- Microbiology Laboratory, Department of Agricultural Chemistry, National Taiwan University, Taipei, Taiwan
Proteases play a key role in numerous bacterial physiological events. Microbial proteases are used in the pharmaceutical industry and in biomedical applications. The genus Vibrio comprises protease-producing bacteria. Proteases transform polypeptides into shorter chains for easier utilization. They also function as a virulence factor in pathogens. The mechanism by which protease genes are regulated in Vibrio parahaemolyticus, an emerging world-wide human pathogen, however, still remains unclear. Quorum sensing is the communication system of bacteria. OpaR is the master quorum-sensing regulator in V. parahaemolyticus. In the present study, quantitative reverse transcriptase-polymerase chain reaction and protease gene promoter-fusion reporter assays revealed that OpaR represses seven protease genes—three metalloprotease genes and four serine protease genes—which are involved in environmental survival and bacterial virulence. Furthermore, the electrophoresis mobility shift assay demonstrated that OpaR is bound directly to the promoter region of each of the seven protease genes. DNase I footprinting identified the sequence of these OpaR-binding sites. ChIP-seq analyses revealed 435 and 835 OpaR-binding sites in the late-log and stationary phases, respectively. These OpaR-binding sequences indicated a conserved OpaR-binding motif: TATTGATAAAATTATCAATA. These results advance our understanding of the protease regulation system in V. parahaemolyticus. This study is the first to reveal the OpaR motif within V. parahaemolyticus in vivo, using ChIP-seq, and to provide a database for OpaR direct regulon.
Introduction
Vibrio parahaemolyticus is a gram-negative bacterium that inhabits marine and estuarine environments. Most cases of seafood-borne gastroenteritis are caused by V. parahaemolyticus (Su and Liu, 2007). The enteral route of V. parahaemolyticus is the consumption of raw or undercooked shellfish. Recent reports have suggested that this bacterium is the main agent of early mortality syndrome (Pérez-Acosta et al., 2018), also known as acute hepatopancreatic necrosis syndrome, an emerging shrimp disease that causes acute mortality in Pacific white shrimp (Litopenaeus vannamei) (Theethakaew et al., 2017). V. parahaemolyticus strains have numerous virulence factors, including thermostable direct hemolysin (TDH), TDH-related hemolysin (TRH), adhesion factors, extracellular proteases, and type III secretion system effectors (Broberg et al., 2011). Two types of flagellar systems assist V. parahaemolyticus with swimming and swarming (Letchumanan et al., 2014).
Vibrio parahaemolyticus expresses extracellular metalloproteases—PrtV, VppC, and VPM—and extracellular serine proteases—VPP1, VpS37, and PrtA (Osei-Adjei et al., 2018). PrtV is a collagenase and can be inhibited by ethylenediaminetetraacetic acid (EDTA) (Yu et al., 2000), VppC acts in the early stationary phase of growth (Miyoshi et al., 2008), VPM is a putative virulence factor (Luan et al., 2007), VVP1 causes cytotoxicity and lethality in mice (Miyoshi, 2013), VpS37 exhibits gelatinolytic activity (Salamone et al., 2015), and PrtA causes abdominal hemorrhage in mice and is toxic to various mammal cells (Lee et al., 2002).
Quorum sensing (QS) is a cell–cell communication system in which bacteria use the production and detection of extracellular chemicals, known as autoinducers, to monitor cell population density (Miller and Bassler, 2001); moreover, gene expression in a microbial community is synchronized by QS (Papenfort and Bassler, 2016). Some bacterial QS-dependent extracellular proteases were used in social cheating to inhibit microbial communities (Whiteley et al., 2017). OpaR and AphA are two QS regulators of V. parahaemolyticus (Gode-Potratz and McCarter, 2011; Sun et al., 2012). In low cell density, AphA controls hundreds of target genes (van Kessel et al., 2013a), whereas OpaR is a dominant regulator in high cell density (Gode-Potratz and McCarter, 2011; Burke et al., 2015). OpaR and AphA repress each other’s gene expression (Sun et al., 2012; Zhang et al., 2012).
Virulence proteases are associated with the QS system in Vibrio spp. Hemagglutinin/protease (HAP) is a well-known virulence factor of V. cholerae (Silva et al., 2003; Liang et al., 2007; Benitez and Silva, 2016) and is regulated by the QS regulator HapR (Wang et al., 2011; Dorman and Dorman, 2018). A previous study reported the purification of a novel serine protease of V. cholerae, which evidently plays a role in hemorrhagic response in the rabbit ileal loop model (Syngkon et al., 2010). Moreover, the serine protease VvpS (Lim et al., 2011) plays a vital role in V. vulnificus autolysis and is activated by the QS regulator SmcR (Kim et al., 2015). OpaR, the QS regulator of V. parahaemolyticus, promotes the expression of PrtA, which is an extracellular alkaline serine protease (Chang and Lee, 2018). OpaR also regulates the quantity of extracellular PrtA (Chang and Lee, 2018). In addition, QS induces the gene expression of Asp (Rui et al., 2009), which is an extracellular alkaline serine protease from V. alginolyticus and the cause of vibriosis in Lutjanus erythopterus (Cai et al., 2007).
Nutrient intake is an essential part of the bacterial life cycle—pathogens ingest nutrient molecules from host cells. A certain minimum level of protease is indispensable for the survival and growth of organisms; however, our understanding of the regulation of protease genes in V. parahaemolyticus is far from comprehensive. Herein, we used ChIP-seq to identify OpaR-binding sites on the genome of V. parahaemolyticus no. 93 (VP93), which is a tdh– and trh– strain. We present a conserved OpaR-binding motif and propose that OpaR directly represses seven protease genes of V. parahaemolyticus.
Results
ChIP-Seq Data Analysis Revealed a Conserved OpaR-Binding Motif
Vibrio parahaemolyticus can be found in multiple cell types, including free-swimming forms and colony formations attached to inert surfaces such as shellfish (Letchumanan et al., 2014). A previous study utilized whole transcriptome next-generation sequencing (RNA-Seq) for analyzing the OpaR regulon in V. parahaemolyticus, which was cultured on a solid growth medium. RNA-Seq data were further validated through qRT-PCR for a select subset of transcription factor genes that were highly regulated by OpaR (Burke et al., 2015). In this study, we investigated the regulation of the protease genes of V. parahaemolyticus, which was cultured in a liquid growth medium.
We first inserted a FLAG-tag in front of the opaR gene on the VP93 chromosome. Western blot results demonstrated that FLAG-OpaR is steadily expressed from the early-log to late-stationary phases (Supplementary Figure 1). We collected bacterial cells from the late-log (OD600 2.8) and stationary (OD600 4) phases to perform ChIP-seq assays. FLAG-OpaR was bound to the genome and cross-linked by anti-FLAG beads; the genome was sheared in 300-bp fragments, and immunoprecipitated DNA was purified and sequenced. Reads were obtained from independent ChIP-seq assays in late-log and stationary phases. The input control of ChIP-seq was the VP93 genome without anti-FLAG antibody enrichment. All reads were mapped to the VP93 genome. Our ChIP-seq data (NCBI Gene Expression Omnibus database, GEO Accession GSE122479) revealed the presence of 435 and 835 OpaR-binding sites in late-log and stationary phases, respectively; there were 432 overlapping OpaR-binding sites between the two phases (Figure 1A).
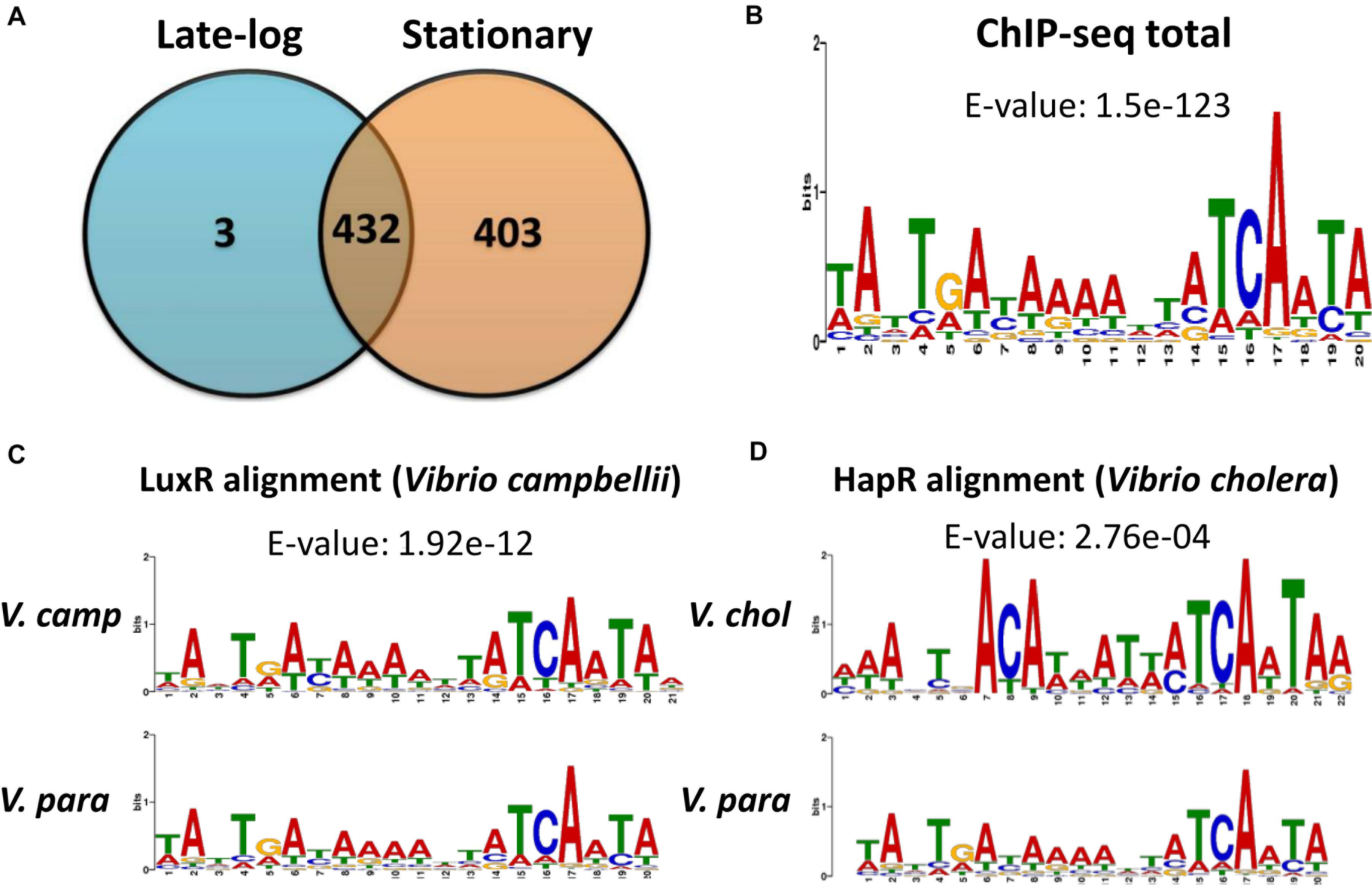
Figure 1. ChIP-seq data revealed in vivo OpaR-binding sites in Vibrio parahaemolyticus. (A) Distribution of OpaR-binding sites of ChIP-seq in the late-log and stationary phases. (B) Conserved OpaR-binding motif was identified from 838 OpaR-binding sequence of ChIP-seq data and analyzed using Multiple Expectation Maximization for Motif Elicitation. The height of each base represents its frequency. Conserved OpaR-binding motif aligned using TOMTOM (http://memesuite.org//tools/tomtom) with other Vibrio quorum-sensing regulators: (C) LuxR of V. campbellii and (D) HapR of V. cholera.
OpaR regulates nine transcription factors in V. parahaemolyticus: CpsR, the Crl family, the Ars family, the CsgD/VpsT family, the FhlA family, the AsnC family, ExsA, AphA, and LafK (Burke et al., 2015). Moreover, transcriptional regulation of opaR, qrr2–4 (encoding QS regulatory small RNAs), and aphA by OpaR has been reported in V. parahaemolyticus (Zhang et al., 2012). In this study, we used Multiple Expectation Maximization for Motif Elicitation (MEME)1 to analyze 838 OpaR-binding sequences, which showed a conserved OpaR-binding motif (TATTGATAAAATTATCAATA) (Figure 1B). From genes situated near to OpaR-binding sites, we identified seven protease genes that might be regulated by OpaR in V. parahaemolyticus. The ChIP-seq-defined regions of the seven protease genes are listed in Table 1. In addition, we used TOMTOM2 to align the conserved OpaR-binding motif with other homologs of QS regulators in Vibrio spp., namely LuxR (Figure 1C) and HapR (Figure 1D). We observed that the OpaR-binding motif showed a high similarity with other QS regulator motifs. Comparison of the three QS regulator motifs—the conserved OpaR-binding motif, LuxR motif, and HapR motif (Figure 1)—indicated that TATTGATAAAATTATCAATA is the most conserved binding region of QS regulators in Vibrio spp.
OpaR Occupies Six Promoters and One 3′ Region of Protease Genes and Their Expression Through qRT-PCR
Table 2 reveals the functions of seven putative OpaR-regulated protease genes classified using Gene Ontology (GO) and Clusters of Orthologous Groups (COG) annotations. These seven genes could be classified into two categories: metalloendopeptidases (LytM, Mcp02, M6 protease) and serine-type endopeptidases (serine protease, DegS, periplasmic protease, protease II). Moreover, they could be classified based on their cellular locations: inner membrane protease, DegS (VP0432) (Gottesman, 2017); periplasmic protease (VP2032) (Baird et al., 1991); and extracellular proteases, LytM (VPA1649) (Gode-Potratz et al., 2011), Mcp02 (VPA0755), M6 protease (VP0907) (Rompikuntal et al., 2015), serine protease (VPA0449) (Chimalapati et al., 2018) and protease II (VPA1467). Three of these—namely Mcp02, serine protease and protease II—are secreted by the type II secretion system (Chimalapati et al., 2018).
The distribution of OpaR-binding peaks relative to the seven protease genes are shown in Figure 2. These peaks overlaid the translational start site of serine protease and degS. OpaR-binding peaks appeared on the upstream of lytM, mcp02, M6 protease, and protease II; however, one OpaR-binding peak presented at the terminator region and overlaid the translational stop site of the periplasmic protease. Predicted OpaR-binding sites in the promoters of lytM, mcp02, M6 protease, serine protease, and degS were found not only in the late-log phase but also in the stationary phase, whereas the OpaR occupancy in the promoter of protease II and terminator of periplasmic protease was only found in the stationary phase. Normalized reads per million (RPM) values [total reads/mapped reads (millions) x region length (bp)] represent the binding strength of OpaR-binding peaks. In addition, the binding length of OpaR ranged from 177 bp (degS) to 372 bp (lytM) (Table 1).
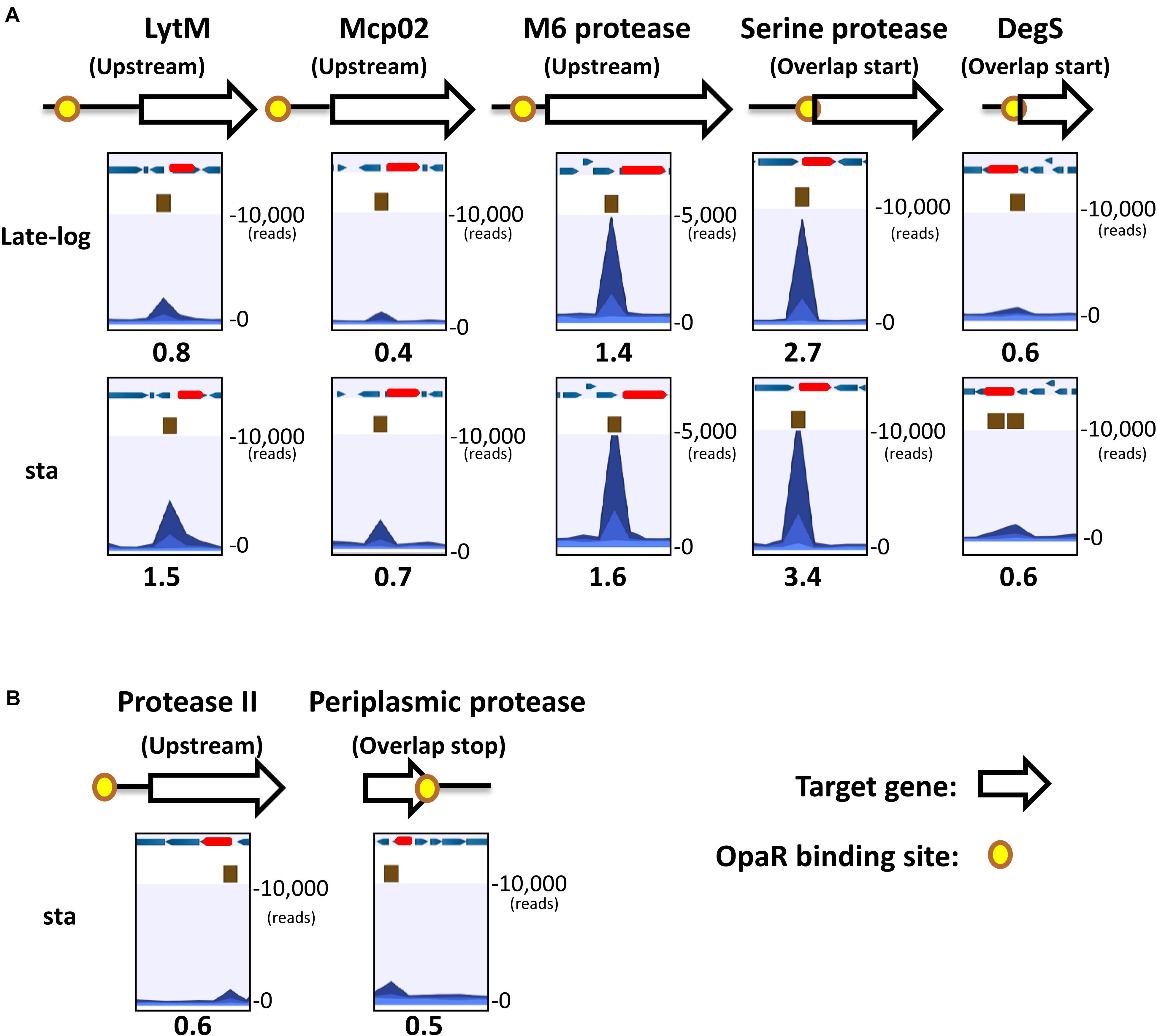
Figure 2. OpaR-binding peak distribution of the seven protease genes. Yellow spots represent in vivo OpaR-binding positions on the protease promoter or 3′region, as identified using ChIP-seq. White and red arrows denote the protease genes, and blue arrows illustrate the nearby genes. Navy blue peak represents the binding strength of the samples (ChIP-seq enriched by anti-FLAG antibody); light blue peak is the control signal (ChIP-seq without anti-FLAG antibody enrichment). Numbers below the peak diagrams represent the reads per million (RPM) value of ChIP-seq. Central OpaR-binding sites are shown in brown boxes. (A) OpaR bound to five protease genes—lytM, mcp02, m6 protease, serine protease, and degS—both in the late-log and stationary phases. (B) OpaR bound to both protease II and periplasmic protease only in the stationary phase.
Each protease gene expression levels at different growth phases were examined using qRT-PCR. Genes encoding LytM (Figure 3A), Mcp02 (Figure 3B), DegS (Figure 3E), and protease II (Figure 3F) were expressed from late-log to stationary phases, whereas those encoding M6 protease (Figure 3C), serine protease (Figure 3D), and periplasmic protease (Figure 3G) were primarily expressed during the late-log phase. Growth curves of VP93 were monitored by measuring OD600 at every growth stage (Figure 3H). Therefore, we used late-log and stationary phase cultures to study the correlation between OpaR and the seven protease genes.
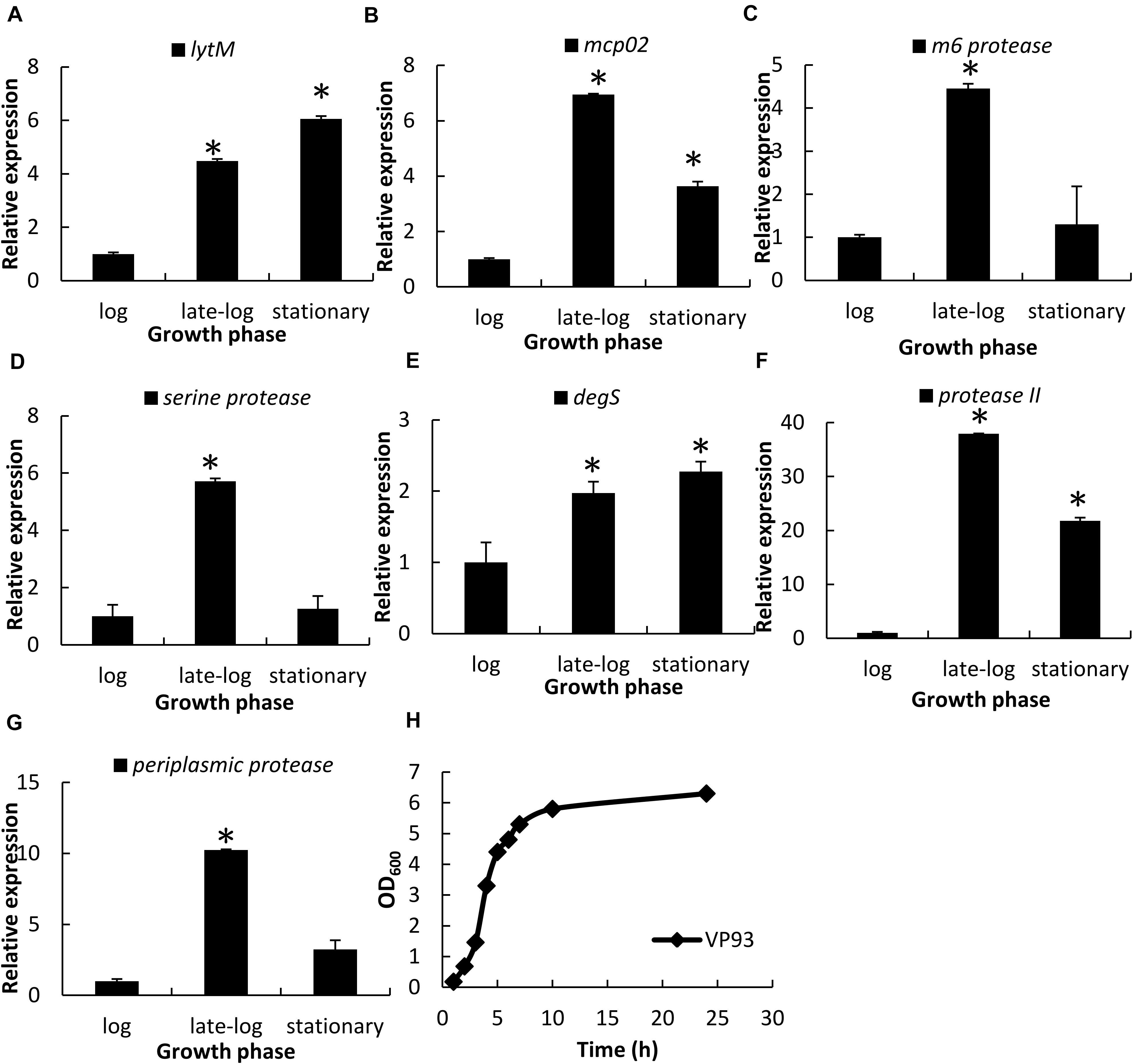
Figure 3. Protease gene expression levels at different growth phases, as examined using qRT-PCR. Total RNA isolated from VP93 at log (OD600 1.7), late-log (OD600 2.8), and stationary (OD600 4) phases was purified and analyzed using specific primer sets for qRT-PCR: (A) lytM, (B) mcp02, (C) m6 protease, (D) serine protease, (E) degS, (F) protease II, and (G) periplasmic protease. (H) Growth curves of VP93 monitored by measuring OD600 at every growth stage. Values represent mean of three independent experiments, and error bars indicate standard deviation. All values were relative to the log phase, which had the value of 1. Asterisks denote statistical significance (*p < 0.01).
OpaR Is a Direct Repressor of the Seven Protease Genes Validated by qRT-PCR, Promoter-Fusion luxAB Reporter Assays, and Electrophoretic Mobility Shift Assay
We used qRT-PCR to measure the relative transcript levels of seven protease genes in wild-type VP93 and mutant ΔopaR strains at late-log and stationary-phase growth. Our results revealed that OpaR represses the expression of genes encoding LytM (1.9-fold, p = 0.0069), Mcp02 (1.5-fold, p = 0.0058), M6 protease (2.0-fold, p = 0.0074), Serine protease (4.8-fold, p = 0.0054), DegS (2.1-fold, p = 0.0085), Protease II (25.7-fold, p = 0.0001), and Periplasmic protease (2.6-fold, p = 0.0072) in the late-log phase (Figure 4A). Moreover, this differential gene expression was also observed in the stationary phase for both Serine protease (repressed 12.7-fold, p = 0.0060) and DegS (repressed 1.5-fold, p = 0.0018) (Figure 4B).
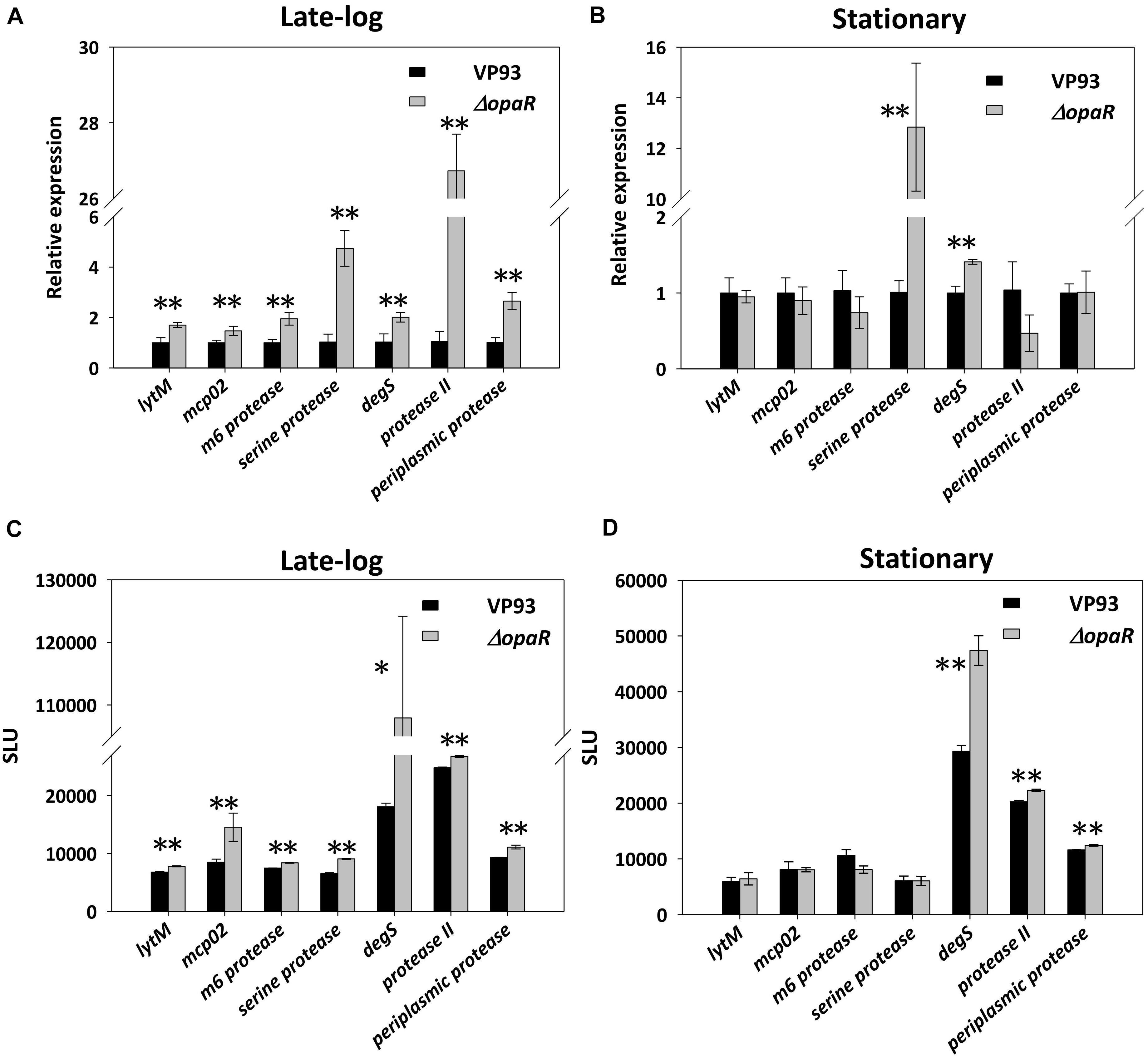
Figure 4. OpaR regulates the expression of the seven protease genes. Total RNA isolated from the VP93 and ΔopaR strains was purified, and gene expression was detected via qRT-PCR at the (A) late-log (OD600 2.8) and (B) stationary (OD600 4) phases. A luciferase assay was performed using VP93 and ΔopaR strains with the protease promoter-luxAB or 3’region-luxAB fusion plasmid at the (C) late-log (OD600 2.8) and (D) stationary (OD600 4) phases. Values represent mean of three independent experiments, and error bars indicate standard deviation. Asterisks denote statistical significance (*p < 0.05, **p < 0.01).
To determine the role of these OpaR-occupied positions of protease genes from Figure 2, the promoter-fusion luxAB reporter assay was conducted. We amplified the aforementioned putative OpaR-binding sequences of each protease gene and cloned them into the promoter-less vector pSAluxAB. Bioluminescence was measured for the wild-type VP93 and the mutant ΔopaR strains at late-log and stationary phases. The results revealed that OpaR represses the bioluminescence expression for protease genes through OpaR-binding sites in the late-log phase, including lytM (1.2-fold, p = 0.0002), mcp02 (1.8-fold, p = 0.0089), m6 protease (1.1-fold, p = 0.0017), serine protease (1.4-fold, p = 0.00001), degS (6.0-fold, p = 0.0106), protease II (1.1-fold, p = 0.0001), and periplasmic protease (1.2-fold, p = 0.0094) (Figure 4C). However, the expressions of degS (1.6-fold, p = 0.0030), protease II (1.1-fold, p = 0.0003), and periplasmic protease (1.1-fold, p = 0.0076) were regulated by OpaR in the stationary phase (Figure 4D).
The electrophoretic mobility shift assay (EMSA) was performed to evaluate the potential of the direct binding of OpaR to the promoters of the protease genes. We amplified the DNA fragments as the probes (P1 and P2) for each target gene, and the positions of EMSA probes are listed in Table 1. EMSA demonstrated that OpaR bound six target protease gene promoters (Figure 5). Two OpaR-binding sites (P1 and P2) were present within lytM, m6 protease, and protease II promoter regions (Figures 5A–C), and only one (P1) was present on degS, mcp02, and serine protease promoter regions (Figures 5D,F,G). Indeed, in OpaR EMSA, two shifted bands were observed in the P1 region of lytM, protease II, and degS promoters (Figures 5A,C,D). These bands are attributable to the presence of more than one individual binding sites in the promoter P1 region; they may also represent two or more different states of DNA binding. Notably, we also observed a periplasmic protease 3’ P1 region that shifted in the presence of 2.8–11.2 pmol of OpaR (Figure 5E). OpaR did not bind to 16S rDNA as the negative control of EMSA (data not shown).
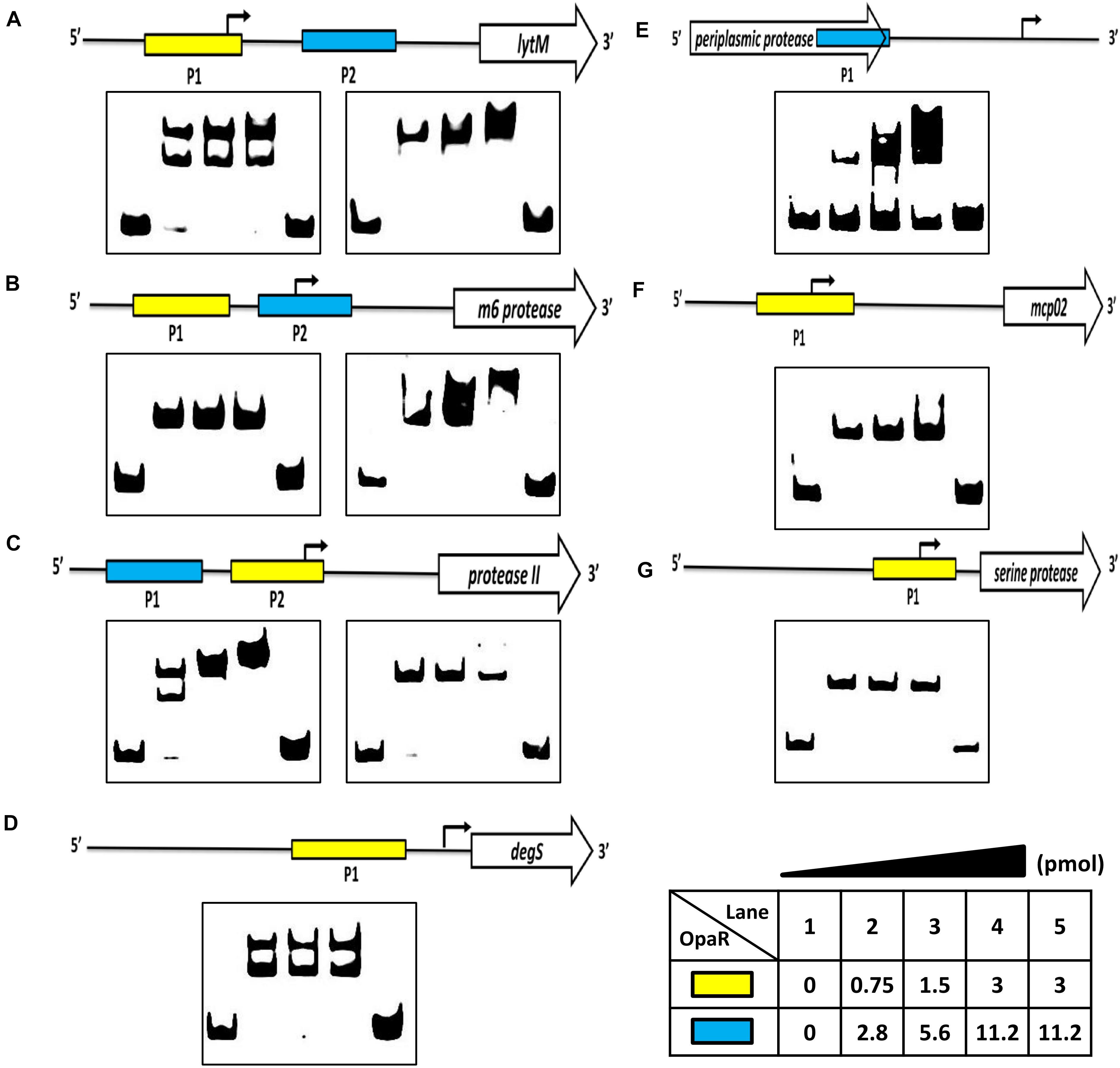
Figure 5. OpaR bound to the promoter region of the protease genes in vitro. An OpaR-binding reaction was performed with the promoter sequences of the protease genes. (A) lytM, (B) m6 protease, (C) protease II, (D) degS, (E) periplasmic protease, (F) mcp02, and (G) serine protease. The more sensitive electrophoretic mobility shift assay (EMSA) probes of each protease gene are labeled with a yellow box. The blue box indicates the other EMSA probe. Digoxigenin-labeled EMSA probes were incubated without (lane 1) or with increasing concentrations of purified OpaR (lanes 2–5) for the yellow and blue boxes. Lane 5 included 100 times more unlabeled probes as competitors.
Identification of OpaR-Binding Sequences in the Seven Protease Genes
We amplified the promoter region containing all the EMSA probe sequences of each protease gene to design footprinting probes. The 5′-end of the sense and antisense footprinting probes were labeled with 6-carboxyfluorescein. Labeled probes were incubated with purified His-OpaR and digested by DNase I. Capillary electrophoresis was used to analyze the digested DNA fragments. The OpaR-binding sequences on the seven protease genes are listed in Table 3, and the specific OpaR-binding positions are presented in Figure 6. The OpaR-binding position was defined by the difference of peak observed between the control without OpaR and samples with OpaR. We found two OpaR-binding sequences for lytM, m6 protease, protease II, degS (Figures 6A–D). Capillary electrophoresis analysis identified one OpaR-binding sequence for periplasmic protease, mcp02 and serine protease (Figures 6E–G). Notably, upon aligning the OpaR-binding sequences obtained from the DNase I footprinting assay with the EMSA probe sequences, degS EMSA revealed a double band shift, and we could locate and identify two OpaR-binding sequences in the DNase I footprinting assay result (Figures 5D, 6D).
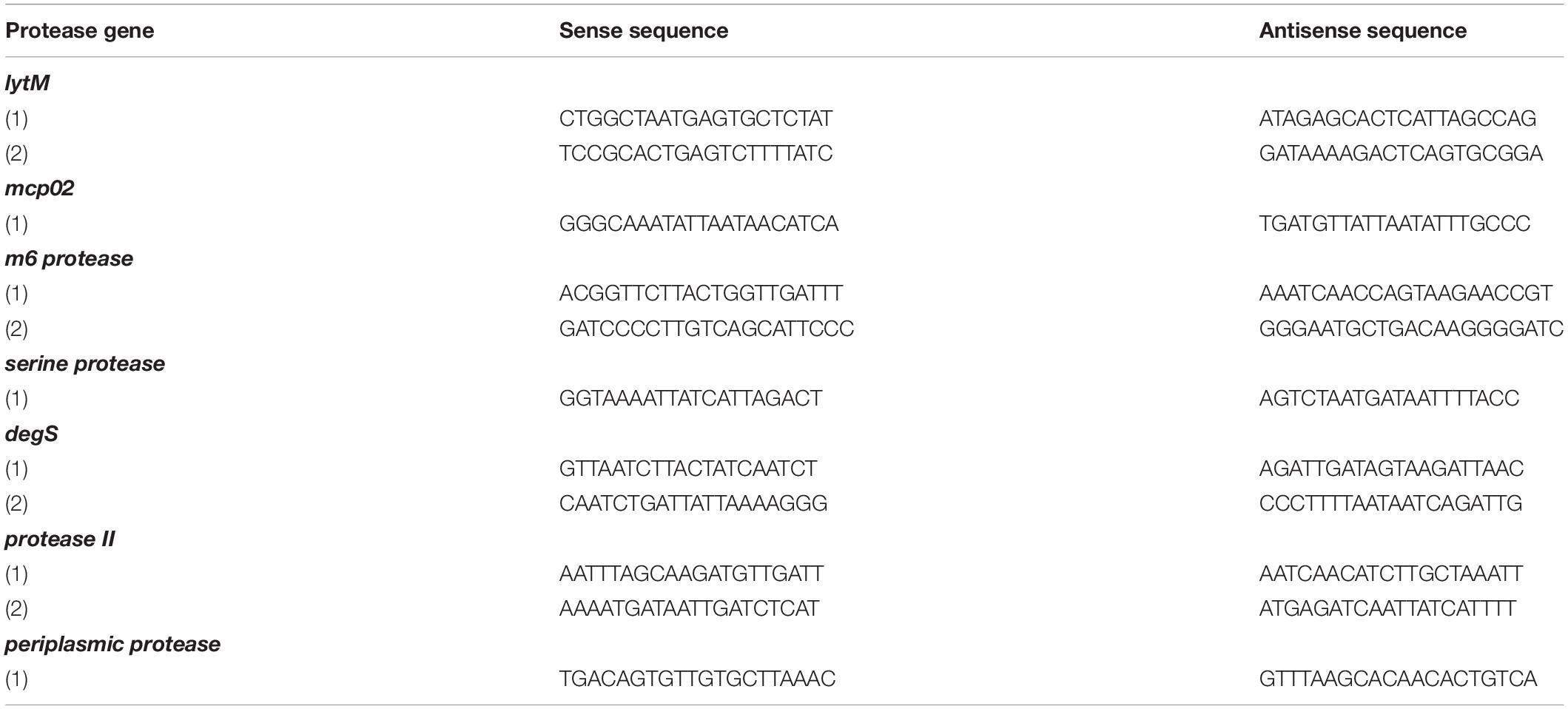
Table 3. OpaR-binding sequences on protease gene promoter regions as revealed using DNase I footprinting assay.
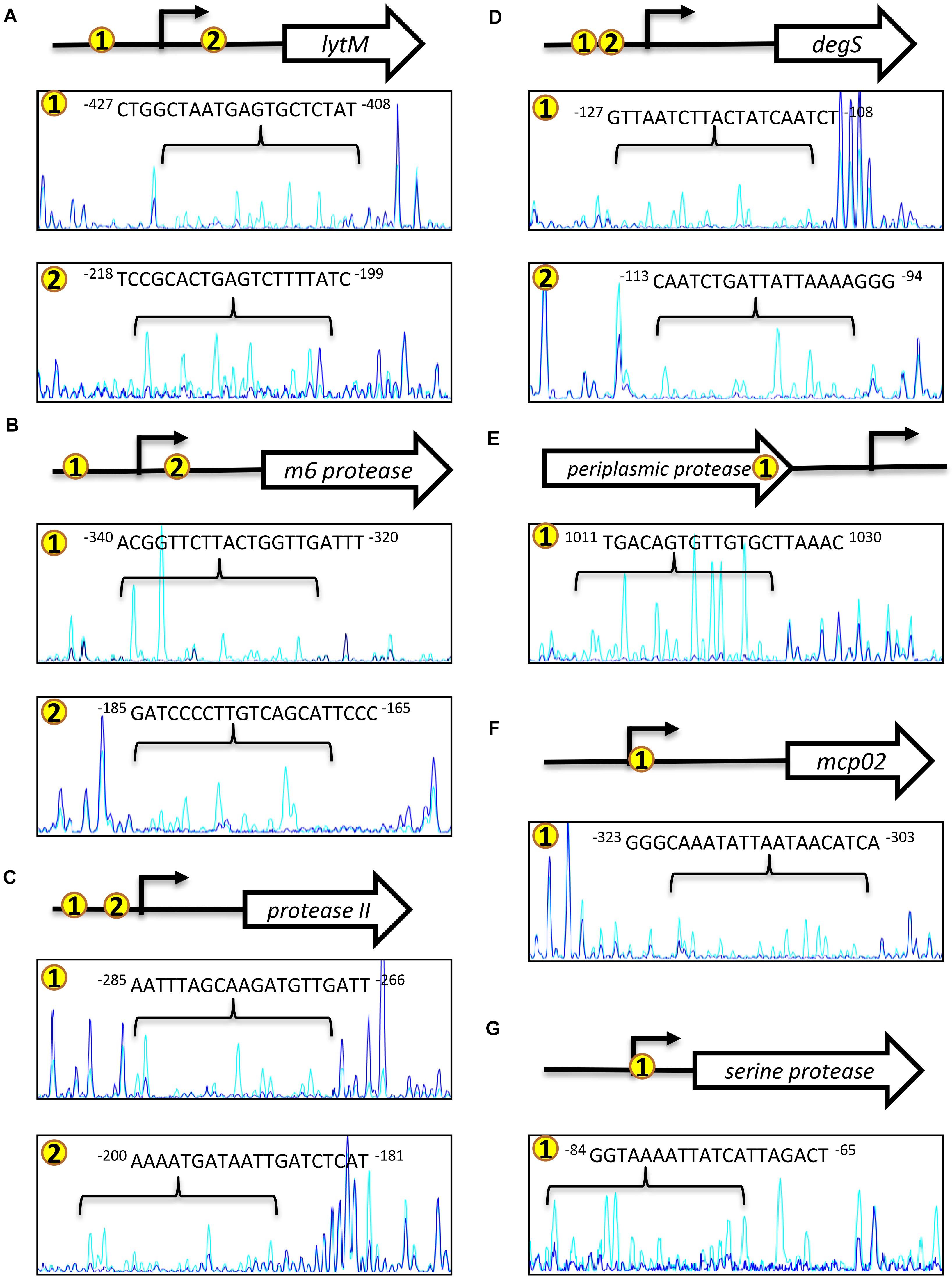
Figure 6. OpaR-binding sequences determined using footprinting assays. The footprinting assay involved capillary electrophoresis analyses on each protease gene promoter sequence containing the ChIP-seq and EMSA-defined OpaR-binding region. Capillary electrophoresis diagrams are shown: (A) lytM, (B) m6 protease, (C) protease II, (D) degS, (E) periplasmic protease, (F) mcp02, and (G) serine protease. Number in yellow circles on the gene promoter and 3’region are consistent with those in the peak diagram. The blue peak shows footprinting results with OpaR. The light blue peak is the control (without OpaR). The arrow (→) represents the predicted transcriptional start site of the protease genes.
To further verify whether the OpaR-binding site appeared at the periplasmic protease 3′region, we performed sequence analysis using ARNold program (Naville et al., 2011). The result revealed that a Rho-independent transcriptional terminator (intrinsic terminator) was present and located 29 to 50-nt downstream of the periplasmic protease stop codon. DNase I footprinting assay result showed that one OpaR-binding sequence located 33 to 52-nt upstream of the stop codon (Figure 6E). OpaR binding close to the stop coden of the ORF may block transcriptional elongation and result in the target gene repression. These results conclude that OpaR binds to the ORF near 3′ region to repress periplasmic protease expression.
Diagram of QS-Dependent Protease Regulation
At a high cell density, OpaR repressed three groups of proteases: extracellular metalloprotease, extracellular serine protease, and periplasmic serine protease. However, PrtA (VPA0227) is an extracellular serine protease that was promoted by OpaR, as reported in a previous study (Chang and Lee, 2018). As shown in Figure 7, OpaR directly regulated the gene expression of the eight protease genes: three environmental survival genes—periplasmic protease (Baird et al., 1991), serine protease, and protease II (Kanatani et al., 1991, 1992)—and five bacterial virulence genes—lytM (Gode-Potratz et al., 2010), mcp02 (Gao et al., 2010; Miyoshi, 2013), m6 protease (Vaitkevicius et al., 2008; Rawlings et al., 2018), prtA (Lee et al., 2002), and degS (Mathur et al., 2007).
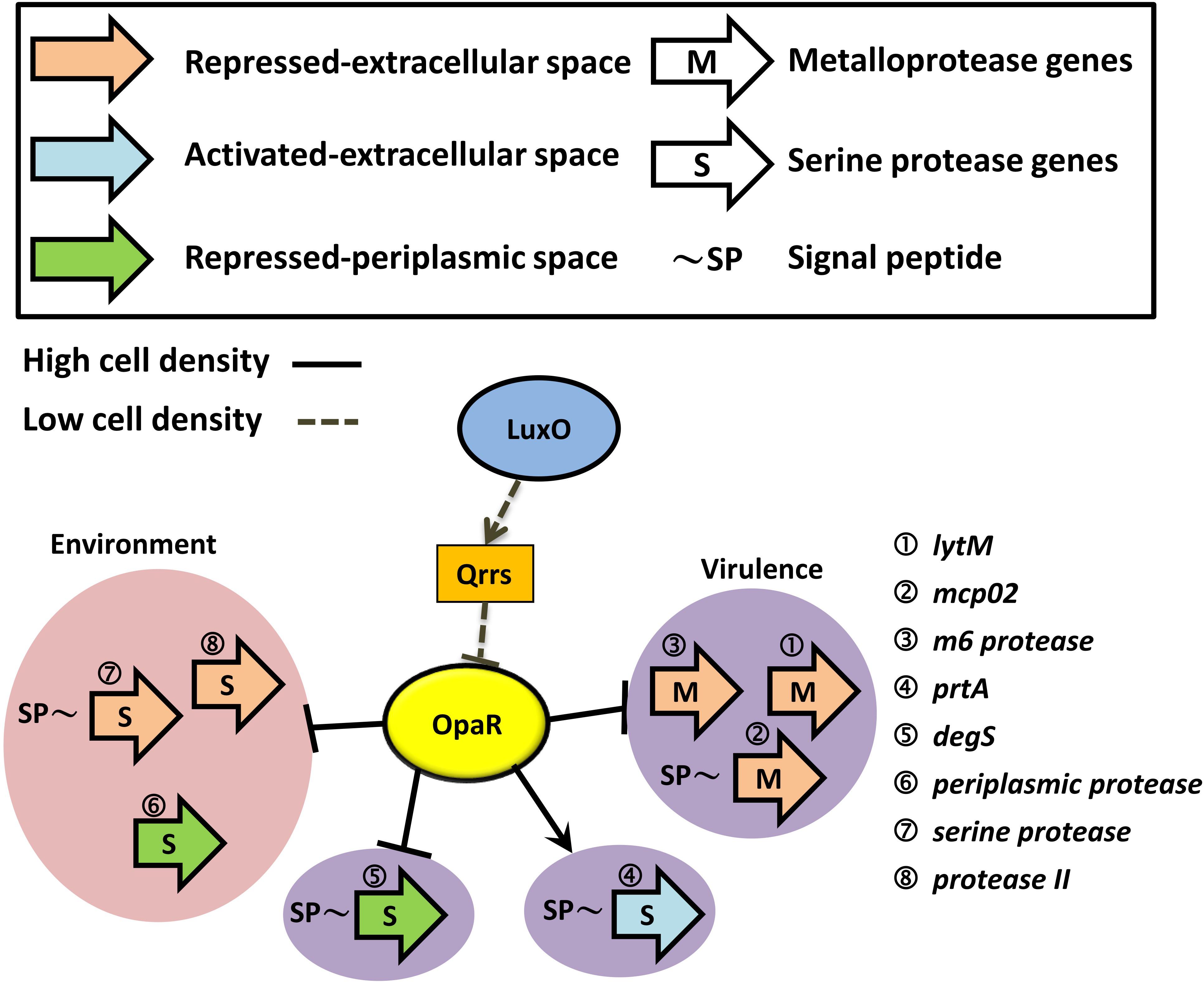
Figure 7. Schematic of how OpaR directly regulates protease genes. Black arrows represent positive regulation of protease genes by OpaR. Protease genes were categorized depending on activation/repression, periplasmic/extracellular localization, and metalloprotease/serine protease. Proteases that might involve bacterial virulence are depicted on a purple background. The pink region includes the environmental survival genes. Dashed and solid lines indicate the regulation pathway at a low and high cell density, respectively. Signal peptides were predicted by SignalP v5.0 (Armenteros et al., 2019) and are labeled as ∼SP.
Discussion
A report using in vitro EMSA with purified His-tagged OpaR demonstrated nine direct OpaR targets (Burke et al., 2015) and indicated that MQSR matrix is too strict to predict OpaR-binding sites (Burke et al., 2015). OpaR-binding sequences have been reported among opaR, qrr2, qrr3, qrr4, aphA, T6SS2 genes, T3SS-associated genes, and flagellar genes (Zhang et al., 2012; Wang et al., 2013; Burke et al., 2015; Lu et al., 2019). ChIP-seq is a powerful method for investigating the binding targets of bacterial transcription factors. Our findings also revealed that 432 OpaR-binding sites overlapped between the late-log and stationary phases and that 403 OpaR-binding sites were present only in the stationary phase (Figure 1A). This finding implied that OpaR occupies more regions of the VP93 genome in the stationary phase than in the late-log phase. OpaR regulates many genes at high cell density, such as lateral flagellar genes, biofilm genes, and T3SS operon genes (Ball et al., 2017). Moreover, the global stress regulator RpoS in the stationary phase induced the expression of a QS regulator (Weber et al., 2008). Stationary-phase regulatory proteins were controlled by the QS system (Lazazzera, 2000). In the present study, we identified 838 in vivo OpaR-binding sites using ChIP-seq assay and suggested that the most conserved OpaR-binding motif was TATTGATAAAATTATCAATA (Figure 1).
The seven protease genes mentioned in this study have a unique function in V. parahaemolyticus. A previous microarray-based study in which V. parahaemolyticus was cultured on a solid growth medium reported that OpaR represses surface-sensing regulon and different secretion systems (Gode-Potratz and McCarter, 2011). LytM reportedly belongs to the surface-sensing regulon and is regulated by the master regulator of the lateral flagellar system LafK (Gode-Potratz et al., 2011). Mcp02 is a putative vibriolysin and causes instant cytotoxicity during infections (Gao et al., 2010). M6 protease has an M6 protease domain in the N-terminal and plays a role in bacterial environmental persistence and survival (Rawlings et al., 2018). M6 protease purified from V. cholera has been reported to cause cytotoxicity and degrade host tissue components (Vaitkevicius et al., 2008). DegS is involved in the periplasmic stress response against antimicrobial peptides (Mathur et al., 2007). Protease II is involved in amino acid transport and metabolism in V. parahaemolyticus (Chimalapati et al., 2018).
Although most binding regulations occur outside of upstream intergenic regions, target gene transcription is more often repressed by binding downstream of the proximal promoter (Babu and Teichmann, 2003). In the present study, ChIP-seq data and EMSA results revealed that OpaR binds to the 3′ end region of the periplasmic protease gene and represses the gene expression. Furthermore, from a report of OpaR RNA-seq data (Burke et al., 2015), we found some genes down regulated by OpaR at greater than 4-fold and noted that OpaR is located at the 3′end region of putatively regulated target genes from our ChIPseq data (data not shown). However, we demonstrated that opaR directly regulates the transcription of the other six protease genes through the OpaR-binding sites in their promoters.
OpaR is a homolog of LuxR, which is a QS regulator in V. harveyi (Pompeani et al., 2008). At a high cell density, QS regulators exhibit maximal gene expression (Waters and Bassler, 2006; Kleinman et al., 2017). They regulate the downstream genes through their activation or repression (van Kessel et al., 2013b). A recent study found that LuxR directly interacts with RNA polymerase to activate transcription of the luxCDABE bioluminescence genes; moreover, LuxR DNA-binding sites that are present in close proximity to the -35 region of the promoter are required for activation at some promoters (Ball and van Kessel, 2019). Notably, our recent report indicated that PrtA, which belongs to the surface-sensing regulon (Ferreira et al., 2012), is a unique OpaR-activated protease gene. The OpaR-binding sequence overlapped the -35 region of the prtA promoter (Chang and Lee, 2018). PrtA is also activated by LafK, which is involved in cytotoxicity and pathogenicity in V. parahaemolyticus.
Herein, we used a strain of V. parahaemolyticus that was a chromosomal FLAG-tag insertion strain, not an overexpression strain with plasmids. Therefore, the in vivo data of this study were affected neither by OpaR overexpression nor by plasmids exhibiting antibiotic resistance. To the best of our knowledge, this is the first study to report a conserved OpaR-binding motif, TATTGATAAAATTATCAATA, and seven OpaR-downregulated protease genes in V. parahaemolyticus.
Materials and Methods
Bacterial Strains and Growth Conditions
All bacterial strains, plasmids, and primers are listed in Supplementary Tables 1, 2. V. parahaemolyticus strains and their derivatives were cultured in tryptic soy bean broth containing 3% NaCl (TSB3) (Difco) at 35°C. Escherichia coli strains were grown in Luria–Bertani (Difco) medium at 35°C. XL1-Blue (Bullock et al., 1987) and S17-1 λpir (Delorenzo et al., 1993) were used for cloning and conjugation, respectively. Mutant and reporter strains were derived from VP93. When necessary, antibiotics were added to the culture medium: ampicillin, 100 μg ml–1; kanamycin, 25 μg ml–1; and chloramphenicol, 20 μg ml–1 for E. coli and 5 μg ml–1 for V. parahaemolyticus.
FLAG-Tag Insertion Before the OpaR Gene
We constructed a FLAG-tag insertion strain using homologous recombination. First, a FLAG-tag (DYKDDDDK) was inserted after the translational start site of opaR using overlapping PCR. The FLAG-opaR fragment was then ligated to pDS132, a suicide vector (Philippe et al., 2004), to yield the pDS132–FLAG-opaR plasmid. This mobilizable plasmid was transferred from E. coli S17-1 λpir to VP93 via conjugation (Schafer et al., 1990). The conjugants were selected from a TSB3 Cm plate for the first step of the homologous recombination. Next, thiosulfate–citrate–bile salts–sucrose plates containing 6% sucrose were used to select the second homologous recombination conjugants. PCR amplification and sequencing were used to confirm the generation of the FLAG-tag insertion strain.
Western Blotting
FLAG-OpaR/VP93 strain culture was collected at every growth stage by centrifugation. Total proteins of the FLAG-OpaR/VP93 strain were separated using 12.5% SDS-PAGE gels and transferred to PVDF membranes (Merck Millipore, Germany). The membrane was blotted with anti-FLAG antibody as the primary antibody. The secondary antibody is an anti-rabbit IgG HRP-linked antibody. Immobilon Western Chemiluminescent Horseradish Peroxidase Substrate (Merck Millipore, Germany) was added, and signals were detected using a chemiluminescence image system, MutiGel-21 (Bio Pioneer Tech, Taipei, Taiwan).
ChIP-Seq and Analyses
FLAG-OpaR/VP93 was grown to the late-log (OD600 3) and stationary (OD600 4.2) phases. Next, the bacterial culture was incubated with 1% formaldehyde for 20 min at 25°C, and cross-linking was ceased using 0.5 M glycine. Bacterial cells were then harvested by centrifugation, washed three times with Tris-buffered saline buffer, and resuspended in a lysis buffer [10 mM Tris, 100 mM NaCl, 1 mM EDTA, 0.5 mM EGTA, 0.1% deoxycholate, and 0.5% N-lauroylsarcosine (pH 8.0)] containing a protease inhibitor cocktail (Sigma). The lysed cells were sonicated using Bioruptor Pico® (Diagenode) for 20 cycles. Cell debris was removed by centrifugation; supernatant DNA fragments ranged from 300 bp to 500 bp. Anti-FLAG antibody beads (Sigma) were incubated with supernatant DNA fragments overnight at 4°C. After the ChIP reaction, samples were washed five times with a radioimmunoprecipitation assay buffer [50 mM hydroxyethyl piperazineethanesulfonic acid buffer, 500 mM LiCl, 1 mM EDTA, 1% Non-idet P-40, and 0.7% deoxycholate (pH 7.5)] and twice with Tris-buffered saline buffer. ChIP DNA was eluted in an elution buffer (50 mM Tris-EDTA buffer, 1% EDTA, pH 7.5) at 65°C for 30 min to de-cross-link. Samples were treated with RNase A and proteinase K (Dong and Mekalanos, 2012), and ChIP DNA was then purified using the Agencourt AMPure XP PCR Purification Kit (Beckman Coulter). Libraries for ChIP-seq were prepared using the Ovation Ultralow Library System V2 1–96 (NuGEN). Sequencing was performed using the HiSeq 2000 sequencing system (Illumina). ChIP-quantitative PCR was performed using SYBR green (BioRad) to determine the quality (S/N ratio) of ChIP-DNA. AphA and VPA0606 were used as positive and negative controls, respectively (Burke et al., 2015).
We used ”Map Reads to Reference” in CLC Genomics Workbench v9.5 platform conducting Reference mapping. We also used ”Transcription factor ChIP-Seq Analysis” conducting the peak finding. Maximum P-value for peak calling is < 0.05. The OpaR-binding motif was generated using MEME1 (Bailey et al., 2009). TOMTOM, a web-based tool2 (Bailey, 2011), was used to align the conserved OpaR-binding motif with other homologs. The ChIP-seq data could be obtained with the GEO accession number GSE122479.
His6-OpaR Protein Expression and Purification
His6-OpaR protein was expressed and purified as previously described (Chang and Lee, 2018). Briefly, to generate the pET28a–opaR plasmid, opaR was cloned into the six-His-tag expression vector pET28a (Novagen) (Supplementary Table 1). The clone was transformed into E. coli BL21 (DE3) to express OpaR with an N-terminal fusion tag. E. coli BL21 (DE3) with pET28a–opaR was grown in 200 mL Luria–Bertani broth supplemented with 25 μg mL–1 kanamycin at 37°C. When OD600 reached 0.4–0.6, 1 mM isopropyl β-D-1-thiogalactopyranoside was used to induce His6-OpaR protein expression at 30°C for 4 h. The cells were then collected and disrupted using the Constant Cell Disrupter System (Constant Systems Ltd., Daventry, United Kingdom), and nickel–nitrilotriacetic acid chromatography (GE Healthcare, Chicago, IL, United States) was used to purify His6-OpaR. His6-OpaR purity was confirmed using sodium dodecyl sulfate polyacrylamide gel electrophoresis. Proteins were quantified using the method described by Bradford (Bradford, 1976).
Quantitative Reverse-Transcriptase PCR
We collected the bacterial cultures from different growth phases of VP93 and ΔopaR. Cells were mixed with RNAprotect Bacteria Reagent (Qiagen) and centrifuged at 5000 g at 4°C for 15 min. Total RNA was isolated using TRIzol. Reverse transcription–PCR (RT-PCR) was performed using HiScript I Reverse Transcriptase (Bionovas Biotechnology), according to the manufacturer’s instructions, and real-time quantitative reverse-transcriptase (qRT-PCR) was performed using SYBR Green Master Mix (iQTM SYBR Green® Supermix, BioRad) on a BioRad CFX96 system. Relative expression values were determined using 2–(Δ Ct Target – Δ Ct Reference). Ct is the fractional threshold cycle, and the reference was 16S ribosomal RNA gene. The specific primer sets used for qRT-PCR are listed in Supplementary Table 2.
Promoter-luxAB Fusion Plasmid and Luciferase Assay
The promoter regions of the six OpaR-regulated protease genes and one 3’ end region of periplasmic protease were amplified using specific primer sets (Supplementary Table 2). The promoter fragments were cloned into a promoter-less vector pSAluxAB (Supplementray Table 1), with SmaI and XbaI restriction sites. These plasmids were transformed into VP93 and ΔopaR by using the Gene Pulser Xcell Electroporation System (BioRad). Luciferase activity was measured by adding n-decanol [final concentration 0.001% (vol/vol)] as the substrate to 2 mL bacterial cultures. Luminescence was detected on a Spectrofluorometry F-2500 device (Hitachi, Tokyo, Japan) and is reported as specific light units (SLU; relative luminescent light units per second per milliliter per OD600 unit).
EMSA
The specific primer sets used to amplify EMSA probes are listed in Supplementary Table 2. These probes were labeled with digoxigenin-11-ddUTP at their 3′-ends using the second generation DIG Gel Shift Kit (Roche Applied Sciences, Mannheim, Germany). In the binding reaction, 0.4 ng labeled fragment was incubated with various quantities of purified His-OpaR protein in 20 μL binding buffer [100 mM hydroxyethyl piperazineethanesulfonic acid (pH 7.6), 5 mM EDTA, 50 mM (NH4)2SO4, 5 mM dithiothreitol, 1% (wt/vol) Tween 20, and 150 mM KCl] at 25°C for 30 min. In competition analyses, the protein–DNA complex was mixed with 100 ng unlabeled fragment. These samples were separated using 6% native polyacrylamide gel electrophoresis in 0.5 × Tris-borate-EDTA buffer and transferred by electroblotting to a positively charged nylon membrane. Finally, immunological treatment and chemiluminescent signal detection were performed according to the instructions for the second generation DIG Gel Shift Kit (Roche Applied Sciences, Mannheim, Germany).
DNase I Footprinting Assay
The DNase I footprinting assay was performed using the method of Zianni et al. (2006). The probes of the DNase I footprinting assay were amplified using specific primer sets (Supplementary Table 2). These probes contained the promoter regions or the 3′ end region of the protease genes. The 5′-ends of the sense and antisense probes were labeled with 6-carboxyfluorescein. In the binding reaction, 75 ng labeled probe and various quantities of His-OpaR protein were mixed in 20 μL binding buffer (second generation DIG Gel Shift Kit) and incubated at 25°C for 30 min. We then added 0.015 U DNase I (Thermo scientific, Germany) at 25°C for 1 min to digest the DNA–protein complex and ceased this reaction using 2.77 μL stop solution (5 mM EDTA) at 65°C for 10 min. The Agencourt AMPure XP PCR Purification Kit (Beckman Coulter) was used to purify the digested DNA fragments. Subsequently, 1 μL digested DNA fragments were added to a mixture of 8.5 μL highly deionized formamide and 0.5 μL GeneScanTM LIZ 600 Size Standard (Applied Biosystems). The samples were analyzed using the ABI 3730xl DNA Analyzer (Applied Biosystems), and the results were analyzed using Peak Scanner Software v1.0 (Applied Biosystems).
Statistical Analysis
Statistical differences between the three samples were measured using Student’s t-test with a two-tailed distribution. P < 0.05 or P < 0.01 indicated statistical significance.
Data Availability Statement
The ChIP-seq data have been submitted to the National Center for Biotechnology Information’s Gene Expression Omnibus (GEO) database (accession number: GSE122479).
Author Contributions
S-CC and C-YL designed the study. S-CC performed the experiments and data analyses. C-YL supervised and coordinated the project. S-CC wrote the preliminary draft of this manuscript and C-YL revised the manuscript. Both authors have reviewed and approved the manuscript.
Funding
This study was supported by the Ministry of Science and Technology of Taiwan under grant no. MOST 104-2313-B-002012 and 106-2313-B-002-024.
Conflict of Interest
The authors declare that the research was conducted in the absence of any commercial or financial relationships that could be construed as a potential conflict of interest.
Acknowledgments
We thank Dr. Hsing-Chen Tsai (National Taiwan University) and Ms. Hsiao-Ting Fu for their assistance of Bioruptor® Pico (Diagenode) shearing machine use in ChIP-seq assay. We used the BioRad CFX96 system at Technology Commons at the College of Life Science of National Taiwan University.
Supplementary Material
The Supplementary Material for this article can be found online at: https://www.frontiersin.org/articles/10.3389/fmicb.2020.534692/full#supplementary-material
Footnotes
References
Armenteros, J. J. A., Tsirigos, K. D., Sonderby, C. K., Petersen, T. N., Winther, O., Brunak, S., et al. (2019). SignalP 5.0 improves signal peptide predictions using deep neural networks. Nat. Biotechnol. 37, 420–430. doi: 10.1038/s41587-019-0036-z
Babu, M. M., and Teichmann, S. A. (2003). Functional determinants of transcription factors in Escherichia coli: protein families and binding sites. Trends Genet. 19, 75–79. doi: 10.1016/s0168-9525(02)00039-2
Bailey, T. L. (2011). DREME: motif discovery in transcription factor ChIP-seq data. Bioinformatics 27, 1653–1659. doi: 10.1093/bioinformatics/btr261
Bailey, T. L., Boden, M., Buske, F. A., Frith, M., Grant, C. E., Clementi, L., et al. (2009). MEME SUITE: tools for motif discovery and searching. Nucl. Acids Res. 37, W202–W208.
Baird, L., Lipinska, B., Raina, S., and Georgopoulos, C. (1991). Identification of the Escherichia coli sohb gene, a multicopy suppressor of the HtrA (Degp) null phenotype. J. Bacteriol. 173, 5763–5770. doi: 10.1128/jb.173.18.5763-5770.1991
Ball, A. S., Chaparian, R. R., and van Kessel, J. C. (2017). Quorum sensing gene regulation by LuxR/HapR master regulators in Vibrios. J. Bacteriol. 199, e105–e117.
Ball, A. S., and van Kessel, J. C. (2019). The master quorum-sensing regulators LuxR/HapR directly interact with the alpha subunit of RNA polymerase to drive transcription activation in Vibrio harveyi and Vibrio cholerae. Mol. Microbiol. 5, 1317–1334. doi: 10.1111/mmi.14223
Benitez, J. A., and Silva, A. J. (2016). Vibrio cholerae hemagglutinin (HA)/protease: an extracellular metalloprotease with multiple pathogenic activities. Toxicon 115, 55–62. doi: 10.1016/j.toxicon.2016.03.003
Bradford, M. M. (1976). Rapid and sensitive method for quantitation of microgram quantities of protein utilizing principle of protein-dye binding. Anal. Biochem. 72, 248–254. doi: 10.1016/0003-2697(76)90527-3
Broberg, C. A., Calder, T. J., and Orth, K. (2011). Vibrio parahaemolyticus cell biology and pathogenicity determinants. Microbes Infect. 13, 992–1001. doi: 10.1016/j.micinf.2011.06.013
Bullock, W. O., Fernandez, J. M., and Short, J. M. (1987). XL1-Blue - a high efficiency plasmid transforming recA Escherichia coli strain with beta-galactosidase selection. Biotechniques 5, 376–379.
Burke, A. K., Guthrie, L. T. C., Modise, T., Cormier, G., Jensen, R. V., McCarter, L. L., et al. (2015). OpaR controls a network of downstream transcription factors in Vibrio parahaemolyticus BB22OP. PLoS One 10:e0121863. doi: 10.1371/journal.pone.0121863
Cai, S., Wu, Z., Jian, J., and Lu, Y. (2007). Cloning and expression of the gene encoding an extracellular alkaline serine protease from Vibrio alginolyticus strain HY9901, the causative agent of vibriosis in Lutjanus erythopterus (Bloch). J. Fish Dis. 30, 493–500. doi: 10.1111/j.1365-2761.2007.00835.x
Chang, S. C., and Lee, C. Y. (2018). OpaR and RpoS are positive regulators of a virulence factor PrtA in Vibrio parahaemolyticus. Microbiol. Sgm 164, 221–231. doi: 10.1099/mic.0.000591
Chimalapati, S., de Souza Santos, M., Servage, K., De Nisco, N. J., Dalia, A. B., and Orth, K. (2018). Natural transformation of Vibrio parahaemolyticus: a rapid method to create genetic deletions. J. Bacteriol. 200, e00032-18.
Delorenzo, V., Eltis, L., Kessler, B., and Timmis, K. N. (1993). Analysis of Pseudomonas gene products using lacIq/Ptrp−lac plasmids and transposons that confer conditional phenotypes. Gene 123, 17–24. doi: 10.1016/0378-1119(93)90533-9
Dong, T. G., and Mekalanos, J. J. (2012). Characterization of the RpoN regulon reveals differential regulation of T6SS and new flagellar operons in Vibrio cholerae O37 strain V52. Nucl. Acids Res. 40, 7766–7775. doi: 10.1093/nar/gks567
Dorman, M. J., and Dorman, C. J. (2018). Regulatory hierarchies controlling virulence gene expression in Shigella flexneri and Vibrio cholerae. Front. Microbiol. 9:2686. doi: 10.3389/fmicb.2018.02686
Ferreira, R. B. R., Chodur, M. D., Antunes, M. C. L., Trimble, J. M., and McCarter, L. (2012). Output targets and transcriptional regulation by a cyclic dimeric GMP-responsive circuit in the Vibrio parahaemolyticus Scr network. J. Bacteriol. 194, 914–924. doi: 10.1128/jb.05807-11
Gao, X. A., Wang, J., Yu, D. Q., Bian, F., Xie, B. B., Chen, X. L., et al. (2010). Structural basis for the autoprocessing of zinc metalloproteases in the thermolysin family. Proc. Natl. Acad. Sci. U.S.A. 107, 17569–17574. doi: 10.1073/pnas.1005681107
Gode-Potratz, C. J., Chodur, D. M., and McCarter, L. L. (2010). Calcium and iron regulate swarming and type III secretion in Vibrio parahaemolyticus. J. Bacteriol. 192, 6025–6038. doi: 10.1128/jb.00654-10
Gode-Potratz, C. J., Kustusch, R. J., Breheny, P. J., Weiss, D. S., and McCarter, L. L. (2011). Surface sensing in Vibrio parahaemolyticus triggers a programme of gene expression that promotes colonization and virulence. Mol. Microbiol. 79, 240–263. doi: 10.1111/j.1365-2958.2010.07445.x
Gode-Potratz, C. J., and McCarter, L. L. (2011). Quorum sensing and silencing in Vibrio parahaemolyticus. J. Bacteriol. 193, 4224–4237. doi: 10.1128/jb.00432-11
Kanatani, A., Masuda, T., Shimoda, T., Misoka, F., Lin, X. S., Yoshimoto, T., et al. (1991). Protease-II from Escherichia coli: sequencing and expression of the enzyme gene and characterization of the expressed enzyme. J. Biochem. Tokyo 110, 315–320. doi: 10.1093/oxfordjournals.jbchem.a123577
Kanatani, A., Yoshimoto, T., Nagai, H., Ito, K., and Tsuru, D. (1992). Location of the protease-II gene (Ptrb) on the physical map of the Escherichia coli chromosome. J. Bacteriol. 174:7881. doi: 10.1128/jb.174.23.788.1992
Kim, J. A., Park, J. H., Lee, M. A., Lee, H. J., Park, S. J., Kim, K. S., et al. (2015). Stationary-phase induction of vvpS expression by three transcription factors: repression by LeuO and activation by SmcR and CRP. Mol. Microbiol. 97, 330–346. doi: 10.1111/mmi.13028
Kleinman, C. L., Sycz, G., Bonomi, H. R., Rodriguez, R. M., Zorreguieta, A., and Sieira, R. (2017). ChIP-seq analysis of the LuxR-type regulator VjbR reveals novel insights into the Brucella virulence gene expression network. Nucl. Acids Res. 45, 5757–5769. doi: 10.1093/nar/gkx165
Lazazzera, B. A. (2000). Quorum sensing and starvation: signals for entry into stationary phase. Curr. Opin. Microbiol. 3, 177–182. doi: 10.1016/s1369-5274(00)00072-2
Lee, C. Y., Cheng, M. F., Yu, M. S., and Pan, M. J. (2002). Purification and characterization of a putative virulence factor, serine protease, from Vibrio parahaemolyticus. FEMS Microbiol. Lett. 209, 31–37. doi: 10.1111/j.1574-6968.2002.tb11105.x
Letchumanan, V., Chan, K. G., and Lee, L. H. (2014). Vibrio parahaemolyticus: a review on the pathogenesis, prevalence, and advance molecular identification techniques. Front. Microbiol. 5:705. doi: 10.3389/fmicb.2014.00705
Liang, W., Pascual-Montano, A., Silva, A. J., and Benitez, J. A. (2007). The cyclic AMP receptor protein modulates quorum sensing, motility and multiple genes that affect intestinal colonization in Vibrio cholerae. Microbiol. Sgm 153, 2964–2975. doi: 10.1099/mic.0.2007/006668-0
Lim, M. S., Kim, J. A., Lim, J. G., Kim, B. S., Jeong, K. C., Lee, K. H., et al. (2011). Identification and characterization of a novel serine protease, VvpS, that contains two functional domains and is essential for autolysis of Vibrio vulnificus. J. Bacteriol. 193, 3722–3732. doi: 10.1128/jb.00314-11
Lu, R., Tang, H., Qiu, Y., Yang, W., Yang, H., Zhou, D., et al. (2019). Quorum sensing regulates the transcription of lateral flagellar genes in Vibrio parahaemolyticus. Fut. Microbiol. 14, 1043–1053. doi: 10.2217/fmb-2019-0048
Luan, X. Y., Chen, J. X., Zhang, X. H., Li, Y., and Hu, G. B. (2007). Expression and characterization of a metalloprotease from a Vibrio parahaemolyticus isolate. Can. J. Microbiol. 53, 1168–1173.
Mathur, J., Davis, B. M., and Waldor, M. K. (2007). Antimicrobial peptides activate the Vibrio cholerae sigma (E) regulon through an OmpU-dependent signalling pathway. Mol. Microbiol. 63, 848–858.
Miller, M. B., and Bassler, B. L. (2001). Quorum sensing in bacteria. Annu. Rev. Microbiol. 55, 165–199.
Miyoshi, S. (2013). Extracellular proteolytic enzymes produced by human pathogenic Vibrio species. Front. Microbiol. 4:339. doi: 10.3389/fmicb.2013.00339
Miyoshi, S., Nitanda, Y., Fujii, K., Kawahara, K., Li, T., Maehara, Y., et al. (2008). Differential gene expression and extracellular secretion of the collagenolytic enzymes by the pathogen Vibrio parahaemolyticus. Fems Microbiol. Lett. 283, 176–181. doi: 10.1111/j.1574-6968.2008.01159.x
Naville, M., Ghuillot-Gaudeffroy, A., Marchais, A., and Gautheret, D. (2011). ARNold: a webtool for the prediction of Rho-independent transcription terminators. RNA Biol. 8, 11–13. doi: 10.4161/rna.8.1.13346
Osei-Adjei, G., Huang, X., and Zhang, Y. (2018). The extracellular proteases produced by Vibrio parahaemolyticus. World J. Microbiol. Biotechnol. 34, 68.
Papenfort, K., and Bassler, B. L. (2016). Quorum sensing signal-response systems in Gram-negative bacteria. Nat. Rev. Microbiol. 14, 576–588. doi: 10.1038/nrmicro.2016.89
Pérez-Acosta, J. A., Martínez-Porchas, M., Elizalde-Contreras, J. M., Leyva, J. M., Ruiz-May, E., Gollas-Galván, T., et al. (2018). Proteomic profiling of integral membrane proteins associated to pathogenicity in Vibrio parahaemolyticus strains. Microbiol Immunol 62, 14–23. doi: 10.1111/1348-0421.12556
Philippe, N., Alcaraz, J. P., Coursange, E., Geiselmann, J., and Schneider, D. (2004). Improvement of pCVD442, a suicide plasmid for gene allele exchange in bacteria. Plasmid 51, 246–255. doi: 10.1016/j.plasmid.2004.02.003
Pompeani, A. J., Irgon, J. J., Berger, M. F., Bulyk, M. L., Wingreen, N. S., and Bassler, B. L. (2008). The Vibrio harveyi master quorum-sensing regulator, LuxR, a TetR-type protein is both an activator and a repressor: DNA recognition and binding specificity at target promoters. Mol. Microbiol. 70, 76–88. doi: 10.1111/j.1365-2958.2008.06389.x
Rawlings, N. D., Alan, J., Thomas, P. D., Huang, X. D., Bateman, A., and Finn, R. D. (2018). The MEROPS database of proteolytic enzymes, their substrates and inhibitors in 2017 and a comparison with peptidases in the PANTHER database. Nucl. Acids Res. 46, D624–D632.
Rompikuntal, P. K., Vdovikova, S., Duperthuy, M., Johnson, T. L., Ahlund, M., Lundmark, R., et al. (2015). Outer membrane vesicle-mediated export of processed PrtV protease from Vibrio cholerae. PLoS One 10:e0134098. doi: 10.1371/journal.pone.0134098
Rui, H., Liu, Q., Wang, Q., Ma, Y., Liu, H., Shi, C., et al. (2009). Role of alkaline serine protease, Asp, in Vibrio alginolyticus virulence and regulation of its expression by LuxO-LuxR regulatory system. J. Microbiol. Biotechnol. 19, 431–438. doi: 10.4014/jmb.0807.404
Salamone, M., Nicosia, A., Bennici, C., Quatrini, P., Catania, V., Mazzola, S., et al. (2015). Comprehensive analysis of a Vibrio parahaemolyticus strain extracellular serine protease VpSP37. PLoS One 10:e0126349. doi: 10.1371/journal.pone.0126349
Schafer, A., Kalinowski, J., Simon, R., Seepfeldhaus, A. H., and Puhler, A. (1990). High-frequency conjugal plasmid transfer from gram-negative Escherichia coli to various gram-positive coryneform bacteria. J. Bacteriol. 172, 1663–1666. doi: 10.1128/jb.172.3.1663-1666.1990
Silva, A. J., Pham, K., and Benitez, J. A. (2003). Haemagglutinin/protease expression and mucin gel penetration in El Tor biotype Vibrio cholerae. Microbiol. Sgm 149, 1883–1891. doi: 10.1099/mic.0.26086-0
Su, Y. C., and Liu, C. C. (2007). Vibrio parahaemolyticus: a concern of seafood safety. Food Microbiol. 24, 549–558. doi: 10.1016/j.fm.2007.01.005
Sun, F., Zhang, Y., Wang, L., Yan, X., Tan, Y., Guo, Z., et al. (2012). Molecular characterization of direct target genes and cis-acting consensus recognized by quorum-sensing regulator AphA in Vibrio parahaemolyticus. PLoS One 7:e44210. doi: 10.1371/journal.pone.0044210
Syngkon, A., Elluri, S., Koley, H., Rompikuntal, P. K., Saha, D. R., Chakrabarti, M. K., et al. (2010). Studies on a novel serine protease of a ΔhapAΔprtV Vibrio cholerae O1 strain and its role in hemorrhagic response in the rabbit ileal loop model. PLoS One 5:e13122. doi: 10.1371/journal.pone.0013122.g004
Theethakaew, C., Nakamura, S., Motooka, D., Matsuda, S., Kodama, T., Chonsin, K., et al. (2017). Plasmid dynamics in Vibrio parahaemolyticus strains related to shrimp acute hepatopancreatic necrosis syndrome (AHPNS). Infect. Genet. Evol. 51, 211–218. doi: 10.1016/j.meegid.2017.04.007
Vaitkevicius, K., Rompikuntal, P. K., Lindmark, B., Vaitkevicius, R., Song, T. Y., and Wai, S. N. (2008). The metalloprotease PrtV from Vibrio cholerae. Febs. J. 275, 3167–3177. doi: 10.1111/j.1742-4658.2008.06470.x
van Kessel, J. C., Rutherford, S. T., Shao, Y., Utria, A. F., and Bassler, B. L. (2013a). Individual and combined roles of the master regulators AphA and LuxR in control of the Vibrio harveyi quorum-sensing regulon. J. Bacteriol. 195, 436–443. doi: 10.1128/jb.01998-12
van Kessel, J. C., Ulrich, L. E., Zhulin, I. B., and Bassler, B. L. (2013b). Analysis of activator and repressor functions reveals the requirements for transcriptional control by LuxR, the master regulator of quorum sensing in Vibrio harveyi. mBio 4, e00378-13.
Wang, H. X., Wu, J. H., Ayala, J. C., Benitez, J. A., and Silva, A. J. (2011). Interplay among cyclic diguanylate, HapR, and the general stress response regulator (RpoS) in the regulation of Vibrio cholerae hemagglutinin/protease. J. Bacteriol. 193, 6529–6538. doi: 10.1128/jb.05166-11
Wang, L., Zhou, D., Mao, P., Zhang, Y., Hou, J., Hu, Y., et al. (2013). Cell density-and quorum sensing-dependent expression of type VI secretion system 2 in Vibrio parahaemolyticus. PLoS One 8:e73363. doi: 10.1371/journal.pone.0073363
Waters, C. M., and Bassler, B. L. (2006). The Vibrio harveyi quorum-sensing system uses shared regulatory components to discriminate between multiple autoinducers. Gene Dev. 20, 2754–2767. doi: 10.1101/gad.1466506
Weber, B., Croxatto, A., Chen, C., and Milton, D. L. (2008). RpoS induces expression of the Vibrio anguillarum quorum-sensing regulator VanT. Microbiol. Sgm 154, 767–780. doi: 10.1099/mic.0.2007/014167-0
Whiteley, M., Diggle, S. P., and Greenberg, E. P. (2017). Progress in and promise of bacterial quorum sensing research. Nature 551, 313–320. doi: 10.1038/nature24624
Yu, M. S., Yap, M. N., and Lee, C. Y. (2000). Metal content and biochemical analyses of a recombinant collagenase PrtV from Vibrio parahaemolyticus. Microbiol. Immunol. 44, 805–813. doi: 10.1111/j.1348-0421.2000.tb02567.x
Zhang, Y. Q., Qiu, Y. F., Tan, Y. F., Guo, Z. B., Yang, R. F., and Zhou, D. S. (2012). Transcriptional Regulation of opaR, qrr2-4 and aphA by the master quorum-sensing regulator OpaR in Vibrio parahaemolyticus. PLoS One 7:e34622. doi: 10.1371/journal.pone.0034622
Keywords: quorum sensing, OpaR-binding motif, Vibrio parahaemolyticus, ChIP-seq, proteases regulation, EMSA, DNase I footprinting
Citation: Chang S-C and Lee C-Y (2020) Quorum-Sensing Regulator OpaR Directly Represses Seven Protease Genes in Vibrio parahaemolyticus. Front. Microbiol. 11:534692. doi: 10.3389/fmicb.2020.534692
Received: 13 February 2020; Accepted: 08 October 2020;
Published: 29 October 2020.
Edited by:
Dieter Jahn, Technische Universitat Braunschweig, GermanyReviewed by:
Pendru Raghunath, Texila American University, GuyanaLuis Caetano Martha Antunes, Oswaldo Cruz Foundation (Fiocruz), Brazil
Copyright © 2020 Chang and Lee. This is an open-access article distributed under the terms of the Creative Commons Attribution License (CC BY). The use, distribution or reproduction in other forums is permitted, provided the original author(s) and the copyright owner(s) are credited and that the original publication in this journal is cited, in accordance with accepted academic practice. No use, distribution or reproduction is permitted which does not comply with these terms.
*Correspondence: Chia-Yin Lee, Y2xlZUBudHUuZWR1LnR3