- 1Microbiology and Infection Unit, Warwick Medical School, The University of Warwick, Coventry, United Kingdom
- 2School of Biological Sciences, University of Reading, Reading, United Kingdom
Temperature plays an important role in bacteria-host interactions and can be a determining factor for host switching. In this study we sought to investigate the reasons behind growth temperature restriction in the entomopathogenic enterobacterium Photorhabdus. Photorhabdus has a complex dual symbiotic and pathogenic life cycle. The genus consists of 19 species but only one subgroup, previously all classed together as Photorhabdus asymbiotica, have been shown to cause human disease. These clinical isolates necessarily need to be able to grow at 37°C, whilst the remaining species are largely restricted to growth temperatures below 34°C and are therefore unable to infect mammalian hosts. Here, we have isolated spontaneous mutant lines of Photorhabdus laumondii DJC that were able to grow up to 36–37°C. Following whole genome sequencing of 29 of these mutants we identified a single gene, encoding a protein with a RecG-like helicase domain that for the majority of isolates contained single nucleotide polymorphisms. Importantly, provision of the wild-type allele of this gene in trans restored the temperature restriction, confirming the mutations are recessive, and the dominant effect of the protein product of this gene. The gene appears to be part of a short three cistron operon, which we have termed the Temperature Restricting Locus (TRL). Transcription reporter strains revealed that this operon is induced upon the switch from 30 to 36°C, leading to replication arrest of the bacteria. TRL is absent from all of the human pathogenic species so far examined, although its presence is not uniform in different strains of the Photorhabdus luminescens subgroup. In a wider context, the presence of this gene is not limited to Photorhabdus, being found in phylogenetically diverse proteobacteria. We therefore suggest that this system may play a more fundamental role in temperature restriction in diverse species, relating to as yet cryptic aspects of their ecological niches and life cycle requirements.
Introduction
Photorhabdus is a Gram-negative bacterium that forms a symbiotic relationship with Heterorhabditis nematodes. The Heterorhabditis-Photorhabdus complex is entomopathogenic with the nematodes infecting insects and releasing the bacteria by regurgitation (Ciche and Ensign, 2003). Photorhabdus then sets up a lethal septicemia, producing an array of toxins and degradative enzymes that combat the immune system and kill the insect. They also elaborate a potent cocktail of antimicrobial compounds to eliminate competition by other microbes in the carcass. The bacteria reproduce using nutrients derived from the insect tissues, reaching very high numbers. The nematodes feed on this bacterial biomass, going through several rounds of hermaphroditic reproduction, before eventually re-establishing the symbiosis with the bacteria (reviewed in Waterfield et al., 2009). The Photorhabdus genus, which belongs to the class of γ-proteobacteria, was thought to consist of three species Photorhabdus asymbiotica, Photorhabdus Luminescens, and Photorhabdus temperata. Recently, however, it has been proposed that several of the subspecies be elevated to species level resulting in a total of 19 species (Machado et al., 2018). Nevertheless, for the purposes of this study we will refer to these as belonging to the P. asymbiotica, P. luminescens, and P. temperata subgroups. Thus, so far, only species belonging to the P. asymbiotica subgroup (that can grow up to 37°C or above) have been identified as the causative agent of human infections (Gerrard and Stevens, 2017). The only exception to this was a strain of P. luminescens, identified using 16S sequencing, which was associated with cutaneous lesions and bacteremia in a hypothermic infant with a body temperature upon admission of 33.6°C (Dutta et al., 2018). No other members of the subgroups P. luminescens and P. temperata, the majority of which are largely restricted to growth temperatures below 37°C (Fischer-Le Saux et al., 1999; Tailliez et al., 2010; Machado et al., 2018), have been reported to cause human disease. The exact reasons behind this discrepancy are unknown.
The establishment and maintenance of symbiosis is also a very important aspect of the Photorhabdus life cycle. There is considerable partner preference in the association between Photorhabdus bacteria and the Heterorhabditis nematodes, although this is not strictly species-specific (Kazimierczak et al., 2017; Maher et al., 2017). Nonetheless, there is evidence for the co-evolution of the two partners (Maneesakorn et al., 2011). It may therefore be of relevance, that the optimum temperature for the nematodes is also relatively low. For example, in our hands the Photorhabdus laumondii DJC Heterorhabditid nematode partner dies in culture above 34°C (unpublished data).
Photorhabdus laumondii subsp. laumondii DJC is a laboratory strain of Photorhabdus which is normally unable to grow at temperatures above 35°C on solid Lysogeny broth (LB) media. In this study we have isolated mutants of P. laumondii DJC with abilities to grow between 36 and 37°C.
Results
Isolation and Characterization of Temperature Tolerant P. laumondii DJC Mutants
Clones of P. laumondii DJC were isolated with the ability to grow on LB agar (LBA) at 36°C. This was following plating of a saturated overnight culture and incubation of the plates at the higher temperature. The process was repeated two more times and colonies were isolated from all three experiments originating from four independent lineages in total. Isolates were re-streaked four times at 36°C to select for stably growing clones. Overall, the average frequency with which stable clones were isolated was 2.6 ± 2.5 × 10–6. Thirty of those were then analyzed by whole genome sequencing to identify any mutations that may give rise to the phenotype. Sequencing was performed successfully using the MiSeq platform for 29 of the 30 clones. The WT strain in the lab was also sequenced to account for variations between isolates in different laboratories. Reads from the temperature tolerant clones were then mapped against the reference to identify single nucleotide polymorphisms (SNPs).
Of the 29 isolates successfully sequenced, 19 had a SNP in an uncharacterized gene PluDJC_RS01875, and an additional isolate (S15) had an IS30-family transposase insertion near the 3′ end of the gene (Table 1). This gene appears in the automated annotation as “recG,” however it should be noted that a full-length ortholog of the bona fide recG is encoded elsewhere in the genome. RecG is a 693 amino acid protein whose homolog in other bacteria has been well studied and is known to be important for DNA repair and recombination (Ishioka et al., 1997; Rudolph et al., 2010). As PluDJC_RS01875 is involved in the temperature restriction phenotype, and to distinguish it from the recG misnomer, we subsequently named it trlG (temperature restriction locus G).
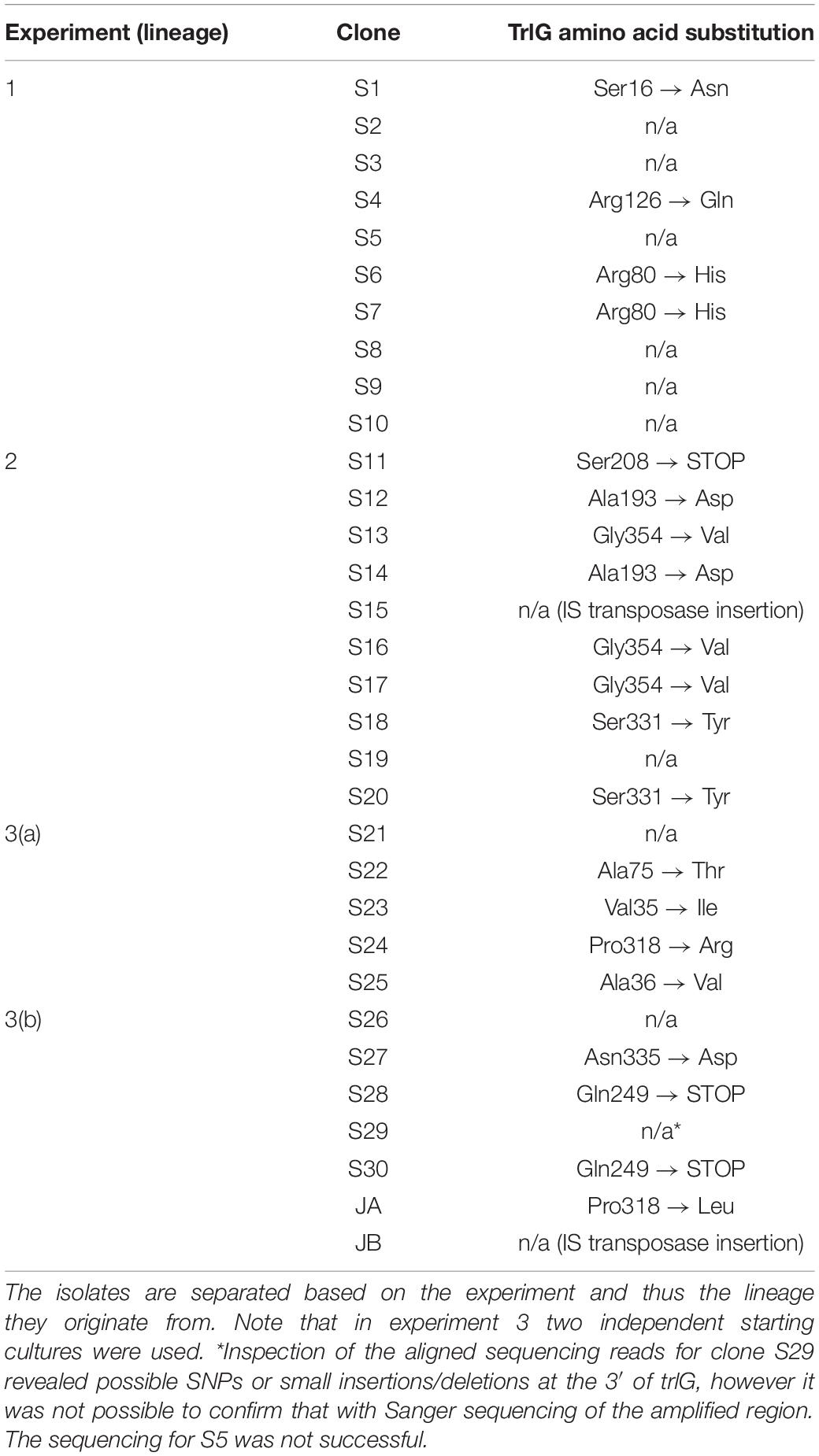
Table 1. TrlG amino acid substitutions that result from the SNPs identified in the sequenced isolates, as well as isolates JA and JB.
Repetition of these experiments aimed at isolating temperature tolerant clones by a collaborator in an independent laboratory (Dr. G. Mulley), gave rise to two additional tolerant isolates, named JA and JB. These were specifically tested for the presence of mutations in trlG by sequencing of a PCR amplicon. Clone JA was found to contain a SNP, while in clone JB an IS66 transposable element had inserted into the 3′ end of trlG (chromosomal position 393014). Homology and structural models of the TrlG protein suggest it comprises two distinct functional domains connected by a flexible loop region. Most of the mutant SNPs were located in either of the two domains. Additionally, three mutants exhibited premature stop codon mutations in the linker region (Figure 1B). Based on a 3D structure prediction of the protein, we can observe that four of the SNPs would result in substitutions in alpha helices potentially disrupting the structure (Figure 1C). The remaining isolates that did not have a mutation in trlG were actually unable to resume growth at 36°C after being frozen (Figure 2A). For the majority of those isolates we were unable to detect any other mutations with the exception of S10 and S21. Clone S10 carries a SNP at position 1454735, resulting in an Ala53Thr substitution in the product of the fur gene. Clone S21 carries a G to T mutation at position 300098, resulting in an Arg131Ser substitution in the product of the spoT gene. The growth of the isolates carrying mutations in trlG was similar to that of the WT bacteria in liquid media at 28°C (Supplementary Figure 1). However at 36°C, while the average growth rate during exponential phase was not different from that of the WT, the mutants were able to carry on growing to reach higher final densities, in contrast to the WT which entered stationary phase early (Supplementary Figure 1). It should be noted that the tolerant isolates are less pigmented and produce less bioluminescence at the higher temperature (Figure 2A and Supplementary Figure 2).
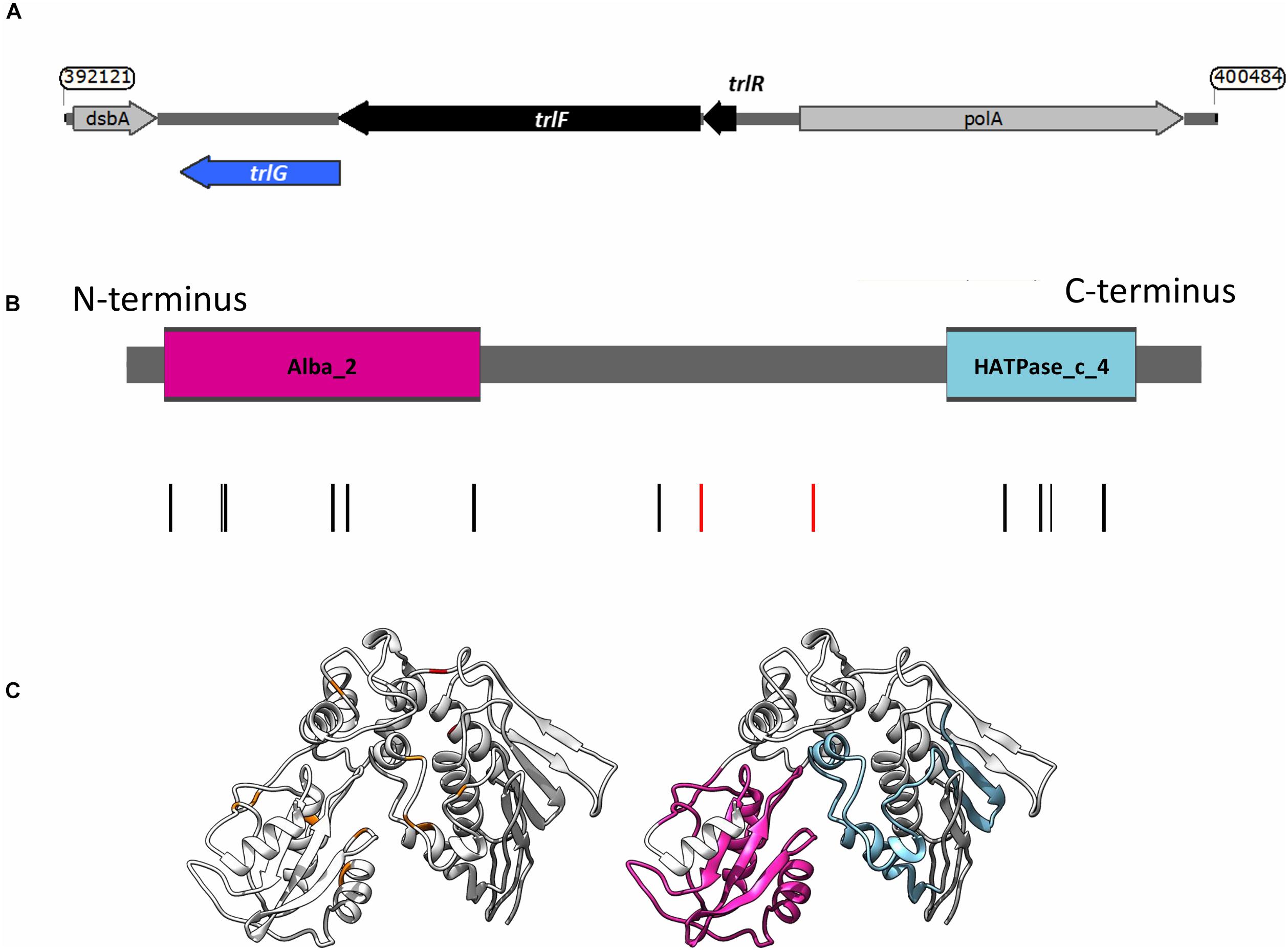
Figure 1. (A) Genetic organization of the temperature restriction locus. (B) Domain architecture of TrlG and the presence of substitution mutations in the temperature tolerant clones. Vertical lines indicate the positions of the mutations; red depicts stop mutations. The colored boxes show the domains identified by Pfam; Magenta: Alba_2 (Pfam domain PF04326, at amino acid positions 14–128) and light blue: HATPase_c_4 (Pfam domain PF13749, at amino acid positions 298–365). (C) (Left) I-TASSER simulation of TrlG with the distribution of mutations mapped. Orange indicates SNP positions, whilst red depicts stop mutations. (Right) The same model as in (Left) with homology domains mapped in color. Images prepared with UCSF Chimera.
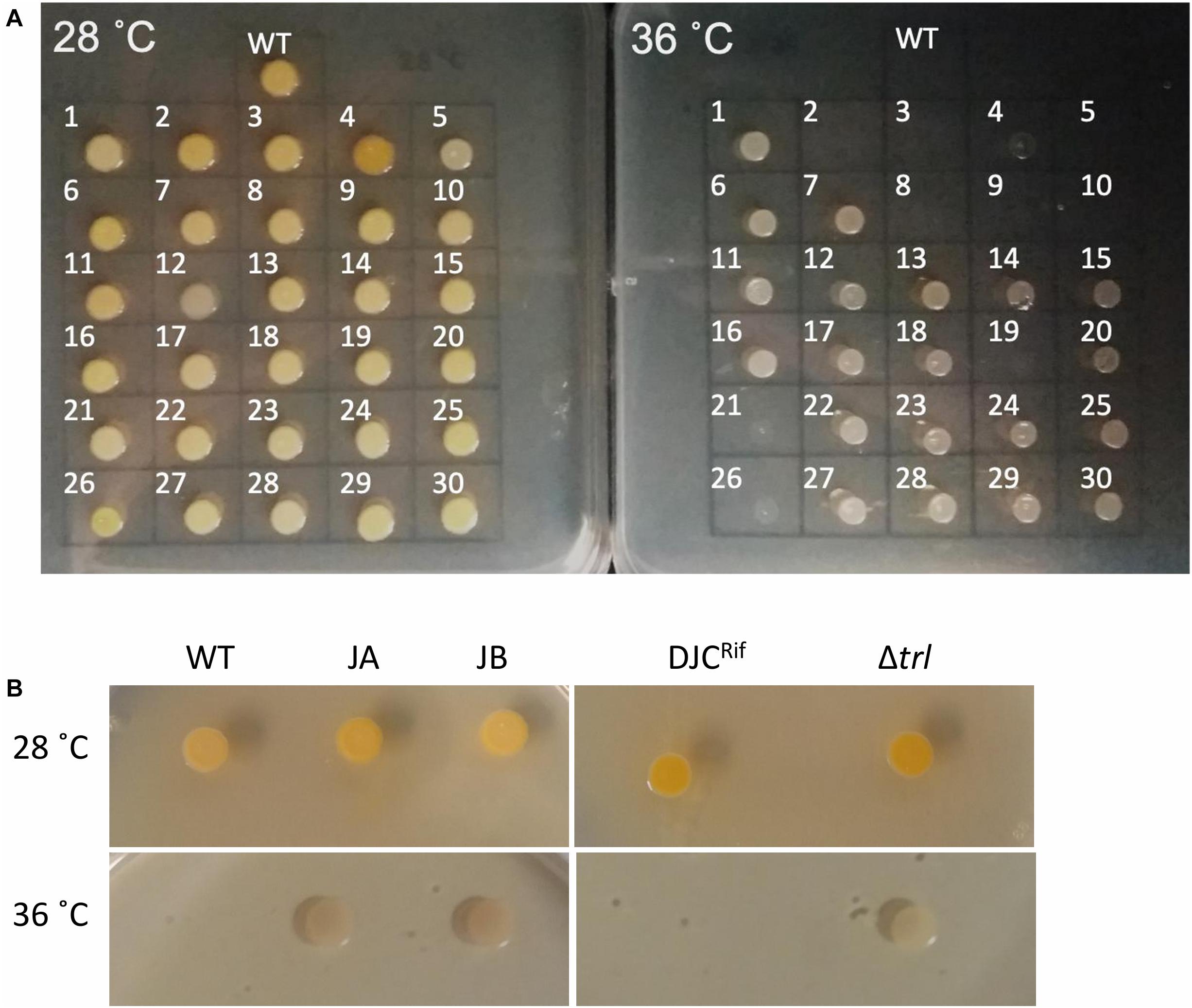
Figure 2. (A) Growth of the 30 sequenced tolerant clones and P. laumondii DJC (WT) on LB agar at 28 and 36°C. (B) Growth of the additional two clones isolated independently (JA and JB) compared to P. laumondii DJC (WT) as well as of the Δtrl deletion strain compared to the isogenic rifampicin resistant P. laumondii DJC (DJCRif) at 28 and 36°C.
The trlG Gene Is Part of a Small Putative Three Gene Operon
Examination of the genomic context of trlG revealed it is part of a small putative operon with PluDJC_RS01880 and PluDJC_RS01885, encoded between the DNA polymerase gene, polA, and dsbA (Figure 1A). PluDJC_RS01880 encodes a protein annotated as a histidinol phosphatase (WP_011144772.1). The product of PluDJC_RS01885 (WP_011144773.1) on the other hand is a small 79 amino acid protein annotated as a hypothetical protein but homologs in other organisms are annotated as transcription factors.
To further confirm the role of trlG in temperature restriction we constructed an unmarked deletion mutant of the entire Temperature Restricting Locus (TRL) operon, Δtrl. This mutant was also able to grow on both solid and liquid media at 36°C (Figure 2B). Importantly, plasmid trans-complementation with the whole operon restored the temperature restricted phenotype in the SNP mutants (Figure 3). Note, we used the whole operon rather than just trlG alone to ensure the stoichiometry of the three gene members was preserved.
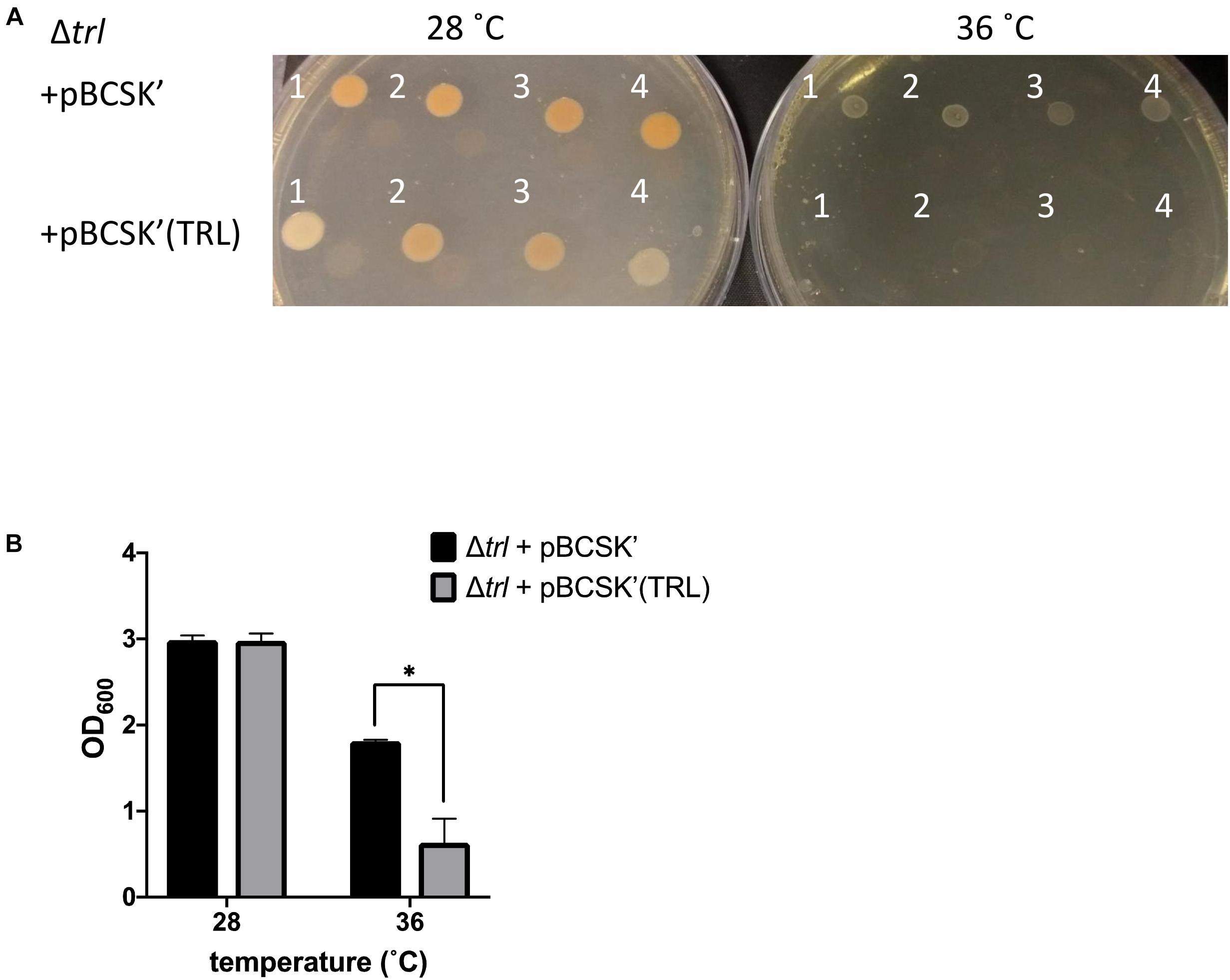
Figure 3. Growth of the Δtrl deletion mutant complemented with either the empty pBCSK′ vector or pBCSK′ carrying the TRL operon on (A) LB agar and (B) liquid LB media at 28 and 36°C. The asterisk denotes significant difference at padj < 0.05. Results show the averages and standard error from four biological replicates.
Finally, since virulence of the bacteria to insects is a crucial aspect of their life cycle, we determined if mutation of trlG would have any effect on virulence against Galleria mellonella larvae. The experiment was performed at both 36°C and room temperature (∼20°C), using a representative SNP mutant as well as the Δtrl deletion strain, compared to WT and the RifR isogenic parent strains, respectively. As expected the WT strain was not able to kill the Galleria at 36°C, although surprisingly, even though they could grow at this temperature in vitro, the mutant strains also failed to establish an infection. All strains had retained the ability to kill Galleria larvae at room temperature (Figure 4).
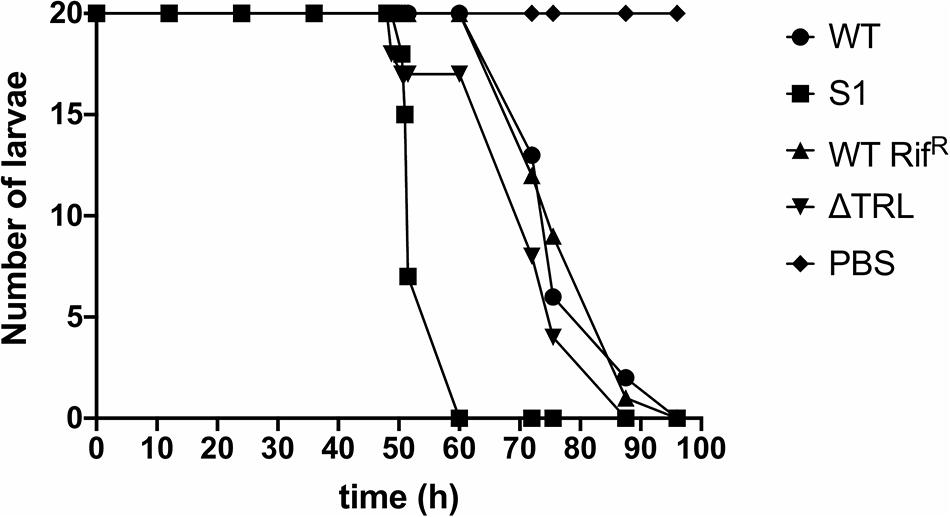
Figure 4. Virulence of the trl mutants at room temperature. The results show the number of surviving Galleria mellonella larvae infected with either the WT strain, the S1 SNP mutant, the RifR WT strain or the Δtrl deletion mutant at different time points. G. mellonella larvae injected with PBS were used as control. Twenty larvae were used for each strain and the experiment was repeated with similar results.
Transcription of the TRL Operon Is Induced by Temperature Up-Shift
To determine whether the TRL operon is specifically expressed in response to exposure to the higher temperature we created reporter constructs whereby we cloned regions upstream of the operon upstream of a promoterless GFP gene. An in silico search for promoters using BPROM (Solovyev and Salamov, 2011) showed the presence of two putative promoters upstream of the first gene in the TRL operon (PluDJC_RS01885, designated here as trlR). Interestingly, one of these promoters seems to be upstream of the predicted, oppositely encoded polA promoter. Analysis of apparent transcription start sites in our unpublished RNAseq analysis of P. laumondii supports the location of these predicted promoters. Additionally, a putative promoter containing a fur box is predicted, which is encoded inside the trlR open reading frame. With TrlR itself showing homology to a transcriptional regulator we hypothesized that this might be a legitimate promoter driving expression of trlF and trlG. We therefore decided to create multiple constructs to ensure that we capture the transcriptional elements that control expression of genes in the TRL operon (Figure 5A). These constructs were separately introduced back into a rifampicin resistant isolate of DJC and the effect of temperature up-shift tested. These experiments confirmed that transcription of the operon is increased upon temperature up-shift (Figures 5B,C). It should be noted that the growth of P. laumondii DJC following temperature up-shift does not immediately cease, but rather continues to increase briefly before reaching a plateau (Figure 5C and Supplementary Figure 3A). Moreover, at the end of the 24 h period and following a ∼20 h incubation at the higher temperature, a large proportion of cells remain viable as shown by live/dead staining (Supplementary Figure 3B). Importantly, the expression of these reporter constructs when the bacteria are grown at 28°C was also monitored and we observed that the operon is also expressed at the permissive growth temperature but expression shows a gradual increase as the bacteria go through late exponential and stationary phase (Figures 5B,C).
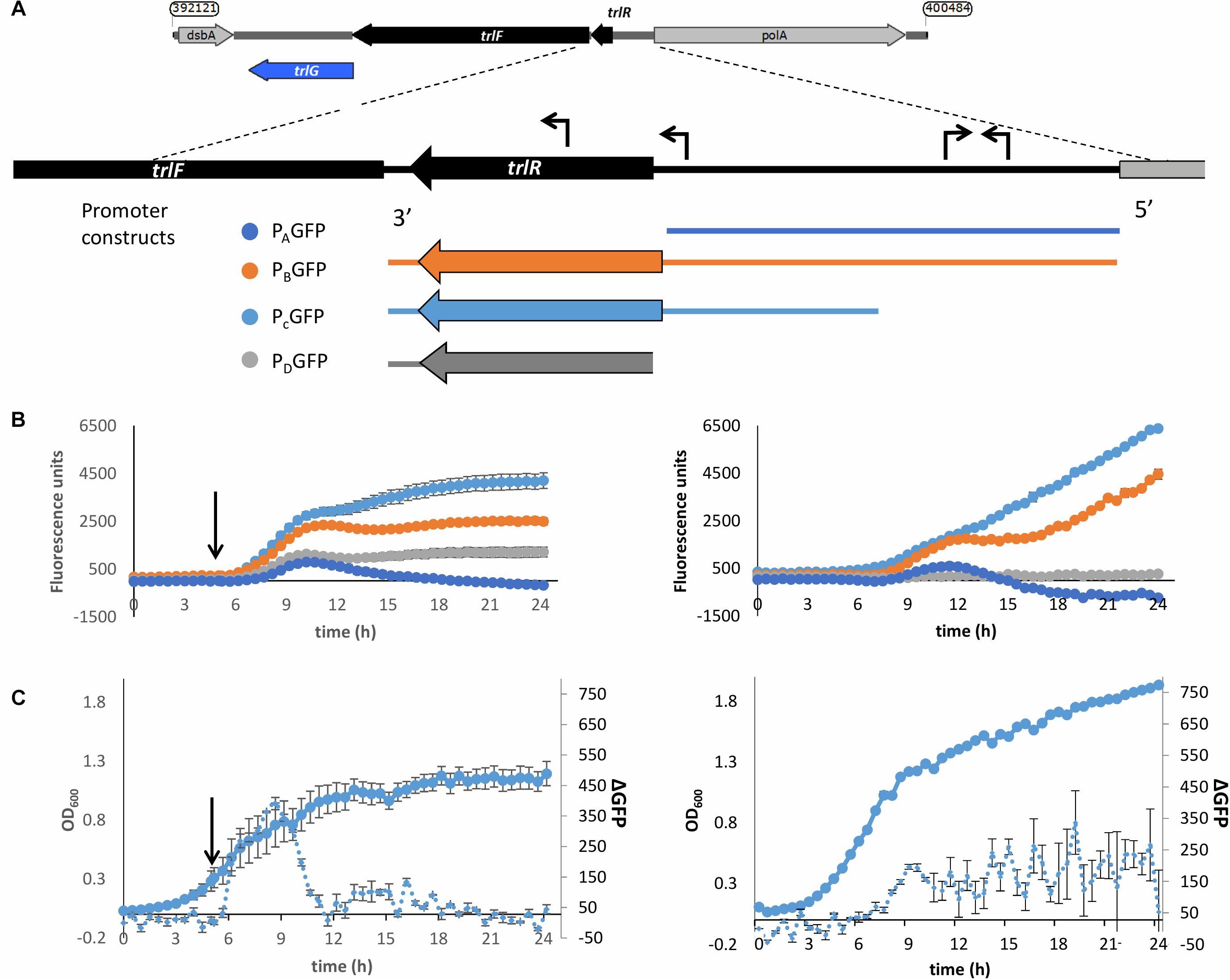
Figure 5. Up-regulation of the promoter of the TRL operon upon shift to 36°C. (A) Schematic diagram showing the different regions used in the promoter reporter constructs. The expanded panel shows the region between trlF (PluDJC_RS01880) and polA with the predicted promoters based on BPROM indicated as arrows on top of the sequence. At the bottom are the regions amplified and introduced separately into pGAG1 upstream of the gfpmut3 gene to create promoter constructs PAGFP-PDGFP. The diagrams are color coded to reflect the colors of the data plotted below. (B) Left: Fluorescence of the P. laumondii DJC reporter strains following a shift in temperature from 28 to 36°C after 5 h of growth as indicated by the arrow. Right: Fluorescence of the P. laumondii DJC reporter strains grown continuously at 28°C. Results on the secondary axis are arbitrary fluorescence units following a subtraction of the fluorescence of the P. laumondii DJC carrying pGAG1 in the absence of any promoter to account for any autofluorescence and they represent the mean of three biological replicates ± standard error. (C)The growth and rate of change in fluorescence in P. laumondii DJC carrying pGAG1(PCGFP), calculated by subtracting the fluorescence at a given time point by the fluorescence of the previous time point; left: following a shift in temperature from 28 to 36°C after 5 h of growth as indicated by the arrow; right: at 28°C. Results represent the mean of three biological replicates ± standard error.
Presence of the TRL in the Photorhabdus Genus
Since mutations in trlG appear to allow P. laumondii to survive in higher temperatures we were interested in investigating the presence of the gene among other Photorhabdus genomes. A trlG homolog was found in 21 out of the 46 Photorhabdus genomes in the RefSeq genome database (Figure 6 and Supplementary Figure 4). However, no trlG homologs were present in any bacteria belonging to the P. asymbiotica or P. temperata subgroups. Within the group of bacteria previously classed as P. luminescens the presence of the gene is not uniform. Those isolates that possess the gene actually encode for the full operon. Even though the majority of those genomes are in the draft sequencing stage, we investigated the location of the operon in the genome and observed that it appears to be conserved in the region between dsbA and polA. Importantly, in the published genome sequence of the type strain P. laumondii (Duchaud et al., 2003) an insertion sequence (IS) element is found upstream of the polA gene. However, we note that this IS element is absent in our WT P. laumondii DJC strain – which is a laboratory selected derivative of the original TT01 isolate (confirmed by PCR). Interestingly, Alien Hunter analysis (Vernikos and Parkhill, 2006) shows no evidence of horizontal gene transfer of this three gene TRL locus, suggesting it is ancestral in this sub-lineage of Photorhabdus. In addition to P. laumondii TT01, only P. laumondii strain DSPV002N possesses an IS630-like element ISPlu10 family transposase directly upstream of the operon. Four other isolates, P. laumondii HP88, Photorhabdus sp. S7-51, Photorhabdus sp. S15-56 and Photorhabdus sp. S14-60, appear to possess a gene encoding for a 61 amino acid hypothetical protein (WP_133148736.1), upstream of the operon but in the opposite strand.
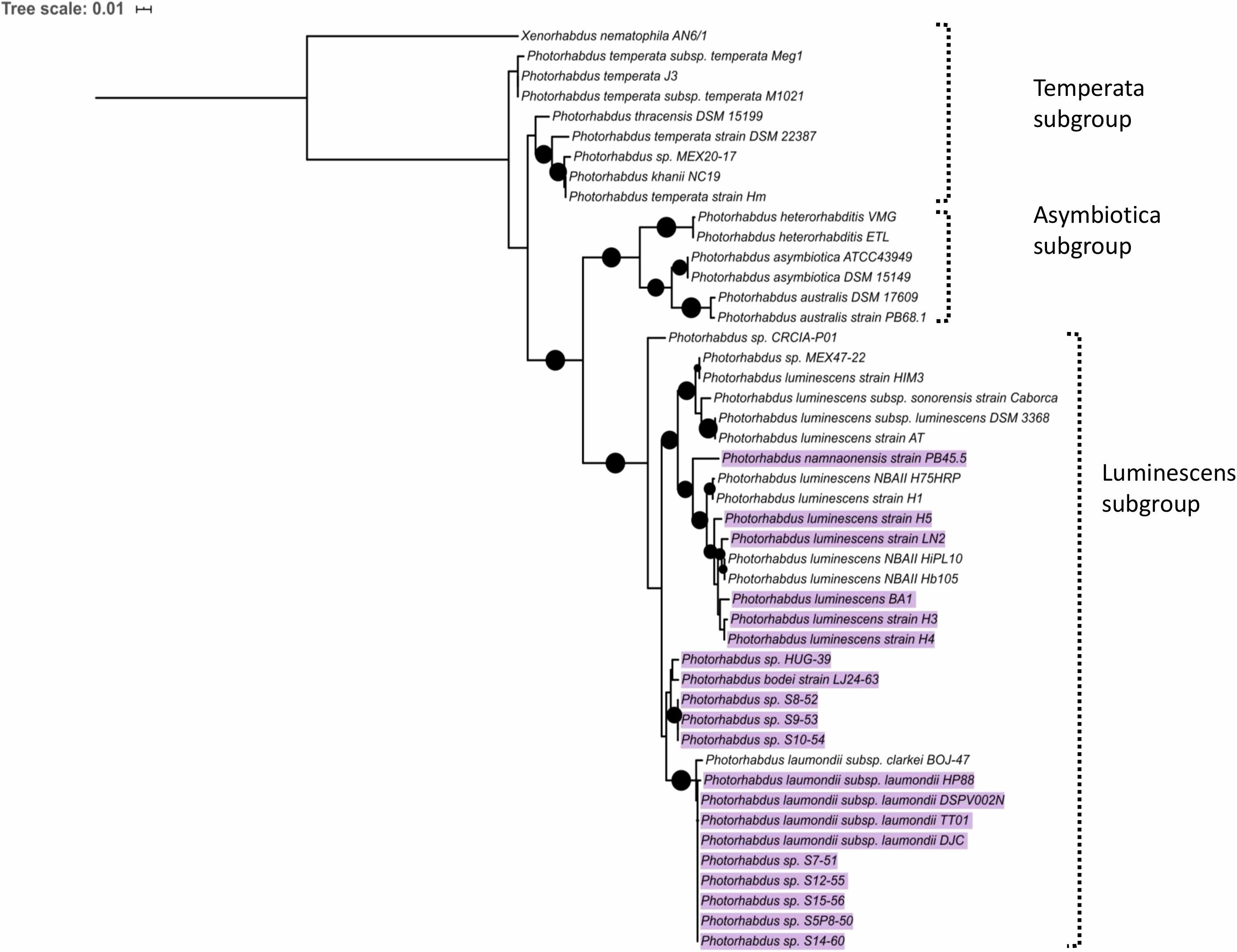
Figure 6. Maximum likelihood tree constructed using the Photorhabdus recA nucleotide sequences from RefSeq genomes. Black circles indicate branch support over 80%. The scale bar represents the number of substitutions per nucleotide position. Highlighted are the strains that were found to contain a copy of the trlG gene.
The TRL Is Conserved Amongst Bacterial Genera
The TRL is of relevance to bacteria beyond the Photorhabdus genus. Blastp analysis against the NCBI reference protein database showed that TrlG is prevalent in proteobacteria (Figure 7). The closest homolog outside of Photorhabdus is found in Vibrio parahaemolyticus followed by proteins encoded by Pseudospirillum japonicum and Nitrincola nitratireducens, which like Photorhabdus belong to the class of γ-proteobacteria. TrlG was, however, absent from the closely related Xenorhabdus, also a nematode endosymbiont. While the TrlG proteins of Photorhabdus and Glaeserella are all clustered together, for other bacteria this does not seem to be the case, suggesting individual acquisition events. Within the Vibrio genus, the gene is found in specific isolates of different species, such as Vibrio cholerae, Vibrio anguillarum, and Vibrio atlanticus. As an example, out of the 1218 V. cholerae genomes found in the RefSeq database, only 12 were found to encode for a homolog of TrlG (with over 40% sequence identity). Of note is the presence of TrlG in various Pseudomonas isolates. Some are classified as strains of the plant pathogens Pseudomonas syringae or Pseudomonas amygdali, however, the gene is also present in some isolates of Pseudomonas aeruginosa.
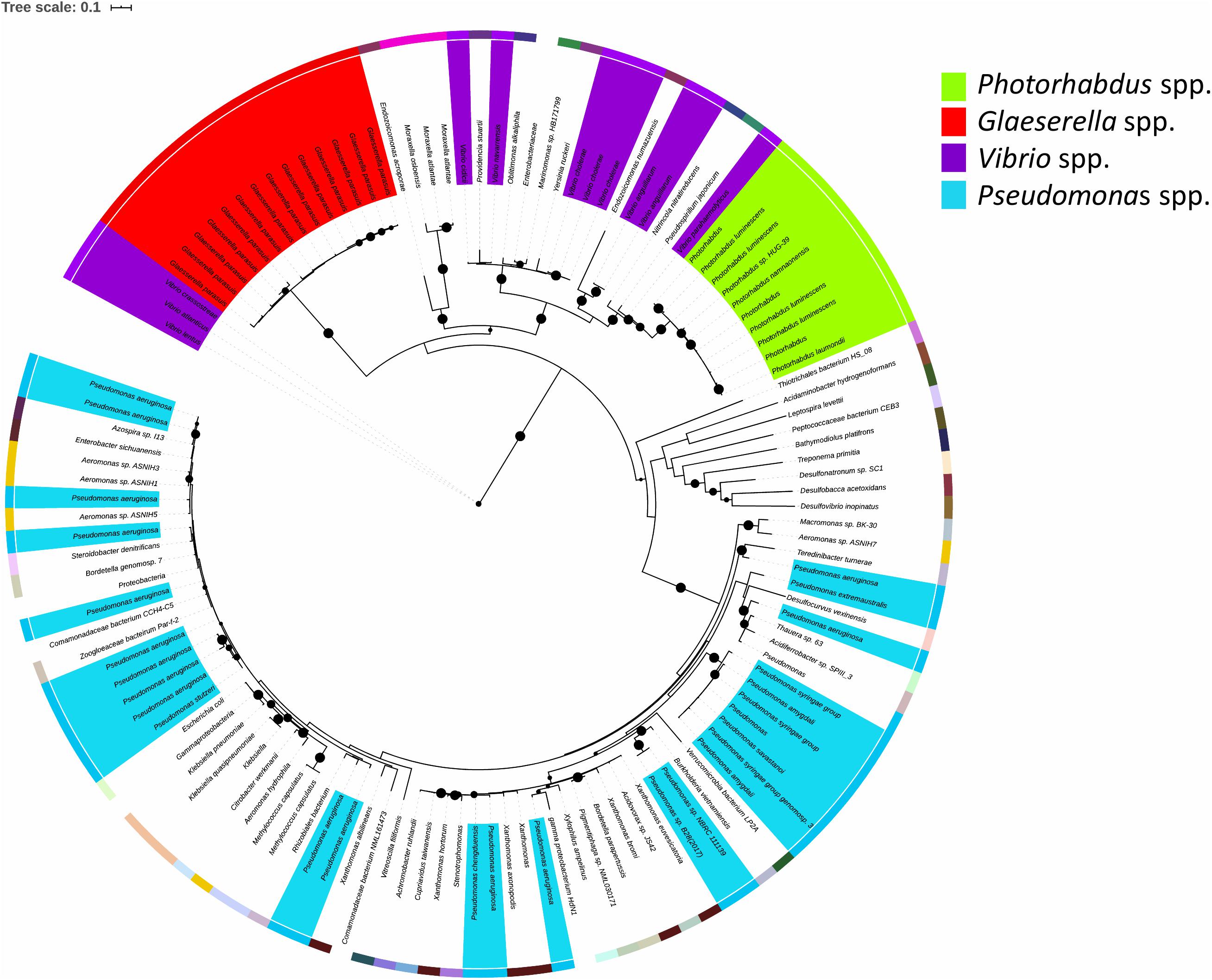
Figure 7. Maximum likelihood tree constructed using protein sequences with over 60% sequence identity to the Photorhabdus laumondii TrlG protein (with a query cover >90%). Nodes with support values higher than 80% are indicated with black circles. The external colored ring represents different genera. The scale bar represents the branch length, indicating the number of substitutions per amino acid position. Protein identifiers for all the entries can be found in Supplementary Table 1.
Analysis of conservation of the entire operon using MultiGeneBlast (Medema et al., 2013) against a GenBank database consisting of complete bacterial genomes showed that outside of the Photorhabdus genus, all three genes, pluDJC_RS01875 – RS01885 are only conserved syntenically in strains of V. anguillarum and on plasmid pYR3 of Yersinia ruckeri strain CSF007-82 (locus tags CSF007_p0360, CSF007_p0365, CSF007_p0345, Figure 8). However, unlike the TRL of P. laumondii where the three genes are located in succession, in pYR3 there are two small genes annotated as encoding Type I restriction-modification system, DNA-methyltransferase subunit M in between CSF007_p0345, the gene encoding the putative transcriptional regulator, and CSF007_p0360.
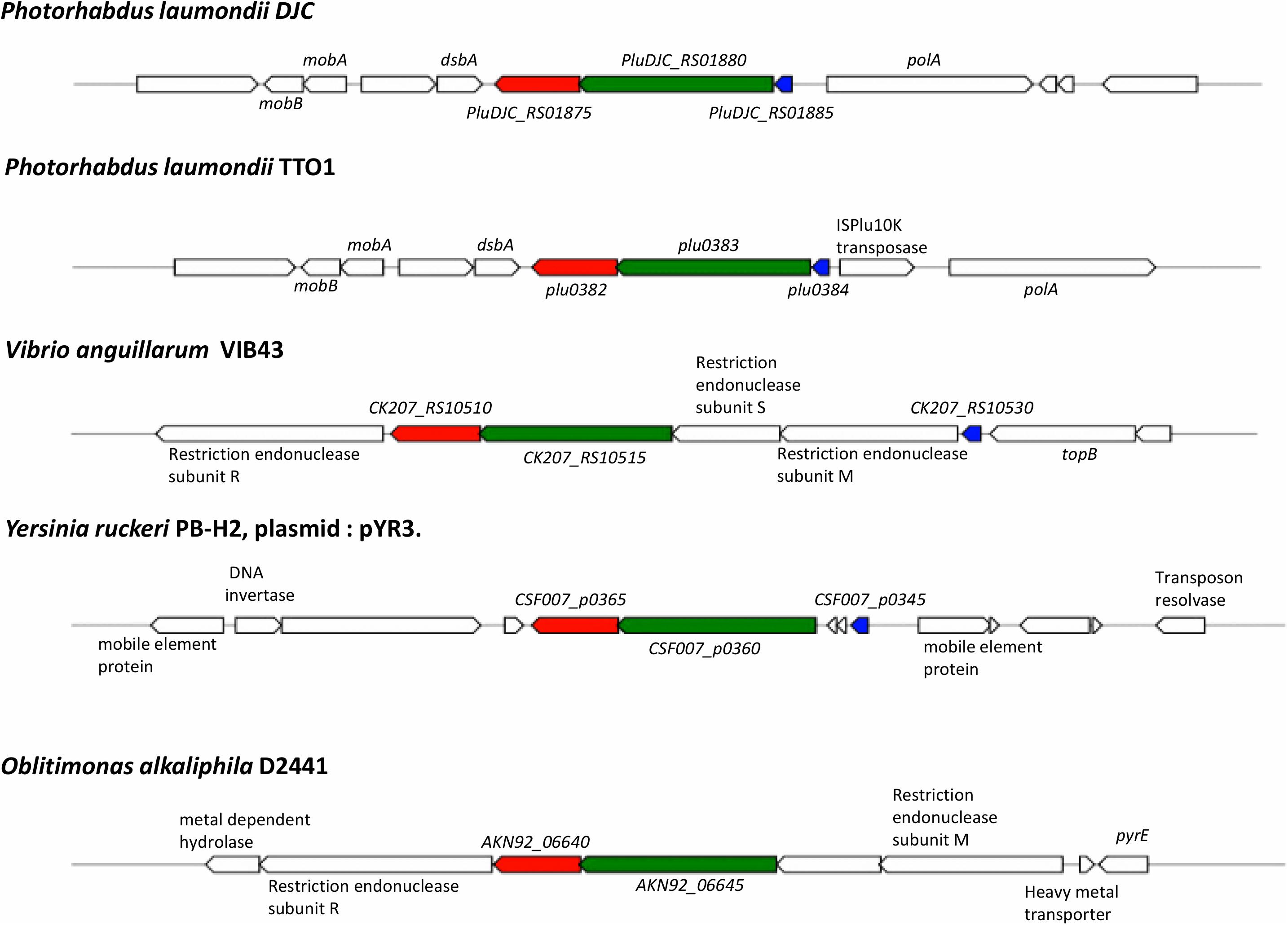
Figure 8. Conservation and genetic organization of the TRL operon in representative species as detected by MultiGeneBlast. In red is trlG, in green is trlF and in blue is trlR; genes colored identically in other organisms denote the identified homologs of trlG, trlF, and trlR, respectively.
Discussion
Members of the genus Photorhabdus dedicate a significant proportion of their genome to the synthesis of virulence factors and resource defense. Indeed, when the first Photorhabdus genome sequence was completed it was noted that there were more predicted toxin genes than in any other bacteria that had been sequenced at the time (Duchaud et al., 2003). In addition, many other genera belonging to the γ-proteobacteria contain multiple examples of mammalian pathogens, both obligate and opportunistic. With these observations in mind it is perhaps unusual that only very few members of the genus Photorhabdus are able to cause infection in humans (Gerrard and Stevens, 2017). Our previous analysis of the adaptations relevant to human pathogenicity in a representative P. asymbiotica strain suggested that no specific mammalian active toxins are necessary to facilitate human infection. Rather it seems that substantive changes in metabolic activity, when cultured at 37°C, are primarily responsible for their expanded host range (Mulley et al., 2015); a phenomenon termed nutritional virulence (Abu Kwaik and Bumann, 2013).
An obvious limitation for mammalian infection in many members of the genus is their inability to grow above ∼35°C, which again is unusual for members of the γ-proteobacteria. Typically, maximum growth temperatures of bacteria are determined by multiple factors that affect bacterial physiology, such as membrane and lipid fluidity, chaperone activities and other enzyme/protein functions. It is therefore possible that for any one species there are multiple functions that fail simultaneously at the non-permissive temperature. However, the ability of P. asymbiotica isolates to grow readily at temperatures of 37°C (and above) and the overall high level of conservation in core genome sequence across the genus indicates that there is likely no general physiological reason for temperature restriction in members of the genus.
Our identification of the TRL genes offers up an alternative explanation to this temperature restriction phenomenon, at least in certain lineages of P. laumondii. The specific induction of the TRL gene transcription upon temperature up-shift, leading to growth arrest, appears prima facia to represent a specific adaptive mechanism. We do not yet know if induction of TRL transcription at higher temperatures is mediated by the TrlR protein alone or if other regulatory pathways are involved. While it remains unclear as to what advantage this temperature dependent growth arrest imparts upon these strains, we may speculate. For example, we note that the nematode symbiont cannot survive above ∼34°C in vitro, although to our knowledge nematode survival at different temperatures in vivo has not been investigated. Therefore, a TRL mediated mechanism to suppress continued exponential growth at increasing temperatures, may represent a mechanism to synchronize life cycles of the bacteria and nematode in fluctuating environmental conditions. It is perhaps no coincidence that the TRL operon is encoded next to the polA gene and that the most 5′ TRL promoter overlaps on the opposing strand with that of the polA gene. Thus, activation of TRL transcription might directly suppress polA transcription by steric hindrance. It is formally possible that the tight linkage between polA and TRL reflects a functional linkage in the gene activities (i.e., replication and temperature). It is interesting in this respect that even at 28°C, we see a gradual increase in TRL transcription as the culture moves toward stationary phase and the eventual cessation of replication.
Interestingly, while ablation of the whole TRL operon did not affect virulence in the Galleria infection model at 28°C when the host temperature was increased to 36°C, the infection failed. This was true for both the S1 SNP and Δtrl strains, despite their ability to grow in vitro. This is consistent with the reported failure of P. asymbiotica to establish a lethal infection in the Manduca sexta model when incubated at 37°C (Mulley et al., 2015). In a natural context 37°C is not a problem for P. asymbiotica ATCC43949 as it would be in a mammalian host, for which it is adapted. Thus, despite the alleviation of growth restriction, there are clearly other temperature dependent changes in gene regulation occurring, as evidenced by the down regulation of luciferase activity and pigment production in these mutants. This effect may be common to both P. asymbiotica and the mutant P. laumondii lineages and regulated through a separate unknown mechanism. In addition, the loss of pigmentation and reduction in bioluminescence of the mutant colonies at 36°C is reminiscent of the secondary phenotypic variants of Photorhabdus (Boemare and Akhurst, 1988) as well as Δmdh and ΔfumC mutants deficient in enzymes of the TCA cycle (Lango and Clarke, 2010) and a ΔrelAspoT strain, deficient in (p)pGpp synthesis (Bager et al., 2016). The aforementioned mutants retain virulence in insect models but are no longer symbiotically competent. On the other hand, absence of pigmentation and bioluminescence is also a characteristic of the M-phase variants of P. luminescens, which are responsible for transmission of the bacteria to infective juvenile nematodes and establishment of symbiosis and these variants are avirulent against insects (Somvanshi et al., 2012).
The majority of random mutations selected from the population were non-synonymous amino acid substitutions in TrlG rather than premature stop codons or frame shifts. It is possible that this simply reflects the lower probability of obtaining a stop codon by random chance as complete deletion of the operon suggested the presence of an altered version of TrlG was not in some way advantageous. More importantly, no mutations were seen in the much larger and tightly linked trlF gene. This suggests that either (i) TrlF does not play a role in the temperature restriction phenotype, or (ii) that if it does, its function can be fully compensated by dominant activity of an intact TrlG protein. The small size of the trlR regulator gene means there was a much lower probability of isolating mutants in this. As we could delete the whole operon, it indicates that mutations in trlF alone are not overtly deleterious if they should occur in isolation. Interestingly, it is possible that the alternative mutation in the fur gene found in isolate S10 may also affect the expression of the trlG gene if there is indeed an interaction with the fur box of the predicted internal promoter in trlR.
As the lifestyles of most Photorhabdus strains are generally similar, it is interesting that the TRL operon appears restricted yet ancestral to a specific species lineage of Photorhabdus. As members of this linage are geographically dispersed (Machado et al., 2018; Mathur et al., 2018), factors such as biogeography, insect prey type or the nature of competing organisms are unlikely to be the reason for maintaining TRL. We therefore speculate that selection for TRL may be a result of specific requirements of the host bacterial genomes, or perhaps in restrictions imposed by the nematode host lineage. For example; as the P. luminescens TT01 genome is heavily infested by IS-elements, we could hypothesize that TRL represents a mechanism to protect against rampant IS-element activity. If a sustained temperature increase was “perceived” as stress to the bacteria (or more importantly the IS-elements), it may lead to undesirable activation of a large number of IS-elements. Therefore, stopping genome replication would likely protect against extensive transposition damage.
An alternative idea is that these strains rely on the activities of a pathway or global regulator which is highly sensitive to temperature. For example, it has been shown that secondary metabolite production is reliant upon the activity of the Hfq protein (Tobias et al., 2017) which, in other Gram-negative bacteria studied, mediates interactions of regulatory ncRNAs with their target mRNA molecules (Zhang et al., 1998; Møller et al., 2002; Lease and Woodson, 2004). Folding and interactions of many ncRNAs are often temperature sensitive. The lack of pigment and bioluminescence in the mutant strains at the higher temperature suggests that this regulation is indeed being perturbed. If all secondary metabolism necessary for virulence and symbiosis ceases at the higher temperature it would make sense for the bacterium to inhibit further replication until normal regulation is re-established.
Finally, the identification of TRL-like operons in members of other genera suggests this represents a widespread general mechanism, regulating the life-cycles of phylogenetically diverse bacteria. However, while some of these organisms are found in marine environments and may have lower temperature optima, we do not know whether strains carrying the TRL are restricted in growth compared to other strains of the same species that do not carry the locus. An investigation of the growth temperature ranges of the different isolates would be required to establish if presence of the gene has any consequence in these organisms.
The two other organisms where the entire TRL gene cluster was found are both fish pathogens. Y. ruckeri is the causative agent of enteric red mouth disease in salmonid fish species (Kumar et al., 2015) and its optimum growth temperature is also 28°C, while its optimum temperature for infectivity is 18°C. Similarly, V. anguillarum has an optimum growth temperature of 25°C whilst the optimum temperature for vibriosis disease is 15°C (Lages et al., 2019). It appears that a lower temperature optimum for virulence is common in fish pathogens and virulence genes are found to be up-regulated at these lower temperatures (Guijarro et al., 2015; Mendez et al., 2018; Lages et al., 2019). The association of TRL operons with pathogens of poikilothermic hosts (e.g., fish) tempts speculation there is an as yet unknown advantage to this mechanism in such niches.
Materials and Methods
Strains and Growth Conditions
The strains used in this study were the rifampicin sensitive parent strain of P. laumondii subsp. laumondii DJC (WT) (Zamora-Lagos et al., 2018), and a rifampicin resistant derivative of this strain, P. luminescens subsp. laumondii DJC (RifR) (Jones et al., 2010). Bacteria were grown in LB at 28°C unless otherwise stated. For growth on solid media, LB was supplemented with 1.5% agar and 0.1% sodium pyruvate and plates were incubated in the dark. When necessary, kanamycin was added to the media at a concentration of 25 μg/ml. To assess growth on solid media, overnight cultures of Photorhabdus were diluted to an OD600 of 0.05 and 5 μl were spotted on solid media. They were then incubated at the appropriate temperature for 48 h. To assess growth in liquid, the diluted cultures were incubated at the appropriate temperature for 24 h, shaking at 180 rpm. For continuous monitoring of growth in liquid, diluted cultures were aliquoted in flat bottomed 96-well plates and placed in a BMG Labtech OMEGA microplate reader at the appropriate temperature, with double orbital shaking at 300 rpm. Measurements of absorbance at 600 nm were taken in 30 min intervals.
Isolation of the Temperature Tolerant Clones
Saturated overnight cultures of P. laumondii DJC grown at 28°C in LB medium were plated on LBA and incubated at 36°C. After approximately 48 h colonies were observed on the plates. These were re-streaked four times on LBA at 36°C and stably grown clones were stored at −80°C in 32% v/v glycerol.
Whole-Genome Sequencing and Bioinformatics Analysis
DNA was isolated from 1 ml of overnight cultures of P. laumondii DJC using the Qiagen Blood and Tissue kit. The Nextera XT kit (Illumina) was used for library preparation and sequencing was performed using a MiSeq v2 cartridge on a MiSeq sequencer (Illumina). Raw data has been deposited in the NCBI database under BioProject ID PRJNA596169, while raw sequencing reads for the WT isolate in our lab can be found in the Short Read Archive using accession number SRR8307122. Fastq reads of both the WT and the tolerant clones were mapped against the NCBI reference sequence for P. laumondii subsp. laumondii DJC NZ_CP024900.1 (Langmead and Salzberg, 2012) in very sensitive mode. SAMtools v0.1.18 (Li et al., 2009) was used to obtain sorted bam files, containing aligned reads, and subsequently pile up format files. VarScan v2.3.6 (Koboldt et al., 2012) was then used to identify SNPs (parameters: p-value 0.1, min-coverage 20 –min-reads2 15 –min-avg-qual 30 –min-var-freq 0.9). A different set of parameters was subsequently used to account for the clones with lower coverage (with modified min-coverage of 10 and –min-reads2 of 6). For clones where no SNP was identified in the trlG gene automatically, manual inspection of the alignment files followed by PCR and Sanger sequencing were used to check for the presence of any insertions/deletions. In particular, to sequence the trlG gene in the JC and JD strains as well as the tolerant clones S15 and S29, either the gene was amplified using primer pair 00323SecF/00323SecR or the entire operon was amplified using recOSeqF/reOSeqR and the resulting PCR products were purified and sent for Sanger sequencing. All primer sequences used in this study are listed in Table 2.
A prediction of the protein structure of TrlG was performed using I-TASSER (Zhang, 2008) with default parameters. The location of the amino acid substitutions on the protein was visualized using UCSF Chimera (Pettersen et al., 2004).
To investigate the presence of the gene in other bacteria, trlG was translated using the standard codon usage and the amino acid sequence was used as input for BLASTP v2.8.1 against the NCBI protein reference sequences using default search parameters. Hits were retained if the showed at least 60% sequence identity over 90% of the query length. Multiple alignments were created using Clustal Omega (Sievers et al., 2011). The Clustal alignment file was then used to infer a maximum likelihood phylogeny using IQ-TREE v 1.6.3 (Nguyen et al., 2015) with the JTT + I + G4 substitution model selected by ModelFinder (Kalyaanamoorthy et al., 2017), and ultrafast bootstrap (Hoang et al., 2018). To reconstruct the Photorhabdus recA phylogeny, recA nucleotide sequences were obtained from the Photorhabdus genomes present in the NCBI reference genomic sequence database and analyzed using the Phylogeny. fr platform. The sequences were aligned using MUSCLE and ambiguity was removed using Gblocks. A maximum likelihood tree was then constructed using PhyML and 500 bootstrap iterations were performed. Phylogenetic trees were visualized using the Interactive Tree Of Life (iTOL) (Letunic and Bork, 2019). For MultiGeneBlast analysis (Medema et al., 2013), a database was created from the bacterial GenBank division and a homology search was performed with default parameters, with the three TRL genes as input.
Construction of a Δtrl Mutant
A markerless Δtrl deletion mutant was created by double homologs recombination using the suicide plasmid pDS132 (Philippe et al., 2004). Regions upstream and downstream of the TRL were amplified using primer pairs DrecOUF/DrecOUR and DrecODF/DrecODR, respectively. The purified PCR products were mixed and used as a template in a second joining PCR with primers DrecOUF/DrecODR. The resulting product was digested using enzymes SacI and PstI and ligated into digested and dephosphorylated pDS132. The resulting plasmid was introduced by transformation into the donor Escherichia coli strain S17.1 lamda-pir. For conjugations into Photorhabdus, the rifampicin resistant P. luminescens DJC strain was used as a recipient. Briefly, exponentially growing cultures of P. laumondii DJC RifR in LB containing 1 mM MgCl2 and E. coli S17.1 lamda-pir harboring pDS132 (Δtrl) were harvested and combined at a ratio 4:1 of acceptor to donor bacteria. Matings were spotted on LBA plates containing 1 mM MgCl2 and incubated overnight at 28°C. Bacteria were then harvested and aliquots were plated on LBA supplemented with 15 μg/ml chloramphenicol and 50 μg/ml rifampicin. Possible transconjugants and hence first recombinants were confirmed by PCR using primer pairs TestRecO1/00323SeqR and polA3F′/TestRecG4. Successful first recombinants were grown in LB overnight and then the culture was diluted in fresh LB and grown to mid exponential. At this point serial dilutions were made and aliquots were plated on LBA supplemented with 0.2% sucrose and incubated at 28°C. The resulting colonies were tested for deletion of the TRL operon by PCR using primers TestRecO1/TestRecG4.
Complementation
For complementation, the entire TRL was amplified using primers recOFEcoRI/recORSpeI and the resulting PCR product was digested with EcoRI and SpeI and ligated into a modified version of vector pBCSK (that had been previously digested with AseI and KpnI to remove Plac). The resulting plasmid, termed pBCSK′ (TRL), and the empty pBCSK′ were introduced separately into the Δtrl deletion mutant or into SNP mutants by transformation. To prepare cells for transformation, bacteria growing exponentially in 100 ml LB were harvested at an OD600 of 0.2–0.3 and kept for 90 min on ice. They were then collected by centrifugation and washed twice with ice-cold SH buffer at pH 7 (10 mM HEPES containing 5% w/v sucrose). The cells were resuspended in 160 μl SH buffer and 50 μl aliquots were used for electroporation with the following conditions: 2.5 kV, 25 μF and 200 Ω in 2 mm cuvettes. This was followed by addition of 1 ml LB and incubation at 28°C, 180 rpm for 3 h. Aliquots were plated on LB agar supplemented with 25 μg/ml chloramphenicol. Colonies were tested for the presence of the plasmid by PCR using primers M13R/Seq03223F.
Infection Assays
The infections were performed using 5th instar larvae of G. mellonella. The bacteria were grown in LB at 28°C and then collected and resuspended in PBS to an OD600 of 1.5. The suspension was then serially diluted to obtain a final suspension with an infection dose of ∼50 colony forming units per 10 μl. The Galleria larvae were infected by injection through the first proleg and incubated either at room temperature or 36°C. For the experiment conducted at room temperature two groups of 10 larvae were infected per strain, while for the experiment at 36°C, 8 larvae were infected per strain. The experiment was conducted twice.
Construction of GFP Promoter Fusions
The TRL promoter regions were amplified using Q5 DNA polymerase and with P. laumondii DJC DNA as a template and using the following primer pairs: Prec2F/PrecR for promoter region PA, Prec2F/Prev00325 for promoter region PB, Prec1f/Prev00325 for promoter region PC and Prec3F/Prev00325 for promoter region PD. PCR products were digested using BamHI and SalI and purified. They were then ligated into the broad range vector pGA-G1, which carries a promoterless gfp-mut3 (Leo Eberl, University of Zurich). The ligations were introduced by transformation into E. coli DH5a lamda-pir. Successful transformants were confirmed by PCR and plasmids were isolated using the Qiagen miniprep kit and introduced into E. coli strain S17.1 lamda-pir to be used as a donor in conjugation, which was performed as described above.
Growth and Fluorescence Measurements of Reporter Strains
Photorhabdus laumondii DJC strains harboring the reporter constructs were grown overnight in LB supplemented with kanamycin at 28°C and the cultures were then diluted to fresh media to an OD600 of ∼0.05. The diluted cultures were aliquoted in black flat-bottomed μClear 96-well plates (CELLSTAR, Greiner) and placed in an Omega Fluostar (BMG Labtech) microplate reader. They were incubated at 28°C with double orbital shaking at 300 rpm. Readings of absorbance at 600 nm as well as GFP fluorescence (gain 1000) were taken every 30 min. For the temperature shift experiment, after 5 h of growth at 28°C the temperature of the microplate reader was increased to 36°C (this took place in ∼ 2 min). Then readings were taken immediately following the shift and at 30 min intervals after that.
Data Availability Statement
The raw sequencing data generated and analyzed in this study has been deposited in the NCBI database under BioProject ID PRJNA596169, while raw sequencing reads for the WT isolate in our lab can be found in the Short-Read Archive (SRA) using accession number SRR8307122. The reference genome sequence for P. luminescens subsp. laumondii DJC is available on NCBI RefSeq with the accession number NZ_CP024900.1.
Author Contributions
AH and NW designed the study and wrote the initial manuscript draft. AH, JH, and GM performed the mutant isolation experiments. JH performed the structural prediction studies. AH performed the remaining experiments and analyzed the data. GM reviewed the manuscript. All authors have approved the final manuscript.
Funding
This work was funded by the Warwick Medical School, University of Warwick, the Leverhulme Trust grant RPG-2015-194 and the EPSRC MOAC Centre for Doctoral Training (DTPEP/F500378/1).
Conflict of Interest
The authors declare that the research was conducted in the absence of any commercial or financial relationships that could be construed as a potential conflict of interest.
Acknowledgments
We would like to thank Prof. Mark Pallen for the use of his MiSeq machine. The previous version of the manuscript, prior to any corrections based on reviewers’ comments, has been made available through the preprint server bioaRxiv (Hapeshi et al., 2020).
Supplementary Material
The Supplementary Material for this article can be found online at: https://www.frontiersin.org/articles/10.3389/fmicb.2020.548800/full#supplementary-material
References
Abu Kwaik, Y., and Bumann, D. (2013). Microbial quest for food in vivo: “Nutritional virulence” as an emerging paradigm. Cell. Microbiol. 15, 882–890. doi: 10.1111/cmi.12138
Bager, R., Roghanian, M., Gerdes, K., and Clarke, D. J. (2016). Alarmone (p)ppGpp regulates the transition from pathogenicity to mutualism in Photorhabdus luminescens. Mol. Microbiol. 100, 735–747. doi: 10.1111/mmi.13345
Boemare, N., and Akhurst, R. (1988). Biochemical and physiological characterization of colony form variants in Xenorhabdus spp. (Enterobacteriaceae). Microbiology 134, 751–761. doi: 10.1099/00221287-134-3-751
Ciche, T. A., and Ensign, J. C. (2003). For the insect pathogen Photorhabdus luminescens, which end of a nematode is out? Appl. Environ. Microbiol. 69, 1890–1897. doi: 10.1128/AEM.69.4.1890-1897.2003
Duchaud, E., Rusniok, C., Frangeul, L., Buchrieser, C., Givaudan, A., Taourit, S., et al. (2003). The genome sequence of the entomopathogenic bacterium Photorhabdus luminescens. Nat. Biotechnol. 21, 1307–1313. doi: 10.1038/nbt886
Dutta, A., Flores, A. R., Revell, P. A., and Owens, L. (2018). Neonatal bacteremia and cutaneous lesions caused by Photorhabdus luminescens: a rare gram-negative bioluminescent bacterium. J. Pediatric Infect. Dis. Soc. 7, e182–e184. doi: 10.1093/jpids/piy064
Fischer-Le Saux, M., Viallard, V., Brunel, B., Normand, P., and Boemare, N. E. (1999). Polyphasic classification of the genus Photorhabdus and proposal of new taxa: P. luminescens subsp. luminescens subsp. nov., P. luminescens subsp. akhurstii subsp. nov., P. luminescens subsp. laumondii subsp. nov., P. temperata sp. nov., P. temperata subs. temperata subsp. nov and P. asymbiotica sp. nov. Int. J. Syst. Bacteriol. 49, 1645–1656. doi: 10.1099/00207713-49-4-1645
Gerrard, J. G., and Stevens, R. P. (2017). “A review of clinical cases of infection with Photorhabdus Asymbiotica,” in The Molecular Biology of Photorhabdus Bacteria, ed. R. H. ffrench-Constant (Cham: Springer International Publishing), 179–191. doi: 10.1007/82_2016_56
Guijarro, J. A., Cascales, D., García-Torrico, A. I., García-Domínguez, M., and Méndez, J. (2015). Temperature-dependent expression of virulence genes in fish-pathogenic bacteria. Front. Microbiol. 6:700. doi: 10.3389/fmicb.2015.00700
Hapeshi, A., Healey, J. R. J., Mulley, G., and Nicholas, R. (2020). Temperature restriction in entomopathogenic bacteria. bioRxiv [Preprint]. doi: 10.1101/2020.06.02.129163
Hoang, D. T., Chernomor, O., von Haeseler, A., Minh, B. Q., and Vinh, L. S. (2018). UFBoot2: improving the ultrafast bootstrap approximation. Mol. Biol. Evol. 35, 518–522. doi: 10.1093/molbev/msx281
Ishioka, K., Iwasaki, H., and Shinagawa, H. (1997). Roles of the recG gene product of Escherichia coli in recombination repair: effects of the ΔrecG mutation on cell division and chromosome partition. Genes Genet. Syst. 72, 91–99. doi: 10.1266/ggs.72.91
Jones, R. T., Sanchez-Contreras, M., Vlisidou, I., Amos, M. R., Yang, G., Muñoz-Berbel, X., et al. (2010). Photorhabdus adhesion modification protein (Pam) binds extracellular polysaccharide and alters bacterial attachment. BMC Microbiol. 10:141. doi: 10.1186/1471-2180-10-141
Kalyaanamoorthy, S., Minh, B. Q., Wong, T. K. F., von Haeseler, A., and Jermiin, L. S. (2017). ModelFinder: fast model selection for accurate phylogenetic estimates. Nat. Methods 14, 587–589. doi: 10.1038/nmeth.4285
Kazimierczak, W., Skrzypek, H., Sajnaga, E., Skowronek, M., Waśko, A., and Kreft, A. (2017). Strains of Photorhabdus spp. associated with polish Heterorhabditis isolates: their molecular and phenotypic characterization and symbiont exchange. Arch. Microbiol. 199, 979–989. doi: 10.1007/s00203-017-1368-z
Koboldt, D. C., Zhang, Q., Larson, D. E., Shen, D., Mclellan, M. D., Lin, L., et al. (2012). VarScan 2: somatic mutation and copy number alteration discovery in cancer by exome sequencing. Genome Res. 22, 568–576. doi: 10.1101/gr.129684.111.568
Kumar, G., Menanteau-Ledouble, S., Saleh, M., and El-Matbouli, M. (2015). Yersinia ruckeri, the causative agent of enteric redmouth disease in fish. Vet. Res. 46, 1–10. doi: 10.1186/s13567-015-0238-4
Lages, M. A., Balado, M., and Lemos, M. L. (2019). The expression of virulence factors in Vibrio anguillarum is dually regulated by iron levels and temperature. Front. Microbiol. 10:2335. doi: 10.3389/fmicb.2019.02335
Langmead, B., and Salzberg, S. L. (2012). Fast gapped-read alignment with Bowtie 2. Nat. Methods 9, 357–359. doi: 10.1038/nmeth.1923
Lango, L., and Clarke, D. J. (2010). A metabolic switch is involved in lifestyle decisions in Photorhabdus luminescens. Mol. Microbiol. 77, 1394–1405. doi: 10.1111/j.1365-2958.2010.07300.x
Lease, R. A., and Woodson, S. A. (2004). Cycling of the Sm-like protein Hfq on the DsrA small regulatory RNA. J. Mol. Biol. 344, 1211–1223. doi: 10.1016/j.jmb.2004.10.006
Letunic, I., and Bork, P. (2019). Interactive tree of life (iTOL) v4: recent updates and new developments. Nucleic Acids Res. 47, W256–W259. doi: 10.1093/nar/gkz239
Li, H., Handsaker, B., Wysoker, A., Fennell, T., Ruan, J., Homer, N., et al. (2009). The sequence Alignment/Map format and SAMtools. Bioinformatics 25, 2078–2079. doi: 10.1093/bioinformatics/btp352
Machado, R. A. R., Wüthrich, D., Kuhnert, P., Arce, C. C. M., Thönen, L., Ruiz, C., et al. (2018). Whole-genome-based revisit of Photorhabdus phylogeny: proposal for the elevation of most Photorhabdus subspecies to the species level and description of one novel species Photorhabdus bodei sp. nov., and one novel subspecies Photorhabdus laumondii subsp. Int. J. Syst. Evol. Microbiol. 68, 2664–2681. doi: 10.1099/ijsem.0.002820
Maher, A. M. D., Asaiyah, M. A. M., Brophy, C., and Griffin, C. T. (2017). An entomopathogenic nematode extends its niche by associating with different symbionts. Microb. Ecol. 73, 211–223. doi: 10.1007/s00248-016-0829-2
Maneesakorn, P., An, R., Daneshvar, H., Taylor, K., Bai, X., Adams, B. J., et al. (2011). Phylogenetic and cophylogenetic relationships of entomopathogenic nematodes (Heterorhabditis: Rhabditida) and their symbiotic bacteria (Photorhabdus: Enterobacteriaceae). Mol. Phylogenet. Evol. 59, 271–280. doi: 10.1016/j.ympev.2011.02.012
Mathur, C., Kushwah, J., Somvanshi, V. S., and Dutta, T. K. (2018). A 37 kDa Txp40 protein characterized from Photorhabdus luminescens sub sp. akhurstii conferred injectable and oral toxicity to greater wax moth, Galleria mellonella. Toxicon 154, 69–73. doi: 10.1016/j.toxicon.2018.09.007
Medema, M. H., Takano, E., and Breitling, R. (2013). Detecting sequence homology at the gene cluster level with multigeneblast. Mol. Biol. Evol. 30, 1218–1223. doi: 10.1093/molbev/mst025
Mendez, J., Cascales, D., Garcia-Torrico, A. I., and Guijarro, J. A. (2018). Temperature-dependent gene expression in Yersinia ruckeri: tracking specific genes by bioluminescence during in vivo colonization. Front. Microbiol. 9:1098. doi: 10.3389/fmicb.2018.01098
Møller, T., Franch, T., Højrup, P., Keene, D. R., Bächinger, H. P., Brennan, R. G., et al. (2002). Hfq: a bacterial Sm-like protein that mediates RNA-RNA interaction. Mol. Cell 9, 23–30. doi: 10.1016/s1097-2765(01)00436-1
Mulley, G., Beeton, M. L., Wilkinson, P., Vlisidou, I., Ockendon-Powell, N., Hapeshi, A., et al. (2015). From insect to man: Photorhabdus sheds light on the emergence of human pathogenicity. PLoS One 10:e0144937. doi: 10.1371/journal.pone.0144937
Nguyen, L. T., Schmidt, H. A., Von Haeseler, A., and Minh, B. Q. (2015). IQ-TREE: a fast and effective stochastic algorithm for estimating maximum-likelihood phylogenies. Mol. Biol. Evol. 32, 268–274. doi: 10.1093/molbev/msu300
Pettersen, E. F., Goddard, T. D., Huang, C. C., Couch, G. S., Greenblatt, D. M., Meng, E. C., et al. (2004). UCSF Chimera – a visualization system for exploratory research and analysis. J. Comput. Chem. 25, 1605–1612. doi: 10.1002/jcc.20084
Philippe, N., Alcaraz, J. P., Coursange, E., Geiselmann, J., and Schneider, D. (2004). Improvement of pCVD442, a suicide plasmid for gene allele exchange in bacteria. Plasmid 51, 246–255. doi: 10.1016/j.plasmid.2004.02.003
Rudolph, C. J., Mahdi, A. A., Upton, A. L., and Lloyd, R. G. (2010). RecG protein and single-strand DNA exonucleases avoid cell lethality associated with PriA helicase activity in Escherichia coli. Genetics 186, 473–492. doi: 10.1534/genetics.110.120691
Sievers, F., Wilm, A., Dineen, D., Gibson, T. J., Karplus, K., Li, W., et al. (2011). Fast, scalable generation of high-quality protein multiple sequence alignments using Clustal Omega. Mol. Syst. Biol. 7:539. doi: 10.1038/msb.2011.75
Solovyev, V., and Salamov, A. (2011). “Automatic annotation of microbial genomes and metagenomic sequences,” in Metagenomics and its Applications in Agriculture, Biomedicine and Environmental Studies, ed. R. W. Li (Hauppauge, NY: Nova Science Publishers).
Somvanshi, V. S., Sloup, R. E., Crawford, J. M., Martin, A. R., Heidt, A. J., Kim, K.-S., et al. (2012). A single promoter inversion switches photorhabdus between pathogenic and mutualistic states. Science 337, 88–93. doi: 10.1126/science.1216641
Tailliez, P., Laroui, C., Ginibre, N., Paule, A., Pages, S., and Boemare, N. (2010). Phylogeny of Photorhabdus and Xenorhabdus based on universally conserved protein-coding sequences and implications for the taxonomy of these two genera. Proposal of new taxa: X. vietnamensis sp. nov., P. luminescens subsp. caribbeanensis subsp. nov., P. l. Int. J. Syst. Evol. Microbiol. 60, 1921–1937. doi: 10.1099/ijs.0.014308-0
Tobias, N. J., Heinrich, A. K., Eresmann, H., Wright, P. R., Neubacher, N., Backofen, R., et al. (2017). Photorhabdus-nematode symbiosis is dependent on hfq-mediated regulation of secondary metabolites. Environ. Microbiol. 19, 119–129. doi: 10.1111/1462-2920.13502
Vernikos, G. S., and Parkhill, J. (2006). Interpolated variable order motifs for identification of horizontally acquired DNA: revisiting the Salmonella pathogenicity islands. Bioinformatics 22, 2196–2203. doi: 10.1093/bioinformatics/btl369
Waterfield, N. R., Ciche, T., and Clarke, D. (2009). Photorhabdus and a host of hosts. Annu. Rev. Microbiol. 63, 557–574. doi: 10.1146/annurev.micro.091208.073507
Zamora-Lagos, M.-A., Eckstein, S., Langer, A., Gazanis, A., Pfeiffer, F., Habermann, B., et al. (2018). Phenotypic and genomic comparison of Photorhabdus luminescens subsp. laumondii TT01 and a widely used rifampicin-resistant Photorhabdus luminescens laboratory strain. BMC Genomics 19:854. doi: 10.1186/s12864-018-5121-z
Zhang, A., Altuvia, S., Tiwari, A., Argaman, L., Hengge-Aronis, R., and Storz, G. (1998). The OxyS regulatory RNA represses rpoS translation and binds the Hfq (HF-I) protein. EMBO J. 17, 6061–6068. doi: 10.1093/emboj/17.20.6061
Keywords: Photorhabdus, temperature restriction, evolution, pathogenicity, mutants
Citation: Hapeshi A, Healey JRJ, Mulley G and Waterfield NR (2020) Temperature Restriction in Entomopathogenic Bacteria. Front. Microbiol. 11:548800. doi: 10.3389/fmicb.2020.548800
Received: 03 April 2020; Accepted: 08 September 2020;
Published: 30 September 2020.
Edited by:
Rekha Seshadri, Lawrence Berkeley National Laboratory, United StatesReviewed by:
Julien Brillard, Institut National de la Recherche Agronomique (INRA), FranceHelge B. Bode, Goethe University Frankfurt, Germany
Copyright © 2020 Hapeshi, Healey, Mulley and Waterfield. This is an open-access article distributed under the terms of the Creative Commons Attribution License (CC BY). The use, distribution or reproduction in other forums is permitted, provided the original author(s) and the copyright owner(s) are credited and that the original publication in this journal is cited, in accordance with accepted academic practice. No use, distribution or reproduction is permitted which does not comply with these terms.
*Correspondence: Nicholas R. Waterfield, n.r.waterfield@warwick.ac.uk
†Present address: Alexia Hapeshi, Chemistry Department, University of Warwick, Coventry, United Kingdom