- 1Marine Ecology and Systematics (MarES), Department of Biology, University of the Balearic Islands, Palma de Mallorca, Spain
- 2Instituto de Ciencias Naturales Alexander von Humboldt, Universidad de Antofagasta, Antofagasta, Chile
- 3Grup de Recerca en Microbiologia, Departament de Biologia, Universitat de les Illes Balears, Palma de Mallorca, Spain
- 4Celomic Unit of the University Institute of Research in Health Sciences of the Balearic Islands, Palma de Mallorca, Spain
- 5Servicio Científico-Técnicos, University of the Balearic Islands, Palma de Mallorca, Spain
Little is known about the direct effects of microplastics (MPs) and their organic additives on marine bacteria, considering their role in the nutrient cycles, e.g., N-cycles through the N2-fixation, or in the microbial food web. To fill this gap of knowledge, we exposed marine bacteria, specifically diazotrophs, to pure MPs which differ in physical properties (e.g., density, hydrophobicity, and/or size), namely, polyethylene, polypropylene, polyvinyl chloride and polystyrene, and to their most abundant associated organic additives (e.g., fluoranthene, 1,2,5,6,9,10-hexabromocyclododecane and dioctyl-phthalate). Growth, protein overproduction, direct physical interactions between MPs and bacteria, phosphorus acquisition mechanisms and/or N2-fixation rates were evaluated. Cyanobacteria were positively affected by environmental and high concentrations of MPs, as opposed to heterotrophic strains, that were only positively affected with high concentrations of ~120 μm-size MPs (detecting the overproduction of proteins related to plastic degradation and C-transport), and negatively affected by 1 μm-size PS beads. Generally, the organic additives had a deleterious effect in both autotrophic and heterotrophic bacteria and the magnitude of the effect is suggested to be dependent on bacterial size. Our results show species-specific responses of the autotrophic and heterotrophic bacteria tested and the responses (beneficial: the “good,” deleterious: the “bad” and/or both: the “double-sword”) were dependent on the type and concentration of MPs and additives. This suggests the need to determine the threshold levels of MPs and additives concentrations starting from which significant effects can be observed for key microbial populations in marine systems, and these data are necessary for effective environmental quality control management.
Introduction
Marine coastal ecosystems are the most impacted zones by the pollution of plastics. Up to 10 million tons of plastic enter annually in the oceans (Almroth and Eggert, 2019). This oceanic “soup” of plastic is composed of different particle sizes: macroplastics (>250 mm), mesoplastics (1–25 mm), microplastics (MPs) (1–1,000 μm), and nanoplastics (NPs) (<1 μm) (Hartmann et al., 2019). The most abundant polymers (at the macro- and micro-size scale) on the surface of the oceans and seas are polyethylene (PE), followed by polypropylene (PP) and then by others such as polyvinyl chloride (PVC) or polystyrene (PS) (Suaria et al., 2016). MPs have associated chemical additives (usually organic) that have been added to them to improve their chemical properties, and these low molecular weight additives can leach from the plastic polymers, being also sorbed onto them (Bakir et al., 2014). Therefore, MPs can also be sources and vectors for these organic pollutants, which are deleterious for marine organisms (Hahladakis et al., 2018).
The abundance of plastic particles declines exponentially with depth according to their densities, resulting in low-density polymers (e.g., PE and PP) predominating in the surface waters and higher density polymers (e.g., PS) in the deeper areas (Erni-Cassola et al., 2019). However, some evidence suggests that much of the small plastic particles at the surface, independently of their density, end up in sediments by transport mechanisms (Reisser et al., 2015; Urbanek et al., 2018). The accumulation of MPs at depth indicates the susceptibility of planktonic and benthic macro- and micro-organisms to the effects of these pollutants.
In eukaryotic microorganisms, the deleterious effects of plastics have already been described (Wang and Zheng, 2008; Cole and Galloway, 2015; Nelms et al., 2018). However, studies investigating the direct effects of plastics, especially MPs, on marine prokaryotic organisms (e.g., in their growth, biochemistry or nutrient acquisition mechanisms) are still scarce (Harrison et al., 2011; Bryant et al., 2016; Romera-Castillo et al., 2018; Tetu et al., 2019; Machado et al., 2020; Piccardo et al., 2020; Sarker et al., 2020; Seeley et al., 2020). None have investigated the direct effect of MPs, for example, on marine diazotrophs, which are capable of converting the nitrogen gas (N2) into ammonia (NH3) through the nitrogenase enzyme complex and playing an important role in the marine N cycle. The few studies that have been done on other microorganisms usually are focused on plastic degradation and biofilm formation (Urbanek et al., 2018). Nonetheless, Machado et al. (2020) and Seeley et al. (2020) suggest that MPs can be anthropogenic stressors affecting microbial diversity and N-cycles. Other studies have reported changes in the microbial communities associated with the floating plastics through metagenomic analysis (Yang et al., 2019), suggesting that the response to plastic pollution can be species-specific. Tetu et al. (2019), Sarker et al. (2020), and Piccardo et al. (2020) also revealed the importance of concentration levels of leached plastic in cyanobacteria and heterotrophic bacteria. Considering these previous results, experimental studies investigating the effect of MPs and their additives should take into account the response of different bacterial test species and different concentration levels of the pollutants. Moreover, the previous studies investigating the effect of plastic pollution use plastics with unknown chemical additives, and to separate the effects of plastics and additives, pure MPs and their known additives must be tested. Furthermore, varying physical properties of MPs have to be considered (e.g., density, hydrophobic, or size) since could affect the response of microorganisms.
Here, we report the responses of different bacterial species, specifically N2-fixing phototrophic and heterotrophic bacteria to different concentrations of pure MPs (i.e., PE, PP, PVC, and PS) and their most predominant organic chemical additives [fluoranthene, 1,2,5,6,9,10-hexabromocyclododecane (HBCD) and dioctyl-phthalate (DEHP)]. Our results show beneficial, detrimental or both effects, depending on the species tested and the type and concentrations of MPs and additives added.
Materials and Methods
Culture Strains Tested
Five marine N2-fixing species (two cyanobacteria and three heterotrophic bacteria), found in association with P. oceanica (Agawin et al., 2017; Fernández-Juárez et al., in prep), were selected according to the experimental design described in Supplementary Table 1. Before the experiments, the cells were acclimatized and cultured in their respective optimal culture media to achieve their exponential phase. Culture media were composed of synthetic seawater medium (ASN-III) + Turks island salts 4X for Halothece sp., BG110 for F. muscicola and marine broth (MB) for the rest of the heterotrophic bacteria (Rippka et al., 1979). The cells were acclimated at 25°C at 120 r.p.m in a rotatory shaker with a photoperiod of 12 h (hours) dark:12 h light under low-intensity fluorescent light (30 μE m−2 s−1).
Experimental Culture Conditions
All the experiments and response variables were performed in triplicate (n = 3) in artificial seawater (ASW) medium following Kim et al. (2007) at pH 7, adding 1 mL−1 per L of trace metal [L−1: 2.86 g H3BO3, 1.81 g MnCl2·4H2O, 0.22 g ZnSO4·7H2O, 0.39 g NaMoO4·2H2O, 0.079 g CuSO4·5H2O and 0.049 g Co(NO3)2·6H2O], glucose (final concentration 0.1% [v/v]), and with the respective MPs and/or organic additives. Inorganic phosphorus (, 0.04 g L−1 K2HPO4), iron (Fe, 0.006 g L−1 ferric citrate) and inorganic nitrogen (NH3, 0.5 g L−1 NH3Cl) were added according to the response variable measured as we described below. Bacteria at their exponential phase were inoculated in the treatments (Supplementary Table 1) and incubated for 72 h under the same conditions as when they were previously acclimatized (i.e., at 25°C, 120 r.p.m in a rotatory shaker with a photoperiod of 12 h dark:12 h light, under low-intensity fluorescent light, 30 μE m−2 s−1).
The pure MPs (low-density polyethylene [PE] with size 109 ± 6.29 μm, polypropylene [PP] with size 90 ± 7.56 μm and low-density polyvinyl chloride [PVC] with size 164 ± 8.03 μm) and organic additives (fluoranthene, 1,2,5,6,9,10-hexabromocyclododecane [HBCD] and dioctyl-phthalate [DEHP]) used were obtained from Sigma-Aldrich. Besides, we used fluorescent polystyrene (PS)-based latex beads (Fluoresbrite® YG Microspheres 1.00 μm, Polysciences, Inc.) as the lowest sized MPs, based on the definition of MPs (Hartmann et al., 2019). The stock solution of MPs was made at 100 mg mL−1, resuspending the MPs (previously UV-sterilized for 15 min) in acetone (98% [v/v]) to avoid aggregation of MPs and for easier manipulation of the workings solutions. To prevent chemical damages of MPs by acetone, the stock solution was rapidly diluted to working solutions of 1 mg mL−1 in ASW. The organic additives, i.e., fluoranthene and HBCD, were initially prepared in 1 mg mL−1 in absolute ethanol and acetone (98% [v/v]), respectively. For the DEHP additive that was in liquid form, a stock solution of 1 mg mL−1 was also prepared. We diluted these stock solutions to working solutions of 3 mg L−1 in ASW. Fluorescent PS beads were sterilized following the manufacturer's instructions, and the different concentrations in ASW were made from a stock solution of 4.55 × 1010 particles mL−1. The controls were made with the respective amounts of acetone and/or ethanol (without any MPs nor organic additives). All the treatments have ≤ 1% acetone or ethanol to avoid any biological effect in the cells, and co-solvents effect in water (Schwarzenbach et al., 2002).
Experiments were performed in two parts (Supplementary Table 1), i.e., (I) under environmentally relevant concentrations (in which we performed an initial screening of the five strains selected) and (II) the “worst-case” scenario (in which we selected two strains, one cyanobacterium and one heterotrophic bacterium as our model strains). Ecotoxicology thresholds for MPs were determined following (Reddy et al., 2006; Suaria et al., 2016; Everaert et al., 2018; Kane et al., 2020), which established that MPs can accumulate up to 29–133 μg mL−1 in the water column and seafloor. For organic additives, we followed the concentrations reported in Hermabessiere et al. (2017), in which it is reported that additives can accumulate between from pg L−1 to μg L−1, finding up to 44.39 μg L−1 in the water column.
Under Environmental Concentrations
In the first part, we made an overall screening of the five culture strains in sterile 2 mL well microplates (with 2 mL of culture media) to study their growth response under marine environmentally relevant concentrations of MPs and additives, with optimal nutrient conditions of , Fe and NH3 (n = 3). We used five concentration for MPs (0, 0.01, 0.1, 1, and 100 μg mL−1) and organic additives (0, 0.3, 3, 30, and 300 μg L−1) (Supplementary Table 1). Additional treatments combining MPs and plastic additives (e.g., PE-fluoranthene, PP-HBCD, and PVC-DEHP) were done by combining the lowest and the highest number of MPs and additives to test for possible interacting effects of MPs and their organic additives (Supplementary Table 1). Growth analysis was assessed through flux cytometry (n = 3).
Under the “Worst-Case” Scenario
In the second part, we selected two strains, one autotrophic (Halothece sp., being our model phototrophic bacteria) and one heterotrophic (Cobetia sp., which was our model heterotrophic bacteria). We established two levels MPs and additives concentration: 100 and 1,000 μg mL−1 for MPs, and 300 and 3,000 μg L−1 for the organic additives. We also selected a concentration of 4.55 × 106 and 4.55 × 107 particles mL−1 for PS beads. We cultured the test bacteria in 50 mL falcon (with 30 mL of culture media) tubes under N2-fixing conditions (limiting NH3 [~ 0.15 mM] and with optimal and Fe) for growth, protein overproduction, microscopical analysis, -uptake and N2-fixation assays, or under -limiting conditions (with optimal NH3 and Fe) for alkaline phosphatase activity (APA) (n = 3, Supplementary Table 1).
Flow Cytometry
Aliquots of cultures from all the experiments were taken initially and after 72 h of incubation and counted in fresh (without freezing nor fixing) with a Becton Dickinson FACS-Verse cytometer (Beckton & Dickinson, Franklin Lakes, New Jersey, USA). Fluorescent beads, BD FACSuite™ CS&T research beads (Beckton & Dickinson and Company BD Biosciences, San Jose, USA), were used as internal standards to calibrate the instrument. Cells were separated by combinations of the scatter plots of the flow cytometer parameters: forward scatter (FSC, reflecting cell size), side scatter (SSC, reflecting internal complexity of the cells), and/or fluorescein isothiocyanate filter (FITC, reflecting fluorescence, 488 nm excitation, 530/30 nm emission). For treatments with fluorescent PS beads, adsorption to them was measured (without detaching cells from the beads) using the FSC and FITC cytometer signals (Supplementary Figure 1). Adsorbed bacteria were those with intermediate intensity fluorescence signals between the free bacteria and the beads (Supplementary Figure 1). In all the experiments, a total of 10,000 cells (or cells recorded in 30 s) were counted in each sample. Changes in growth were calculated as the changes in cell concentrations after 72 h.
Protein Identification: MALDI-TOF Assay and Protein Structure Prediction
Crude cell extracts were done following the methods described in Ivleva and Golden (2007), using the cultures of Halothece sp. and Cobetia sp. after 72 h of incubation in the “worst-case” scenario (at the highest concentration treatment of MPs/additives and the control). Protein concentrations were determined with Bradford protein assay (Thermofisher), following the manufacturer's instructions. The protein extracts were run into polyacrylamide gels, 4–20% (p/v) Amersham ECL Gel (GE Healthcare, Chicago, Illinois, EEUU), using the ECL Gel Box system (GE Healthcare, Chicago, Illinois, EEUU) following the manufacturer's instructions.
The different bands detected only in Cobetia sp. exposed to high concentrations of MPs (1,000 ug mL−1 of PE, PP, and PVC), which did not appear at the control, were excised from the gel with a clean scalpel and sent to MALDI-TOF analysis. Each gel slice was cut into small pieces and then transferred to a clean and sterile Eppendorf tube. Protein identification was performed following Jaén-Luchoro et al. (2017). The samples were then analyzed with an Autoflex III MALDI-TOF-TOF (BrukerDaltonics) spectrometer using the software Compassflex series v1.4 (flexControl v3.4, flexAnalysis v3.4 and BioTools 3.2). The spectra were calibrated using the peptide calibration standard (BrukerDaltonics). The obtained mass spectra were used for the protein identification and the in-house database was created with the predicted protein sequence of the annotated genome of Cobetia sp. The search process was performed with the algorithm MASCOT (MatrixSciences).
Fasta sequence of the alcohol dehydrogenase (ADH) (detected through MALDI-TOF) was sent to the I-Tasser server for protein 3D-structure prediction (Zhang, 2008), with their domains previously checked in Pfam 32.0 (Finn et al., 2016). The predicted structure was sent to the FunFOLD2 server for the prediction of protein–ligand interactions (Roche et al., 2013). Besides, we detected the potential sites of ligand or “pockets” through MetaPocket 2.0 (Huang, 2009). Finally, we predicted the orientation and position of the protein-ligand complex between polyethylene glycol (PEG) and ADH, docking these with Swissdock (Zoete and Michielin, 2011). All the structures were visualized by Pymol (DeLano, 2002).
Microscopic Observations
At the final time (after 72 h), the five strains tested were placed onto a microplate for inverted microscopy visualization of the physical interactions between bacterial cells and the MPs (i.e., PE, PP, and PVC) at 100x objective (Leica DM IRB). For Halothece sp. and Cobetia sp., their interaction with PS fluorescent beads (with an excitation of 441 nm and emission of 485 nm) were visualized by confocal microscopy (Leica TCS SPE, Leica Microsystems). Images were processed using the software Leica application suite (Leica Microsystems). The specific autofluorescence for Halothece sp. was observed with an excitation of 532 nm and an emission of 555–619 nm. For Cobetia sp., the cells were stained with Sybr green (Sigma-Aldrich), or propidium iodide (Sigma) to properly visualize the cells distinguishing them from the PS beads.
P-Metabolism Analysis
Alkaline phosphatase activity (APA) was evaluated through fluorometric assay following the hydrolysis of the substrate (S) 4-methylumbelliferyl phosphate (MUF-P, Sigma-Aldrich) to 4-methylumbelliferyl (MUF), following Fernández-Juárez et al. (2019). The culture media, i.e., in the “worst-case” scenario, was limited (but with optimal NH3 and Fe) to promote APA. Saturation curves of velocity (V, fmol MUF cell−1 h−1) vs. substrate (S, μM) were made for each experimental condition for each of the two selected strains (Halothece sp. and Cobetia sp.). We used different concentrations of MUF-P: 0, 0.05, and 5 μM of MUF-P. After 1 h incubation in darkness at room temperature, APA was measured in a microtiter plate that contained buffer borated pH 10 (3:1 of sample: buffer). The MUF production (fmol MUF cell−1 h−1) was measured with a Cary Eclipse spectrofluorometer (FL0902M009, Agilent Technologies) at 359 nm (excitation) and 449 nm (emission), using a calibration standard curve with commercial MUF (Sigma-Aldrich). The maximum velocity (Vmax) at saturating substrate concentrations was obtained from each plot of V vs. S, using the Lineweaver-Burk plot.
For the determination of inorganic concentrations for Halothece sp. and Cobetia sp. experiments, 1 mL of the culture was centrifuged for 15 min at 16,000 × g under 4°C. The bacteria-free clear supernatant was collected and used for determinations following standard spectrophotometric methods (Hansen and Koroleff, 2007). The concentrations in the culture media were determined at the initial and final time (after 72 h). Specific -uptake rates (pmol cell−1 d−1) were calculated as described in Fernández-Juárez et al. (2019) and Ghaffar et al. (2017).
Determination of N2-Fixation Rates Through Acetylene Reduction Assay (ARA)
Rates of N2-fixation were measured for Halothece sp. as our model strain, described in Fernández-Juárez et al. (2019, 2020), under the “worst-case” scenario. A known volume of culture (8 mL) was sampled during the dark photoperiod and transferred to a closed hermetic vial and oxygen was flushed from the sample through bubbling with N2 gas. A medium with saturated acetylene was injected at 20% (v/v) final concentration in each vial with a sterile syringe. Samples were incubated for 3 h at room temperature (24°C) in the dark. After the 3 h incubation time, 10 mL of liquid was removed and transferred to Hungate tubes containing 1.25 mL of 20% trichloroacetic acid (Agawin et al., 2014). Prior to analysis with the gas chromatograph (GC), the Hungate tubes were incubated at 37°C overnight in a water bath. Ethylene and acetylene gas from the gas phase of the Hungate tubes were determined using a GC (model GC-7890, Agilent Technologies) equipped with a flame ionization detector (FID), following the set up described in Fernández-Juárez et al. (2019, 2020). Ethylene produced was calculated using the equation in Stal (1988). The acetylene reduction rates were converted to N2-fixation rates (nmol mL−1 h−1) using a factor of 4:1 (Jensen and Cox, 1983).
Statistical Analysis
Non-normally distributed data, the Kruskal-Wallis rank-sum non-parametric test was used since the size sample was n < 20. An unpaired two-sample Wilcoxon test was used to determine the significant effects among different concentrations of MPs and additives. All analyses were done in R-Studio, R version 3.5.3 (2019-03-11).
Results and Discussion
Effects of Varying Concentrations of MPs and Additives, Under Relevant Concentrations in the Marine Environment
The addition of PE within the range of 0–100 μg mL−1 did not significantly affect all the diazotrophs tested (p > 0.05, n = 3, Figure 1A), in agreement with Machado et al. (2020). Moreover, PP and PVC within the range of 0–100 μg mL−1 did not significantly affect the growth of heterotrophic bacteria (Cobetia sp., Marinobacterium litorale and Pseudomonas azotifigens) (p > 0.05, n = 3, Figures 1B,C). This is consistent with the results obtained by Piccardo et al. (2020), in which PET microparticles at 100 μg mL−1 do not have affect in the heterotrophic bacteria (e.g., Vibrio fischeri). However, species-specific growth responses of the bacteria tested with the addition of MPs were exemplified here when PVC and PP addition (at 100 μg mL−1) significantly enhanced the growth of the autotrophic cyanobacterial diazotrophs (Halothece sp. and Fischerella muscicola) by 1.5- to 4- fold (p < 0.05, n = 3, Figures 1B,C). This supports the idea that MPs may be selecting bacterial communities in the ocean (Seeley et al., 2020).
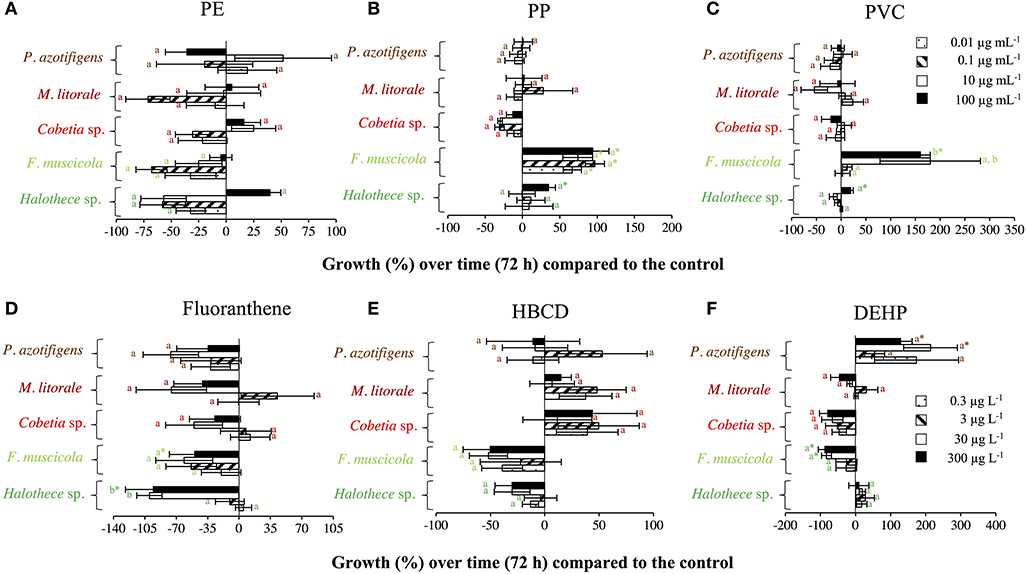
Figure 1. Growth responses of diazotrophs subject to MPs and organic additives under relevant concentrations in the marine environment after 72 h, compared with the control value. (A) PE, (B) PP, and (C) PVC, with 0, 0.01, 0.1, 10, and 100 μg mL−1. (D) Fluoranthene, (E) HBCD, and (F) DEHP, with 0, 0.3, 3, 30, and 300 μg L−1. Values are the mean, and the error bar is the standard error between the replicates (n = 3). Letters indicate pairwise analysis among the variables (i.e., concentration) inside each strain (see the different colors), and asterisks (*) indicate pairwise significant differences between variables and the control (p < 0.05), using a post-hoc test (Wilcoxon) after Kruskal-Wallis over the whole dataset.
The effect of organic additives on bacterial growth is suggested here to be dependent on the type of additive (i.e., fluoranthene, HBCD and DEHP) (Figures 1D–F). Fluoranthene reduced the growth of Halothece sp. and F. muscicola, by 22- and 7- fold, respectively, at the highest concentrations compared to the control (p < 0.05, n = 3, Figure 1D). Besides, DEHP significantly reduced the growth of F. muscicola at the highest concentrations compared to the control (p < 0.05, n = 3, Figure 1F). On the contrary, the additive HBCD did not affect the growth of any species (p > 0.05, n = 3, Figure 1E), while the additive DEHP significantly enhanced the growth of the heterotrophic P. azotifigens by 4-fold, being significative at 30 and 300 μg L−1 compared to the control (p < 0.05, n = 3, Figure 1F). Additives can be sorbed and/or liberated by MPs in marine environments, with contrasting consequences (Gallo et al., 2018; Hahladakis et al., 2018). If sorbed, these chemicals may be less available for cells, being less harmful to sensitive species (Hahladakis et al., 2018), but detrimental to species making use of them as a C-source (Cao et al., 2015; Wang et al., 2015). If liberated, the increased availability of additives may be more harmful to sensitive species (Tetu et al., 2019; Sarker et al., 2020), but benefit species using them (Cao et al., 2015; Wang et al., 2015). In our experiments, PVC + DEHP significantly enhanced the growth of P. azotifigens and Cobetia sp. (synergism), but significantly altered it when PE + fluoranthene were added (antagonism) (p < 0.05, n = 3, Figure 2), while the addition of PP + HBCD did not have any further effect (p > 0.05, n = 3, Figure 2).
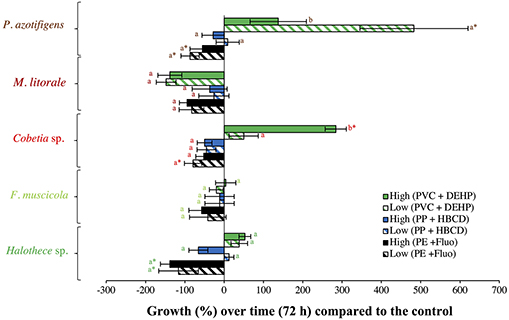
Figure 2. Growth responses of diazotrophs subject to MP-additive interactions (PE + fluoranthene, PP + HBCD, and PVC + DEHP) after 72 h (under relevant concentrations), compared with the control value. “Low” and “High” represent low and high concentrations of MPs (0.01 and 100 μg mL−1, respectively) and plastic additive (0.3 and 300 μg L−1, respectively). Values are the mean, and the error bar is the standard error between the replicates (n = 3). Letters indicate pairwise analysis among the variables (i.e., concentration) inside each strain (see the different colors), and asterisks (*) indicate pairwise significant differences between variables and the control (p < 0.05), using a post-hoc test (Wilcoxon) after Kruskal-Wallis over the whole dataset.
Effect of Varying Concentrations of MPs and Additives, Under the “Worst-Case” Scenario
Effect of High Concentration of MPs
High MPs concentrations stimulated cell growth, especially with the addition of PVC in both strains compared with the control by 6- to 8- fold (p < 0.05, n = 3, Figure 3A). The enhancement of the bacterial growth, especially in the heterotrophic strain, Cobetia sp., after 72 h of incubation can be due to increased dissolved organic carbon (DOC) pool in the medium that could have leached from the MPs added (Romera-Castillo et al., 2018). Unfortunately, DOC was not measured.
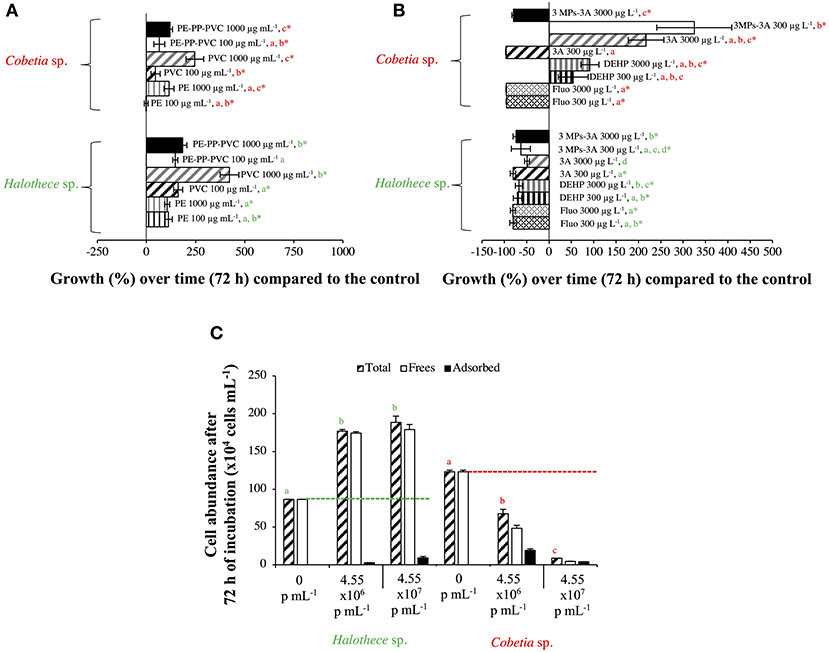
Figure 3. Growth responses of diazotrophs subject to high concentrations (the “worst-case” scenario) of MPs and additives after 72 h, compared with the control value. (A) Effect of MPs (PE, PP, and/or PVC, 100/1,000 μg mL−1). The effect of PP alone with non-significant results is not included in the graph. (B) Effect of organic additives (fluoranthene, HBCD, and/or DEHP, 300/3,000 μg L−1). The effect of HBCD alone with non-significant results is not included in the graph. (C) Effect of PS beads. Total, free and adsorbed bacteria (to the beads) are differentiated. Values are the mean, and the error bar is the standard error between the replicates (n = 3). Letters indicate pairwise analysis among the variables (i.e., concentration) inside each strain (see the different colors), and asterisks (*) indicate pairwise significant differences between variables and the control (p < 0.05), using a post-hoc test (Wilcoxon) after Kruskal-Wallis over the whole dataset.
Species-specific responses based on protein production profile are also shown here when two proteins related to plastic degradation (alcohol dehydrogenase [ADH] of 342 amino acids, HA399_02440) and carbon transport (C4-dicarboxylate ABC transporter substrate-binding protein [C4-ABCS] of 329 amino acids, HA399_06715) were overproduced in the heterotroph Cobetia sp., but were not detectable with the MALDI-TOF analyses in the cyanobacteria Halothece sp. after the addition of MPs at high concentrations. ADHs from Rhodopseudomonas acidophila M402 and Pseudomonas oleovorans have been shown to oxidize plastic polymers (e.g., PEG), being NAD-dependent (Ohta et al., 2006; Kawai, 2010), consistent with our in silico structural analysis for Cobetia sp. (Figures 4A–C). Further experimental studies have to be performed to evaluate if Cobetia sp. can degrade PEG polymers or similar ones, and indeed if the carbon released by ADH is transported by C4-ABCS inside the cells since C4-ABCS is a carbon transporter (Rosa et al., 2019).
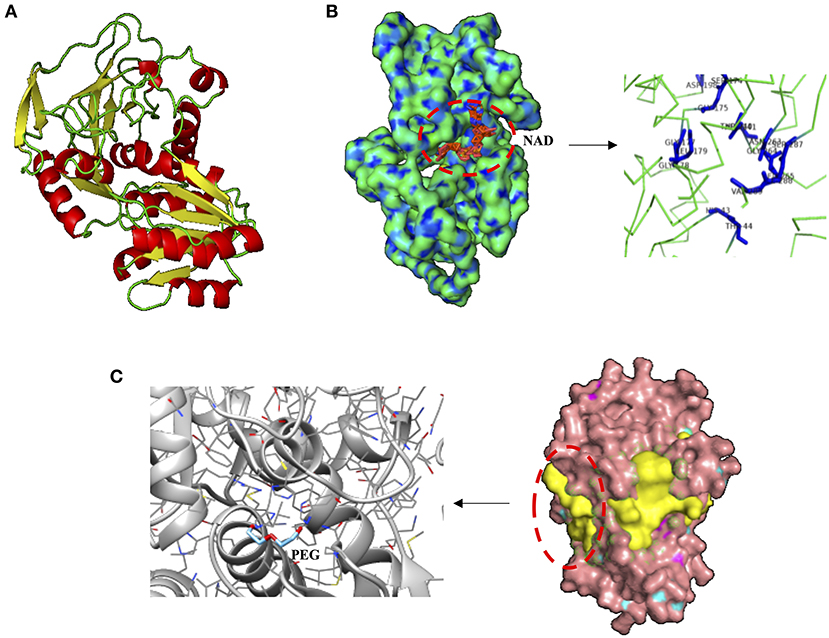
Figure 4. Structural analysis of alcohol dehydrogenase (ADH) from Cobetia sp. (HA399_02440). (A) Protein prediction of ADH showing, (B) the nicotinamide-adenine-dinucleotide (NAD) dependence, displaying the ligand binding residues HIS 43, THR 44, SER 174, GLY 175, GLY 177, GLY 178, LEU 179, ASP 198, THR 240, ASP 241, ASN 263, GLY 264, LYS 265, SER 287, ILE 288, and VAL 289. (C) The three of the most probable pockets of the ADH (potential sites of ligand binding, in yellow), and the docking site (prediction of the orientation and position of protein-ligand complex) between the polyethylene glycol (PEG) and ADH.
Effect of the Size of MPs
Larger-sized MPs (i.e., 120 μm) enhanced the growth of autotropic and heterotrophic bacteria at high concentrations of MPs (Figure 3A). Larger-sized MPs may provide more surface area for the cells to adhere and attach as observed in the heterotrophs tested here (Figures 5B–E). Surface attachment of the cells to the MPs through hydrodynamic and electrostatic interactions may enhance growth and facilitate nutrient uptake (especially under oligotrophic conditions), in most of the cases by the biofilm formation, increasing the surface of the substrates. This can aid the uptake of the necessary metabolites and co-factors, as suggested in Tuson and Weibel (2013). Smaller-sized MPs (i.e., PS beads of 1 μm-size), however, affected negatively the smallest size heterotrophic bacteria (i.e., Cobetia sp. of ~ 1 μm-size, p < 0.05, n = 3, Figure 3C), and not the unicellular cyanobacteria (i.e., Halothece sp. of ~ 4 to 7 μm-size) (p > 0.05, n = 3, Figure 3C). This can be due to the differences in the degree of physical adsorption between the PS beads and the different bacterial species. Approximately 40–87% of Cobetia sp. cells were adsorbed to PS (Figures 3C, 5H,I), while only ~2–5% of Halothece sp. cells were adsorbed (Figure 3C). The mechanisms behind the negative effect of small-sized MP in small-sized bacteria needs to be further studied and can possibly be due to disruption of bacterial cell division by the aggregation of the cells and beads. Although fewer PS beads were adsorbed on Halothece sp. cells, an invagination of the cell membranes by PS beads has been observed, possibly being engulfed or included as a carbon source (Figures 5F,G).
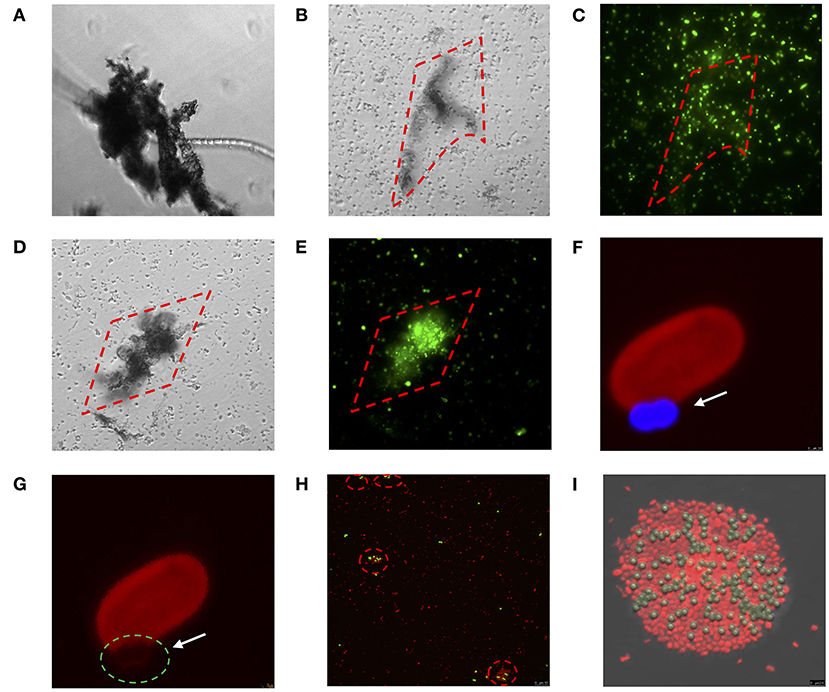
Figure 5. Direct physical interaction between MPs and bacterial species tested in which (A–E) show the interaction between diazotrophs and mix of MPs (PE, PP, and PVC) at 100 μg mL−1 through inverted microscopy: (A) Fischerella muscicola under bright-field (BF), (B,C) Cobetia sp. under BF and Sybr-green channel, respectively, (D,E) Marinobacterium litorale under BF and Sybr-green channel, respectively; (F–I) show the physical interaction between diazotrophs and PS 1 μm beads (excitation of 441 nm and emission of 485 nm) through confocal microscopy: (F,G) Halothece sp. under excitation of 532 nm wavelength and emission of 555–619 nm. The arrows and green dashed circle show where the cell membrane is interacting with the PS bead (blue). (H,I) Cobetia sp. under excitation of 493 and 636 nm of emission. The dashed red circles show cell agglomeration on the PS beads (yellow). Images were taken at 1000x (A–E and H) and further magnified (F,G,I).
Physicochemical Properties of MPs
The physicochemical properties (e.g., hydrophobicity, electrostatic attraction, or roughness) of different MPs may affect the responses of bacterial communities (Ogonowski et al., 2018). Hydrophobicity, for example, can directly affect the bacterial colonization of MPs. PS polymers, which have aromatic phenyl groups, are one of the most hydrophobic polymers (Ogonowski et al., 2018), and this may explain the adherence of both autotrophic and heterotrophic bacteria to PS beads (Figures 5F–I). Contrary to the cyanobacterial diazotrophs (Halothece sp. and F. muscicola), which were not capable of adhering to the MPs, heterotrophic N2-fixers (Cobetia sp., M. litorale, and P. azotifigens) tested were able to adhere to other types of MPs (i.e., PE, PP, and PVC, Figures 5A–E) which are less hydrophobic than PS. Moreover, PVC polymers are slightly more hydrophilic than PE (Kennedy, 2014), suggesting that they can be less available for adhesion and more available for bacterial growth. This may explain why PVC polymers were the MPs that most enhanced bacteria growth (Figure 3A).
Effect of High Concentrations of Organic Additives
Contrary to the effects of MPs, the addition of different types of organic additives (fluoranthene, HBCD and/or DEHP up to 3,000 μg L−1) affected negatively the growth of Halothece sp. by 8-fold (p < 0.05, n = 3, Figure 3B). For the heterotrophic bacteria, different responses were observed, being the fluoranthene the most toxic pollutant (p < 0.05, n = 3, Figure 3B). The differing sensitivities of different species of bacteria to a particular type of additive can be due to cell-size dependent toxicity. For example, here we found an increasing toxicity to a PAH additive, fluoranthene, from bigger- to smaller-sized: Cobetia sp. (~1 μm) > unicellular cyanobacteria Halothece sp. (4–7 μm), > filamentous cyanobacteria F. muscicola (~7 μm) (p < 0.05, Spearman's correlation, n = 21, r2 = 0.7) (Figures 1D, 3B). The negative correlation between cell size and PAH toxicity is consistent with the study of Echeveste et al. (2010), and may be due to the higher surface to volume ratio of smaller-sized cells which increases the potential of the additives to be adsorbed or consumed by the cells. The DEHP additive (at high concentrations) and the interaction of the three MPs with the three plastic additives at low concentrations enhanced the growth of Cobetia sp. (p < 0.05, n = 3, Figure 3B), indicating that this species might use DEHP as a C-source and can be a possible bioremediator of DEHP-contaminated environments like Rhodococcus (Wang et al., 2015). Nevertheless, here we did not intend to reproduce an environmental situation since it may be improbable to find such high concentrations in the water column due to their solubility. However in marine sediments, concentrations of up to 2,988 μg Kg−1 of organic pollutants can be found in extremely impacted areas (Hermabessiere et al., 2017), and thus our results provide useful data to understand the response of the microorganisms associated with the benthic organisms.
Effect of MPs Pollution in the P and N-Metabolism
P-Acquisitions Mechanisms
The MPs and their plastic additives generally enhanced the alkaline phosphatase activity (APA) of Halothece sp. The addition of PS beads (at 4.55 × 107 particles mL−1) increased the maximum rate of reaction (Vmax) up to 0.21 fmol MUF cell−1 h−1, significantly higher than controls with a Vmax of 0.033 fmol MUF cell−1 h−1 (p < 0.05, n = 3, Figure 6A). In previous experiments, we described that the cyanobacterial N2-fixer Halothece sp. synthesizes an alkaline phosphatase D (PhoD) that is Fe dependent (Fe as metal co-factor) (Fernández-Juárez et al., 2019). Since metals (i.e., Fe) can be accumulated onto the plastics (Rochman et al., 2014), it is hypothesized that MPs may promote an environment rich in Fe co-factors. Considering the P-dependence of important processes (e.g., N2-fixation, Fernández-Juárez et al., 2019), stimulation of APA can promote the growth of Halothece sp. For this unicellular cyanobacterium, -uptake rates were significantly downregulated by the addition of MPs and their plastic additives (p < 0.05, n = 3, Figure 6B). Comparisons between treatments were made (Supplementary Table 2), showing that the combination of the three MPs (i.e., high levels) and the three additives (i.e., low levels) were the treatments with lower reduction of the -uptake. The decreased -uptake rates observed in Halothece sp. may be due to the adsorption of phosphate ions by PE and PVC (Hassenteufel et al., 1963).
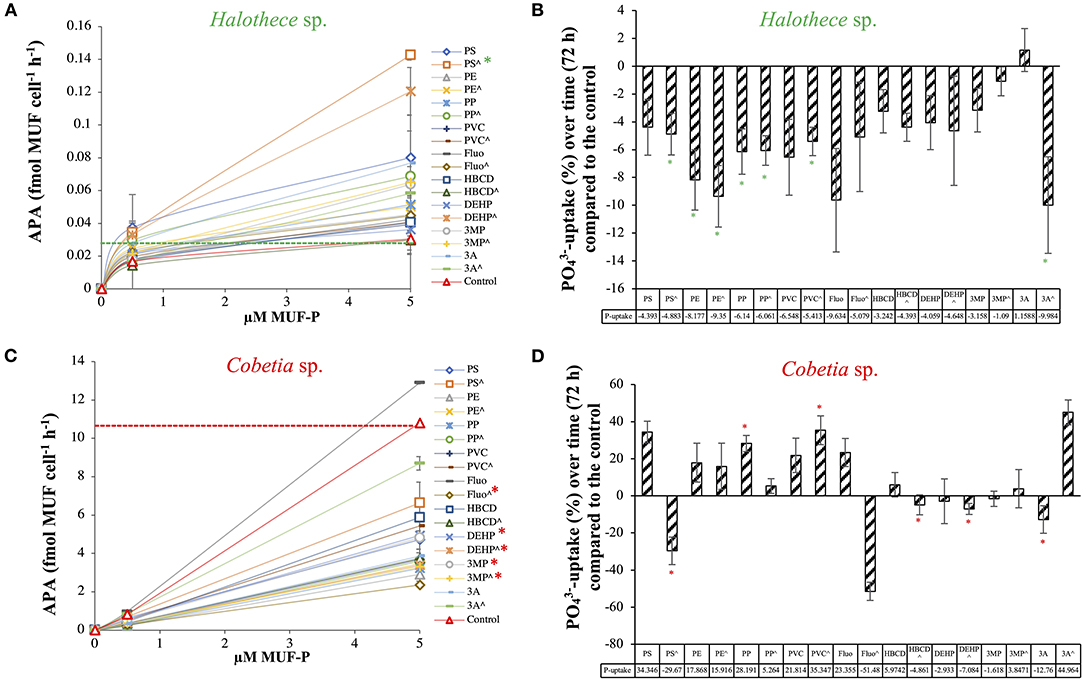
Figure 6. Phosphorus acquisition mechanisms for two species of N2-fixers exposed to different MPs and their plastic additives: (A) Alkaline phosphatase activity (APA, fmol MUF cell−1 h−1) and (B) -uptake (pmol cell−1 d−1) for Halothece sp., using relative values (with the control as reference). (C) APA and (D) -uptake for Cobetia sp., using relative values (with the control as reference). (∧) indicates treatments up to 1,000 μg mL−1 (MPs) and 3,000 μg L−1 (organic additives), without (∧) indicates treatments with 100 μg mL−1 (MPs) and 300 μg L−1 (organic additives). Values are the mean, and the error bar is the standard error between the replicates (n = 3). Asterisks (*) indicate significant differences (p < 0.05) compared with the controls, using a post-hoc test (Wilcoxon) after Kruskal-Wallis over the whole dataset.
Unlike Halothece sp., APA for Cobetia sp. was generally reduced by MPs and their organic additives (p < 0.05, n = 3, Figure 6C). Among the MPs, PE addition at high concentrations caused the most significant decrease in APA (Vmax = 8.52 fmol MUF cell−1 h−1) compared to controls (Vmax = 32.78 fmol MUF cell−1 h−1) (p < 0.05, Figure 6C). Among the plastic additives, fluoranthene caused the highest decrease in APA (Vmax = 11.52 fmol MUF cell−1 h−1) compared to controls (p < 0.05, Figure 6C). Significant differences in -uptake rates were observed among the treatments tested (p < 0.05, n = 3, Figure 6D and Supplementary Table 2). Contrary to Halothece sp., -uptake was increased with the addition of PP and PVC (p < 0.05, n = 3, Figure 6D), maybe as a consequence of higher nutrient and energy requirements for growth. Hence, we show species-specific differences of the P-mechanisms and P-requirements and the responses of these processes to MPs and their additives, showing that P-homeostasis can be disturbed with the addition of MPs and their organic additives associated.
Effect on N2-Fixation in Cyanobacteria
In a seminal paper, Bryant et al. (2016) claimed that MPs may be hot spots of N2-fixing autotrophic bacteria, based on the high abundances of N2-fixation genes (nifH, nifD, and nifK) in the metagenomes associated with the plastic. Unfortunately, the authors did not measure the N2-fixation activities, considering that N2-fixation rates in the open ocean are largely maintained by cyanobacteria (Zehr and Capone, 2020). Hence, cyanobacteria N2-fixers can be one of the most impacted groups. Here, the effects of MPs and their additives on N2-fixation rates are reported for the first time in cyanobacteria (i.e., Halothece sp., Figure 7). However, MPs and their additives did not have a significant effect on specific N2-fixation rates of Halothece sp. (p > 0.05, n = 3, Figure 7), but as we showed that growth was positively enhanced with the addition of MPs (Figure 3A), or negatively affected by the addition of organic additives (Figure 3B), these pollutants could eventually enhance/inhibit global N2-fixation rates in the environment.
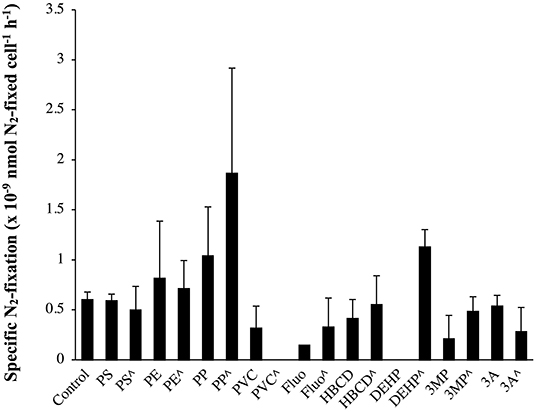
Figure 7. Specific N2-fixation rates (nmol N2-fixed cell−1 h−1) in the unicellular cyanobacteria Halothece sp. exposed to MPs and their plastic additives. (∧) indicates treatments up to 1,000 μg mL−1 (MPs) and 3,000 μg L−1 (organic additives), and without (∧) indicates treatments with 100 μg mL−1 (MPs) and 300 μg L−1 (organic additives). Values are the mean, and the error bar is the standard error between the replicates (n = 3).
In summary, this study shows that the most predominant MPs (e.g., PE, PP, PVC, and PS) in the oceans and their commonly associated organic additives (i.e., fluoranthene, HBCD, and DEHP) can be beneficial (the “good”), deleterious (the “bad”), or both (the “double-sword”) to marine bacteria. Our study provides useful data to understand the response of marine bacteria, especially the diazotrophs to MPs pollution. Nevertheless, the transposition of the results obtained under in vitro controlled conditions must be taken with precautions since our study used concentrations that may not be representative of all marine environments. Open questions such as how the hydrophobicity of MPs can affect the growth responses, or if N2-fixers may have another important environmental role of biodegrading synthetic plastic polymers aside from their important ecological role of providing new N into marine ecosystems, have to be addressed. The use of next-generation analysis (i.e., transcriptomic or proteomic assays) to identify changes in gene expression or protein profiles derived from MPs and plastic additives may allow a better comprehension of the molecular responses behind the plastic threat in oceans.
Data Availability Statement
The datasets presented in this study can be found in online repositories. The names of the repository/repositories and accession number(s) can be found in the article/Supplementary Material.
Author Contributions
VF-J and XL-A conducted all experiments with the help of AF-C, PE, AB-F, GR-M, and RG in the various parameters measured in the study. VF-J and NA led the writing of the MS. NA is the supervisor of the laboratory. All authors contributed to the article and approved the submitted version.
Funding
This work was supported by funding to NA through the Ministerio de Economía, Industria y Competitividad-Agencia Estatal de Investigación, and the European Regional Development Funds project (CTM2016-75457-P).
Conflict of Interest
The authors declare that the research was conducted in the absence of any commercial or financial relationships that could be construed as a potential conflict of interest.
Acknowledgments
We acknowledge the support and help of scientific technical service (Maria Trinidad Garcia Barceló) of the University of the Balearic Islands for gas-chromatography analyses. We also thank Pere Ferriol Buñola and Alba Coma Ninot for the help in the acquisition of the cultures, and project AAEE117/2017 of the Dirección General Innovación y Recerca CAIB.
Supplementary Material
The Supplementary Material for this article can be found online at: https://www.frontiersin.org/articles/10.3389/fmicb.2020.581118/full#supplementary-material
References
Agawin, N. S. R., Benavides, M., Busquets, A., Ferriol, P., Stal, L. J., and Arístegui, J. (2014). Dominance of unicellular cyanobacteria in the diazotrophic community in the Atlantic Ocean. Limnol. Oceanogr. 59, 623–637. doi: 10.4319/lo.2014.59.2.0623
Agawin, N. S. R., Ferriol, P., Sintes, E., and Moyà, G. (2017). Temporal and spatial variability of in situ nitrogen fixation activities associated with the Mediterranean seagrass Posidonia oceanica meadows. Limnol. Oceanogr. 62, 2575–2592. doi: 10.1002/lno.10591
Almroth, B.C., and Eggert, H. (2019). Marine plastic pollution: sources, impacts, and policy issues. Rev. Environ. Econ. Policy, 13, 2, 317–326, doi: 10.1093/reep/rez012
Bakir, A., Rowland, S.J., and Thompson, R.C. (2014). Enhanced desorption of persistent organic pollutants from microplastics under simulated physiological conditions. Environ. Pollut. 185, 16–23. doi: 10.1016/j.envpol.2013.10.007
Bryant, J.A., Clemente, T.M., Viviani, D.A., Fong, A.A., Thomas, K.A., Kemp, P., et al. (2016). Diversity and activity of communities inhabiting plastic debris in the North Pacific Gyre. mSystems 1, 1–19. doi: 10.1128/mSystems.00024-16
Cao, J., Lai, Q., Yuan, J., and Shao, Z. (2015). Genomic and metabolic analysis of fluoranthene degradation pathway in Celeribacter indicus P73 T. Sci. Rep. 5, 1–12. doi: 10.1038/srep07741
Cole, M., and Galloway, T.S. (2015). Ingestion of nanoplastics and microplastics by pacific oyster larvae. Environ. Sci. Technol. 49, 14625–14632. doi: 10.1021/acs.est.5b04099
DeLano, W. (2002). The PyMOL Molecular Graphics System. Schrödinger, LLC. Available online at: http://www.pymol.org
Echeveste, P., Agustí, S., and Dachs, J. (2010). Cell size dependent toxicity thresholds of polycyclic aromatic hydrocarbons to natural and cultured phytoplankton populations. Environ. Pollut. 158, 299–307. doi: 10.1016/j.envpol.2009.07.006
Erni-Cassola, G., Zadjelovic, V., Gibson, M. I., and Christie-oleza, J. A. (2019). Distribution of plastic polymer types in the marine environment : a meta- analysis. J. Hazard. Mater. 369, 691–698. doi: 10.1016/j.jhazmat.2019.02.067
Everaert, G., Van Cauwenberghe, L., De Rijcke, M., Koelmans, A. A., Mees, J., Vandegehuchte, M., et al. (2018). Risk assessment of microplastics in the ocean: Modelling approach and first conclusions. Environ. Pollut. 242, 1930–1938. doi: 10.1016/j.envpol.2018.07.069
Fernández-Juárez, V., Bennasar-Figueras, A., Sureda-Gomila, A., Ramis-Munar, G., and Agawin, N. S. R. (2020). Differential effects of varying concentrations of phosphorus, Iion, and nitrogen in N2-Fixing Cyanobacteria. Front. Microbiol. 11, 1–19. doi: 10.3389/fmicb.2020.541558
Fernández-Juárez, V., Bennasar-Figueras, A., Tovar-Sanchez, A., and R. Agawin, N. S. (2019). The role of iron in the P-acquisition mechanisms of the unicellular N2-fixing cyanobacteria Halothece sp. found in association with the Mediterranean seagrass Posidonia oceanica. Front. Microbiol. 10, 1–22. doi: 10.3389/fmicb.2019.01903
Finn, R.D., Coggill, P., Eberhardt, R.Y., Eddy, S.R., Mistry, J., Mitchell, A.L., et al. (2016). The Pfam protein families database: towards a more sustainable future. Nucleic Acids Res. 44, D279–D285. doi: 10.1093/nar/gkv1344
Gallo, F., Fossi, C., Weber, R., Santillo, D., Sousa, J., Ingram, I., et al. (2018). Marine litter plastics and microplastics and their toxic chemicals components: the need for urgent preventive measures. Environ. Sci. Eur. 30:139. doi: 10.1186/s12302-018-0139-z
Ghaffar, S., Stevenson, R.J., and Khan, Z. (2017). Effect of phosphorus stress on Microcystis aeruginosa growth and phosphorus uptake. PLoS ONE 12:174349. doi: 10.1371/journal.pone.0174349
Hahladakis, J.N., Velis, C.A., Weber, R., Iacovidou, E., and Purnell, P. (2018). An overview of chemical additives present in plastics: migration, release, fate and environmental impact during their use, disposal and recycling. J. Hazard. Mater. 344, 179–199. doi: 10.1016/j.jhazmat.2017.10.014
Hansen, H. P., and Koroleff, F. (2007). “Determination of nutrients,” in Methods of Seawater Analysis, 3rd Edn, eds K. Grasshoff, K. Kremling, and M. Ehrhard (Weinheim: Wiley – VCH Verlag), 159–228. doi: 10.1002/9783527613984.ch10
Harrison, P., Sapp, M., Schratzberger, M., and Osborn, A.M. (2011). Interactions between microorganisms and marine microplastics: a call for re- search. Mar. Technol. Soc. J. 45, 12–20. doi: 10.4031/MTSJ.45.2.2
Hartmann, N.B., Hüffer, T., Thompson, R.C., Hassellöv, M., Verschoor, A., Daugaard, A.E., et al. (2019). Are we speaking the same language? Recommendations for a definition and categorization framework for plastic debris. Environ. Sci. Technol. 53, 1039–1047. doi: 10.1021/acs.est.8b05297
Hassenteufel, W., Jagitsch, R., and Koczy, F. F. (1963). Impregnation of glass surfacae against sortion of phospahate traces. Limnol. Oceanogr. 8, 152–156. doi: 10.4319/lo.1963.8.2.0152
Hermabessiere, L., Dehaut, A., Paul-Pont, I., Lacroix, C., Jezequel, R., Soudant, P., et al. (2017). Occurrence and effects of plastic additives on marine environments and organisms: a review. Chemosphere 182, 781–793. doi: 10.1016/j.chemosphere.2017.05.096
Huang, B. (2009). MetaPocket: a meta approach to improve protein ligand binding site prediction. OMICS 13, 325–330. doi: 10.1089/omi.2009.0045
Ivleva, N. B., and Golden, S.S. (2007). Protein extraction, fractionation, and purification from cyanobacteria. Methods Mol. Biol. 362, 365-73. doi: 10.1007/978-1-59745-257-1_26
Jaén-Luchoro, D., Aliaga-Lozano, F., Gomila, R. M., Gomila, M., Salvà-Serra, F., Lalucat, J., et al. (2017). First insights into a type II toxin-antitoxin system from the clinical isolate Mycobacterium sp. MHSD3, similar to epsilon/zeta systems. PLoS ONE 12, 1–20. doi: 10.1371/journal.pone.0189459
Jensen, B. B., and Cox, R. P. (1983). Direct measurements of steady-state kinetics of cyanobacterial N2 uptake by membrane-leak mass spectrometry and comparisons between nitrogen fixation and acetylene reduction. Appl. Environ. Microbiol. 45, 1331–1337. doi: 10.1128/AEM.45.4.1331-1337.1983
Kane, I.A., Clare, M.A., Miramontes, E., Wogelius, R., Rothwell, J.J., Garreau, P., et al. (2020). Seafloor microplastic hotspots controlled by deep-sea circulation. Science 5899:aba5899. doi: 10.1126/science.aba5899
Kawai, F. (2010). The biochemistry and molecular biology of xenobiotic polymer degradation by microorganisms. Biosci. Biotechnol. Biochem. 74, 1743–1759. doi: 10.1271/bbb.100394
Kennedy, E. J. (2014). Biological drug products: development and strategies. ChemMedChem 9, 2814–2815. doi: 10.1002/cmdc.201402432
Kim, H., Choo, Y. J., Song, J., Lee, J. S., Lee, K. C., and Cho, J. C. (2007). Marinobacterium litorale sp. nov. in the order Oceanospirillales. Int. J. Syst. Evol. Microbiol. 57, 1659–1662. doi: 10.1099/ijs.0.64892-0
Machado, M. C., Vimbela, G. V., Silva-Oliveira, T. T., Bose, A., and Tripathi, A. (2020). The response of Synechococcus sp. PCC 7002 to micro-/nano polyethylene particles - investigation of a key anthropogenic stressor. PLoS ONE 15, 1–14. doi: 10.1371/journal.pone.0232745
Nelms, S. E., Galloway, T. S., Godley, B. J., Jarvis, D. S., and Lindeque, P. K. (2018). Investigating microplastic trophic transfer in marine top predators. Environ. Pollut. 238, 999–1007. doi: 10.1016/j.envpol.2018.02.016
Ogonowski, M., Motiei, A., Ininbergs, K., Hell, E., Gerdes, Z., Udekwu, K. I., et al. (2018). Evidence for selective bacterial community structuring on microplastics. Environ. Microbiol. 20, 2796–2808. doi: 10.1111/1462-2920.14120
Ohta, T., Kawabata, T., Nishikawa, K., Tani, A., Kimbara, K., and Kawai, F. (2006). Analysis of amino acid residues involved in catalysis of polyethylene glycol dehydrogenase from Sphingopyxis terrae, using three-dimensional molecular modeling-based kinetic characterization of mutants. Appl. Environ. Microbiol. 72, 4388–4396. doi: 10.1128/AEM.02174-05
Piccardo, M., Provenza, F., Grazioli, E., Cavallo, A., Terlizzi, A., and Renzi, M. (2020). PET microplastics toxicity on marine key species is influenced by pH, particle size and food variations. Sci. Total Environ. 715, 136947. doi: 10.1016/j.scitotenv.2020.136947
Reddy, M.S., Shaik Basha, Adimurthy, S., and Ramachandraiah, G. (2006). Description of the small plastics fragments in marine sediments along the Alang-Sosiya ship-breaking yard, India. Estuar. Coast. Shelf Sci. 68, 656–660. doi: 10.1016/j.ecss.2006.03.018
Reisser, J., Slat, B., Noble, K., Plessis, K., Epp, M., Proietti, M., et al. (2015). The vertical distribution of buoyant plastics at sea: an observational study in the North Atlantic Gyre. Biogeosciences 12, 1249–1256. doi: 10.5194/bg-12-1249-2015
Rippka, R., Deruelles, J., Waterbury, J. B., Herdman, M., and Stanier, R. Y. (1979). Generic assignments, strain histories and properties of pure cultures of cyanobacteria. Microbiology 111, 1–61. doi: 10.1099/00221287-111-1-1
Roche, D. B., Buenavista, M. T., and McGuffin, L. J. (2013). The FunFOLD2 server for the prediction of protein-ligand interactions. Nucleic Acids Res. 41, 303–307. doi: 10.1093/nar/gkt498
Rochman, C.M., Hentschel, B.T., and Teh, S.J. (2014). Long-term sorption of metals is similar among plastic types: implications for plastic debris in aquatic environments. PLoS ONE 9:e85433. doi: 10.1371/journal.pone.0085433
Romera-Castillo, C., Pinto, M., Langer, T.M., Álvarez-Salgado, X.A., and Herndl, G.J. (2018). Dissolved organic carbon leaching from plastics stimulates microbial activity in the ocean. Nat. Commun. 9:1430. doi: 10.1038/s41467-018-03798-5
Rosa, L. T., Dix, S. R., Rafferty, J. B., and Kelly, D. J. (2019). A New Mechanism for high-affinity uptake of C4-dicarboxylates in Bacteria revealed by the structure of Rhodopseudomonas palustris MatC (RPA3494), a periplasmic binding protein of the Tripartite Tricarboxylate Transporter (TTT) Family. J. Mol. Biol. 431, 351–367. doi: 10.1016/j.jmb.2018.11.016
Sarker, I., Moore, L. R., Paulsen, I. T., and Tetu, S. G. (2020). Assessing the toxicity of leachates from weathered plastics on photosynthetic marine bacteria prochlorococcus. Front. Mar. Sci. 7, 1–14. doi: 10.3389/fmars.2020.571929
Schwarzenbach, R. P., Gschwend, P. M., and Imboden, D. M. (2002). Environmental Organic Chemistry. Hoboken, NJ: John Wiley & Sons, Wiley-Interscience, 2003. doi: 10.1002/0471649643
Seeley, M. E., Song, B., Passie, R., and Hale, R. C. (2020). Microplastics affect sedimentary microbial communities and nitrogen cycling. Nat. Commun. 11, 1–10. doi: 10.1038/s41467-020-16235-3
Stal, L. J. (1988). Acetylene reduction technique for assay of nitrogenase. Methods Enzymol. 167, 474–484. doi: 10.1016/0076-6879(88)67052-2
Suaria, G., Avio, C. G., Mineo, A., Lattin, G. L., Magaldi, M. G., Belmonte, G., et al. (2016). The Mediterranean Plastic Soup: synthetic polymers in Mediterranean surface waters. Sci. Rep. 6:37551. doi: 10.1038/srep37551
Tetu, S.G., Sarker, I., Schrameyer, V., Pickford, R., Elbourne, L.D.H., Moore, L.R., et al. (2019). Plastic leachates impair growth and oxygen production in Prochlorococcus, the ocean's most abundant photosynthetic bacteria. Commun. Biol. 2:184. doi: 10.1038/s42003-019-0410-x
Tuson, H. H., and Weibel, D. B. (2013). Bacteria-surface interactions. Soft Matter 9, 4368–4380. doi: 10.1039/c3sm27705d
Urbanek, A. K., Rymowicz, W., and Mirończuk, A. M. (2018). Degradation of plastics and plastic-degrading bacteria in cold marine habitats. Appl. Microbiol. Biotechnol. 102, 7669–7678. doi: 10.1007/s00253-018-9195-y
Wang, J., Zhang, M. Y., Chen, T., Zhu, Y., Teng, Y., Luo, Y. M., et al. (2015). Isolation and identification of a di-(2-ethylhexyl) phthalate-degrading bacterium and its role in the bioremediation of a contaminated soil. Pedosphere 25, 202–211. doi: 10.1016/S1002-0160(15)60005-4
Wang, L., and Zheng, B. (2008). Toxic effects of fluoranthene and copper on marine diatom Phaeodactylum tricornutum. J. Environ. Sci. 20, 1363–1372. doi: 10.1016/S1001-0742(08)62234-2
Yang, Y., Liu, G., Song, W., Ye, C., Lin, H., Li, Z., et al. (2019). Plastics in the marine environment are reservoirs for antibiotic and metal resistance genes. Environ. Int. 123, 79–86. doi: 10.1016/j.envint.2018.11.061
Zehr, J. P., and Capone, D. G. (2020). Changing perspectives in marine nitrogen fixation. Science 368:aay9514. doi: 10.1126/science.aay9514
Zhang, Y. (2008). I-TASSER server for protein 3D structure prediction. BMC Bioinformatics 9:40. doi: 10.1186/1471-2105-9-40
Keywords: microplastics, organic additives, marine pollution, cyanobacteria and heterotrophic bacteria, N2-fixing bacteria
Citation: Fernández-Juárez V, López-Alforja X, Frank-Comas A, Echeveste P, Bennasar-Figueras A, Ramis-Munar G, Gomila RM and Agawin NSR (2021) “The Good, the Bad and the Double-Sword” Effects of Microplastics and Their Organic Additives in Marine Bacteria. Front. Microbiol. 11:581118. doi: 10.3389/fmicb.2020.581118
Received: 07 July 2020; Accepted: 21 December 2020;
Published: 20 January 2021.
Edited by:
Ondrej Prasil, Academy of Sciences of the Czech Republic (ASCR), CzechiaReviewed by:
Edo Bar Zeev, Ben-Gurion University of the Negev, IsraelOlivier Pringault, Institut de Recherche Pour le Développement (IRD), France
Copyright © 2021 Fernández-Juárez, López-Alforja, Frank-Comas, Echeveste, Bennasar-Figueras, Ramis-Munar, Gomila and Agawin. This is an open-access article distributed under the terms of the Creative Commons Attribution License (CC BY). The use, distribution or reproduction in other forums is permitted, provided the original author(s) and the copyright owner(s) are credited and that the original publication in this journal is cited, in accordance with accepted academic practice. No use, distribution or reproduction is permitted which does not comply with these terms.
*Correspondence: Víctor Fernández-Juárez, dmljdG9yZmpAaG90bWFpbC5lcw==; Nona S. R. Agawin, bm9uYS5hZ2F3aW5AdWliLmVz