- Department of Biology, University of Copenhagen, Copenhagen, Denmark
The stringent response regulates bacterial growth rate and is important for cell survival under changing environmental conditions. The effect of the stringent response is pleiotropic, affecting almost all biological processes in the cell including transcriptional downregulation of genes involved in stable RNA synthesis, DNA replication, and metabolic pathways, as well as the upregulation of stress-related genes. In this Review, we discuss how the stringent response affects chromosome replication and DNA repair activities in bacteria. Importantly, we address how accumulation of (p)ppGpp during the stringent response shuts down chromosome replication using highly different strategies in the evolutionary distant Gram-negative Escherichia coli and Gram-positive Bacillus subtilis. Interestingly, (p)ppGpp-mediated replication inhibition occurs downstream of the origin in B. subtilis, whereas replication inhibition in E. coli takes place at the initiation level, suggesting that stringent cell cycle arrest acts at different phases of the replication cycle between E. coli and B. subtilis. Furthermore, we address the role of (p)ppGpp in facilitating DNA repair activities and cell survival during exposure to UV and other DNA damaging agents. In particular, (p)ppGpp seems to stimulate the efficiency of nucleotide excision repair (NER)-dependent repair of DNA lesions. Finally, we discuss whether (p)ppGpp-mediated cell survival during DNA damage is related to the ability of (p)ppGpp accumulation to inhibit chromosome replication.
Introduction
Bacteria respond to a variety of changing environmental conditions by inducing the stringent response. Known inducers of the stringent response include nutrient limitations such as amino acids, fatty acids, carbon and nitrogen starvation, and other stresses such as high temperature and low pH (Gallant et al., 1977; Gentry and Cashel, 1996; Wells and Gaynor, 2006; Winther et al., 2018; Sinha et al., 2019; Schafer et al., 2020). The hallmark of stringent response is the accumulation of guanosine tetra- or pentaphosphate, ppGpp and pppGpp, respectively [collectively called (p)ppGpp or alarmone], which leads to reprogramming of cell physiology facilitating cell survival under stress (Potrykus and Cashel, 2008; Hauryliuk et al., 2015). Importantly, (p)ppGpp plays a role in antibiotic tolerance and is essential for virulence in pathogenic bacteria (Dalebroux et al., 2010; Hauryliuk et al., 2015). Additionally, (p)ppGpp regulates bacterial growth rates even in the absence of external environmental stress (Potrykus et al., 2011).
Alarmones are synthesized and hydrolyzed by the long RelA/SpoT Homolog (RSH) protein superfamily. In the Gram-negative γ–proteobacterium Escherichia coli, two paralogous enzymes modulate (p)ppGpp levels; monofunctional RelA, which has only synthetase activity, and bifunctional SpoT, which has both synthetase and hydrolase activities. In the spore-forming Gram-positive bacterium, Bacillus subtilis, (p)ppGpp levels are metabolized by one long RSH superfamily protein Rel and two small alarmone synthetases (SASs) called RelP and RelQ (Liu et al., 2015; reviewed in Ronneau and Hallez, 2019). Accumulation of (p)ppGpp rapidly alters the levels of a wide range of gene transcripts and metabolites to allow cell survival and adaptation to new growth conditions (Eymann et al., 2002; Traxler et al., 2008). The major changes involve transcriptional down-regulation of genes involved in stable RNA (rRNA and tRNA) synthesis, DNA replication, and metabolic pathways, whereas genes engaged in stress and amino-acid biosynthesis are activated (Sanchez-Vazquez et al., 2019; Gummesson et al., 2020). In E. coli, (p)ppGpp directly binds two sites on RNA polymerase (RNAP) to allosterically alter its binding to- and efficiency at different gene promoters, which results in genome-wide transcriptional reprogramming. (p)ppGpp binding to RNAP and the consequent RNAP-driven transcriptional response is potentiated by another small RNAP binding protein, DksA (reviewed in Gourse et al., 2018). In B. subtilis, RNAP lacks critical (p)ppGpp binding sites and no DskA homologs have been identified. As a consequence, (p)ppGpp does not directly target B. subtilis RNAP. Instead (p)ppGpp synthesis strongly depletes the pool of available GTP, which leads to an indirect inhibition of stable RNA promoter activity since GTP is used as start nucleotide for most of the stable RNAs (Krasny and Gourse, 2004; Gourse et al., 2018; Sanchez-Vazquez et al., 2019). Importantly, apart from transcriptional responses, (p)ppGpp directly targets many other proteins to affect metabolic processes such as nucleotide metabolism and biosynthetic pathways (Zhang et al., 2018, 2019; Wang et al., 2019).
Here, we discuss how the stringent response affects chromosome replication, DNA damage and repair activities, focusing mainly on recent studies done in the evolutionarily distant E. coli and B. subtilis.
Role of the Stringent Response in Chromosome Replication
In E. coli, chromosome replication initiates at a single origin of replication, oriC, which contains an AT-rich region and multiple binding-sites for the initiator protein, DnaA (Leonard and Mechali, 2013). DnaA belongs to the family of AAA + proteins and binds ATP and ADP with similar affinity (Sekimizu et al., 1987), of which only the ATP-bound form, DnaAATP, is required for oligomerization at oriC, and hence active for initiation (reviewed in Skarstad and Katayama, 2013; Riber et al., 2016). Origin unwinding leads to loading of DNA helicase, DnaB, onto single-stranded DNA (ssDNA) by the helicase loader, DnaC, followed by recruitment of primase, DnaG, as well as assembly of two replisomes to direct replication bidirectionally, until the replication forks meet and terminate at the terminus region, opposite to oriC (Kornberg and Baker, 1992). In B. subtilis, chromosome replication is mediated by the same overall steps, but the bipartite replication origin, containing two DnaA-box clusters separated by the dnaA gene (Moriya et al., 1992), is structurally different as compared to the continuous replication origin of E. coli. Also, assembly of the helicase, DnaC, onto ssDNA by the helicase loader, DnaI, occurs via a different mechanism known as “ring assembly” (Soultanas, 2012), but the following recruitment of DnaG primase and assembly of the replication elongation machinery is largely similar to that of E. coli (reviewed by Jameson and Wilkinson, 2017).
Highly different strategies have been adopted for (p)ppGpp-mediated chromosome replication inhibition in E. coli and B. subtilis. It is widely accepted that replication arrest in B. subtilis occurs downstream from the origin (i.e., on the elongation level), whereas replication inhibition in E. coli occurs at the initiation level, suggesting that stringent cell cycle arrest points differ between E. coli and B. subtilis (Levine et al., 1991).
(p)ppGpp-Mediated Inhibition of Initiation of Chromosome Replication
High levels of (p)ppGpp inhibit chromosome replication initiation in E. coli (Levine et al., 1991; Schreiber et al., 1995; Ferullo and Lovett, 2008; Riber and Lobner-Olesen, 2020), but the exact mechanism responsible for this inhibition has been somewhat unclear. However, several recent papers have made crucial discoveries adding valuable insight into this area of research.
Previously, the transcriptional activity of both dnaA operon promoters was reported to be stringently controlled (Chiaramello and Zyskind, 1990; Zyskind and Smith, 1992), suggesting that reduced dnaA gene transcription, and hence lowered de novo DnaA protein synthesis, could explain the initiation arrest observed in the presence of elevated (p)ppGpp levels. This was supported by a recent study, reporting that continued DnaA synthesis, expressed from a (p)ppGpp-insensitive T7 RNAP-dependent promoter, allowed for replication initiation during (p)ppGpp accumulation (Riber and Lobner-Olesen, 2020). Additionally, it was reported that polyphosphate during the stringent response activates Lon protease to degrade DnaAADP. As several regulatory systems work in concert to convert DnaAATP into DnaAADP (Katayama et al., 1998; Kato and Katayama, 2001; Kasho and Katayama, 2013), this indirectly lowers the amount of active DnaAATP, causing replication initiation to cease (Gross and Konieczny, 2020). However, degradation of DnaA has been reported only for Caulobacter crescentus, and not for E. coli (Gorbatyuk and Marczynski, 2005; Katayama et al., 2010). Also, recent data give no indication of DnaA degradation during (p)ppGpp accumulation (Riber and Lobner-Olesen, 2020).
Interestingly, several studies address the importance of DnaA activity, i.e., the DnaAATP-to-DnaAADP ratio, during (p)ppGpp accumulation. Continuous de novo DnaA synthesis was found to allow for new rounds of replication initiation during (p)ppGpp accumulation (Riber and Lobner-Olesen, 2020). As the level of ATP is more abundant than ADP in the cell (Petersen and Møller, 2000), and because DnaA binds these nucleotides with similar affinity (Sekimizu et al., 1987), de novo synthesized DnaA will be mainly ATP-bound, which ensures that the pool of DnaAATP is continuously being replenished. Thus, while overall cell growth ceases due to (p)ppGpp accumulation DnaAATP continues to increase due to de novo synthesis, which in turn allows for continued replication initiation during high levels of (p)ppGpp. In contrast, overproduction of DnaA during otherwise normal cell growth does not notably increase the DnaAATP level (Flatten et al., 2015). Following induction of (p)ppGpp in such cells, transcription of dnaA will be repressed, which results in insufficient accumulation of active DnaAATP to sustain further initiations (Kraemer et al., 2019).
Altogether, these observations suggest that (p)ppGpp-mediated replication initiation inhibition occurs through prevention of de novo DnaA synthesis, which lowers both the amount and activity (i.e., ATP-bound status) of DnaA. In agreement with this, (p)ppGpp fails to arrest replication initiation in cells where a hyperactive DnaA protein, mimicking ATP-bound DnaA, is overproduced (Kraemer et al., 2019).
Limitation of DnaA does, however, not seem to be the sole mechanism responsible of (p)ppGpp-mediated replication initiation inhibition. Recent studies emphasize lack of transcriptional activation of oriC to explain the negative effect of (p)ppGpp on initiation. Here, (p)ppGpp-driven reduction in transcriptional activity of promoters located close to oriC, presumably preventing introduction of negative supercoils in the wake of the migrating RNA polymerase complex, was suggested to cause less transcriptional activation of the origin, hence inhibiting initiation (Kraemer et al., 2019). Also, DNA gyrase (gyrA) and topoisomerase IV (parC) expression was found to be inhibited by high levels of (p)ppGpp, and the negative superhelicity of oriC was suggested to be lowered, despite not actually being measured (Fernandez-Coll et al., 2020).
Both mioC and gidA promoters, located adjacent to oriC, can be deleted without measurable effects (Lobner-Olesen and Boye, 1992; Bates et al., 1997; Lies et al., 2015), showing that they are dispensable for replication initiation during normal growth. However, when oriC becomes sufficiently impaired for initiation, such as when DnaA box R4 is deleted, transcription from these promoters becomes important (Bates et al., 1997). This is supported by the initiation kinetics of rifampicin and chloramphenicol. As rifampicin inhibits transcription initiation (Hartmann et al., 1967) rifampicin-treated cells will gradually stop to accumulate DnaA, but translation will continue as long as intact dnaA mRNA is present. On the other hand, chloramphenicol treatment will immediately block DnaA translation (Vazquez, 1979). Yet, chloramphenicol did not inhibit initiation as fast as rifampicin (Lark, 1972; Messer, 1972; Riber and Lobner-Olesen, 2020). As transcription is still on-going in chloramphenicol treated cells, this supports the ability of transcriptional activation of oriC to allow for extra initiations during suboptimal, e.g., DnaA limiting, conditions.
In conclusion, failure to de novo synthesize DnaA (i.e., reduced dnaA transcription) and to replenish the DnaAATP pool along with lowered transcriptional activation of oriC (i.e., reduced gidA/mioC and/or gyrA/parC transcription) contribute in arresting replication initiation during (p)ppGpp accumulation in E. coli (Figure 1A; left). However, it is difficult to quantitate the exact contribution from each of those mechanisms.
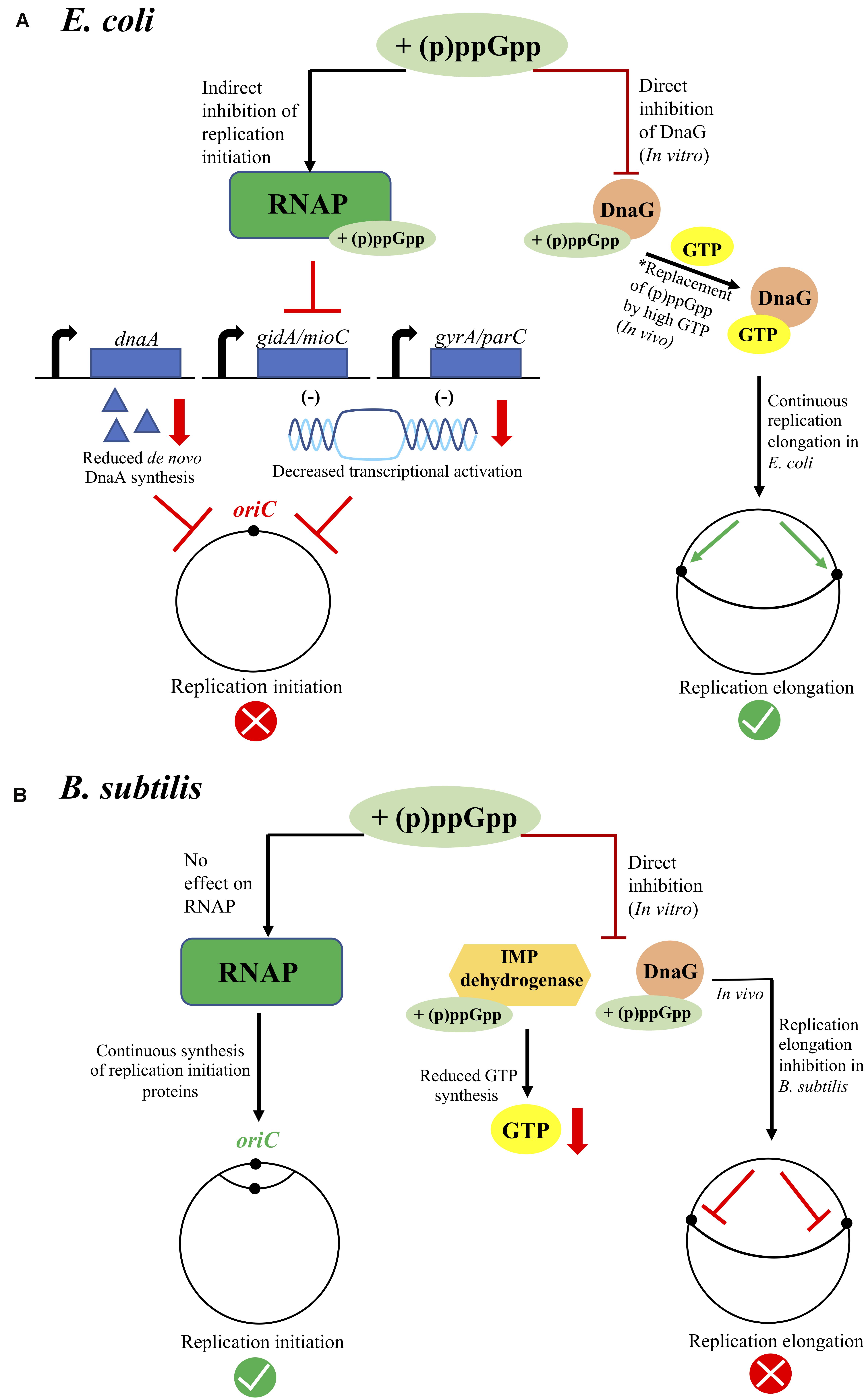
Figure 1. Overview of (p)ppGpp-meditated inhibition of chromosome replication in E. coli (A) and B. subtilis (B). In E. coli (A) replication inhibition occurs at the initiation level during (p)ppGpp accumulation. Here, (p)ppGpp binds the RNA Polymerase (RNAP), which indirectly affects the global gene expression profile through RNAP-driven transcriptional reprogramming. Downregulated gene transcripts include dnaA, gidA, mioC, gyrA, and parC, leading to lack of de novo DnaA synthesis and possibly lowered transcriptional activation of oriC, which all together contribute in arresting replication initiation during (p)ppGpp accumulation. Also, (p)ppGpp binds DnaG primase in vitro, but replication elongation remains unaffected in vivo. As GTP levels are not significantly reduced in E. coli during (p)ppGpp accumulation, and since GTP also binds DnaG, we hypothesize that GTP might outcompete (p)ppGpp in binding DnaG in vivo (this hypothesis is marked as *). In B. subtilis (B) replication inhibition occurs at the elongation level during (p)ppGpp accumulation. Here, (p)ppGpp binds IMP dehydrogenase, lowering the pool of available GTP, as well as DnaG. The significantly reduced level of GTP leads to DnaG being susceptible to strongly binding (p)ppGpp in vivo. Substantial replication occurs at the B. subtilis origin during (p)ppGpp accumulation, possibly because (p)ppGpp does not directly bind RNAP, excluding any RNAP-driven transcriptional reprogramming, or any replication initiation proteins.
(p)ppGpp-Mediated Inhibition of Elongation of Chromosome Replication
In contrast to E. coli, substantial replication occurs at the B. subtilis origin following induction of the stringent response. Also, regulation of chromosome replication initiation was shown to be independent of (p)ppGpp accumulation in B. subtilis (Levine et al., 1991; Murray and Koh, 2014). This indicates that (p)ppGpp might not regulate the synthesis of replication initiation proteins and/or transcriptional activation of oriC in B. subtilis. The lack of RNAP-driven transcriptional reprogramming due to B. subtilis RNAP not being a direct target of (p)ppGpp partly supports the latter (Figure 1B; left). Replication was instead shown to be arrested at distinct termination sites located approximately 200 kb downstream on either side of oriC (Levine et al., 1991), suggesting (p)ppGpp-mediated inhibition of chromosome replication in B. subtilis to be regulated at the post-initiation level.
By using genomic microarrays to monitor the progression of replication forks in synchronized cell cultures of B. subtilis, it was later revealed that starvation-induced replication arrest occurred throughout the chromosome, irrespective of the location of the replication forks. A direct (p)ppGpp-mediated inhibition of DNA primase (DnaG) activity, known to affect replication fork progression (Wu et al., 1992; Lee et al., 2006), was proposed to underlie the observed replication elongation arrest (Wang et al., 2007). This inhibition was found to be dose-dependent, suggesting that the severity of stress (i.e., concentration of (p)ppGpp) is tightly coupled to an equivalent reduction in replication progression rate, thus providing a tunable stress response (Wang et al., 2007; Denapoli et al., 2013). Interestingly, replication forks arrested in the presence of high levels of (p)ppGpp did not recruit the SOS response protein RecA, indicating that stalled forks were not disrupted, but reversibly halted with the ability to restart replication upon nutrient availability (Wang et al., 2007). These observations support that (p)ppGpp-mediated primase inhibition serves to maintain genome integrity during periods of stress.
Another factor that might contribute to the strong (p)ppGpp inhibition of progressing replication forks in B. subtilis is the equivalent decrease in the cellular pool of GTP available for continued DNA strand extension. This decrease is caused by increased consumption of GTP during (p)ppGpp biosynthesis, and by a direct inhibition of the activity of inosine monophosphate (IMP) dehydrogenase that catalyzes an early step in GTP biosynthesis (Lopez et al., 1981; Figure 1B; right).
(p)ppGpp binds and inhibits the E. coli DnaG primase in vitro (Maciag et al., 2010; Rymer et al., 2012). To date, no other replication proteins in E. coli, including DnaA, have been reported as direct targets for (p)ppGpp (Zhang et al., 2018; Wang et al., 2019). Obviously, this finding contradicts decades of research stating that ongoing rounds of replication are continued until completion following induction of the stringent response in E. coli, proposing that DNA replication elongation is not arrested during (p)ppGpp accumulation in vivo (Schreiber et al., 1995; Ferullo and Lovett, 2008; Kraemer et al., 2019; Riber and Lobner-Olesen, 2020). DeNapoli et al. did quantify genome-wide replication fork progression in E. coli and revealed that the replication elongation rate was modestly reduced by (p)ppGpp induction, but possibly the response was restricted to acute stress conditions (Denapoli et al., 2013).
Factors preventing binding of (p)ppGpp to DnaG, or the competing action between RNAP and DnaG in binding (p)ppGpp, were suggested to explain the lack of effect on DnaG activity in vivo (Maciag et al., 2010). Indeed, (p)ppGpp was found to bind DnaG at partially overlapping sites with nucleotides and inhibit primase activity in a GTP-concentration dependent manner (Rymer et al., 2012). As GTP levels are not reduced by more than 50% in E. coli during the stringent response (Varik et al., 2017), whereas B. subtilis experiences a significant drop in GTP concomitant with (p)ppGpp accumulation (Ochi et al., 1982), this supports a stronger (p)ppGpp-mediated binding to- and inhibition of DnaG in B. subtilis, hence leading to a more potent inhibition of replication elongation as compared to E. coli (Figures 1A,B; right).
Role of the Stringent Response in DNA Damage and Repair
Bacterial genomic integrity is often threatened by DNA damage induced either by natural fork breakage, fork stalling, replication-transcription collision, or by external threats such as radiation and DNA modifying drugs (Kuzminov, 1999). Faithful damage repair orchestrated by DNA repair proteins is essential to maintain genomic integrity, chromosomal replication and cell viability. Accordingly, mutants lacking repair proteins are sensitive to DNA damaging agents and are less viable (Van Houten, 1990; Kuzminov, 1999; Sinha et al., 2020). Since (p)ppGpp binding to RNAP in E. coli destabilizes the open promoter complexes, it is expected to modulate replication-transcription collision and to play a role in maintaining genomic integrity.
The observation that loss of both RelA and SpoT (ppGpp0 strain), i.e., inability to synthesize (p)ppGpp, enhanced UV sensitivity of an E. coli ruvAB mutant, suggested a possible role of (p)ppGpp in facilitating DNA repair (McGlynn and Lloyd, 2000). RuvAB along with RuvC play a role in branch migration and resolution of Holliday junctions, formed during RecBCD-RecA-mediated DNA double-strand break (DSB) repair and RecFOR-RecA-mediated gap repair (Kuzminov, 1999; Sinha et al., 2020). Interestingly, a slight increase in the basal level of (p)ppGpp by using the spoT1 allele, having reduced (p)ppGpp hydrolytic activity, improved UV survival of the ruvAB mutant (McGlynn and Lloyd, 2000). Thus, high (p)ppGpp increases/promotes viability, whereas no (p)ppGpp increases UV sensitivity of the ruvAB mutant. The ppGpp0 strain alone was also found to be UV sensitive (McGlynn and Lloyd, 2000).
The ppGpp0 strain displays an amino acid auxotrophy phenotype and accumulates suppressor mutations (known as “stringent mutants”) that allow cells to grow in minimal medium lacking amino acids. These suppressor mutations occur in RNAP subunits encoded by rpoB and rpoC (Zhou and Jin, 1998; McGlynn and Lloyd, 2000), and were shown to destabilize the transcriptional complex in a manner similar to (p)ppGpp binding to RNAP (Trautinger et al., 2005). Remarkably, some of these suppressor mutations (denoted rpo∗) significantly improved survival of the ΔrelA ΔspoT ΔruvAB strain after UV treatment (McGlynn and Lloyd, 2000).
Thus, it was proposed that (p)ppGpp/rpo∗-mediated destabilization of transcriptional complexes reduces the occurrence of stalled RNAP on DNA, hence allowing free space for efficient excision repair of UV-induced DNA lesions and for simultaneous facilitation of replication fork progression by avoiding replication-transcription conflicts (McGlynn and Lloyd, 2000; Trautinger and Lloyd, 2002; Trautinger et al., 2005). Additionally, it was shown that (p)ppGpp-mediated suppression of ruvAB mutant UV sensitivity is complex and requires RecA, RecG, and PriA, but not RecBCD, and was proposed to involve replication fork stalling, regression and restart (McGlynn and Lloyd, 2000). Since replication fork stalling, regression and restart are the major reactions following UV irradiation in E. coli cells (Khan and Kuzminov, 2012), the most plausible explanation for the UV resistance phenotype of spoT1 ruvAB (or rpo∗ ruvAB) cells would be destabilization of the RNAP array allowing replication forks to directly encounter DNA lesions followed by an active fork regression and lesion bypass, instead of fork breakage, to facilitate replication restart (Trautinger et al., 2005; Figure 2).
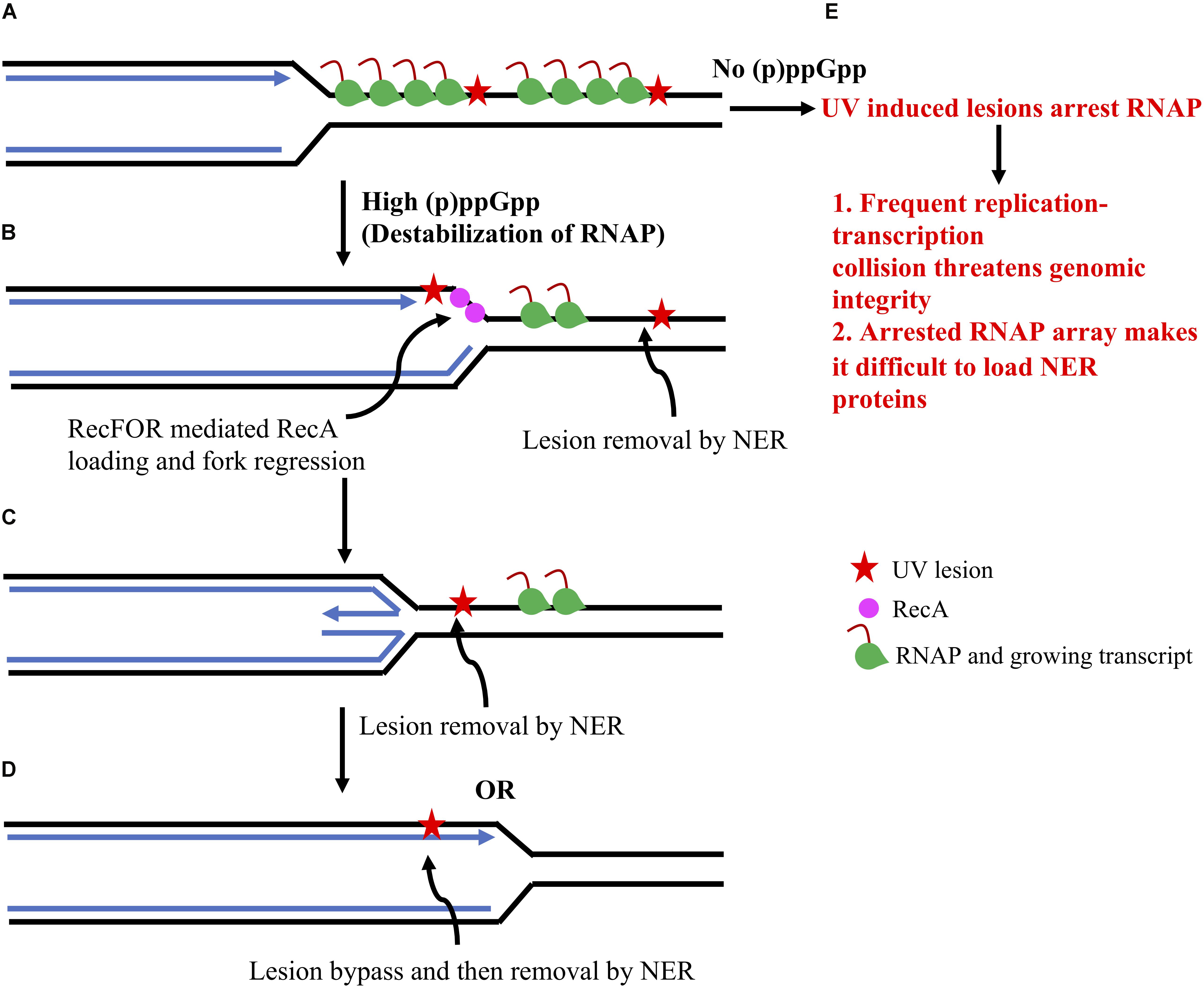
Figure 2. Overview of (p)ppGpp-meditated repair of UV induced DNA damage in E. coli. The UV induced DNA lesions arrest RNAP and halt transcription progression (A). This can lead to frequent replication-transcription collision. In (A), only co-directional collision has been shown but there is an equal possibility for head-on collision and both threaten genomic integrity. This scenario will probably be escalated in absence of (p)ppGpp since the RNAP array will be stably arrested for a long time in absence of (p)ppGpp (A–E). Whereas, (p)ppGpp binding to RNAP will destabilize it and remove it from the DNA template. Removal of RNAP will help in two ways: 1. It will create space to load NER proteins and remove/repair DNA lesions. 2. It will help the replication fork to progress toward DNA lesions (B). Arrested replication forks can get reversed with the help of RecFOR mediated RecA loading and fork regression (C). DNA synthesis and resetting of the replication fork will help in lesion bypass (D). DNA lesions can be removed and repaired by NER pathways either at the (C,D) step. This model is adapted from Trautinger et al. (2005).
In contrast to UV, high (p)ppGpp (or rpo∗) cannot suppress sensitivity of the ΔruvAB strain against exposure to mitomycin C (MMC) or γ rays (McGlynn and Lloyd, 2000). It should be noted that DNA lesions generated by both UV and MMC are removed/repaired by nucleotide excision repair (NER) (Van Houten, 1990). However, MMC treatment generates inter-strand crosslinks that most often get converted into DSBs, whereas UV treatment induces intra-strand pyrimidine dimers with generation of DSBs being primarily dependent on replication fork stalling at the lesion site (Khan and Kuzminov, 2012). These observations exclude a direct role of (p)ppGpp in DSBs repair.
Transcription-Coupled DNA Repair (TCR)
Another study, corroborating the above finding, confirmed that E. coli ppGpp0 cells were highly sensitive to UV radiation, 4-nitroquinoline-1-oxide (4NQO), and nitrofurazone (NFZ) (Kamarthapu et al., 2016). These agents induce formation of DNA adducts, which are mainly removed and repaired by NER pathways (Ikenaga et al., 1975; Ona et al., 2009). Remarkably, wild-type cells rapidly accumulated a 20-fold increase in (p)ppGpp when treated with 4NQO or NFZ, suggesting that DNA lesions induce (p)ppGpp synthesis. However, the mechanism of (p)ppGpp synthesis during these treatments remains to be determined (Kamarthapu et al., 2016).
TCR is defined by an active transcription-dependent increase in excision repair of lesions on the transcribed DNA strand in comparison to the non-transcribed strand (Mellon and Hanawalt, 1989). Two factors, Mfd and UvrD, promote TCR by two different pathways: by pushing RNAP forward of the DNA lesion and by promoting RNAP backtracking, respectively, followed by recruitment of NER proteins, such as UvrAB at the lesion site (Mellon and Hanawalt, 1989; Kamarthapu and Nudler, 2015). Interestingly, the preference for repairing the transcribed strand rather than the non-transcribed strand was abolished in ppGpp0 cells suggesting that (p)ppGpp is crucial for TCR. Since the sensitivity of ppGpp0 cells to UV, 4NQO or NFZ was epistatic to uvrD mutant sensitivity, it was proposed that (p)ppGpp potentiates the pro-backtracking activity of UvrD (Kamarthapu et al., 2016). The role of (p)ppGpp in facilitating TCR can also occur independent of UvrD either by promoting RNAP backtracking by destabilizing and removing RNAP complexes from tightly packed arrays at the highly transcribed ribosomal genes, thus creating space for backtracking, or by reducing the number of ribosomes trailing RNAP to make space for backtracking (Rasouly et al., 2017). However, extensive backtracked RNAP might increase the risk of replication-transcription collision and has the capacity to induce DSBs and genomic instability (Dutta et al., 2011). The conundrum is perhaps resolved by (p)ppGpp-mediated inhibition of replication initiation, thus minimizing the frequency of replication-transcription collisions when RNAP backtracking is needed to repair genotoxic lesions on DNA.
In B. subtilis, the SMC-ScpAB complex is important for chromosome condensation and segregation, and Δsmc mutants exhibit pleiotropic phenotypes including defects in chromosome condensation, segregation, DNA repair and viability at high temperature. Upregulation of the stringent response has been shown to suppress chromosome segregation defects, hypersensitivity to gyrase inhibitors and restore viability of Δsmc mutants (Benoist et al., 2015). Since the stringent response slows down replication elongation in B. subtilis, it might be possible that slow replication allows chromosome segregation to occur even in the absence of the SMC-ScpAB complex. This hypothesis finds support as Δsmc mutant cells grow well in minimal medium (i.e., slow growth conditions) as compared to no growth in rich medium (i.e., fast growth conditions) at 37°C (Benoist et al., 2015). Similar studies for the role of the stringent response in chromosome segregation mutant cells of E. coli have not been reported.
Concluding Remarks
Based on the highlights presented throughout this review, the stringent response has clearly proven to affect both bacterial chromosome replication and DNA repair activities. However, whereas (p)ppGpp accumulation negatively affects replication initiation and replication elongation in E. coli and B. subtilis, respectively, the effect of (p)ppGpp-mediated modulation of DNA repair activities seems positive. Indeed, the absence of (p)ppGpp makes E. coli cells sensitive to UV and other DNA damaging agents, and studies suggest a role of (p)ppGpp in enhancing the efficiency of NER-dependent repair of DNA lesions, most likely by destabilizing RNAP complexes and making space for recruitment of NER proteins. Interestingly, these observations might be coupled to (p)ppGpp-mediated replication inhibition, which prevents replication-transcription collisions and/or reduces the frequency of replication forks meeting the UV lesions, thus assisting efficient NER-mediated repair. This intriguing hypothesis, connecting the negative effect of (p)ppGpp on replication to (p)ppGpp-driven stimulation of DNA repair activity, can easily be tested by using a system where (p)ppGpp-dependent replication inhibition is abrogated as recently described (Riber and Lobner-Olesen, 2020).
Author Contributions
AS, AL-O, and LR wrote the manuscript. AS and LR designed and prepared the figures. All authors contributed to the article and approved the submitted version.
Funding
For this research, AS was funded by the Center for Bacterial Stress Response and Persistence (BASP) supported by grants from the Novo Nordisk Foundation and the Danish National Research Foundation (DNRF120). AL-O was funded by grants from the Danish National Research Foundation (DNRF120) and from the Novo Nordisk Foundation through the Challenge Center for Peptide-Based Antibiotics NNF16OC0021700 (Cepan). LR was funded by a Lundbeck Foundation Experiment 2019 Grant (R324-2019-2001) from the Lundbeck Foundation.
Conflict of Interest
The authors declare that the research was conducted in the absence of any commercial or financial relationships that could be construed as a potential conflict of interest.
References
Bates, D. B., Boye, E., Asai, T., and Kogoma, T. (1997). The absence of effect of gid or mioC transcription on the initiation of chromosomal replication in Escherichia coli. Proc. Natl. Acad. Sci. U.S.A. 94, 12497–12502. doi: 10.1073/pnas.94.23.12497
Benoist, C., Guérin, C., Noirot, P., and Dervyn, E. (2015). Constitutive stringent response restores viability of Bacillus subtilis lacking structural maintenance of chromosome protein. PLoS One 10:e0142308. doi: 10.1371/journal.pone.0142308
Chiaramello, A. E., and Zyskind, J. W. (1990). Coupling of DNA replication to growth rate in Escherichia coli: a possible role for guanosine tetraphosphate. J. Bacteriol. 172, 2013–2019. doi: 10.1128/jb.172.4.2013-2019.1990
Dalebroux, Z. D., Svensson, S. L., Gaynor, E. C., and Swanson, M. S. (2010). ppGpp conjures bacterial virulence. Microbiol. Mol. Biol. Rev. 74, 171–199. doi: 10.1128/mmbr.00046-09
Denapoli, J., Tehranchi, A. K., and Wang, J. D. (2013). Dose-dependent reduction of replication elongation rate by (p)ppGpp in Escherichia coli and Bacillus subtilis. Mol. Microbiol. 88, 93–104. doi: 10.1111/mmi.12172
Dutta, D., Shatalin, K., Epshtein, V., Gottesman, M. E., and Nudler, E. (2011). Linking RNA polymerase backtracking to genome instability in E. coli. Cell 146, 533–543. doi: 10.1016/j.cell.2011.07.034
Eymann, C., Homuth, G., Scharf, C., and Hecker, M. (2002). Bacillus subtilis functional genomics: global characterization of the stringent response by proteome and transcriptome analysis. J. Bacteriol. 184, 2500–2520. doi: 10.1128/jb.184.9.2500-2520.2002
Fernandez-Coll, L., Maciag-Dorszynska, M., Tailor, K., Vadia, S., Levin, P. A., Szalewska-Palasz, A., et al. (2020). The Absence of (p)ppGpp Renders Initiation of Escherichia coli Chromosomal DNA Synthesis Independent of Growth Rates. mBio 11:e03223-19.
Ferullo, D. J., and Lovett, S. T. (2008). The stringent response and cell cycle arrest in Escherichia coli. PLoS. Genet. 4:e1000300. doi: 10.1371/journal.pgen.1000300
Flatten, I., Fossum-Raunehaug, S., Taipale, R., Martinsen, S., and Skarstad, K. (2015). The DnaA Protein Is Not the Limiting Factor for Initiation of Replication in Escherichia coli. PLoS.Genet. 11:e1005276. doi: 10.1371/journal.pgen.1005276
Gallant, J., Palmer, L., and Pao, C. C. (1977). Anomalous synthesis of ppGpp in growing cells. Cell 11, 181–185. doi: 10.1016/0092-8674(77)90329-4
Gentry, D. R., and Cashel, M. (1996). Mutational analysis of the Escherichia coli spoT gene identifies distinct but overlapping regions involved in ppGpp synthesis and degradation. Mol. Microbiol. 19, 1373–1384. doi: 10.1111/j.1365-2958.1996.tb02480.x
Gorbatyuk, B., and Marczynski, G. T. (2005). Regulated degradation of chromosome replication proteins DnaA and CtrA in Caulobacter crescentus. Mol. Microbiol. 55, 1233–1245. doi: 10.1111/j.1365-2958.2004.04459.x
Gourse, R. L., Chen, A. Y., Gopalkrishnan, S., Sanchez-Vazquez, P., Myers, A., and Ross, W. (2018). Transcriptional Responses to ppGpp and DksA. Annu. Rev. Microbiol. 72, 163–184. doi: 10.1146/annurev-micro-090817-062444
Gross, M. H., and Konieczny, I. (2020). Polyphosphate induces the proteolysis of ADP-bound fraction of initiator to inhibit DNA replication initiation upon stress in Escherichia coli. Nucleic Acids Res. 48, 5457–5466. doi: 10.1093/nar/gkaa217
Gummesson, B., Shah, S. A., Borum, A. S., Fessler, M., Mitarai, N., Sorensen, M. A., et al. (2020). Valine-induced isoleucine starvation in Escherichia coli K-12 studied by spike-in normalized RNA sequencing. Front. Genet. 11:144. doi: 10.3389/fgene.2020.00144
Hartmann, G., Honikel, K. O., Knusel, F., and Nuesch, J. (1967). The specific inhibition of the DNA-directed RNA synthesis by rifamycin. Biochim. Biophys. Acta 145, 843–844. doi: 10.1016/0005-2787(67)90147-5
Hauryliuk, V., Atkinson, G. C., Murakami, K. S., Tenson, T., and Gerdes, K. (2015). Recent functional insights into the role of (p)ppGpp in bacterial physiology. Nat. Rev. Microbiol. 13, 298–309. doi: 10.1038/nrmicro3448
Ikenaga, M., Ishii, Y., Tada, M., Kakunaga, T., and Takebe, H. (1975). Excision-repair of 4-nitroquinolin-1-oxide damage responsible for killing, mutation, and cancer. Basic Life Sci. 5b, 763–771. doi: 10.1007/978-1-4684-2898-8_54
Jameson, K. H., and Wilkinson, A. J. (2017). Control of Initiation of DNA Replication in Bacillus subtilis and Escherichia coli. Genes 8:22. doi: 10.3390/genes8010022
Kamarthapu, V., Epshtein, V., Benjamin, B., Proshkin, S., Mironov, A., Cashel, M., et al. (2016). ppGpp couples transcription to DNA repair in E. coli. Science 352, 993–996. doi: 10.1126/science.aad6945
Kamarthapu, V., and Nudler, E. (2015). Rethinking transcription coupled DNA repair. Curr. Opin. Microbiol. 24, 15–20. doi: 10.1016/j.mib.2014.12.005
Kasho, K., and Katayama, T. (2013). DnaA binding locus datA promotes DnaA-ATP hydrolysis to enable cell cycle-coordinated replication initiation. Proc. Natl. Acad. Sci. U.S.A. 110, 936–941. doi: 10.1073/pnas.1212070110
Katayama, T., Kubota, T., Kurokawa, K., Crooke, E., and Sekimizu, K. (1998). The initiator function of DnaA protein is negatively regulated by the sliding clamp of the E. coli chromosomal replicase. Cell 94, 61–71. doi: 10.1016/s0092-8674(00)81222-2
Katayama, T., Ozaki, S., Keyamura, K., and Fujimitsu, K. (2010). Regulation of the replication cycle: conserved and diverse regulatory systems for DnaA and oriC. Nat. Rev. Microbiol. 8, 163–170. doi: 10.1038/nrmicro2314
Kato, J., and Katayama, T. (2001). Hda, a novel DnaA-related protein, regulates the replication cycle in Escherichia coli. EMBO J. 20, 4253–4262. doi: 10.1093/emboj/20.15.4253
Khan, S. R., and Kuzminov, A. (2012). Replication forks stalled at ultraviolet lesions are rescued via RecA and RuvABC protein-catalyzed disintegration in Escherichia coli. J. Biol. Chem. 287, 6250–6265. doi: 10.1074/jbc.m111.322990
Kraemer, J. A., Sanderlin, A. G., and Laub, M. T. (2019). The stringent response inhibits DNA replication initiation in E. coli by modulating supercoiling of oriC. mBio 10:e01330-19.
Krasny, L., and Gourse, R. L. (2004). An alternative strategy for bacterial ribosome synthesis: Bacillus subtilis rRNA transcription regulation. EMBO J. 23, 4473–4483. doi: 10.1038/sj.emboj.7600423
Kuzminov, A. (1999). Recombinational repair of DNA damage in Escherichia coli and bacteriophage lambda. Microbiol. Mol. Biol. Rev. 63, 751–813. doi: 10.1128/mmbr.63.4.751-813.1999
Lark, K. G. (1972). Evidence for direct involvement of RNA in the initiation of DNA replication in E. coli 15T. J. Mol. Biol. 64, 47–60. doi: 10.1016/0022-2836(72)90320-8
Lee, J. B., Hite, R. K., Hamdan, S. M., Xie, X. S., Richardson, C. C., and Van Oijen, A. M. (2006). DNA primase acts as a molecular brake in DNA replication. Nature 439, 621–624. doi: 10.1038/nature04317
Leonard, A. C., and Mechali, M. (2013). DNA replication origins. Cold Spring Harb. Perspect. Biol. 5:a010116.
Levine, A., Vannier, F., Dehbi, M., Henckes, G., and Séror, S. J. (1991). The stringent response blocks DNA replication outside the ori region in Bacillus subtilis and at the origin in Escherichia coli. J. Mol. Biol. 219, 605–613. doi: 10.1016/0022-2836(91)90657-r
Lies, M., Visser, B. J., Joshi, M. C., Magnan, D., and Bates, D. (2015). MioC and GidA proteins promote cell division in E. coli. Front. Microbiol. 6:516. doi: 10.3389/fmicb.2015.00516
Liu, K., Bittner, A. N., and Wang, J. D. (2015). Diversity in (p)ppGpp metabolism and effectors. Curr. Opin. Microbiol. 24, 72–79. doi: 10.1016/j.mib.2015.01.012
Lobner-Olesen, A., and Boye, E. (1992). Different effects of mioC transcription on initiation of chromosomal and minichromosomal replication in Escherichia coli. Nucleic Acids Res. 20, 3029–3036. doi: 10.1093/nar/20.12.3029
Lopez, J. M., Dromerick, A., and Freese, E. (1981). Response of guanosine 5’-triphosphate concentration to nutritional changes and its significance for Bacillus subtilis sporulation. J. Bacteriol. 146, 605–613. doi: 10.1128/jb.146.2.605-613.1981
Maciag, M., Kochanowska, M., Lyzen, R., Wegrzyn, G., and Szalewska-Palasz, A. (2010). ppGpp inhibits the activity of Escherichia coli DnaG primase. Plasmid 63, 61–67. doi: 10.1016/j.plasmid.2009.11.002
McGlynn, P., and Lloyd, R. G. (2000). Modulation of RNA polymerase by (p)ppGpp reveals a RecG-dependent mechanism for replication fork progression. Cell 101, 35–45. doi: 10.1016/s0092-8674(00)80621-2
Mellon, I., and Hanawalt, P. C. (1989). Induction of the Escherichia coli lactose operon selectively increases repair of its transcribed DNA strand. Nature 342, 95–98. doi: 10.1038/342095a0
Messer, W. (1972). Initiation of deoxyribonucleic acid replication in Escherichia coli B-r: chronology of events and transcriptional control of initiation. J. Bacteriol. 112, 7–12. doi: 10.1128/jb.112.1.7-12.1972
Moriya, S., Atlung, T., Hansen, F. G., Yoshikawa, H., and Ogasawara, N. (1992). Cloning of an autonomously replicating sequence (ars) from the Bacillus subtilis chromosome. Mol. Microbiol. 6, 309–315. doi: 10.1111/j.1365-2958.1992.tb01473.x
Murray, H., and Koh, A. (2014). Multiple regulatory systems coordinate DNA replication with cell growth in Bacillus subtilis. PLoS Genet. 10:e1004731. doi: 10.1371/journal.pgen.1004731
Ochi, K., Kandala, J., and Freese, E. (1982). Evidence that Bacillus subtilis sporulation induced by the stringent response is caused by the decrease in GTP or GDP. J. Bacteriol. 151, 1062–1065. doi: 10.1128/jb.151.2.1062-1065.1982
Ona, K. R., Courcelle, C. T., and Courcelle, J. (2009). Nucleotide excision repair is a predominant mechanism for processing nitrofurazone-induced DNA damage in Escherichia coli. J. Bacteriol. 191, 4959–4965. doi: 10.1128/jb.00495-09
Petersen, C., and Møller, L. B. (2000). Invariance of the nucleoside triphosphate pools of Escherichia coli with growth rate. J. Biol. Chem. 275, 3931–3935. doi: 10.1074/jbc.275.6.3931
Potrykus, K., and Cashel, M. (2008). (p)ppGpp: still magical? Annu. Rev. Microbiol. 62, 35–51. doi: 10.1146/annurev.micro.62.081307.162903
Potrykus, K., Murphy, H., Philippe, N., and Cashel, M. (2011). ppGpp is the major source of growth rate control in E. coli. Environ. Microbiol. 13, 563–575. doi: 10.1111/j.1462-2920.2010.02357.x
Rasouly, A., Pani, B., and Nudler, E. (2017). A magic spot in genome maintenance. Trends Genet. 33, 58–67. doi: 10.1016/j.tig.2016.11.002
Riber, L., Frimodt-Moller, J., Charbon, G., and Lobner-Olesen, A. (2016). Multiple DNA Binding Proteins Contribute to Timing of Chromosome Replication in E. coli. Front. Mol. Biosci. 3:29. doi: 10.3389/fmolb.2016.00029
Riber, L., and Lobner-Olesen, A. (2020). Inhibition of Escherichia coli chromosome replication by rifampicin treatment or during the stringent response is overcome by de novo DnaA protein synthesis. Mol. Microbiol. doi: 10.1111/mmi.14531 [Epub ahead of print].
Ronneau, S., and Hallez, R. (2019). Make and break the alarmone: regulation of (p)ppGpp synthetase/hydrolase enzymes in bacteria. FEMS Microbiol. Rev. 43, 389–400. doi: 10.1093/femsre/fuz009
Rymer, R. U., Solorio, F. A., Tehranchi, A. K., Chu, C., Corn, J. E., Keck, J. L., et al. (2012). Binding mechanism of metalNTP substrates and stringent-response alarmones to bacterial DnaG-type primases. Structure 20, 1478–1489. doi: 10.1016/j.str.2012.05.017
Sanchez-Vazquez, P., Dewey, C. N., Kitten, N., Ross, W., and Gourse, R. L. (2019). Genome-wide effects on Escherichia coli transcription from ppGpp binding to its two sites on RNA polymerase. Proc. Natl. Acad. Sci. U.S.A. 116, 8310–8319. doi: 10.1073/pnas.1819682116
Schafer, H., Beckert, B., Frese, C. K., Steinchen, W., Nuss, A. M., Beckstette, M., et al. (2020). The alarmones (p)ppGpp are part of the heat shock response of Bacillus subtilis. PLoS Genet. 16:e1008275. doi: 10.1371/journal.pgen.1008275
Schreiber, G., Ron, E. Z., and Glaser, G. (1995). ppGpp-mediated regulation of DNA replication and cell division in Escherichia coli. Curr. Microbiol. 30, 27–32. doi: 10.1007/bf00294520
Sekimizu, K., Bramhill, D., and Kornberg, A. (1987). ATP activates dnaA protein in initiating replication of plasmids bearing the origin of the E. coli chromosome. Cell 50, 259–265. doi: 10.1016/0092-8674(87)90221-2
Sinha, A. K., Possoz, C., and Leach, D. R. F. (2020). The roles of bacterial DNA double-strand break repair proteins in chromosomal DNA replication. FEMS Microbiol. Rev. 44, 351–368. doi: 10.1093/femsre/fuaa009
Sinha, A. K., Winther, K. S., Roghanian, M., and Gerdes, K. (2019). Fatty acid starvation activates RelA by depleting lysine precursor pyruvate. Mol. Microbiol. 112, 1339–1349. doi: 10.1111/mmi.14366
Skarstad, K., and Katayama, T. (2013). Regulating DNA replication in bacteria. Cold Spring Harb. Perspect. Biol. 5:a012922. doi: 10.1101/cshperspect.a012922
Soultanas, P. (2012). Loading mechanisms of ring helicases at replication origins. Mol. Microbiol. 84, 6–16. doi: 10.1111/j.1365-2958.2012.08012.x
Trautinger, B. W., Jaktaji, R. P., Rusakova, E., and Lloyd, R. G. (2005). RNA polymerase modulators and DNA repair activities resolve conflicts between DNA replication and transcription. Mol. Cell 19, 247–258. doi: 10.1016/j.molcel.2005.06.004
Trautinger, B. W., and Lloyd, R. G. (2002). Modulation of DNA repair by mutations flanking the DNA channel through RNA polymerase. EMBO J. 21, 6944–6953. doi: 10.1093/emboj/cdf654
Traxler, M. F., Summers, S. M., Nguyen, H. T., Zacharia, V. M., Hightower, G. A., Smith, J. T., et al. (2008). The global, ppGpp-mediated stringent response to amino acid starvation in Escherichia coli. Mol. Microbiol. 68, 1128–1148. doi: 10.1111/j.1365-2958.2008.06229.x
Van Houten, B. (1990). Nucleotide excision repair in Escherichia coli. Microbiol. Rev. 54, 18–51. doi: 10.1128/mmbr.54.1.18-51.1990
Varik, V., Oliveira, S. R. A., Hauryliuk, V., and Tenson, T. (2017). HPLC-based quantification of bacterial housekeeping nucleotides and alarmone messengers ppGpp and pppGpp. Sci. Rep. 7:11022.
Vazquez, D. (1979). “Inhibitors of protein biosynthesis,” in Molecular Biology, Biochemistry and Biophysics, eds A. Kleinzeller, G. F. Springer, and H. G. W. Wittman (Berlin: Springer Verlag), 108–112.
Wang, B., Dai, P., Ding, D., Del Rosario, A., Grant, R. A., Pentelute, B. L., et al. (2019). Affinity-based capture and identification of protein effectors of the growth regulator ppGpp. Nat. Chem. Biol. 15, 141–150. doi: 10.1038/s41589-018-0183-4
Wang, J. D., Sanders, G. M., and Grossman, A. D. (2007). Nutritional control of elongation of DNA replication by (p)ppGpp. Cell 128, 865–875. doi: 10.1016/j.cell.2006.12.043
Wells, D. H., and Gaynor, E. C. (2006). Helicobacter pylori initiates the stringent response upon nutrient and pH downshift. J. Bacteriol. 188, 3726–3729. doi: 10.1128/jb.188.10.3726-3729.2006
Winther, K. S., Roghanian, M., and Gerdes, K. (2018). Activation of the Stringent Response by Loading of RelA-tRNA Complexes at the Ribosomal A-Site. Mol. Cell. 70:95-105.e104.
Wu, C. A., Zechner, E. L., Reems, J. A., Mchenry, C. S., and Marians, K. J. (1992). Coordinated leading- and lagging-strand synthesis at the Escherichia coli DNA replication fork. V. Primase action regulates the cycle of Okazaki fragment synthesis. J. Biol. Chem. 267, 4074–4083.
Zhang, Y., Zbornikova, E., Rejman, D., and Gerdes, K. (2018). Novel (p)ppGpp Binding and Metabolizing Proteins of Escherichia coli. mBio 9:e02188-17.
Zhang, Y. E., Baerentsen, R. L., Fuhrer, T., Sauer, U., Gerdes, K., and Brodersen, D. E. (2019). (p)ppGpp Regulates a Bacterial Nucleosidase by an Allosteric Two-Domain Switch. Mol. Cell 74, 1239-1249.e1234.
Zhou, Y. N., and Jin, D. J. (1998). The rpoB mutants destabilizing initiation complexes at stringently controlled promoters behave like “stringent”. RNA polymerases in Escherichia coli. Proc. Natl. Acad. Sci. U.S.A. 95, 2908–2913. doi: 10.1073/pnas.95.6.2908
Keywords: (p)ppGpp, DNA replication, DNA repair, stringent response, genome stability, Escherichia coli, Bacillus subtilis
Citation: Sinha AK, Løbner-Olesen A and Riber L (2020) Bacterial Chromosome Replication and DNA Repair During the Stringent Response. Front. Microbiol. 11:582113. doi: 10.3389/fmicb.2020.582113
Received: 10 July 2020; Accepted: 13 August 2020;
Published: 28 August 2020.
Edited by:
Feng Gao, Tianjin University, ChinaReviewed by:
Gregory Marczynski, McGill University, CanadaGert Bange, University of Marburg, Germany
Copyright © 2020 Sinha, Løbner-Olesen and Riber. This is an open-access article distributed under the terms of the Creative Commons Attribution License (CC BY). The use, distribution or reproduction in other forums is permitted, provided the original author(s) and the copyright owner(s) are credited and that the original publication in this journal is cited, in accordance with accepted academic practice. No use, distribution or reproduction is permitted which does not comply with these terms.
*Correspondence: Anurag Kumar Sinha, YWt1c2lAZm9vZC5kdHUuZGs=; Leise Riber, bHJpYmVyQHBsZW4ua3UuZGs=
†Present address: Anurag Kumar Sinha, National Food Institute, Technical University of Denmark, Lyngby, Denmark; Leise Riber, Department of Plant and Environmental Sciences, University of Copenhagen, Copenhagen, Denmark
‡These authors have contributed equally to this work