- State Key Laboratory of Silkworm Genome Biology, Southwest University, Chongqing, China
Scleromitrula shiraiana is a necrotrophic fungus with a narrow host range, and is one of the main causal pathogens of mulberry sclerotial disease. However, its molecular mechanisms and pathogenesis are unclear. Here, we report a 39.0 Mb high-quality genome sequence for S. shiraiana strain SX-001. The S. shiraiana genome contains 11,327 protein-coding genes. The number of genes and genome size of S. shiraiana are similar to most other Ascomycetes. The cross-similarities and differences of S. shiraiana with the closely related Sclerotinia sclerotiorum and Botrytis cinerea indicated that S. shiraiana differentiated earlier from their common ancestor. A comparative genomic analysis showed that S. shiraiana has fewer genes encoding cell wall-degrading enzymes (CWDEs) and effector proteins than that of S. sclerotiorum and B. cinerea, as well as many other Ascomycetes. This is probably a key factor in the weaker aggressiveness of S. shiraiana to other plants. S. shiraiana has many species-specific genes encoding secondary metabolism core enzymes. The diversity of secondary metabolites may be related to the adaptation of these pathogens to specific ecological niches. However, melanin and oxalic acid are conserved metabolites among many Sclerotiniaceae fungi, and may be essential for survival and infection. Our results provide insights into the narrow host range of S. shiraiana and its adaptation to mulberries.
Introduction
Mulberry (Morus spp.) is the feed crop for silkworms (Bombyx mori L.). In addition, mulberry fruit also has great economic and medicinal value because it is rich in secondary metabolites that are beneficial to human health (Chen et al., 2016, 2017; Choi et al., 2016). Mulberry sclerotial disease is the most serious fungal disease of Morus spp., and it severely reduces fruit yield. However, effective prevention and control measures are lacking. Three fungi in the Sclerotiniaceae, Ciboria carunculoides, Ciboria shiraiana, and Scleromitrula shiraiana, cause mulberry sclerotial disease (Whetzel and Wolf, 1945; Hong et al., 2007). S. shiraiana is widely distributed in major mulberry cultivation areas in East Asia, such as China, South Korea, and Japan (Schumacher and Holst-Jensen, 1997). S. shiraiana has a similar life cycle to that of C. carunculoides and C. shiraiana, and forms sclerotia in infected drupelets. Ascospores, which are produced by the fruiting bodies that germinate from the sclerotia, are the source of primary infection.
Scleromitrula shiraiana is a typical necrotrophic plant pathogenic fungus, which is phylogenetically closely related to the notorious Sclerotinia sclerotiorum and Botrytis cinerea. Their life cycles are also similar, and all of them belong to the Sclerotinaceae family (Ascomycota) (Figure 1). Both S. sclerotiorum and B. cinerea have considerably broader host ranges than that of S. shiraiana, causing diseases in more than 400 and 1000 plant species, respectively, including many important crops (Bolton et al., 2006; Williamson et al., 2007; Elad et al., 2016). However, S. shiraiana causes disease only in mulberry. S. shiraiana can produce conidia by asexual propagation like B. cinerea, but different from S. sclerotiorum. However, in S. shiraiana, the role of conidia in the infection cycle is insignificant, because the time window suitable for pathogen infection is narrow. In addition, conidia of S. shiraiana are formed in a liquid environment, which is not conducive to spreading. Another obvious difference between S. shiraiana and its relatives S. sclerotiorum and B. cinerea is that S. shiraiana shows is very slow growth on potato dextrose agar or other artificial media. Sexual reproduction is essential for the inheritance and variation of fungi. S. sclerotiorum is self-fertilized and homothallic. However, the sexual process of most B. cinerea strains is heterothallic, i.e., it needs two different mating-type strains. The different mating types of Ascomycetes are determined by the mating-type (MAT) gene at the MAT locus. The transcription factors encoded by the MAT gene are required for sexual reproduction (Debuchy et al., 2010). Homothallic S. sclerotiorum has two types of MAT genes at one MAT locus, namely MAT1-1 and MAT1-2 (Malvárez et al., 2007). However, like heterothallic B. cinerea, different idiomorphs of S. shiraiana are in two types of strains.
Necrotrophic plant pathogens extract nutrients from dead cells that are killed prior to or during colonization. Phytotoxic compounds and cell wall-degrading enzymes (CWDEs) are common attack weapons of necrotrophs, and are deployed to induce plant cell necrosis and cause leakage of nutrients (Mengiste, 2012). Many CWDEs contribute to the pathogenicity of S. sclerotiorum and B. cinerea (Riou et al., 1991; Alghisi and Favaron, 1995; Cotton et al., 2003; Kars et al., 2005; Brito et al., 2006). The decrease in the production of numerous CWDEs was associated with a concomitant decrease in virulence of pathogens (Kubicek et al., 2014). Genomic analyses have revealed abundant genes encoding CWDEs in the genomes of S. sclerotiorum and B. cinerea (Amselem et al., 2011; Derbyshire et al., 2017; Van Kan et al., 2017). For many necrotrophs, CWDEs are their common weapons, while many phytotoxins are specific to particular pathogens. Botrydial and botcinic acid are two types of phytotoxins produced by B. cinerea (Colmenares et al., 2002; Tani et al., 2006). Several relevant biosynthetic genes have been identified, such as the polyketide synthase gene BcPKS6 and BcPKS9 (also known as Bcboa6 and Bcboa9), and BcBOT1 to BcBOT5, respectively (Dalmais et al., 2011; Collado and Viaud, 2016). Another phytotoxin, oxalic acid, is a crucial virulence factor shared by S. sclerotiorum and B. cinerea. Oxalic acid can promote virulence in several ways: It enhances the activity of polygalacturonases to promote cell wall degradation, suppresses the plant oxidative burst, promotes programmed cell death (PCD) in the host, and alters the redox in plant cells (Cessna et al., 2000; Favaron et al., 2004; Kim et al., 2008; Williams et al., 2011). Oxalic acid has also been detected in S. shiraiana, and may contribute to its pathogenicity during infection of mulberry fruits. Furthermore, there is a positive correlation between oxalic acid and melanin contents in S. shiraiana (Lü et al., 2017). Melanin plays roles in the self-protection and pathogenicity of pathogens (Butler and Day, 1998; Henson et al., 1999; Jacobson, 2000; Butler et al., 2001). Pathogens with melanized appressoria have stronger pathogenicity (Ebbole, 2007; Butler et al., 2009). For sclerotia-forming fungi, such as S. sclerotiorum, B. cinerea, and S. shiraiana, melanin is an essential component of the sclerotia (Butler et al., 2009; Liang et al., 2018). The genes involved in the biosynthesis of 1,8-dihydroxynaphthalene melanin are conserved in corresponding fungi.
A growing body of evidence suggests that the effector proteins of necrotrophic fungi suppress the plant immune response and modulate plant physiology to accommodate fungal invaders (Presti et al., 2015). Since the interaction between the necrotrophic effectors and their hosts operate opposite of the classic gene-for-gene interaction observed in host-biotroph systems, it is called inverse gene-gene interaction (Faris and Friesen, 2020). Cerato-platanins (CP) are a group of small, secreted, cysteine-rich proteins that have been found only in fungi (Pazzagli et al., 2014). Most CP proteins have been shown to act either as virulence factors or as elicitors. However, in necrotrophic fungi, the CP protein is related to fungal virulence. A previous study showed that BcSpl1 is required for virulence in B. cinerea and promotes pathogenic infection by inducing hypersensitive cell death in the host (Marcos et al., 2011). SsSm1 and SsCP1 are two members of the CP family in S. sclerotiorum. Transient expression of SsSm1 and SsCP1 in tobacco leaves was found to cause a hypersensitive response (Pan et al., 2018; Yang et al., 2018). SsCP1 was shown to interact directly with the pathogenesis-related protein PR1 in the apoplast, thereby inhibiting its potential antifungal activity. However, the functions of most fungal effector proteins are unknown, and they lack conserved domains or homologs in other fungi.
It is noteworthy that both BcSpl1 and SsCP1 induce an increase in the salicylic acid (SA) concentration in the host (Marcos et al., 2013; Yang et al., 2018). In plants, SA is required to activate defenses against biotrophic and hemi-biotrophic pathogens, and it is essential in local resistance and systemic acquired resistance (SAR) (Qi et al., 2018). Local resistance induces hypersensitive response (HR)-like cell death at the infected site, making the plant resistant to biotrophic and hemi-biotrophic pathogens, but susceptible to necrotrophic pathogens. However, SAR is broad-spectrum resistance to a variety of pathogens, including necrotrophs (Vlot et al., 2009). Thus, SA may be an ancient plant hormone involved in defense, but it may also play a more complex role in the arms race between plants and necrotrophic pathogens.
As mentioned above, S. shiraiana is a pathogen with a narrow host spectrum unlike S. sclerotiorum and B. cinerea. High-quality and almost complete genome sequences are available for S. sclerotiorum and B. cinerea. Previous studies have also reported the genomes of some obligate parasitic fungi and restricted host range necrotrophs such as powdery mildew fungi, rust fungi, Parastagonospora nodorum, and Pyrenophora teres f. teres (Hane et al., 2007; Ellwood et al., 2010; Spanu et al., 2010; Duplessis et al., 2011; Zheng et al., 2013; Schwessinger et al., 2018). Genomic analyses of pathogenic fungi with different nutritional styles can provide clues about the reasons for the differences in their host ranges.
In this study, we sequenced the genome of S. shiraiana, which is only known to infect mulberry. Our results show that the number of genes and genome size of S. shiraiana are similar to those of other necrotrophic phytopathogenic fungi. Effector proteins and CWDEs are common offensive weapons of necrotrophic pathogens. However, S. shiraiana has fewer genes encoding effectors and CWDEs than do S. sclerotiorum and B. cinerea, even though they are taxonomically closely related. Many phytotoxins of pathogens are secondary metabolites, and we detected major differences in secondary metabolism genes among S. shiraiana, S. sclerotiorum, and B. cinerea. This suggests that S. shiraiana may produce specific toxins to promote infection. In summary, the reduced number of key pathogenicity-related genes and the differences in secondary metabolism genes may jointly contribute to the adaptation of S. shiraiana to mulberry.
Materials and Methods
Strains, Culture Conditions, and Nucleic Acid Isolation
Scleromitrula shiraiana strain SX-001 was isolated from diseased mulberry fruit in our laboratory (Lü et al., 2017). The strain was routinely sub-cultured on potato dextrose agar (PDA) medium at 25°C to maintain vigor. Mycelia were collected from the media and ground in liquid nitrogen. Genomic DNA was extracted using a modified cetyltrimethylammonium bromide (CTAB) method (Umesha et al., 2016). Total RNA was extracted with TRIzol reagent (Invitrogen, Carlsbad, CA, United States) according to the manufacturer’s instructions and treated with RNase-free DNase I to remove contaminating DNA.
Genome Sequencing and Assembly
The genome of S. shiraiana strain SX-001 was sequenced by Single Molecule Real-Time (SMRT) technology at the Beijing Novogene Bioinformatics Technology Co., Ltd. (Beijing, China). The DNA sample used for genome sequencing was assessed by Qubit Fluorometer and agarose gel electrophoresis. The 350-bp library was constructed following the standard protocols of Illumina (San Diego, CA, United States). The 350-bp library was quantified using an Agilent 2100 Bioanalyzer (Agilent, Palo Alto, CA, United States) and subjected to paired-ended 150-bp sequencing by Illumina HiSeq PE150. For long reads sequencing, 20-kb SMRTbell libraries were constructed following the standard methods of PacBio (Menlo Park, CA, United States). The 20-kb libraries were sequenced on a PacBio RSII instrument. The low quality reads were filtered by the SMRT Link v5.0.1 and the filtered reads were assembled to generate one contig without gaps. Finally, 21 high quality contigs were obtained.
Genome Annotations
Protein-coding genes were annotated from the genome sequences using MAKER2 pipeline (Holt and Yandell, 2011). First, interspersed repetitive sequences were predicted by the RepeatMasker (Version open-4.0.5) combined with RepBase-20181026. Tandem Repeats were analyzed by TRF (Tandem repeats finder, Version 4.07b). Second, ab initio gene prediction was performed with protein-coding sequences from two strains in B. cinerea (B05.10 and T4), S. sclerotiorum, S. borealis, Botrytis paeoniae, and Botrytis tulipae (Amselem et al., 2011; Mardanov et al., 2014; Valero-Jiménez et al., 2019). For transcriptome sequences, we used a locally assembled transcriptome from RNA-Seq data using Trinity v2.11.0 (Grabherr et al., 2011). Third, two gene predictors were used in the pipeline: GeneMark-ES version 4.62 and Augustus version 3.3.3 (Ter-Hovhannisyan et al., 2008; Keller et al., 2011).
The following seven databases were used to predict gene functions: GO (Gene Ontology), KEGG (Kyoto Encyclopedia of Genes and Genomes), KOG (Clusters of Orthologous Groups), NR (Non-Redundant Protein Database databases), TCDB (Transporter Classification Database), P450, and, Swiss-Prot. A whole genome BLAST search (E-value < 1e-5, minimal alignment length percentage >40%) was also performed against the above seven databases.
Comparative Analyses of Genes Encoding Carbohydrate-Active Enzymes and Secondary Metabolism Clusters and Genes
The identification and annotation of all 16 fungal carbohydrate-active enzymes were based on the Carbohydrate-active enzymes (CAZy) database and performed on the dbCAN2 meta server (Lombard et al., 2013; Zhang et al., 2018). All 16 fungal secondary metabolism clusters and genes were predicted by antiSMASH fungal version with default parameters (Blin et al., 2019). Genes encoding polyketide synthase (PKS), non-ribosomal peptide synthetase (NRPS), PKS-NRPS hybrid (HYBRID), terpene synthase (TS), and dimethylallyl tryptophan synthase (DMATS) were confirmed by NCBI BLASTP and CDD searches. The fungal genomes used for comparative analyses were downloaded from DOE Joint Genome Institute (JGI)1 and Ensembl Fungi2.
Gene Family and Phylogenetic Analysis
Ortholog families in the S. shiraiana genome and 15 other fungal genomes were identified by OrthoFinder v2.4.0 (Emms and Kelly, 2019). The phylogenetic analysis was conducted using STAG (Species Tree Inference from All Genes) and STRIDE (Species Tree Root Inference from gene Duplication Events) methods, which infer a species tree from sets of multi-copy genes (Emms and Kelly, 2017, 2018).
Melanin Synthesis Gene Cluster Analysis
Two PKS genes (sshi00009473 and sshi00001626), which are associated with 1,8-dihydroxynaphthalene (DHN) melanin synthesis in S. shiraiana, were identified based on homologous genes in B. cinerea and S. sclerotiorum. Genes encoding two other key enzymes for melanin synthesis, 1,3,6,8-tetrahydroxynaphthalene reductase (4HNR), and scytalone dehydratase (SCD), were identified by BLASTP in the genome of B. cinerea and S. sclerotiorum. ShPKS13 (sshi00001626) is located in the same gene cluster as ShSCD (sshi00001628) and Sh4HNR (sshi00001627).
Secretory Proteins and Putative Effectors
The secretory proteins were predicted using SignalP 5.0 (Almagro Armenteros et al., 2019). Then, TMHMM 2.0 was used to filter out those containing transmembrane domains (Krogh et al., 2001). Potential virulence-related proteins were identified by searching against the pathogen-host interaction database (PHI base) by BLASTP (Urban et al., 2016). To predict effectors, known secretory protein sequences were entered into the Big-PI Fungal Predictor to filter out proteins harboring a putative glycophosphatidylinositol membrane-anchoring domain (Eisenhaber et al., 2004). Finally, EffectorP 2.0 was used to predict potential effectors in the remaining proteins (Sperschneider et al., 2018).
Transient Expression Analysis of Putative Effectors in Nicotiana benthamiana
The putative effector genes were cloned into pGR107 by homologous recombination with the pEASY Basic Seamless Cloning and Assembly Kit (TransGen, Beijing, China) according to the manufacturer instructions. The GR107 vector was linearized by digestion with ClaI and SalI. Then, each construct was transformed into Agrobacterium tumefaciens strain GV3101 containing the helper plasmid pJIC SA_Rep. Infiltration experiments were performed on 4- to 6-weeks-old N. benthamiana plants using needleless syringes as described before (Lv et al., 2018). The negative control was GFP and the positive control was the pro-apoptotic mouse protein BAX. Cell death symptoms were photographed at 6 days after infiltration. The results are representative of three biological replicates. The primer sequences are listed in Supplementary Table 8.
Obtaining and Analysis of ShSCD-Deletion Mutants
Deletion of ShSCD was performed as described previously by Yu et al. (2016). The gene deletion utilizes pSKH vector. The primer pair SCD3XhoIF and SCD3KpnIR were used to amplify a c. 800 bp sequence of 3′ untranslated region (UTR) of ShSCD gene. The fragment was digested with XhoI and KpnI and then inserted into pSKH to produce the pSKHSCD1. The primer pair SCD5SacIF and SCD5NotIR were used to amplify a c. 800 bp sequence of 5′ UTR of ShSCD gene. The fragment was digested with SacI and NotI and then inserted into pSKHSCD1 to produce the pSKHSCD2. The pSKHSCD2 plasmid was used as a PCR template, and the 5′UTR:N-HY and 3′UTR:C-YG fragments were amplified using primer pairs SCD5-F/HY-R and YG-F/SCD3-R, respectively. Then two fragments were transformed into protoplasts of wild-type S. shiraiana SX-001 strain as described by Rollins (2003). The ShSCD-deletion mutants were identified by PCR and Southern blot hybridization. For Southern blotting, probes were designed based on the gene sequence of ShSCD and the CDS of the gene encoding the hygromycin B phosphotransferase (HYG). The probes were labeled with the DIG High Prime DNA Labeling and Detection Starter Kit II (Roche, Indianapolis, IN, United States) according to the manufacturer’s instructions. Genomic DNA was extracted from fungal strains using the CTAB method (Umesha et al., 2016). Southern blotting was performed based on the method of Qi et al. (2014). The relative yield of melanin was measured with a spectrophotometer at 420 nm as described by Lü et al. (2017). The primers used are listed in Supplementary Table 8.
ShSCD-deletion mutants and wild-type S. shiraiana were inoculated in PDA medium containing 5 mM and 10 mM hydrogen peroxide, respectively. There were six plates in each group, and colony photography and diameter measurement were conducted after 9 days of culture. The experiments were repeated at least three times.
Plant Treatment and Fungal Inoculation
Each group of ten 6-week-old Arabidopsis leaves were pretreated with CWDE solutions I (3.0% cellulase R-10), II (1.0% pectinase) or III (2.0% hemicellulase) for 30 h, respectively. The buffer used in the enzyme solution comprised 0.6 M D-mannitol, 20 mM potassium chloride, and 20 mM MES monohydrate (pH 5.7). Leaves pre-treated with this buffer served as negative controls. The pre-treated Arabidopsis leaves were washed with double-distilled water, and then excess water was removed with filter paper. The leaves were transferred to Petri dishes lined with moist filter paper and then inoculated with S. shiraiana. The leaves were photographed at 4 days after inoculation. The experiments were repeated at least three times.
Accession Code
The S. shiraiana genome data have been deposited in the Genome Warehouse (GWH) in National Genomics Data Center, Beijing Institute of Genomics (BIG), Chinese Academy of Sciences, under accession number GWHACFG00000000 that is publicly accessible at https://bigd.big.ac.cn/gwh.
Results
Genome Sequencing and Assembly
To investigate the infection mechanism and to generate a high-quality reference genome for S. shiraiana, the genome of S. shiraiana strain SX-001 was sequenced by SMRT technology. A total of 3.40 Gb data were de novo assembled using SMRT Link v5.1.0. The genome sequence assembly contained 21 contigs with a total length of 39.0 Mb and a coverage of 87-fold (Table 1). The contig N50 was 3.61 Mb and the max-length contig was 4.96 Mb. The genome size and predicted gene number of S. shiraiana was similar to those of other related fungi, such as S. sclerotiorum, B. cinerea, and Magnaporthe oryzae, except for biotrophic Blumeria graminis f. sp. hordei (Figure 2 and Supplementary Table 1). However, the GC content in the genome sequence was lower in S. shiraiana (38.9%) than in other fungi (Supplementary Table 1).
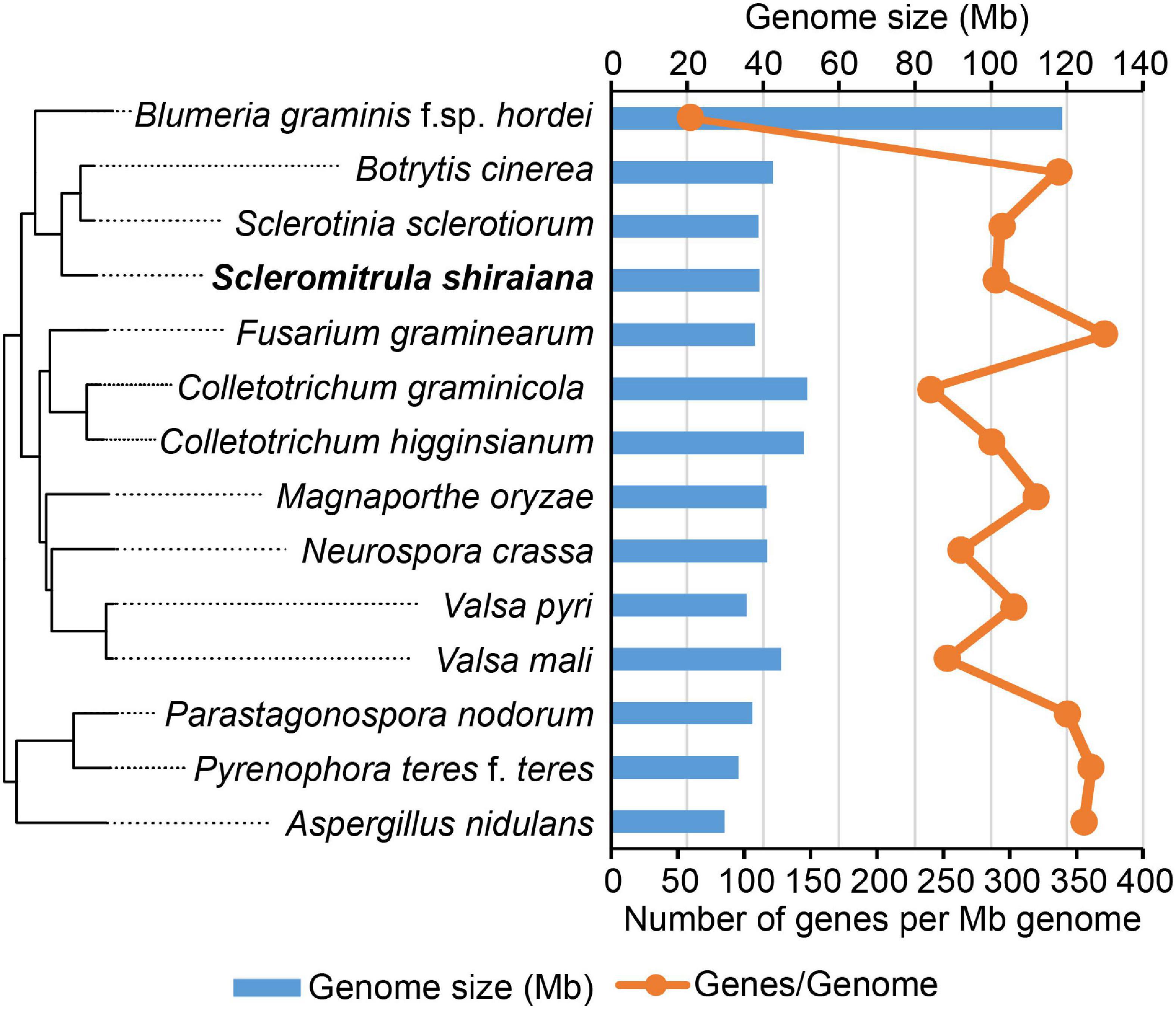
Figure 2. Genome size and the number of genes per mega genome sequence of Scleromitrula shiraiana and 13 other Ascomycetes.
The proportion of repetitive sequences in the genome of S. shiraiana (4.45%) was comparable to that in the genome of B. cinerea (4.4%), and lower than that in the genome of S. sclerotiorum (7.7%) (Figure 3). However, only 0.07% of the repetitive sequences were predicted to be transposable elements, which is significantly lower than that in S. sclerotiorum (7%) and in B. cinerea (0.9%) (Figure 3B).
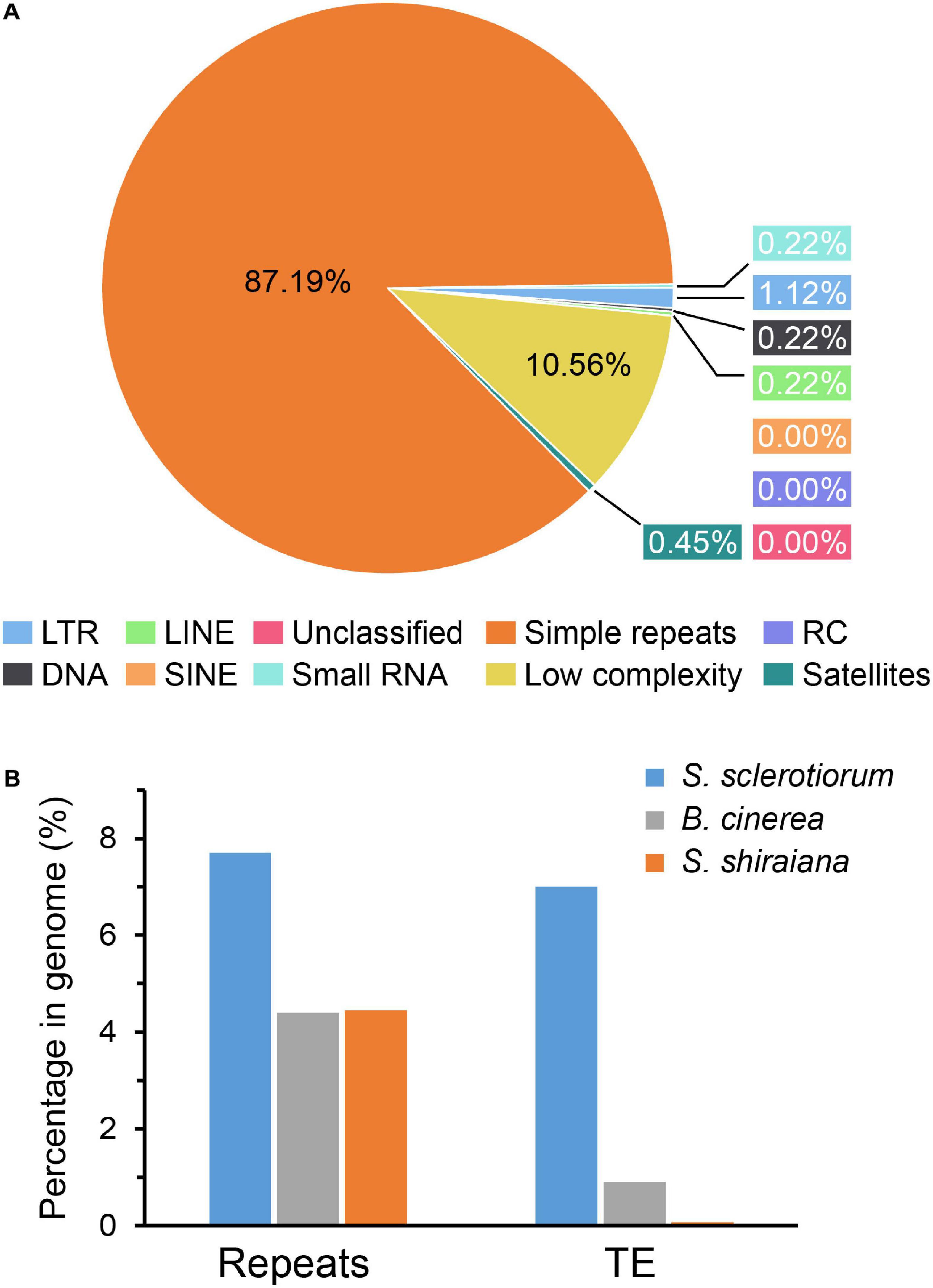
Figure 3. Repetitive sequences and transposable elements (TEs) in Scleromitrula shiraiana genome. (A) Repetitive sequences content of the genomes of S. shiraiana genome. (B) Comparison of the proportion of repeats and transposable elements among genomes of Sclerotinia sclerotiorum, Botrytis cinerea, and S. shiraiana. TEs include LTR, LINE, SINE, DNA, RC, and Unclassified. LTR, long terminal repeat; LINE, long interspersed nuclear element; SINE, short interspersed nuclear element; DNA, DNA transposon; RC, rolling circle; Unclassified, unclassified transposable elements.
Mating Type (MAT) of S. shiraiana
Sclerotinia sclerotiorum contains two types of MAT idiomorphs, MAT1-1 and MAT1-2. The products encoded by MAT1-1-1 and MAT1-2-1 contain a conserved alpha domain and a high mobility group (HMG) domain, respectively. B. cinerea Strain B05.10 contains a MAT1-1 idiomorph including a characteristic MAT1-1-1 alpha-domain gene and MAT1-1-5. The B. cinerea strain T4 contains a MAT1-2 idiomorph including a characteristic MAT1-2-1 HMG-domain gene and MAT1-2-4 (Faretra et al., 1988). The arrangement of the MAT locus of S. shiraiana SX-001 was similar to that of B. cinerea T4 (Figure 4). The MAT1-2 idiomorph of S. shiraiana SX-001 contained MAT1-2-1 and MAT1-2-4, but their encoded products showed only 37.0% and 29.1% similarity to their homologs in B. cinerea T4, respectively. These values were significantly lower than the similarity of MAT1 products between B. cinerea T4 and S. sclerotiorum (strain 1980), 77.0% and 65.1%, respectively (Supplementary Figure 1).
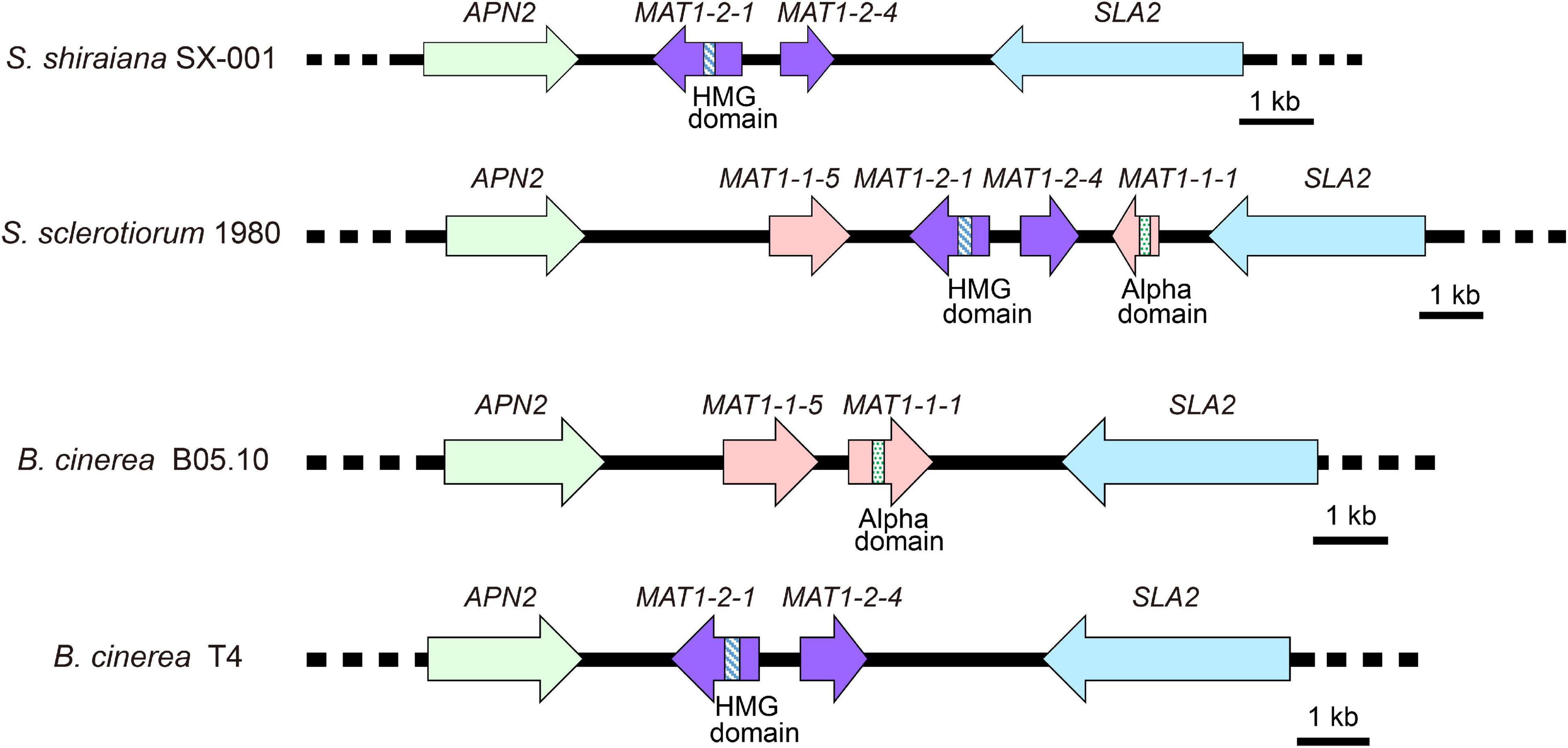
Figure 4. Comparative analysis of MAT loci among Scleromitrula shiraiana SX-001, Sclerotinia sclerotiorum 1980, and Botrytis cinerea (B05.10 and T4). Orthologous genes are shown with same color and pattern.
Comparative Genomic Analysis
The orthologous protein families were identified by OrthoFinder. The proteomes of S. shiraiana and 15 other fungi were clustered, and 15,558 ortholog families were obtained (Supplementary Tables 2,3). Of these ortholog families, 1266 single-copy orthologs were identified in all 16 fungi (Supplementary Table 3). In addition, there were 39,345 unassigned genes, accounting for 20.0% of the total number of genes. These 16 fungi shared 2,605 ortholog families and contained 157,162 genes (Figure 5B and Supplementary Table 3). A phylogenetic tree of these 16 fungi was constructed with STAG (Species Tree inference from All Genes) and STRIDE (Species Tree Root Inference from gene Duplication Events) methods (Figure 5A). In the phylogenetic tree, S. shiraiana was clustered with three other Leotiomycetes; B. cinerea, S. sclerotiorum, and B. graminis f. sp. hordei. Among the 16 fungi, S. shiraiana has more missing orthogroups than the closely related S. sclerotiorum and B. cinerea (Figure 5B).
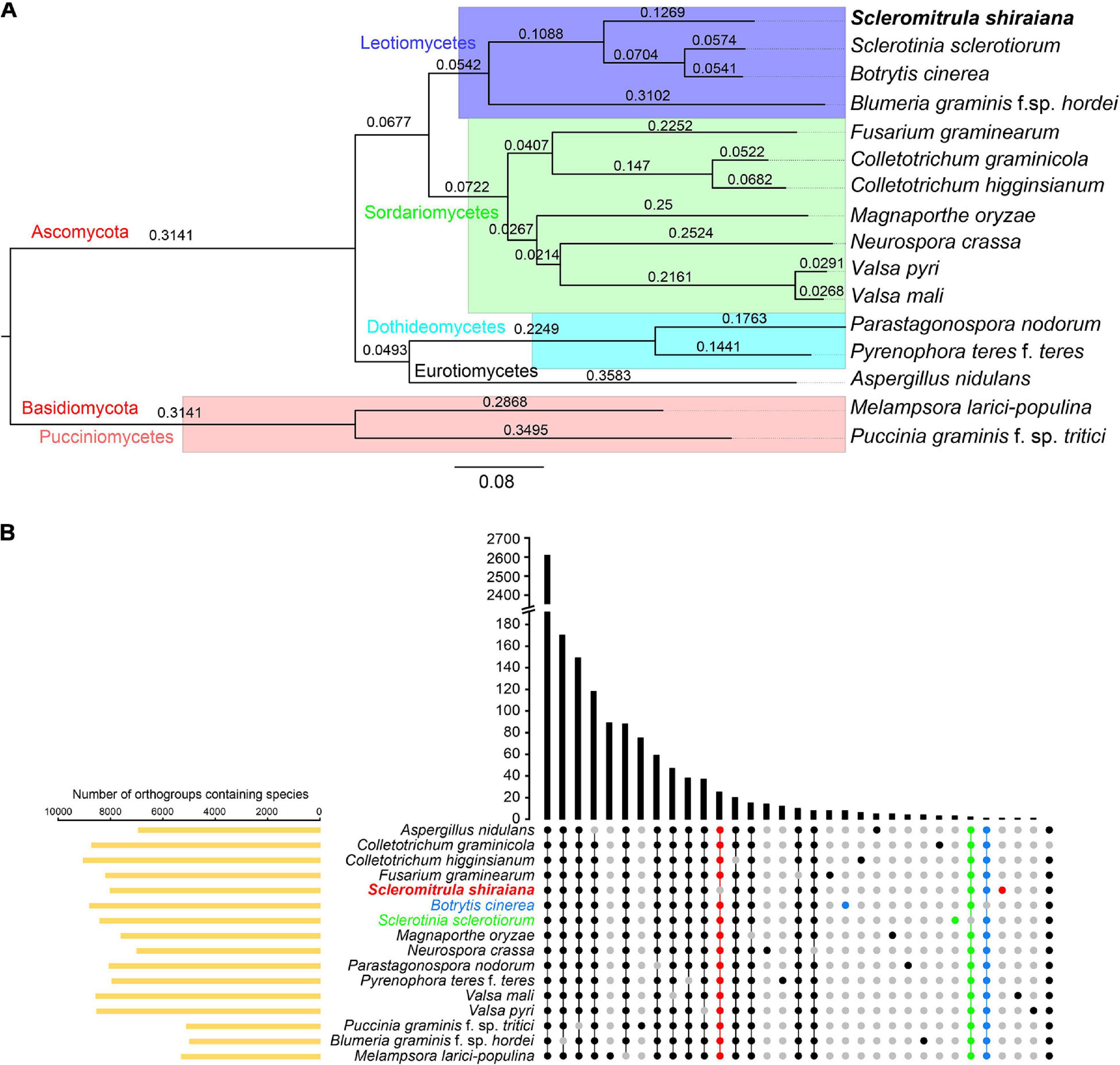
Figure 5. Phylogenetic and orthogroups analysis of Scleromitrula shiraiana and other fungal species. (A) Phylogenetic tree of different fungal species. Phylogenetic tree was constructed with STAG (Species Tree inference from All Genes) and STRIDE (Species Tree Root Inference from gene Duplication Events) methods with OrthoFinder2. Melampsora larici-populina and Puccinia graminis f. sp. tritici were used as outgroups. (B) Statistical analysis of Orthogroups of S. shiraiana and other 15 fungi. Orthogroups in each genome were identified using OrthoFinder with default parameters. Species-specific orthogroups and species-missing orthogroups in S. shiraiana, Botrytis cinerea, Sclerotinia sclerotiorum are highlighted.
Since S. shiraiana, B. cinerea, S. sclerotiorum, and B. graminis f. sp. hordei were clustered in the phylogenetic tree, and B. graminis f. sp. hordei is a special Leotiomycetes member (obligate biotroph, huge genomes, and few protein-coding genes), so S. shiraiana, B. cinerea, and S. sclerotiorum were analyzed separately. S. shiraiana, B. cinerea, and S. sclerotiorum belong to the same family (Sclerotiniaceae), but they are classified into three different genera. These three species shared 7,402 ortholog families (Supplementary Figure 2). Although S. shiraiana is evolutionally close to B. cinerea and S. sclerotiorum, genome coverage was only 20.9% and 21.4%, respectively (Supplementary Table 4). These values were lower than the coverage between B. cinerea and S. sclerotiorum (72.3%) (Amselem et al., 2011), and between Colletotrichum higginsianum and Colletotrichum graminicola (35.3%) (O’connell et al., 2012). These results suggest that S. shiraiana may have differentiated from its ancestors earlier than did S. sclerotiorum and B. cinerea.
Oxalic Acid and Secondary Metabolism
Oxalic acid is an important pathogenic metabolite in S. sclerotiorum (Cessna et al., 2000; Kim et al., 2008; Williams et al., 2011). The enzyme OAH (oxaloacetate acetyl hydrolase) is associated with oxalic acid accumulation (Han et al., 2007; Liang et al., 2015; Xu et al., 2015). Oxalic acid is also produced by S. shiraiana (Lü et al., 2017). An OAH gene (Ssh_07163) was identified in the S. shiraiana genome. Further research is required to identify the key genes for oxalic acid biosynthesis and degradation and the role of oxalic acid in the pathogenicity of S. shiraiana.
To identify the pathways involved in the production of secondary metabolites in S. shiraiana, a genome-wide search was conducted to identify genes encoding key enzymes such as PKS (polyketide synthase), NRPS (non-ribosomal peptide synthetase), HYBRID (hybrid NRPS–PKS enzyme), TS (terpene synthase), and DMATS (dimethylallyl tryptophan synthase). We detected 33 core biosynthetic genes encoding these five key enzymes, which were similar in number to those in S. sclerotiorum, but less than those in B. cinerea (Figure 6 and Supplementary Table 5). However, 36.3% of the core biosynthetic genes of S. shiraiana are species-specific and their function is unknown, indicating that S. shiraiana may produce species-specific secondary metabolites.
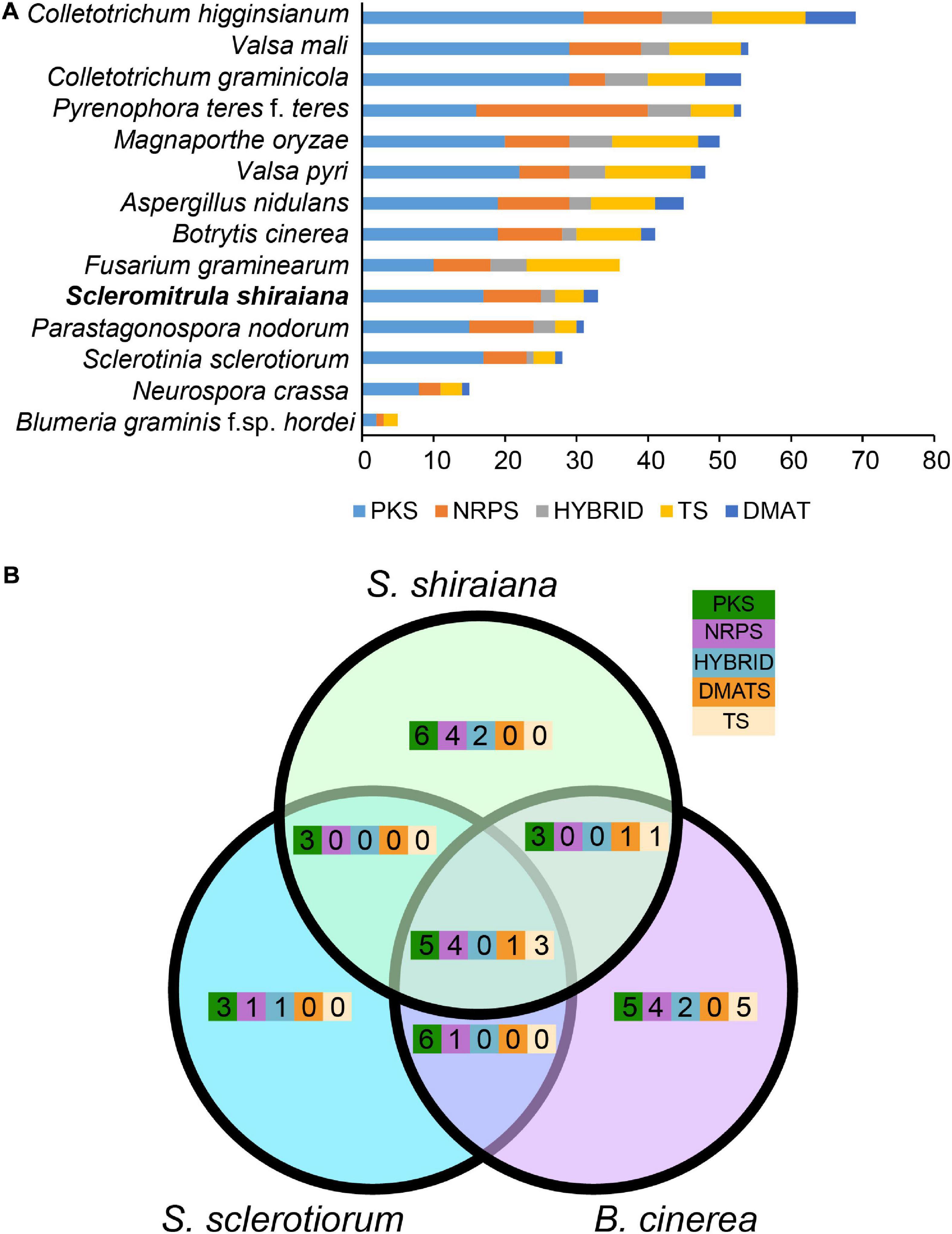
Figure 6. Comparison of secondary metabolism genes between Scleromitrula shiraiana and other Ascomycetes. (A) Comparison of genes encoding PKS, NRPS, HYBRID, TS, and DMATS between S. shiraiana and other 13 Ascomycetes. (B) Comparison of genes encoding PKS, NRPS, HYBRID, TS, and DMATS among S. shiraiana, Botrytis cinerea, and Sclerotinia sclerotiorum. PKS, NRPS, HYBRID, TS, and DMATS are represented by five different color rectangles. PKS, polyketide synthase; NRPS, non-ribosomal peptide synthase; HYBRID, hybrid NRPS–PKS enzyme; TS, terpene synthase; DMATS, dimethylallyl tryptophan synthase.
Melanin is necessary for pathogen fitness (Henson et al., 1999; Liu and Nizet, 2009). The key genes for melanin synthesis in fungi are PKS, SCD (encoding scytalone dehydratase), and 4HNR (encoding 1,3,6,8-tetrahydroxynaphthalene reductase). Homologs of these were found in S. shiraiana, and like in other fungi, they were arranged in the same gene cluster. However, the arrangement of these genes differed between S. shiraiana and S. sclerotiorum/B. cinerea (Figure 7A), suggesting that genomic rearrangement has occurred in S. shiraiana. To verify the effect of melanin on S. shiraiana, ShSCD-deletion mutants were obtained (Figure 7B and Supplementary Figure 4). Melanin production was significantly reduced in the ShSCD-deletion mutants (Figures 7B,C). Reactive oxygen species are related to plant defense against pathogens. This study showed that the ability of the ShSCD-deletion mutants to resist oxidative stress (H2O2) was lower than that of the wild type (Supplementary Figure 4). Unfortunately, pathogenicity assays have not been performed because the filamentous hyphae of S. shiraiana were difficult to inoculate mulberry, and S. shiraiana cannot form viable sclerotia in artificial medium (Lü et al., 2017). This may be related to the fact that the sclerotium of S. shiraiana is pseudosclerotium (Lü et al., 2017). Therefore, the pathogenicity of ShSCD-deletion mutants requires further determination.
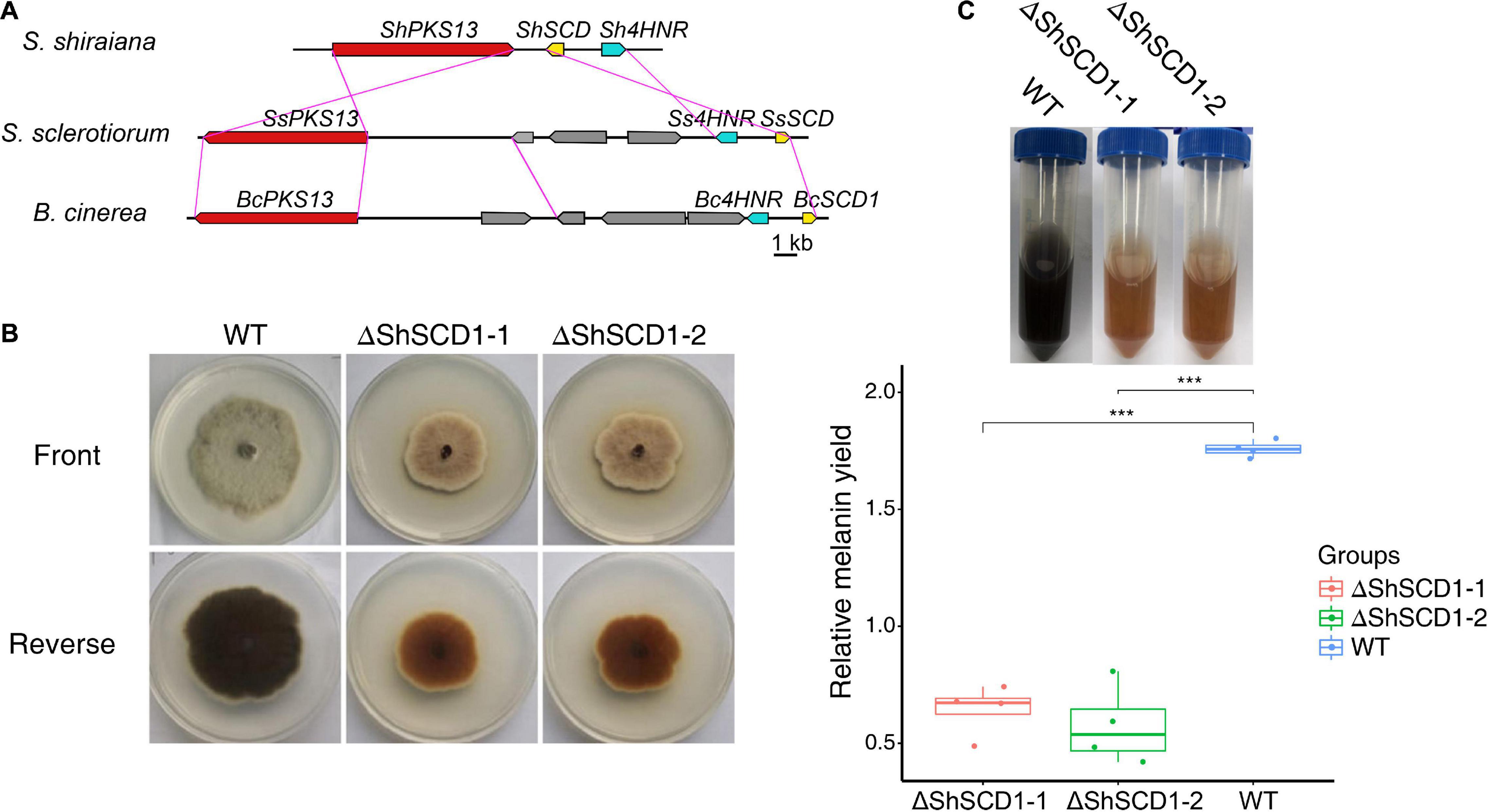
Figure 7. Cluster analysis of melanin biosynthetic genes in Scleromitrula shiraiana and functional analysis of ShSCD. (A) Comparison and analysis of melanin synthesis gene clusters among S. shiraiana, Botrytis cinerea, and Sclerotinia sclerotiorum. (B) Phenotypes of wild-type strain and ShSCD-deletion strains on potato dextrose agar (PDA) medium. (C), Relative melanin yield in wild-type strain and ShSCD-deletion strains. Asterisks indicate a statistically significant difference (***p < 0.001) according to Student’s t-test (n = 4). Experiments were repeated at least three times.
Reduced Number of Genes Encoding Plant CWDEs in S. shiraiana
The plant cell wall is an important barrier against pathogen attack (Kubicek et al., 2014). To overcome this barrier, phytopathogenic fungi produce a wide variety of secreted enzymes to degrade the major structural polysaccharide components of the plant cell wall. A comparative analysis showed that there were fewer genes encoding carbohydrate-active enzymes (CAZymes) in S. shiraiana than in the other hemibiotrophic and necrotrophic fungi being analyzed. The comparison also showed that the number of CAZymes in necrotrophic pathogenic fungi was similar or slightly less than that in the hemibiotrophic pathogenic fungi, but more than that in biotrophic and saprophytic fungi. However, there were significantly fewer CAZymes in S. shiraiana than those of restricted host range necrotrophic P. nodorum and P. teres f. teres (Table 2).
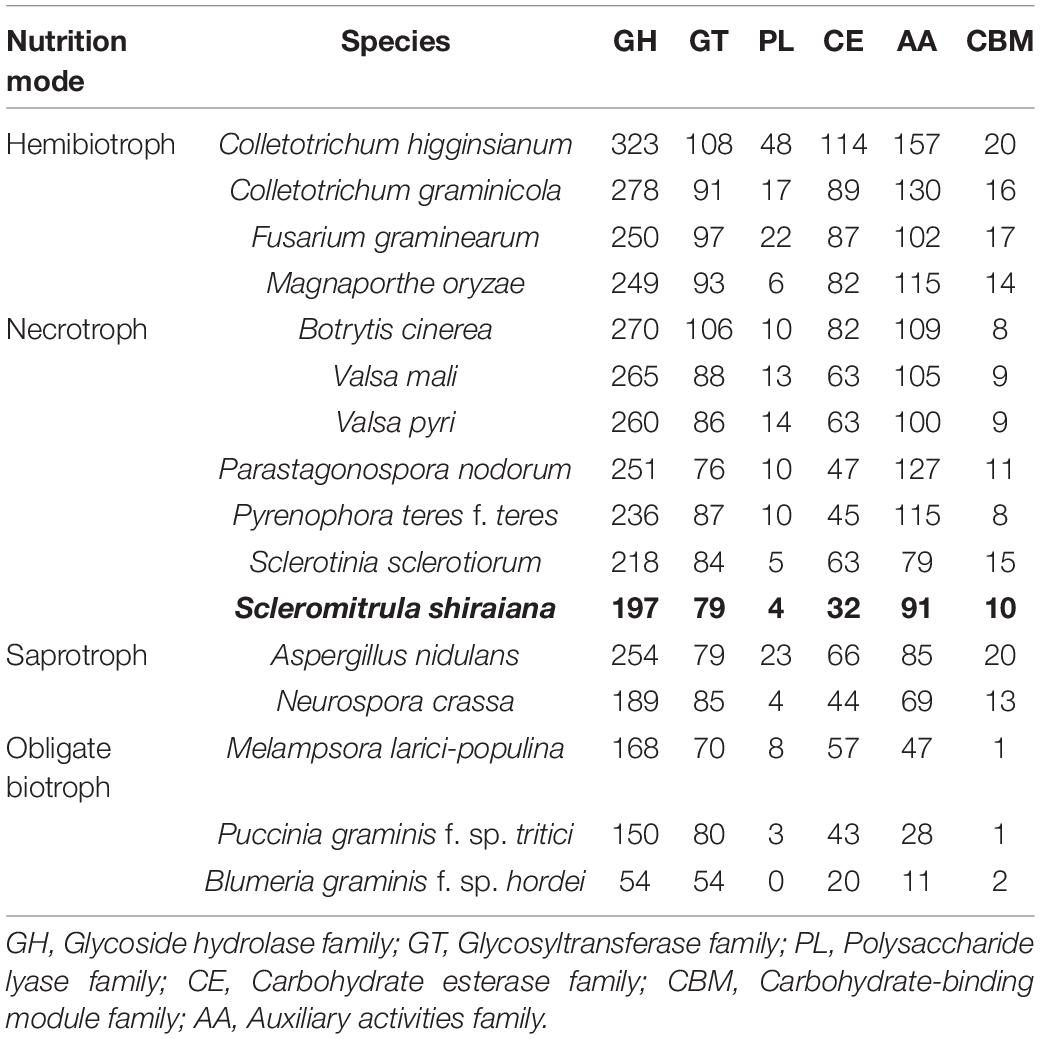
Table 2. Comparison of carbohydrate-active enzyme (CAZyme) classes from S. shiraiana and 15 other fungal genomes.
The number of genes encoding CWDEs of S. shiraiana was 67.3% of that of B. cinerea and 77.2% of that of S. sclerotiorum (Supplementary Table 6). Among all the necrotrophic pathogens compared, S. shiraiana had the smallest number of genes encoding CWDEs (Figure 8A and Supplementary Table 6). Some Ascomycetes, such as S. sclerotiorum and B. cinerea, Valsa mali, and Valsa pyri, are suited for pectin decomposition. However, S. shiraiana had significantly fewer genes encoding polygalacturonases and rhamnogalacturonases (GH28), and pectate lyase and pectin lyase (PL1), which are necessary for efficient degradation of pectin (Figure 8A and Supplementary Table 6). The number of genes encoding hemicellulases also differed widely among the 16 fungal genomes. Among Ascomycetes, there is only one gene encoding acetyl xylan esterase (CE1) and endo-β-1,4-xylanase (GH11) in S. shiraiana, while other fungi contain two or more (Supplementary Table 6). Acetylesterases (CE16) are also less abundant in S. shiraiana than in S. sclerotiorum and B. cinerea. In addition, β-mannanase (GH26) is absent in S. shiraiana, as well as P. nodorum and P. teres f. teres, B. graminis f. sp. hordei, Fusarium graminearum, and M. oryzae. Interestingly, with the exception of S. shiraiana, the other five fungi are restricted or obligate infecting gramineous plants.
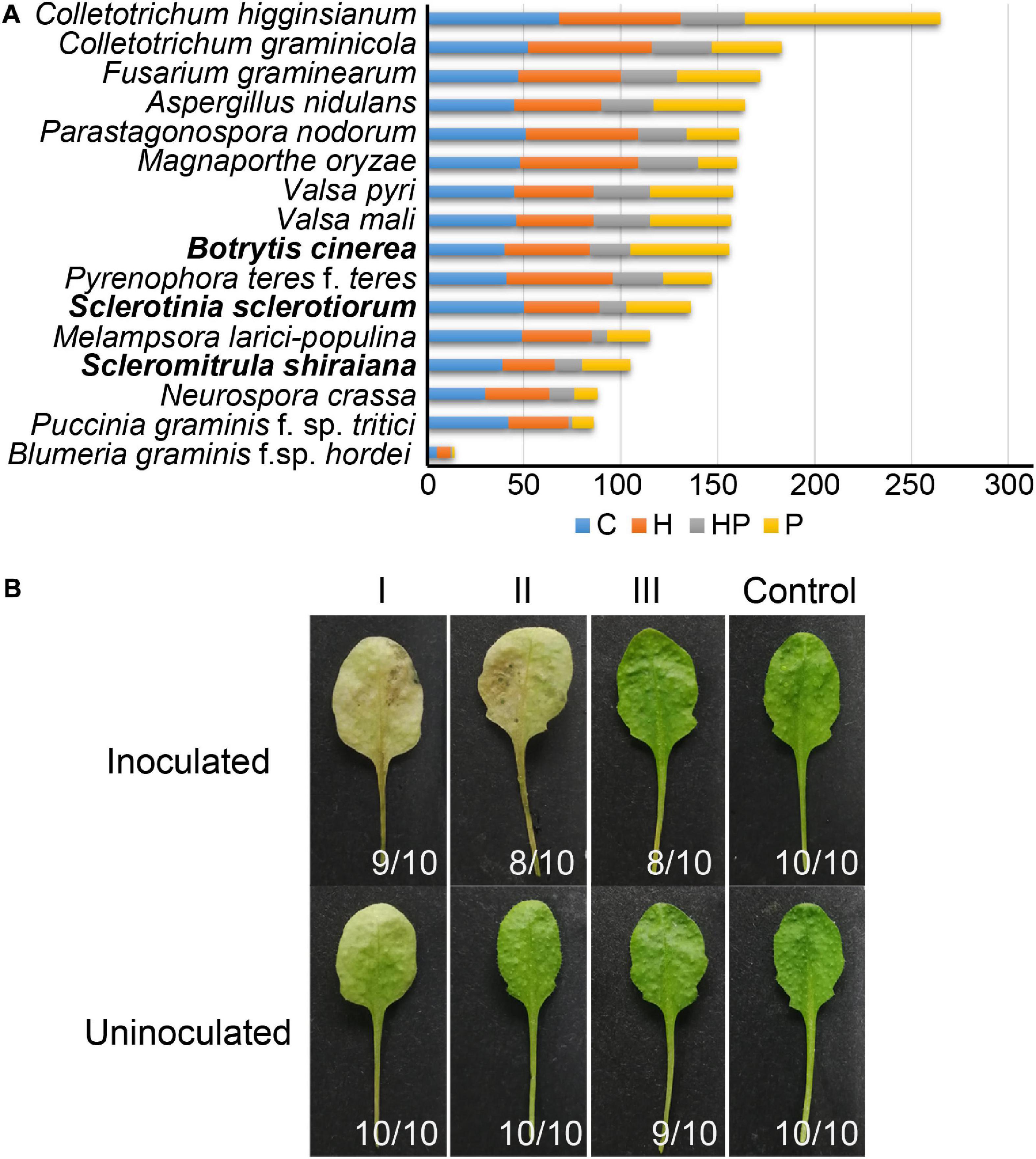
Figure 8. Genes encoding plant cell wall-degrading enzymes (CWDEs) in Scleromitrula shiraiana. (A) Comparison of genes encoding CWDEs between S. shiraiana and 15 other fungi. C, cellulase; H, hemicellulase; HP, hemicellulase or pectinase (degrade hemicellulose and pectin side chains); P, pectinase. (B) Effects of additional application of cell wall-degrading enzymes on pathogenicity of S. shiraiana against Arabidopsis. Arabidopsis leaves were pretreated buffer (negative control) or CWDE solution I (3.0% cellulase R-10), II (1.0% pectinase), or III (2.0% hemicellulase) for 30 h, washed with double-distilled water, inoculated with S. shiraiana, and photographed at 4 days after inoculation. The number in the lower right corner of each leaf indicates the number of leaves with the corresponding phenotype/number of all detected leaves. The experiments were repeated at least three times.
We hypothesized that the small number of genes encoding CWDEs may explain the weak pathogenicity of S. shiraiana. To test this hypothesis, we conducted experiments using Arabidopsis leaves insensitive to S. shiraiana. The Arabidopsis leaves were pretreated with cellulase, pectinase, and hemicellulase, and then inoculated with S. shiraiana. The application of cellulase and pectinase allowed S. shiraiana to infect Arabidopsis leaves, but the application of hemicellulase did not (Figure 8B). This result suggests that the small number of CWDEs in S. shiraiana may be involved in its limited host range.
Functional Analysis of Putative Effectors
Plant pathogens secrete effector proteins to suppress plant defense responses and modulate host cellular processes to promote colonization (Lo Presti and Kahmann, 2017). The function of most fungal effector proteins is unknown, and most of them lack conserved domains or homologs in other species. We detected 68 genes encoding putative effector proteins in S. shiraiana, which was less than 78 in S. sclerotiorum and 109 in B. cinerea (Figure 9A). As expected, most of the 68 predicted effector proteins lacked functional or conserved domains. Moreover, there are 26 effector proteins that were species-specific (Supplementary Table 7). In order to initially determine which ones cause host cell death, 20 effector protein genes were randomly selected and transiently expressed in Nicotiana benthamiana by Agrobacterium infiltration. The effectors sshi00003413 (E5) and sshi00010565 (E20) strongly induced cell death (Figure 9B and Supplementary Figure 5), suggesting that these two effector proteins may also cause cell death in the mulberry, thereby promoting the colonization and infection of pathogen. Both effector proteins have no conserved domains.
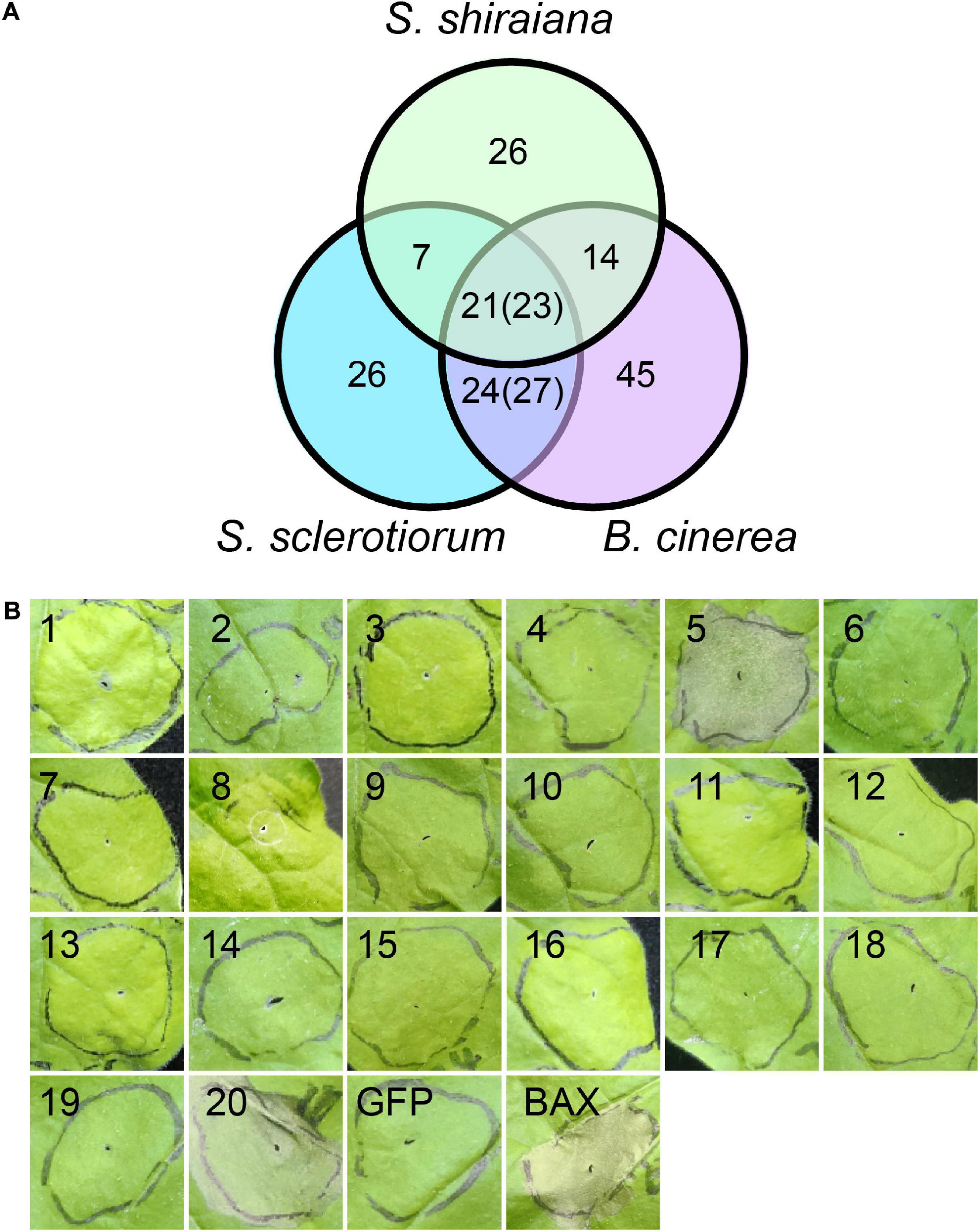
Figure 9. Prediction and analysis of effector proteins in Scleromitrula shiraiana. (A) Comparison of effectors predicted in S. shiraiana, Sclerotinia sclerotiorum, and Botrytis cinerea. Numbers in parentheses indicate that multiple effector proteins in B. cinerea are shared with S. sclerotiorum and/or S. shiraiana. (B) Identification of putative S. shiraiana effectors that can induce cell death in Nicotiana benthamiana. Leaves of 4–6-week-old N. benthamiana plants were infiltrated with Agrobacterium carrying S. shiraiana effector genes using a 1-ml needleless syringe (positive control, BAX; negative control, GFP). Cell death symptoms were photographed at 6 days after infiltration. Results are representative of three biological replicates.
Discussion
Mulberry sclerotial disease is the most destructive fungal disease of mulberry, and often causes high yield losses. S. shiraiana is one of the causal pathogens of mulberry sclerotial disease, and is also the one that is easiest to isolate and cultivate artificially. In addition, S. shiraiana is a necrotrophic pathogenic fungus with a restricted host range; it is only found on mulberry. In this study, we sequenced the genome of S. shiraiana and obtained a high-quality 39.0 Mb assembly with 21 contigs. The genome size and predicted gene number of S. shiraiana is similar to that of most other Ascomycetes, including the taxonomically closely related S. sclerotiorum and B. cinerea. S. shiraiana, S. sclerotiorum, and B. cinerea belong to the Sclerotiniaceae, but S. shiraiana may have emerged earlier from their common ancestor species. Navaud et al. (2018) divided Sclerotiniaceae into three major macro-evolutionary regimes according to the variation of diversification rate during the evolution, which have contrasted proportions of broad host range species. The fungi of the regime G1 including S. shiraiana are early diverging, and showed low diversification rates and low host jump frequencies resulted in a narrow host range (Navaud et al., 2018). The emergence of broad host range fungi (such as S. sclerotiorum in regime G2 and B. cinerea in regime G3) is largely driven by high jump frequency and probably combined with low diversification rate (Navaud et al., 2018). S. shiraiana shares some characteristics with S. sclerotiorum and B. cinerea. First, S. shiraiana and B. cinerea are heterothallic, while S. sclerotiorum is homothallic. Second, the sexual stages of S. shiraiana and S. sclerotiorum are critical to their life cycle, and the ascospores produced in their sexual process are the main primary infection source. However, the sexual stage of B. cinerea is very rare in nature (Dewey and Grant-Downton, 2016). Third, S. shiraiana and B. cinerea can produce conidiospores in the asexual stage, while S. sclerotiorum cannot (Saharan and Mehta, 2008). Fourth, in terms of genome composition, there are more transposable elements in the S. sclerotiorum genome than in the genomes of S. shiraiana and B. cinerea. Of course, S. shiraiana also has many differences from S. sclerotiorum and B. cinerea. First and foremost, S. shiraiana has a narrow host range, and it is likely to infect only Morus plants. The adaptation of S. shiraiana to mulberry restricted its infection to other potential hosts. In addition, the hyphae of S. shiraiana grow very slowly and easily produce a large amount of melanin in artificial culture medium. This suggests that S. shiraiana is weak in competitiveness and environmental adaptability. Another difference is that, B. cinerea depends on its vigorous conidia to infect plants. Although S. shiraiana also produces conidia, their role in its life cycle is dispensable or negligible. One of the reasons is that the mulberry flowering period is short and a very narrow time window is available for conidia reinfection. The other and the main reason is that the conidia of S. shiraiana are produced in a liquid environment, which severely limits its spread (Lü et al., 2017). This may also be one of the reasons that restrict S. shiraiana from jumping to and infecting other potential host plants.
The plant cell wall is a vital defense barrier and a main component of the defense monitoring system (Bacete et al., 2018). The plant cell wall is a heterogeneous structure composed of polysaccharides, proteins, and aromatic polymers. The main components of polysaccharides are cellulose, hemicellulose, and pectin. Degradation of plant cell wall polysaccharides is an important infection strategy for necrotrophs (Glass et al., 2013). In addition, degraded polysaccharides serve as their carbon sources. As shown in the comparative genomic analysis, there are fewer genes encoding carbohydrate-active enzymes in the S. shiraiana genome than in the genomes of other necrotrophs. Cellulase, hemicellulase, and pectinase target cellulose, hemicellulose, and pectin in the plant cell wall network complex, respectively. The number of genes encoding CWDEs in the S. shiraiana genome is significantly less than that of S. sclerotiorum, B. cinerea and restricted host range necrotrophic P. nodorum and P. teres f. teres. In fact, except for B. cinerea, most other Botrytis spp. have a narrow host range. The difference in the number of CWDEs of Botrytis spp. is closely related to the content of their host cell wall components (Valero-Jiménez et al., 2019). The initial colonization of S. shiraiana started from the stigma of decayed mulberry female flowers. The adaptation to mulberry allows S. shiraiana to successfully infect the host with less CWDEs. Our results show that cellulase and pectinase, rather than hemicellulase, significantly promote the infection of A. thaliana by S. shiraiana. However, this experiment is preliminary and has limitations. Because CWDEs pretreatment can enhance the susceptibility of plants to many microorganisms. So, we have not been able to determine the role of cellulase, pectinase, and even hemicellulase in the process of S. shiraiana infecting mulberries. The role of CWDEs in the colonization and pathogenicity of S. shiraiana needs further study.
Many plant pathogens, including bacteria, fungi, and oomycetes, secrete effector proteins that function in the apoplast or cytoplasm of host cells to suppress the immune response of plants (Macho and Zipfel, 2015; Toruno et al., 2016; Wang et al., 2019). Some isolates of M. oryzae can infect Oryza sativa japonica varieties because of their abundant effector proteins (Liao et al., 2016). We detected 68 predicted effector proteins in S. shiraiana, fewer than in S. sclerotiorum, B. cinerea, and other pathogens (Schirawski et al., 2010; O’connell et al., 2012; Yin et al., 2015; Derbyshire et al., 2017). The small number of CWDEs and effector proteins in S. shiraiana may be the one of the main reasons for its weak infectivity, which results in a narrow host range. However, some Botrytis spp. with a narrow host range and some regionally diverged isolates (such as S. sclerotiorum and B. cinerea) have specific effectors or abnormal numbers of CWDEs, which may have potentially undergone some level of host-specific adaptation (Mousavi-Derazmahalleh et al., 2019; Valero-Jiménez et al., 2019). Some reports indicate that S. sclerotiorum and other suspected pathogens are present on diseased mulberry fruit, but it is unknown whether these fungi cause mulberry sclerotial disease. Because S. sclerotiorum and B. cinerea often cause the rot of infected tissues, this is not conducive to the formation of their sclerotia in mulberries.
The comparative genomic analysis showed that one third of the genes encoding secondary metabolism core enzymes in S. shiraiana were species-specific. Different necrotrophic fungi secrete different types of phytotoxins to suppress the host immune response and promote disease. Botrydial produced by B. cinerea induces a hypersensitive response in the host plant, which contributes to pathogen infection (Rossi et al., 2011). ToxA, a host-specific toxin produced by Pyrenophora tritici-repentis and Stagonospora nodorum, enhances virulence against wheat (Faris et al., 2010). As a pathogen with a narrow host range, S. shiraiana may produce certain phytotoxins that make it more suitable for infecting mulberry.
In this study, we sequenced the necrotrophic pathogen that causes mulberry sclerotial disease, S. shiraiana. Usually, necrotrophic phytopathogenic fungi deploy phytotoxins, CWDEs, and effector proteins to cause plant cell necrosis and infect plants. However, the genome of S. shiraiana encodes far fewer CWDEs and effector proteins than do the genomes of closely related species. This may be one reason for the restricted host range of S. shiraiana. The differences in secondary metabolism genes between S. shiraiana and other Ascomycetes may be responsible its narrow host range. Although S. shiraiana has a small arsenal, it retains highly targeted virulence weapons for pathogenicity against mulberry.
Data Availability Statement
The datasets presented in this study can be found in online repositories. The names of the repository/repositories and accession number(s) can be found below: https://bigd.big.ac.cn/gwh, GWHACFG00000000.
Author Contributions
NH and ZL contributed to the conception and design of the work. ZL, ZH, LH, XK, and HL performed the experiments. ZH performed effector screening. ZL and LH performed cell wall degrading enzymes detection. XK performed ShSCD functional analysis. ZL, BM, YL, and JY analyzed the data. YL and JY contributed reagents and plant materials. ZL and ZH wrote the manuscript. NH, ZL, and BM produced the final version of the manuscript. All authors contributed to the article and approved the submitted version.
Funding
This work was funded by the National Key Research and Development Program (No. 2018YFD1000602), the National Natural Science Foundation of China (No. 31572323), and the Chongqing Postdoctoral Science Foundation (No. cstc2019jcyj-bshX0096).
Conflict of Interest
The authors declare that the research was conducted in the absence of any commercial or financial relationships that could be construed as a potential conflict of interest.
Supplementary Material
The Supplementary Material for this article can be found online at: https://www.frontiersin.org/articles/10.3389/fmicb.2020.603927/full#supplementary-material
Supplementary Figure 1 | Sequence alignment of products encoded by genes at MAT1-2 loci of Scleromitrula shiraiana SX-001, Sclerotinia sclerotiorum 1980, and Botrytis cinerea T4. (A,B), Sequence alignment of MAT1-2-1 and MAT1-2-4 in S. shiraiana SX-001, S. sclerotiorum 1980 and B. cinerea T4, respectively. Sequence identity of SshMAT1-2-1 vs. BcMAT1-2-1, SshMAT1-2-1 vs. SscMAT1-2-1 and SscMAT1-2-1 vs. BcMAT1-2-1 is 37.0%, 41.6%, and 77.0%, respectively. Sequence identity of SshMAT1-2-4 vs. BcMAT1-2-4, SshMAT1-2-4 vs. SscMAT1-2-4 and SscMAT1-2-4 vs. BcMAT1-2-4 is 29.1%, 32.1% and 65.1%, respectively. Ssh, S. shiraiana SX-001; Bc, B. cinerea T4; Ssc, S. sclerotiorum 1980.
Supplementary Figure 2 | Statistical analysis of orthogroups in Scleromitrula shiraiana SX-001, Sclerotinia sclerotiorum 1980, and Botrytis cinerea B05.10.
Supplementary Figure 3 | Southern blotting verification of ShSCD-deletion strains.
Supplementary Figure 4 | The tolerance test of ShSCD-deletion mutant to hydrogen peroxide. (A) High concentrations of hydrogen peroxide significantly inhibit the growth of ShSCD-deletion mutants. The colony inhibition rate = | DT - DC| /DC. DT, the colony diameter of treatment group. DC, the colony diameter of control group. Different letters (a and b) indicate statistical differences (p < 0.05). (B) The colony morphology of ShSCD-deletion mutants and wild-type strain on PDA medium containing different concentrations of hydrogen peroxide. Colony diameter measurement and colony photography were performed after 9 days of culture. The experiments were repeated at least three times.
Supplementary Figure 5 | The relative expression level of 20 effector genes transiently expressed in N. benthamiana.
Supplementary Table 1 | Information for fungal genome used in this study.
Supplementary Table 2 | Orthogroups gene count in 16 fungi.
Supplementary Table 3 | Statistics of orthogroups and genes per species.
Supplementary Table 4 | Coverage of Scleromitrula shiraiana sequences to those of Sclerotinia sclerotiorum and Botrytis cinerea.
Supplementary Table 5 | Secondary metabolism key enzymes encoding genes in Scleromitrula shiraiana, Sclerotinia sclerotiorum, and Botrytis cinerea.
Supplementary Table 6 | Comparison of plant and fungal cell wall-degrading enzymes between Scleromitrula shiraiana and 15 other fungi.
Supplementary Table 7 | Protein domain analysis of 68 putative effectors of Scleromitrula shiraiana.
Supplementary Table 8 | Primers used in this study.
Footnotes
References
Alghisi, P., and Favaron, F. (1995). Pectin-degrading enzymes and plant-parasite interactions. Eur. J. Plant Pathol. 101, 365–375. doi: 10.1007/bf01874850
Almagro Armenteros, J. J., Tsirigos, K. D., Sønderby, C. K., Petersen, T. N., Winther, O., Brunak, S., et al. (2019). SignalP 5.0 improves signal peptide predictions using deep neural networks. Nat. Biotechnol. 37, 420–423. doi: 10.1038/s41587-019-0036-z
Amselem, J., Cuomo, C. A., Van Kan, J. A. L., Viaud, M., Benito, E. P., Couloux, A., et al. (2011). Genomic analysis of the necrotrophic fungal pathogens Sclerotinia sclerotiorum and Botrytis cinerea. PLoS Genet. 7, e1002230.
Bacete, L., Melida, H., Miedes, E., and Molina, A. (2018). Plant cell wall-mediated immunity: cell wall changes trigger disease resistance responses. Plant J. 93, 614–636. doi: 10.1111/tpj.13807
Blin, K., Shaw, S., Steinke, K., Villebro, R., Ziemert, N., Lee, S. Y., et al. (2019). antiSMASH 5.0: updates to the secondary metabolite genome mining pipeline. Nucleic Acids Res. 47, W81–W87.
Bolton, M. D., Thomma, B. P. H. J., and Nelson, B. D. (2006). Sclerotinia sclerotiorum (Lib.) de Bary: biology and molecular traits of a cosmopolitan pathogen. Mole. Plant Pathol. 7, 1–16. doi: 10.1111/j.1364-3703.2005.00316.x
Brito, N., Espino, J. J., and González, C. (2006). The endo-β-1,4-xylanase Xyn11A is required for virulence in Botrytis cinerea. Mol. Plant Microb. Int. 19, 25–32. doi: 10.1094/mpmi-19-0025
Butler, M. J., and Day, A. W. (1998). Fungal melanins: a review. Can. J. Microb. 44, 1115–1136. doi: 10.1139/w98-119
Butler, M. J., Day, A. W., Henson, J. M., and Money, N. P. (2001). Pathogenic properties of fungal melanins. Mycologia 93, 1–8. doi: 10.2307/3761599
Butler, M. J., Gardiner, R. B., and Day, A. W. (2009). Melanin synthesis by Sclerotinia sclerotiorum. Mycologia 101, 296–304. doi: 10.3852/08-120
Cessna, S. G., Sears, V. E., Dickman, M. B., and Low, P. S. (2000). Oxalic acid, a pathogenicity factor for Sclerotinia sclerotiorum, suppresses the oxidative burst of the host plant. Plant cell 12, 2191–2200. doi: 10.2307/3871114
Chen, H., Pu, J., Liu, D., Yu, W., Shao, Y., Yang, G., et al. (2016). Anti-inflammatory and antinociceptive properties of flavonoids from the fruits of black mulberry (Morus nigra L.). PLoS One 11:e0153080. doi: 10.1371/journal.pone.0153080
Chen, H., Yu, W., Chen, G., Meng, S., Xiang, Z., and He, N. (2017). Antinociceptive and antibacterial properties of anthocyanins and flavonols from fruits of black and non-black mulberries. Molecules 23:4. doi: 10.3390/molecules23010004
Choi, J. W., Synytsya, A., Capek, P., Bleha, R., Pohl, R., and Park, Y. I. (2016). Structural analysis and anti-obesity effect of a pectic polysaccharide isolated from Korean mulberry fruit Oddi (Morus alba L.). Carbohydrate Poly. 146, 187–196. doi: 10.1016/j.carbpol.2016.03.043
Collado, I. G., and Viaud, M. (2016). “Secondary metabolism in Botrytis cinerea: combining genomic and metabolomic approaches,” in Botrytis – the fungus, the pathogen and its management in agricultural systems, eds S. Fillinger and Y. Elad (Cham: Springer International Publishing), 291–313. doi: 10.1007/978-3-319-23371-0_15
Colmenares, A. J., Aleu, J., Duran-Patron, R., Collado, I. G., and Hernandez-Galan, R. (2002). The putative role of botrydial and related metabolites in the infection mechanism of Botrytis cinerea. J. Chem. Ecol. 28, 997–1005.
Cotton, P., Kasza, Z., Bruel, C., Rascle, C., and Fèvre, M. (2003). Ambient pH controls the expression of endopolygalacturonase genes in the necrotrophic fungus Sclerotinia sclerotiorum. FEMS Microbiol. Lett. 227, 163–169. doi: 10.1016/s0378-1097(03)00582-2
Dalmais, B., Schumacher, J., Moraga, J. P. L. E. P., Tudzynski, B., Collado, I. G., and Viaud, M. (2011). The Botrytis cinerea phytotoxin botcinic acid requires two polyketide synthases for production and has a redundant role in virulence with botrydial. Mol. Plant Pathol. 12, 564–579. doi: 10.1111/j.1364-3703.2010.00692.x
Debuchy, R., Berteaux-Lecellier, V., and Silar, P. (2010). Mating systems and sexual morphogenesis in ascomycetes,” in Cellular and Molecular Biology of Filamentous Fungi. Am. Soc. Microb. 2010, 501–535.
Derbyshire, M., Denton-Giles, M., Hegedus, D., Seifbarghy, S., Rollins, J., Van Kan, J., et al. (2017). The complete genome sequence of the phytopathogenic fungus Sclerotinia sclerotiorum reveals insights into the genome architecture of broad host range pathogens. Genome Biol. Evol. 9, 593–618. doi: 10.1093/gbe/evx030
Dewey, M. F. M., and Grant-Downton, R. (2016). “Botrytis-biology, detection and quantification,” in Botrytis – the fungus, the pathogen and its management in agricultural systems, eds S. Fillinger and Y. Elad (Cham: Springer International Publishing), 17–34. doi: 10.1007/978-3-319-23371-0_2
Duplessis, S., Cuomo, C. A., Lin, Y.-C., Aerts, A., Tisserant, E., Veneault-Fourrey, C., et al. (2011). Obligate biotrophy features unraveled by the genomic analysis of rust fungi. Proc. Natl. Acad. Sci. 108, 9166–9171. doi: 10.1073/pnas.1019315108
Ebbole, D. J. (2007). Magnaporthe as a model for understanding host-pathogen interactions. Ann. Rev. Phytopathol. 45, 437–456. doi: 10.1146/annurev.phyto.45.062806.094346
Eisenhaber, B., Schneider, G., Wildpaner, M., and Eisenhaber, F. (2004). A sensitive predictor for potential GPI lipid modification sites in fungal protein sequences and its application to genome-wide studies for Aspergillus nidulans, Candida albicans, Neurospora crassa, Saccharomyces cerevisiae and Schizosaccharomyces pombe. J. Mole. Biol. 337, 243–253. doi: 10.1016/j.jmb.2004.01.025
Elad, Y., Pertot, I., Cotes Prado, A. M., and Stewart, A. (2016). “Plant hosts of Botrytis spp,” in Botrytis – the Fungus, the pathogen and its management in agricultural systems, eds S. Fillinger and Y. Elad (Cham: Sp), 413–486. doi: 10.1007/978-3-319-23371-0_20
Ellwood, S. R., Liu, Z., Syme, R. A., Lai, Z., Hane, J. K., Keiper, F., et al. (2010). A first genome assembly of the barley fungal pathogen Pyrenophora teres f. teres. Genome Biol. 11:R109.
Emms, D. M., and Kelly, S. (2017). STRIDE: species tree root inference from gene duplication events. Mole. Biol. Evol. 34, 3267–3278. doi: 10.1093/molbev/msx259
Emms, D. M., and Kelly, S. (2018). STAG: species tree inference from all genes. bioRxiv. doi: 10.1101/267914
Emms, D. M., and Kelly, S. (2019). OrthoFinder: phylogenetic orthology inference for comparative genomics. Genome Biol. 20:238.
Faretra, F., Antonacci, E., and Pollastro, S. (1988). Sexual behavior and mating system of Botryotinia fuckeliana, teleomorph of Botrytis cinerea. J. Gen. Microbiol. 134, 2543–2550. doi: 10.1099/00221287-134-9-2543
Faris, J. D., and Friesen, T. L. (2020). Plant genes hijacked by necrotrophic fungal pathogens. Curr. Opin. Plant Biol. 56, 74–80. doi: 10.1016/j.pbi.2020.04.003
Faris, J. D., Zhang, Z., Lu, H., Lu, S., Reddy, L., Cloutier, S., et al. (2010). A unique wheat disease resistance-like gene governs effector-triggered susceptibility to necrotrophic pathogens. Proc. Natl. Acad. Sci. USA 107, 13544–13549. doi: 10.1073/pnas.1004090107
Favaron, F., Sella, L., and D’ovidio, R. (2004). Relationships among endo-polygalacturonase, oxalate, pH, and plant polygalacturonase-inhibiting protein (PGIP) in the interaction between Sclerotinia sclerotiorum and Soybean. Mol. Plant Microb. Int. 17, 1402–1409. doi: 10.1094/mpmi.2004.17.12.1402
Glass, N. L., Schmoll, M., Cate, J. H., and Coradetti, S. (2013). Plant cell wall deconstruction by ascomycete fungi. Ann. Rev. Microbiol. 67, 477–498. doi: 10.1146/annurev-micro-092611-150044
Grabherr, M. G., Haas, B. J., Yassour, M., Levin, J. Z., Thompson, D. A., Amit, I., et al. (2011). Full-length transcriptome assembly from RNA-Seq data without a reference genome. Nat. Biotechnol. 29, 644–652. doi: 10.1038/nbt.1883
Han, Y., Joosten, H.-J., Niu, W., Zhao, Z., Mariano, P. S., Mccalman, M., et al. (2007). Oxaloacetate hydrolase, the C–C bond lyase of oxalate secreting fungi. J. Biol. Chem. 282, 9581–9590. doi: 10.1074/jbc.m608961200
Hane, J. K., Lowe, R. G. T., Solomon, P. S., Tan, K.-C., Schoch, C. L., Spatafora, J. W., et al. (2007). Dothideomycete–plant interactions illuminated by genome sequencing and EST analysis of the wheat pathogen Stagonospora nodorum. Plant cell 19, 3347–3368. doi: 10.1105/tpc.107.052829
Henson, J. M., Butler, M. J., and Day, A. W. (1999). The dark side of the mycelium: melanins of phytopathogenic fungi. Ann. Rev. Phytopathol. 37, 447–471. doi: 10.1146/annurev.phyto.37.1.447
Holt, C., and Yandell, M. (2011). MAKER2: an annotation pipeline and genome-database management tool for second-generation genome projects. BMC Bioinform. 12:491.
Hong, S. K., Kim, W. G., Sung, G. B., and Nam, S. H. (2007). Identification and distribution of two fungal species causing sclerotial disease on mulberry fruits in Korea. Mycobiology 35, 87–90. doi: 10.4489/myco.2007.35.2.087
Jacobson, E. S. (2000). Pathogenic roles for fungal melanins. Clin. Microbiol. Rev. 13, 708–717. doi: 10.1128/cmr.13.4.708
Kars, I., Krooshof, G. H., Wagemakers, L., Joosten, R., Benen, J. A. E., and Van Kan, J. A. L. (2005). Necrotizing activity of five Botrytis cinerea endopolygalacturonases produced in Pichia pastoris. Plant J. 43, 213–225. doi: 10.1111/j.1365-313x.2005.02436.x
Keller, O., Kollmar, M., Stanke, M., and Waack, S. (2011). A novel hybrid gene prediction method employing protein multiple sequence alignments. Bioinformatics 27, 757–763. doi: 10.1093/bioinformatics/btr010
Kim, K. S., Min, J.-Y., and Dickman, M. B. (2008). Oxalic acid is an elicitor of plant programmed cell death during Sclerotinia sclerotiorum disease development. Mol. Plant Microb. Int. 21, 605–612. doi: 10.1094/mpmi-21-5-0605
Krogh, A., Larsson, B., Von Heijne, G., and Sonnhammer, E. L. L. (2001). Predicting transmembrane protein topology with a hidden markov model: application to complete genomes. J. Mole. Biol. 305, 567–580. doi: 10.1006/jmbi.2000.4315
Kubicek, C. P., Starr, T. L., and Glass, N. L. (2014). Plant cell wall–degrading enzymes and their secretion in plant-pathogenic fungi. Ann. Rev. Phytopathol. 52, 427–451. doi: 10.1146/annurev-phyto-102313-045831
Liang, X., Liberti, D., Li, M., Kim, Y.-T., Hutchens, A., Wilson, R., et al. (2015). Oxaloacetate acetylhydrolase gene mutants of Sclerotinia sclerotiorum do not accumulate oxalic acid, but do produce limited lesions on host plants. Mol. Plant Pathol. 16, 559–571. doi: 10.1111/mpp.12211
Liang, Y., Xiong, W., Steinkellner, S., and Feng, J. (2018). Deficiency of the melanin biosynthesis genes SCD1 and THR1 affects sclerotial development and vegetative growth, but not pathogenicity, in Sclerotinia sclerotiorum. Mol. Plant Pathol. 19, 1444–1453. doi: 10.1111/mpp.12627
Liao, J., Huang, H., Meusnier, I., Adreit, H., Ducasse, A., Bonnot, F., et al. (2016). Pathogen effectors and plant immunity determine specialization of the blast fungus to rice subspecies. eLife 5:e19377.
Liu, G. Y., and Nizet, V. (2009). Color me bad: microbial pigments as virulence factors. Trends Microbiol. 17, 406–413. doi: 10.1016/j.tim.2009.06.006
Lo Presti, L., and Kahmann, R. (2017). How filamentous plant pathogen effectors are translocated to host cells. Curr. Opin. Plant Biol. 38, 19–24. doi: 10.1016/j.pbi.2017.04.005
Lombard, V., Golaconda Ramulu, H., Drula, E., Coutinho, P. M., and Henrissat, B. (2013). The carbohydrate-active enzymes database (CAZy) in 2013. Nucleic Acids Res. 42, D490–D495.
Lü, Z., Kang, X., Xiang, Z., and He, N. (2017). Laccase gene Sh-lac is involved in the growth and melanin biosynthesis of Scleromitrula shiraiana. Phytopathology 107, 353–361. doi: 10.1094/phyto-04-16-0180-r
Lv, Z., Huang, Y., Ma, B., Xiang, Z., and He, N. (2018). LysM1 in MmLYK2 is a motif required for the interaction of MmLYP1 and MmLYK2 in the chitin signaling. Plant Cell Rep. 37, 1101–1112. doi: 10.1007/s00299-018-2295-4
Macho, A. P., and Zipfel, C. (2015). Targeting of plant pattern recognition receptor-triggered immunity by bacterial type-III secretion system effectors. Curr. Opin. Microbiol. 23, 14–22. doi: 10.1016/j.mib.2014.10.009
Malvárez, G., Carbone, I., Grünwald, N. J., Subbarao, K. V., Schafer, M., and Kohn, L. M. (2007). New populations of Sclerotinia sclerotiorum from lettuce in California and peas and lentils in Washington. Phytopathology 97, 470–483. doi: 10.1094/phyto-97-4-0470
Marcos, F., Celedonio, G., and Nélida, B. (2011). BcSpl1, a cerato-platanin family protein, contributes to Botrytis cinerea virulence and elicits the hypersensitive response in the host. N. Phytol. 192, 483–495. doi: 10.1111/j.1469-8137.2011.03802.x
Marcos, F., Nélida, B., and Celedonio, G. (2013). The Botrytis cinerea cerato-platanin BcSpl1 is a potent inducer of systemic acquired resistance (SAR) in tobacco and generates a wave of salicylic acid expanding from the site of application. Mole. Plant Pathol. 14, 191–196. doi: 10.1111/j.1364-3703.2012.00842.x
Mardanov, A. V., Beletsky, A. V., Kadnikov, V. V., Ignatov, A. N., and Ravin, N. V. (2014). Draft genome sequence of Sclerotinia borealis, a psychrophilic plant pathogenic fungus. Genome Announc. 2, e1175–e1113.
Mengiste, T. (2012). Plant immunity to necrotrophs. Ann. Rev. Phytopathol. 50, 267–294. doi: 10.1146/annurev-phyto-081211-172955
Mousavi-Derazmahalleh, M., Chang, S., Thomas, G., Derbyshire, M., Bayer, P. E., Edwards, D., et al. (2019). Prediction of pathogenicity genes involved in adaptation to a lupin host in the fungal pathogens Botrytis cinerea and Sclerotinia sclerotiorum via comparative genomics. BMC Genom. 20:385.
Navaud, O., Barbacci, A., Taylor, A., Clarkson, J. P., and Raffaele, S. (2018). Shifts in diversification rates and host jump frequencies shaped the diversity of host range among Sclerotiniaceae fungal plant pathogens. Mol. Ecol. 27, 1309–1323. doi: 10.1111/mec.14523
O’connell, R. J., Thon, M. R., Hacquard, S., Amyotte, S. G., Kleemann, J., Torres, M. F., et al. (2012). Lifestyle transitions in plant pathogenic Colletotrichum fungi deciphered by genome and transcriptome analyses. Nat. Genet. 44:1060.
Pan, Y., Wei, J., Yao, C., Reng, H., and Gao, Z. (2018). SsSm1, a Cerato-platanin family protein, is involved in the hyphal development and pathogenic process of Sclerotinia sclerotiorum. Plant Sci. 270, 37–46. doi: 10.1016/j.plantsci.2018.02.001
Pazzagli, L., Seidl-Seiboth, V., Barsottini, M., Vargas, W. A., Scala, A., and Mukherjee, P. K. (2014). Cerato-platanins: elicitors and effectors. Plant Sci. 228, 79–87. doi: 10.1016/j.plantsci.2014.02.009
Presti, L. L., Lanver, D., Schweizer, G., Tanaka, S., Liang, L., Tollot, M., et al. (2015). Fungal effectors and plant susceptibility. Ann. Rev. Plant Biol. 66, 513–545. doi: 10.1146/annurev-arplant-043014-114623
Qi, G., Chen, J., Chang, M., Chen, H., Hall, K., Korin, J., et al. (2018). Pandemonium breaks out: disruption of salicylic acid-mediated defense by plant pathogens. Mole. Plant 11, 1427–1439. doi: 10.1016/j.molp.2018.10.002
Qi, X., Shuai, Q., Chen, H., Fan, L., Zeng, Q., and He, N. (2014). Cloning and expression analyses of the anthocyanin biosynthetic genes in mulberry plants. Mole. Genet. Genom. 289, 783–793. doi: 10.1007/s00438-014-0851-3
Riou, C., Freyssinet, G., and Fevre, M. (1991). Production of cell wall-degrading enzymes by the phytopathogenic fungus Sclerotinia sclerotiorum. Appl. Environ. Microbiol. 57, 1478–1484. doi: 10.1128/aem.57.5.1478-1484.1991
Rollins, J. A. (2003). The Sclerotinia sclerotiorum pac1 gene is required for sclerotial development and virulence. Mole. Plant-Microbe Int. 16, 785–795. doi: 10.1094/mpmi.2003.16.9.785
Rossi, F. R., Garriz, A., Marina, M., Romero, F. M., Gonzalez, M. E., Collado, I. G., et al. (2011). The sesquiterpene botrydial produced by Botrytis cinerea induces the hypersensitive response on plant tissues and its action is modulated by salicylic acid and jasmonic acid signaling. Mol. Plant Microb. Int. 24, 888–896. doi: 10.1094/mpmi-10-10-0248
Saharan, G. S., and Mehta, N. (2008). The Pathogen – Sclerotinia in Sclerotinia diseases of crop plants: biology, ecology and disease management. Dordrecht: Springer, 77–111.
Schirawski, J., Mannhaupt, G., Münch, K., Brefort, T., Schipper, K., Doehlemann, G., et al. (2010). Pathogenicity determinants in smut fungi revealed by genome comparison. Science 330, 1546–1548. doi: 10.1126/science.1195330
Schumacher, T., and Holst-Jensen, A. (1997). A synopsis of the genus Scleromitrula (=Verpatinia) (Ascomycotina: Helotiales: Sclerotiniaceae). Mycoscience 38, 55–69. doi: 10.1007/bf02464969
Schwessinger, B., Sperschneider, J., Cuddy, W. S., Garnica, D. P., Miller, M. E., Taylor, J. M., et al. (2018). A near-complete haplotype-phased genome of the dikaryotic wheat stripe rust fungus Puccinia striiformis f. sp. tritici reveals high interhaplotype diversity. mBio 9, e2275–e2217.
Spanu, P. D., Abbott, J. C., Amselem, J., Burgis, T. A., Soanes, D. M., Stüber, K., et al. (2010). Genome expansion and gene loss in powdery mildew fungi reveal tradeoffs in extreme parasitism. Science 330, 1543–1546.
Sperschneider, J., Dodds, P. N., Gardiner, D. M., Singh, K. B., and Taylor, J. M. (2018). Improved prediction of fungal effector proteins from secretomes with EffectorP 2.0. Mol. Plant Pathol. 19, 2094–2110. doi: 10.1111/mpp.12682
Tani, H., Koshino, H., Sakuno, E., Cutler, H. G., and Nakajima, H. (2006). Botcinins E and F and botcinolide from Botrytis cinerea and structural revision of botcinolides. J. Nat. Produc. 69, 722–725. doi: 10.1021/np060071x
Ter-Hovhannisyan, V., Lomsadze, A., Chernoff, Y. O., and Borodovsky, M. (2008). Gene prediction in novel fungal genomes using an ab initio algorithm with unsupervised training. Genome Res. 18, 1979–1990. doi: 10.1101/gr.081612.108
Toruno, T. Y., Stergiopoulos, I., and Coaker, G. (2016). Plant-pathogen effectors: cellular probes interfering with plant defenses in spatial and temporal manners. Annu. Rev. Phytopathol. 54, 419–441. doi: 10.1146/annurev-phyto-080615-100204
Umesha, S., Manukumar, H. M., and Raghava, S. (2016). A rapid method for isolation of genomic DNA from food-borne fungal pathogens. 3 Biotech 6:123.
Urban, M., Cuzick, A., Rutherford, K., Irvine, A., Pedro, H., Pant, R., et al. (2016). PHI-base: a new interface and further additions for the multi-species pathogen–host interactions database. Nucleic Acids Res. 45, D604–D610.
Valero-Jiménez, C. A., Veloso, J., Staats, M., and Van Kan, J. A. L. (2019). Comparative genomics of plant pathogenic Botrytis species with distinct host specificity. BMC Genomics 20:203.
Van Kan, J. A. L., Stassen, J. H. M., Mosbach, A., Van Der Lee, T.a.J, Faino, L., Farmer, A. D., et al. (2017). A gapless genome sequence of the fungus Botrytis cinerea. Mol. Plant Pathol. 18, 75–89. doi: 10.1111/mpp.12384
Vlot, A. C., Dempsey, D. M. A., and Klessig, D. F. (2009). Salicylic acid, a multifaceted hormone to combat disease. Ann. Rev. Phytopathol. 47, 177–206. doi: 10.1146/annurev.phyto.050908.135202
Wang, Y., Tyler, B. M., and Wang, Y. (2019). Defense and counterdefense during plant-pathogenic oomycete infection. Ann. Rev. Microbiol. 73, 667–696. doi: 10.1146/annurev-micro-020518-120022
Whetzel, H. H., and Wolf, F. A. (1945). The cup fungus, Ciboria carunculoides, pathogenic on mulberry fruits. Mycologia 37, 476–491. doi: 10.2307/3754633
Williams, B., Kabbage, M., Kim, H.-J., Britt, R., and Dickman, M. B. (2011). Tipping the balance: Sclerotinia sclerotiorum secreted oxalic acid suppresses host defenses by manipulating the host redox environment. PLoS Pathogens 7:e1002107. doi: 10.1371/journal.ppat.1002107
Williamson, B., Tudzynski, B., Tudzynski, P., and Van Kan, J. A. L. (2007). Botrytis cinerea: the cause of grey mould disease. Mol. Plant Pathol. 8, 561–580. doi: 10.1111/j.1364-3703.2007.00417.x
Xu, L., Xiang, M., White, D., and Chen, W. (2015). pH dependency of sclerotial development and pathogenicity revealed by using genetically defined oxalate-minus mutants of Sclerotinia sclerotiorum. Environ. Microbiol. 17, 2896–2909. doi: 10.1111/1462-2920.12818
Yang, G., Tang, L., Gong, Y., Xie, J., Fu, Y., Jiang, D., et al. (2018). A cerato-platanin protein SsCP1 targets plant PR1 and contributes to virulence of Sclerotinia sclerotiorum. N. Phytolog. 217, 739–755. doi: 10.1111/nph.14842
Yin, Z., Liu, H., Li, Z., Ke, X., Dou, D., Gao, X., et al. (2015). Genome sequence of Valsa canker pathogens uncovers a potential adaptation of colonization of woody bark. N. Phytolog. 208, 1202–1216. doi: 10.1111/nph.13544
Yu, Y., Xiao, J., Du, J., Yang, Y., Bi, C., and Qing, L. (2016). Disruption of the gene encoding endo-β-1, 4-xylanase affects the growth and virulence of Sclerotinia sclerotiorum. Front. Microbiol. 7:1787.
Zhang, H., Yohe, T., Huang, L., Entwistle, S., Wu, P., Yang, Z., et al. (2018). dbCAN2: a meta server for automated carbohydrate-active enzyme annotation. Nucleic Acids Res. 46, W95–W101.
Keywords: mulberry sclerotial disease, fungal genomics, necrotrophic fungus, secondary metabolism, cell wall-degrading enzymes, effector
Citation: Lv Z, He Z, Hao L, Kang X, Ma B, Li H, Luo Y, Yuan J and He N (2021) Genome Sequencing Analysis of Scleromitrula shiraiana, a Causal Agent of Mulberry Sclerotial Disease With Narrow Host Range. Front. Microbiol. 11:603927. doi: 10.3389/fmicb.2020.603927
Received: 08 September 2020; Accepted: 16 December 2020;
Published: 14 January 2021.
Edited by:
Hossein Borhan, Agriculture and Agri-Food Canada (AAFC), CanadaReviewed by:
Jose Sebastian Rufian, Shanghai Institutes for Biological Sciences, Chinese Academy of Sciences (CAS), ChinaStefan Kusch, RWTH Aachen University, Germany
Copyright © 2021 Lv, He, Hao, Kang, Ma, Li, Luo, Yuan and He. This is an open-access article distributed under the terms of the Creative Commons Attribution License (CC BY). The use, distribution or reproduction in other forums is permitted, provided the original author(s) and the copyright owner(s) are credited and that the original publication in this journal is cited, in accordance with accepted academic practice. No use, distribution or reproduction is permitted which does not comply with these terms.
*Correspondence: Ningjia He, aGVqaWFAc3d1LmVkdS5jbg==