- 1Institute of Engineering Biology and Health, Collaborative Innovation Center of Yangtze River Delta Region Green Pharmaceuticals, College of Pharmaceutical Sciences, Zhejiang University of Technology, Hangzhou, China
- 2Lab of Biosystems and Microanalysis, State Key Laboratory of Bioreactor Engineering, East China University of Science and Technology, Shanghai, China
Mycobacterium tuberculosis is a global human pathogen that infects macrophages and can establish a latent infection. Emerging evidence has established the nutrients metabolism as a key point to study the pathogenesis of M. tuberculosis and host immunity. It was reported that fatty acids and cholesterol are the major nutrient sources of M. tuberculosis in the period of infection. However, the mechanism by which M. tuberculosis utilizes lipids for maintaining life activities in nutrient-deficiency macrophages is poorly understood. Mycobacterium smegmatis is fast-growing and generally used to study its pathogenic counterpart, M. tuberculosis. In this work, we found that the phosphate sensing regulator RegX3 of M. smegmatis is required for its growing on propionate and surviving in macrophages. We further demonstrated that the expression of prpR and related genes (prpDBC) in methylcitrate cycle could be enhanced by RegX3 in response to the phosphate-starvation condition. The binding sites of the promoter region of prpR for RegX3 and PrpR were investigated. In addition, cell morphology assay showed that RegX3 is responsible for cell morphological elongation, thus promoting the proliferation and survival of M. smegmatis in macrophages. Taken together, our findings revealed a novel transcriptional regulation mechanism of RegX3 on propionate metabolism, and uncovered that the nutrients-sensing regulatory system puts bacteria at metabolic steady state by altering cell morphology. More importantly, since we observed that M. tuberculosis RegX3 also binds to the prpR operon in vitro, the RegX3-mediated regulation might be general in M. tuberculosis and other mycobacteria for nutrient sensing and environmental adaptation.
Introduction
Tuberculosis, a high lethality illness induced by Mycobacterium tuberculosis (M. tuberculosis), is responsible for millions of deaths every year. As extremely successful intracellular pathogen, M. tuberculosis exerts a latent infection in macrophages by a series of adaptive mechanisms. These strategies enable M. tuberculosis to quickly adapt to environmental changes, including the acidic conditions (pH 5.8-6.2), the nutrient depleted and hypoxic center of granulomas (Sturgill-Koszycki et al., 1994; Russell et al., 2010; Flynn et al., 2011). Notably, two-component regulatory systems (TCRSs) are the major adaptive mechanisms that affect global regulation in response to the specific environmental stimulus, thereby contributing to the long-term survival of M. tuberculosis within host cells (Rickman et al., 2004). Genomic analysis of M. tuberculosis has identified eleven kinds of TCRSs as well as several unpaired HKs and RRs. It was reported that some of these components are crucial for the adaptive responses under stress conditions and the pathogen virulence of M. tuberculosis (James et al., 2012).
SenX3-RegX3 system is the first identified TCRS in M. tuberculosis (Wren et al., 1992). Like other highly conserved TCRS, Mycobacteria SenX3-RegX3 is an auto-regulation operon and consist of a sensor (senX3) and its regulator regX3 (Himpens et al., 2000). When sensor protein detects environmental signals, the conserved histidine is self-phosphorylated and then transfers a phosphoryl group to response regulator to activate its DNA-binding capability, thus leading to the induction or suppression of target genes (Stock and Guhaniyogi, 2006). Interestingly, the intergenic region (IR) between senX3 and regX3 consists of several mycobacterial interspersed repetitive units (MIRUs), which may not only participate in regulation of gene expression by stabilizing upstream mRNA and controlling translation of downstream genes within a polycistronic operon, but also modulate host–pathogen interactions (Rifat and Karakousis, 2014). It was found that M. tuberculosis senX3-regX3 null mutant in infected macrophages and a mice model showed an apparent decline. Consequently, SenX3-RegX3 system is essential for M. tuberculosis virulence, and also indispensable for bacillary survival in host cells and lungs (Parish et al., 2003; Rickman et al., 2004; Rifat et al., 2009). More importantly, SenX3-RegX3 is a regulator of the genes referred to phosphate uptake in Mycobacterium. In this system, the dual roles of SenX3 as a phosphatase or phosphate donor of response regulator RegX3 depend on phosphate availability, while inorganic phosphate assimilation, regulated by the inorganic phosphate concentration within environment, is required for Mycobacterium growth and infection (Glover et al., 2007; Guo et al., 2009; Tischler et al., 2013). It is noticed that senX3-regX3 expression is not activated when dealing with a wide range of stress conditions like extremes of the pH scale, antibiotics and hydrogen peroxide, but is significantly increased in the case of low phosphorus. Therefore, SenX3-RegX3 controls the genes expression of Phosphate dose-dependence in Mycobacterium and is vital for optimum growth under phosphate-restricted condition (Glover et al., 2007). Moreover, it was reported that M. tuberculosis relies on a Pst system to regulate RegX3 activity in response to the available phosphate in vivo. The inorganic phosphate, a common phosphate source for bacteria to replicate in lungs, is a crucial signal regulating the M. tuberculosis gene expression through the Pst-SenX3-RegX3 signal transduction system (Tischler et al., 2013). Microarray analysis showed that SenX3-RegX3 regulated nearly 100 genes in M. tuberculosis, which were related to large numbers of important processes, including biosynthesis of biological macromolecules (DNA and RNA), oxidative stress, synthesis of PE/PPE family proteins, cell wall synthesis, fatty acid degradation and toxicity (Guo et al., 2009). The observation indicated that RegX3 might be a potential global regulator in mycobacteria.
Emerging evidence has demonstrated that M. tuberculosis utilizes fatty acids and cholesterol as main carbon sources during infection (Bloch and Segal, 1956; Mckinney et al., 2000; Schnappinger et al., 2003; Lee et al., 2017). In Mycobacterium metabolism, fatty acids enter the bacteria via membrane free diffusion and relevant transporters, and then go into β-oxidation cycle for gradual breakdown (Munoz-Elias and McKinney, 2006). Through continuous cycles of β-oxidation, even-chain and odd-chain fatty acids were converted into acetyl-coenzyme A (acetyl-CoA) and propionyl-coenzyme A (propionyl-CoA), which could be further oxidized through glyoxylate shunt and methylcitrate cycle, respectively (Rhee et al., 2011; Anthony and Kyu, 2014). It should be emphasized that methylcitrate cycle makes great contribution to the Mycobacterium growth in propionate and odd-chain fatty acids, since the propionate accumulated in environment and the propionate metabolites generated by β-oxidation are toxic, thus significantly inhibiting bacterial growth. The methylcitrate cycle can convert propionate into propionyl-CoA, which is subsequently oxidized into pyruvate by three specific enzymes, 2-methylisocitrate lyase (PrpB), methylcitrate synthase (PrpC) and methylcitrate dehydratase (PrpD) (Munoz-Elias and McKinney, 2006; Upton and McKinney, 2007; Puckett et al., 2017). Moreover, a novel transcription factor PrpR has been first described by Datta et al. and is important for the metabolism of odd-chain fatty acids and cholesterol in M. tuberculosis (Datta et al., 2011). PrpR can directly regulate the encoding genes for methylcitrate (prpC, prpD) and glyoxylate (isocitrate lyase, Icl1) circulations (Masiewicz et al., 2012). When utilizing propionate as the sole carbon source, the prpR-deletion strain of M. tuberculosis exhibited a compromised growth in vitro. PrpR activates the transcription of prpDC and icl1 genes, but also negatively regulates ramB, the prpR homolog controlling glyoxylate cycle. Furthermore, it has been underlined that M. tuberculosis prpR is crucial for the bacterial growth and survival in hypoxic hollow fiber model in mice (Klinkenberg et al., 2008).
The current studies about RegX3 mainly focus on its regulation of phosphorus metabolism in M. tuberculosis. However, the regulation effects of RegX3 on other physiological processes were poorly understood. A previous study uncovered a regulatory role of RegX3 in the expression of prpC in M. tuberculosis, suggesting that RegX3 might regulate the methylcitrate cycle (Roberts et al., 2011). In this work, we expanded the research scope of RegX3 regulation and explored its influence on carbon metabolism route. Due to the high pathogenicity and long growth cycle of M. tuberculosis, we selected its fast-growing counterpart Mycobacterium smegmatis (M. smegmatis) as the experimental object. M. smegmatis, a common model used for rapid screening of new antituberculotic drugs, is an atypical mycobacterium with high-speed proliferation and no pathogenicity (Sweeney et al., 2011). Using this model, we found that RegX3 is necessary for the M. smegmatis survival and growth in propionate. RegX3 directly up-regulated PrpR to promote the transcription of prpC, prpD and prpB encoding key enzymes of methylcitrate, thereby activating the whole methylcitrate cycle. This regulation mode also responds to the environmental phosphorus concentration and relies on the phosphorus availability. Furthermore, it was indicated that RegX3 promoted M. smegmatis proliferation and survival by changing the strain morphology in macrophages. Our data thus establishes a comprehensive regulatory network mediated by RegX3, and reveals the metabolic status of fatty acid intermediate metabolites in M. smegmatis.
Results
RegX3 Promotes the Growth of M. smegmatis on Propionate
To investigate the role of the MSMEG_0937-encoded RegX3 in M. smegmatis propionate metabolism, we constructed a regX3 knock-down strain by the CRISPR interference (CRISPRi)-based genetic repression, as well as a regX3 overexpression strain. Changes in regX3 transcription levels of the engineered stains were measured (Supplementary Figure 1). The growth curves of M. smegmatis wild-type (WT), the knock-down strain (i regX3) and the overexpression strain (OE regX3) in MOPS-propionate (10 mM) minimal medium with 100 μM K2HPO4 as its phosphorus source were measured. As shown in Figure 1A, the differences were observed among the growth of three strains. Compared to WT, the biomass of OE regX3 increased to a nearly 1.5-fold after 24 h, while i regX3 hardly grew on propionate. However, the three strains of M. smegmatis exhibited similar growth curves when they were cultured in 10 mM glucose (as sole carbon source) or 10 mM K2HPO4 (as higher phosphate condition) (Figures 1B,C). These data indicated an essential role of RegX3 in the propionate catabolism, which is consistent with the previous findings that RegX3 is involved in the regulation of fatty acid metabolism (Parish et al., 2003).
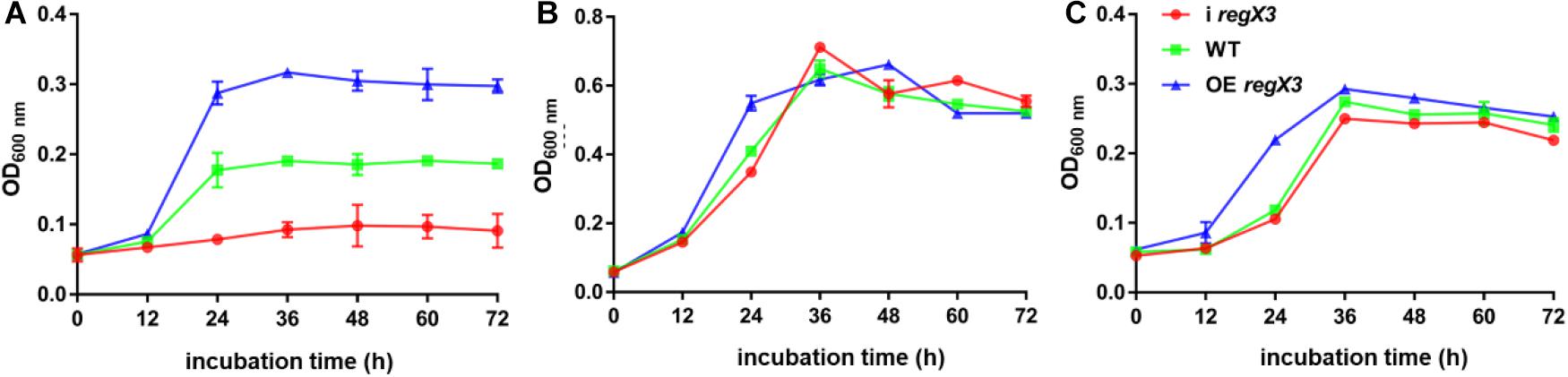
Figure 1. Growth curves of diverse M. smegmatis strains grown under different conditions. The three M. smegmatis, WT, i regX3, and OE regX3, were cultured in panel (A) MOPS-propionate (10 mM) minimal medium with low concentration phosphate (100 μM K2HPO4), (B) MOPS-glucose (10 mM) minimal medium with a low concentration of phosphate (100 μM K2HPO4) and (C) MOPS-propionate (10 mM) minimal medium with a high concentration of phosphate (10 mM K2HPO4), respectively. The mean of OD600 nm was calculated at each time point. Data are presented as means ± SEM calculated from three independent experiments.
Mycobacterium smegmatis RegX3 Binds to the Promoter Region of prpR Gene
Next, we sought to explore the underlying mechanism by which RegX3 promotes M. smegmatis growth on propionate. Electrophoretic mobility shift assay (EMSA) showed that recombinant RegX3 of M. smegmatis specifically bound to the upstream promoter region of prpR (MSMEG_6643) gene in vitro (Figures 2A,B). Since PrpR is a transcription factor referred to the regulation of methylcitrate route in M. smegmatis (Masiewicz et al., 2012), we suspected that RegX3 affects the whole methylcitrate cycle in M. smegmatis by regulating prpR gene. In addition, the binding of RegX3 to the promoter region of prpR gene in M. tuberculosis was validated (Supplementary Figure 2), indicating that the RegX3-mediated transcriptional regulation of methylcitrate cycle might be conserved in M. tuberculosis.
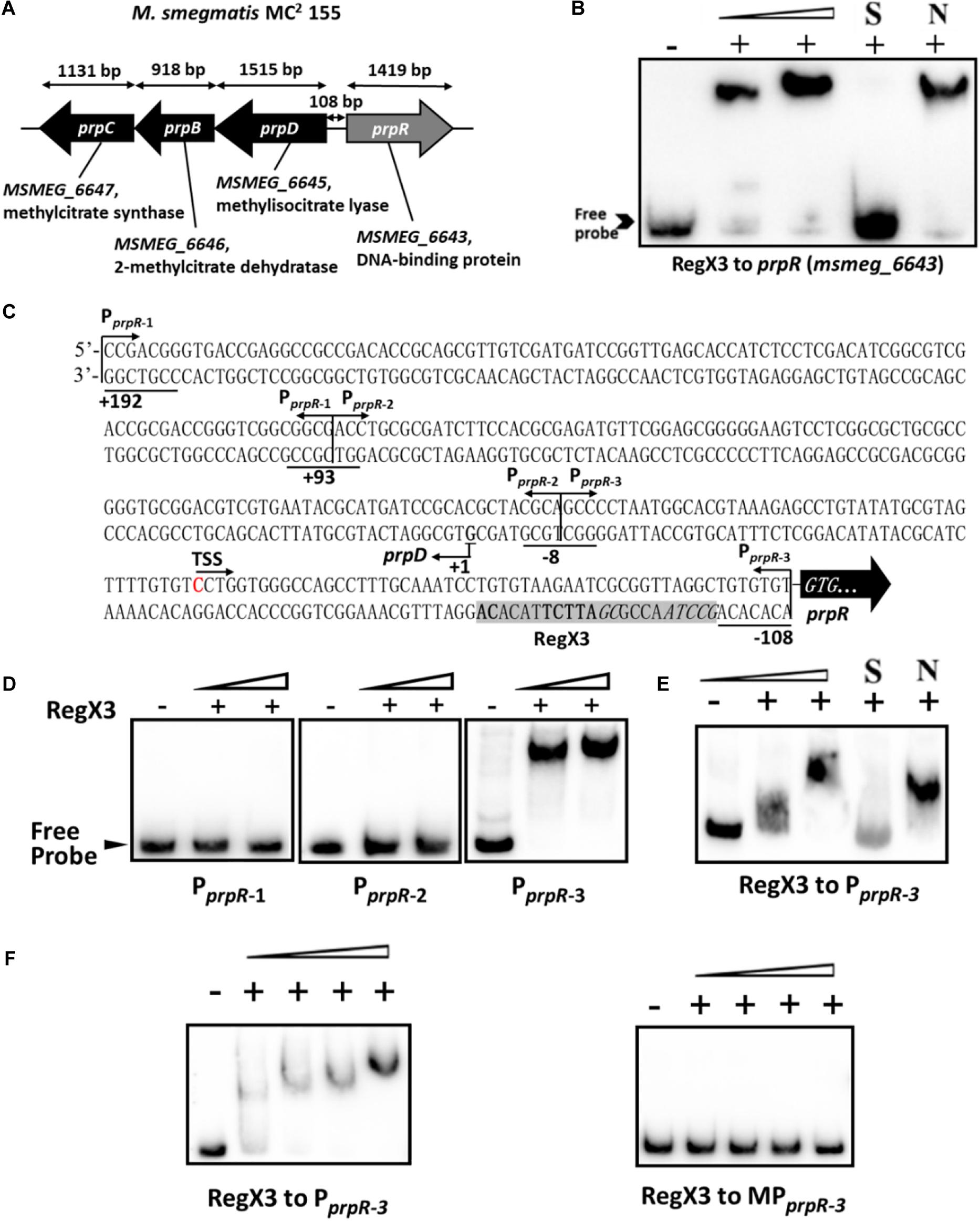
Figure 2. Binding of RegX3 to the regulatory region of the genes related to the methylcitrate cycle. (A) The schematic depiction of prpDBC (msmeg_6645-6647) and prpR (msmeg_6643) regions on the M. smegmatis MC2 155 chromosome. The open reading frame prpR is located in close proximity to the prpDBC operon. (B) EMSA showing the binding of His-RegX3 with the promoter of prpR. The biotin-labeled probe, a 300-bp DNA fragment corresponding to the upstream region (-300 to 0 bp) of prpR, was co-incubated with different concentrations of recombinant His-RegX3 protein (0, 0.6, and 1.2 μM). S represents the unlabeled specific probe, N represents the non-specific competitor DNA (Salmon sperm DNA). (C) Putative binding site (shadowed) of RegX3 in the promoter regions of prpR. The +192, +93, -8, and -108 positions are underlined; the three short fragments (PprpR–1, PprpR–2, and PprpR–3) and TSS (transcriptional start site) are marked. (D) EMSA showing the binding of His-RegX3 (0, 3.6, and 7.1 μM) with three biotin-labeled short fragments from prpR upstream promoter region. (E) Binding of biotin labeled PprpR–3 with increasing concentration of His-RegX3 (0, 1.6, and 3.2 μM). (F) EMSA showing the binding of His-RegX3 (0, 0.35, 0.7, 1.4, and 2.8 μM) with biotin-labeled PprpR–3 containing predicted consensus binding motif (GCCTAACCGCGATTCTTACACA) (left) or biotin-labeled MPprpR–3 containing mutate binding motif (TCCTCACCGCGAGGATTACACA) (right).
Previous studies have identified the DNA-binding site of RegX3 with a conservative binding sequence, GT/GTCAcc-n4-cG (Glover et al., 2007). Using MAST/MEME tools and PREDetector software, we identified an unknown putative RegX3 binding motif in the promoter region of prpR (Figure 2C, marked by shadow background). To precisely determine the RegX3 binding position in prpR operon, we generated three about 100 bp DNA fragments derived from the prpR gene upstream and promoter region by PCR, named PprpR–1, PprpR–2, and PprpR–3. As expected, PprpR–3 harboring the predicted RegX3 binding site was bound to RegX3 (Figures 2D,E). To further confirm the specific binding, we introduced point mutations in the conserved sites of the binding motif. No shift band was observed in EMSA assays for MPprpR–3, a biotin-labeled probe derived from PprpR–3 with mutant motif (Figure 2F). Altogether, our data characterized a previous unknown RegX3-binding motif in the promoter region of prpR in M. smegmatis and M. tuberculosis.
Induction of the Methylcitrate Cycle-Related Genes in Response to Phosphate Starvation
As previously reported, the phosphate acquisition in M. smegmatis is mediated by a two-component regulatory system SenX3-RegX3 in response to environmental inorganic phosphate concentration. We further investigated the transcriptional profile of operon prpDBC and the activity of MCS enzyme in M. smegmatis under the conditions of different phosphate availabilities. As described in section “Materials and Methods,” the M. smegmatis WT strain harvested at logarithmic phase in LB broth with 0.05% Tween 80 were transferred to modified MOPS-propionate (10 mM) minimal media (100 μM K2HPO4 of low-phosphate condition and 10 mM K2HPO4 of high-phosphate condition) for 12-h incubation. The significant increase of regX3 transcription level (3.1-fold, p < 0.001) was observed responding to phosphate starvation, which induced expression of the genes participating in methylcitrate pathway, including prpR (2.4-fold, p < 0.01), prpB (7.1-fold, p < 0.001), prpC (2.6-fold, p < 0.01), and prpD (3.7-fold, p < 0.01) (Figure 3A). In addition, intracellular total activity of MCS enzyme was increased in phosphate-limited medium, approximately 1.8-fold, over that in phosphate-rich medium (Figure 3B). These results indicated that the expression of methylcitrate cycle genes can be induced in response to phosphate starvation.
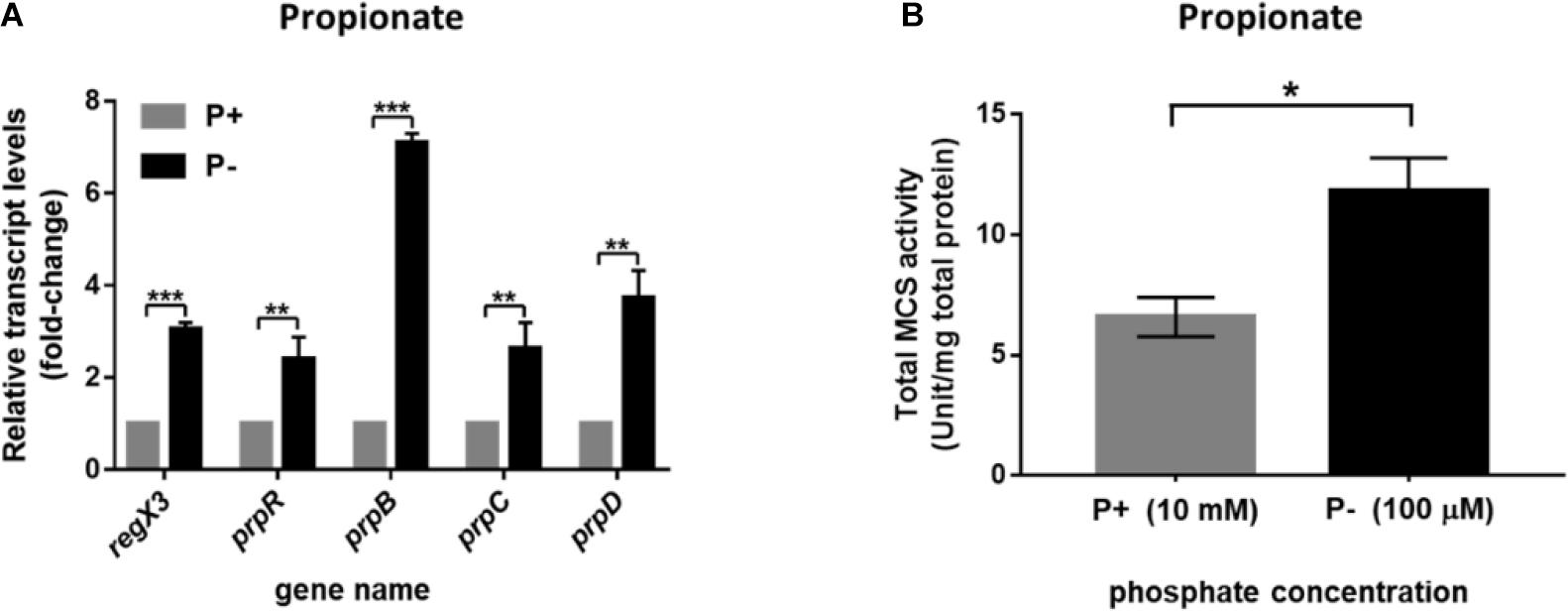
Figure 3. Induction of the methylcitrate cycle-related genes and enzyme activity in M. smegmatis in response to different phosphate availabilities. (A) The transcriptional profiles of regX3 and methylcitrate-cycle-related genes when M. smegmatis was grown in MOPS-propionate (10 mM) medium without phosphate (P-) or with a high phosphate level (P+, 10 mM K2HPO4). RNA was collected at 12 h after incubation. Relative fold change was tested using 16S rRNA as housekeeping gene. The data of relative fold change under the high phosphate condition was set to 1.0 (arbitrary unit). (B) Comparison analysis of total MCS activity in M. smegmatis grown at the low- and high-phosphate level. Ten micrograms of total protein were used in the MCS activity assay. Data are presented as means ± SEM calculated from three independent experiments. Unpaired two-tailed Student’s t-test, *p < 0.05; **p < 0.01; and ***p < 0.001.
RegX3 Activates the Methylcitrate Cycle by Up-Regulating prpR Transcription During M. smegmatis Growth on Propionate
Methylcitrate cycle is the most widely studied pathway of propionyl-CoA metabolism in M. smegmatis (Munoz-Elias and McKinney, 2006; Munoz-Elias et al., 2006). Besides, it was reported that when propionate is the main carbon source, PrpR acted as a transcriptional activator of prpD, prpB, prpC encoding three key enzymes in methylcitrate pathway (Masiewicz et al., 2012). To investigate the effect of RegX3 on the methylcitrate cycle in M. smegmatis, we determined transcriptional levels of gene prpR and operon prpDBC and enzyme activity of MCS in the three strains (WT, i regX3 and OE regX3) of M. smegmatis. Under the limiting culture conditions, diverse M. smegmatis strains were collected at 6- and 12-h post incubation. Compared to WT, i regX3 resulted in down-regulation of methylcitrate cycle-related genes; while overexpression of regX3 remarkably induced the expression of these genes (Figure 4A), indicating that RegX3 was a transcriptional activator of prpR gene. However, the impacts of RegX3 on the transcription of methylcitrate cycle related genes were disappeared when the three M. smegmatis strains grew on glucose (Supplementary Figure 3), indicating that the RegX3-mediated regulation of the methylcitrate cycle at low phosphate is propionate-dependent.
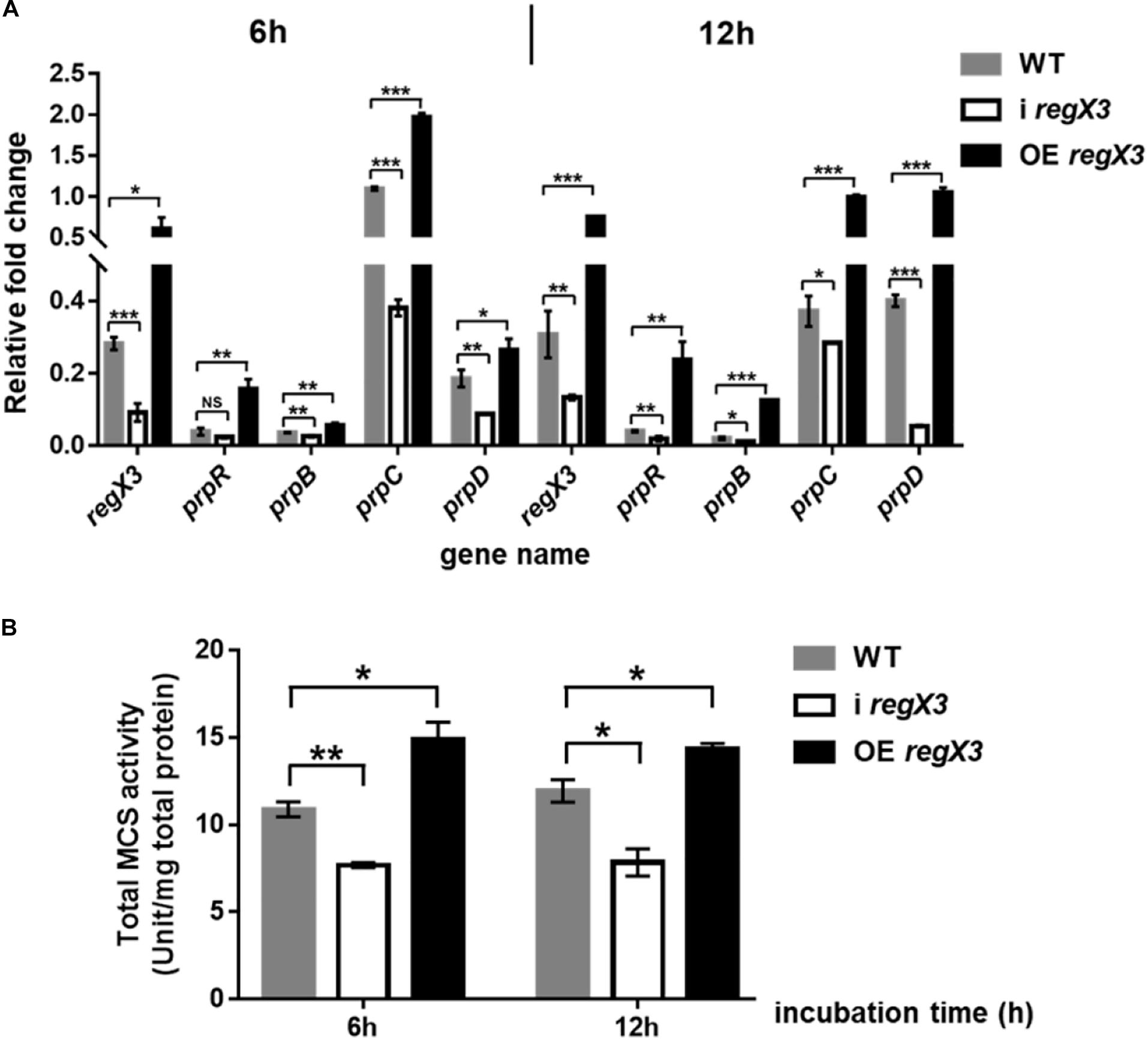
Figure 4. Induction of the methylcitrate cycle-related gene and enzyme activity by RegX3 in M. smegmatis growing on propionate under the low-phosphate condition. (A) Transcriptional profiles of regX3 and the methylcitrate cycle-related genes in WT, i regX3, and OE regX3 strains of M. smegmatis grown in MOPS-propionate (10 mM) medium supplemented with 100 μM K2HPO4. Cells were harvested for RNA extraction at 6 and 12 h after incubation. 16S rRNA was selected as a reference and fold change was analyzed by qRT-PCR. (B) Total activity of MCS enzyme in diverse M. smegmatis strains grown on propionate. Strains were incubated and collected under the same conditions as tested for the qRT-PCR analysis. 10 μg total protein were used. Data are presented as means ± SEM calculated from three independent experiments. Unpaired two-tailed Student’s t-test, *p < 0.05; **p < 0.01; and ***p < 0.001.
Next, we examined if RegX3 affects the activity of MCS, the key enzyme required for the first step that propionyl-CoA enters methylcitrate cycle. Consistent with the positive effects at transcriptional level, the transcription inhibition of regX3 resulted in obvious decreases in total MCS activities compared to WT. On the contrary, overexpression of regX3 enhanced MCS activities to about 1.4-fold (p < 0.05) and 1.2-fold (p < 0.05) after 6- and 12-h incubation, respectively (Figure 4B). These results demonstrated that RegX3 activates the methylcitrate cycle of M. smegmatis growing on propionate in response to phosphate starvation, through up-regulating the prpR transcription.
RegX3 Competes With PrpR for Binding to the prpR Promoter
Previous studies identified a highly conserved PrpR-binding palindromic sequence (TTTGCAAA) in the promoter region of prpR, resulting in autoregulation of the PrpR protein (Masiewicz et al., 2012). As described in Figure 2C, RegX3 bound to the prpR promoter within the upstream region of a 22-bp sequence, which is similar to the typical RegX3-binding box GT/GTCAcc-n4-cG. Indeed, PrpR and RegX3 binding sites are very close to each other with only three bases in space (Figure 5A), indicating a potential competition for the prpR promoter between RegX3 and PrpR.
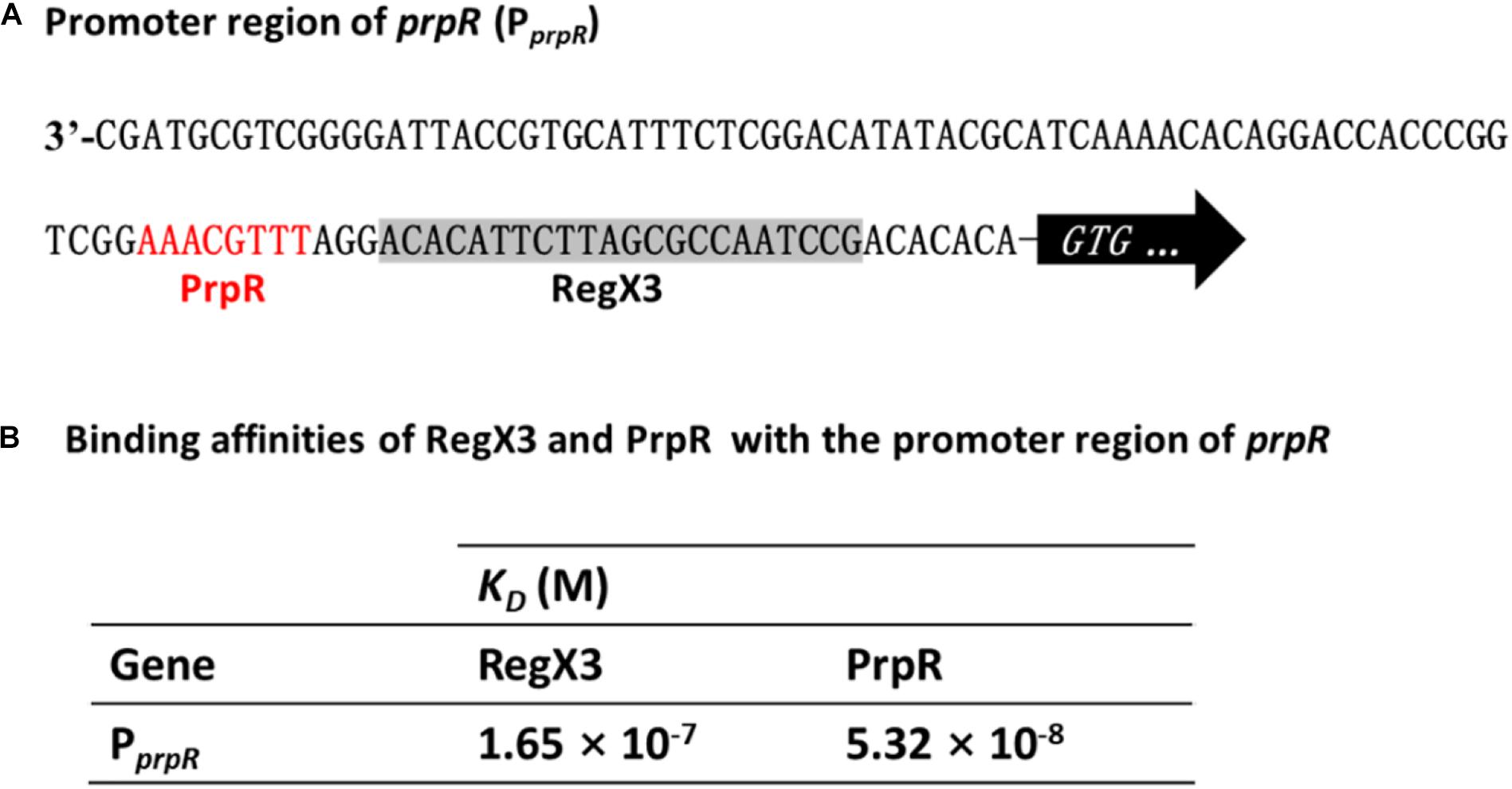
Figure 5. Competitive binding of RegX3 and PrpR to the prpR promoter. (A) Overview of PprpR showing the RegX3 box (shaded) and PrpR binding site (red). (B) Binding affinities of the promoter region of prpR for RegX3 and PrpR. The interactions of recombinant His-RegX3 and His-PrpR with the prpR (encoding MCS) were determined using the Octet Macromolecular Interaction Platform. The KD value of RegX3 and PrpR was 165 and 53.2 nM, respectively, which demonstrates that the PrpR has a stronger binding capacity than RegX3.
To further investigate which regulator protein has higher affinity and plays a major role in the competitive regulation of prpR gene, the kinetic binding analysis of RegX3 and PrpR to the prpR promoter were conducted using Bio-layer Interferometry. As shown in Supplementary Figures 4, 5B, serial concentrations of recombinant RegX3 or PrpR were incubated with DNA probes, and the affinity constant KD was calculated by the measurement of association and dissociation. As described in Figure 5B, PrpR (KD = 165 nM) had a 2-fold higher affinity for the prpR promoter than that of RegX3 (KD = 53.2 nM), suggesting that PrpR prevents RegX3 from binding to the putative RegX3-Box in the promoter of prpR.
RegX3 Promotes the Proliferation and Survival of M. smegmatis in Macrophages
Considering that RegX3 is required for M. tuberculosis resistance to phosphate-limiting stress and indispensable for M. tuberculosis survival in humans (Gomez and McKinney, 2004), we reasoned that the RegX3 of M. smegmatis might be also essential for its survival in macrophage cells. To investigate the effect of RegX3 on survival of M. smegmatis in macrophage, THP-1 macrophage cells were co-cultured with M. smegmatis WT, i regX3, and OE regX3 strains. Survival of these strains were tested at different time points (2, 12, 24, 36, and 48 h). All these bacteria underwent growth and clearance in macrophages.
As shown in Figure 6, the overexpression strain has a higher growth rate and a lower clearance rate during the macrophage infection period. No significant difference in the survival of the three strains in macrophages was observed at 2 h after infection. But the obvious differences appeared with the extension of infection time. The overexpression strain in macrophages doubled in the first 12 h of infection, and the CFU of bacteria at 12 h is almost twice of that at 2 h. At the same time, the WT strain had a slight increase, while the amount of i regX3 decreased steadily. After 24 h of infection, the three strains in macrophages began to decline remarkably and maintained a stable difference in survival rate. At the 48 h time point, the CFU of OE regX3 was about 2-fold (p < 0.001) higher than that of WT strain, while i regX3 CFU was decreased approximately at one-half of WT CFU (p < 0.001). These results revealed that OE regX3 has a higher growth rate and a lower clearance rate during infection of macrophage, suggesting that RegX3 exhibits a positive effect on the proliferation and survival of M. smegmatis in macrophages.
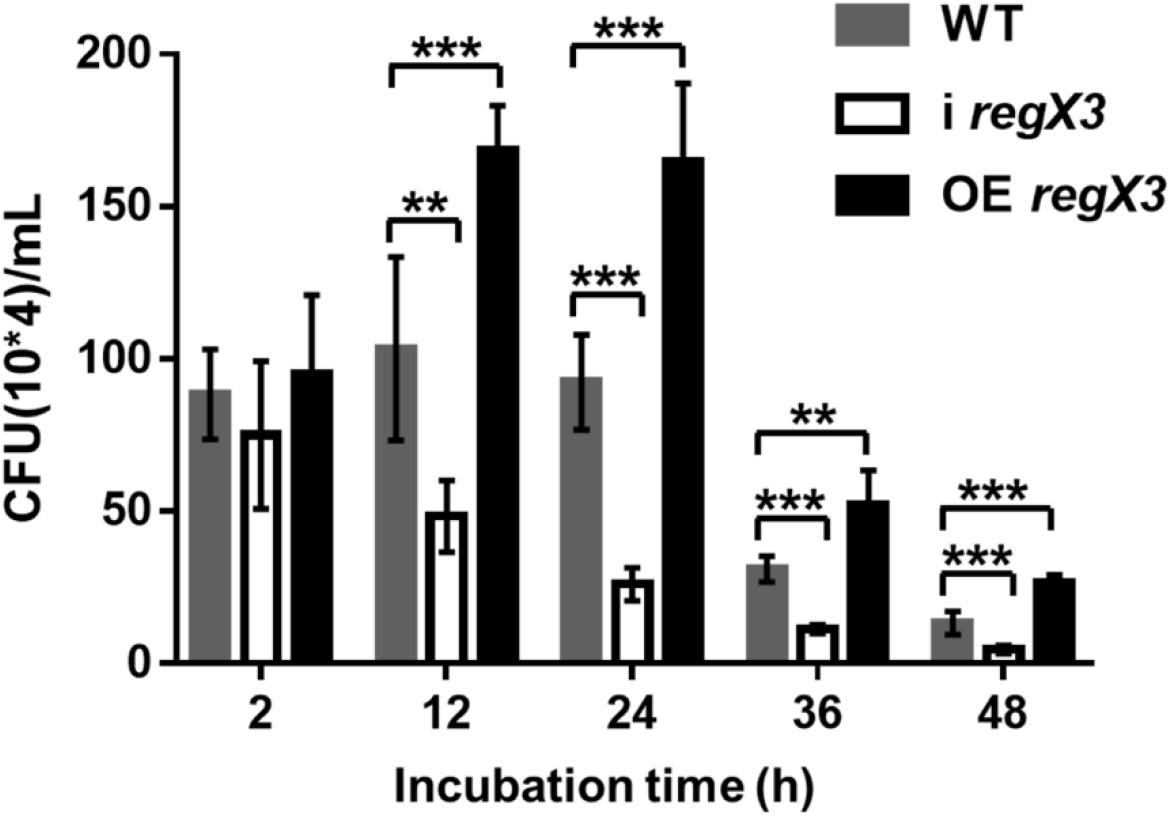
Figure 6. Survival of diverse M. smegmatis strains in macrophages. THP-1-derived macrophage cells were infected with M. smegmatis WT, i regX3, and OE regX3 strains. Survival of these strains were determined in different time, 2, 12, 24, 36, and 48 h. Cells were harvested at different time points to determine the concentrations of live M. smegmatis (n = 6). Data are presented as means ± SEM calculated from three independent experiments. Unpaired two-tailed Student’s t-test, **p < 0.01 and ***p < 0.001.
RegX3 Affects the Cell Morphology of M. smegmatis Growing on Propionate
It was previously reported that M. tuberculosis undergoes elongation following Pi depletion and this physiological change is closely related to the effect of SenX3-RegX3 (Rifat et al., 2009, 2014). To explore whether RegX3 also plays an essential role in the cell morphology of M. smegmatis growing on propionate, we measured the bacterial cell length of WT, i regX3, and OE regX3 strains by using transmission electron microscopy (TEM). Images of thirty bacilli of each strain were randomly capture after cultivated for 12 h in the modified MOPS-propionate (10 mM) or the glucose (10 mM) minimal media supplemented with a low concentration of phosphate (100 μM K2HPO4) (Supplementary Figures 5A,B). As shown in Figures 7A–C, the mean length of i regX3 (2.58 ± 0.05 μm) was shorter than that of either WT (2.88 ± 0.12 μm) or OE regX3 (3.14 ± 0.07 μm) bacilli during growth on propionate in response to phosphate starvation. However, no significant difference was observed in the length of WT (2.84 ± 0.1 μm), i regX3 (2.78 ± 0.05 μm), and OE regX3 bacilli (2.93 ± 0.02 μm) cultured in glucose with a low concentration of phosphate. The morphological change related to regX3 was disappeared when the three M. smegmatis strains sensed the high-phosphate condition (Supplementary Figures 5C–E). Collectively, these data indicated that RegX3 triggers morphological change of M. smegmatis growing on propionate in response to phosphate starvation.
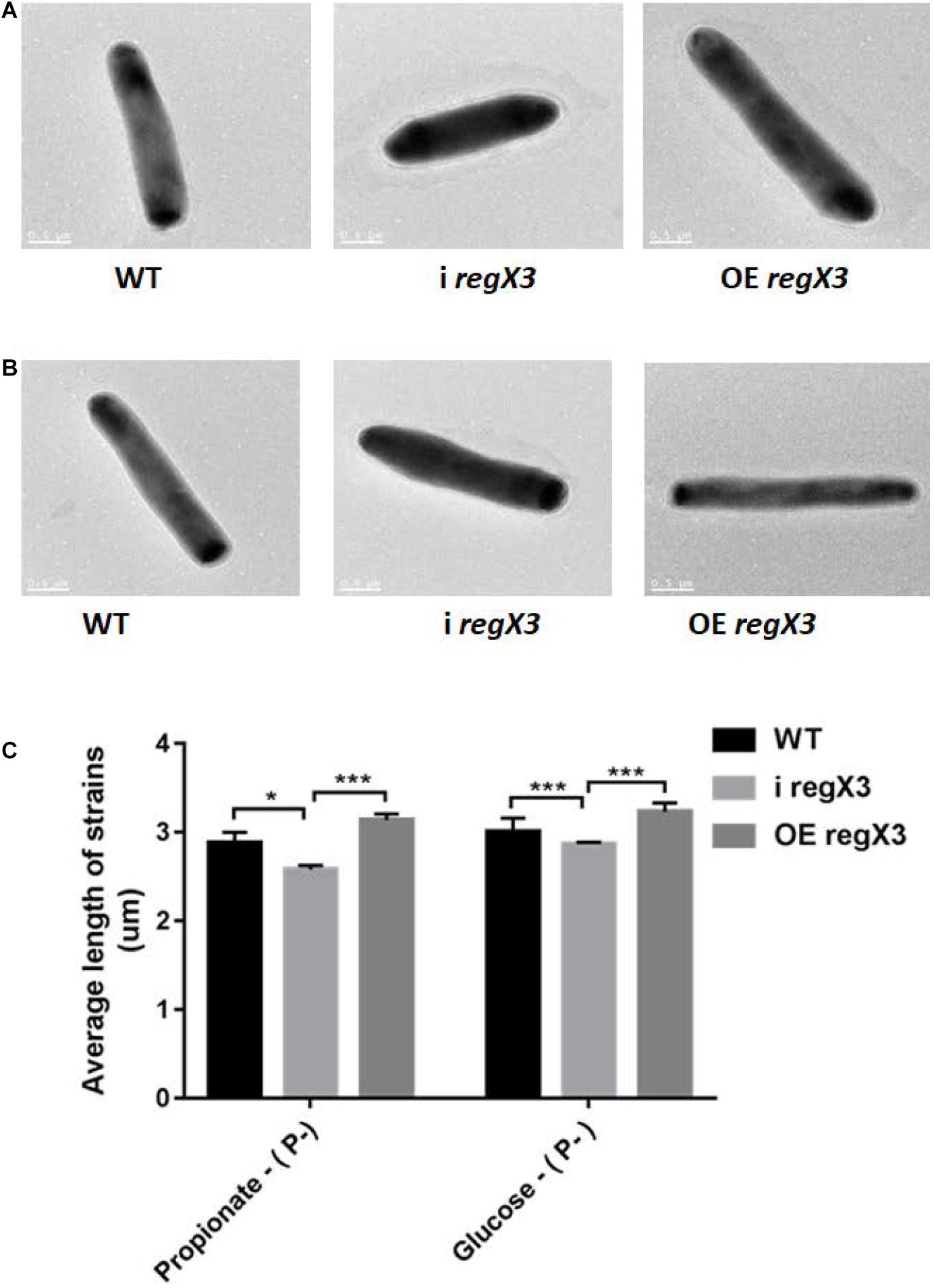
Figure 7. The morphology of three M. smegmatis strains growing on different carbon resources in response to phosphate starvation. Transmission electron microscopy (TEM) images of single bacillus from M. smegmatis WT, i regX3, and OE regX3 strains after incubated for 12 h in the 10 mM MOPS-propionate (A), or the 10 mM MOPS-glucose (B) minimal medium with a low concentration of phosphate (100 μM K2HPO4). Scale bar represents 0.5 μm. See also Supplementary Figures 5A,B. (C) Length of bacteria cells (n = 30) as shown in Figure S5A and S5B was measured by manual evaluation, using Image J on the electron microscopic images. Data are presented as means ± SEM. Unpaired two-tailed Student’s t-test, *p < 0.05 and ***p < 0.001.
Discussion
It has been previously reported that M. tuberculosis utilizes fatty acids and cholesterol as main energy source during the course of infection. In M. tuberculosis-infected alveolar macrophages, the long-chain fatty acids such as dipalmitoyl phosphatidylcholine from the lung surfactant can be metabolized by M. tuberculosis through the glyoxylate cycle (Grabner and Meerbach, 1991; Munoz-Elias and McKinney, 2005). In addition, M. tuberculosis can not only hydrolyze the lipids in phagosomal membrane and release free fatty acids (Kondo et al., 1985), but also utilize the macrophage triacylglycerol stores motivated during phagocytosis (Mason et al., 1972). Therefore, fatty acids metabolism plays an important role in M. tuberculosis survival within host cells. When lipids act as the primary nutrient of strain, the metabolites acetyl-CoA and propionyl-CoA were degraded by glyoxylate and methylcitrate cycles. It is worth mentioning that the production of propionyl-CoA could be degraded by other way like citrate and methylmalonyl-CoA routes, but the studies showed that methylcitrate rounds is the primary route (Textor et al., 1997; Horswill and Escalante-Semerena, 1999; Claes et al., 2002). As illustrated in Figure 8, when propionyl-CoA enters into the methylcitrate cycle, it would react with the oxaloacetate from TCA cycle to generate methylcitrate under the function of methylcitrate synthase (MCS). With the assist of methylcitrate dehydratase (MCD), the methylcitrate would subsequently be converted into methylisocitrate. And methylisocitrate lyase (MCL) would catalyze the cleavage of methylisocitrate to free pyruvate and the succinate which would reflow to TCA cycle. All homologs enzymes of methylcitrate cycle were encoded in the M. tuberculosis, except for MCL, which is characterized by ICL1 and ICL2 enzymes essential for the for survival of M. tuberculosis on propionate (Cole et al., 1998). In the M. smegmatis genome, a dedicated MCL, which is arranged in the prpDBC operon encoding MCS and MCD orthologs, can be potentially encoded (Upton and McKinney, 2007). In addition, the similar arranged orthologs of prpDBC are present in the Mycobacterium marinum and Mycobacterium avium genomes (Li et al., 2005). In summary, the methylcitrate cycle of M. smegmatis is generally similar to that of M. tuberculosis, it is suspected that these two genetically related mycobacteria share the way of propionate metabolism.
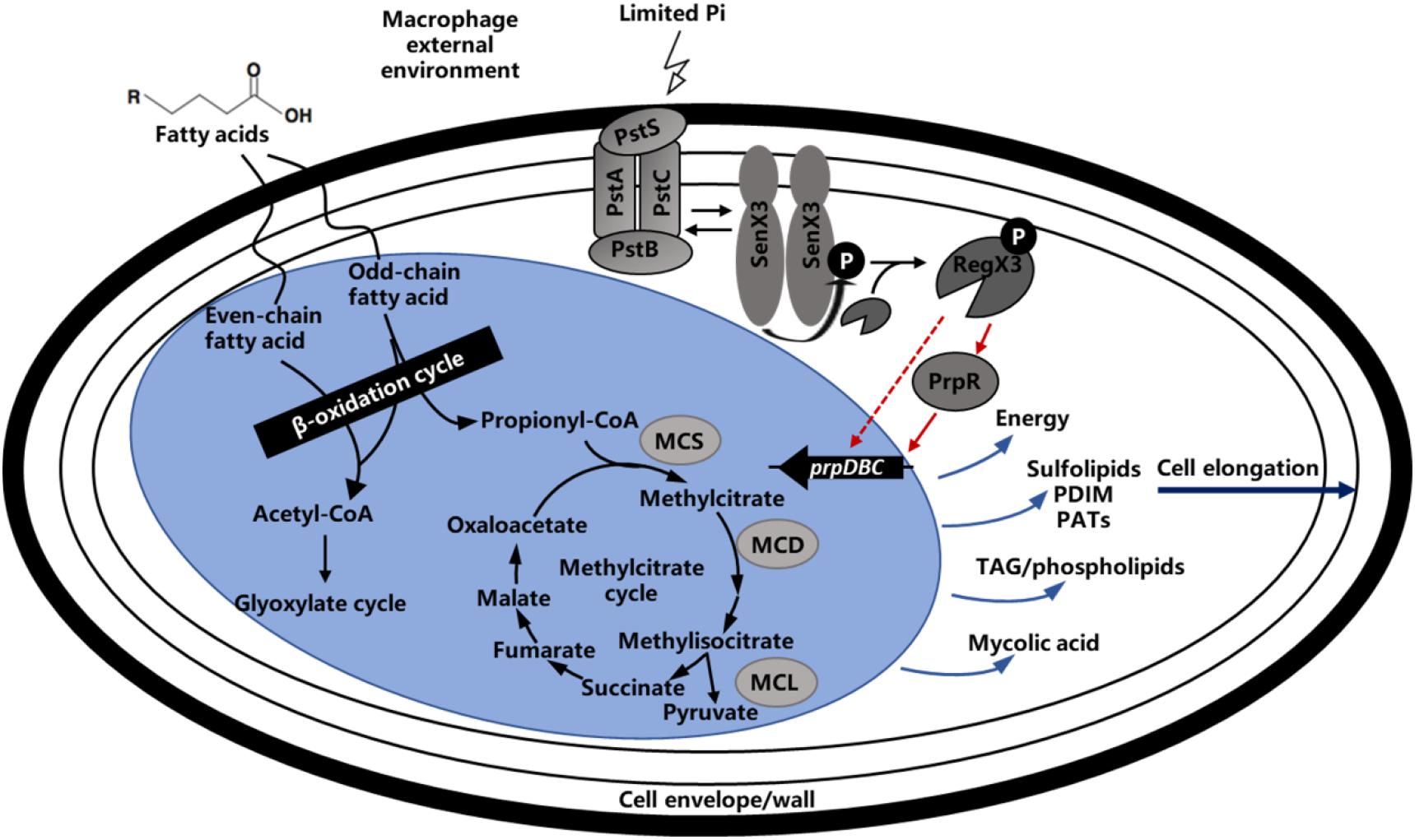
Figure 8. Regulatory model of methylcitrate pathway by RegX3 in Mycobacteria. Phosphate-responsive RegX3 and propionate-responsive PrpR competitively regulate the transcription of prpDBC operon involved in methylcitrate pathway (MCS, MCD, and MCL), which play an important role in metabolism of cholesterol and fatty acids as the main nutrient sources of mycobacteria during infection. It was suggested that RegX3 contributed to establish a direct association between phosphate and carbon metabolisms, and also modulate the C-P balance in mycobacteria. The wave lines indicate transmembrane transport. The fold line with arrow indicates signal stimulus. The red lines with arrows indicate positive transcriptional regulation while the light blue lines with arrows indicate compound synthesis process.
In this work, we showed that the important nutrient-sensing regulator of M. smegmatis, RegX3, directly activated the transcription of prpR gene involved in methylcitrate cycle in response to phosphate starvation. It was suggested that RegX3 contributed to establish a direct connection between P and C metabolisms, and also modulate the C-P balance in mycobacteria. Carbon and phosphorus are the basic components of microbial nutrition. Pathogenic bacteria may frequently encounter limited supplies of phosphate after ingested by macrophage, although the concentration of soluble phosphate in macrophage has not been determined yet. The regulation of propionate utilization in M. smegmatis is under RegX3 control, indicating that phosphate limitation triggers the RegX3-mediated response, which results in the expression of genes involved in propionate degradation and utilization. It was previously reported that PrpR exerts a regulatory effect on the uptake and utilization of propionate in M. tuberculosis and influences the expression of MCD and MCS enzymes in methylcitrate pathway by directly regulating the transcription of the local prpDC target (Masiewicz et al., 2012). Meanwhile, the prpDBC (encode MCD, MCL, and MCS, respectively) operon could also be upregulated by PrpR in M. smegmatis. Therefore, we suspected that the increased expression of RegX3, which responded to phosphate restriction, could activate the transcription levels of prpBCD genes by its direct regulation of prpR, to promote the whole methylcitrate pathway. We found that the phosphate limitation and overexpression of regX3 increased the activity of MCS, thereby enhancing the propionyl-CoA assimilation (Figures 3B, 4B). Besides, RegX3 was previously shown to regulate the methylcitrate cycle by activating prpC expression in M. tuberculosis (Roberts et al., 2011). These collective results suggested that the phosphate regulator RegX3 functions at two different levels (prpR and prpC) in the methylcitrate cycle, thus playing a dual role in both carbon and phosphate metabolism in mycobacteria. More importantly, our data clearly demonstrated that the RegX3-mediated regulatory effect on methylcitrate pathway is conserved in M. tuberculosis, which provides insights into the molecular mechanisms for nutrient sensing and metabolism in M. tuberculosis. These mechanisms might be general in mycobacteria for nutrient sensing and environmental adaptation.
Transcription inhibition of the regX3 gene resulted in growth defect on propionate as the sole carbon source. The macrophage infection experiment confirmed that RegX3 is required for the proliferation and survival of M. smegmatis in macrophages. How RegX3 enables bacteria to quickly adapt to the harsh macrophage microenvironment and keep infective activity for a long term? On one hand, actinomycetes (including mycobacteria) accumulate large amount of triacylglycerols in response to the starvation condition (Alvarez and Steinbuchel, 2002), so RegX3 stimulated by low-phosphorus signal might accelerate the methylcitrate cycle to prevent the accumulation of toxic metabolites caused by incomplete propionyl-CoA metabolism. On the other hand, it has been discovered that M. tuberculosis undergoes elongation following phosphate depletion and the SenX3-RegX3 regulatory system has a great effect on the bacterial morphology (Rifat et al., 2009; Rifat and Karakousis, 2014). The similar cases were also observed when the M. smegmatis was cultured on propionate as the main carbon source. There is a reasonable interpretation that RegX3 can elongate bacteria and increase the ratio of surface area to volume so that the bacteria can be more exposed to the surrounding environment to increase the chance of obtaining propionate and other nutrients. Therefore, the RegX3-mediated C-P balance in mycobacteria probably mentions the effect of RegX3 on cell wall or relevant precursor synthesis pathway, which means that the M. tuberculosis inside host may control the metabolic level by sensing the change of environmental phosphorus concentration, altering the cell morphology for a stable nutritional status. Furthermore, M. tuberculosis cell wall contains various lipid components that can serve as virulence factors, regulate host immune response, counteract anti-TB antibiotics and toxic molecules derived from host cells, it is thus suggested that RegX3 might have some connection with the pathogen-associated molecular patterns (PAMPs) of M. tuberculosis (Siegrist and Bertozzi, 2014). It is quite possible for RegX3 to be involved in the interaction between M. tuberculosis lipid metabolism and hots immune response, representing a complex and dynamic host-pathogen interplay (Figure 8).
Here, we carried out the research about the regulation mode of transcription regulator RegX3 on methylcitrate cycle in M. smegmatis. The experimental results verified by this work could match with the corresponding regulatory network of M. tuberculosis to a certain extent, thus reflecting the following metabolism of fatty acid intermediate metabolites in M. tuberculosis when the bacteria infect lung cells or survive in macrophages. It is the most important that the research can help us to comprehend how M. tuberculosis survives in a harsh host environment, thus extending our understanding of the toxicity mechanism of M. tuberculosis and providing new insights into the development of anti-tuberculosis drug.
Materials and Methods
Bacterial Strains, Plasmids, and Growth Conditions
Different strains, plasmids referenced in the work are showed in Table 1. E.coli used for cloning and protein expression were grown in liquid or onto solid (with the addition of 15 g agar l–1) Luria Broth (LB) medium. Different M. smegmatis strains, including wild-type M. smegmatis MC2 155 (Munoz-Elias and McKinney, 2005), regX3 knock-down strain and regX3 overexpression strain, were grown on LB media with 0.05% Tween-80 for strain activation. Phosphate restrictive media were prepared using a MOPS-propionate basal media (5.232 g MOPS, pH 7.2, 1.864 g KCl, 0.584 g NaCl, 1.321 g (NH4)2SO4, 0.017 g FeCl3, 0.241 g MgSO4, 0.011 g CaCl2, 0.961 g Sodium propionate, 0.02% tyloxapol) supplemented with NaH2PO4 as Pi source. The growth of M. smegmatis strains was investigated in the media MOPS-glucose (10 mM) minimal medium with low concentration phosphate (100 μM K2HPO4) and MOPS-propionate (10 mM) minimal medium with high concentration phosphate (10 mM K2HPO4). The medium were cultured at 37°C with agitation of 220 rpm and sterilized at 121°C for 20 min.
Overexpression and Purification of His6-RegX3 and His-PrpR Protein in E. coli
The genes (regX3, prpR, senX3, and regX3-Mtb) were amplified from M. smegmatis MC2 155 (Munoz-Elias and McKinney, 2005) and M. tuberculosis genome by PCR using a couple of primers (Table 2). The fragments were obtained through digestion of the PCR products using the EcoRI and HindIII for regX3 and prpR, NdeI and XhoI for senX3 and regX3-Mtb, and inserted into pET-28a(+) vector. The pET-28a(+)-regX3 plasmid (p0937), pET-28a(+)-prpR plasmid (p6643), pET-28a(+)-senX3 plasmid (p0936), or pET-28a(+)-regX3-mtb plasmid (p0467) were transferred to BL21 E. coli. E. coli strains newly transferred with p0937/p6643/p0936/p0467 were cultured in LB media at 37°C with agitation of 220 rpm (OD600 = 0.6). The following overexpression and purification of RegX3, PrpR, SenX3, and RegX3-mtb in BL21 were operated as previously described (Yao and Ye, 2016). The expression of proteins were analyzed by SDS-PAGE, and the concentration was quantified using the Bradford method (Transgen).
Electrophoretic Mobility Shift Assays
The upstream regions from -300 bp to 0 bp of detected genes (predicted to contain a RegX3-binding site) were amplified by PCR using gene-specific primers and the biotin-labeled universal primer. The probes were confirmed by 1.5% agarose gel and cleaned up using the PCR Purification Kit (Qiagen), whose concentration was detected with microplate reader (Biotek, United States). EMSAs were processed on the basis of the protocol attached to Chemiluminescent EMSA Kit (Beyotime Biotechnology, China). The binding system, containing 1.576 g Tris-HCl (pH = 8.0), 2.38 g Magnesium chloride, 2.92 g Sodium chloride, 1 mM DTT, 0.292 g Ethylene Diamine Tetraacetic Acid, 0.01% Non-idet P40, 50 μg ml–1 poly[d(I-C)] and 100 ml glycerol. The reaction system was prepared that probes labeled with biotin cultured separately with different regulator protein at room temperature (20 min) prior to separating on a 6% non-denaturing PAGE gel in ice-bathed TBE at 170 V, and bands were checked by BeyoECL Plus. Sequences of primers are listed in Table 2.
Construction of the RegX3 Knock-Down and Overexpression Strains
The regX3 gene overexpression mutants were generated by protoplast transformation with E. coli–M. smegmatis integrative shuttle vector pMV261 containing a strong constitutive hsp60 promoter by inserting the 678 bp of the regX3 open reading frame (MSMEG_0937, amplified with primers OregX3-F/R) between EcoRI and HindIII, to obtain the regX3 (pMV261-regX3) overexpression plasmid (Table 1; Stover et al., 1991). The plasmids were entered into the M. smegmatis WT strain protoplasts by electrotransformation. Kanamycin-resistant was checked by supplemented with 25 μg/ml Kanamycin (final concentration) after transfer for 3∼4 h. The selected recombinant strains of above two kinds of M. smegmatis were verified by their relative transcription levels with WT strain via the combination of RNA extraction and real-time RT-PCR. The fold change of regX3 expression between the overexpression strain and wild-type in rich and nutrient-limiting media were 1.2-fold and 2.5-fold (p < 0.05), respectively.
The CRISPRi technique (Yao et al., 2016) was used to construct a mutant with inhibited transcription level of regX3. First, we assembled the functional plasmid pPR27-hsp60-dCas9-sgRNA, where the pPR27 plasmid is a shuttle plasmid (Bigi et al., 2004) and the hsp60 is a strong constitutive promoter for M. smegmatis, while the complex consisted of the deactivated Cas9 (dCas9) and the sgRNA could bind to target gene and impede the occurrence of transcription. Second, the recombinant plasmids were electroporated into the M. smegmatis WT strain protoplasts and the strains successfully introduced plasmid were screened out with gentamycin at a final concentration of 20 μg ⋅ ml–1. The regX3 transcript fold change of the mutants were also verified by comparing with WT strain.
All the primers used for construction of the M. smegmatis mutants are listed in Table 2. The designed sgRNA-regX3 forward sequence (in Table 2) was synthesized by Sangon Biotech (Shanghai) Co., Ltd.
RNA Preparation and Real-Time RT-PCR
Frozen bacterial stocks were inoculated into LB-medium supplemented with 0.05% Tween 80 and cultured to OD600 of 0.6. Cultures were adjusted to OD600 = 0.2 in MOPS-Pi and OD600 measurements were taken every 12-h for 3 days. IE regX3 strains including the pPR27 plasmid were cultured in medium with 50 mg/ml kanamycin (Sigma). overexpression strain including the pMV261 plasmid were cultured in with 50 mg/ml gentamycin (Sigma). For RNA preparation, M. smegmatis WT, i regX3 and OE rgX3 strains were precultured in LB + 0.05% Tween-80 broth to an OD600 of 0.6-0.8 and subsequently transferred to the MOPS-propionate (final OD600 0.2) minimal medium described above with 100 μM K2HPO4 as unique phosphate resource for several-hour incubation at 37°C after washed three times with sterile physiological saline. Cell pellets were harvested after 10min of centrifugation at 6,000 rpm and 4°C accompanying three-time washing with phosphate buffer saline (PBS). Total RNA was extracted using RNAprep Pure Cell/Bacteria kit (Tiangen Biotech, Beijing, China), with the RNA integrality tested by 1% agarose gel. The obtained RNA was reverse-transcribed to cDNA using RT reagent kit (Takara, Shiga, Japan) following the manufacturer’s instructions. The SYBR premix Ex TaqTM GC Kit (Takara) was chosen as a reaction reagent and final concentration of cDNA was 50 ng/uL. The PCR was conducted using CFX96 real-time system (Bio-Rad, United States) with the primers listed in Table 2. The RT-qPCR experiment methods were described below: 95°C (3 min), then 40 rounds of 95°C (15 s), 60°C (15 s), and 72°C (30 s), finally 72°C (10 min). The 16S rRNA selected as an reference and fold change were analyzed using the (2–ΔΔCT) method or another (2–ΔCT) method when sigA gene was used as an internal reference (Elliott and Tischler, 2016).
Methylcitrate Synthase (MCS) Assays
Frozen WT strain was inoculated into LB-media with 0.05% Tween 80 and cultured to logarithmic phase (OD600 = 0.6). After incubation in Pi-rich (10 mM) and Pi-limiting (100 μM), the WT strain was harvested (OD600 0.6) for MCS extraction. The harvested strain was ultrasonic crushing (200 W, 3 s ultraphonic, 10 s interval, repeat for 30 times) at the ratio of strain: extraction buffer 1000: 1. Then samples were centrifuged (8000 rpm, 10 min, 4oC) and the supernatant was harvested for MCS reaction. MCS activity was analyzed by the free CoA accumulation, which produced by the reaction of propionyl-CoA and oxaloacetate. While the free CoA accumulation was checked by the reaction of CoA with DTNB (Lu et al., 2019). Reactions System containing 11.92 g HEPES pH 8.0, 5.84 g Sodium chloride, 0.584 g Ethylene Diamine Tetraacetic Acid, 0.04 g DTNB, 0.035 mM propionyl-CoA, 0.4 mM oxaloacetate, and the extract 1-6 ug/ul. After system cultured at 30°C for 10 min, the TNB anion were tested at 412 nm, using a microplate reader (BioTek, United States).
Kinetic Binding Analysis
The binding affinities of His-RegX3 or His-PrpR with the promoter of prpR (encoding MCS) were determined using the Octet Macromolecular Interaction Platform (Fortebio, United States). Firstly, the upstream promoter regions of prpR with a concentration of 50 ng μl–1 was dissolved in loading buffer (2.383 g HEPES, 0.19 g Magnesium chloride, 0.029 g Ethylene Diamine Tetraacetic Acid, 14.91 g Potassium chloride, pH 8.0). Next, the biotin-labeled promoter was immobilized on a streptavidin-coated bioprobe (Fortebio), and then the bioprobe with the prpR promoter fragment was inserted into the purified His-RegX3 (1.7, 3.4, 7, and 14 μM) or His-PrpR (0.9, 1.8, 3.5, and 7 μM) of different concentration gradient, dissolved in running buffer (2.383 g HEPES, 0.19 g Magnesium chloride, 0.029 g Ethylene Diamine Tetraacetic Acid, 14.91 g Potassium chloride, 10 μg/ml bovine serum albumin (BSA), 0.02% Tween-20, pH 8.0). After the protein binding curve tended to be stable, the bioprobe was moved into running buffer to initiate the dissociation reaction. Finally, the raw data processing was performed using Octet Data Analysis Software Version 7.0 to calculate the kinetic binding affinities (KD). The KD values of RegX3 and PrpR were 165 and 53.2 nM, respectively. The KD value demonstrates that the PrpR has a stronger binding capacity than RegX3. The detailed protocol has been described in previous research (Liao et al., 2014).
Mycobacterium smegmatis Survival in THP-1 Cell
Bacterial infection model of macrophages was mainly modified with reference to the previous report (Jiang et al., 2018). Human THP-1 cell lines (Conservation Genetics, CAS Cell Bank) were grown in 1640 media (Hyclone, United States) containing 10% FBS (Gibco, United States), and adjusted to a final total of 2 × 106 cells. For the induction of a macrophage-like state, the cells were exposed to a 12-h treatment with 10 ng/ml polymethyl acrylate (PMA). After the renewal of medium, adherent cells cultured 8-12 h, and then co-incubated with different M. smegmatis at OD600 of 0.4 for 2 h (MOI = 10). The infected cells were washed three times with RPMI 1640 media to remove free bacteria and then grown in novel RPMI 1640 media (15 μg/ml gentamicin) for 2, 12, 24, 36, and 48 h. THP-1 cell were washed two times with LB medium before lysed in LB culture with 0.05% SDS (10 min, 37°C). In different time point, the cell lysates were diluted to three appropriate sets of tenfold serial gradients (102, 103, and 104) and portions were uniformly spread on LB agar plates. The CFU counting was performed on the plates which were Incubated at 37°C for 72–120 h.
Transmission Electron Microscopy
Bacterial morphology was evaluated by electron microscopy studies. The bacterial liquid was dry on grids and stained using 1% phosphotungstate (PTA). The profile was captured with JEOL JEM-1400 TEM [120 kV]. The data of each bacteria was tested with ImageJ software. The mean of the length of the bacilli for each strain was calculated.
Statistical Analysis
All data are presented as mean values with error bars indicating standard deviations (±SD) calculated from three independent experiments. Statistical significance between two groups was determined by unpaired two-tailed Student’s t-test using GraphPad Prism 7. Differences were significant when p < 0.05.
Importance
Mycobacterium tuberculosis is the pathogen of tuberculosis, which causes millions of deaths and infections every year. Despite the importance of host lipids for maintaining M. tuberculosis activities, little is known about the regulation mechanism of lipid metabolism. Here we uncover a novel metabolic regulation mechanism mediated by the phosphate regulator RegX3 of Mycobacterium smegmatis, a fast-growing counterpart of M. tuberculosis. RegX3 regulates the propionate metabolism responding to environmental phosphate concentration, and alters cell morphology to maintain nutritional steady state. Moreover, the regulation mechanism has been preliminarily proved to be general for M. tuberculosis, and other Mycobacteria. Our findings shed lights on how M. tuberculosis utilizes lipids to sustain life in nutrient-deficient macrophage, and provide insights for exploring anti-tuberculosis drug targets.
Data Availability Statement
The original data presented in the study are included in the article/Supplementary Material, further inquiries can be directed to the corresponding author/s.
Author Contributions
J-FP and Y-XL performed the experiments. B-CY conceived the concept, and supervised and discussed the experiments with JW and NQ. J-FP, Y-XL, and B-CY wrote the manuscript. All authors contributed to the article and approved the submitted version.
Funding
This study was supported by grants from the National Key Research and Development Program of China (2018YFA0900404), the National Natural Science Foundation of China (31730004 to B-CY and 31870864 to NQ), and Fundamental Research Funds for the Provincial Universities of Zhejiang (RF-B2020003).
Conflict of Interest
The authors declare that the research was conducted in the absence of any commercial or financial relationships that could be construed as a potential conflict of interest.
Supplementary Material
The Supplementary Material for this article can be found online at: https://www.frontiersin.org/articles/10.3389/fmicb.2021.619387/full#supplementary-material
References
Alvarez, H. M., and Steinbuchel, A. (2002). Triacylglycerols in prokaryotic microorganisms. Appl. Microbiol. Biotechnol. 60, 367–376. doi: 10.1007/s00253-002-1135-0
Anthony, D. B., and Kyu, Y. R. (2014). Metabolomics of central carbon metabolism in Mycobacterium tuberculosis. Microbiol. Spec. 2, 1–16. doi: 10.1128/microbiolspec.MGM2-0026-2013
Bigi, F., Gioffre, A., Klepp, L., Santangelo, M. D., Cataldi, A., Alito, A., et al. (2004). The knockout of the lprG-Rv1410 operon produces strong attenuation of Mycobacterium tuberculosis. Microbes Infect. 6, 182–187. doi: 10.1016/j.micinf.2003.10.010
Bloch, H., and Segal, W. (1956). Biochemical differentiation of Mycobacterium tuberculosis grown in vivo and in vitro. J. Bacteriol. 72, 132–141. doi: 10.1128/jb.72.2.132-141.1956
Claes, W. A., Puhler, A., and Kalinowski, J. (2002). Identication of two prpDBC gene clusters in Corynebacterium glutamicum and their involvement in propionate degradation via the 2-methylcitrate cycle. J. Bacteriol. 184, 2728–2739. doi: 10.1128/jb.184.10.2728-2739.2002
Cole, S. T., Brosch, R., Parkhill, J., Garnier, T., Churcher, C., Harris, D., et al. (1998). Deciphering the biology of Mycobacterium tuberculosis from the complete genome sequence. Nature 393, 537–543.
Datta, P., Shi, L., Bibi, N., Balazsi, G., and Gennaro, M. L. (2011). Regulation of central metabolism genes of Mycobacterium tuberculosis by parallel feed-forward loops controlled by sigma factor E (sigma(E)). J. Bacteriol. 193, 1154–1160. doi: 10.1128/jb.00459-10
Elliott, S. R., and Tischler, A. D. (2016). Phosphate starvation: a novel signal that triggers ESX-5 secretion in Mycobacterium tuberculosis. Mol. Microbiol. 100, 510–526. doi: 10.1111/mmi.13332
Flynn, J. L., Chan, J., and Lin, P. L. (2011). Macrophages and control of granulomatous inflammation in tuberculosis. Mucosal Immunol. 4, 271–278. doi: 10.1038/mi.2011.14
Glover, R. T., Kriakov, J., Garforth, S. J., Baughn, A. D., and Jacobs, W. R. (2007). The two-component regulatory system senX3-regX3 regulates phosphate-dependent gene expression in Mycobacterium smegmatis. J. Bacteriol. 189, 5495–5503. doi: 10.1128/jb.00190-07
Gomez, J. E., and McKinney, J. D. (2004). M. tuberculosis persistence, latency, and drug tolerance. Tuberculosis 84, 29–44. doi: 10.1016/j.tube.2003.08.003
Grabner, R., and Meerbach, W. (1991). Phagocytosis of surfactant by alveolar macrophages in vitro. Am. J. Physiol. Lung Cell Mol. Physiol. 261, 472–477.
Guo, M. M., Feng, H., Zhang, J., Wang, W. Q., Wang, Y., He, Z. G., et al. (2009). Dissecting transcription regulatory pathways through a new bacterial one-hybrid reporter system. Genome Res. 19, 1301–1308. doi: 10.1101/gr.086595.108
Himpens, S., Locht, C., and Supply, P. (2000). Molecular characterization of the mycobacterial senx3-regx3 two-component system: evidence for autoregulation. Microbiology 146, 3091–3098. doi: 10.1099/00221287-146-12-3091
Horswill, A. R., and Escalante-Semerena, J. C. (1999). Salmonella typhimurium LT2 catabolizes propionate via the 2-methylcitric acid cycle. J. Bacteriol. 181, 5615–5623. doi: 10.1128/jb.181.18.5615-5623.1999
James, J. N., Zeeshaan-ul, H., Ioerger, T. R., Brown, A. C., Personne, Y., Carroll, P., et al. (2012). Deletion of SenX3-RegX3, a key two-component regulatory system of Mycobacterium smegmatis, results in growth defects under phosphate-limiting conditions. Microbiology 158, 2724–2731. doi: 10.1099/mic.0.060319-0
Jenkins, V. A., Robertson, B. D., and Williams, K. J. (2012). Aspartate D48 is essential for the GlnR-mediated transcriptional response to nitrogen limitation in Mycobacterium smegmatis. FEMS Microbiol. Lett. 330, 38–45. doi: 10.1111/j.1574-6968.2012.02530.x
Jiang, H. W., Czajkowsky, D. M., Wang, T., Wang, X. D., Wang, J. B., Zhang, H. N., et al. (2018). Identification of serine 119 as an effective inhibitor binding site of M. tuberculosis ubiquitin-like protein ligase PafA using purified proteins and M. smegmatis. EBioMedicine 30, 225–236. doi: 10.1016/j.ebiom.2018.03.025
Klinkenberg, L. G., Sutherland, L. A., Bishai, W. R., and Karakousis, P. C. (2008). Metronidazole lacks activity against Mycobacterium tuberculosis in an in vivo hypoxic granuloma model of latency. J. Infect. Dis. 198, 275–283. doi: 10.1086/589515
Kondo, E., Suzuki, K., Kanai, K., and Yasuda, T. (1985). Liposomes-mycobacteria incubation systems as a partial model of host–parasite interaction at cell membrane level. Jpn. J. Med. Sci. Biol. 38, 169–180. doi: 10.7883/yoken1952.38.169
Lee, W., VanderVen, B. C., Walker, S., and Russell, D. G. (2017). Novel protein acetyltransferase, Rv2170, modulates carbon and energy metabolism in Mycobacterium tuberculosis. Sci. Rep. 7:72.
Li, L., Bannantine, J. P., Zhang, Q., Amonsin, A., May, B. J., Alt, D., et al. (2005). The complete genome sequence of Mycobacterium avium subspecies paratuberculosis. Proc. Natl. Acad. Sci. U.S.A. 102, 12344–12349.
Liao, C. H., Yao, L. L., and Ye, B. C. (2014). Three genes encoding citrate synthases in Saccharopolyspora erythraea are regulated by the global nutrient-sensing regulators GlnR, DasR, and CRP. Mol. Microbiol. 94, 1065–1084. doi: 10.1111/mmi.12818
Lu, X. Y., Liu, Y. W., Yang, Y. Q., Wang, S. S., Wang, Q., Wang, X. Y., et al. (2019). Constructing a synthetic pathway for acetylcoenzyme A from one-carbon through enzyme design. Nat. Commun. 10, 1378–1388.
Masiewicz, P., Brzostek, A., Wolański, M., Dziadek, J., and Zakrzewska-Czerwińska, J. (2012). A novel role of the PrpR as a transcription factor involved in the regulation of methylcitrate pathway in Mycobacterium tuberculosis. PLoS One 7:e43651. doi: 10.1371/journal.pone.0043651
Mason, R. J., Stossel, T. P., and Vaughan, M. (1972). Lipids of alveolar macrophages, polymorphonuclear leukocytes, and their phagocytic vesicles. J. Clin. Invest. 51, 2399–2407. doi: 10.1172/jci107052
Mckinney, J. D., Zu Bentrup, K. H., Munoz-Elias, E. J., Miczak, A., Chen, B., Chan, W. T., et al. (2000). Persistence of Mycobacterium tuberculosis in macrophages and mice requires the glyoxylate shunt enzyme isocitrate lyase. Nature 406, 735–738. doi: 10.1038/35021074
Munoz-Elias, E. J., and McKinney, J. D. (2005). Mycobacterium tuberculosis isocitrate lyases 1 and 2 are jointly required for in vivo growth and virulence. Nat. Med. 11, 638–644. doi: 10.1038/nm1252
Munoz-Elias, E. J., and McKinney, J. D. (2006). Carbon metabolism of intracellular bacteria. Cell. Microbiol. 8, 10–22. doi: 10.1111/j.1462-5822.2005.00648.x
Munoz-Elias, E. J., Upton, A. M., Cherian, J., and McKinney, J. D. (2006). Role of the methylcitrate cycle in Mycobacterium tuberculosis metabolism, intracellular growth, and virulence. Mol. Microbiol. 60, 1109–1122. doi: 10.1111/j.1365-2958.2006.05155.x
Parish, T., Smith, D. A., Roberts, G., Betts, J., and Stoker, N. G. (2003). The senX3-regX3 two-component regulatory system of Mycobacterium tuberculosis is required for virulence. Microbiology 149, 1423–1435. doi: 10.1099/mic.0.26245-0
Puckett, S., Trujillo, C., Wang, Z., Eoh, H., Ioerger, T. R., Krieger, I., et al. (2017). Glyoxylate detoxification is an essential function of malate synthase required for carbon assimilation in Mycobacterium tuberculosis. Proc. Natl. Acad. Sci. U.S.A. 114, 2225–2232.
Rhee, K. Y., Bryk, R., Ehrt, S., Marrero, J., Park, S. W., Schnappinger, D., et al. (2011). Central carbon metabolism in Mycobacterium tuberculosis: an unexpected frontier. Trends Microbiol. 19, 307–316. doi: 10.1016/j.tim.2011.03.008
Rickman, L., Saldanha, J. W., Hunt, D. M., Hoar, D. N., Colston, M. J., Millar, J. B., et al. (2004). A two-component signal transduction system with a PAS domain-containing sensor is required for virulence of Mycobacterium tuberculosis in mice. Biochem. Biophys. Res. Commun. 314, 259–267. doi: 10.1016/j.bbrc.2003.12.082
Rifat, D., Belchis, D. A., and Karakousis, P. C. (2014). senX3-independent contribution of regX3 to Mycobacterium tuberculosis virulence. BMC Microbiol. 14:265. doi: 10.1186/s12866-014-0265-8
Rifat, D., Bishai, W. R., and Karakousis, P. C. (2009). Phosphate depletion: a novel trigger for Mycobacterium tuberculosis persistence. J. Infect. Dis. 200, 1126–1135.
Rifat, D., and Karakousis, P. C. (2014). Differential regulation of the two-component regulatory system senX3-regX3 in Mycobacterium tuberculosis. Microbiology 160, 1125–1133. doi: 10.1099/mic.0.077180-0
Roberts, G., Vadrevu, I. S., Madiraju, M. V., and Parish, T. (2011). Control of CydB and GltA1 expression by the SenX3 RegX3 two component regulatory system of Mycobacterium tuberculosis. PLoS One 6:e21090. doi: 10.1371/journal.pone.0021090
Russell, D. G., VanderVen, B. C., Lee, W., Abramovitch, R. B., Kim, M. J., Homolka, S., et al. (2010). Mycobacterium tuberculosis wears what it eats. Cell Host Microbe 8, 68–76. doi: 10.1016/j.chom.2010.06.002
Schnappinger, D., Ehrt, S., Voskuil, M. I., Liu, Y., Mangan, J. A., Monahan, I. M., et al. (2003). Transcriptional adaptation of Mycobacterium tuberculosis within macrophages: insights into the phagosomal environment. Exp. Med. 198, 693–704. doi: 10.1084/jem.20030846
Stock, A. M., and Guhaniyogi, J. (2006). A new perspective on response regulator activation. J. Bacteriol. 188, 7328–7330.
Stover, C. K., Delacruz, V. F., Fuerst, T. R., Burlein, J. E., Benson, L. A., Bennett, L. T., et al. (1991). New use of BCG for recombinant vaccines. Nature 351, 456–460.
Sturgill-Koszycki, S., Schlesinger, P., Chakraborty, P., Haddix, P. L., Collins, H. L., Fok, A. K., et al. (1994). Lack of acidification in Mycobacterium phagosomes produced by exclusion of the vesicular proton-ATPase. Science 263, 678–681.
Sweeney, K. A., Dao, D. N., Goldberg, M. F., Hsu, T., Venkataswamy, M. M., Henao-Tamayo, M., et al. (2011). A recombinant Mycobacterium smegmatis induces potent bactericidal immunity against Mycobacterium tuberculosis. Nat. Med. 17, 1261–1268.
Textor, S., Wendisch, V. F., De Graaf, A. A., Muller, U., Linder, M. I., Linder, D., et al. (1997). Propionate oxidation in Escherichia coli: evidence for operation of a methylcitrate cycle in bacteria. Arch. Microbiol. 168, 428–436.
Tischler, A. D., Leistikow, R. L., Kirksey, M. A., Voskuil, M. I., and Mckinney, J. D. (2013). Mycobacterium tuberculosis requires phosphate-responsive gene regulation to resist host immunity. Infect. Immun. 81, 317–328.
Upton, A. M., and McKinney, J. D. (2007). Role of the methylcitrate cycle in propionate metabolism and detoxification in Mycobacterium smegmatis. Microbiology 153, 3973–3982.
Wren, B. W., Colby, S. M., Cubberley, R. R., and Pallen, M. J. (1992). Degenerate PCR primers for the amplification of fragments from genes encoding response regulators from a range of pathogenic bacteria. FEMS Microbiol. Lett. 99, 287–292.
Yao, L., Cengic, I., Anfelt, J., and Hudson, E. P. (2016). Multiple gene repression in cyanobacteria using CRISPRi. ACS Synth. Biol. 5, 207–212.
Keywords: Mycobacterium smegmatis, phosphate metabolism, regX3 regulator, methylcitrate cycle, propionate metabolism
Citation: Pei J-F, Qi N, Li Y-X, Wo J and Ye B-C (2021) RegX3-Mediated Regulation of Methylcitrate Cycle in Mycobacterium smegmatis. Front. Microbiol. 12:619387. doi: 10.3389/fmicb.2021.619387
Received: 20 October 2020; Accepted: 13 January 2021;
Published: 02 February 2021.
Edited by:
Patrick Eichenberger, New York University, United StatesReviewed by:
Tania Lupoli, New York University, United StatesSabine Ehrt, Cornell University, United States
Copyright © 2021 Pei, Qi, Li, Wo and Ye. This is an open-access article distributed under the terms of the Creative Commons Attribution License (CC BY). The use, distribution or reproduction in other forums is permitted, provided the original author(s) and the copyright owner(s) are credited and that the original publication in this journal is cited, in accordance with accepted academic practice. No use, distribution or reproduction is permitted which does not comply with these terms.
*Correspondence: Bang-Ce Ye, bcye@ecust.edu.cn; orcid.org/0000-0002-5555-5359
†These authors have contributed equally to this work