- 1Max Perutz Labs, Vienna Biocenter (VBC), Department of Microbiology, Immunobiology and Genetics, University of Vienna, Vienna, Austria
- 2Institute for Theoretical Chemistry, University of Vienna, Vienna, Austria
- 3Research Group Bioinformatics and Computational Biology, Faculty of Computer Science, University of Vienna, Vienna, Austria
Pseudomonas aeruginosa (Pae) is notorious for its high-level resistance toward clinically used antibiotics. In fact, Pae has rendered most antimicrobials ineffective, leaving polymyxins and aminoglycosides as last resort antibiotics. Although several resistance mechanisms of Pae are known toward these drugs, a profounder knowledge of hitherto unidentified factors and pathways appears crucial to develop novel strategies to increase their efficacy. Here, we have performed for the first time transcriptome analyses and ribosome profiling in parallel with strain PA14 grown in synthetic cystic fibrosis medium upon exposure to polymyxin E (colistin) and tobramycin. This approach did not only confirm known mechanisms involved in colistin and tobramycin susceptibility but revealed also as yet unknown functions/pathways. Colistin treatment resulted primarily in an anti-oxidative stress response and in the de-regulation of the MexT and AlgU regulons, whereas exposure to tobramycin led predominantly to a rewiring of the expression of multiple amino acid catabolic genes, lower tricarboxylic acid (TCA) cycle genes, type II and VI secretion system genes and genes involved in bacterial motility and attachment, which could potentially lead to a decrease in drug uptake. Moreover, we report that the adverse effects of tobramycin on translation are countered with enhanced expression of genes involved in stalled ribosome rescue, tRNA methylation and type II toxin-antitoxin (TA) systems.
Introduction
Pseudomonas aeruginosa (Pae) is an opportunistic pathogen known to cause nosocomial infections that are particularly detrimental to immunocompromised individuals and to patients suffering from cystic fibrosis (CF) (Williams et al., 2010). On the one hand, the pathogenic potential of Pae is based on its metabolic versatility, permitting fast adaptation to changing environmental conditions. On the other hand Pae can form biofilms and produce multiple virulence factors (Kerr and Snelling, 2009; Gellatly and Hancock, 2013). Pae is characterized by high intrinsic resistance to a wide variety of antibiotics. It can further develop resistance by acquisition of genetic determinants through horizontal gene transfer, as well as by mutational processes affecting “resistance genes” that are collectively termed the resistome (Wright, 2007; Fajardo et al., 2008; Breidenstein et al., 2011; Jaillard et al., 2017). In this way, Pae has rendered most antibiotics ineffective, leaving polymyxins and aminoglycosides as last resort antibiotics.
Polymyxins are polycationic cyclic antimicrobial peptides. Owing to their positively charged 2,4-diaminobuteric acid (Dab) moieties they can electrostatically interact with the negatively charged lipopolysaccharide (LPS) of the outer membrane (OM) of Gram-negative bacteria, causing the displacement of LPS-stabilizing divalent cations, Ca2+ and Mg2+. This interaction is followed by insertion of the hydrophobic segments of the drug into the OM and its penetration via a self-promoted uptake mechanism. Cell death subsequently occurs by disintegration of the inner membrane (IM) and leakage of cellular components (El-Sayed Ahmed et al., 2020). Moreover, it has been reported that polymyxins can exert their toxic effects by causing phospholipid exchange between the OM and IM (Velkov et al., 2013), inhibition of respiratory enzymes of the NADH oxidase family (Deris et al., 2014), binding to bacterial DNA and disrupting its synthesis (Kong et al., 2011) and/or formation of reactive oxygen species (ROS) (Sampson et al., 2012; Yu et al., 2017). Nevertheless, the mode of bactericidal action of polymyxins in Pae remains controversial. For instance, a recent study (O’Driscoll et al., 2018) indicated that polymyxin E (colistin) does not exert its antibacterial effect by puncturing the IM or by inhibiting DNA replication and transcription. In addition, the exact contribution of polymyxin induced ROS to lethality of Pae is largely inconclusive (Brochmann et al., 2014; Lima et al., 2019).
In contrast, the regulatory circuits underlying polymyxin resistance are well understood in Pae. An increased resistance is conveyed by reduction of the net negative charge of LPS, resulting in diminished polymyxin binding (Jeannot et al., 2017; Poirel et al., 2017). The cellular machinery for covalent modification of negatively charged lipid A of LPS with positively charged 4-amino-L-arabinose (Lara4N) is encoded by the arn (pmr) operon. This operon is activated by at least five two-component systems (TCS) including PhoP/PhoQ (Barrow and Kwon, 2009), PmrA/PmrB (McPhee et al., 2003), ParR/ParS (Fernández et al., 2010), ColR/ColS and CprR/CprS (Fernández et al., 2012; Gutu et al., 2013). In addition, the cprA gene product was found to be required for polymyxin resistance conferred by the PhoP/PhoQ, PmrA/PmrB, and CprR/CprS TCSs (Gutu et al., 2013). Furthermore, a number of other functions contributing to intrinsic polymyxin resistance have been identified, which mainly affect LPS biosynthesis-related functions (regulatory functions, metabolism, synthesis and transport) (Fernández et al., 2013; Zhang et al., 2017; Sherry and Howden, 2018). Moreover, overproduction of spermidine and of the OM protein OprH have been shown to contribute as well to polymyxin susceptibility, as they can interact with divalent cation-binding sites of LPS, making them inaccessible for polymyxin binding (Young et al., 1992; Johnson et al., 2011). On the other hand, a reduced expression of oprD increased cell survival in the presence of polymyxins through an unknown mechanism (Mlynarcik and Kolar, 2019). Additionally, the MexXY-OprM and MexAB-OprM efflux pump systems can provide low to moderate polymyxin resistance and tolerance respectively (Pamp et al., 2008; Muller et al., 2011; Poole et al., 2015).
Aminoglycosides are positively charged antibiotics that initially interact with LPS of Gram-negative Bacteria. Aminoglycosides require an energized membrane for translocation into the cytoplasm. Once inside the cells, they bind to 16S rRNA at the A-site of the 30S ribosomal subunit, disrupting translation and causing the synthesis of aberrant polypeptides. These polypeptides can be inserted into the cell membrane, causing membrane damage, which leads to further intracellular accumulation of aminoglycosides. The established autocatalytic loop of membrane damage and their increased uptake results in stalling of ribosomes, and in complete inhibition of protein synthesis (Krause et al., 2016).
Covalent modifications of the negatively charged moieties of LPS, 16S rRNA methylation by RNA methyltransferases, ribosomal mutations and aminoglycoside modifying enzymes (AMEs) are exploited by Pae to counteract aminoglycosides (Poole, 2005; Garneau-Tsodikova and Labby, 2016; Krause et al., 2016; Valderrama-Carmona et al., 2019). The main efflux system responsible for the extrusion of aminoglycosides is MexXY-OprM (Poole, 2005). Its synthesis is controlled by PA5471, an anti-repressor of the mexXY operon repressor MexZ (Morita et al., 2006; Hay et al., 2013). Additional efflux systems include MexAB-OprM and an ortholog of the EmrE multidrug transporter of Escherichia coli (Poole, 2005; Nasie et al., 2012). Moreover, protein chaperones such as GroEL/ES, GrpE and HtpX, as well as the AmgR/AmgS TCS have been implicated in protecting the cells from polypeptides arising from drug induced mistranslation (Hinz et al., 2011; Wu et al., 2015).
A number of studies have confirmed the safety of colistin for treatment of acute pulmonary infections, while tobramycin was proven effective in suppressing chronic Pae airway infections in CF patients (Ramsey et al., 1999; Garnacho-Montero et al., 2003). However, in recent years a gradual decrease in baseline susceptibility of Pae to these last resort antibiotics was observed (Obritsch et al., 2004; Wi et al., 2017; Jain, 2018). As a refined understanding of the molecular regulatory circuits that contribute to resistance, tolerance and persister cell formation is key to develop new strategies/tools to combat Pae, we have employed RNA-seq and Ribo-seq in parallel to monitor gene expression responses of the clinical Pae isolate PA14 grown in synthetic cystic fibrosis sputum medium (SCFM) to inhibitory concentrations of colistin and tobramycin.
In addition to arn operon activation, which is known to result in reduced drug uptake, Pae responds to colistin by launching an anti-oxidative response, and by de-regulating genes belonging to the MexT and AlgU regulons. Concerning tobramycin, Pae seemingly goes through metabolic changes and envelope remodeling to prevent drug uptake, whereas its ramifications on translational processes are met with the stalled ribosome rescue response and the activation of type II toxin-antitoxin (TA) systems.
Materials and Methods
Bacterial Strains and Growth Conditions
The clinical isolate Pae PA14 (Rahme et al., 1995) was used in all gene expression profiling experiments. Synthetic cystic fibrosis sputum medium (SCFM) was prepared as previously described (Palmer et al., 2007) with the modification specified in Tata et al. (2016). PA14 cells were grown aerobically in 500 ml SCFM at 37°C. At an OD600 of 1.7, the cultures were treated with inhibitory concentrations of colistin (8 μg/ml; Sigma) and tobramycin (64 μg/ml; Sigma), respectively, or water was added as a control. The cultures reached OD600 of 2 approximately 2 h after exposure to the antibiotics, as can be inferred from Supplementary Figure 1. 10 ml samples were withdrawn for RNA-seq analyses, while the remaining culture volume was used for the Ribo-seq experiments. The strain PA14ΔalgU was constructed as described in the Supplementary Text.
RNA-Seq
Total RNA was isolated from two biological replicates using the Trizol method (Ambion) according to the manufacturer’s instructions. The samples were treated with DNase I (TURBOTM DNase, Thermo Scientific), followed by phenol-chloroform-isoamyl alcohol (25:24:1) extraction and ethanol precipitation. Ribosomal RNA was depleted with The Ribo-ZeroTM rRNA Removal Kit. The libraries were constructed using the NEBNext® UltraTM Directional RNA Library Prep Kit for Illumina®. Hundred bp single end sequence reads were generated using the Illumina HiSeq200 platform at the in house Next Generation Sequencing Facility (VBCF, Vienna, Austria1). Quality control assessment of the raw reads using FastqQC2 obviated further pre-processing. Sequencing adapter removal was performed with cutadap (Martin, 2011). Mapping of the samples against the PA14 reference genome (NCBI accession number NC_008463.1) was performed with Segemehl (Hoffmann et al., 2009) with default parameters. Reads mapping to rRNA or tRNA genes were discarded from all data and ignored for all follow-up analyses. The mapped sequencing data were prepared for visualization using the ViennaNGS tool box and visualized with the UCSC Genome Browser (Wolfinger et al., 2015). Reads per gene were counted using BEDTools (Quinlan and Hall, 2010) and the Refseq annotation of Pae (NC_002516.2). Differential gene expression analysis was performed with DESeq (Anders and Huber, 2010). All genes with a fold-change (FC) greater than ±2 and a multiple testing adjusted p-value below 0.05 were considered to be significantly modulated. The raw sequencing data were deposited in the European Nucleotide Archive (ENA) under accession number PRJEB41029.
Ribo-Seq
Ribosome profiling of elongating ribosomes (Ribo-seq; Ingolia et al., 2009) was performed with the same cultures as used for the RNA-seq analyses. Upon culture growth, the cells were treated for 10 min with chloramphenicol (300 μg/ml) to stop translation, and then harvested by centrifugation at 8,000 g for 15 min at 0°C. The cells were washed in 50 ml ice cold lysis buffer (10 mM MgOAc, 60 mM NH4Cl, 10 mM TRIS-HCl, pH 7.6) and again pelleted by centrifugation at 5000 g for 15 min at 4°C. The pellets were re-suspended in 1 ml ice cold lysis buffer containing 0.2% Triton X-100, 100 μg/ml chloramphenicol and 100 U/ml DNAse I, frozen in liquid nitrogen and cryogenically pulverized by repeated cycles of grinding in a pre-chilled mortar and freezing in a dry ice/ethanol bath. These lysates were centrifuged at 15,000 g for 30 min at 4°C to remove cellular debris. Hundred μl aliquots of the cleared lysates were treated with 4 μl of Micrococcal Nuclease (MNase, NEB) and 6 μl of the RiboLock RNase inhibitor (Thermo Scientific) for 1 h at 25°C with continuous shaking at 450 rpm. The lysates were then layered onto 10–40% linear sucrose density gradients in lysis buffer and centrifuged at 256,000 g for 3 h at 4°C. Five hundred μl gradient fractions were collected by continuously monitoring the absorbance at 260 nm. The RNA was extracted from fractions containing 70S ribosomes with phenol-chloroform-isoamyl alcohol (25:24:1), and precipitated with ethanol. The samples were then treated with DNase I (TURBOTM DNase, Thermo Scientific) and separated on a 15% polyacrylamide gel containing 8M urea. Ribosome protected mRNA fragments (ribosomal footprints) ranging in size of 20–40 nucleotides were removed and eluted from the polyacrylamide gel by overnight incubation in elution buffer (0.3 M NaOAc, 1 mM EDTA) at 4°C, which was followed by an additional round of phenol-chloroform-isoamyl alcohol (25:24:1) extraction and ethanol precipitation. The quality of RNA samples was subsequently analyzed with a 2100 Bioanalyzer and an Aligned RNA 6000 Pico Kit (Aligned Technologies). The RNA was further processed into cDNA libraries with NEBNextTM Small RNA Library Prep Set for Illumina® and their quality was assessed with the 2100 Bioanalyzer and a High Sensitivity DNA Kit (Agilent Technologies). Pipin PrepTM was used to purify the 140–160 bp cDNA products which corresponded to adapter-ligated 20–40 nucleotide long ribosomal footprints. RNA sequencing and data processing was performed as described above. The raw sequencing data were deposited in the ENA under accession number PRJEB41027.
Results and Discussion
Quality Assessment and Data Analysis
To determine the effect of colistin and tobramycin on gene expression, parallel RNA-seq and Ribo-seq experiments were performed with planktonically grown PA14. The cultures reached OD600 of 2 approximately 2 h after exposure to the antibiotics, as can be inferred from Supplementary Figure 1. As a control, total RNA and ribosome protected mRNA fragments (ribosomal footprints) were isolated from cultures grown without antibiotics. As the number of ribosomal footprint sequencing reads have been shown to correlate with those obtained from RNA-seq experiments (Ingolia et al., 2009), we first determined the representative gene expression correlations between RNA-seq and Ribo-seq. The number of RNA-seq and Ribo-seq sequencing reads were normalized (BaseMean), and the Spearman correlation value (ρ-value) between them was assessed for each condition (controls, colistin and tobramycin treatment). The correlation coefficient between the average Ribo-seq and RNA-seq BaseMean expression values was 0.68 for the control, 0.81 for colistin, and 0.91 for tobramycin treated samples, respectively (Figure 1). Similar ρ-values have been also reported by other studies (Blevins et al., 2018).
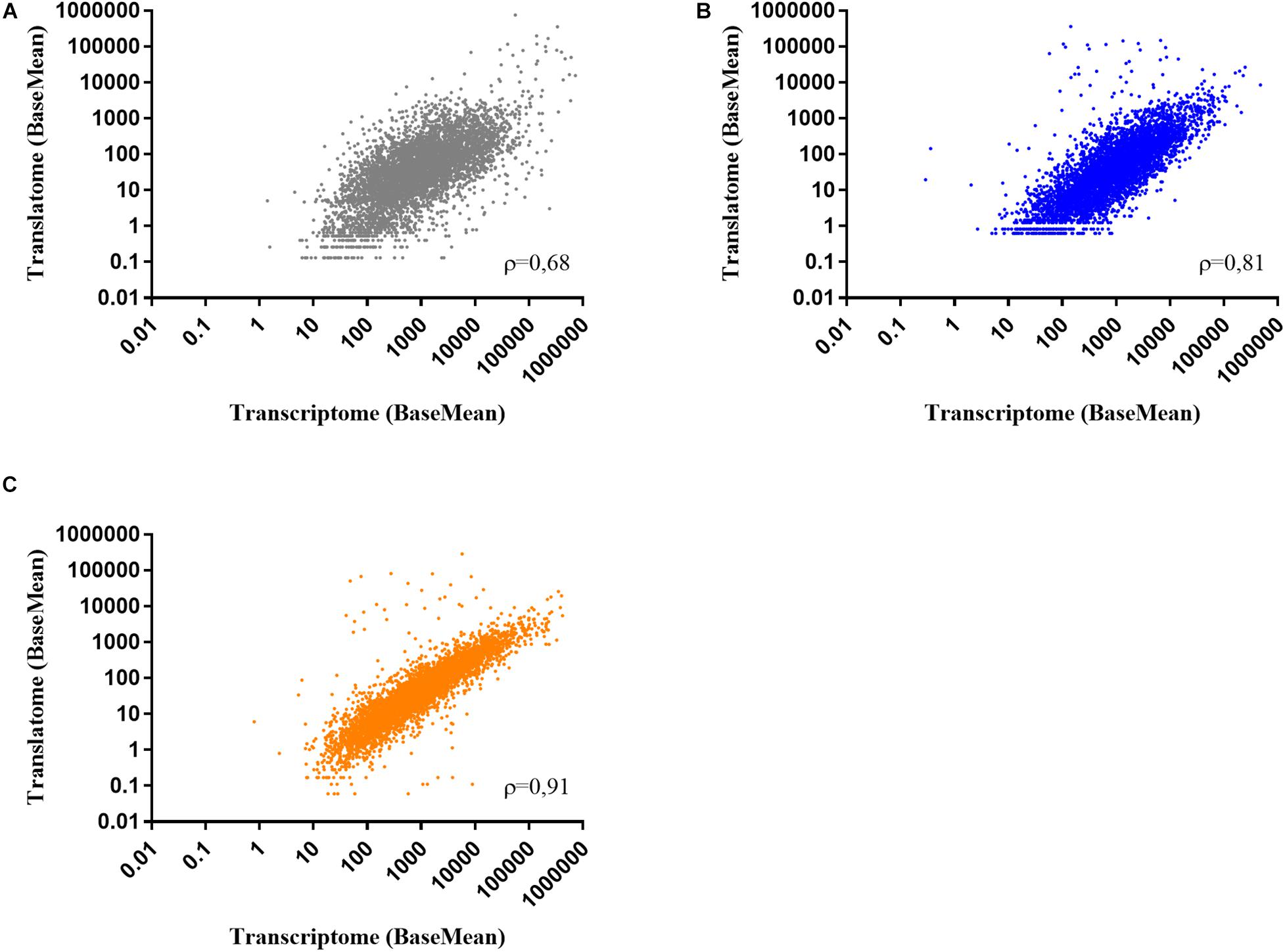
Figure 1. Scatter plots showing the correlation between normalized RNA-seq and Ribo-seq sequencing reads (BaseMean) obtained from two biological replicates of (A) control, (B) colistin, and (C) tobramycin treated samples. ρ – Spearman correlation value.
Next, the FC in transcript abundance between antibiotic treated and untreated samples was calculated. The following criteria were applied for differential gene expression analysis and interpretation: (i) only annotated genes deposited in the Pseudomonas genome database (Winsor et al., 2016) were considered for comparison; (ii) genes with a low expression level (less than 100 RNA-seq or 50 Ribo-seq reads) were disregarded; (iii) for all data sets a p-value (adjusted for multiple testing) of 0.05 was set as a threshold for significance and (iv) the change in FC had to exceed ± 2 for a given gene to be regarded as differentially expressed. When compared with the sequencing data acquired from the non-treated samples, 2056 and 3558 genes were found to be differentially abundant in RNA-seq after exposure to colistin and tobramycin, respectively, whereas that number in Ribo-seq amounted to 1124 and 1045 (Figure 2 and Supplementary Table 1). The scatter plots depicting the correlation between RNA-seq and Ribo-seq gene FC values are shown in Figure 3. Discrepancies in the number of de-regulated genes in RNA-seq when compared to Ribo-seq data have been reported before (Blevins et al., 2018), highlighting the importance of parallel application of these methods for assessment of gene expression. Interestingly, the vast majority of genes were significantly differentially expressed solely at the transcriptional or at the translational level by colistin and tobramycin. 1546 genes were de-regulated by colistin exclusively at the transcriptional level, whereas 614 genes were only affected at the translational level. In case of tobramycin 2778 genes showed FC values that exceeded ± 2 only in the RNA-seq data, while 274 genes were differentially expressed only in the Ribo-seq data. Moreover, 173 and 75 transcripts displayed opposite FC values in the two data sets after treatment with colistin and tobramycin, respectively (Figure 2 and Supplementary Table 1). These results showed that the differentially abundant transcripts observed with RNA-seq did not highly correlate with the outcome of the Ribo-seq analyses and vice versa. An explanation for this observation could be that the expression of these genes is post-transcriptionally regulated. In any case, the patterns of PseudoCAP functional class distribution of annotated transcripts with altered expression in response to colistin or tobramycin were similar for the transcriptome and translatome data (Figure 4).
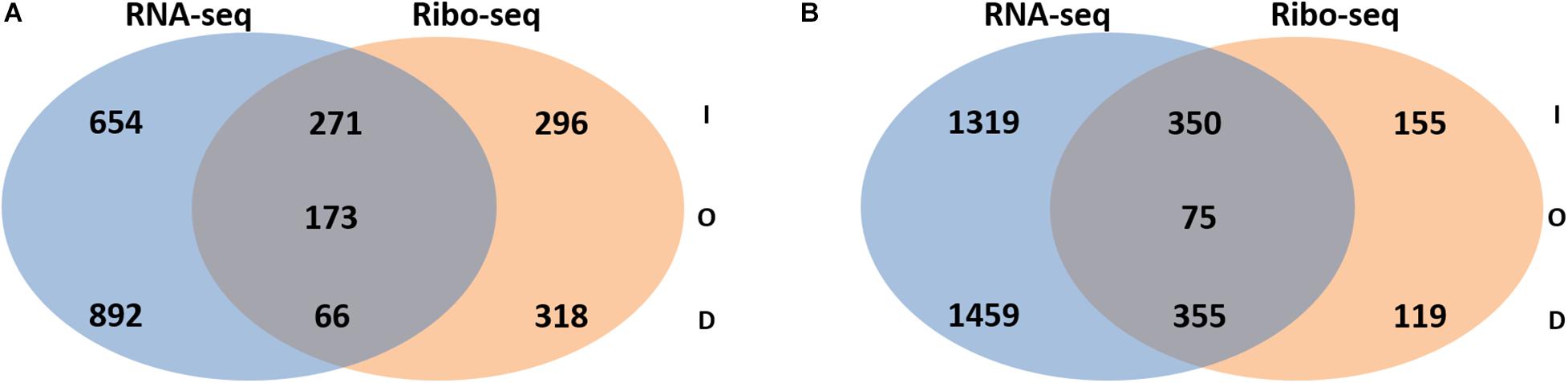
Figure 2. Venn diagram showing the number of transcripts with increased (I), decreased (D) or opposite (O) abundance in RNA-seq and Ribo-seq data obtained after (A) colistin treatment and (B) tobramycin treatment. For significance, only transcripts with a fold-change ≥ 2 or ≤ –2 and a multiple testing adjusted p-value ≤ 0.05 were considered. The corresponding transcripts and ribosomal footprints with increased, decreased and opposite abundance are listed in Supplementary Table 1.
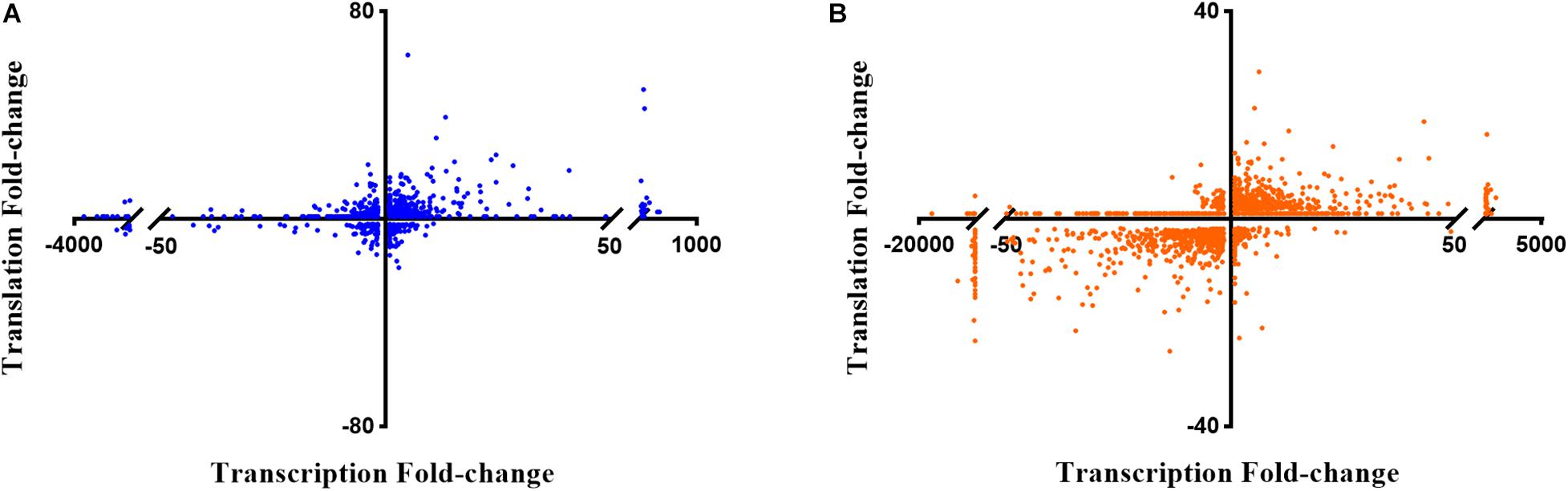
Figure 3. Scatter plots showing the correlation between gene expression fold-changes in RNA-seq and Ribo-seq data obtained after (A) colistin treatment and (B) tobramycin treatment. The X-axis corresponds to the RNA-seq data, or transcriptome, and the Y-axis to the Ribo-seq data, or translatome.
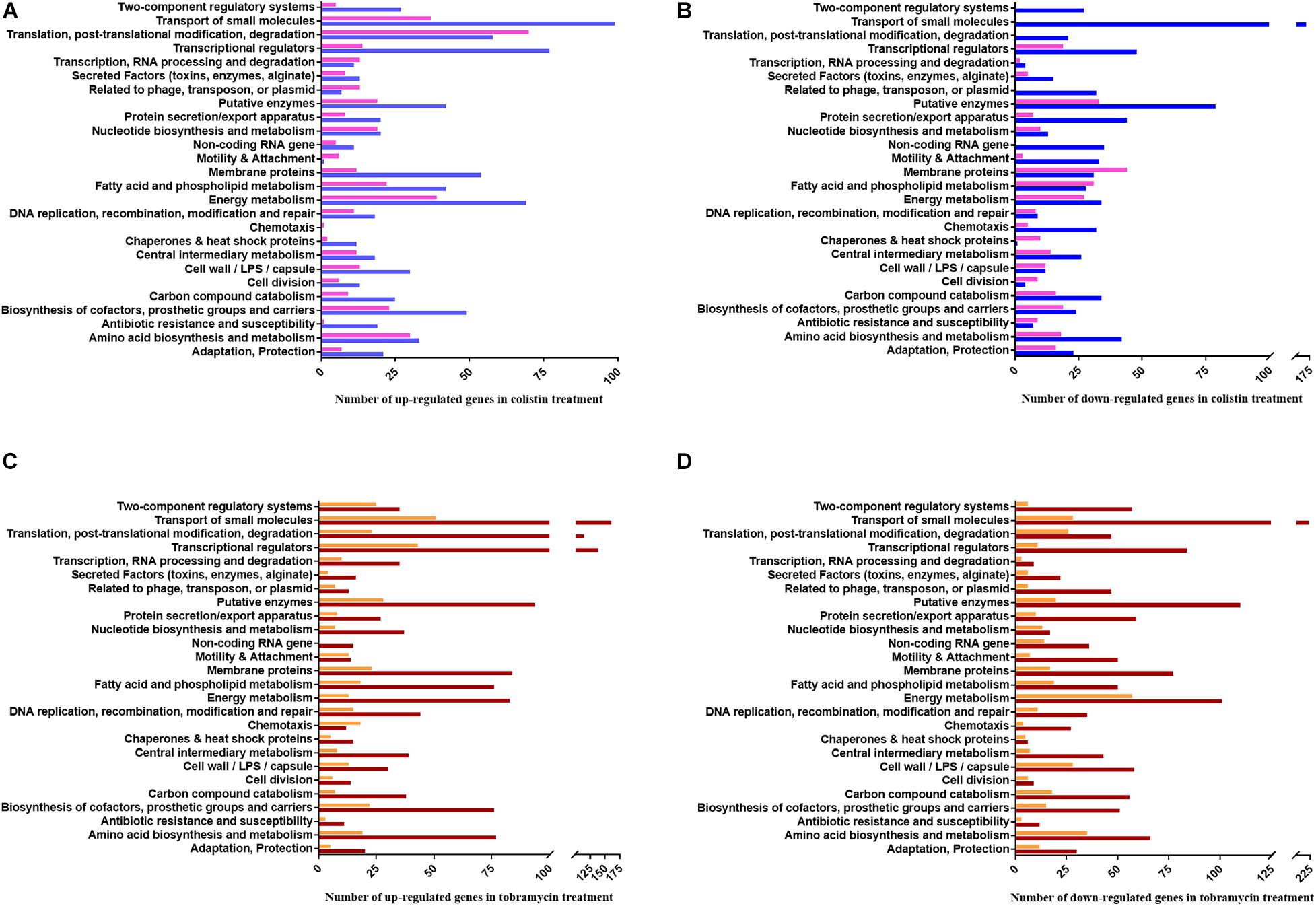
Figure 4. PseudoCAP functional class distribution of annotated genes with altered expression in response to colistin or tobramycin. (A) Up-regulated and (B) down-regulated genes in colistin treated samples. Blue and pink bars indicate the number of de-regulated genes based on the RNA-seq and Ribo-seq data, respectively. (C) Up-regulated and (D) down-regulated genes in tobramycin treated samples. Brown and orange bars indicate the number of de-regulated genes based on the RNA-seq and Ribo-seq data, respectively.
Known Gene Expression Responses to Colistin and Tobramycin
To validate our data, we first scrutinized an assortment of genes known to be involved in maintenance of intrinsic and/or adaptive resistance of Pae toward colistin and tobramycin. In the case of colistin, we assessed the expression levels of the oprD, pmrA, and pmrB transcripts and of genes involved in the synthesis (i) and modification of LPS (such as the arn operon, pagL, lpxO2, lpxC, and galU), (ii) of spermidine (PA14_63110 – PA14_63120), (iii) of the short-chain dehydrogenase/reductase family protein CprA (PA14_43311) and (iv) of the MexXY (PA14_38395-AmrB) and MexAB-OprM efflux pumps. As anticipated, the above mentioned genes were up-regulated upon colistin treatment, with the exception of oprD whose expression was down-regulated (Supplementary Table 2).
In the case of tobramycin, the abundance of genes known to be involved in (i) drug modification (aph), (ii) target binding inhibition (rsmE), (iii) extrusion (mexXY operon anti-repressor PA14_72210), (iv) maintenance of the cell membrane (groEL/ES, grpE, and htpX) as well as the genes encoding the AmgR/AmgS (OmpR/EnvZ) TCS were scrutinized. The transcription and/or translation of all the above mentioned genes was enhanced upon tobramycin treatment (Supplementary Table 2).
At a glance, energy metabolism-, translation-, and transcription- functional classes of genes were up-regulated after colistin exposure. On the other hand, colistin appears to negatively affect the abundance of mRNAs encoding functions involved in transport of small molecules, motility and attachment. Moreover, it translationally impaired expression of membrane protein genes (Figure 4).
The functional classes representing the majority of predominantly positively affected genes by tobramycin are related to transcriptional regulators, RNA processing and translation, whereas the most down-regulated gene functions are involved in energy metabolism, carbon compound catabolism and cell wall/LPS/capsule synthesis. Interestingly, motility and attachment genes were prominently down-regulated by tobramycin at the transcriptional level, whereas amino acid biosynthesis and metabolism genes were apparently more negatively affected at the translational level (Figure 4).
To find additional players and pathways involved in colistin and/or tobramycin resistance in Pae, we next took a closer look at all genes which displayed a ±10 FC in transcript abundance in antibiotic treated samples in the RNA-seq and/or Ribo-seq data sets (Supplementary Table 1).
Colistin Induces Oxidative Stress Response Genes
The accepted mode of action of polymyxins, i.e., causing a lesion in the IM, has been challenged by the finding that even supra-bactericidal colistin concentrations induced minor loss of intracellular components (O’Driscoll et al., 2018). However, polymyxins are also known to elicit oxidative damage in Bacteria through the production of ROS, such as superoxide O2–, hydrogenperoxide H2O2 and hydroxy radicals ⋅OH (El-Sayed Ahmed et al., 2020). Both O2– and H2O2 can injure proteins that possess iron–sulfur ([Fe–S]) clusters as cofactors. The maintenance of [Fe–S] proteins is of importance as they are required for many biological processes, including protein biosynthesis, respiration, central metabolism, photosynthesis, nitrogen fixation, DNA repair, RNA modification and gene regulation (Roche et al., 2013; Kimura and Suzuki, 2015). Polymyxin induced oxidative damage has been reported for Gram-negative and Gram-positive species, including Acinetobacter boumannii (Sampson et al., 2012), Pae (Brochmann et al., 2014; Lima et al., 2019), Bacillus subtilis, and the natural producer Paenibacillus polymyxa (Yu et al., 2019). Studies performed on the Gram-positive P. polymyxa provided a detailed explanation of how polymyxins might lead to ROS production. It has been hypothesized that colistin stimulates the tricarboxylic acid (TCA) cycle through an increase in the production of isocitrate (icdA), α-ketoglutaric (sucB), and malate (mdh) dehydrogenases, which in turn leads to increased NADH production and enhanced respiration rates (Yu et al., 2019). Accordingly, the concentration of O2– surges intracellularly, where it can be converted to H2O2 by the superoxide dismutase (SOD). The sodA (Mn-SOD) and sodB (Fe-SOD) genes were up-regulated in P. polymyxa in the presence of colistin (Yu et al., 2017), while inactivation of sodB in the Gram-negative bacterium A. boumannii augmented its susceptibility to the same drug (Heindorf et al., 2014). Moreover, the involvement of sodC (CuZn-SOD) and catalase encoding katA genes in polymyxin resistance was observed in A. boumannii and Staphylococcus aureus, respectively (Antonic et al., 2013; Pournaras et al., 2014).
In our study, exposure of Pae to inhibitory concentration of colistin resulted in an up-regulation of genes involved in the oxidative stress response (Table 1 and Supplementary Table 1). These genes include aphF (Ochsner et al., 2000b), iscR (PA14_14710) (Romsang et al., 2014), the PA14_21570-PA14_21580-PA14_21590-PA14_21600 operon (Farrant et al., 2020), and PA14_22320 (Salunkhe et al., 2005). Next, we assessed whether additional genes required for alleviation of ROS were differentially expressed upon colistin treatment, but were initially not accounted for due to the set ±10 FC threshold. The catalase encoding katA and katB genes (Brown et al., 1995; Ma et al., 1999), as well as the gene encoding their regulator OxyR (Wei et al., 2012) were up-regulated at both the transcriptional and translational level (Table 1 and Supplementary Table 1). However, the alkyl hydroperoxide reductase gene ahpB (Ochsner et al., 2000b) and the superoxide dismutase gene sodB (Hassett et al., 1993, 1995) were only found to be up-regulated in the RNA-seq data (Table 1 and Supplementary Table 1). Moreover, the soxR gene and the majority of genes regulated by the redox-responsive SoxR regulator (mexG, mexH, mexI, PA14_16310, and PA14_35160) (Palma et al., 2005) displayed an increased transcript and ribosomal footprint abundance (Table 1 and Supplementary Table 1).

Table 1. Gene expression response of PA14 grown in the presence of colistin versus untreated control.
The toxicity of ROS-generating agents is magnified by ferrous ions (Fe2+) through the Fenton reaction (Kohanski et al., 2007; Yeom et al., 2010), wherein H2O2 is oxidized by Fe2+ to generate OH radicals. These can inactivate enzymes and cause DNA and membrane damage, leading to growth arrest and ultimately to cell death (Yeom et al., 2010). Thus, Bacteria generally establish a tight control on expression of iron homeostasis genes. For instance, in P. polymyxa the levels of the transcriptional regulator Fur, which represses iron acquisition genes, are increased upon colistin treatment (Yu et al., 2017). Fe2+ can be directly taken up from environment or it can be generated through reduction of free intracellular ferric (Fe3+) ions bound to siderophores such as pyoveridine (PVD) or pyochelin (PCH), to iron-sulfur ([Fe–S]) cluster proteins or to heme (Ochsner et al., 2000a; Ratledge and Dover, 2000; Wandersman and Delepelaire, 2004; Cartron et al., 2006). Therefore, we also scrutinized the levels of transcripts encoding genes for iron acquisition and storage upon exposure to colistin. As judged from the RNA-seq data, the genes required for PVD biosynthesis and transport (Lamont and Martin, 2003), for heme uptake (has locus; Ochsner et al., 2000a), the Feo system of Fe2+ uptake and the TonB2-ExbB1-ExbD1 complex (Zhao and Poole, 2000), which serves as an energy coupler for active iron transport across the outer membrane, were down-regulated (Table 1 and Supplementary Table 1). In addition, the majority of these genes was apparently not efficiently translated in neither the control nor in the colistin treated samples (≤50 Ribo-seq reads). Visual inspection of their sequencing profiles in the UCSC Genome Browser (Wolfinger et al., 2015) revealed a very low ribosomal coverage (Supplementary Figure 2), which might be caused by the applied iron rich culturing conditions. For example, siderophore synthesis in Pseudomonas sp. is fully inhibited at >4–10 μM iron (Meyer, 1978; Dumas et al., 2013), a concentration far below of what was used in our experimental setup (100 μM FeSO4). Counterintuitively, the genes for pyochelin synthesis and uptake are apparently translated in the presence of colistin (Table 1 and Supplementary Table 1). As pyochelin has a weaker affinity for iron when compared with pyoveridine (Dumas et al., 2013), ongoing synthesis could be necessary to meet sufficient metabolic requirements for iron.
In most Gram-negative Bacteria the ferric uptake regulator Fur complexed with Fe2+ is responsible for preventing the synthesis of PVD and PCH in iron replete conditions (Ochsner and Vasil, 1996; Vasil and Ochsner, 1999). Analogously to P. polymixa, fur was slightly up-regulated in both the RNA-seq and Ribo-seq data (Supplementary Table 1). Therefore, an explanation for the apparent translation of the PCH genes remains elusive.
An additional link between colistin resistance and iron homeostasis can be found in the increased synthesis of PA14_04180 (Table 1 and Supplementary Table 1), a putative periplasmic protein with a bacterial oligonucleotide/oligosaccharide-binding (OB-fold) domain, which can bind cationic ligands (Ginalski et al., 2004). Gene PA14_04180 was found to be regulated by the calcium responsive TCS CarS/CarR and the ferrous iron responsive BqsS/BqsR TCS (Kreamer et al., 2012; Guragain et al., 2016). The BqsS/BqsR system contributes to cationic stress tolerance as it is regulating the expression of several genes with known or predicted functions in polyamine biosynthesis/transport or polymyxin resistance in Pae (i.e., arnB, oprH, and PA14_63110) (Kreamer et al., 2015). Moreover, a periplasmic OB-fold protein OmdA, similar to PA14_04180, is controlled by the PmrA/PmrB TCS and was found to confer resistance to polymyxin B (Pilonieta et al., 2009).
In contrast to P. polymyxa (Yu et al., 2019), we did not notice an up-regulation of TCA cycle genes or drastic changes in expression of the NADH oxidase family genes (Supplementary Table 1). Alternatively, it has been hypothesized that O2– production could be induced in Gram-negative Bacteria during transit of polymyxin molecules through the cell envelope (Kohanski et al., 2007; El-Sayed Ahmed et al., 2020) and via inhibition of type II NADH-quinone oxidoreductases (NDH-2) (Deris et al., 2014).
Denitrification Pathway Genes Are De-Regulated in the Presence of Colistin
The transcripts encoding for enzymes required for the denitrification pathway (Schreiber et al., 2007), which include the nitrite reductase encoding nir-operon, the nitric oxide reductase encoding nor-operon and the nitrous dioxide reductase encoding nos-operon, were highly abundant relative to their representation in cells growing in the absence of colistin. Similar trends in expression were observed in the Ribo-seq data set, albeit not to the same degree (Table 1 and Supplementary Table 1). Surprisingly, the master regulator of the denitrification pathway ANR and the genes of the nar-operon, which encode nitrate reductase, a complex that catalyzes the first step of denitrification, were down-regulated after colistin treatment (Table 1 and Supplementary Table 1). It is possible that the ParR/ParS TCS positively regulates several genes involved in anaerobic respiration (nirC, norC, norB, nosZ, and nosL) (Fernández et al., 2010), however the reasons for activating the anaerobic respiratory chain in presence of colistin remain to be elucidated.
Colistin Induced Up-Regulation of the MexT Regulon
Colistin caused a significant up-regulation of the PA14 genes PA14_22420, PA14_22740, PA14_28410, mexF, mexE, PA14_32480, PA14_32490, PA14_39060, PA14_39420, PA14_41990, PA14_56620, PA14_56640, and PA14_64530 (Table 1 and Supplementary Table 1), all of which belong to the MexT regulon (Tian et al., 2009a; Hill et al., 2019). MexT is a transcriptional regulator of the LysR family known to control the expression of pathogenicity, virulence and antibiotic resistance determinants in Pae (Köhler et al., 1997a,b, 1999; Tian et al., 2009a; Huang et al., 2019). MexT regulates gene expression either directly through binding to the promoter region of distinct target genes, or indirectly through the activation of the MexEF-OprN efflux pump (Tian et al., 2009a,b; Olivares et al., 2012). Furthermore, MexT is a redox-responsive transcriptional activator implicated in diamide stress tolerance, in defense against the innate immune system-derived oxidant hypochlorous acid and against nitrosative stress (Fetar et al., 2011; Fargier et al., 2012; Farrant et al., 2020). Thus, the observed activation of MexT regulated genes might be a result of a defense mechanism being triggered against oxidative stress that arises as a consequence of colistin activity. As mentioned above, the denitrification pathway (nir, nor, and nos genes) was up-regulated in the presence of colistin (Table 1 and Supplementary Table 1), hence it is tempting to speculate whether this antibiotic can additionally inflict nitrosative stress to Pae. Moreover, Wang et al. (2013) showed that the deletion of parR and parS in Pae strain PAO1 negatively impacts the transcript abundance of genes belonging to the MexT regulon, without affecting the expression levels of mexT.
Colistin Impacts the AlgU Regulon
Schulz et al. (2015) predicted that the primary regulon of the alternative sigma factor σ22 (AlgU or AlgT) in PA14 comprises 341 genes, while their mRNA profiling approach uncovered 222 genes that were down-regulated in an algU deletion- and up-regulated in an algU overexpressing strain, or vice versa. Our RNA-seq and Ribo-seq data sets show that colistin caused a change in expression at the transcriptional and/or the translational level of 141 out of those 222 AlgU-dependent genes (Supplementary Table 3). Envelope stress inducing agents cause proteolytic degradation of the AlgU anti-sigma factor MucA through regulated intramembrane proteolysis (RIP), which leads to the release of AlgU from the IM, and ultimately to the activation of the AlgU regulon (Wood et al., 2006; Damron and Goldberg, 2012). It is possible that the genes controlled by AlgU play a significant role in colistin susceptibility in Pae, as polymyxins have long been implicated in triggering envelope stress in Gram-negative Bacteria. As the transcription of algU itself was only slightly increased (2.65- fold) upon exposure to colistin (Supplementary Table 1), the regulation of the AlgU activity through RIP might explain the observed alterations in expression of the AlgU regulon. In view of our studies, we compared the susceptibility toward colistin of PA14 with an isogenic in frame algU deletion mutant. When compared with the PA14 WT strain, the minimal inhibitory concentration of colistin for PA14ΔalgU was approximately fourfold reduced (Supplementary Figure 3), showing that the AlgU-dependent response counteracts the deleterious effects of colistin. In line with our observations, Murray et al. (2015) reported that a transposon insertion in algU affects the fitness of Pae in the presence of polymyxin B.
Colistin Affects Multiple Efflux-Pump Genes
Besides the aforementioned MexXY-OprM, MexAB-OprM, MexEF-OprN, and MexGHI-OpmD efflux pumps, a strong colistin-dependent induction of the mexCD-oprJ and mexJK operons was observed (Table 1 and Supplementary Table 1). Expression of mexCD-oprJ was shown to be enhanced by polymyxin B in an AlgU-dependent manner (Fraud et al., 2008), whereas the MexJK efflux system has so far not been linked to polymyxin susceptibility.
Tobramycin Down-Regulates Amino Acid Catabolism and Lower Tricarboxylic Acid Cycle Genes
The insertional inactivation of the genes encoding the Nuo and Nqr dehydrogenases was shown to increase tobramycin resistance of Pae (Schurek et al., 2008; Kindrachuk et al., 2011). It was hypothesized that their inactivation causes a reduction in the proton motive force and energy production, hence limiting the active uptake of tobramycin. The nuo and nqr genes were among the most down-regulated genes in the RNA-seq and Ribo-seq data after tobramycin treatment (Table 2 and Supplementary Table 1).

Table 2. Gene expression response of PA14 grown in the presence of tobramycin versus untreated control.
The catalytic activities of the isocitrate dehydrogenase Idh, the dihydrolipoamide succinyltransferase SucB and the aconitate hydratase PA14_53970 result in an increased NADH content and promote cellular respiration (Kohanski et al., 2007; Meylan et al., 2017). Upon tobramycin treatment, a down-regulation was observed for these genes of the lower part of the tricarboxylic acid (TCA) cycle (idh, sucB, and PA14_53970) (Table 2 and Supplementary Table 1). The diminished synthesis of enzymes of the lower part of TCA cycle upon tobramycin treatment is in agreement with a recent study, which suggested that Pae can bypass the decarboxylation steps of the cycle to reduce the NADH content, thus decreasing energy production. Growth of Pae on glyoxylate as a sole carbon source leads to the activation of this bypass, and consequently an increase in tobramycin resistance (Meylan et al., 2017).
Amino acid catabolism promotes the production of intermediate metabolites like fumarate, pyruvate, acetyl-CoA and α-ketoglutarate that fuel the TCA cycle, and thus could promote aminoglycosides uptake. Indeed, Pae responds to tobramycin by down-regulating genes encoding enzymes required for glycine and serine (glyA2, gcvT2, sdaA), phenylalanine (pheB, pheC, maiA, fahA), arginine (arcABD) and branched-chain amino acid (bkdA1A2B-lpdV, gnyRBDHL and ldh) catabolism (Table 2 and Supplementary Table 1).
Moreover, we also noted that the utilization pathways of D-alanine for peptidoglycan synthesis and transport (ddl, mraY murC, murG, murD, murE) and for glycogen metabolism (PA14_36570, PA14_36580, PA14_36590, PA14_36605, PA14_36620, PA14_36630, glgB, PA14_36730) were down-regulated after exposure to tobramycin (Table 2 and Supplementary Table 1).
Tobramycin Affects the Expression of Functions Involved in Pathogenicity, Virulence and Transport
The comparative transcriptome and translatome analyses of PA14 treated with tobramycin uncovered a significantly reduced abundance of several virulence and pathogenicity related genes (Table 2 and Supplementary Table 1). The genes encoding the type II (xcp locus) and type VI (tss, hsi, and hcp-1 locus) secretion systems, the quinolone-based quorum-sensing system (pqs genes) as well as the esterase (estA) were down-regulated in the RNA-seq and Ribo-seq data sets. Additionally, genes encoding for functions required for motility, attachment, pilus and fimbrial assembly (PA14_55780-PA14_55940 including tad locus, pil, flg, fli, and flh genes) were primarily down-regulated at the transcriptional level. Pae may prevent tobramycin uptake through the down-regulation of genes of different secretion systems, as they are believed to be the entry gates for several structurally unrelated antimicrobial agents (Tzeng et al., 2005; Mulcahy et al., 2006). Although insertional inactivation of pilZ and fimV have been confirmed to confer low-level tobramycin resistance, it seems interesting to assess the contribution of other motility and attachment genes to aminoglycoside permeability, such as those of the tad locus (Schurek et al., 2008).
The genes encoding ABC transporters such as the dipeptide permease Dpp, involved in the uptake of kasugamycin in E. coli (Shiver et al., 2016), the permease Npp, which plays a role in the translocation of the uridyl peptide antibiotic pacidamycin in Pae (Luckett et al., 2012; Pletzer et al., 2015) and the ATP-binding protein OpuC, showed a tobramycin-dependent down-regulation in both, the RNA-seq and Ribo-seq data. Moreover, the Na+/H+ antiporter pha (sha) operon important for the homeostasis of monovalent cations (Kosono et al., 2005) was also strongly down-regulated after exposure to tobramycin (Table 2 and Supplementary Table 1). The Na+/H+ Sha antiporter shows similarity to the membrane subunits of the respiratory Nuo complex and could therefore be of interest for further analysis with regard to its potential impact on the proton motive force and thus aminoglycoside uptake (Mathiesen and Hägerhäll, 2003).
Tobramycin Impacts the Abundance of Genes Involved in Translation
Tobramycin promotes mistranslation, stop codon read-through and ribosome stalling (Aboa et al., 2002; Thompson et al., 2002; Harms et al., 2003; Vioque and Cruz, 2003). A number of genes related to translation were strongly up-regulated in the RNA-seq and Ribo-seq data, including the genes encoding translation initiation factor 3 (infC), ribosomal proteins (rpsB, rplC, rplD), a putative ribosomal maturation factor (yhbC), elongation factor EF-Tu (tufB) and ribonuclease P (rnpA) (Table 2 and Supplementary Table 1).
tRNA modifications can play an important role in the modulation of antibiotic resistance by regulating translational processes (Chopra and Reader, 2015). The genes encoding the tRNA methyltransferases TrmD, Orf2, and RimM were significantly up-regulated by tobramycin (Table 2 and Supplementary Table 1). TrmD is involved in the m1G37 methylation of proline tRNA (Gamper H.B. et al., 2015; Gamper H. et al., 2015), and recent studies in E. coli and S. enterica revealed that the translation of several membrane-associated proteins is controlled by m1G37 methylation at proline codons near the start of their respective open reading frames. TmrD deficient strains exhibit a decrease in membrane protein content resulting in a higher susceptibility to aminoglycosides (Masuda et al., 2019).
Trans-translation is a process adopted by the cell to rescue stalled ribosomes that requires the specialized tmRNA SsrA and the small accessory protein SmpB (Withey and Friedman, 2003; Haebel et al., 2004). Pathogenic bacteria lacking this system display an enhanced sensitivity toward aminoglycosides (de la Cruz and Vioque, 2001; Aboa et al., 2002; Morita et al., 2006). In this study, several genes encoding effectors of stalled ribosome rescue (PA14_72200, tmRNA ssrA and smpB encoding an accessory protein) were up-regulated after tobramycin exposure (Table 2 and Supplementary Table 1).
Furthermore, the gene encoding ObgE, a conserved ribosomal associated GTPase with unknown function (Verstraeten et al., 2015), was up-regulated upon tobramycin treatment (Table 2 and Supplementary Table 1). Verstraeten et al. (2015) showed that over-expression of obgE confers tobramycin and ofloxacin tolerance to Pae and E. coli. They further reported that in E. coli Obg-mediated tolerance requires activation of the type I hokB-sokB TA system. Although a hokB ortholog is not present in Pae, we found multiple genes associated with type II TA systems including HigA (VapI) and ParE-ParD (PA14_01510-PA14_01520) that are up-regulated in the presence of tobramycin (Table 2 and Supplementary Table 1).
Comparison With Previous Transcriptome Studies
When compared with previous Pae transcriptome studies performed in the presence of polymyxins and tobramycin (Cummins et al., 2009; Fernández et al., 2010; Kindrachuk et al., 2011; Murray et al., 2015; Han et al., 2019; Ben Jeddou et al., 2020) this study revealed a larger number of de-regulated genes. Despite some variances, overlaps concerning de-regulated genes exist. The arn operon, the speD2-speE2 (PA14_63110- PA14_63120) genes, the mexAB-oprN, the mexC, the mexXY (PA14_38395 and amrB), the galU, the cprA (PA14_43311) and the genes of unknown function PA2358 (PA14_34170), PA1797 (PA14_41280), PA14_41290 and PA4782 (PA14_63220) were also previously found to be de-regulated in response to polymyxins or in Pae strains harboring mutations that impact polymyxin resistance (Cummins et al., 2009; Fernández et al., 2010; Murray et al., 2015; Han et al., 2019; Ben Jeddou et al., 2020) (Table 1 and Supplementary Table 1). Kindrachuk et al. (2011) reported that bacteriostatic and bactericidal concentrations of tobramycin stimulate the expression of several heat shock genes and genes encoding transcriptional regulators, whereas genes involved in energy metabolism (i.e., nuo, nqr, and suc genes), motility and attachment (i.e., pil and flg genes) were down-regulated. Our RNA-seq and Ribo-seq results closely mirror these previous findings (Figure 4 and Supplementary Table 1).
The transcriptional repression of iron homeostasis- (i.e., has, pvd, pch, fpt, fpv) and sulfonate utilization genes (ssu) (Tralau et al., 2007) and an up-regulation of the denitrification pathway genes (nir, nor, nos) upon exposure to polymyxin B has been reported for PA14 grown in Mueller–Hinton broth (Ben Jeddou et al., 2020). However, this study did not reveal a positive effect on expression of oxidative stress response genes by polymyxin B. On the other hand, transcription of PA14_24360, ahpF, and ahpB was seemingly induced when PA14 was exposed to synthetic antimicrobial peptide dendrimers (Ben Jeddou et al., 2020).
Conclusion
In this study, SCFM was used for culturing Pae, a medium that approximates the environment in the lungs of CF patients (Palmer et al., 2007). To the best of our knowledge, no other gene profiling study has offered a more comprehensive view of Pae’s cellular responses to colistin and tobramycin, and especially under these culturing conditions.
Although the potential of colistin to instigate ROS production in Pae is known, this study revealed for the first time its impact on the expression of distinct oxidative stress response genes. Moreover, the study disclosed a colistin-dependent de-regulation of the AlgU regulon and an up-regulation of the MexT regulon taking on a previously undescribed roles in defense against polymyxin antibiotics (Figure 5).
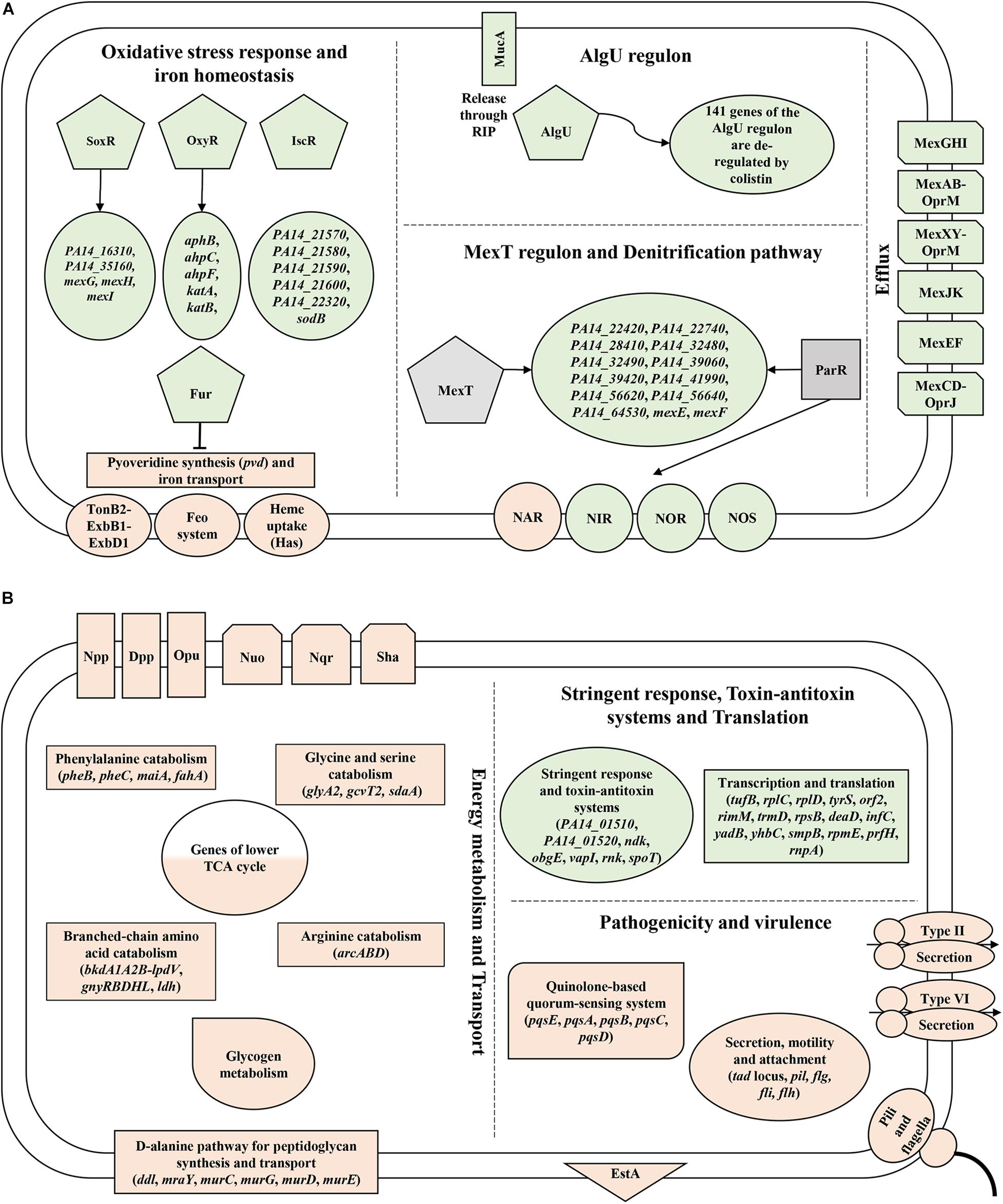
Figure 5. Depiction of novel functions/pathways revealed in this study that are de-regulated upon (A) colistin and (B) tobramycin treatment. Major genes/pathways that are down-regulated and up-regulated based on the RNA-seq and/or Ribo-seq data are highlighted in rose and green, respectively. Positive- and negative regulation of gene expression is denoted by arrows and blocked lines, respectively. RIP - regulated intramembrane proteolysis.
The transcriptome and translatome studies further indicated that the expression of multiple amino acid catabolism genes, lower TCA cycle genes, type II and VI secretion system genes and genes involved in motility and attachment are rewired in response to tobramycin, presumably to reduce drug uptake. Moreover, we discussed that the adverse effects of tobramycin on translation are countered through the expression of functions involved in stalled ribosome rescue, tRNA methylation and type II TA systems. These findings might aid toward the optimization of strategies to increase the efficacy of these last resort drugs against Pae (Figure 5).
Moreover; our results implicate a number of hypothetical genes of unknown function in colistin and tobramycin resistance (Supplementary Table 1). Deciphering their roles could be the basis for future research to elucidate additional mechanisms of action and resistance to colistin and tobramycin.
Data Availability Statement
The datasets presented in this study can be found in online repositories. The names of the repository/repositories and accession number(s) can be found in the article/ Supplementary Material.
Author Contributions
ACS, BL, ES, and UB conceived and designed the experiments. ACS and BL performed the experiments. ACS, BL, FA, MW, and UB analyzed the data. ACS, BL, and UB wrote the manuscript. All the authors contributed to the article and approved the submitted version.
Funding
The work was supported by the Austrian Science Fund (www.fwf.ac.at/en) through project P33617-B (UB and ES). ACS and BL were supported through the FWF funded doctoral program RNA-Biology W-1207.
Conflict of Interest
The authors declare that the research was conducted in the absence of any commercial or financial relationships that could be construed as a potential conflict of interest.
Acknowledgments
We are grateful to Petra Pusic and Marlena Rozner for their help with the RNA-seq and Ribo-seq experiments.
Supplementary Material
The Supplementary Material for this article can be found online at: https://www.frontiersin.org/articles/10.3389/fmicb.2021.626715/full#supplementary-material
Supplementary Figure 1 | Activity of (A) 8 μg/ml colistin and (B) 64 μg/ml tobramycin on growth of Pseudomonas aeruginosa PA14. The arrows represent the points at which antibiotics were added to growing cultures (OD600 of 1.7). Error bars indicate standard deviations obtained from two biological replicates.
Supplementary Figure 2 | Superimposition of the (A) tonB2-exbB1-exbD1, (B) feo, (C) has, and (D) pvd genes with the ribosome profiling data. In pink – open reading frames (ORF) of corresponding genes located on the negative strand of PA14 genomic DNA; in orange – ORFs of corresponding genes located on the positive strand of PA14 genomic DNA; in light green – mapped ribosomal footprints obtained from control samples, in dark green – mapped ribosomal footprints obtained from colistin treated samples.
Supplementary Figure 3 | Increased susceptibility of PA14ΔalgU toward colistin. (A) The microdilution assay was performed in duplicate with strains PA14 and PA14ΔalgU, aerobically grown in SCFM medium to an OD600 of ∼2.0. Then, 0.5 ml of the culture was mixed with 1.5 ml of SCFM medium, containing serial dilutions of colistin (concentration 4–64 μg/ml). The cultures were shaken at 37°C for 20 h and the pictures were taken. The minimal inhibitory concentrations (MICs; marked by red edging) correspond to the lowest concentration of colistin that visibly impeded growth. Control, no colistin added. (B) Graphical representation of the results shown in (A). The outcome of the duplicate assay was identical.
Supplementary Table 1 | RNA-seq and Ribo-seq differential gene expression analysis of Pae treated with colistin or tobramycin versus untreated control.
Supplementary Table 2 | Changes in transcript and ribosomal footprint abundance of genes which contribute to polymyxin and aminoglycoside resistance/susceptibility in Pae after exposure to colistin and tobramycin.
Supplementary Table 3 | Changes in transcript and ribosomal footprint abundance of genes belonging to the AlgU regulon in Pae (Schulz et al., 2015) in the presence of 8 μg/ml colistin.
Footnotes
References
Aboa, T., Ueda, K., Sunohara, T., Ogawa, K., and Aiba, H. (2002). SsrA-mediated protein tagging in the presence of miscoding drugs and its physiological role in Escherichia coli. Genes Cells 7, 629–638. doi: 10.1046/j.1365-2443.2002.00549.x
Anders, S., and Huber, W. (2010). Differential expression analysis for sequence count data. Genome Biol. 11:R106. doi: 10.1186/gb-2010-11-10-r106
Antonic, V., Stojadinovic, A., Zhang, B., Izadjoo, M. J., and Alavi, M. (2013). Pseudomonas aeruginosa induces pigment production and enhances virulence in a white phenotypic variant of Staphylococcus aureus. Infect. Drug Resist. 6, 175–186. doi: 10.2147/IDR.S49039
Barrow, K., and Kwon, D. H. (2009). Alterations in two-component regulatory systems of phoPQ and pmrAB are associated with polymyxin B resistance in clinical isolates of Pseudomonas aeruginosa. Antimicrob. Agents Chemother. 53, 5150–5154. doi: 10.1128/AAC.00893-09
Ben Jeddou, F., Falconnet, L., Luscher, A., Siriwardena, T., Reymond, J. L., Van Delden, C., et al. (2020). Adaptive and mutational responses to peptide dendrimer antimicrobials in Pseudomonas aeruginosa. Antimicrob. Agents Chemother. 64, e02040-19. doi: 10.1128/AAC.02040-19
Blevins, W. R., Tavella, T., Moro, S. G., Blasco-Moreno, B., Closa-Mosquera, A., Diez, J., et al. (2018). Using ribosome profiling to quantify differences in protein expression: a case study in Saccharomyces cerevisiae oxidative stress conditions. bioRxiv [Preprint]. 501478. doi: 10.1101/501478
Breidenstein, E. B. M., de la Fuente-Núñez, C., and Hancock, R. E. W. (2011). Pseudomonas aeruginosa: all roads lead to resistance. Trends Microbiol. 19, 419–426. doi: 10.1016/j.tim.2011.04.005
Brochmann, R. P., Toft, A., Ciofu, O., Briales, A., Kolpen, M., Hempel, C., et al. (2014). Bactericidal effect of colistin on planktonic Pseudomonas aeruginosa is independent of hydroxyl radical formation. Int. J. Antimicrob. Agents 43, 140–147. doi: 10.1016/j.ijantimicag.2013.10.015
Brown, S. M., Howell, M. L., Vasil, M. L., Anderson, A. J., and Hassett, D. J. (1995). Cloning and characterization of the katB gene of Pseudomonas aeruginosa encoding a hydrogen peroxide-inducible catalase: purification of KatB, cellular localization, and demonstration that it is essential for optimal resistance to hydrogen p. J. Bacteriol. 177, 6536–6544. doi: 10.1128/jb.177.22.6536-6544.1995
Cartron, M. L., Maddocks, S., Gillingham, P., Craven, C. J., and Andrews, S. C. (2006). Feo - Transport of ferrous iron into bacteria. BioMetals 19, 143–157. doi: 10.1007/s10534-006-0003-2
Chopra, S., and Reader, J. (2015). tRNAs as antibiotic targets. Int. J. Mol. Sci. 16, 321–349. doi: 10.3390/ijms16010321
Cummins, J., Reen, F. J., Baysse, C., Mooij, M. J., and O’Gara, F. (2009). Subinhibitory concentrations of the cationic antimicrobial peptide colistin induce the pseudomonas quinolone signal in Pseudomonas aeruginosa. Microbiology 155, 2826–2837. doi: 10.1099/mic.0.025643-0
Damron, F. H., and Goldberg, J. B. (2012). Proteolytic regulation of alginate overproduction in Pseudomonas aeruginosa. Mol. Microbiol. 84, 595–607. doi: 10.1111/j.1365-2958.2012.08049.x
de la Cruz, J., and Vioque, A. (2001). Increased sensitivity to protein synthesis inhibitors in cells lacking tmRNA. RNA 7, 1708–1716.
Deris, Z. Z., Akter, J., Sivanesan, S., Roberts, K. D., Thompson, P. E., Nation, R. L., et al. (2014). A secondary mode of action of polymyxins against Gram-negative bacteria involves the inhibition of NADH-quinone oxidoreductase activity. J. Antibiot. 67, 147–151. doi: 10.1038/ja.2013.111
Dumas, Z., Ross-Gillespie, A., and Kümmerli, R. (2013). Switching between apparently redundant iron-uptake mechanisms benefits bacteria in changeable environments. Proc. R. Soc. B Biol. Sci. 280, 20131055. doi: 10.1098/rspb.2013.1055
El-Sayed Ahmed, M. A. E.-G., Zhong, L.-L., Shen, C., Yang, Y., Doi, Y., and Tian, G.-B. (2020). Colistin and its Role in the Era of Antibiotic Resistance: an extended review (2000-2019). Emerg. Microbes Infect. 9, 868-885. doi: 10.1080/22221751.2020.1754133
Fajardo, A., Martínez-Martín, N., Mercadillo, M., Galán, J. C., Ghysels, B., Matthijs, S., et al. (2008). The Neglected Intrinsic Resistome of Bacterial Pathogens. PLoS One 3:e1619. doi: 10.1371/journal.pone.0001619
Fargier, E., Mac Aogáin, M., Mooij, M. J., Woods, D. F., Morrissey, J. P., Dobson, A. D. W., et al. (2012). MexT Functions as a Redox-Responsive Regulator Modulating Disulfide Stress Resistance in Pseudomonas aeruginosa. J. Bacteriol. 194, 3502–3511. doi: 10.1128/JB.06632-11
Farrant, K. V., Spiga, L., Davies, J. C., and Williams, H. D. (2020). Response of Pseudomonas aeruginosa to the innate immune system-derived oxidants hypochlorous acid and hypothiocyanous acid. bioRxiv. [Preprint]. doi: 10.1101/2020.01.09.900639
Fernández, L., Álvarez-Ortega, C., Wiegand, I., Olivares, J., Kocíncová, D., Lam, J. S., et al. (2013). Characterization of the polymyxin B resistome of Pseudomonas aeruginosa. Antimicrob. Agents Chemother. 57, 110–119. doi: 10.1128/AAC.01583-12
Fernández, L., Gooderham, W. J., Bains, M., McPhee, J. B., Wiegand, I., and Hancock, R. E. W. (2010). Adaptive resistance to the “last hope” antibiotics polymyxin B and colistin in Pseudomonas aeruginosa is mediated by the novel two-component regulatory system ParR-ParS. Antimicrob. Agents Chemother. 54, 3372–3382. doi: 10.1128/AAC.00242-10
Fernández, L., Jenssen, H., Bains, M., Wiegand, I., Gooderham, W. J., and Hancock, R. E. W. (2012). The two-component system CprRS senses cationic peptides and triggers adaptive resistance in Pseudomonas aeruginosa independently of ParRS. Antimicrob. Agents Chemother. 56, 6212–6222. doi: 10.1128/AAC.01530-12
Fetar, H., Gilmour, C., Klinoski, R., Daigle, D. M., Dean, C. R., and Poole, K. (2011). mexEF-oprN multidrug efflux operon of Pseudomonas aeruginosa: regulation by the MexT activator in response to nitrosative stress and chloramphenicol. Antimicrob. Agents Chemother. 55, 508–514. doi: 10.1128/AAC.00830-10
Fraud, S., Campigotto, A. J., Chen, Z., and Poole, K. (2008). MexCD-OprJ multidrug efflux system of Pseudomonas aeruginosa: involvement in chlorhexidine resistance and induction by membrane-damaging agents dependent upon the AlgU stress response sigma factor. Antimicrob. Agents Chemother. 52, 4478–4482. doi: 10.1128/AAC.01072-08
Gamper, H. B., Masuda, I., Frenkel-Morgenstern, M., and Hou, Y. M. (2015). Maintenance of protein synthesis reading frame by EF-P and m 1 G37-tRNA. Nat. Commun. 6:7226. doi: 10.1038/ncomms8226
Gamper, H., Masuda, I., Frenkel-Morgenstern, M., and Hou, Y.-M. (2015). The UGG Isoacceptor of tRNAPro Is Naturally Prone to Frameshifts. Int. J. Mol. Sci. 16, 14866–14883. doi: 10.3390/ijms160714866
Garnacho-Montero, J., Garcia-Garmendia, J. L., Barrero-Almodovar, A., Jimenez-Jimenez, F. J., Perez-Paredes, C., and Ortiz-Leyba, C. (2003). Impact of adequate empirical antibiotic therapy on the outcome of patients admitted to the intensive care unit with sepsis. Crit. Care Med. 31, 2742–2751. doi: 10.1097/01.CCM.0000098031.24329.10
Garneau-Tsodikova, S., and Labby, K. J. (2016). Mechanisms of resistance to aminoglycoside antibiotics: overview and perspectives. Medchemcomm 7, 11–27. doi: 10.1039/c5md00344j
Gellatly, S. L., and Hancock, R. E. W. (2013). Pseudomonas aeruginosa: new insights into pathogenesis and host defenses. Pathog. Dis. 67, 159–173. doi: 10.1111/2049-632X.12033
Ginalski, K., Kinch, L., Rychlewski, L., and Grishin, N. V. (2004). BOF: a novel family of bacterial OB-fold proteins. FEBS Lett. 567, 297–301. doi: 10.1016/j.febslet.2004.04.086
Guragain, M., King, M. M., Williamson, K. S., Pérez-Osorio, A. C., Akiyama, T., Khanam, S., et al. (2016). The Pseudomonas aeruginosa PAO1 two-component regulator CarSR regulates calcium homeostasis and calcium induced virulence factor production through its regulatory targets CarO and CarP. J. Bacteriol. 198, 951–963. doi: 10.1128/JB.00963-15
Gutu, A. D., Sgambati, N., Strasbourger, P., Brannon, M. K., Jacobs, M. A., Haugen, E., et al. (2013). Polymyxin resistance of Pseudomonas aeruginosa phoQ mutants is dependent on additional two-component regulatory systems. Antimicrob. Agents Chemother. 57, 2204–2215. doi: 10.1128/AAC.02353-12
Haebel, P. W., Gutmann, S., and Ban, N. (2004). Dial tm for rescue: tmRNA engages ribosomes stalled on defective mRNAs. Curr. Opin. Struct. Biol. 14, 58–65. doi: 10.1016/j.sbi.2004.01.010
Han, M.-L., Zhu, Y., Creek, D. J., Lin, Y.-W., Gutu, A. D., Hertzog, P., et al. (2019). Comparative Metabolomics and Transcriptomics Reveal Multiple Pathways Associated with Polymyxin Killing in Pseudomonas aeruginosa. mSystems 4, e00149-18. doi: 10.1128/msystems.00149-18
Harms, J. M., Bartels, H., Schlünzen, F., and Yonath, A. (2003). Antibiotics acting on the translational machinery. J. Cell Sci. 116, 1391–1393. doi: 10.1242/jcs.00365
Hassett, D. J., Schweizer, H. P., and Ohman, D. E. (1995). Pseudomonas aeruginosa sodA and sodB mutants defective in manganese- and iron-cofactored superoxide dismutase activity demonstrate the importance of the iron-cofactored form in aerobic metabolism. J. Bacteriol. 177, 6330–6337. doi: 10.1128/jb.177.22.6330-6337.1995
Hassett, D. J., Woodruff, W. A., Wozniak, D. J., Vasil, M. L., Cohen, M. S., and Ohman, D. E. (1993). Cloning and characterization of the Pseudomonas aeruginosa sodA and sodB genes encoding manganese- and iron-cofactored superoxide dismutase: demonstration of increased manganese superoxide dismutase activity in alginate-producing bact. J. Bacteriol. 175, 7658–7665. doi: 10.1128/jb.175.23.7658-7665.1993
Hay, T., Fraud, S., Lau, C. H. F., Gilmour, C., and Poole, K. (2013). Antibiotic Inducibility of the mexXY Multidrug Efflux Operon of Pseudomonas aeruginosa: involvement of the MexZ Anti-Repressor ArmZ. PLoS One 8:e56858. doi: 10.1371/journal.pone.0056858
Heindorf, M., Kadari, M., Heider, C., Skiebe, E., and Wilharm, G. (2014). Impact of Acinetobacter baumannii superoxide dismutase on motility, virulence, oxidative stress resistance and susceptibility to antibiotics. PLoS One 9:e101033. doi: 10.1371/journal.pone.0101033
Hill, I. T., Tallo, T., Dorman, M. J., and Dove, S. L. (2019). Loss of RNA chaperone Hfq unveils a toxic pathway in Pseudomonas aeruginosa. J. Bacteriol. 201, e00232–19. doi: 10.1128/JB.00232-19
Hinz, A., Lee, S., Jacoby, K., and Manoil, C. (2011). Membrane proteases and aminoglycoside antibiotic resistance. J. Bacteriol. 193, 4790–4797. doi: 10.1128/JB.05133-11
Hoffmann, S., Otto, C., Kurtz, S., Sharma, C. M., Khaitovich, P., Vogel, J., et al. (2009). Fast mapping of short sequences with mismatches, insertions and deletions using index structures. PLoS Comput. Biol. 5:e1000502. doi: 10.1371/journal.pcbi.1000502
Huang, H., Shao, X., Xie, Y., Wang, T., Zhang, Y., Wang, X., et al. (2019). An integrated genomic regulatory network of virulence-related transcriptional factors in Pseudomonas aeruginosa. Nat. Commun. 10:2931. doi: 10.1038/s41467-019-10778-w
Ingolia, N. T., Ghaemmaghami, S., Newman, J. R. S., and Weissman, J. S. (2009). Genome-wide analysis in vivo of translation with nucleotide resolution using ribosome profiling. Science 324, 218–223. doi: 10.1126/science.1168978
Jaillard, M., van Belkum, A., Cady, K. C., Creely, D., Shortridge, D., Blanc, B., et al. (2017). Correlation between phenotypic antibiotic susceptibility and the resistome in Pseudomonas aeruginosa. Int. J. Antimicrob. Agents 50, 210–218. doi: 10.1016/j.ijantimicag.2017.02.026
Jain, S. (2018). Emergence of Colistin Resistance among Gram Negative Bacteria in Urinary Tract Infections from Super Specialty Hospital of North India. Int. J. Infect. Dis. 73:133. doi: 10.1016/j.ijid.2018.04.3716
Jeannot, K., Bolard, A., and Plésiat, P. (2017). Resistance to polymyxins in Gram-negative organisms. Int. J. Antimicrob. Agents 49, 526–535. doi: 10.1016/j.ijantimicag.2016.11.029
Johnson, L., Mulcahy, H., Kanevets, U., Shi, Y., and Lewenza, S. (2011). Surface-localized spermidine protects the Pseudomonas aeruginosa: outer membrane from antibiotic treatment and oxidative stress. J. Bacteriol. 194, 813–826. doi: 10.1128/JB.05230-11
Kerr, K. G., and Snelling, A. M. (2009). Pseudomonas aeruginosa: a formidable and ever-present adversary. J. Hosp. Infect. 73, 338–344. doi: 10.1016/j.jhin.2009.04.020
Kimura, S., and Suzuki, T. (2015). Iron-sulfur proteins responsible for RNA modifications. Biochim. Biophys. Acta Mol. Cell Res. 1853, 1272–1283. doi: 10.1016/j.bbamcr.2014.12.010
Kindrachuk, K. N., Fernández, L., Bains, M., and Hancock, R. E. W. (2011). Involvement of an ATP-dependent protease, PA0779/AsrA, in inducing heat shock in response to tobramycin in Pseudomonas aeruginosa. Antimicrob. Agents Chemother. 55, 1874–1882. doi: 10.1128/AAC.00935-10
Kohanski, M., Dwyer, D., Hayete, B., Lawrence, C., and Collins, J. (2007). A common mechanism of cellular death induced by bactericidal antibiotics. Cell 130, 797–810.
Köhler, T., Epp, S. F., Curty, L. K., and Pechère, J. C. (1999). Characterization of MexT, the regulator of the MexE-MexF-OprN multidrug efflux system of Pseudomonas aeruginosa. J. Bacteriol. 181, 6300–6305. doi: 10.1128/jb.181.20.6300-6305.1999
Köhler, T., Michéa-Hamzehpour, M., Henze, U., Gotoh, N., Curty, L. K., and Pechère, J. C. (1997a). Characterization of MexE-MexF-OprN, a positively regulated multidrug efflux system of Pseudomonas aeruginosa. Mol. Microbiol. 23, 345–354. doi: 10.1046/j.1365-2958.1997.2281594.x
Köhler, T., Michea-Hamzehpour, M., Plesiat, P., Kahr, A. L., and Pechere, J. C. (1997b). Differential selection of multidrug efflux systems by quinolones in Pseudomonas aeruginosa. Antimicrob. Agents Chemother. 41, 2540–2543. doi: 10.1128/aac.41.11.2540
Kong, L., Liu, Z., Hu, X., and Liu, S. (2011). Interaction of polymyxin B with ds-DNA, and determination of DNA or polymyxin B via resonance Rayleigh scattering and resonance non-linear scattering spectra. Microchim. Acta 173, 207–213. doi: 10.1007/s00604-011-0547-x
Kosono, S., Haga, K., Tomizawa, R., Kajiyama, Y., Hatano, K., Takeda, S., et al. (2005). Characterization of a multigene-encoded sodium/hydrogen antiporter (Sha) from Pseudomonas aeruginosa: its involvement in pathogenesis. J. Bacteriol. 187, 5242–5248. doi: 10.1128/JB.187.15.5242-5248.2005
Krause, K. M., Serio, A. W., Kane, T. R., and Connolly, L. E. (2016). Aminoglycosides: an overview. Cold Spring Harb. Perspect. Med. 6:a027029. doi: 10.1101/cshperspect.a027029
Kreamer, N. N., Costa, F., and Newman, D. K. (2015). The ferrous iron-responsive BqsRS two-component system activates genes that promote cationic stress tolerance. MBio 6, e02549. doi: 10.1128/mBio.02549-14
Kreamer, N. N. K., Wilks, J. C., Marlow, J. J., Coleman, M. L., and Newman, D. K. (2012). BqsR/BqsS Constitute a Two-Component System That Senses Extracellular Fe(II) in Pseudomonas aeruginosa. J Bacteriol. 194, 1195–1204. doi: 10.1128/JB.05634-11
Lamont, I. L., and Martin, L. W. (2003). Identification and characterization of novel pyoverdine synthesis genes in Pseudomonas aeruginosa. Microbiology 149, 833–842. doi: 10.1099/mic.0.26085-0
Lima, M. R., Ferreira, G. F., Nunes Neto, W. R., Monteiro, J. D. M., Santos, ÁR. C., Tavares, P. B., et al. (2019). Evaluation of the interaction between polymyxin B and Pseudomonas aeruginosa biofilm and planktonic cells: reactive oxygen species induction and zeta potential. BMC Microbiol 19:115. doi: 10.1186/s12866-019-1485-8
Luckett, J. C. A., Darch, O., Watters, C., AbuOun, M., Wright, V., Paredes-Osses, E., et al. (2012). A novel virulence strategy for Pseudomonas aeruginosa mediated by an autotransporter with arginine-specific aminopeptidase activity. PLoS Pathog. 8:e1002854. doi: 10.1371/journal.ppat.1002854
Ma, J. F., Ochsner, U. A., Klotz, M. G., Nanayakkara, V. K., Howell, M. L., Johnson, Z., et al. (1999). Bacterioferritin A modulates catalase A (KatA) activity and resistance to hydrogen peroxide in Pseudomonas aeruginosa. J. Bacteriol. 181, 3730–3742. doi: 10.1128/jb.181.12.3730-3742.1999
Martin, M. (2011). Cutadapt removes adapter sequences from high-throughput sequencing reads. EMBnet J. 17:10. doi: 10.14806/ej.17.1.200
Masuda, I., Matsubara, R., Christian, T., Rojas, E. R., Yadavalli, S. S., Zhang, L., et al. (2019). tRNA Methylation Is a Global Determinant of Bacterial Multi-drug Resistance. Cell Syst. 8, 302–314. doi: 10.1016/j.cels.2019.03.008
Mathiesen, C., and Hägerhäll, C. (2003). The “antiporter module” of respiratory chain Complex I includes the MrpC/NuoK subunit - A revision of the modular evolution scheme. FEBS Lett. 549, 7–13. doi: 10.1016/S0014-5793(03)00767-1
McPhee, J. B., Lewenza, S., and Hancock, R. E. W. (2003). Cationic antimicrobial peptides activate a two-component regulatory system, PmrA-PmrB, that regulates resistance to polymyxin B and cationic antimicrobial peptides in Pseudomonas aeruginosa. Mol. Microbiol. 50, 205–217. doi: 10.1046/j.1365-2958.2003.03673.x
Meyer, J. M. (1978). The Fluorescent Pigment of Pseudomonas fluorescens: biosynthesis, Purification and Physicochemical Properties. J. Gen. Microbiol. 107, 319–328.
Meylan, S., Porter, C. B. M., Yang, J. H., Belenky, P., Gutierrez, A., Lobritz, M. A., et al. (2017). Carbon Sources Tune Antibiotic Susceptibility in Pseudomonas aeruginosa via Tricarboxylic Acid Cycle Control. Cell Chem. Biol. 24, 195–206. doi: 10.1016/j.chembiol.2016.12.015
Mlynarcik, P., and Kolar, M. (2019). Molecular mechanisms of polymyxin resistance and detection of mcr genes. Biomed. Pap. 163, 28–38. doi: 10.5507/bp.2018.070
Morita, Y., Sobel, M. L., and Poole, K. (2006). Antibiotic inducibility of the MexXY multidrug efflux system of Pseudomonas aeruginosa: involvement of the antibiotic-inducible PA5471 gene product. J. Bacteriol. 188, 1847–1855. doi: 10.1128/JB.188.5.1847-1855.2006
Mulcahy, H., O’Callaghan, J., O’Grady, E. P., Adams, C., and O’Gara, F. (2006). The posttranscriptional regulator RsmA plays a role in the interaction between Pseudomonas aeruginosa and human airway epithelial cells by positively regulating the type III secretion system. Infect. Immun. 74, 3012–3015. doi: 10.1128/IAI.74.5.3012-3015.2006
Muller, C., Plésiat, P., and Jeannot, K. (2011). A two-component regulatory system interconnects resistance to polymyxins, aminoglycosides, fluoroquinolones, and β-lactams in Pseudomonas aeruginosa. Antimicrob. Agents Chemother. 55, 1211–1221. doi: 10.1128/AAC.01252-10
Murray, J. L., Kwon, T., Marcotte, E. M., and Whiteley, M. (2015). Intrinsic antimicrobial resistance determinants in the superbug pseudomonas aeruginosa. MBio 6, e01603-15. doi: 10.1128/mBio.01603-15
Nasie, I., Steiner-Mordoch, S., and Schuldiner, S. (2012). New substrates on the block: clinically relevant resistances for EmrE and homologues. J. Bacteriol. 194, 6766–6770. doi: 10.1128/JB.01318-12
Obritsch, M. D., Fish, D. N., MacLaren, R., and Jung, R. (2004). National surveillance of antimicrobial resistance in Pseudomonas aeruginosa isolates obtained from intensive care unit patients from 1993 to 2002. Antimicrob. Agents Chemother. 48, 4606–4610. doi: 10.1128/AAC.48.12.4606-4610.2004
Ochsner, U. A., Johnson, Z., and Vasil, M. L. (2000a). Genetics and regulation of two distinct haem-uptake systems, phu and has, in Pseudomonas aeruginosa. Microbiology 146, 185–198. doi: 10.1099/00221287-146-1-185
Ochsner, U. A., Vasil, M. L., Alsabbagh, E., Parvatiyar, K., and Hassett, D. J. (2000b). Role of the Pseudomonas aeruginosa oxyR-recG operon in oxidative stress defense and DNA repair: oxyR-dependent regulation of katB-ankB, ahpB, and ahpC-ahpF. J. Bacteriol. 182, 4533–4544. doi: 10.1128/JB.182.16.4533-4544.2000
Ochsner, U. A., and Vasil, M. L. (1996). Gene repression by the ferric uptake regulator in Pseudomonas aeruginosa: cycle selection of iron-regulated genes. Proc. Natl. Acad. Sci. U. S. A. 93, 4409–4414. doi: 10.1073/pnas.93.9.4409
O’Driscoll, N. H., Cushnie, T. P. T., Matthews, K. H., and Lamb, A. J. (2018). Colistin causes profound morphological alteration but minimal cytoplasmic membrane perforation in populations of Escherichia coli and Pseudomonas aeruginosa. Arch. Microbiol. 200, 793–802. doi: 10.1007/s00203-018-1485-3
Olivares, J., Alvarez-Ortega, C., Linares, J. F., Rojo, F., Köhler, T., and Martínez, J. L. (2012). Overproduction of the multidrug efflux pump MexEF-OprN does not impair Pseudomonas aeruginosa fitness in competition tests, but produces specific changes in bacterial regulatory networks. Environ. Microbiol. 14, 1968–1981. doi: 10.1111/j.1462-2920.2012.02727.x
Palma, M., Zurita, J., Ferreras, J. A., Worgall, S., Larone, D. H., Shi, L., et al. (2005). Pseudomonas aeruginosa SoxR does not conform to the archetypal paradigm for SoxR-dependent regulation of the bacterial oxidative stress adaptive response. Infect. Immun. 73, 2958–2966. doi: 10.1128/IAI.73.5.2958-2966.2005
Palmer, K. L., Aye, L. M., and Whiteley, M. (2007). Nutritional cues control Pseudomonas aeruginosa multicellular behavior in cystic fibrosis sputum. J. Bacteriol. 189, 8079–8087. doi: 10.1128/JB.01138-07
Pamp, S. J., Gjermansen, M., Johansen, H. K., and Tolker-Nielsen, T. (2008). Tolerance to the antimicrobial peptide colistin in Pseudomonas aeruginosa biofilms is linked to metabolically active cells, and depends on the pmr and mexAB-oprM genes. Mol. Microbiol. 68, 223–240. doi: 10.1111/j.1365-2958.2008.06152.x
Pilonieta, M. C., Erickson, K. D., Ernst, R. K., and Detweiler, C. S. (2009). A protein important for antimicrobial peptide resistance, YdeI/OmdA, is in the periplasm and interacts with OmpD/NmpC. J. Bacteriol. 191, 7243–7252. doi: 10.1128/JB.00688-09
Pletzer, D., Braun, Y., Dubiley, S., Lafon, C., Köhler, T., Page, M. G. P., et al. (2015). The Pseudomonas aeruginosa PA14 ABC transporter NppA1A2BCD is required for uptake of peptidyl nucleoside antibiotics. J. Bacteriol. 197, 2217–2228. doi: 10.1128/JB.00234-15
Poirel, L., Jayol, A., and Nordmanna, P. (2017). Polymyxins: antibacterial activity, susceptibility testing, and resistance mechanisms encoded by plasmids or chromosomes. Clin. Microbiol. Rev. 30, 557–596. doi: 10.1128/CMR.00064-16
Poole, K. (2005). Aminoglycoside resistance in Pseudomonas aeruginosa. Antimicrob. Agents Chemother. 49, 479–487. doi: 10.1128/AAC.49.2.479-487.2005
Poole, K., Lau, C. H. F., Gilmour, C., Hao, Y., and Lam, J. S. (2015). Polymyxin susceptibility in Pseudomonas aeruginosa linked to the MexXY-OprM multidrug efflux system. Antimicrob. Agents Chemother. 59, 7276–7289. doi: 10.1128/AAC.01785-15
Pournaras, S., Poulou, A., Dafopoulou, K., Chabane, Y. N., Kristo, I., Makris, D., et al. (2014). Growth retardation, reduced invasiveness, and impaired colistin-mediated cell death associated with colistin resistance development in Acinetobacter baumannii. Antimicrob. Agents Chemother. 58, 828–832. doi: 10.1128/AAC.01439-13
Quinlan, A. R., and Hall, I. M. (2010). BEDTools: a flexible suite of utilities for comparing genomic features. Bioinformatics 26, 841–842.
Rahme, L. G., Stevens, E. J., Wolfort, S. F., Shao, J., Tompkins, R. G., and Ausubel, F. M. (1995). Common virulence factors for bacterial pathogenicity in plants and animals. Science 268, 1899–1902. doi: 10.1126/science.7604262
Ramsey, B. W., Pepe, M. S., Quan, J. M., Otto, K. L., Montgomery, A. B., Williams-Warren, J., et al. (1999). Intermittent administration of inhaled tobramycin in patients with cystic fibrosis. N. Engl. J. Med. 340, 23–30. doi: 10.1056/NEJM199901073400104
Ratledge, C., and Dover, L. G. (2000). Iron Metabolism in Pathogenic Bacteria. Annu. Rev. Microbiol. 54, 881–941. doi: 10.1146/annurev.micro.54.1.881
Roche, B., Aussel, L., Ezraty, B., Mandin, P., Py, B., and Barras, F. (2013). Reprint of: iron/sulfur proteins biogenesis in prokaryotes: formation, regulation and diversity. Biochim. Biophys. Acta Bioenerg. 1827, 923–937. doi: 10.1016/j.bbabio.2013.05.001
Romsang, A., Duang-Nkern, J., Leesukon, P., Saninjuk, K., Vattanaviboon, P., and Mongkolsuk, S. (2014). The iron-sulphur cluster biosynthesis regulator IscR contributes to iron homeostasis and resistance to oxidants in Pseudomonas aeruginosa. PLoS One 9:e86763. doi: 10.1371/journal.pone.0086763
Salunkhe, P., Salunkhe, P., To, T., To, T., Buer, J., Buer, J., et al. (2005). Genome-Wide Transcriptional Profiling of the Steady-State Response of Pseudomonas aeruginosa to Hydrogen Peroxide. Society 187, 2565–2572. doi: 10.1128/JB.187.8.2565
Sampson, T. R., Liu, X., Schroeder, M. R., Kraft, C. S., Burd, E. M., and Weiss, D. S. (2012). Rapid killing of Acinetobacter baumannii by polymyxins is mediated by a hydroxyl radical death pathway. Antimicrob Agents Chemother 56, 5642–5649. doi: 10.1128/AAC.00756-12
Schreiber, K., Krieger, R., Benkert, B., Eschbach, M., Arai, H., Schobert, M., et al. (2007). The anaerobic regulatory network required for Pseudomonas aeruginosa nitrate respiration. J. Bacteriol. 189, 4310–4314. doi: 10.1128/JB.00240-07
Schulz, S., Eckweiler, D., Bielecka, A., Nicolai, T., Franke, R., Dötsch, A., et al. (2015). Elucidation of Sigma Factor-Associated Networks in Pseudomonas aeruginosa Reveals a Modular Architecture with Limited and Function-Specific Crosstalk. PLoS Pathog. 11:e1004744. doi: 10.1371/journal.ppat.1004744
Schurek, K. N., Marr, A. K., Taylor, P. K., Wiegand, I., Semenec, L., Khaira, B. K., et al. (2008). Novel genetic determinants of low-level aminoglycoside resistance in Pseudomonas aeruginosa. Antimicrob. Agents Chemother. 52, 4213–4219. doi: 10.1128/AAC.00507-08
Sherry, N., and Howden, B. (2018). Emerging Gram negative resistance to last-line antimicrobial agents fosfomycin, colistin and ceftazidime-avibactam–epidemiology, laboratory detection and treatment implications. Expert Rev. Anti. Infect. Ther. 16, 289–306. doi: 10.1080/14787210.2018.1453807
Shiver, A. L., Osadnik, H., Kritikos, G., Li, B., Krogan, N., Typas, A., et al. (2016). A Chemical-Genomic Screen of Neglected Antibiotics Reveals Illicit Transport of Kasugamycin and Blasticidin S. PLoS Genet. 12:e1006124. doi: 10.1371/journal.pgen.1006124
Tata, M., Wolfinger, M. T., Amman, F., Roschanski, N., Dötsch, A., Sonnleitner, E., et al. (2016). RNASeq Based Transcriptional Profiling of Pseudomonas aeruginosa PA14 after Short- and Long-Term Anoxic Cultivation in Synthetic Cystic Fibrosis Sputum Medium. PLoS One 11:e0147811. doi: 10.1371/journal.pone.0147811
Thompson, J., O’Connor, M., Mills, J. A., and Dahlberg, A. E. (2002). The protein synthesis inhibitors, oxazolidinones and chloramphenicol, cause extensive translational inaccuracy in vivo. J. Mol. Biol. 322, 273–279. doi: 10.1016/S0022-2836(02)00784-2
Tian, Z.-X., Fargier, E., Mac Aogá in, M., Adams, C., and Wang, Y.-P. (2009a). Transcriptome profiling defines a novel regulon modulated by the LysR-type transcriptional regulator MexT in Pseudomonas aeruginosa. Nucleic Acids Res. 37, 7546–7559. doi: 10.1093/nar/gkp828
Tian, Z. X., Mac Aogáin, M., O’Connor, H. F., Fargier, E., Mooij, M. J., Adams, C., et al. (2009b). MexT modulates virulence determinants in Pseudomonas aeruginosa independent of the MexEF-OprN efflux pump. Microb. Pathog. 47, 237–241. doi: 10.1016/j.micpath.2009.08.003
Tralau, T., Vuilleumier, S., Thibault, C., Campbell, B. J., Hart, C. A., and Kertesz, M. A. (2007). Transcriptomic analysis of the sulfate starvation response of Pseudomonas aeruginosa. J. Bacteriol. 189, 6743–6750. doi: 10.1128/JB.00889-07
Tzeng, Y. L., Ambrose, K. D., Zughaier, S., Zhou, X., Miller, Y. K., Shafer, W. M., et al. (2005). Cationic antimicrobial peptide resistance in Neisseria meningitidis. J. Bacteriol. 187, 5387–5396. doi: 10.1128/JB.187.15.5387-5396.2005
Valderrama-Carmona, P., Cuartas, J. H., Carolina Castaño, D., and Corredor, M. (2019). The Role of Pseudomonas aeruginosa RNA Methyltransferases in Antibiotic Resistance,” in Pseudomonas Aeruginosa - An Armory Within. London: IntechOpen.
Vasil, M. L., and Ochsner, U. A. (1999). The response of Pseudomonas aeruginosa to iron: genetics, biochemistry and virulence. Mol. Microbiol. 34, 399–413. doi: 10.1046/j.1365-2958.1999.01586.x
Velkov, T., Roberts, K. D., Nation, R. L., Thompson, P. E., and Li, J. (2013). Pharmacology of polymyxins: new insights into an ’old class of antibiotics. Future Microbiol. 8, 711–724. doi: 10.2217/fmb.13.39
Verstraeten, N., Knapen, W. J., Kint, C. I., Liebens, V., Van den Bergh, B., Dewachter, L., et al. (2015). Obg and Membrane Depolarization Are Part of a Microbial Bet-Hedging Strategy that Leads to Antibiotic Tolerance. Mol. Cell 59, 9–21. doi: 10.1016/j.molcel.2015.05.011
Vioque, A., and Cruz, J. (2003). Trans -translation and protein synthesis inhibitors. FEMS Microbiol. Lett. 218, 9–14. doi: 10.1111/j.1574-6968.2003.tb11491.x
Wandersman, C., and Delepelaire, P. (2004). Bacterial Iron Sources: from Siderophores to Hemophores. Annu. Rev. Microbiol. 58, 611–647. doi: 10.1146/annurev.micro.58.030603.123811
Wang, D., Seeve, C., Pierson, L. S., and Pierson, E. A. (2013). Transcriptome profiling reveals links between ParS/ParR, MexEF-OprN, and quorum sensing in the regulation of adaptation and virulence in Pseudomonas aeruginosa. BMC Genomics 14:618. doi: 10.1186/1471-2164-14-618
Wei, Q., Le Minh, P. N., Dö Tsch, A., Hildebrand, F., Panmanee, W., Elfarash, A., et al. (2012). Global regulation of gene expression by OxyR in an important human opportunistic pathogen. Nucleic Acids Res. 40, 4320–4333. doi: 10.1093/nar/gks017
Wi, Y. M., Choi, J. Y., Lee, J. Y., Kang, C. I., Chung, D. R., Peck, K. R., et al. (2017). Emergence of colistin resistance in Pseudomonas aeruginosa ST235 clone in South Korea. Int. J. Antimicrob. Agents 49, 767–769. doi: 10.1016/j.ijantimicag.2017.01.023
Williams, B. J., Dehnbostel, J., and Blackwell, T. S. (2010). Pseudomonas aeruginosa: host defence in lung diseases. Respirology 15, 1037–1056. doi: 10.1111/j.1440-1843.2010.01819.x
Winsor, G. L., Griffiths, E. J., Lo, R., Dhillon, B. K., Shay, J. A., and Brinkman, F. S. L. (2016). Enhanced annotations and features for comparing thousands of Pseudomonas genomes in the Pseudomonas genome database. Nucleic Acids Res. 44, D646-53. doi: 10.1093/nar/gkv1227
Withey, J. H., and Friedman, D. I. (2003). A Salvage Pathway for Protein Synthesis: tmRNA and Trans -Translation. Annu. Rev. Microbiol. 57, 101–123. doi: 10.1146/annurev.micro.57.030502.090945
Wolfinger, M. T., Fallmann, J., Eggenhofer, F., and Amman, F. (2015). ViennaNGS: a toolbox for building efficient next-generation sequencing analysis pipelines. F1000Res. 4:50. doi: 10.12688/f1000research.6157.2
Wood, L. F., Leech, A. J., and Ohman, D. E. (2006). Cell wall-inhibitory antibiotics activate the alginate biosynthesis operon in Pseudomonas aeruginosa: roles of σ22 (AlgT) and the AlgW and Prc proteases. Mol. Microbiol. 62, 412–426. doi: 10.1111/j.1365-2958.2006.05390.x
Wright, G. D. (2007). The antibiotic resistome: the nexus of chemical and genetic diversity. Nat. Rev. Microbiol. 5, 175–186. doi: 10.1038/nrmicro1614
Wu, X., Held, K., Zheng, C., Staudinger, B. J., Chavez, J. D., Weisbrod, C. R., et al. (2015). Dynamic proteome response of Pseudomonas aeruginosa to tobramycin antibiotic treatment. Mol. Cell. Proteomics 14, 2126–2137. doi: 10.1074/mcp.M115.050161
Yeom, J., Imlay, J. A., and Park, W. (2010). Iron homeostasis affects antibiotic-mediated cell death in Pseudomonas species. J. Biol. Chem. 285, 22689–22695. doi: 10.1074/jbc.M110.127456
Young, M. L., Bains, M., Bell, A., and Hancock, R. E. W. (1992). Role of Pseudomonas aeruginosa outer membrane protein OprH in polymyxin and gentamicin resistance: isolation of an OprH-deficient mutant by gene replacement techniques. Antimicrob. Agents Chemother. 36, 2566–2568. doi: 10.1128/AAC.36.11.2566
Yu, Z., Zhu, Y., Fu, J., Qiu, J., and Yin, J. (2019). Enhanced NADH metabolism involves colistin-induced killing of Bacillus subtilis and Paenibacillus polymyxa. Molecules 24:387. doi: 10.3390/molecules24030387
Yu, Z., Zhu, Y., Qin, W., Yin, J., and Qiu, J. (2017). Oxidative stress induced by polymyxin E is involved in rapid killing of Paenibacillus polymyxa. Biomed Res. Int. 2017, 1–12.
Zhang, Y. F., Han, K., Chandler, C. E., Tjaden, B., Ernst, R. K., and Lory, S. (2017). Probing the sRNA regulatory landscape of P. aeruginosa: post-transcriptional control of determinants of pathogenicity and antibiotic susceptibility. Mol. Microbiol. 106, 919–937. doi: 10.1111/mmi.13857
Keywords: Pseudomonas aeruginosa, colistin, tobramycin, RNA-Seq, ribosome profiling, Ribo-seq
Citation: Cianciulli Sesso A, Lilić B, Amman F, Wolfinger MT, Sonnleitner E and Bläsi U (2021) Gene Expression Profiling of Pseudomonas aeruginosa Upon Exposure to Colistin and Tobramycin. Front. Microbiol. 12:626715. doi: 10.3389/fmicb.2021.626715
Received: 06 November 2020; Accepted: 31 March 2021;
Published: 30 April 2021.
Edited by:
Giuseppantonio Maisetta, University of Pisa, ItalyReviewed by:
Dinesh Sriramulu, Independent Researcher, Chennai, IndiaKatherina Sewald, Fraunhofer Institute for Toxicology and Experimental Medicine (FHG), Germany
Copyright © 2021 Cianciulli Sesso, Lilić, Amman, Wolfinger, Sonnleitner and Bläsi. This is an open-access article distributed under the terms of the Creative Commons Attribution License (CC BY). The use, distribution or reproduction in other forums is permitted, provided the original author(s) and the copyright owner(s) are credited and that the original publication in this journal is cited, in accordance with accepted academic practice. No use, distribution or reproduction is permitted which does not comply with these terms.
*Correspondence: Udo Bläsi, udo.blaesi@univie.ac.at
†These authors have contributed equally to this work