- Laboratory of Food Microbiology and Fermentation, Department of Food Science, University of Copenhagen, Copenhagen, Denmark
Fungal spoilage of dairy products is a major concern due to food waste and economical losses, some fungal metabolites may furthermore have adverse effects on human health. The use of lactic acid bacteria (LAB) is emerging as a potential clean label alternative to chemical preservatives. Here, our aim was to characterize the growth potential at three storage temperatures (5, 16, and 25°C) of a panel of molds (four Mucor and nine Penicillium strains) isolated from dairy products, then investigate the susceptibility of the molds toward 12 LAB cultures. Fungal cell growth and morphology in malt extract broth was monitored using oCelloScope at 25°C for 24 h. Mucor plumbeus 01180036 was the fastest growing and Penicillium roqueforti ISI4 (P. roqueforti ISI4) the slowest of the tested molds. On yogurt-agar plates, all molds grew at 5, 16, and 25°C in a temperature-dependent manner with Mucor strains growing faster than Penicillium strains regardless of temperature. The sensitivity toward 12 LAB cultures was tested using high-throughput overlay method and here all the molds except P. roqueforti ISI4 were strongly inhibited. The antifungal action of these LAB was confirmed when spotting mold spores on agar plates containing live cells of the LAB strains. However, if cells were removed from the fermentates, the inhibitory effects decreased markedly. The antifungal effects of volatiles tested in a plate-on-plate system without direct contact between mold and LAB culture media were modest. Some LAB binary combinations improved the antifungal activity against the growth of several molds beyond that of single cultures in yogurt serum. The role of competitive exclusion due to manganese depletion was examined as a possible antifungal mechanism for six Penicillium and two Mucor strains. It was shown that this mechanism was a major inhibition factor for the molds tested apart from the non-inhibited P. roqueforti ISI4 since addition of manganese with increasing concentrations of up to 0.1 mM resulted in partly or fully restored mold growth in yogurt. These findings help to understand the parameters influencing the mold spoilage of dairy products and the interactions between the contaminating strains, substrate, and bioprotective LAB cultures.
Introduction
Mold spoilage is a major concern in dairy industry, resulting in significant food waste and substantial economic losses (Schnürer and Magnusson, 2005). Dairy products provide a favorable niche for mold growth, and visible alterations can be found due to the outgrowth on the surface of products. Some of the most common molds, such as Penicillium and Mucor genera, are involved in dairy product spoilage (Garnier et al., 2017). Penicillium spp. are mainly isolated from cheese but they are also found in other product types including yogurt, butter, and milk. Mucor spp. are common spoilage agents of yogurt and cheese (Pitt and Hocking, 2009). Apart from the negative impacts on food quality, such as visible growth and off-flavors, some fungal genera possess the ability to produce mycotoxins, which constitute a threat to human health. For instance, Mucor circinelloides (M. circinelloides) is recognized as an opportunistic pathogen, which can produce the mycotoxin 3-nitropropionic acid (Hollmann et al., 2008). It has been suspected of causing nausea, vomiting and diarrhea, and even a rhinocerebral mucormycosis in an immunosuppressed person, following ingestion of contaminated yogurt (Lazar et al., 2014). Several Penicillium strains are also known to be capable of producing mycotoxins, including wild-type Penicillium roqueforti (P. roqueforti) strains (Pitt and Hocking, 2009). Prevention of mold spoilage is therefore a vital part of the quality assurance in dairy industry.
To avoid fungal spoilage in food industry, much effort is used to prevent contamination and inhibit fungal growth during product manufacturing. Chemical preservatives have been used in food industry to protect food from undesirable growth and thus extend product shelf-life (Garnier et al., 2017), but there is a growing public concern about extensive use of chemical preservatives. Furthermore, an increasing number of molds are becoming resistant not only to antibiotics, but also to food preservatives, such as sorbic acid and benzoic acids (Schnürer and Magnusson, 2005). In recent years, there has been a rising demand from consumers for minimally processed or preservative-free food, which has resulted in a growing interest in natural fungal control alternatives, such as antifungal bioprotective cultures (Luz et al., 2018).
Selected lactic acid bacteria (LAB) have commonly been reported as potential biopreservation tools to inhibit fungal growth in different foodstuffs, extend shelf life, and sometimes even improve the nutritional value and sensory characteristics (Pelyuntha et al., 2019). For example, Lactobacillus rhamnosus (L. rhamnosus) was shown to have growth inhibitory effect on Penicillium aurantioviolaceum (P. aurantioviolaceum) and Mucor plumbeus (M. plumbeus) (Bazukyan et al., 2018) and Lactobacillus paracasei (L. paracasei) DGCC 2132 displayed antifungal properties against Penicillium solitum (P. solitum) DCS 302 and Penicillium sp. nov. DCS 1541 in yogurt (Aunsbjerg et al., 2015a). The mechanism underlying the activity of LAB strains against spoilage molds has been suggested to be multifactorial and include the production of a variety of metabolites including volatile compounds and non-volatile compounds (Campana et al., 2019). Leyva Salas et al. (2019) highlighted the diversity of antifungal molecules produced by bioprotective cultures in different dairy products but the concentrations of the individual compounds found in products have not been able to fully explain the inhibitive effect. Recently, Siedler et al. (2020) showed that competition for manganese is a major limiting factor for the growth of several spoilage organisms in yogurt when grown with a bioprotective culture consisting of L. paracasei and L. rhamnosus as the antifungal effect could be counteracted with the addition of manganese in increasing concentrations of up to 6 mg/L.
The aim of the present study was to: (a) characterize the growth potential at three temperatures of a panel of molds isolated from freshly fermented dairy products such as yogurt or skyr; (b) investigate the susceptibility of the molds toward different preparations of LAB by assessment of the direct interactive effect of molds with the LAB fermentates with or without living cells, as well as no direct contact assessing the role of volatiles; (c) evaluate the inhibitory effects of 12 LAB cultures in selected combinations on the growth of these molds and (d) investigate the role of competitive exclusion due to manganese depletion in inhibition.
Materials and Methods
Strains and Chemicals
The 12 LAB strains used in this study are listed in Table 1. The stock cultures were kept at −80°C in De Man, Rogosa and Sharpe (MRS) broth containing 20% glycerol. Thirteen molds isolated from freshly fermented dairy products were used as target organisms (Table 1). Malt extract agar (MEA, 30 g/L malt extract, 5 g/L peptone, 15 g/L agar, pH 5.6 ± 0.2) and malt extract broth (MEB, 17 g/L malt extract, 3 g/L peptone, pH 5.6 ± 0.2) were used to support fungal growth. Molds were grown on MEA plates for 7 days at 25°C, and the spore suspension of each mold was collected in sterile 0.9% saline solution (pH 7.0) and counted with a Malassez counting chamber. Suspension concentration of each mold was adjusted to 1.0 × 106 spores/mL and stored at −80°C until further use. Manganese chloride (MnCl2) was obtained from Sigma-Aldrich (Schnelldorf, Germany) and used for the evaluation of manganese depletion. Commercial plain yogurt (3.5% fat, no sugar or additives, pH 4.6) was purchased from the local supermarket (Arla Foods, Copenhagen, Denmark).
Growth Potential Assessment of 13 Dairy-Associated Molds
Growth Curves of 13 Molds Monitored by oCelloScope
Mold cell growth was monitored at 25°C for 24 h as described by Aunsbjerg et al. (2015b) using the oCelloScope detection system (BioSense Solutions ApS, Farum, Denmark), a newly developed optical detection system based on a unique optical scanning technology FluidScopeTM combining optical techniques. One hundred microliters of spore suspension of each mold (1.0 × 103 spores/mL) in MEB medium were added into a 96-well microplate (approximately 100 spores/well). The 96-well microplate was standing at room temperature for around 1 h after inoculation to allow the spores to settle in these wells, and then placed in the oCelloScope system. The samples were measured automatically and the instrument-derived growth values were recorded every 60 min for 24 h. Automated growth kinetic analyses were performed using the Background Corrected Absorption (BCA) algorithm {BCA = log10[Σ(corrected absorption pixel histograms)]} of the oCelloScope-specific software, UniExplorer (v.5.0.3). For each mold strain, growth testing was performed with three technical replicates.
Mold Growth Potential on Yogurt-Agar Plates at Different Temperatures
Mold growth potential in plain yogurt was assessed by spotting 20 μL of spore suspension of each mold (1.0 × 105 spores/mL) on yogurt-agar plates (yogurt was tempered in a 48°C water bath and then mixed with melted, tempered agar) in triplicate (2.0 × 103 spores/spot) (Aunsbjerg et al., 2015a). Plates were incubated at 5, 16, and 25°C, respectively, for up to 24 days, to evaluate the effect of temperature on fungal growth. Multispectral images of plates with spotted molds were captured by a Videometer Lab2 spectral imaging instrument (Videometer ApS, Hørsholm, Denmark), and mold growth was expressed by the average size of three colonies in pixel units calculated according to Ebrahimi et al. (2015) using MATLAB 2018b software (MathWorks, inc., Natick, MA, United States).
Sensitivity of Molds Toward 12 LAB Cultures in MRS
High-Throughput Overlay Test
The antifungal activity of 12 LAB cultures against 13 molds was initially tested using the high-throughput overlay method developed by Garnier et al. (2018). The test was performed in 24-well microplates (Sigma-Aldrich) containing 500 μL of 1.5% MRS agar. One microliter of each culture suspension (1.0 × 107 CFU/mL) was spotted on the center of each well and the 24-well microplates were incubated at 37°C for 48 h under anaerobic conditions. Thereafter, wells were overlaid with 100 μL of 0.5% malt extract soft agar inoculated with the individual mold at a final concentration of 1.0 × 103 spores/mL (100 spores/well). Wells inoculated with molds but without LAB cultures were used as control. The 24-well microplates were incubated for up to 8 days at 25°C. The antifungal activity was determined by visually evaluating fungal growth as strong inhibition (+++), weak inhibition (++), fungal growth with delayed sporulation (+), and no inhibition (−) on the basis of the inhibition zone (Figure 1A). For each mold strain, growth inhibition testing was performed with three well replicates.
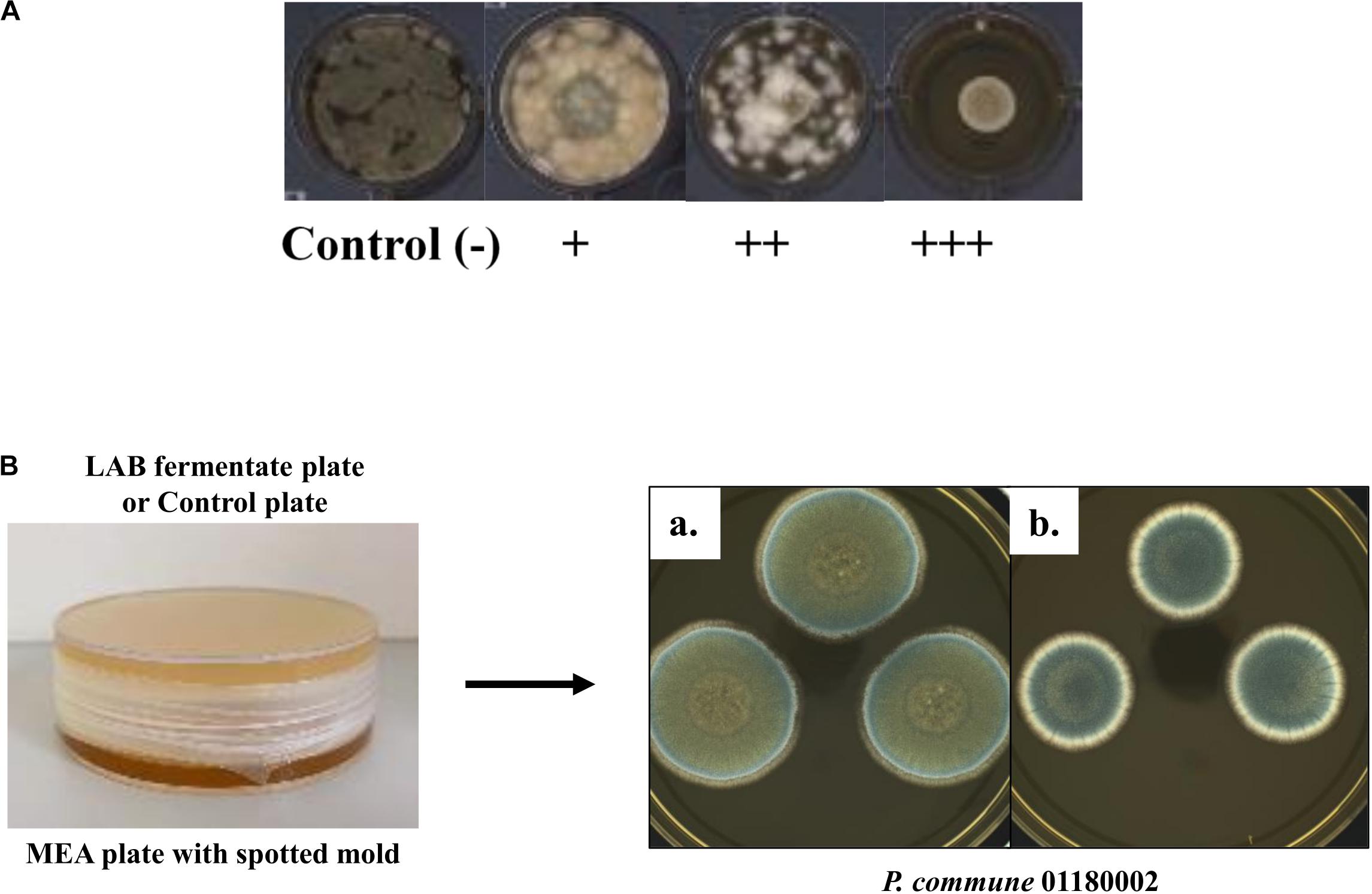
Figure 1. (A) Example of the antifungal inhibition scoring of LAB cultures overlaid by 0.5% malt extract soft agar containing P. roqueforti ISI4. Control: MRS agar in the absence of antifungal culture overlaid by molds; “+++”: strong inhibition; “++”: weak inhibition; “+”: growth but delayed sporulation; “–”: no inhibition. (B) A plate-on-plate system. Inhibitory effect of volatile compounds produced by L. parabuchneri LPB02 (plate on top) on growth of P. commune 01180002 on a MEA plate (bottom). Growth of P. commune 01180002 on the bottom MEA plate with a MRS fermentate of L. parabuchneri LPB02 plate on top (b) and a control plate on top (a) in a closed chamber, respectively, was assessed.
Sensitivity of the Molds Toward Different Preparations of LAB
In this test, the sensitivity of the target molds toward different preparations of LAB in MRS was evaluated by assessing the direct interactive effect of molds with the LAB fermentates containing live cells, with the LAB fermentates without cells, and with no direct contact between the molds and the LAB culture medium. MRS broth was inoculated with individual 12 LAB cultures at a final concentration of 1.0 × 107 CFU/mL in 250 mL blue cap flasks and then fermented at 37°C for 22 h, which was regarded as sufficient to display antifungal activity (Aunsbjerg et al., 2015a), in order to obtain LAB fermentates. The pH and the number of CFU/mL of 12 LAB cultures were determined before and after fermentation, respectively. Cell-free fermentates were prepared by centrifugation of LAB fermentates with cells at 4, 400 × g for 20 min at 4°C, followed by filtration through a 0.45 μm filter (Syringe Filter Q-Max, Frisenette ApS, Denmark). Un-inoculated MRS broth kept at 37°C for 22 h was used as control. The antifungal activity of 12 LAB fermentates with or without live cells against target molds was measured using an agar spot method, and the contribution of volatile compounds produced by LAB to the antifungal activity was assessed in a “Plate-on-Plate” test system (Figure 1B) without direct contact between molds and LAB fermentates with cells (Aunsbjerg et al., 2015a).
Interaction of molds with LAB fermentates containing live cells
The agar plates (1% agar) of each LAB fermentate with bacterial cells were prepared by mixing with melted, tempered MEA (in a 48°C water bath). These agar plates were used after solidification and a short drying period. The antifungal activity of each LAB culture was tested by spotting 20 μL of each spore suspension (1.0 × 105 spores/mL) on these LAB fermentates agar plates in triplicate and incubating at 25°C for 5 days. Control groups were prepared by spotting each mold suspension on the plates prepared by mixing un-inoculated MRS broth with MEA. Multispectral images of plates with spotted molds were captured by Videometer Lab2 spectral imaging instrument and the size of each mold colony was calculated based on the number of pixels using MATLAB 2018b software (Ebrahimi et al., 2015). The inhibitory effect was calculated according to the formula:
P indicates the total number of pixels of each colony in either control or sample groups.
Interaction of molds with cell-free LAB fermentates
The tests were performed as described above using the individual cell-free fermentates for the agar plates.
Interaction of molds with volatiles
An MEA plate was spotted with 20 μL of each mold spore suspension (1.0 × 105 spores/mL) in triplicate. On top of the MEA plate, a plate with LAB fermentate agar containing live cells was placed upside down and the two plates were sealed together with parafilm. Control plates were prepared by mixing MRS broth with agar. The inhibitory effect of volatiles produced by bacterial cells on mold growth was assessed after 5 days at 25°C as described above.
Based on the results of antifungal activity of the 12 LAB culture, Lactiplantibacillus plantarum LP37 was selected as one representative of the most inhibitive cultures and used as the main antifungal culture in the test of antifungal activity of LAB binary combinations. According to the provider of the strain, the addition of the strain to a mild yogurt culture did not negatively affect the sensory attributes during a shelf life of 42 days at 10°C.
Antifungal Activity of LAB Binary Combinations in Yogurt Serum
Yogurt serum, which was used as a transparent yogurt surrogate in this assay, was prepared by centrifugation of the commercial plain yogurt for 30 min at 4, 400 × g (4°C) and subsequent filtration of the supernatant through a 0.22-μm pore size filter for sterilization (Syringe Filter Q-Max, Frisenette ApS, Denmark). The serum was kept at 4°C prior to use.
The antifungal activity of LAB binary combinations in yogurt serum was tested by pre-fermentation of LAB cultures either alone or in combination (Aunsbjerg et al., 2015c). Here, L. plantarum LP37 was selected as the main culture and combined with each of the other eleven cultures. Yogurt serum was inoculated with the single or combined cultures (in the ratio of 1:1) with the initial inoculum level of 105 CFU/mL, and then fermented at 37°C for 22 h. After fermentation, the agar plates were prepared by mixing the fermentates of single or combined cultures, respectively, with melted, tempered agar. Un-inoculated yogurt serum agar plates (1% agar) were used as control. The inhibitory effect of LAB binary combinations and each LAB single culture was assessed by spotting spore suspension with 20 μL of 1.0 × 105 spores/mL of each mold on these fermentate-agar plates, respectively. The four Mucor strains were incubated at 25°C for 2 days due to their rapid growth while the nine Penicillium strains were incubated for 5 days.
Manganese Depletion as a Possible Antifungal Mechanism
In this test, L. plantarum LP37 was selected as a representative, inhibitive strain for exploring the role of manganese depletion in the growth inhibition of six Penicillium strains and two Mucor strains, representing different species of spoilage strains. The commercial plain yogurt was inoculated with L. plantarum LP37 at a final concentration of 1.0 × 107 CFU/mL, and then distributed into four bottles, which were subsequently supplemented with manganese ion at the final concentration of 0, 0.001, 0.01, and 0.1 mM, respectively (Siedler et al., 2020). Yogurt without L. plantarum LP37 and manganese was used as control. The yogurt agar plates were prepared by mixing yogurt samples with melted, tempered agar. Then 20 μL of spore suspension (1.0 × 104 spores/mL) of each mold were spotted on these yogurt agar plates in triplicate. After incubation for 5 days at 25°C, quantification of mold growth was performed as described in Subsection “Sensitivity of the Molds Toward Different Preparations of LAB.”
In addition, manganese depletion test was carried out in yogurt serum as well after pre-fermentation with L. plantarum LP37. In brief, L. plantarum LP37 was inoculated in yogurt serum at a final concentration of 1.0 × 107 CFU/mL and then fermented at 37°C for 22 h prior to use. Both L. plantarum LP37 fermentates with or without bacterial cells were used for testing the manganese depletion. Manganese ion was added into yogurt serum as described above and the agar plates were prepared by mixing with agar. The pH and number of CFU/mL of L. plantarum LP37 were determined before and after fermentation. Un-inoculated yogurt serum kept at 37°C for 22 h was used as control. Each spore suspension was spotted as described above.
Statistical Analyses
All the tests in this study were done in triplicate and the values were expressed as mean values ± standard deviation (SD). The statistical significances of experimental antifungal activity of LAB combinations were evaluated by one-way analysis of variance (ANOVA), differences were considered statistically significant at P < 0.05 (∗P < 0.05, ∗∗P < 0.01). Statistical analyses were performed using SPSS software ver. 26.0 (IBM, Armonk, NY, United States).
Results
Real-Time Monitoring of Cell Growth Using oCelloScope
Growth kinetics analysis of 13 molds during 24 h at 25°C in MEB medium (Supplementary Figure 1A) showed that the initial inoculum size of all the tested molds was approximately at the same level (100 spores/well), while large variations were observed in the growth potential. The Mucor strains generally grew faster than the Penicillium strains with M. plumbeus 01180036 as the most rapidly growing isolate. M. circinelloides 01180023 was the first isolate to reach stationary phase after approximately 10 h but reached a lower maximum level than the other strains. The fastest growing isolate among Penicillium strains was Penicillium glabrum ISI3, which grew almost as fast as the M. plumbeus strains. In contrast, P. roqueforti ISI4 cells displayed the slowest initial growth with respect to other strains tested. In addition, the morphological changes of molds, such as hyphae development, could also be studied visually from the oCelloScope images and quantified using morphological descriptors (Aunsbjerg et al., 2015b) as exemplified in Supplementary Figure 1B, showing the growth of P. solitum ISI5 and M. circinelloides 01180023 in MEB medium. Here, hyphae formation could be observed in the Mucor strain already within 4 h.
Mold Growth Potential on Yogurt-Agar Plates
At the three tested temperatures, 5, 16, and 25°C, all tested molds were capable of growth on yogurt agar plates (Figure 2) in a temperature-dependent manner. For example, M. circinelloides 01180023 required around 14 days to reach a specific colony size of 1.5 × 105 (in pixels) at 5°C, while this took 4 days at 16°C and 3 days at 25°C. At 5°C (Figure 2A), mold growth with sporulation was observed after incubation ranging from 9 to 11 days. Large variations were observed with Mucor strains generally growing faster than the Penicillium strains, and with M. circinelloides 01180023 as the fastest growing isolate. Growth of P. roqueforti ISI4 started out slowly, as also seen in the 24 h oCelloScope test, but it increased rapidly after some days and this strain had the highest growth recorded of the Penicillium tested after day 20 at 5°C and day 5 at 25°C, respectively. Also at 16°C (Figure 2B), the growth rate of Mucor strains was higher than Penicillium strains, and M. circinelloides 01180023 was still the faster growing mold. The results at 25°C (Figure 2C) were in accordance with the results in oCelloScope test. The four Mucor strains grew faster both in MEB medium and yogurt than the Penicillium strains regardless of temperature. Noteworthy, P. glabrum ISI3 grew rapidly at 25°C but it was the slowest isolate at 5°C, indicating that this strain is more sensitive to chilling. Moreover, P. roqueforti ISI4, initially the slowest growing mold, became the fastest growing Penicillium strain after incubation for 4 days. The mean values of the size of mold colonies were shown in Supplementary Table 1, and the values differed significantly (P < 0.05).
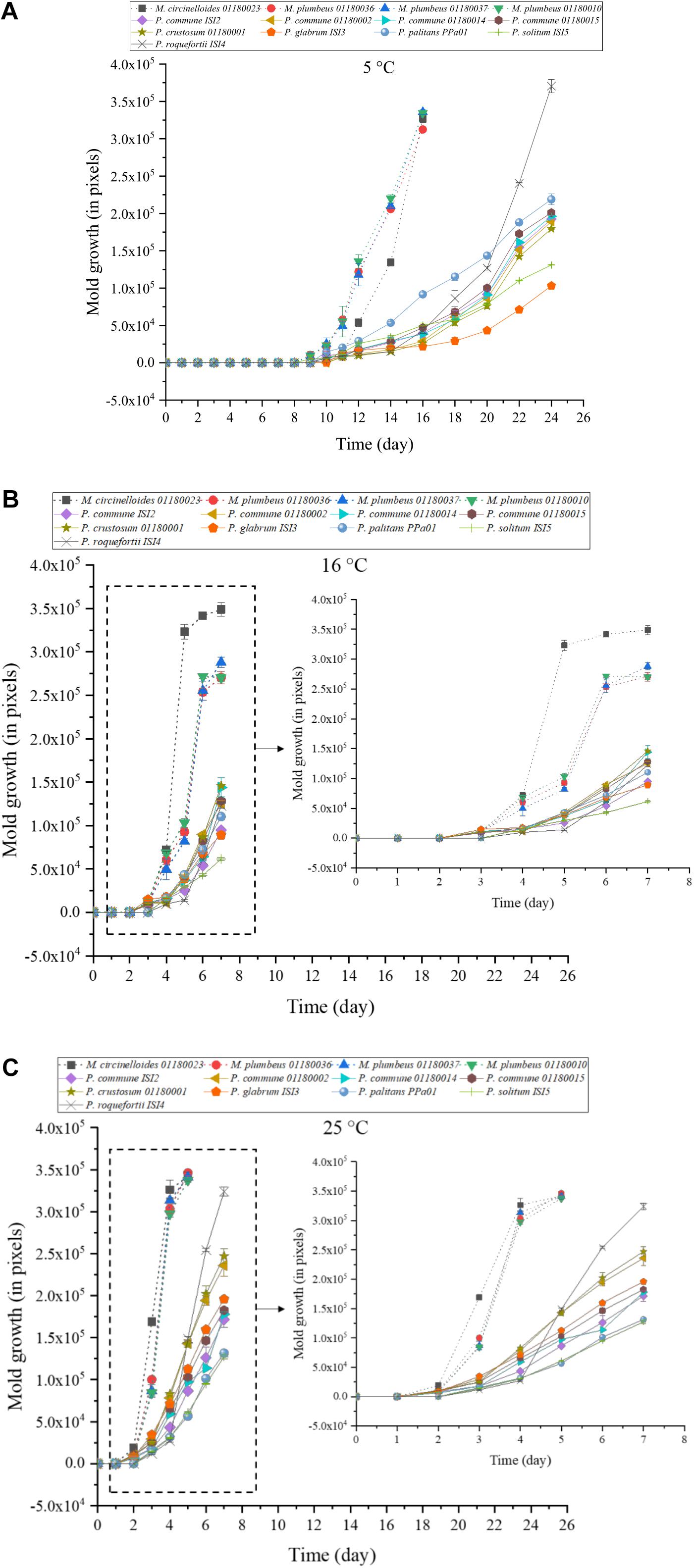
Figure 2. Growth potential of 13 molds on yogurt-agar plates incubated for up to 24 days at 5°C (A), 16°C (B), and 25°C (C), respectively. The test was determined by spotting each spore suspension (20 μL of 1.0 × 105 spores/mL) on yogurt-agar plates in triplicate. Bars represent the standard error of mean of three replicates. Four Mucor strains are marked with dotted lines; nine Penicillium strains with solid lines. The growth curves in (B,C) in the dotted box are magnified.
Sensitivity Toward 12 LAB Cultures
High-Throughput Overlay Method
When employing a high-throughput overlay screening technique to detect antifungal activity of 12 LAB cultures against 13 molds, results obtained after 5 days were similar to those after 8 days of incubation. In this test, all tested molds were greatly inhibited (+++) by all the 12 LAB cultures at the concentration of 107 CFU/mL except P. roqueforti ISI4, which showed resistance to all the tested LAB cultures except for a weak inhibition when exposed to Lentilactobacillus parabuchneri LPB04 (Table 2). The sporulation of this isolate was, however, delayed in all cases.
Sensitivity Toward 12 LAB Fermentates With Live Cells
The antifungal activity of 12 LAB fermentates with live cells in MRS against tested molds was tested. Results indicated that the inhibitory effect of the LAB fermentates with cells varied drastically (Figure 3A). In general, the 12 LAB cultures exhibited strong inhibition on the growth of all molds except P. roqueforti ISI4, which showed no or very weak inhibition as also seen previously in the high-throughput overlay test. In comparison with the results of the high-throughput overlay test, more differentiation of the response was observed since some strains (M. circinelloides 01180023, Penicillium commune 01180015 and Penicillium palitans PPa01) were only inhibited to a lesser degree (up to 50%). Comparing with other LAB cultures, L. plantarum LP48 showed weaker inhibition on P. commune 01180015 and P. palitans PPa01 with inhibitory effect of around 60 and 75%, respectively. The inhibitory effect of L. paracasei LPC46 on M. circinelloides 01180023 was only around 50%. Although some fermentates (e.g., L. parabuchneri LPB02 and L. plantarum LP48) displayed a slight inhibitory effect on P. roqueforti ISI4 of around 20–25%, these LAB cultures did not have the capacity to strongly inhibit the growth of this mold. After incubation for 9 days (data not shown), some tested molds were still strongly inhibited by the fermentates, while for other molds, some growth was observed after the 9 days, indicating a more fungistatic rather than fungicidal effect.
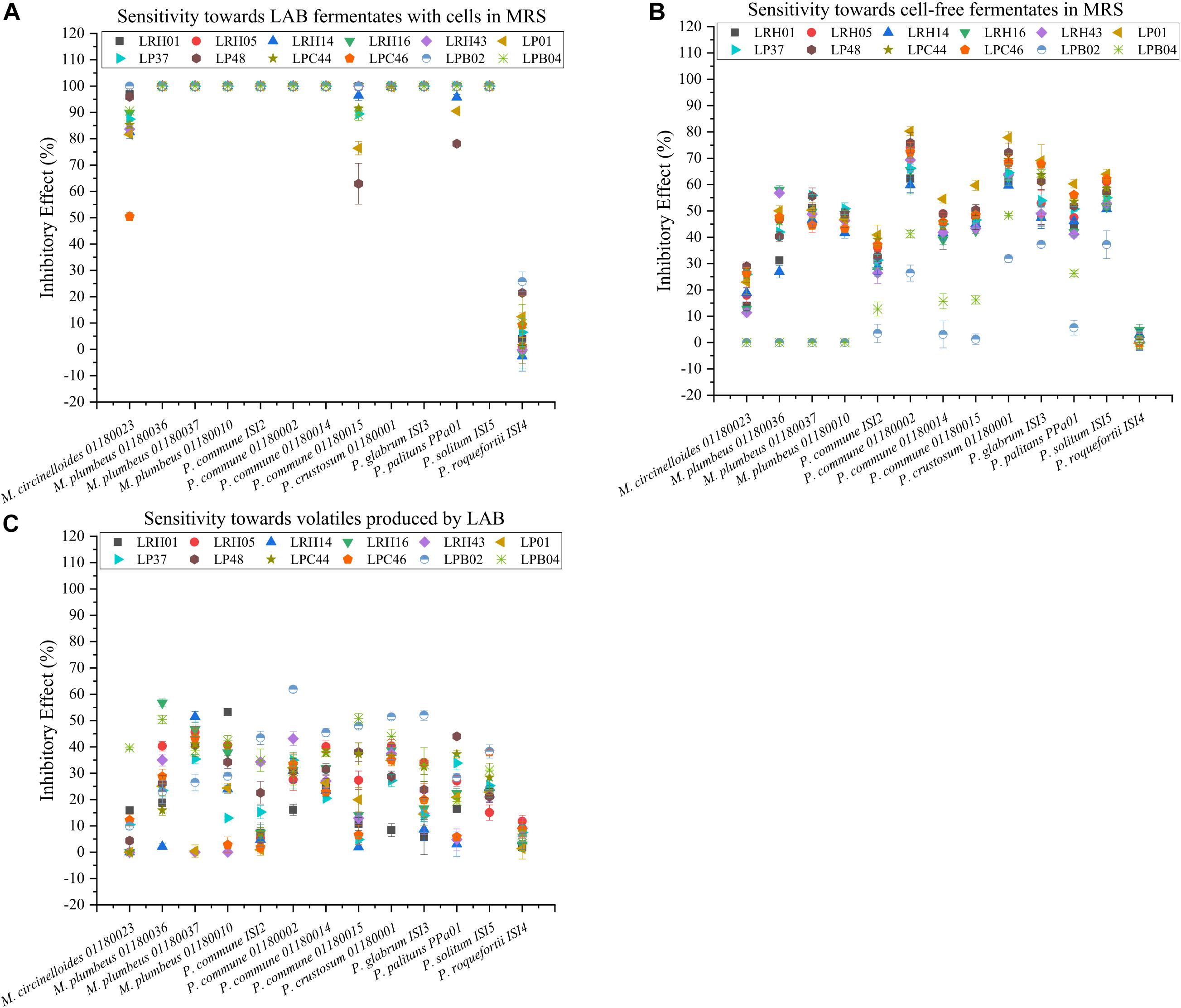
Figure 3. Sensitivity of 13 molds toward 12 LAB fermentates with live cells (A), LAB cell-free fermentates (B), and volatiles from LAB cultures in MRS (C), respectively. The LAB strain code: LRH01, LRH05, LRH14, LRH16, and LRH43 are L. rhamnosus strains, LP01, LP37, and LP48 L. plantarum strains, LPC44 and LPC46 L. paracasei strains, and LPB02 and LPB04 L. parabuchneri strains. Bars represent the standard error of mean of three replicates.
Sensitivity of the Molds to Cell-Free Fermentates
In this assay, the sensitivity of molds to LAB cell-free fermentates was determined in MRS medium. As seen in Figure 3B, the removal of cells resulted in decreased inhibition efficacy. Large variations of the inhibitory effect were seen with P. commune 01180002 being more sensitive, followed by P. solitum ISI5, whereas P. roqueforti ISI4 again was largely unaffected. Among the four LAB species tested, the L. plantarum cell-free fermentates gave rise to the strongest inhibitory effects whereas L. parabuchneri cell-free fermentates lost most of their effect. In addition, the antifungal compounds produced by L. plantarum LP01 displayed stronger inhibition on P. commune strains.
Sensitivity of the Molds to Volatiles Produced by Bacterial Cells
In order to test the antifungal volatiles, a plate-on-plate system was employed. Here, the inhibitory effect of volatile compounds produced by the 12 LAB cultures was modest, ranging from 0 to around 60% (Figure 3C). In contrast with the results in cell-free fermentates, the L. parabuchneri cultures here displayed higher inhibitory effect compared with other LAB cultures. The volatiles produced by L. parabuchneri LPB02 showed the highest inhibitory effects on P. commune 01180002 (60%), followed by Penicillium crustosum 01180001 and P. glabrum ISI3. Since the cell-free fermentate of the same strain, L. parabuchneri LPB02, gave rise to a relatively weak inhibition of these molds, this indicated that the activity of L. parabuchneri LPB02 grown in MRS against molds partly relied on volatiles.
Inhibitory Effect of LAB Combinations in Yogurt Serum
Based on the results in MRS, L. plantarum LP37 was selected as a representative for the inhibitory cultures and used in binary combinations with each of the other eleven LAB cultures with an initial approximate inoculum level of 105 CFU/mL. As shown in Table 3, 3 out of 11 binary combinations, including the binary combinations of L. plantarum LP37 combined with either L. rhamnosus LRH14, L. plantarum LP01, or L. plantarum LP48, showed significant improvement of antifungal activity against P. commune strains and/or P. palitans PPa01 compared with the corresponding single cultures (P < 0.05). P. roqueforti ISI4, which was neither inhibited by L. plantarum LP37 nor L. paracasei LPC46 on their own, was weakly inhibited by their combination, indicating some synergistic effect (Le Lay et al., 2016).
Manganese Depletion by L. plantarum LP37 in Yogurt and Yogurt Serum
In comparison with the control groups, the molds displayed various levels of sensitivity when grown with L. plantarum LP37 in yogurt-agar plates. However, the additions of increasing manganese concentrations (up to 0.1 mM) partly or fully restored the growth of the five sensitive Penicillium and two Mucor strains, suggesting that manganese depletion by L. plantarum LP37 plays a dominating role in the inhibition observed in these strains (Figure 4). Again, P. roqueforti ISI4 was almost non-affected by both L. plantarum LP37 and manganese.
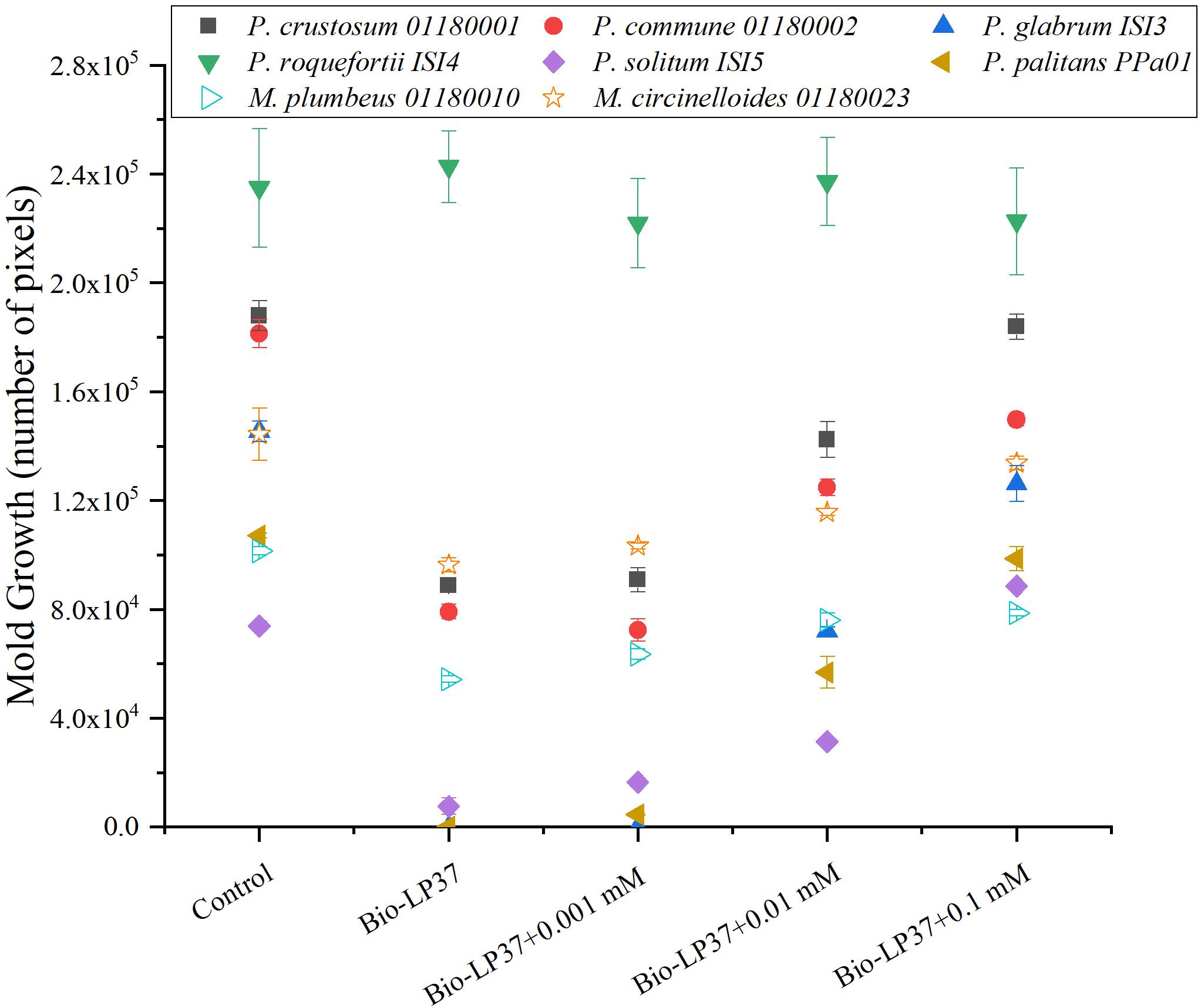
Figure 4. Manganese depletion by L. plantarum LP37 in yogurt. Different manganese concentrations (0.001, 0.01, and 0.1 mM) were added as indicated. The plates with spotted Penicillium strains and Mucor strains (200 spores/spot) were incubated at 25°C for 5 days. Bars represent the standard error of mean of three replicates. Bio-LP37 indicated the culture L. plantarum LP37. Closed symbols indicate Penicillium strains (6); Open symbols indicate Mucor strains (2).
In addition, yogurt serum was used as the culture medium to ferment LAB cultures. In Figure 5A, as expected, mold growth was partly or fully restored with addition of manganese in a concentration dependent manner, while P. roqueforti ISI4 was almost non-affected. Results here were in agreement with test carried out in yogurt, indicating that the manganese depletion of the culture medium was a limiting factor in both scenarios naturally low in manganese content. If cells were removed (Figure 5B), the antifungal activity of the cell-free yogurt serum was almost lost, indicating limited influence of any preformed compounds in the fermentate.
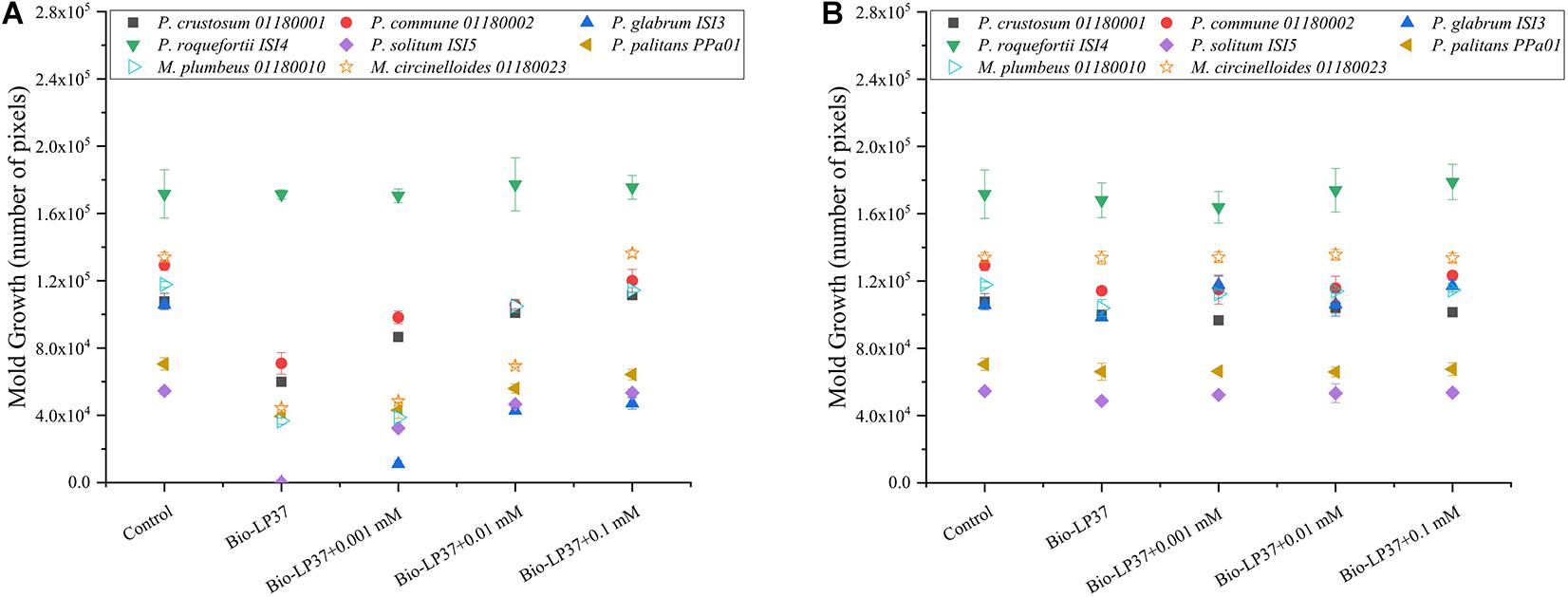
Figure 5. Manganese depletion by L. plantarum LP37 fermentate in yogurt serum with (A) or without bacterial cells (B). Different manganese concentrations (0.001, 0.01, and 0.1 mM) were added as indicated. The plates with spotted Penicillium strains and Mucor strains (200 spores/spot) were incubated at 25°C for 5 days. Bio-LP37 indicates the bioprotective culture L. plantarum LP37. Closed symbols indicate six Penicillium strains; Open symbols indicate two Mucor strains.
Discussion
Different molds can spoil fresh, fermented dairy products but they may have different characteristics affecting their response. Here, the growth potential of Penicillium and Mucor species isolated from relevant products was determined as well as their response toward the activity of a range of LAB cultures. Firstly, the growth curves of molds were monitored in real time using the oCelloScope detection system, a small portable platform based on an automated optical detection system (Aunsbjerg et al., 2015b; Bouillon et al., 2019), during initial fungal growth at 25°C. The method is useful for early detection of growth before a three dimensional hyphae network has been formed (Aunsbjerg et al., 2015a). Growth was also followed on yogurt agar plates incubated at different temperatures, where 5°C was chosen to simulate a refrigeration storage temperature, 16°C an abuse cold chain temperature, and 25°C room temperature. Results from the oCelloScope test and the yogurt-agar spot test were in good agreement. However, the oCelloScope only covered the early growth. While P. roqueforti ISI4 grew slowly initially in both tests, the yogurt spot test, which ran for several days, revealed a later fast increase in the growth of this mold compared with the other Penicillium strains tested. The final high growth level supports Leggieri et al. (2017), who reported that P. roqueforti exhibited optimal growth at 25°C. Regardless of test method or temperatures, the growth of the Mucor strains seemed generally faster than the Penicillium strains. Similarly, Morin-Sardin et al. (2016) examined the growth of seven Mucor strains and found that they displayed strain differences in temperature optima, but they could, nevertheless, all be defined as fast. Gougouli et al. (2011) monitored mycelium growth rates of 12 mold strains grown in yogurt including six Penicillium spp. and one M. circinelloides, and found that although there were considerable differences within the Penicillium strains, the M. circinelloides strain still grew faster within a temperature range of 5–35°C. It has been suggested that the lack of septate formation in Mucor strains facilitates translocations within the cell and thereby promotes very rapid mycelium formation (Pitt and Hocking, 2009). All strains grew at 5°C underlining their potential for chilled storage spoilage. They did, however, grow slower on yogurt-agar than on MEA plates (Supplementary Figure 2). For example, the growth of P. commune 011800015 and P. solitum ISI5 with sporulation was observed after 7 days of incubation on MEA plates, whereas this took 10 and 9 days, respectively, on yogurt-agar plates. The differences could be partly due to the difference in pH as several previous studies such as Gock et al. (2003) and Morin-Sardin et al. (2016), who showed that the optimal pH for a variety of Mucor and Penicillium strains is closer to that of MEA (pH 5.6) rather than the pH 4.6 of yogurt.
The antifungal activity of 12 LAB cultures against the molds was screened in MRS medium using a high-throughput overlay method in 24-well microplates. The high-throughput antifungal overlay method allows for a rapid screening of a large variety of antifungal agents and the approach is feasible for many laboratories due to the ease and low equipment cost. In this system, all the LAB cultures had strong activity toward all the molds except P. roqueforti ISI4. The observed robustness of P. roqueforti toward a number of LAB cultures may be a common characteristics within this species, since this feature has also been observed in other P. roqueforti strains (Magnusson et al., 2003; Cheong et al., 2014; Russo et al., 2017; Quattrini et al., 2018). Although there is major intraspecific diversity in P. roqueforti, they are known to tolerate low concentrations of oxygen and high levels of CO2 or weak acid preservatives (Ropars et al., 2017; Coton et al., 2020). Using the overlay method, several studies have found different species of LAB to have strong antifungal activity. This includes Inglin et al. (2015), who reported both antifungal and antibacterial activity of LAB species; Garnier et al. (2018), who found that Mucor racemosus and P. commune could be inhibited by certain L. rhamnosus and L. plantarum cultures, and Salas et al. (2018), who found that a mixture of Lactobacillus harbinensis (L. harbinensis) and L. plantarum exhibited inhibitory effect on Mucor and Penicillium strains.
The sensitivity of these molds, apart from P. roqueforti ISI4, toward the 12 LAB cultures was confirmed in an agar spot test on fermentates with cells grown in MRS. This test, however, allowed for a slightly more differentiated response. When the cells were removed from the fermentates, the mold growth was still partly suppressed but the antifungal activity decreased markedly in comparison with that of fermentates with live cells. The remaining antifungal activity of cell-free fermentates in MRS is probably due to the presence of various active compounds produced by these LAB cultures in the supernatant such as organic acids, fatty acids, phenolic compounds, proteinaceous compounds and hydrogen peroxide (H2O2) which have been described as being antifungal (Dalié et al., 2010). Belguesmia et al. (2013) found that the cell-free culture supernatant of L. harbinensis in MRS had robust antifungal activities against Penicillium expansum, and observed that the supernatant contained high amounts of organic acids such as lactic and acetic acids as well as hexanoic acid. Guimarães et al. (2018) showed that the cell-free supernatants of L. plantarum and Lactobacillus buchneri inhibited Penicillium nordicum radial growth and suggested that this was due to the production of specific organic acids, such as lactic acid, phenyllactic acid, hydroxyphenyllactic acid and indole lactic acid. Apart from antifungal compounds in the cell-free fermentates, it is also possible that nutrient depletion has taken place although MRS is a rich medium. As reported by previous study, Honoré et al. (2016) indicated that glucose and glutamine in a chemically defined medium were almost fully consumed by L. paracasei strains, while other nutrients remained at 50% or more of the initial content, which was speculated to correlate with the decreased mold growth. In nature, competitive exclusion due to the competition for nutrients (Honoré et al., 2016), space (Golowczyc et al., 2007), and essential trace ions is a widespread phenomenon also between bacteria and fungi (Mille-Lindblom et al., 2006). Very recently, Siedler et al. (2020) has pointed toward manganese depletion as a major antifungal mechanism in a yogurt context. Compared with yogurt, MRS has a high amount of trace ions making depletion less likely to explain all the inhibition observed. The specific contribution of volatile metabolites produced by LAB cultures to the antifungal activity was estimated in a non-contact assay. Here, some inhibitory activity of volatile compounds was observed although the effect was much lower than that of cell-containing fermentates of LAB cultures in MRS. Our results here confirm that some of the bacterial volatiles (Fernando et al., 2005) have a measurable antifungal effect, albeit modest in this system. Leyva Salas et al. (2019) suggested that the inhibition of P. commune in sour cream was associated with higher amounts of three volatile compounds produced by LAB: diacetyl, acetoin and an unidentified volatile compound; Arrebola et al. (2010) likewise reported the antifungal properties of volatile compounds such as diacetyl, acetoin, dimethyl sulfone, 2-butanone, and volatile fatty acids and Aunsbjerg et al. (2015a) found that in a defined medium, the main volatile compound measured in the headspace of a cell-containing fermentate of L. paracasei during fermentation was diacetyl, which had a strong inhibitory effect on two Penicillium strains.
Although laboratory media optimized for growth of LAB cultures are well suited for an initial screening of the antifungal activity of the cultures, they do not reflect the composition of the relevant foods nor previous starter culture activity. Further investigations were therefore conducted in yogurt and yogurt serum in order to simulate the conditions in yogurt better. Manganese, an essential trace element, is a key cofactor for the growth of mold (Avila et al., 2013) and the level in milk is low (0.03 mg/L) (Siedler et al., 2020). According to Böhmer et al. (2013), due to the absence of superoxide dismutase, L. plantarum requires a relatively high level of manganese ions for optimal growth (up to 30 mM) under aerobic conditions. L. plantarum LP37 was selected as a representative of an inhibitory LAB and was capable of inhibiting growth of 5 out of 6 selected Penicillium spp. (P. roqueforti was unaffected) and two Mucor strains in yogurt and yogurt serum. In all cases of inhibition, the growth could be partly or fully restored by addition of manganese up to 0.1 mM, thus illustrating that not only the Penicillium but also the Mucor strains were inhibited by the lack of accessible manganese. The inhibition of mold growth in yogurt therefore seems to be associated with the ability of the L. plantarum culture to efficiently scavenge manganese, further supporting that in dairy products the removal of manganese accessible to molds is a main inhibitory mechanism of bioprotective cultures. This also implies that the same cultures may not have the same effect if the products are based on or mixed with materials with high manganese content such as plant materials.
In comparison with the inhibitory effect of the 12 LAB fermentates with live cells in MRS (Figure 3A), the sensitivity of these target molds decreased when the LAB fermentates were based on yogurt serum (Table 3). It was also observed that L. plantarum LP37 exhibited stronger antifungal activity against both Mucor and Penicillium strains in MRS medium than observed in yogurt or yogurt serum (Supplementary Figure 5). In the cell-free L. plantarum LP37 yogurt serum fermentate, mold growth was not inhibited (Figure 5B) in contrast to what was observed in the MRS based media (Figure 3B). The lack of inhibition may partly be due to the less optimal growth conditions for the LAB culture in yogurt serum, where both pH (pH 4.6) and nutrient levels are lower than in MRS (start pH 5.6). It was observed that in MRS medium, the number of bacterial cells of L. plantarum LP37 reached 109 CFU/mL after the 22 h fermentation (Supplementary Figure 3), while only a level of approximately 108 CFU/mL was reached in the yogurt serum (Supplementary Figure 4). Production of compounds with antifungal effect would therefore also be predicted to be present in much higher concentrations and thereby potentially plays a larger role in the MRS fermentates compared with the yogurt serum. This further illustrates the importance of testing in matrices relevant to the food in question when evaluating interactions. A broad panel of target organisms is also desirable since it showed that both Penicillium and Mucor species were sensitive to the manganese depletion, but also that some molds such as P. roqueforti are not affected and will require different countermeasures.
The enhancement of the inhibitory effect by employment of combinations of different LAB cultures or mixed with propionibacteria has been noted in several studies (Schwenninger and Meile, 2004; Salas et al., 2018) and several mixed bioprotective cultures are commercially available. The mixtures may have the advantage of employing different mechanisms or support mutual growth without having a negative impact on the sensory properties. The option of using different LAB combinations can therefore increase the technological flexibility in terms of products and target organisms and may also decrease costs, if it is possible to use lower inocula than seen for single cultures. Here, binary combinations containing L. plantarum LP37 were tested in yogurt serum to investigate potential synergistic effects. Results indicated that the binary combination of L. plantarum LP37 and L. plantarum LP48 in yogurt serum had significantly improved antifungal activity (P < 0.05) against some molds, two P. commune strains, compared with single cultures (Table 3). It is noteworthy that the inhibitory effects observed in mixed cultures were obtained with relatively low start inocula, which could make the use of bioprotective cultures more economically feasible. On the other hand, higher start inocula would most likely ensure stronger inhibitory effects.
Overall, the interaction between LAB and spoilage fungi is very complex and the LAB will exert antifungal activity in different modes depending on the circumstances making it challenging to develop all-purpose bioprotective cultures. On the other hand, selection of cultures for a particular environment such as plain yogurt or similar dairy products, can be facilitated by recognizing the role of manganese accumulation capacity in this environment. Further work should be undertaken to define which interaction mechanisms are at play in different scenarios and how factors such as oxygen and production of reactive oxygen species, as well as compositional changes of the matrix, influence the effect of the bioprotective cultures.
Conclusion
Dairy products are very susceptible to spoilage molds, and it is therefore crucial to characterize the growth potential of molds. Here, Mucor strains grew faster than Penicillium strains both in MEB medium and yogurt. All tested molds, except P. roqueforti ISI4, were strongly inhibited by the 12 LAB strains when they were grown in MRS. After removal of the LAB living cells from MRS based fermentates, the inhibitory effect decreased, and the effect of volatiles alone was even less, indicating that preformed compounds played a role but they were not the main inhibitory factor. When yogurt serum was employed as a medium, a reduced LAB growth and a weaker inhibition of mold growth were observed, while removal of the cells caused almost complete loss of activity. The inhibition efficacy could in some instances be enhanced by combining cultures. In yogurt, it was shown that manganese depletion by L. plantarum LP37 played an important role in the growth inhibition of both the Penicillium and the Mucor strains tested. Apart from the non-affected P. roqueforti ISI4, it therefore seems that these spoilage molds are generally sensitive to bioprotective cultures capable of making the manganese unavailable in the matrix. Elucidation of the importance of growth conditions, manganese sources, and the rate of manganese transport would further help to expand the use of bioprotective cultures in similar matrices. The inhibitory effect and mode of action of the bioprotective cultures thus seems to depend not only on the target organisms, and growth of the culture but also on the composition of the matrix.
Data Availability Statement
The raw data supporting the conclusions of this article will be made available by the authors, without undue reservation.
Author Contributions
CS designed and performed the experiments, contributed to the conception and data analysis, and prepared the manuscript. SK designed and supervised the project, analyzed the data, and reviewed the manuscript. Both authors read and approved the final manuscript.
Funding
This work was financially supported by Project “Antifungal Dairy Product Bioprotection” financed by the Danish Dairy Research Foundation, Faculty of Science, University of Copenhagen, and the China Scholarship Council (grant: 201706170083).
Conflict of Interest
The authors declare that the research was conducted in the absence of any commercial or financial relationships that could be construed as a potential conflict of interest.
Acknowledgments
We gratefully acknowledge F. W. J. van der Berg (Department of Food Science, University of Copenhagen, Denmark) for help with the analysis of Videometer data using MATLAB software. We also sincerely acknowledge SACCO S.r.l. (Italy) for providing the bioprotective cultures.
Supplementary Material
The Supplementary Material for this article can be found online at: https://www.frontiersin.org/articles/10.3389/fmicb.2021.631730/full#supplementary-material
References
Arrebola, E., Sivakumar, D., and Korsten, L. (2010). Effect of volatile compounds produced by Bacillus strains on postharvest decay in citrus. Biol. Control. 53, 122–128. doi: 10.1016/j.biocontrol.2009.11.010
Aunsbjerg, S. D., Andersen, K. R., and Knøchel, S. (2015a). Real-time monitoring of fungal inhibition and morphological changes. J. Microbiol. Methods 119, 196–202. doi: 10.1016/j.mimet.2015.10.024
Aunsbjerg, S. D., Honoré, A. H., Marcussen, J., Ebrahimi, P., Vogensen, F. K., Benfeldt, C., et al. (2015b). Contribution of volatiles to the antifungal effect of Lactobacillus paracasei in defined medium and yogurt. Int. J. Food Microbiol. 194, 46–53. doi: 10.1016/j.ijfoodmicro.2014.11.007
Aunsbjerg, S. D., Honoré, A. H., Vogensen, F. K., and Knøchel, S. (2015c). Development of a chemically defined medium for studying foodborne bacterial-fungal interactions. Int. Dairy J. 45, 48–55. doi: 10.1016/j.idairyj.2015.01.019
Avila, D. S., Puntel, R. L., and Aschner, M. (2013). Manganese in health and disease. Met. Ions Life Sci. 13, 199–227. doi: 10.1007/978-94-007-7500-8-7
Bazukyan, I., Matevosyan, L., Toplaghaltsyan, A., and Trchounian, A. (2018). Antifungal activity of lactobacilli isolated from Armenian dairy products: an effective strain and its probable nature. AMB Express 8:87. doi: 10.1186/s13568-018-0619-y
Belguesmia, Y., Rabesona, H., Mounier, J., Pawtowsky, A., Le Blay, G., Barbier, G., et al. (2013). Characterization of antifungal organic acids produced by Lactobacillus harbinensis K.V9.3.1Np immobilized in gellan-xanthan beads during batch fermentation. Food Control 36, 205–211. doi: 10.1016/j.foodcont.2013.08.028
Bouillon, G. A., Gåserød, O., and Rattray, F. P. (2019). Evaluation of the inhibitory effect of alginate oligosaccharide on yeast and mould in yoghurt. Int. Dairy J. 99:104554. doi: 10.1016/j.idairyj.2019.104554
Böhmer, N., König, S., and Fischer, L. (2013). A novel manganese starvation-inducible expression system for Lactobacillus plantarum. FEMS Microbiol. Lett. 342, 37–44. doi: 10.1111/1574-6968.12105
Campana, R., Federici, S., Ciandrini, E., Manti, A., and Baffone, W. (2019). Lactobacillus spp. inhibit the growth of Cronobacter sakazakii ATCC 29544 by altering its membrane integrity. J. Food Sci. Technol. 56, 3962–3967. doi: 10.1007/s13197-019-03928-x
Cheong, E. Y. L., Sandhu, A., Jayabalan, J., Kieu Le, T. T., Nhiep, N. T., My Ho, H. T., et al. (2014). Isolation of lactic acid bacteria with antifungal activity against the common cheese spoilage mould Penicillium commune and their potential as biopreservatives in cheese. Food Control 46, 91–97. doi: 10.1016/j.foodcont.2014.05.011
Coton, E., Coton, M., Hymery, N., Mounier, J., and Jany, J. L. (2020). Penicillium roqueforti: an overview of its genetics, physiology, metabolism and biotechnological applications. Fungal Biol. Rev. 34, 59–73. doi: 10.1016/j.fbr.2020.03.001
Dalié, D. K. D., Deschamps, A. M., and Richard-Forget, F. (2010). Lactic acid bacteria - potential for control of mould growth and mycotoxins: a review. Food Control 21, 370–380. doi: 10.1016/j.foodcont.2009.07.011
Ebrahimi, P., van den Berg, F., Aunsbjerg, S. D., Honoré, A., Benfeldt, C., Jensen, H. M., et al. (2015). Quantitative determination of mold growth and inhibition by multispectral imaging. Food Control 55, 82–89. doi: 10.1016/j.foodcont.2015.01.050
Fernando, W. G. D., Ramarathnam, R., Krishnamoorthy, A. S., and Savchuk, S. C. (2005). Identification and use of potential bacterial organic antifungal volatiles in biocontrol. Soil Biol. Biochem. 37, 955–964. doi: 10.1016/j.soilbio.2004.10.021
Garnier, L., Salas, M. L., Pinon, N., Wiernasz, N., Pawtowski, A., Coton, E., et al. (2018). Technical note: high-throughput method for antifungal activity screening in a cheese-mimicking model. J. Dairy Sci. 101, 4971–4976. doi: 10.3168/jds.2017-13518
Garnier, L., Valence, F., and Mounier, J. (2017). Diversity and control of spoilage fungi in dairy products: an update. Microorganisms 5:42. doi: 10.3390/microorganisms5030042
Gock, M. A., Hocking, A. D., Pitt, J. I., and Poulos, P. G. (2003). Influence of temperature, water activity and pH on growth of some xerophilic fungi. Int. J. Food Microbiol. 81, 11–19. doi: 10.1016/S0168-1605(02)00166-6
Golowczyc, M. A., Mobili, P., Garrote, G. L., Abraham, A. G., and De Antoni, G. L. (2007). Protective action of Lactobacillus kefir carrying S-layer protein against Salmonella enterica serovar enteritidis. Int. J. Food Microbiol. 118, 264–273. doi: 10.1016/j.ijfoodmicro.2007.07.042
Gougouli, M., Kalantzi, K., Beletsiotis, E., and Koutsoumanis, K. P. (2011). Development and application of predictive models for fungal growth as tools to improve quality control in yogurt production. Food Microbiol. 28, 1453–1462. doi: 10.1016/j.fm.2011.07.006
Guimarães, A., Venancio, A., and Abrunhosa, L. (2018). Antifungal effect of organic acids from lactic acid bacteria on Penicillium nordicum. Food Addit. Contam. Part A Chem. Anal. Control Expo. Risk Assess. 35, 1803–1818. doi: 10.1080/19440049.2018.1500718
Hollmann, M., Razzazi-Fazeli, E., Grajewski, J., Twaruzek, M., Sulyok, M., and Böhm, J. (2008). Detection of 3-nitropropionic acid and cytotoxicity in Mucor circinelloides. Mycotoxin Res. 24, 140–150. doi: 10.1007/BF03032341
Honoré, A. H., Aunsbjerg, S. D., Ebrahimi, P., Thorsen, M., Benfeldt, C., Knøchel, S., et al. (2016). Metabolic footprinting for investigation of antifungal properties of Lactobacillus paracasei. Anal. Bioanal. Chem. 408, 83–96. doi: 10.1007/s00216-015-9103-6
Inglin, R. C., Stevens, M. J. A., Meile, L., Lacroix, C., and Meile, L. (2015). High-throughput screening assays for antibacterial and antifungal activities of Lactobacillus species. J. Microbiol. Methods 114, 26–29. doi: 10.1016/j.mimet.2015.04.011
Lazar, S. P., Lukaszewicz, J. M., Persad, K. A., and Reinhardt, J. F. (2014). Rhinocerebral Mucor circinelloides infection in immunocompromised patient following yogurt ingestion. Del. Med. J. 86, 245–248.
Le Lay, C., Coton, E., Le Blay, G., Chobert, J. M., Haertlé, T., Choiset, Y., et al. (2016). Identification and quantification of antifungal compounds produced by lactic acid bacteria and propionibacteria. Int. J. Food Microbiol. 239, 79–85. doi: 10.1016/j.ijfoodmicro.2016.06.020
Leggieri, M. C., Decontardi, S., Bertuzzi, T., Pietri, A., and Battilani, P. (2017). Modeling growth and toxin production of toxigenic fungi signaled in cheese under different temperature and water activity regimes. Toxins 9:4. doi: 10.3390/toxins9010004
Leyva Salas, M., Mounier, J., Maillard, M. B., Valence, F., Coton, E., and Thierry, A. (2019). Identification and quantification of natural compounds produced by antifungal bioprotective cultures in dairy products. Food Chem. 301:125260. doi: 10.1016/j.foodchem.2019.125260
Luz, C., Ferrer, J., Mañes, J., and Meca, G. (2018). Toxicity reduction of ochratoxin A by lactic acid bacteria. Food Chem. Toxicol. 112, 60–66. doi: 10.1016/j.fct.2017.12.030
Magnusson, J., Ström, K., Roos, S., Sjögren, J., and Schnürer, J. (2003). Broad and complex antifungal activity among environmental isolates of lactic acid bacteria. FEMS Microbiol. Lett. 219, 129–135. doi: 10.1016/S0378-1097(02)01207-7
Mille-Lindblom, C., Fischer, H., and Tranvik, L. J. (2006). Antagonism between bacteria and fungi: substrate competition and a possible tradeoff between fungal growth and tolerance towards bacteria. Oikos 113, 233–242. doi: 10.1111/j.2006.0030-1299.14337.x
Morin-Sardin, S., Rigalma, K., Coroller, L., Jany, J. L., and Coton, E. (2016). Effect of temperature, pH, and water activity on Mucor spp. growth on synthetic medium, cheese analog and cheese. Food Microbiol. 56, 69–79. doi: 10.1016/j.fm.2015.11.019
Pelyuntha, W., Chaiyasut, C., Kantachote, D., and Sirilun, S. (2019). Cell-free supernatants from cultures of lactic acid bacteria isolated from fermented grape as biocontrol against Salmonella Typhi and Salmonella Typhimurium virulence via autoinducer-2 and biofilm interference. PeerJ 26:e7555. doi: 10.7717/peerj.7555
Pitt, J. I., and Hocking, A. D. (2009). Fungi and Food Spoilage, 3rd Edn. London: Springer, 519. doi: 10.1007/978-0-387-92207-2
Quattrini, M., Bernardi, C., Stuknytë, M., Masotti, F., Passera, A., Ricci, G., et al. (2018). Functional characterization of Lactobacillus plantarum ITEM 17215: a potential biocontrol agent of fungi with plant growth promoting traits, able to enhance the nutritional value of cereal products. Food Res. Int. 106, 936–944. doi: 10.1016/j.foodres.2018.01.074
Ropars, J., López-Villavicencio, M., Snirc, A., Lacoste, S., and Giraud, T. (2017). Blue cheese-making has shaped the population genetic structure of the mould Penicillium roqueforti. PLoS One 12:e0171387. doi: 10.1371/journal.pone.0171387
Russo, P., Arena, M. P., Fiocco, D., Capozzi, V., Drider, D., and Spano, G. (2017). Lactobacillus plantarum with broad antifungal activity: a promising approach to increase safety and shelf-life of cereal-based products. Int. J. Food Microbiol. 247, 48–54. doi: 10.1016/j.ijfoodmicro.2016.04.027
Salas, M. L., Thierry, A., Lemaître, M., Garric, G., Harel-Oger, M., Chatel, M., et al. (2018). Antifungal activity of lactic acid bacteria combinations in dairy mimicking models and their potential as bioprotective cultures in pilot scale applications. Front. Microbiol. 9:1787. doi: 10.3389/fmicb.2018.01787
Schnürer, J., and Magnusson, J. (2005). Antifungal lactic acid bacteria as biopreservatives. Trends Food Sci. Technol. 16, 70–78. doi: 10.1016/j.tifs.2004.02.014
Schwenninger, S. M., and Meile, L. (2004). A mixed culture of Propionibacterium jensenii and Lactobacillus paracasei subsp. paracasei inhibits food spoilage yeasts. Syst. Appl. Microbiol. 27, 229–237. doi: 10.1078/072320204322881853
Siedler, S., Rau, M. H., Bidstrup, S., Vento, J. M., Aunsbjerg, S. D., Bosma, E. F., et al. (2020). Competitive exclusion is a major bioprotective mechanism of lactobacilli against fungal spoilage in fermented milk products. Appl. Environ. Microbiol. 86, e2312–e2319. doi: 10.1128/AEM.02312-19
Zheng, J., Wittouck, S., Salvetti, E., Franz, C. M. A. P., Harris, H. M. B., Mattarelli, P., et al. (2020). A taxonomic note on the genus Lactobacillus: description of 23 novel genera, emended description of the genus Lactobacillus beijerinck 1901, and union of Lactobacillaceae and Leuconostocaceae. Int. J. Syst. Evol. Microbiol. 70, 2782–2858. doi: 10.1099/ijsem.0.004107
Keywords: bioprotection, spoilage molds, microbial interaction, antifungal activity, dairy products, manganese depletion
Citation: Shi C and Knøchel S (2021) Sensitivity of Molds From Spoiled Dairy Products Towards Bioprotective Lactic Acid Bacteria Cultures. Front. Microbiol. 12:631730. doi: 10.3389/fmicb.2021.631730
Received: 20 November 2020; Accepted: 22 January 2021;
Published: 10 February 2021.
Edited by:
Jörg Hummerjohann, Agroscope, SwitzerlandReviewed by:
Pasquale Russo, University of Foggia, ItalyFrancesca Valerio, Institute of Sciences of Food Production, Italian National Research Council, Italy
Copyright © 2021 Shi and Knøchel. This is an open-access article distributed under the terms of the Creative Commons Attribution License (CC BY). The use, distribution or reproduction in other forums is permitted, provided the original author(s) and the copyright owner(s) are credited and that the original publication in this journal is cited, in accordance with accepted academic practice. No use, distribution or reproduction is permitted which does not comply with these terms.
*Correspondence: Ce Shi, Y2VzaGlAZm9vZC5rdS5kaw==