- 1Institute for Veterinary Medical Research, Centre for Agricultural Research, Budapest, Hungary
- 2Institute of Hygiene, University of Münster, Münster, Germany
Introduction: Commensal and pathogenic strains of multidrug-resistant (MDR) Escherichia coli and non-typhoid strains of Salmonella represent a growing foodborne threat from foods of poultry origin. MDR strains of Salmonella Infantis and E. coli are frequently isolated from broiler chicks and the simultaneous presence of these two enteric bacterial species would potentially allow the exchange of mobile resistance determinants.
Objectives: In order to understand possible genomic relations and to obtain a first insight into the potential interplay of resistance genes between enteric bacteria, we compared genomic diversity and mobile resistomes of S. Infantis and E. coli from broiler sources.
Results: The core genome MLST analysis of 56 S. Infantis and 90 E. coli contemporary strains revealed a high genomic heterogeneity of broiler E. coli. It also allowed the first insight into the genomic diversity of the MDR clone B2 of S. Infantis, which is endemic in Hungary. We also identified new MDR lineages for S. Infantis (ST7081 and ST7082) and for E. coli (ST8702 and ST10088). Comparative analysis of antibiotic resistance genes and plasmid types revealed a relatively narrow interface between the mobile resistomes of E. coli and S. Infantis. The mobile resistance genes tet(A), aadA1, and sul1 were identified at an overall high prevalence in both species. This gene association is characteristic to the plasmid pSI54/04 of the epidemic clone B2 of S. Infantis. Simultaneous presence of these genes and of IncI plasmids of the same subtype in cohabitant caecal strains of E. coli and S. Infantis suggests an important role of these plasmid families in a possible interplay of resistance genes between S. Infantis and E. coli in broilers.
Conclusion: This is the first comparative genomic analysis of contemporary broiler strains of S. Infantis and E. coli. The diversity of mobile resistomes suggests that commensal E. coli could be potential reservoirs of resistance for S. Infantis, but so far only a few plasmid types and mobile resistance genes could be considered as potentially exchangeable between these two species. Among these, IncI1 plasmids could make the greatest contribution to the microevolution and genetic interaction between E. coli and S. Infantis.
Introduction
Reoccurring emergence of multidrug-resistant (MDR) strains of Salmonella enterica serovar Infantis is a global challenge for public health and food safety. Although it is accepted that the use of antibiotics in animal industry is one of the major driving forces of this process, there are several factors influencing development and emergence of MDR non-typhoidal Salmonella in food-producing animals. In most industrialised countries the poultry (primarily layer and broiler as well as fattening turkey) is the major source of foodborne salmonellosis. During the last two decades, MDR clones of S. Infantis have emerged and became established in the broiler industries of several countries in Europe (EFSA and ECDC, 2020), in Israel, Turkey, Japan and in the United States (Shahada et al., 2006; Gal-Mor et al., 2010; Tate et al., 2017; Acar et al., 2019), respectively.
In general, enteric bacteria such as Escherichia coli and Salmonella are readily responding to the frequent use of antibiotics by developing resistance. Due to their great genomic plasticity, E. coli strains can be more adaptive to the unfavourable antibiotic environment (Mathew et al., 2002). Part of this flexibility is the ability to acquire resistance determinants by horizontal gene transfer, and to develop new MDR lineages by vertical gene transfer ensuring survival and persistence. Based on this difference between Salmonella and E. coli, it is logical to assume that the resistome of E. coli in animals may serve as a reservoir for drug resistance determinants for Salmonella (Szmolka and Nagy, 2013). This could be especially true for the broiler industry where the high stocking density and the frequently necessitated antimicrobial treatments could fuel the mobilisation and uptake of resistance determinants within the intestinal E. coli population and between E. coli and Salmonella (Gyles, 2008). Indeed, some studies revealed in vivo or semi in vivo AMR gene transfers between Salmonella and E. coli in chicks (Oladeinde et al., 2019) and in turkey poults (Poppe et al., 2005) or in simulated intestinal environment of pigs (Blake et al., 2003) with or without simultaneous application of antibiotics.
Regarding the growing economic and public health importance of MDR Salmonella Infantis and the situation of MDR E. coli of broilers described above, it seems to be logical to ask how much the mobile resistomes of these two important MDR enterobacterial species overlap in real life as an indication of possible exchanges of resistance genes. So far, only a few studies compared the resistance phenotype and genotype of contemporary Salmonella spp. and E. coli isolates from poultry (Varga et al., 2019) and from broilers (Ahmed et al., 2009), but they have been mainly carried out on a phenotypic basis or have only investigated selected resistance genes of a relatively low number of strains.
Therefore, we performed a comparative resistome analysis at the whole-genome level of contemporary of S. Infantis isolates from humans and broilers and of commensal and extraintestinal pathogenic E. coli (ExPEC) strains isolated from broilers. With these studies, we aimed to gain a first insight into the possible interspecies exchange of mobile resistance determinants between E. coli and S. Infantis circulating in the broiler industry. Our findings indicated that certain plasmid types and resistance genes or gene cassettes could be considered in a possible genomic interaction between E. coli and S. Infantis.
Materials and Methods
Sampling of S. Infantis and E. coli Isolates
Whole-genome sequences of 56 S. Infantis and 90 E. coli isolates were analysed in this study. The collection was established in order to provide a genome-based comparison of antibiotic resistance in S. Infantis and E. coli from different broiler sources in Hungary.
Salmonella Infantis strains were mostly isolated from the faeces of broiler chickens (n = 31) and from human samples (n = 25) representing sporadic clinical cases (Supplementary Table 1). The majority of these strains (n = 19) were part of the basic collection of Szmolka et al. (2018) describing the molecular epidemiology of S. Infantis between 2011 and 2013 in Hungary. Additionally, we included 12 broiler strains of S. Infantis isolated between 2016 and 2018, the latter being of caecal origin (see next section).
Escherichia coli strains were isolated from the faeces, caecum and bone marrow of broiler chickens and day-old chicks (Supplementary Table 1). Commensal strains from faeces (n = 22) were isolated in 2013 from broiler chickens processed at three different slaughterhouses in North-Central Hungary. At slaughter 15 animals were randomly sampled at each slaughterhouse.
Caecal strains of E. coli (n = 43) were isolated in 2018 from broiler chickens and from day-old chicks. Caecal samples from broiler chickens were provided by the Veterinary Diagnostic Directorate, National Food Chain Safety Office (NÉBIH), as part of the national Salmonella Monitoring Programme (2018–2019). Here we tested 27 strains of E. coli isolated from the caecum of six broiler chickens, representing six different farms.
Escherichia coli isolates from the caecum of day-old chicks (IntEC) were provided by colleagues from private veterinary services in Hungary (2018–2019). These strains were isolated from the caecum of nine chick carcasses, representing seven chicken farms (Supplementary Table 1).
Extraintestinal pathogenic E. coli (n = 25) strains were isolated from the bone marrow of day-old chicks that died from E. coli septicaemia in two different periods. Old strains of ExPEC were isolated in Hungary between 1998 and 2000, and their virulence gene patterns were partly reported earlier (Tóth et al., 2012). Recent (2018) strains of ExPEC (2018) were isolated from the bone marrow of the same day-old chicks (n = 9) from which the above mentioned IntEC strains also derived. Both, the caecal- and the bone marrow samples of day-old chicks were used to isolate multiple IntEC and ExPEC strains (Supplementary Table 1), that were selected to represent antibiotic resistance phenotypes (see below). All S. Infantis and E. coli isolates were stored at −80°C in lysogeny broth (LB) (Becton Dickinson) containing 10% glycerol.
Isolation and Identification of Cohabitant Strains of S. Infantis and E. coli
Caecal samples of broiler chickens (n = 6) served as a common source for simultaneous isolation of S. Infantis and E. coli strains, that were designated here as cohabitant strains (Supplementary Table 1). Each caecal sample was used to isolate multiple strains of S. Infantis and E. coli, to represent the intra-community resistance diversity and to predict potentially exchangeable genes and plasmid types between the two species.
Detection of Salmonella in the caecum of broilers was performed according to the international Salmonella standard ISO 6579:2006 with little modifications. Briefly, 10 g caecal content was enriched in 100 ml LB broth for 18 h at 37°C. After incubation, 10 ml RV (Rappaport-Vassiliadis) selective enrichment broth was inoculated with 100 μl of the overnight LB culture, and incubated for 24 h at 42°C. Finally, 10 μl of the overnight RV culture was streaked onto XLD (Xylose Lysine Deoxycholate) agar plates (Becton Dickinson), and after 27 h of incubation (37°C) ten individual Salmonella-like colonies were randomly picked to represent each sample. The molecular confirmation of S. Infantis was performed by serovar-specific PCR as described by Kardos et al. (2007).
Cohabitant strains of E. coli were isolated from the overnight LB cultures of the Salmonella-positive caecal samples. For this, 10 μl of the LB culture was streaked onto Chromocult® Coliform agar plates (Merck). After overnight incubation (18 h, 37°C) ten individual E. coli-like colonies were picked randomly to represent each caecal sample. The molecular confirmation of all E. coli isolates was carried out by multiplex PCR on the basis of the simultaneous presence of the marker genes lacZ (beta-galactosidase) and uidA (beta-glucuronidase) (Bej et al., 1991).
Selection for Multiresistance and Determination of the Pulsotype
Collections of S. Infantis and E. coli were assembled based on the antibiotic resistance of the strains to represent the diversity of phenotypes identified for different sample sources. For S. Infantis, the pulsotype was also considered by the selection of strains to represent clonal diversity.
The resistance phenotype was determined by disc diffusion against nine antibiotic compounds that were selected in order to detect plasmid-mediated phenotypes conferred by genes of the mobile resistomes. For this, the following antibiotic compounds were used: ampicillin (AMP10), cefotaxime (CTX5), chloramphenicol (CHL30), ciprofloxacin (CIP5), gentamicin (GEN10), meropenem (MEM10) nalidixic acid (NAL30), sulphonamide compounds (SUL300), tetracycline (TET30) and trimethoprim (TMP5). Antibiotic susceptibility testing was performed according to the guidelines and interpretation criteria of the European Committee on Antimicrobial Susceptibility Testing (EUCAST, 2018). E. coli ATCC 25922 was used as a reference strain. The intermediate category was interpreted as susceptible, while multiresistance was defined as simultaneous resistance against at least three antibiotic classes.
Pulsed-field gel electrophoresis (PFGE) of S. Infantis strains was carried out according to the CDC Pulse Net standardised Salmonella protocol using S. Braenderup H9812 as a molecular standard. XbaI restriction profiles were analysed by the Fingerprinting II Software (Bio-Rad Laboratories, Ventura, CA, United States). Cluster analysis was performed by the un-weighted pair-group method (UPGMA) with arithmetic means. Clonal distances were calculated on the basis of the Dice’s coefficient. A 1.0% position tolerance and 1.5% optimisation setting were applied.
DNA Extraction and Whole-Genome Sequencing
Total genomic DNA was isolated using the MagAttract® HMW DNA kit (Qiagen, Hilden, Germany). To prepare 500 bp paired-end libraries of all isolates we used the Nextera XT DNA Library Preparation kit (Illumina, San Diego, CA, United States). Libraries were sequenced on the Illumina MiSeq sequencing platform using v2 sequencing chemistry. The quality of the raw sequencing data was then analysed using FastQC v0.11.5 (Andrews, 2010). Raw reads were trimmed using Sickle v1.331. Genome assembly of the processed reads was carried out with SPAdes v3.10.1 (Bankevich et al., 2012). The genome sequence reads for all strains included into this study were combined in Bioproject number PRJNA694299 and are publicly available in the NCBI Sequence Read Archive (SRA). The accession numbers of the genome sequence reads of the individual isolates are also indicated in Supplementary Table 1.
In silico Genome Analysis
The Salmonella serovar identity was confirmed in silico on the basis of contig sequences by using the web-based application SeqSero v1.2 (Zhang et al., 2015). The S. Infantis-specific antigenic profile 7:r:1,5 (O:H1:H2) was thereby predicted for all the 56 Salmonella strains, perfectly corresponding to the Infantis-specific PCR. The E. coli serotype was determined by SerotypeFinder v.1.1 (Joensen et al., 2015) by using threshold values: identity 90%; minimum length 80%. The E. coli phylogroup was predicted by the in silico Clermont Phylotyper (Waters et al., 2018) for the differentiation of the seven phylogroups (A, B1, B2, C, D, E and F).
For molecular epidemiological analysis of S. Infantis and E. coli strains the Ridom SeqSphere+ software was used (Jünemann et al., 2013). Sequence types (STs) were determined by multilocus sequence typing (MLST) based on the polymorphism of the seven housekeeping genes according to the Warwick MLST scheme for E. coli (Wirth et al., 2006) and to the Achtman MLST scheme for S. Infantis (Achtman et al., 2012). Strains with unknown allelic profiles were submitted to Enterobase (Zhou et al., 2019) for the confirmation and identification of the new STs. The relatedness of S. Infantis and E. coli strains was further analysed by core genome (cg)MLST. The genome of the earliest sequenced S. Infantis strain 1326/28 (GenBank accession no. LN649235) was used as reference for the cgMLST of S. Infantis, while core genes of E. coli were analysed by blasting all genome sequences against the E. coli reference strain K-12 MG1655 (GenBank accession no. NC_000913).
Web-based tools ResFinder v.3.0 (Zankari et al., 2012) and PlasmidFinder v.2.0 (Carattoli et al., 2014) were used for the in silico detection of acquired antibiotic resistance genes and for typing of plasmids on the basis of the replicon type. Thresholds for the prediction of resistance genes were set to a minimum of 90% sequence identity and a minimum of 80% length coverage, while plasmid replicon types were detected at a minimum of 95% sequence identity and at a minimum of 80% length coverage. Cluster analysis based on the resistance genes was performed by using the PAST software v.4 (Hammer et al., 2001). The Kruskal–Wallis test was performed to statistically support results of multiple group comparisons, by using the OriginPro 2021 software (OriginLab Corporation). Differences were considered to be significant at the 0.05 level.
In order to reveal whether certain clones are associated with an increased prevalence of resistance genes the Multiple Antibiotic Resistance (MAR) index was calculated for all E. coli STs. The MAR index is the ratio between the total number of resistance genes, number of antibiotics tested and number of isolates (Krumperman, 1983). A higher MAR index indicates a greater abundance of resistance genes.
Results
Diversity of Serotypes, STs and Phylogroups of E. coli and of S. Infantis From Broilers and Humans
The SerotypeFinder predicted a large diversity of serotypes among the tested E. coli strains. Strains were assigned to 40 different O-types, out of which O8 and O9 were most frequently identified in eight and seven strains, respectively. The O-antigen-associated genes wzx/wzy were not typable (nt) for additional eight strains (Supplementary Table 2). Similar to the O-serotype determinants, the H-type gene (fliC) was also variable, resulting in the identification of 31 H-types in the E. coli collection. The in silico phylogroup prediction resulted in the identification of predominant phylogroups A and B1 in 27 and 37% of the strains, respectively, most of them being isolated from the caecum. E. coli serotypes were compared to the phylogroup and the ST of the strains with regard to the source of isolation. In general, there was no strong correlation found between serotypes and phylogroups or STs of these E. coli strains. Serogroups O78, O88 and O115 showed a tendency for extraintestinal (bone marrow) isolates designated as ExPEC (Supplementary Table 2).
In order to compare the diversity of the isolated E. coli and S. Infantis strains, the STs of 90 E. coli and of 56 S. Infantis strains were determined by MLST on the basis of whole genome sequences.
The E. coli strains were allocated to 49 STs (Figure 1). The majority of them represented individual STs with one isolate each. STs containing at least four strains were regarded here as large STs. Commensal and extraintestinal strains were both grouped in larger STs such as ST10, ST93, ST117 and ST162, while ST155 contained commensal (faecal and caecal) strains only. The new E. coli STs ST8702 and ST10088 representing caecal isolates belong to the clonal complexes (CCs) CC10 and CC155, respectively (Supplementary Table 1 and Figure 1).
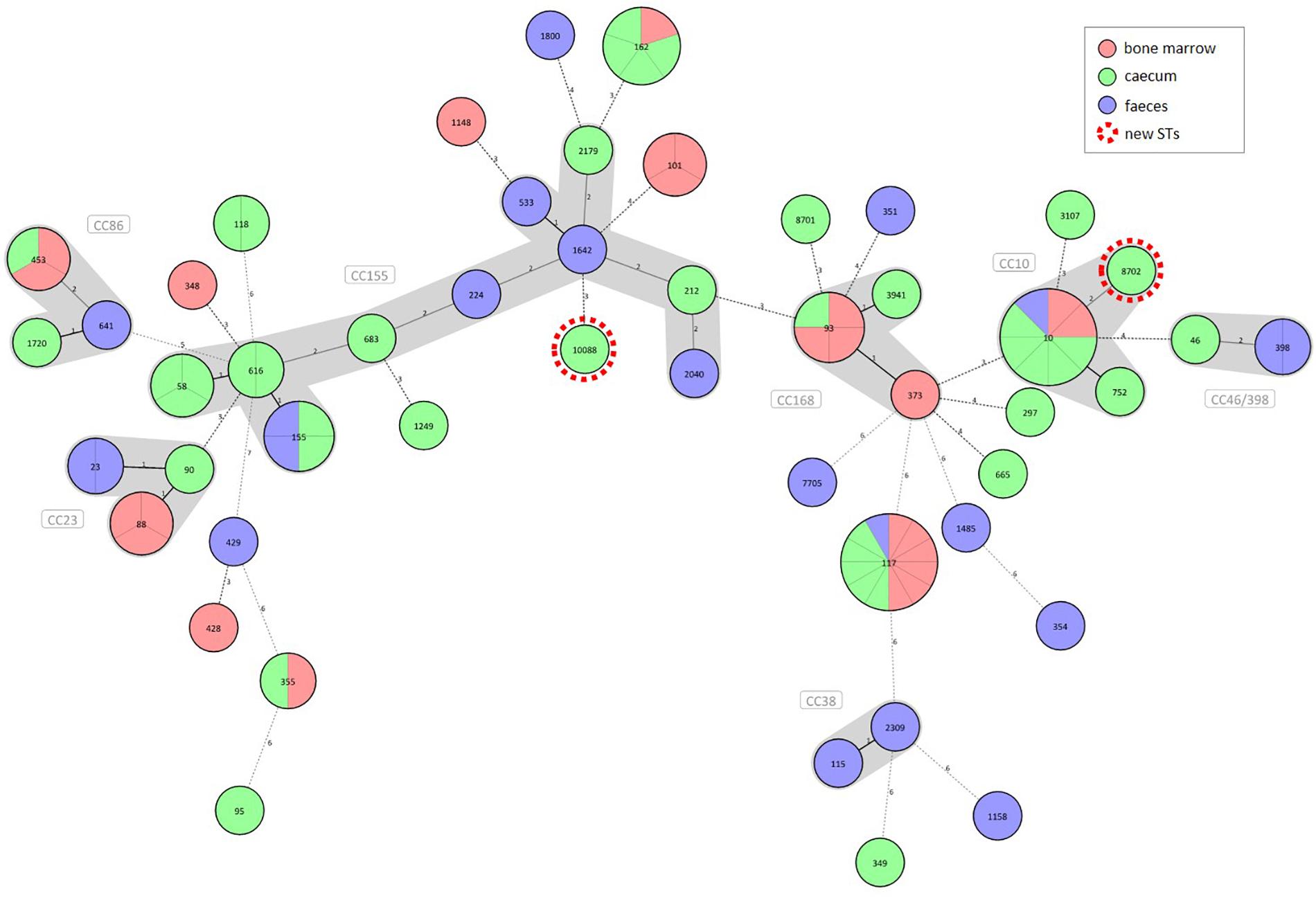
Figure 1. Diversity of commensal (caecal and faecal) and extraintestinal (bone marrow) E. coli strains from broilers according to the sample source. A Minimum Spanning Tree of 90 commensal and clinical E. coli strains was generated based on the polymorphism of seven housekeeping genes. Strains are separated by grey lines in nodes with multiple strains. Allelic patterns with missing values represented a category of its own and were designated as novel clones highlighted with red dotted circles. Distance lines change from black to dotted grey as the phylogenetic distance between the strains increases. Maximum distance in cluster was set to 2, making it therefore possible to group STs into clonal complexes (CCs). The thickness of the distance line is inversely proportional to the distance value.
In contrast to E. coli, most S. Infantis strains were assigned to ST32. Exceptions were two strains for which ST7081 (from human) and ST7082 (from broiler) were identified as new Salmonella spp. STs (Supplementary Table 1).
Genomic Diversity of E. coli and S. Infantis Strains From Broilers
To reveal genomic diversity of broiler strains of E. coli, cgMLST was carried out based on the polymorphism of 2398 genes of the core genome, by using the strain E. coli K-12 MG1655 as a reference. The core genome-tree showed that E. coli genomes are grouped into three main clusters according to their phylogenetic background (Figure 2). Cluster 1 and Cluster 2 represent the dominant phylogroups A and B1 (27.8 and 37.8%), while most strains of Cluster 3 were assigned to phylogroup F (14.4%). Closely related strains from the large STs ST10, ST93 (Cluster 1) and ST155, ST162 (Cluster 2) showed a high level of genomic diversity on the basis of the core genome comparison. Commensal (intestinal) and extraintestinal (clinical) E. coli strains of ST117 displayed the most homogenous core genome sequences (Figure 2). ST10 and ST93 belong to phylogroup A, ST117 to phylogroup F, while ST155 and ST162 belong to phylogroup B1 (Figure 2).
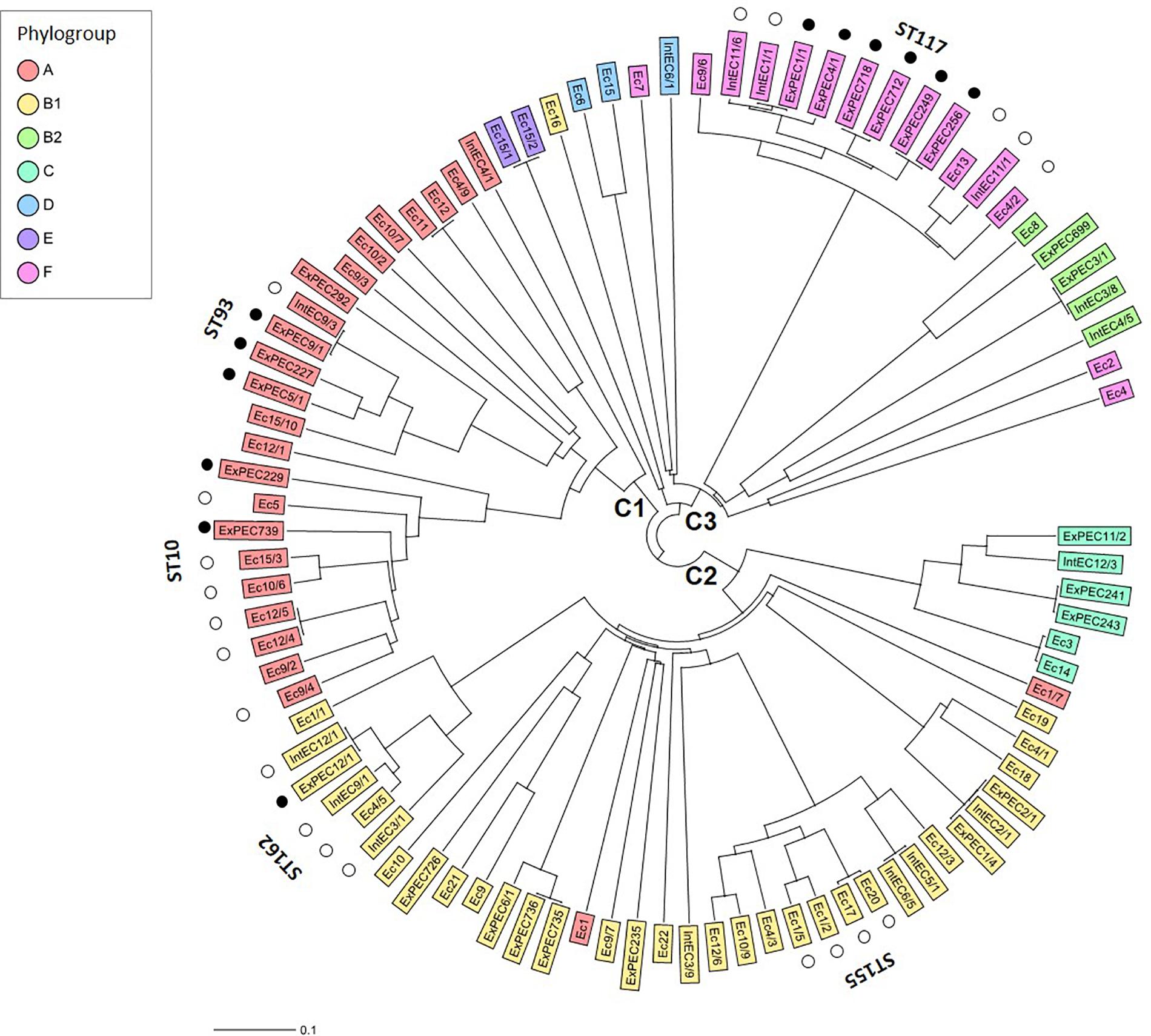
Figure 2. Core genome diversity and phylogroups of commensal (caecal and faecal) and extraintestinal (bone marrow) strains of E. coli from broilers. The Neighbour Joining Tree showing the genomic diversity of 90 E. coli strains was calculated based on the polymorphism of 2398 target genes of the core genome. Core genes were identified by blasting all genome sequences against the reference strain E. coli K-12 MG1655 (GenBank accession no. NC_000913). By distance calculation, gene columns with missing values were removed. Only the large STs of E. coli with at least four isolates are indicated. Black and white dots indicate extraintestinal and commensal (caecal and faecal) strains, respectively, representing larger STs. C1-3 describes Clusters 1–3.
For S. Infantis, the core genome analysis was performed to reveal the genomic diversity within the PFGE clones (Figure 3), with special regard to the epidemic PFGE clone B2 (Supplementary Table 1). The cgMLST was based on the sequence polymorphism of 3850 core genome targets by using the strain S. Infantis 1326/28 as a reference. Core genomes of 56 S. Infantis strains were analysed in comparison with the S. Infantis strains SI69/94 and SI54/04 representing the ancient, pansensitive strains of PFGE clone (pulsotype) A and of the emergent epidemic multiresistant PFGE clone B2, respectively.
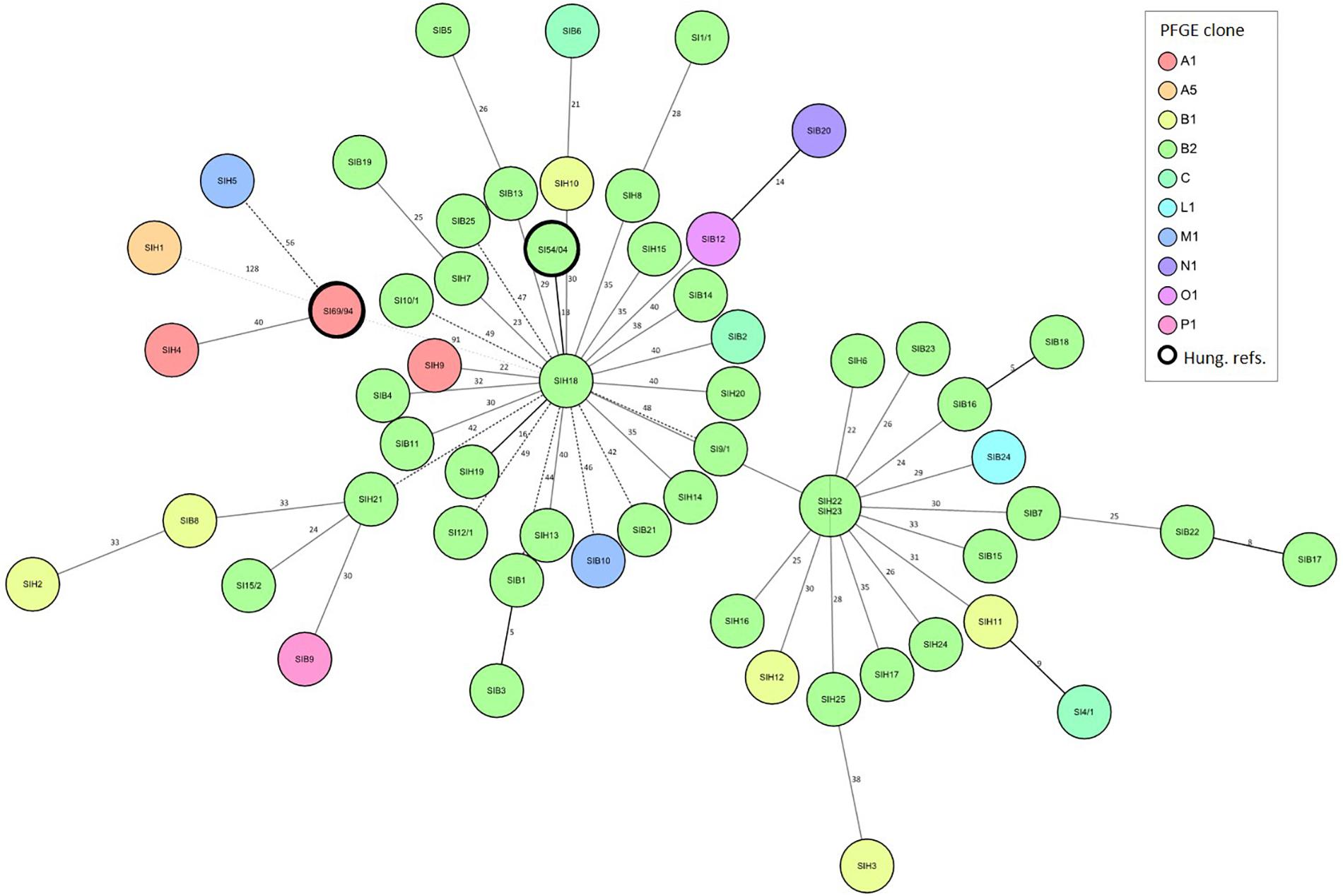
Figure 3. Relation between the genomic diversity and pulsotype in broiler and human strains of S. Infantis. Minimum Spanning Tree showing the core genome diversity of S. Infantis strains was generated based on the polymorphism of 3647 target genes of the core genome. By distance calculation, gene columns with missing values were removed. Distances are shown by the line style, distance numbers are also indicated. Distance lines change from black to dotted grey as the phylogenetic distance between the strains increases. Logarithmic scale was used for distance line length calculation. Strains are separated by grey lines in nodes with multiple strains. Besides the 56 S. Infantis strains studied here, the whole-genome sequences of S. Infantis strains SI69/94 (GenBank accession no. JRXB00000000) and SI54/04 (GenBank accession no. JRXC00000000) were also included, and blasted against the reference strain S. Infantis 1326/28 (GenBank accession no. LN649235). Both of these S. Infantis strains (framed by thick black line) were included as a Hungarian references for the ancient, pansensitive strains of the late 1990s (SI69/94) and for strains representing the emergent endemic MDR clone B2 (SI54/04) (Olasz et al., 2015).
The cgMLST analysis showed a high level of genomic diversity within the epidemic clone B2 of S. Infantis (Figure 3). There were no relations between cgMLST and pulsotype. The S. Infantis strains grouped in two large clusters comprising strains from broilers and humans, and centred around the human strains SIH22/SIH23 or SIH18. This latter cluster contained the Hungarian MDR reference strain SI54/04. Core genomes of tested strains of S. Infantis strongly differed from that of the ancient reference strain SI69/94 (PFGE clone A). Exceptions were the human strains SIH1, SIH4 and SIH5 that clustered together with this ancient, pansensitive strain SI69/94, and one of them also represented the PFGE clone A.
The Mobile Resistome of E. coli and S. Infantis: Differences and Overlaps
To describe the diversity and distribution of acquired resistance genes and of plasmid types, the genome sequences of the tested E. coli and S. Infantis strains were analysed by the web-based programmes ResFinder and PlasmidFinder. The in silico analysis showed that mobile resistomes of E. coli and S. Infantis strains were comparable on the basis of 34 resistance genes (17 gene families) and of 14 plasmid types (Table 1 and Figure 4).
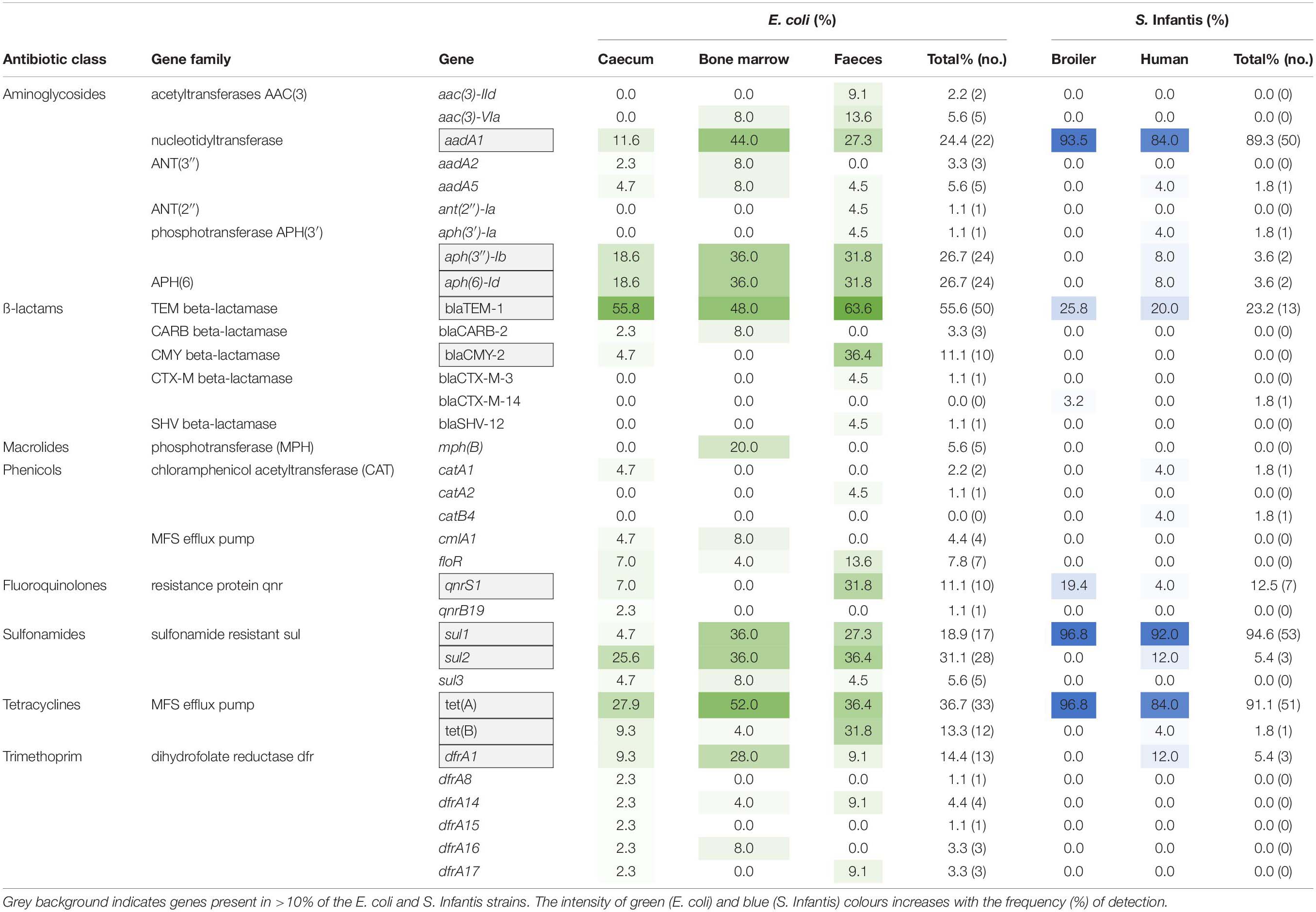
Table 1. Diversity and distribution of resistance genes of the mobile resistomes in S. Infantis and E. coli strains isolated from broilers and humans.
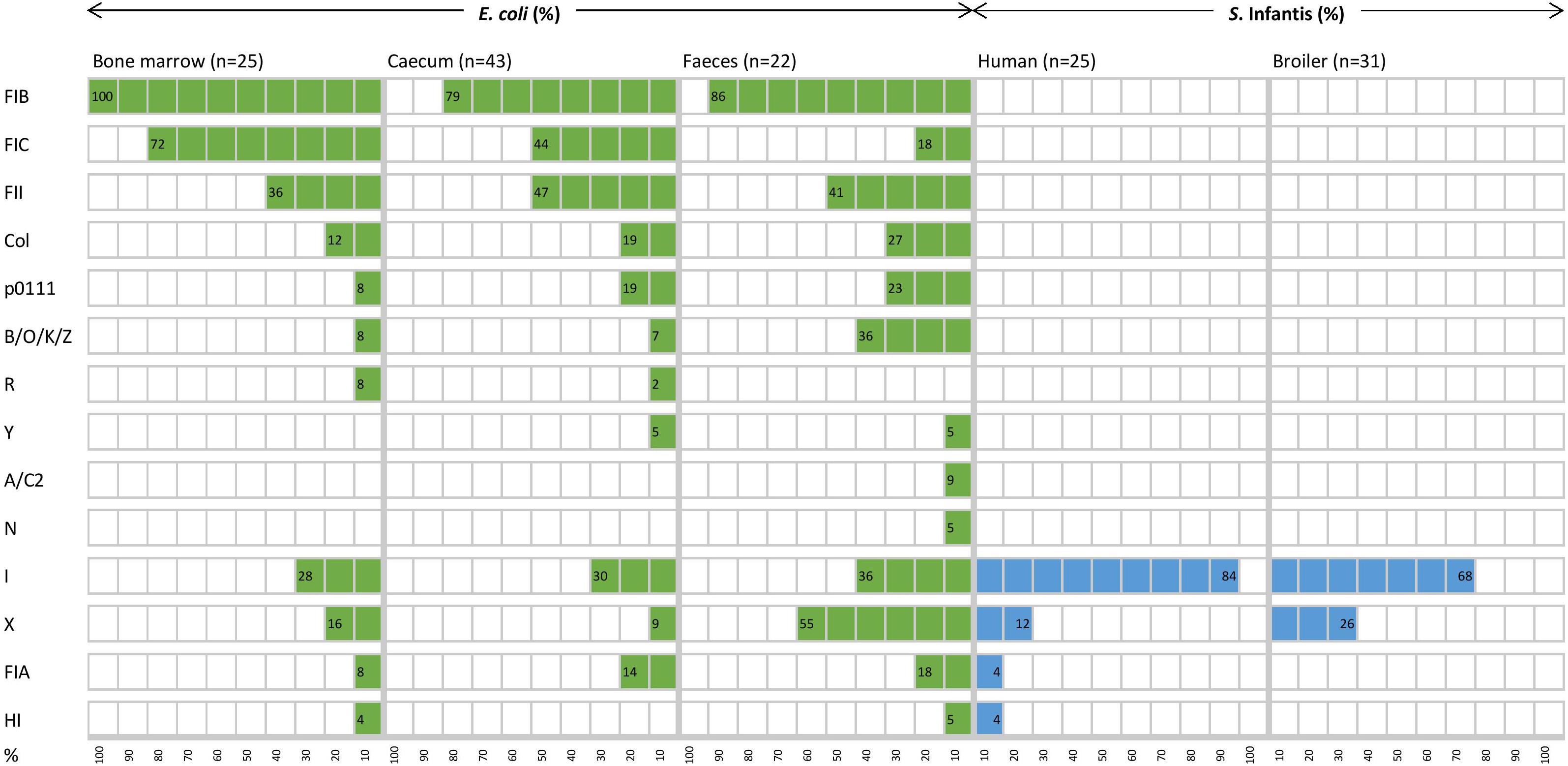
Figure 4. Diversity and distribution (%) of plasmid replicon types of E. coli and S. Infantis strains from different host sources.
The mobile resistome analysis of E. coli showed a large diversity of acquired resistance genes and plasmid types in the majority of E. coli strains. All of the 34 resistance genes were identified in the E. coli strains (Table 1). Eleven of these genes were detected in more than 10% of the E. coli strains, conferring resistance to aminoglycosides, β-lactams, fluoroquinolone, sulphonamides, tetracyclines and trimethoprim. The distribution of resistance genes was not related to the sample type, the overall prevalence of resistance genes did not differ significantly between sample sites (Table 1).
The ampicillin resistance gene blaTEM–1 and the tetracycline resistance gene tet(A) were most frequently detected, with a prevalence of 48.0–63.6% and 36.4–52.0% according to the source of isolation (Table 1). Extraintestinal strains (from bone marrow) demonstrated the highest frequency of genes aadA1 (44.4%), sul1 (36.0%) and dfrA1 (28.0%) related to class 1 integrons. Besides, the macrolide phosphotransferase gene mph(B) was also detected exclusively in five extraintestinal E. coli strains. Only commensal (especially the faecal) strains of E. coli carried genes related to emerging plasmids such as qnrS1 (31.8%) and blaCMY–2 (36.4%). The latter was identified only in E. coli strains. In comparison with S. Infantis, E. coli strains were also abundantly carrying genes coding for aminoglycoside-, sulphonamide- and tetracycline resistances. In this context, the aph(3″)-Ib, aph(6)-Id, sul2 and tet(B) genes should be mentioned as characterising 13.3–26.7% of the E. coli strains, but being carried by only a few S. Infantis strains (Table 1).
In contrast, broiler and human strains of S. Infantis carried a more reduced set of resistance genes and plasmid types (Table 1 and Figure 4). A total of 15 resistance genes were identified in the collection representing ten gene families and five of these genes were detected in more than 10% of the S. Infantis strains, conferring resistance to aminoglycosides, β-lactams, fluoroquinolone, sulphonamides and tetracyclines (Table 1). The diversity of resistance genes was higher in human strains than in broiler strains. The tetracycline resistance gene tet(A) and the class 1 integron genes aadA1 and sul1 were predominantly identified (89.3–91.1%) in S. Infantis strains regardless of their host. These genes were also frequently identified in E. coli but at a considerably lower prevalence (Table 1). Additional resistance genes detected in E. coli as well as in S. Infantis were the ampicillin- and fluoroquinolone resistance genes blaTEM–1 (23.2%) and qnrS1 (12.5%), respectively. The prevalence of blaTEM–1 was much higher in E. coli than in S. Infantis, while qnrS1 gene was detected more frequently in broiler strains than in human strains of S. Infantis. The emerging CTX-M type resistance genes were also present in both species. According to this, the blaCTX–M–14 gene was identified in one broiler faecal strain of S. Infantis, while blaCTX–M–3 was detected in one faecal E. coli strain (Table 1).
Consistent with the high diversity of resistance genes, the coexistence of multiple plasmids was predicted for the majority of E. coli strains. Most plasmids belonged to the F replicon type, and plasmid diversity was not significantly higher in commensal strains than in extraintestinal ones (Figure 4). Because at least two different plasmid types were detected in almost all strains, no correlation could be established between certain resistance genes and plasmid types, except for one strain which carried the blaTEM–1 gene on an IncI1 plasmid (Figure 5).
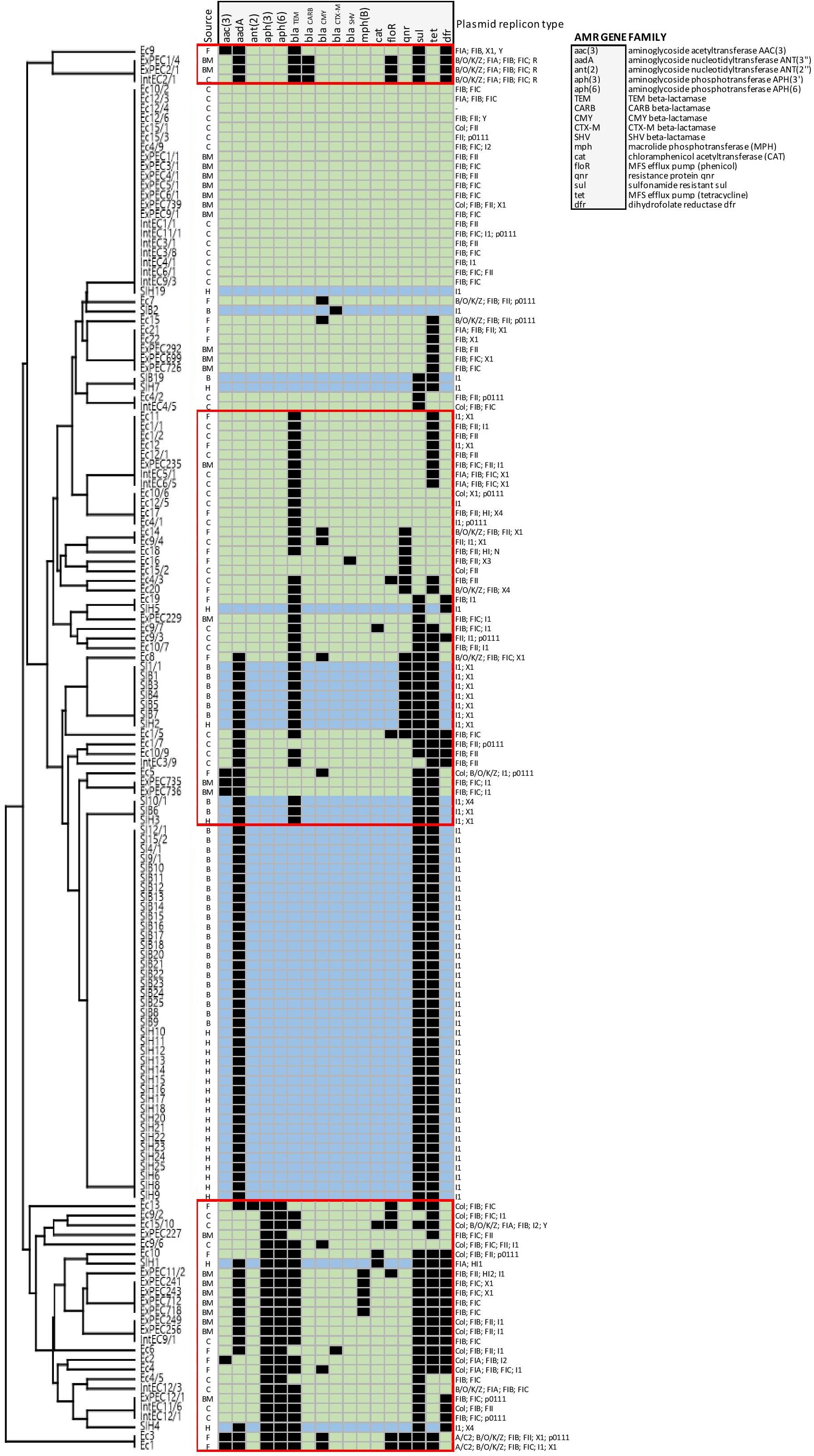
Figure 5. Mobile resistome tree of S. Infantis isolated from broilers and humans and of E. coli from broilers. E. coli strains are coloured in light green, while light blue represents S. Infantis strains. Abbreviations for the source of isolation are: F, faeces; BM, bone marrow; C, caecum; B, broiler; and H, human. The identified E. coli clusters are framed by red lines.
In contrast to E. coli, most S. Infantis strains were identified as monoplasmidic, and the coexistence of two replicon types was predicted for only two strains from humans. The MDR plasmid pSI54/04 of S. Infantis dominant in Hungary (Szmolka et al., 2018) was identified with the replicon type I1, similar to the CTX-M-14 plasmid carried by a broiler S. Infantis isolate. Despite of the above mentioned remarkable differences regarding the plasmid diversity of the tested E. coli and S. Infantis strains, plasmids with replicon families IncI and IncX were most commonly detected in both species (Figure 4).
We also wanted to find out if increased resistance was associated with certain E. coli STs. As a result, we determined that altogether 10 STs (including up to three strains each) proved to comprise potential carriers of multiresistance (MAR index >0.3) (Supplementary Figure 1).
Relations Between Resistance Genes and Plasmids of E. coli and S. Infantis and Comparison of Their Cohabitant Strains
To obtain deeper insight into resistance relationships in E. coli and S. Infantis, the strains were grouped according to the pattern of acquired resistance genes. This cluster analysis showed that the composition of the E. coli resistomes was very heterogenous, even with the association of up to 10 resistance genes in some of the strains (Figure 5). According to the resistance phenotype, MDR strains of E. coli were characterised by several combinations of associated resistance genes. Based on these constellations, E. coli strains were grouped into 3 well-separable clusters: (i) cluster CARB characterised by the coexistence of genes blaCARB–2 – blaTEM–1, (ii) cluster TEM/TET with the associated genotype of blaTEM–1 – tet(A) – qnrS and (iii) cluster APH, that could be regarded as a “super-MDR clade” as it is characterised by multiple combinations of associated genes around the aph(3″)-Ib and aph(6)-Id genes. Genes related to class 1 integrons were commonly detected in all of these three clusters (Figure 5).
In contrast, the composition of the of S. Infantis resistomes was quite homogenous, with the association of maximum of five genes in the majority of strains. Most S. Infantis isolates from broilers and humans grouped into a large cluster designated here as cluster TET, characterised by the MDR genotype tet(A)-aadA1-sul1. Some strains isolated from broilers were grouped into the clade TEM/TET identified for E. coli. Accordingly, these strains carried the blaTEM–1 – qnrS1 genes in addition to the tet(A)-aadA1-sul1 genes. The simultaneous presence of six to eight resistance genes was detected in only two human S. Infantis isolates, both of them were grouped in the above mentioned “super-MDR clade” identified for E. coli (Figure 5).
For the prediction of potentially exchangeable resistance genes/plasmids the comparison of the mobile resistomes of cohabitant E. coli and S. Infantis strains was performed. Cohabitant strains of E. coli and S. Infantis were isolated from the same samples, more exactly from the caecum of six broiler chickens representing six different farms (Figure 6). From each caecal sample it was possible to isolate one MDR strain of S. Infantis together with at least four cohabitant E. coli strains with different resistance phenotypes. The comparative resistome analysis of the cohabitant strains showed that the class 1 integron genes aadA-sul1 and the resistance plasmid genes tet(A), blaTEM–1 and qnrS1 can be potentially exchangeable between E. coli and S. Infantis. Furthermore, certain plasmids, especially of IncI1 type could be involved in the interaction between these two species (Figure 6). To address the question whether the same plasmids can be found in cohabitant S. Infantis and E. coli strains, we compared the sequence identity of plasmid contigs which include the aadA1, sul1, tet(A), blaTEM–1 or qnrS1 genes. The multiple sequence alignment shows that the plasmid contigs of cohabitant S. Infantis and E. coli strains are not identical (Supplementary Figure 2). Future comparative studies including a high number of cohabitant strains will be addressed to reveal plasmid sequence similarities between E. coli and S. Infantis.
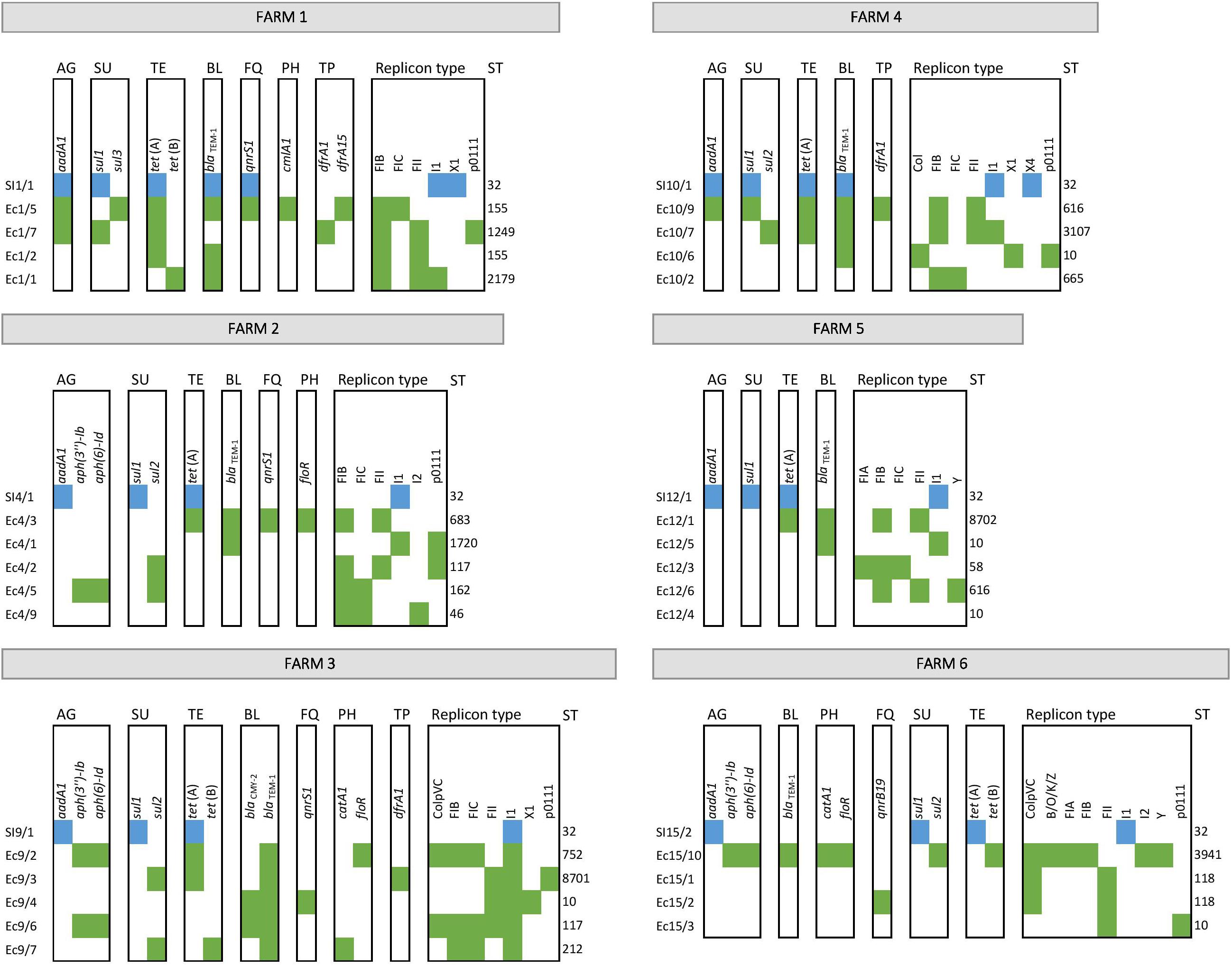
Figure 6. Antibiotic resistance genes as potentially exchangeable between cohabitant strains of E. coli and S. Infantis from caecal samples of broilers. S. Infantis strains are coloured in blue, while green represents E. coli strains. Abbreviations for antibiotic resistances are: AG, aminoglycosides; SU, sulfonamides; TE, tetracyclines; BL, beta-lactams; FQ, fluoroquinolones; PH, phenicols; and TP, trimethoprim.
Discussion
In the last decade the multiresistance of S. Infantis has grown into a global problem of the broiler industry. MDR strains of S. Infantis are frequently co-isolated with MDR E. coli in broilers, which increases the food safety risk of chicken meat and contributes to decreasing its consumer value and market potential.
The simultaneous presence of both bacterial species may allow an active intraspecies and intergeneric interplay of mobile resistance elements between S. Infantis and E. coli in broilers. Here we provided a genome-based comparison of mobile resistomes and genomic diversity of S. Infantis and E. coli isolates in order to gather new information about relations between these two cohabitant MDR enterobacteria of food safety importance. The core genome-based analysis identified a high genomic heterogeneity of broiler E. coli strains.
This high-resolution analysis allowed the first insight into the relatedness of S. Infantis isolates of PFGE clone B2, which is epidemic in Hungary among broilers and humans. At the same time, we were able to provide an overview on emerging lineages and mobile resistance determinants characterising populations of E. coli and S. Infantis in Hungarian broiler flocks. By the comparative analysis of mobile resistomes in cohabitant strains, the first snapshot was taken on a possible intergeneric interaction and resistance gene exchange between E. coli and S. Infantis in the caecum of chickens.
Genomic diversity of E. coli was not related to the source of isolation. Strains from both, caecum and bone marrow, displayed a high diversity in STs and serotypes. None of the E. coli STs represented MDR high-risk lineages, such as ST131 known to cause extraintestinal infection of humans, but also colonising several animal hosts (Mathers et al., 2015; Yamaji et al., 2018b). ST10, ST93, ST117 and ST162 were identified here as larger closely related groups, comprising both intestinal and extraintestinal strains, while ST155 contained intestinal strains only. ST10 is known as one of the most widespread MDR lineages of E. coli from animals (Liu et al., 2018), and can also be isolated from urine of patients suffering from urinary tract infection (Yamaji et al., 2018a). ST93 was described among others to include ESBL-/ and mcr-1 expressing E. coli isolates from foods of animal origin (Zhang et al., 2019). Similarly, mcr-1 positive E. coli strains of ST162 were isolated from food in China (Zhang et al., 2019) and from pigs in Mexico (Furlan et al., 2019). Furthermore, ST117 and ST155 have also been recently reported to include many human pathogenic and MDR E. coli strains with zoonotic potential (Alonso et al., 2017; Yang et al., 2017). ST117 was also described as the primary causative agent of cellulitis in poultry in Denmark (Poulsen et al., 2018). Here, we identified two new MDR clones, i.e. ST8702 and ST10088, of the CCs CC10 and CC155, respectively. The above mentioned ST117 and ST155 could not be considered as reservoirs for genetic determinants of high priority resistance.
Regarding the O-type diversity, we most frequently identified O8, O9 and O78 in E. coli strains from broilers. From among these, the O78 group is widely recognised for ExPEC in chickens and man (Schouler et al., 2012; Tóth et al., 2012) and as such, may constitute a zoonotic risk (Moulin-Schouleur et al., 2007; Huja et al., 2015). Moreover, it seems that avian E. coli O78:H4/ST117 detected here in the bone marrow and in caecal contents could represent an epidemic lineage, as several reports indicate that O78:H4 is an epidemiologically significant serotype of avian ExPEC representing the ST117 (Ronco et al., 2017; Poulsen et al., 2018). Although, E. coli strains of serogroup O8 also seem to be implicated in avian colibacillosis (Gross, 1991), much less is known about extraintestinal pathogenicity of this group of E. coli isolates in the avian host (Schouler et al., 2012). This is in good agreement with our finding that only one of the eight MDR E. coli O8 strains was derived from the bone marrow. There seem to be no data available on avian E. coli O9 strains, which were all caecal (commensal) isolates in our study. The presence of these three O serogroups (especially of O78) will justify an in depth analysis of virulence genes of these E. coli isolates, which should be the subject of further genomic analysis in the near future.
It could also be stated that strains from the large STs such as ST10, ST93 (Cluster 1) and ST155, ST162 (Cluster 2) displayed a high level of genomic diversity on the basis of the core genome comparison, but E. coli ST117 strains were the most homogenous in their genome content (Cluster 3). Finally, it should also be mentioned that ExPEC strains were relatively most frequently grouped to ST117 in Cluster 3 (6 of 14 such strains: 42.8%) in line with previous data on avian pathogenic E. coli of ST117 (Alonso et al., 2017; Yang et al., 2017; Poulsen et al., 2018). This contrasts with the lower proportional occurrence of ExPEC among phylogroup A strains of Cluster 1 (6 of 23 such strains: 26.1%) and of ExPEC among phylogroup B1 strains of Cluster 2 (8 of 33 such strains: 24.2%).
In contrast to the heterogeneity of E. coli described above, strains of S. Infantis have shown a very homogenous clonal structure by almost all being assigned to ST32, which is considered the predominant ST of this serovar worldwide. However, it appears that S. Infantis is genetically “open” for a clonal diversification, as indicated by the emergence of MDR STs such as the ST2283, which has recently spread in the broiler population in Germany (García-Soto et al., 2020). Our data also contribute to the global clonal diversity of S. Infantis through the identification of two new MDR STs ST7081 and ST7082 for a human and a broiler strain, respectively.
The predominant prevalence of the MDR genotype of tet(A)-aadA1-sul1 indicates the constant circulation of the dominant PFGE clone B2 and its MDR plasmid in Hungary since its emergence two decades ago (Nógrády et al., 2007; Szmolka et al., 2018). By using the high discriminatory cgMLST genotyping tool, we revealed for the first time the internal genomic structure of the epidemic PFGE clone B2, showing two main clusters each with a high genomic plasticity and potential for clonal diversification. This finding is in line with other reports, stating that the S. Infantis population is heterogeneous at the genomic level, with a more homogeneous repertoire of MDR plasmids, and with a potential to reflect geographical differences (Gymoese et al., 2019; Alba et al., 2020; Nagy et al., 2020).
Our results from comparative mobile resistome analysis describes avian E. coli strains as genetically diverse with a high prevalence and diversity of plasmid types and of mobile resistance genes, while S. Infantis strains proved to be much poorer in antibiotic resistance determinants and in different plasmid types. Such a discrepancy between these two bacterial populations is reflecting the genetic differences between these two species that may be explained by their divergent evolution about 100–150 million years ago (Ochman and Wilson, 1987). Besides, E. coli is a genetically extremely flexible long-term intestinal coloniser and member of the normal intestinal microbiota, perfectly adapted to changing conditions in the intestinal tract, while S. Infantis is a rather transient invader in the gut, having to compete with the resident microbiota of chicks.
We identified plasmid replicon types in the S. Infantis and E. coli isolates to get an overview of the interspecies distribution of plasmid types without the need to assign individual resistance genes to plasmid types. From all the plasmid types detected in our study, IncF plasmids represented the most striking difference between E. coli and S. Infantis. IncF plasmids are restricted to Enterobacteriaceae and are associated with virulence in pathogenic E. coli and Salmonella (Johnson and Nolan, 2009; McMillan et al., 2020). They are also well-known as MDR plasmids. Moreover, the emergence of some dominant E. coli STs has been driven by extended-spectrum β-lactamases (ESBL) plasmids of the IncF family (Villa et al., 2010; Carattoli, 2013; Dunn et al., 2019). We detected this replicon family almost exclusively in E. coli strains with the exception of one human S. Infantis isolate, suggesting that the prevalence of this plasmid type is greatly determined by the bacterial host species. The IncF plasmid family has rarely been reported in Salmonella, e.g. the spvB virulence plasmid of S. Typhimurium belongs to the IncF plasmid family (Oluwadare et al., 2020). IncI1 plasmids, however, were predominantly identified in S. Infantis, but this plasmid family was also frequently detected in E coli strains.
For both species the mobile resistance genes tet(A), aadA1 and sul1 were identified with an overall high prevalence, conferring resistance to classical antibiotic classes such as tetracyclines, aminoglycosides and sulphonamides, while the blaTEM–1 gene encoding ampicillin resistance was detected mostly in E. coli regardless of the sample source. These findings on the most frequently identified resistance genes fully support the latest EU report on the prevalence of antibiotic resistance phenotypes in poultry (EFSA and ECDC, 2020). The abundance of these genes and of the corresponding MDR genotypes is not surprising, as a number of compounds belonging to these antibiotic classes are approved and largely used in some EU countries for disease prevention in broilers (Roth et al., 2019). Thus, the coexistence of genes blaTEM–1, tet(A), aadA1 and sul1 is not something particularly noteworthy for E. coli, and the combination of these associations is increasingly considered as a common feature of both commensal and pathogenic strains (Szmolka and Nagy, 2013; Vila et al., 2016). But for S. Infantis, the tet(A)-aadA1-sul1 genotype indicated the emergence of a new epidemic MDR clone (pulsotype B2) of S. Infantis, that was first reported in Hungary (Nógrády et al., 2007, 2012) and subsequently in other countries (Franco et al., 2015; Hindermann et al., 2017).
The epidemiological success and persistence of the clone B2 still seems to be unbroken, as we predominantly identified the MDR genotype tet(A)-aadA1-sul1 among broiler and human strains of S. Infantis. This is in line with our previous report, in which we traced the molecular epidemiology of Hungarian strains of S. Infantis between 2011 and 2013 (Szmolka et al., 2018). The coexistence of these mobile resistance marker genes and of the specific virulence genes identified previously (Szmolka et al., 2018) indicated the indisputable presence of the plasmid pSI54/04 in most of the S. Infantis strains. Plasmid pSI54/04 was first defined as an IncP plasmid by the PCR-based replicon typing system developed by Carattoli et al. (2005), however, the WGS-based pMLST (Carattoli et al., 2014) identified this plasmid as belonging to the IncI1 family.
Plasmid pSI54/04 is regarded as a pESI-like plasmid (plasmid for emerging S. Infantis), because of showing high sequence similarity with the resistance-virulence megaplasmid pESI endemic in S. Infantis in Israel (Aviv et al., 2014; Szmolka et al., 2018). The real incompatibility group of some pESI-like plasmids is not clearly definable, because the replication origin of these plasmids is not conventional. It results from the substitution of the IncI1 oriV by an IncP-1alpha oriV (Aviv et al., 2014; Dionisi et al., 2016). This could be one reason for the controversial findings described above regarding incompatibility of pSI54/04, basically confirming findings of Bogomazova et al. (2019) in relation to comparative analysis of a selected set of pESI-like plasmid-bearing strains on the basis of whole-genome sequencing. It is conceivable that this mosaic replicon type may be an advantage for pESI-like plasmids, in facilitating selection and global spread of certain successful MDR clones of S. Infantis such as the clone B2, which is epidemic in Hungary.
pESI-like plasmids of S. Infantis gain further importance, because of their ability to coexist with certain resistance plasmids and/or to incorporate multiple resistance genes including those conferring resistance to ESBLs (Bogomazova et al., 2019).
As an example for plasmid coexistence, we describe the association between the tetracycline resistance plasmid pSI54/04 and a small blaTEM–1-qnrS1 plasmid of IncX incompatibility group responsible for β-lactam- and fluoroquinolone resistance in a small group of broiler strains. Kehrenberg et al. (2006) described for the first time a conjugative blaTEM–1-qnrS1 plasmid pINF5 in a chicken isolate of S. Infantis, but the emergence of new alleles such as blaTEM–20, blaTEM–52, blaTEM–70, blaTEM–148 and blaTEM–198 seems to diminish the scientific importance of the wild-type TEM-1 plasmids for this serovar (Cloeckaert et al., 2007; Shahada et al., 2010; Chuma et al., 2013). Very recently, the coexistence of a pESI-like megaplasmid with an IncX plasmid carrying the mcr-1 gene coding for colistin resistance has been reported in S. Infantis isolated from broilers (Carfora et al., 2018). Moreover, the ESBL gene blaCTX–M–1 was identified on a pESI-like plasmid of the IncP incompatibility group. Indeed, some successful MDR clones of S. Infantis in Europe and the United States carry pESI-like plasmids conferring ESBL resistance by genes blaCTX–M–1 and blaCTX–M–65 (Franco et al., 2015; Hindermann et al., 2017; Tate et al., 2017; Alba et al., 2020). In contrast, the plasmid pSI54/04 is constantly and predominantly present in the Hungarian broiler and human S. Infantis population, but does not carry ESBL genes.
In comparison to the overall high-level resistance to the above mentioned “classical” antibiotics, resistances to high-priority antibiotic classes such as fluoroquinolones, third-generation cephalosporins, carbapenems, polymyxins and macrolides were detected at a lower level or were not detected at all. The plasmid-mediated fluoroquinolone resistance gene qnrS1 characterised our broiler intestinal strains of E. coli and S. Infantis relatively frequently, but it was absent from extraintestinal E. coli strains. In harmony with these findings, the EU member states also reported a high prevalence of fluoroquinolone resistance in commensal (indicator) E. coli from broilers (EFSA and ECDC, 2020). The E. coli resistance to third-generation cephalosporins was conferred here by the ESBL gene blaCTX–M–3 and the AmpC beta-lactamase gene blaCMY–2 detected in a small number of strains with origin from caecum and faeces. In Europe, resistance to cephalosporins is also reported at a low incidence (EFSA and ECDC, 2020), but in some countries outside Europe the intestine of the broiler chickens is regarded as a reservoir of ESBL-producing E. coli (Li et al., 2016; Vinueza-Burgos et al., 2019). For S. Infantis, we report the first ESBL-producing human strain in Hungary that carries the cefotaxime resistance gene blaCTX–M–14 on an IncI1 type plasmid. This allele is rarely identified in broiler strains of S. Infantis (Kameyama et al., 2012; Bogomazova et al., 2019), and it is more likely embedded in conjugative plasmids of S. Enteritidis of human origin (Romero et al., 2004; Izumiya et al., 2005; Bado et al., 2012). The mobile resistance gene mph(B) encoding a macrolide efflux pump was identified in five ExPEC strains that were grouped in the so-called “super-MDR clade” characterised by multiple combinations of associated resistance genes and mechanisms. Besides, certain ExPEC strains are effective in causing severe diseases in chickens and posing a serious concern to food safety and to human health (Gao et al., 2018).
The WGS-based comparison of antibiotic resistance genes and plasmid types revealed a relatively narrow interface between exchangeable plasmid types of E. coli and of S. Infantis that may transfer antibiotic resistance genes. According to this, we found that the resistance genes tet(A)-aadA1-sul1 specific to the S. Infantis plasmid pSI54/04 were also frequently identified in E. coli strains, but the association of genes blaTEM–1 and qnrS1 were also commonly carried by members of both species. Nevertheless, the plasmid contigs of cohabitant S. Infantis and E. coli strains, which include the tet(A), aadA1, sul1, blaTEM–1 and qnrS1 genes are not identical in these two species. By the comparative mobile resistome analysis of the cohabitant strains, we were able to confirm the presence of these genes and of plasmid families IncI and IncX in strains of E. coli and S. Infantis isolated from the same caecum of chicken. Within these plasmid families, IncI1 plasmids were detected for both species, while IncX plasmids did not overlap at a subgroup level. These findings suggest that the potential for an interspecies transmission exists for certain resistance genes or even for whole plasmids such as the pSI54/04 or the blaTEM–1 plasmid. Indeed, it was demonstrated that the pESI plasmid endemic for S. Infantis in Israel can be transferred in vivo to E. coli strains as members of the normal gut microflora in mouse (Aviv et al., 2016), but so far there is a general lack of knowledge regarding the transferability of pESI-like plasmids of S. Infantis to E. coli in broilers.
We believe the results indicate that the comparative characterisation of cohabitant strains is promising, and we are fully aware that the in-depth analysis of transferable resistance elements in cohabitant S. Infantis and E. coli strains requires a larger number of strains representing the genomic diversity of relevant S. Infantis and E. coli isolates to be tested. However, our results so far can be considered as preliminary and conjugation experiments along this line are representing our future task.
Conclusion
This is the first report on the comparative genomic analysis of contemporary strains of S. Infantis and of E. coli from broilers. The present analysis confirms the continuous persistence and reveals the high genomic diversity of multiresistant E. coli and S. Infantis endemic in broiler flocks in Hungary. Our findings on the diversity of the mobile resistomes indicate that commensal E. coli can be regarded as a potential reservoir of resistance genes for Salmonella, but so far only a few plasmid families and genes of mobile resistomes of E. coli and S. Infantis could be considered as potentially exchangeable. Among these, certain IncI1 plasmids could play the most important role in resistance gene/plasmid exchange and the evolution of multiresistance between E. coli and S. Infantis. Future active molecular monitoring of MDR E. coli and cohabitant S. Infantis strains will certainly shed more light on the microevolution of emerging clones and plasmids of MDR S. Infantis in broilers.
Data Availability Statement
The genome sequence reads for all strains included into this study were combined in Bioproject number PRJNA694299 and are publicly available in the NCBI Sequence Read Archive (SRA). The accession numbers of the genome sequence reads of the individual isolates are also indicated in Supplementary Table 1.
Author Contributions
AS and UD conceived the project and wrote the manuscript. HW and AS performed the bioinformatic and resistome analyses, respectively. All authors reviewed the manuscript.
Funding
This work was supported by the national research fund NKFI K 128600, by a research fellowship of the Heinrich Hertz-Foundation to AS and by the Deutsche Forschungsgemeinschaft (DFG, German Research Foundation) – RTG2220 – project number 281125614. HW was supported by a Ph.D. scholarship of Pharma-Zentrale GmbH (Herdecke).
Conflict of Interest
The authors declare that this study received funding from Pharma-Zentrale GmbH (Herdecke). The funder was not involved in the study design, collection, analysis, interpretation of data, the writing of the article, or the decision to submit it for publication.
Acknowledgments
The authors thank Béla Nagy for his essential help during this project and for the critical review of the manuscript. Thanks are also due to Szilárd Jánosi and Judit Szolyák for providing the chicken caecal samples for the isolation of cohabitant strains of S. Infantis and E. coli. The authors thank Judit Pászti for providing the PFGE analysis for S. Infantis strains isolated in 2016 and 2018. Further thanks are due to Csaba Szalay and Béla Markos for providing E. coli cultures from the caecum and the bone marrow of chicks. The authors also thank Karin Tegelkamp for the excellent performance of the genome sequencing of the S. Infantis and E. coli isolates.
Supplementary Material
The Supplementary Material for this article can be found online at: https://www.frontiersin.org/articles/10.3389/fmicb.2021.642125/full#supplementary-material
Supplementary Figure 1 | Relation between the sequence type (ST) and abundance of acquired resistance genes. In bold are indicated STs that are potentially associated with increased abundance of acquired resistance genes (MAR index > 0.3). Circle sizes are proportional to the prevalence (%) of the given gene for each ST. “Empty” STs (ST46, 349, 355, and 665) are not presented.
Supplementary Figure 2 | Multiple sequence alignment of plasmid contigs carrying antibiotic resistance genes that overlap between cohabitant strains of S. Infantis and E. coli. The sequence identity of plasmid contigs including the aadA1, sul1, tet(A), blaTEM–1, or qnrS1 genes was compared between cohabitant S. Infantis and E. coli strains. S. Infantis strains are coloured in blue, while green represents E. coli strains.
Supplementary Table 1 | Sequenced strains of broiler and human S. Infantis (SI) and of broiler E. coli (Ec)
with origin from different sample sources. Black dots indicate cohabitant strains of SI and Ec isolated from six caecal samples representing one farm each. Cip (ciprofloxacin) resistance also means resistance to nalidixic acid (Nal).
Supplementary Table 2 | Sero- and phylogroups of E. coli strains isolated from different broiler sources.
Footnotes
References
Acar, S., Bulut, E., Stasiewicz, M. J., and Soyer, Y. (2019). Genome analysis of antimicrobial resistance, virulence, and plasmid presence in turkish Salmonella serovar infantis isolates. Int. J. Food Microbiol. 307:108275. doi: 10.1016/j.ijfoodmicro.2019.108275
Achtman, M., Wain, J., Weill, F.-X., Nair, S., Zhou, Z., Sangal, V., et al. (2012). Multilocus sequence typing as a replacement for serotyping in Salmonella enterica. PLoS Pathog. 8:e1002776. doi: 10.1371/journal.ppat.1002776
Ahmed, A. M., Shimabukuro, H., and Shimamoto, T. (2009). Isolation and molecular characterization of multidrug-resistant strains of Escherichia coli and Salmonella from retail chicken meat in japan. J. Food Sci. 74, M405–M410. doi: 10.1111/j.1750-3841.2009.01291.x
Alba, P., Leekitcharoenphon, P., Carfora, V., Amoruso, R., Cordaro, G., Matteo, P. D., et al. (2020). Molecular epidemiology of Salmonella Infantis in Europe: insights into the success of the bacterial host and its parasitic pESI-like megaplasmid. Microb. Genom. 6:e000365. doi: 10.1099/mgen.0.000365
Alonso, C. A., Michael, G. B., Li, J., Somalo, S., Simón, C., Wang, Y., et al. (2017). Analysis of blaSHV-12-carrying Escherichia coli clones and plasmids from human, animal and food sources. J. Antimicrob. Chemoth. 72, 1589–1596. doi: 10.1093/jac/dkx024
Andrews, S. (2010). FastQC: a quality control tool for high throughput sequence data. Available online at: http://www.bioinformatics.babraham.ac.uk/projects/fastqc
Aviv, G., Rahav, G., and Gal-Mor, O. (2016). Horizontal transfer of the Salmonella enterica serovar infantis resistance and virulence plasmid pESI to the gut microbiota of warm-blooded hosts. mBio 7, e1316–e1395. doi: 10.1128/mbio.01395-16
Aviv, G., Tsyba, K., Steck, N., Salmon-Divon, M., Cornelius, A., Rahav, G., et al. (2014). A unique megaplasmid contributes to stress tolerance and pathogenicity of an emergent Salmonella enterica serovar Infantis strain. Environ. Microbiol. 16, 977–994. doi: 10.1111/1462-2920.12351
Bado, I., García-Fulgueiras, V., Cordeiro, N. F., Betancor, L., Caiata, L., Seija, V., et al. (2012). First human isolate of Salmonella enterica serotype enteritidis harboring blaCTX-M-14 in south america. Antimicrob. Agents Chemother. 56, 2132–2134. doi: 10.1128/aac.05530-11
Bankevich, A., Nurk, S., Antipov, D., Gurevich, A. A., Dvorkin, M., Kulikov, A. S., et al. (2012). SPAdes: A new genome assembly algorithm and its applications to single-cell sequencing. J. Comp. Biol. 19, 455–477. doi: 10.1089/cmb.2012.0021
Bej, A. K., McCarty, S. C., and Atlas, R. M. (1991). Detection of coliform bacteria and Escherichia coli by multiplex polymerase chain reaction: comparison with defined substrate and plating methods for water quality monitoring. Appl. Environ. Microbiol. 57, 2429–2432.
Blake, D. P., Hillman, K., Fenlon, D. R., and Low, J. C. (2003). Transfer of antibiotic resistance between commensal and pathogenic members of the Enterobacteriaceae under ileal conditions. J. Appl. Microbiol. 95, 428–436. doi: 10.1046/j.1365-2672.2003.01988.x
Bogomazova, A. N., Gordeeva, V. D., Krylova, E. V., Soltynskaya, I. V., Davydova, E. E., Ivanova, O. E., et al. (2019). Mega-plasmid found worldwide confers multiple antimicrobial resistance in Salmonella Infantis of broiler origin in russia. Int. J. Food Microbiol. 319:108497. doi: 10.1016/j.ijfoodmicro.2019.108497
Carattoli, A. (2013). Plasmids and the spread of resistance. Int. J. Med. Microbiol. 303, 298–304. doi: 10.1016/j.ijmm.2013.02.001
Carattoli, A., Bertini, A., Villa, L., Falbo, V., Hopkins, K. L., and Threlfall, E. (2005). Identification of plasmids by PCR-based replicon typing. J. Microbiol. Methods 63, 219–228. doi: 10.1016/j.mimet.2005.03.018
Carattoli, A., Zankari, E., García-Fernández, A., Larsen, M. V., Lund, O., Villa, L., et al. (2014). In silico detection and typing of plasmids using plasmidfinder and plasmid multilocus sequence typing. Antimicrob. Agents Chemother. 58, 3895–3903. doi: 10.1128/AAC.02412-14
Carfora, V., Alba, P., Leekitcharoenphon, P., Ballarò, D., Cordaro, G., Matteo, P. D., et al. (2018). Colistin resistance mediated by mcr-1 in ESBL-producing, multidrug resistant Salmonella infantis in broiler chicken industry, italy (2016–2017). Front. Microbiol. 9:1880. doi: 10.3389/fmicb.2018.01880
Chuma, T., Miyasako, D., Dahshan, H., Takayama, T., Nakamoto, Y., Shahada, F., et al. (2013). Chronological change of resistance to β-lactams in Salmonella enterica serovar infantis isolated from broilers in japan. Front. Microbiol. 4:113. doi: 10.3389/fmicb.2013.00113
Cloeckaert, A., Praud, K., Doublet, B., Bertini, A., Carattoli, A., Butaye, P., et al. (2007). Dissemination of an extended-spectrum-β-lactamase blaTEM-52 gene-carrying IncI1 plasmid in various Salmonella enterica serovars isolated from poultry and humans in belgium and france between 2001 and 2005. Antimicrob. Agents Chemother. 51, 1872–1875. doi: 10.1128/AAC.01514-06
Dionisi, A. M., Owczarek, S., Benedetti, I., Luzzi, I., and García-Fernández, A. (2016). Extended-spectrum β-lactamase-producing Salmonella enterica serovar Infantis from humans in Italy. Int. J. Antimicrob. Agents 48, 345–346. doi: 10.1016/j.ijantimicag.2016.06.025
Dunn, S. J., Connor, C., and McNally, A. (2019). The evolution and transmission of multi-drug resistant Escherichia coli and Klebsiella pneumoniae: the complexity of clones and plasmids. Curr. Opin. Microbiol. 51, 51–56. doi: 10.1016/j.mib.2019.06.004
EUCAST. (2018). The European Committee on Antimicrobial Susceptibility Testing. Breakpoint tables for interpretation of MICs and zone diameters. Version 8.0. Available Online at: http://www.eucast.org
EFSA and ECDC (2020). The european union summary report on antimicrobial resistance in zoonotic and indicator bacteria from humans, animals and food in 2017/2018. EFSA J. 18:e06007. doi: 10.2903/j.efsa.2020.6007
Franco, A., Leekitcharoenphon, P., Feltrin, F., Alba, P., Cordaro, G., Iurescia, M., et al. (2015). Emergence of a clonal lineage of multidrug-resistant ESBL-producing Salmonella infantis transmitted from broilers and broiler meat to humans in italy between 2011 and 2014. PLoS One 10:e0144802. doi: 10.1371/journal.pone.0144802
Furlan, J. P. R., Gallo, I. F. L., Campos, A. C. L. P., de, Passaglia, J., Falcão, J. P., Navarro, A., et al. (2019). Molecular characterization of multidrug-resistant Shiga toxin-producing Escherichia coli harboring antimicrobial resistance genes obtained from a farmhouse. Pathog. Glob. Health 113, 1–7. doi: 10.1080/20477724.2019.1693712
Gal-Mor, O., Valinsky, L., Weinberger, M., Guy, S., Jaffe, J., Schorr, Y., et al. (2010). Multidrug-Resistant Salmonella enterica serovar infantis, israel. Emerg. Infect. Dis. 16, 1754–1757. doi: 10.3201/eid1611.100100
Gao, J., Duan, X., Li, X., Cao, H., Wang, Y., and Zheng, S. J. (2018). Emerging of a highly pathogenic and multi-drug resistant strain of Escherichia coli causing an outbreak of colibacillosis in chickens. Infect. Genet. Evol. 65, 392–398. doi: 10.1016/j.meegid.2018.08.026
García-Soto, S., Abdel-Glil, M. Y., Tomaso, H., Linde, J., and Methner, U. (2020). Emergence of multidrug-resistant Salmonella enterica subspecies enterica serovar infantis of multilocus sequence type 2283 in german broiler farms. Front. Microbiol. 11:1741. doi: 10.3389/fmicb.2020.01741
Gross, W. B. (1991). “Colibacillosis,” in Disease of Poultry, 9th Edn, eds B. W. Calnek, H. J. Barnes, C. W. Beard, W. M. Reid, and J. H. W. Yoder (Ames: Iowa State University Press), 138–144.
Gyles, C. L. (2008). Antimicrobial resistance in selected bacteria from poultry. Anim. Health Res. Rev. 9, 149–158. doi: 10.1017/s1466252308001552
Gymoese, P., Kiil, K., Torpdahl, M., Østerlund, M. T., Sørensen, G., Olsen, J. E., et al. (2019). WGS based study of the population structure of Salmonella enterica serovar Infantis. BMC Genom. 20:870. doi: 10.1186/s12864-019-6260-6
Hammer, Ø, Harper, D. A. T., and Ryan, P. D. (2001). PAST: Paleontological statistics software package for education and data analysis. Palaeontol. Electron 4:9.
Hindermann, D., Gopinath, G., Chase, H., Negrete, F., Althaus, D., Zurfluh, K., et al. (2017). Salmonella enterica serovar infantis from food and human infections, switzerland, 2010–2015: poultry-related multidrug resistant clones and an emerging ESBL producing clonal lineage. Front. Microbiol. 8:1322. doi: 10.3389/fmicb.2017.01322
Huja, S., Oren, Y., Trost, E., Brzuszkiewicz, E., Biran, D., Blom, J., et al. (2015). Genomic avenue to avian colisepticemia. Mbio 6, e1614–e1681. doi: 10.1128/mbio.01681-14
Izumiya, H., Mori, K., Higashide, M., Tamura, K., Takai, N., Hirose, K., et al. (2005). Identification of CTX-M-14 β-Lactamase in a Salmonella enterica serovar enteritidis isolate from japan. Antimicrob. Agents Chemother. 49, 2568–2570. doi: 10.1128/aac.49.6.2568-2570.2005
Joensen, K. G., Tetzschner, A. M. M., Iguchi, A., Aarestrup, F. M., and Scheutz, F. (2015). Rapid and easy in Silico serotyping of Escherichia coli isolates by use of whole-genome sequencing data. J. Clin. Microbiol. 53, 2410–2426. doi: 10.1128/jcm.00008-15
Johnson, T. J., and Nolan, L. K. (2009). Pathogenomics of the virulence plasmids of Escherichia coli. Microbiol. Mol. Biol. Rev. 73, 750–774. doi: 10.1128/mmbr.00015-09
Jünemann, S., Sedlazeck, F. J., Prior, K., Albersmeier, A., John, U., Kalinowski, J., et al. (2013). Updating benchtop sequencing performance comparison. Nat. Biotechnol. 31, 294–296. doi: 10.1038/nbt.2522
Kameyama, M., Chuma, T., Yokoi, T., Yata, J., Tominaga, K., Miyasako, D., et al. (2012). Emergence of Salmonella enterica serovar infantis harboring inci1 plasmid with blaCTX-M-14 in a broiler farm in japan. J. Vet. Med. Sci. 74, 1213–1216. doi: 10.1292/jvms.11-0488
Kardos, G., Farkas, T., Antal, M., Nógrády, N., and Kiss, I. (2007). Novel PCR assay for identification of Salmonella enterica serovar Infantis. Lett. Appl. Microbiol. 45, 421–425. doi: 10.1111/j.1472-765X.2007.02220.x
Kehrenberg, C., Friederichs, S., Jong, A., de, Michael, G. B., and Schwarz, S. (2006). Identification of the plasmid-borne quinolone resistance gene qnrS in Salmonella enterica serovar Infantis. J. Antimicrob. Chemother. 58, 18–22. doi: 10.1093/jac/dkl213
Krumperman, P. H. (1983). Multiple antibiotic resistance indexing of Escherichia coli to identify high-risk sources of fecal contamination of foods. Appl. Environ. Microbiol. 46, 165–170.
Li, S., Zhao, M., Liu, J., Zhou, Y., and Miao, Z. (2016). Prevalence and antibiotic resistance profiles of extended-spectrum β-lactamase–producing Escherichia coli isolated from healthy broilers in shandong province, china. J. Food Protect. 79, 1169–1173. doi: 10.4315/0362-028x.jfp-16-025
Liu, X., Liu, H., Wang, L., Peng, Q., Li, Y., Zhou, H., et al. (2018). Molecular characterization of extended-spectrum β-lactamase-producing multidrug resistant Escherichia coli from swine in northwest china. Front. Microbiol. 9:1756. doi: 10.3389/fmicb.2018.01756
Mathers, A. J., Peirano, G., and Pitout, J. D. D. (2015). Chapter four Escherichia coli ST131: the quintessential example of an international multiresistant high-risk clone. Adv. Appl. Microbiol. 90, 109–154. doi: 10.1016/bs.aambs.2014.09.002
Mathew, A. G., Jackson, F., and Saxton, A. M. (2002). Effects of antibiotic regimens on resistance of Escherichia coli and Salmonella serovar typhimurium in swine. J. Swine Health Prod. 10, 7–13.
McMillan, E. A., Jackson, C. R., and Frye, J. G. (2020). Transferable plasmids of Salmonella enterica associated with antibiotic resistance genes. Front. Microbiol. 11:562181. doi: 10.3389/fmicb.2020.562181
Moulin-Schouleur, M., Répérant, M., Laurent, S., Brée, A., Mignon-Grasteau, S., Germon, P., et al. (2007). Extraintestinal pathogenic Escherichia coli strains of avian and human origin: link between phylogenetic relationships and common virulence patterns. J. Clin. Microbiol. 45, 3366–3376. doi: 10.1128/jcm.00037-07
Nagy, T., Szmolka, A., Wilk, T., Kiss, J., Szabó, M., Pászti, J., et al. (2020). Comparative genome analysis of hungarian and global strains of Salmonella infantis. Front. Microbiol. 11:539. doi: 10.3389/fmicb.2020.00539
Nógrády, N., Király, M., Davies, R., and Nagy, B. (2012). Multidrug resistant clones of Salmonella Infantis of broiler origin in europe. Int. J. Food Microbiol. 157, 108–112. doi: 10.1016/j.ijfoodmicro.2012.04.007
Nógrády, N., Tóth, Á, Kostyák, Á, Pászti, J., and Nagy, B. (2007). Emergence of multidrug-resistant clones of Salmonella Infantis in broiler chickens and humans in Hungary. J. Antimicrob. Chemoth. 60, 645–648. doi: 10.1093/jac/dkm249
Ochman, H., and Wilson, A. C. (1987). Evolution in bacteria: evidence for a universal substitution rate in cellular genomes. J. Mol. Evol. 26:377. doi: 10.1007/bf02101157
Oladeinde, A., Cook, K., Lakin, S. M., Woyda, R., Abdo, Z., Looft, T., et al. (2019). Horizontal gene transfer and acquired antibiotic resistance in Salmonella enterica serovar heidelberg following in vitro incubation in broiler ceca. Appl. Environ. Microb. 85, e01903–e01919. doi: 10.1128/aem.01903-19
Olasz, F., Nagy, T., Szabó, M., Kiss, J., Szmolka, A., Barta, E., et al. (2015). Genome sequences of three Salmonella enterica subsp. enterica serovar infantis strains from healthy broiler chicks in hungary and in the united kingdom. Genome Announc. 3, e1414–e1468. doi: 10.1128/genomea.01468-14
Oluwadare, M., Lee, M. D., Grim, C. J., Lipp, E. K., Cheng, Y., and Maurer, J. J. (2020). The role of the Salmonella spvB IncF plasmid and its resident entry exclusion gene traS on plasmid exclusion. Front. Microbiol. 11:949. doi: 10.3389/fmicb.2020.00949
Poppe, C., Martin, L. C., Gyles, C. L., Reid-Smith, R., Boerlin, P., McEwen, S. A., et al. (2005). Acquisition of resistance to extended-spectrum cephalosporins by Salmonella enterica subsp. enterica serovar newport and Escherichia coli in the turkey poult intestinal tract. Appl. Environ. Microb. 71, 1184–1192. doi: 10.1128/aem.71.3.1184-1192.2005
Poulsen, L. L., Bisgaard, M., Jørgensen, S. L., Dideriksen, T., Pedersen, J. R., and Christensen, H. (2018). Characterization of Escherichia coli causing cellulitis in broilers. Vet. Microbiol. 225, 72–78. doi: 10.1016/j.vetmic.2018.09.011
Romero, L., López, L., Martínez-Martínez, L., Guerra, B., Hernández, J. R., and Pascual, A. (2004). Characterization of the first CTX-M-14-producing Salmonella enterica serotype Enteritidis isolate. J. Antimicrob. Chemoth. 53, 1113–1114. doi: 10.1093/jac/dkh246
Ronco, T., Stegger, M., Olsen, R. H., Sekse, C., Nordstoga, A. B., Pohjanvirta, T., et al. (2017). Spread of avian pathogenic Escherichia coli ST117 O78:H4 in Nordic broiler production. BMC Genom. 18:13. doi: 10.1186/s12864-016-3415-6
Roth, N., Käsbohrer, A., Mayrhofer, S., Zitz, U., Hofacre, C., and Domig, K. J. (2019). The application of antibiotics in broiler production and the resulting antibiotic resistance in Escherichia coli: A global overview. Poultry Sci. 98, 1791–1804. doi: 10.3382/ps/pey539
Schouler, C., Schaeffer, B., Brée, A., Mora, A., Dahbi, G., Biet, F., et al. (2012). Diagnostic strategy for identifying avian pathogenic Escherichia coli based on four patterns of virulence genes. J. Clin. Microbiol. 50, 1673–1678. doi: 10.1128/jcm.05057-11
Shahada, F., Chuma, T., Dahshan, H., Akiba, M., Sueyoshi, M., and Okamoto, K. (2010). Detection and characterization of extended-spectrum beta-lactamase (TEM-52)-producing Salmonella serotype Infantis from broilers in Japan. Foodborne Pathog. Dis. 7, 515–521. doi: 10.1089/fpd.2009.0454
Shahada, F., Chuma, T., Tobata, T., Okamoto, K., Sueyoshi, M., and Takase, K. (2006). Molecular epidemiology of antimicrobial resistance among Salmonella enterica serovar Infantis from poultry in Kagoshima, Japan. Int. J. Antimicrob. Agents 28, 302–307. doi: 10.1016/j.ijantimicag.2006.07.003
Szmolka, A., and Nagy, B. (2013). Multidrug resistant commensal Escherichia coli in animals and its impact for public health. Front. Microbiol. 4:258. doi: 10.3389/fmicb.2013.00258
Szmolka, A., Szabó, M., Kiss, J., Pászti, J., Adrián, E., Olasz, F., et al. (2018). Molecular epidemiology of the endemic multiresistance plasmid pSI54/04 of Salmonella Infantis in broiler and human population in hungary. Food Microbiol. 71, 25–31. doi: 10.1016/j.fm.2017.03.011
Tate, H., Folster, J. P., Hsu, C.-H. H., Chen, J., Hoffmann, M., Li, C., et al. (2017). Comparative analysis of extended-spectrum-β-Lactamase CTX-M-65-producing Salmonella enterica serovar infantis isolates from humans, food animals, and retail chickens in the united states. Antimicrob. Agents Chemother. 61, e417–e488. doi: 10.1128/aac.00488-17
Tóth, I., Dobrindt, U., Koscsó, B., Kósa, A., Herpay, M., and Nagy, B. (2012). Genetic and phylogenetic analysis of avian extraintestinal and intestinal Escherichia coli. Acta Microbiol. Imm. H. 59, 393–409. doi: 10.1556/amicr.59.2012.3.10
Varga, C., Guerin, M. T., Brash, M. L., Slavic, D., Boerlin, P., and Susta, L. (2019). Antimicrobial resistance in fecal Escherichia coli and Salmonella enterica isolates: a two-year prospective study of small poultry flocks in ontario, canada. BMC Vet. Res. 15:464. doi: 10.1186/s12917-019-2187-z
Vila, J., Sáez-López, E., Johnson, J., Römling, U., Dobrindt, U., Cantón, R., et al. (2016). Escherichia coli: an old friend with new tidings. FEMS Microbiol. Rev. 40, 437–463. doi: 10.1093/femsre/fuw005
Villa, L., García-Fernández, A., Fortini, D., and Carattoli, A. (2010). Replicon sequence typing of IncF plasmids carrying virulence and resistance determinants. J. Antimicrob. Chemoth. 65, 2518–2529. doi: 10.1093/jac/dkq347
Vinueza-Burgos, C., Ortega-Paredes, D., Narváez, C., Zutter, L. D., and Zurita, J. (2019). Characterization of cefotaxime resistant Escherichia coli isolated from broiler farms in ecuador. PLos One 14:e0207567. doi: 10.1371/journal.pone.0207567
Waters, N., Brennan, F., Holmes, A., Abram, F., and Pritchard, L. (2018). Easily phylotyping E. coli via the EzClermont web app and command-line tool. biorxiv 2018:317610. doi: 10.1101/317610
Wirth, T., Falush, D., Lan, R., Colles, F., Mensa, P., Wieler, L. H., et al. (2006). Sex and virulence in Escherichia coli: an evolutionary perspective. Mol. Microbiol. 60, 1136–1151. doi: 10.1111/j.1365-2958.2006.05172.x
Yamaji, R., Friedman, C. R., Rubin, J., Suh, J., Thys, E., McDermott, P., et al. (2018a). A population-based surveillance study of shared genotypes of Escherichia coli isolates from retail meat and suspected cases of urinary tract infections. Msphere 3, e118–e179. doi: 10.1128/msphere.00179-18
Yamaji, R., Rubin, J., Thys, E., Friedman, C. R., and Riley, L. W. (2018b). Persistent pandemic lineages of uropathogenic Escherichia coli in a college community from 1999 to 2017. J. Clin. Microbiol. 56, e1817–e1834. doi: 10.1128/jcm.01834-17
Yang, Y.-Q., Li, Y.-X., Song, T., Yang, Y.-X., Jiang, W., Zhang, A.-Y., et al. (2017). Colistin resistance gene mcr-1 and its variant in Escherichia coli isolates from chickens in china. Antimicrob. Agents Ch. 61, e1204–e1216. doi: 10.1128/aac.01204-16
Zankari, E., Hasman, H., Cosentino, S., Vestergaard, M., Rasmussen, S., Lund, O., et al. (2012). Identification of acquired antimicrobial resistance genes. J. Antimicrob. Chemoth. 67, 2640–2644. doi: 10.1093/jac/dks261
Zhang, P., Wang, J., Wang, X., Bai, X., Ma, J., Dang, R., et al. (2019). Characterization of five Escherichia coli isolates Co-expressing ESBL and MCR-1 resistance mechanisms from different origins in china. Front. Microbiol. 10:1994. doi: 10.3389/fmicb.2019.01994
Zhang, S., Yin, Y., Jones, M. B., Zhang, Z., Kaiser, B. L., Dinsmore, B. A., et al. (2015). Salmonella serotype determination utilizing high-throughput genome sequencing data. J. Clin. Microbiol. 53, 1685–1692. doi: 10.1128/JCM.00323-15
Keywords: Salmonella Infantis, Escherichia coli, antibiotic resistance, resistome, core genome, plasmid
Citation: Szmolka A, Wami H and Dobrindt U (2021) Comparative Genomics of Emerging Lineages and Mobile Resistomes of Contemporary Broiler Strains of Salmonella Infantis and E. coli. Front. Microbiol. 12:642125. doi: 10.3389/fmicb.2021.642125
Received: 15 December 2020; Accepted: 03 February 2021;
Published: 25 February 2021.
Edited by:
Axel Cloeckaert, Institut National de Recherche pour l’Agriculture, l’Alimentation et l’Environnement (INRAE), FranceReviewed by:
Jonathan Gray Frye, U.S. National Poultry Research Center (USDA-ARS), United StatesJorge Blanco, University of Santiago de Compostela, Spain
Eelco Franz, Centre for Infectious Disease Control (RIVM), Netherlands
Nicole Ricker, University of Guelph, Canada
Copyright © 2021 Szmolka, Wami and Dobrindt. This is an open-access article distributed under the terms of the Creative Commons Attribution License (CC BY). The use, distribution or reproduction in other forums is permitted, provided the original author(s) and the copyright owner(s) are credited and that the original publication in this journal is cited, in accordance with accepted academic practice. No use, distribution or reproduction is permitted which does not comply with these terms.
*Correspondence: Ama Szmolka, c3ptb2xrYS5hbm5hbWFyaWFAYXRrLmh1