- Departamento de Genética, Facultad de Biología, Universidad de Sevilla, Sevilla, Spain
Genes annotated as ygfE and yiiU in the genome of Salmonella enterica serovar Typhimurium encode proteins homologous to Escherichia coli cell division factors ZapA and ZapB, respectively. ZapA− and ZapB− mutants of S. enterica are bile-sensitive. The amount of zapB mRNA increases in the presence of a sublethal concentration of sodium deoxycholate (DOC) while zapA mRNA remains unaffected. Increased zapB mRNA level in the presence of DOC is not caused by upregulation of zapB transcription but by increased stability of zapB mRNA. This increase is suppressed by an hfq mutation, suggesting the involvement of a small regulatory RNA. We provide evidence that such sRNA is MicA. The ZapB protein is degraded in the presence of DOC, and degradation appears to involve the Lon protease. We propose that increased stability of zapB mRNA in the presence of DOC may counter degradation of bile-damaged ZapB, thereby providing sufficient level of functional ZapB protein to permit Z-ring assembly in the presence of bile.
Introduction
During infection of the gastrointestinal tract, enteric pathogens must endure harsh environments such as pH variations, low oxygen levels, elevated osmolarity, and nutrient limitation (Alvarez-Ordonez et al., 2011; Fang et al., 2016). In the small intestine, periodical secretion of bile poses another challenge due to the antibacterial activity of bile salts (Hofmann and Hagey, 2008). Salmonella serovars that cause systemic and chronic infections also encounter bile in the gall bladder, at concentrations higher and more steady than in the intestine (Hofmann and Hagey, 2008; Dougan and Baker, 2014). Bile acids/salts act as detergents that disrupt membrane phospholipids, cause misfolding and denaturation of proteins as well as oxidative DNA damage, and interfere with the formation of secondary RNA structures (Bernstein et al., 1999; Gunn, 2000; Prieto et al., 2004; Begley et al., 2005; Merritt and Donaldson, 2009; Urdaneta and Casadesus, 2017; Bustos et al., 2018).
Resistance to bile can be studied under laboratory conditions by adding ox bile or individual bile salts to microbiological culture media. In both Escherichia coli and Salmonella, isolation of bile-sensitive mutants has identified loci required for bile resistance, and suppressor analysis has contributed to understand the mechanisms involved (Picken and Beacham, 1977; Thanassi et al., 1997; van Velkinburgh and Gunn, 1999; Wibbenmeyer et al., 2002; Ramos-Morales et al., 2003; Buckley et al., 2006; Crawford et al., 2012; Hernandez et al., 2012; May and Groisman, 2014; Hernandez et al., 2015; Urdaneta and Casadesus, 2018). In the last decade, bacterial responses to bile have been investigated also by high throughput analysis of gene expression, and the combination of genetics, biochemistry, and transcriptomics has provided a picture of the cell components and bacterial responses that permit bile resistance (Prouty et al., 2004; Hernandez et al., 2012; Kroger et al., 2013; Johnson et al., 2017). Envelope structures such as the lipopolysaccharide (Picken and Beacham, 1977; van Velkinburgh and Gunn, 1999; Crawford et al., 2012), the enterobacterial common antigen (Ramos-Morales et al., 2003), and the peptidoglycan (Hernandez et al., 2015) provide barriers that reduce intake of bile salts. In addition, bile salts are transported outside the cell by efflux pumps, especially AcrAB-TolC (Thanassi et al., 1997; Buckley et al., 2006; Urdaneta and Casadesus, 2018). Exposure to bile also activates stress responses (Thanassi et al., 1997; Bernstein et al., 1999; Hernandez et al., 2012), remodeling of the cell wall (Hernandez et al., 2015), and DNA repair (Prieto et al., 2006).
Among the bile-upregulated genes identified by transcriptomic analysis, a Salmonella enterica locus annotated as yiiU was found (Hernandez et al., 2012). In this study, we show that S. enterica YiiU is a homolog of the E. coli ZapB protein, a non-essential cell division factor involved in Z-ring formation and nucleoid segregation (Ebersbach et al., 2008; Buss et al., 2015; Mannik et al., 2016; Du and Lutkenhaus, 2017). Salmonella ZapB− mutants are sensitive to sodium deoxycholate (DOC), indicating that ZapB plays a role in bile resistance. We also show that the S. enterica ygfE gene is a homolog of E. coli ZapA, and that ZapA− mutants are sensitive to bile. However, zapA expression is not regulated by bile.
Upregulation of the zapB mRNA level in the presence of DOC appears to involve interaction with MicA, a small regulatory RNA. We tentatively propose that increased stability of zapB mRNA in the presence of DOC may counter degradation of bile-damaged ZapB by the Lon protease.
Materials and Methods
Bacterial Strains, Bacteriophages, Plasmids, and Strain Construction
Strains of S. enterica used in this study (Table 1) belong to serovar Typhimurium and derive from a His+ derivative of SL1344 (Hoiseth and Stocker, 1981; Cano et al., 2002). Escherichia coli RK4353 [Δ(argF-lac)169 Δ(fimB-fimE)632(::IS1) Δ(fruK-yeiR) F− λ− [araD139] deoC1 flhD5301 gyrA219 rbsR22 relA1 rpsL150] was the host of pBAD18 (Guzman et al., 1995). Escherichia coli DH5α [endA1 hsdR17 supE44 thi1 recA1 gyrA96 relA1 ΔlacU189 (Ф80 lacZΔM15)] was also used for cloning. E. coli CC118 λ pir [phoA20 thi-1 rspE rpoB argE(Am) recA1 (λ pir)] and E. coli S17-1 λ pir [recA pro hsdR RP4-2-Tc::Mu-Km::Tn7 (λ pir)] were used for directed construction of point mutations. Plasmid pDMS197 is a suicide vector containing the sacB gene of Bacillus spp. (Edwards et al., 1998). Transduction was performed with S. enterica phage P22 HT 105/1 int201 (Schmieger, 1972). The P22 HT transduction protocol was described elsewhere (Torreblanca et al., 1999). To obtain phage-free isolates, transductants were purified by streaking on green plates (Garzon et al., 1995). Phage sensitivity was tested by cross-streaking with P22 H5.
Targeted disruption of yiiU (zapB) and ygfE (zapA) was achieved using the pKD13 plasmid (Datsenko and Wanner, 2000) and oligonucleotides listed in Supplementary Table S1. The antibiotic resistance cassette introduced during strain construction was excised by recombination with pCP20 (Datsenko and Wanner, 2000). For construction of the lacZ fusions in the Salmonella chromosome, FRT sites generated by excision of the KmR cassette were used to integrate plasmid pCE40 (Ellermeier et al., 2002). Addition of 3xFLAG tag to the protein-coding zapB DNA sequence was carried out using the pSUB11 plasmid as template (Uzzau et al., 2001) and the oligonucleotides zapB-FLAG-FOR and zapB-FLAG-REV. Introduction of the lon::Tn10 allele into SL1344 was achieved by P22 HT-mediated transduction from the ATCC 14028 derivative SV5663 (Garcia-Calderon et al., 2009). The PLtetO-zapB construct was engineered by inserting the PLtetO promoter from the pXG1 plasmid (Lutz and Bujard, 1997) upstream of the transcriptional start site of zapB::lacZ on the Salmonella chromosome using primers zapB-PLtetO-FOR and zapB-PLtetO-REV. Details on the procedure have been described elsewhere (Lopez-Garrido and Casadesus, 2012).
To clone the zapB promoter with its 5' untranslated region (UTR) upstream of the lacZ gene of pIC552 (Macian et al., 1994), the primers pIC-zapB-FOR and pIC-zapB-REV were used. Plasmids carrying the S. enterica and E. coli zapA and zapB genes were constructed by cloning the respective genes under the control of the arabinose-dependent pBAD promoter of pBAD18 (Guzman et al., 1995). Plasmids pBR328-CyaR, pBR328-InvR, pBR328-MicA, pBR328-RyhB-1, and pBR328-RyhB-2 are pBR328 derivatives.
Media and Culture Conditions
Lysogeny broth (LB) and M9 minimal medium (Sambrook and Russell, 2001) were used as liquid media. Solid LB contained agar at 1.5% final concentration. To prepare LB containing sodium DOC (Sigma-Aldrich), an appropriate volume from a 25% stock was added. Liquid cultures were grown with aeration by shaking in an orbital incubator. Antibiotics were used at concentrations described previously (Garcia-Calderon et al., 2005). Arabinose was used at a final concentration of 0.2%.
Subcellular Fractionation
Subcellular fractionation was performed as described elsewhere (Pucciarelli et al., 2002), with some modifications. Briefly, bacteria were grown in LB medium at 37°C, spun down by centrifugation at 15,000 × g for 5 min at 4°C and resuspended twice in cold phosphate-buffered saline (PBS, pH 7.4). The bacterial suspension was disrupted by sonication, and unbroken cells were further removed by low-speed centrifugation (5,000 × g, 5 min, 4°C). The supernatant was centrifuged at high speed (100,000 × g, 30 min, 4°C) and the new supernatant was recovered as the cytosol fraction. The pellet containing envelope material was suspended in PBS with 0.4% Triton X-100 and incubated for 2 h at 4°C. The sample was centrifuged again (100,000 × g, 30 min, 4°C) and divided into the supernatant containing mostly inner membrane proteins and the insoluble fraction corresponding to the outer membrane fraction. An appropriate volume of Laemmli buffer was added to each fraction. After heating (100°C, 5 min) and clearing by centrifugation (15,000 × g, 5 min, room temperature), the protein content was analyzed by SDS-PAGE.
Determination of Minimal Inhibitory Concentration of Sodium Deoxycholate
Aliquots from exponential cultures in LB, each containing around 3 × 102 colony-forming-units (CFUs) were transferred to polypropylene microtiter plates (Soria Genlab, Valdemoro, Spain) containing known amounts of DOC. After 12 h incubation at 37°C, growth was visually monitored.
β-Galactosidase Assays
Levels of β-galactosidase activity were determined using the CHCl3-sodium dodecyl sulfate permeabilization procedure (Miller, 1972).
Quantitative Reverse Transcriptase PCR
For quantitative reverse transcriptase-PCR (qRT-PCR), Salmonella RNA was extracted from late exponential cultures (OD600 ≅ 1). Gene-specific primers (Supplementary Table S1) were designed with ProbeFinder software1 from Roche Applied Science. The efficiency of each primer pair was determined to be higher than 90%, following the procedure described in the real-time quantitative PCR (qPCR) guide of Applied Biosystems. Relative RNA levels were determined using the ∆∆Ct method. Each ∆∆Ct determination was performed at least in three different RNA samples.
Preparation of Protein Extracts and Western Blot Analysis
Total protein extracts were prepared from cultures grown until stationary phase (OD600 > 1) at 37°C in LB or LB + 5% DOC. Around 3 × 109 bacterial cells were collected by centrifugation (16,000 × g, 2 min), resuspended in 50 μl of Laemmli sample buffer [1.3% SDS, 10% (v/v) glycerol, 50 mM Tris-HCl, 1.8% β-mercaptoethanol, 0.02% bromophenol blue, pH 6.8] and boiled (100°C, 5 min). Before loading, the samples were cleared by centrifugation (15,000 × g, 5 min). For analysis of protein stability, cultures were grown in LB at 37°C with shaking to a OD600 of 0.6–0.8. Chloramphenicol (40 μg ml−1) was added, and the culture was divided into two types: the DOC sample, to which 25% of DOC was added to reach a final concentration of 5%, and the LB sample, to which LB was added to equalize the volume with the DOC sample. A 1 ml aliquot was removed as zero-time control, and the samples were further incubated at 37°C without shaking. One-milliliter samples were extracted after 10, 20, 30, 45, 60, 75, and 90 min and treated as indicated above. Proteins were resolved by Tris-Tricine-PAGE (12%) electrophoresis and transferred onto polyvinylidene-difluoride membranes using a semidry electrophoresis transfer apparatus (Bio-Rad). Specific proteins were detected with anti-Flag M2 monoclonal antibody (1:5,000, Sigma-Aldrich) and anti-GroEL polyclonal antibody (1:20,000; Sigma-Aldrich). Goat anti-mouse horseradish peroxidase-conjugated antibody (1:5,000; Bio-Rad) or goat anti-rabbit horseradish peroxidase-conjugated antibody (1:20,000; Santa Cruz Biotechnology) were used as secondary antibodies. Proteins recognized by the antibodies were visualized by chemoluminiscence using the luciferin-luminol reagents (Thermo Scientific) in a LAS 3000 Mini Imaging System (Fujifilm). For quantification, the intensity of the hybridization bands was determined using MultiGauge software (Fujifilm). GroEL was used as loading control.
RNA Extraction and Northern Blot Analysis
Aliquots from exponential cultures in LB and LB + 5% DOC were centrifuged at 16,000 × g, 37°C, during 2 min, and washed twice with 500 μl of NaCl 0.9%. The pellets were resuspended in 100 μl of a solution of lysozyme (Sigma-Aldrich), 3 mg ml−1. Cell lysis was facilitated by freezing at −20°C for >2 h. After lysis, RNA was extracted using 1 ml of TRIsure reagent (Bioline). Lastly, total RNA was resuspended in 25 μl of RNase-free water. The quality of the preparation and the RNA concentration were determined using a ND-1000 spectrophotometer (NanoDrop Technologies).
For Northern blot analysis, 10 μg of total RNA was loaded per well and electrophoresis was performed in denaturing 1% agarose-formaldehyde gels. Vacuum transfer and fixation to Hybond-N+ membranes (GE Healthcare) were performed using 0.05 M NaOH. UV crosslinking was used to immobilize RNAs on the membrane. For mRNA stability experiments, rifampicin (500 mg ml−1) was added to exponential cultures grown in LB at 37°C (zero-time control). At this point, the culture was divided into two types: the DOC sample, to which 25% of DOC was added to reach a final concentration of 5%, and the LB sample, to which LB was added to equalize the volume with the DOC sample. Incubation was continued, and culture aliquots were taken at appropriate times. DNA oligonucleotides (Racer2-zapB for zapB mRNA and 5S-probe for the loading control) were labeled with [32P]-γ-ATP using T4 polynucleotide kinase (Biolabs). Labeled probes were purified over microspin G-25 columns (GE Healthcare) to remove unincorporated nucleotides. As a control of RNA loading and transfer efficiency, the filters were hybridized with an oligoprobe for the 5S rRNA. Membranes were hybridized with oligoprobes at 42°C. Signals were visualized with a FLA-5100 image system (Fujifilm), and quantification was performed using MultiGauge software (Fujifilm).
5' Rapid Amplification of cDNA Ends
RNA was isolated from S. enterica stationary cells (DO600 ~2) grown in LB. Fifteen microgram of RNA was used to determine cDNA ends using a standard protocol. RNAs were prepared either with or without tobacco acid pyrophosphatase (TAP) to distinguish primary transcript 5' ends from internal 5' processing sites. The sequences of the primers are listed in Supplementary Table S1. The DNA primer for cDNA synthesis, Racer2-zapB, is complementary to zapB RNA. A second DNA primer for subsequent PCR amplification of cDNAs, Racer-out, is homologous to the adaptor RNA primer Racer-RNA-adaptor used for 5' rapid amplification of cDNA ends (5'-RACE). PCR products detected both with and without TAP treatment were further amplified with the internal primers Racer-nested and Racer1-zapB, and purified. PCR products were cloned on pGEM-T Easy (Promega), and 29 clones from two independent assays were sequenced.
Site-Directed Mutagenesis of zapB and micA
Introduction of point mutations into MicA sRNA was performed by PCR using as template the wild type micA gene cloned on pBR328. Two pairs of primers were employed: MicA5101319-FOR and micAREVSalI; and MicA5101319-REV and micAFORBamHI. MicA5101319-FOR and MicA5101319-REV are complementary primers that introduce the point mutations in the gene, while micAREVSalI and micAFORBamHI introduce ad hoc restriction sites. The resulting DNA fragments were ligated into one using Gibson assembly (New England Biolabs, Ipswich, MA, United States). The fragment was digested with SalI and BamHI and cloned onto pBR328. The plasmid containing substitutions in MicA sRNA (5, G→C; 8, G→C; 13, U→A; and 19, A→U) was transformed into the zapB + 18::lacZ background to yield strain SV9486 (zapB + 18::lacZ pBR328-MicA*).
To insert point mutations into the chromosomal zapB gene, PCR was performed as described for MicA, using the pairs of primers For-zapB(MicA)-50,545,661 and zapB-XbaI-REV; and Rev-zapB(MicA)-50,545,661 and zapB-SacI-FOR. As above, ligation by Gibson assembly was followed by digestion and cloning onto pDMS197. The strains carrying pMDS197 derivatives were used as donors in matings with the S. enterica strain SV9265 (which harbors a KmR cassette replacing the zapB coding sequence) as recipient. TcR transconjugants were selected on M9 plates supplemented with tetracycline. Several TcR transconjugants were grown in nutrient broth containing 5% sucrose. Individual tetracycline-sensitive segregants were then screened for kanamycin sensitivity and examined for the incorporation of the mutant zapB allele by DNA sequencing using external oligonucleotides (zapB-E1 and zapB-E2). The resulting mutant (SV9647, zapB*50A54T58A61G) contained four substitutions (50: U→A, 54: A→U, 58: U→A, and 61, C→G) that restored complementation with mutant MicA.
In vitro Transcription and Gel Mobility Shift Assay
DNA templates for in vitro transcription of zapB mRNA and MicA sRNA were generated by PCR using chromosomal DNA from both the wild type and a zapB mutant (SV9647), and plasmid DNA from strains containing wild type and mutant variants of sRNA MicA cloned onto pBR328. The primers used were Transc-zapB-FOR and Transc-zapB-REV, and Transc-micA-FOR and Transc-micA-REV (Supplementary Table S1). The PCR products were purified with the Wizard® SV Clean-Up System (Promega). For the in vitro transcription reaction, the HiScribe T7 High Yield RNA Synthesis kit was employed. Two sets of reactions were prepared: (i) for synthesis of non-labeled zapB transcripts, the zapB PCR product was mixed with equimolar concentrations of all four ribonucleotides (10 mM) and (ii) for synthesis of internally labeled MicA sRNA and zapB mRNA, each PCR product was mixed with ATP, GTP, and CTP 10 mM and 0.4 mM of UTP. Radioactive labelling was performed by adding 3 μl of EasyTide® uridine 5'-triphosphate (α-32P) 3,000 Ci mmol−1 (PerkinElmer) to a final concentration of 0.25 μM. One microliter of T7 RNA Polymerase Mix and 4 μl of Master Mix were added to each reaction tube. Nuclease-free water was added to a final volume of 40 μl. The reactions were then incubated at 37°C for 2 h and treated with DNase (TURBO DNase Ambion 2 U μl−1) for 15–20 min. To discard unincorporated nucleotides, the reactions were passed through GE Healthcare ilustra™ MicroSpin™ G-25 columns. Subsequently, the RNA transcripts (both labeled and unlabelled) were purified by electrophoresis on a 7.9 M urea, 8% polyacrylamide denaturing gel. The hybridization fragments were visualized using flour-coated TLC plates (Ambion). Gel slices were crushed, and RNA was eluted overnight at 4°C with crush-soak solution (200 mM NaCl, 10 mM Tris-HCl, and 10 mM EDTA). The RNA was ethanol-precipitated and resuspended in nuclease-free water.
Gel mobility shift assays were performed with 0.015 pmol of [α-32P]-labeled wild type or mutant MicA (MicA*) RNA, and 0.075 pmol of unlabelled zapB mRNA transcript in 1× binding buffer (200 mM Tris-HCl pH 8, 10 mM DTT, 10 mM MgCl2, 200 mM KCl, and 100 mM Na2HPO4-NaH2PO4) at 37°C. The reactions were incubated, and samples were taken at 10, 30, 60, and 100 min. The binding reactions were mixed with 2 μl of loading dye (48% glycerol and 0.01% bromophenol blue) and subjected to electrophoresis at room temperature in a 6% non-denaturing polyacrylamide Tris-borate EDTA (TBE) gel for 2 h at 55 V, 15 mA in 1x TBE buffer (90 mM Tris-borate/2 mM EDTA). In parallel to the binding reactions, a positive control was prepared by mixing zapB mRNA and [α-32P]-labeled MicA (mutant and wild type) followed by boiling denaturation and renaturation at 37°C. Single [α-32P]-labeled zapB RNA and MicA were also loaded on the gel. After electrophoresis, gels were dried and analyzed in a FLA-5100 Scanner (Fujifilm).
Statistical Analysis
Student’s t-test (unpaired) was performed using GraphPad Prism version 8.0 for Mac to determine the statistical differences between two groups. Ordinary one-way ANOVA was also performed using GraphPad Prism.
Results
The ZapB and ZapA Proteins of S. enterica Are Necessary for Bile Resistance
The zapB gene of E. coli encodes an 81-amino-acid protein that acts as a non-essential cell division factor involved in Z-ring assembly (Adams and Errington, 2009). The name zapB is for “Z ring-associated protein B” (Ebersbach et al., 2008). Alignment of the amino acid sequences of the ZapB protein of E. coli and the predicted protein encoded by the yiiU locus of Salmonella enterica reveals a 91% identity value. For this reason, the S. enterica yiiU locus will be henceforth named zapB.
Minimal inhibitory concentration (MIC) analysis was performed to determine whether the S. enterica ZapB protein is necessary for bile resistance. A null ZapB− mutant (SV6269) was found to be sensitive to DOC (Table 2). The conclusion that ZapB is necessary for bile resistance was confirmed by complementation analysis with plasmid-borne E. coli and S. enterica zapB genes expressed from the arabinose-inducible promoter of pBAD18 (Table 2).
The observation that a ZapB− mutant is sensitive to bile raised the possibility that lack of another Z-ring assembly modulator, ZapA, might also cause bile sensitivity. A bioinformatic search followed by Clustal W alignment revealed that the ygfE gene of S. enterica encodes a protein with 88% amino acid identity with E. coli ZapA. YgfE was thus renamed ZapA. Disruption of the zapA gene caused sensitivity to DOC, and complementation tests using plasmid-borne E. coli and S. enterica zapA genes restored bile resistance (Table 2).
The MIC of DOC for a zapA zapB mutant was similar or identical to that of a zapB mutant, an epistatic effect which suggests that bile sensitivity in the absence of either ZapA or ZapB results from disruption of the same cellular process.
Expression of zapB Is Upregulated in the Presence of Bile
The levels of zapA and zapB mRNAs in LB and LB + DOC were monitored by qPCR. Relative RNA levels, averaged from six independent experiments, were as follows: zapA, 1.00 (arbitrary) in LB and 1.21 ± 0.36 in LB + DOC; and zapB, 2.09 ± 0.49 in LB and 8.80 ± 1.78 in LB + DOC. These experiments confirmed previous observations indicating that expression of zapB (but not of zapA) increases in the presence of bile (Hernandez et al., 2012). Further work was centered on zapB only.
The ZapB Protein Is Degraded by the Lon Protease in the Presence of Bile
The stability of the ZapB protein in the presence and in the absence of DOC was analyzed by Western blot analysis using a ZapB version tagged with the 3 × FLAG epitope (ZapB::3 × FLAG). Note that the strain carrying this ZapB variant is resistant to DOC and the protein may be, therefore, considered wild type (Table 2). The amount of ZapB protein detected in LB + DOC was lower than in LB (Figure 1A), suggesting that ZapB might be degraded in the presence of DOC. To investigate this possibility, we carried out protein stability assays to monitor the half-life of the ZapB::3 × FLAG protein upon addition of DOC. As shown in Figures 1B,C, ZapB is degraded faster in LB + DOC (half-life around 30 min) than in LB (half-life >90 min).
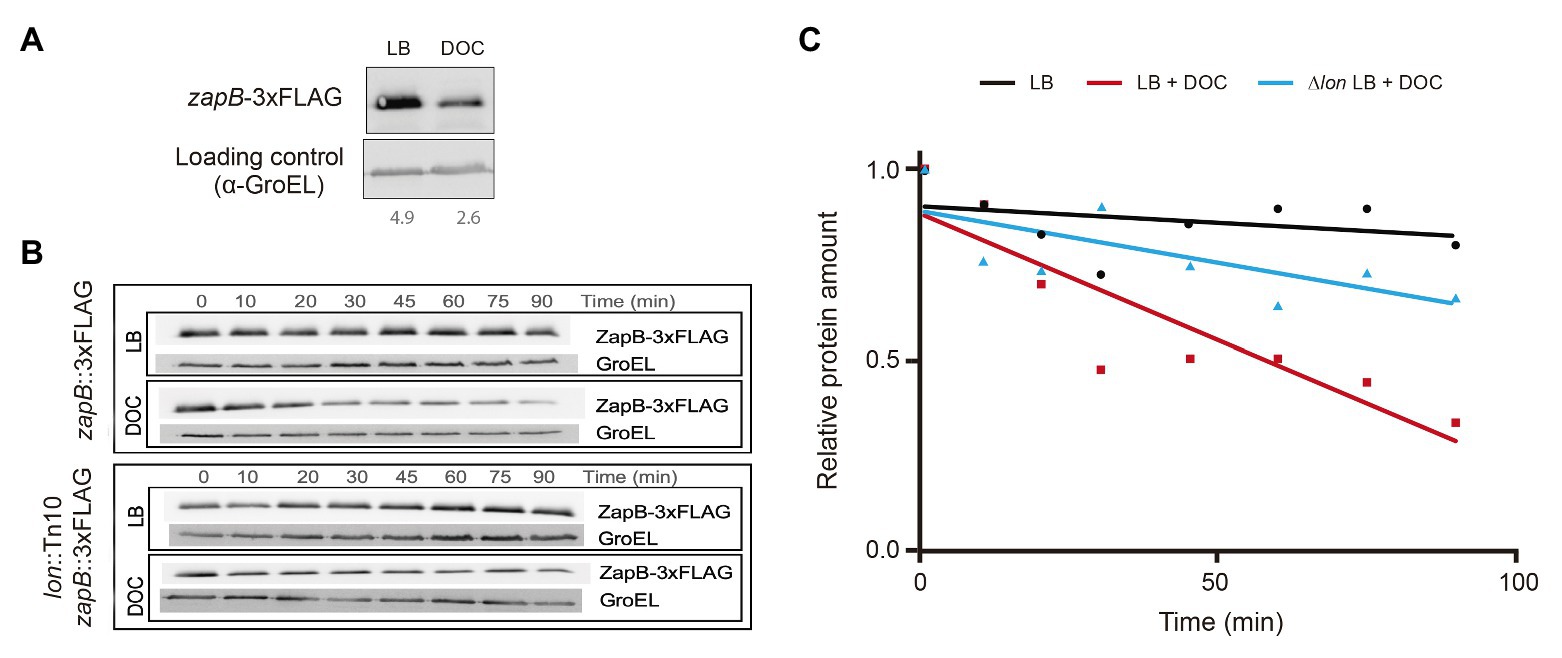
Figure 1. Degradation of ZapB protein by the Lon protease in the presence of DOC. (A) Western blot analysis of the levels of ZapB-3 × FLAG protein in lysogeny broth (LB) and LB + 5% DOC. (B) Stability of the ZapB-3 × FLAG protein in exponential cultures grown in LB and LB + 5% DOC in wild type and Lon− backgrounds. Aliquots were extracted 10, 20, 30, 45, 60, 75, and 90 min after addition of DOC and chloramphenicol. (C) Graphical representation of the data of panel B, showing the stability of the ZapB-3 × FLAG protein in LB and LB + DOC and the effect of a lon mutation.
Because the Lon protease is involved in the degradation of unfolded and misfolded proteins (Tsilibaris et al., 2006; Gur et al., 2011) and bile salts cause protein misfolding and denaturation (Bernstein et al., 1999; Leverrier et al., 2003), the stability of ZapB protein was monitored in a Lon− background (strain SV7017). In the absence of Lon protease, the ZapB stability in LB + DOC increased (Figures 1B,C). Hence, we tentatively conclude that the Lon protease may degrade ZapB in the presence of DOC.
Postranscriptional Regulation of zapB
To investigate the mechanism underlying zapB upregulation in the presence of DOC, the transcriptional start site of the zapB gene was identified by 5'-RACE. Twenty-four out of twenty-nine clones analyzed from two independent 5'-RACE assays identified a 5'-end A residue located 56 nucleotides upstream of the zapB translational start codon. In silico analysis of the region revealed the occurrence of nucleotide sequences compatible with the consensus sequences of −35 and −10 modules in σ70-dependent promoters, and with appropriate spacing (Collado-Vides, 1996). The proposed structure of the zapB promoter is presented in Figure 2A.
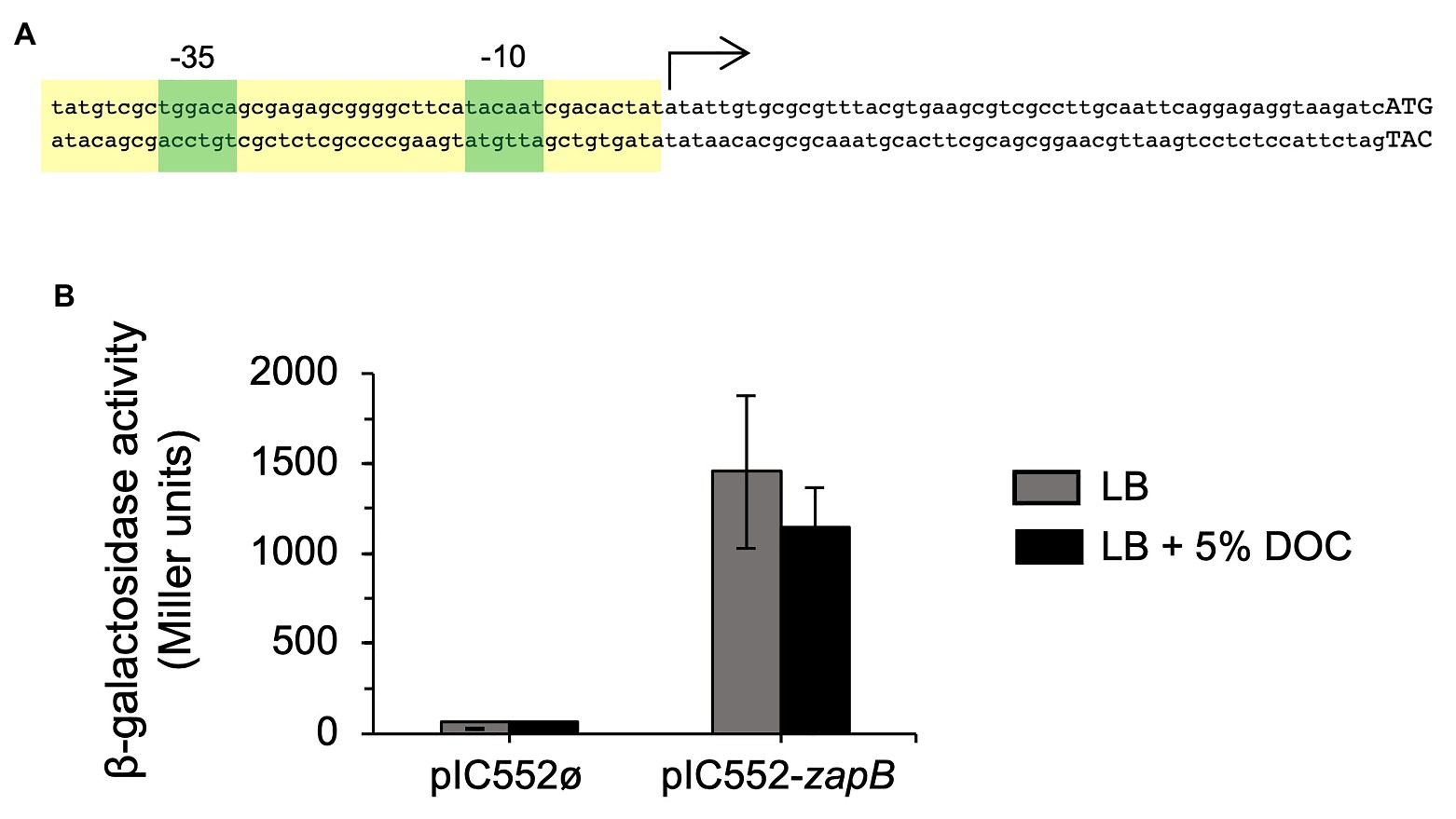
Figure 2. Identification of the zapB promoter. (A) Diagram of the putative promoter region of zapB. The transcription initiation site (arrow), and the putative −35 and −10 modules (green boxes) are shown. Capital letters are nucleotides of the putative zapB coding sequence. The region that was cloned on the promoter-probe vector pIC552 to generate a transcriptional fusion with the lacZ gene is outlined in yellow. (B) β-Galactosidase activities of strain SV7339 (carrying the pIC552-zapB derivative) in LB and in LB + 5% sodium DOC. Strain SV7338 (carrying the empty vector) was included as control. Data are averages and SD from three independent experiments.
To confirm the existence of the predicted zapB promoter, the putative promoter region was cloned on the promoter-probe vector pIC552 (Macian et al., 1994) to generate a transcriptional fusion with the lacZ gene. The pIC552 derivative bearing the zapB promoter was introduced into the wild type strain, and β-galactosidase activity measurements confirmed that the cloned region was able to drive lacZ expression. Interestingly, transcription of the pIC552-borne pzapB-lacZ gene was not regulated by DOC (Figure 2B). This observation raised the possibility that upregulation of zapB expression in the presence of DOC might be postranscriptional.
Upregulation of zapB mRNA in the presence of bile was confirmed by β-galactosidase assays using a translational zapB::lacZ fusion and by Northern blot analysis (Figures 3A,B). When the translational zapB::lacZ fusion was placed under the control of a heterologous promoter, pLtetO (Lutz and Bujard, 1997), increased zapB expression was still observed in the presence of DOC (Figure 3C). This observation was in agreement with the above observation that the zapB promoter cloned on pIC552 was not responsive to DOC (Figure 2B), leading us to conclude that DOC-dependent regulation of zapB is not transcriptional.
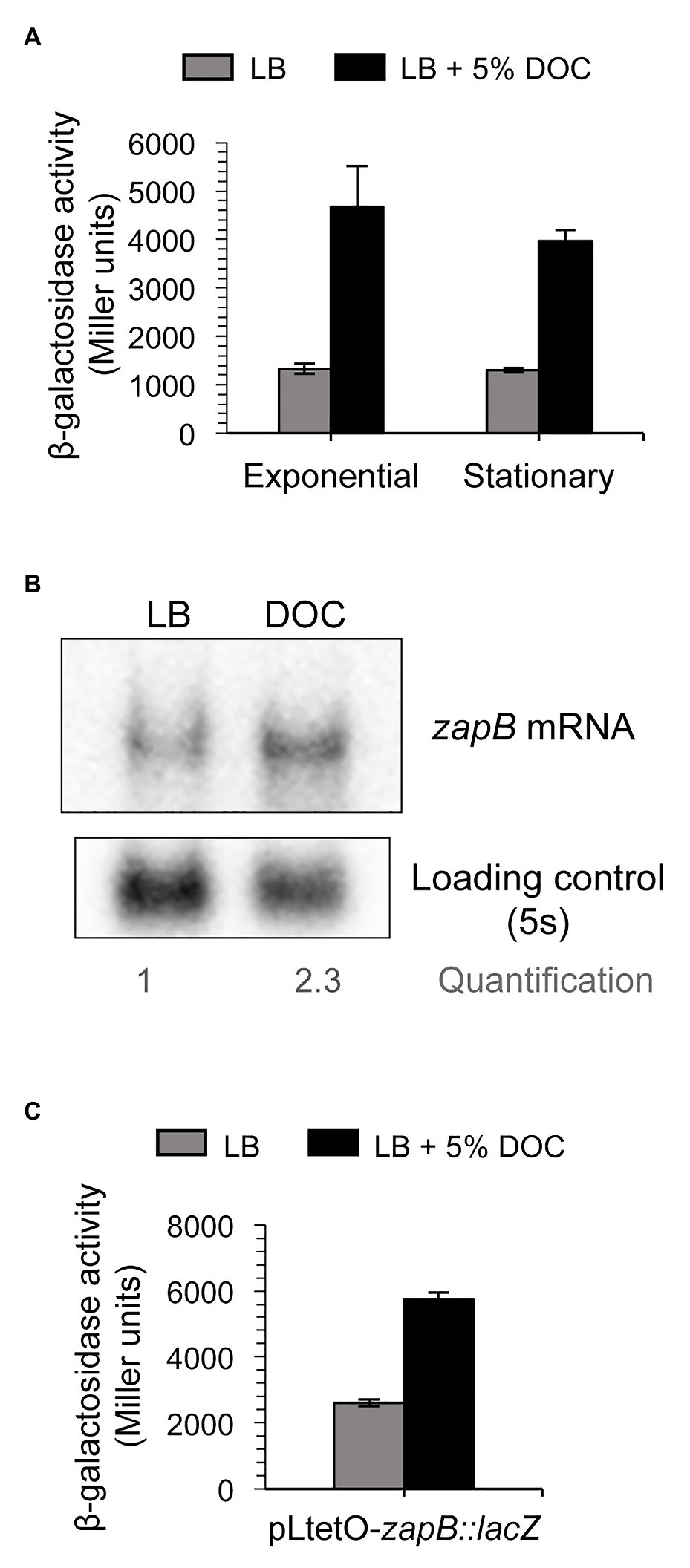
Figure 3. Evidence for postranscriptional control of zapB. Regulation of zapB expression by bile. (A) β-Galactosidase activity of the translational zapB::lacZ fusion of strain SV6270 in exponential and stationary cultures in LB (gray histograms) and LB + 5% DOC (black histograms). (B) Northern blot analysis of the levels of zapB mRNA in extracts from stationary cultures in LB and LB + 5% DOC. (C) β-Galactosidase activity of a zapB::lacZ fusion under the control of the pLtetO promoter (strain SV7429) in LB (gray histograms) and LB + 5% DOC (black histograms).
Bile Stabilizes zapB mRNA by a Mechanism Involving Hfq
Evidence for postranscriptional upregulation of zapB in the presence of bile prompted a comparison of the stability of the zapB transcript in LB and in LB + DOC. For this purpose, Northern blot analysis was performed. Decay of zapB mRNA was faster in LB than in LB + DOC (Figure 4), suggesting that the higher amount of zapB mRNA found in the presence of DOC might result from increased mRNA stability.
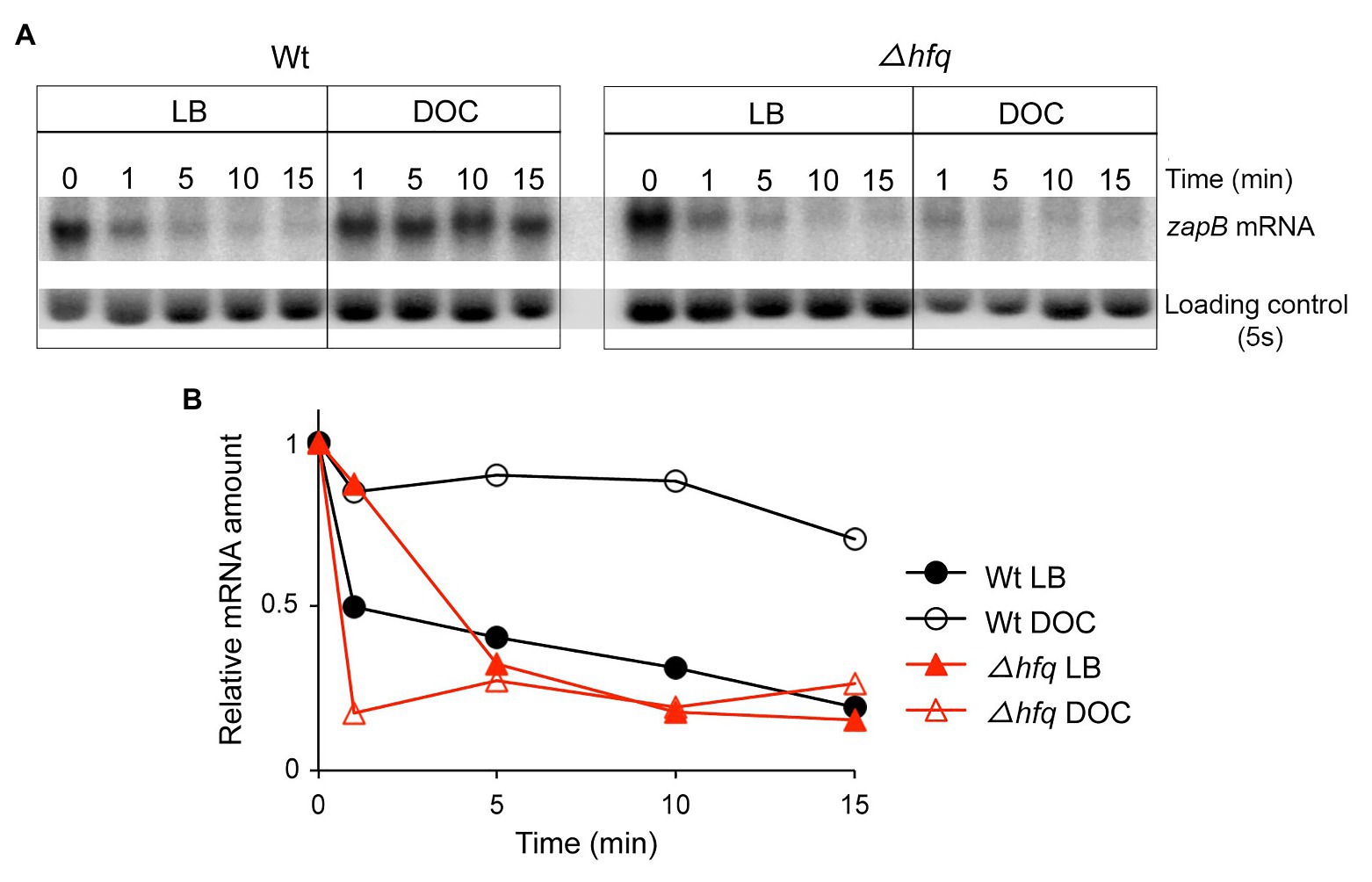
Figure 4. Stability of zapB mRNA. (A) Levels of zapB mRNA in RNA extracts from exponential cultures in LB and LB + 5% DOC. Samples were taken 1, 5, 10, and 15 min after addition of rifampicin. (B) Quantification of the level of zapB mRNA relative to the loading control and to time zero.
Among the mechanisms that control bacterial mRNA stability, interaction with small regulatory RNAs (sRNAs) is common (Storz et al., 2004, 2011; Caron et al., 2010; Podkaminski and Vogel, 2010; Prevost et al., 2011). Hence, we considered the possibility that the stability of zapB mRNA might be controlled by a sRNA, either destabilizing the transcript in LB or stabilizing the transcript in the presence of DOC. Because most bacterial small regulatory RNAs require the Hfq chaperone for interaction with the target and for stability of the sRNA itself (Valentin-Hansen et al., 2004; McCullen et al., 2010; Soper et al., 2011), we analyzed the stability of zapB mRNA in an Hfq− strain using Northern blotting (Figure 4). In the Hfq− background, the stability of the zapB transcript with and without DOC was similar to the stability of zapB mRNA in the wild type strain grown in LB, suggesting that stabilization of zapB mRNA in the presence of DOC requires the Hfq RNA chaperone (Figures 4A,B).
Identification of the zapB mRNA Target Region Involved in Postranscriptional Control
To identify the hypothetical zapB mRNA region that might be subjected to postranscriptional control, we constructed a set of translational fusions inserting the lacZ gene at different locations within the zapB coding sequence: 9, 18, 27, 30, and 66 nucleotides after the start codon (Supplementary Figure S1). Because 3'UTRs are often targets for sRNA binding (Opdyke et al., 2004; Papenfort and Vanderpool, 2015), we also constructed a strain in which the 3'UTR of zapB was deleted downstream of the zapB::lacZ fusion (Supplementary Figure S1). Measurement of β-galactosidase activities upon growth in LB and in LB + DOC provided the following observations:
1. The zapB::lacZ fusion that conserves only nine nucleotides of the zapB coding region showed reduced expression in both LB and LB + DOC (Figure 5A) and was not regulated by DOC.
2. Deoxycholate-dependent regulation was observed in the remaining lacZ fusions (Figure 5A), including the strain that maintains 18 nucleotides of the zapB coding region, suggesting that the upstream (5') 18 nucleotides of the zapB mRNA coding region are required for regulation by DOC.
3. The 3'UTR was found to be dispensable for zapB regulation by DOC (Figure 5A).
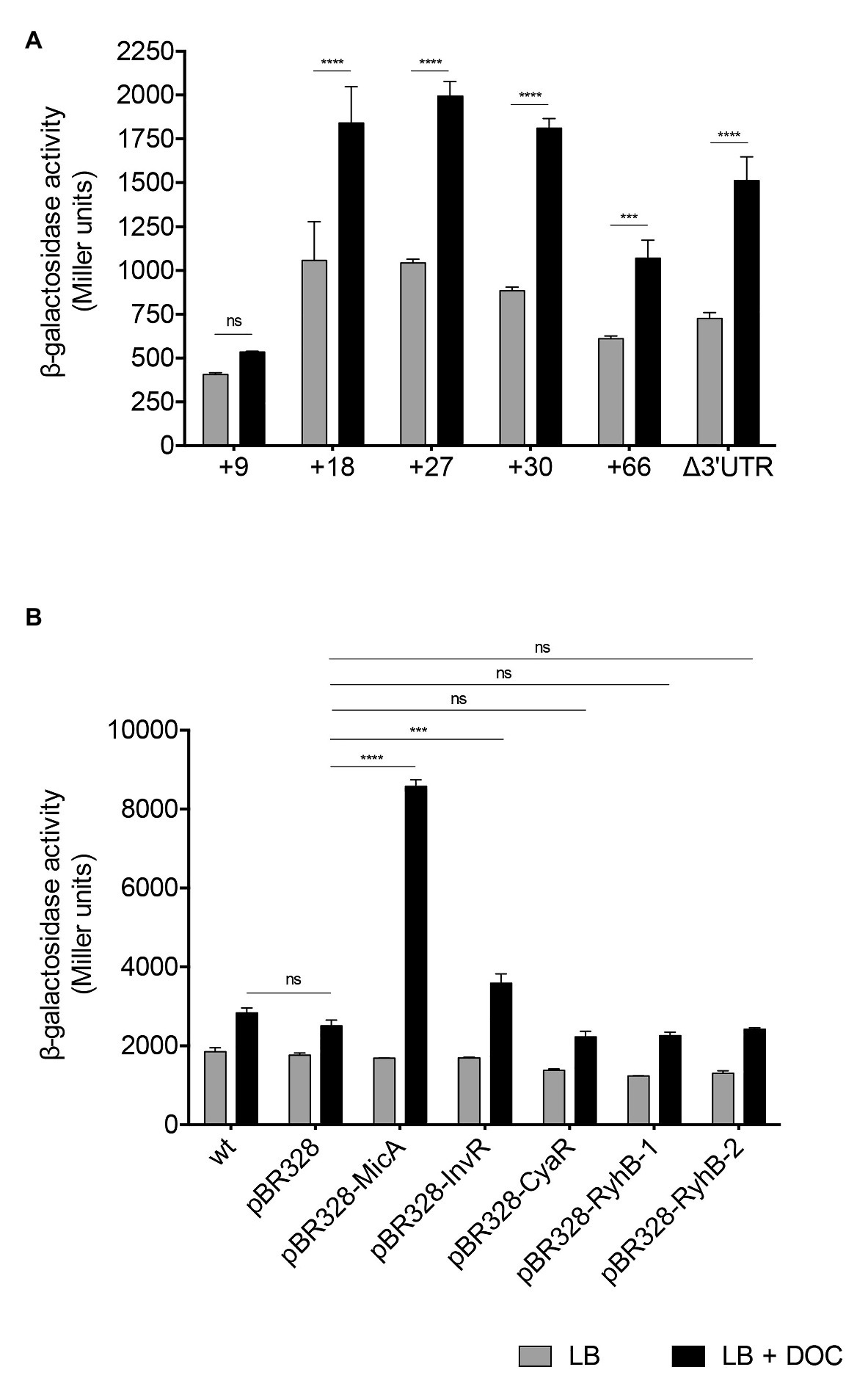
Figure 5. Identification of a zapB region involved in regulation by DOC. (A) β-Galactosidase activities of zapB::lacZ fusions inserted 9, 18, 27, 30, and 66 nucleotides downstream of the start codon of the zapB coding sequence, and of a zapB::lacZ fusion lacking the 3'UTR of the zapB transcript. (B) β-Galactosidase activities of zapB::lacZ fusions in strains overexpressing the sRNAs MicA, InvR, CyaR, RyhB-1, and RyhB-2 cloned onto the pBR328 plasmid. As controls, the zapB::lacZ strain (SV6270, named wild type) and a strain carrying the empty vector were assayed. The strains were grown in LB (gray histograms) and in LB + 5% DOC (black histograms). Statistical indications: ns, not significantly different; significantly different, ****p < 0.001; ***p < 0.01.
Altogether, the above observations provide evidence that the 5'UTR and the upstream (5') region of the zapB coding sequence (CDS) may be the main (perhaps the only) region of zapB mRNA involved in postranscriptional control by DOC.
Search for Small Regulatory RNA(s) That Interact With zapB mRNA
We did not rule out the possibility that Hfq binding might be sufficient to stabilize zapB mRNA. However, this possibility seemed unlikely as Hfq often acts in concert with sRNAs (Basineni et al., 2009; Chao and Vogel, 2010; Soper et al., 2010; Storz et al., 2011). A computational search for potential interactions between Salmonella sRNA candidates and zapB mRNA using the IntaRNA program (Smith et al., 2010) identified sRNAs with potential targets in zapB mRNA (Supplementary Table S2). Such sRNA candidates were shortlisted using two criteria: (i) the potentially interacting mRNA sequence should be located in the 5' region of the zapB transcript and (ii) the potentially interacting sRNA should be regulated by bile. These criteria were met by five candidates: MicA, InvR, CyaR, RyhB-1, and RyhB-2, previously reported to be upregulated in the presence of bile (Kroger et al., 2013).
MicA, InvR, CyaR, RyhB-1, and RyhB-2 sRNAs were cloned onto pBR328, and the resulting plasmids were transformed into a Salmonella strain harboring a zapB::lacZ fusion. β-Galactosidase activities in LB and in LB + DOC were then determined (Figure 5B). The presence of DOC resulted in higher β-galactosidase activities when the sRNAs MicA and InvR were overexpressed, and the MicA effect was especially strong. The other sRNAs tested in the assay did not show any effect. These results suggest that MicA may be involved in stabilization of zapB mRNA in the presence of DOC.
Interaction Between zapB mRNA and MicA
In silico analysis with the IntaRNA tool (Smith et al., 2010) predicts that MicA might interact with zapB mRNA by base pairing at a zapB mRNA region that contains a putative ribosome binding site (5'GAGG3') and the AUG start codon of the coding sequence (Figure 6A). To probe the predicted interaction, point mutations were introduced in MicA sRNA by site-directed mutagenesis: 5, G→C; 8, G→C; 13, U→A; and 19, A→U. The mutant version of micA (micA*) was then cloned on pBR328 and transformed into a strain carrying a zapB::lacZ fusion. Expression of zapB::lacZ in LB and in LB + DOC was compared with that of a strain that overexpressed wild type MicA. β-Galactosidase activity analysis indicated that the upregulation of the zapB transcript detected upon MicA overexpression disappeared when MicA was mutated (Figure 6B). Actually, the β-galactosidase activity in the presence of MicA* was similar to that of the control harboring the empty vector (Figure 6B). These observations suggest that the mutations introduced into MicA had disrupted the interaction with zapB mRNA, thus supporting the existence of a zapB mRNA:MicA sRNA interaction at the region predicted by bioinformatic analysis.
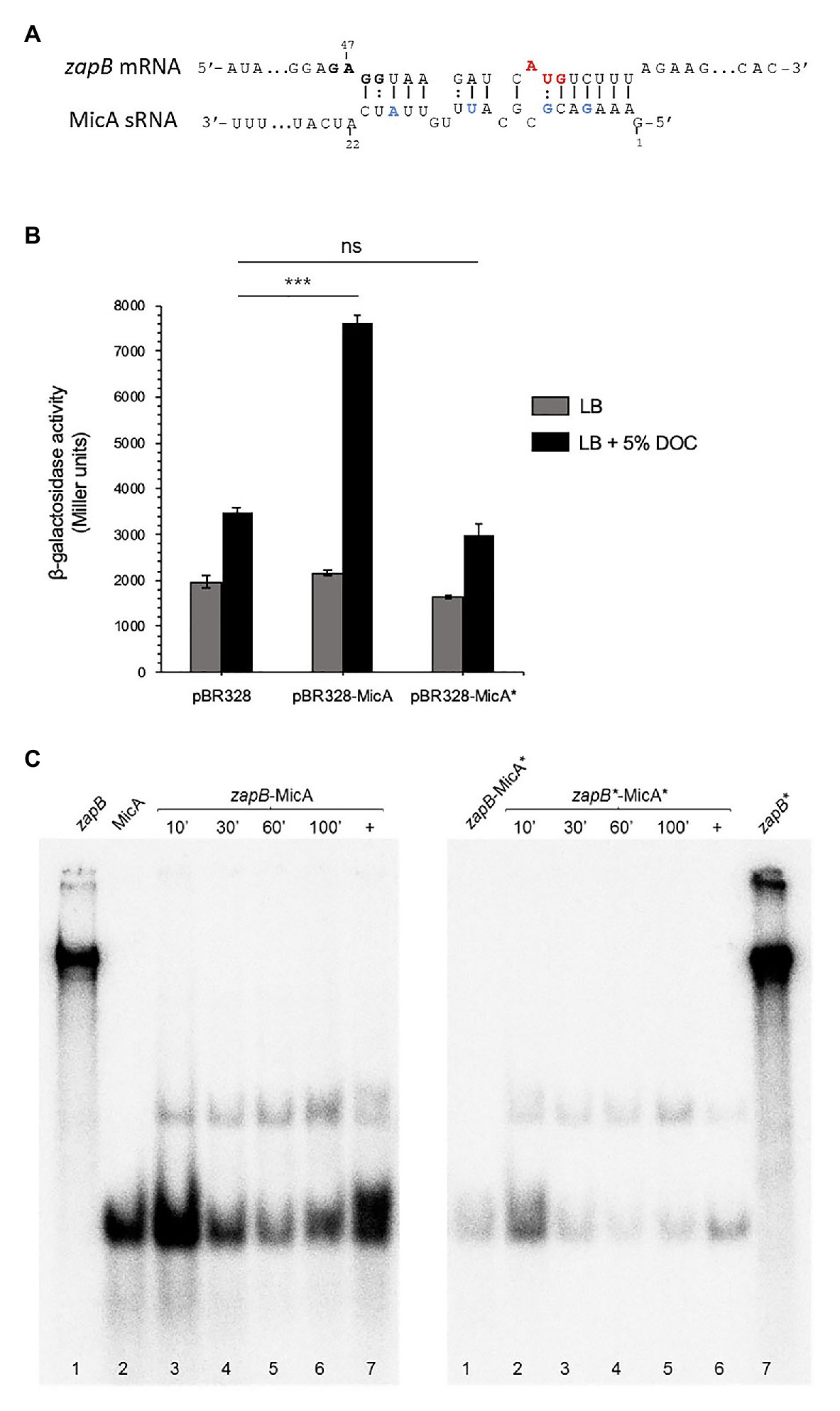
Figure 6. zapB-MicA interaction. (A) Diagram of putative interacting regions in sRNA MicA and zapB mRNA predicted by IntaRNA. The putative ribosome binding site and start codon are outlined (bold and red, respectively). MicA nucleotides subjected to site-specific mutagenesis are outlined in blue. (B) β-Galactosidase activities of zapB::lacZ fusions in strains overexpressing either MicA or MicA*. As controls, a zapB::lacZ strain (SV9372, “wild type”) and a strain carrying the empty vector were included. The strains were grown in LB (gray histograms) and in LB + 5% DOC (black histograms). (C) Gel mobility shift assay (EMSA) showing in vitro interactions between zapB-MicA and zapB*-MicA*. Samples were taken at 10, 30, 60, and 100 min. Lanes 1 and 2 in the left panel and lane 7 in the right panel show labeled zapB, MicA, and zapB* individual samples, respectively. As positive controls (+), zapB and MicA RNAs were treated to force association (lane 7, left and lane 6, right). As negative control, a mix of wild type zapB mRNA with mutant MicA (zapB-MicA*) was included (lane 1, right). Statistical indications: ns, not significantly different; significantly different, ***p < 0.01.
Further evidence for zapB mRNA:MicA sRNA interaction was obtained in vitro. An electrophoretic mobility shift assay (EMSA) was carried out using wild type and mutant versions of both zapB mRNA and MicA sRNA, the latter labeled with [α-32P] (Figure 6C). The mutations introduced in zapB*, the mutant version of zapB mRNA (50: U→A, 54: A→U, 58: U→A, and 61, C→G) were complementary to those present in MicA*, so that the interaction predicted by IntaRNA could be restored. Binding was detected for both zapB mRNA:MicA and zapB* mRNA:MicA*, and the fact that binding was largely independent from the incubation time may suggest a strong interaction (Figure 6C). Altogether, the experiments shown in Figures 5, 6 support the following tentative conclusions: (i) zapB mRNA and MicA sRNA do interact; (ii) the main (perhaps only) interaction involves the upstream region of zapB mRNA; and (iii) the zapB:MicA interaction increases zapB expression in the presence of DOC, probably by MicA-mediated stabilization of zapB mRNA.
Discussion
Initial evidence that the Z-ring might be involved in bile resistance was provided by transcriptomic analysis: the zapB gene, which in Salmonella was still annotated as a locus of unknown function (yiiU), was found to be upregulated in the presence of a sublethal concentration of DOC (Hernandez et al., 2012). Disruption of the yiiU locus causes sensitivity to DOC (Table 1), indicating that yiiU is not merely a bile-induced locus but a gene necessary for bile resistance. Lack of the S. enterica ygfE gene product likewise causes bile sensitivity (Table 1). Change of the ygfE and yiiU gene designations to zapA and zapB is supported by the 88 and 91% identity between the predicted Salmonella and E. coli gene products, respectively. Unlike zapB, the S. enterica zapA gene is not upregulated by bile.
Upregulation of S. enterica zapB expression in the presence of DOC is still observed when zapB transcription is driven by a heterologous promoter (Figure 3C), thereby suggesting the involvement of a postranscriptional mechanism. Comparison of zapB mRNA decay in LB and LB + 5% DOC reveals that zapB mRNA is more stable in the presence of DOC, thus explaining the higher mRNA level detected both in the initial transcriptomic analysis (Hernandez et al., 2012) and in Northern blots (Figures 3B, 4). Stabilization of the zapB transcript in the presence of DOC requires the Hfq RNA chaperone (Figure 4), an effect that admits two alternative explanations: (i) Hfq binding might protect zapB mRNA from degradation and (ii) Hfq might catalyze the interaction of zapB mRNA with a small regulatory RNA, and the mRNA:sRNA interaction might increase zapB mRNA stability.
Construction of lacZ fusions in truncated forms of the zapB transcript defined the 5'UTR and the upstream (5') 18 nucleotides of the coding sequence as a region necessary for upregulation in the presence of bile salts (Figure 5A). This interpretation is in agreement with the fact that activating sRNAs often target upstream mRNA regions (Papenfort and Vanderpool, 2015).
In silico search for sRNAs that might interact with zapB mRNA by base pairing provided the candidates listed in Supplementary Table S2. Upregulation in the presence of bile (Kroger et al., 2013) and putative interaction at the 5' region of zapB narrowed the number of candidate sRNAs down to five: CyaR, InvR, MicA, RyhB-1, and RyhB-2. When overexpression of these sRNAs was tested, MicA conspicuously increased the β-galactosidase activity of a zapB::lacZ fusion in the presence of DOC (Figure 5B). Occurrence of a zapB mRNA:MicA sRNA interaction was further supported by the existence of a potential base pairing region (Figure 6A) and by the fact that introduction of point mutations in MicA abolished zapB::lacZ upregulation in the presence of DOC (Figure 6B). Interaction between zapB mRNA and MicA sRNA was also detected in vitro (Figure 6C). Furthermore, site-directed mutagenesis of MicA disrupted the interaction, which was restored upon introduction of compensatory mutations in zapB mRNA (Figure 6C).
MicA is a small regulatory RNA that participates in the bacterial envelope stress response mediated by the sigma factor RpoE (Figueroa-Bossi et al., 2006). MicA binds omp mRNAs accelerating their decay and enabling outer membrane remodeling upon envelope stress (Papenfort et al., 2006; Bossi and Figueroa-Bossi, 2007). Identification of additional mRNA targets has linked MicA to the phoPQ regulon and to additional functions (Coornaert et al., 2010; Kint et al., 2010; Gogol et al., 2011; Van Puyvelde et al., 2015). Our observation that MicA protects the zapB transcript from degradation in the presence of DOC departs from previous examples of MicA-mediated regulation, which involved stimulation of mRNA turnover (Papenfort et al., 2006; Bossi and Figueroa-Bossi, 2007). This difference is further emphasized by the observation that the region of MicA involved in protection of zapB mRNA (nt 2–21) overlaps with regions involved in MicA-mediated turnover in other mRNAs: 1–22 in ompX (Gogol et al., 2011) and 8–24 in ompA (Udekwu et al., 2005). These mechanistic differences may seem paradoxical. However, MicA-mediated stabilization of zapB mRNA in the presence of bile is anything but paradoxical: a known role of MicA in the bacterial cell is protection from envelope stress (Figueroa-Bossi et al., 2006; Papenfort et al., 2006; Bossi and Figueroa-Bossi, 2007). On the other hand, transcript stabilization upon interaction with the mRNA 5' region has been described for other sRNAs (Papenfort and Vogel, 2009; Storz et al., 2011).
Evidence that ZapB is degraded by the Lon protease in the presence of DOC is presented in Figure 1. Lon degrades abnormally folded proteins (Gottesman, 1996), and bile salts cause misfolding and denaturation of proteins (Bernstein et al., 1999). We thus propose that bile salts may cause ZapB misfolding, and that misfolding may trigger degradation by Lon. If this view is correct, increased zapB mRNA stability in the presence of bile may compensate for Lon-mediated degradation. Stabilization of zapB mRNA may be in turn facilitated by the existence of a higher concentration of MicA in the presence of bile (Kroger et al., 2013).
The epistatic effect of a zapB mutation over a zapA mutation suggests that the ZapA and ZapB gene products participate in the same cellular process. Because ZapA and ZapB are Z-ring assembly modulators (Adams and Errington, 2009), we tentatively propose that perturbation of Z-ring assembly may render the Salmonella cell bile-sensitive. The immediate cause of bile sensitivity in the absence of Z-ring modulators remains unknown. However, it is remarkable that another cell division factor, DamX, is also necessary for bile resistance in both S. enterica (Lopez-Garrido et al., 2010) and E. coli (Arends et al., 2010).
Data Availability Statement
The raw data supporting the conclusions of this article will be made available by the authors, without undue reservation.
Author Contributions
RF-F, SH, VU, and MS-R carried out the experiments. RF-F, SH, EP-F, MS-R, VU, and JC designed the experiments and interpreted results. JC wrote the manuscript. All authors contributed to the article and approved the submitted version.
Funding
This study was supported by grant BIO2016-75235-P from the Spanish Ministerio de Economía, Industria y Competitividad - Agencia Estatal de Investigación and the European Regional Fund. VU was supported by a postdoctoral contract associated to grant CVI-5879 from the Consejería de Innovación, Ciencia y Empresa, Junta de Andalucía, Spain. MS-R was supported by the VI Plan Propio de Investigación y Transferencia de la Universidad de Sevilla.
Conflict of Interest
The authors declare that the research was conducted in the absence of any commercial or financial relationships that could be construed as a potential conflict of interest.
Acknowledgments
We are grateful to Miguel A. de Pedro, Laurent Aussel, and Kai Papenfort for advice and discussions, and to Modesto Carballo, Laura Navarro, and Cristina Reyes of the SGI Biología, CITIUS, Universidad de Sevilla, for help with experiments performed at the facility.
Supplementary Material
The Supplementary Material for this article can be found online at: https://www.frontiersin.org/articles/10.3389/fmicb.2021.647305/full#supplementary-material
Footnotes
References
Adams, D. W., and Errington, J. (2009). Bacterial cell division: assembly, maintenance and disassembly of the Z ring. Nat. Rev. Microbiol. 7, 642–653. doi: 10.1038/nrmicro2198
Alvarez-Ordonez, A., Begley, M., Prieto, M., Messens, W., Lopez, M., Bernardo, A., et al. (2011). Salmonella spp. survival strategies within the host gastrointestinal tract. Microbiology 157, 3268–3281. doi: 10.1099/mic.0.050351-0
Arends, S. J., Williams, K., Scott, R. J., Rolong, S., Popham, D. L., and Weiss, D. S. (2010). Discovery and characterization of three new Escherichia coli septal ring proteins that contain a SPOR domain: DamX, DedD, and RlpA. J. Bacteriol. 192, 242–255. doi: 10.1128/JB.01244-09
Basineni, S. R., Madhugiri, R., Kolmsee, T., Hengge, R., and Klug, G. (2009). The influence of Hfq and ribonucleases on the stability of the small non-coding RNA OxyS and its target rpoS in E. coli is growth phase dependent. RNA Biol. 6, 584–594. doi: 10.4161/rna.6.5.10082
Begley, M., Gahan, C. G., and Hill, C. (2005). The interaction between bacteria and bile. FEMS Microbiol. Rev. 29, 625–651. doi: 10.1016/j.femsre.2004.09.003
Bernstein, C., Bernstein, H., Payne, C. M., Beard, S. E., and Schneider, J. (1999). Bile salt activation of stress response promoters in Escherichia coli. Curr. Microbiol. 39, 68–72. doi: 10.1007/s002849900420
Bossi, L., and Figueroa-Bossi, N. (2007). A small RNA downregulates LamB maltoporin in Salmonella. Mol. Microbiol. 65, 799–810. doi: 10.1111/j.1365-2958.2007.05829.x
Buckley, A. M., Webber, M. A., Cooles, S., Randall, L. P., La Ragione, R. M., Woodward, M. J., et al. (2006). The AcrAB-TolC efflux system of Salmonella enterica serovar Typhimurium plays a role in pathogenesis. Cell. Microbiol. 8, 847–856. doi: 10.1111/j.1462-5822.2005.00671.x
Buss, J., Coltharp, C., Shtengel, G., Yang, X., Hess, H., and Xiao, J. (2015). A multi-layered protein network stabilizes the Escherichia coli FtsZ-ring and modulates constriction dynamics. PLoS Genet. 11:e1005128. doi: 10.1371/journal.pgen.1005128
Bustos, A. Y., Font de Valdez, G., Fadda, S., and Taranto, M. P. (2018). New insights into bacterial bile resistance mechanisms: the role of bile salt hydrolase and its impact on human health. Food Res. Int. 112, 250–262. doi: 10.1016/j.foodres.2018.06.035
Cano, D. A., Pucciarelli, M. G., Garcia-del Portillo, F., and Casadesus, J. (2002). Role of the RecBCD recombination pathway in Salmonella virulence. J. Bacteriol. 184, 592–595. doi: 10.1128/JB.184.2.592-595.2002
Caron, M. P., Lafontaine, D. A., and Masse, E. (2010). Small RNA-mediated regulation at the level of transcript stability. RNA Biol. 7, 140–144. doi: 10.4161/rna.7.2.11056
Chao, Y., and Vogel, J. (2010). The role of Hfq in bacterial pathogens. Curr. Opin. Microbiol. 13, 24–33. doi: 10.1016/j.mib.2010.01.001
Collado-Vides, J. (1996). Towards a unified grammatical model of sigma 70 and sigma 54 bacterial promoters. Biochimie 78, 351–363. doi: 10.1016/0300-9084(96)84767-5
Coornaert, A., Lu, A., Mandin, P., Springer, M., Gottesman, S., and Guillier, M. (2010). MicA sRNA links the PhoP regulon to cell envelope stress. Mol. Microbiol. 76, 467–479. doi: 10.1111/j.1365-2958.2010.07115.x
Crawford, R. W., Keestra, A. M., Winter, S. E., Xavier, M. N., Tsolis, R. M., Tolstikov, V., et al. (2012). Very long O-antigen chains enhance fitness during Salmonella-induced colitis by increasing bile resistance. PLoS Pathog. 8:e1002918. doi: 10.1371/journal.ppat.1002918
Datsenko, K. A., and Wanner, B. L. (2000). One-step inactivation of chromosomal genes in Escherichia coli K-12 using PCR products. Proc. Natl. Acad. Sci. U. S. A. 90, 6640–6645. doi: 10.1073/pnas.120163297
Dougan, G., and Baker, S. (2014). Salmonella enterica serovar Typhi and the pathogenesis of typhoid fever. Annu. Rev. Microbiol. 68, 317–336. doi: 10.1146/annurev-micro-091313-103739
Du, S., and Lutkenhaus, J. (2017). The N-succinyl-l,l-diaminopimelic acid desuccinylase DapE acts through ZapB to promote septum formation in Escherichia coli. Mol. Microbiol. 105, 326–345. doi: 10.1111/mmi.13703
Ebersbach, G., Galli, E., Moller-Jensen, J., Lowe, J., and Gerdes, K. (2008). Novel coiled-coil cell division factor ZapB stimulates Z ring assembly and cell division. Mol. Microbiol. 68, 720–735. doi: 10.1111/j.1365-2958.2008.06190.x
Edwards, R. A., Keller, L. H., and Schifferli, D. M. (1998). Improved allelic exchange vectors and their use to analyze 987P fimbria gene expression. Gene 207, 149–157. doi: 10.1016/S0378-1119(97)00619-7
Ellermeier, C. D., Janakiram, A., and Slauch, J. M. (2002). Construction of targeted single copy lac fusions using lambda red and FLP-mediated site-specific recombination in bacteria. Gene 290, 153–161. doi: 10.1016/s0378-1119(02)00551-6
Fang, F. C., Frawley, E. R., Tapscott, T., and Vazquez-Torres, A. (2016). Bacterial stress responses during host infection. Cell Host Microbe 20, 133–143. doi: 10.1016/j.chom.2016.07.009
Figueroa-Bossi, N., Lemire, S., Maloriol, D., Balbontin, R., Casadesus, J., and Bossi, L. (2006). Loss of Hfq activates the sigmaE-dependent envelope stress response in Salmonella enterica. Mol. Microbiol. 62, 838–852. doi: 10.1111/j.1365-2958.2006.05413.x
Garcia-Calderon, C. B., Casadesus, J., and Ramos-Morales, F. (2009). Regulation of igaA and the Rcs system by the MviA response regulator in Salmonella enterica. J. Bacteriol. 191, 2743–2752. doi: 10.1128/JB.01519-08
Garcia-Calderon, C. B., Garcia-Quintanilla, M., Casadesus, J., and Ramos-Morales, F. (2005). Virulence attenuation in Salmonella enterica rcsC mutants with constitutive activation of the Rcs system. Microbiology 151, 579–588. doi: 10.1099/mic.0.27520-0
Garzon, A., Cano, D. A., and Casadesus, J. (1995). Role of Erf recombinase in P22-mediated plasmid transduction. Genetics 140, 427–434. doi: 10.1093/genetics/140.2.427
Gogol, E. B., Rhodius, V. A., Papenfort, K., Vogel, J., and Gross, C. A. (2011). Small RNAs endow a transcriptional activator with essential repressor functions for single-tier control of a global stress regulon. Proc. Natl. Acad. Sci. U. S. A. 108, 12875–12880. doi: 10.1073/pnas.1109379108
Gottesman, S. (1996). Proteases and their targets in Escherichia coli. Annu. Rev. Genet. 30, 465–506. doi: 10.1146/annurev.genet.30.1.465
Gunn, J. S. (2000). Mechanisms of bacterial resistance and response to bile. Microbes Infect. 2, 907–913. doi: 10.1016/S1286-4579(00)00392-0
Gur, E., Biran, D., and Ron, E. Z. (2011). Regulated proteolysis in gram-negative bacteria—how and when? Nat. Rev. Microbiol. 9, 839–848. doi: 10.1038/nrmicro2669
Guzman, L. M., Belin, D., Carson, M. J., and Beckwith, J. (1995). Tight regulation, modulation, and high-level expression by vectors containing the arabinose PBAD promoter. J. Bacteriol. 177, 4121–4130. doi: 10.1128/JB.177.14.4121-4130.1995
Hernandez, S. B., Cava, F., Pucciarelli, M. G., Garcia-Del Portillo, F., de Pedro, M. A., and Casadesus, J. (2015). Bile-induced peptidoglycan remodelling in Salmonella enterica. Environ. Microbiol. 17, 1081–1089. doi: 10.1111/1462-2920.12491
Hernandez, S. B., Cota, I., Ducret, A., Aussel, L., and Casadesus, J. (2012). Adaptation and preadaptation of Salmonella enterica to bile. PLoS Genet. 8:e1002459. doi: 10.1371/journal.pgen.1002459
Hofmann, A. F., and Hagey, L. R. (2008). Bile acids: chemistry, pathochemistry, biology, pathobiology, and therapeutics. Cell. Mol. Life Sci. 65, 2461–2483. doi: 10.1007/s00018-008-7568-6
Hoiseth, S. K., and Stocker, B. A. (1981). Aromatic-dependent Salmonella typhimurium are non-virulent and effective as live vaccines. Nature 291, 238–239. doi: 10.1038/291238a0
Johnson, R., Ravenhall, M., Pickard, D., Dougan, G., Byrne, A., and Frankel, G. (2017). Comparison of Salmonella enterica serovars Typhi and Typhimurium reveals typhoidal serovar-specific responses to bile. Infect. Immun. 86, e00490–e00517. doi: 10.1128/IAI.00490-17
Kint, G., De Coster, D., Marchal, K., Vanderleyden, J., and De Keersmaecker, S. C. (2010). The small regulatory RNA molecule MicA is involved in Salmonella enterica serovar Typhimurium biofilm formation. BMC Microbiol. 10:276. doi: 10.1186/1471-2180-10-276
Kroger, C., Colgan, A., Srikumar, S., Handler, K., Sivasankaran, S. K., Hammarlof, D. L., et al. (2013). An infection-relevant transcriptomic compendium for Salmonella enterica Serovar Typhimurium. Cell Host Microbe 14, 683–695. doi: 10.1016/j.chom.2013.11.010
Leverrier, P., Dimova, D., Pichereau, V., Auffray, Y., Boyaval, P., and Jan, G. (2003). Susceptibility and adaptive response to bile salts in Propionibacterium freudenreichii: physiological and proteomic analysis. Appl. Environ. Microbiol. 69, 3809–3818. doi: 10.1128/AEM.69.7.3809-3818.2003
Lopez-Garrido, J., and Casadesus, J. (2012). Crosstalk between virulence loci: regulation of Salmonella enterica pathogenicity island 1 (SPI-1) by products of the std fimbrial operon. PLoS One 7:e30499. doi: 10.1371/journal.pone.0030499
Lopez-Garrido, J., Cheng, N., Garcia-Quintanilla, F., Garcia-del Portillo, F., and Casadesus, J. (2010). Identification of the Salmonella enterica damX gene product, an inner membrane protein involved in bile resistance. J. Bacteriol. 192, 893–895. doi: 10.1128/JB.01220-09
Lutz, R., and Bujard, H. (1997). Independent and tight regulation of transcriptional units in Escherichia coli via the LacR/O, the TetR/O and AraC/I1-I2 regulatory elements. Nucleic Acids Res. 25, 1203–1210. doi: 10.1093/nar/25.6.1203
Macian, F., Perez-Roger, I., and Armengod, M. E. (1994). An improved vector system for constructing transcriptional lacZ fusions: analysis of regulation of the dnaA, dnaN, recF and gyrB genes of Escherichia coli. Gene 145, 17–24. doi: 10.1016/0378-1119(94)90317-4
Mannik, J., Castillo, D. E., Yang, D., and Siopsis, G. (2016). The role of MatP, ZapA and ZapB in chromosomal organization and dynamics in Escherichia coli. Nucleic Acids Res. 44, 1216–1226. doi: 10.1093/nar/gkv1484
May, J. F., and Groisman, E. A. (2014). Conflicting roles for a cell surface modification in Salmonella. Mol. Microbiol. 88, 970–983. doi: 10.1111/mmi.12236
McCullen, C. A., Benhammou, J. N., Majdalani, N., and Gottesman, S. (2010). Mechanism of positive regulation by DsrA and RprA small noncoding RNAs: pairing increases translation and protects rpoS mRNA from degradation. J. Bacteriol. 192, 5559–5571. doi: 10.1128/JB.00464-10
Merritt, M. E., and Donaldson, J. R. (2009). Effect of bile salts on the DNA and membrane integrity of enteric bacteria. J. Med. Microbiol. 58, 1533–1541. doi: 10.1099/jmm.0.014092-0
Miller, J. H. (1972). Experiments in molecular genetics. Cold Spring Harbor, New York: Cold Spring Harbor Laboratory Press.
Opdyke, J. A., Kang, J. G., and Storz, G. (2004). GadY, a small-RNA regulator of acid response genes in Escherichia coli. J. Bacteriol. 186, 6698–6705. doi: 10.1128/JB.186.20.6698-6705.2004
Papenfort, K., Pfeiffer, V., Mika, F., Lucchini, S., Hinton, J. C., and Vogel, J. (2006). SigmaE-dependent small RNAs of Salmonella respond to membrane stress by accelerating global omp mRNA decay. Mol. Microbiol. 62, 1674–1688. doi: 10.1111/j.1365-2958.2006.05524.x
Papenfort, K., and Vanderpool, C. K. (2015). Target activation by regulatory RNAs in bacteria. FEMS Microbiol. Rev. 39, 362–378. doi: 10.1093/femsre/fuv016
Papenfort, K., and Vogel, J. (2009). Multiple target regulation by small noncoding RNAs rewires gene expression at the post-transcriptional level. Res. Microbiol. 160, 278–287. doi: 10.1016/j.resmic.2009.03.004
Picken, R. N., and Beacham, I. R. (1977). Bacteriophage-resistant mutants of Escherichia coli K12. Location of receptors within the lipopolysaccharide. J. Gen. Microbiol. 102, 305–318. doi: 10.1099/00221287-102-2-305
Podkaminski, D., and Vogel, J. (2010). Small RNAs promote mRNA stability to activate the synthesis of virulence factors. Mol. Microbiol. 78, 1327–1331. doi: 10.1111/j.1365-2958.2010.07428.x
Prevost, K., Desnoyers, G., Jacques, J. F., Lavoie, F., and Masse, E. (2011). Small RNA-induced mRNA degradation achieved through both translation block and activated cleavage. Genes Dev. 25, 385–396. doi: 10.1101/gad.2001711
Prieto, A. I., Ramos-Morales, F., and Casadesus, J. (2004). Bile-induced DNA damage in Salmonella enterica. Genetics 168, 1787–1794. doi: 10.1534/genetics.104.031062
Prieto, A. I., Ramos-Morales, F., and Casadesus, J. (2006). Repair of DNA damage induced by bile salts in Salmonella enterica. Genetics 174, 575–584. doi: 10.1534/genetics.106.060889
Prouty, A. M., Brodsky, I. E., Manos, J., Belas, R., Falkow, S., and Gunn, J. S. (2004). Transcriptional regulation of Salmonella enterica serovar Typhimurium genes by bile. FEMS Immunol. Med. Microbiol. 41, 177–185. doi: 10.1016/j.femsim.2004.03.002
Pucciarelli, M. G., Prieto, A. I., Casadesús, J., and García-del-Portillo, F. (2002). Envelope instability in DNA adenine methylase mutants of Salmonella enterica. Microbiology 148, 1171–1182. doi: 10.1099/00221287-148-4-1171
Ramos-Morales, F., Prieto, A. I., Beuzon, C. R., Holden, D. W., and Casadesus, J. (2003). Role for Salmonella enterica enterobacterial common antigen in bile resistance and virulence. J. Bacteriol. 185, 5328–5332. doi: 10.1128/JB.185.17.5328-5332.2003
Sambrook, J., and Russell, D. W. (2001). Molecular cloning: A laboratory manual. Cold Spring Harbor, New York: Cold Spring Harbor Laboratory Press.
Schmieger, H. (1972). Phage P22-mutants with increased or decreased transduction abilities. Mol. Gen. Genet. 119, 75–88. doi: 10.1007/BF00270447
Smith, C., Heyne, S., Richter, A. S., Will, S., and Backofen, R. (2010). Freiburg RNA tools: a web server integrating INTARNA, EXPARNA and LOCARNA. Nucleic Acids Res. 38, W373–W377. doi: 10.1093/nar/gkq316
Soper, T. J., Doxzen, K., and Woodson, S. A. (2011). Major role for mRNA binding and restructuring in sRNA recruitment by Hfq. RNA 17, 1544–1550. doi: 10.1261/rna.2767211
Soper, T., Mandin, P., Majdalani, N., Gottesman, S., and Woodson, S. A. (2010). Positive regulation by small RNAs and the role of Hfq. Proc. Natl. Acad. Sci. U. S. A. 107, 9602–9607. doi: 10.1073/pnas.1004435107
Storz, G., Opdyke, J. A., and Zhang, A. (2004). Controlling mRNA stability and translation with small, noncoding RNAs. Curr. Opin. Microbiol. 7, 140–144. doi: 10.1016/j.mib.2004.02.015
Storz, G., Vogel, J., and Wassarman, K. M. (2011). Regulation by small RNAs in bacteria: expanding frontiers. Mol. Cell 43, 880–891. doi: 10.1016/j.molcel.2011.08.022
Thanassi, D. G., Cheng, L. W., and Nikaido, H. (1997). Active efflux of bile salts by Escherichia coli. J. Bacteriol. 179, 2512–2518. doi: 10.1128/JB.179.8.2512-2518.1997
Torreblanca, J., Marques, S., and Casadesus, J. (1999). Synthesis of FinP RNA by plasmids F and pSLT is regulated by DNA adenine methylation. Genetics 152, 31–45.
Tsilibaris, V., Maenhaut-Michel, G., and Van Melderen, L. (2006). Biological roles of the Lon ATP-dependent protease. Res. Microbiol. 157, 701–713. doi: 10.1016/j.resmic.2006.05.004
Udekwu, K. I., Darfeuille, F., Vogel, J., Reimegard, J., Holmqvist, E., and Wagner, E. G. (2005). Hfq-dependent regulation of OmpA synthesis is mediated by an antisense RNA. Genes Dev. 19, 2355–2366. doi: 10.1101/gad.354405
Urdaneta, V., and Casadesus, J. (2017). Interactions between bacteria and bile salts in the gastrointestinal and hepatobiliary tracts. Front. Med. 4:163. doi: 10.3389/fmed.2017.00163
Urdaneta, V., and Casadesus, J. (2018). Adaptation of Salmonella enterica to bile: essential role of AcrAB-mediated efflux. Environ. Microbiol. 20, 1405–1418. doi: 10.1111/1462-2920.14047
Uzzau, S., Figueroa-Bossi, N., Rubino, S., and Bossi, L. (2001). Epitope tagging of chromosomal genes in Salmonella. Proc. Natl. Acad. Sci. U. S. A. 98, 15264–15269. doi: 10.1073/pnas.261348198
Valentin-Hansen, P., Eriksen, M., and Udesen, C. (2004). The bacterial Sm-like protein Hfq: a key player in RNA transactions. Mol. Microbiol. 51, 1525–1533. doi: 10.1111/j.1365-2958.2003.03935.x
Van Puyvelde, S., Vanderleyden, J., and De Keersmaecker, S. C. (2015). Experimental approaches to identify small RNAs and their diverse roles in bacteria—what we have learnt in one decade of MicA research. Microbiology 4, 699–711. doi: 10.1002/mbo3.263
van Velkinburgh, J. C., and Gunn, J. S. (1999). PhoP-PhoQ-regulated loci are required for enhanced bile resistance in Salmonella spp. Infect. Immun. 67, 1614–1622. doi: 10.1128/IAI.67.4.1614-1622.1999
Keywords: Salmonella, bile resistance, Z-ring, Lon protease, MicA sRNA
Citation: Fernández-Fernández R, Hernández SB, Puerta-Fernández E, Sánchez-Romero MA, Urdaneta V and Casadesús J (2021) Evidence for Involvement of the Salmonella enterica Z-Ring Assembly Factors ZapA and ZapB in Resistance to Bile. Front. Microbiol. 12:647305. doi: 10.3389/fmicb.2021.647305
Edited by:
Axel Cloeckaert, Institut National de Recherche pour l’agriculture, l’alimentation et l’environnement (INRAE), FranceReviewed by:
Juan A. Ayala, Autonomous University of Madrid, SpainLaurent Aussel, Aix-Marseille Université, France
Copyright © 2021 Fernández-Fernández, Hernández, Puerta-Fernández, Sánchez-Romero, Urdaneta and Casadesús. This is an open-access article distributed under the terms of the Creative Commons Attribution License (CC BY). The use, distribution or reproduction in other forums is permitted, provided the original author(s) and the copyright owner(s) are credited and that the original publication in this journal is cited, in accordance with accepted academic practice. No use, distribution or reproduction is permitted which does not comply with these terms.
*Correspondence: Josep Casadesús, Y2FzYWRlc3VzQHVzLmVz; Verónica Urdaneta, dmVyb25pY2F1cmRhbmV0YUBnbWFpbC5jb20=
†These authors have contributed equally to this work
‡Present address: Sara B. Hernández, Department of Molecular Biology, Umeå University, Umeå, Sweden
Elena Puerta-Fernández, Instituto de Recursos Naturales y Agrobiología de Sevilla (IRNAS, CSIC), Sevilla, Spain
María A. Sánchez-Romero, Departamento de Microbiología y Parasitología, Facultad de Farmacia, Universidad de Sevilla, Sevilla, Spain
Verónica Urdaneta, Department of Infectious Diseases, Yale School of Medicine, The Anlyan Center, New Haven, CT, United States