- 1Departamento de Genética e Evolução, Centro de Ciências Biológicas e da Saúde, Universidade Federal de São Carlos, São Carlos, Brazil
- 2Department of Microbiology and Immunology, Stony Brook University, Stony Brook, NY, United States
- 3Laboratório Nacional de Biorrenováveis (LNBR), Centro Nacional de Pesquisa em Energia e Materiais (CNPEM), Campinas, São Paulo, Brazil
- 4MRC Centre for Medical Mycology, University of Exeter, Exeter, United Kingdom
- 5Faculdade de Ciências Farmacêuticas de Ribeirão Preto, Universidade de São Paulo, São Paulo, Brazil
- 6Division of Infectious Diseases, School of Medicine, Stony Brook University, Stony Brook, NY, United States
- 7Institute of Chemical Biology and Drug Discovery, Stony Brook University, Stony Brook, NY, United States
- 8Veterans Administration Medical Center, Northport, NY, United States
The deleterious effects of human-induced climate change have long been predicted. However, the imminent emergence and spread of new diseases, including fungal infections through the rise of thermotolerant strains, is still neglected, despite being a potential consequence of global warming. Thermotolerance is a remarkable virulence attribute of the mold Aspergillus fumigatus. Under high-temperature stress, opportunistic fungal pathogens deploy an adaptive mechanism known as heat shock (HS) response controlled by heat shock transcription factors (HSFs). In eukaryotes, HSFs regulate the expression of several heat shock proteins (HSPs), such as the chaperone Hsp90, which is part of the cellular program for heat adaptation and a direct target of HSFs. We recently observed that the perturbation in cell wall integrity (CWI) causes concomitant susceptibility to elevated temperatures in A. fumigatus, although the mechanisms underpinning the HS response and CWI cross talking are not elucidated. Here, we aim at further deciphering the interplay between HS and CWI. Our results show that cell wall ultrastructure is severely modified when A. fumigatus is exposed to HS. We identify the transcription factor HsfA as essential for A. fumigatus viability, thermotolerance, and CWI. Indeed, HS and cell wall stress trigger the coordinated expression of both hsfA and hsp90. Furthermore, the CWI signaling pathway components PkcA and MpkA were shown to be important for HsfA and Hsp90 expression in the A. fumigatus biofilms. Lastly, RNA-sequencing confirmed that hsfA regulates the expression of genes related to the HS response, cell wall biosynthesis and remodeling, and lipid homeostasis. Our studies collectively demonstrate the connection between the HS and the CWI pathway, with HsfA playing a crucial role in this cross-pathway regulation, reinforcing the importance of the cell wall in A. fumigatus thermophily.
Introduction
Fungal human diseases and their impacts on human health and world economy have frequently been overlooked (Brown et al., 2012). It is believed that fungal infections kill more than 1.5 million people worldwide per year (Benedict et al., 2019), and prospects are even worse if the last decades’ environmental changes are considered. For instance, it has been proposed that global warming may significantly enhance the adaptation of fungal populations to higher temperatures, which may cause the emergence of new fungal diseases associated with difficult treatment (Garcia-Solache and Casadevall, 2010; Casadevall et al., 2019).
In this worrisome scenario, the saprophytic mold and opportunistic human pathogen Aspergillus fumigatus stands out for its intrinsic thermophilic and thermotolerance traits and the rise of resistant isolates to available antifungal drugs (van Paassen et al., 2016). Unlike other Aspergillus species, A. fumigatus can germinate under temperatures above 40°C, and its conidia remain viable up to 70°C (Araujo and Rodrigues, 2004). These attributes of A. fumigatus biology help to explain its high prevalence in the environment and support the thermotolerance as an essential determinant for its pathogenicity since it allows the adaptation of the fungus to temperatures found before and after the infection of mammalian host and favors the persistence of this fungus inside the human lungs (Albrecht et al., 2010; Haas et al., 2016).
Living organisms continuously monitor the environmental temperature to survive (Leach and Cowen, 2013). In response to temperature rise, gene expression is adjusted, allowing the cells to synthesize a specific group of proteins called heat shock proteins (HSPs) that deal with this stressing condition (Parsell and Lindquist, 1993). During heat shock (HS), these molecular chaperones prevent the aggregation of denatured proteins and restore their native conformations and function (Hartl, 1996). To allow this orchestrated response, an influential group of conserved DNA binding proteins, the heat shock transcription factors (HSFs), regulate gene transcription during HS and other stress sources (Albrecht et al., 2010; Sueiro-Olivares et al., 2015; Gomez-Pastor et al., 2018). One member of this family, the heat shock transcription factor 1 (Hsf1), is a key player in mediating the cell transcriptional response to HS and has been widely studied in humans, Saccharomyces cerevisiae, and Candida albicans (Sarge et al., 1993; Eastmond and Nelson, 2006; Nicholls et al., 2009; Leach et al., 2016). Hsf1 is essential for viability and it is hyperphosphorylated in both fungal organisms in response to HS (Wiederrecht et al., 1988; Hashikawa and Sakurai, 2004; Nicholls et al., 2009, 2011). Under stressing conditions, Hsf1 homotrimers bind to specific DNA motifs named HS elements (HSE) located in target gene promoters, inducing the expression of molecular chaperones and other genes related to thermal adaptation (Sorger and Pelham, 1988; Sorger and Nelson, 1989; Yamamoto et al., 2005; Leach et al., 2016). Concomitantly, chaperones such as Hsp70 and Hsp90 are known regulators of Hsf1 activity via a well-described feedback regulatory loop in which Hsp90 binds to Hsf1 to keep it in an inactive state (Leach et al., 2012a; Schopf et al., 2017; Kijima et al., 2018; Krakowiak et al., 2018). In conditions where the chaperones are required for other functions and are no longer available for Hsf1 inhibition, the transcription of target HS genes increases (Veri et al., 2018). In A. fumigatus, putative HSEs were found in genes encoding HSPs and enzymes involved in the oxidative stress response, protein translation, carbohydrate and nitrogen metabolism, and signal transduction (Albrecht et al., 2010).
Thermoadaptation has also been directly associated with the activation of signaling pathways that govern cellular processes such as fungal morphogenesis and dimorphism, plasma membrane fluidity, and cell wall integrity (CWI) [reviewed in Brown et al. (2010); Leach and Cowen (2013); Fabri et al. (2020)]. The CWI pathway, which comprises the apical kinase PkcA, the three-component mitogen-activated protein kinases (MAPKs) Bck1, Mkk2, and MpkA, and the transcription factor RlmA (Valiante et al., 2009; Rocha et al., 2015, 2016), is one of the signaling cascades responsible for the regulation of cell wall biosynthesis and maintenance (Valiante et al., 2015). This signaling pathway also sustains the fungal cell wall viability during heat stress (Kamada et al., 1995; Fuchs and Mylonakis, 2009; Rocha et al., 2020b). However, despite the significance of the interplay between thermotolerance and CWI, little is known about the molecular events that reciprocally govern these two essential A. fumigatus virulence attributes.
Precedent exists indicating that the Hsf1-Hsp90 circuit is a connecting point between thermotolerance and the fungal CWI pathway (LaFayette et al., 2010; Lamoth et al., 2012; Leach et al., 2012a). For instance, without the proper expression of Hsp90 driven by Hsf1 in S. cerevisiae and C. albicans, CWI is compromised, leading to deficient expression of genes that promote CWI during exposure to high temperatures (Truman et al., 2007; Nicholls et al., 2009; Leach et al., 2012a). Also, Hsp90 potentiates the resistance to echinocandins in different fungal pathogens, including A. fumigatus, since depletion of this chaperone increases the susceptibility to these antifungals (Cowen, 2009; LaFayette et al., 2010; Lamoth et al., 2013, 2015; Chatterjee and Tatu, 2017). We have previously demonstrated that three critical components of the A. fumigatus CWI pathway (PkcA, MpkA, and RlmA) are Hsp90 clients (Rocha et al., 2020b), suggesting an involvement of the Hsf1-Hsp90 regulatory function over the A. fumigatus CWI pathway. However, it remained unclear how hsfAHSF1 contributes to thermal adaptation and the disturbed cell wall homeostasis observed in the CWI pathway mutants. This is a follow-up study to our previous work (Rocha et al., 2020b), and our results show that cell wall ultrastructure is severely modified when cells are exposed to HS. We observe that hsfA is essential for thermotolerance, impacts the expression of genes related to the cell wall biosynthesis, and genetically interacts with the main players of the CWI and HOG pathway. We show a clear link between the HS and cell wall stress modulated by HsfA and the CWI pathway in A. fumigatus biofilms in vitro by following the fluctuations in HsfA expression. Our results also show that cell integrity genes are modulated when hsfA expression is repressed.
Materials and Methods
Strains and Culture Conditions
The A. fumigatus strains used in this study are described in Supplementary Table 1. Strains were maintained in complete medium [YG; glucose 2% (w/w), 0.5% yeast extract (w/w), 1 × trace elements)] or minimal medium [MM; glucose 1% (w/w), 1 × high-nitrate salts, and 1 × trace elements (pH 6.5)]. Trace elements and high nitrate salt compositions were as described previously (Malavazi and Goldman, 2012). For solid media, agar 2% (w/w) was added. To grow the ΔKU80 pyrG1 strain, the media was supplemented with 1.2 g/L of uridine and uracil. When required, pyrithiamine (Sigma) or hygromycin B (Merck) was added to a final concentration of 0.2 μg/ml or 200 μg/ml, respectively. Different xylose concentrations were used depending on the experiment to induce hsfA expression in the xylP::hsfA conditional strain.
The wild-type and xylP::hsfA strains were grown and analyzed by DIC (Differential Interference Contrast) microscopy to analyze hyphal growth. Accordingly, 1 × 105 conidia of each strain were inoculated in glass-bottom dishes (MatTek Corporation) containing 2 ml of MM supplemented with different xylose concentrations at 37°C for 12 h. Images were captured with an AxioCam MRm camera (Zeiss) and processed using ZEN software.
Construction of the A. fumigatus Mutants
For the xylP::hsfA cassette construction, two fragments spanning the 5′ UTR region of hsfA (Afu5g01900) gene were PCR-amplified from genomic DNA of the CEA17 strain, according to Supplementary Figure 1A. The primers used are listed in Supplementary Table 2. The opposite sites of the 5′ regions contained a short sequence homologous to the multiple cloning site of the pRS426 plasmid (the small bold letters indicated in Supplementary Table 2). The pyrG gene inserted into the cassette was amplified from pCDA21 plasmid (Chaveroche et al., 2000) and used as a prototrophy marker. The pYES-hph-pXyl devR vector was used to amplify the Penicillium chrysogenum xylose reductase gene promoter (xylP), which is activated in the presence of xylose and repressed in the presence of glucose (Zadra et al., 2000). The substitution cassette was generated by in vivo recombination in S. cerevisiae, as reported previously (Malavazi and Goldman, 2012). All the PCR amplifications were performed using Phusion High-Fidelity DNA Polymerase (Thermo Scientific). The cassette was transformed into protoplasts of the A. fumigatus ΔKU80 pyrG1 according to previously described procedures (Malavazi and Goldman, 2012). Transformants were carefully tested by PCR (Supplementary Figure 1B) and Southern blot analysis, using the 5′ flanking region as a probe (Supplementary Figure 1C). An endogenous hsfA promoter region spanning 467 bp was maintained before the hsfA gene because we previously observed that complete replacement of the native hsfA promoter resulted in transformants with growth defects and inconsistent control of hsfA transcription (data not shown).
To generate the double mutant ΔmpkA xylP::hsfA, the mpkA deletion cassette was amplified from the genomic DNA of the ΔmpkA strain (Valiante et al., 2009) using primers MpkA 5F and MpkA 3′ REV and transformed into the xylP::hsfA strain selected for pyrithiamine resistance. The hsfA locus in this strain was checked using the primers HsfA 600 ups and HsfA 2 5UTR REV pRS426 (Supplementary Figure 1D), while the mpkA replacement was checked by using the primers MpkA FW and MpkA REV (Supplementary Table 2 and Supplementary Figure 1E). Likewise, to construct the double mutant pkcAG579R xylP::hsfA, the xylP::hsfA cassette was transformed into the pkcAG579R pyrG- strain (Rocha et al., 2020b). The xylP::hsfA replacement in this mutant was checked using the primers HsfA 5 FW and xylP REV (Supplementary Figure 1F), while the primers pkcA GC FW and Afu5g11970 3R (Supplementary Figure 1G) were used to check the mutated pkcA locus. The pkcAG579R mutation is a Gly579Arg substitution in the PkcA protein, and the strain carrying this mutation is defective in the activation of MpkA and consequently the activation of the CWI pathway, resulting in altered expression of genes encoding cell wall-related proteins (Rocha et al., 2015). To construct the double mutant ΔsakA xylP::hsfA, the sakA deletion cassette was amplified from the genomic DNA of the ΔsakA strain (Altwasser et al., 2015) using primers sakA yes FW and sakA yes REV and transformed into the xylP::hsfA strain selected for hygromycin resistance. The xylP::hsfA locus in this mutant was checked using the primers HsfA 5 FW and xylP REV (Supplementary Figure 1H), while the primers IM-563 and IM-566 (Supplementary Figure 1I) were used to check the sakA locus.
To generate HsfA fusion with the luciferase gene (luc), a substitution cassette was constructed in which the hsfA genomic sequence without stop codon was cloned in-frame with the luc gene in a C-terminal fusion (Supplementary Figure 1J). The luc gene (1665 bp) without spacer was PCR-amplified from the pUC57 plasmid (Jacobsen et al., 2014) using the primers Luc FW and Luc REV. The pyrG gene was also used as a marker for prototrophy. The cassette was constructed in vivo in S. cerevisiae and transformed in A. fumigatus wild-type (Supplementary Figures 1K,L) and pkcAG579R pyrG- (Supplementary Figures 1M,N) strains. The ΔmpkA cassette was also used to transform the hsfA::luc strain to obtain the double mutant (Supplementary Figures 1O,P).
To generate the reporter strain in which the hsp90 promoter (hsp90P) was fused with the luciferase gene (luc), a substitution cassette was constructed by cloning the hsp90P (975 bp) in-frame with the luc gene (Supplementary Figure 1Q) flanked by 5′ UTR (0.833 kb) and 3′ UTR (0.573 kb) regions of the pyrG gene to allow integration of the construct at the pyrG locus. hsp90P was amplified from the genomic DNA of the CEA17 strain using the primers Hsp90P pyrG FW and Hsp90P RV luc, and the 5’ UTR of pyrG was amplified by the primers pRS426 5UTR pyrG FW and 5UTR pyrG RV. The fragment containing the luc gene, the hygromycin resistance marker gene, and the 3′ UTR region of pyrG was amplified from the pNB04 plasmid (Rocha et al., 2016) using primers luc 2 FW and pyrG 3UTR pRS426 RV. The hsp90P::luc cassette was generated by recombination in S. cerevisiae and transformed into the A. fumigatus wild-type (Supplementary Figures 1R,S), pkcAG579R (Supplementary Figures 1T,U), ΔrlmA (Supplementary Figures 1T,V), and ΔmpkA (Supplementary Figures 1T,W) strains to obtain relevant single and double mutants.
DNA Manipulation and Southern Blot Analysis
Southern blot analysis was used to confirm that the xylP::hsfA cassette integrated homologously at the targeted locus. Genomic DNA from A. fumigatus was extracted as previously described (Malavazi and Goldman, 2012). For Southern blot analysis, XhoI-restricted chromosomal DNA fragments were separated on a 1% agarose gel and blotted onto Hybond N+ nylon membranes (GE Healthcare), following standard techniques. Probe labeling was performed using AlkPhos Direct Labelling and Detection System (GE Healthcare) according to the manufacturer’s description. Labeled membranes were exposed to ChemiDoc XRS gel imaging system (BioRad) to generate the images.
Transmission Electron Microscopy (TEM) Analysis
A total of 1 × 107 conidia of the wild-type strain were statically grown in 10 ml of liquid YG for 36 h at 30°C. HS was induced by transferring the mycelia to fresh pre-heated YG (48°C) for 5, 10, 15, 30, and 60 min of incubation at 48°C. The control was left at 30°C. To analyze the cell wall organization in the conditional hsfA mutant, wild-type and xylP::hsfA strains were statically grown in liquid MM supplemented with xylose 1% for 36 h at 30°C. The mycelia were collected by centrifugation, washed twice with fresh MM, and further incubated in MM (repression) or MM lacking glucose but supplemented with xylose 0.06% (induction) for 4 h at 30°C. Finally, the repressed samples were heat-shocked at 48°C for 15, 30, and 60 min or treated with 2 μg/ml of caspofungin (CASP) for 1 h to induce cell wall stress. The untreated control remained at 30°C. Next, the mycelia were fixed in 3% EM grade glutaraldehyde in 0.1 M sodium cacodylate buffer, pH 7.4, for 24 h at 4°C. Cells were processed as described previously (Munshi et al., 2018), and TEM image acquisition was achieved using a FEI TeCnai12 BioTwinG2 microscope at an acceleration voltage of 120 kV. Digital images were acquired with an AMT XR-60 CCD Digital Camera system. Cell wall thicknesses of 50 sections of different germlings were measured using ImageJ software.
Phenotypic Assays
The radial growth of the wild-type and xylP::hsfA strains at different temperatures was analyzed by spotting 1 × 104 conidia of each strain onto the center of 90 mm Petri dishes containing solid MM supplemented with different concentrations of xylose. The plates were incubated for 72 h at 30°C, 37°C, or 48°C and analyzed. Similarly, to investigate the susceptibility of the strains to agents that impair cell wall maintenance or cell membrane [caffeine (CAF), calcofluor white (CFW), congo red (CR), caspofungin (CASP), and sodium dodecyl sulfate (SDS), respectively], 1 × 104 conidia of each strain were inoculated on solid MM supplemented with xylose 0.06% and increasing concentrations of the drugs. The same procedures were employed to test susceptibility to osmotic stress caused by sorbitol and oxidative stress induced by paraquat and menadione. All the Petri dishes were incubated at 37°C for 72 h. As an alternative protocol, the susceptibility to H2O2, diamide, and the crop fungicide fludioxonil was investigated in 96-well plate assays containing 200 μl of liquid MM supplemented with xylose 0.06% and different concentrations of the agents mentioned above.
For the genetic analysis of double and single mutants, 10-fold dilution series (1 × 105–1 × 102) of the conidia were spotted on Petri dishes containing MM supplemented with different xylose concentrations and CASP. The plates were incubated at 30°C, 37°C, or 48°C for 48 h and analyzed.
Luciferase Activity Assay
Luminescence quantification of the relevant strains was performed as described elsewhere (Rocha et al., 2016), with minor modifications. For the luciferase activity assay during HS, 2 × 105 conidia of each strain were cultured in white, clear bottomed 96-well plates (Greiner Bio-one) containing 200 μl of MM supplemented with yeast extract 0.006% and grown at 30°C for 12 h for initial biofilm formation. Next, luciferin (0.5 mM) was added to each well and the plates were read initially at 30°C and incubated at 37°C or 48°C for 2 h. Luminescence readings were taken at 2-min intervals. For the cell wall stress assay, the same procedures were followed except that the plates were cultured at 37°C for 5 h. Subsequently, 2 μg/ml of CASP were added to each well along with luciferin 0.5 mM. The plates were incubated at 37°C for 4 h, and readings were recorded at 2-min intervals. All the luciferase activity experiments were read in Luminescence mode on the SpectraMax M5 (Molecular Devices). Normalization was made by the number of conidia. Mean ± SEM are shown in the graphs.
RNA Extraction and RT-qPCR Procedures
To evaluate the hsfA expression in the conditional xylP::hsfA mutant, 1 × 108 conidia of the wild-type and xylP::hsfA strains were grown for 24 h at 37°C in 50 ml of liquid MM supplemented with xylose 1% and transferred to fresh MM or MM without glucose supplemented with different concentrations of xylose (0.06, 0.2, 1, and 5%) for additional 4 h of growth. HS stress induction was achieved by incubating 1 × 108 conidia of the wild-type strain in MM for 24 h at 30°C. Subsequently, mycelia were transferred to fresh pre-heated MM (48°C) for 2, 5, 10, 15, 30, 60, and 120 min of incubation at 48°C. The control was left at 30°C. Cell wall stress was achieved by incubating 2 × 107 conidia of the wild-type strain in YG for 16 h at 37°C. Subsequently, the samples were subjected to CASP or CR exposure for 60 or 30 min, respectively. Mycelia from each culture condition were collected via vacuum filtration, frozen in liquid nitrogen, and stored at −80°C until used for RNA extractions.
The total RNA was extracted with Trizol reagent (Thermo Scientific) according to the manufacturer’s protocol. RNA was processed as described previously (Rocha et al., 2015). DNAse-treated total RNA from each strain was reverse-transcribed with High-Capacity cDNA Reverse Transcription kit (Thermo Scientific) as described elsewhere (Rocha et al., 2015). RT-qPCR was conducted with Power Sybr Green PCR Master Mix (Thermo Scientific). The primers for the individual genes were designed using Primer Express 3.0 software (Life Technologies) and are listed in Supplementary Table 3. RT-qPCR was performed in duplicate from three independent biological samples in a StepOne Plus Real-time PCR System (Thermo Scientific). The fold change in mRNA abundance was calculated using 2–ΔΔCt equation, and all the values were normalized to the expression of β-tubulin (tubA).
RNA-Sequencing
To induce heat stress, 1 × 108 conidia from wild-type and xylP::hsfA strains were incubated in 50 ml of liquid MM supplemented with xylose 1% for 24 h at 30°C. Subsequently, mycelia were washed twice with MM and incubated for 4 h at 30°C in MM for hsfA repression (Baldin et al., 2015). HS was induced by transferring the mycelia to fresh pre-heated MM for 15 and 60 min at 48°C. The control was left at 30°C. Mycelium from each time point for both pre- and post-stress exposure was collected via vacuum filtration, immediately frozen in liquid nitrogen, and stored at −80°C until used for RNA extraction.
Total RNA was extracted using Trizol reagent, treated with DNase I (Qiagen) and purified using the RNeasy kit (Qiagen), according to the manufacturer’s instructions. RNA from each treatment was quantified using NanoDrop (Thermo Scientific) and analyzed on an Agilent 2100 Bioanalyzer system to assess RNA integrity (RIN = 9.0–9.5). Sample preparation and library construction were performed as described previously (Alves de Castro et al., 2016) using Illumina TruSeq stranded mRNA sample preparation kit. Libraries were quantified on Step One Plus equipment (Applied Biosystems) and sequenced (2 × 100 bp) on a HiSeq 2500 instrument. Short reads were submitted to the NCBI’s Short Read Archive under the Bioproject PRJNA690780. Obtained fastq files were quality checked with FastQC1 and cleaned with Trimmomatic (Bolger et al., 2014). Ribosomal RNA was removed using SortMeRNA (Kopylova et al., 2012), and high-quality reads were mapped to the A. fumigatus Af293 genome sequence using Tophat2 (Kim et al., 2013) in strand-specific mode. The saturation of sequencing effort was assessed by counting the number of detected exon–exon junctions at different subsampling levels of the total high-quality reads, using RSeQC (Wang et al., 2012). All samples achieved saturation of known exon–exon junctions. To assess transcript abundance, exonic reads were counted in a strand-specific way using the Rsubread library (Liao et al., 2013) from the Bioconductor suite (Huber et al., 2015). Calling of differentially expressed genes was carried out in EdgeR (Robinson et al., 2010), controlling for an FDR of 0.01. The fold changes and the statistical significances of all genes for each comparison are shown in Supplementary Table 4. Gene ontology (GO) enrichment analysis was performed using the KOBAS tool kobas.cbi.pku.edu.cn (Xie et al., 2011). Cluster analysis of the differently expressed genes was performed using hierarchical clustering in Multiple Experiment Viewer (MeV) software2.
Results
Heat Stress Affects the Structure of A. fumigatus Cell Wall
Our previous results indicated that the A. fumigatus CWI pathway mutants are less tolerant to HS and PkcA signaling is required for early adaptation to HS (Rocha et al., 2020b). To gain insights about the early and prolonged effects of HS on the cell wall, we further analyzed the cell wall organization in the wild-type strain before and after HS at 48°C using TEM (Figure 1A). We observed a remarkable increase in the thickness of the fungus cell wall after HS exposure. Surprisingly, this increase is noticeable as early as after 5 min of exposure to high temperature resulting in a 27% increase in the cell wall thickness. Consistently, this increase is more evident after 10–15 min (60% increase) and 60 min (75% increase) of HS (Figure 1B). These results suggest that the sudden increase in temperature triggers the cell wall remodeling, indicating that this structure is highly dynamic and responsive to fluctuations in the surrounding temperature, potentially underlying a survival mechanism to counteract HS represented by the dramatic expansion of the cell wall.
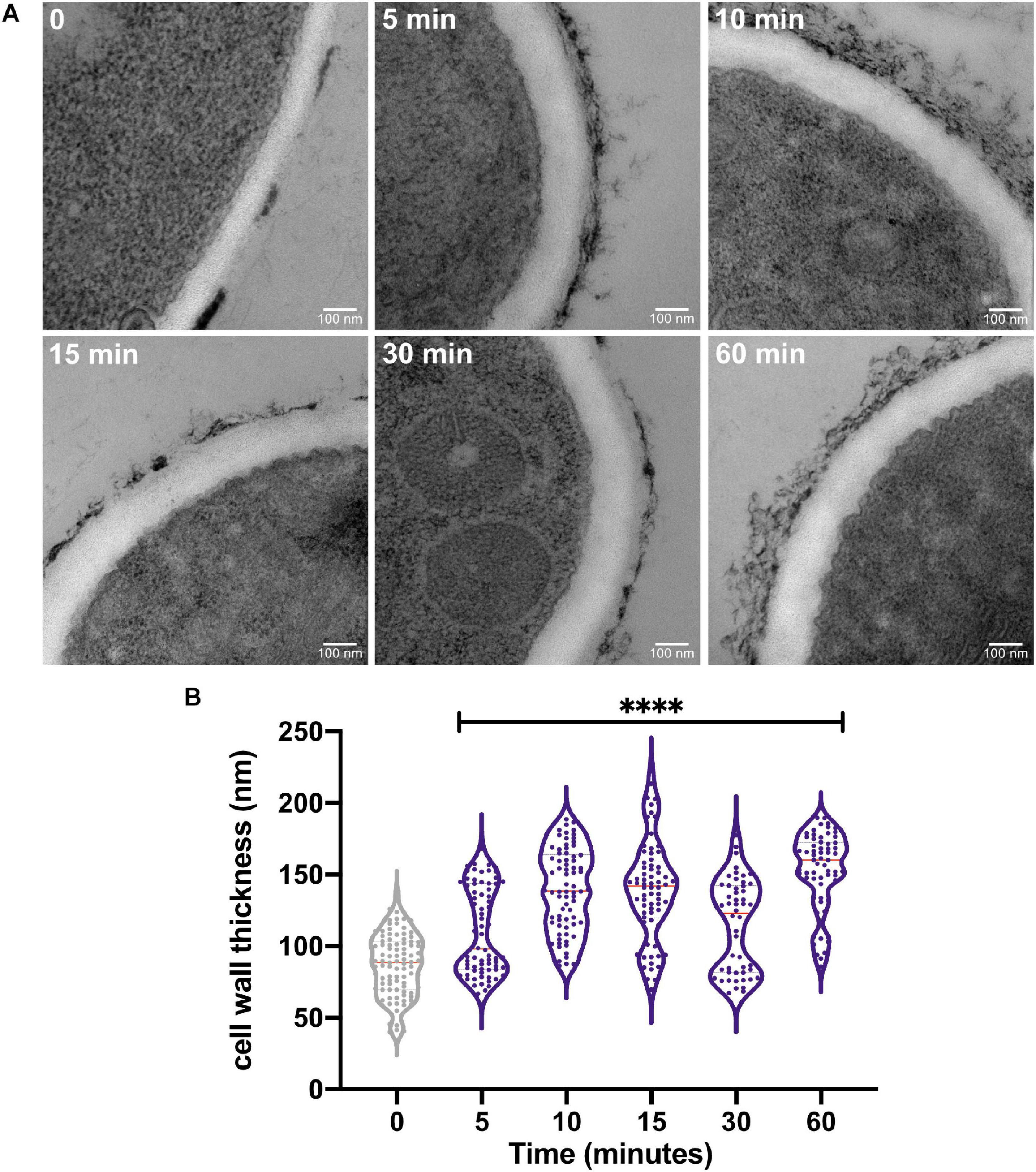
Figure 1. The heat shock stress causes an increase in the cell wall thickness of A. fumigatus. (A) The A. fumigatus wild-type strain was statically grown in complete liquid media for 36 h at 30°C, heat-shocked at 48°C for the indicated times, and prepared for transmission electron microscopy analysis. (B) Cell wall thickness measurement of the germlings shown in violin plot of 50 sections of different germlings. Dashed lines indicate the quartiles and red lines indicate the median for each point. ****p ≤ 0.0001 (one-way ANOVA and Dunnett’s multiple comparisons test).
The hsfA Gene Is Essential for Thermotolerance and Required for Cell Wall Stress Resistance in A. fumigatus
The dramatic and quick changes in the cell wall architecture observed above when cells are challenged with HS prompted us to look for the impact of known regulators of thermoadaptation on the CWI of A. fumigatus. We have previously shown that the CWI pathway is activated when the cells are exposed to elevated temperatures and that the molecular chaperone Hsp90 interacts with the main components of this signaling pathway, assisting both the adaptation to HS and cell wall stress (Rocha et al., 2020b). Since, in eukaryotes, the HSP90 gene expression is tightly governed by the transcription factor Hsf1 and this protein is one of the most important regulators of the HS response (Leach et al., 2016; Schopf et al., 2017), we searched for the hsf1 homolog in A. fumigatus. A BLASTp search of the A. fumigatus A1163 genome database using S. cerevisiae and C. albicans HSF1 as queries revealed a single open reading frame with significant similarity. The potential homolog, Afu5g01900 (hereafter named hsfA), shares 30% identity and 51% similarity (e-value 5e–26) with the ScHsf1 and 27% identity and 46% similarity (e-value 9e–29) with the CaHsf1. Furthermore, HsfA shows significant identity with Hsf1 of A. nidulans and Aspergillus niger (70% identity and 79% similarity, e-value 0.0; and 74% identity and 83% similarity, e-value 0.0, respectively). The HsfA region with the highest identity comprises the HSF-type DNA-binding domain of both yeast and human proteins (Veri et al., 2018), from residues 150 to 252 (2.8e-28; Pfam 00447).
As a first approach to study the role of HsfA in A. fumigatus, we analyzed the expression of hsfA in the A. fumigatus wild-type strain during HS and cell wall stress. We also assessed the mRNA levels of the hsp90 gene, which is one of the most significant transcriptional targets of Hsf1 in eukaryotes (Erkine et al., 1999; Leach et al., 2016; Prodromou, 2016). We found that both hsfA and hsp90 mRNA accumulation were rapidly induced in response to HS (Figure 2A), with hsfA reaching maximum expression after 15 min and hsp90 after 30 min post-HS treatment compared to the control condition (30°C). After reaching the peak, hsfA mRNA levels decrease over time while high hsp90 expression levels are sustained post-60 min of HS. These results are consistent with the role of hsfA as a transcription factor potentially upregulated when Hsp90-mediated hsfA inhibition is relieved upon HS, culminating with subsequent HsfA-mediated enhanced transcription of HSP, including Hsp90. When cells were challenged with cell wall stress induced by the addition of increasing concentrations of CASP, hsfA mRNA levels were significantly increased in the presence of 2 μg/ml and 4 μg/ml of the drug compared to the non-treated control condition (Figure 2B). Curiously, the hsp90 accumulation under these conditions did not significantly change in comparison to the non-treated control. A similar expression profile for both genes was observed when different CR (congo red) concentrations were employed (Figure 2C), where an increase in hsfA mRNA abundance was detected at CR concentration ranging from 50 μg/ml to 200 μg/ml. These results indicate that hsfA is required to cope with the cell wall stress, but without a dramatic increase in mRNA hsp90 abundance, suggesting a different regulatory mechanism for this TF in the presence of cell wall damage compared to temperature stress.
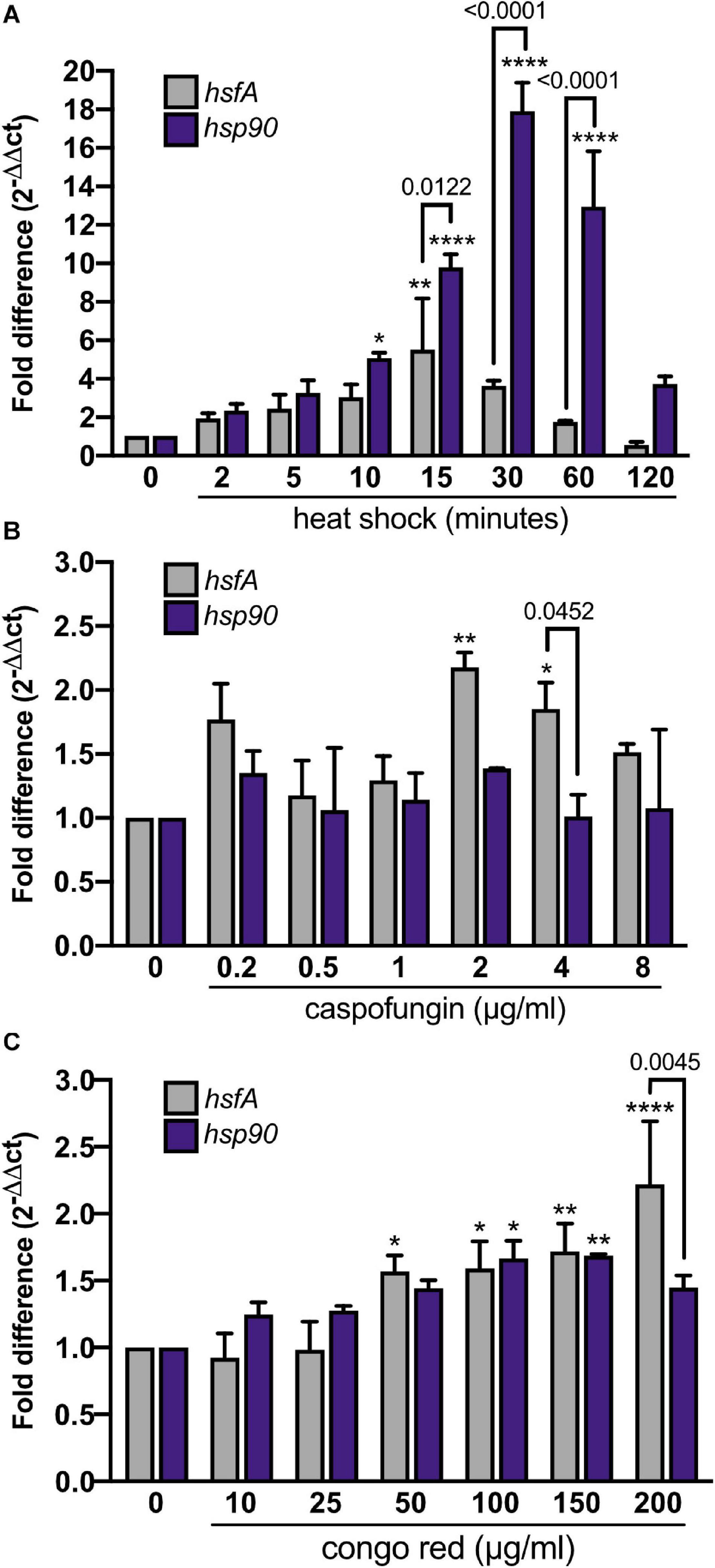
Figure 2. hsfA and hsp90 expression respond to heat shock and cell wall stress. Expression of hsfA and hsp90 was investigated by RT-qPCR. (A) The wild-type strain was grown in MM for 24 h at 30°C and heat-shocked for the indicated time points (min) at 48°C. The wild-type strain was grown in YG for 16 h at 37°C, and cell wall stress was achieved using increasing caspofungin concentrations for 60 min (B) or congo red for 30 min (C). The fold difference of each gene represents the normalized total mRNA in relation to the same gene in the control condition. Mean ± SD (n = 3) are shown. Significant differences were observed by using two-way ANOVA followed by Sidak’s posttest. *p < 0.05, **p < 0.01, and ****p < 0.0001 indicate significant differences from comparisons to the same gene at the control condition. Differences between the genes are indicated by the bars.
To further explore the role of hsfA in the A. fumigatus HS and cell wall stress response, we next attempted to generate an hsfA null mutant. Various deletion strategies and constructions were not successful, and after repeated attempts, no positive transformants were obtained (data not shown), suggesting that under standard laboratory conditions, hsfA is essential for viability in A. fumigatus, as observed for other fungal species, such as S. cerevisiae and C. albicans (Wiederrecht et al., 1988; Nicholls et al., 2009). Subsequently, we decided to generate a conditional xylP::hsfA mutant, in which the hsfA gene is under the control of the P. chrysogenum xylP promoter (Zadra et al., 2000). The substitution cassette contained the pyrG gene as the prototrophy marker, fused to the xylP promoter containing the first 467 bp of the native hsfA promoter (Supplementary Figure 1A). Diagnostic PCR and Southern blot analysis confirmed a single integration event in the conditional mutant (Supplementary Figures 1B,C).
To validate the conditional expression of hsfA in this mutant, the mRNA abundance of hsfA was determined by RT-qPCR, both in the presence of glucose 1% (MM) for repression and in MM supplemented with increasing concentrations of xylose for xylP promoter induction (Figure 3A). The hsfA expression levels in the conditional lethal mutant increased in a xylose concentration-dependent manner (up to 5%), while in the wild-type strain, it remained constant. The results showed that at low xylose concentration (0.06%) hsfA mRNA abundance in the xylP::hsfA mutant was similar to that recorded for the wild-type strain. Consistently, hsfA expression was significantly suppressed in the absence of xylose.
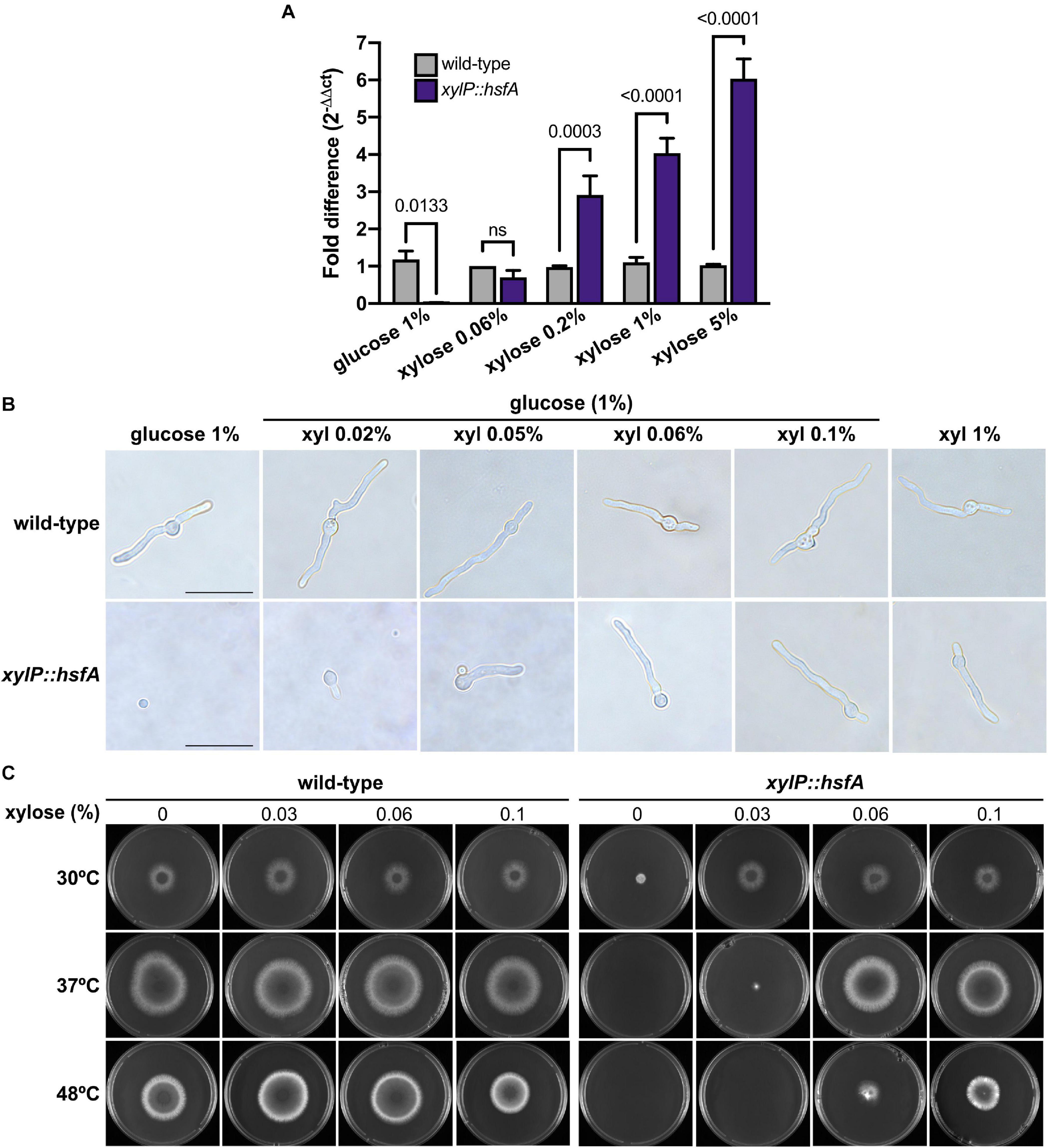
Figure 3. hsfA is essential for vegetative growth and thermotolerance. (A) hsfA expression in the wild-type and conditional lethal xylP::hsfA strain. The strains were grown for 24 h at 37°C in liquid MM supplemented with xylose 1% and transferred to fresh MM or MM lacking glucose supplemented with different xylose concentrations for 4 h. The fold difference of each condition represents the normalized mRNA abundance in comparison to the wild-type strain. Mean ± SD (n = 3) are shown. Statistics are indicated by the bars and p values are depicted (ns: non-significant; two-way ANOVA and Sidak’s multiple comparison test). (B) hsfA is required for A. fumigatus germination. The wild-type and xylP::hsfA strains were cultivated for 12 h at 37°C in glass-bottom dishes containing 2 ml of MM (glucose 1%) supplemented with increasing concentrations of xylose (xyl), or in xylose 1% as the sole carbon source. Magnification 100×; bar = 10 μm. (C) Radial growth of the wild-type and xylP::hsfA strains under repressive (glucose) or inducing conditions (xylose). A total of 1 × 104 conidia of wild-type and xylP::hsfA strains were inoculated into the center of solid MM plates supplemented with the indicated concentrations of xylose and incubated at 30°C, 37°C, or 48°C for 72 h.
In order to evaluate if hsfA repression causes any changes in the germination and structure of A. fumigatus hyphae such as lysis, which could be consistent with cell wall defects, an optical microscopy experiment was conducted in which both the wild-type and the xylP::hsfA strains were cultivated in MM containing different concentrations of xylose for 12 h at 37°C. As expected, the absence of xylose completely inhibited growth of the conditional mutant without noticeably affecting germination of the conidia (Figure 3B). In contrast, the xylP::hsfA mutant started germination with an increase in xylose concentration, achieving a comparable wild-type growth with 0.06 to 0.1% of xylose in the medium. These results indicate that HsfA is essential for A. fumigatus viability. Except for germination inhibition at low xylose concentrations, no change in the mutant hyphae morphology was observed. For comparison, in a medium lacking glucose and supplemented with xylose 1% as the sole carbon source, the conditional mutant grew normally, without the emergence of any hyphae structural alteration (Figure 3B).
Because thermotolerance is a key feature for the A. fumigatus biology (Bhabhra and Askew, 2005) and HsfA may be a major regulator of this attribute, we initially attempted to understand the role played by hsfA in ensuring thermotolerance. The wild-type and the xylP::hsfA strains were cultured in MM supplemented with varying xylose concentrations at different temperatures (Figure 3C). Although under basal condition (30°C), the xylP::hsfA strain under repressive condition showed decreased radial growth compared to the wild-type strain, growth was comparable to that of the wild-type strain when a small concentration of xylose was added to the medium (0.03%). However, at 37°C, the conditional mutant could not grow under repressive conditions, further suggesting the crucial role of hsfA for thermoadaptation of the fungus. The radial growth of the mutant was equivalent to the wild-type strain in the presence of at least 0.06% xylose. For this reason, this concentration of xylose was chosen for the next phenotypic assays below. The xylP::hsfA mutant susceptibility was even higher at 48°C since it could only grow properly when more than 0.1% of xylose was added to the medium (Figure 3C). Altogether, these results suggest that the cell viability is highly dependent on hsfA upon temperature increase.
As our qPCR analysis hinted at the importance of hsfA as an adjunctive transcription factor required to cope with cell wall stress, we next investigated the relevance of hsfA for cell wall maintenance by evaluating the susceptibility of the conditional lethal mutant when grown in low hsfA expression level conditions (0.06% xylose, Figure 3). We observed differences in the conditional mutant susceptibility profile compared to the wild-type strain in the presence of cell wall stressing agents. Among the drugs tested, the highest growth inhibition was recorded for CASP, CAF, and CR treatments (Figure 4A). For these experiments, small increments in the amount of xylose added to the culture media caused recovery of the lethal phenotype, thus making it difficult to determine a xylose concentration to balance viability of the mutant and repression of hsfA to assess susceptibility to these cell wall stressors. Thus, we suggest that the narrow range of xylose concentration that results in a dramatic difference in growth (for instance, compare xylose concentration of 0.03 and 0.06% in Figure 3C) can partially explain the subtle cell wall phenotypes we present here.
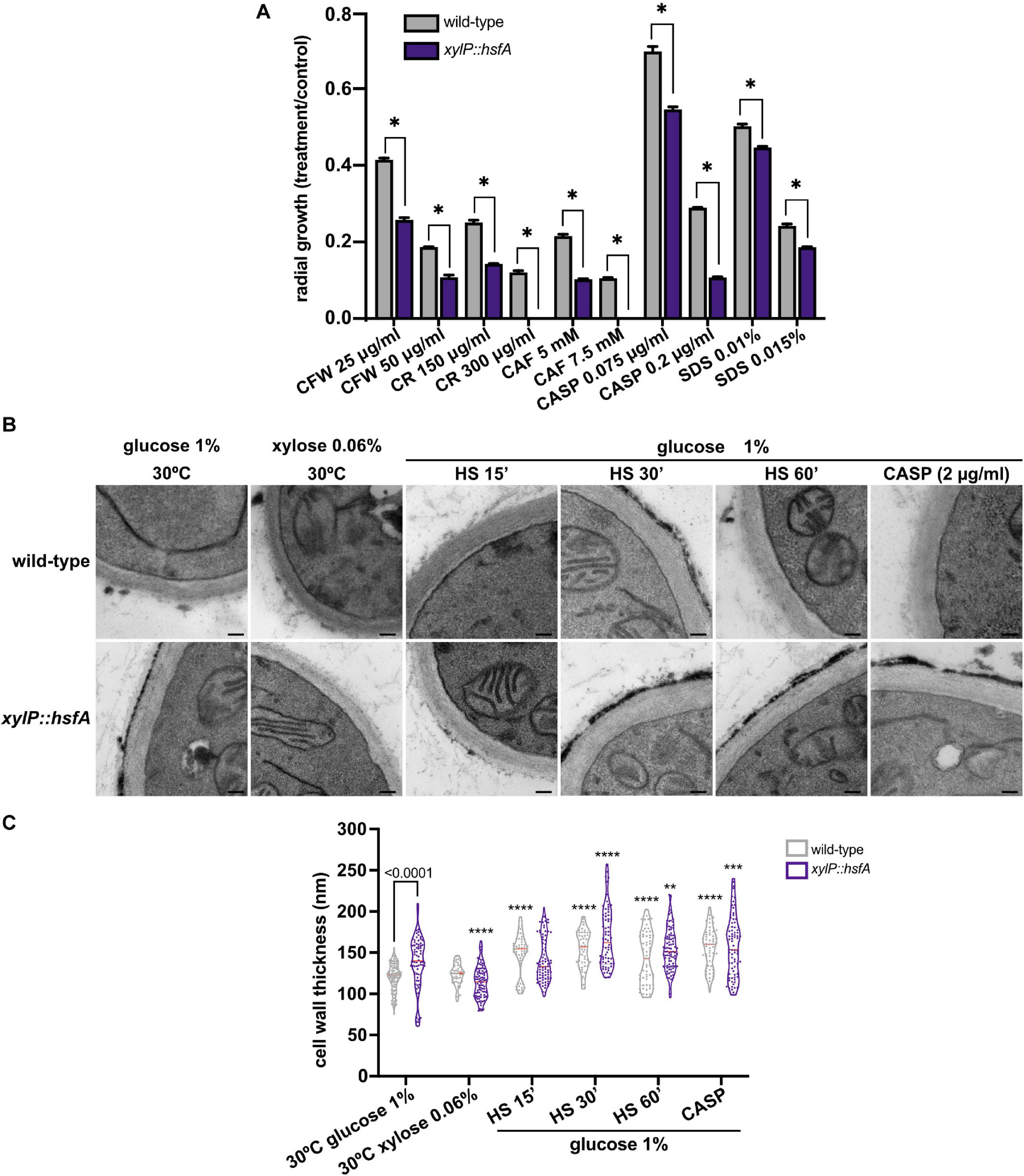
Figure 4. The xylP::hsfA mutant is more susceptible to cell wall stress. (A) A total of 1 × 104 conidia of the wild-type and xylP::hsfA strains were inoculated onto solid MM supplemented with 0.06% xylose (low hsfA expression conditions) and varying concentrations of calcofluor white (CFW), congo red (CR), caffeine (CAF), caspofungin (CASP), and sodium dodecil sulfate (SDS). The plates were incubated at 37°C for 72 h and the ratio of radial growth of treated to the control condition was calculated. The results were expressed as mean ± SD, n = 3. *p ≤ 0.0001 (two-way ANOVA and Sidak’s posttest). (B) HsfA depletion increases cell wall thickness in A. fumigatus. The wild-type and xylP::hsfA strains were grown in liquid MM supplemented with 1% xylose for 36 h at 30°C and further incubated in MM (repression) or MM lacking glucose supplemented with xylose 0.06% (induction) for 4 h at 30°C. Next, the repressed samples were heat-shocked at 48°C for 15, 30, and 60 min or treated with 2 μg/ml of CASP for 60 min, and prepared for transmission electron microscopy analysis. Black bars: 100 nm. (C) Cell wall thickness measurement of the germlings shown in violin plot of 50 sections of different germlings. Dashed lines indicate the quartiles and red lines indicate the median for each condition. Significant differences were observed by using two-way ANOVA followed by Sidak’s posttest. **p < 0.01, ***p < 0.001, and ****p < 0.0001 indicate significant differences from comparisons to the same strain at the control (30°C, glucose 1%) condition. Differences between the strains in the same growth condition are indicated by the bars.
Nevertheless, the results shown in Figure 4A point to a role of hsfA in cell wall homeostasis. In line with this evidence, when the xylP::hsfA conditional lethal mutant was grown under repressive conditions (MM) and analyzed by TEM, we observed a significant cell wall thickening (15%) at non-HS basal condition (30°C), which was fully recovered when 0.06% xylose was added to the culture medium (Figures 4B,C), further suggesting that hsfA plays a crucial role controlling the expression of cell wall-related genes. As expected, the cell wall thickness of the wild-type strain increased during the HS and during cell wall stress caused by CASP. In contrast, the cell wall thickening of the xylP::hsfA conditional mutant was similar to the wild-type strain under the two stress conditions, although significantly different from the control condition (1% glucose, 30°C), where the cell wall is constitutively thicker. These results suggest that hsfA is required for typical cell wall architecture under basal conditions, while hsfA loss of function retains the ability to remodel the cell wall ultrastructure when challenged with CASP and HS.
In addition to temperature stress tolerance, Hsf1 in yeast is also induced by oxidative stress, ethanol exposure, and glucose starvation (Liu and Thiele, 1996; Hahn and Thiele, 2004; Hashikawa et al., 2007). Consistently, the xylP::hsfA strain showed increased sensitivity to all oxidative stress compounds tested (menadione, hydrogen peroxide, and diamide) in comparison to the wild-type strain, except for paraquat (Supplementary Figure 2). The conditional mutant was also more susceptible to the crop fungicide fludioxonil (Supplementary Figure 2B). In contrast, there were no growth differences between the conditional mutant and the wild-type strain in the presence of osmotic stress caused by high concentrations of sorbitol (data not shown). These results suggest a role for hsfA in oxidative stress detoxification in A. fumigatus.
HsfA Genetically Interacts With the MAPKs mpkA and sakA
Previous reports suggested that A. fumigatus cell wall undergoes continuous remodeling in response to environmental stimuli or stress conditions (Rocha et al., 2020a). Under these circumstances, the biosynthesis and reinforcement of the cell wall rely on the concerted actions of the CWI pathway and the High Osmolarity Glycerol (HOG) pathway since both cascades have overlapping functions to promote adaptation to cell wall-targeting and temperature stresses (Altwasser et al., 2015; Rocha et al., 2015, 2020b; Bruder Nascimento et al., 2016). Also, the MAPK cascades associated with these circuits crosstalk with the Hsf1 transcription factor through the action of the Hsp90 in S. cerevisiae and C. albicans (Lamoth et al., 2012; Leach et al., 2012a). As hsfA repression caused cell wall phenotypes, we asked whether this transcription factor genetically interacts with the apical kinase (pkcA) and the MAPK (mpkA) of the CWI pathway and the MAPK of the HOG pathway (sakA). Accordingly, double conditional mutants of xylP::hsfA with pkcAG579R allele and ΔmpkA, or ΔsakA were constructed (Supplementary Figures 1D–I).
Phenotypic tests with these double mutants were conducted under low xylose concentration to titrate out the expression of hsfA and score genetic interactions when hsfA is limiting inside the cell, since the recovery of hsfA expression occurs in largely low xylose concentration, as mentioned above (Figure 3). We observed that mpkA and sakA genetically interact with hsfA upon growth at 37°C and 48°C since the respective double conditional mutants grew less at lower xylose concentrations than the parental strains (Figure 5A). The same results were observed during cell wall stress caused by CASP (Figure 5B), CR, and CFW (Supplementary Figures 3A,B), as well as in the presence of SDS (Supplementary Figure 3C). Consistently, when the xylose concentration is increased to 0.1–0.5%, growth of the double conditional mutant is completely rescued, indicating that restoration of hsfA expression to wild-type levels is sufficient to fully recover the phenotype in the absence of either mpkA or sakA. These results also point out that mpkA and sakA are not essential for hsfA activation during thermoadaptation and cell wall stress.
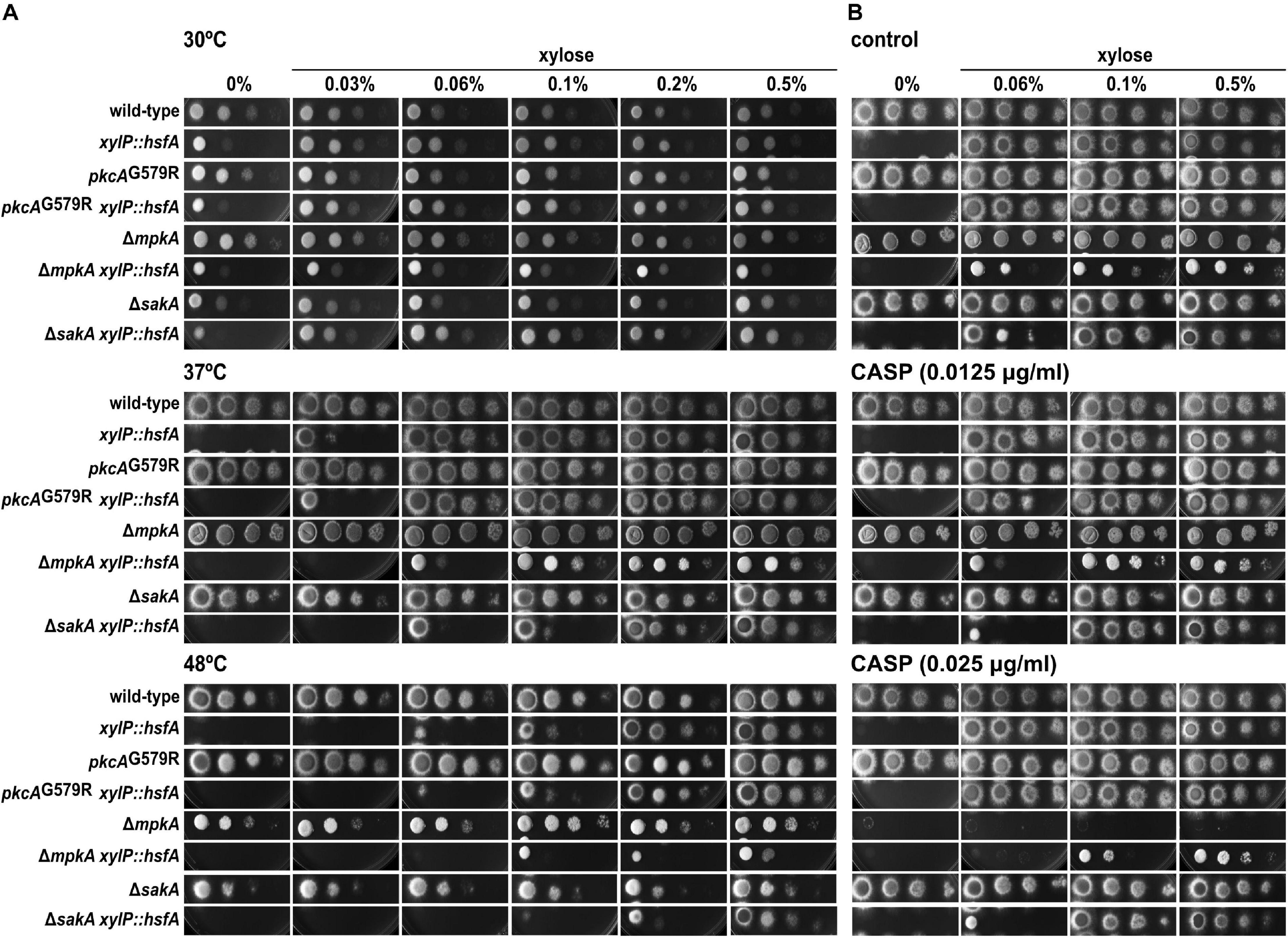
Figure 5. hsfA genetically interacts with mpkA and sakA during temperature and cell wall stresses. Tenfold dilution series of conidia from the wild-type, xylP::hsfA, pkcAG579R, pkcAG579R xylP::hsfA, ΔmpkA, ΔmpkA xylP::hsfA, ΔsakA, and ΔsakA xylP::hsfA strains was inoculated into solid MM containing different concentrations of xylose. (A) Plates were incubated at 30°C, 37°C, or 48°C for 48 h. (B) 0.0125 μg/ml and 0.025 μg/ml of caspofungin (CASP) were used to induce cell wall stress and the plates were incubated at 37°C for 48 h.
HsfA and Hsp90 Act Together to Endure Cell Wall Stress and HS via the CWI Pathway
Given the above reported synthetically sick genetic interactions between hsfA and the MAPKs of the CWI and HOG pathways, we used the luciferase (luc) reporter gene to investigate differences in HsfA protein expression during HS and cell wall stress induced by CASP. The rationale behind this experiment was to examine a role of these MAPKs in regulating posttranscriptional levels of HsfA. Hence, single and double mutants containing HsfA protein controlled by its native promoter and C-terminally fused to the luciferase protein were constructed (Supplementary Figures 1J–P). All the reporter strains, with the exception of ΔsakA hsfA::luc, showed no growth defects compared to the parental strains, indicating that the replaced alleles are fully functional (Supplementary Figure 4). Since the viability and growth of several ΔsakA hsfA::luc transformation mutants were impaired under HS conditions compared to the parental strains (data not shown), the evaluation of the hsfA expression was not possible in this mutant.
The luciferase activity was recorded after exposing the germlings of the reporter strains to HS and CASP. We observed that HsfA protein expression was rapidly induced after 15 min of HS at both 37°C (Figure 6A) and 48°C (Figure 6B) in the biofilm of all strains. These results are consistent with the peak in mRNA abundance recorded under the same conditions for the wild-type strain (Figure 2A), thus validating our HsfA reporter strain. However, the luciferase signal was more intense in the strains carrying the pkcAG579R and ΔmpkA mutations at both temperatures. In these genetic backgrounds, sustained high HsfA levels were recorded at 37°C up to 120 min of HS. Although, at 48°C, the same HsfA expression peak was detected after 15 min, the luciferase signal returned to wild-type levels after the 30 min of HS adaptation. These results highlight the importance of hsfA and the CWI pathway in the thermoadaptation of A. fumigatus to the mammalian host environment.
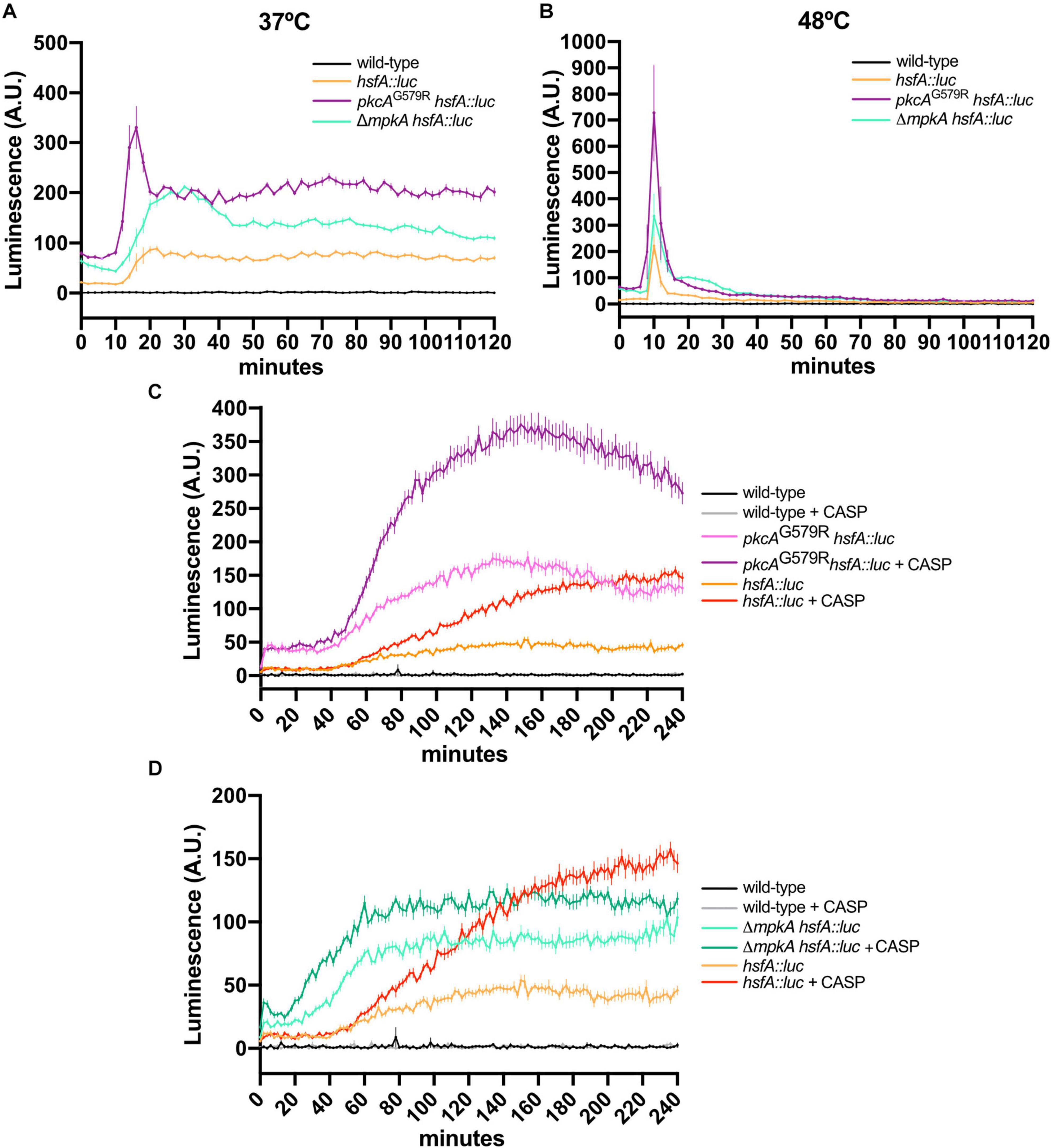
Figure 6. The loss of function of CWI regulators increases HsfA expression in A. fumigatus biofilms under HS and cell wall stress. (A,B) Luciferase activity assay of the hsfA::luc, pkcAG579R hsfA::luc, and ΔmpkA hsfA::luc strains during the HS at 37°C (A) and 48°C (B). (C,D) Luciferase activity assay of the pkcAG579R hsfA::luc (D) and ΔmpkA hsfA::luc strains during cell wall stress caused by CASP. The wild-type strain without luciferase gene was used as the negative control in all experiments. Mean ± SEM (n ≥ 8) are shown. The results were normalized by the number of conidia (2 × 105 per assay) and are expressed as luminescence (arbitrary units). CASP: caspofungin (2 μg/ml).
The expression of HsfA was also significantly induced by CASP treatment after 60 min of drug exposure (Figures 6C,D), similarly to the mRNA hsfA levels recorded under the same conditions in the wild-type strain (Figure 2B). Interestingly, the HsfA high levels were maintained up to 240 min of treatment. Again, we observed a positive synergistic effect in the HsfA expression when the pkcAG579R mutant allele was combined with the CASP exposure (Figure 6C). The same synergistic effect was also observed in the combination of CASP and the ΔmpkA mutant (Figure 6D). We also conclude that pkcA and mpkA are not required for HsfA expression, and the loss of function of these kinases enhances the accumulation of HsfA possibly via undescribed compensatory mechanisms to retain the CWI and thermotolerance.
Given the hsp90 levels are critical for the HsfA transcription and to probe further into the potential mechanisms underlying the enhanced HsfA expression in the CWI pathway mutants, we assessed the activity of the hsp90 promoter (hsp90P) under the same conditions we used above to trace the HsfA protein accumulation. We constructed CWI pathway mutants in which the hsp90P was fused to the luciferase gene to generate relevant reporter strains (Supplementary Figures 1Q–W). For these experiments, we also included the deletion strain of the CWI pathway-associated transcription factor RlmA. As expected, hsp90P activity was rapidly upregulated during HS in the hsp90P::luc strain at 37°C (Figure 7A) and 48°C (Figure 7B), peaking at 15–20 min post-HS. At 37°C, there were no significant differences in the luminescence signal between the wild-type, pkcAG579R, and ΔrlmA backgrounds.
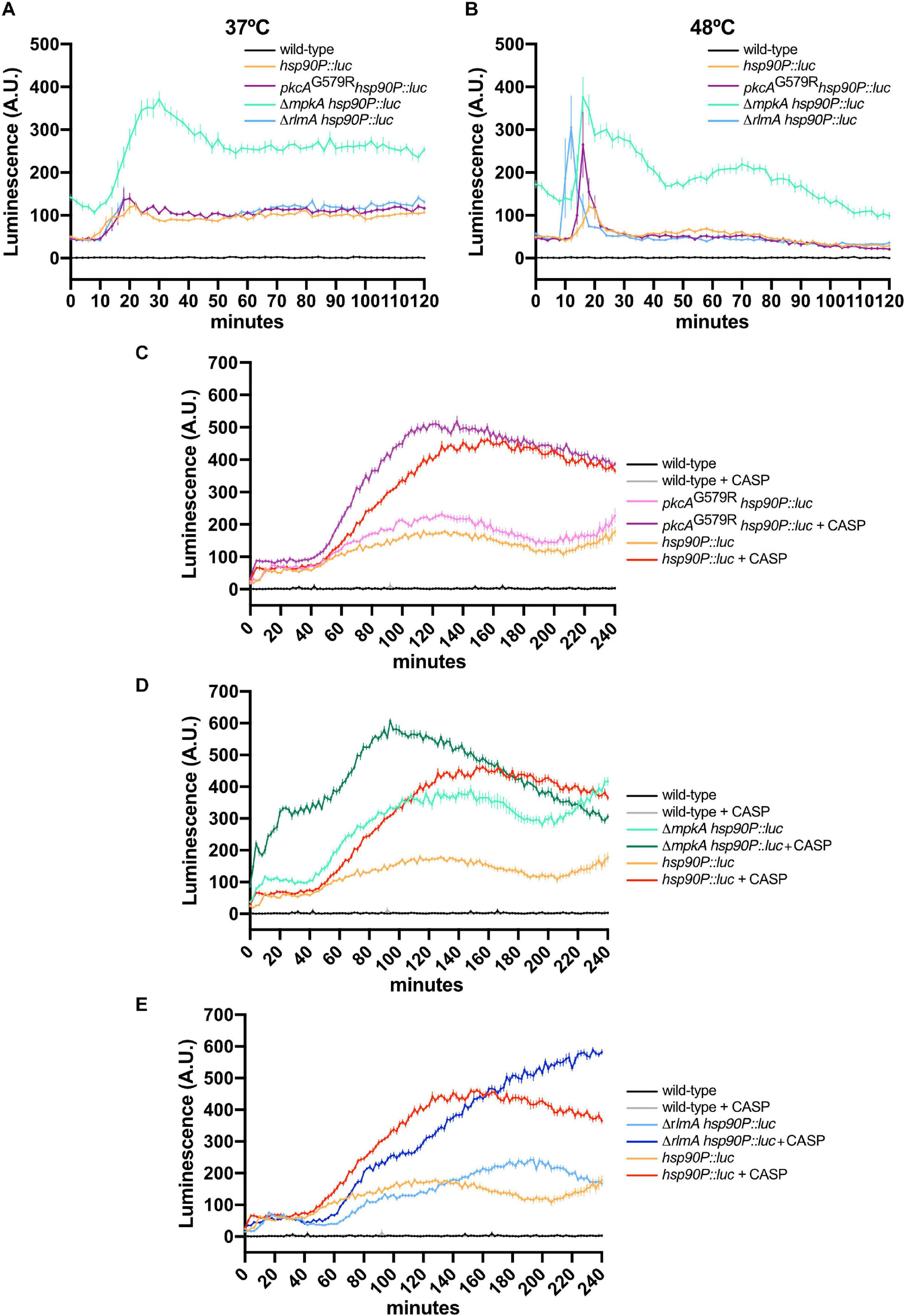
Figure 7. The loss of function of CWI regulators increases hsp90 expression in A. fumigatus biofilms under HS and cell wall stress. (A,B) Luciferase activity assay of the hsp90P::luc, pkcAG579R hsp90P::luc, ΔmpkA hsp90P::luc, and ΔrlmA hsp90P::luc strains during the HS at 37°C (A) and 48°C (B). (C–E) Luciferase activity assay of the pkcAG579R hsp90P::luc (C), ΔmpkA hsp90P::luc (D), and ΔrlmA hsp90P::luc (E) strains during cell wall stress caused by CASP. The wild-type strain without luciferase gene was used as the negative control in all experiments. Mean ± SEM (n ≥ 8) are shown. The results were normalized by the number of conidia (2 × 105 per assay) and are expressed as luminescence (arbitrary units). CASP: caspofungin (2 μg/ml).
In contrast, the mpkA deletion strongly induced the hsp90P throughout the experiment, even under basal conditions (time point 0). At 48°C, a peak of hsp90P activity occurred earlier in all mutant strains compared to the wild-type reporter, and this effect was more evident in the ΔrlmA deletion background. Similar to the results scored at 37°C, the higher induction of hsp90P occurred in the mpkA deletion strain. Altogether, these data (Figures 6, 7) confirm our previous results that the HS response in the A. fumigatus biofilm is dysregulated when the CWI pathway is impaired (Rocha et al., 2020b) and reveal that pkcA and mpkA are also the primary sensors for the compensatory activation of both hsfA and hsp90 expression.
Finally, hsp90P activity was also highly induced during the cell wall stress caused by CASP (Figure 7). Interestingly, this effect was similar for the pkcAG579R and ΔmpkA mutants, which peaked after 100–120 min post-CASP treatment. However, the luminescence signal was higher in the ΔmpkA strain under basal conditions. In contrast, the luciferase signal increased later in the ΔrlmA strain and was kept at high levels after the signal of the hsp90P activity decreased in the other CWI pathway mutants (Figures 7C,D). Collectively, the luciferase assays indicate that the expression of HsfA and Hsp90 is connected, and both jointly work in response to HS and cell wall stress. Additionally, the results suggest a direct HsfA role in governing the expression of genes involved in the cell adaptation to these stressing conditions as noted by the compensatory increase in the HsfA expression in the CWI pathway mutants.
HsfA Repression Modulates the Expression of Genes Related to Cell Wall Homeostasis and HS Response
In order to assess HsfA-dependent gene expression targets during HS, the wild-type and xylP::hsfA transcriptomes were investigated during HS exposure for 15 and 60 min at 48°C, after xylP::hsfA was repressed in MM lacking xylose, as described in the Materials and Methods section. The first time point (15 min) corresponds to the peak in hsfA expression at mRNA and protein levels (Figures 2A, 6B). The exposure of A. fumigatus for 60 min causes an overall 20% reduction of the metabolic activity of mature wild-type A. fumigatus biofilm (Rocha et al., 2020b) and does not cause loss of viability of the xylP::hsfA conditional lethal mutant when shifted to repressive conditions. Thus, these two time points allow us to identify early and late genes induced by HS.
HS elicited rapid modulation of gene expression since 1,362 genes were upregulated (log2FC ≥ 1.0) while 1,499 genes were downregulated (log2FC ≤ −1.0) in the wild-type strain post-15 min. After 60 min of HS, these numbers increased to 1,384 and 1,549, respectively (Figures 8A,B). In the xylP::hsfA strain under repression, 1,375 genes were upregulated (log2FC ≥ 1.0) while 1,729 genes were downregulated (log2FC ≤ −1.0) post-15 min of HS. After 60 min HS, these numbers decreased to 1,136 and 1,474, respectively (Figures 8A,B).
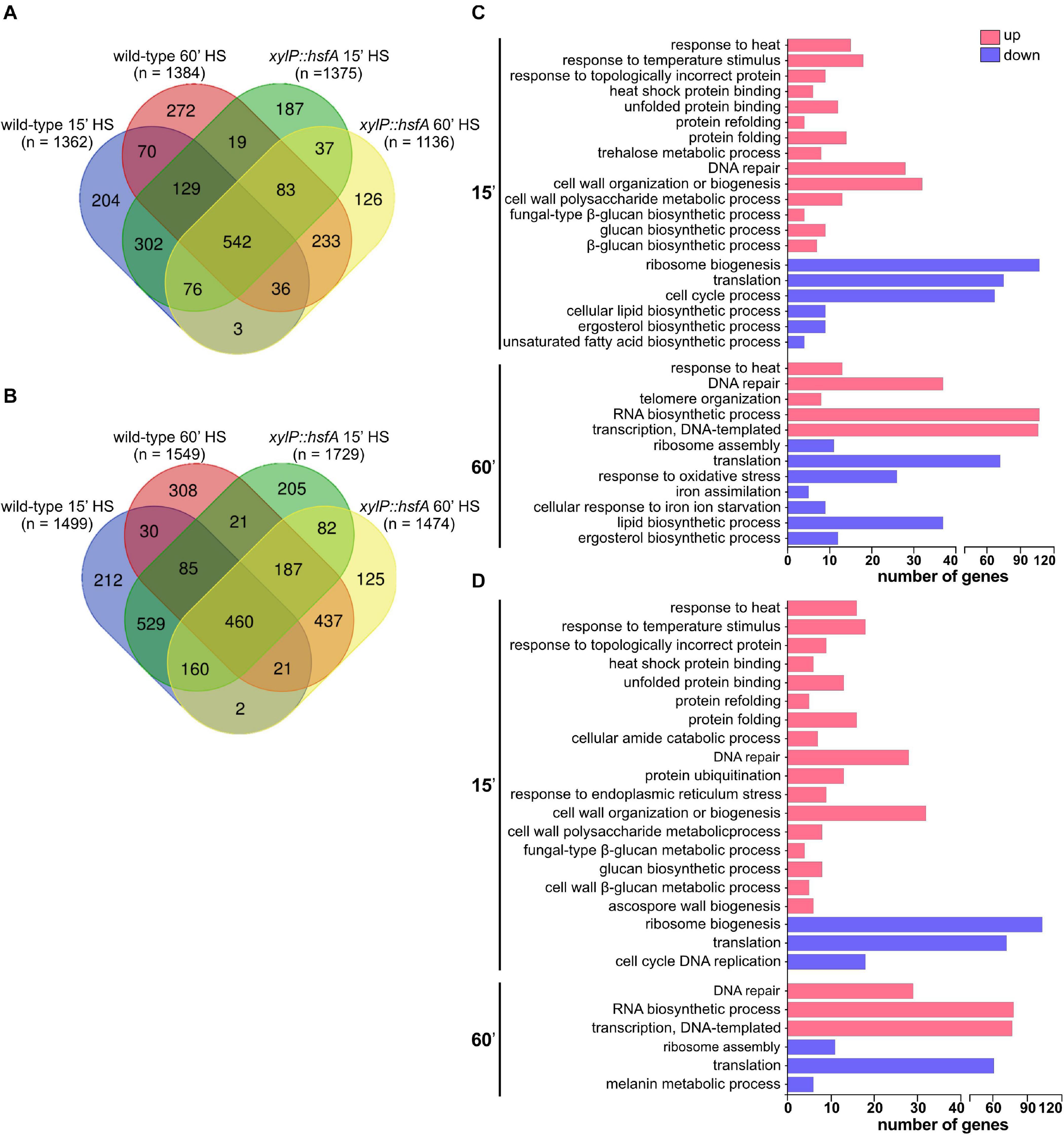
Figure 8. Global transcriptional response of the wild-type and xylP::hsfA strains to HS. Venn diagram depicting the number of upregulated (log2FC ≥ 1.0) (A) and downregulated (log2FC ≤ –1.0) (B) genes in the wild type and xylP::hsfA strains post-15 min and 60 min of HS. (C) Selected Biological Process Gene Ontology terms enriched from significantly differently expressed genes (log2FC ≥ 1.0 or log2FC ≤ –1.0) in the wild-type strain during HS (15 min and 60 min time points), in comparison to control condition (30°C). (D) Selected Biological Process Gene Ontology terms enriched from significantly differently expressed genes (log2FC ≥ 1.0 or log2FC ≤ –1.0) in the xylP::hsfA strain during HS compared to control condition (30°C). For the full Biological Process Gene Ontology terms list, refer to Supplementary Table 5.
Intriguingly, the xylP::hsfA mutant showed a GO enrichment similar to the wild-type strain after 15 min of HS. Both strains demonstrated a transcriptional upregulation of genes involved in the cellular response to heat, protein folding, and refolding (Figures 8C,D), which are expected biological processes activated in response to temperature increase (Albrecht et al., 2010). Some of these genes were also differentially expressed at the protein level under HS, such as the chaperones Hsp90 (Afu5g04170), Hsp30 (Afu3g14540), Hsp70 (Afu1g07440), Hsp60 (Afu2g09290), Hsp78 (Afu1g11180), Hsp88 (Afu1g12610), Ssc70 (Afu2g09960), and Sti1 (Afu7g01860), as previously reported (Albrecht et al., 2010). The early HS response also caused significant upregulation of genes involved in glucan biosynthetic process and cell wall organization or biogenesis in both strains (Figures 8C,D), including the CWI pathway transcription factor rlmA (Afu3g08520); the catalytic subunit of the β-1,3-glucan synthase fksA (Afu6g12400); the β-1,3-glucanosyltransferases gelA (Afu2g01170) and gel7 (Afu6g12410); the chitin synthases chsE (Afu2g13440), chsF (Afu8g05630), and csmB (Afu2g13430); and the MAPK kinase of the HOG signaling pathway pbs2 (Afu1g15950). These results support the cell wall remodeling observed during HS (Figure 1). In contrast, only the wild-type strain showed enrichment of genes involved in trehalose biosynthesis (Figure 8C), suggesting the participation of HsfA in this process. At the same time, protein ubiquitination and the response to endoplasmic reticulum stress were processes enriched only in the repressed xylP::hsfA mutant (Figure 8D). Among the downregulated genes post-15 min of HS, there was an enrichment of ribosome biogenesis and translation processes in both the wild-type and mutant strains (Figures 8C,D), suggesting an abrogation of protein synthesis to sustain thermoadaptation. The GO analysis of downregulated genes also showed enrichment in the category involved in the ergosterol biosynthetic process and unsaturated fatty acid biosynthetic process in the wild-type strain (Figure 8C). These findings reflect the cellular balance to overcome increased membrane fluidity caused by temperature increase and suggest that HsfA has a role in lipid homeostasis and the balance in the content of saturated/unsaturated fatty acids ratio elicited by the HS.
After 60 min of HS, the upregulated genes in both strains were enriched for different metabolic processes, such as DNA repair, RNA biosynthetic process, and transcription, while the downregulated genes were involved in ribosome assembly and translation (Figures 8C,D). The profile of genes modulated after prolonged HS exposure excluded the categories involved in cell wall metabolism. Interestingly, only the wild-type strain retained an enrichment of upregulated genes involved in response to HS after 60 min, supporting the evidence of a late hsfA role during the HS. Again, the lipid and ergosterol biosynthetic processes were also found downregulated only in the wild-type strain. The iron assimilation and cellular response to iron ion starvation categories were also downregulated exclusively in the wild type (Figure 8C), which is in agreement with previous studies that have shown a relationship between Hsf1 and the cellular iron pool in C. albicans (Nair et al., 2017, 2018).
Subsequently, to identify genes whose expression was directly influenced by hsfA, we compared the xylP::hsfA mutant grown on repressive conditions with the wild-type strain, and we observe that several genes are modulated either at the non-HS control condition (30°C) or during temperature stress (Figures 9A,B). Some of them were constitutively repressed or induced in the mutant strain, including the hsfA gene, which is repressed throughout the experiment, thus validating our experimental conditions (Supplementary Figure 5). Notably, four genes of the pyripyropene biosynthetic gene cluster (pyr4, pyr5, pyr6, and pyr9) were highly induced in the mutant strain. Consistently, other genes belonging to this secondary metabolite cluster, such as pyr1 (Afu6g13920), pyr3 (Afu6g13940), and pyr8 (Afu6g14000), were upregulated both in the non-HS and at the 60-min time point (Supplementary Table 4). These data suggest that HsfA has a role in the biosynthesis of this meroterpenoid.
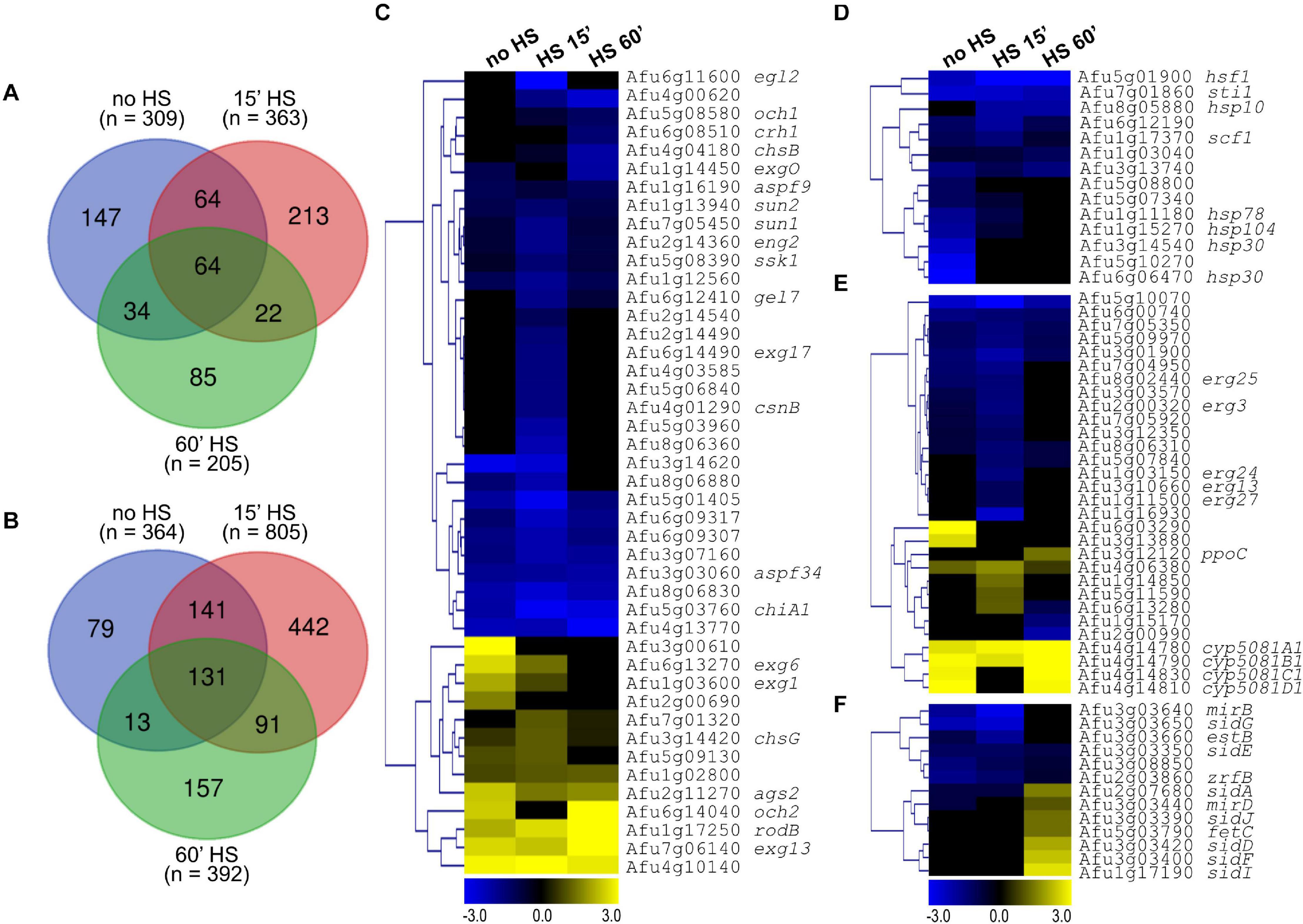
Figure 9. hsfA repression impacts the expression of genes related to HS, cell wall, lipid, and iron metabolism. Venn diagrams comparing the genes upregulated (log2FC ≥ 1.0) (A) and downregulated (log2FC ≤ –1.0) (B) in the xylP::hsfA mutant strain relative to the wild-type strain at no HS condition and post-15 min or 60 min of HS. (C–F) Hierarchical clustering analysis showing selections of differently expressed genes (log2FC ≥ 1.0 or log2FC ≤ –1.0). The heat maps are divided by processes and categories of interest: cell wall biosynthesis and organization (C); heat shock response, chaperone activity, and protein folding (D); lipid metabolism (E); and iron metabolism (F). All the heat maps were created in the Multiple Experiment Viewer (MeV) platform, using hierarchical clustering and Euclidean Distance with average linkage clustering.
Focusing on the genes with participation on the cell wall organization, we observed few genes involved in the biosynthesis of cell wall, such as the α-1,3-glucan synthase ags2 (Afu2g11270) and the chitin synthase chsG (Afu3g14420), which were upregulated, and β-1,3-glucanosyltransferase gel7 (Afu6g12410) and the chitin synthase chsB (Afu4g04180), which were downregulated in at least one time point. In contrast, a number of genes involved in the remodeling of the cell wall were identified including the β-1,3-exoglucanases exg1 (Afu1g03600) and exg6 (Afu6g13270), the glycosyl hydrolase Afu4g13770, the chitinases chiA1 (Afu5g03760), Afu5g03960 and Afu5g06840, and the glucanases eg12 (Afu6g11600), aspf9 (Afu1g16190), exgO (Afu1g14450), Afu1g12560, Afu8g06830, Afu2g14490, and Afu8g06360 (Figure 9C). Noteworthy, the more evident alterations in the mRNA abundance of most of these cell wall-related genes were a noticeable repression post-15 min of HS, when the expression of HsfA reaches its peak (Figures 2A, 6B), suggesting that hsfA has a role in the transcriptional activation of such genes and is important for cell wall metabolism during thermoadaptation.
As expected, many HSPs, such as sti1 (Afu7g01860), hsp10 (Afu8g05880), scf1 (Afu1g17370), hsp78 (Afu1g11180), hsp104 (Afu1g15270), hsp30/42 (Afu3g14540), hsp20/26 (Afu5g10270) and hsp30 (Afu6g06470), were repressed in the mutant strain in comparison to the wild type (Figure 9D), also indicating that they are putative HsfA transcriptional targets. In addition, several genes related to lipid metabolism, mainly those involved in ergosterol and fatty acids biosynthesis, were downregulated, especially at no HS condition and post-15 min of HS (Figure 9E), pointing to a rearrangement of the cellular plasma membrane to increase its fluidity in response to thermal insult (Leach and Cowen, 2014). Finally, siderophore metabolic genes were also considerably modulated and divided into two groups: one containing downregulated genes at basal condition and post-15 min of HS, and another comprising upregulated genes post-60 min of HS (Figure 9F). This last group coincides with the repression of the GO terms related to iron metabolism in the wild-type strain at that time point (Figure 8C). Independent RT-qPCR experiments validated the RNA-seq results for the six selected genes that represent the GO categories shown in Figure 9. We investigated the mRNA levels of hsfA, hsp30, ags2, chsG, ppoC, and sidA and presented the results as the ratio xylP::hsfA/wild-type (Supplementary Figure 6).
Collectively, our RNA-seq results strongly suggest that temperature stress causes major changes in the A. fumigatus transcriptome, and the transcription factor HsfA is a key regulator in the modulation of a significant number of these genes, being especially important for the response to HS, cell wall remodeling, plasma membrane homeostasis, and iron metabolism.
Given that thermotolerance and CWI are reciprocally controlled, and multiple transcription factors are intertwined to regulate these two polygenic biological processes, we analyzed our dataset to identify transcription factors differentially expressed in the wild-type and the xylP::hsfA strains exposed to HS. We concentrated our analysis in the transcription factors that presented differential expression values (log2FC ≥ 1.0 or log2FC ≤ −1.0) in at least one time point by comparing in this analysis the wild-type versus the xylP::hsfA. This search identified many transcription factors, including several of them uncharacterized (Supplementary Figure 7).
The putative transcription factors encoded by the genes Afu17060, Afu1g17150, Afu1g15370, Afu4g06420, and Afu4g07090 (znfA) were upregulated only in the mutant strain in at least one time point of HS, suggesting that they may play a role similar to that of HsfA during the HS response when hsfA is repressed. Concomitantly, Afu2g03020, Afu5g01662, Afu6g01840, Afu7g01820, and Afu1g01340 were genes upregulated only in the wild-type strain (Supplementary Figure 7), indicating that these genes may be direct or indirect regulated by HsfA. Afu7g06590, Afu3g06940, Afu8g05010 (zpfA), Afu1g11290, Afu5g00435, Afu5g10040, and Afu1g03210 (flbD) were among the downregulated genes only in the xylP::hsfA mutant strain in at least one time point of HS (Supplementary Figure 7). RgdA (Afu3g13920) is a transcriptional factor important for conidiation and cell wall architecture (Jun et al., 2020). Interestingly, in our analysis, this gene was considerably upregulated after 60 min of HS only in the wild-type strain, suggesting a possible target of HsfA regulatory network. Moreover, the gene encoding the transcription factor RttA (Afu7g04740) was also recently identified as playing a role in adaptation to the azole tebuconazole (Toyotome et al., 2020), and here it was downregulated in the wild-type strain post-60 min of HS. Altogether, the transcriptome analysis of transcription factors revealed probable additional regulators of the A. fumigatus HS response.
Discussion
Although endothermy is a mammalian protective mechanism against fungal infections (Robert and Casadevall, 2009; Bergman and Casadevall, 2010), thermophilic fungi such as A. fumigatus can overcome this obstacle, indicating that thermotolerance in this fungus may be related to the expression of stress response genes that support its persistence inside the host. However, the cellular consequences of A. fumigatus adaptation to heat, the impact on the cell wall organization, and the participation of the signaling pathways that coordinate these two events are not fully understood.
Recently, we demonstrated that in addition to the regulation of cell wall biosynthesis and maintenance, the CWI pathway activity is modulated during early regulation of A. fumigatus thermotolerance and showed that Hsp90 is necessary for this crosstalk by interacting with the mains players of the CWI signaling cascade (Rocha et al., 2020b). Hsp90 is fundamental for the eukaryotic HS response by promoting the folding and assembly of newly synthesized proteins (Taipale et al., 2010). Since the conserved transcription factor Hsf1 controls HSP90 transcription (Sarge et al., 1993; Eastmond and Nelson, 2006; Nicholls et al., 2009; Leach et al., 2016), here we identified and characterized the putative A. fumigatus HSF1 homolog and assigned its function in thermotolerance and the associate cell wall stress response, which we hypothesize is a key fungal response mechanism to heat (Figure 10). We revealed that HsfA is an essential transcription factor necessary for thermotolerance and vegetative growth, as observed in other fungi (Wiederrecht et al., 1988; Thompson et al., 2008; Nicholls et al., 2009; Yang et al., 2017).
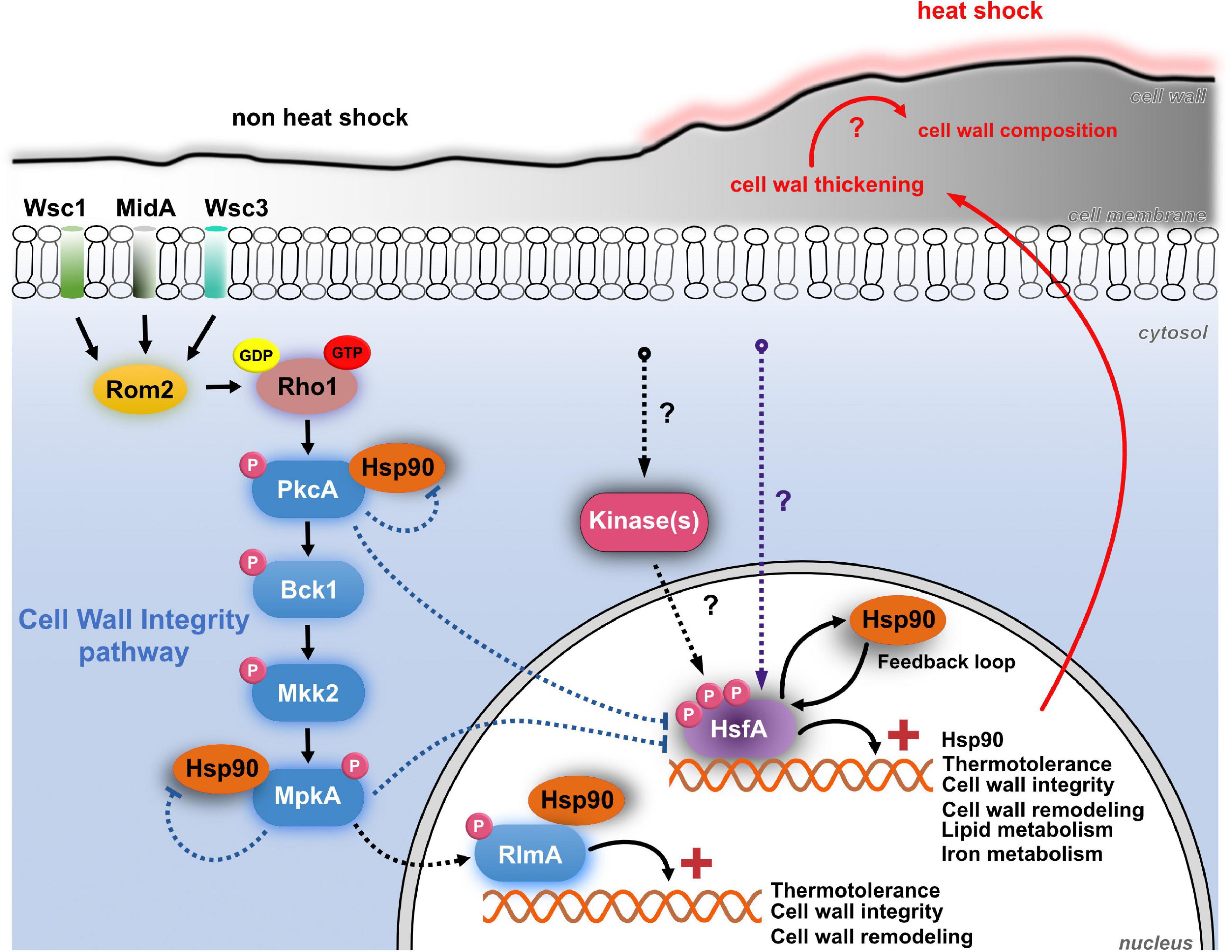
Figure 10. The crosstalk between HsfA-Hsp90 and CWI pathway in A. fumigatus during HS. The CWI pathway is activated during early adaptation to HS, possibly via MidA mechanosensor located at the cell membrane. This signal funnels into Rom2, a guanine nucleotide exchange factor, and the small Rho GTPAse Rho1, leading to the activation of PkcA and the CWI pathway. These events culminate with the phosphorylation of the MAP kinase MpkA, which, in turn, phosphorylates the transcription factor RlmA, thus activating the transcription of genes (+) important for thermotolerance, cell wall integrity, and remodeling. The CWI pathway proteins PkcA, MpkA, and RlmA are constitutive Hsp90 clients. A direct consequence of the HS is the thickening of the cell wall after 5 min of temperature increase, which may have consequences to the remodeling of the cell wall and exposure of carbohydrate to the cell surface. However, these consequences remain to be investigated (red question marks). Also, the cellular plasma membrane becomes more fluid in response to thermal insult. Although not necessary for HsfA activation, PkcA and MpkA negatively regulate the expression of HsfA and Hsp90, both during the HS (dashed blue arrows) and upon cell wall stress induced by caspofungin (not shown in the Figure; see text for details). The resulting increase in protein abundance of HsfA and Hsp90 in the pkcA and mpkA mutants possibly occurs via undescribed compensatory mechanisms accompanied by the activation of additional signaling cascades to retain the CWI and thermotolerance (purple dashed line). In the face of heat stress, mRNA abundance of HsfA increases and triggers the expression of genes related to thermotolerance, cell wall integrity, cell wall remodeling, lipid, and iron metabolism. One of its transcriptional targets, Hsp90, is also induced to deal with HS and assist the stabilization of client proteins. After about 15–30 min of HS, the HsfA levels decrease, possibly due to the action of Hsp90 in the regulatory feedback loop. This regulatory mechanism comprises the Hsf1/Hsp90 protein–protein interaction (not investigated here), which is reversed during the HS, when Hsp90 protein levels increase and decouple from Hsf1 to assist the folding of client proteins. The activity of Hsf1 remains until Hsp90 again binds to Hsf1, negatively regulating Hsf1 (see text for details). Hyperphosphorylation (not investigated here) is another regulatory event that modulates the transcriptional activity of HsfA orthologs in other fungal pathogens. The protein(s) kinase(s) responsible for HsfA phosphorylation are still unknown in A. fumigatus. This diagram is based on data from this article and the references (Dichtl et al., 2012; Leach et al., 2012b; Rocha et al., 2020a, b).
Here, we provide strong evidence that an outcome of the heat stress is the exacerbated thickening of the cell wall in A. fumigatus (Figures 1, 4) accompanied by the induction of genes involved in cell wall biogenesis and remodeling (Figure 8). These results suggest that the cell wall expansion is necessary to sustain thermoadaptation without loss of viability. Increased cell wall thickness has also been recently reported in C. albicans biofilm hyphae during mild heat stress (Ikezaki et al., 2019). However, our results reveal that such modifications in cell wall architecture are very rapidly sensed by the cells since we detect a thickening of this structure as early as after 5 min of HS exposure (Figure 1). However, we should also consider that this rearrangement process in the cell wall observed at the initial times, such as after 5 min of HS, can be an event not exclusively directed by the activity of the CWI pathway or the transcriptional activity of HsfA. For instance, it could also be related to physical changes in the cell surface, such as increased Brownian movement of water molecules or expansion of proteins and polysaccharides in the cell wall. Additional experimentation is required to address this hypothesis. Nevertheless, these data point out that the morphological alterations of the cell wall during HS potentially result in modifications in the synthesis or exposure of specific sugars on the cell surface, e.g., α-glucan, β-glucan, chitin, mannoproteins, or galactosaminogalactan (Figure 10). Since the invading conidia germinate and eventually proliferate at high-temperature conditions inside the host, compared to the environmental niche, such cell wall adaptation may also exist during the initial steps of fungal infection and can be a determinant for immune recognition. However, the consequences of these alterations for the immune recognition of the fungus still need rigorous investigation. In C. albicans, repression of HSP90 expression in a tetO-HSP90 conditional mutant leads to increased detection of chitin by CFW fluorescence binding assay (Leach et al., 2012a; Nair et al., 2017). Likewise, depletion of Hsp90 in A. fumigatus caused increased sensitivity to cell wall stress (Lamoth et al., 2012).
Interestingly, our data demonstrate that the ability of the cell wall to be remodeled during the heat stress or CASP treatment was maintained in the xylP::hsfA mutant, indicating that this process is not entirely dependent on this transcription factor, even though the conditional lethal mutant xylP::hsfA is sensitive to cell wall-damaging agents, including CASP. However, under the non-HS condition (30°C), the repression of hsfA instantly causes a thickening of the cell wall (Figure 4), similar to the C. albicans HSF1 (Nair et al., 2017) and HSP90 conditional mutants (Leach et al., 2012a). In our case, we show that the cell wall thickening in the xylP::hsfA mutant is completely rescued if hsfA expression is restored by low xylose concentration, again reinforcing the crosstalk between resistance to heat and the cell wall structure. Despite the subtle increase in hsfA expression recorded in a wild-type strain challenged with CASP and CR (Figure 2), a peak in hsfA mRNA abundance occurred after 15 min of HS, coinciding with the higher thickening of the cell wall and the HS response establish by the concomitant increase in hsp90 expression (Figures 2, 7). Similar patterns of gene expression are observed for other transcription factors, which are regulated posttranscriptionally and undergo minimal transcriptional changes. In this case, the intracellular pools of these transcription factors are likely present at all times in order to respond to stress quickly. A similar scenario may be the case for HsfA in the presence of cell wall stress. Whether posttranscriptional regulation of HsfA instead of transcriptional activation is a key event to deal with cell wall stress would require additional experiments, including investigation of the phosphorylation status and the activity of HsfA in response to cell wall-damaging agents. In line with this idea, very slight changes in xylose concentration can rescue the conditional lethal phenotype of xylP::hsfA mutant, suggesting that low levels of hsfA provide a survival benefit (Figures 3, 5).
Concomitantly to the dramatic changes in the cell wall structure, we observed increased expression of hsp90 in response to hsfA upregulation, followed by a subsequent drop in hsfA mRNA accumulation (Figures 2, 6, 7), suggesting that the expected feedback loop between HsfA and Hsp90 described in C. albicans also occurs in A. fumigatus (Nicholls et al., 2009; Leach et al., 2012a, 2016). Consistently, the protein levels of Hsp90 remain high up to 240 min post-HS (Rocha et al., 2020b). In addition, HsfA was also shown to be important in controlling the expression of genes encoding HSPs (Figure 9) and enzymes involved in trehalose biosynthesis (Figure 8 and Supplementary Table 4), which together make up part of the canonical HS response (Takahashi et al., 2017). However, the genes encoding chaperones such as hsp60, hsp70, and hsp90, although modulated by HS, were not significantly impacted by the repression of hsfA (Supplementary Table 4), differently from what was observed in C. albicans (Nicholls et al., 2009; Leach et al., 2016). Possibly, the small increase in hsfA mRNA levels recorded in our RNA-seq samples under repressive conditions (1.5-fold; 15 min HS, Supplementary Table 4) could have elicited upregulation of these HSPs in the xylP::hsfA conditional mutant. The hypothesis that other transcription factors may be acting should also be considered, and Supplementary Figure 7 shows some possible candidates, such as znfA, which was more expressed in depleted hsfA cells and recently identified as important for the regulation of the CASP paradoxical effect (Valero et al., 2020). Noteworthy, human transcription factors involved in the immune response, such as STAT1, STAT3, NF-IL6, and NF-kB, can induce HSP90 expression, either synergistically or antagonistically to HSF1 [reviewed in Prodromou (2016)].
Our present study results indicate that the thickening of the cell wall is a consequence of HS, and the mechanisms by which HsfA participates in this event are intertwined with the main signaling pathways that control cell integrity, including the CWI and HOG pathways. We found synthetically sick genetic interaction between hsfA and mpkA or sakA both under temperature and cell wall stresses, but not with the hypomorphic allele pkcAG579R (Rocha et al., 2015), suggesting that these MAPKs act upstream hsfA in response to these stressors.
However, our luciferase assays indicate that PkcA and MpkA are not crucial for HsfA activation since the expression of HsfA and hsp90P activity were boosted during the HS and CASP treatment in the CWI pathway mutant backgrounds (Figures 6, 7). We argue that functional impairment of any CWI pathway component is sufficient to increase HsfA expression to likely activate downstream targets, and some of them could be shared with the CWI pathway. To this end, another observation of our study is that the HsfA signal was more intense in the pkcAG579R background at both stress conditions. In contrast, the activity of hsp90P was more intense in the ΔmpkA only in the HS, given the signals recorded in the pkcAG579R and ΔmpkA strains were comparable in the presence of CASP. These observations again point to the crucial role of PkcA in the early adaptation to HS as described previously (Rocha et al., 2020b). Supporting this idea, overexpression of Pkc1PkcA, but not the MAPKs Mpk1MpkA, Mkk1Mkk1, and Bck1Bck1 in S. cerevisiae, can suppress the Hsf1HsfA defects in response to heat stress, meaning that the signaling emerging from Pkc1 and not Pkc1-regulated MAPK cascade was necessary for HSF1 suppression (Imazu and Sakurai, 2005). Although a collection of kinases that phosphorylate and modulate the ScHsf1 activity (reviewed in Veri et al., 2018) is known, the enzymes that phosphorylate HsfA during the HS or cell wall stress are still unknown, thus requiring further experimentation (Figure 10).
Noteworthy, our RNA-seq indicates that HsfA is also important for the plasma membrane homeostasis in the face of a temperature upshift (Figures 8, 9). Since the fungal plasma membrane adapts to temperature changes [reviewed in Fabri et al. (2020)], our data suggested that HsfA has a repressive role in the biosynthesis of ergosterol and lipids in general, especially the unsaturated fatty acids, indicating that this transcription factor may regulate the chemical and consequently physical changes of the plasma membrane during HS. Not surprisingly, a molecular link between fatty acid synthesis and the HS response governed by Hsf1 in C. albicans was described previously (Leach and Cowen, 2014) and is an open field of investigation in A. fumigatus.
In summary, we characterized the essential HSF HsfA and demonstrated its contribution to cell wall maintenance. We observed a crosstalk between HsfA-Hsp90 and CWI and provided evidence that the kinases PkcA and MpkA are not essential for the HsfA activation during the cell wall and heat stresses. Additionally, we identified genes modulated by HsfA, including those related to HS response, cell wall biogenesis, lipid metabolism, and iron homeostasis. Our results reinforce the concept that cell wall adaptation contributes to the thermotolerance of A. fumigatus via an integrated mechanism encompassing HS response and the regulation of the CWI signaling pathways in fungi.
Data Availability Statement
The datasets presented in this study can be found in online repositories. The names of the repository/repositories and accession number(s) can be found in the article/Supplementary Material.
Author Contributions
IM planned and designed research. JF, MR, CF, and LR performed research. IM, AC, GG, LR, and MD contributed reagents/analytic tools. IM, JF, AC, LR, GG, CF, and MD analyzed and validated the data. GP conducted RNA-seq analyses. JF and IM wrote the original draft of the manuscript. All authors discussed the data, and edited and approved the manuscript.
Funding
This study was supported by Fundação de Amparo à Pesquisa do Estado de São Paulo (FAPESP grant numbers: 2015/17541-0, 2016/07870-9, and 2017/19694-3) and Conselho Nacional de Desenvolvimento Científico e Tecnológico (CNPq grant number 462383/2014-8) to IM. This work was also supported by NIH grants AI136934 and AI125770, and by Merit Grant I01BX002924 from the Veterans Affairs Program to MD. The funding body had no role in designing the study or in collecting, analyzing, and interpreting the data, or in writing the manuscript.
Conflict of Interest
MD is a Co-Founder and Chief Scientific Officer (CSO) of MicroRid Technologies Inc.
The remaining authors declare that the research was conducted in the absence of any commercial or financial relationships that could be construed as a potential conflict of interest.
Acknowledgments
We thank Fundação de Amparo à Pesquisa do Estado de São Paulo (FAPESP) and Conselho Nacional de Desenvolvimento Científico e Tecnológico (CNPq) for financial support. We are indebted to Dr. Vito Valiante for providing the pYES-hph-pXyl devR vector and the ΔmpkA and ΔsakA strains and to Dr. Matthias Brock for providing the pUC57 plasmid containing the luciferase gene. We are grateful to Magda Regina Ometto Patricio for technical assistance (FAPESP 2019/00967-5). We also thank Douglas Antonio Alvaredo Paixão and Brazilian Bioethanol Science and Technology Laboratory CNPEM/MCTIC NGS Sequencing Facility for generating the sequencing data described here. JF was awarded a FAPESP Research Internships Abroad (BEPE) fellowship (2018/22755-7).
Supplementary Material
The Supplementary Material for this article can be found online at: https://www.frontiersin.org/articles/10.3389/fmicb.2021.656548/full#supplementary-material
Footnotes
References
Albrecht, D., Guthke, R., Brakhage, A. A., and Kniemeyer, O. (2010). Integrative analysis of the heat shock response in Aspergillus fumigatus. BMC Genomics 11:32. doi: 10.1186/1471-2164-11-32
Altwasser, R., Baldin, C., Weber, J., Guthke, R., Kniemeyer, O., Brakhage, A. A., et al. (2015). Network Modeling Reveals Cross Talk of MAP Kinases during Adaptation to Caspofungin Stress in Aspergillus fumigatus. PLoS One 10:e0136932. doi: 10.1371/journal.pone.0136932
Alves de Castro, P., Dos Reis, T. F., Dolan, S. K., Oliveira Manfiolli, A., Brown, N. A., et al. (2016). The Aspergillus fumigatus SchA(SCH9) kinase modulates SakA(HOG1) MAP kinase activity and it is essential for virulence. Mol. Microbiol. 102, 642–671. doi: 10.1111/mmi.13484
Araujo, R., and Rodrigues, A. G. (2004). Variability of germinative potential among pathogenic species of Aspergillus. J. Clin. Microbiol. 42, 4335–4337. doi: 10.1128/JCM.42.9.4335-4337.2004
Baldin, C., Valiante, V., Kruger, T., Schafferer, L., Haas, H., Kniemeyer, O., et al. (2015). Comparative proteomics of a tor inducible Aspergillus fumigatus mutant reveals involvement of the Tor kinase in iron regulation. Proteomics 15, 2230–2243. doi: 10.1002/pmic.201400584
Benedict, K., Jackson, B. R., Chiller, T., and Beer, K. D. (2019). Estimation of Direct Healthcare Costs of Fungal Diseases in the United States. Clin. Infect. Dis. 68, 1791–1797. doi: 10.1093/cid/ciy776
Bergman, A., and Casadevall, A. (2010). Mammalian endothermy optimally restricts fungi and metabolic costs. MBio 1:10. doi: 10.1128/mBio.00212-10
Bhabhra, R., and Askew, D. S. (2005). Thermotolerance and virulence of Aspergillus fumigatus: role of the fungal nucleolus. Med. Mycol. 43, (Suppl. 1), S87–S93. doi: 10.1080/13693780400029486
Bolger, A. M., Lohse, M., and Usadel, B. (2014). Trimmomatic: a flexible trimmer for Illumina sequence data. Bioinformatics 30, 2114–2120. doi: 10.1093/bioinformatics/btu170
Brown, A. J., Leach, M. D., and Nicholls, S. (2010). The relevance of heat shock regulation in fungal pathogens of humans. Virulence 1, 330–332. doi: 10.4161/viru.1.4.12364
Brown, G. D., Denning, D. W., Gow, N. A., Levitz, S. M., Netea, M. G., and White, T. C. (2012). Hidden killers: human fungal infections. Sci. Transl. Med. 4:165rv113. doi: 10.1126/scitranslmed.3004404
Bruder Nascimento, A. C., Dos Reis, T. F., de Castro, P. A., Hori, J. I., Bom, V. L., de Assis, L. J., et al. (2016). Mitogen activated protein kinases SakA(HOG1) and MpkC collaborate for Aspergillus fumigatus virulence. Mol. Microbiol. 100, 841–859. doi: 10.1111/mmi.13354
Casadevall, A., Kontoyiannis, D. P., and Robert, V. (2019). On the Emergence of Candida auris: Climate Change, Azoles, Swamps, and Birds. MBio 10:19. doi: 10.1128/mBio.01397-19
Chatterjee, S., and Tatu, U. (2017). Heat shock protein 90 localizes to the surface and augments virulence factors of Cryptococcus neoformans. PLoS Negl. Trop. Dis. 11:e0005836. doi: 10.1371/journal.pntd.0005836
Chaveroche, M. K., Ghigo, J. M., and d’Enfert, C. (2000). A rapid method for efficient gene replacement in the filamentous fungus Aspergillus nidulans. Nucleic Acids Res. 28:E97.
Cowen, L. E. (2009). Hsp90 orchestrates stress response signaling governing fungal drug resistance. PLoS Pathog. 5:e1000471. doi: 10.1371/journal.ppat.1000471
Dichtl, K., Helmschrott, C., Dirr, F., and Wagener, J. (2012). Deciphering cell wall integrity signalling in Aspergillus fumigatus: identification and functional characterization of cell wall stress sensors and relevant Rho GTPases. Mol. Microbiol. 83, 506–519. doi: 10.1111/j.1365-2958.2011.07946.x
Eastmond, D. L., and Nelson, H. C. (2006). Genome-wide analysis reveals new roles for the activation domains of the Saccharomyces cerevisiae heat shock transcription factor (Hsf1) during the transient heat shock response. J. Biol. Chem. 281, 32909–32921. doi: 10.1074/jbc.M602454200
Erkine, A. M., Magrogan, S. F., Sekinger, E. A., and Gross, D. S. (1999). Cooperative binding of heat shock factor to the yeast HSP82 promoter in vivo and in vitro. Mol. Cell Biol. 19, 1627–1639. doi: 10.1128/mcb.19.3.1627
Fabri, J., de Sa, N. P., Malavazi, I., and Del Poeta, M. (2020). The dynamics and role of sphingolipids in eukaryotic organisms upon thermal adaptation. Prog. Lipid Res. 80:101063. doi: 10.1016/j.plipres.2020.101063
Fuchs, B. B., and Mylonakis, E. (2009). Our paths might cross: the role of the fungal cell wall integrity pathway in stress response and cross talk with other stress response pathways. Eukaryot Cell 8, 1616–1625. doi: 10.1128/EC.00193-09
Garcia-Solache, M. A., and Casadevall, A. (2010). Global warming will bring new fungal diseases for mammals. MBio 1:10. doi: 10.1128/mBio.00061-10
Gomez-Pastor, R., Burchfiel, E. T., and Thiele, D. J. (2018). Regulation of heat shock transcription factors and their roles in physiology and disease. Nat. Rev. Mol. Cell Biol. 19, 4–19. doi: 10.1038/nrm.2017.73
Haas, D., Lesch, S., Buzina, W., Galler, H., Gutschi, A. M., Habib, J., et al. (2016). Culturable fungi in potting soils and compost. Med. Mycol. 54, 825–834. doi: 10.1093/mmy/myw047
Hahn, J. S., and Thiele, D. J. (2004). Activation of the Saccharomyces cerevisiae heat shock transcription factor under glucose starvation conditions by Snf1 protein kinase. J. Biol. Chem. 279, 5169–5176. doi: 10.1074/jbc.M311005200
Hartl, F. U. (1996). Molecular chaperones in cellular protein folding. Nature 381, 571–579. doi: 10.1038/381571a0
Hashikawa, N., and Sakurai, H. (2004). Phosphorylation of the yeast heat shock transcription factor is implicated in gene-specific activation dependent on the architecture of the heat shock element. Mol. Cell Biol. 24, 3648–3659. doi: 10.1128/mcb.24.9.3648-3659.2004
Hashikawa, N., Yamamoto, N., and Sakurai, H. (2007). Different mechanisms are involved in the transcriptional activation by yeast heat shock transcription factor through two different types of heat shock elements. J. Biol. Chem. 282, 10333–10340. doi: 10.1074/jbc.M609708200
Huber, W., Carey, V. J., Gentleman, R., Anders, S., Carlson, M., Carvalho, B. S., et al. (2015). Orchestrating high-throughput genomic analysis with Bioconductor. Nat. Methods 12, 115–121. doi: 10.1038/nmeth.3252
Ikezaki, S., Cho, T., Nagao, J. I., Tasaki, S., Yamaguchi, M., Arita-Morioka, K. I., et al. (2019). Mild Heat Stress Affects on the Cell Wall Structure in Candida albicans Biofilm. Med. Mycol. J. 60, 29–37. doi: 10.3314/mmj.19-00001
Imazu, H., and Sakurai, H. (2005). Saccharomyces cerevisiae heat shock transcription factor regulates cell wall remodeling in response to heat shock. Eukaryot. Cell 4, 1050–1056. doi: 10.1128/EC.4.6.1050-1056.2005
Jacobsen, I. D., Luttich, A., Kurzai, O., Hube, B., and Brock, M. (2014). In vivo imaging of disseminated murine Candida albicans infection reveals unexpected host sites of fungal persistence during antifungal therapy. J. Antimicrob. Chemother. 69, 2785–2796. doi: 10.1093/jac/dku198
Jun, S. C., Choi, Y. H., Lee, M. W., Yu, J. H., and Shin, K. S. (2020). The Putative APSES Transcription Factor RgdA Governs Growth, Development, Toxigenesis, and Virulence in Aspergillus fumigatus. mSphere 5:20. doi: 10.1128/mSphere.00998-20
Kamada, Y., Jung, U. S., Piotrowski, J., and Levin, D. E. (1995). The protein kinase C-activated MAP kinase pathway of Saccharomyces cerevisiae mediates a novel aspect of the heat shock response. Genes Dev. 9, 1559–1571. doi: 10.1101/gad.9.13.1559
Kijima, T., Prince, T. L., Tigue, M. L., Yim, K. H., Schwartz, H., Beebe, K., et al. (2018). HSP90 inhibitors disrupt a transient HSP90-HSF1 interaction and identify a noncanonical model of HSP90-mediated HSF1 regulation. Sci. Rep. 8:6976. doi: 10.1038/s41598-018-25404-w
Kim, D., Pertea, G., Trapnell, C., Pimentel, H., Kelley, R., and Salzberg, S. L. (2013). TopHat2: accurate alignment of transcriptomes in the presence of insertions, deletions and gene fusions. Genome Biol. 14:R36. doi: 10.1186/gb-2013-14-4-r36
Kopylova, E., Noe, L., and Touzet, H. (2012). SortMeRNA: fast and accurate filtering of ribosomal RNAs in metatranscriptomic data. Bioinformatics 28, 3211–3217. doi: 10.1093/bioinformatics/bts611
Krakowiak, J., Zheng, X., Patel, N., Feder, Z. A., Anandhakumar, J., Valerius, K., et al. (2018). Hsf1 and Hsp70 constitute a two-component feedback loop that regulates the yeast heat shock response. Elife 7:31668. doi: 10.7554/eLife.31668
LaFayette, S. L., Collins, C., Zaas, A. K., Schell, W. A., Betancourt-Quiroz, M., Gunatilaka, A. A., et al. (2010). PKC signaling regulates drug resistance of the fungal pathogen Candida albicans via circuitry comprised of Mkc1, calcineurin, and Hsp90. PLoS Pathog. 6:e1001069. doi: 10.1371/journal.ppat.1001069
Lamoth, F., Juvvadi, P. R., Fortwendel, J. R., and Steinbach, W. J. (2012). Heat shock protein 90 is required for conidiation and cell wall integrity in Aspergillus fumigatus. Eukaryot. Cell 11, 1324–1332. doi: 10.1128/EC.00032-12
Lamoth, F., Juvvadi, P. R., Gehrke, C., and Steinbach, W. J. (2013). In vitro activity of calcineurin and heat shock protein 90 Inhibitors against Aspergillus fumigatus azole- and echinocandin-resistant strains. Antimicrob. Agents Chemother. 57, 1035–1039. doi: 10.1128/AAC.01857-12
Lamoth, F., Juvvadi, P. R., Soderblom, E. J., Moseley, M. A., and Steinbach, W. J. (2015). Hsp70 and the Cochaperone StiA (Hop) Orchestrate Hsp90-Mediated Caspofungin Tolerance in Aspergillus fumigatus. Antimicrob. Agents Chemother. 59, 4727–4733. doi: 10.1128/AAC.00946-15
Leach, M. D., and Cowen, L. E. (2013). Surviving the heat of the moment: a fungal pathogens perspective. PLoS Pathog. 9:e1003163. doi: 10.1371/journal.ppat.1003163
Leach, M. D., and Cowen, L. E. (2014). Membrane fluidity and temperature sensing are coupled via circuitry comprised of Ole1, Rsp5, and Hsf1 in Candida albicans. Eukaryot. Cell 13, 1077–1084. doi: 10.1128/EC.00138-14
Leach, M. D., Budge, S., Walker, L., Munro, C., Cowen, L. E., and Brown, A. J. (2012a). Hsp90 orchestrates transcriptional regulation by Hsf1 and cell wall remodelling by MAPK signalling during thermal adaptation in a pathogenic yeast. PLoS Pathog. 8:e1003069. doi: 10.1371/journal.ppat.1003069
Leach, M. D., Farrer, R. A., Tan, K., Miao, Z., Walker, L. A., Cuomo, C. A., et al. (2016). Hsf1 and Hsp90 orchestrate temperature-dependent global transcriptional remodelling and chromatin architecture in Candida albicans. Nat. Commun. 7:11704. doi: 10.1038/ncomms11704
Leach, M. D., Klipp, E., Cowen, L. E., and Brown, A. J. (2012b). Fungal Hsp90: a biological transistor that tunes cellular outputs to thermal inputs. Nat. Rev. Microbiol. 10, 693–704. doi: 10.1038/nrmicro2875
Liao, Y., Smyth, G. K., and Shi, W. (2013). The Subread aligner: fast, accurate and scalable read mapping by seed-and-vote. Nucleic Acids Res. 41:e108. doi: 10.1093/nar/gkt214
Liu, X. D., and Thiele, D. J. (1996). Oxidative stress induced heat shock factor phosphorylation and HSF-dependent activation of yeast metallothionein gene transcription. Genes Dev. 10, 592–603.
Malavazi, I., and Goldman, G. H. (2012). Gene disruption in Aspergillus fumigatus using a PCR-based strategy and in vivo recombination in yeast. Methods Mol. Biol. 845, 99–118. doi: 10.1007/978-1-61779-539-8_7
Munshi, M. A., Gardin, J. M., Singh, A., Luberto, C., Rieger, R., Bouklas, T., et al. (2018). The Role of Ceramide Synthases in the Pathogenicity of Cryptococcus neoformans. Cell Rep. 22, 1392–1400. doi: 10.1016/j.celrep.2018.01.035
Nair, R., Khandelwal, N. K., Shariq, M., Redhu, A. K., Gaur, N. A., Shaikh, S., et al. (2018). Identification of genome-wide binding sites of heat shock factor 1, Hsf1, under basal conditions in the human pathogenic yeast, Candida albicans. AMB Express 8:116. doi: 10.1186/s13568-018-0647-7
Nair, R., Shariq, M., Dhamgaye, S., Mukhopadhyay, C. K., Shaikh, S., and Prasad, R. (2017). Non-heat shock responsive roles of HSF1 in Candida albicans are essential under iron deprivation and drug defense. Biochim. Biophys. Acta Mol. Cell Res. 1864, 345–354. doi: 10.1016/j.bbamcr.2016.11.021
Nicholls, S., Leach, M. D., Priest, C. L., and Brown, A. J. (2009). Role of the heat shock transcription factor, Hsf1, in a major fungal pathogen that is obligately associated with warm-blooded animals. Mol. Microbiol. 74, 844–861. doi: 10.1111/j.1365-2958.2009.06883.x
Nicholls, S., MacCallum, D. M., Kaffarnik, F. A., Selway, L., Peck, S. C., and Brown, A. J. (2011). Activation of the heat shock transcription factor Hsf1 is essential for the full virulence of the fungal pathogen Candida albicans. Fungal Genet. Biol. 48, 297–305. doi: 10.1016/j.fgb.2010.08.010
Parsell, D. A., and Lindquist, S. (1993). The function of heat-shock proteins in stress tolerance: degradation and reactivation of damaged proteins. Annu. Rev. Genet. 27, 437–496. doi: 10.1146/annurev.ge.27.120193.002253
Prodromou, C. (2016). Mechanisms of Hsp90 regulation. Biochem. J. 473, 2439–2452. doi: 10.1042/BCJ20160005
Robert, V. A., and Casadevall, A. (2009). Vertebrate endothermy restricts most fungi as potential pathogens. J. Infect. Dis. 200, 1623–1626. doi: 10.1086/644642
Robinson, M. D., McCarthy, D. J., and Smyth, G. K. (2010). edgeR: a Bioconductor package for differential expression analysis of digital gene expression data. Bioinformatics 26, 139–140. doi: 10.1093/bioinformatics/btp616
Rocha, M. C., Fabri, J. H., Franco, de Godoy, K., Alves, de Castro, P., et al. (2016). Aspergillus fumigatus MADS-Box Transcription Factor rlmA Is Required for Regulation of the Cell Wall Integrity and Virulence. G3 6, 2983–3002. doi: 10.1534/g3.116.031112
Rocha, M. C., Fabri, J., Simoes, I. T., Silva-Rocha, R., Hagiwara, D., da Cunha, A. F., et al. (2020a). The Cell Wall Integrity Pathway Contributes to the Early Stages of Aspergillus fumigatus Asexual Development. Appl. Environ. Microbiol. 86:19. doi: 10.1128/AEM.02347-19
Rocha, M. C., Godoy, K. F., de Castro, P. A., Hori, J. I., Bom, V. L., Brown, N. A., et al. (2015). The Aspergillus fumigatus pkcA G579R Mutant Is Defective in the Activation of the Cell Wall Integrity Pathway but Is Dispensable for Virulence in a Neutropenic Mouse Infection Model. PLoS One 10:e0135195. doi: 10.1371/journal.pone.0135195
Rocha, M. C., Minari, K., Fabri, J., Kerkaert, J. D., Gava, L. M., da Cunha, A. F., et al. (2020b). Aspergillus fumigatus Hsp90 interacts with the main components of the cell wall integrity pathway and cooperates in heat shock and cell wall stress adaptation. Cell Microbiol. 23:e13273. doi: 10.1111/cmi.13273
Sarge, K. D., Murphy, S. P., and Morimoto, R. I. (1993). Activation of heat shock gene transcription by heat shock factor 1 involves oligomerization, acquisition of DNA-binding activity, and nuclear localization and can occur in the absence of stress. Mol. Cell Biol. 13, 1392–1407. doi: 10.1128/mcb.13.3.1392
Schopf, F. H., Biebl, M. M., and Buchner, J. (2017). The HSP90 chaperone machinery. Nat. Rev. Mol. Cell Biol. 18, 345–360. doi: 10.1038/nrm.2017.20
Sorger, P. K., and Nelson, H. C. (1989). Trimerization of a yeast transcriptional activator via a coiled-coil motif. Cell 59, 807–813.
Sorger, P. K., and Pelham, H. R. (1988). Yeast heat shock factor is an essential DNA-binding protein that exhibits temperature-dependent phosphorylation. Cell 54, 855–864.
Sueiro-Olivares, M., Fernandez-Molina, J. V., Abad-Diaz-de-Cerio, A., Gorospe, E., Pascual, E., Guruceaga, X., et al. (2015). Aspergillus fumigatus transcriptome response to a higher temperature during the earliest steps of germination monitored using a new customized expression microarray. Microbiology 161(Pt 3), 490–502. doi: 10.1099/mic.0.000021
Taipale, M., Jarosz, D. F., and Lindquist, S. (2010). HSP90 at the hub of protein homeostasis: emerging mechanistic insights. Nat. Rev. Mol. Cell Biol. 11, 515–528. doi: 10.1038/nrm2918
Takahashi, H., Kusuya, Y., Hagiwara, D., Takahashi-Nakaguchi, A., Sakai, K., and Gonoi, T. (2017). Global gene expression reveals stress-responsive genes in Aspergillus fumigatus mycelia. BMC Genomics 18:942. doi: 10.1186/s12864-017-4316-z
Thompson, S., Croft, N. J., Sotiriou, A., Piggins, H. D., and Crosthwaite, S. K. (2008). Neurospora crassa heat shock factor 1 Is an essential gene; a second heat shock factor-like gene, hsf2, is required for asexual spore formation. Eukaryot. Cell 7, 1573–1581. doi: 10.1128/EC.00427-07
Toyotome, T., Onishi, K., Sato, M., Kusuya, Y., Hagiwara, D., Watanabe, A., et al. (2020). Identification of novel mutations contributing to azole tolerance of Aspergillus fumigatus through in vitro exposure to tebuconazole. Preprint. doi: 10.1101/2020.07.20.213256
Truman, A. W., Millson, S. H., Nuttall, J. M., Mollapour, M., Prodromou, C., and Piper, P. W. (2007). In the yeast heat shock response, Hsf1-directed induction of Hsp90 facilitates the activation of the Slt2 (Mpk1) mitogen-activated protein kinase required for cell integrity. Eukaryot. Cell 6, 744–752. doi: 10.1128/EC.00009-07
Valero, C., Colabardini, A. C., Chiaratto, J., Pardeshi, L., de Castro, P. A., Ferreira Filho, J. A., et al. (2020). Aspergillus fumigatus Transcription Factors Involved in the Caspofungin Paradoxical Effect. mBio 11:20. doi: 10.1128/mBio.00816-20
Valiante, V., Jain, R., Heinekamp, T., and Brakhage, A. A. (2009). The MpkA MAP kinase module regulates cell wall integrity signaling and pyomelanin formation in Aspergillus fumigatus. Fungal Genet. Biol. 46, 909–918. doi: 10.1016/j.fgb.2009.08.005
Valiante, V., Macheleidt, J., Foge, M., and Brakhage, A. A. (2015). The Aspergillus fumigatus cell wall integrity signaling pathway: drug target, compensatory pathways, and virulence. Front. Microbiol. 6:325. doi: 10.3389/fmicb.2015.00325
van Paassen, J., Russcher, A., In ’t Veld-van Wingerden, A. W., Verweij, P. E., and Kuijper, E. J. (2016). Emerging aspergillosis by azole-resistant Aspergillus fumigatus at an intensive care unit in the Netherlands, 2010 to 2013. Euro Surveill. 21:30300. doi: 10.2807/1560-7917.ES.2016.21.30.30300
Veri, A. O., Robbins, N., and Cowen, L. E. (2018). Regulation of the heat shock transcription factor Hsf1 in fungi: implications for temperature-dependent virulence traits. FEMS Yeast Res. 18:foy041. doi: 10.1093/femsyr/foy041
Wang, L., Wang, S., and Li, W. (2012). RSeQC: quality control of RNA-seq experiments. Bioinformatics 28, 2184–2185. doi: 10.1093/bioinformatics/bts356
Wiederrecht, G., Seto, D., and Parker, C. S. (1988). Isolation of the gene encoding the S. cerevisiae heat shock transcription factor. Cell 54, 841–853.
Xie, C., Mao, X., Huang, J., Ding, Y., Wu, J., Dong, S., et al. (2011). KOBAS 2.0: a web server for annotation and identification of enriched pathways and diseases. Nucleic Acids Res. 39, W316–W322. doi: 10.1093/nar/gkr483
Yamamoto, A., Mizukami, Y., and Sakurai, H. (2005). Identification of a novel class of target genes and a novel type of binding sequence of heat shock transcription factor in Saccharomyces cerevisiae. J. Biol. Chem. 280, 11911–11919. doi: 10.1074/jbc.M411256200
Yang, D. H., Jung, K. W., Bang, S., Lee, J. W., Song, M. H., Floyd-Averette, A., et al. (2017). Rewiring of Signaling Networks Modulating Thermotolerance in the Human Pathogen Cryptococcus neoformans. Genetics 205, 201–219. doi: 10.1534/genetics.116.190595
Keywords: Aspergillus fumigatus, HsfA, thermotolerance, heat shock (HS), transcription factor, cell wall integrity (CWI)
Citation: Fabri JHTM, Rocha MC, Fernandes CM, Persinoti GF, Ries LNA, Cunha AF, Goldman GH, Del Poeta M and Malavazi I (2021) The Heat Shock Transcription Factor HsfA Is Essential for Thermotolerance and Regulates Cell Wall Integrity in Aspergillus fumigatus. Front. Microbiol. 12:656548. doi: 10.3389/fmicb.2021.656548
Received: 21 January 2021; Accepted: 11 March 2021;
Published: 09 April 2021.
Edited by:
Allan J. Guimaraes, Fluminense Federal University, BrazilReviewed by:
Louise Walker, University of Aberdeen, United KingdomNir Osherov, Tel Aviv University, Israel
Copyright © 2021 Fabri, Rocha, Fernandes, Persinoti, Ries, Cunha, Goldman, Del Poeta and Malavazi. This is an open-access article distributed under the terms of the Creative Commons Attribution License (CC BY). The use, distribution or reproduction in other forums is permitted, provided the original author(s) and the copyright owner(s) are credited and that the original publication in this journal is cited, in accordance with accepted academic practice. No use, distribution or reproduction is permitted which does not comply with these terms.
*Correspondence: Iran Malavazi, aW1hbGF2YXppQHVmc2Nhci5icg==