- 1Beijing Advanced Innovation Center for Tree Breeding by Molecular Design, Beijing University of Agriculture, Beijing, China
- 2College of Plant Science and Technology, Beijing University of Agriculture, Beijing, China
- 3Beijing Key Laboratory for Agricultural Application and New Technique, Beijing, China
In order to increase O2 concentration in the rhizosphere and reduce the continuous cropping obstacles under high-density cultivation, ventilation is often used to increase soil aeration. Yet, the effect of ventilation on soil microbial communities and nutrient cycling and, further, the extent to which they influence strawberry growth under greenhouse conditions are still poorly understood. Thus, four treatments—no ventilation + low planting density (LD), ventilation + LD, no ventilation + high planting density (HD), and ventilation + HD—of strawberry “Red cheeks” (Fragaria × ananassa Duch. cv. “Benihopp”) were studied in a greenhouse for 3 years. The ventilation pipe (diameter = 10 cm) was buried in the soil at a depth of 15 cm from the surface and fresh air was sent to the root zone through the pipe by a blower. Ten pipes (one pipeline in a row) were attached to a blower. Soil samples were collected using a stainless-steel corer (five-point intra-row sampling) for the nutrient and microbial analyses. The composition and structure of the soil bacterial and fungal communities were analyzed by high-throughput sequencing of the 16S and 18S rRNA genes, and functional profiles were predicted using PICRUSt and FUNGuild, respectively. The results showed that soil ventilation increased the net photosynthetic rate (Pn), transpiration rate (Tr), and water use efficiency (WUE) of strawberry plants across two growth stages [vegetative growth stage (VGS) and fruit development stage (FDS)]. Soil ventilation increased its available nutrient contents, but the available nutrient contents were reduced under the high planting density compared with low planting density. Both the O2 concentration and O2:CO2 ratio were increased by ventilation; these were positively correlated with the relative abundance of Bacilli, Gamma-proteobacteria, Blastocatella, as well as Chytridiomycota and Pezizomycetes. Conversely, ventilation decreased soil CO2 concentration and the abundance of Beta-proteobacteria and Gemmatimonadetes. The greater planting density increased the relative abundance of Acidobacteria (oligotrophic group). Ventilation altered soil temperature and pH along with carbon and nitrogen functional profiles in the VGS (more nitrogen components) and FDS (more carbon components), which benefited strawberry plant growth under high planting density. The practice of soil ventilation provides a strategy to alleviate hypoxia stress and continuous cropping obstacles for improving crop production in greenhouse settings.
Introduction
Over the past century, intensive tillage (such as high-density planting) has often been used in greenhouses to increase the crop yield of per unit land area (Maboko and Du Plooy, 2013). However, long-term use of intensive tillage has major negative impacts on the ecosystem and leads to significant soil degradation, including soil compaction, soil organic carbon (SOC) loss, and essential soil function reduction, which can restrict plant growth and crop yield (Puerta et al., 2018; Stefan et al., 2021).
It is well-known that mesophytic plant roots require soil oxygen (O2) in order to respire, grow, develop, and function normally (Li et al., 2016a, b; Ben-Noah and Friedman, 2018). Most of O2 supply is obtained directly through diffusion gas exchange from the atmosphere to the rhizosphere soil; meanwhile, the root and microbial respiration is constrained by O2 in the root zone (Ityel et al., 2014; Ben-Noah and Friedman, 2018). Under high-density planting condition, reduced O2 and elevated carbon dioxide (CO2) concentrations negatively affect plant growth and productivity partly (Kitaya et al., 1984; Li et al., 2020). Furthermore, the soil respiration and microbial biomass decreased in most cases of high CO2 treatments (Santruckova and Simek, 1997).
Increased O2 concentration of root zone can improve bell pepper biomass and fruit yield (Ityel et al., 2014). Meanwhile soil aeration has been reported to accelerate the growth and increase the crop yield by improving O2 concentration, such as muskmelon and tomato (Bhattarai et al., 2006; Li et al., 2016, 2020). Thus, aerated irrigation has been widely used in agricultural systems, e.g., orchards, greenhouse, field, and pot experiments (Bhattarai et al., 2004; Friedman and Naftaliev, 2012; Hou et al., 2016; Du et al., 2019). Aerated irrigation promoted the growth of new leaves, fine roots, and new branches of grape, and accelerated air exchange in rhizosphere soil (Zhao et al., 2019). It also has been reported that ventilation (every 3 days) improved leaf functions and increased the net photosynthetic rate (Pn) of young peach trees (Xiao et al., 2015). Thus, soil aeration is considered the third most important factor affecting soil fertility after water and nutrient availability (Glinski and Stepniewski, 1985; Ben-Noah et al., 2021), and its status is an important aspect of soil quality and soil ecology (Niu et al., 2012). Soil aeration elevates the concentration of O2 and relieves the high concentrations of CO2 formed during respiration under anaerobic soil conditions (Ben-Noah and Friedman, 2018). Tillage is the only commonly used agricultural practice for improving soil aeration, despite its adverse effect on soil structure (Stępniewski and Stępniewska, 2009).
In addition, long-term continuous cropping usually leads to increased soil-borne disease and decreased soil nutrient content of strawberry, which may be caused by the decreased beneficial microorganisms and accumulated fungal pathogen (Fusarium oxysporum) in the greenhouse (Huang et al., 2018; Li and Liu, 2019). Intermittent aeration can promote functional bacteria growth in N removal process of wetlands (Liu et al., 2019). Meanwhile, aeration irrigation significantly increased the abundance of aerobic bacteria and promoted the increase of Pseudomonas and Aspergillus related to phosphate solubilization, that of Bacillus related to potassium solubilization, and that of Fusarium related to organic matter (OM) decomposition (Zhao et al., 2019).
To alleviate the negative effects of high planting density and continuous cropping, we aimed to improve soil aeration of strawberry through in situ ventilation using a blower. Strawberry (Fragaria ananassa Duch.) is classified as a plant with high oxygen demand root (Iwasaki, 2008) and is more susceptible to root hypoxic stress. However, few studies are reported that apply root zone aeration as a technique to improve strawberry production (Iwasaki, 2008). Furthermore, the mechanism by which soil aeration may facilitate strawberry growth is largely unknown, especially how it affects soil microbial community composition, and functional structure or alter nutrient concentrations under a high-density planting.
Here, we speculated that in situ ventilation is able to influence the soil microbial community, soil nutrient cycling, and their interaction, to benefit the growth of strawberry at high-density planting. To analyze the effect mechanism of soil ventilation on strawberry plant growth, we evaluated the response of soil microorganisms and nutrient content to cultivation management (soil ventilation and planting density treatments) at the strawberry vegetative growth stage (VGS) and fruit development stage (FDS) in greenhouses. The objectives of this study were (i) to evaluate the impact of intermittent soil ventilation on the abundance, diversity, and function of the microbial community under rational high planting density (HD) vs. low planting density (LD) conditions; (ii) to evaluate the impact of intermittent soil ventilation on the soil C and N cycling at the VGS and FDS; and (iii) to evaluate the impact of intermittent soil ventilation on strawberry plant growth.
Materials and Methods
Study Site and Plant Materials
The field experiment was conducted in plastic greenhouses in Changping (40°19′ N, 116°16′ E), China, a city located northwest of Beijing. The site lies at an elevation of 57 m above sea level (asl), in a region that has a warm continental monsoonal climate, whose mean temperature ranges from −4 to 26°C, with a mean annual precipitation about 556.4 mm. The soil type here is a typical light sandy loam, with a SOC content of 2.10%, and available N, P, and K concentrations of 143.95, 15.68, and 225.75 mg kg–1, respectively. Strawberries were first cultivated at the study site in 2008; hence, by 2016, strawberries have been growing in the area continuously for nearly 10 years. Seedlings of the strawberry “Red cheeks” (Fragaria × ananassa Duch. cv. “Benihopp”) similar in size (i.e., with approximately five compound leaves per plant) were selected and planted in the experimental greenhouses on the ridges in square-shaped rows. The rows were covered with black plastic film. The planting cycle lasts approximately 10 months, from August to May of the next year, amounting to a single growing season with corresponding growth stages for VGS (September to December) and FDS (January to May).
Experimental Design
Four treatments were set up in the greenhouse: no ventilation + LD, ventilation + LD, no ventilation + HD, and ventilation + HD. The randomized block design was repeated three times, with a total of 12 cultivation plots. The greenhouse was 65.0 m long and 8.0 m wide with an east–west orientation, while each cultivation plot was 5.5 m long and 1.0 m wide with a total planting area of 5.5 m2, and crop rows were aligned north–south. The ventilation pipe (diameter = 10 cm, made of nylon fabric and supported with spiral iron wire ring) was buried in the soil at a depth of 15 cm from the surface and fresh air was sent to the root zone through the pipe by a blower (output: 0.75 kw, flow rate: 50 L min–1, SCL k05-MS MOR; FBZ, Ferrara, Italy). Ten pipes (one pipeline per row) were attached to a blower, and most air in the pipe can pass through the nylon cloth to the soil (Supplementary Figures 1–3). The ventilation was applied every 2 h and each time lasted for 1 h in 09:00–17:00 during the growing season. The ventilation treatment was administered for 3 years from 2013 to 2016. There are two densities: low planting density (LD, with two plants per row and a plant × row spacing of 10.0 cm × 25.0 cm) vs. high planting density (HD, with four plants per row and a plant × row spacing of 10.0 cm × 12.5 cm) (see Supplementary Figure 1 for details). Every year, seedlings were planted at the end of August and harvested in May of the following year.
Photosynthetic Characteristics and Leaf Nutrient Content of Strawberry
Leaf photosynthetic activity of strawberry plants was measured using a portable photosynthesis system (LI-6400; LI-COR Biosciences, Lincoln, NE, United States) between 09:00 a.m. and 11:00 a.m. during VGS (November 10, 2015) and FDS (April 10, 2016). Net photosynthetic rate (Pn) and transpiration rate (Tr) were measured and water use efficiency (WUE) was calculated as WUE = Pn/Tr (Ribeiro et al., 2009). Three fully expanded leaves were selected from each plant per plot for the measurements, and average values were calculated for each plant. During the measurements, the photosynthetic photon flux density was 1,200 μmol m–2 s–1; the CO2 concentration in the leaf chamber was 380 ± 5 μmol mol–1; and the leaf temperature was 25°C. The leaf area (LA) was measured with a leaf area meter (LI-3000A; LI-COR Biosciences) (Nardini et al., 2014). Leaf N was determined by the Kjeldahl method and leaf P and K were determined by inductively coupled plasma-optical emission spectrometry (ICP-OES) (iCAP 6000; Thermo Fisher Scientific, Waltham, MA, United States) (Rivelli et al., 2012) for 15 leaves per plant that had been oven-dried at 70°C for 24 h.
Soil Sampling and Processing
Soil samples were collected on two occasions (November 10, 2015 and April 10, 2016). After removing the litter layer, five replicate samples (top 0–20 cm depth) were collected in an “S” shape on the ridge, using a standard soil auger (4.5 cm inner diameter) and then homogenized to obtain a composite sample for each plot. The samples were immediately transported to the laboratory, sieved (<2 mm), and divided into two subsamples. One was immediately stored at −80°C for soil microbial and enzymatic activity analyses; the other portion was air-dried and stored at room temperature until chemically analyzed.
Soil Physicochemical Parameters
The O2 and CO2 concentrations in the treated soils were measured with a gas detector (GasAlertMicro 5 IR; BW Technologies Honeywell, Calgary, AB, Canada). Soil temperature was measured with an electronic temperature and humidity recorders (RTR53A; T&D Corporation, Matsumoto, Japan). The pH was measured with a compound electrode (PE 10, Sartorius, Göttingen, Germany) at a soil-to-water ratio of 1:2.5. The SOC content was determined by the Walkley–Black method (Nelson and Sommers, 1996), albeit with some slight modifications. Total nitrogen (TN) content was determined with the Kjeldahl method (Bremner, 1996), and soil available nitrogen (AN) was determined by applying the alkaline hydrolysis diffusion method (Lu, 2000). Total and available phosphorus (TP and AP, respectively), and total and available potassium (TK and AK, respectively) concentrations were measured by ICP-OES (Rivelli et al., 2012). Soil invertase (INV), catalase (CAT), and urease (URE) activity were determined as described by Jin et al. (2009), with some slight modifications applied. To estimate the INV activity, the 3,5-dinitrosalicylic acid method was implemented, using a sucrose substrate, after which 5 g of air-dried soil was incubated at 37°C for 24 h. Catalase activity was measured using the back titration of residual H2O2 added to soil (2 g) with 0.1 M KMnO4. URE activity was determined after incubating the soil (5 g) for 24 h at 37°C with a 10% urea solution as the substrate.
Soil DNA Extraction and Amplification
From each sample, total genomic DNA was extracted from 0.25 g of soil using the TIANamp Soil DNA Kit (Tiangen Biotech, Beijing, China) according to the manufacturer’s protocol. The quality and quantity of DNA were evaluated based on the A260/280 ratio, measured on a spectrophotometer (NanoDrop 2000; Thermo Fisher Scientific) and by electrophoresis (1% agarose gel). The V3–V4 hypervariable regions of the bacterial 16S rRNA gene was amplified using the barcoded primers 341F (5′-CCTACGGGNGGC WGCAG-3′) and 785R (GACTACHVGGGTATCTAATCC) (Klindworth et al., 2013), and the fungal 18S rRNA gene sequences were PCR-amplified using the barcoded primers EF4 (5′-GGAAGGGRTGTATTTATTAG-3′) and NS2 (5′-GGCTGCTGGCACCAGACTTGC-3′) (Smit et al., 1999; Rashad et al., 2012). The purified Polymerase Chain Reaction (PCR) amplicons were then sequenced on the MiSeq platform (300-bp paired-end reads) (Illumina, San Diego, CA, United States) by Ori-Gene Technology (Beijing, China).
Soil Microorganisms Sequence Data Analysis
High-quality paired-end reads of the 16S and 18S sequences were merged using the FLASH software (Magoč and Salzberg, 2011) and Mothur1 was used to filter the sequences and remove the barcodes. Operational taxonomic units (OTUs) were obtained using the UPARSE pipeline based on the merged sequences (Edgar, 2013); those with ≥97% similarity were assigned to the same OTU. To obtain taxonomic information, representative 16S and 18S sequences of each OTU were generated and aligned to the SILVA and UNITE databases, respectively, using the Ribosomal Database Project (RDP) classifier (Pruesse et al., 2007).2 The raw sequences were submitted to NCBI Sequence Read Archive under the identification PRJNA721522.
Alpha-diversity indices, including the number of species observed (Sobs) and the Chao and Shannon indices, were calculated with Mothur v. 1.34.4 (Schloss et al., 2009). The functional groups related to soil C and N cycles of the bacteria were obtained using the Functional Annotation of Prokaryotic Taxa (FAPROTAX) database,3 which could map prokaryotic clades to metabolic and ecologically relevant functions based on the literature of cultured strains, by transforming the OUT tables into putative functional profiles (Louca et al., 2016; Xu et al., 2021). Based on the OTUs of fungi, a trophic classification of pathotrophs, saprotrophs, and symbiotrophs was performed using FUNGuild,4 as previously described (Nguyen et al., 2016).
Statistical Analysis
The soil multifunctionality index (SMF) comprises nine soil nutrition indicators and the respective activity of the three enzymes mentioned in the earlier section Soil Physicochemical Parameters (Delgado-Baquerizo et al., 2016; Bastida et al., 2017). To obtain the SMF for each plot, individual functions underwent a Z-score transformation, and standardized rates of soil functions were then averaged.
The variables were subjected to a three-way analysis of variance (ANOVA). The three factors included in this experimental design were as follows: (i) Ventilation, which had two levels: ventilation and no ventilation; (ii) Density, which had two levels: HD and LD; and (iii) Stage: VGS and FDS. Three-way ANOVA of the abundance of bacterial and fungal populations is provided in the Supporting Information (Supplementary Tables 3, 4, respectively). All data were subjected to one-way ANOVA followed by post hoc analyses with Tukey’s Honestly Significant Difference (HSD) test. Differences between the means were considered statistically significant at p < 0.05. Different lowercase letters represent significant differences between eight treatments.
Microbial community structure was analyzed by a principal component analysis (PCA) based on relative abundances of the dominant populations at the phylum, class, family, and genus levels. Permutational multivariate (PERM) ANOVA with 9,999 permutations was used to evaluate the influence of factor analysis upon microbial community structure. Redundancy analysis (RDA) was performed to elucidate the relationships between the environmental variables (O2, CO2, O2:CO2, temperature, and pH) and the soil chemical factors. Spearman correlation coefficients between soil, plant, and microbial (bacterial and fungal) variables and O2 (CO2, O2:CO2, or SMF) were also calculated. Figures were generated using R software v.3.5.3.5
Results
Photosynthetic Characteristics and Leaf Nutrient Contents of Strawberry
Compared with no ventilation, soil ventilation increased the Pn, Tr, and WUE of strawberry plants in its two growth stages. Pn and WUE were slightly higher in the FDS than VGS, with no significant difference between LD and HD. Soil ventilation increased LA in both VGS and FDS stages. The leaf N concentration was increased in the VGS yet decreased in the FDS, whereas the leaf P and K concentration were both increased by ventilation in both growth stages (Table 1).
Soil Physicochemical Properties
Soil ventilation significantly increased the soil O2 concentration and O2:CO2 ratio, while reducing the soil CO2 concentration. It also increased soil temperature in the FDS, but had no significant effect on soil pH in either growth stage. Planting density significantly influenced the soil O2:CO2 ratio, in that it was reduced when a greater planting density of strawberry was used. Significant differences in soil properties (soil O2, CO2 concentrations, O2:CO2 ratio, temperature, and pH) were evident between the two growth stages (Figures 1A–E and Supplementary Table 1).
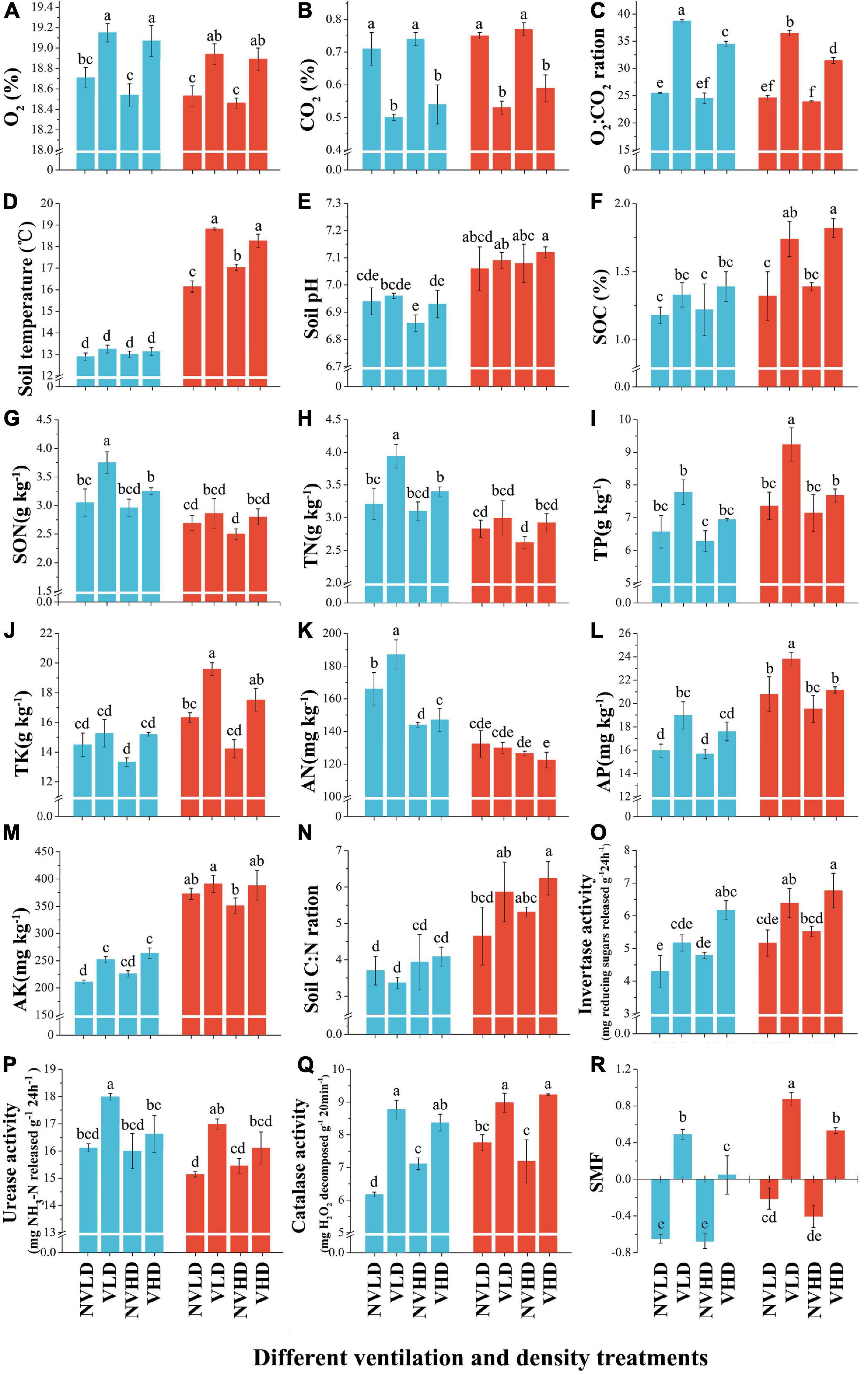
Figure 1. Soil physicochemical index, nutrient index, enzyme activities, and soil multifunctional index (SMF) at two growth stages (vegetative growth stage and fruit development stage) of strawberry under different soil ventilation and planting density treatments. (A) O2 concentration, %; (B) CO2 concentration, %; (C) O2:CO2 ratio; (D) soil temperature, °C; (E) soil pH; (F) SOC (soil organic carbon), %; (G) SON (soil organic nitrogen), g kg–1; (H) TN (soil total nitrogen), g kg–1; (I) TP (soil total phosphorus), g kg–1; (J) TK (soil total potassium), g kg–1; (K) AN (soil available nitrogen), g kg–1; (L) AP (soil available phosphorus), g kg–1; (M) AK (soil available potassium), g kg–1; (N) soil C/N ratio (soil organic carbon-to-total nitrogen ratio); (O) invertase activity, mg reducing sugars released g–1 24 h–1; (P) urease (URE) activity, mg NH3-N released g–1 24 h–1; (Q) catalase activity, mg H2O2 decomposed g–1 20 min–1; (R) SMF (soil multifunctionality index), mg H2O2 decomposed g–1 20 min–1. The vegetative growth stage (VGS) and fruit development stage (FDS) are shown in sky blue and red, respectively. Results are shown as mean ± SD. Error bars represent standard deviation (SD) (n = 3). Different letters indicate significant differences between the means of each index (p < 0.05, Tukey’s test).
Soil ventilation and plant density influenced the levels of most soil nutrients in the two growth stages of strawberry, as seen in Figure 1 and Supplementary Table 2. Soil ventilation increased the SOC content, and this effect was significant in the FDS. It also increased soil organic nitrogen (SON), TN, TP, TK, AP, and AK contents, all of which were diminished by a higher planting density. Soil TN content was higher in the VGS than FDS, while soil TP and TK contents showed the opposite trend (Figures 1G–J).
Soil ventilation enhanced the activities of three soil enzymes (URE, INV, and CAT). The INV activity was increased with a greater planting density. URE activity was slightly higher while INV activity was lower in the VGS than FDS (Figures 1O–Q). Soil ventilation increased the SMF, and it was higher in the FDS than VGS (Figure 1R and Supplementary Table 2).
The PCA revealed significant differences between the two growth stages of strawberry plants. The soil N cycle dominated in the VGS whereas the soil C cycle (including the P and K cycles) was dominated in the FDS (Figure 2A). The RDA showed that soil O2 concentration was positively correlated with those soil nutrient variables related to N cycling; soil temperature and pH were redundant among the soil nutrient variables that are related to C cycling (Figure 2B).
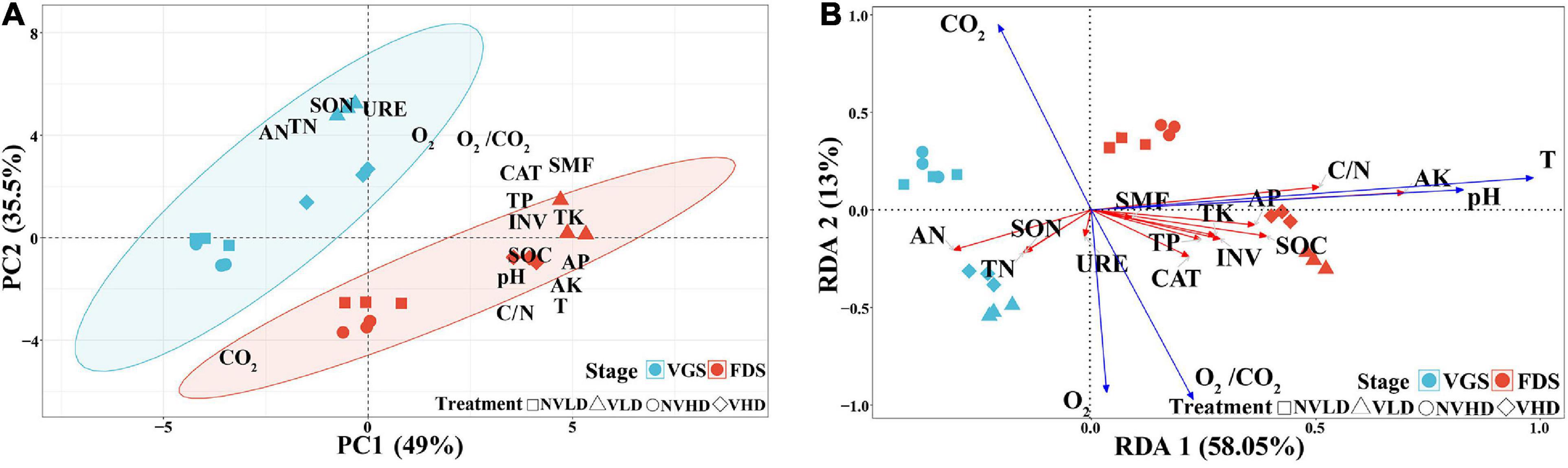
Figure 2. Principal component analysis (PCA) based on soil chemical and physicochemical parameters as variables and redundancy analysis (RDA) of the soil chemical parameters data as explained by environmental variables (O2, CO2, O2/CO2, T, and pH) at two growth stages (vegetative growth stage and fruit development stage) of strawberry under different soil ventilation and planting density treatments. (A) PCA; (B) RDA. The vegetative growth stage (VGS) and fruit development stage (FDS) are shown in sky blue and red, respectively.
Diversity, Composition, and Structure of Soil Bacterial Community
The Sobs, Chao, and Shannon indices for the total bacterial community, estimated in terms of its OTUs and relative abundances, were not significantly affected by ventilation according to the PERMANOVA results (Supplementary Table 3). However, the bacterial diversity (Shannon index) and richness were higher at HD than LD in the VGS, but there was no significant difference at the FDS (Figure 3).
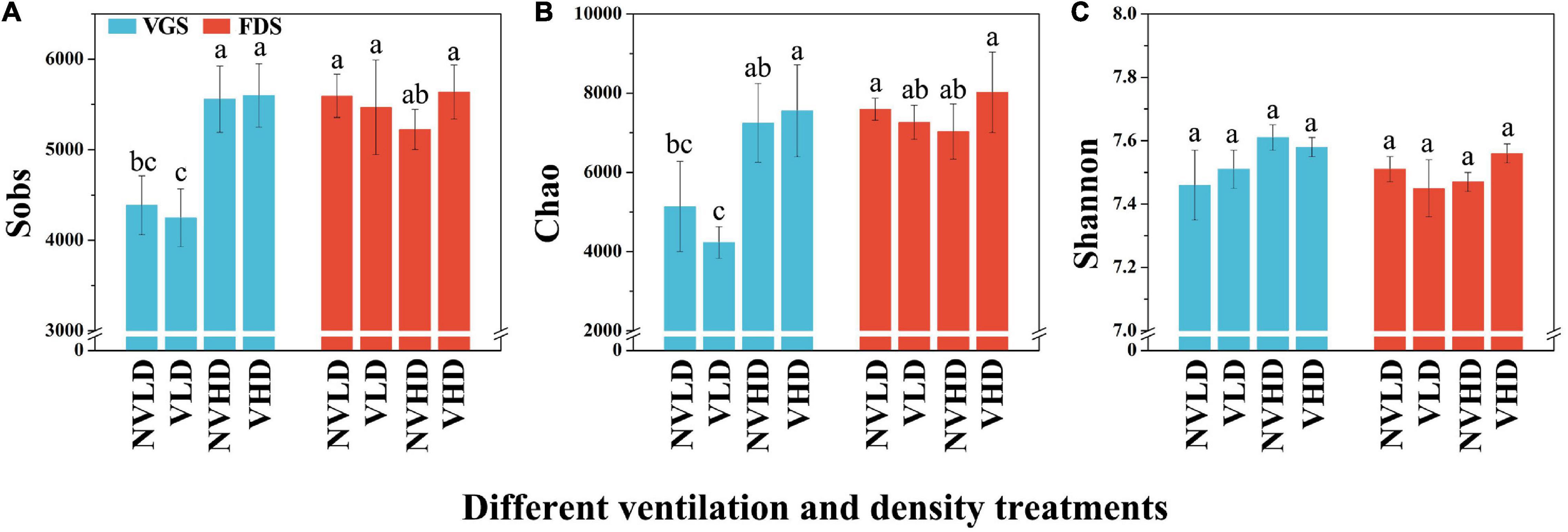
Figure 3. Sobs (A), Chao (B), and Shannon (C) indices of the bacterial community in the studied soils. The vegetative growth stage (VGS) and fruit development stage (FDS) are shown in sky blue and red, respectively. Sobs is the observed operational taxonomic units (OTUs); Chao was used to evaluate community richness based on OTUs; the Shannon index was used to assess community diversity, considering the contributions of rare taxa. Results are shown as mean ± SD. Error bars represent standard deviation (SD) (n = 3). Different letters indicate significant differences between the means of each index (p < 0.05, Tukey’s test).
The soil bacterial community was dominated by the following phyla: Proteobacteria (34%), Planctomycetes (12%), Acidobacteria (9%), Bacteroidetes (8%), and Actinobacteria (5%). Ventilation markedly reduced the relative abundance of Proteobacteria, Gemmatimonadetes, and Chloroflexi, and increased that of Firmicutes. Compared with LD, a greater planting density (HD) increased the relative abundance of Acidobacteria but decreased that of Bacteroidetes in the VGS. The significant differences were found between the two growth stages of strawberry. For example, the relative abundances of Proteobacteria, Acidobacteria, and Chlamydiae were all lower while those of Planctomycetes, Actinobacteria, Chloroflexi, and Firmicutes were higher in the VGS than FDS (Figure 4A). The dominant phyla were significantly influenced by the interaction term of ventilation × density.
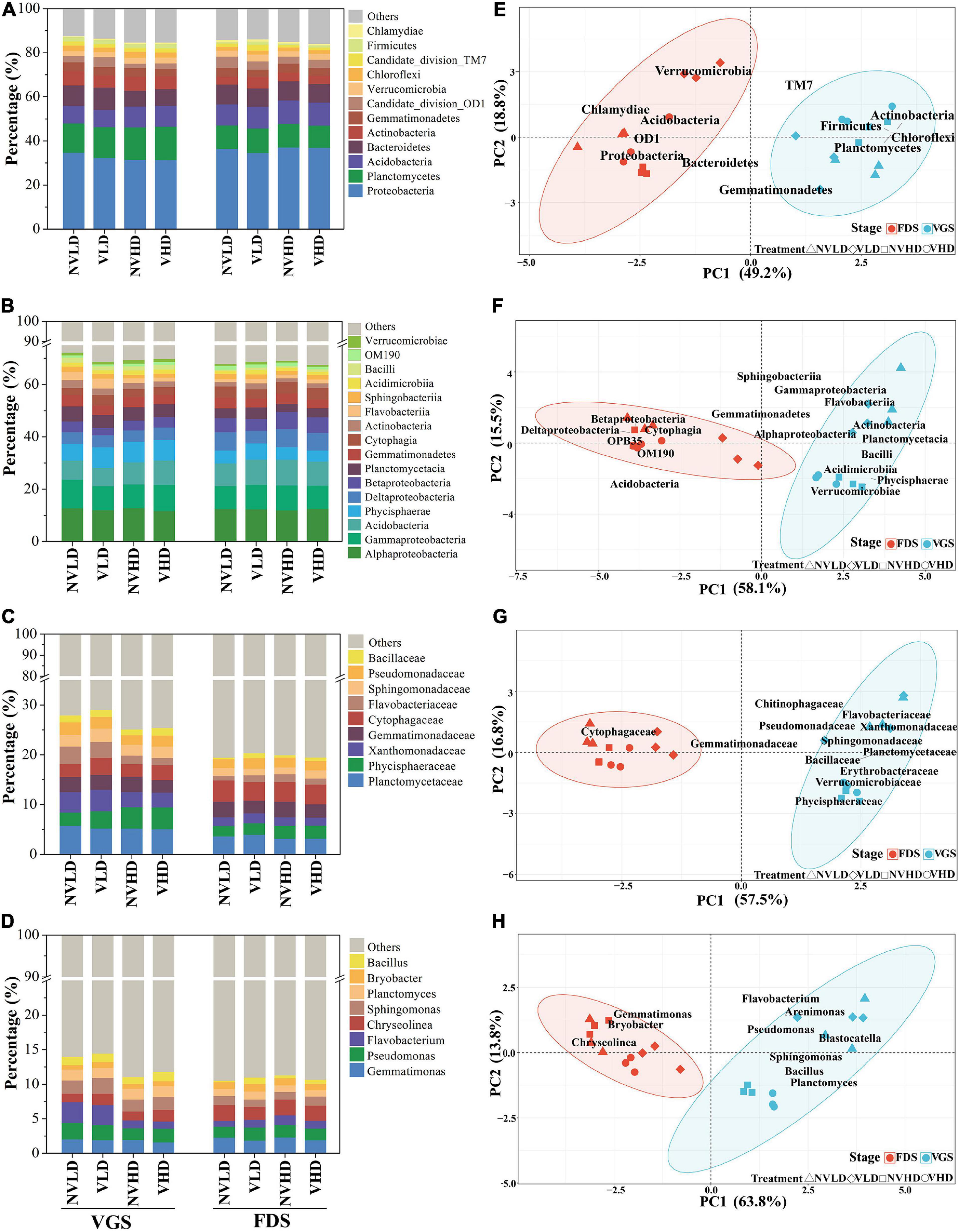
Figure 4. Total bacterial community composition as determined by 16S rRNA gene sequencing. (A–D) Community composition at the phylum (A), class (B), family (C), and genus (D) levels. (E–H) Community structure at the phylum (E), class (F), family (G), and genus (H) levels. Only dominant populations (as long as one of the treatments has a relative abundance > 1%) are represented. The vegetative growth stage (VGS) and fruit development stage (FDS) are shown in sky blue and red, respectively.
The relative abundances of the top 16 dominant classes of bacteria were influenced by ventilation and planting density at the two growth stages. Ventilation altered the relative abundances of seven classes, with increases in Phycisphaerae and Bacilli and decreases in Alphaproteobacteria, Betaproteobacteria, Deltaproteobacteria, and Gemmatimonadetes. Planting density markedly influenced the relative abundances of 11 classes: in this respect, Acidobacteria and Verrucomicrobiae were higher whereas Flavobacteriia and Sphingobacteriia were lower at the HD than LD in the VGS (Figure 4B). Ventilation significantly increased the relative abundance of Phycisphaeraceae in the VGS and Bacillaceae in the FDS, though it reduced that of Gemmatimonadaceae in the FDS (Figure 4C and Supplementary Table 4C). The relative abundance of Bacillus was increased by soil ventilation whereas that of Pseudomonas and Flavobacterium was reduced by higher planting density at the VGS (Figure 4D).
The PERMANOVA revealed significant effects of ventilation, density, and growth stage, as well as their interactions, on microbial community structure at different taxonomic levels (Supplementary Table 5). Ventilation, mainly affected the structure of the total bacterial community at family and genus levels, while planting density did so at the class and genus levels. Significant differences between the two growth stages arose mainly at the genus level (Figures 4E–H and Supplementary Table 5).
Diversity, Composition, and Structure of Soil Fungal Community
The Sobs, Chao, and Shannon indices of the total fungal community were also not significantly affected by ventilation (Supplementary Table 3). The fungal diversity (Shannon index) was influenced by planting density and growth stage of strawberry plants and interactions among the three factors, being lower in the FDS than VGS (Figure 5 and Supplementary Table 3).
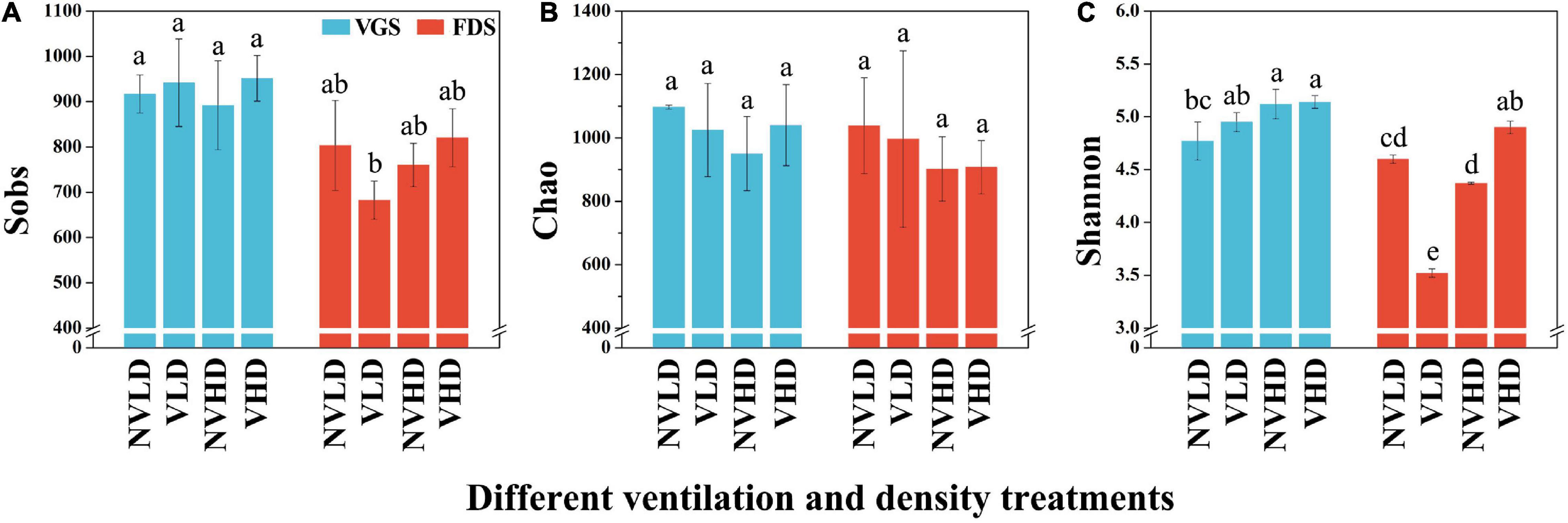
Figure 5. Sobs (A), Chao (B), and Shannon (C) indices of the fungal community in the studied soils. The vegetative growth stage (VGS) and fruit development stage (FDS) are shown in sky blue and red, respectively. Sobs is the observed operational taxonomic units (OTUs); Chao was used to evaluate community richness based on OTUs; the Shannon index was used to assess community diversity, considering the contributions of rare taxa. Results are shown as mean ± SD. Error bars represent standard deviation (SD) (n = 3). Different letters indicate significant differences between the means of each index (p < 0.05, Tukey’s test).
The fungal community was dominated by Zygomycota (9%), Chytridiomycota (6%), Basidiomycota (6%), and Ascomycota (3%) in all four treatment groups (Figure 6A). Ventilation reduced the relative abundance of Basidiomycota and increased that of Chytridiomycota. The relative abundance of Basidiomycota was higher, whereas that of Ascomycota and Zygomycota were both lower at the HD than LD. Furthermore, the interactions of the three factors influenced the relative abundance of the fungal community to different degrees at the phylum level (Figure 6A and Supplementary Table 6).
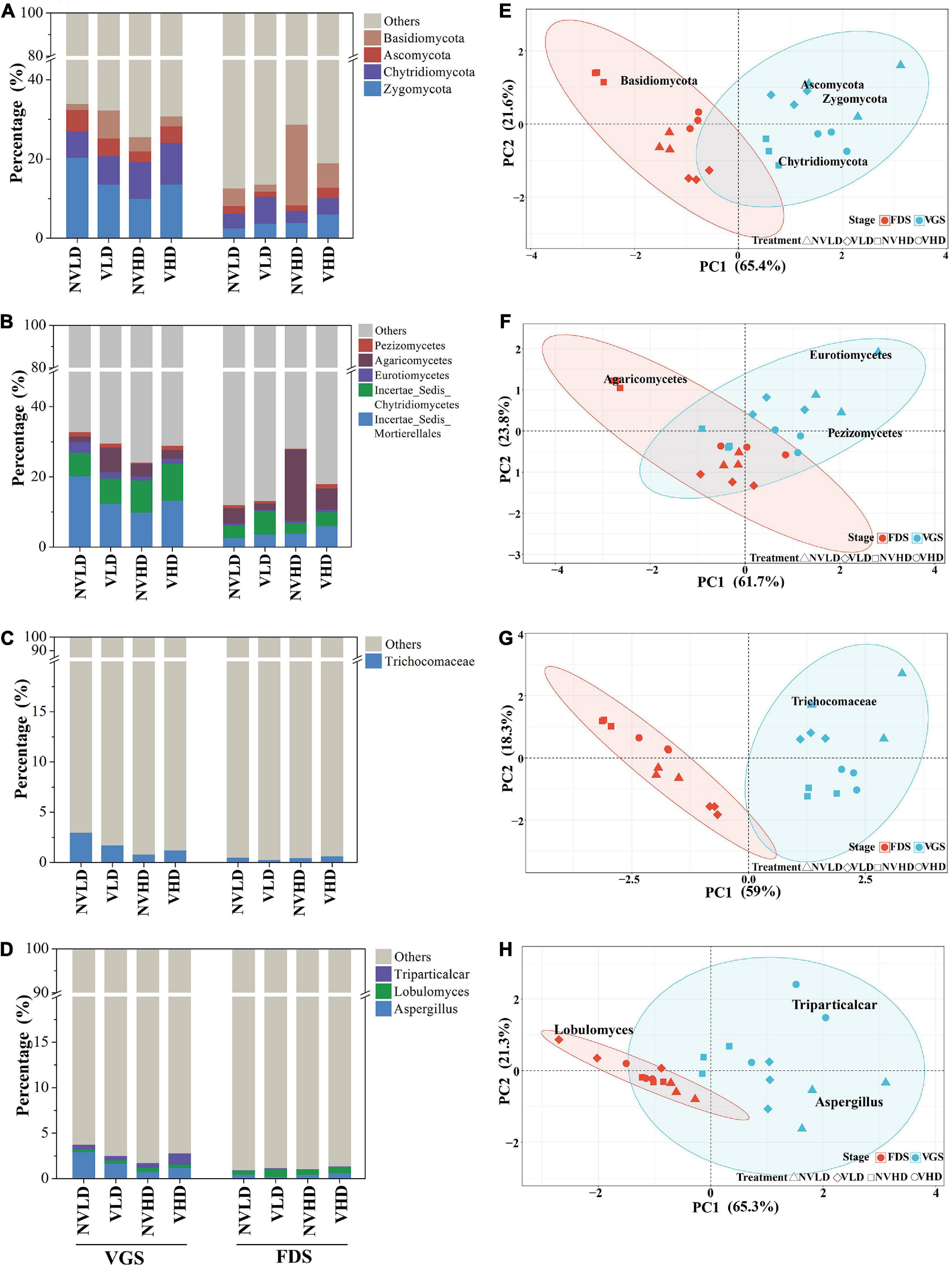
Figure 6. Total fungal community composition as determined by 18S rRNA gene sequencing. (A–D) Community composition at the phylum (A), class (B), family (C), and genus (D) levels. (E–H) Community structure at the phylum (E), class (F), family (G), and genus (H) levels. Only dominant populations (as long as one of the treatments has a relative abundance > 1%) are represented. The vegetative growth stage (VGS) and fruit development stage (FDS) are shown in sky blue and red, respectively.
The relative abundances of three dominant classes of fungi were altered by the treatments. For instance, soil ventilation reduced the relative abundance of Agaricomycetes and increased that of Pezizomycetes. The relative abundance of Eurotiomycetes and Pezizomycetes was higher, while that of Agaricomycetes was lower in the VGS than in the FDS (Figure 6B). Aspergillus, Lobulomyces, and Triparticalcar were the three dominant genera in soil. The relative abundance of Aspergillus was affected by density and growth stage and their interactions; that of Lobulomyces was increased by ventilation in the FDS (Figure 6D).
Functional Diversity of Microbial Community
Functional Groups Related to the Soil C Cycle
Among the 14 functional groups related to the C cycle in the bacterial community identified by FAPROTAX analysis, chemoheterotrophy and aerobic chemoheterotrophy assemblages were dominant. Ventilation reduced the enrichment of predatory or exoparasitic and methylotrophy groups. However, enrichment of the chemoheterotrophy, aerobic chemoheterotrophy, intracellular parasites, photoautotrophy, cyanobacteria, oxygenic photoautotrophy, and aromatic compound degradation functional groups all decreased with an increased planting density. Growth stage influenced the assemblage of 13 observed functional groups, including chemoheterotrophy, chitinolysis, and aromatic compound degradation, all of which were higher in the VGS than FDS, and vice versa for the photoautotrophy, cyanobacteria, and methylotrophy trends (Figure 7A).
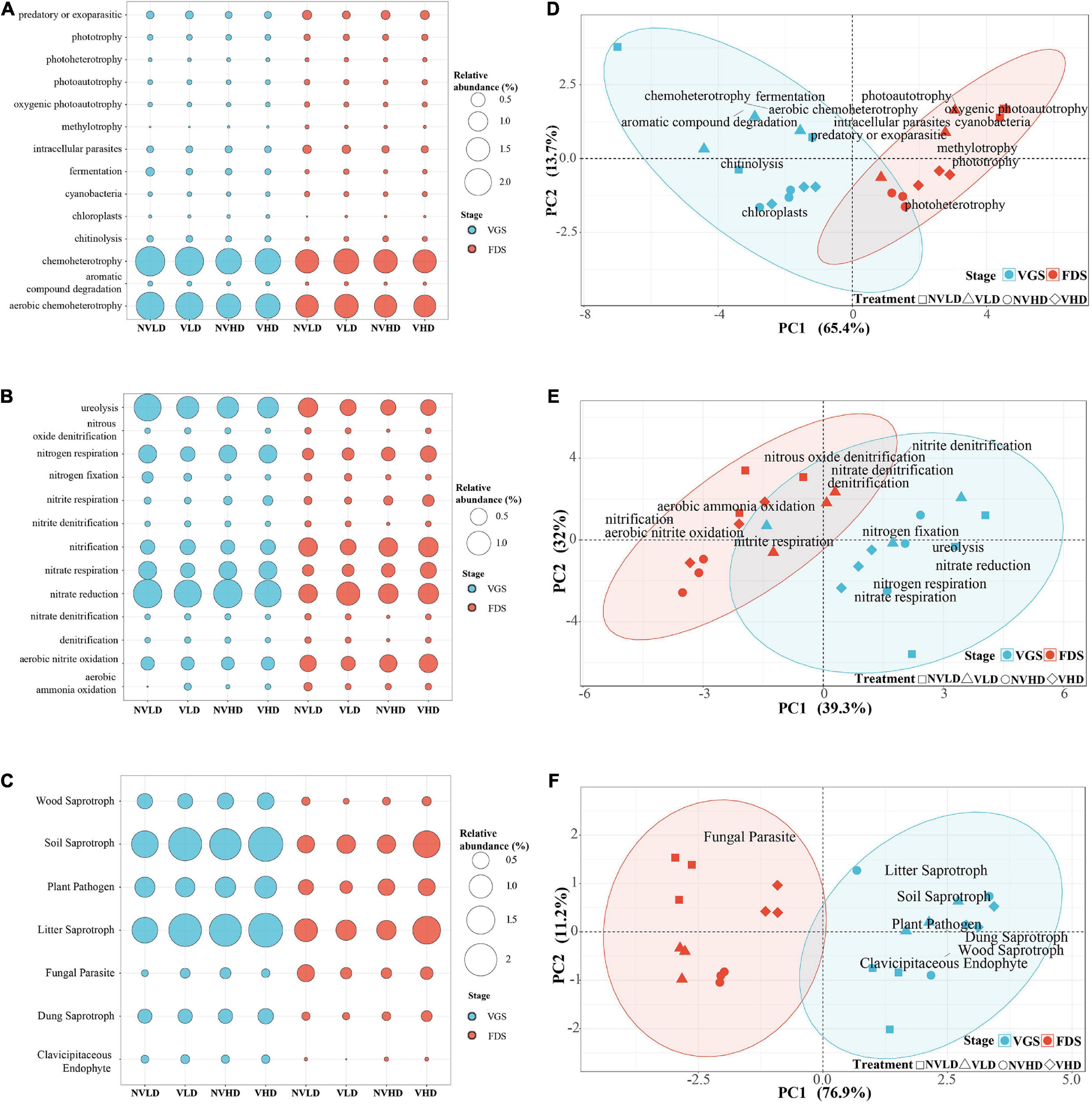
Figure 7. Composition and structure of the functional groups in microbial communities. (A–C) Composition of the bacterial community related to the C cycle (A) and N cycle (B) and of the fungal community (C). (D–F) Functional structure of the bacterial community related to the C cycle (D) and N cycle (E) and of the fungal community (F). The vegetative growth stage (VGS) and fruit development stage (FDS) are shown in sky blue and red, respectively.
Functional Groups Related to the Soil N Cycle
Functional groups related to the N cycle in the bacterial community indicated that the dominant assemblages of bacterial groups were associated with nitrate reduction, ureolysis, aerobic nitrite oxidation, nitrate respiration, N respiration, nitrification, N fixation, and nitrite respiration in the studied soils. Ventilation inhibited the assemblage of the ureolysis group yet promoted that of the nitrite respiration group. When compared with LD, HD reduced the assemblage of ureolysis and N fixation groups, but it increased that of the nitrification, nitrate respiration, and nitrite respiration groups. Growth stage influenced the assemblage of eight functional groups related to N cycle (Figure 7B and Supplementary Table 3).
Functional Diversity of Fungal Community
Functional groups identified by FUNGuild analysis in the fungal community showed that the dominant assemblages in soil were litter saprotroph, soil saprotroph, and plant pathogen. Ventilation significantly reduced the assemblages of fungal parasite groups and enhanced that of soil saprotrophs, which were increased by the HD (Figure 7C).
The PCA of the relative abundances of bacterial and fungal functional groups revealed that they sorted according to the growth stage (S) of strawberry along PC1 (Figures 7D–F and Supplementary Table 8).
Correlations Between Soil Ventilation and Measured Variables
Soil O2 concentration was positively correlated with soil N components. Importantly, it was positively correlated with the relative abundances of Firmicutes (Bacilli, Bacillaceae, and Bacillus); Pseudomonadaceae (Pseudomonas); Arenimonas, Blastocatella, Sphingomonadaceae, Sphingomonas, Chytridiomycota (Triparticalcar), and Pezizomycetes, but negatively correlated with those Betaproteobacteria and Gemmatimonadetes (Gemmatimonadetes, Gemmatimonadaceae, and Gemmatimonas). Soil O2 concentration was positively correlated with litter and soil saprotrophs in the fungal community, but not correlated with the C and N functional groups of the bacterial community. Additionally, soil O2 concentration was negatively correlated with the Tr of strawberry (Figure 8A).
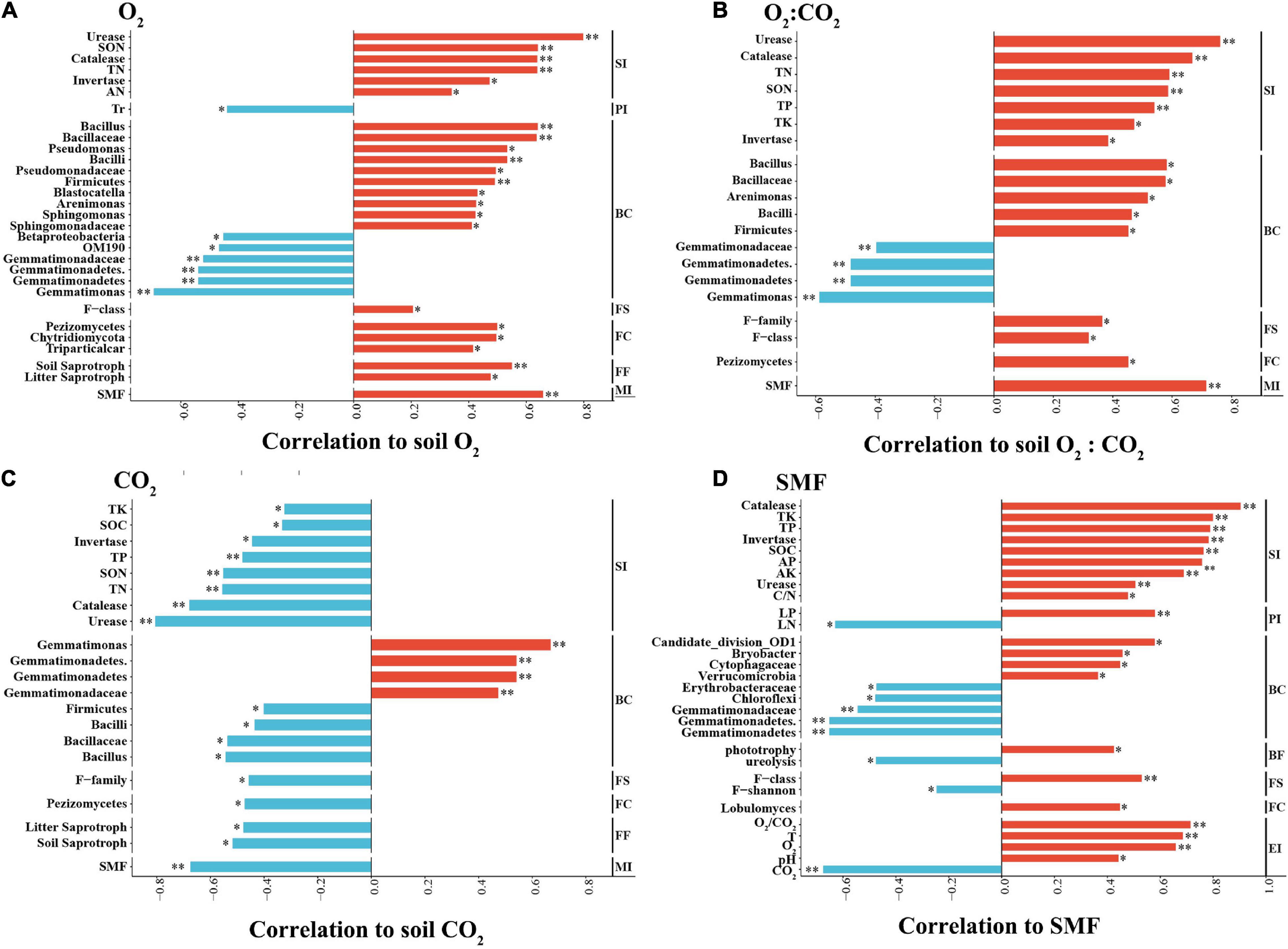
Figure 8. Spearman correlation coefficients between soil environmental factors and soil physicochemical parameters and microbial community. (A) Soil O2 concentration; (B) soil CO2 concentration; (C) soil O2:CO2 ratio; (D) SMF (soil multifunctionality index). The abundance of total bacterial and fungal populations was analyzed by their 16S and 18S rRNA gene sequencing, respectively. BC, bacterial composition; BF, bacterial function; BS, bacterial structure; FC, fungal composition; FF, fungal function; FS, fungal structure; MI, multifunctional index; PI, plant index; SI, soil index; EI, environmental index. The negative and positive correlations are shown in sky blue and red, respectively. ∗p < 0.05; ∗∗p < 0.01.
For soil CO2 concentration, the pattern of its correlations with the above indices was the opposite of that found for soil O2 concentration. More specifically, soil CO2 concentration was negatively correlated with the SOC content and TP and TK concentrations (Figure 8B). Soil temperature and pH were negatively correlated with soil CO2 concentration. SMF was positively correlated with O2 concentration and with the relative abundances of Verrucomicrobia and Bryobacter, yet negatively correlated with those of Chloroflexi and Gemmatimonadetes, as well as with the functional group capable of ureolysis. Additionally, the SMF was positively correlated with the leaf P concentration of strawberry plants (Figure 8D).
Discussion
Soil Ventilation Effects on the Profile of Soil Microbial Community
In this study, in situ ventilation increased the concentration of soil O2 and decreased that of soil CO2 (Figure 1). O2 concentration and supply rates in the root zone plays a critical role in root respiration, root elongation, nutrient absorption and transpiration, and plant growth (Zhao et al., 2019). In this process, soil bacterial and fungal communities also play vital roles in the turnover of SOC and C cycling (Zhao et al., 2018). In the present work, OM decomposition driven by the soil microbial community was activated by ventilation. It has been reported that soil microbial communities regulate many ecosystem processes and contribute to nutrient cycling through the decomposition of OM (Bender et al., 2016; Hartman et al., 2018). Moreover, the positive (soil O2) and negative (soil CO2) correlation with soil N components suggest that soil O2 and CO2 have antagonistic effects on N mineralization through soil enzyme activities. This may be consistent with the fact that rhizosphere ventilation can improve the potted tomato root zone environment, increase the soil enzyme activity, and promote the nutrient uptake (Niu et al., 2012).
In this study, ventilation increases relative abundance of Firmicutes phyla and its Bacilli (class, family, and genus) and decreases that of Gemmatimonadetes (Figure 4). It has been reported that aeration irrigation significantly increased the abundance of aerobic bacteria, such as Nitrospira and Cytophagia (Zhao et al., 2019). Furthermore, the abundance of Bacillus was positively correlated with soil O2 concentrations (Figure 8A). Bacteria of the genera Pseudomonas and Bacillus can promote plant growth and protect plants from pathogens. A high relative abundance of aerobic Bacillus promotes plant growth and inhibits the growth of pathogenic bacteria via the decomposition of labile OM in soil (Razanamalala et al., 2018; Molina-Santiago et al., 2019). Conversely, Gemmatimonadetes abundance was negatively correlated with soil O2 concentration (Figure 8A). This result is consistent with the finding that the relative abundance of Gemmatimonadetes decreased in the presence of wheat residues (Bernard et al., 2007) and that Gemmatimonadetes was more active in soils without leaf litter than in the litter treatments (Pfeiffer et al., 2013). Thus, members of the Gemmatimonadetes phylum may be adapted to a lifestyle associated with OM sources that are challenging to mineralize along O2 gradients (Whitman et al., 2016).
Ventilation did not alter the fungal alpha-diversity in soil (Supplementary Table 3), but enhanced Chytridiomycota and reduced Basidiomycota at the phylum level, reduced Pezizomycetes and Agaricomycetes at the class level, and increased Triparticalcar at the genus level (Figure 6). The OM decomposition rate was positively correlated with the relative abundance of Chytridiomycota, perhaps because of the association between the availability of labile C and the soil O2 concentration, which is related to the recruitment of Chytridiomycota (Procter et al., 2014). The relative abundance of Basidiomycota decreased with soil O2 concentration, suggesting that wood decay and plant litter decomposition were slowed in the ventilated soil. Ventilation increased abundances of litter and soil saprotrophs groups, which were positively correlated with soil O2 concentrations (Figure 8), which may promote the decomposition of soil labile OM and inhibit the occurrence of plant pathogens, resulting in accelerated N mineralization rates (Razanamalala et al., 2018). It also has been demonstrated that although some fungi are sensitive, the community remains stable upon changes in soil O2 or CO2 (Kaisermann et al., 2014; Zhang et al., 2016; Zi et al., 2018). Thus, aerobic bacteria and functional fungi of soil play major roles in accelerating N mineralization under ventilated conditions.
Planting Density Effects on Soil Oligotrophic and Copiotrophic Groups
The crop cultivation model can influence soil microbial attributes (Hartmann et al., 2015; García-Delgado et al., 2019; Xiao et al., 2019). For example, intra- and inter-specific plant competition, especially at a high planting density, as well as the identity of the plant species themselves, can influence the rhizosphere bacterial community (Cavalieri et al., 2020). In our study, the planting density affected soil CO2 concentration, and the TN and AN concentration and URE activity were all higher at LD when compared to HD in the VGS (Figure 1). Planting density has been shown to alter soil nutrient supply in the forest: increased N mineralization was beneficial to plant morphogenesis and increased the N content of leaves, while C mineralization enhanced plant C assimilation and water utilization and increased the leaf P content (Bastida et al., 2017). Consistent with those results for soil nutrients, compared with HD, we also found that the relative abundances of oligotrophic groups (Acidobacteria and Chloroflexi) were lower while those of copiotrophic groups (Bacteroidetes, Actinobacteria, and Gammaproteobacteria) were higher at LD. Similar results were reported recently, in that Proteobacteria was more abundant under a high-intensity thinning treatment, whereas Acidobacteria was more abundant under low-intensity thinning and control treatments (Dang et al., 2018). The changes to copiotrophic and oligotrophic groups according to planting density suggests that the soil microbial community composition depends on soil nutrient levels, further indicating a cooperative effect among microbial communities in adapting to the external environment. This is because N inputs significantly increased the relative abundance of the predicted copiotrophic groups (Proteobacteria and Firmicutes) but reduced those of predicted oligotrophic groups (Acidobacteria, Nitrospirae, and Chloroflexi) (Ling et al., 2017). By contrast, HD reduced the enrichment of soil functional groups related to C (cyanobacteria, photoautotrophy) and N (ureolysis, N fixation) cycling. These functional groups may be sensitive to high C input in aerated soil, resulting in decreased C and N contents in aerated HD soil, because of soil resource competition during plant growth (Dang et al., 2018; Muñoz-Rojas et al., 2018). Soil, litter, and dung saprotrophs were enriched at HD, and this probably accelerated the C and N cycling dynamics. It is likely that LD slowed the degradation of organic substances due to low C input and reduced competition for nutrients between plants and microbes (Dang et al., 2018; Mommer et al., 2018).
Growth Stage Effects Soil Nutrient Composition via Soil Temperature and pH
Soil temperature and pH were both higher in FDS than in VGS during the strawberry growth stage (Figure 1). They are important environmental factors influencing soil microflora (Cheng et al., 2017; Lammel et al., 2018; Walker et al., 2018). These caused significant differences in the soil C and N compositions and enzyme activities in the ventilation soil. Usually, soil temperature and pH also co-vary with some confounding factors, such as land use and management practices (e.g., tillage, fertilizer application), plant cover, and climatic conditions. We found that soil temperature and pH play important roles in shaping the soil nutrient composition to meet the stage requirements for plant growth and development in ventilated soil. A more comprehensive analysis of the direct (e.g., Actinobacteria) and indirect effects of pH can clarify the mechanisms that shape soil microbial communities (Lammel et al., 2018). In this study, the soil N cycle was dominant in the VGS whereas the soil C cycle (accompanied by P and K cycles) was dominant in the FDS (Figure 2A), with corresponding trends found for the functional groups related to soil C and N cycles. Temperature-induced differences in gross N flux are related to total C availability and the soil microbiome profile (Cookson et al., 2007). Rhizospheric microbial community profiles of a variety of plants (e.g., pea, wheat, sugar beet, and alfalfa) are altered according to the plant developmental gradient (Mougel et al., 2006; Houlden et al., 2008); for example, the root microbiota of rice varies over time during the life cycle of rice plants in the field (Zhang et al., 2018). This potential selection of microbes in the rhizosphere through plant aging may be associated with the ability of beneficial microbes to adapt to intrinsic requirements through plant growth and development by promoting systemic tolerance to abiotic stress, increasing plant’s innate immunity (Zamioudis and Pieterse, 2012), and enhancing mineral nutrition (van der Heijden et al., 2008).
Conclusion
Our study shows that soil ventilation altered the microbial community profile and drove differences in C and N contents between VGS (more N components) and FDS (more C components), which enhanced strawberry growth under high-density planting environment. The soil aerobic bacteria (e.g., Bacillus) and functional fungi (e.g., litter and soil saprotrophs groups) play a major role in accelerating N mineralization under ventilation treatments. The copiotrophic and oligotrophic groups seem to cooperate to benefit strawberry growth mediated by planting density. However, further and more investigation of copiotrophic and oligotrophic bacterial groups are asked to test this hypothesis. More N components should be supplied in the VGS while both C and N components should be applied in the FDS to meet the requirements for normal strawberry plant and fruit development. Soil ventilation can be used as a tillage practice for improving soil quality, keeping soil health and maximize crop production in greenhouse ecosystems with high-density planting (hypoxia stress) or long-term continuous cropping. In order to reveal the underlying mechanism, the effect of ventilation on soil aggregate and functional genes of soil microbial community participating in N mineralization require further study. Meanwhile, it is crucial to consider the soil aeration and their effect on the whole soil microbiome when planning optimal agricultural management practices in greenhouse.
Data Availability Statement
The microbiota sequencing data presented in the study are deposited in the National Center for Biotechnology Information (NCBI) repository, accession number PRJNA721522.
Author Contributions
YY and JZ designed the study. YZ, YH, ZY, and ZLL performed the greenhouse and laboratory experiments. ZLL, MH, and ZML performed the data analysis. YZ, JZ, and YY wrote the first draft of the manuscript. All the authors contributed to the writing of this manuscript and approved the final version.
Funding
This study was supported by grants from the National Key Project of the Research and Development Plan (2016YFD0201116), the Beijing Key Project of the Science and Technology Plan (Z181100002418003/004), the National Natural Science Foundation of China (31171922 and 31301736), the Beijing Natural Science Fund-Municipal Education Commission (KZ201910020022), the Construction of Beijing Science and Technology Innovation and Service Capacity in Top Subjects (CEFF-PXM2019_014207_000032), the Beijing Key Laboratory of New Technique in Agricultural Application (NYYYXJS201704), and the China Postdoctoral Science Foundation (2019M650283).
Conflict of Interest
The authors declare that the research was conducted in the absence of any commercial or financial relationships that could be construed as a potential conflict of interest.
Publisher’s Note
All claims expressed in this article are solely those of the authors and do not necessarily represent those of their affiliated organizations, or those of the publisher, the editors and the reviewers. Any product that may be evaluated in this article, or claim that may be made by its manufacturer, is not guaranteed or endorsed by the publisher.
Acknowledgments
We thank the Beijing Collaborative Innovation Center for Eco-Environmental Improvement of Forestry and Fruit Trees; the Key Laboratory of Pomology, Beijing Nursery Engineering Research Center for Fruit Crops; the Key Laboratory of Agricultural Applications at the Beijing University of Agriculture; and the technicians at the Changping District Forestry Administration in Beijing.
Supplementary Material
The Supplementary Material for this article can be found online at: https://www.frontiersin.org/articles/10.3389/fmicb.2021.666982/full#supplementary-material
Footnotes
- ^ https://mothur.org
- ^ https://rdp.cme.msu.edu/
- ^ www.zoology.ubc.ca/louca/FAPROTAX
- ^ https://github.com/UMNFuN/FUNGuild
- ^ https://www.r-project.org/
References
Bastida, F., Torres, I. F., Andrés-Abellán, M., Baldrian, P., López-Mondéjar, R., Větrovský, T., et al. (2017). Differential sensitivity of total and active soil microbial communities to drought and forest management. Glob. Chang. Biol. 23, 4185–4203. doi: 10.1111/gcb.13790
Bender, S. F., Wagg, C., and van der Heijden, M. G. A. (2016). An underground revolution: biodiversity and soil ecological engineering for agricultural sustainability. Trends Ecol. Evol. 31, 440–452. doi: 10.1016/j.tree.2016.02.016
Ben-Noah, I., and Friedman, S. P. (2018). Review and evaluation of root respiration and of natural and agricultural processes of soil aeration. Vad. Zone J. 17, 1–47. doi: 10.2136/vzj2017.06.0119
Ben-Noah, I., Nitsan, I., Cohen, B., Kaplan, G., and Friedman, S. P. (2021). Soil aeration using air injection in a citrus orchard with shallow groundwater. Agric. Water Manag. 245:106664. doi: 10.1016/j.agwat.2020.106664
Bernard, L., Mougel, C., Maron, P., Nowak, V., Lévêque, J., Henault, C., et al. (2007). Dynamics and identification of soil microbial populations actively assimilating carbon from 13C-labelled wheat residue as estimated by DNA-and RNA-SIP techniques. Environ. Microbiol. 9, 752–764. doi: 10.1111/j.1462-2920.2006.01197.x
Bhattarai, S. P., Huber, S., and Midmore, D. J. (2004). Aerated subsurface irrigation water gives growth and yield benefits to zucchini, vegetable soybean and cotton in heavy clay soils. Ann. Appl. Biol. 144, 285–298. doi: 10.1111/j.1744-7348.2004.tb00344.x
Bhattarai, S. P., Pendergast, L., and Midmore, D. J. (2006). Root aeration improves yield and water use efficiency of tomato in heavy clay and saline soils. Sci. Hortic. 108, 278–288. doi: 10.1016/j.scienta.2006.02.011
Bremner, J. M. (1996). “Nitrogen total,” in Methods of Soil Analysis Part 3: Chemical Methods, SSSA Book Series 5, ed. D. L. Sparks (Madison, WI: Soil Science Society of America), 1085–1122.
Cavalieri, A., Bak, F., Garcia-Lemos, A. M., Weiner, J., Nicolaisen, M. H., Nybroe, O., et al. (2020). Effects of intra- and interspecific plant density on rhizosphere bacterial communities. Front. Microbiol. 11:1045. doi: 10.3389/fmicb.2020.01045
Cheng, L., Zhang, N., Yuan, M., Xiao, J., Qin, Y., Deng, Y., et al. (2017). Warming enhances old organic carbon decomposition through altering functional microbial communities. ISME J. 11, 1825–1835. doi: 10.1038/ismej.2017.48
Cookson, W. R., Osman, M., Marschner, P., Abaye, D. A., Clark, I., Murphy, D. V., et al. (2007). Controls on soil nitrogen cycling and microbial community composition across land use and incubation temperature. Soil Biol. Biochem. 39, 744–756. doi: 10.1016/j.soilbio.2006.09.022
Dang, P., Gao, Y., Liu, J., Yu, S., and Zhao, Z. (2018). Effects of thinning intensity on understory vegetation and soil microbial communities of a mature Chinese pine plantation in the Loess Plateau. Sci. Total Environ. 630, 171–180. doi: 10.1016/j.scitotenv.2018.02.197
Delgado-Baquerizo, M., Maestre, F. T., Reich, P. B., Jeffries, T. C., Gaitan, J. J., Encinar, D., et al. (2016). Microbial diversity drives multifunctionality in terrestrial ecosystems. Nat. Commun. 7:10541. doi: 10.1038/ncomms10541
Du, Y., Gu, X., Wang, J., and Niu, W. (2019). Yield and gas exchange of greenhouse tomato at different nitrogen levels under aerated irrigation. Sci. Total Environ. 668, 1156–1164. doi: 10.1016/j.scitotenv.2019.03.098
Edgar, R. C. (2013). UPARSE: highly accurate OTU sequences from microbial amplicon reads. Nat. Methods 10, 996–998. doi: 10.1038/NMETH.2604
Friedman, S. P., and Naftaliev, B. (2012). A survey of the aeration status of drip-irrigated orchards. Agric. Water Manag. 115, 132–147. doi: 10.1016/j.agwat.2012.08.015
García-Delgado, C., Barba-Vicente, V., Marín-Benito, J. M., Mariano Igual, J., Sánchez-Martín, M. J., Rodríguez-Cruz, S., et al. (2019). Influence of different agricultural management practices on soil microbial community over dissipation time of two herbicides. Sci. Total Environ. 646, 1478–1488. doi: 10.1016/j.scitotenv.2018.07.395
Glinski, J., and Stepniewski, W. (1985). Soil Aeration and Its Role for Plants. Boca Raton, FL: CRC Press.
Hartman, K., van der Heijden, M. G. A., Wittwer, R. A., Banerjee, S., Walser, J., Schlaeppi, K., et al. (2018). Cropping practices manipulate abundance patterns of root and soil microbiome members paving the way to smart farming. Microbiome 6:14. doi: 10.1186/s40168-017-0389-9
Hartmann, M., Frey, B., Mayer, J., Mäder, P., and Widmer, F. (2015). Distinct soil microbial diversity under long-term organic and conventional farming. ISME J. 9, 1177–1194. doi: 10.1038/ismej.2014.210
Hou, H. J., Chen, H., Cai, H. J., Yang, F., Li, D., and Wang, F. T. (2016). CO2 and N2O emissions from Lou soils of greenhouse tomato fields under aerated irrigation. Atmos. Environ. 132, 69–76. doi: 10.1016/j.atmosenv.2016.02.027
Houlden, A., Timms-Wilson, T. M., Day, M. J., and Bailey, M. J. (2008). Influence of plant developmental stage on microbial community structure and activity in the rhizosphere of three field crops. FEMS Microbiol. Ecol. 65, 193–201. doi: 10.1111/j.1574-6941.2008.00535.x
Huang, Y., Xiao, X., Huang, H., Jing, J., Zhao, H., Long, X.-E., et al. (2018). Contrasting beneficial and pathogenic microbial communities across consecutive cropping fields of greenhouse strawberry. Appl. Microbiol. Biot. 102, 5717–5729. doi: 10.1007/s00253-018-9013-6
Ityel, E., Ben-Gal, A., Silberbush, M., and Lazarovitch, N. (2014). Increased root zone oxygen by a capillary barrier is beneficial to bell pepper irrigated with brackish water in an arid region. Agric. Water Manag. 131, 108–114. doi: 10.1016/j.agwat.2013.09.018
Iwasaki, Y. (2008). Root zone aeration improves growth and yields of coir-cultured strawberry (Fragaria ananassa Duch.) during summer. Acta Hortic. 319, 251–254. doi: 10.17660/ActaHortic.2008.779.30
Jin, K., Sleutel, S., Buchan, D., De Neve, S., Cai, D. X., Gabriels, D., et al. (2009). Changes of soil enzyme activities under different tillage practices in the Chinese Loess Plateau. Soil Till. Res. 104, 115–120. doi: 10.1016/j.still.2009.02.004
Kaisermann, A., Maron, P. A., Beaumelle, L., and Lata, J. C. (2014). Fungal communities are more sensitive indicators to non-extreme soil moisture variations than bacterial communities. Appl. Soil Ecol. 86, 158–164. doi: 10.1016/j.apsoil.2014.10.009
Kitaya, Y., Yabuki, K., and Kiyata, M. (1984). Studies on the control of gaseous environment in the rhizosphere. Effect of carbon dioxide in the rhizosphere on growth of cucumber. J. Agric. Met. 40, 119–124. doi: 10.2480/agrmet.40.119
Klindworth, A., Pruesse, E., Schweer, T., Peplies, J., Quast, C., Horn, M., et al. (2013). Evaluation of general 16S ribosomal RNA gene PCR primers for classical and next-generation sequencing-based diversity studies. Nucleic Acids Res. 41:e1. doi: 10.1093/nar/gks808
Lammel, D. R., Barth, G., Ovaskainen, O., Cruz, L. M., Zanatta, J. A., Ryo, M., et al. (2018). Direct and indirect effects of a pH gradient bring insights into the mechanisms driving prokaryotic community structures. Microbiome 6:106. doi: 10.1186/s40168-018-0482-8
Li, W., and Liu, Q. (2019). Changes in fungal community and diversity in strawberry rhizosphere soil after 12 years in the greenhouse. J. Integr. Agric. 18, 677–687. doi: 10.1016/S2095-3119(18)62003-9
Li, Y., Niu, W., Cao, X., Zhang, M., Wang, J., Zhang, Z., et al. (2020). Growth response of greenhouse-produced muskmelon and tomato to sub-surface drip irrigation and soil aeration management factors. BMC Plant Biol. 20:141. doi: 10.1186/s12870-020-02346-y
Li, Y., Niu, W., Dyck, M., Wang, J., and Zou, X. (2016a). Yields and nutritional of greenhouse tomato in response to different soil aeration volume at two depths of subsurface drip irrigation. Sci. Rep-UK 6:39307. doi: 10.1038/srep39307
Li, Y., Niu, W., Xu, J., Wang, J., Zhang, M., Wang, V., et al. (2016b). Root morphology of greenhouse produced muskmelon under sub-surface drip irrigation with supplemental soil aeration. Sci. Hortic. 201, 287–294. doi: 10.1016/j.scienta.2016.02.018
Ling, N., Chen, D., Guo, H., Wei, J., Bai, Y., Wei, J., et al. (2017). Differential responses of soil bacterial communities to long-term N and P inputs in a semi-arid steppe. Geoderma 292, 25–33. doi: 10.1016/j.geoderma.2017.01.013
Liu, F., Fan, J., Du, J., Shi, X., Zhang, J., Shen, Y., et al. (2019). Intensified nitrogen transformation in intermittently aerated constructed wetlands: removal pathways and microbial response mechanism. Sci. Total Environ. 650, 2880–2887. doi: 10.1016/j.scitotenv.2018.10.037
Louca, S., Parfrey, L. W., and Doebeli, M. (2016). Decoupling function and taxonomy in the global ocean microbiome. Science 353, 1272–1277. doi: 10.1126/science.aaf4507
Lu, R. K. (2000). Methods of Soil And Agro-Chemical Analysis. Beijing: China Agricultural Science and Technology Press.
Maboko, M. M., and Du Plooy, C. P. (2013). High-density planting of tomato cultivar’s with early decapitation of growing point increased yield in a closed hydroponic system. Acta Agric. Scand. Sect. B Soil Plant Sci. 63, 676–682. doi: 10.1080/09064710.2013.851276
Magoč, T., and Salzberg, S. L. (2011). FLASH: fast length adjustment of short reads to improve genome assemblies. Bioinformatics 27, 2957–2963. doi: 10.1093/bioinformatics/btr507
Molina-Santiago, C., Pearson, J. R., Navarro, Y., Berlanga-Clavero, M. V., Caraballo-Rodriguez, A. M., Petras, D., et al. (2019). The extracellular matrix protects Bacillus subtilis colonies from Pseudomonas invasion and modulates plant co-colonization. Nat. Commun. 1011:1919. doi: 10.1038/s41467-019-09944-x
Mommer, L., Cotton, T. E. A., Raaijmakers, J. M., Termorshuizen, A. J., Ruijven, J., Hendriks, M., et al. (2018). Lost in diversity: the interactions between soil-borne fungi, biodiversity and plant productivity. New Phytol. 218, 542–553. doi: 10.1111/nph.15036
Mougel, C., Offre, P., Ranjard, L., Corberand, T., Gamalero, E., Robin, C., et al. (2006). Dynamic of the genetic structure of bacterial and fungal communities at different developmental stages of Medicago truncatula Gaertn. cv. Jemalong Line J5. New Phytol. 170, 165–175. doi: 10.2307/3694642
Muñoz-Rojas, M., Román, J. R., Roncero-Ramos, B., Erickson, T. E., Merritt, D. J., Aguila-Carricondo, P., et al. (2018). Cyanobacteria inoculation enhances carbon sequestration in soil substrates used in dryland restoration. Sci. Total Environ. 636, 1149–1154. doi: 10.1016/j.scitotenv.2018.04.265
Nardini, A., Õunapuu-Pikas, E., and Savi, T. (2014). When smaller is better: leaf hydraulic conductance and drought vulnerability correlate to leaf size and venation density across four Coffea arabica genotypes. Funct. Plant Biol. 41, 972–982. doi: 10.1071/FP13302
Nelson, D. W., and Sommers, L. E. (1996). “Total carbon, organic carbon, and organic matter,” in Methods of Soil Analysis, Part 3. Chemical methods, ed. D. L. Sparks (Madison: American Society of Agronomy Inc.), 961–1010.
Nguyen, N. H., Song, Z., Bates, S. T., Branco, S., Tedersoo, L., Menke, J., et al. (2016). FUNGuild: an open annotation tool for parsing fungal community datasets by ecological guild. Fungal Ecol. 20, 241–248. doi: 10.1016/j.funeco.2015.06.006
Niu, W. Q., Jia, Z. X., Xuan, Z., and Shao, H. B. (2012). Effects of soil rhizosphere aeration on the root growth and water absorption of tomato. Clean–Soil Air Water 40, 1364–1371. doi: 10.1002/clen.201100417
Pfeiffer, B., Fender, A., Lasota, S., Hertel, D., Jungkunst, H. F., and Daniel, R. (2013). Leaf litter is the main driver for changes in bacterial community structures in the rhizosphere of ash and beech. Appl. Soil Ecol. 72, 150–160. doi: 10.1016/j.apsoil.2013.06.008
Procter, A. C., Ellis, J. C., Fay, P. A., Polley, H. W., and Jackson, R. B. (2014). Fungal community responses to past and future atmospheric CO2 differ by soil type. Appl. Environ. Microb. 80, 7364–7377. doi: 10.1128/AEM.02083-14
Pruesse, E., Quast, C., Knittel, K., Fuchs, B. M., Ludwig, W., Peplies, J., et al. (2007). SILVA: a comprehensive online resource for quality checked and aligned ribosomal RNA sequence data compatible with ARB. Nucleic Acids Res. 35, 7188–7196. doi: 10.1093/nar/gkm864
Puerta, V. L., Oereira, E. I. P., Wittwer, R., Heijden, M., and Six, J. (2018). Improvement of soil structure through organic crop management, conservation tillage and grass-clover ley. Soil Till. Res. 180, 1–9. doi: 10.1016/j.still.2018.02.007
Rashad, Y. M., Abdel-Fattah, G., and Hafez, E. E. (2012). Diversity among some Egyptian isolates of Rhizoctonia solani based on anastomosis grouping, molecular identification and virulence on common bean. Afr. J. Microbiol. Res. 6, 6661–6667. doi: 10.5897/AJMR12.1096
Razanamalala, K., Razafimbelo, T., Maron, P., Ranjard, L., Chemidlin, N., Lelièvre, M., et al. (2018). Soil microbial diversity drives the priming effect along climate gradients: a case study in Madagascar. ISME J. 12, 451–462. doi: 10.1038/ismej.2017.178
Ribeiro, R. V., Machado, E. C., Santos, M. G., and Oliveira, R. F. (2009). Photosynthesis and water relations of well-watered orange plants as affected by winter and summer conditions. Photosynthetica 47, 215–222. doi: 10.1007/s11099-009-0035-2
Rivelli, A. R., De Maria, S., Puschenreiter, M., and Gherbin, P. (2012). Accumulation of cadmium, zinc, and copper by Helianthus Annuus L.: impact on plant growth and uptake of nutritional elements. Int. J. Phytoremediat. 14, 320–334. doi: 10.1080/15226514.2011.620649
Santruckova, H., and Simek, M. (1997). Effect of soil CO2 concentration on microbial biomass. Biol. Fertil. Soils 25, 269–273. doi: 10.1007/s003740050313
Schloss, P. D., Westcott, S. L., Ryabin, T., Hall, J. R., Hartmann, M., Hollister, E. B., et al. (2009). Introducing mothur: open-source, platform-independent, community-supported software for describing and comparing microbial communities. Appl. Environ. Microb. 75, 7537–7541. doi: 10.1128/AEM.01541-09
Smit, E., Leeflang, P., Glandorf, B., van Elsas, J. D., and Wernars, K. (1999). Analysis of fungal diversity in the wheat rhizosphere by sequencing of cloned PCR-amplified genes encoding 18S rRNA and temperature gradient gel electrophoresis. Appl. Environ. Microb. 65, 2614–2621. doi: 10.1128/AEM.65.6.2614-2621.1999
Stefan, L., Hartmann, M., Engbersen, N., Six, J., and Schöb, C. (2021). Positive effects of crop diversity on productivity driven by changes in soil microbial composition. Front. Microbiol. 12:660749. doi: 10.3389/fmicb.2021.660749
Stępniewski, W., and Stępniewska, Z. (2009). Selected oxygen-dependent process—response to soil management and tillage. Soil Till. Res. 102, 193–200. doi: 10.1016/j.still.2008.07.006
van der Heijden, M. G. A., Bardgett, R. D., and van Straalen, N. M. (2008). The unseen majority: soil microbes as drivers of plant diversity and productivity in terrestrial ecosystems. Ecol. Lett. 11, 296–310. doi: 10.1111/j.1461-0248.2007.01139.x
Walker, T. W. N., Kaiser, C., Strasser, F., Herbold, C. W., Leblans, N. I. W., Woebken, D., et al. (2018). Microbial temperature sensitivity and biomass change explain soil carbon loss with warming. Nat. Clim. Chang. 8, 885–889. doi: 10.1038/s41558-018-0259-x
Whitman, T., Pepe-Ranney, C., Enders, A., Koechli, C., Campbell, A., Buckley, D. H., et al. (2016). Dynamics of microbial community composition and soil organic carbon mineralization in soil following addition of pyrogenic and fresh organic matter. ISME J. 10, 2918–2930. doi: 10.1038/ismej.2016.68
Xiao, D., Tan, Y., Liu, X., Yang, R., Zhang, W., He, X., et al. (2019). Effects of different legume species and densities on arbuscular mycorrhizal fungal communities in a karst grassland ecosystem. Sci. Total Environ. 678, 551–558. doi: 10.1016/j.scitotenv.2019.04.293
Xiao, Y., Peng, F., Dang, Z., Jiang, X., Zhang, J., Zhang, Y., et al. (2015). Influence of rhizosphere ventilation on soil nutrient status, root architecture and the growth of young peach trees. Soil Sci. Plant Nutr. 61, 775–787. doi: 10.1080/00380768.2015.1045404
Xu, Z., Ma, Y., Zhang, L., Han, Y., Yuan, J., Li, G., et al. (2021). Relating bacterial dynamics and functions to gaseous emissions during composting of kitchen and garden wastes. Sci. Total Environ. 767:144210. doi: 10.1016/j.scitotenv.2020.144210
Zamioudis, C., and Pieterse, C. M. J. (2012). Modulation of host immunity by beneficial microbes. Mol. Plant Microb. Int. 25, 139–150. doi: 10.1094/mpmi-06-11-0179
Zhang, J., Zhang, N., Liu, Y., Zhang, X., Hu, B., Qin, Y., et al. (2018). Root microbiota shift in rice correlates with resident time in the field and developmental stage. Sci. China Life Sci. 61, 613–621. doi: 10.1007/s11427-018-9284-4
Zhang, K., Shi, Y., Jing, X., He, J., Sun, R., Yang, Y., et al. (2016). Effects of short-term warming and altered precipitation on soil microbial communities in alpine grassland of the Tibetan Plateau. Front. Microbiol. 7:1032. doi: 10.3389/fmicb.2016.01032
Zhao, F., Sun, J., Yu, S., Liu, H., and Yu, K. (2019). Aeration irrigation can improve growth of table grape cv. Red Globe (Vitis vinifera L.) in greenhouse. Hortscience 54, 732–737. doi: 10.21273/HORTSCI13732-18
Zhao, F. Z., Ren, C. J., Zhang, L., Han, X. H., Yang, G. H., and Wang, J. (2018). Changes in soil microbial community are linked to soil carbon fractions after afforestation. Eur. J. Soil Sci. 69, 370–379. doi: 10.1111/ejss.12525
Keywords: microbial communities, strawberry growth, soil O2 and CO2, planting density, nutrient cycling, multifunctional index
Citation: Zhang Y, Hu Y, You Z, Li Z, Kong M, Han M, Liu Z, Zhang J and Yao Y (2021) Soil Ventilation Benefited Strawberry Growth via Microbial Communities and Nutrient Cycling Under High-Density Planting. Front. Microbiol. 12:666982. doi: 10.3389/fmicb.2021.666982
Received: 11 February 2021; Accepted: 22 September 2021;
Published: 18 October 2021.
Edited by:
Yong Wang, Guizhou University, ChinaReviewed by:
Zhiqin Zhou, Southwest University, ChinaYongzhong Feng, Northwest A&F University, China
Copyright © 2021 Zhang, Hu, You, Li, Kong, Han, Liu, Zhang and Yao. This is an open-access article distributed under the terms of the Creative Commons Attribution License (CC BY). The use, distribution or reproduction in other forums is permitted, provided the original author(s) and the copyright owner(s) are credited and that the original publication in this journal is cited, in accordance with accepted academic practice. No use, distribution or reproduction is permitted which does not comply with these terms.
*Correspondence: Yuncong Yao, eWFveWNfMjBAMTI2LmNvbQ==; Jie Zhang, YW55emowMzRAMTI2LmNvbQ==
†These authors have contributed equally to this work