- 1State Key Laboratory for Managing Biotic and Chemical Threats to the Quality and Safety of Agro-products, Key Laboratory of Biotechnology in Plant Protection of Ministry of Agriculture and Zhejiang Province, Institute of Plant Virology, Ningbo University, Ningbo, China
- 2Yantai Academy of Agricultural Science, Yantai, China
Protein lysine acetylation (Kac) is an important post-translational modification mechanism in eukaryotes that is involved in cellular regulation. To investigate the role of Kac in virus-infected plants, we characterized the lysine acetylome of Nicotiana benthamiana plants with or without a Chinese wheat mosaic virus (CWMV) infection. We identified 4,803 acetylated lysine sites on 1,964 proteins. A comparison of the acetylation levels of the CWMV-infected group with those of the uninfected group revealed that 747 sites were upregulated on 422 proteins, including chloroplast localization proteins and histone H3, and 150 sites were downregulated on 102 proteins. Nineteen conserved motifs were extracted and 51 percent of the acetylated proteins located on chloroplast. Nineteen Kac sites were located on histone proteins, including 10 Kac sites on histone 3. Bioinformatics analysis results indicated that lysine acetylation occurs on a large number of proteins involved in biological processes, especially photosynthesis. Furthermore, we found that the acetylation level of chloroplast proteins, histone 3 and some metabolic pathway-related proteins were significantly higher in CWMV-infected plants than in uninfected plants. In summary, our results reveal the regulatory roles of Kac in response to CWMV infection.
Introduction
Protein post-translational modifications (PTMs) are dynamic and reversible protein processing events that occur in prokaryotic and eukaryotic cells (Rao et al., 2014). Previous studies shown that PTMs involved in plenty of biological processes, including gene expression, enzymatic activity, protein stability and cell signaling (Khoury et al., 2011). To date, more than 450 distinct PTMs have been identified, including acetylation, succinylation, malonylation, butyrylation, and propionylation. Lysine residues acetylation, is an important regulatory mechanisms that broaden and fine-tune protein functions (Xing and Poirier, 2012). Furthermore, protein acetylation plays an important role in cellular biological activities, such as cell growth, apoptosis, cytokinetics, cell metabolism (Choudhary et al., 2009; Wang et al., 2010), enzymatic activity (Starai and Escalante-Semerena, 2004; Nambi et al., 2013), protein interactions (Choudhary et al., 2009), protein stability (Liang et al., 2011), and metabolic pathways. The balance of lysine acetylation depends on the regulation of specific lysine acetyl-acetylases and deacetylases (Choudhary et al., 2009; Xing and Poirier, 2012; Konig et al., 2014; Hartl et al., 2017).
Protein lysine acetylation (Kac) of histones was first confirmed to control gene expression and regulate epigenetic in 1963 (Phillips, 1963). Early studies of protein acetylation in Arabidopsis and rice (Oryza sativa) reported that multi-lysine residues are acetylated in histone H3, including K4, K9, K14, K18, K23, K27, and K36. Lysine residues in histone H4, such as K5, K8, K12, K16, and K20 are acetylated (Benhamed et al., 2006; Fuchs et al., 2006; Hollender and Liu, 2008; Servet et al., 2010; Tan et al., 2011; Xue et al., 2018). Recently, with the development of proteomic techniques more and more modifications have been discovered, including non-histone acetylation. For example, a lot of Kac proteins in rice was found, including 1,669 Kac sites on 1,024 proteins in vegetative cells and reproductive organs (Li et al., 2018) and 1,337 Kac sites on 716 proteins in whole seedlings (Xiong et al., 2016). In addition, 2,057 Kac sites on 1,022 proteins have been identified in Arabidopsis (Hartl et al., 2017), including many respiratory chain proteins and tricarboxylic acid cycle enzymes. In addition to acetylated proteins mentioned above, many have other functions, such as photorespiration, regulating redox and metabolism (Konig et al., 2014). Kac proteins have also been identified in strawberry (Fragaria × ananassa) (Fang et al., 2015), grape (Vitis vinifera) (Melo-Braga et al., 2012), and wheat (Triticum aestivum) (Zhang et al., 2016; Table 1). However, compared with rice and Arabidopsis, acetylomes of Nicotiana benthamiana and of chinese wheat mosaic virus (CWMV)-infected N. benthamiana plants have been poorly studied. Although there are several studies about lysine acetylation in plant, the impact and regulation on pathogen interactions, particularly virus infection, has not been investigated in plants to date (Hartl et al., 2017).
CWMV belong to family Virgaviridae, the genus Furovirus (Adams et al., 2009), and causes a damaging disease on wheat with light chlorotic streaking of young leaves. In old leaves, CWMV infection cause bright-yellow chlorotic streaking or even purple chlorotic stripes are observed. Severely infected plants become stunted, wilt, and eventually die (Chen, 1993; Diao et al., 1999). CWMV Has a bipartite positive-sense single-stranded RNA genome and its particle is rigid rod-shaped (Diao et al., 1999). There are three proteins encoded by RNA1 (7,147 nt), including a 153-kDa replicase protein, a 212-kDa protein contain a RNA-dependent RNA polymerase domain, and cell-to-cell movement protein. There are four proteins encoded by RNA2 (3,564 nt), including a major coat protein, two minor CP-related proteins, and a 19 kDa cysteine-rich RNA silencing suppressor (Diao et al., 1999; Andika et al., 2013). Recently, the CWMV infectious cDNA clones have been developed (Yang et al., 2016), making investigations of CWMV and the CWMV-host interaction possible. However, to date, only a few host factors have been identified to interact with CWMV encoding proteins and involved in the CWMV infection, and no PTM of the host protein related to the CWMV infection response has been identified.
In this study, we explored lysine acetylation in CWMV-infected N. benthamiana leaves. We used 2D-LC-MS/MS and advanced bioinformatic analyses to enrichment Kac peptides, and to determine the Kac landscape in N. benthamiana. In total, our analysis yielded 4,803 acetylated lysine sites on 1,964 proteins. Further proteomic analysis revealed that the acetylated proteins involved in varied biological processes, cellular functions and subcellular localization. Among these, most upregulated Kac proteins are involved in photosynthetic processes whereas the downregulated Kac proteins participate in metabolic processes. This study have broaden the understanding of acetylomes in N. benthamiana and reveals a lot of acetylation sites involved in specific defense processes against virus infection.
Experimental Procedures
CWMV Inoculation and Plant Growth
For the agroinfiltration of Nicotiana benthamiana leaves, we use electroporation method to transform the plasmids into Agrobacterium tumefaciens strain (GV3101). Individual bacterial cultures with plasmid were grown overnight, collected, and resuspended in the induction buffer (100 mM acetosyringone, 1 M MgCl2, 10 mM MES, pH 5.6). The bacterial cultures were incubation for 3 h at room temperature, and then infiltrated individually into two leaves of four-leaf-stage N. benthamiana using needleless syringes. Three plants were infiltrated with the bacterial culture. Three plants inoculated with induction buffer only were used as control plants. After inoculation, N. benthamiana plants were grown at 15∘C and under 16/8 h light/dark conditions for 14 days.
Nicotiana benthamiana Protein Extraction
Sample proteins were extracted from 1 g leaves. Firstly, we ground samples to form powder in liquid nitrogen and then add lysis buffer (1% protease inhibitor cocktail, 8 M urea, 1% Triton-100, 10 mM dithiothreitol, 3 μM trichostatin A and 50 mM nicotinamide for acetylation) to the powdered sample, which was then placed on ice and sonicated using ultrasonic crusher (Scientz, Ningbo, China) for three times. Secondly, the suspension was centrifuged at 20,000 g for 10 min at 4∘C to remove the remaining debris. Thirdly, the protein was precipitated by incubating the lysate with cold 20% trichloroacetic acid for 2 h at –20∘C. The precipitate was collected after 12,000 g centrifugation for 10 min at 4∘C and then washed with cold acetone for three times. Finally, the protein was dissolved in 8 M urea and the protein concentration was determined using a BCA kit according to the manufacturer’s instructions.
Database Search
The resulting 2D-LC-MS/MS data were processed using the MaxQuant search engine (v. 1.5.2.8). Tandem mass spectra were searched against the Nicotiana tabacum database1 concatenated with a reverse decoy database. We set the mass tolerance as 20 ppm in “First search,” the “Main search” set as 5 ppm, and set fragment ions as 0.02 Da. The false discovery rate was adjusted to lower than 1% and the minimum score for modified peptides was set as > 40.
Bioinformatics Analysis
For bioinformatics analysis, we use the UniProt-GOA database2 to unify the gene and gene product attributes and to assimilate and disseminate annotation data of acetylation-modified peptides and proteins. Next, we use Gene Ontology (GO) annotation to classify identified proteins into three categories: molecular function, biological process and cellular component. The corrected p < 0.05 was considered to be significant. The online KEGG database3 was used for pathway and protein domain analyses of the identified N. benthamiana proteins. The corrected p < 0.05 was considered to be significant in pathway. These pathways were classified into hierarchical categories according to the KEGG website.
InterProScan software was used to annotate the functional description of the identified protein domains based on the InterPro4 domain database and the protein sequence alignment method. The InterPro database and two-tailed Fisher’s exact test was used for each protein category. The corrected p < 0.05 of protein domains was considered significant. The subcellular localization of all peptides and identified proteins was predicted in Wolfpsort software.
The Kac amino acids positions motif of modifier-21-mers in all protein sequences was analyzed and displayed using motif-x software. All the database protein sequences were used as background database parameters; default values were used for other parameters.
Protein–Protein Interaction Network
The protein-protein interaction analysis is based on STRING database (version 11.0) and only select the searched proteins that present interactions in the dataset. Set a confidence score > 0.7 between all interactions to define the interaction confidence. The densely connected regions of searched proteins was analyzed by a graph theoretical clustering algorithm and plug-in tool kit part molecular complex detection (MCODE).
Protein Expression
To investigate the acetylation of proteins A0A1S4CHI1, A0A1S4BE66, and A0A1S4CLZ9, these proteins were fused with a green fluorescent protein (GFP) tag at the C-terminus. The proteins were expressed in CWMV-infected and uninfected N. benthamiana leaves. Forty eight hours after inoculation, green fluorescence was observed under a confocal fluorescence microscope (Nikon, Tokyo, Japan; A1 + A1R) before extracting proteins from leaves.
Western Blot Analysis
Total proteins were extracted from 0.1 g fresh leaf tissues by ground them in liquid nitrogen and then add protein extraction buffer (1% Triton-100, 10 mM dithiothreitol and 1% protease inhibitor cocktail). The mixture liquid was centrifuged at 18,000 g for 10 min at 4∘C. Next, collect 160 μl supernatant of each sample and add 40μl SDS-PAGE buffer, then boiled in at 100∘C for 10 min. The supernatant was loaded onto a 4–20% SDS-PAGE gel, followed by western blot analysis using Histone H3ac (pan-acetyl) antibody (rabbit, Active Motif, Carlsbad, CA, United States), anti-acetyl-lysine antibody (mouse, PTM Biolabs, Hangzhou, China), histone H3K9ac (pan-acetyl) antibody (rabbit, Active Motif), histone H3K18ac (pan-acetyl) antibody (rabbit, Active Motif), histone H3K27ac (pan-acetyl) antibody (rabbit, Active Motif), histone H3K79ac (pan-acetyl) antibody (rabbit, Active Motif).
Results
Proteome-Wide Analysis of Lysine Acetylation Peptides and Proteins in CWMV-Infected N. benthamiana
Nicotiana benthamiana leaves infected with CWMV show some physiological changes (Figure 1A and Supplementary Figure 1). To determine the overall number of acetylated proteins and to compare protein acetylation levels in CWMV-infected N. benthamiana leaves 14 days post infection with those of uninfected leaves, a western blot assay was performed using protein extracts from N. benthamiana leaves with or without CWMV infection. Multiple lysine-acetylated protein bands were detected using anti-acetyl-lysine antibodies; and then, the CWMV-infected leaves showed stronger reactions to the anti-acetyl-lysine than the uninfected control leaves (Figure 1B). To analyze the lysine acetylation peptides, we performed LC-MS/MS analysis (Figure 1C). LC-MS/MS spectra of acetylated peptides (upregulation of chloroplastic protein 29 kDa ribonucleoprotein B) are shown as an example (Figure 1D). To confirm the validity of the LC-MS/MS data, we checked the quality errors of acetyl-peptides and sample repeatability. The distribution of quality errors and the relative standard deviation coefficient (RSD) were close to zero (Figures 2A,B), which confirmed that the LC-MS/MS data were highly accurate. The length of most acetylation peptides ranged from 7 to 25 (Figure 2C). In total, our analysis yielded 4,803 acetylated lysine sites on 1,964 N. benthamiana proteins. A comparison of the acetylation levels of the CWMV-infected group with those of the uninfected group revealed that 747 sites were upregulated on 422 proteins and 150 sites were downregulated on 102 proteins when using a change threshold of at least 1.2 times and a t-test p < 0.05 (Figure 2D). Among all the acetylation level changed proteins, 19 Kac sites were combined in core histones, including 10 Kac sites on histone H3, and 278 Kac sites were combined in chloroplast proteins (Supplementary Table 1).
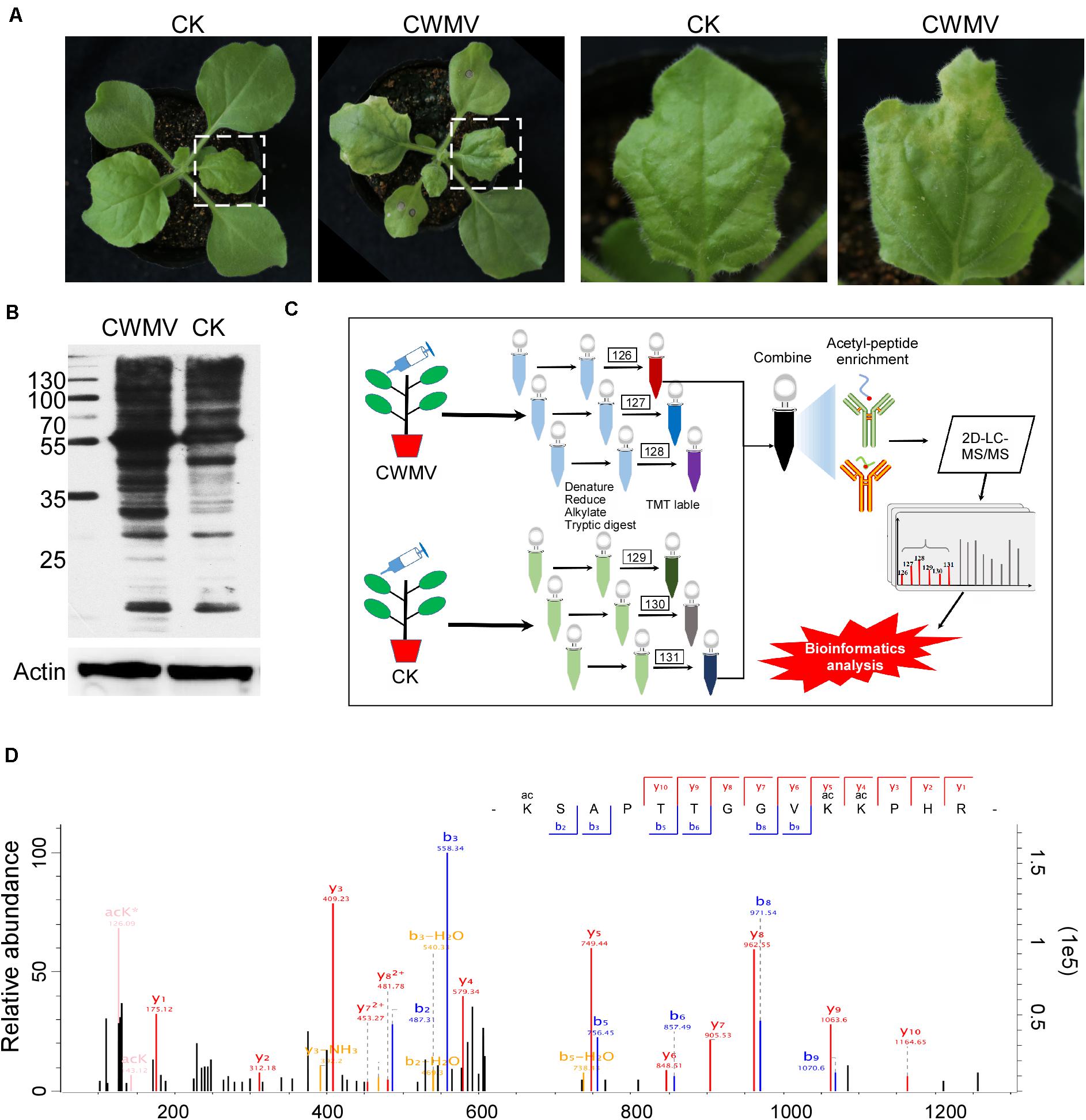
Figure 1. Proteome-wide analysis of lysine acetylation peptides and proteins. (A) Photograph of Nicotiana benthamiana 14 days post-inoculation with CWMV. (B) Detection of Lys-acetylated proteins in CWMV-infected N. benthamiana leaf samples and uninfected leaf samples by western blot assays using anti-acetyl-Lys antibodies. (C) The workflow used for the Kac proteomic experiments. (D) LC-MS/MS spectra of acetyl-peptides from a upregulated chloroplastic protein named 29 kDa ribonucleoprotein B; acetyl-peptide VEVIYDK(ac)LTGR with one acetylation site at K122.
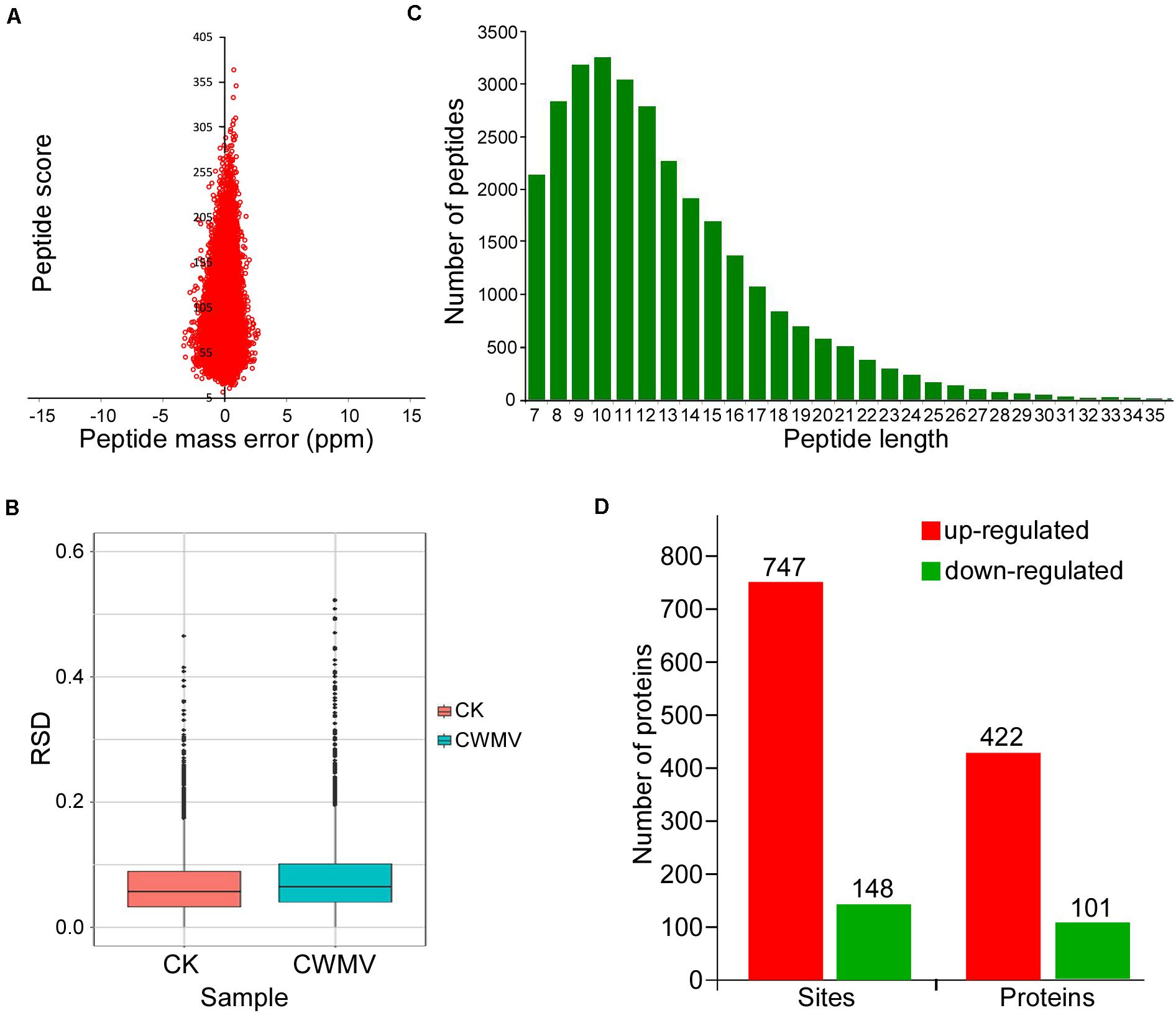
Figure 2. Distribution and motif analysis of lysine acetylation sites. (A) Mass error distribution of all identified peptides. (B) Boxplot showing the sequence coverage of proteins that were quantified under each extraction condition. RSD, relative standard deviation coefficient. (C) Distribution of peptide length. (D) Number of lysine acetylation sites on peptides and proteins identified in CWMV-infected N. benthamiana leaf samples and uninfected leaf samples.
Distribution Characterization and Motif Analysis of Acetylation Sites
To evaluate the number of acetylation sites per protein in CWMV-infected N. benthamiana leaves, we calculated the acetylation sites on proteins. The results indicated that 52.7% (1,035/1,964) contained a single acetylation site, 18.3% (359/1,964) contained two acetylation sites, 10.1% (198/1,964) contained three acetylation sites, and 18.9% (372/1,964) contained more than three acetylation sites (Figure 3A). Furthermore, 53 proteins contained at least 10 acetylation sites, including Q76N23, histone H3, which contained 10 acetylation sites, A0A1S4CB32, a chloroplastic heat shock-related protein, which contained 22 acetylation sites, and A0A1S3Y0T4, an elongation factor 2, which contained 27 acetylation sites (Supplementary Table 2).
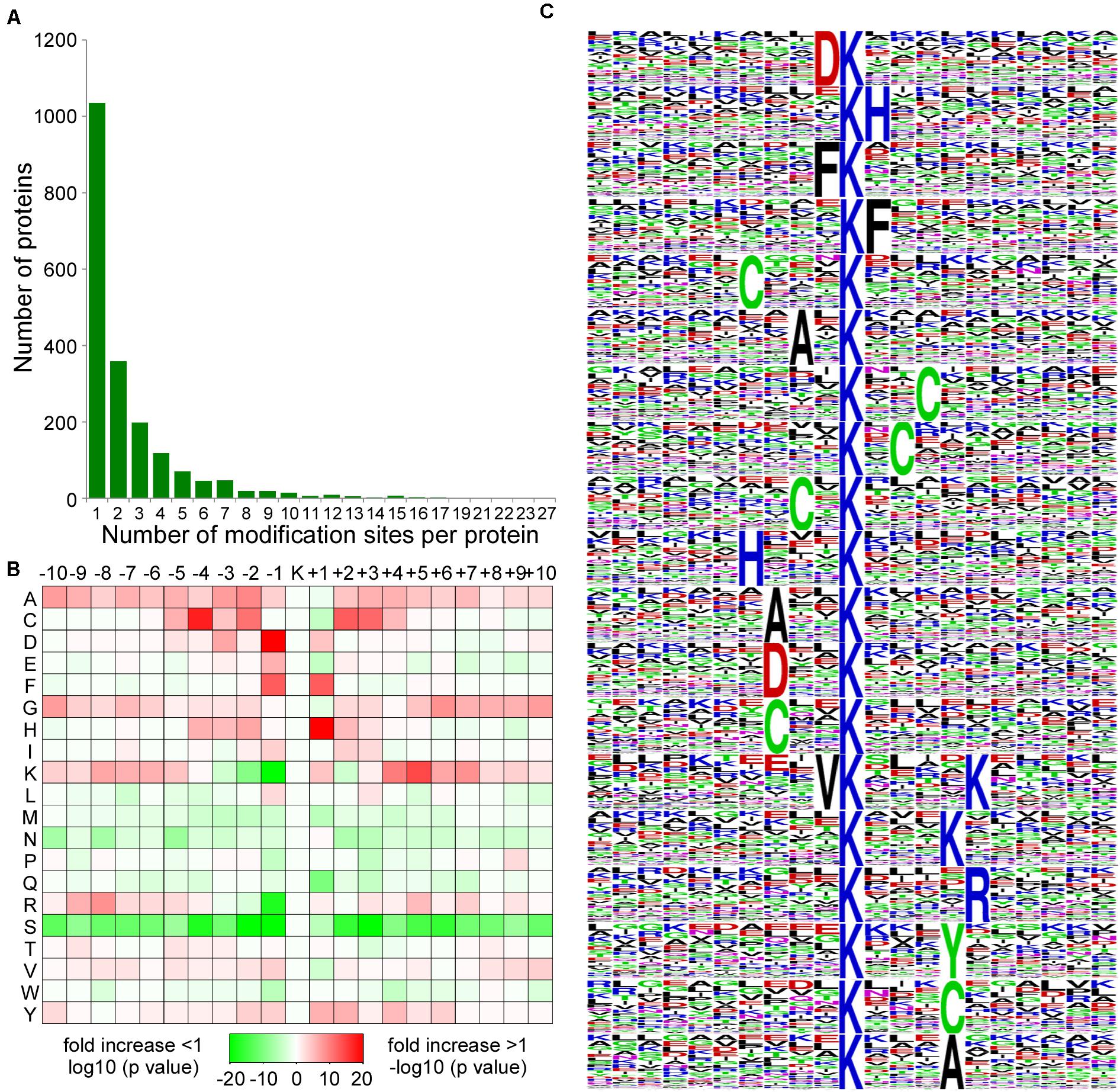
Figure 3. Distribution and enrichment analysis of lysine acetylation sites. (A) The number of acetylation sites on acetylated proteins. (B) Enrichments of amino acids around the acetylation sites. The intensity map shows the relative abundance for 10 amino acids from the lysine-acetylated site. The colors in the intensity map represent the log10 of the ratio of frequencies within acetyl-21-mers vs. non-acetylated (red shows enrichment, green shows depletion). (C) Sequence probability logos of significantly enriched acetylation site motifs for ± 10 amino acids around the lysine acetylation sites. The size of each letter corresponds to the frequency of that amino acid residue in that position.
To further evaluate the characteristics and particular domain of acetylation sites, we analyzed the motif of sequences comprising lysine residues and amino acids in specific positions by using Motif-x program. A position-specific intensity map was generated to assess the significantly enriched amino acids around the acetylation sites. The concentration of motif with amino acid frequencies showed that the + 1, + 2, or + 3 positions have a preference for H, F, or C residues and that the –1, –2, or –3 positions have a preference for A, C, D, F, or V residues. However, serine residues were significantly lacking from all 20 positions around acetylation sites (Figure 3B). A total of 19 conserved motifs in D∗Kac, Kac∗H, H∗Kac, F∗Kac, Kac∗F, C∗Kac, Kac∗C, A∗Kac, Kac∗A, V∗Kac∗K, Kac∗R, Kac∗K, and Kac∗Y were summarized from 3,360 acetylated peptides. Among these motifs, D∗Kac represented 379 (11.3%) of the enrichment motifs to be the most common combination (Figure 3C). These newly identified amino acid residues surrounding acetylation sites in N. benthamiana could potentially provide acetylation binding sites for future studies.
Functional Distribution and Subcellular Localization of Acetylation Proteins in CWMV-Infected N. benthamiana
We detected 524 novel acetylated proteins in CWMV-infected N. benthamiana. To better understand the potential functions of these proteins, we further assessed the GO analysis using DAVID (Huang da et al., 2009). Proteins were categorized based on their biological process, cellular component, and molecular function. We found that 37% of acetylated proteins were involved in metabolic process, 30% in cellular process, and 22% in single-organism process according to biological process category (Figure 4A). There are 38% of acetylated proteins were located in the cell, 24% in a macromolecular complex, 24% in organelles, and 13% in the membrane according to the cellular component category (Figure 4B). In the molecular function category, most acetylated proteins were either associated with catalytic activity (46%) or binding (39%) (Figure 4C). These results indicated that the enzyme metabolism related proteins might be a high probability of acetylation in CWMV-infected N. benthamiana. For the subcellular localization, we found that most acetylation proteins were located in the chloroplast (51%) in CWMV-infected N. benthamiana (Figure 4D). In addition, some of the remaining acetylation proteins were mainly located in the cytoplasm (24%), nucleus (8%), and mitochondria (5%), respectively.
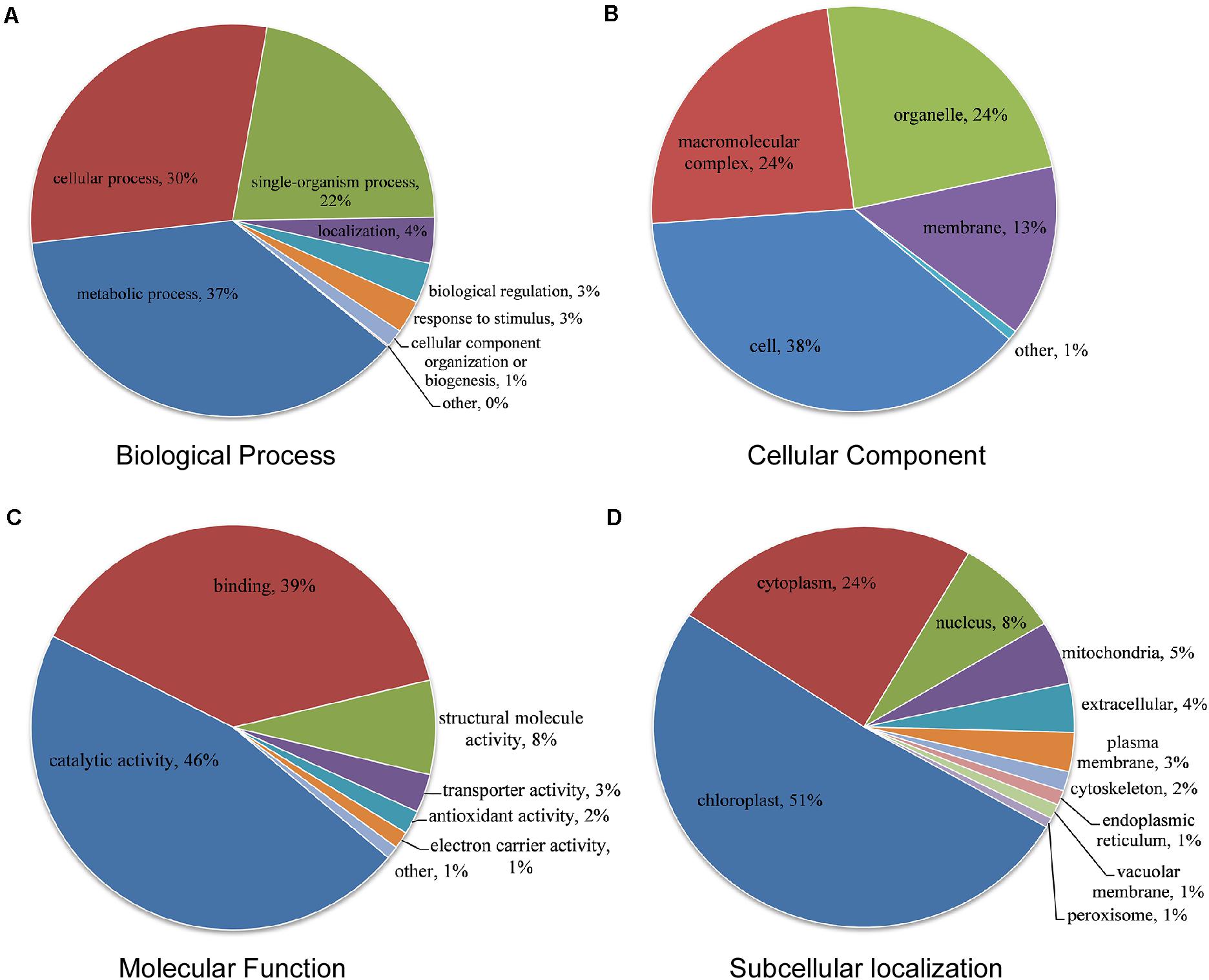
Figure 4. Functional distribution and subcellular localization of lysine acetylated proteins in CWMV-infected N. benthamiana. (A) Lysine acetylated proteins classification according to their biological process. (B) Lysine acetylated proteins classification based on their cellular component. (C) Lysine acetylated proteins classification according to their molecular function. (D) Subcellular localization of lysine acetylated proteins.
Enrichment Analysis and Domain Enrichment of Lysine-Acetylated Proteins in CWMV-Infected N. benthamiana Plants
To investigate the general functions of acetylation proteins in the N. benthamiana response to CWMV infection, acetylated proteins detected in CWMV-infected versus uninfected (CK) plants were mapped to KEGG metabolic pathways. KEGG metabolic pathway analysis showed that most acetylated proteins participated in the regulation of photosynthesis, carbon fixation in photosynthetic organisms, nitrogen metabolism, porphyrin and chlorophyll metabolism, and glyoxylate and dicarboxylate metabolism (Figure 5A). The acetylation proteins participated in the regulation of photosynthesis, carbon fixation in photosynthetic organisms, glyoxylate and dicarboxylate metabolism, and nitrogen metabolism were upregulated (Figure 5B). Downregulated acetylated proteins participated in the regulation of terpenoid backbone biosynthesis, fatty acid degradation, and protein processing in the endoplasmic reticulum (Figure 5C).
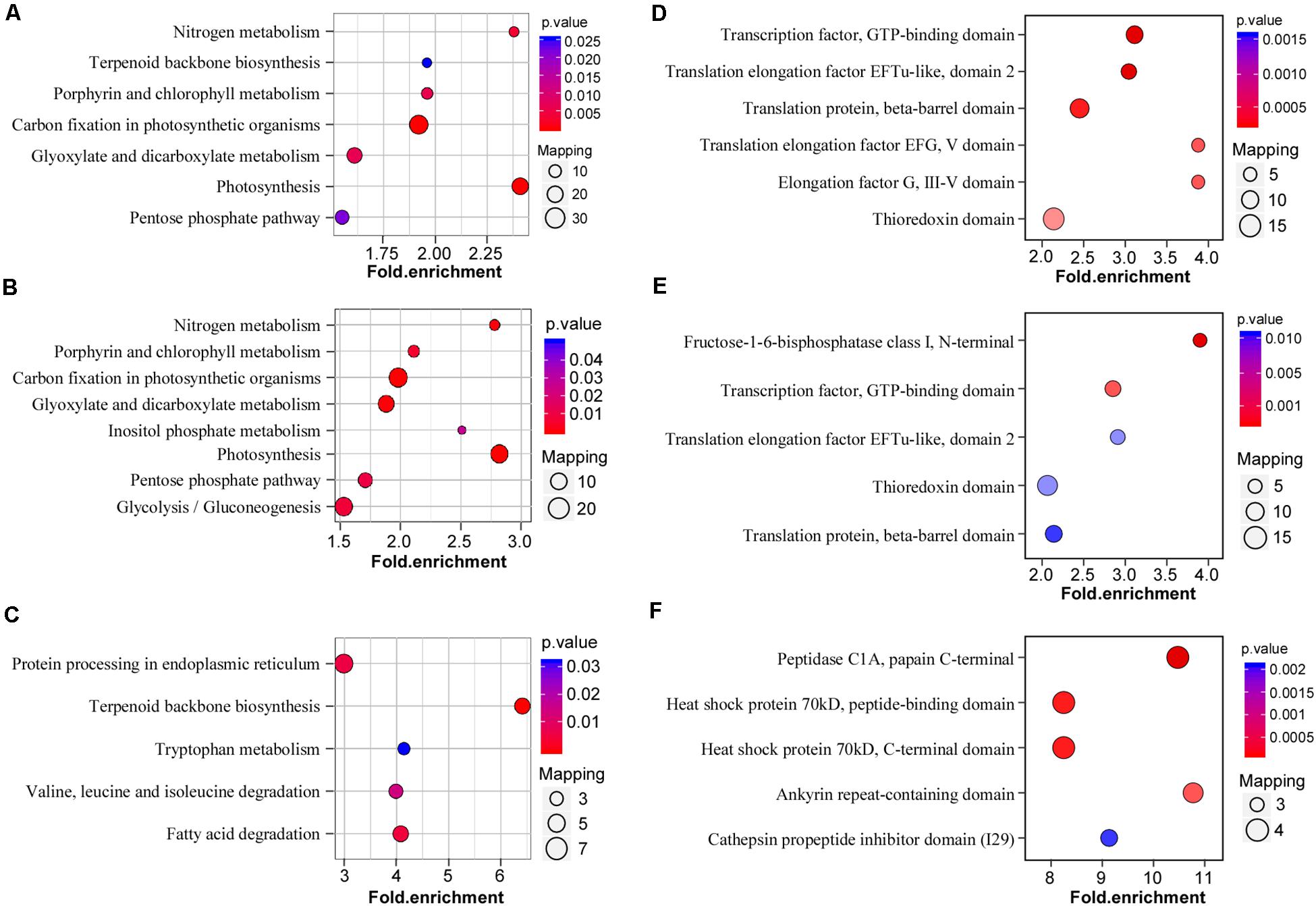
Figure 5. Enrichment analysis and domain enrichment of lysine-acetylated proteins in CWMV-infected N. benthamiana plants. (A) KEGG pathway-based enrichment analysis of proteins in CWMV-infected versus uninfected (CK) plants (–log10 p > 1.5). (B) KEGG pathway enrichment analysis of upregulated proteins in CWMV-infected versus CK plants (–log10 p > 1.5). (C) KEGG pathway-based enrichment analysis of downregulated proteins in CWMV-infected versus CK plants (–log10 p > 1.5). (D) Protein domain enrichment analysis of CWMV-infected versus CK plants. (E) Protein domain enrichment analysis of upregulated proteins in CWMV-infected versus CK plants. (F) Protein domain enrichment analysis of downregulated proteins in CWMV-infected versus CK plants.
To verify the lysine acetylation preferred targets, protein domains were analyzed using the InterPro domain database. The protein domain enrichment results revealed that 21 domains were enriched (Supplementary Table 3), mainly domains that included an elongation factor or translation elongation factor, and metabolic protein domains, such as the transcription factor GTP-binding domain, translation elongation factor EFTu-like domain 2, and translation protein beta-barrel domain (Figure 5D). Among these, approximately 20% of proteins were identified as chloroplast-related protein domains (Supplementary Table 4), suggesting that Kac regulate chloroplastic functions in response to CWMV infection. Meanwhile, fructose-1-6-bisphosphatase class I domain, transcription factor, GTP-binding domain and translation elongation factor EFTu-like domain 2 were enriched in upregulated lysine acetylated proteins (Figure 5E). Furthermore, the peptidase C1A papain C-terminal domain, heat shock protein peptide-binding domain, and heat shock protein 70 kDa, C-terminal domain were enriched in downregulated lysine acetylated proteins (Figure 5F).
Analysis of the Interaction Network of Acetylated Proteins
To further clarify whether the acetylated proteins are associated with the protein component and the crosslink between we used the STRING database5 to construct protein–protein interaction (PPI) networks for acetylated proteins. According to the STRING construct results, we used MCODE tool to obtain entire interaction network and extracted three highly interactive clusters from them. A comparison of CWMV-infected leaves with uninfected leaves revealed 200 acetylated proteins involved in interactions that mapped to the protein interaction database (Figure 6A and Supplementary Table 5). Of these, 178 were upregulated, including HSP70, psbO, PSBP2, RPL16 and RPL2, and 22 were downregulated. The top group consisted of acetylated ribosome proteins (Figure 6B). Furthermore, a comparison of CWMV-infected leaves with uninfected leaves revealed 34 acetylated photosynthesis-related proteins in interactions that mapped to the protein interaction database. These photosynthesis-related proteins could be classified into several groups, such as photosystem I related proteins, chlorophyll a/b binding proteins, photosystem II related proteins and ATP synthase proteins (Figure 6C and Supplementary Table 6).
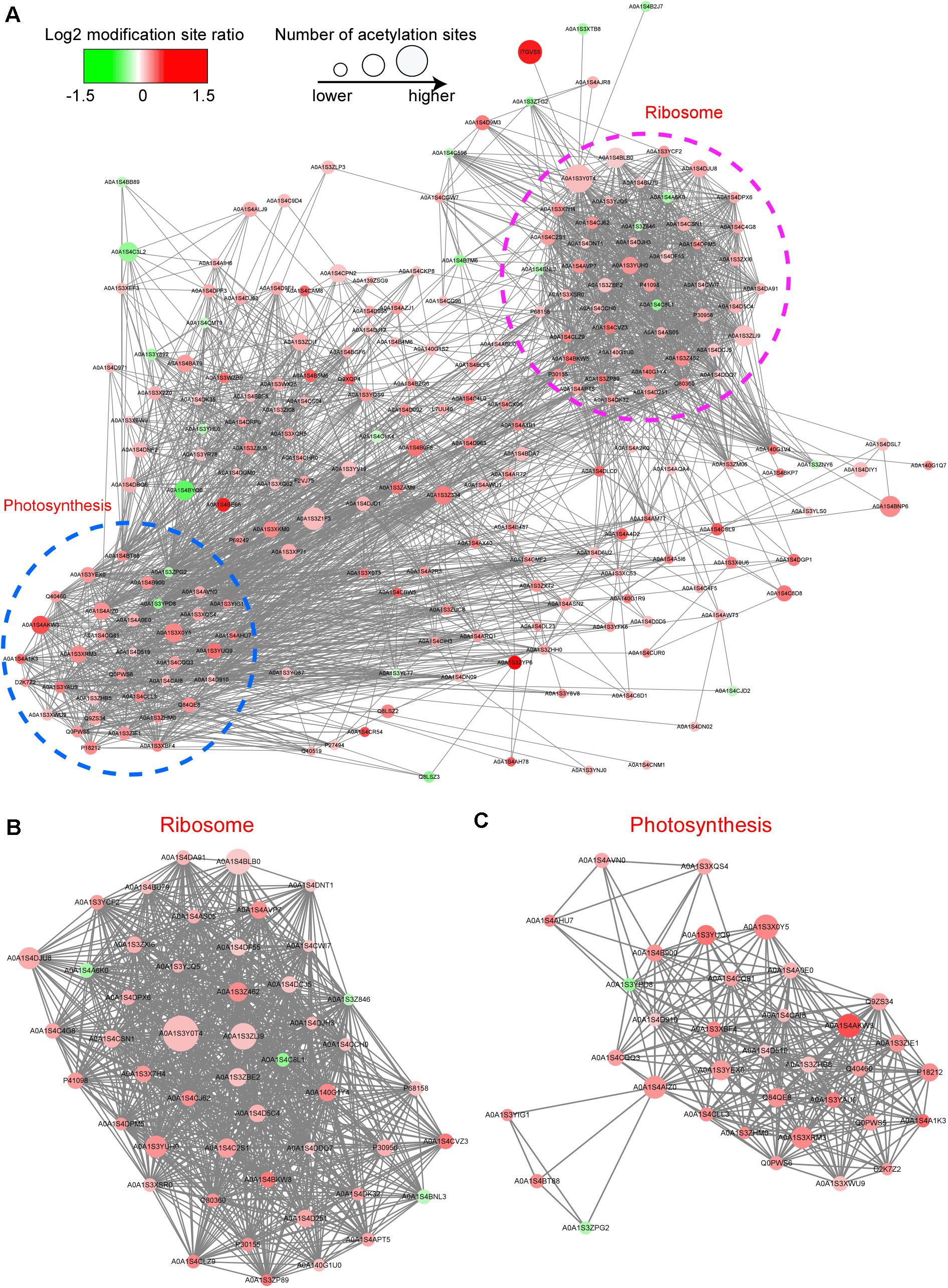
Figure 6. Interaction networks of differentially expressed acetylated proteins in CWMV-infected plants compared with expression in uninfected N. benthamiana plants. (A) The entire protein–protein interaction network of acetylated proteins of CWMV-infected versus uninfected CK plants. (B) Interaction network of acetylated proteins associated with the ribosome of CWMV-infected versus uninfected CK plants. (C) Interaction network of acetylated proteins involved in the photosynthesis of CWMV-infected versus uninfected CK plants.
Acetylated Proteins Involved in Photosynthesis
Given that a large number of acetylated proteins associated with photosynthesis pathway were found by KEGG pathway enrichment analysis. Further analysis showed that photosystem II (PSII) protein complex members, including psbB, psbD, psbO, psbP, psbQ, psbR, and psbS, were identified as acetylated proteins in N. benthamiana and were upregulated in CWMV-infected plants. In addition, photosystem I (PSI) subunits, including PsaA, PsaB, PsaC, PsaD, PsaN, and PsaH, were also found to be acetylated. Among these, only one site on the PsaB subunit was downregulated, whereas the other sites were upregulated in CWMV-infected plants. Similarly, the acetylation level of some chloroplast ATP synthases, such as alpha, beta, gamma, and ATPF0B, were found to be upregulated in CWMV-infected plants (Figure 7 and Supplementary Table 7). These results indicate that CWMV infection induces acetylation of proteins associated with photosynthesis.
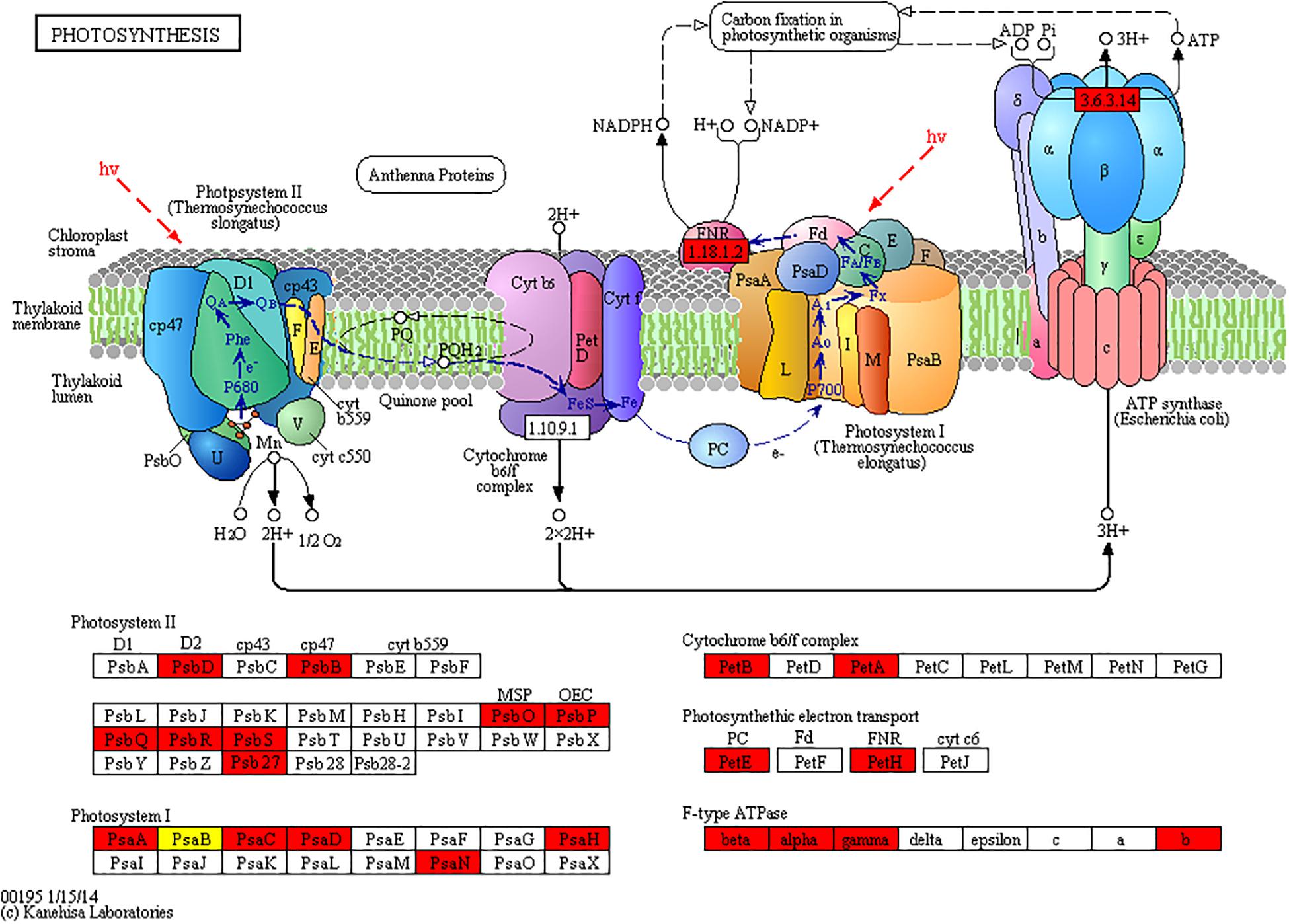
Figure 7. KEGG pathways of significantly enriched photosynthesis. The red highlights mean the acetylated proteins that were significantly upregulated in the photosynthesis pathway of CWMV-infected N. benthamiana plants compared with those of uninfected N. benthamiana (CK) plants. The yellow highlight means that acetylated protein with one lysine site downregulated and one sites upregulated in CWMV-infected plants.
Histone 3 and Chloroplastic Protein Acetylation Levels Were Upregulated in N. benthamiana After CWMV Infection
To confirm that the acetylation level of histone 3 was upregulated in CWMV-infected N. benthamiana leaves compared with levels in uninfected leaves, a western blot assay was performed using protein extracts from N. benthamiana leaves with or without CWMV infection. Although an acetylated histone 3 protein band was detected for both CWMV-infected and uninfected N. benthamiana leaves, CWMV-infected leaves showed stronger reactions to anti-histone H3ac than uninfected control leaves. To confirm the candidate acetylation sites on H3, western blot assays were performed using anti-H3K9ac, anti-H3K14ac, anti-H3K18ac, and anti-H3K79ac antibodies. The analysis revealed that levels of H3K9ac and H3K79ac in CWMV-infected N. benthamiana leaves were obviously higher than those in uninfected N. benthamiana leaves. However, the levels of H3K14ac and H3K18ac were similar in CWMV-infected and uninfected N. benthamiana leaves (Figure 8A). In addition, we measured the acetylation level of chloroplast proteins by performing a western blot assay using chloroplast proteins extracted from N. benthamiana leaves with or without CWMV infection. Although multiple lysine-acetylated chloroplast protein bands were detected, CWMV-infected leaves showed stronger reactions to the anti-acetyl-lysine than uninfected control leaves (Figure 8B). Furthermore, we selected three upregulated lysine-acetylated proteins (A0A1S4CHI1, A0A1S4BE66, and A0A1S4CLZ9) at random from proteins identified as significantly upregulated when using a change threshold of at least 1.2 times and a t-test p < 0.05 (Supplementary Table 1). We measured the acetylation level of these proteins by performing a western blot assay using purified transient expression proteins extracted from N. benthamiana leaves with or without CWMV infection. A specific lysine-acetylated protein band was detected for all leaves. Although levels of A0A1S4CHI1 and A0A1S4CLZ9 lysine-acetylation were obviously higher in CWMV-infected N. benthamiana leaves than in uninfected N. benthamiana leaves, levels of lysine-acetylation in A0A1S4BE66 in CWMV-infected N. benthamiana leaves were only slightly higher than those in uninfected N. benthamiana leaves (Figure 8C). These results were confirmed by LC-MS/MS analyses (Supplementary Table 8). Our findings indicate that CWMV infection induced the acetylation of quite a few proteins in the host plant.
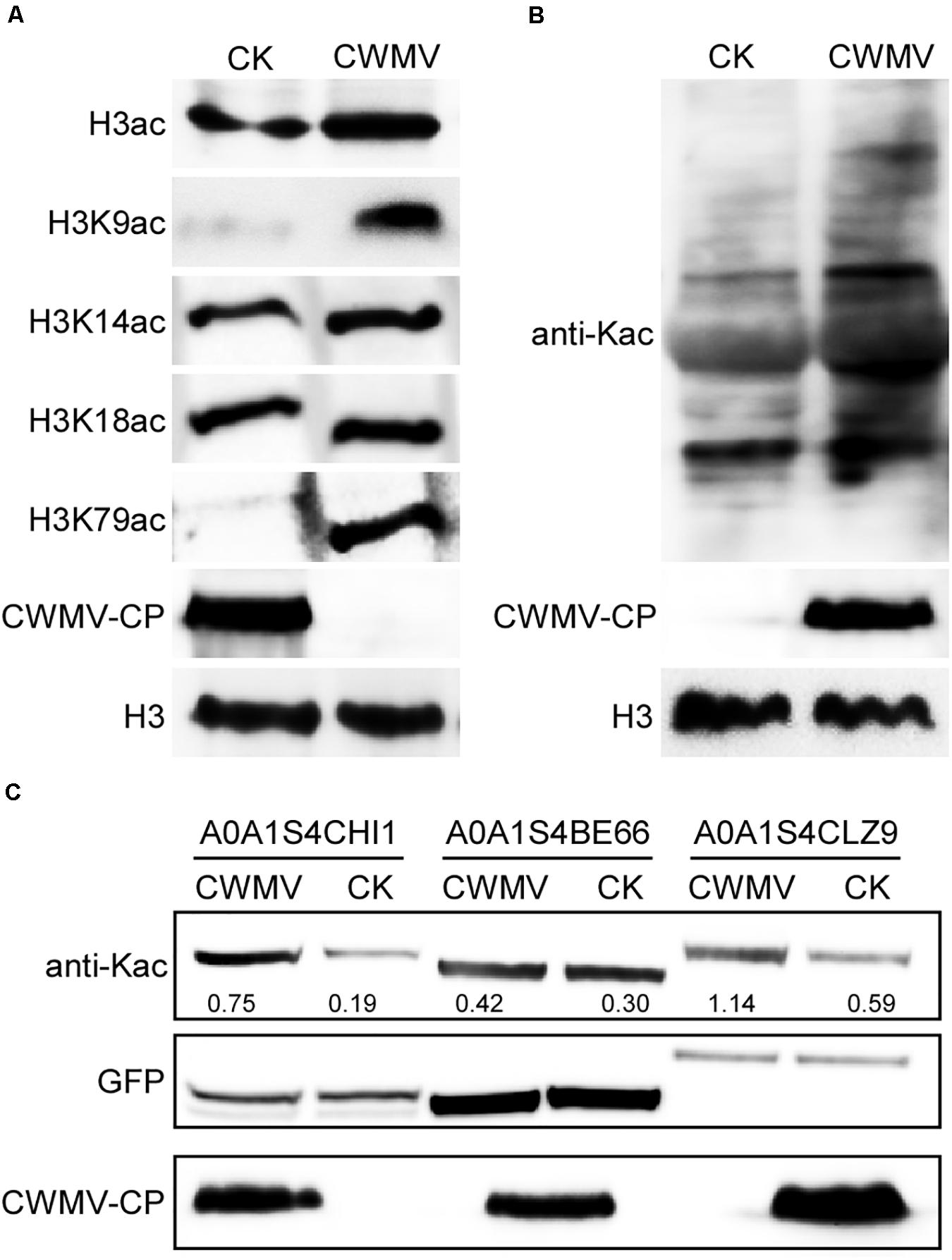
Figure 8. Protein acetylation levels in CWMV-infected and uninfected N. benthamiana. (A) Western blot analyses of different acetylated lysine sites on histone H3 in CWMV-infected and uninfected N. benthamiana leaf samples using H3K9ac-, H3K14ac-, H3K18ac-, and H3K79ac-specific antibodies. The expression level of CWMV-CP in the same samples was used to confirm the infection. The expression level of histone H3 in the same samples was used as an internal control. (B) Western blot analyses of chloroplast proteins extracted from CWMV-infected and the uninfected N. benthamiana leaves using anti-Kac antibodies. The expression level of CWMV-CP in the same samples was used to confirm the infection. The expression level of histone H3 in the same samples was used as an internal control. (C) Western blot analyses of selected proteins extracted from CWMV-infected and the uninfected N. benthamiana leaves using anti-Kac antibodies and anti-GFP antibodies. The expression level of CWMV-CP in the same samples was used to confirm the infection. The expression level of protein fused with GFP in the samples was used as an internal control.
Discussion
Lysine acetylation of proteins, a dynamic and reversible post-translational modification, plays a critical regulatory role in both eukaryotes and prokaryotes (Zhang et al., 2016). This is the first attempt to catalog acetylated proteins in N. benthamiana and the first insight into acetylation level changes in response to CWMV infection. Here, we detected 4,803 acetylated lysine sites on 1,964 proteins. These modified proteins in N. benthamiana are involved in different metabolic processes and participate in various biological processes and single-organism processes. Furthermore, the analysis of protein interaction network demonstrated that a wide range of interactions were modulated by lysine acetylation. These suggesting that protein acetylation might be a frequently event in plants associated with pathogen infection, responses to environmental stress, and metabolic processes. These results were similar to previous studies of acetylated proteins in plants, including rice (Nallamilli et al., 2014; He et al., 2016; Xiong et al., 2016; Li et al., 2018), strawberry (Fang et al., 2015), and Arabidopsis (Finkemeier et al., 2011; Wu et al., 2011; Konig et al., 2014; Hartl et al., 2017).
The chloroplast organelle in mesophyll cells of higher plants represents a sunlight-driven metabolic factory that eventually fuels life on our planet (Kirchhoff, 2019). In this study, a large proportion of acetylated proteins were located in chloroplasts (51%), suggesting that there is a close relationship between virus infection and chloroplast proteins acetylation. According to previous studies, chloroplasts could effectively activate the defensive hormonal responses in the process of plant-pathogen interaction and viral proteins located to chloroplast could promote viral pathogenesis. For example, the tomato yellow leaf curl virus-encoded C4 protein re-localizes from the plasma membrane to chloroplasts when the plant defense response is activated, interfering with chloroplast-dependent anti-viral salicylic acid biosynthesis (Medina-Puche et al., 2020). Previous studies have shown that the chloroplast provides a compartment for plant virus replication as well as membrane contents, and might help viruses evading the RNA-mediated defense response (Ahlquist et al., 2003; Dreher, 2004; Torrance et al., 2006). Another gemini-virus betasatellite-encoded protein, βC1, interacts with PsbP and subverts PsbP-mediated antiviral defense in plants (Gnanasekaran et al., 2019). The beta satellite-mediated impediments at different stages of chloroplast functionality affect the photosynthetic efficiency of N. benthamiana (Bhattacharyya et al., 2015). The viral replication complexes targeting to chloroplast require many special chloroplast components. For example, lipid in chloroplast membranes is associate with the localization of Potato mop-top virus (PTMV) encoding protein TGB2 to chloroplast membranes for replication (Cowan et al., 2012). Based on these previous findings, we hypothesized that chloroplastic protein acetylation plays an important role in virus infection.
In this study, large amount of photosynthesis related proteins acetylation were found to be up- or downregulated in N. benthamiana leaves infected with CWMV, including several light-harvesting complexes (LHCs) proteins, several PSII subunits and multiple PSI subunits. LHCs are important constituents in light energy transferring of photosystems (Yang et al., 2000). Study in wheat showed that many LHCs located to the chloroplast are associated with photosynthesis, including Lhca1, Lhcb3, Lhcb5, and Lhcb6 (Zhang et al., 2016). PSII is a complete membrane protein complex with more than 20 subunit proteins and a large number of cofactors (Umena et al., 2011). A previous study of Synechocystis suggested that PsaD could work with multiple PSI subunits, and suggested that acetylation regulates Fd activity by affecting PsaD interaction with Fd (Mo et al., 2015). Similarly, the acetylation levels of some chloroplast ATP synthases, such as alpha, beta, gamma, and ATPF0B, were different in CWMV-infected plants to those in uninfected plants. The cytochrome complex has previously been shown to affects the ATP and NADPH production by transfer electrons from PSII to PSI (Rowland et al., 2010). Accordingly, we speculate that the acetylated proteins may transfer electrons from PSII to PSI in N. benthamiana to participate in photosynthesis.
Protein lysine acetylation (Kac) of histones was first confirmed acetylation event (Phillips, 1963). Here, we found that 19 Kac sites were combined in core histones, including 10 Kac sites on histone 3, which suggests that acetylation plays a role in gene regulation by affecting chromatin structure. Previous reports have shown that a large amount of lysine residues in histone proteins are acetylated, especially histone H3 and histone H4 (Benhamed et al., 2006; Fuchs et al., 2006; Hollender and Liu, 2008; Servet et al., 2010; Tan et al., 2011; Xue et al., 2018). The conservation of histone acetylation among different species highlights the importance of studying the function of histone acetylation in plant biological processes. In addition, a large amount of precise Kac sites of non-histone proteins were demonstrated in this study, which provided valuable information for further studies on the role of protein acetylation in plant-virus interaction.
In conclusion, this is the first extensive dataset of lysine acetylated sites on proteins of CWMV-infected and uninfected N. benthamiana plants. A large number of histone lysine residues and many proteins located in the chloroplast are acetylated. Some of these chloroplast proteins are related to photosynthesis, including members of PSI and PSII, as well as different subunits of ATP synthase. This study not only broadens our understanding of lysine acetylation regulates metabolic processes in N. benthamiana infected with CWMV but also provides a rich resource for the functional investigation of proteins with Kac sites during virus infection. However, more studies are needed to uncover the role and effects of protein acetylation and to interpret the underlying mechanisms behind protein acetylation in CWMV-infected N. benthamiana.
Data Availability Statement
The datasets generated and analyzed during the current study are available in the ProteomeXchange Consortium via the PRIDE partner repository (https://www.ebi.ac.uk/pride/archive/projects/PXD012537) with the dataset identifier PXD012537.
Author Contributions
JY and KZ initiated and designed the experiments. BY, KZ, SG, LL, TL, and LC performed the experiments and collected the data. KZ analyzed the data and wrote the manuscript. JY, YC, and JC revised the manuscript. All authors read and approved the final manuscript.
Funding
This work was funded by the National Key R&D Plan in China (2017YFD-0201701), National Natural Science Foundation of China (31901954), Public Projects of Ningbo City (202002N3004), Natural Science Foundation of Ningbo City (2019A610415), Ningbo Science and Technology Innovation 2025 Major Project (2019B10004), China Modern Agricultural Industry Technology System (CARS-03), and sponsored by K. C. Wong Magna Fund Ningbo University.
Conflict of Interest
The authors declare that the research was conducted in the absence of any commercial or financial relationships that could be construed as a potential conflict of interest.
Supplementary Material
The Supplementary Material for this article can be found online at: https://www.frontiersin.org/articles/10.3389/fmicb.2021.672559/full#supplementary-material
Supplementary Figure 1 | Determination of CWMV infection.
Footnotes
- ^ https://www.solgenomics.net/tools/blast/
- ^ http://www.ebi.ac.uk/GOA/
- ^ http://www.kegg.jp/kegg/pathway.html
- ^ http://www.ebi.ac.uk/interpro/
- ^ https://string-db.org
References
Adams, M. J., Antoniw, J. F., and Kreuze, J. (2009). Virgaviridae: a new family of rod-shaped plant viruses. Arch. Virol. 154, 1967–1972. doi: 10.1007/s00705-009-0506-6
Ahlquist, P., Noueiry, A. O., Lee, W. M., Kushner, D. B., and Dye, B. T. (2003). Host factors in positive-strand RNA virus genome replication. J. Virol. 77, 8181–8186. doi: 10.1128/jvi.77.15.8181-8186.2003
Andika, I. B., Sun, L., Xiang, R., Li, J., and Chen, J. (2013). Root-specific role for Nicotiana benthamiana RDR6 in the inhibition of Chinese wheat mosaic virus accumulation at higher temperatures. Mol. Plant Microbe Interact. 26, 1165–1175. doi: 10.1094/mpmi-05-13-0137-r
Benhamed, M., Bertrand, C., Servet, C., and Zhou, D. X. (2006). Arabidopsis GCN5, HD1, and TAF1/HAF2 interact to regulate histone acetylation required for light-responsive gene expression. Plant Cell 18, 2893–2903. doi: 10.1105/tpc.106.043489
Bhattacharyya, D., Gnanasekaran, P., Kumar, R. K., Kushwaha, N. K., Sharma, V. K., Yusuf, M. A., et al. (2015). A geminivirus betasatellite damages the structural and functional integrity of chloroplasts leading to symptom formation and inhibition of photosynthesis. J. Exp. Bot. 66, 5881–5895. doi: 10.1093/jxb/erv299
Chen, J. (1993). Occurrence of fungally transmitted wheat mosaic viruses in China. Ann. Appl. Biol. 123, 55–61. doi: 10.1111/j.1744-7348.1993.tb04072.x
Choudhary, C., Kumar, C., Gnad, F., Nielsen, M. L., Rehman, M., Walther, T. C., et al. (2009). Lysine acetylation targets protein complexes and co-regulates major cellular functions. Science 325, 834–840. doi: 10.1126/science.1175371
Cowan, G. H., Roberts, A. G., Chapman, S. N., Ziegler, A., Savenkov, E. I., and Torrance, L. (2012). The potato mop-top virus TGB2 protein and viral RNA associate with chloroplasts and viral infection induces inclusions in the plastids. Front. Plant Sci. 3:290. doi: 10.3389/fpls.2012.00290
Diao, A., Chen, J., Ye, R., Zheng, T., Yu, S., Antoniw, J. F., et al. (1999). Complete sequence and genome properties of Chinese wheat mosaic virus, a new furovirus from China. J. Gen. Virol. 80(Pt 5), 1141–1145. doi: 10.1099/0022-1317-80-5-1141
Dreher, T. W. (2004). Turnip yellow mosaic virus: transfer RNA mimicry, chloroplasts and a C-rich genome. Mol. Plant Pathol. 5, 367–375. doi: 10.1111/j.1364-3703.2004.00236.x
Fang, X., Chen, W., Zhao, Y., Ruan, S., Zhang, H., Yan, C., et al. (2015). Global analysis of lysine acetylation in strawberry leaves. Front. Plant Sci. 6:739. doi: 10.3389/fpls.2015.00739
Finkemeier, I., Laxa, M., Miguet, L., Howden, A. J., and Sweetlove, L. J. (2011). Proteins of diverse function and subcellular location are lysine acetylated in Arabidopsis. Plant Physiol. 155, 1779–1790. doi: 10.1104/pp.110.171595
Fuchs, J., Demidov, D., Houben, A., and Schubert, I. (2006). Chromosomal histone modification patterns–from conservation to diversity. Trends Plant Sci. 11, 199–208. doi: 10.1016/j.tplants.2006.02.008
Gnanasekaran, P., Ponnusamy, K., and Chakraborty, S. (2019). A geminivirus betasatellite encoded βC1 protein interacts with PsbP and subverts PsbP-mediated antiviral defence in plants. Mol. Plant Pathol. 20, 943–960. doi: 10.1111/mpp.12804
Hartl, M., Fussl, M., Boersema, P. J., Jost, J. O., Kramer, K., Bakirbas, A., et al. (2017). Lysine acetylome profiling uncovers novel histone deacetylase substrate proteins in Arabidopsis. Mol. Syst. Biol. 13:949. doi: 10.15252/msb.20177819
He, D., Wang, Q., Li, M., Damaris, R. N., Yi, X., Cheng, Z., et al. (2016). Global proteome analyses of lysine acetylation and succinylation reveal the widespread involvement of both modification in metabolism in the embryo of germinating rice seed. J. Proteome Res. 15, 879–890. doi: 10.1021/acs.jproteome.5b00805
Hollender, C., and Liu, Z. (2008). Histone deacetylase genes in Arabidopsis development. J. Integr. Plant Biol. 50, 875–885.
Huang da, W., Sherman, B. T., and Lempicki, R. A. (2009). Bioinformatics enrichment tools: paths toward the comprehensive functional analysis of large gene lists. Nucleic Acids Res. 37, 1–13. doi: 10.1093/nar/gkn923
Khoury, G. A., Baliban, R. C., and Floudas, C. A. (2011). Proteome-wide post-translational modification statistics: frequency analysis and curation of the swiss-prot database. Sci. Rep. 1:90.
Kirchhoff, H. (2019). Chloroplast ultrastructure in plants. New Phytol. 223, 565–574. doi: 10.1111/nph.15730
Konig, A. C., Hartl, M., Boersema, P. J., Mann, M., and Finkemeier, I. (2014). The mitochondrial lysine acetylome of Arabidopsis. Mitochondrion 19, 252–260. doi: 10.1016/j.mito.2014.03.004
Li, X., Ye, J., Ma, H., and Lu, P. (2018). Proteomic analysis of lysine acetylation provides strong evidence for involvement of acetylated proteins in plant meiosis and tapetum function. Plant J. 93, 142–154. doi: 10.1111/tpj.13766
Liang, W., Malhotra, A., and Deutscher, M. P. (2011). Acetylation regulates the stability of a bacterial protein: growth stage-dependent modification of RNase R. Mol. Cell 44, 160–166. doi: 10.1016/j.molcel.2011.06.037
Medina-Puche, L., Tan, H., Dogra, V., Wu, M., Rosas-Diaz, T., Wang, L., et al. (2020). A defense pathway linking plasma membrane and chloroplasts and co-opted by pathogens. Cell 182, 1109–1124. doi: 10.1016/j.cell.2020.07.020
Melo-Braga, M. N., Verano-Braga, T. I, Leon, R., Antonacci, D., Nogueira, F. C., Thelen, J. J., et al. (2012). Modulation of protein phosphorylation, N-glycosylation and Lys-acetylation in grape (Vitis vinifera) mesocarp and exocarp owing to Lobesia botrana infection. Mol. Cell. Proteomics 11, 945–956. doi: 10.1074/mcp.m112.020214
Mo, R., Yang, M., Chen, Z., Cheng, Z., Yi, X., Li, C., et al. (2015). Acetylome analysis reveals the involvement of lysine acetylation in photosynthesis and carbon metabolism in the model cyanobacterium Synechocystis sp. PCC 6803. J. Proteome Res. 14, 1275–1286. doi: 10.1021/pr501275a
Nallamilli, B. R., Edelmann, M. J., Zhong, X., Tan, F., Mujahid, H., Zhang, J., et al. (2014). Global analysis of lysine acetylation suggests the involvement of protein acetylation in diverse biological processes in rice (Oryza sativa). PLoS One 9:e89283. doi: 10.1371/journal.pone.0089283
Nambi, S., Gupta, K., Bhattacharyya, M., Ramakrishnan, P., Ravikumar, V., Siddiqui, N., et al. (2013). Cyclic AMP-dependent protein lysine acylation in mycobacteria regulates fatty acid and propionate metabolism. J. Biol. Chem. 288, 14114–14124. doi: 10.1074/jbc.m113.463992
Phillips, D. M. (1963). The presence of acetyl groups of histones. Biochem. J. 87, 258–263. doi: 10.1042/bj0870258
Rao, R. S., Thelen, J. J., and Miernyk, J. A. (2014). Is Lys-Nvarepsilon-acetylation the next big thing in post-translational modifications? Trends Plant Sci. 19, 550–553. doi: 10.1016/j.tplants.2014.05.001
Rowland, J. G., Simon, W. J., Nishiyama, Y., and Slabas, A. R. (2010). Differential proteomic analysis using iTRAQ reveals changes in thylakoids associated with photosystem II-acquired thermotolerance in Synechocystis sp. PCC 6803. Proteomics 10, 1917–1929. doi: 10.1002/pmic.200900337
Servet, C., Conde e Silva, N., and Zhou, D. X. (2010). Histone acetyltransferase AtGCN5/HAG1 is a versatile regulator of developmental and inducible gene expression in Arabidopsis. Mol. Plant 3, 670–677. doi: 10.1093/mp/ssq018
Starai, V. J., and Escalante-Semerena, J. C. (2004). Identification of the protein acetyltransferase (Pat) enzyme that acetylates acetyl-CoA synthetase in Salmonella enterica. J. Mol. Biol. 340, 1005–1012. doi: 10.1016/j.jmb.2004.05.010
Tan, F., Zhang, K., Mujahid, H., Verma, D. P., and Peng, Z. (2011). Differential histone modification and protein expression associated with cell wall removal and regeneration in rice (Oryza sativa). J. Proteome Res. 10, 551–563. doi: 10.1021/pr100748e
Torrance, L., Cowan, G. H., Gillespie, T., Ziegler, A., and Lacomme, C. (2006). Barley stripe mosaic virus-encoded proteins triple-gene block 2 and gammab localize to chloroplasts in virus-infected monocot and dicot plants, revealing hitherto-unknown roles in virus replication. J. Gen. Virol. 87, 2403–2411. doi: 10.1099/vir.0.81975-0
Umena, Y., Kawakami, K., Shen, J. R., and Kamiya, N. (2011). Crystal structure of oxygen-evolving photosystem II at a resolution of 1.9 A. Nature 473, 55–60. doi: 10.1038/nature09913
Wang, Q., Zhang, Y., Yang, C., Xiong, H., Lin, Y., Yao, J., et al. (2010). Acetylation of metabolic enzymes coordinates carbon source utilization and metabolic flux. Science 327, 1004–1007. doi: 10.1126/science.1179687
Wu, X., Oh, M. H., Schwarz, E. M., Larue, C. T., Sivaguru, M., Imai, B. S., et al. (2011). Lysine acetylation is a widespread protein modification for diverse proteins in Arabidopsis. Plant Physiol. 155, 1769–1778. doi: 10.1104/pp.110.165852
Xing, S., and Poirier, Y. (2012). The protein acetylome and the regulation of metabolism. Trends Plant Sci. 17, 423–430. doi: 10.1016/j.tplants.2012.03.008
Xiong, Y., Peng, X., Cheng, Z., Liu, W., and Wang, G. L. (2016). A comprehensive catalog of the lysine-acetylation targets in rice (Oryza sativa) based on proteomic analyses. J. Proteomics 138, 20–29. doi: 10.1016/j.jprot.2016.01.019
Xue, C., Liu, S., Chen, C., Zhu, J., Yang, X., Zhou, Y., et al. (2018). Global proteome analysis links lysine acetylation to diverse functions in Oryza sativa. Proteomics 18:1700036. doi: 10.1002/pmic.201700036
Yang, D. H., Paulsen, H., and Andersson, B. (2000). The N-terminal domain of the light-harvesting chlorophyll a/b-binding protein complex (LHCII) is essential for its acclimative proteolysis. FEBS Lett. 466, 385–388. doi: 10.1016/s0014-5793(00)01107-8
Yang, J., Zhang, F., Xie, L., Song, X. J., Li, J., Chen, J. P., et al. (2016). Functional identification of two minor capsid proteins from Chinese wheat mosaic virus using its infectious full-length cDNA clones. J. Gen. Virol. 97, 2441–2450. doi: 10.1099/jgv.0.000532
Keywords: CWMV, lysine acetylation, chloroplast, photosynthesis, histone 3
Citation: Yuan B, Liu T, Cheng Y, Gao S, Li L, Cai L, Yang J, Chen J and Zhong K (2021) Comprehensive Proteomic Analysis of Lysine Acetylation in Nicotiana benthamiana After Sensing CWMV Infection. Front. Microbiol. 12:672559. doi: 10.3389/fmicb.2021.672559
Received: 05 March 2021; Accepted: 15 April 2021;
Published: 17 May 2021.
Edited by:
Xiuling Yang, Institute of Plant Protection, Chinese Academy of Agricultural Sciences, ChinaReviewed by:
Yongliang Zhang, China Agricultural University, ChinaWende Liu, Institute of Plant Protection, Chinese Academy of Agricultural Sciences, China
Copyright © 2021 Yuan, Liu, Cheng, Gao, Li, Cai, Yang, Chen and Zhong. This is an open-access article distributed under the terms of the Creative Commons Attribution License (CC BY). The use, distribution or reproduction in other forums is permitted, provided the original author(s) and the copyright owner(s) are credited and that the original publication in this journal is cited, in accordance with accepted academic practice. No use, distribution or reproduction is permitted which does not comply with these terms.
*Correspondence: Jianping Chen, jpchen2001@126.com; Kaili Zhong, zhongkaili@nbu.edu.cn