- 1Department of Laboratory Medicine, Second Medical Center, Chinese PLA General Hospital, Beijing, China
- 2Department of Gastroenterology, Second Medical Center, Chinese PLA General Hospital, National Clinical Research Center for Geriatric Diseases, Beijing, China
- 3Department of Oncology, Fifth Medical Center, Chinese PLA General Hospital, Beijing, China
- 4Department of Surgery, First Medical Center, Chinese PLA General Hospital, Beijing, China
- 5British Heart Foundation Cardiovascular Epidemiology Unit, Department of Public Health and Primary Care, University of Cambridge, Cambridge, United Kingdom
- 6Royal Free Hospital, London, United Kingdom
Efficacy of Helicobacter pylori (H. pylori) eradication therapy has declined due to rapid rises in antibiotic resistance. We investigated how increased temperature affected H. pylori (NCTC 11637) growth and its sensitivity to metronidazole in vitro. We performed transcriptomic profiling using RNA-sequencing to identify differentially expressed genes (DEGs) associated with increased temperature. Transcriptional pathways involved in temperature-driven metronidazole resistance changes were analyzed through bioinformatic and literature curation approaches. We showed that H. pylori growth was inhibited at 41°C and inhibition was more apparent with prolonged incubation. Resistance to metronidazole was also reduced—minimum inhibitory concentration for metronidazole decreased from > 256 μg/ml at 37°C to 8 μg/ml at 41°C after culturing for 3 days. RNA-sequencing results, which were highly concordant within treatment conditions, revealed more than one third of genes (583/1,552) to be differentially expressed at increased temperatures with similar proportions up and down-regulated. Quantitative real-time PCR validation for 8 out of 10 DEGs tested gave consistent direction in gene expression changes. We found enrichment for redox and oxygen radical pathways, highlighting a mechanistic pathway driving temperature-related metronidazole resistance. Independent literature review of published genes associated with metronidazole resistance revealed 46 gene candidates, 21 of which showed differential expression and 7 out of 9 DEGs associated with “redox” resistance pathways. Sanger sequencing did not detect any changes in genetic sequences for known resistance genes rdxA, frxA nor fdxB. Our findings suggest that temperature increase can inhibit the growth and reduce H. pylori resistance to metronidazole. Redox pathways are possible potential drivers in metronidazole resistance change induced by temperature. Our study provides insight into potential novel approaches in treating antibiotic resistant H. pylori.
Introduction
Helicobacter pylori (H. pylori) infection has been established as the main cause of various gastroduodenal diseases including chronic gastric inflammation, peptic ulcer disease and gastric cancer (GC) and it is classified as a class I carcinogen (Ishaq and Nunn, 2015). H. pylori resides in human gastric mucosa and affects nearly half of the human population worldwide with prevalence exceeding 80% in certain regions such as parts of Asia (Hooi et al., 2017). Eradication of H. pylori has been shown to reduce GC incidence across a range of risk groups (Takenaka et al., 2007; Kosunen et al., 2011).
The standard triple therapy, consisting of a proton pump inhibitor combined with clarithromycin and amoxicillin or metronidazole, has been the mainstay of treatment for H. pylori infection over the last two decades. However, rising antibiotic resistance has made standard triple therapy less effective—bismuth and non-bismuth based quadruple therapies have also been introduced depending on efficacy and resistance patterns locally (Graham and Fischbach, 2010). Resistance to clarithromycin and metronidazole also varies between regions across the world and have hampered the elimination of H. pylori (Kim et al., 2015). In China, the resistance rate for clarithromycin is around 50% whilst resistance to metronidazole ranges from 41.6 to 95.4% in Southeast China (Su et al., 2013; Thung et al., 2016). Various mechanisms have been shown to affect H. pylori antibiotic resistance under physiological conditions (Hu et al., 2016; Alba et al., 2017). Point mutations in 23S rRNA and changes in efflux pump systems have been shown to confer resistance to macrolides such as clarithromycin (Francesco et al., 2011). In addition, reduced activities in nitro-reductase (rdxA), flavinoxido reductase (frxA) and ferrodoxin-like enzymes (frxB) lead to reduced activation of metronidazole (Francesco et al., 2011).
Changes in environmental conditions lead to alterations in transcriptomic profiles of H. pylori (Thompson et al., 2003; De la Cruz et al., 2017). Previous evidence has demonstrated local nanoparticle heating inhibits H. pylori growth and virulence as a potential alternate approach to treating H. pylori infections (Wu et al., 2019; Zhi et al., 2019). Whilst temperature differences have led to changes leading to increased antibiotic resistance in other bacteria species (Liang et al., 2016; Loughman et al., 2016; De Silva et al., 2018), the effects of temperature on antibiotic resistance and transcriptomic changes in H. pylori have not been investigated previously.
In this study, we examine the effect of changes in temperature on the growth of H. pylori and its sensitivity to metronidazole using a reference resistant strain. In addition, we investigate perturbations in transcriptomic profiles underlying changes in temperature-driven antibiotic susceptibility.
Materials and Methods
Bacterial Strain and Culture Conditions
H. pylori type strain NCTC 11637 (Lock et al., 2001) was used for this study and 16S rRNA identification was used to verify the strains. Bacteria was cultured on Karmali Agar Base (CM0935, Oxoid, United Kingdom) supplemented with 5% sterile defibrinated sheep blood (Beijing XLF Medical Sales Co. Ltd, China) for 3–5 days at 37°C, under microaerophilic conditions: microaerobic gas mixture composed of 5% oxygen, 10% carbon dioxide, and 85% nitrogen (GEN bag microaer, BioMérieux, France). H. pylori were subcultured three times before each experiment.
Comparison of H. pylori Growth at Different Temperatures
H. pylori NCTC 11637 was cultured at 37 and 41°C to evaluate the effects of elevated temperature on bacteria growth. Bacteria was resuspended in phosphate-buffered saline (PBS) to 8 different dilutional concentrations (0.5, 0.25, 0.125, 0.0625, 0.03125, 0.015, 0.0075, 0.003 McFarland). 90 mm plates seeded with 0.1 ml of bacterial suspensions were incubated at 37°C as controls. In the treatment group, 0.1 ml bacterial suspensions at the 8 dilution concentrations were seeded on 3 sets of 8 plates. After inoculation, the 3 sets of plates (each set consists of 8 plates of different bacterial concentrations) were incubated at 41°C for 1, 3, and 5 days, respectively, and then incubated at 37°C for a further 3 days. Colonies in each plate were counted after the last incubation.
Antibiotics Susceptibility Testing
In vitro minimum inhibitory concentrations (MICs) of four antibiotics (amoxicillin, clarithromycin, metronidazole, tetracycline) against H. pylori NCTC 11637 were tested. All experiments were performed in triplicate. The MICs of amoxicillin, metronidazole and tetracycline against H. pylori were determined via the Epsilometer test (E-test) using an E-strip (BioMerieux SA, France) and the Kirby-Bauer method was used for clarithromycin sensitivity. H. pylori NCTC 11637 was cultured as described above, and the third-generation colonies were selected and suspended in PBS to the turbidity of a 2 McFarland standard. Then, 0.1 ml of the bacterial suspension was evenly coated on Karmali Agar Base. Each agar plate was left to dry for 15 min before E-strip was affixed and the plates were incubated as described above. MICs were defined as the lowest concentration that allowed no visible growth after 72 h of incubation at 37°C. The clinical breakpoints for amoxicillin, clarithromycin, metronidazole, and tetracycline are defined as: >0.125, >0.5, >8, and >1 mg/L, respectively, as per European Committee on Antimicrobial Susceptibility Testing Breakpoints version 8 (European Committee on Antimicrobial Susceptibility Testing [ECUCAST], 2018).
RNA Extraction and Transcriptomic Analysis
H. pylori NCTC 11637 strain was divided into two groups in triplicate. The experimental group was treated at 41°C for 3 days. The control group was treated at 37°C for 3 days. The cells were harvested, and total RNA was extracted and purified using the Bacterial RNA kit (Omega Bio-tek, GA, United States) according to manufacturer protocols. Quality control of each RNA sample was performed with Agilent 2100 Bioanalyzer (Agilent Technologies, Beijing, China). The cDNA libraries were constructed using NEBNext® UltraTM RNA Library Prep Kit (New England Biolabs, Ipswich, MA, United States) and submitted for sequencing using IlluminaHiseq 4000. Library construction and sequencing were performed by Allwegene BioTech Co., Ltd. (Beijing, China). Raw reads were filtered using Trimmomatic v0.33 (Bolger et al., 2014) and mapped to the H. pylori NCTC 11637 genome (National Center for Biotechnology Information [NCBI], 2012) using Bowtie2 v2.2.6 (Langmead and Salzberg, 2012) with default parameters. Genes were quantified using HTSeq v0.6.0 (Anders et al., 2015). Differentially expressed genes (DEGs) were analyzed by DESeq2 v1.22.1 in R (Love et al., 2014). Genes with absolute log2 fold-changes > 1 and multiple testing adjusted (Benjamini–Hochberg procedure) q < 0.05 were considered as DEGs.
Gene Expression Using qRT-PCR
Quantitative real-time polymerase chain reaction (qRT-PCR) assays were performed using the same samples analyzed (3 controls at 37°C and 3 at 41°C for 3 days) as in the RNA-seq transcriptomic analyses. 10 genes with differential expression levels identified using RNA sequencing were selected for subsequent validation (5 of the most significantly associated genes and 5 associated with metronidazole resistance from bioinformatic screening as described in Curation of resistance related genes). Gene-specific primers (Supplementary Table 1A) were designed and purchased from Invitrogen (Beijing, China). Three technical replicates were performed for each biological sample. First strand cDNA was synthesized using PrimeScriptTM RT reagent Kit with gDNA Eraser (Takara, Japan) according to manufacturer instructions. qRT-PCR reactions were performed using an ABI Prism 7500 Sequence Detection System (Perkin-Elmer Applied Biosystems, Foster City, CA). H. pylori 16S rRNA was used as housekeeping internal control. Gene expression and log2 fold-changes were analyzed using the 2–ΔΔCT algorithm (Livak and Schmittgen, 2001). After quality control, one biological sample at 37°C exhibited low readouts across all genes compared to the other 2 biological samples (which did not show low readouts in RNA-seq) and was excluded from analysis.
GO and KEGG Pathway Analysis
We mapped the genes to Entrez Gene symbols first. Gene Ontology (GO) enrichment analysis comprising cellular component (CC), molecular function (MF), and biological process (BP) were conducted for DEGs in R with GOSeq v1.26.0 and topGO v2.26.0 package, using the H. pylori 11637 reference strain annotated by Pfam (protein families database) (El-Gebali et al., 2019) as background. KOBAS v3.0 (Xie et al., 2011) was used to perform Kyoto Encyclopedia of Genes and Genomes (KEGG) pathway enrichment analysis for DEGs using H. pylori 26695 reference strain as background. The p < 0.05 adjusted for multiple testing (q-value) using the Benjamini-Hochberg method was used as significance thresholds for GO and KEGG pathway enrichment analyses. We also performed sensitivity analyses using absolute log2 fold-change > 1.5, q < 0.05 as cut-offs for DEGs.
Curation of Resistance Related Genes
Curation of All Mapped Genes Into Metronidazole Resistance Pathways
Metronidazole antibiotic resistance pathways in H. pylori based on four groups of broad mechanisms: (1) reduced activity of nitro-reductases, (2) increased activity of the oxygen radical scavenger system, (3) reduced uptake and increased efflux, and (4) increased activity of the DNA repair enzymes, were determined from current literature (Hu et al., 2016; Alba et al., 2017). All mapped genes (n = 1,552) were manually curated by two independent reviewers to determine whether each is related to one of the four mechanisms based on gene function and gene description. Genes with assignments which agree between the two reviewers were included.
Curation of Genes Associated With Metronidazole Resistance in Literature
Queries were made in PubMed using keywords “helicobacter pylori,” “metronidazole,” and “resist∗” and subsequently manually curated to create a list of reported H. pylori metronidazole resistance genes. DEGs identified in this study were then mapped to the list of literature reported genes. Enrichment tests were performed by permutation testing (10,000 times).
Detection and Sequencing of rdxA, frxA, and fdxB
For both 37°C and 41°C conditions, conventional polymerase chain reaction (PCR) amplification was performed. Specific reagents, primers and conditions are detailed in Supplementary Table 1B. PCR, gel electrophoresis and DNA sequencing via Sanger sequencing method were performed following standard manufacturer protocols.
Results
Increased Temperature Inhibits H. pylori Growth
The effect of elevated temperature on H. pylori (NCTC 11637 strain) growth was evaluated at 37°C (control group) and at 41°C for 1, 3, and 5 days. Growth of H. pylori was significantly inhibited after incubation at 41°C with inhibition more apparent with prolonged incubation (Figure 1A). At an inoculation concentration of 0.002 McFarland, bacterial colony count of the control group was 4,288 ± 184 CFU/ml. The counts decreased to 2,970 ± 462, 2,255 ± 575, and 1,990 ± 187 CFU/ml after 1, 3, and 5 days of treatment under 41°C, respectively (p = 0.026 [1 day], p = 0.018 [3 days], p = 0.00011 [5 days], compared with control group).
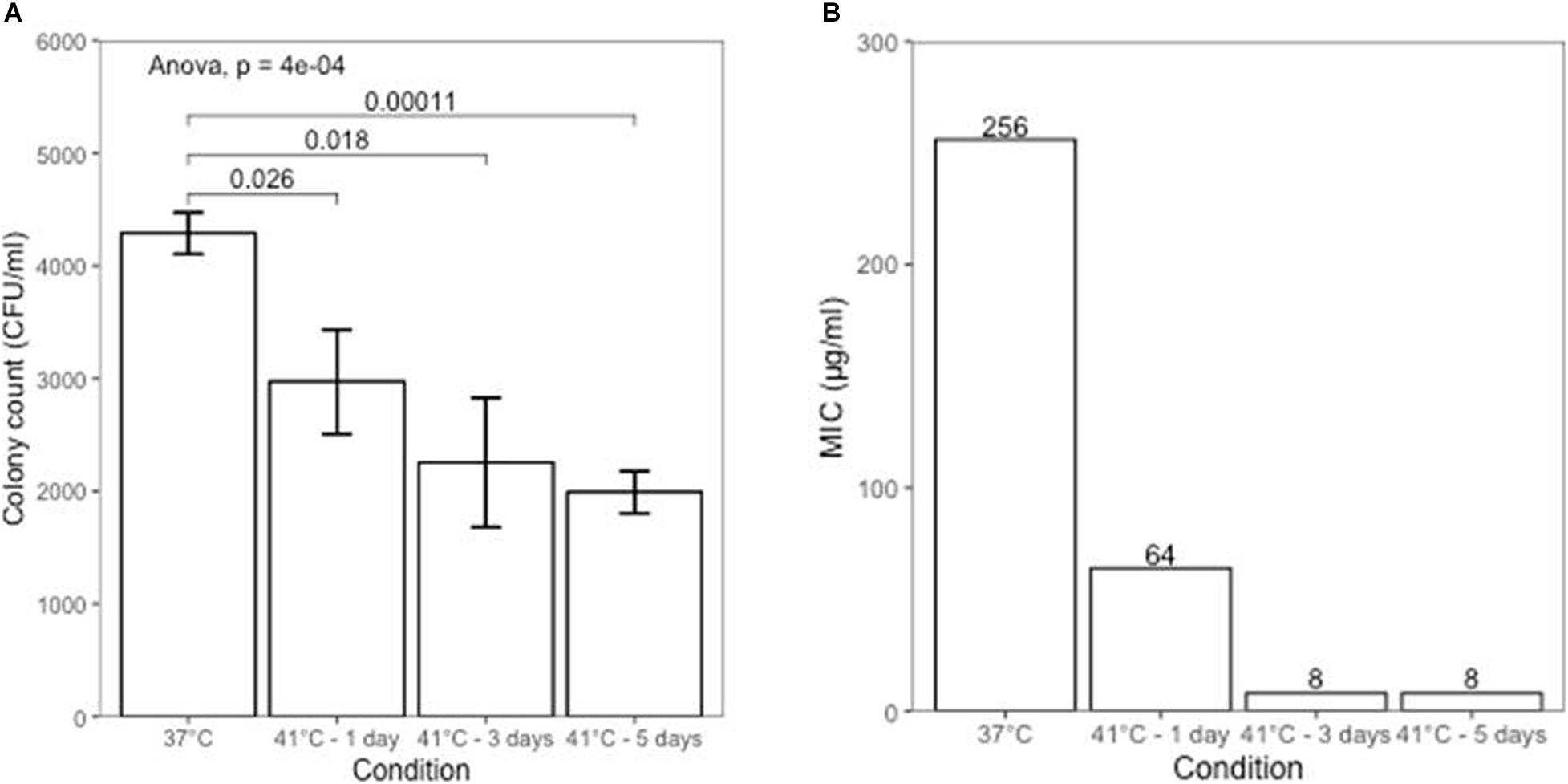
Figure 1. (A) Growth inhibition of H. pylori at different temperature conditions. (B) Changes in metronidazole susceptibility at different temperature conditions.
Temperature Change Increases Sensitivity of H. pylori to Metronidazole
We investigated the effect of increased temperature on sensitivities of the metronidazole-resistant H. pylori NCTC 11637 strain to metronidazole using the E-test strip. The minimum inhibitory concentration (MIC) for metronidazole under the 37°C culture condition (control group) was > 256 μg/ml. After culturing at 41°C for 3 days, MIC of H. pylori to metronidazole decreased to 8 μg/ml (Figure 1B), which is the breakpoint of metronidazole resistance. The NCTC 11637 strain was sensitive to clarithromycin, amoxicillin and tetracycline and this did not change under increased culture temperature conditions (Supplementary Table 2). There was no growth in the H. pylori subculture inoculation after returning from 41 to 37°C.
Transcriptome Analyses Identify Changes in Drug Resistance Genes
Identification of Differentially Expressed Genes by RNA-Sequencing
To study the transcriptomic changes which may drive decreased resistance to increased temperature, we used RNA sequencing to assess changes in gene expression between 37 and 41°C. Illumina paired-end sequencing of 6 samples (3 cultured at 37°C and 3 at 41°C) yielded a total of 252,222,378 clean reads. 70.9–80.1% reads of samples were mapped to the annotated H. pylori NCTC 11637 genome culminating in 1,552 mapped genes. Gene expression measurements were highly consistent between biological replicates within each temperature condition (Pearson’s r: 0.97–0.99 within condition, Figure 2A). 583 out of 1,552 mapped genes were significantly differentially expressed at absolute log2 fold-change > 1 and q < 0.05 after incubation at 41°C for 3 days compared to 37°C, of which 292 were up-regulated and 291 were down-regulated (Figures 2B,C and Supplementary Table 3).
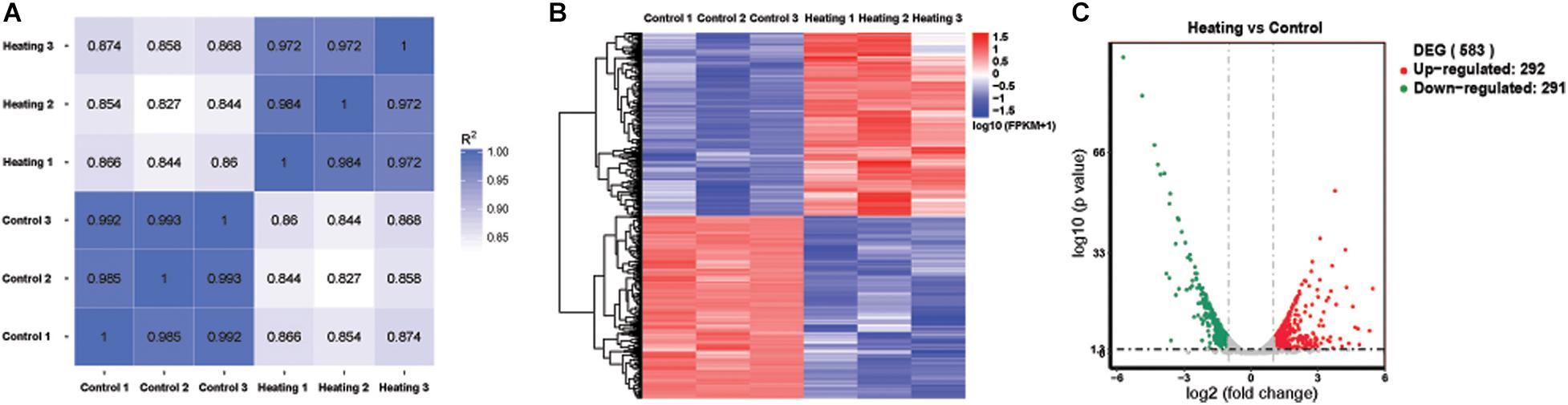
Figure 2. Differentially expressed genes (DEGs) by RNA-seq. (A) Pearson’s correlation heatmap of expression of all mapped genes (B) Hierarchical clustered heat map of significantly differentially expressed genes (583). (C) Volcano plot displaying DEGs. The vertical axis corresponds to the q-value, the horizontal axis displays the log2 fold-change. The red dots represent the up-regulated expressed transcripts (292), the green dots represent the down-regulated transcripts (291). Vertical and horizontal dashed lines indicate absolute log2 fold-change = 1 and adjusted p = 0.05, respectively.
Gene Expression Measurements Using qRT-PCR
We selected 10 DEGs, 5 most significantly associated genes and 5 related to metronidazole resistance (Materials and Methods) for measurement using qRT-PCR. 8 out of the 10 DEGs showed consistent direction in log fold gene expression changes (Figure 3). All 5 of DEGs with the strongest statistical significance of association [HP17_RS13720 (HP1076), HP_RS12585 (HP0115), flgL, HP17_RS17120 (HP0630), and HP17_RS13130 (HP1286)] showed directionally concordant changes using qRT-PCR.
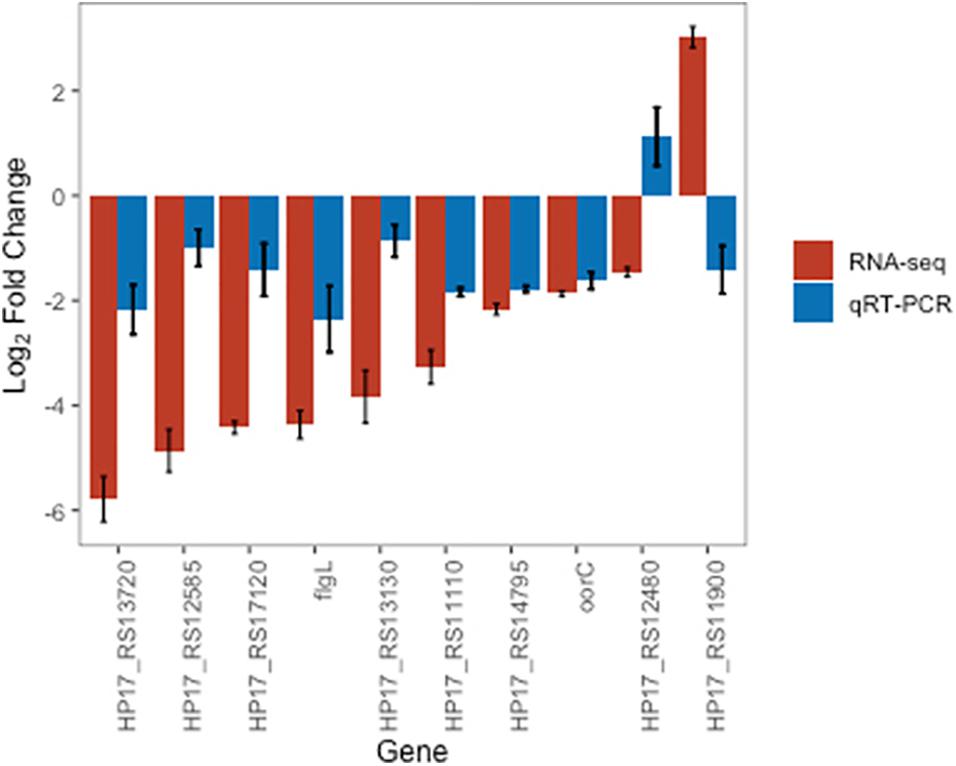
Figure 3. Barplot showing log2 fold-changes at incubation of 41°C for 3 days compared to 37°C using RNA-Seq and qRT-PCR methods for 10 selected DEGs. Error bars indicate standard deviations from the mean. Genes ordered by RNA-Seq log2 fold-changes.
GO and KEGG Pathway Enrichment Analysis
To investigate molecular and functional pathway changes as result of increased temperature, GO and KEGG pathway enrichment analyses of DEGs were performed. Whilst no pathways were significantly enriched after adjusting for multiple testing, we found enrichment at nominal significance (p < 0.05) for drug, vitamin, superoxide and reactive oxygen species metabolic GO processes. Cellular component was enriched for ribosome, non-membrane-bounded organelle and cytoplasm components at nominal threshold. Molecular function enrichment included structural constituent of ribosome, oxidoreductase activity, superoxide dismutase activity and various symporter activities (Supplementary Tables 4.1, 4.2). Sensitivity analyses, using DEGs defined using more stringent thresholds of absolute log2 fold-change > 1.5 adjusted q < 0.05 showed similar results. Both analyses showed GO enrichment for reactive oxygen species and metabolic processes (Supplementary Table 5).
DEGs Are Enriched for Specific Metronidazole Resistance Pathways
All mapped genes were curated base on gene description and function into four broad metronidazole resistance pathways: (1) nitro-reductases, (2) oxygen radical scavenger, (3) drug uptake and efflux and (4) DNA repair related. 126 genes were related to one of the four pathways (Supplementary Table 6 and Table 1). Nitro-reductase related pathways were enriched for DEGs under increased temperature condition at 41°C (permutation 10,000 times, empirical p = 0.0038). Oxygen radical scavenger pathways were borderline significant for enrichment of DEGs (empirical p = 0.05).
In addition, we performed independent literature search for published genes associated with metronidazole resistance. Our literature search revealed 46 gene candidates related to metronidazole resistance in H. pylori which were classified into 10 categories (Supplementary Table 7). These genes were all found within the 1,552 genes sequenced in our study. 21 of the 46 genes showed differential expression. Permutation testing did not reveal significant enrichment amongst resistance genes overall for DEGs (empirical p = 0.15). However, genes associated with “redox” resistance pathways were significantly enriched for DEGs with 7 out of 9 genes differentially expressed (empirical p = 0.016). Other pathways with more than 5 genes in each category did not show enrichment for DEGs.
Metronidazole is a prodrug which is first activated by nitro-reductase (mutated in resistant H. pylori NCTC 11637 strains; Debets-Ossenkopp et al., 1999) to produce oxygen radicals toxic to bacteria through DNA damage (Cederbrant et al., 1992; van der Wouden et al., 2001). We found that expression of NAD(P)H-dependent oxidoreductase (rdxA [HP17_RS12480]) was downregulated (log2 fold-change: −1.51, p = 3.8 × 10–5). In addition, we also found that superoxidase dismutase (Sod [HP17_RS11110]) expression, an important protein in detoxifying free oxygen radicals, was downregulated (log2 fold-change: −3.29, p = 4.1 × 10–45) and expression of its transcriptional repressor, ferric uptake regulator (Fur [HP17_RS13980]) (Tsugawa et al., 2011), was upregulated (log2 fold-change: 2.14, p = 1.7 × 10–8).
Key Resistance Gene Sequences Not Altered by Increased Temperature
We independently tested established H. pylori resistance genes rdxA, frxA, and fdxB through Sanger sequencing to see if increased temperature conditions led to structural changes in these genes. All three genes were successfully detected and amplified though PCR in both temperature conditions (Supplementary Figure 1). Sanger sequencing did not detect any changes in genetic sequences for rdxA, frxA nor fdxB (Supplementary Figures 2–4).
Discussion
Our study showed that increased temperature inhibited the growth of metronidazole resistant H. pylori strain (NCTC 11637) consistent with previous reported changes in growth of sensitive H. pylori strains (26695 strain) under elevated temperatures (Jiang and Doyle, 1998). In addition, we present the novel finding that elevated temperature (41°C) increased sensitivities of the resistant strain to metronidazole which has not been reported previously. Studies have identified thermoregulated antibiotic resistance in some species. Francisella tularensis showed increased resistance to gentamicin at ambient temperature (26°C) compared to mammalian body temperature (37°C) (Loughman et al., 2016). Temperature associated expression changes in antibiotic resistance were observed in Acinetobacter baumannii ATCC 17978 (De Silva et al., 2018).
This is the first study investigating gene expression changes with increased temperature in H. pylori. Transcriptomic profiling showed good internal consistency within treatment conditions and widespread changes in gene expression with more than one third of all genes differentially expressed. Previous literature showed transcriptomic changes in H. pylori with sudden decreased temperature (Han et al., 2009). Approximately equal proportion of DEGs was up and down-regulated similar to findings seen with decreased temperature.
Whilst no specific GO or KEGG pathways were enriched for DEGs after multiple testing correction, we found nominal statistical evidence indicating that metabolic processes may be enriched. Furthermore, permutation analyses based on annotation of genes for metronidazole resistance mechanisms and specific metronidazole resistance genes published in the literature showed enrichment for redox (oxygen radical scavenger) and nitro-reductase pathways. Metronidazole is a prodrug which is first activated by nitro-reductase to produce oxygen radicals toxic to bacteria through DNA damage (van der Wouden et al., 2001). Increased nitro-reductase activity in theory leads to reduced metronidazole resistance. We found reduced expression of nitro-reductases, such as NAD(P)H-dependent oxidoreductase (rdxA, HP17_RS12480) with heating. The H. pylori NCTC 11637 strain used in this study contains a transposon-induced deletion in rdxA which plays a key role conferring resistance (Debets-Ossenkopp et al., 1999), thus the NAD(P)H-dependent oxidoreductase produced by the mutated gene does not provide normal functions regardless of expression levels.
In addition we also found Sod expression to be downregulated with elevated temperature. Sod contributes to metronidazole resistance through mitigating oxygen radical related damage and increased Sod levels have been associated with development of metronidazole resistance (Wassmann and Bruchhaus, 2000; van der Wouden et al., 2001). Interestingly, we observed an increased expression of a negative transcription regulator of Sod, Fur, which may also contribute to decreased Sod expression. Therefore, another plausible mechanism by which higher temperature leads to reduced metronidazole resistance may be through decreased Sod expression which leads to increased susceptibility to superoxide damage. DEGs within other resistance pathways such as DNA repair, efflux pump complexes, bacterial flagellar mobility, changes in metabolism or potential novel pathways may also play a role in temperature induced reduction in metronidazole resistance. Lastly, we confirmed that key resistance genes, rdxA, frxA, and fdxB, are not mutated by increased temperature conditions, suggesting that resistance changes can be attributed to gene expression or protein function changes. Further studies would be needed to elucidate these functional mechanisms in detail. Our approach of studying transcriptomic changes associated with temperature-related antibiotic resistance can highlight perturbed gene pathways as well as suggest candidate genes and pathways as potential novel drug targets for antimicrobials.
Our study examined the effect of 41°C temperature on H. pylori growth, metronidazole resistance and transcriptomic changes. Further studies are needed to investigate the detailed mechanisms driving reduced resistance to metronidazole. Effects at other temperatures as well as using strains with different profiles and mechanisms of resistance to various other antibiotics commonly used in H. pylori eradication could also be explored. Whether in vitro effects of increased temperature are also reflected in vivo remain to be elucidated.
At present, antibiotics treatment combined with proton-pump inhibitors is the main approach to treating H. pylori infections. Unfortunately, efficacy has decreased, due to the rapid rise in resistance rates (Fallone et al., 2019). Emergence of multi-drug resistance strains further exacerbates the problem (Boyanova et al., 2019). H. pylori colonizes and grows in gastric mucosa in humans which makes local treatment possible. Since the stomach mucosa of mammal species can withstand temperatures as high as 46°C (Kang et al., 2004), raising local temperatures have been postulated to help eradicate H. pylori in vivo complementing antibiotic-based therapies. One way to achieve this may be through well-controlled local photothermal/magnetic thermal treatments using nanomaterials which have shown early promise in suppressing bacterial growths (Mocan et al., 2017; Chen et al., 2018), including H. pylori (Wu et al., 2019). It is recently reported that gold nanostars@H. pylori-antibodies nanoprobes could target and kill H. pylori in the stomach in model animals under near-infrared laser irradiation (Zhi et al., 2019). However, feasibility and safety of sustained localized thermal treatment within human gastric mucosa need to be ascertained in a clinical setting in order for it to become a viable strategy for H. pylori eradication.
In conclusion, growth of H. pylori and resistance to metronidazole was significantly inhibited when cultured in vitro at 41°C. Transcriptomic analyses showed differential gene expression changes in more than one third of the genes with evidence of enrichment pointing to redox pathways as potential drivers of temperature related reduction in metronidazole resistance. Expression changes for genes in other resistance pathways may also play a role in temperature induced metronidazole sensitivity. Our findings suggest a novel approach in treating metronidazole and multi-drug resistant H. pylori in vivo.
Data Availability Statement
The original contributions presented in the study are publicly available. This data can be found here: https://www.ncbi.nlm.nih.gov/sra/PRJNA718481.
Author Contributions
MG carried out H. pylori growth experiments and antibiotics susceptibility testing, and drafted the manuscript. YH performed bioinformatic analysis and curation of related genes. XW participated in bioinformatic analysis. HT, FM, and BH participated in antibiotics susceptibility testing and gene expression analysis. BS and GW designed and conceived of the study, participated in its coordination, and wrote the manuscript. GW had primary responsibility for final content. All authors read and approved the final manuscript.
Funding
This study was supported by the National Science Foundation of China (grant no. 51471186) and the National Clinical Research Center for Geriatric Diseases (grant no. NCRCG-PLAGH-2019015). The funder had no role in the study design, data collection, data analysis, data interpretation, or writing of the article.
Conflict of Interest
The authors declare that the research was conducted in the absence of any commercial or financial relationships that could be construed as a potential conflict of interest.
Supplementary Material
The Supplementary Material for this article can be found online at: https://www.frontiersin.org/articles/10.3389/fmicb.2021.681911/full#supplementary-material
Supplementary Figure 1 | Agarose gel electrophoresis of gene fragments of H. pylori NCTC 11637 amplified by PCR. (A) Lanes 1–6, PCR fragments of gene rdxA; (B) Lane 1, PCR fragments of gene frxA of H. pylori NCTC 11637 cultured in 37°C; Lane 2, PCR fragments of gene frxA of H. pylori NCTC 11637 cultured in 41°C; Lanes 3–5, PCR fragments of gene fdxB of H. pylori NCTC 11637 cultured in 37°C with primer pairs 1–3, respectively, Lanes 6–8, PCR fragments of gene fdxB of H. pylori NCTC 11637 cultured in 41°C with primer pairs 1–3, respectively.
Supplementary Figure 2 | The DNA sequence blast of rdxA gene in H. pylori 26695 and H. pylori NCTC 11637. The H. pylori 26695 is sensitive to metronidazole but H. pylori NCTC 11637 is resistant. Compared with H. pylori 26695, the structural variation of rdxA gene in H. pylori NCTC 11637 included point mutations and the insertion of mini-IS605, which caused rdxA inactivation and thus metronidazole resistance. 11637-rdxA-37: the rdxA gene sequence of H. pylori NCTC 11637 cultured in 37°C. 11637-rdxA-41: the rdxA gene sequence of H. pylori NCTC 11637 cultured in 41°C. 26695-rdxA: the rdxA gene reference sequence of H. pylori 26695. The dashed rectangle showed the start site of rdxA gene and the solid rectangle showed the termination site of rdxA gene. The figure showed that the 11637-rdxA-37 and 11637-rdxA-41 were exactly the same. The high light region showed the insertion of mini-IS605, one of the endogenous transposable elements, in H. pylori NCTC 11637.
Supplementary Figure 3 | The DNA sequence blast of frxA gene in H. pylori 26695 and H. pylori NCTC 11637. 11637-frxA-37: the frxA gene sequence of H. pylori NCTC 11637 cultured in 37°C. 11637-frxA-41: the frxA gene sequence of H. pylori NCTC 11637 cultured in 41°C. 26695-frxA: the frxA gene reference sequence of H. pylori 26695. The figure showed that the 11637-frxA-37 and 11637-frxA-41 were exactly the same.
Supplementary Figure 4 | The DNA sequence blast of fdxB gene in H. pylori 26695 and H. pylori NCTC 11637. 11637-fdxB-37: the fdxB gene sequence of H. pylori NCTC 11637 cultured in 37°C. 11637-fdxB-41: the fdxB gene sequence of H. pylori NCTC 11637 cultured in 41°C. 26695-fdxB: the fdxB gene reference sequence of H. pylori 26695. The figure showed that the 11637-fdxB-37 and 11637-fdxB-41 were exactly the same.
Abbreviations
BP, biological process; CC, cellular component; CFU, colony forming units; DEGs, differentially expressed genes; E-test, Epsilometer test; GC, gastric cancer; GO, Gene Ontology; H. pylori, Helicobacter pylori; KEGG, Kyoto Encyclopedia of Genes and Genomes; MF, molecular function; MIC, minimum inhibitory concentration; PBS, phosphate-buffered saline; PCR, polymerase chain reaction; Pfam, protein families database.
References
Alba, C., Blanco, A., and Alarcon, T. (2017). Antibiotic resistance in Helicobacter pylori. Curr. Opin. Infect. Dis. 30, 489–497. doi: 10.1097/QCO.0000000000000396
Anders, S., Pyl, P. T., and Huber, W. (2015). HTSeq–a Python framework to work with high-throughput sequencing data. Bioinformatics 31, 166–169. doi: 10.1093/bioinformatics/btu638
Bolger, A. M., Lohse, M., and Usadel, B. (2014). Trimmomatic: a flexible trimmer for Illumina sequence data. Bioinformatics 30, 2114–2120. doi: 10.1093/bioinformatics/btu170
Boyanova, L., Hadzhiyski, P., Kandilarov, N., Markovska, R., and Mitov, I. (2019). Multidrug resistance in Helicobacter pylori: current state and future directions. Expert. Rev. Clin. Pharmacol. 12, 909–915. doi: 10.1080/17512433.2019.1654858
Cederbrant, G., Kahlmeter, G., and Ljungh, A. (1992). Proposed mechanism for metronidazole resistance in Helicobacter pylori. J. Antimicrob. Chemother. 29, 115–120. doi: 10.1093/jac/29.2.115
Chen, W., Wang, Y., Qin, M., Zhang, X., Zhang, Z., Sun, X., et al. (2018). Bacteria-driven hypoxia targeting for combined biotherapy and photothermal therapy. ACS Nano 12, 5995–6005. doi: 10.1021/acsnano.8b02235
De la Cruz, M. A., Ares, M. A., von Bargen, K., Panunzi, L. G., Martinez-Cruz, J., Valdez-Salazar, H. A., et al. (2017). Gene expression profiling of transcription factors of Helicobacter pylori under different environmental conditions. Front. Microbiol. 8:615. doi: 10.3389/fmicb.2017.00615
De Silva, P. M., Chong, P., Fernando, D. M., Westmacott, G., and Kumar, A. (2018). Effect of incubation temperature on antibiotic resistance and virulence factors of Acinetobacter baumannii ATCC 17978. Antimicrob. Agents Chemother. 62:e01514–17. doi: 10.1128/AAC.01514-17
Debets-Ossenkopp, Y. J., Pot, R. G., van Westerloo, D. J., Goodwin, A., Vandenbroucke-Grauls, C. M., Berg, D. E., et al. (1999). Insertion of mini-IS605 and deletion of adjacent sequences in the nitroreductase (rdxA) gene cause metronidazole resistance in Helicobacter pylori NCTC11637. Antimicrob. Agents Chemother. 43, 2657–2662. doi: 10.1128/AAC.43.11.2657
El-Gebali, S., Mistry, J., Bateman, A., Eddy, S. R., Luciani, A., Potter, S. C., et al. (2019). The Pfam protein families database in 2019. Nucleic Acids Res. 47, D427–D432. doi: 10.1093/nar/gky995
European Committee on Antimicrobial Susceptibility Testing [ECUCAST] (2018). Clinical Breakpoints – Bacteria (V 8.0). Available online at: https://www.eucast.org/clinical_breakpoints/ (accessed January 6, 2018).
Fallone, C. A., Moss, S. F., and Malfertheiner, P. (2019). Reconciliation of recent Helicobacter pylori treatment guidelines in a time of increasing resistance to antibiotics. Gastroenterology 157, 44–53. doi: 10.1053/j.gastro.2019.04.011
Francesco, V. D., Zullo, A., Hassan, C., Giorgio, F., Rosania, R., and Ierardi, E. (2011). Mechanisms of Helicobacter pylori antibiotic resistance: an updated appraisal. World J. Gastrointest. Pathophysiol. 2, 35–41. doi: 10.4291/wjgp.v2.i3.35
Graham, D. Y., and Fischbach, L. (2010). Helicobacter pylori treatment in the era of increasing antibiotic resistance. Gut 59, 1143–1153. doi: 10.1136/gut.2009.192757
Han, Y. H., Liu, W. Z., Shi, Y. Z., Lu, L. Q., Xiao, S. D., and Zhang, Q. H. (2009). Gene expression profile of Helicobacter pylori in response to growth temperature variation. J. Microbiol. 47, 455–465.
Hooi, J. K. Y., Lai, W. Y., Ng, W. K., Suen, M. M. Y., Underwood, F. E., Tanyingoh, D., et al. (2017). Global prevalence of Helicobacter pylori infection: systematic review and meta-analysis. Gastroenterology 153, 420–429. doi: 10.1053/j.gastro.2017.04.022
Hu, Y., Zhang, M., Lu, B., and Dai, J. (2016). Helicobacter pylori and antibiotic resistance, a continuing and intractable problem. Helicobacter 21, 349–363. doi: 10.1111/hel.12299
Ishaq, S., and Nunn, L. (2015). Helicobacter pylori and gastric cancer: a state of the art review. Gastroenterol. Hepatol. Bed Bench 8(Suppl 1), S6–S14.
Jiang, X., and Doyle, M. P. (1998). Effect of environmental and substrate factors on survival and growth of Helicobacter pylori. J. Food Prot. 61, 929–933. doi: 10.4315/0362-028x-61.8.929
Kang, Y. M., Bielefeldt, K., and Gebhart, G. F. (2004). Sensitization of mechanosensitive gastric vagal afferent fibers in the rat by thermal and chemical stimuli and gastric ulcers. J. Neurophysiol. 91, 1981–1989.
Kim, S. Y., Choi, D. J., and Chung, J. W. (2015). Antibiotic treatment for Helicobacter pylori: is the end coming? World J. Gastrointest. Pharmacol. Ther. 6, 183–198. doi: 10.4292/wjgpt.v6.i4.183
Kosunen, T. U., Pukkala, E., Sarna, S., Seppala, K., Aromaa, A., Knekt, P., et al. (2011). Gastric cancers in Finnish patients after cure of Helicobacter pylori infection: a cohort study. Int. J. Cancer 128, 433–439. doi: 10.1002/ijc.25337
Langmead, B., and Salzberg, S. L. (2012). Fast gapped-read alignment with Bowtie 2. Nat. Methods. 9, 357–359. doi: 10.1038/nmeth.1923
Liang, Y., Guo, Z., Gao, L., Guo, Q., Wang, L., Han, Y., et al. (2016). The role of the temperature-regulated acyltransferase (PA3242) on growth, antibiotic resistance and virulence in Pseudomonas aeruginosa. Microb. Pathog. 101, 126–135. doi: 10.1016/j.micpath.2016.09.019
Livak, K. J., and Schmittgen, T. D. (2001). Analysis of relative gene expression data using real-time quantitative PCR and the 2(-Delta Delta C(T)) Method. Methods 25, 402–408. doi: 10.1006/meth.2001.1262
Lock, R. A., Cordwell, S. J., Coombs, G. W., Walsh, B. J., and Forbes, G. M. (2001). Proteome analysis of Helicobacter pylori: major proteins of type strain NCTC 11637. Pathology 33, 365–374.
Loughman, K., Hall, J., Knowlton, S., Sindeldecker, D., Gilson, T., Schmitt, D. M., et al. (2016). Temperature-dependent gentamicin resistance of Francisella tularensis is mediated by uptake modulation. Front. Microbiol. 7:37. doi: 10.3389/fmicb.2016.00037
Love, M. I., Huber, W., and Anders, S. (2014). Moderated estimation of fold change and dispersion for RNA-seq data with DESeq2. Genome Biol. 15:550. doi: 10.1186/s13059-014-0550-8
Mocan, L., Tabaran, F. A., Mocan, T., Pop, T., Mosteanu, O., Agoston-Coldea, L., et al. (2017). Laser thermal ablation of multidrug-resistant bacteria using functionalized gold nanoparticles. Int. J. Nanomed. 12, 2255–2263. doi: 10.2147/IJN.S124778
National Center for Biotechnology Information [NCBI] (2012). Helicobacter pylori NCTC 11637 Genome Sequencing and Assembly. Available online at: https://www.ncbi.nlm.nih.gov/bioproject/PRJNA76569 (accessed October 16, 2019).
Su, P., Li, Y., Li, H., Zhang, J., Lin, L., Wang, Q., et al. (2013). Antibiotic resistance of Helicobacter pylori isolated in the Southeast Coastal Region of China. Helicobacter 18, 274–279. doi: 10.1111/hel.12046
Takenaka, R., Okada, H., Kato, J., Makidono, C., Hori, S., Kawahara, Y., et al. (2007). Helicobacter pylori eradication reduced the incidence of gastric cancer, especially of the intestinal type. Aliment Pharmacol. Ther. 25, 805–812. doi: 10.1111/j.1365-2036.2007.03268.x
Thompson, L. J., Merrell, D. S., Neilan, B. A., Mitchell, H., Lee, A., and Falkow, S. (2003). Gene expression profiling of Helicobacter pylori reveals a growth-phase-dependent switch in virulence gene expression. Infect. Immun. 71, 2643–2655. doi: 10.1128/iai.71.5.2643-2655.2003
Thung, I., Aramin, H., Vavinskaya, V., Gupta, S., Park, J. Y., Crowe, S. E., et al. (2016). Review article: the global emergence of Helicobacter pylori antibiotic resistance. Aliment Pharmacol. Ther. 43, 514–533. doi: 10.1111/apt.13497
Tsugawa, H., Suzuki, H., Satoh, K., Hirata, K., Matsuzaki, J., Saito, Y., et al. (2011). Two amino acids mutation of ferric uptake regulator determines Helicobacter pylori resistance to metronidazole. Antioxid. Redox. Signal. 14, 15–23. doi: 10.1089/ars.2010.3146
van der Wouden, E. J., Thijs, J. C., Kusters, J. G., van Zwet, A. A., and Kleibeuker, J. H. (2001). Mechanism and clinical significance of metronidazole resistance in Helicobacter pylori. Scand. J. Gastroenterol. Suppl. 234, 10–14. doi: 10.1080/003655201753265055
Wassmann, C., and Bruchhaus, I. (2000). Superoxide dismutase reduces susceptibility to metronidazole of the pathogenic protozoan Entamoeba histolytica under microaerophilic but not under anaerobic conditions. Arch. Biochem. Biophys. 376, 236–238. doi: 10.1006/abbi.2000.1707
Wu, T., Wang, L., Gong, M., Lin, Y., Xu, Y., Ye, L., et al. (2019). Synergistic effects of nanoparticle heating and amoxicillin on H. pylori inhibition. J. Magn. Magn. Mater. 485, 95–104. doi: 10.1016/j.jmmm.2019.04.076
Xie, C., Mao, X., Huang, J., Ding, Y., Wu, J., Dong, S., et al. (2011). KOBAS 2.0: a web server for annotation and identification of enriched pathways and diseases. Nucleic Acids Res. 39, W316–W322. doi: 10.1093/nar/gkr483
Keywords: Helicobacter pylori, temperature, transcriptomics, antibiotic resistance, metronidazole
Citation: Gong M, Han Y, Wang X, Tao H, Meng F, Hou B, Sun BB and Wang G (2021) Effect of Temperature on Metronidazole Resistance in Helicobacter pylori. Front. Microbiol. 12:681911. doi: 10.3389/fmicb.2021.681911
Received: 17 March 2021; Accepted: 23 April 2021;
Published: 19 May 2021.
Edited by:
Jong H. Kim, Agricultural Research Service, United StatesReviewed by:
Alba Trespalacios, Pontifical Javeriana University, ColombiaXiaoming Wang, Nanjing Agricultural University, China
Copyright © 2021 Gong, Han, Wang, Tao, Meng, Hou, Sun and Wang. This is an open-access article distributed under the terms of the Creative Commons Attribution License (CC BY). The use, distribution or reproduction in other forums is permitted, provided the original author(s) and the copyright owner(s) are credited and that the original publication in this journal is cited, in accordance with accepted academic practice. No use, distribution or reproduction is permitted which does not comply with these terms.
*Correspondence: Benjamin B. Sun, YnM0NDZAbWVkc2NobC5jYW0uYWMudWs=; Gangshi Wang, d2FuZ2dhbmdzaGlAaG90bWFpbC5jb20=
†These authors have contributed equally to this work