- 1College of Chemical Engineering, Beijing University of Chemical Technology, Beijing, China
- 2TEDA Institute of Biological Sciences and Biotechnology, Nankai University, Tianjin, China
Having been generated with a tremendous amount annually, paper waste (PW) represents a large proportion in municipal solid waste (MSW) and also a potential source of renewable energy production through the application of anaerobic digestion (AD). However, the recalcitrant lignocellulosic structure poses obstacles to efficient utilization in this way. Recently, anaerobic and microaerobic pretreatment have attracted attention as approaches to overcome the obstacles of biogas production. This study was set out to present a systematic comparison and assessment of anaerobic and microaerobic pretreatment of PW with different oxygen loadings by five microbial agents: composting inoculum (CI), straw-decomposing inoculum (SI), cow manure (CM), sheep manure (SM), and digestate effluent (DE). The hints of microbial community evolution during the pretreatment and AD were tracked by 16S rRNA high-throughput sequencing. The results demonstrated that PW pretreated by DE with an oxygen loading of 15 ml/gVS showed the highest cumulative methane yield (CMY) of 343.2 ml/gVS, with a BD of 79.3%. In addition to DE, SI and SM were also regarded as outstanding microbial agents for pretreatment because of the acceleration of methane production at the early stage of AD. The microbial community analysis showed that Clostridium sensu stricto 1 and Clostridium sensu stricto 10 possessed high relative abundance after anaerobic pretreatment by SI, while Bacteroides and Macellibacteroides were enriched after microaerobic pretreatment by SM, which were all contributable to the cellulose degradation. Besides, aerobic Bacillus in SI and Acinetobacter in SM and DE probably promoted lignin degradation only under microaerobic conditions. During AD, VadinBC27, Ruminococcaceae Incertae Sedis, Clostridium sensu stricto 1, Fastidiosipila, and Caldicoprobacter were the crucial bacteria that facilitated the biodegradation of PW. By comparing the groups with same microbial agent, it could be found that changing the oxygen loading might result in the alternation between hydrogenotrophic and acetoclastic methanogens, which possibly affected the methanogenesis stage. This study not only devised a promising tactic for making full use of PW but also provided a greater understanding of the evolution of microbial community in the pretreatment and AD processes, targeting the efficient utilization of lignocellulosic biomass in full-scale applications.
Introduction
In modern society, paper materials have become indispensable consumer products with a wide variety of civil and industrial uses (Bajpai, 2014). With the unprecedented economic growth and social improvement in the past 20 years, China has become one of the largest paper-producing and paper-consuming countries (NationMaster, 2021), resulting in tremendous production of paper waste (PW) annually. In 2016, the production of PW in China was over 18 million tons, accounting for 9.15% of total municipal solid waste (MSW) (Zhu et al., 2020). At present, the pathway for the environmentally friendly utilization of PW focuses on the recycling and reproduction for new paper products. However, only for limited cycles can the PW be reused due to the decreased fiber strength, whiteness, and quality in the reproduction (Li et al., 2020). Besides, the PW tainted with glue, paint, food wastes, and other residues cannot be recycled, much of which is simply discarded as MSW (Ma et al., 2020; Zhao et al., 2020). Although other treatment methods, such as incineration, landfilling, and composting, have been gradually developed, the environmental impacts and low energy recovery place a heavy burden on their broader popularization (Manfredi et al., 2011; Sanscartier et al., 2012). Therefore, it is of high significance to develop alternative approaches to make the utmost utilization of PW.
For decades, anaerobic digestion (AD) has been emerging as an efficient and viable solution for biowastes to alleviate environmental pollution and produce renewable energy (Wang et al., 2021). Originated from wood, grass, and other plants, PW is mainly composed of lignocellulosic matter and can be applied in AD (Li et al., 2020), whereas, similar to other feedstocks, the intrinsic lignocellulosic structure retards the decomposition of organic matter and negatively affects the biodegradation process (Abraham et al., 2020). Therefore, many pretreatment strategies have been proposed to break the ceiling of their digestibility. Microbial pretreatment, as a means of biological pretreatment, has recently aroused great interest. The various microbes inoculated into the biowaste produce functional enzymes and metabolites, causing the destruction of the lignocellulosic structure. As a result, enzymatic hydrolysis and biomethanation efficiency could be enhanced (Zhao et al., 2019; Lee et al., 2020). Compared with physical and chemical-based pretreatment methods, microbial pretreatment possesses advantages of low cost, safety, and non-pollution along with the absence of intensive heating or chemicals (Carrere et al., 2016; Amin et al., 2017). Up to now, diverse fungi, bacteria, and microbial consortia have been introduced for pretreatment to assist in the bioconversion of biowaste. Kainthola et al. reported that the methane yield of rice straw was significantly increased by microbial pretreatment using Phanerochaete chrysosporium (Kainthola et al., 2019). It was reported by Ali et al. that the methane yield of sawdust pretreated by bacterial consortium increased by 92.2% compared with the control (Ali et al., 2019). The constructed microbial consortia described by Patel et al. led to a 3.7-fold polyhydroxybutyrate yield improvement during the dark fermentation of pea-shell slurry (Patel et al., 2015). However, there are still drawbacks undermining the feasibility of microbial pretreatment in large-scale applications. The mild biochemical reactions by microbes make the degradation rate to be slower than that of physical and chemical solutions (Den et al., 2018). Besides, the hydraulic retention time of microbial pretreatment is naturally extended due to microbial growth and propagation, especially for fungi (Amin et al., 2017). Such disadvantages will consequently increase the equipment scale, energy consumption, and capital investment to an extent.
Recently, microaerobic pretreatment, that is, supplying a small amount of oxygen during microbial pretreatment, has been given much attention. Previous studies have shown that under the microaerobic condition, the stimulated microbes showed higher hydrolysis and acidogenesis activity (Lim and Wang, 2013). Therefore, the shorter pretreatment period and lower energy costs can be realized (He et al., 2017; Xu et al., 2018; Wang et al., 2020). However, the microbes for pretreatment were not adaptive to every substrate to improve methane production, nor was it under every oxygen content. For instance, Zhen et al. (2020) stated that cumulative methane yield (CMY) of kitchen wastes did not show any improvement after the microaerobic pretreatment. It was reported by Fu et al. that microaerobic pretreatment with excessive oxygen resulted in decreased methane production of corn straw (Fu et al., 2016). During the microbial pretreatment, there existed a complicated microbial relationship created by a variety of microbes with distinct functions, some of which possibly exhibited different response to the oxygen loading and therefore affected the AD process. Zhen et al. stated that after the microaerobic pretreatment, the Firmicute and Bacteroidetes were the predominant phyla during the AD of rice straw (Zhen et al., 2021). However, Ruan et al. (2019) found that the digestate from the anaerobic sludge digestion possessed higher abundances of Proteobacteria and Chloroflexi. It can be deduced that the substrate, microbial consortia, and oxygen loadings are crucial factors that contribute to this difference. However, the systematic comparison in terms of these factors was still insufficient due to the various experimental conditions. In this work, five different microbial agents enriched with various microbes, namely, composting inoculum (CI), straw-decomposing inoculum (SI), cow manure (CM), sheep manure (SM), and digestate effluent (DE), were introduced to achieve the following aims:
1. Construct an optimal microbial pretreatment tactic that combines the microbial agent with suitable oxygen loading to attain improved methane production performance of PW.
2. Explore the evolution of the microbial community in anaerobic pretreatment, microaerobic pretreatment, and AD.
3. Propose the functional microbes that synergistically facilitate the biodegradation of lignocellulosic materials during the pretreatment and AD.
Materials and Methods
Substrates, Microbial Agents, and Inoculum
The corrugated board (CB) and tissue paper (TP) bought in a supermarket were cut into pieces about 5 mm in length. The CI and SI were liquid and solid microbial consortia commercially designed for composting crop residues. The CM and SM were directly delivered from a farm in Shunyi District, Beijing. DE and inoculum for AD were the activated anaerobic sludge obtained from a biogas plant in Shunyi District, Beijing, which was naturally degassed for 20 days to eliminate the residual biogas. The characteristics of the substrates and microbial agents are shown in Table 1.
Anaerobic and Microaerobic Pretreatment
The pretreatment was conducted in 20 digesters, which were split into four groups. In each group, the five digesters were filled with each 5 g (based on total solids, TS) of corrugated board and tissue paper and then assigned to the five microbial agents, respectively. Five milliliters of CI and 2 g (based on volatile solids, VS) of SI, CM, SM, and DE were added into assigned digesters. A proper amount of deionized water was also filled into all digesters to reach a TS of 15% and then shaken well to homogenize the mixture. The digesters were purged with high-purity nitrogen (99.99%) for 3 min before a specific amount of pure oxygen was injected into the digesters of each group, that is, 0, 5, 15, and 30 ml/gVS, respectively. All digesters were labeled as “microbial agent—oxygen loading” and placed in a 37°C thermostatic incubator, and the concentrations of oxygen and carbon dioxide were measured every 12 h. The experiments were conducted in triplicate.
Anaerobic Digestion
The preparation of AD started when the oxygen in all digesters was almost exhausted. The substrate-to-inoculum ratio was set to 1:1 (based on VS), and the anaerobic inoculum was added to each digester. Besides, some water was also added into the digester to keep the TS at 15% (organic loading at 47.7 gVS/L). The digesters were purged with pure nitrogen to eliminate other gases, carefully sealed, and placed in a 37°C incubator to start the AD process. Simultaneously, the untreated group (labeled as UN) and blank group for the AD experiment were set up. In the untreated group, PW was directly mixed with inoculum and each microbial agent in five digesters, whose dosages were consistent with what we used for the pretreatment. The blank group was established using five digesters containing inoculum and each microbial agent to calculate the net methane production without the substrates. The untreated and blank groups were also flushed with nitrogen gas and placed in the incubator. Before the biogas pressure test, all digesters were made to shake for 1 min at a low speed. A flowsheet of the experimental setup is shown in Figure 1.
Analytical Methods
Basic Characteristics
The TS and VS for substrates and inoculum could be calculated by Eqs. (1) and (2), based on the standard test methods from APHA (APHA, 2005):
where m1 represents the weight of dish (mg), m2 is equal to the weight of fresh sample and dish (mg), m3 is the weight of dried residue and dish (mg), and m4 is assigned to the weight of residue and dish after ignition (mg). A fiber analyzer (ANKOM, New York) was installed to calculate the cellulose, hemicellulose, and lignin contents according to the conventional method (Van Soest et al., 1991; Zhao et al., 2017). The determination of organic elements, including carbon (C), nitrogen (N), hydrogen (H), and sulfur (S), relied on an elemental analyzer (Vario EL cube, Elementar, Germany) (Ning et al., 2018). The mass balance equation supported the calculation of O content, that is, C + H + O + N = 99.5% (based on VS) (Rincón et al., 2012). Since then, it was reasonable to compute the theoretical maximum methane yield (TMY, ml/gVS) of substrates and microbial agents using Buswell’s formula (Li et al., 2018), as shown in Eqs. (3) and (4).
Production of Methane and Volatile Fatty Acids
The daily biogas yield was calculated following the ideal gas law as shown in Eq. (5) and modified into the standard conditions (0°C, 101 kPa):
where Vbiogas stands for the daily biogas yield (L), P refers to the pressure difference (kPa) before and after discharging biogas, Vheadspace is defined as the headspace volume of the digester (L), and T is the absolute temperature (K). C and R are physical constants referring to the molar volume (22.41 L/mol) and universal gas constant (8.314 L kPa/K/mol), respectively.
The biogas compositions and volatile fatty acids (VFAs) contents were measured daily by a gas chromatograph (GC) system (7890B, Agilent, USA), and the detailed instruments were reported elsewhere (Li et al., 2020). The biodegradability (BD) was defined as the ratio of the experimental methane yield (EMY), namely, the highest CMY, divided by the TMY, as shown in Eq. (6):
Microbial Community Analysis
To identify the functional microbes and evolution of the microbial community during pretreatment and AD, the groups that possessed relatively higher methane yield after AD were selected to conduct the 16S rRNA high-throughput sequencing for the microbial agents, pretreated PW, and digestates after AD. The PCR reaction mixture (30 μl) consisted of 10 ng of extracted DNA, 0.5 μl of Taq DNA polymerase (5 U/μl), 5 μl of deoxyribonucleotide triphosphate (10 mM), and 10 pmol of each primer. 805R (5′-GACTACHVGGGTATCTAATCC-3′) and 341F (5′-15CTACGGGNGGCWGCAG-3′) were considered as the primer pair of amplification for the analysis of total bacteria (Wu et al., 2020). The identification of archaea contained two rounds. 340F (5′-15CCTAYGGGGYGCASCAG-3′) and 1000R (5′-GGCCATGCACYWCYTCTC-3′) were used for the first round of amplification, and 349F (5′-GYGCASCAGKCGMGAAW-3′) and 806R (5′-GGACTACVSGGGTATCTAAT-3′) were used for the second round (Wang et al., 2018).
The Cutadapt software was adopted for the amplicon sequences from the original sequenced fragments. The PEAR program with default settings was adopted for merging the high-quality paired-end reads. The reads abandoned from the raw sequencing data were shorter than 200 bp and contained ambiguous nucleotides, incorrect barcodes, or primers. The UCHIME program was introduced to remove the potential chimera and then cluster the remaining reads into operational taxonomic units (OTUs) with a minimum identity of 97%. The taxonomic assignment of OTUs was supported by the RDP Resource, SILVA rRNA database, and NCBI taxonomy database. The species diversity was estimated by Shannon index, while the species richness was reflected by Chao1 and ACE indexes, which were all calculated using MOTHUR. The relative abundance (RA) was defined as the ratio of the number of sequences affiliated with a taxonomic category to the total number of sequences per sample.
Statistical Analysis
The results are shown with the average value ± standard deviation and organized by Origin pro 2021 Learning Edition. Circos graphs of microbial community structure were generated by Circos Online1.
Results and Discussion
Variation in O2 and CO2 Concentrations During Pretreatment
Figure 2 exhibits the variation in O2 and CO2 concentrations in the digesters during the pretreatment with five microbial agents. The trends showed that regardless of the microbial agent used, CO2 concentration steadily increased at the beginning of pretreatment along with the consumption of O2. CO2 and O2 concentrations finally reached a stable state within 5 days. Besides, the greater amounts of O2 injected into digesters corresponded to higher CO2 production. Thus, it could be inferred that all of these agents contained various aerobic or facultative microbes that converted O2 into CO2 for their growth. More interestingly, at the same oxygen loading, the rates of O2 consumption and CO2 production in SI, SM, and DE groups were faster than those of CI and CM groups, and the time to exhaust O2 was 2 days, even for the highest oxygen loading groups (30 ml/gVS). The results indicated that the aerobic or facultative microbes in SI, SM, and DE groups were more active or abundant, which might possibly contribute to the higher hydrolysis rate.
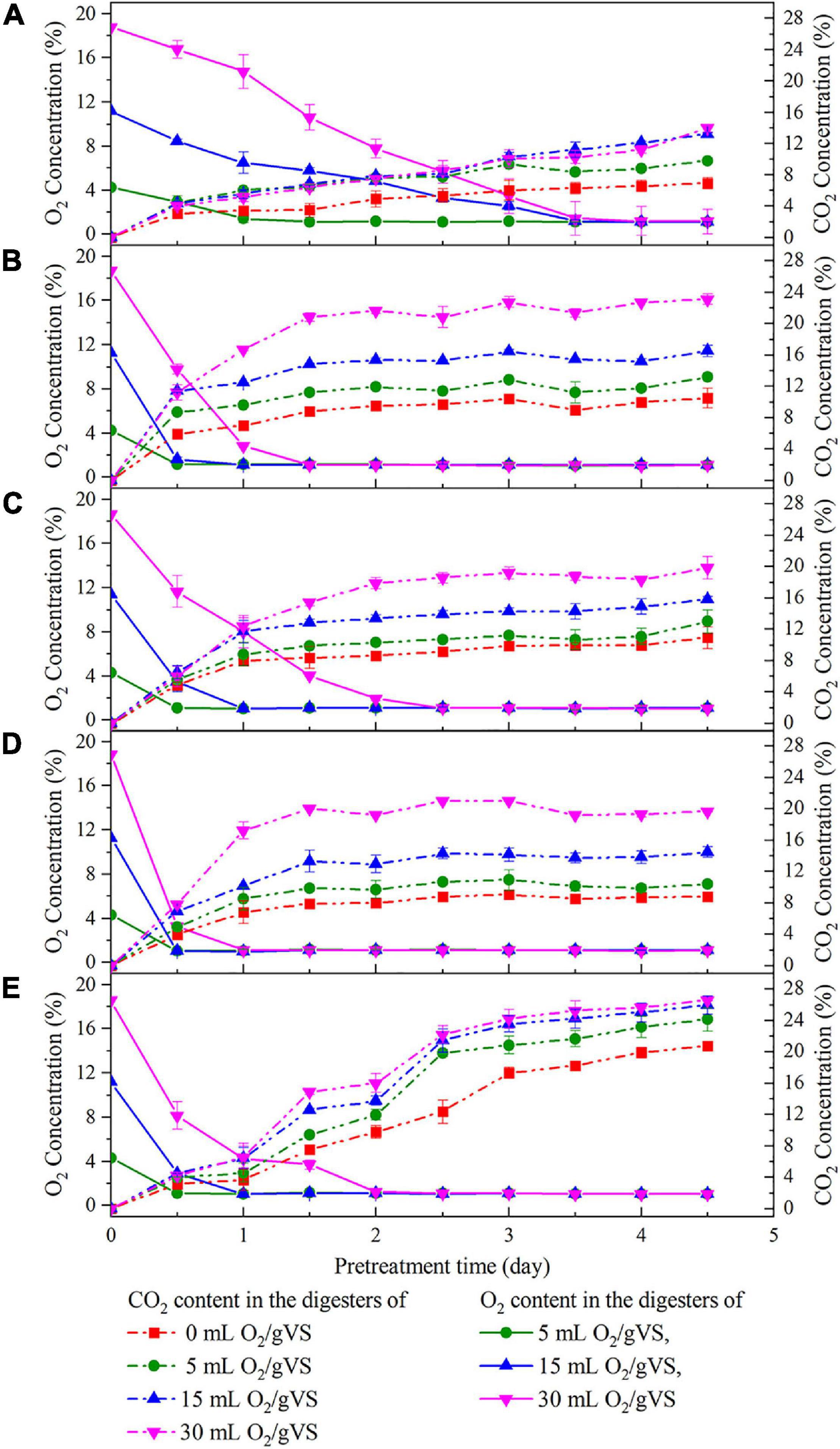
Figure 2. Changes of O2 and CO2 concentration in the digesters during the pretreatment by CI (A), SI (B), CM (C), SM (D), and DE (E).
Effect of Microbial Pretreatment on Daily Methane Yield
The trends in daily methane yield (DMY) of PW pretreated by different microbial agents are shown in Figure 3. The DMY of PW pretreated by CI exhibited an outstanding peak between the 5th and 10th day. Then, there was a swift decrease until another small peak appeared around the 35th day. However, the trends of DMY of PW pretreated by SI, CM, SM, and DE were quite different. There are two peaks observed: one was around the 5th day, and another was between the 10th and 20th days. This phenomenon was related to the accumulation of intermediate products (e.g., VFAs), which has already been reported by Li et al. (2020). The maximum DMY of untreated PW mixed with CI, SI, CM, SM, and DE were 15.1, 16.9, 22.0, 18.9, and 13.5 ml/gVS, respectively. The maximum DMY of PW pretreated by different microbial agents were observed in the groups of CI-0 (21.7 ml/gVS), SI-5 (26.8 ml/gVS), CM-15 (28.2 ml/gVS), SM-15 (26.0 ml/gVS), and DE-15 (39.4 ml/gVS), respectively, with the increase of 43.7, 58.6, 28.2, 37.6, and 191.9% compared with untreated, respectively. The noticeable improvement might be related to diverse microbes that degrade the organic matter to small molecules such as VFAs during the pretreatment.
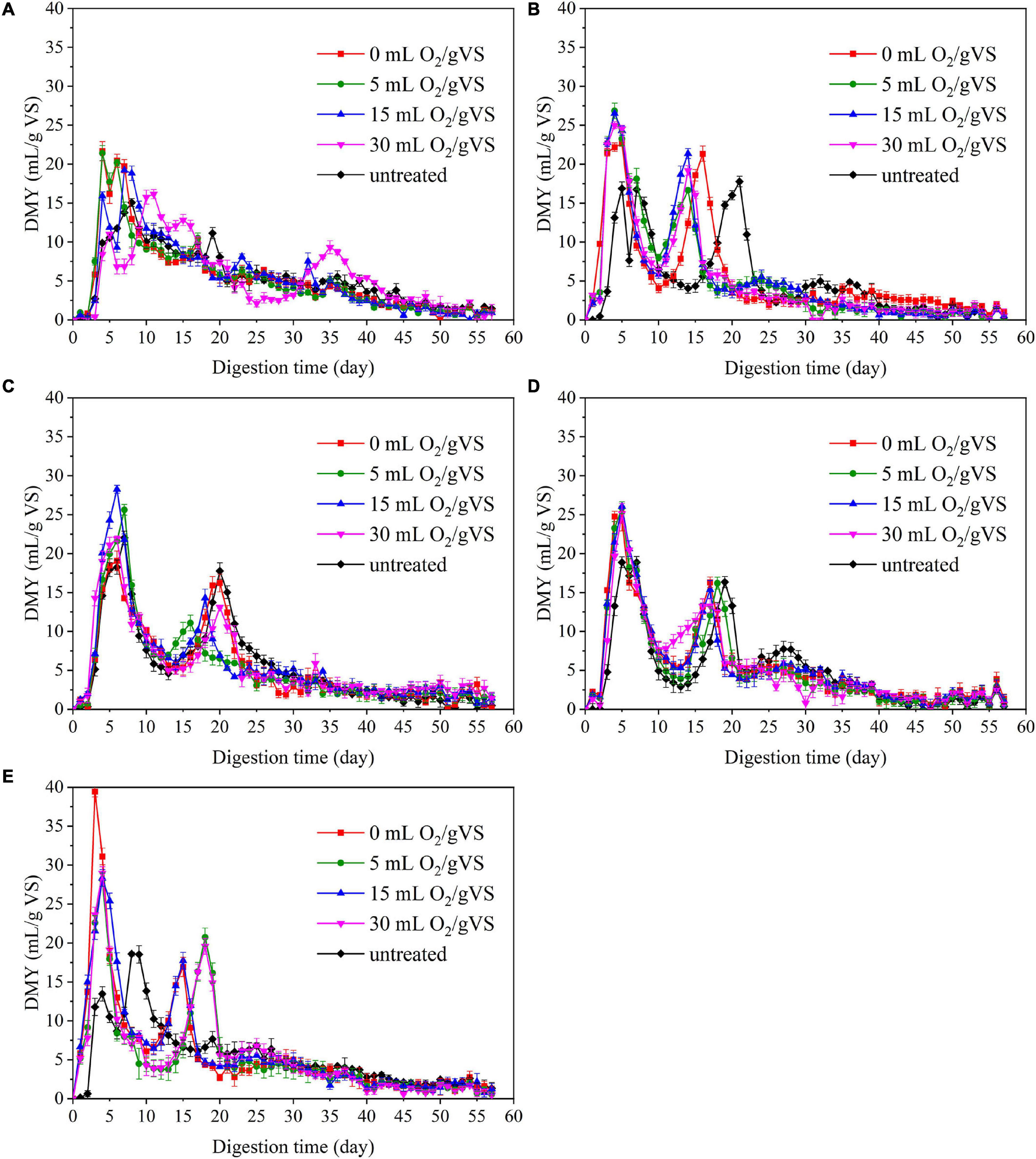
Figure 3. The daily methane yield (DMY) untreated and pretreated PW by CI (A), SI (B), CM (C), SM (D), and DE (E).
Effect of Microbial Pretreatment on Cumulative Methane Yield and Biodegradability
Figure 4 and Table 2 present the cumulative methane production of untreated and pretreated PW by microbial agents. Generally, the AD experiment can be divided into two stages by taking the 20th day as the dividing line. During the first 20 days of AD, when DMY remained relatively high, the increase of CMY of all groups was faster than those in the next 40 days. The CMY in the first 20 days (CMY20) of CI-UN, SI-UN, CM-UN, SM-UN, and DE-UN were 177.5, 168.1, 194.7, 173.0, and 181.1 ml/gVS, respectively. The enhanced CMY20 was achieved after microbial pretreatment. The CMY20 of PW in CI-0 was 195.3 ml/gVS, with the highest increase of 10.0% compared with the untreated. The maximum CMY20 of PW pretreated by CM was 222.0 ml/gVS in CM-15, with an increase of 14.0% compared with the untreated. However, when the oxygen loading increased to 30 ml/gVS, the CMY20 of PW pretreated by CI and CM gradually fell, with an increase of only 0.9 and 3.5%, respectively. Compared with CI and CM, the microbial pretreatments by SI, SM, and DE were more effective in enhancing methane yield at the early stage of AD. The maximum CMY20 of PW pretreated by SI, SM, and DE were 232.7 ml/gVS in SI-5, 219.6 ml/gVS in SM-30, and 235.8 ml/gVS in DE-15, with an increase of 38.4, 26.9, and 30.2% compared with untreated. Besides, it is found that changes in oxygen loading during the pretreatment of SI, SM, and DE had a slight influence on the improvement of CMY20.
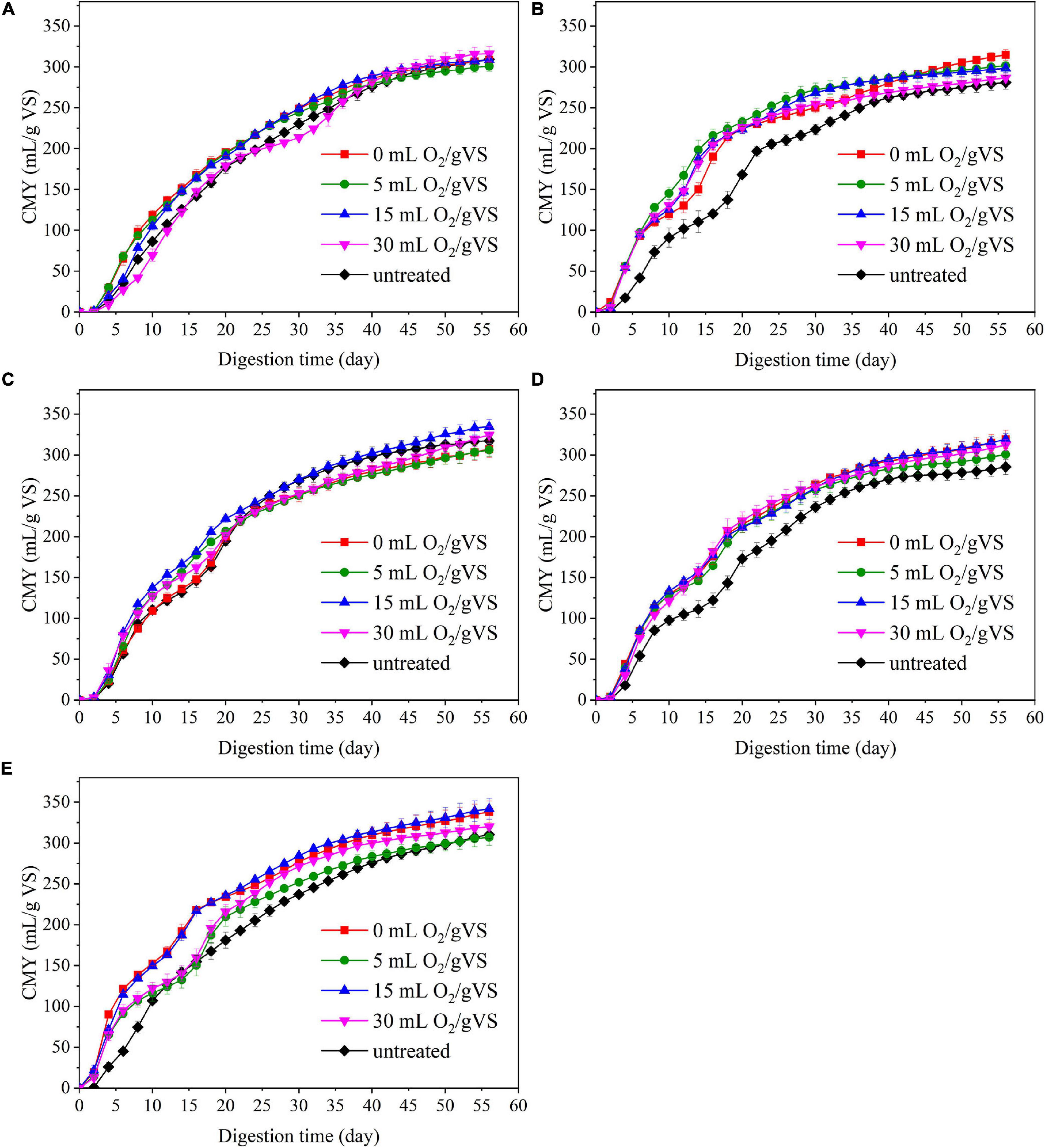
Figure 4. The cumulative methane yield (CMY) of untreated and pretreated PW by CI (A), SI (B), CM (C), SM (D), and DE (E).
When the AD process came to an end, the EMY of each group could be attained. The highest EMY of pretreated PW was 343.2 ml/gVS in DE-15, with an increase of 10.1% compared with the untreated. The maximum BD of 79.3% was also from this group. Table 3 provides a literature summary regarding the methane production of PW. It is clear that the organic loading in this study was the highest (47.7 gVS/L), followed by 25 gVS/L in the study of Yuan et al. (2014) and 15 gVS/L in that of Li et al. (2020). On the other hand, the methane yield of 343.2 ml/gVS in this study was higher than the estimated methane yield (315.4 ml/gVS) of untreated PW, which was the weighted sum of maximum methane yield of untreated corrugated paper (Krause et al., 2018) and tissue paper (Li et al., 2020) at a ratio of 1 to 1. These findings indicated that with the help of microaerobic pretreatment by DE, the AD of PW might be conducted at a smaller reactor with high organic loading and achieve satisfactory biomethanation efficiency. In addition, the maximum EMY of PW pretreated by other microbial agents were 316.9 ml/gVS in CI-30, 315.6 ml/gVS in SI-0, 336.0 ml/gVS in CM-15, and 320.6 ml/gVS in SM-0, increasing by 2.0, 12.3, 5.8, and 12.2% compared with untreated, respectively. It was noteworthy that the improvement in EMY was not as great compared with that of CMY20 for all groups except CI-30, demonstrating that microbial pretreatment tended to accelerate the methane production rate at the early stage of AD. Taken into account the amount or improvement of DMY, CMY20, and EMY, the anaerobic pretreatment or microaerobic pretreatment by SI, SM, and DE were attractive schemes for attaining better methane production performance of PW and deserved deeper investigation in the next sections.
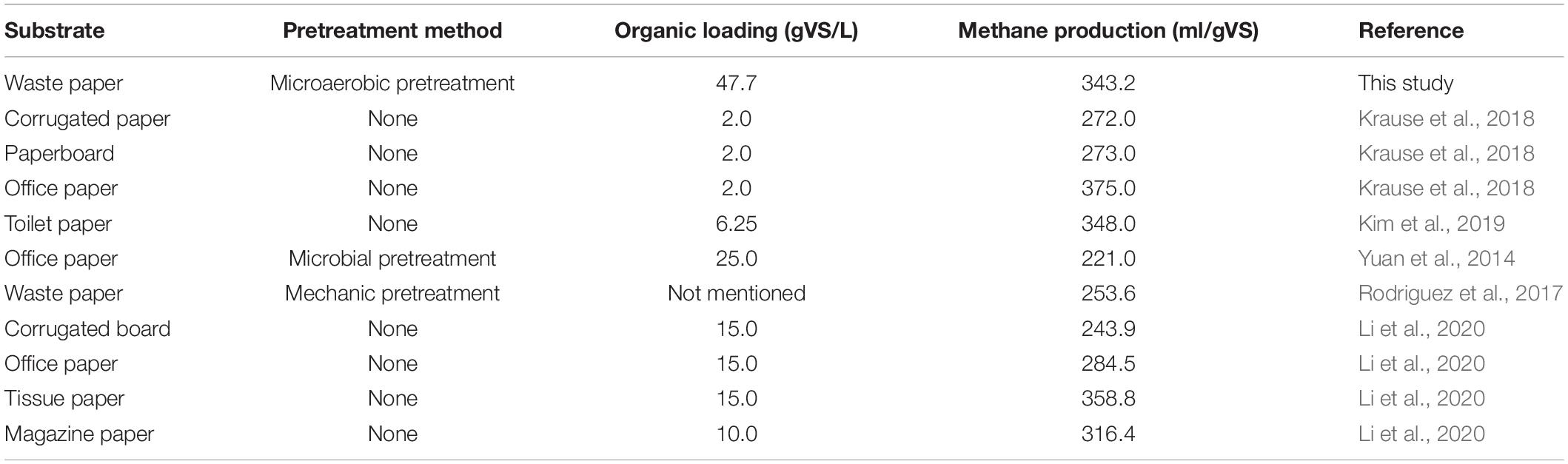
Table 3. Comparison of highest methane production of various paper wastes from reported literature and this study.
VFA Compositions After Microbial Pretreatment by SI, SM, and DE
VFAs represent a series of intermediate products generated from digestible substances and are precursors for methane production. The VFA compositions and concentrations in the anaerobic and microaerobic pretreatment groups by SI, SM, and DE are shown in Table 4. Evidently, the total acid concentration after the microaerobic pretreatment was higher than those of anaerobic pretreatment, indicating that a small amount of oxygen during the pretreatment could help microbes degrade PW into VFAs. At the same oxygen loading level, the pretreated groups by SI possessed the highest total acid concentration in comparison with the SM and DE, implying that SI might harbor more microbes that were efficient in the acidogenesis of PW. The composition of VFAs included various short-chain fatty acids, among which acetic acid took the highest percentage of total acids in all groups. The highest acetic acid concentration was 2846.7 mg/L in SI-0, followed by 2522.8 mg/L in SI-15 and 2456.6 mg/L in DE-15. Since acetic acid could be efficiently converted into methane by acetotrophic methanogens, its production during the pretreatment had positive effects on the AD performance of PW. By comparison, the groups with SM and DE possessed a relatively higher concentration of propionic acid, while n-butyric acid and isovaleric acid were abundant in SI and SM groups. The findings suggested that there existed many microbes in SI, SM, and DE capable of converting the substrates into different VFAs.
Microbial Community Analysis During Pretreatment and AD
Evolution of Microbial Community During Microbial Pretreatment by SI, SM, and DE
The overview of RA of predominant bacteria in SI, SM, DE, and pretreated PW is provided in Figure 5 and Supplementary Table S1. Staphylococcus and Bacillus were the predominant genera in SI, whose RAs were 51.42 and 9.07%, respectively. After the anaerobic pretreatment, the RA of Bacillus decreased slightly to 6.82%, while that of Staphylococcus decreased significantly to 31.52%. Besides, Clostridium sensu stricto 1 and Clostridium sensu stricto 10 appeared in SI-0 with the RAs of 25.10 and 15.27%, respectively. Members of Bacillus are aerobes or facultative anaerobes that produce cellulase, hemicellulase, ligninase, amylase, protease, and pectinase (Dastager et al., 2015; Yang et al., 2017; Xu et al., 2018). Therefore, this genus might result in the rapid consumption of oxygen during the pretreatment (as shown in Figure 2B) and induce cellulose and lignin degradation. Staphylococcus was regarded as a facultative anaerobic organism that could convert various sugars to organic acids (Supré et al., 2010; De Bel et al., 2013) and was possibly the main contributor to anaerobic pretreatment by SI. Clostridium sensu stricto 1 and Clostridium sensu stricto 10 accounted for a large proportion in SI-0. Clostridium included a variety of bacteria that specialized in utilizing multiple sugars as carbon and energy sources to generate methanogenic precursors such as acetic acid, butyric acid, H2, and CO2 (Lanjekar et al., 2015; Amin et al., 2021). Compared with SI-UN, the higher RA of Clostridium in SI-0 might contribute to the acceleration of methane production at the early stage, as shown in Figure 4B. The SM and DE also contained various hydrolysis and acidogenesis bacteria. The RAs of Bacteroides and Macellibacteroides in SM were 0.63 and 0.15%, respectively, but increased by dozens and hundreds of times in SM-15, respectively. Many bacteria in these genera could convert cellulose into various VFAs (Jabari et al., 2012; Sakamoto and Ohkuma, 2013; Hatamoto et al., 2014). The RAs of Proteiniphilum in SM and DE were 0.08 and 1.24%, respectively, but significantly increased after microbial pretreatment. It was reported that Proteiniphilum functioned by converting the proteins and pyruvate into acetate and propionate (Chen and Dong, 2005). Noticeably, Acinetobacter was abundant in SM (15.87%) and SM-15 (31.16%). Most bacteria from this genus were aerobic, and some were associated with lignin degradation (Radolfova-Krizova et al., 2016; Yang et al., 2017), contributing to the rapid oxygen consumption (shown in Figure 2D) and higher methane production of pretreated PW.
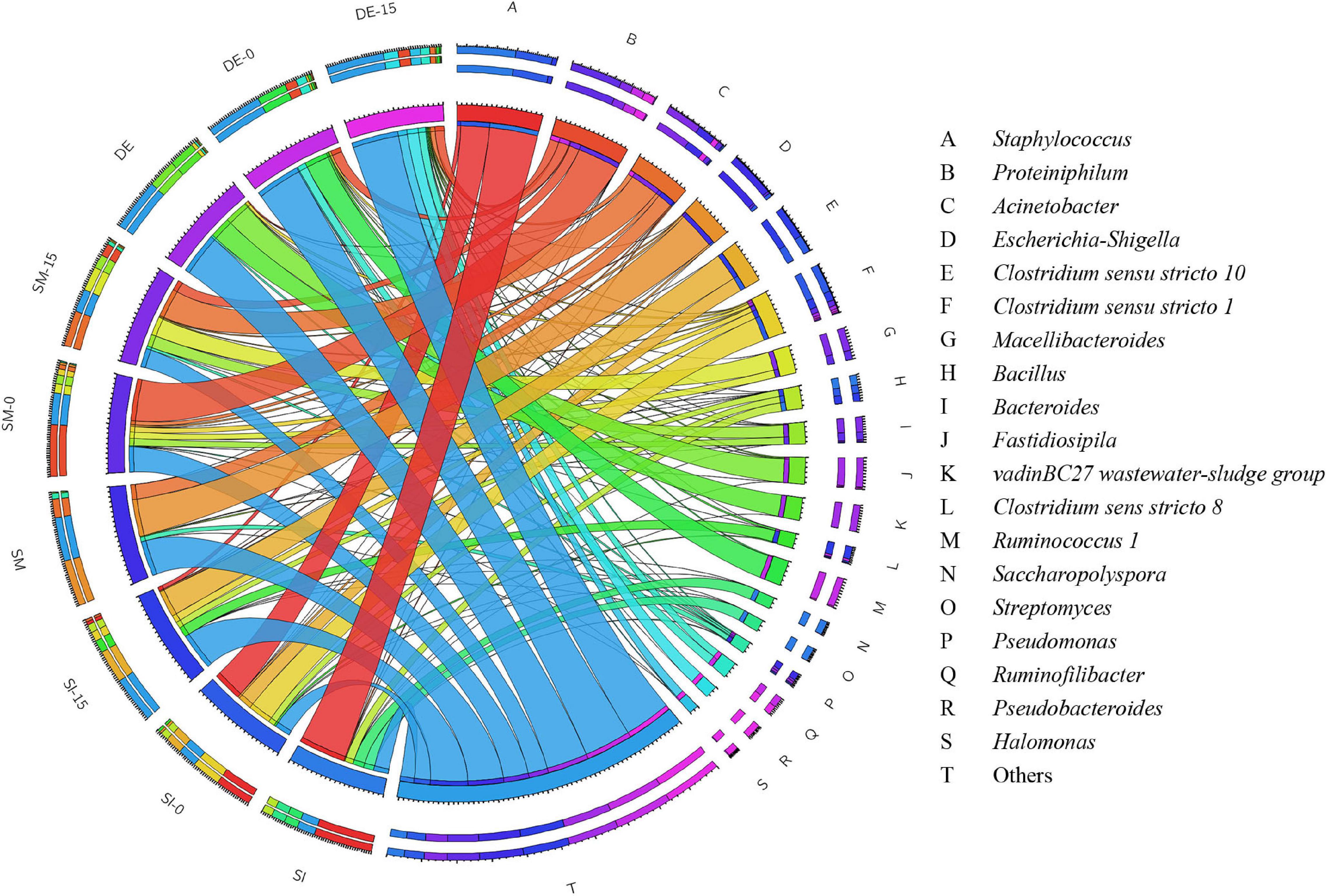
Figure 5. The relative abundance of bacteria in the SI, SM, DE, and pretreated PW at the genus level.
Changes of Microbial Community After AD
Table 5 provides information regarding the species diversity and richness of bacteria and archaea in selected groups after AD, including sequence number, OTU number, ACE, Chao1, Shannon, and Simpson indexes. After the pretreatment by SI, the highest sequence number, OTU number, Shannon, ACE, and Chao1 indexes of bacteria in bacteria were achieved in SI-15, followed by SI-UN and SI-0. However, the observed outcomes in the groups of SM were quite different. These indexes of bacteria in SM-UN were the highest, followed by SM-0 and SM-15. A possible explanation for this result might be that the vulnerable bacteria in SM gradually disappeared due to the changes in the environment in the digester. The Shannon, ACE, and Chao1 indexes of bacteria in DE-0 and DE-15 showed a minor difference with those of DE-UN, indicating that many bacteria in the digestate were not susceptible to interference during the pretreatment and AD. The variations of species diversity and richness of archaea were quite different from those of bacteria. For SI and DE, the sequence number of archaea in the groups of anaerobic pretreatments higher than the untreated group and microaerobic pretreatment group. Besides, the sequence number and OTU number of archaea in SM-0 was higher than that in SM-15. It could be inferred that the anaerobic pretreatment was beneficial for methanogens to keep their activity during the AD.
Figure 6 and Supplementary Table S2 exhibit the overview of abundant bacteria in selected groups after AD. Evidently, the microbial community in each digester was significantly changed. Some remarkable bacteria for pretreatment, like Proteiniphilum, Bacillus, and Staphylococcus, were nearly absent, while VadinBC27 and Ruminococcaceae Incertae Sedis appeared as the predominant bacteria in all digesters. From previous studies, VadinBC27 was recognized as degrading amino acids in syntrophic association with hydrogenotrophic methanogens (Li et al., 2015). Ruminococcaceae Incertae Sedis was previously identified as a functional bacterium associated with cellulose and hemicellulose degradation (Ecem Öner et al., 2018). Therefore, the microbial community containing these bacteria enabled the enhancement of methane production. Clostridium sensu stricto 1 was another primary bacterium in the digesters with SI. The RA of Clostridium sensu stricto 1 in SI-0 was 16.23%, with an increase of 58.6% compared with SI-UN. Noticeably, Clostridium sensu stricto 1 took up a large part after the pretreatment and possessed high abundance after AD. These results suggested that it was adaptive to the environment in digesters and might play critical roles in both microbial pretreatment and AD. Besides, Fastidiosipila and Caldicoprobacter were also observed in the digesters with SM and DE after AD. The former was able to produce acetate and butyrate from carbohydrates and protein (Falsen et al., 2005), and the latter could metabolize cellulose and hemicellulose into oligosaccharides or monosaccharides (Zhou et al., 2018). Aside from the predominant bacteria mentioned above, others detected in these digestates include Sedimentibacter, Syntrophomonas, Owenweeksia, and Petrimonas. The RAs of Sedimentibacter in SI-0 and SI-15 were 3.70 and 3.89%, respectively, with an increase of 30.0 and 36.9% compared with the untreated (2.84%). It has been reported that Sedimentibacter was identified as a strict anaerobe that could degrade amino acids into ethanol and organic acids (Lechner, 2015; Imachi et al., 2016). Syntrophomonas facilitates the conversion of butyric acid into acetate, propionate, and H2 under methanogenic conditions (Sekiguchi, 2015), and its RA ranged between 1.54 and 5.41% in the digestates we collected. The RA of Petrimonas experienced a minor difference in the groups of SM (1.67–2.47%) and DE (3.69–4.23%). This genus was an important participant during AD as it could ferment many sugars and organic acids into methane production precursors like acetate, H2, and CO2 (Grabowski et al., 2005).
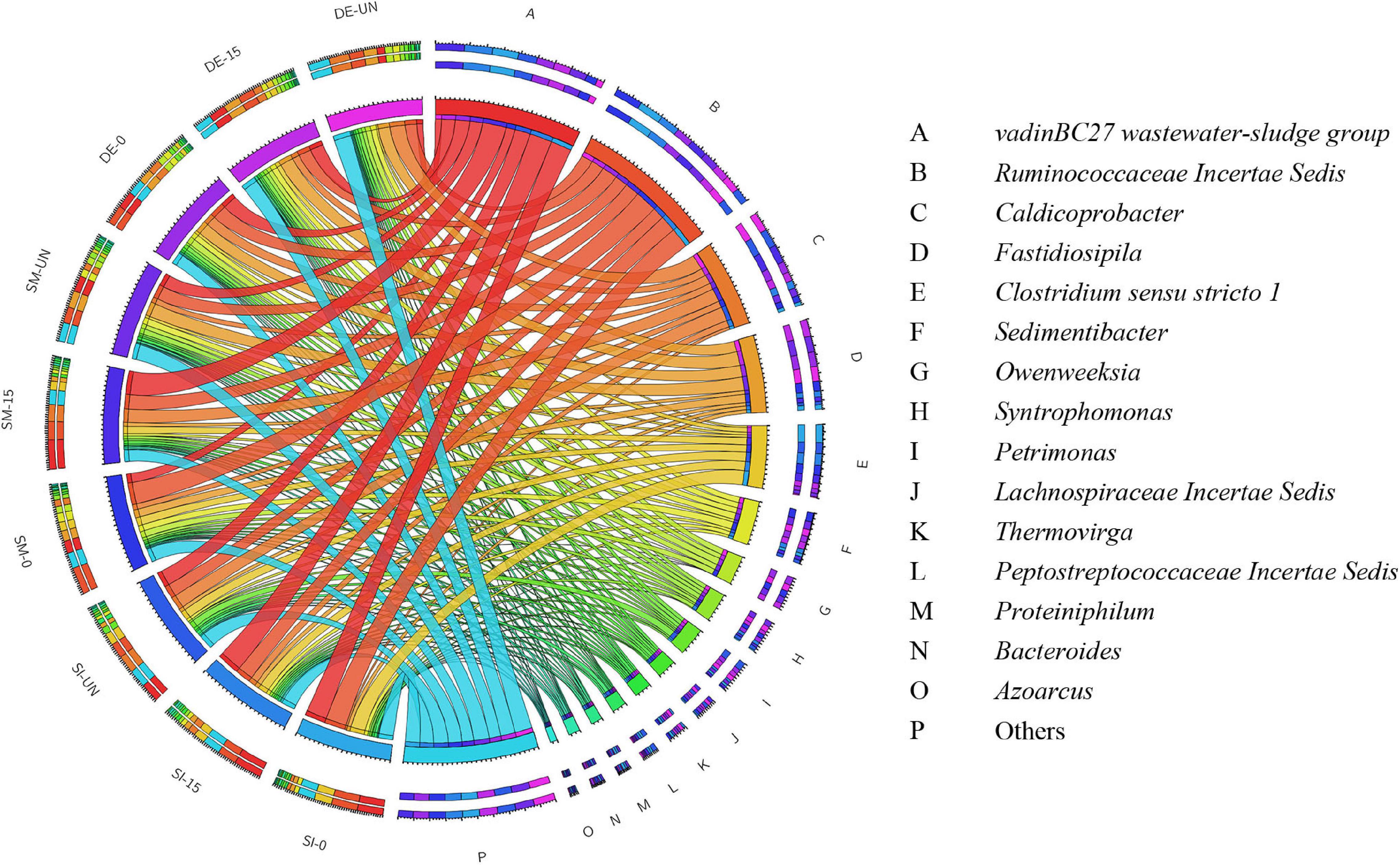
Figure 6. The relative abundance of bacteria in the selected digestates after AD at the genus level.
The community structures of archaea in selected groups after AD are presented in Figure 7 and Supplementary Table S3. Obviously, Methanosarcina, and Methanosaeta were the main methanogens in all groups regardless of microbial agents and oxygen loading for pretreatment. By comparing these two genera in the same microbial agent groups, it could be found that the groups with anaerobic pretreatment possessed the highest RA of Methanosarcina, followed by those with microaerobic pretreatment and without pretreatment, while the RA of Methanosaeta showed opposite trends. For example, the RAs of Methanosarcina and Methanosaeta in SM-UN were 59.13 and 31.60%, respectively. Methanosarcina significantly increased to 62.51 and 75.53% in SM-15 and SM-0, respectively, while the Methanosaeta decreased to 28.27 and 21.33%. Methanosarcina is known as the only methanogen compatible with the acetoclastic and hydrogenotrophic methanogenesis routes, while Methanosaeta has the ability to follow the acetoclastic pathway only (Zakaria and Dhar, 2019). The shift between Methanosarcina and Methanosaeta might probably result from the changes of precursors produced by the pretreatment with different microbial agents and oxygen loading and consequently bring about better performance of methane production of PW.
Conclusion
This study was designed to investigate the methane production performance of PW subjected to anaerobic and microaerobic pretreatments by five microbial agents, namely, CI, SI, CM, SM, and DE. Results showed the diverse efficacy of these microbial agents and oxygen loadings on the methane production performance of PW. The EMY of pretreated PW was enhanced by the pretreatments of SI, SM, and DE under an optimal oxygen loading. The microbial community analysis revealed that microbial agents provided functional bacteria for enhancing the hydrolytic and acidogenic process, which exhibited a different response to the oxygen loading during the anaerobic and microaerobic pretreatment. Besides, the anaerobic and microaerobic pretreatment had a profound effect on the microbial community in AD digesters, leading to the enhanced methane production performance. The work not only provided a promising technique to make full use of PW but also gave a reference for further studies on biodegradation mechanisms of lignocellulosic biowastes during the microbial pretreatment at various oxygen concentrations and AD processes.
Data Availability Statement
The raw data supporting the conclusions of this article will be made available by the authors, without undue reservation.
Author Contributions
CS and WL conducted the experiment and wrote the original draft. FC performed the statistical analysis. GL provided the resources for this experiment. CC contributed to the conception and supervision of this study. All authors contributed to manuscript revision, read, and approved the submitted version.
Conflict of Interest
The authors declare that the research was conducted in the absence of any commercial or financial relationships that could be construed as a potential conflict of interest.
Supplementary Material
The Supplementary Material for this article can be found online at: https://www.frontiersin.org/articles/10.3389/fmicb.2021.688290/full#supplementary-material
Footnotes
References
Abraham, A., Mathew, A. K., Park, H., Choi, O., Sindhu, R., Parameswaran, B., et al. (2020). Pretreatment strategies for enhanced biogas production from lignocellulosic biomass. Bioresour. Technol. 301:122725. doi: 10.1016/j.biortech.2019.122725
Ali, S. S., Al-Tohamy, R., Manni, A., Luz, F. C., Elsamahy, T., and Sun, J. (2019). Enhanced digestion of bio-pretreated sawdust using a novel bacterial consortium: microbial community structure and methane-producing pathways. Fuel 254:115604. doi: 10.1016/j.fuel.2019.06.012
Amin, F. R., Khalid, H., El-Mashad, H. M., Chen, C., Liu, G., and Zhang, R. (2021). Functions of bacteria and archaea participating in the bioconversion of organic waste for methane production. Sci. Total Environ. 763:143007. doi: 10.1016/j.scitotenv.2020.143007
Amin, F. R., Khalid, H., Zhang, H., Rahman, S., Zhang, R., Liu, G., et al. (2017). Pretreatment methods of lignocellulosic biomass for anaerobic digestion. AMB Exp. 7:72. doi: 10.1186/s13568-017-0375-4
APHA (2005). Standard Methods for the Examination of Water and Wastewater, 21 Edn. Washington, DC: American Public Health Association.
Bajpai, P. (2014). “Uses of recovered paper other than papermaking,” in Recycling and Deinking of Recovered Paper, ed. P. Bajpai (Oxford: Elsevier), 283–295. doi: 10.1016/b978-0-12-416998-2.00016-7
Carrere, H., Antonopoulou, G., Affes, R., Passos, F., Battimelli, A., Lyberatos, G., et al. (2016). Review of feedstock pretreatment strategies for improved anaerobic digestion: From lab-scale research to full-scale application. Bioresour. Technol. 199, 386–397. doi: 10.1016/j.biortech.2015.09.007
Chen, S., and Dong, X. (2005). Proteiniphilum acetatigenes gen. nov., sp. nov., from a UASB reactor treating brewery wastewater. Int. J. Syst. Evol. Microbiol. 55, 2257–2261. doi: 10.1099/ijs.0.63807-0
Dastager, S. G., Mawlankar, R., Mual, P., Verma, A., Krishnamurthi, S., Joseph, N., et al. (2015). Bacillus encimensis sp. nov. isolated from marine sediment. Int. J. Syst. Evol. Microbiol. 65, 1421–1425. doi: 10.1099/ijs.0.000114
De Bel, A., Van Hoorde, K., Wybo, I., Vandoorslaer, K., Echahidi, F., De Brandt, E., et al. (2013). Staphylococcus jettensis sp. nov., a coagulasenegative staphylococcal species isolated from human clinical specimens. Int. J. Syst. Evol. Microbiol. 63, 3250–3256. doi: 10.1099/ijs.0.044438-0
Den, W., Sharma, V. K., Lee, M., Nadadur, G., and Varma, R. S. (2018). Lignocellulosic biomass transformations via greener oxidative pretreatment processes: access to energy and value added chemicals. Front. Chem. 6:141. doi: 10.3389/fchem.2018.00141
Ecem Öner, B., Akyol, Ç, Bozan, M., Ince, O., Aydin, S., and Ince, B. (2018). Bioaugmentation with Clostridium thermocellum to enhance the anaerobic biodegradation of lignocellulosic agricultural residues. Bioresour. Technol. 249, 620–625. doi: 10.1016/j.biortech.2017.10.040
Falsen, E., Collins, M. D., Welinder-Olsson, C., Song, Y., Finegold, S. M., and Lawson, P. A. (2005). Fastidiosipila sanguinis gen. nov., sp. nov., a new Gram-positive, coccus-shaped organism from human blood. Int. J. Syst. Evol. Microbiol. 55, 853–858. doi: 10.1099/ijs.0.63327-0
Fu, S., Wang, F., Shi, X., and Guo, R. (2016). Impacts of microaeration on the anaerobic digestion of corn straw and the microbial community structure. Chem. Eng. J. 287, 523–528. doi: 10.1016/j.cej.2015.11.070
Grabowski, A., Tindall, B. J., Bardin, V., Blanchet, D., and Jeanthon, C. (2005). Petrimonas sulfuriphila gen. nov., sp. nov., a mesophilic fermentative bacterium isolated from a biodegraded oil reservoir. Int. J. Syst. Evol. Microbiol. 55, 1113–1121. doi: 10.1099/ijs.0.63426-0
Hatamoto, M., Kaneshige, M., Nakamura, A., and Yamaguchi, T. (2014). Bacteroides luti sp. nov., an anaerobic, cellulolytic and xylanolytic bacterium isolated from methanogenic sludge. Int. J. Syst. Evol. Microbiol. 64, 1770–1774. doi: 10.1099/ijs.0.056630-0
He, S., Fan, X., Luo, S., Katukuri, N. R., and Guo, R. (2017). Enhanced the energy outcomes from microalgal biomass by the novel biopretreatment. Energy Convers. Manag. 135, 291–296. doi: 10.1016/j.enconman.2016.12.049
Imachi, H., Sakai, S., Kubota, T., Miyazaki, M., Saito, Y., and Takai, K. (2016). Sedimentibacter acidaminivorans sp. nov., an anaerobic, amino-acid-utilizing bacterium isolated from marine subsurface sediment. Int. J. Syst. Evol. Microbiol. 66, 1293–1300. doi: 10.1099/ijsem.0.000878
Jabari, L., Gannoun, H., Cayol, J. L., Hedi, A., Sakamoto, M., Falsen, E., et al. (2012). Macellibacteroides fermentans gen. nov., sp. nov., a member of the family Porphyromonadaceae isolated from an upflow anaerobic filter treating abattoir wastewaters. Int. J. Syst. Evol. Microbiol. 62, 2522–2527. doi: 10.1099/ijs.0.032508-0
Kainthola, J., Kalamdhad, A. S., Goud, V. V., and Goel, R. (2019). Fungal pretreatment and associated kinetics of rice straw hydrolysis to accelerate methane yield from anaerobic digestion. Bioresour. Technol. 286:121368. doi: 10.1016/j.biortech.2019.121368
Kim, J., Kim, J., and Lee, C. (2019). Anaerobic co-digestion of food waste, human feces, and toilet paper: methane potential and synergistic effect. Fuel 248, 189–195. doi: 10.1016/j.fuel.2019.03.081
Krause, M. J., Chickering, G. W., Townsend, T. G., and Pullammanappallil, P. (2018). Effects of temperature and particle size on the biochemical methane potential of municipal solid waste components. Waste Manag. 71, 25–30. doi: 10.1016/j.wasman.2017.11.015
Lanjekar, V. B., Marathe, N. P., Shouche, Y. S., and Ranade, D. R. (2015). Clostridium punense sp. Nov., an obligate anaerobe isolated from healthy human faeces. Int. J. Syst. Evol. Microbiol. 65, 4749–4756. doi: 10.1099/ijsem.0.000644
Lechner, U. (2015). “Sedimentibacter,” in Bergey’s Manual of Systematics of Archaea and Bacteria, ed. W. B. Whitman (New York, NY: John Wiley & Sons, Ltd.), 1–7. doi: 10.1002/9781118960608.gbm00718
Lee, J. K., Patel, S. K. S., Sung, B. H., and Kalia, V. C. (2020). Biomolecules from municipal and food industry wastes: an overview. Bioresour. Technol. 298:122346. doi: 10.1016/j.biortech.2019.122346
Li, L., He, Q., Ma, Y., Wang, X., and Peng, X. (2015). Dynamics of microbial community in a mesophilic anaerobic digester treating food waste: relationship between community structure and process stability. Bioresour. Technol. 189, 113–120. doi: 10.1016/j.biortech.2015.04.015
Li, W., Khalid, H., Amin, F. R., Zhang, H., Dai, Z., Chen, C., et al. (2020). Biomethane production characteristics, kinetic analysis, and energy potential of different paper wastes in anaerobic digestion. Renew. Energy 157, 1081–1088. doi: 10.1016/j.renene.2020.04.035
Li, W., Khalid, H., Zhu, Z., Zhang, R., Liu, G., Chen, C., et al. (2018). Methane production through anaerobic digestion: participation and digestion characteristics of cellulose, hemicellulose and lignin. Appl. Energy 226, 1219-1228. doi: 10.1016/j.apenergy.2018.05.055
Lim, J. W., and Wang, J.-Y. (2013). Enhanced hydrolysis and methane yield by applying microaeration pretreatment to the anaerobic co-digestion of brown water and food waste. Waste Manag. 33, 813–819. doi: 10.1016/j.wasman.2012.11.013
Ma, S., Zhou, C., Chi, C., Liu, Y., and Yang, G. (2020). Estimating physical composition of municipal solid waste in China by applying artificial neural network method. Environ. Sci. Technol. 54, 9609–9617. doi: 10.1021/acs.est.0c01802
Manfredi, S., Tonini, D., and Christensen, T. H. (2011). Environmental assessment of different management options for individual waste fractions by means of life-cycle assessment modelling. Resour. Conserv. Recycl. 55, 995–1004. doi: 10.1016/j.resconrec.2011.05.009
NationMaster (2021). Top Countries for Paperboard and Packaging Paper Production. NationMaster. Available online at: https://www.nationmaster.com/nmx/ranking/paperboard-and-packaging-paper-production (accessed May 3, 2021).
Ning, Z., Zhang, H., Li, W., Zhang, R., Liu, G., and Chen, C. (2018). Anaerobic digestion of lipid-rich swine slaughterhouse waste: methane production performance, long-chain fatty acids profile and predominant microorganisms. Bioresour. Technol. 269, 426–433. doi: 10.1016/j.biortech.2018.08.001
Patel, S. K. S., Kumar, P., Singh, M., Lee, J. K., and Kalia, V. C. (2015). Integrative approach to produce hydrogen and polyhydroxybutyrate from biowaste using defined bacterial cultures. Bioresour. Technol. 176, 136–141. doi: 10.1016/j.biortech.2014.11.029
Radolfova-Krizova, L., Maixnerova, M., and Nemec, A. (2016). Acinetobacter pragensis sp. nov., found in soil and water ecosystems. Int. J. Syst. Evol. Microbiol. 66, 3897–3903. doi: 10.1099/ijsem.0.001285
Rincón, B., Heaven, S., Banks, C. J., and Zhang, Y. (2012). Anaerobic digestion of whole-crop winter wheat silage for renewable energy production. Energy Fuels 26, 2357–2364. doi: 10.1021/ef201985x
Rodriguez, C., Alaswad, A., El-Hassan, Z., and Olabi, A. G. (2017). Mechanical pretreatment of waste paper for biogas production. Waste Manag. 68, 157–164. doi: 10.1016/j.wasman.2017.06.040
Ruan, D., Zhou, Z., Pang, H., Yao, J., Chen, G., and Qiu, Z. (2019). Enhancing methane production of anaerobic sludge digestion by microaeration: enzyme activity stimulation, semi-continuous reactor validation and microbial community analysis. Bioresour. Technol. 289:121643. doi: 10.1016/j.biortech.2019.121643
Sakamoto, M., and Ohkuma, M. (2013). Bacteroides reticulotermitis sp. nov., isolated from the gut of a subterranean termite (Reticulitermes speratus). Int. J. Syst. Evol. Microbiol. 63, 691–695. doi: 10.1099/ijs.0.040931-0
Sanscartier, D., MacLean, H. L., and Saville, B. (2012). Electricity production from anaerobic digestion of household organic waste in Ontario: techno-economic and GHG emission analyses. Environ. Sci. Technol. 46, 1233–1242. doi: 10.1021/es2016268
Sekiguchi, Y. (2015). “Syntrophomonas,” in Bergey’s Manual of Systematics of Archaea and Bacteria, ed. W. B. Whitman (New York, NY: John Wiley & Sons, Ltd.), 1–11. doi: 10.1002/9781118960608.gbm00682
Supré, K., De Vliegher, S., Cleenwerck, I., Engelbeen, K., Van Trappen, S., Piepers, S., et al. (2010). Staphylococcus devriesei sp. nov., isolated from teat apices and milk of dairy cows. Int. J. Syst. Evol. Microbiol. 60, 2739–2744. doi: 10.1099/ijs.0.015982-0
Van Soest, P. J., Robertson, J. B., and Lewis, B. A. (1991). Methods for dietary fiber, neutral detergent fiber, and nonstarch polysaccharides in relation to animal nutrition. J. Dairy Sci. 74, 3583–3597. doi: 10.3168/jds.S0022-0302(91)78551-2
Wang, C., Zhang, J., Hu, F., Zhang, S., Lu, J., and Liu, S. (2020). Bio-pretreatment promote hydrolysis and acidification of oilseed rape straw: roles of fermentation broth and micro-oxygen. Bioresour. Technol. 308:123272. doi: 10.1016/j.biortech.2020.123272
Wang, G., Li, Q., Gao, X., and Wang, X. C. (2018). Synergetic promotion of syntrophic methane production from anaerobic digestion of complex organic wastes by biochar: performance and associated mechanisms. Bioresour. Technol. 250, 812–820. doi: 10.1016/j.biortech.2017.12.004
Wang, L., Zhang, H., Dai, Z., Liu, Y., Chen, C., and Liu, G. (2021). Effect of nicotine inhibition on anaerobic digestion and the co-digestion performance of tobacco stalks with different animal manures. Process Saf. Environ. Prot. 146, 377–382. doi: 10.1016/j.psep.2020.09.005
Wu, Y., Wang, L., Jin, M., and Zhang, K. (2020). Simultaneous copper removal and electricity production and microbial community in microbial fuel cells with different cathode catalysts. Bioresour. Technol. 305:123166. doi: 10.1016/j.biortech.2020.123166
Xu, W., Fu, S., Yang, Z., Lu, J., and Guo, R. (2018). Improved methane production from corn straw by microaerobic pretreatment with a pure bacteria system. Bioresour. Technol. 259, 18–23. doi: 10.1016/j.biortech.2018.02.046
Yang, C. X., Wang, T., Gao, L. N., Yin, H. J., and Lü, X. (2017). Isolation, identification and characterization of lignin-degrading bacteria from Qinling. China. J. Appl. Microbiol. 123, 1447–1460. doi: 10.1111/jam.13562
Yuan, X., Wen, B., Ma, X., Zhu, W., Wang, X., Chen, S., et al. (2014). Enhancing the anaerobic digestion of lignocellulose of municipal solid waste using a microbial pretreatment method. Bioresour. Technol. 154, 1–9. doi: 10.1016/j.biortech.2013.11.090
Zakaria, B. S., and Dhar, B. R. (2019). Progress towards catalyzing electro-methanogenesis in anaerobic digestion process: fundamentals, process optimization, design and scale-up considerations. Bioresour. Technol. 289:121738. doi: 10.1016/j.biortech.2019.121738
Zhao, C., Zhang, R., He, Y., Wang, W., Chen, C., Liu, G., et al. (2017). Maximization of the methane production from durian shell during anaerobic digestion. Bioresour. Technol. 238, 433–438. doi: 10.1016/j.biortech.2017.03.184
Zhao, H. L., Liu, F., Liu, H. Q., Wang, L., Zhang, R., and Hao, Y. (2020). Comparative life cycle assessment of two ceramsite production technologies for reusing municipal solid waste incinerator fly ash in China. Waste Manag 113, 447–455. doi: 10.1016/j.wasman.2020.06.016
Zhao, Y., Xu, C., Ai, S., Wang, H., Gao, Y., Yan, L., et al. (2019). Biological pretreatment enhances the activity of functional microorganisms and the ability of methanogenesis during anaerobic digestion. Bioresour. Technol. 290:121660. doi: 10.1016/j.biortech.2019.121660
Zhen, F., Luo, X., Xing, T., Sun, Y., Kong, X., and Li, W. (2021). Performance evaluation and microbial community analysis of microaerobic pretreatment on thermophilic dry anaerobic digestion. Biochem. Eng. J. 167, 107873. doi: 10.1016/j.bej.2020.107873
Zhen, X., Zhang, X., Li, S., Li, M., and Kang, J. (2020). Effect of micro-oxygen pretreatment on gas production characteristics of anaerobic digestion of kitchen waste. J. Mater. Cycles Waste Manag. 22, 1852–1858. doi: 10.1007/s10163-020-01072-9
Zhou, Y., Xu, Z., Zhao, M., Shi, W., Huang, Z., He, D., et al. (2018). Construction and evaluation of efficient solid-state anaerobic digestion system via vinegar residue. Int. Biodeterior. Biodegrad. 133, 142–150. doi: 10.1016/j.ibiod.2018.06.020
Keywords: paper waste, anaerobic pretreatment, microaerobic pretreatment, microbial community analysis, anaerobic digestion
Citation: Song C, Li W, Cai F, Liu G and Chen C (2021) Anaerobic and Microaerobic Pretreatment for Improving Methane Production From Paper Waste in Anaerobic Digestion. Front. Microbiol. 12:688290. doi: 10.3389/fmicb.2021.688290
Received: 30 March 2021; Accepted: 18 May 2021;
Published: 06 July 2021.
Edited by:
Yi Zheng, Kansas State University, United StatesReviewed by:
Sanjay Kumar Singh Patel, Konkuk University, South KoreaVivekanand Vivekanand, Malaviya National Institute of Technology, India
Copyright © 2021 Song, Li, Cai, Liu and Chen. This is an open-access article distributed under the terms of the Creative Commons Attribution License (CC BY). The use, distribution or reproduction in other forums is permitted, provided the original author(s) and the copyright owner(s) are credited and that the original publication in this journal is cited, in accordance with accepted academic practice. No use, distribution or reproduction is permitted which does not comply with these terms.
*Correspondence: Chang Chen, Y2hlbmNoYW5nQG1haWwuYnVjdC5lZHUuY24=
†These authors have contributed equally to this work