- 1Council for Agricultural Research and Economics, Research Centre for Agriculture and Environment, Rome, Italy
- 2National Research Institute of Horticulture, Skierniewice, Poland
- 3Council for Agricultural Research and Economics, Research Centre for Viticulture and Enology, Conegliano, Italy
- 4Council for Agricultural Research and Analysis of the Agricultural Economy, Research Centre for Engineering and Agro-Food Processing, Monterotondo, Italy
- 5Institute for Biological Systems, Council of National Research of Italy (CNR), Rome, Italy
- 6Life Sciences Department, Natural History Museum, London, United Kingdom
Microorganisms promised to lead the bio-based revolution for a more sustainable agriculture. Beneficial microorganisms could be a valid alternative to the use of chemical fertilizers or pesticides. However, the increasing use of microbial inoculants is also raising several questions about their efficacy and their effects on the autochthonous soil microorganisms. There are two major issues on the application of bioinoculants to soil: (i) their detection in soil, and the analysis of their persistence and fate; (ii) the monitoring of the impact of the introduced bioinoculant on native soil microbial communities. This review explores the strategies and methods that can be applied to the detection of microbial inoculants and to soil monitoring. The discussion includes a comprehensive critical assessment of the available tools, based on morpho-phenological, molecular, and microscopic analyses. The prospects for future development of protocols for regulatory or commercial purposes are also discussed, underlining the need for a multi-method (polyphasic) approach to ensure the necessary level of discrimination required to track and monitor bioinoculants in soil.
Introduction
Microbial-based products (also named bioinoculants, biofertilisers, and biopesticides) used to support plant nutrition and protection from abiotic and biotic stress have received considerable attention in the last decades by researchers, manufacturers and farmers, particularly because they help to reduce the use of chemicals in agriculture (Adesemoye et al., 2009; Lacey et al., 2015; Bargaz et al., 2018; Harman and Uphoff, 2019; Singh and Prabha, 2019; Basu et al., 2021). However, the inoculation of the soil with beneficial microorganisms may affect its native microbial populations (Trabelsi and Mhamdi, 2013; Mawarda et al., 2020); with effects that depend on the soil chemical and physical characteristics and the environmental conditions (i.e., climate, agronomic practices, and cropping systems, etc.). Furthermore, considering the pivotal role of soil microbial diversity for life-supporting functions, changes occurring to the soil microbial structure after applying microbial-based formulations may affect the overall soil quality and fertility with varying effects (Trabelsi and Mhamdi, 2013), which can impact crop productivity and quality.
On the other hand, manufacturers are interested in understanding the interactions between the bioinocula and the native soil microbiota since it can improve their application methods and efficacy. Moreover, particularly in the case of biopesticides, the knowledge of their effects on soil microbial diversity and composition must comply with ecotoxicological standards during the registration process (e.g., European Union Regulation 1107/2009). In this framework, which encompasses scientific, commercial and regulatory aspects, the development of tools to monitor the introduced microbial species becomes of paramount importance, particularly to assure a correct risk assessment in relation to the environment and human health (Sessitsch et al., 2019; Mitter et al., 2021). The monitoring tools should support two critical aspects of microbial inocula application: (i) the bioinoculant detection in the soil to evaluate its persistence and fate; (ii) the assessment of the impact of the introduced bioinoculant species on native soil microbial communities. Evaluating the effects on native soil microbial communities and detect the inoculants once applied in the soil can add a piece in the complex puzzle of biofertilizer development.
The present review focuses on the methods so far applied or with future potential for tracking the bioinoculants in soil, and evaluating their impact on the local microbial communities. The aim is to provide critical and comprehensive analysis of the tools currently available, and also a bibliometric screening of the literature dealing with detection and monitoring of microbial inocula. A description and comparison of the methods based on morpho-phenological, molecular and microscopic approaches is then provided. The prospects for the future development of protocols suitable for regulatory or commercial purposes are also discussed. A multi-faced approach capable of assuring the necessary level of discrimination that is required for tracking and monitoring bioinocula and biopesticides in the soil is then suggested.
Trends in Soil Microbial Inoculants Detection and Monitoring: a Science Mapping Approach
A science mapping analysis of published research papers was used to appraise the research trends on microbial inoculant detection and monitoring in the soil in 1991–2020. The following keywords and strings were used to retrieve the relevant publications from the SCOPUS database, which was consulted on October 12th, 2020: detection, tracking, traceability, monitoring, inoculants (OR bioproducts OR biofertiliser OR consortia OR microorganisms OR bioinocula), soil, technique (OR tool OR method) in the combined fields of “title,” “abstract,” and “keywords.”
The software VOSviewer, version 1.6.5.0 (freely available at www.vosviewer.com), was used to create bibliometric maps based on the retrieved publications and to conduct a general quantitative analysis. Before starting the analysis, a thesaurus file was created to ensure consistency for different term spelling and synonyms. Term maps were produced following the method used by van Eck and Waltman (2010). The EndNote file of this search is attached as Supplementary Information Data 1 and VosViewerMap, and Network files necessary to navigate the maps with labels are available as Supplementary Information Data 2, 3 respectively.
A total number of 681 scientific publications were retrieved, providing in total 17,617 terms. Only the terms occurring at least eight times in the combined fields per publication were extracted from the 681 retrieved publications. Figure 1 shows the yearly total number and frequencies of publications in the concerned period. It emerged that during the first 5 years, only 1 to 3 articles were published yearly on these subjects, while from the beginning of the century, the interest in the topic, and thus the number of publications, increased, generally in parallel to the research on microbial bioinocula (Santos et al., 2019; Canfora et al., 2021). Almost 70% of the retrieved publications (474) were research papers, while the remaining 207 included both reviews (114) and books or chapters. Interestingly, the countries traditionally concerned with soil microbiology studies and the introduction of microbial inocula in the agronomical practice, also having relevant legislation in place, resulted at the top of the list: the European Union (21.64%, with Germany accounting alone for the highest percentage – 6.31%), India (11.32%), United States of America (9.92%), China (7.52), and United Kingdom (5.41).
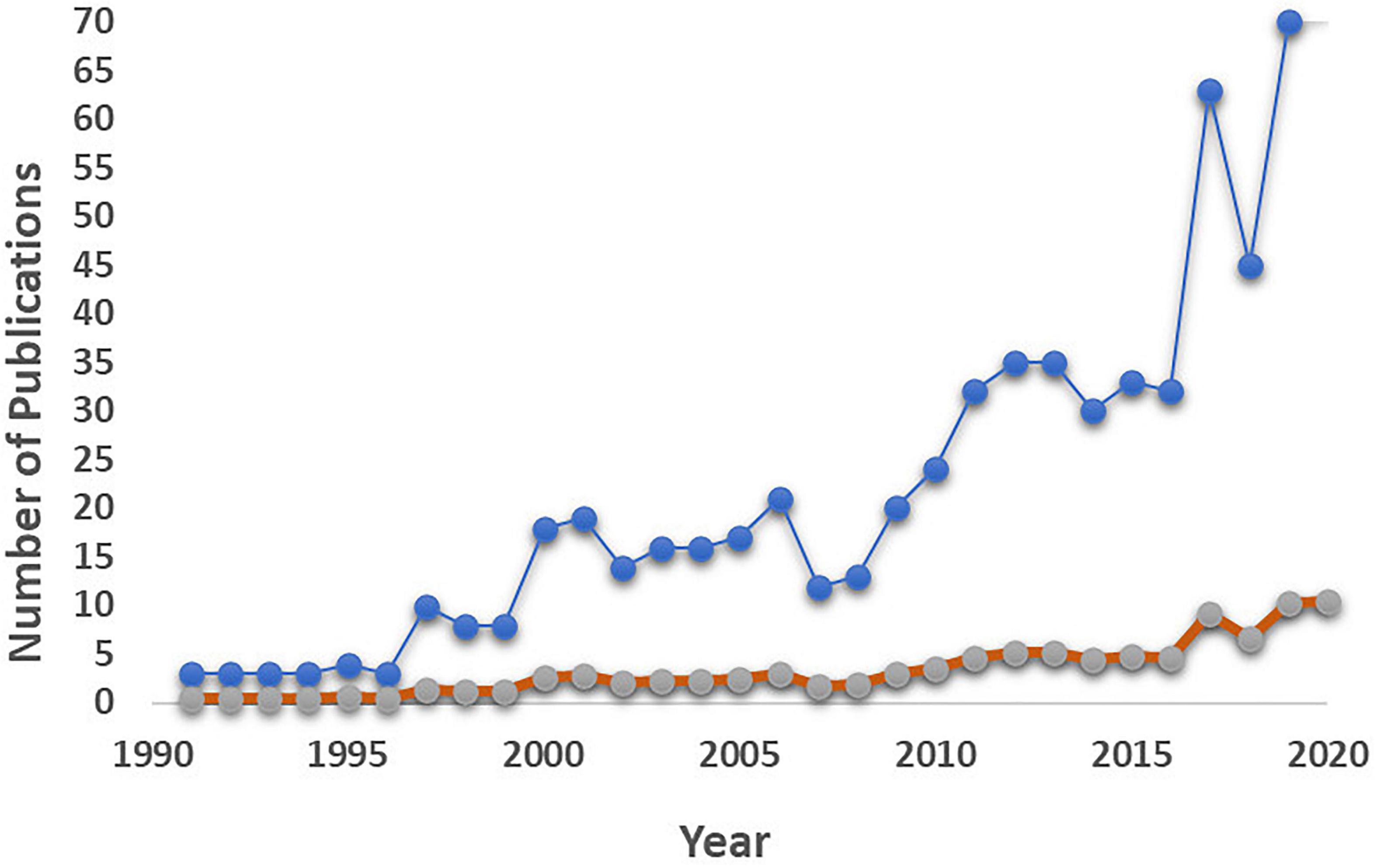
Figure 1. Trend of publications on soil microbial inoculants tracking and monitoring in the period from 1991 to 2020. The blue line represents the number of publications per year; the orange line represents the frequencies of publications per year.
Term maps showing closeness among terms were calculated based on terms co-occurrence within the same publication, according to Costa et al. (2019). Three clusters emerged from the analysis, discriminating methods-based terms (e.g., qPCR, marker, PCR, sequence, and T-RFLP; green circles), microorganism-related terms (e.g., Pseudomonas fluorescens, Rhizobacteria, plant growth promoting bacteria, and activity; blue circles), and use-related terms (e.g., plant biomass, nutrient availability, remediation, and pollutant; red dots; Figure 2). A number of terms formed a bridge between clusters (i.e., strain, inoculation, bacteria, and rhizosphere), while others appeared quite scattered (i.e., drought, phytohormone, farmer, human health, and field release).
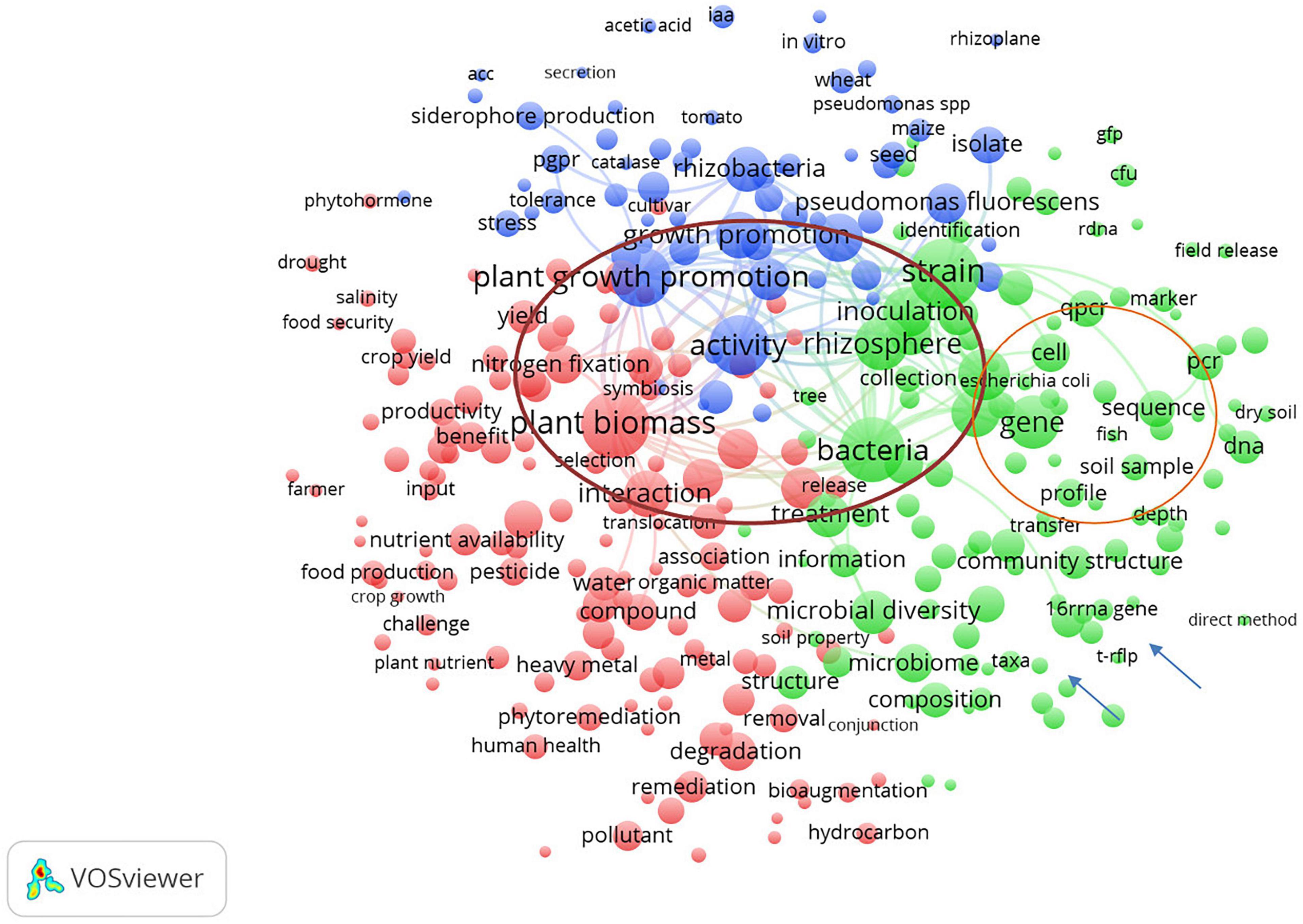
Figure 2. The terms clustering map based on the analysis of publications concerned with soil microbial inoculants tracking and monitoring retrieved from Scopus database from the period 1991–2020. Red, blue, green colors represent the terms belonging to different clusters. The dot size of each term is based on the number of its occurrence. The connecting lines indicate the 100 strongest co-occurrence links between terms.
It is noteworthy that the term “field release” and “farmer” are set apart from each other, which could suggest a limited connection between research and field application of bioinocula or a limited number of long-term studies under field conditions assessing the impact caused by or persistence of microbial inoculants on the soil ecosystem and microbiome as also pointed out recently elsewhere (Canfora et al., 2021).
It shall be highlighted that the term “detection” appeared first in 1991 (Pickup, 1991), and a review on the microbiological and molecular techniques for monitoring the genetically modified and unmodified microorganisms in the soil appeared in 1998 (Van Elsas et al., 1998), pointing out questions about risk assessment concerning the release of cells in the soil environment. However, terms like “regulation” and “legislation,” which should be widely expected, were missing from the analyzed dataset of publications. On the other hand, terms related to results of soil inoculation with bioinocula were associated only with beneficial effects.
The term map created according to the publication year (Figure 3) clearly showed the evolution and trends in research during the last decade. The attention toward the study of the interaction between native soil microbial communities and bioinocula represented by terms such as “interaction,” “plant growth promotion,” and “activity” has marked the new trend in the detection and monitoring of microbial soil inoculants. The increased sensibility during the last decade with respect to the environmental impact of bioinocula also emerged from the map representing the scientific impact of the subjects addressed by researchers over the years (Figure 4).
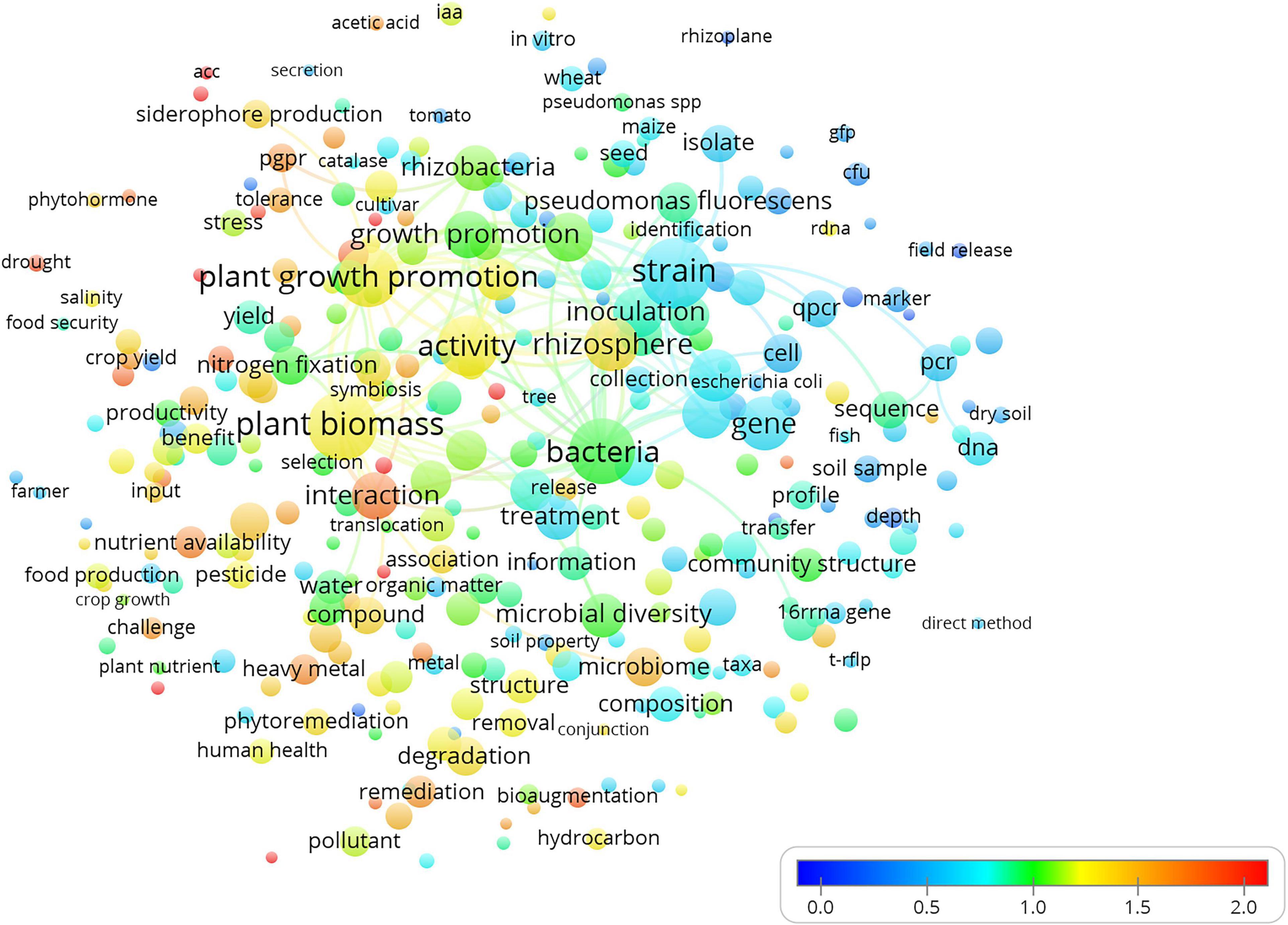
Figure 3. Term map based indexed per citation rate based on all the Scopus soil microbial inoculants tracking and monitoring publications/citations. The different colors represent the normalized citation rate scale. The size of each term is based on the number of its occurrence. The connecting lines indicate the 100 strongest co-occurrence links between terms.
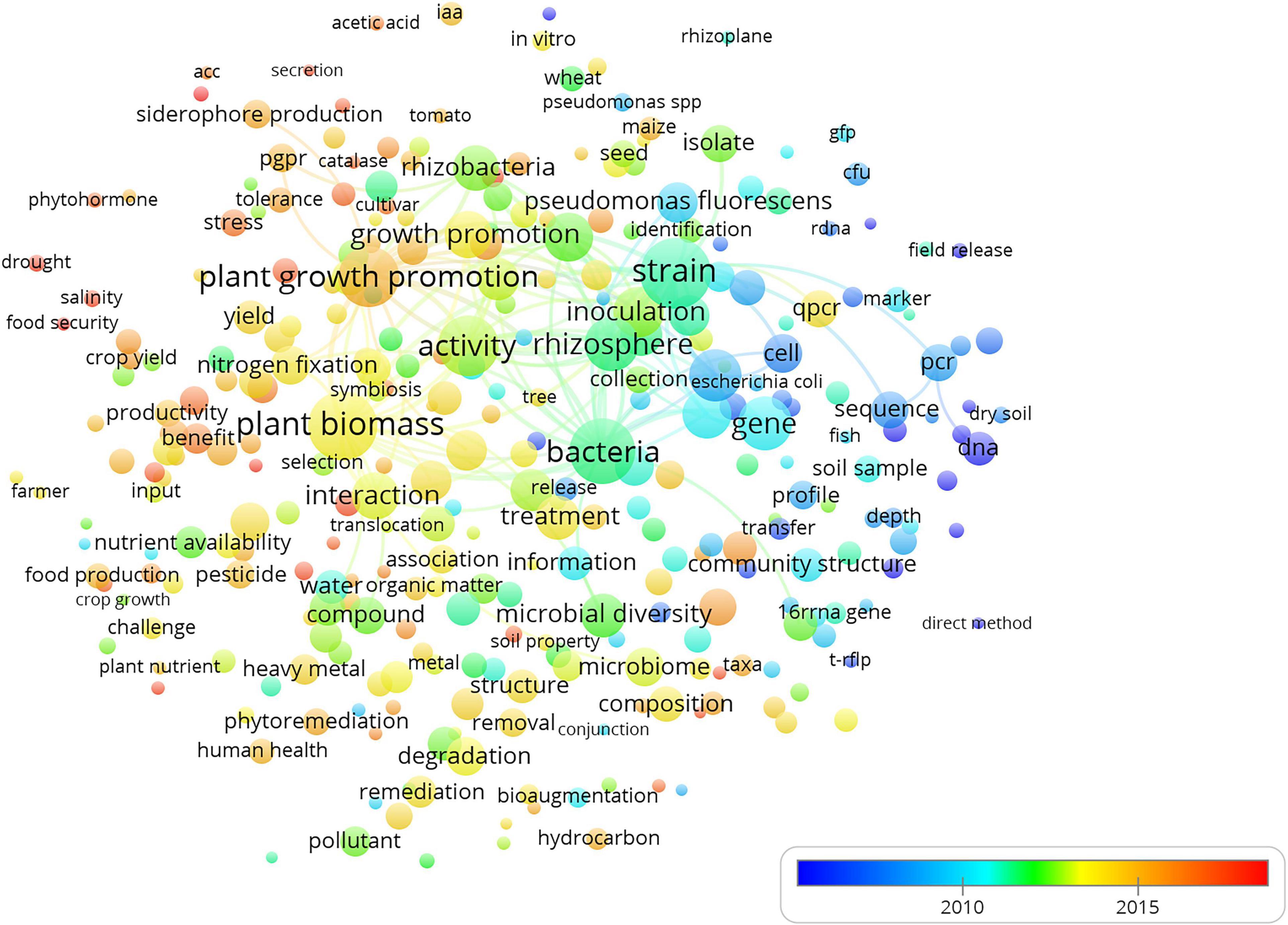
Figure 4. Term map created according to publication year on soil microbial inoculant detection and monitoring based on the Scopus database. The time scale is represented by different colors. The dot size of each term is based on the number of its occurrence. The connecting lines indicate the 100 strongest co-occurrence links between terms.
A striking result of the analysis concerned the unbalance of studies with terms associated with bacteria, which overrated those dedicated to fungi. This outcome confirmed another recent metadata analysis, which found that among 108 studies addressing the use of microbial inoculant in soil, only 22 were dealing with inoculated fungi and two the co-inoculation of bacteria and fungi, while the remaining concerned bacteria (Mawarda et al., 2020). Even though both groups of microorganisms have a long history of field application as in the case of mycorrhizal fungi (Wamberg et al., 2003; Bardi and Malusá, 2012; Malusá et al., 2016) or N-fixing bacteria (Lucy et al., 2004; Naseer et al., 2019), the easiness and/or wide availability of strains inducing growth promotion or protection among the bacteria could have been accounted for this result, also confirming the conclusions of other analyses (Canfora et al., 2021).
The most recurrent methods for detection and monitoring in the publications retrieved were based on PCR techniques. The terms PCR/qPCR showed the highest occurrence (150 items), while culture-dependent and fingerprinting [e.g., terminal-restriction fragment length polymorphism (TRFLP), Denaturing Gradient Gel Electrophoresis (DGGE)] methods occurred only about 50 times each. Mawarda et al. (2020), by using a literature search-mapping approach, reported that 72% of studies addressing the impact of bioinocula on resident microbial communities used profiling methods including TRFLP, and 4% used quantitative PCR targeting particular taxonomic or functional groups. The use of a single methodology for the detection and monitoring of the inoculated strain was already argued by Van Elsas et al. (1998). They recommended a polyphasic approach, combining culture-dependent and culture-independent methods, to evaluate the dynamics of non-native microorganisms introduced in the soil environment and their interaction with the autochthonous microbiome to understand their survival and performance. However, the current and previous bibliometric analyses showed that a monophasic approach had been generally used to address these goals. The effort expressed by this review in presenting and discussing comprehensively different available and underutilized or perspective methods for detection and monitoring of bioinoculants also aimed at fostering a better understanding of the opportunities and risks associated with the use of bioinocula.
What to Keep in Mind When Monitoring the Impact of Microbial Inoculants in Soil Interactions Between Bioinocula and Autochthonous Soil Microorganisms
The mechanisms underlying the fate and persistence of bioinoculants in soil can result from the sum of multiple variables and therefore be difficult to understand and predict. The introduction of any microbial inoculant to the soil should be considered as a disturbance to native microbiota. In ecology, the term “disturbance” usually refers to causal events that may directly or indirectly impact the environment/community and may occur at different spatial and temporal scales with different frequencies, intensities, and extents (Shade et al., 2012). A community’s response to disturbance is defined as “stability” that comprises both “resistance” and “resilience.” The scientific literature includes many definitions of stability, resistance and resilience, and a complete examination of these definitions is available elsewhere. However, in this paper, the term “resistance” is defined as the degree to which a community is insensitive to a disturbance, and “resilience” is the rate at which a community can return to the original state after a disturbance. Many studies have investigated the resilience of microbial communities to various disturbances, including human activities such as land use and agricultural practices (Mocali et al., 2015; Morriën et al., 2017) but also natural disturbances such as fire or freeze-thaw and desiccation (Shade et al., 2012; Griffiths and Philippot, 2013). However, to the best of our knowledge, the impact of microbial inoculation on the soil microbiome has been poorly addressed so far. In particular, the extent to which these changes affect soil ecosystem functioning remains largely unknown (Ray et al., 2020).
Bioinocula releases into soil often result in transient loads of the microbial strain/s that generally fade away with time (Bankhead et al., 2004), even though it has been shown that an entomopathogenic fungal strain was detected up to 15 years after the application (Enkerli et al., 2004; Mayerhofer et al., 2015). Given the supposed transitory survival of bioinocula, many scientists and practitioners assume that soil microbial inoculants would have negligible effects on the autochthonous soil microbial communities (Mawarda et al., 2020). However, the quick disappearance of inoculum in the soil does not necessarily imply a lack of long-lasting changes in the soil resident community. For example, Rhizobium inoculation to promote soybean productivity significantly influenced the bacterial community in the crop rhizosphere (Zhong et al., 2019) and the fungal community (Xu et al., 2020). Furthermore, the introduction of non-pathogenic Escherichia coli into soil shifted the niche structure. It increased the niche breadth of resident bacterial communities, leading to changes in significant soil bacterial genera’s relative abundances, such as Bacillus, Pseudomonas, Burkholderia, and Bradyrhizobium (Mallon et al., 2018). On the other hand, it is logical to assume that persisting microbial inoculants will have a more prolonged impact than short-lived inoculants. For instance, long-lasting changes were reported in microbial composition with the inoculant Bacillus amyloliquefaciens NJN-6, which showed relatively stable abundance (between 2.5 and 3.0 log copies of 16S rRNA gene/gram of soil) within 3 years after application (Fu et al., 2017). However, it remained unclear whether the measured changes were due to direct effects from the inoculant or indirect effect, for instance, because of nutrients released from dead inoculant cells or the inoculant-root interactions described for other bioinoculants (Castro-Sowinski et al., 2007; Hartmann et al., 2009).
The survival of microbial inoculants in soil depends on the soil native microbiota: it is high when the autochthonous microbial community’s diversity is low and vice versa (Van Elsas et al., 2012; Mallon et al., 2015). However, the importance of evaluating the impact of inoculation in a temporal context should be kept in mind since the effects might change over time. For example, most of the studies addressing the impact of microbial inoculants on soil native communities measured the effects only over a short period after inoculation, usually within the vegetative season (Mawarda et al., 2020). In most cases, the inoculation led to changes in the structure of the resident communities, but it remains unclear whether the impact could persist for a more extended time, thus indicating the actual resilience capacity after the disturbance (Xing et al., 2020). However, some studies have shown a longer-term impact on resident rhizobacteria diversity due to residual effects of antibiotic-producing PGPR P. fluorescens F113Rif (Walsh et al., 2003) or on the community structure of some specific bacterial groups (Robleto et al., 1998). Moreover, the method applied for the assessment could differently represent the impact of the bioinocula. For example, the T-RFLP-based methodology and also the quantification of the gene copy numbers of a specific set of fungal genes showed that the application of two different species of entomopathogenic fungi did not alter the composition of fungal communities considerably neither after the first application nor at the end of the second season in comparison to untreated soils (Tartanus et al., 2021) but appeared to modify the bacterial community. Remarkably, even unsuccessful application of microbial inoculants might still leave a legacy effect on the resident community (Mallon et al., 2015; Xing et al., 2020). Therefore, although largely unexplored, this issue might have implications in areas where microbial invasions are sought, such as on the efficacy of probiotic, biocontrol, and biofertiliser agents, because the legacy of past invasion attempts may alter the present community structure, functioning, and, ultimately, mechanisms of resistance.
Considering the role of the resident soil microbiome for an effective use of microbial inoculants, it is quite surprising that the term “resilience” was not evidenced by our literature survey and science mapping approach. It confirms that this perspective is still largely overlooked, thus suggesting the need for an effort to evaluate the impact of inoculants on soil native community resilience, especially from a functional perspective. A broad range of “omic” approaches (metagenomics, metatranscriptomics, metabolomics, and metaproteomics) applied in long-term experiments under field conditions would allow an in-depth assessment. An example of such effort is represented by the EXCALIBUR project1, aiming to deepen our understanding of the mechanisms underlying the soil microbiome changes composition and functioning upon bioinocula application (Allison and Martiny, 2009).
Good Field Practices to Track Soil Microbial Inoculants: the Sampling Approach Comes First
The complex web of soil microorganisms represents the primary biodiversity source on Earth (Shoemaker et al., 2017). Research in soil microbial ecology has revealed the tremendous diversity and complexity of microbial communities across space and time in the soil (Green and Bohannan, 2006). The difficulties associated with the soil matrix (uneven physical and chemical composition), which require a specific effort when studying microbial diversity, are even more challenging when tracking or monitoring a bioinoculum is concerned (Nannipieri et al., 2019).
Therefore, the sampling method used for tracing and monitoring the fate of a microbial inoculant in the soil represents the most critical step of the process, which shall be designed to assure the quality and the robustness of the data generated.
The sampling methodology shall consider whether bulk or rhizospheric soil (or almost rhizospheric, i.e., in the root zone) soil is best suited to assess the strain fate. For strains used as biofertilisers, the soil near the roots is considered more suitable because the plant-microbe interactions (Bhattacharjee et al., 2008; Hartmann et al., 2009) can enhance the bioinoculant survival and root colonization. Inoculants used as biopesticides, particularly those based on fungi able to behave saprophytically (e.g., Beauveria bassiana, Trichoderma spp., etc.), can also be monitored by sampling either the bulk soil (Enkerli et al., 2004; Kessler et al., 2004; Dolci et al., 2006; Mayerhofer et al., 2015), or the soil near the root system in case of poly-annual/perennial crops (Canfora et al., 2016; Tartanus et al., 2021).
Sampling the bulk or soil near the root zone will dictate the depth at which the soil has to be collected. However, the soil texture and composition should also be taken into consideration in defining the sampling depth due to the possible effect of oxygen (air) availability and organic matter content on bioinoculant persistence (Walstad et al., 1970; Studdert and Kaya, 1990; Vänninen et al., 2000; Weyman-Kaczmarkowa and Pȩdziwilk, 2000; Kessler et al., 2003; Rousk and Bååth, 2007; Fàtu et al., 2015). The frequency of sampling is a variable that can be affected by several factors, including the formulation of the bioinoculum, the crop duration, the strain growth potential, and the pedo-climatic conditions of the site (Horaczek and Viernstein, 2004; Malusá et al., 2020). All of them are quite intuitive to understand; however, a proper sampling strategy cannot disregard them to avoid reducing of the validity of the analytical results. The number of samples and sub-samples to be collected for each individual analysis is generally constrained by the resources available for the analytical determination. However, the minimum number of samples should comply with the classical soil microbiology methods (Bloem et al., 2009; Elsas et al., 2019).
Examples of sampling strategies to monitor the persistence and fate of bioinocula in soil are available, particularly for biopesticides. This is mainly due to the registration requirements because of concerns about non-target organisms’ risks (e.g., EU Regulation 1107/2009, US EPA Act – 7 U.S.C. §136 et seq. 1996; Pelaez et al., 2013). For example, a randomized block design with 4 replicates, sampling the soil near the root zone at a depth of 0–20 cm, with each soil sample composed by 25 points randomly distributed within each treatment was used to follow the persistence of formulations made with two Beauveria spp. in strawberries (Canfora et al., 2016; Tartanus et al., 2021). The persistence and fate of five B. brongniartii strains in the soil for 2 years were monitored collecting 12 soil samples from a depth of 5–20 cm in eight apple orchards (Dolci et al., 2006). However, the increasing awareness about the implications derived from the field application of microorganisms could also foster the introduction of similar requirements for biofertilisers [EFSA project GP/EFSA/ENCO/2020/02 on “Evaluating the impact on/by environmental microbiomes (plants, wildlife, and soil) in assessments under EFSA’s remit”].
Soil handling also requires a specific procedure after sampling, particularly when molecular techniques are planned to be used for the analysis. It must be kept in a sterile plastic bag or tube DNase and RNase free with minimal manipulation, placed in a cooler with ice or ice bags and, once in the laboratory, it shall be stored immediately at 4°C or frozen in case of prolonged storage periods.
Overview of the Methodological Tools to Detect and Monitor Bioinocula in Soil: Challenges, Limitations, and Controversial Issues
Earlier detection approaches from culture-dependent tools, such as direct microscopic examination (Stefani et al., 2015), plate profiling (Davis et al., 2005; Eichorst et al., 2007; Bloem et al., 2009), and FISH (Fluorescent in situ Hybridization; Cerqueira et al., 2008; Schmidt and Eickhorst, 2014), have provided essential insights into detection and localization of target microbial species in soil. These approaches led the way to culture-independent tools addressing the analysis of target microbial species of bioinocula and evaluating the bioinocula effect on microbial communities’ structure and diversity. The rapid development of DNA and RNA-based analytical methods has offered new opportunities to monitor microbial inoculants survival and interactions within a specific soil community. Indeed, a high degree of resolution is fundamental to evaluate the success or failure of bacteria or fungi inoculation, tracing the “introduced DNA” in a mixture of genomes from thousands of different organisms. Culture-independent methods have also effectively characterized the soil microbial assemblages in space and time, evaluating their functional and trophic interactions (references herein included). Recent research in the “omic” era has expanded our knowledge and understanding of microbial community assembly but tracing the bioinocula in the soil is still not a straightforward task. A plethora of methods have been developed to enable inventories of microbial species composition and a good understanding of dynamics and processes of biodiversity (Philippot et al., 2012; Nemergut et al., 2013; Cristescu, 2014) in bulk or rhizospheric soil, but generally are not suitable to follow the fate of a single species.
However, any method utilized for tracking inoculants introduced in the soil presents some advantages and disadvantage, which we try to point out to highlight possible procedures implementing the multi-phasic approach mentioned above.
Microscopy-Based Techniques: Challenges and Opportunities
Given the wide variability of microbial species, the observation, identification, and enumeration of microorganisms not as pure isolates but embedded in complex matrices, such as plant cell tissues, biofilms, soil, compost, and mineral matrices, require the use of rigorous procedures. Different species or strains may differ in morphology, nutrition, physiology, reproduction and growth, metabolism, pathogenesis, antigenicity, and genetic properties (Moore et al., 2010). Techniques developed to morphologically resolve and trace individual species or even particular strains of microorganisms in a chemically and physically complex system generally exploit one or more organisms’ properties, combining their resolving power to achieve maximum discrimination. Morphology, including cell size, arrangement and shape, is not sufficient on its own to typify and trace individual species of microorganisms, primarily if they are embedded in complex matrices that are opaque to the source of observation (Moore et al., 2010).
Tracing individual species or strains of fungi or bacteria in environmental matrices leads back to the fundamental concepts of taxonomy (or biosystematics), which consists of four main parts: classification, nomenclature, identification and phylogeny, which is now an integral part of the classification process (Naranjo-Ortiz and Gabaldón, 2019). However, prokaryotic microorganisms vary widely in size, and their cells are often close to the resolution limits of the light microscope; also, when observing environmental samples, cells may become confused with particulate matter or be obscured by internal and external structures of larger cells, as is the case with animal and plant tissues (Johnson and Criss, 2013). Furthermore, it is often impossible to distinguish living cells from dead cells or cells from inanimate objects in environmental samples.
Compared to bacteria, fungi have larger cells that often display characters with a diagnostic value, but the systematics of fungi is complicated by intraspecific morphological and physiological variation and the limited number of morphological markers available (Naranjo-Ortiz and Gabaldón, 2019). Therefore, the use of microscopy to locate, identify and count individual microbial taxa is limited by the systematic weight of the characters used in the identification.
Fluorescence microscopy (FM) has represented a significant advance in tracking and localizing specific microorganisms in complex matrices. Using fluorescent dyes (fluorophores) and markers derived from them that indicate the presence of specific substances or cellular activities, it is possible to visualize microorganisms under the microscope and distinguish them from the background or dead cells in the same sample. These protocols combine membrane-permeable fluorescent dyes [i.e., SYTO9 and 4′,6-diamidino-2-phenylindole (DAPI)], which penetrate all the microbial cells in the sample, with membraneimpermeable fluorescent dyes, that instead are impermeable only to viable membranes (i.e., propidium iodide and SYTOX Green), and which therefore enter only the non-viable cells (Johnson and Criss, 2013). These techniques work with both fungi and bacteria, but some cells and resistant structures could require specific protocols, as well as with the complicated wall structure of many fungal taxa (Villa et al., 2009). Many fluorescent probes have been developed, which, when used in combination with the FM, allow various cellular parameters of microorganisms to be measured, thereby tracking their presence and activity. For example, fluorescence detection techniques have been widely used to map intracellular pH. Fluorescence lifetime imaging microscopy (FLIM) allow characterizing pH regulation mechanisms in different cellular compartments. Carboxy–(C–) SNAFL1, C–SNAFL2, fluorescein, and C–fluorescein are fluorescent compounds that allow real–time cellular response to pH changes in the medium, at different pH. They are widely used to visualize pH gradients in microbial biofilms (Lin et al., 2003). However, unless it is sufficient to generically track a cellular function or enzymatic activity to detect the presence of the microorganism of interest, chemical–only fluorescent markers do not have the resolution power required of a system capable of discriminating between different microorganism species. One of the limitations of FM and derived techniques is the spatial resolution, which is usually in the order of 30–100 nm (Huang et al., 2009).
The use of fluorescence in situ hybridization (FISH) techniques, with oligonucleotide probes that bind only to complementary nucleic acid sequences to target rRNA molecules, is a key method for detecting and sometimes identifying microorganisms in environmental samples. Probes can be designed for any gene or sequence within a gene to visualize target mRNA in microbial cells. FM combined with FISH can then be used to visualize the target microorganism in the sample where the fluorescent probe has bound. There are many FISH techniques with application–specific protocols and different fluorochrome combinations (Schimak et al., 2016). FISH is widely used in microbial ecology to identify microorganisms in complex matrices, although one of the best–known applications is the characterization of multispecies biofilms (Almstrand et al., 2013). With FISH, by using different colored probes for each species present in a sample, it is possible to localize their distribution and document their interaction by FM. Recently, multicolor FISH approaches have been developed using up to eight fluorophores with distinct spectral properties. The technique allowed to unambiguously discriminate the seven phylogenetically distinct microbial populations that were composing an artificial community (Lukumbuzya et al., 2019).
Oligonucleotide probes targeting ribosomal RNA (rRNA) are typically used to identify bacteria, being among the most conserved macromolecules in nature and found with a high copy number in every cell. Comparative analysis of rRNA sequences allows identifying short signature sequences that are unique to different groups of microorganisms. These signatures are used as targets for fluorochrome–labeled probes made of short complementary oligonucleotides (15–30 nucleotides). A comprehensive online resource for information on the identification of single microbial cells by FISH is available on the SILVA website2.
Raman micro-spectroscopy, a vibrational spectroscopy technique integrated with microscopy systems, is another microscopy-based technique suitable for tracking microorganisms in complex matrices that allow the chemical fingerprinting of individual eukaryotic organelles or bacterial cells. The chemical information derived from a Raman spectrum makes it possible to identify substances accumulated in cells, such as lipids, sugars, cytochromes, and pigments, within seconds (Gruber-Vodicka et al., 2011; Majed et al., 2012; Milucka et al., 2012). The incorporation of stable 13C, deuterium and 15N isotopes into target cells allows their detection by characteristic band shifts in Raman spectra (Wang et al., 2016). The combination of Raman micro-spectroscopy with FISH techniques allowed the authors to recognize in situ, in a microbial community, cells capable of degrading naphthalene and attributing them to an uncultured bacterial species (Huang et al., 2007).
Another spectroscopic technique associated with microscopy and capable of discriminating between different microorganisms’ species is the Fourier-Transformed Infra-Red spectrometry integrated with a microscope (FT-IR microspectroscopy). The technique is used to create a chemical fingerprint of the target microorganism, which is then used to discriminate it from other strains (Adiani et al., 2020). This technique was used to discriminate E. coli strains from different sources, and its efficiency was comparable with the BOX-PCR genomic fingerprinting method (Carlos et al., 2011). The bands in the FT-IR spectra that were responsible for the strain’s discrimination were in the region between 2,816 and 3,026 cm–1 wavenumbers, corresponding to fatty acids.
Discrimination between cells of different microbial species that coexist requires a spatial resolution that can only be achieved by electron microscopy (EM). However, the limited field view and small area visualized in EM images represent a limitation for the technique. To monitor larger areas of the sample by EM and understand specific patterns of microorganism distribution or assess their abundance, it is necessary to scan complete EM grids with a resolution that would require the collection of thousands of images. This limitation can be overcome by correlative microscopy, which combines fluorescence and EM (Verkade, 2008). FM is used to identify and localize features of interest, and EM is used to map their ultrastructure (Schwarz and Humbel, 2007). The development of increasingly advanced correlative microscopy systems goes hand in hand with software and computing power to handle complex sample coordinates and manage in parallel several microscope software (Agronskaia et al., 2008). Correlative microscopy initially combined optical or FM with transmission EM, but it is now available in integrated systems that combine different microscopy and spectroscopy types, making it possible to find and analyze the same points in a sample with nanometric precision. Wrede et al. (2008) used a scanning electron microscope in conjunction with an optical fluorescence system to study microbial mats from Cold Seep communities, simultaneously visualizing and distinguishing bacterial and archaeal microorganisms and their associated biogenic minerals. Instruments in which FM is correlated with scanning EM are often complemented by additional techniques that exploit the possibility of performing different measurements at the same point of interest in a sample, switching between instruments, or integrating multiple analysis methods in the same instrument, with software that manages coordinates and recognizes image patterns (Kommnick et al., 2019). For example, Liu et al. (2020) used confocal FM, time-of-flight secondary ion mass spectrometry, X-ray photoelectron spectroscopy, and scanning electron microscopy (SEM) in an integrated system to document, through imaging and both organic and inorganic chemical analysis, the interaction of Brachypodium roots with a strain of Pseudomonas spp., a typical plant growth-promoting soil bacterium.
For tracking microorganisms in environmental samples and tissues, such as roots and plant organs, in situ hybridization systems with nucleic acid-based metal probes allow the observation of samples directly with a scanning electron microscope (SEM-ISH) is of particular interest. The technique involves hybridizing target cells with gold- or platinum-labeled oligonucleotide or polynucleotide probes. It is, therefore, similar to FISH but allows observation directly at the resolution offered by scanning electron microscopes, without necessarily using correlative microscopy equipment. A comprehensive discussion of the technique was addressed by Kenzaka and Tani (2012), and effectively used to track bacteria hybridized with DNA-targeted polynucleotide probes increasing the method sensitivity by applying a signal amplification (Kenzaka et al., 2009). The SEM-ISH protocol proposed by Kenzaka and Tani (2012) allows detecting of cells with a low number of target DNA sequences, enabling to determine the spatial distribution of target cells in complex communities and on different materials. With SEM-ISH technique, SEM instruments can also provide phylogenetic or genetic information on target microbes in addition to information on the substrate on which they grow, both in terms of high-resolution imaging and chemical imaging (i.e., with backscattered electrons, that allow distinguishing objects with a different atomic number; Goldstein et al., 2018).
Culture-Dependent Methods
Culture-dependent methods have several advantages as practical techniques to quantify bio-inoculants. They permit the detection of only viable cells and, therefore, inoculants that are competitive and persist over time. However, using these methods, detecting the inoculated strain from field samples is a difficult task (Pitkäranta et al., 2011; Al-Awadhi et al., 2013; Ngom and Liu, 2014). Culture-dependent methods cannot provide a comprehensive analysis of the endophytic ability of strains under unsterilized conditions, and epiphytes resistant to sterilizing agents could determine an overestimation of their counts (Kandel et al., 2017).
To fully understand the power and limitations of culture-dependent methods in monitoring specific bioinocula in soil, it is necessary to consider that although ongoing cultivation efforts continue to yield between 600 to 800 novel bacterial species per year (Overmann, 2013), global bacterial diversity ranges between 107 and 109 species (Curtis et al., 2002). The same situation applies to fungal species, which are estimated between 70,000 and 1.5 million (Feofilova, 2001), with only 5% of them described and cataloged (Guarro et al., 1999). Notwithstanding the abundance of microbial species in soil, more than 99% cannot be cultured by traditional techniques. Therefore, the culturable species are not representative of the total phylogenetic diversity. Nevertheless, considering that commercial or potential bioinocula are produced by fermentation (i.e., must be culturable), the culture-dependent methods are technically suitable for bioinocula monitoring or tracking (Table 1). Indeed, since the beginning of microbiology, culture-based methods have been used to assess microorganisms’ viability. However, bacterial cells that cannot grow on routinely used laboratory media were considered to be dead and described as “viable” but non- “culturable” (Xu et al., 1982; Bodor et al., 2020).
Moreover, the potential of culture-dependent methods were shown to depend also on the experimental conditions utilized. For example, Castanheira et al. (2017) were able to quantify the colonization of a consortium of bacteria with a cultivation-based approach. However, they utilized FISH and GFP-labeling, combined with confocal microscopy to follow their behavior and coexistence in the plants. Sharma et al. (2011) conducted a field experiment on apple plants using a culture-dependent approach to evaluate the microbial changes due to the application of a consortium formed by bacteria (A. chroococcum AZ1 and AZ2) and fungi (G. fasciculatum and G. mosseae; Table 1).
For all detection methods based on cell growth, the organism’s viability under culture conditions is essential. However, optimal culturing conditions in the lab may not be optimal for retrieving viable microorganisms from the environment: only those that can adapt from the environmental conditions to culture conditions will be possible to observe. Factors affecting the adaptation of an individual species may include lag time or the formation of cooperative clusters in spatially structures environments, or the different strategy of growth kinetics (oligotrophy, copiotrophy, and the r-K continuum; Pianka, 1970, 1972; Hengeveld et al., 2002), or interactions based on a substrate (Canfora et al., 2017).
The plate-count approach strongly underestimates the active microbial biomass. By analogy, the population sizes of bioinocula could be severely underestimated by cultivation methods (Ayrapetyan et al., 2015). Consequently, isolation in liquid cultures or on solid agar media is mainly used to monitor the potential activity, morphology, and physiological characteristics of microbial groups that perform specific functions in soil (Blagodatskaya and Kuzyakov, 2013) rather than verifying the presence of specific species or strains. However, the use of specific liquid media for isolation often results in enrichment cultures: although not yet growing in axenic culture, the target organism may tend to occur in enhanced abundance. Sequential use of the same selective liquid medium may result in a highly enriched population of target microorganisms that can subsequently be placed on solidified medium for purification (Van Kessel et al., 2015). In some cases, using solid agar media reduces the stress imposed by inter-organism competition allowing single populations to better grow out in colonies for further purification and isolation. This approach has been traditionally helpful and is still being used to recover novel isolates in pure culture (Pascual et al., 2016).
New methods have been developed to isolate new strains, which can be potentially used to track them in the soil. Cells’ encapsulation in gel microdroplets, for massively parallel microbial cultivation under low nutrient flux conditions, followed by flow cytometry to detect microdroplets containing microcolonies, allowed to detect previously uncultured strains (Zengler et al., 2002). A cost-effective and easy method that allowed on-site determination of live and dead bacterial cells’ concentration using a fiber-based spectroscopic device (optrode) was used to analyze a sample containing live and dead bacteria at varying ratios and calculate the concentration of each population (Ou et al., 2019).
Previously uncultivated microorganisms were detected using a simulated natural environment (Kaeberlein et al., 2002). A novel method of in situ cultivation (ichip) led to a significant increase of colony count compared to that observed on synthetic media, favoring species commonly not detected in standard Petri dishes (Nichols et al., 2010). Chaudhary and coworkers (Chaudhary et al., 2019) developed a new diffusion bioreactor to cultivate hidden bacterial communities in their natural environment and were able to recover more than 400 bacteria isolates, including 35 previously uncultured strains.
They may be present in the soil as unculturable and non-sporulating fungi, such as the endophytic and mycorrhiza forming species (Tsui et al., 2011). Indeed, he arbuscular mycorrhizal fungi (AMF) are not able to be cultivated on laboratory growth media and can only be recovered from the soil with trap plants and then counted using different methods such as the magnified intersections method (McGonigle et al., 1990), the grid-line intersect method (Newman, 1966; Marsh, 1971; Trouvelot et al., 1986), or gradient centrifugation to recover spores (Hayman, 1984; Schenck and Perez, 1990). Identification of these fungi was traditionally performed based on their spore morphology, which is time-consuming and requires considerable expertise (Pagano et al., 2016). However, monitoring of AMF can be performed by assessing root colonization. Phillips and Hayman (1970) developed an easy standard method to stain AMF in roots, which has undergone several modifications to adapt it to the roots’ characteristics from diverse plant species (e.g., Derkowska et al., 2015). Other methods based on biochemical analyses have also been proposed to monitor AMF. For example, ergosterol has been used as a biomass indicator to compare the growth of different AMF (Frey et al., 1994; Fujiyoshi et al., 2000; Hart and Reader, 2002; Lovelock et al., 2004). A method for quantification of glomalin, a fungal protein (or protein class) deposited in the soil by AMF (Rillig, 2004), which content is correlated to AMF presence (Steinberg and Rillig, 2003), based on the Bradford protein analysis (Bradford, 1976), was improved by using ELISA or SDS-page (Rillig, 2004). The analysis of AMF population size in soil, without discriminating between species, can be determined with the MPN (Most Probable Number) bioassay, which is similar to the dilution method used for culturable microorganisms (Alexander and Clark, 2016).
Most of the fungi used as biopesticides, such as the entomopathogenic species, are easily cultivated and isolated, growing optimally on standard culture media. Laboratory techniques to isolate the commercially most exploited genera of entomopathogenic fungi and bacteria from soil relying on culture-based methodologies were recently reviewed (Sharma et al., 2021) to isolate entomopathogenic fungi and bacteria from soil.
To improve the isolation of microorganisms in soil, a particular culture medium (SIA) allowing the isolation of viable but non-culturable strains within 1–3 months of incubation at 28°C could help develop different cultural methods for the diagnosis of unculturable fungi (Sia et al., 2018). However, different co-formulants could behave differently on the survival of bacteria in formulations (Omer, 2010; Vassilev et al., 2020). As alternatives, or in combination, to the classical cultivation methods, the MicroResp (Creamer et al., 2016), and community-level physiological profiles (Frąc et al., 2017) methods can be used for the identification of bacteria species. The latter method can be performed using the Biolog system (Al-Dhabaan and Bakhali, 2017) which is based on patterns of single carbon source utilization. A method based on multiple culture conditions combined with the rapid identification of bacteria (by MALDI-TOF mass spectrometry and 16S rRNA sequencing), named “culturomics,” which was developed for the identification of previously unculturable bacterial species of the human gut microbiome (Lagier et al., 2016, 2018) could be applied to isolate less abundant and unculturable microorganisms from the soil.
DNA-Based Technologies for Tracking of Bioinoculants in Soil and Assessing Their Impact on the Microbial Community
The concept of DNA barcode as “a small piece of the genome found in a broad range of species,” is not new and has been widely used for many years across all life forms, including microorganisms, to distinguish a species from another (Chakraborty et al., 2014; Kress et al., 2015). The barcode is derived from a PCR amplicon of a target sequence used to identify (or barcoding) a microorganism distinguishing it from other species. However, DNA barcodes are not error-free, and single species barcoding needs to be designed based on robust genetic distances to obtain unique and highly discriminant markers. The genetic variability of individual strains, sometimes closely related but different in genomic traits, is exploited to discriminate the individual species but may, as well, provide inaccurate identifications (Hebert et al., 2003; Chakraborty et al., 2014). Markers based on sequences characterized amplified regions (SCAR) have been widely used as molecular probes for tracking the fate of fungi (Abbasi et al., 1999; Dauch et al., 2003). SCAR markers are based on universal primers, i.e., sequences universally present with highly conserved flanking regions, which, however, are able to discriminate only at genera or species groups levels, but not at species level within a pool of microorganisms (Barberio et al., 2001; references included in Table 2). However, markers suitable to monitor or discriminate the introduced bioinocula from native soil strains should be species-specific (Salazar et al., 2000; Grosch et al., 2007).
Specificity for a target organism is a critical element in the use of any PCR-based technique. PCR-based techniques that proved to be successful in targeting single species in complex communities is focused on the natural polymorphisms of genomes. Short unique sequences allowed the use of species-specific probes to discriminate the introduced organisms in soil (Felici et al., 2008; Öpik et al., 2010; Stockinger et al., 2010; Sýkorová et al., 2012; Trabelsi and Mhamdi, 2013; Canfora et al., 2016, 2017; Malusá et al., 2016; Tartanus et al., 2021).
The possibility of targeting short sequences allowed to bypass the disadvantages of a simple PCR-based tool and the technical gap of universal primers. Simple sequence repeats have a polymorphic character that allows producing highly discriminant fingerprints (Rehner and Buckley, 2003; Schwarzenbach et al., 2007; Babić et al., 2008), showing a good discrimination power also for eukaryotic microorganisms such as filamentous fungi (Canfora et al., 2016).
Real-time quantitative PCR (qPCR) is a technique allowing quantifying genetic biomarkers at species, genera or domain-specific levels through the detection of the fluorescence produced by a reporter molecule increases linearly as the PCR cycles proceed in real time. In this framework, quantitative PCR-based techniques offer some opportunities for monitoring single species persistence or functions across the soil communities’ complexity (Schwieger and Tebbe, 2000; Hirsch et al., 2010; Han et al., 2012; Trabelsi and Mhamdi, 2013).
The fluorescence detected is directly proportional to the amount of labeled DNA template present in the PCR (Wen et al., 2009; Blaya et al., 2016). Fluorescent reporters include dyes (i.e., SYBR green) that intercalate with any (non-specific) double-stranded DNA or sequence-specific DNA probe (TaqMan probe), allowing the detection, after hybridization, of the probe with its complementary sequence. The preparation of the qPCR standard and the calibration of the qPCR assay are the two critical steps of the method, affecting the occurrence of false-positive results. qPCR benefits of direct DNA extraction followed by PCR amplification in real-time; this approach is faster than simple PCR and, when coupled with trait-based PCR primers, unique for the microorganisms of interest, allows the simultaneous detection and quantification of the strain.
Several examples in the literature reported that using Real-Time PCR coupled with trait-based PCR permit the detection of the introduced species, simultaneously evaluating their relative abundance within the microbial community (Clesceri et al., 1999; Babić et al., 2008; Couillerot et al., 2010; Paulo et al., 2014; Canfora et al., 2016, 2017; Mandakovic et al., 2018; Tartanus et al., 2021). However, the suitability of a polyphasic approach based on DNA-based methods emerged clearly from a 3-year long study on the persistence of two entomopathogenic fungi (Beauveria brongniartii and B. bassiana) on two sites (Canfora et al., 2016; Tartanus et al., 2021). The abundance of the fungi assessed by mean of a culture-dependent approach, resulted to be lower compared to culture-independent methods. It is noteworthy that previous studies focusing on the detection of the same species by using culture-dependent methods were still able to demonstrate the survival and the establishment of B. brongniartii over 24 months (Dolci et al., 2006) or 7 years after its application (Enkerli et al., 2004; Figure 5).
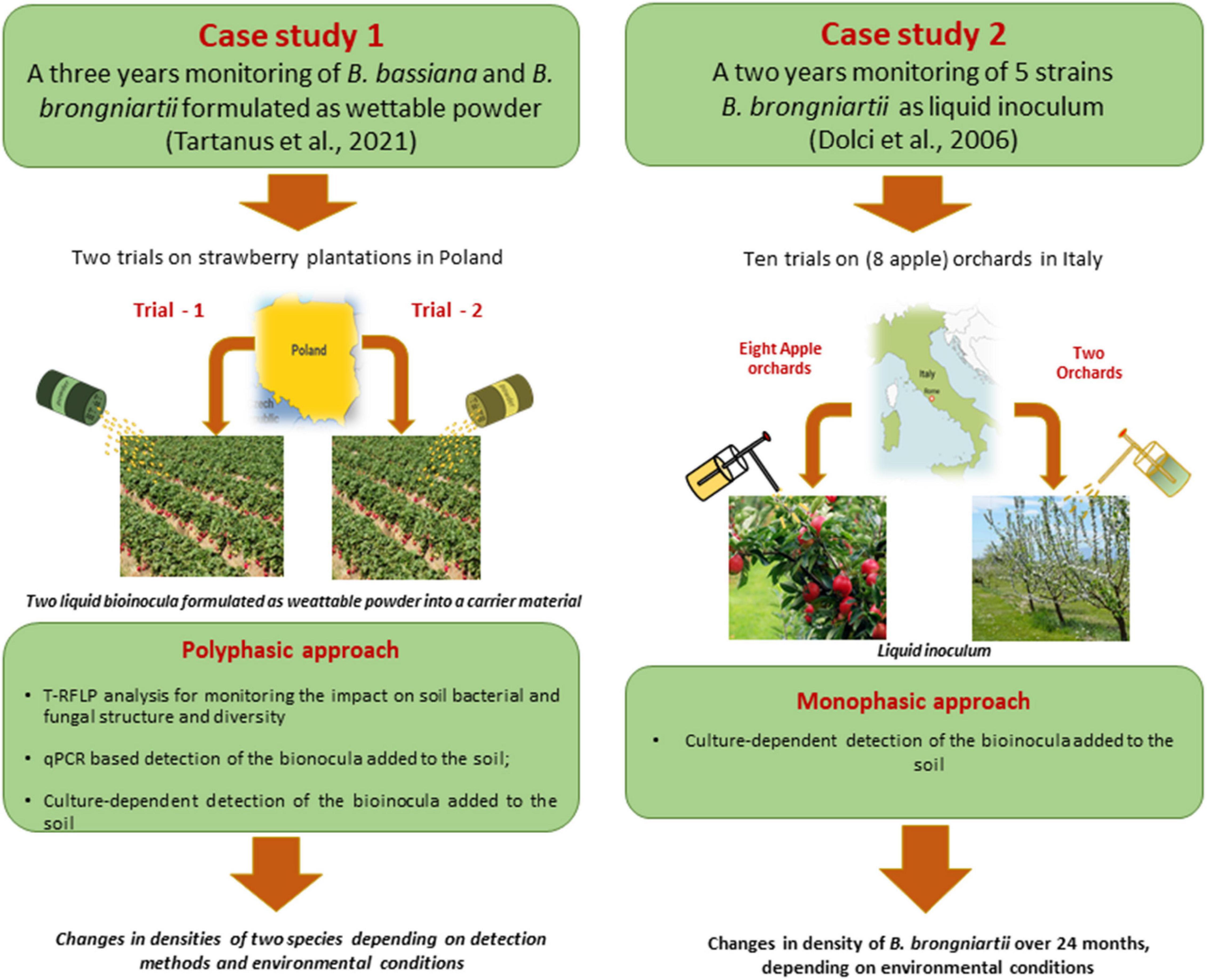
Figure 5. Two case studies of different methods for detection and monitoring of inoculum persistence.
The Real-Time PCR can be successfully used to determine functional groups and have a great potential to estimate the real activity status of certain microorganisms by quantifying relevant functional genes. Although qPCR-real time can be helpful for monitoring and directly quantifying bacteria or fungi introduced in the soil, it needs primer pairs specifically designed to obtain a highly discriminating amplicon. A summary of the major advantages and drawbacks of PCR-based methods is presented in Table 2.
In recent years, increasing efforts have been devoted to improve the knowledge of species-specific marker gene sequences (Yilmaz et al., 2011) or the species genome sequence (Field et al., 2008). The whole genome of a microbial inoculant can be used to design qPCR primers and probes based on the core genome sequences that do not match with other sequences present on BLAST (Rillig et al., 2019). Alternatively, it is possible to exploit the 16S or 18S sequence for bacterial or fungi, respectively, to design specific qPCR primers. Irrespective of the species concerned, there is a clear need for a broader knowledge of microorganisms’ genome sequence that could also be exploited for monitoring of the strains used in microbial inoculants formulations. This approach is currently developed in the work of the project Excalibur1.
Different methods based on the analysis of genetic polymorphisms (T-RFLP; DGGE) have been extensively used to study complex microbial communities in diverse soil ecosystems and environments but proved to be unable to discriminate single target species (Schwieger and Tebbe, 2000; Hirsch et al., 2010; Han et al., 2012; Pellegrino et al., 2012; Trabelsi and Mhamdi, 2013). Nevertheless, they resulted suitable to evaluate the impact of bioinocula on autochthonous microbial communities’ structure and diversity (Michaelsen et al., 2010; Canfora et al., 2015). For example, T-RFLP analysis, which is based on the detection of a single restriction fragment within conserved genomic sequences, amplified directly from the soil DNA is capable of surveying dominant members within the microbial population that comprise at least 1% of the entire community. The combination of PCR, restriction enzyme digestion and electrophoresis on an automated sequencer produces patterns of terminal restriction fragments that can be used to examine microbial community structure and community dynamics in response to bioinocula application (Gao et al., 2012; Trabelsi et al., 2012) or changes in environmental conditions (Mengoni et al., 2006) as well as to study microbial population composition in natural habitats (Canfora et al., 2015). The fingerprint of soil can be obtained by DGGE analysis under DGGE, which enable the separation of DNA fragments of identical length but different sequence (Pellegrino et al., 2012; Pangallo et al., 2014). T-RFLP and DGGE are capable to detect 95–99% of the microbial community, and biomarkers can be derived from sequences present on 16S or 18S or 23s rRNA genes, or ribosomal ITS regions, in the case of bacteria, archaea or fungi, respectively. The presence of discriminant bands could indicate putative species that may be characteristic of the soil sample; however, only cloning and sequencing of the DNA band can confirm the result.
In the last decade, metagenomic methods have been increasingly applied to characterize microbial communities or target groups of microorganisms in different soil ecosystems and environmental compartments (Mocali and Benedetti, 2010; Nesme et al., 2016; Jansson and Hofmockel, 2018; Taş et al., 2021). Sequencing the whole DNA [NGS, third-generation sequencing (TGS) techniques] isolated from a specific soil environment allows to evaluate the total genomic diversity, which is a different concept compared to total species diversity assessed with conventional molecular methods. The metagenomic approach has been applied to study the structure and composition of uncultivated and unculturable microbial populations in specific ecosystems in response to selective pressures and spatio-temporal factors (Daniel, 2005; Fierer et al., 2013; Howe et al., 2014; Canfora et al., 2014b; Feng et al., 2018). Bharti and Grimm (2021) reviewed the sequencing technologies used in the last 25 years, trying to list challenges and best practice protocols using sequencing-based technologies for microbiome analysis. A drawback of the NGS method lies in the possibility of generating overlapped short-read sequences since the DNA is partially digested with enzymes into lengths that are readily sequenced. In this framework, TGS platform like PacBio (Pacific Biosciences RS II/Sequel) and Oxford Nanopore MinION sequencing technologies are attracting the attention of the molecular ecologist showing promising results since they produce very long reads (from 1 to 100 kb; Santos et al., 2020; Bharti and Grimm, 2021). However, despite the recent output improvement of Sequel from ∼0.5–1 to ∼5–10 Gb, the application remains scarce. The Illumina sequencing platform produces high read counts. However, computational power and intensive time-consuming work is required to assign taxonomic identity due to the large and multivariate data structure. The sequences can be assembled to recreate the entire sequence of targeted microorganisms’ genomes in soil, but the results cannot be used as specific and discriminant characters for single microbial inoculants. Moreover, the estimation of microbial diversity obtained with the analysis depends on the resolution and length of the sequence of the rRNA gene marker used and the quality of sequenced libraries, the computational bioinformatics parameters used and the whole process workflow. On the other side, long reads sequence allows identifying rare genomes inaccessible compared to the short-reads platform. However, both sequencing generations platform suffer from many biases in sample collection, nucleic acid extraction, experimental errors and bioinformatic analysis. In the last years, modern sequencing technologies have taken over the Sanger classical technologies for metagenomic profiling of microbial communities. Specific genetic markers (Shen et al., 2021) used to identify target microbes from several environments (63F-1087r of 16S rRNA gene; ITS1-4 or ITS2-4, β-tubulin, calmodulin gene) with the classical Sanger approach do not overlap with standardised barcodes (V3-V4 or V3-V4 or V7-V8 for 16S rRNA, ITS1f- ITS2) used to build an international collection of sequences derived from the taxonomical classification of the communities with NGS platform (Cristescu, 2014). This generates an increasing gap between classical morphological and DNA-based identification (Cristescu, 2014). Owing to this gap, the identification process consisting of taxonomic assignment based on the alignment of sequences with international database (NCBI) suffers from errors that can influence microbial diversity estimates. A robust taxonomic inference can help us calibrate microbial diversity estimates and prevent erroneous interpretations. It easy to imagine that if we start from a good knowledge based on morphological, physiological, or functional traits, we will likely able to generate specie-specific barcode. Overall, we regard metabarcoding of complex bacterial and fungal communities as a highly promising approach to the discovery of patterns of complex communities.
Future Perspectives and Conclusion
The prospect of manipulating crop microbial populations by inoculating beneficial bacteria and fungi to increase plant growth has shown considerable aptitude in laboratory and greenhouse studies; however, responses have been variable in the field (e.g., Arora, 2015; Kaminsky et al., 2019; Malusá et al., 2020). Recent progress in understanding the biological interactions between the practical requirements for microbial inoculant formulation and delivery systems will increase this technology’s evenness in the field and facilitate its commercial development.
The use of multi-strain inocula of microorganisms with known functions and bioinocula with different desirable traits and tolerance to environmental conditions (Ahmad et al., 2008; Vassileva et al., 2020) may increase the consistency of results in the field. Nevertheless, tracking and monitoring the fate and metabolic activity of microbial inoculants and their impact on rhizosphere and soil microbial communities are needed to assure their safe and trustworthy application to crops. A combination of classical and molecular techniques should be used to monitor any inoculant’s strain after its release into the soil. Both culture-based and culture-independent approaches have their specific advantages and limitations. Appropriate metabolic and physiological characterization of the microorganisms used for the formulation of bioinoculants would bridge the culture-independent and the culture-dependent methods. The chance of designing optimized and custom specific probes with high specificity increases when a proper identification and genetic characterization of the species involved in the formulation of a soil microbial inoculant is available. This strongly contributes to the success of detection and monitoring analysis that also depends on a proper sampling method which shall assure the quality and the robustness of the data generated.
The genetic or functional characterization of the inoculant allows to fully exploit the potential of PCR-based methods that tackle species-specific functional traits or genome polymorphism, increasing the power of sensibility, specificity and sensitivity of the technique. However, although many available methods can be coupled to address the request of a discriminant and specific detection tool to monitor introduced bioinocula strains in soil, it is generally acknowledged that accurate and standards methods are yet to be developed. An impetus toward this goal could derive from the field of microfluidics applications and tools like the “lab-on-chip” (Aleklett et al., 2018) could have the potential to address some of the major challenges of tracking and monitoring of bioinoculants. The “lab-on-chip” (microarray) technology opens the avenue for the parallel, high-throughput multiple identification of target microorganisms and genes in environmental samples (Wilson et al., 2002; Chandler et al., 2010; Schreiner et al., 2010; Mocali et al., 2020). Data obtained with amplification microarrays correlated well with conventional small subunit rRNA qPCR results (Chandler et al., 2010). Microarrays would also evaluate bioinocula functional activity; in the case of expressed genes, they represent one of the most powerful approaches due to their unique ability to query the mRNA expression. Environmental DNA barcoding can be used to monitor the impacts of introduced species on soil autochthonous microbial diversity, evaluating the sensitivity of vulnerable microbial native species, and better identify their distribution. However, it is not valuable for species-specific tracking.
For all these reasons, a polyphasic approach, comprising the use of different techniques, would be most practical and suitable for monitoring microbial inoculants in soil. This approach would allow fine-tuning the application method for different kinds of bioinocula to better foster the efficacy of the bioinocula, to better assess the bioinocula impact on native microbial communities, finally, resulting in an increased practical application of biofertilisers and biopesticides.
Author Contributions
LC and AM: conceptualization. AM, LC, FPi, CC, and FPa: methodology. LC, CC, and FPa: formal analysis. LC, AM, FPi, EM, and SM: writing—original draft preparation. EM, LC, AM, and FPi: writing—review and editing. All authors have read and agreed to the published version of the manuscript.
Funding
This work was conducted in the frame of the EXCALIBUR project, funded by the European Union’s Horizon 2020 Research and Innovation Program (Grant Agreement No. 817946) and the H2020- MSCA-IF-EF-SE project “AlienInSoil” N.892048 awarded to FPi.
Conflict of Interest
The authors declare that the research was conducted in the absence of any commercial or financial relationships that could be construed as a potential conflict of interest.
Publisher’s Note
All claims expressed in this article are solely those of the authors and do not necessarily represent those of their affiliated organizations, or those of the publisher, the editors and the reviewers. Any product that may be evaluated in this article, or claim that may be made by its manufacturer, is not guaranteed or endorsed by the publisher.
Supplementary Material
The Supplementary Material for this article can be found online at: https://www.frontiersin.org/articles/10.3389/fmicb.2021.698491/full#supplementary-material
Supplementary Table 1 | EndNote file.
Footnotes
References
Abbasi, P. A., Miller, S. A., Meulia, T., Hoitink, H. A. J., and Kim, J. M. (1999). Precise detection and tracing of Trichoderma hamatum 382 in compost- amended potting mixes by using molecular markers. Appl. Environ. Microbiol. 65, 5421–5426. doi: 10.1128/aem.65.12.5421-5426.1999
Adesemoye, A. O., Torbert, H. A., and Kloepper, J. W. (2009). Plant growth-promoting rhizobacteria allow reduced application rates of chemical fertilizers. Microb. Ecol. 58, 921–929. doi: 10.1007/s00248-009-9531-y
Adiani, V., Gupta, S., and Variyar, P. S. (2020). Microbial quality assessment of minimally processed pineapple using GCMS and FTIR in tandem with chemometrics. Sci. Rep. 10:6203. doi: 10.1038/s41598-020-62895-y
Agronskaia, A. V., Valentijn, J. A., van Driel, L. F., Schneijdenberg, C. T. W. M., Humbel, B. M., van Bergen en Henegouwen, P. M. P., et al. (2008). Integrated fluorescence and transmission electron microscopy. J. Struct. Biol. 164, 183–189. doi: 10.1016/j.jsb.2008.07.003
Ahmad, F., Ahmad, I., and Khan, M. S. (2008). Screening of free-living rhizospheric bacteria for their multiple plant growth promoting activities. Microbiol. Res. 163, 173–181. doi: 10.1016/j.micres.2006.04.001
Al-Awadhi, H., Dashti, N., Khanafer, M., Al-Mailem, D., Ali, N., and Radwan, S. (2013). Bias problems in culture-independent analysis of environmental bacterial communities: a representative study on hydrocarbonoclastic bacteria. Springerplus 2, 1–11. doi: 10.1186/2193-1801-2-369
Al-Dhabaan, F. A. M., and Bakhali, A. H. (2017). Analysis of the bacterial strains using Biolog plates in the contaminated soil from Riyadh community. Saudi J. Biol. Sci. 24, 901–906. doi: 10.1016/j.sjbs.2016.01.043
Aleklett, K., Kiers, E. T., Ohlsson, P., Shimizu, T. S., Caldas, V. E., and Hammer, E. C. (2018). Build your own soil: exploring microfluidics to create microbial habitat structures. ISME J. 12, 312–319. doi: 10.1038/ismej.2017.184
Alexander, M., and Clark, F. E. (2016). Nitrifying bacteria. 1477–1483. doi: 10.2134/agronmonogr9.2.c51
Allison, S. D., and Martiny, J. B. H. (2009). Resistance, resilience, and redundancy in microbial communities. Light Evol. 2, 149–166. doi: 10.17226/12501
Almstrand, R., Daims, H., Persson, F., Sörensson, F., and Hermansson, M. (2013). New methods for analysis of spatial distribution and coaggregation of microbial populations in complex biofilms. Appl. Environ. Microbiol. 79, 5978–5987. doi: 10.1128/AEM.01727-1713
Arora, N. K. (2015). Plant Microbes Symbiosis: Applied Facets. Berlin: Springer. doi: 10.1007/978-81-322-2068-2068
Ayrapetyan, M., Williams, T. C., Baxter, R., and Oliver, J. D. (2015). Viable but nonculturable and persister cells coexist stochastically and are induced by human serum. Infect. Immun. 83, 4194–4203. doi: 10.1128/IAI.00404-415
Babić, K. H., Schauss, K., Hai, B., Sikora, S., Redžepović, S., Radl, V., et al. (2008). Influence of different Sinorhizobium meliloti inocula on abundance of genes involved in nitrogen transformations in the rhizosphere of alfalfa (Medicago sativa L.). Environ. Microbiol. 10, 2922–2930. doi: 10.1111/j.1462-2920.2008.01762.x
Bankhead, S. B., Landa, B. B., Lutton, E., Weller, D. M., and Gardener, B. B. M. S. (2004). Minimal changes in rhizobacterial population structure following root colonization by wild type and transgenic biocontrol strains. FEMS Microbiol. Ecol. 49, 307–318. doi: 10.1016/j.femsec.2004.04.005
Barberio, C., Pagliai, L., Cavalieri, D., and Fani, R. (2001). Biodiversity and horizontal gene transfer in culturable bacteria isolated from activated sludge enriched in nonylphenol ethoxylates. Res. Microbiol. 152, 105–112. doi: 10.1016/S0923-2508(00)01173-1176
Bardi, L., and Malusá, E. (2012). “Drought and nutritional stresses in plant: alleviating role of rhizospheric microorganisms”, in Abiotic Stress: New Research eds N. Haryana and S. Punj (Hauppauge, NY: Nova Science Publishers, Inc.), 1–57.
Bargaz, A., Lyamlouli, K., Chtouki, M., Zeroual, Y., and Dhiba, D. (2018). Soil microbial resources for improving fertilizers efficiency in an integrated plant nutrient management system. Front. Microbiol. 9:1606. doi: 10.3389/fmicb.2018.01606
Basu, A., Prasad, P., Das, S. N., Kalam, S., Sayyed, R. Z., Reddy, M. S., et al. (2021). Plant growth promoting rhizobacteria (Pgpr) as green bioinoculants: recent developments, constraints, and prospects. Sustain 13, 1–20. doi: 10.3390/su13031140
Bharti, R., and Grimm, D. G. (2021). Current challenges and best-practice protocols for microbiome analysis. Brief. Bioinform. 22, 178–193. doi: 10.1093/bib/bbz155
Bhattacharjee, R. B., Singh, A., and Mukhopadhyay, S. N. (2008). Use of nitrogen-fixing bacteria as biofertiliser for non-legumes: prospects and challenges. Appl. Microbiol. Biotechnol. 80, 199–209. doi: 10.1007/s00253-008-1567-1562
Blagodatskaya, E., and Kuzyakov, Y. (2013). Active microorganisms in soil: critical review of estimation criteria and approaches. Soil Biol. Biochem. 67, 192–211. doi: 10.1016/j.soilbio.2013.08.024
Blaya, J., Lloret, E., Santísima-Trinidad, A. B., Ros, M., and Pascual, J. A. (2016). Molecular methods (digital PCR and real-time PCR) for the quantification of low copy DNA of Phytophthora nicotianae in environmental samples. Pest Manag. Sci. 72, 747–753. doi: 10.1002/ps.4048
Bloem, J., Schouten, A. J., Sørensen, S. J., Rutgers, M., Werf, A., and van der, et al. (2009). “Monitoring and evaluating soil quality,” in Microbiological Methods for Assessing Soil Quality, eds J. Bloem, D. W. Hopkins, and A. Benedetti (Wallingford: CABI), doi: 10.1079/9780851990989.0023
Bodor, A., Bounedjoum, N., Vincze, G. E., Erdeiné Kis, Á, Laczi, K., Bende, G., et al. (2020). Challenges of unculturable bacteria: environmental perspectives. Rev. Environ. Sci. Biotechnol. 19, 1–22. doi: 10.1007/s11157-020-09522-9524
Bradford, M. M. (1976). A rapid and sensitive method for the quantitation of microgram quantities of protein utilizing the principle of protein-dye binding. Anal. Biochem. 72, 248–254. doi: 10.1016/0003-2697(76)90527-90523
Canfora, L., Abu-Samra, N., Tartanus, M., Łabanowska, B. H., Benedetti, A., Pinzari, F., et al. (2017). Co-inoculum of Beauveria brongniartii and B. bassiana shows in vitro different metabolic behaviour in comparison to single inoculums. Sci. Rep. 7:13102. doi: 10.1038/s41598-017-12700-12700
Canfora, L., Bacci, G., Pinzari, F., Lo Papa, G., Dazzi, C., and Benedetti, A. (2014a). Salinity and bacterial diversity: to what extent does the concentration of salt affect the bacterial community in a saline soil? PLoS One 9:e114658. doi: 10.1371/journal.pone.0106662
Canfora, L., Costa, C., Pallottino, F., and Mocali, S. (2021). Trends in soil microbial inoculants research: a science mapping approach to unravel strengths and weaknesses of their application. Agric 11:58. doi: 10.3390/agriculture11020158
Canfora, L., Lo Papa, G., Vittori Antisari, L., Bazan, G., Dazzi, C., and Benedetti, A. (2015). Spatial microbial community structure and biodiversity analysis in “extreme” hypersaline soils of a semiarid Mediterranean area. Appl. Soil Ecol. 93, 120–129. doi: 10.1016/j.apsoil.2015.04.014
Canfora, L., Malusà, E., Tkaczuk, C., Tartanus, M., Łabanowska, B. H., and Pinzari, F. (2016). Development of a method for detection and quantification of B. brongniartii and B. bassiana in soil. Sci. Rep. 6, 96–100. doi: 10.1038/srep22933
Canfora, L., Sbrana, C., Avio, L., Felici, B., Scatà, M. C., Neri, U., et al. (2014b). Risk management tools and the case study Brassica napus: evaluating possible effects of genetically modified plants on soil microbial diversity. Sci. Total Environ. 493, 983–994. doi: 10.1016/j.scitotenv.2014.06.086
Canfora, L., Vendramin, E., Felici, B., Tarricone, L., Florio, A., and Benedetti, A. (2018). Vineyard microbiome variations during different fertilisation practices revealed by 16s rRNA gene sequencing. Appl. Soil Ecol. 125, 71–80. doi: 10.1016/j.apsoil.2017.12.019
Carlos, C., Maretto, D. A., Poppi, R. J., Sato, M. I. Z., and Ottoboni, L. M. M. (2011). Fourier transform infrared microspectroscopy as a bacterial source tracking tool to discriminate fecal E. coli strains. Microchem. J. 99, 15–19. doi: 10.1016/j.microc.2011.03.002
Castanheira, N. L., Dourado, A. C., Pais, I., Semedo, J., Scotti-Campos, P., Borges, N., et al. (2017). Colonization and beneficial effects on annual ryegrass by mixed inoculation with plant growth promoting bacteria. Microbiol. Res. 198, 47–55. doi: 10.1016/j.micres.2017.01.009
Castro-Sowinski, S., Herschkovitz, Y., Okon, Y., and Jurkevitch, E. (2007). Effects of inoculation with plant growth-promoting rhizobacteria on resident rhizosphere microorganisms. FEMS Microbiol. Lett. 276, 1–11. doi: 10.1111/j.1574-6968.2007.00878.x
Cerqueira, L., Azevedo, N. F., Almeida, C., Jardim, T., Keevil, C. W., and Vieira, M. J. (2008). DNA mimics for the rapid identification of microorganisms by fluorescence in situ hybridization (FISH). Int. J. Mol. Sci. 9, 1944–1960. doi: 10.3390/ijms9101944
Chakraborty, C., Doss, C. G. P., Patra, B. C., and Bandyopadhyay, S. (2014). DNA barcoding to map the microbial communities: current advances and future directions. Appl. Microbiol. Biotechnol. 98, 3425–3436. doi: 10.1007/s00253-014-5550-5559
Chandler, D. P., Kukhtin, A., Mokhiber, R., Knickerbocker, C., Ogles, D., Rudy, G., et al. (2010). Monitoring microbial community structure and dynamics during in situ u(vi) bioremediation with a field-portable microarray analysis system. Environ. Sci. Technol. 44, 5516–5522. doi: 10.1021/es1006498
Chaudhary, D. K., Khulan, A., and Kim, J. (2019). Development of a novel cultivation technique for uncultured soil bacteria. Sci. Rep. 9:6666. doi: 10.1038/s41598-019-43182-x
Chaudhary, D., Narula, N., Sindhu, S. S., and Behl, R. K. (2013). Plant growth stimulation of wheat (Triticum aestivum L.) by inoculation of salinity tolerant Azotobacter strains. Physiol. Mol. Biol. Plants 19, 515–519. doi: 10.1007/s12298-013-0178-172
Clesceri, L., Greenberg, A., and Eaton, A. (1999). “Standard methods for the examination of water and wastewater,” in Standard Methods for the Examination of Water and Wastewater, 20 th Edn, eds L. Clesceri, A. Greenberg, and A. Eaton (Washington, DC: American Public Health Association).
Costa, C., Schurr, U., Loreto, F., Menesatti, P., and Carpentier, S. (2019). Plant phenotyping research trends, a science mapping approach. Front. Plant Sci. 9:1933. doi: 10.3389/fpls.2018.01933
Couillerot, O., Poirier, M. A., Prigent-Combaret, C., Mavingui, P., Caballero-Mellado, J., and Moënne-Loccoz, Y. (2010). Assessment of SCAR markers to design real-time PCR primers for rhizosphere quantification of Azospirillum brasilense phytostimulatory inoculants of maize. J. Appl. Microbiol. 109, 528–538. doi: 10.1111/j.1365-2672.2010.04673.x
Creamer, R. E., Hannula, S. E., Leeuwen, J. P. V., Stone, D., Rutgers, M., Schmelz, R. M., et al. (2016). Ecological network analysis reveals the inter-connection between soil biodiversity and ecosystem function as affected by land use across Europe. Appl. Soil Ecol. 97, 112–124. doi: 10.1016/j.apsoil.2015.08.006
Cristescu, M. E. (2014). From barcoding single individuals to metabarcoding biological communities: towards an integrative approach to the study of global biodiversity. Trends Ecol. Evol. 29, 566–571. doi: 10.1016/j.tree.2014.08.001
Curtis, T. P., Sloan, W. T., and Scannell, J. W. (2002). Estimating prokaryotic diversity and its limits. Proc. Natl. Acad. Sci. U. S. A. 99, 10494–10499. doi: 10.1073/pnas.142680199
Daniel, R. (2005). The metagenomics of soil. Nat. Rev. Microbiol. 3, 470–478. doi: 10.1038/nrmicro1160
Dauch, A. L., Watson, A. K., and Jabaji-Hare, S. H. (2003). Detection of the biocontrol agent Colletotrichum coccodes (183088) from the target weed velvetleaf and from soil by strain-specific PCR markers. J. Microbiol. Methods 55, 51–64. doi: 10.1016/S0167-7012(03)00116-117
Davis, K. E. R., Joseph, S. J., and Janssen, P. H. (2005). Effects of growth medium, inoculum size, and incubation time on culturability and isolation of soil bacteria. Appl. Environ. Microbiol. 71, 826–834. doi: 10.1128/AEM.71.2.826-834.2005
Derkowska, E., Paszt, L. S., Dyki, B., and Sumorok, B. (2015). Assessment of mycorrhizal frequency in the roots of fruit plants using different dyes. Adv. Microbiol. 05, 54–64. doi: 10.4236/aim.2015.51006
Dolci, P., Guglielmo, F., Secchi, F., and Ozino, O. I. (2006). Persistence and efficacy of Beauveria brongniartii strains applied as biocontrol agents against melolontha melolontha in the valley of aosta (northwest Italy). J. Appl. Microbiol. 100, 1063–1072. doi: 10.1111/j.1365-2672.2006.02808.x
Eichorst, S. A., Breznak, J. A., and Schmidt, T. M. (2007). Isolation and characterization of soil bacteria that define Terriglobus gen. nov., in the phylum Acidobacteria. Appl. Environ. Microbiol. 73, 2708–2717. doi: 10.1128/AEM.02140-2146
Elsas, J. D., Travors, J. T., Rosado, A. S., and Nannipieri, P. (2019). Modern Soil Microbiology, 3rd Edn. Boca Raton, FL: CRC Press.
Enkerli, J., Widmer, F., and Keller, S. (2004). Long-term field persistence of Beauveria brongniartii strains applied as biocontrol agents against European cockchafer larvae in Switzerland. Biol. Control 29, 115–123. doi: 10.1016/S1049-9644(03)00131-132
Enkerli, J., Widmer, F., Gessler, C., and Keller, S. (2001). Strain-specific microsatellite markers in the entomopathogenic fungus Beauveria brongniartii. Mycol. Res. 105, 1079–1087. doi: 10.1017/S0953756201004543
Fàtu, A. F. Ă, Dinu, M., Ciornei, C., and Andrei, A. (2015). Biological control of Melolontha melolontha L. larvae with entomopathogenic bioinsecticide based on beauveria brongniartii. AgroLife Sci. J. 4, 64–69.
Felici, C., Vettori, L., Toffanin, A., and Nuti, M. (2008). Development of a strain-specific genomic marker for monitoring a Bacillus subtilis biocontrol strain in the rhizosphere of tomato. FEMS Microbiol. Ecol. 65, 289–298. doi: 10.1111/j.1574-6941.2008.00489.x
Feng, G., Xie, T., Wang, X., Bai, J., Tang, L., Zhao, H., et al. (2018). Metagenomic analysis of microbial community and function involved in cd-contaminated soil. BMC Microbiol. 18:11. doi: 10.1186/s12866-018-1152-1155
Feofilova, E. P. (2001). The kingdom fungi: heterogeneity of physiological and biochemical properties and relationships with plants, animals, and prokaryotes (review). Appl. Biochem. Microbiol. 37, 124–137. doi: 10.1023/A:1002863311534
Field, D., Garrity, G., Gray, T., Morrison, N., Selengut, J., Tatusova, T., et al. (2008). The minimun information about a genome sequence. Nat. Biotechnol. 26, 541–547.
Fierer, N., Ladau, J., Clemente, J. C., Leff, J. W., Owens, S. M., Pollard, K. S., et al. (2013). Reconstructing the microbial diversity and function of pre-agricultural tallgrass prairie soils in the United States. Science 342, 621–624. doi: 10.1126/science.1243768
Frąc, M., Weber, J., Gryta, A., Dębicka, M., Kocowicz, A., Jamroz, E., et al. (2017). Microbial functional diversity in podzol ectohumus horizons affected by alkaline fly ash in the vicinity of electric power plant. Geomicrobiol. J. 34, 579–586. doi: 10.1080/01490451.2016.1220651
Frey, B., Vilariño, A., SchÜepp, H., and Arines, J. (1994). Chitin and ergosterol content of extraradical and intraradical mycelium of the vesicular-arbuscular mycorrhizal fungus Glomus intraradices. Soil Biol. Biochem. 26, 711–717. doi: 10.1016/0038-0717(94)90263-90261
Fu, L., Penton, C. R., Ruan, Y., Shen, Z., Xue, C., Li, R., et al. (2017). Inducing the rhizosphere microbiome by biofertilizer application to suppress banana Fusarium wilt disease. Soil Biol. Biochem. 104, 39–48. doi: 10.1016/j.soilbio.2016.10.008
Fujiyoshi, M., Nakatsubo, T., Ogura, S., and Horikoshi, T. (2000). Estimation of mycelial biomass of arbuscular mycorrhizal fungi associated with the annual legume Kummerowia striata by ergosterol analysis. Ecol. Res. 15, 121–131. doi: 10.1046/j.1440-1703.2000.00329.x
Gao, G., Yin, D., Chen, S., Xia, F., Yang, J., Li, Q., et al. (2012). Effect of biocontrol agent Pseudomonas fluorescens 2P24 on soil fungal community in cucumber rhizosphere using T-RFLP and DGGE. PLoS One 7:e31806. doi: 10.1371/journal.pone.0031806
Germaine, K., Keogh, E., Garcia-Cabellos, G., Borremans, B., Van Der Lelie, D., Barac, T., et al. (2004). Colonisation of poplar trees by gfp expressing bacterial endophytes. FEMS Microbiol. Ecol. 48, 109–118. doi: 10.1016/j.femsec.2003.12.009
Goldstein, J. I., Newbury, D. E., Michael, J. R., Ritchie, N. W. M., Scott, J. H. J., and Joy, D. C. (2018). Microscopy and X-Ray Microanalysis. New York, NY: Springer-Verlag.
Green, J., and Bohannan, B. J. M. (2006). Spatial scaling of microbial biodiversity. Trends Ecol. Evol. 21, 501–507. doi: 10.1016/j.tree.2006.06.012
Griffiths, B. S., and Philippot, L. (2013). Insights into the resistance and resilience of the soil microbial community. FEMS Microbiol. Rev. 37, 112–129. doi: 10.1111/j.1574-6976.2012.00343.x
Grosch, R., Schneider, J. H. M., Peth, A., Waschke, A., Franken, P., Kofoet, A., et al. (2007). Development of a specific PCR assay for the detection of Rhizoctonia solani AG 1-IB using SCAR primers. J. Appl. Microbiol. 102, 806–819. doi: 10.1111/j.1365-2672.2006.03125.x
Gruber-Vodicka, H. R., Dirks, U., Leisch, N., Baranyi, C., Stoecker, K., Bulgheresi, S., et al. (2011). Paracatenula, an ancient symbiosis between thiotrophic Alphaproteobacteria and catenulid flatworms. Proc. Natl. Acad. Sci. U. S. A. 108, 12078–12083. doi: 10.1073/pnas.1105347108
Guarro, J., Gené, J., and Stchigel, A. M. (1999). Developments in fungal taxonomy. Clin. Microbiol. Rev. 12, 454–500. doi: 10.1128/cmr.12.3.454
Han, I., Lee, T. K., Han, J., Doan, T., Van, Kim, S. B., et al. (2012). Improved detection of microbial risk of releasing genetically modified bacteria in soil by using massive sequencing and antibiotic resistance selection. J. Hazard. Mater. 22, 172–178. doi: 10.1016/j.jhazmat.2012.05.031
Harman, G. E., and Uphoff, N. (2019). Symbiotic root-endophytic soil microbes improve crop productivity and provide environmental benefits. Scientifica (Cairo). 2019:9106395. doi: 10.1155/2019/9106395
Hart, M. M., and Reader, R. J. (2002). Taxonomic basis for variation in the colonization strategy of arbuscular mycorrhizal fungi. New Phytol. 153, 335–344. doi: 10.1046/j.0028-646X.2001.00312.x
Hartmann, A., Schmid, M., van Tuinen, D., and Berg, G. (2009). Plant-driven selection of microbes. Plant Soil 321, 235–257. doi: 10.1007/s11104-008-9814-y
Hayman, D. S. (1984). “Methods for evaluating and manipulating vesicular-arbuscular mycorrhiza,” in Microbiological Methods for Environmental Microbiology, eds J. M. Lynch and J. M. Grainger (London: Academic Press).
Hebert, P. D. N., Cywinska, A., Ball, S. L., and DeWaard, J. R. (2003). Biological identifications through DNA barcodes. Proc. R. Soc. B Biol. Sci. 270, 313–321. doi: 10.1098/rspb.2002.2218
Hengeveld, R., MacArthur, R. H., and Wilson, E. O. (2002). The theory of island biogeography. Acta Biotheor. 50, 133–136. doi: 10.1023/A:1016393430551
Hirsch, P. R., Mauchline, T. H., and Clark, I. M. (2010). Culture-independent molecular techniques for soil microbial ecology. Soil Biol. Biochem. 42, 878–887. doi: 10.1016/j.soilbio.2010.02.019
Horaczek, A., and Viernstein, H. (2004). Comparison of three commonly used drying technologies with respect to activity and longevity of aerial conidia of Beauveria brongniartii and Metarhizium anisopliae. Biol. Control 31, 65–71. doi: 10.1016/j.biocontrol.2004.04.016
Howe, A. C., Jansson, J. K., Malfatti, S. A., Tringe, S. G., Tiedje, J. M., and Brown, C. T. (2014). Tackling soil diversity with the assembly of large, complex metagenomes. Proc. Natl. Acad. Sci. U. S. A. 111, 4904–4909. doi: 10.1073/pnas.1402564111
Huang, B., Bates, M., and Zhuang, X. (2009). Super-resolution fluorescence microscopy. Annu. Rev. Biochem. 78, 993–1016. doi: 10.1146/annurev.biochem.77.061906.092014
Huang, W. E., Stoecker, K., Griffiths, R., Newbold, L., Daims, H., Whiteley, A. S., et al. (2007). Raman-FISH: combining stable-isotope Raman spectroscopy and fluorescence in situ hybridization for the single cell analysis of identity and function. Environ. Microbiol. 9, 1878–1889. doi: 10.1111/j.1462-2920.2007.01352.x
Jansson, J. K., and Hofmockel, K. S. (2018). The soil microbiome — from metagenomics to metaphenomics. Curr. Opin. Microbiol. 43, 162–168. doi: 10.1016/j.mib.2018.01.013
Johnson, M.B, and Criss, A. K. (2013). Fluorescence microscopy methods for determining the viability of bacteria in association with mammalian cells. J. Vis. Exp. 79:50729. doi: 10.3791/50729
Kaeberlein, T., Lewis, K., and Epstein, S. S. (2002). Isolating “uncultivabte” microorganisms in pure culture in a simulated natural environment. Science 296, 1127–1129. doi: 10.1126/science.1070633
Kaminsky, L. M., Trexler, R. V., Malik, R. J., Hockett, K. L., and Bell, T. H. (2019). The inherent conflicts in developing soil microbial inoculants. Trends Biotechnol. 37, 140–151. doi: 10.1016/j.tibtech.2018.11.011
Kandel, S. L., Herschberger, N., Kim, S. H., and Doty, S. L. (2015). Diazotrophic endophytes of poplar and willow for growth promotion of rice plants in nitrogen-limited conditions. Crop Sci. 55, 1765–1772. doi: 10.2135/cropsci2014.08.0570
Kandel, S., Joubert, P., and Doty, S. (2017). Bacterial endophyte colonization and distribution within plants. Microorganisms 5:77. doi: 10.3390/microorganisms5040077
Kenzaka, T., and Tani, K. (2012). Scanning electron microscopy imaging of bacteria based on nucleic acid sequences. Scan. Electron Microsc. 49, 796–799. doi: 10.5772/36638
Kenzaka, T., Ishidoshiro, A., Tani, K., and Nasu, M. (2009). Scanning electron microscope imaging of bacteria based on DNA sequence. Lett. Appl. Microbiol. 49, 796–799. doi: 10.1111/j.1472-765X.2009.02680.x
Kessler, P., Enkerl, J., Schweize, C., and Keller, S. (2004). Survival of Beauveria brongniartii in the soil after application as a biocontrol agent against the European cockchafer Melolontha melolontha. BioControl 49, 563–581. doi: 10.1023/B:BICO.0000036441.40227.ed
Kessler, P., Matzke, H., and Keller, S. (2003). The effect of application time and soil factors on the occurrence of Beauveria brongniartii applied as a biological control agent in soil. J. Invertebr. Pathol. 84, 15–23. doi: 10.1016/j.jip.2003.08.003
Khalid, M., Hassani, D., Bilal, M., Asad, F., and Huang, D. (2017). Influence of bio-fertilizer containing beneficial fungi and rhizospheric bacteria on health promoting compounds and antioxidant activity of Spinacia oleracea L. Bot. Stud. 58:35. doi: 10.1186/s40529-017-0189-183
Kim, M., Lee, K.-H., Yoon, S.-W., Kim, B.-S., Chun, J., and Yi, H. (2013). Analytical tools and databases for metagenomics in the next-generation sequencing era. Genomics Inform. 11:102. doi: 10.5808/gi.2013.11.3.102
Kommnick, C., Lepper, A., and Hensel, M. (2019). Correlative light and scanning electron microscopy (CLSEM) for analysis of bacterial infection of polarized epithelial cells. Sci. Rep. 9:17079. doi: 10.1038/s41598-019-53085-53086
Kowalchuk, G. A., Hol, W. H. G., and Van Veena, J. A. (2006). Rhizosphere fungal communities are influenced by Senecio jacobaea pyrrolizidine alkaloid content and composition. Soil Biol. Biochem. 38, 2852–2859. doi: 10.1016/j.soilbio.2006.04.043
Kress, W. J., García-Robledo, C., Uriarte, M., and Erickson, D. L. (2015). DNA barcodes for ecology, evolution, and conservation. Trends Ecol. Evol. 30, 25–35. doi: 10.1016/j.tree.2014.10.008
Lacey, L. A., Grzywacz, D., Shapiro-Ilan, D. I., Frutos, R., Brownbridge, M., and Goettel, M. S. (2015). Insect pathogens as biological control agents: back to the future. J. Invertebr. Pathol. 132, 1–41. doi: 10.1016/j.jip.2015.07.009
Lagier, J. C., Dubourg, G., Million, M., Cadoret, F., Bilen, M., Fenollar, F., et al. (2018). Culturing the human microbiota and culturomics. Nat. Rev. Microbiol. 16, 540–550. doi: 10.1038/s41579-018-0041-40
Lagier, J. C., Khelaifia, S., Alou, M. T., Ndongo, S., Dione, N., Hugon, P., et al. (2016). Culture of previously uncultured members of the human gut microbiota by culturomics. Nat. Microbiol. 1:16203. doi: 10.1038/nmicrobiol.2016.203
Lee, J., Kim, H.-S. I., Young, Jo, H., and Jae Kwon, M. I. (2021). Revisiting soil bacterial counting methods: optimal soil storage and pretreatment methods and comparison of culture-dependent and -independent methods. PLoS One 16:e0246142. doi: 10.1371/journal.pone.0246142
Lin, H. J., Herman, P., and Lakowicz, J. R. (2003). Fluorescence lifetime-resolved pH imaging of living cells. Cytom. Part A 52, 77–89. doi: 10.1002/cyto.a.10028
Liu, W., Huang, L., Komorek, R., Handakumbura, P. P., Zhou, Y., Hu, D., et al. (2020). Correlative surface imaging reveals chemical signatures for bacterial hotspots on plant roots. Analyst 145, 393–401. doi: 10.1039/c9an01954e
Lombard, N., Prestat, E., van Elsas, J. D., and Simonet, P. (2011). Soil-specific limitations for access and analysis of soil microbial communities by metagenomics. FEMS Microbiol. Ecol. 78, 31–49. doi: 10.1111/j.1574-6941.2011.01140.x
Lovelock, C. E., Wright, S. F., and Nichols, K. A. (2004). Using glomalin as an indicator for arbuscular mycorrhizal hyphal growth: an example from a tropical rain forest soil. Soil Biol. Biochem. 36, 1009–1012. doi: 10.1016/j.soilbio.2004.02.010
Lucy, M., Reed, E., and Glick, B. R. (2004). Applications of free living plant growth-promoting rhizobacteria. Antonie van Leeuwenhoek Int. J. Gen. Mol. Microbiol. 86, 1–25. doi: 10.1023/B:ANTO.0000024903.10757.6e
Lueders, T., and Friedrich, M. W. (2003). Evaluation of PCR amplification bias by terminal restriction fragment length polymorphism analysis of small-subunit rRNA and mcrA genes by using defined template mixtures of methanogenic pure cultures and soil DNA extracts. Appl. Environ. Microbiol. 69, 320–326. doi: 10.1128/AEM.69.1.320-326.2003
Lukumbuzya, M., Schmid, M., Pjevac, P., and Daims, H. (2019). A multicolor fluorescence in situ hybridization approach using an extended set of fluorophores to visualize microorganisms. Front. Microbiol. 10:1383. doi: 10.3389/fmicb.2019.01383
Majed, N., Chernenko, T., Diem, M., and Gu, A. Z. (2012). Identification of functionally relevant populations in enhanced biological phosphorus removal processes based on intracellular polymers profiles and insights into the metabolic diversity and heterogeneity. Environ. Sci. Technol. 46, 5010–5017.
Mallon, C. A., Le Roux, X., Van Doorn, G. S., Dini-Andreote, F., Poly, F., and Salles, J. F. (2018). The impact of failure: unsuccessful bacterial invasions steer the soil microbial community away from the invader’s niche. ISME J. 12, 728–741. doi: 10.1038/s41396-017-0003-y
Mallon, C., Poly, F., Le Roux, X., Marring, I., van Elsas, J. D., and Salles, J. F. (2015). Resource pulses can alleviate the biodiversity – invasion relationship in soil microbial communities. Ecology 96, 915–926. doi: 10.1890/14-1001.1
Malusá, E., Pinzari, F., and Canfora, L. (2016). “Efficacy of biofertilizers: challenges to improve crop production,” in Microbial Inoculants in Sustainable Agricultural Productivity (New Delhi: Springer), 17–40. doi: 10.1007/978-81-322-2644-4_2
Malusá, E., Tartanus, M., Furmanczyk, E. M., and Łabanowska, B. H. (2020). Holistic approach to control Melolontha spp. in organic strawberry plantations. Org. Agric. 10, 13–22. doi: 10.1007/s13165-020-00295-292
Mandakovic, D., Rojas, C., Maldonado, J., Latorre, M., Travisany, D., Delage, E., et al. (2018). Structure and co-occurrence patterns in microbial communities under acute environmental stress reveal ecological factors fostering resilience. Sci. Rep. 8:5875. doi: 10.1038/s41598-018-23931-23930
Marsh, B. A’B. (1971). Measurement of length in random arrangements of lines. J. App. Ecol. 8, 265–267. doi: 10.2307/2402144
Mawarda, P. C., Le Roux, X., Dirk van Elsas, J., and Salles, J. F. (2020). Deliberate introduction of invisible invaders: a critical appraisal of the impact of microbial inoculants on soil microbial communities. Soil Biol. Biochem. 148:107874. doi: 10.1016/j.soilbio.2020.107874
Mayerhofer, J., Enkerli, J., Zelger, R., and Strasser, H. (2015). Biological control of the European cockchafer: persistence of Beauveria brongniartii after long-term applications in the Euroregion Tyrol. BioControl 60, 617–629. doi: 10.1007/s10526-015-9671-9676
McGonigle, T. P., Miller, M. H., Evans, D. G., Fairchild, G. L., and Swan, J. A. (1990). A new method which gives an objective measure of colonization of roots by vesicular—arbuscular mycorrhizal fungi. New Phytol. 115, 495–501. doi: 10.1111/j.1469-8137.1990.tb00476.x
McKinnon, A. C., Glare, T. R., Ridgway, H. J., Mendoza-Mendoza, A., Holyoake, A., Godsoe, W. K., et al. (2018). Detection of the entomopathogenic fungus Beauveria bassiana in the rhizosphere of wound-stressed Zea mays plants. Front. Microbiol. 9:1161. doi: 10.3389/fmicb.2018.01161
Mengoni, A., Selvi, F., Cusimano, N., Galardi, F., and Gonnelli, C. (2006). Genetic diversity inferred from AFLP fingerprinting in populations of Onosma echioides (Boraginaceae) from serpentine and calcareous soils. Plant Biosyst. 140, 211–219. doi: 10.1080/11263500600756660
Michaelsen, A., Piñar, G., and Pinzari, F. (2010). Molecular and microscopical investigation of the microflora inhabiting a deteriorated Italian manuscript dated from the thirteenth century. Microb. Ecol. 60, 69–80. doi: 10.1007/s00248-010-9667-9669
Milucka, J., Ferdelman, T. G., Polerecky, L., Franzke, D., Wegener, G., Schmid, M., et al. (2012). Zero-valent sulphur is a key intermediate in marine methane oxidation. Nature 491, 541–546. doi: 10.1038/nature11656
Mitter, E. K., Tosi, M., Obregón, D., Dunfield, K. E., and Germida, J. J. (2021). Rethinking crop nutrition in times of modern microbiology: innovative biofertilizer technologies. Front. Sustain. Food Syst. 5:606815. doi: 10.3389/fsufs.2021.606815
Mocali, S., and Benedetti, A. (2010). Exploring research frontiers in microbiology: the challenge of metagenomics in soil microbiology. Res. Microbiol. 161, 497–505. doi: 10.1016/j.resmic.2010.04.010
Mocali, S., Kuramae, E. E., Kowalchuk, G. A., Fornasier, F., and Priori, S. (2020). Microbial functional diversity in vineyard soils: sulfur metabolism and links with grapevine plants and wine quality. Front. Environ. Sci. 8:75. doi: 10.3389/fenvs.2020.00075
Mocali, S., Landi, S., Curto, G., Dallavalle, E., Infantino, A., Colzi, C., et al. (2015). Resilience of soil microbial and nematode communities after biofumigant treatment with defatted seed meals. Ind. Crops Prod. 75, 79–90. doi: 10.1016/j.indcrop.2015.04.031
Moore, E. R. B., Mihaylova, S. A., Vandamme, P., Krichevsky, M. I., and Dijkshoorn, L. (2010). Microbial systematics and taxonomy: relevance for a microbial commons. Res. Microbiol. 161, 430–438. doi: 10.1016/j.resmic.2010.05.007
Morgan, J. L., Darling, A. E., and Eisen, J. A. (2010). Metagenomic sequencing of an in vitro-simulated microbial community. PLoS One 5:e10209. doi: 10.1371/journal.pone.0010209
Morriën, E., Hannula, S. E., Snoek, L. B., Helmsing, N. R., Zweers, H., De Hollander, M., et al. (2017). Soil networks become more connected and take up more carbon as nature restoration progresses. Nat. Commun. 8:14349. doi: 10.1038/ncomms14349
Nannipieri, P., Penton, C. R., Purahong, W., Schloter, M., and van Elsas, J. D. (2019). Recommendations for soil microbiome analyses. Biol. Fertil. Soils 55, 765–766. doi: 10.1007/s00374-019-01409-z
Naranjo-Ortiz, M. A., and Gabaldón, T. (2019). Fungal evolution: diversity, taxonomy and phylogeny of the Fungi. Biol. Rev. 94, 2101–2137. doi: 10.1111/brv.12550
Naseer, I., Ahmad, M., Nadeem, S. M., Ahmad, I., and Najm-ul-Seher, and Zahir, Z. A. (2019). “Rhizobial inoculants for sustainable agriculture: prospects and applications,” in Biofertilizers for Sustainable Agriculture and Environment. Soil Biology, Vol. 55, eds B. Giri, R. Prasad, Q. S. Wu, and A. Varma (Cham: Springer), doi: 10.1007/978-3-030-18933-4_11
Nemergut, D. R., Schmidt, S. K., Fukami, T., O’Neill, S. P., Bilinski, T. M., Stanish, L. F., et al. (2013). Patterns and processes of microbial community assembly. Microbiol. Mol. Biol. Rev. 77, 342–356. doi: 10.1128/mmbr.00051-12
Nesme, J., Achouak, W., Agathos, S. N., Bailey, M., Baldrian, P., Brunel, D., et al. (2016). Back to the future of soil metagenomics. Front. Microbiol. 7:73. doi: 10.3389/fmicb.2016.00073
Newman, E. I. (1966). A method of estimating the total length of root in a sample. J. Appl. Ecol. 3:139. doi: 10.2307/2401670
Ngom, B., and Liu, X. (2014). Techniques for tracking microbial community structure and function in natural environment and engineered systems. Int. J. Sci. Res. 3, 800–807.
Nichols, D., Cahoon, N., Trakhtenberg, E. M., Pham, L., Mehta, A., Belanger, A., et al. (2010). Use of ichip for high-throughput in situ cultivation of “uncultivable” microbial species. Appl. Environ. Microbiol. 76, 2445–2450. doi: 10.1128/AEM.01754-1759
Nowrotek, M., Jałowiecki, OvermanŁ, Harnisz, M., and Płaza, G. A. (2019). Culturomics and metagenomics: in understanding of environmental resistome. Front. Environ. Sci. Eng. 13:40. doi: 10.1007/s11783-019-1121-8
Omer, A. M. (2010). Bioformulations of Bacillus spores for using as Biofertilizer. Life Sci. J. 7, 124–131.
Öpik, M., Vanatoa, A., Vanatoa, E., Moora, M., Davison, J., Kalwij, J. M., et al. (2010). The online database MaarjAM reveals global and ecosystemic distribution patterns in arbuscular mycorrhizal fungi (Glomeromycota). New Phytol. 188, 223–241. doi: 10.1111/j.1469-8137.2010.03334.x
Ou, F., McGoverin, C., Swift, S., and Vanholsbeeck, F. (2019). Rapid and cost-effective evaluation of bacterial viability using fluorescence spectroscopy. Anal. Bioanal. Chem. 411, 3653–3663. doi: 10.1007/s00216-019-01848-1845
Overmann, J. (2013). “Principles of enrichment, isolation, cultivation, and preservation of prokaryotes,” in The Prokaryotes: Prokaryotic Biology and Symbiotic Associations, eds. E. Rosenberg, E. F. DeLong, S. Lory, E. Stackebrandt, and F. Thompson (Berlin: Springer), 149–207. doi: 10.1007/978-3-642-30194-0_7
Pace, N. R. (1997). A molecular view of microbial diversity and the biosphere. Science 276, 734–740. doi: 10.1126/science.276.5313.734
Pagano, M., Oehl, F., Silva, G., Maia, L., Silva, D. K., and Cabello, M. (2016). “Advances in arbuscular mycorrhizal taxonomy,” in Recent Advances on Mycorrhizal Fungi. Fungal Biology, ed. M. Pagano (Cham: Springer), doi: 10.1007/978-3-319-24355-9_2
Pangallo, D., Šaková, N., Koreňová, J., Puškárová, A., Kraková, L., Valík, L., et al. (2014). Microbial diversity and dynamics during the production of May bryndza cheese. Int. J. Food Microbiol. 170, 38–43. doi: 10.1016/j.ijfoodmicro.2013.10.015
Pascual, J., Blanco, S., García-López, M., García-Salamanca, A., Bursakov, S. A., Genilloud, O., et al. (2016). Assessing bacterial diversity in the rhizosphere of Thymus zygis growing in the Sierra Nevada National Park (Spain) through culture-dependent and independent approaches. PLoS One 11:e0146558. doi: 10.1371/journal.pone.0146558
Paulo, M. F. B. S., Jean, L. S.-A., Leona, H. V., de, M., Pricles, de, S. G., et al. (2014). Development of a real-time PCR assay for the detection and quantification of Gluconacetobacter diazotrophicus in sugarcane grown under field conditions. African J. Microbiol. Res. 8, 2937–2946. doi: 10.5897/ajmr2014.6779
Pelaez, V., da Silva, L. R., and Araújo, E. B. (2013). Regulation of pesticides: a comparative analysis. Sci. Public Policy 40, 644–656. doi: 10.1093/scipol/sct020
Pellegrino, E., Turrini, A., Gamper, H. A., Cafà, G., Bonari, E., Young, J. P. W., et al. (2012). Establishment, persistence and effectiveness of arbuscular mycorrhizal fungal inoculants in the field revealed using molecular genetic tracing and measurement of yield components. New Phytol. 194, 810–822. doi: 10.1111/j.1469-8137.2012.04090.x
Philippot, L., Ritz, K., Pandard, P., Hallin, S., and Martin-Laurent, F. (2012). Standardisation of methods in soil microbiology: progress and challenges. FEMS Microbiol. Ecol. 82, 1–10. doi: 10.1111/j.1574-6941.2012.01436.x
Phillips, J. M., and Hayman, D. S. (1970). Improved procedures for clearing roots and staining parasitic and vesicular-arbuscular mycorrhizal fungi for rapid assessment of infection. Trans. Br. Mycol. Soc. 55, 158–161, IN16-IN18. doi: 10.1016/s0007-1536(70)80110-80113
Pianka, E. R. (1972). R and K selection or b and d selection? Am. Nat. 106, 581–588. doi: 10.1086/282798
Pickup, R. W. (1991). Development of molecular methods for the detection of specific bacteria in the environment. J. Gen. Microbiol. 137, 1009–1019. doi: 10.1099/00221287-137-5-1009
Pitkäranta, M., Meklin, T., Hyvärinen, A., Nevalainen, A., Paulin, L., Auvinen, P., et al. (2011). Molecular profiling of fungal communities in moisture damaged buildings before and after remediation - a comparison of culture-dependent and culture-independent methods. BMC Microbiol. 11:235. doi: 10.1186/1471-2180-11-235
Ray, P., Lakshmanan, V., Labbé, J. L., and Craven, K. D. (2020). Microbe to microbiome: a paradigm shift in the application of microorganisms for sustainable agriculture. Front. Microbiol. 11:622926. doi: 10.3389/fmicb.2020.622926
Rehner, S. A., and Buckley, E. P. (2003). Isolation and characterization of microsatellite loci from the entomopathogenic fungus Beauveria bassiana (Ascomycota: Hypocreales). Mol. Ecol. Notes 3, 409–411. doi: 10.1046/j.1471-8286.2003.00464.x
Rillig, M. C. (2004). Arbuscular mycorrhizae, glomalin, and soil aggregation. Can. J. Soil Sci. 84, 355–363. doi: 10.4141/S04-003
Rillig, M. C., Ryo, M., Lehmann, A., Aguilar-Trigueros, C. A., Buchert, S., Wulf, A., et al. (2019). The role of multiple global change factors in driving soil functions and microbial biodiversity. Science 366, 886–890. doi: 10.1126/science.aay2832
Robleto, E. A., Borneman, J., and Triplett, E. W. (1998). Effects of bacterial antibiotic production on rhizosphere microbial communities from a culture-independent perspective. Appl. Environ. Microbiol. 64, 5020–5022. doi: 10.1128/aem.64.12.5020-5022.1998
Roesch, L. F. W., Fulthorpe, R. R., Riva, A., Casella, G., Hadwin, A. K. M., Kent, A. D., et al. (2007). Pyrosequencing enumerates and contrasts soil microbial diversity. ISME J. 1, 283–290. doi: 10.1038/ismej.2007.53
Rousk, J., and Bååth, E. (2007). Fungal and bacterial growth in soil with plant materials of different C/N ratios. FEMS Microbiol. Ecol. 62, 258–267. doi: 10.1111/j.1574-6941.2007.00398.x
Salazar, O., Julián, M. C., Hyakumachi, M., and Rubio, V. (2000). Phylogenetic grouping of cultural types of Rhizoctonia solani AG 2–2 based on ribosomal ITS sequences. Mycologia 92, 505–509. doi: 10.1080/00275514.2000.12061186
Santos, A., van Aerle, R., Barrientos, L., and Martinez-Urtaza, J. (2020). Computational methods for 16S metabarcoding studies using Nanopore sequencing data. Comput. Struct. Biotechnol. J. 18, 296–305. doi: 10.1016/j.csbj.2020.01.005
Santos, M. S., Nogueira, M. A., and Hungria, M. (2019). Microbial inoculants: reviewing the past, discussing the present and previewing an outstanding future for the use of beneficial bacteria in agriculture. AMB Express 9:205. doi: 10.1186/s13568-019-0932-930
Schenck, N. C., and Perez, Y. (1990). Manual for the Identification of VA Mycorrhizal Fungi. Gainesville, FL: INVAM.
Schimak, M. P., Kleiner, M., Wetzel, S., Liebeke, M., Dubilier, N., and Fuchs, B. M. (2016). MiL-FISH: multilabeled oligonucleotides for fluorescence in situ hybridization improve visualization of bacterial cells. Appl. Environ. Microbiol. 82, 62–70. doi: 10.1128/AEM.02776-2715
Schmidt, H., and Eickhorst, T. (2014). Detection and quantification of native microbial populations on soil-grown rice roots by catalyzed reporter deposition-fluorescence in situ hybridization. FEMS Microbiol. Ecol. 87, 390–402. doi: 10.1111/1574-6941.12232
Schreiner, K., Hagn, A., Kyselková, M., Moënne-Loccoz, Y., Welzl, G., Munch, J. C., et al. (2010). Comparison of barley succession and take-all disease as environmental factors shaping the rhizobacterial community during take-all decline. Appl. Environ. Microbiol. 76, 4703–4712. doi: 10.1128/AEM.00481-410
Schwarz, H., and Humbel, B. M. (2007). Correlative light and electron microscopy using immunolabeled resin sections. Methods Mol. Biol. 369, 229–256. doi: 10.1385/1-59745-294-7
Schwarzenbach, K., Widmer, F., and Enkerli, J. (2007). Cultivation-independent analysis of fungal genotypes in soil by using simple sequence repeat markers. Appl. Environ. Microbiol. 73, 6519–6525. doi: 10.1128/AEM.01405-1407
Schwieger, F., and Tebbe, C. C. (2000). Effect of field inoculation with Sinorhizobium meliloti L33 on the composition of bacterial communities in rhizospheres of a target plant (Medicago sativa) and a non-target plant (Chenopodium album) - Linking of 16S rRNA gene-based single-strand conforma. Appl. Environ. Microbiol. 66, 3556–3565. doi: 10.1128/AEM.66.8.3556-3565.2000
Sessitsch, A., Pfaffenbichler, N., and Mitter, B. (2019). Microbiome applications from lab to field: facing complexity. Trends Plant Sci. 24, 194–198. doi: 10.1016/j.tplants.2018.12.004
Shade, A., Peter, H., Allison, S. D., Baho, D. L., Berga, M., Bürgmann, H., et al. (2012). Fundamentals of microbial community resistance and resilience. Front. Microbiol. 3:417. doi: 10.3389/fmicb.2012.00417
Sharma, L., Bohra, N., Rajput, V. D., Quiroz-Figueroa, F. R., Singh, R. K., and Marques, G. (2021). Advances in entomopathogen isolation: a case of bacteria and fungi. Microorganisms 9, 1–25. doi: 10.3390/microorganisms9010016
Sharma, S. D., Kumar, P., Bhardwaj, S. K., and Yadav, S. K. (2011). Screening and selecting novel AM fungi and Azotobacter strain for inoculating apple under soil solarization and chemical disinfestation with mulch practices for sustainable nursery management. Sci. Hortic. (Amsterdam). 130, 164–174. doi: 10.1016/j.scienta.2011.06.032
Shen, Y., Nie, J., Kuang, L., Zhang, J., and Li, H. (2021). DNA sequencing, genomes and genetic markers of microbes on fruits and vegetables. Microb. Biotechnol. 14, 323–362. doi: 10.1111/1751-7915.13560
Shoemaker, W. R., Locey, K. J., and Lennon, J. T. (2017). A macroecological theory of microbial biodiversity. Nat. Ecol. Evol. 1:107. doi: 10.1038/s41559-017-0107
Sia, A., Ali, W. A., Al-jaff, B. M. A., and Al-, S. S. S. (2018). Cultivation and detection of unculturable fungi in soil using soil infusion agar (SIA). J. Univ. Anbar Pure Sci. 12, 9–18.
Singh, D. P., and Prabha, R. (2019). Microbial Interventions in Agriculture and Environment. Rhizosphere, Microbiome and Agro-Ecology. Singapore: Springer, doi: 10.1007/978-981-32-9084-9086
Singh, P. K., Singh, M., and Tripathi, B. N. (2013). Glomalin: an arbuscular mycorrhizal fungal soil protein. Protoplasma 250, 663–669. doi: 10.1007/s00709-012-0453-z
Smalla, K., Wieland, G., Buchner, A., Zock, A., Parzy, J., Kaiser, S., et al. (2001). Bulk and rhizosphere soil bacterial communities studied by denaturing gradient gel electrophoresis: plant-dependent enrichment and seasonal shifts revealed. Appl. Environ. Microbiol. 67, 4742–4751. doi: 10.1128/AEM.67.10.4742-4751.2001
Solanki, M., and Garg, F. C. (2014). The use of lacZ marker in enumeration of Azotobacter chroococcum in carrier based inoculants. Brazilian J. Microbiol. 45, 595–601. doi: 10.1590/S1517-83822014000200030
Stefani, F. O. P., Bell, T. H., Marchand, C., De La Providencia, I. E., El Yassimi, A., St-Arnaud, M., et al. (2015). Culture-dependent and -independent methods capture different microbial community fractions in hydrocarbon-contaminated soils. PLoS One 10:e0128272. doi: 10.1371/journal.pone.0128272
Steinberg, P. D., and Rillig, M. C. (2003). Differential decomposition of arbuscular mycorrhizal fungal hyphae and glomalin. Soil Biol. Biochem. 35, 191–194. doi: 10.1016/S0038-0717(02)00249-243
Stockinger, H., Krüger, M., and Schüßler, A. (2010). DNA barcoding of arbuscular mycorrhizal fungi. New Phytol. 187, 461–474. doi: 10.1111/j.1469-8137.2010.03262.x
Studdert, J. P., and Kaya, H. K. (1990). Water potential, temperature, and soil type on the formation of Beauveria bassiana soil colonies. J. Invertebr. Pathol. 56, 380–386. doi: 10.1016/0022-2011(90)90125-P
Sýkorová, Z., Börstler, B., Zvolenská, S., Fehrer, J., Gryndler, M., Vosátka, M., et al. (2012). Long-term tracing of Rhizophagus irregularis isolate BEG140 inoculated on Phalaris arundinacea in a coal mine spoil bank, using mitochondrial large subunit rDNA markers. Mycorrhiza 22, 69–80. doi: 10.1007/s00572-011-0375-371
Tartanus, M., Furmanczyk, E. M., Canfora, L., Pinzari, F., Tkaczuk, C., Majchrowska-Safaryan, A., et al. (2021). Biocontrol of melolontha spp. Grubs in organic strawberry plantations by entomopathogenic fungi as affected by environmental and metabolic factors and the interaction with soil microbial biodiversity. Insects 12, 1–23. doi: 10.3390/insects12020127
Taş, N., de Jong, A. E., Li, Y., Trubl, G., Xue, Y., and Dove, N. C. (2021). Metagenomic tools in microbial ecology research. Curr. Opin. Biotechnol. 67, 184–191. doi: 10.1016/j.copbio.2021.01.019
Timmusk, S., Paalme, V., Lagercrantz, U., and Nevo, E. (2009). Detection and quantification of Paenibacillus polymyxa in the rhizosphere of wild barley (Hordeum spontaneum) with real-time PCR. J. Appl. Microbiol. 107, 736–745. doi: 10.1111/j.1365-2672.2009.04265.x
Trabelsi, D., and Mhamdi, R. (2013). Microbial inoculants and their impact on soil microbial communities: a review. Biomed Res. Int. 2013:11. doi: 10.1155/2013/863240
Trabelsi, D., Ben Ammar, H., Mengoni, A., and Mhamdi, R. (2012). Appraisal of the crop-rotation effect of rhizobial inoculation on potato cropping systems in relation to soil bacterial communities. Soil Biol. Biochem. 54, 1–6. doi: 10.1016/j.soilbio.2012.05.013
Trouvelot, A., Kough, J. L., and Gianinazzi-Pearson, V. (1986). “Mesure du taux de mycorhization VA d’un systeme radiculaire. recherche de methodes d’estimation ayant une signification fonctionnelle,” in Proceedings of the Physiological and Genetical Aspects of Mycorrhizae. Proceedings of the 1st European Symposium on Mycorrhizae, eds V. Gianinazzi-Pearson and S. Gianinazzi (Paris: Institut National de la Recherche Agronomique), 217–221.
Tsui, C. K. M., Woodhall, J., Chen, W., et al. (2011). Molecular techniques for pathogen identification and fungus detection in the environment. IMA Fungus 2, 177–189. doi: 10.5598/imafungus.2011.02.02.09
van Eck, N. J., and Waltman, L. (2010). Software survey: VOSviewer, a computer program for bibliometric mapping. Scientometrics 84, 523–538. doi: 10.1007/s11192-009-0146-143
Van Elsas, J. D., Chiurazzi, M., Mallon, C. A., Elhottova, D., Krištůfek, V., and Salles, J. F. (2012). Microbial diversity determines the invasion of soil by a bacterial pathogen. Proc. Natl. Acad. Sci. U. S. A. 109, 1159–1164. doi: 10.1073/pnas.1109326109
Van Elsas, J. D., Duarte, G. F., Rosado, A. S., and Smalla, K. (1998). Microbiological and molecular biological methods for monitoring microbial inoculants and their effects in the soil environment. J. Microbiol. Methods 32, 133–154. doi: 10.1016/S0167-7012(98)00025-26
Van Kessel, M. A. H. J., Speth, D. R., Albertsen, M., Nielsen, P. H., Op Den, Camp, H. J. M., et al. (2015). Complete nitrification by a single microorganism Europe PMC Funders Group. Nature 528, 555–559. doi: 10.1038/nature16459
Van Oosten, M. J., Di Stasio, E., Cirillo, V., Silletti, S., Ventorino, V., Pepe, O., et al. (2018). Root inoculation with Azotobacter chroococcum 76A enhances tomato plants adaptation to salt stress under low N conditions. BMC Plant Biol. 18:205. doi: 10.1186/s12870-018-1411-1415
Vänninen, I., Tyni-Juslin, J., and Hokkanen, H. (2000). Persistence of augmented Metarhizium anisopliae and Beauveria bassiana in finnish agricultural soils. BioControl 45, 201–222. doi: 10.1023/A:1009998919531
Varjani, S., Srivastava, V. K., Sindhu, R., Thakur, I. S., and Gnansounou, E. (2018). Culture based approaches, dependent and independent, for microbial community fractions in petroleum oil reservoirs. Indian J. Exp. Biol. 56, 444–450.
Vassilev, N., Vassileva, M., Martos, V., Garcia, del Moral, L. F., Kowalska, J., et al. (2020). Formulation of microbial inoculants by encapsulation in natural polysaccharides: focus on beneficial properties of carrier additives and derivatives. Front. Plant Sci. 11:270. doi: 10.3389/fpls.2020.00270
Vassileva, M., Flor-Peregrin, E., Malusá, E., and Vassilev, N. (2020). Towards better understanding of the interactions and efficient application of plant beneficial prebiotics, probiotics, postbiotics and synbiotics. Front. Plant Sci. 11:1068. doi: 10.3389/fpls.2020.01068
Verkade, P. (2008). Moving EM: the rapid transfer system as a new tool for correlative light and electron microscopy and high throughput for high-pressure freezing. J Microsc. 230(Pt 2), 317–328. doi: 10.1111/j.1365-2818.2008.01989.x
Villa, F., Cappitelli, F., Principi, P., Polo, A., and Sorlini, C. (2009). Permeabilization method for in-situ investigation of fungal conidia on surfaces. Lett. Appl. Microbiol. 48, 234–240. doi: 10.1111/j.1472-765X.2008.02520.x
Walsh, U. F., Moënne-Loccoz, Y., Tichy, H. V., Gardner, A., Corkery, D. M., Lorkhe, S., et al. (2003). Residual impact of the biocontrol inoculant Pseudomonas fluorescens F113 on the resident population of rhizobia nodulating a red clover rotation crop. Microb. Ecol. 45, 145–155. doi: 10.1007/s00248-002-2026-2028
Walstad, J. D., Anderson, R. F., and Stambaugh, W. J. (1970). Effects of environmental conditions on two species of muscardine fungi (Beauveria bassiana and Metarrhizium anisopliae). J. Invertebr. Pathol. 16, 221–226. doi: 10.1016/0022-2011(70)90063-90067
Wamberg, C., Christensen, S., and Jakobsen, I. (2003). Interaction between foliar-feeding insects, mycorrhizal fungi, and rhizosphere protozoa on pea plants. Pedobiologia (Jena) 47, 281–287. doi: 10.1078/0031-4056-4191
Wang, Z., Zervas, M. N., Bartlett, P. N., and Wilkinson, J. S. (2016). Surface and waveguide collection of Raman emission in waveguide-enhanced Raman spectroscopy. Opt. Lett. 41:4146. doi: 10.1364/ol.41.004146
Wen, A., Mallik, I., Alvarado, V. Y., Pasche, J. S., Wang, X., Li, W., et al. (2009). Detection, distribution, and genetic variability of “candidatus liberibacter” species associated with zebra complex disease of potato in north america. Plant Dis. 93, 1102–1115. doi: 10.1094/PDIS-93-11-1102
Weyman-Kaczmarkowa, W., and Pȩdziwilk, Z. (2000). The development of fungi as affected by pH and type of soil in relation to the occurrence of bacteria and soil fungistatic activity. Microbiol. Res. 155, 107–112. doi: 10.1016/S0944-5013(00)80045-80042
Wilson, K. H., Wilson, W. J., Radosevich, J. L., DeSantis, T. Z., Viswanathan, V. S., Kuczmarski, T. A., et al. (2002). High-density microarray of small-subunit ribosomal DNA probes. Appl. Environ. Microbiol. 68, 2535–2541. doi: 10.1128/AEM.68.5.2535-2541.2002
Wrede, C., Heller, C., Reitner, J., and Hoppert, M. (2008). Correlative light/electron microscopy for the investigation of microbial mats from Black Sea Cold Seeps. J. Microbiol. Methods 73, 85–91. doi: 10.1016/j.mimet.2008.02.020
Wu, S. C., Cao, Z. H., Li, Z. G., Cheung, K. C., and Wong, M. H. (2005). Effects of biofertilizer containing N-fixer, P and K solubilizers and AM fungi on maize growth: a greenhouse trial. Geoderma 125, 155–166. doi: 10.1016/j.geoderma.2004.07.003
Xing, J. J., Sun, S. S., Wang, H. Z., Brookes, P. C., and Xu, J. M. (2020). Response of soil native microbial community to Escherichia coli O157:H7 invasion. Environ. Pollut. 261:114225. doi: 10.1016/j.envpol.2020.114225
Xu, H. S., Roberts, N., Singleton, F. L., Attwell, R. W., Grimes, D. J., and Colwell, R. R. (1982). Survival and viability of nonculturable Escherichia coli and Vibrio cholerae in the estuarine and marine environment. Microb. Ecol. 8, 313–323. doi: 10.1007/BF02010671
Xu, H., Yang, Y., Tian, Y., Xu, R., Zhong, Y., and Liao, H. (2020). Rhizobium inoculation drives the shifting of rhizosphere fungal community in a host genotype dependent manner. Front. Microbiol. 10:3135. doi: 10.3389/fmicb.2019.03135
Yilmaz, P., Kottmann, R., Field, D., Knight, R., Cole, J. R., Amaral-Zettler, L., et al. (2011). Minimum information about a marker gene sequence (MIMARKS) and minimum information about any (x) sequence (MIxS) specifications. Nat. Biotechnol. 29, 415–420. doi: 10.1038/nbt.1823
Zengler, K., Toledo, G., Rappé, M., Elkins, J., Mathur, E. J., Short, J. M., et al. (2002). Cultivating the uncultured. Proc. Natl. Acad. Sci. U. S. A. 99, 15681–15686. doi: 10.1073/pnas.252630999
Zhong, Y., Yang, Y., Liu, P., Xu, R., Rensing, C., Fu, X., et al. (2019). Genotype and Rhizobium inoculation modulate the assembly of soybean rhizobacterial communities. Plant Cell Environ. 42, 2028–2044. doi: 10.1111/pce.13519
Keywords: soil, detection, microbial inoculants, bacteria, fungi, biofertilisers, biopesticides, microorganisms
Citation: Manfredini A, Malusà E, Costa C, Pallottino F, Mocali S, Pinzari F and Canfora L (2021) Current Methods, Common Practices, and Perspectives in Tracking and Monitoring Bioinoculants in Soil. Front. Microbiol. 12:698491. doi: 10.3389/fmicb.2021.698491
Received: 21 April 2021; Accepted: 28 June 2021;
Published: 31 August 2021.
Edited by:
Jeanette M. Norton, Utah State University, United StatesReviewed by:
Zisis Vryzas, Democritus University of Thrace, GreeceCharles Oluwaseun Adetunji, Edo University, Nigeria
Copyright © 2021 Manfredini, Malusà, Costa, Pallottino, Mocali, Pinzari and Canfora. This is an open-access article distributed under the terms of the Creative Commons Attribution License (CC BY). The use, distribution or reproduction in other forums is permitted, provided the original author(s) and the copyright owner(s) are credited and that the original publication in this journal is cited, in accordance with accepted academic practice. No use, distribution or reproduction is permitted which does not comply with these terms.
*Correspondence: Loredana Canfora, bG9yZWRhbmEuY2FuZm9yYUBjcmVhLmdvdi5pdA==