- 1State Key Laboratory for Conservation and Utilization of Bio-Resources in Yunnan, Key Laboratory for Southwest Microbial Diversity of the Ministry of Education, Yunnan University, Kunming, China
- 2School of Life Sciences, Yunnan University, Kunming, China
- 3Qicai Yunnan Primary School Affiliated with Yunnan Normal University, Kunming, China
- 4Kunming Edible Fungi Institute of All-China Federation of Supply and Marketing Cooperatives, Kunming, China
- 5Research Institute of Nutrition and Food Science, Kunming Medical University, Kunming, China
- 6Department of Biology, McMaster University, Hamilton, ON, Canada
Mitochondrial genes and genomes have patterns of inheritance that are distinctly different from those of nuclear genes and genomes. In nature, the mitochondrial genomes in eukaryotes are generally considered non-recombining and homoplasmic. If heteroplasmy and recombination exist, they are typically very limited in both space and time. Here we show that mitochondrial heteroplasmy and recombination may not be limited to a specific population nor exit only transiently in the basidiomycete Cantharellus cibarius and related species. These edible yellow chanterelles are an ecologically very important group of fungi and among the most prominent wild edible mushrooms in the Northern Hemisphere. At present, very little is known about the genetics and population biology of these fungia cross large geographical distances. Our study here analyzed a total of 363 specimens of edible yellow chanterelles from 24 geographic locations in Yunnan in southwestern China and six geographic locations in five countries in Europe. For each mushroom sample, we obtained the DNA sequences at two genes, one in the nuclear genome and one in the mitochondrial genome. Our analyses of the nuclear gene, translation elongation factor 1-alpha (tef-1) and the DNA barcode of C. cibarius and related species, suggested these samples belong to four known species and five potential new species. Interestingly, analyses of the mitochondrial ATP synthase subunit 6 (atp6) gene fragment revealed evidence of heteroplasmy in two geographic samples in Yunnan and recombination within the two new putative species in Yunnan. Specifically, all four possible haplotypes at two polymorphic nucleotide sites within the mitochondrial atp6 gene were found distributed across several geographic locations in Yunnan. Furthermore, these four haplotypes were broadly distributed across multiple phylogenetic clades constructed based on nuclear tef-1 sequences. Our results suggest that heteroplasmy and mitochondrial recombination might have happened repeatedly during the evolution of the yellow chanterelles. Together, our results suggest that the edible yellow chanterelles represent an excellent system from which to study the evolution of mitochondrial-nuclear genome relationships.
Introduction
Fungi are important components of natural ecosystems. Many fungi also play significant roles in human and animal health, agriculture, biotechnology, and forestry. Some of these fungi grow in a mutually beneficial relationship with the root tips of plants, forming mycorrhizal associations. In these mycorrhizal fungi, their mycelia help plants obtain essential minerals, nitrogen and water from the soil and contribute to the plants’ nutrition, disease resistance and drought tolerance (Brundrett, 2004). In return, plants provide their fungal partners with carbohydrates for their growth and reproduction. In forest ecosystems, one of the most common fungal-plant root associations (called mycorrhiza) involves the Basidiomycetes. Many of these basidiomycete fungi also produce conspicuous fruiting bodies—mushrooms. Some of these mushrooms, such as the chanterelles, are harvested as a source of highly prized food for humans.
Mitochondria are the powerhouse of all eukaryotes, responsible for generating the universal cellular energy currency ATP through oxidative phosphorylation. In fungi, previous studies have shown that mitochondria play many other roles, including aging in eukaryotes (Stojkovic et al., 2017), and virulence (Verma et al., 2018) and resistance to antifungal drugs in fungal pathogens (Vielba-Fernandez et al., 2018). In addition, mitochondrial genomes provide excellent materials for population and evolutionary studies. This is especially true in fungi where diverse patterns of mitochondrial inheritance have been observed, from uniparental to biparental inheritance (Zhou et al., 2010; de la Providencia et al., 2013; Xu and Li, 2015; Fourie et al., 2018; Mendoza et al., 2020). However, aside from a few model organisms, our knowledge about the mechanisms of fungal mitochondrial genomes and their inheritance is very limited (Basse, 2010; Wilson and Xu, 2012; Xu and Wang, 2015). In general, in contrast to the Mendelian inheritance of nuclear genes and genomes, the mitochondrial genes and genomes are inherited uniparentally with a preference for the maternal parent, with little or no evidence of recombination in most fungi, animals, and plants (Birky, 1995). As a consequence, all copies of the mitochondrial DNA (mtDNA) of an individual are typically identical to each other and to one of the parental mitochondrial genomes, a condition known as homoplasmy (Ling et al., 2011). Homoplasmy could be achieved through processes that prevent mtDNA transmission from one of the two parents, including no contribution from one parent due to fertilization mechanisms, targeted elimination of mtDNA from one parent through mitophagy, and/or the restriction-modification system (Shibata and Ling, 2007; Sato and Sato, 2011).
In filamentous fungi, the cells at the junctions of mating where the two parental homokaryons meet may be heteroplasmic, with each mated cell containing mtDNA from both parents [e.g., in the button mushroom Agaricus bisporus (Xu et al., 1996)]. In Baker’s yeast Saccharomyces cerevisiae, the zygotes are almost universally heteroplasmic. However, in the human yeast pathogen Cryptococcus neoformans, heteroplasmy is only found in the zygotes when sex-determining genes are knocked out (Yan and Xu, 2003; Sun et al., 2020). In most instances of observed heteroplasmy, the heteroplasmic cells are typically transient, with rapid segregation of parental mtDNA genotypes into homoplasmic progeny cells to form homoplasmic mycelia and fruiting bodies (Birky, 2001; Barr et al., 2005; Wilson and Xu, 2012; Xu and Wang, 2015). However, while most reported heteroplasmy in fungi were from laboratory crosses, heteroplasmy has also been found in natural strains in a few basidiomycete fungi. For example, in our ongoing investigation of population genetics on edible basidiomycete mushrooms in southwestern China, over 87% of Thelephora ganbajun samples showed evidence of heterozygosity in the mitochondrial genes cox1 or cox3, representing the first evidence of stable heteroplasmy in natural populations of basidiomycete fungi (Wang et al., 2017). In addition, we found clear evidences of phylogenetic incompatibility between two mitochondrial genes (atp6 and cox3) among geographic populations of another basidiomycete mushroom, an Russula virescens ally (Cao et al., 2013). At present, the dynamics of heteroplasmy and recombination, while critical for advancing our knowledge of mitochondrial evolution, has not been critically investigated (Li et al., 2018).
The basidiomycete Cantharellus cibarius and related species are broadly found in the northern hemisphere, from Europe to North America and Asia (Buyck et al., 2014). These mushrooms can form symbiotic ectomycorrhizal relationships with many plants and are often found in mossy coniferous and birch forests. They are among the most prominent wild edible mushrooms in the northern hemisphere. Recently, phylogenetic analyses based on the translation elongation factor 1 (tef-1) has identified that the following 21 species C. altipes, C. tenuithrix, C. lilacinopruinatus, C. ferruginascens, C. amethysteus, C. lewisii, C. lateritius, C. confluens, C. spectaculus, C. roseocanus, C. flavus, C. phasmatis, C. isabellinus, C. tomentosus, C. avellaneus, C. tabernensis, C. appalachiensis, C. decolorans, C. texensis, C. cinnabarinus, were phylogenetically related to C. cibarius (Buyck and Hofstetter, 2011; Foltz et al., 2013; Buyck et al., 2014). However, most of these species appear to be geographically specific. For example, C. cibarius sensu stricto is only found in Scandinavia and northern Japan (Ogawa et al., 2018). Therefore, the idea that Europe and the warm-temperate and subtropical parts of North America have species of Cantharellus in common, has been progressively abandoned. Consequently, it’s increasingly recognized that the use of European species names of Cantharellus for those found in North America and Asia is likely incorrect, especially for those closely related to C. amethysteus and C. cibarius (Redhead et al., 1998; Buyck and Hofstetter, 2011). At present, while updates on Cantharellus taxonomy have progressed relatively quickly in western Europe and North America, that in Asia remains very fragmented. However, recent studies have reported several new chanterelles from China (Shao et al., 2011; Tian et al., 2012; Shao et al., 2014, 2016a, b; An et al., 2017; Jian et al., 2020), Japan (Suhara and Kurogi, 2015; Ogawa et al., 2018), Korea (Antonin et al., 2017; Buyck et al., 2020), Malaysia (Eyssartier et al., 2009; Buyck et al., 2014), India (Das et al., 2015; Buyck et al., 2018), and Iran (Parad et al., 2018). Recently, a large-scale survey of wild edible mushrooms in local markets in Yunnan province, southwestern China, revealed that there was at least one cryptic species within this group of species in this region (Zhang et al., 2021). This study analyzed 96 samples using sequence information at both the tef-1 locus and the internal transcribed spacer (ITS) regions of the ribosomal RNA gene cluster. However, a very conservative cutoff for species delimitation was used in new species estimation in this study (Zhang et al., 2021). Another study identified that most of the edible yellow chanterelles old in the Yunnan local markets belonged to C. Yunnanensis based on both morphological and tef-1sequences, and not the traditionally assumed C. cibarius (Shao et al., 2021). Unfortunately, the rRNA gene cluster often fail to distinguish closely related species in Cantharellus due to unreadable sequence chromatographs and/or limited DNA sequence variations. Sequence information from other genes as well as more extensive sampling is needed to obtain a better understanding of the evolutionary history of Cantharellus and better insights on dispersal routes and speciation events in the Northern Hemisphere (Olariaga et al., 2017).
Chanterelle is the common name for mushrooms in the genus Cantharellus and in other morphologically related genera. The edible yellow chanterelles are among the most popular edible wild mushrooms in the world and include C. cibarius and related species. There are specialized companies that commercialize fruit bodies of chanterelles of different sizes and presentations, e.g., as fresh, frozen, dry, or salted, with geographic specifications. Morphologically, species in yellow chanterelles are very similar to each other and all species are harvested as food and some of them are globally traded. The combined global commercial value of yellow chanterelles has been estimated at more than one billion US dollars annually (Watling, 1997; Wang and Hall, 2004). One of the main global centers of production and harvesting of edible yellow chanterelles is Yunnan Province in Southwestern China. Due to its highly variable climate and diverse topography, Southwestern China (mainly Yunnan Province) is recognized as one of the world’s 34 biodiversity hotspots (Myers et al., 2000). Relevant to this study, Yunnan is also one of the world’s most important areas for wild mushroom harvesting and trading. For example, of the 2,000 or so wild edible mushroom species in the world, over 900 are found in Yunnan (Yang, 2002; Feng and Yang, 2018). Based on ITS barcoding, our recent survey identified a high species diversity of wild mushrooms in local markets, including a large number of putative new taxa (Zhang et al., 2021). Some of these mushrooms are very important to both the local and regional economy. Most of these species are ectomycorrhizal, contribute significantly to the health of trees and forest ecosystems. Examples of these economically important ectomycorrhizal mushrooms around the globe include Boletus edulis, Thelephora ganbajun, Tricholoma matsutake, Russula virescens, Russulavinosa and C. cibarius etc.
In this study, we analyzed the DNA sequence variation in the mitochondrial atp6 gene fragment for a total of 363 mushroom samples of edible yellow chanterelles. Our analyses found that two nucleotide sites within this gene fragment contained sequence heterogeneity within two individual fruiting bodies and that all four possible genotypes at these two sites were found in several geographic populations from Yunnan. Similarly, three additional heterozygous loci were found in another fruiting body collected in a different geographic population. Interestingly, seven out of all eight possible haplotypes at these three nucleotide sites were found in our samples, consistent with recombination. Finally, the phylogenetic distributions of two polymorphic nucleotide sites (sites 101 and 109) within the atp6 gene fragment were compared with sequence variation at the DNA barcode tef-1 for edible yellow chanterelles in Yunnan and Europe.
Materials and Methods
Mushroom Sampling, DNA Isolation and Gene Sequencing
In this study, we obtained 219 fruiting bodies with macro-morphological characteristics similar to C. cibarius and related species from 24 counties in Yunnan province. The sampled areas in Yunnan spanned about 480 km from east to west and about 550 km from north to south. In addition, we obtained 144 yellow chanterelle samples from local markets in five countries (Finland, France, Hungary, Portugal, and Russia) in Europe. These 363 samples were all included in the study. The geographic coordinates and the sample size from each site are presented in Table 1.
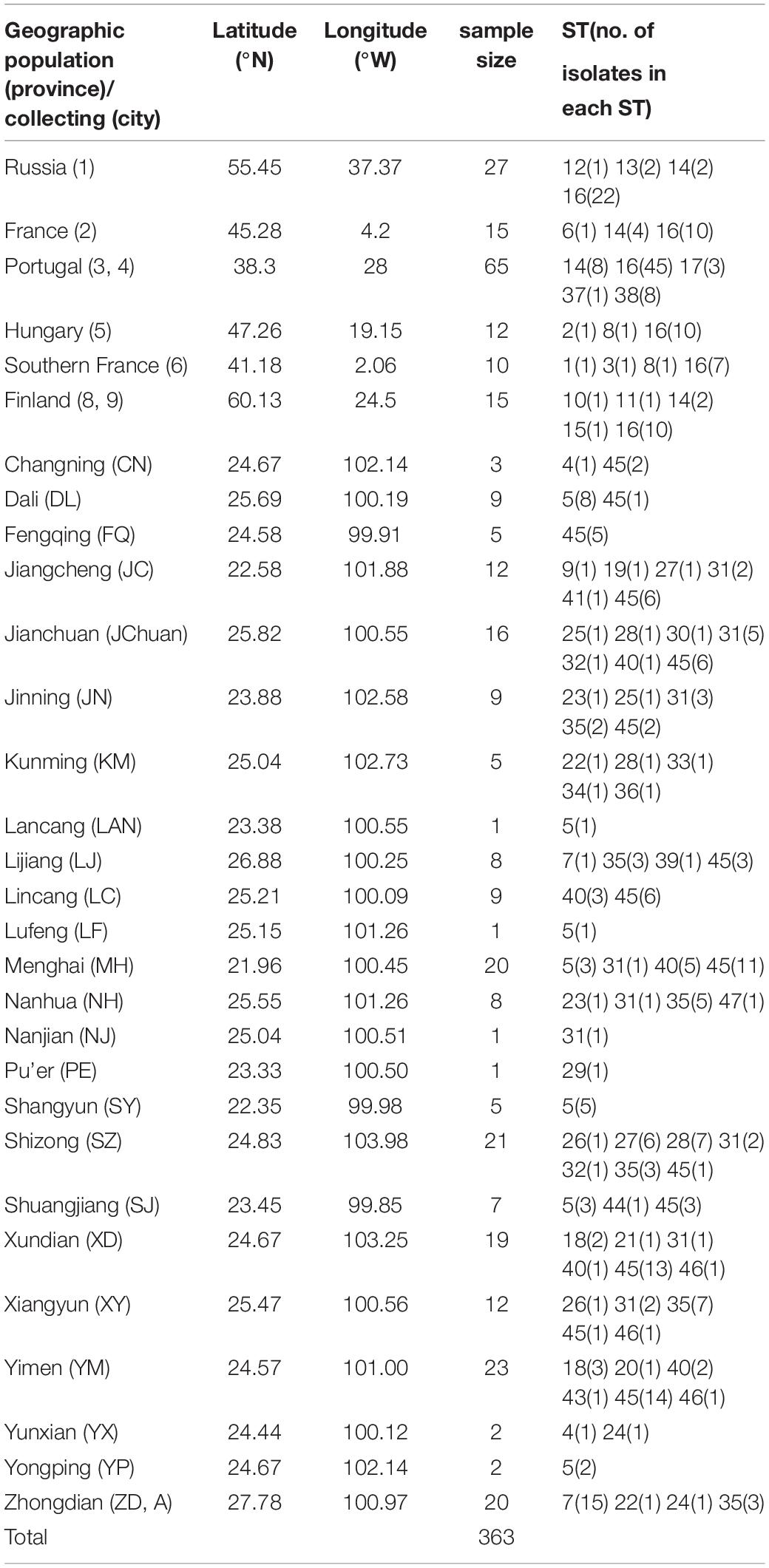
Table 1. Distribution and diversity of tef-1 sequence types for yellow chanterelles across southwestern China and Europe.
DNA extraction, PCR, sequencing, and sequence alignment all followed those of Wang et al. (2017). These samples were first analyzed based on their sequences at the tef-1 gene, the preferred DNA barcode for Cantharellus (Buyck and Hofstetter, 2011; Antonin et al., 2017; Olariaga et al., 2017). In our study, DNA sequences at the nucleartef-1gene were used to confirm phylogenetic affiliations of all our specimens (see below). In addition, the mitochondrial atp6 gene sequences were obtained for 327 specimens. The primer pairs, tef-1-S (5′ ACCAGAACGACGCCGCTCAA 3′) and tef-1-A (5′ TTGTCGCCGTGCCAACCAG 3′), ATP6-S (5′ AACACCGGGTACATTTCT 3′) and ATP6-A (5′ TACTTACGGCGATTTCTA 3′) were used to amplify and sequences the tef-1 and atp6 gene fragments, respectively.
mtDNA Heterozygosity
We observed mtDNA heterozygosity, shown as double peaks at specific nucleotide sites in the chromatograms of the atp6gene, in several specimens. We investigated whether the observed mtDNA heterozygosity was due to nuclear mt paralogs (numts) or the presence of more than one allele in the mitogenome within each of these specimens. To distinguish these two possibilities, we used two approaches. In the first, we confirmed the nucleotide sequence similarity between our sequences and that of the sequenced genome of C. cibarius/sample MG75 (GenBank number: QOWL00000000.1) through Basic Local Alignment Search Tool (BLAST). [Note: Based on its tef-1 sequence, sample MG75 likely belongs to C. tuberculosporus.] The second approach was through copy number assessment using a technique called absolute quantification PCR (AQ-PCR) (Leong et al., 2007).
In the second approach, for samples with evidence of heterogeneity at the atp6 gene, we determined their relative abundances in the fruiting bodies between the two combinations, one combination with peaks (TT) and the second combination with peaks (CA) respectively (Figure 1A) using AQ-PCR. Their abundances were compared with that of a nuclear gene, β-tubulin (tub). Since chanterelle mushrooms are diploids, each cell contains two haploid nuclei and multiple mitochondrial genomes. Thus, there would be two copies of each nuclear gene such as tub within each cell while multiple copies of mitochondrial genes. In this case, the relative copy numbers of the two atp6 alleles can be compared with the copy number of tub to determine within each sample whether the atp6 alleles are located in the nuclear genome or the mitochondrial genome. Our specific protocols are described as follows.
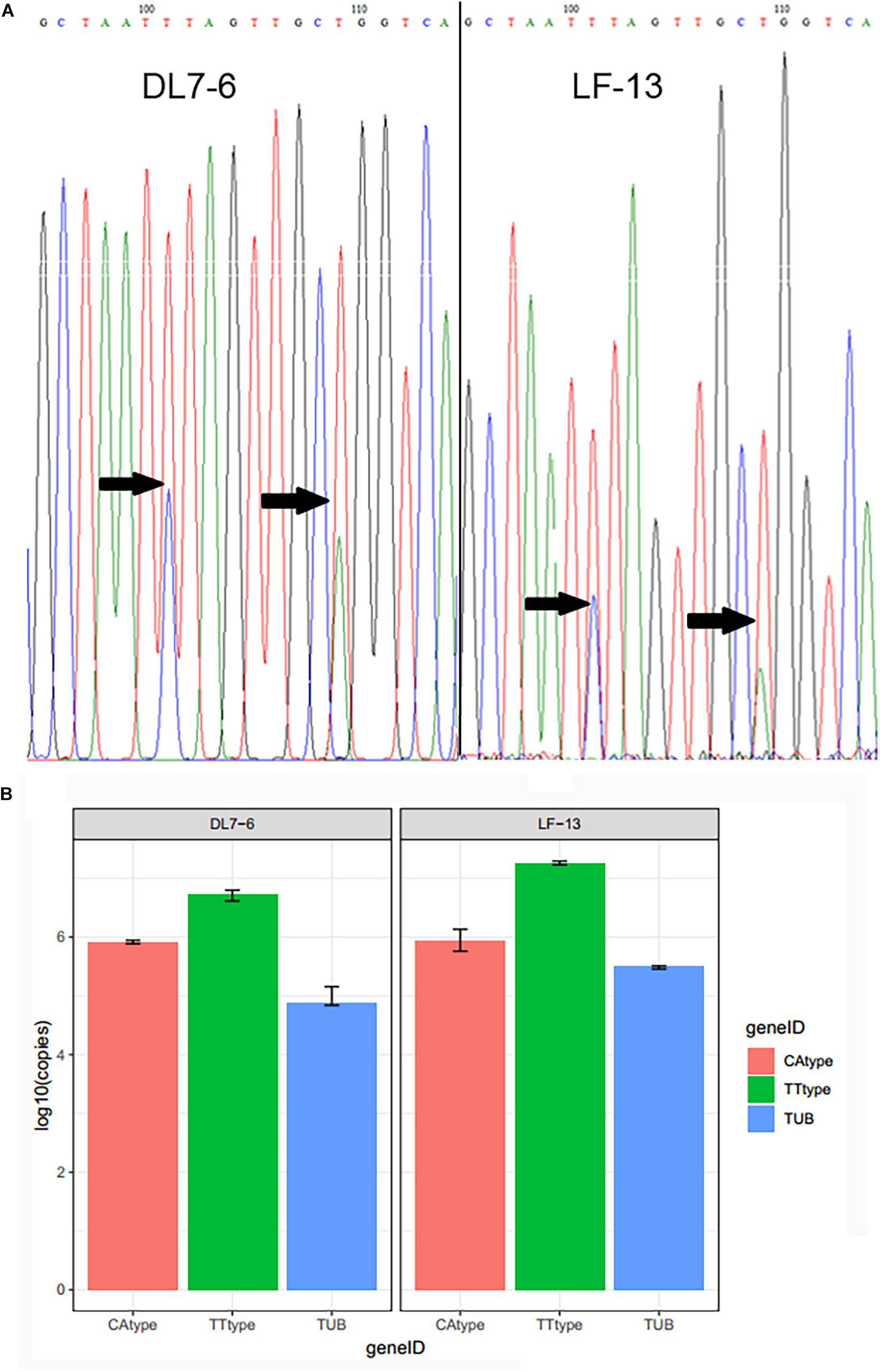
Figure 1. (A) Heterozygous sites of mitochondrial gene atp6 and (B) copy numbers of higher peak and lower peak sequences relative to the reference nuclear tub gene in samples DL7-6 and LF-13 from Yunnan.
To detect the relative copy numbers of the two atp6 alleles, we first amplified the atp6 gene fragment using primers ATP6H1 (5′ TGTAGCTAGAGCTTTCTCTTTAGGAGT 3′) and ATP6H2 (5′CCACTTGTAAATAATTTAGTTAAAAGACC3′). Similarly, the nuclear reference gene β-tubulin was amplified using primers TUB1 (5′CAGGAGGGTATGGATGAG3′) and TUB2 (5′TAACTGGAGGGGAGAATG3′). The amplified products containing the DNA targets were excised from 1% agarose gel under UV illumination and purified, then confirmed by sequencing to ensure the unique polymorphism is included in each atp6 products. Furthermore, the concentration of the amplified products was measured with a spectrophotometer, using the average molecular weight of the product and Avogadro’s constant. The copy number per unit volume was then calculated by comparing to a standard curve.
To establish the standard curve for gene copy number in a sample, both the tub and atp6 genes were first cloned separately using the PMD18-T cloning vector kit (CAT. 6011, TaKaRa, JAPAN) according to the manufacturer’s instructions. The recombinant vector was transformed into competent Escherichia coli cells and 25 μL of transformed culture was spread onto LB plates containing ampicillin (75 μg/ml) and X-gal/IPTG (CAT. R1171, ThermoFisher, United States). Transformed (white) colonies were picked and processed for plasmid isolation. Plasmid purification was done using a Plasmid Mini kit (CAT. TP01100, GENERAL BIOSYSTEMS, China). The presence of the insert in the recombinant clones was confirmed by restriction digestion. The cloned circular plasmid was quantified using a spectrophotometer and linearized with restriction enzyme Hind III (CAT.ER0501, ThermoFisher, United States). The stock solutions of linearized plasmid DNAs were serially diluted to obtain a standard series from 109 to 10 copies per μL with each step differing by 10 folds. Then, standard curves were built using 6 serial dilutions of each of the three plasmid DNA containing three different DNA fragments, atp6-TT, atp6-CA and tub. For AQ-PCR, the corresponding standards series was run under the same conditions and the copy numbers of each gene in each specimen was calculated using the following formula (Godornes et al., 2007):
6.022 × 1023 (molecules/mole) Avogadro’s number
660 Da: the average weight of a single base pair.
After the standard curves are established, we used quantitative-PCR (qPCR) to determines the actual copy numbers of target genes by relating the Ct values of each gene in each specimen to the standard curves (Xue et al., 2014). Each qPCR reaction was performed three times using the CFX96® system (Bio-Rad, United States) that contained the AceQ qPCR TaqMan Master Mix (without ROX) (CAT.Q112, Vazyme, China), 10 uM of dual labeled hydrolysis probe (Probe-TT: FAM-TTTAGTTGCTGGTCACACATTACT-MGB for the TT combination at nucleotide sites 101 and 109 of atp6, probe-CA: FAM-TCTAGTTGCAGGTCATACATTATT-MGB for the CA combination at nucleotide sites 101 and 109 of atp6, and probe-TUB:FAM-AGCACATACACGGCATCCTGG-BHQ1 at tub locus), and 10 uM each of forward and reverse primers. During each round of PCR, the target and reference sequences were simultaneously amplified by AmpliTaq® Gold DNA Polymerase. This enzyme has a 5′ nuclease activity that cleaves probes that are hybridized to each amplicon sequence. When an oligonucleotide probe is cleaved by the AmpliTaq Gold DNA Polymerase 5′ nuclease activity, the quencher is separated from the reporter dye, and thus increasing the fluorescence of the reporter dye. Accumulation of PCR products can be detected in real time by monitoring the increase in fluorescence of each reporter dye at each PCR cycle. Ct, slope, PCR efficiency, correlation coefficient (R2) and percentage of variance in copy numbers were calculated by using the default settings of Bio-Rad CFX Manager Version 3.1 (Bio-Rad, United States).
Data Analysis
Species Identification
Because of the relatively high heterogeneity of the primary fungal DNA barcode ITS within individual samples of C. cibarius and related species, high quality ITS sequences are often not available for species identification within this species complex (Buyck et al., 2020; Zhang et al., 2021). Instead, as in previous studies (Buyck et al., 2011; Buyck and Hofstetter, 2011), the tef-1 gene was used as the DNA barcode to determine the taxonomic placements of our Cantharellus specimens. However, at present, a tef-1 sequence-based new species identification system is not yet available. Because the systematics and taxonomy of Asian Cantharellus remains largely unresolved, for this study, we chose tef-1 sequence variations among samples within each of several closely related known species to establish a cutoff value for defining putative phylogenetic species limits.
To establish the cutoff value, we first retrieved all known tef-1 sequences of C. cibarius and related species from GenBank. The following 13 known species within C. cibarius and related species were found to contain tef-1 sequences from three or more specimens each: C. anzutake, C. tabernensis, C. appalachiensis, C. tenuithrix, C. ferruginascens, C. cinnabarinus, C. cibarius s.s., C. tuberculosporus, C.texensis, C.decolorans, C. amethysteus, C. lateritius, and C. lewisii. The distributions of these eight species have been well-documented and their morphological features and phylogenetic placements are known (Buyck et al., 2014). These sequences were used to identify the range of intraspecific tef-1 sequence variation and establish a conservative sequence-based cutoff value for putative phylogenetic species identification within the yellow chanterelles. Here, the highest intraspecific variation within these eight species was chosen as our cutoff value to define species limits. Subsequently, tef-1 sequence variations between our samples and those published previously for the known species of the yellow chanterelles were compared to the cutoff value. Specifically, we consider any specimen with a greater divergence than the cutoff from all known species and from each other in our sample as a potential new phylogenetic species. In contrast, specimens with tef-1 sequence divergence lower than the cutoff value were considered as belonging to the same species.
For each of our specimens, DNA sequences obtained from both forward and reverse primers of the tef-1 gene were assembled using the SeqMan sequence analysis software (DNASTAR, Inc.). All tef-1 sequences obtained for our specimens and those from GenBank representing the diversity of species within the yellow chanterelles were aligned by using MAFFT 6.0 (Misawa et al., 2002) and checked manually by BioEdit 7.0.9 (Hall, 1999). Ambiguous positions at the two ends of each gene fragment were excluded from the analysis. For the tef-1 dataset, maximum likelihood (ML) and Bayesian inference (BI) analyses were performed using RAxML 7.2.6 (Stamatakis, 2006) and MrBAYES3.1.2 (Huelsenbeck and Ronquist, 2001). The best partition schemes and evolutionary models were selected using MrModeltest v2.3. ML analyses were run with all parameters set to the default settings. GTR+G was used as the most appropriate model and the bootstrap analysis run with 1,000 replicates. BI analysis consisted of four simultaneous Markov chain Monte Carlo (MCMC) chains was run over 5 × 106 generations with trees sampled every 100 generations when the average standard deviation of split frequencies was lower than 0.01. By omitting the first 25% of trees as burn-ins using the “sump” and “sumt” commands, a majority rule consensus tree was generated. The percentage of sequence divergence for each specimen from all other specimens and known species were calculated and used to define the taxonomic placements of each of our specimens.
Haplotype Inference of tef-1 and atp6 Genotype Distribution
In this study, like most other mushrooms, we consider each individual mushroom as derived from a mating event between two genetically different homokaryons. Thus, each mushroom is a heterokaryon, with each cell having two different haploid nuclei and each locus having two alleles. In the case of single nucleotide polymorphisms (SNPs), each nucleotide site in each individual specimen may be homozygous for a specific nucleotide or heterozygous, containing two different nucleotides. In the sequenced tef-1 fragment, there are multiple SNP sites within many specimens. To better infer the relationships among specimens, we also inferred the tef-1 haplotypes for each specimen using the Bayesian method implemented in the program PHASE 2.1 (Stephens and Donnelly, 2003). The inferred haplotype sequences were then imported into MrBAYES 3.2 to analyze the relationships among alleles from within the same and different mushroom fruiting bodies, theatp6 sequence of each sample was assigned to one of the five atp6 genotypes at the two polymorphic nucleotide sites (positions 101 and 109): heterozygous genotype with both CA and TT or homozygous genotypes CA, TA, CT, or TT. For specimens containing haplotypes with ambiguous phylogenetic placements, we also cloned their tef-1 alleles and sequenced each cloned allele separately, following the protocol described for obtaining pure sequences of atp6 and tub. The generated phylogenetic relationships were analyzed together with their geographic origins to identify geographic patterns of (putative) species distributions.
Results
In this study, we analyzed a total of 363 mushroom specimens of the yellow chanterelles from 30 geographic locations in six countries. 24 of the locations were in Yunnan province in China while the remaining six were in Europe. The six European geographic locations had at least 10 specimens in each, with a range of 10 to 65. Among the 24 geographic locations from Yunnan, the sample sizes ranged from 1 to 23; with four having only one specimen each, 12 having 2 to 9 specimens each, and eight having 10 or more specimens each (Table 1). Each of these specimens were analyzed for its nuclear tef-1 and mitochondrial atp6 sequences. Below we describe the patterns of sequence variation among these specimens, with an emphasis on mitochondrial DNA polymorphisms.
tef-1 Genotyping of the 363 Yellow Chanterelle Specimens
Our sequence analyses identified that out of the 303 aligned nucleotide sites among the 363 tef-1 sequences, 123 sites were polymorphic. These 123 SNPs resolved the 363 specimens into 47 tef-1 sequence types (STs) (Peakall and Smouse, 2012). Among the 47 STs, 24 were each shared by more than one specimen each while the remaining 23 were found only once each. The most frequent ST was sequence type16, shared by 104 specimens from seven geographic populations. The second most frequent ST was sequence type45, shared by 75 specimens from 15 geographic populations. Among the 30 geographic samples, 23 contained more than one ST each while the remaining seven had only one ST in each local population. However, four of the seven geographic samples with only one ST each had only one specimen each, with the remaining three geographic samples each having two to five specimens. Samples from two geographic locations from Jianchuan and Shizhong contained most tef-1 STs, both including seven STs each (Table 1).
Phylogenetic Reconstruction and Phylogenetic Species Recognition
For phylogenetic analyses, we retrieved the tef-1 sequences of C. cibarius and those closely related species as revealed based on previous studies (Buyck and Hofstetter, 2011; Foltz et al., 2013; Buyck et al., 2014). Specifically, except C. confluens which didn’t have any tef-1sequence deposited in GenBank, all known species of chanterelles were included in our comparisons. In addition, C. anzutake, C. tuberculosporus, and C. yunnanensis, which were recently described as very closely related species to C. cibarius s.s. and some of our Yunnan samples (Ogawa et al., 2018; Shao et al., 2021), was also included in the comparisons. In total,115 tef-1 sequences were included in our phylogenetic analyses, with 47 sequences representing the 47 unique STs found in our current samples while the remaining 68 sequences were retrieved from NCBI to represent the diverse species within or closely related to C. cibarius s.s.
The ML and BI analyses of tef-1 dataset yielded identical tree topologies for the 115 tef-1 sequences. The tree inferred from BI analysis is shown in Figure 2. As shown in Supplementary Table 1, among the 21 known reference species, C. anzutake showed the highest value (0.8%) of intra-specific variation of tef-1 sequences. Thus, 0.8% sequence variation at tef-1 locus was chosen as the cutoff value for our putative phylogenetic species identification using tef-1 sequences within this group of chanterelles. Taking this value as a threshold, the largest interspecific genetic distances between C. tenuithrix, C. phasmatis, and C. flavus (0.1%) were all lower than 0.8%. Furthermore, these three species formed a single clade in our tef-1 phylogeny. Two of these species, C.phasmatis and C. flavus, were shown to share a more recent common ancestor than with the recently described species from the southern United States C. tenuithrix (Foltz, 2011). Therefore, based on the 0.8% tef-1 sequence divergence criteria to define putative phylogenetic species, C. tenuithrix, C. phasmatis, and C. flavus would be combined as one species/species complex. Similarly, genetic distance between C. ferruginascens and C. lilacinopruinatus was 0.1%. Indeed, both C. ferruginaseens and C. lilacinopruinatus are characterized by having a white stipe with similar pileus margin and hymenophore (Olariaga and Salcedo, 2008). Thus, these two species could be combined as one species too. The same applies to C. roseocanus and C. cibarius, which were recently separated from each other as distinct species (Foltz, 2011; Thorn et al., 2017). Thus, our criterion of requiring greater than 0.8% sequence divergence at the tef-1 locus represents a conservative cutoff value that minimizes the number of potential new species in our collection. Based on this conservative criterion, there are likely nine putative species in our samples. Among these nine putative species, two (Cantharellus sp.1 and Cantharellus sp. 4) clustered with known species C. tuberculosporus and C. cibarius, respectively. Two others, Cantharellus sp. 6 and Cantharellus sp. 8, have <0.8% of genetic distance with the phylogenetically related species C. amethysteus and C. lewisii, respectively. The remaining five had genetic divergences from known species at a much higher than the 0.8% cutoff value, thus likely representing new putative phylogenetic species (Figure 2, Supplementary Tables 1, 2). Among the proposed new putative species, two (Cantharellus sp. 2 and 5) were only based on one sample each, while the other three were each based on more than one samples. Thus, to formally establish these putative species as new species, more samples need to be collected and analyzed, especially for sp. 2 and 5, in their natural habitats. Regardless of the final number of species, these results suggest a significant number of undescribed species and genotypic diversity in our analyzed yellow chanterelles.
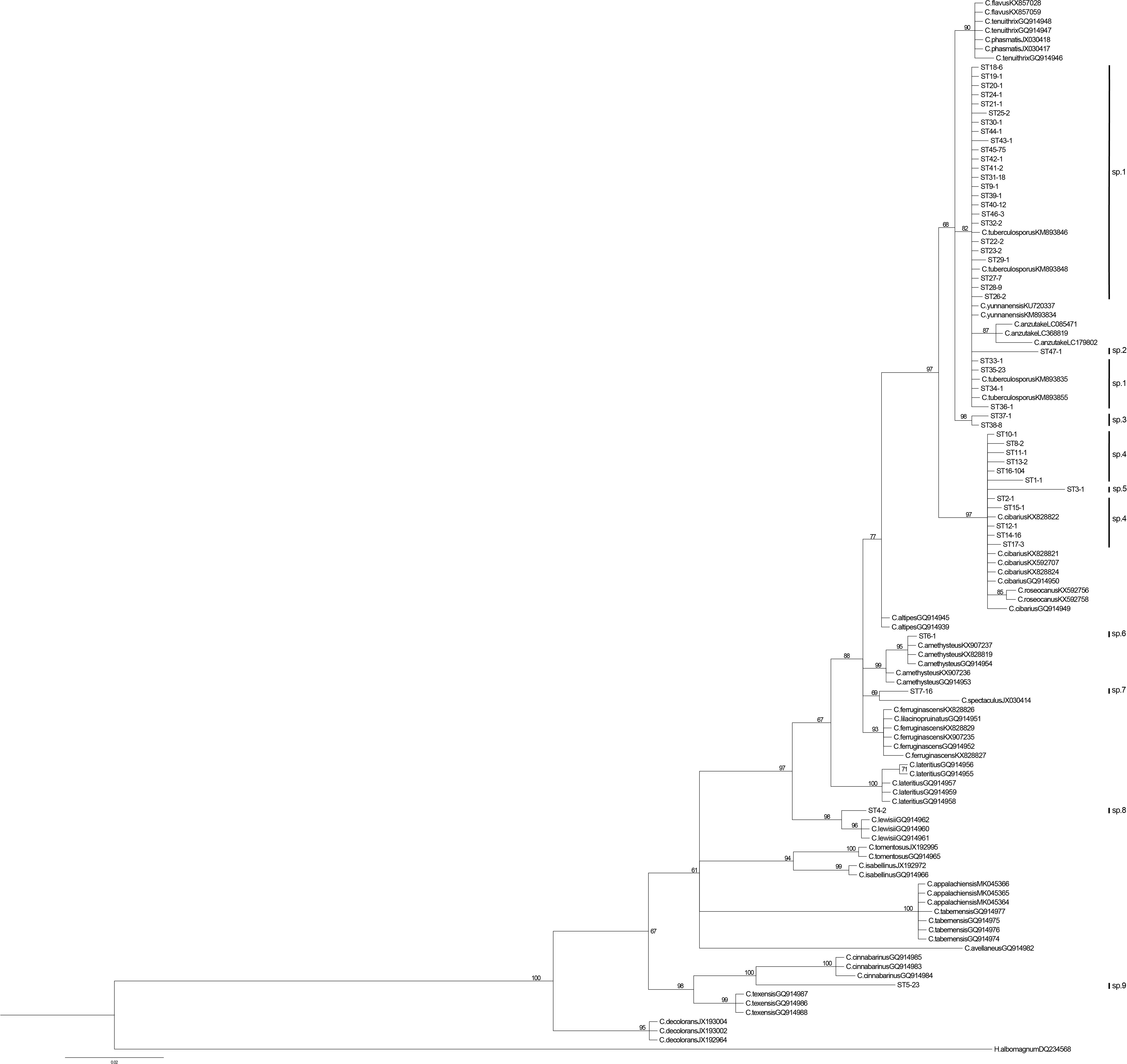
Figure 2. tef-1 phylogenetic tree of the C. cibarius species complex. For each tef-1 sequence type (ST) in our samples, the name or abbreviation (full name can be found in Supplementary Table 2) represents the county/community from where the sample was obtained, the number after represents the ST code assignment; the last number represents the total number of samples belonging to the specific ST.
Evidence for Heteroplasmy Within Mitochondrial atp6
We successfully obtained atp6 sequences from 327 of the 363 specimens. When we examined the atp6 sequence chromatographs, two specimens, DL7-6 and LF-13 (both belonging to Cantharellus sp. 9), were found to have double peaks at sites 101 and 109, while another specimen SY6-4 had double peaks at sites 62, 95, and 132, consistent with heterozygosity at these sites. At all sites, the lower peaks were significantly higher than the baseline noise levels at neighboring homozygous sites within the chromatograph (Figure 1A). To exclude the possibility of cross-contamination, we re-sampled DNA from additional tissues of these three specimens and amplified and sequenced the atp6 gene fragment again to confirm. For controls, genomic DNA from two other samples (ZD12-13 and YM3-30, both are in Cantharellus sp. 1) that initially did not show any double peaks in their atp6 chromatographs were also re-extracted and re-sequenced. Our sequence analyses confirmed the double peaks at the same nucleotide sites in the additional tissues of DL7-6, LF-13, and SY6-4, but no evidence of heterozygosity was found in specimens ZD12-13 and YM3-30. Our results excluded the possibility of sample contamination as a cause for the observed double peaks in specimens DL7-6, LF-13, and SY6-4. In both the original chromatographs and the new chromatographs, the remaining 301 nucleotides of the sequenced atp6 gene fragment each had only one nucleotide in specimens DL7-6, LF-13, and SY6-4, with no evidence of heterozygosity. Below, we systematically analyzed the distribution and variation patterns of heterozygosity at these sites (101 and 109). The three heterozygous sites found in specimen SY6-4 were separated from sites 101 and 109 in the following phylogenetic analyses of atp6 gene sequences.
Comparisons using BLAST showed that both nucleotide sequences with the higher and lower peaks were most similar to the atp6 gene from Cantharellus cibarius sample MG75 (GenBank number: QOWL00000000.1). The sequence identity of the atp6 haplotype with the higher peaks to atp6 gene of sample MG75 was 94% (E-value is 5e-099), and that with the lower peaks was 95% (E-value is 1e-101). The results are consistent with both sequences (one has the combination of the high peaks TT and the other has the combination of low peaks CA) are homologous sequences from the mitochondrial genome.
Our copy number analyses confirmed that both the TT allele and the CA allele of the atp6 gene are more numerous than the nuclear tub gene in both specimens DL7-6 and LF-13. Figure 1B summarized the copy numbers of the CA and TT haplotypes and of the nuclear tub gene from specimens DL7-6 and LF-13. Specifically, in specimen DL7-6, the CA and TT allele copy numbers were 10.9- and 69.9-fold higher than tub. Similarly, in specimen LF-13, the CA and TT allele copy numbers were significantly higher than that of tub gene, at 2.7- and 57.1- folds of tub. The differential ratios of the two alleles of atp6 gene both within each specimen and between the two specimens are also consistent with their non-nuclear nature (Sandor et al., 2018).
Distribution of atp6 Genotypes Along the tef-1 Phylogeny
The phylogenetic distributions of atp6 haplotypes at the two nucleotide sites (sites 101 and 109) were mapped onto the Bayesian tree constructed based on the tef-1 dataset (Figure 3). The results showed that the four different haplotype combinations (CA, CT, TA, and TT) at these two sites were broadly distributed throughout the tef-1 sequence-based phylogenetic tree. For example, among the European samples of the yellow chanterelles, three haplotypes CA, CT, and TT were found at these two nucleotide sites. Similarly, in multiple clades of specimens from Yunnan, three to four haplotypes out of all four possible haplotypes were found at these two atp6 nucleotide sites. Specifically, except for two clades Cantharellus sp. 2 and Cantharellus sp. 5 (both with just one sample each, Figure 2), other seven putative phylogenetic species recognized by tef-1 sequences have two or more different atp6 haplotype combinations. Within Cantharellus sp. 1 and Cantharellus sp. 9, all four haplotypes at the two sites were found, consistent with recombination within this mitochondrial gene fragment in the population. However, if true, during subsequent evolution and diversification, multiple parallel mutations and/or reversions at the two sites must have occurred to account for the observed patterns of distribution. However, given the low sequence diversity among the samples at atp6 gene fragment, we believe that the chance of parallel mutation or reversal at exactly these two sites in multiple clades is extremely small. The second possibility is that mitochondrial recombination is frequent within and among contemporary populations of the yellow chanterelles throughout their distribution range, a single recombination event from the heteroplasmic parent followed by segregation could have generated all four possible genotype combinations at these two sites. However, this second possibility requires most of the proposed species to hybridize with each other to generate recombinant mitochondrial genotypes. The third possibility is that the ancestor of the yellow chanterelles was heteroplasmic and recombined to produce four haplotypes between these two polymorphic nucleotide sites. These recombinants and heteroplasmic individuals then diversified among descendent lineages but with incomplete lineage sorting, resulting in some lineages maintaining the heteroplasmic state while others containing one to four of the four possible haplotypes.
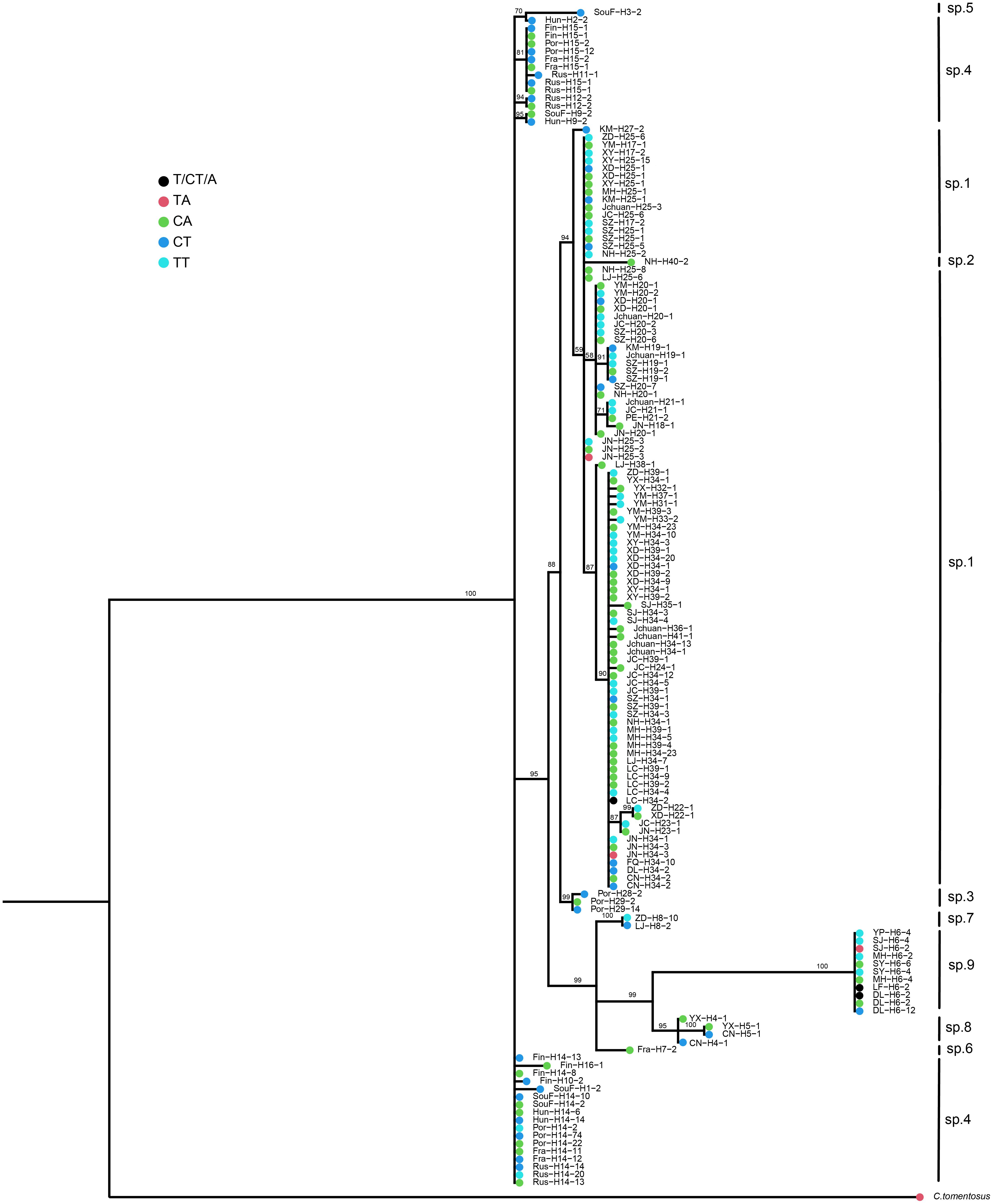
Figure 3. Bayesian tef-1 haplotype cladogram showing the broad distributions ofatp6 genotypes at two polymorphic nucleotide sites. Termina branch colors represent the different atp6genotypes at the two polymorphic sites; For each tef-1 haplotype in our samples, the name or abbreviation (full name can be found in Supplementary Table 2) represents the county/community from where the sample was obtained, the number after “H” represent the haplotype assignment; the last number represents the total number of samples belonging to the specific haplotype from each geographic location and for each unique atp6 genotype. Only representative sequences of unique haplotypes from each geographic location and unique atp6 genotypes are shown.
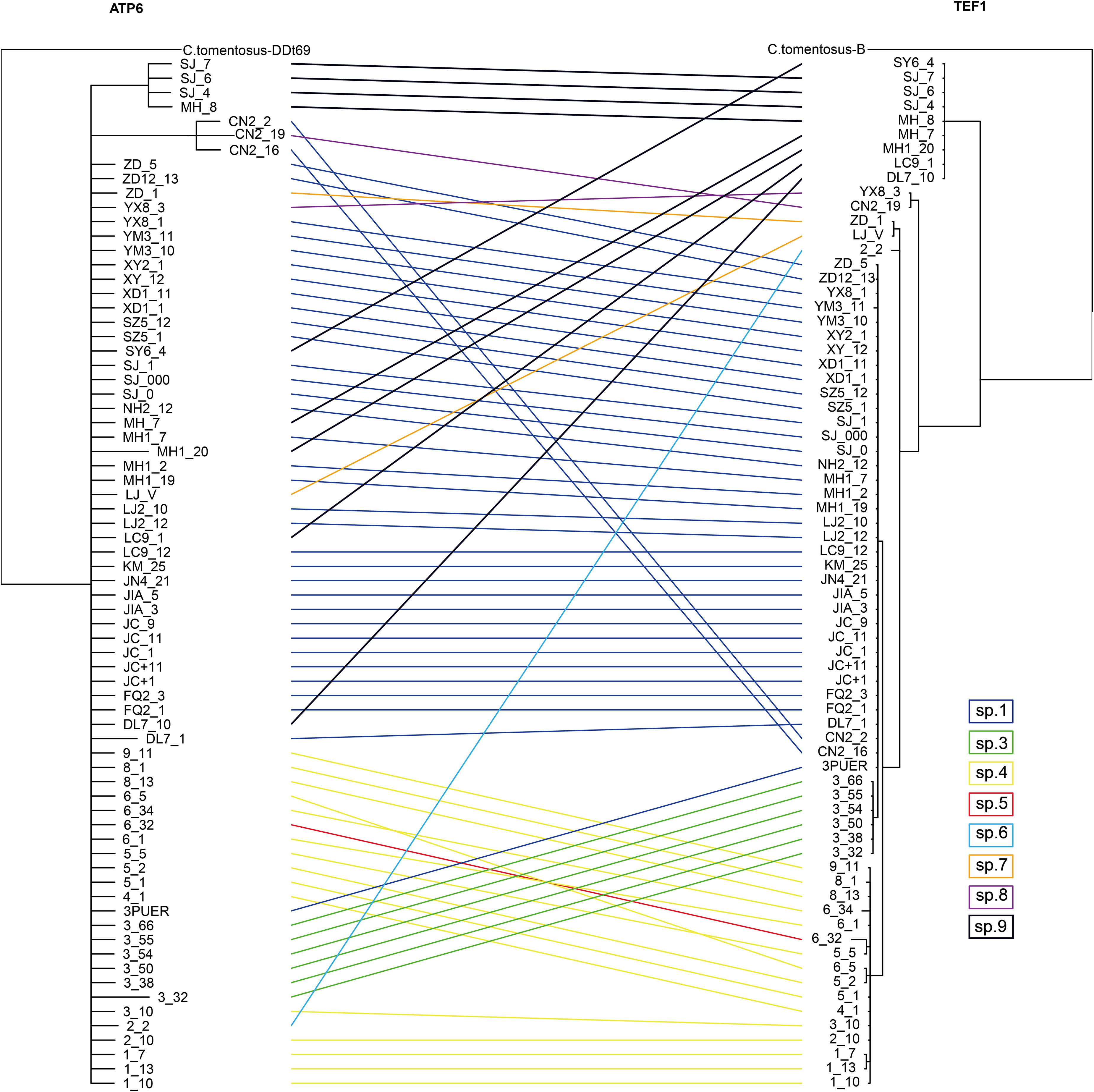
Figure 4. The tanglegram between tef-1 and atp6 phylogenies. The full name can be found in Supplementary Table 2.
To further investigate evidence of recombination among polymorphic nucleotide sites within the mitochondrial atp6 gene, we measured the degree of association between alleles at seven polymorphic nucleotide sites within this DNA fragment using the program Multilocus 1.3 (Agapow and Burt, 2001). The analyses were conducted for both the total samples and samples from individual phylogenetically distinct clusters as revealed by the tef-1 phylogeny. Only clades with >5 samples were analyzed. Because IA can be influenced by the number of polymorphic loci and samples, we standardized the IA value by the rBarD value for comparisons among populations. The null hypothesis for IA is that there is random association (recombination) among alleles at different loci, a p value of <0.05 would indicate that the null hypothesis should be rejected. Phylogenetic incompatibility (PrC) is another indicator of recombination at the population level, the lack of phylogenetic incompatibility implies no recombination (Agapow and Burt, 2001). Results of IA and PrC tests showed that while random recombination was rejected in all samples, evidence for recombination was found in both the total sample as well as in Cantharellus sp. 9 and C. tuberculosporus (Table 2). Specifically, they showed clear evidence of phylogenetic incompatibility (Table 2). Overall, our results are consistent with non-random recombination in the mitochondrial genome of several distinct clades within the yellow chanterelles.
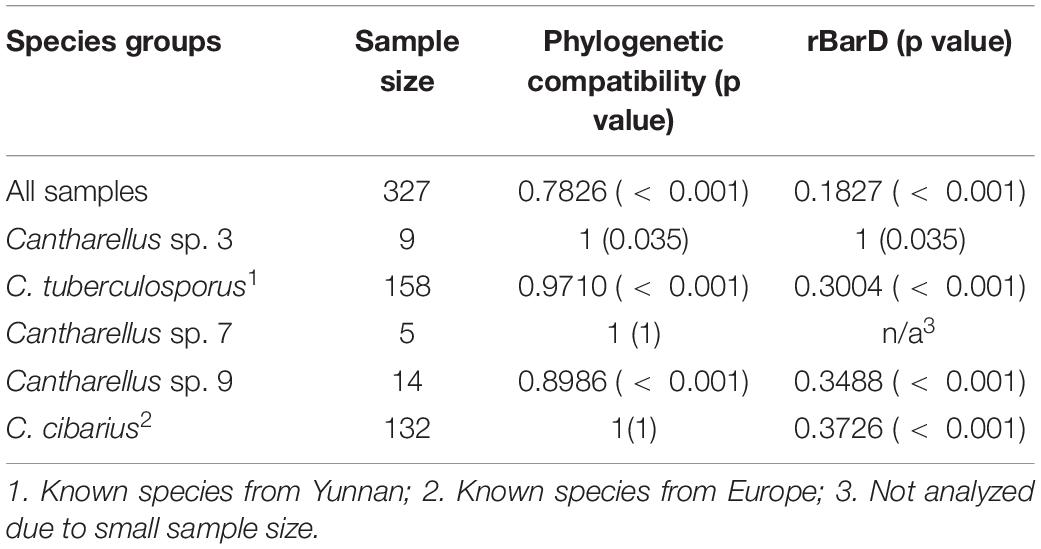
Table 2. Association among SNPs at the atp6 gene for C. cibarius and related species across southwestern China and Europe.
Using the same seven non-heteroplasmic sites within the atp6 gene fragment, we constructed the phylogenetic relationships of all clone-corrected samples and compared it with that constructed using tef-1 SNPs. The phylogenetic comparisons revealed evidence for phylogenetic in congruences between the two gene trees among most phylogenetic species. An example is strain SY6-4 that showed distinctly incongruent phylogenetic placements between the tef-1 and atp6 phylogenies. The results further support recombination and hybridization within and between several clades within the yellow chanterelles.
Discussion
Species and Genetic Diversity
In this study, 363 fruiting bodies of the edible yellow chanterelles were obtained from Yunnan, Southwestern China and five countries in Europe. Phylogenetic analyses of our tef-1 sequence types and closely related sequences from GenBank identified that while many of our samples belonged to two known species C. cibarius (most of our European samples) and C. tuberculosporus (many of our Yunnan samples), our specimens belonged to seven additional putative phylogenetic species.
Previous studies have shown that the broad application of the name C. cibarius to chanterelles found in North America and other areas outside of Europe were inaccurate. As a result, several new species have been described (Buyck and Hofstetter, 2011). The phylogenetic analyses of our samples from Southwest China along with representative samples of C. cibarius and related species from other parts of world indicated that the Yunnan and European samples contained a total of nine putative phylogenetic species. However, due to the lack of ITS, tef-1, and other gene sequences in public databases for several known species in this group of mushrooms, we are unable to confidently finalize the phylogenetic species status of the four clades. Indeed, though ITS shows higher resolution for species identification after cloning step (Ogawa et al., 2018), the difficulties in the direct amplification and sequencing of this gene, as well as the shortage of ITS data in the public database due to its high frequency of heterogeneity in many Cantharellus samples, have made ITS not an ideal barcode locus for this group of fungi (Xu, 2016). Instead, tef-1 has been recognized as the DNA barcode for C. cibarius and related species (Olariaga et al., 2017).
By constructing the tef-1 phylogenetic tree with the reference sequences representing the few current known species within the edible yellow chanterelles, we made several novel observations. First, several known species distinguished based on morphological characters showed no or very limited difference between each other at the tef-1 gene fragment. Indeed, the lack of a clear barcode gap at the tef-1 gene among several groups of morphological species suggest that additional genes or whole-genome sequences should be used to confirm the genetic uniqueness of these species (Xu, 2020). Second, most of the samples from Yunnan had distinct tef-1 sequences from those from other parts of the world. For example, most of the samples from Yunnan belonged to one clade that clustered with C. anzutake, C. tuberculosporus, and C. yunnanensis (Ogawa et al., 2018; Shao et al., 2021; Zhang et al., 2021). However, until recently, the common chanterelles in Yunnan province were called C. cibarius, the species found only in Europe (Liu et al., 2009; Wang et al., 2009). The known species C. tuberculosporus and C. yunnanensis showed very low interspecies genetic distance (0.3%) from each other, and with only 0.8% between C. yunnanensis and C. anzutake. According to the cutoff value we established for species delimitation in this study, the three species could be combined into one monophyletic species. Thus, our analyses indicate that the name C. cibarius is a misnomer when applied based on morphological characteristics to chanterelles in Yunnan, a conclusion similar to what was found recently through morphological investigations (Shao et al., 2021). With such a stringent criterion, several specimens from Zhongdian (Cantharellus sp. 7) and Nanhua (Cantharellus sp. 2) in Yunnan most likely belonged to novel species. Further comparisons using morphological characteristics and additional DNA sequences are needed to confirm their genetic uniqueness and cryptic speciation.
Persistent Heteroplasmy and Ancient Mitochondrial Recombination
We found evidence for heteroplasmy and recombination within the mitochondrial gene atp6 in our samples of C. cibarius and related species. The identification of heterozygous nucleotide sites at the atp6 locus in samples DL7-6 and LF-13 is consistent with heteroplasmy. In laboratory crosses, heteroplasmy is a pre-requisite for mitochondrial recombination (Basse, 2010). Furthermore, according to the tef-1 phylogeny, although the two heteroplasmic specimens DL7-6 and LF-13 belonged to the same tef-1 clade, the four atp6 haplotypes as observed from the yellow chanterelles had wide geographic and phylogenetic distributions, indicating that recombination was likely ancient. For recombination to occur, heteroplasmy must have been present in the ancestor to drive the recombination event. However, during subsequent evolution and diversification, most lineages may have lost heteroplasmy and/or some of the recombinant atp6 genotypes. The exceptions are two specimens DL7-6 and LF-13 that maintained their heteroplasmy.
Advances in DNA sequencing and polymorphism detection technology have documented the presence of recombinant mtDNA for a wide range of eukaryotic taxa. In the Baker’s yeast Saccharomyces cerevisiae, there is a large body of data showing evidence of heteroplasmy and recombination in laboratory crosses. However, mitochondrial heterogeneity was always transient, with the offspring of the zygote becoming completely homozygous within 20 mitotic cell divisions (Hermann and Shaw, 1998). A few other studies have also provided evidence of mtDNA recombination in natural populations of certain fungal species, such as Armillaria gallica (Saville et al., 1998), Cryptococcus gattii (Xu et al., 2009), Agaricus bisporus (Xu et al., 2013) and recently, Thelephora ganbajun (Wang et al., 2017). However, there has been no report showing evidence of potential stable heteroplasmy and broad mitochondrial recombination over repeated speciation events in nature.
In the human pathogenic yeast C. neoformans, a progeny population with diverse mitochondrial genotypes created due to biparental mitochondrial inheritance was found to show greater phenotypic variation than that with a uniparental mitochondrial inheritance (Yan et al., 2007). Mitochondrial genomes are commonly inherited uniparentally in the majority of sexual eukaryotes. As a result, these genomes are considered effectively asexual that may be prone to Muller’s Rachet, an irreversible accumulation of deleterious mutations (Lynch, 1996; Xu, 2005). Interestingly, our analyses identified signatures of heteroplasmy and mtDNA recombination in the yellow chanterelles, suggesting these organisms may have an effective mechanism to prevent Muller’s Rachet from operating in their mitochondrial genomes (Matsumoto and Fukumasa-Nakai, 1996; Xu, 2007).
At present, the selective pressure responsible for the maintenance of heteroplasmy and mitochondrial recombination in the yellow chanterelles is not known. In the human pathogenic yeast C. neoformans, high temperatures and UV exposure have shown to be capable of changing mitochondrial inheritance from uniparental to biparental (Yan et al., 2007), which also included mitochondrial heteroplasmy and the generation of mitochondrial recombinants. The high altitudes in Yunnan where these mushroom samples are from may be exposed to significant UV irradiations or other stress factors that favor mitochondrial heteroplasmy and recombination as an adaptive response to reduce the rate of accumulation of deleterious mutations in the mitochondrial genomes in these species. Indeed, evidence for mitochondrial recombination have been found in several other mushroom species (e.g., T. ganbajun and a Russula virescens ally) in the same geographic regions (Cao et al., 2013; Wang et al., 2017). Further investigations of the relationships between environmental factors and mitochondrial genetic variations are needed to determine the extent of mitochondrial recombination and the potential adaptive significance of such recombination in these mushrooms.
Comparison Between Mitochondrial and Nuclear Genetic Polymorphisms
In our analyses, there were overall lower sequence divergences among clades at the atp6 locus than those at tef-1 locus. This result is consistent with what has been reported in many other fungi that showed lower rates of evolution in the mitochondrial genome than that in the nuclear genome (Sandor et al., 2018, Sandor et al., 2020). Indeed, distinct genotypes and lineages identified based on the nuclear tef-1 sequences were often found to share the same atp6 sequences. However, the lower sequence diversity for mitochondrial genes than nuclear genes in these mushrooms doesn’t mean that the mitochondrial genomes in these fungi are static. Contrary, the mitochondrial genome size variation among strains in the same or closely related species can be very large (up to ±25% of the mean genome size), usually much greater than the nuclear genome size variation within many fungal species (Sandor et al., 2018; Wang and Xu, 2020; Zhang et al., 2020). The mitochondrial genome size variations in fungi were mainly due to differences in the number and size of introns and mobile genetic elements (Sandor et al., 2018; Wang and Xu, 2020; Zhang et al., 2020). Indeed, in our initial screening for mitochondrial genetic markers for analyzing the yellow chanterelles, polymorphisms in intron distributions were found in several genes, including cox1, cox2, cox3, nad1, nad5, cob, and rnl (Supplementary Table 3 and unpublished data). Unfortunately, such intron distribution polymorphisms in these genes limited the success of our direct PCR and sequencing efforts for these specimens. Consequently, these gene fragments were not selected as markers to further investigate the mitochondrial genetic polymorphisms in our study.
We would like to mention that while the low rate of evolution at the mitochondrial atp6 gene may be responsible for some of the haplotype sharing, an alternative explanation is recent hybridization among some of the lineages. Indeed, our analyses revealed evidence of incongruence between the nuclear tef-1 phylogeny and the mitochondrial atp6 phylogeny, consistent with hybridization among lineages. Analyses of whole-genome sequences, including both the nuclear and mitochondrial genome sequences at the population level, is needed in order to distinguish these two possibilities.
Data Availability Statement
The data presented in the study are deposited in the GenBank database, accession number(s) can be found in Supplementary Table 2.
Author Contributions
YZ and JX: Conceptualization, validation, visualization, supervision, project administration, and funding acquisition. JX, SW, and MM: sample collection. SW, HL, CL, FM, RW, and MM: methodology. SW and HL: software and formal analysis. YZ, JX, and SW: investigation. YZ and SW: writing—original draft preparation. JX: writing—review and editing. All authors have read and agreed to the published version of the manuscript.
Funding
This research was jointly supported by the National Natural Science Foundation of China (31870009 and 31760010) to YZ, the “Double First Class” Research Project in Yunnan University (C176280107) to JX, and the Top Young Talents Program of the Ten Thousand Talents Plan in Yunnan Province to YZ.
Conflict of Interest
The authors declare that the research was conducted in the absence of any commercial or financial relationships that could be construed as a potential conflict of interest.
Acknowledgments
We thank Vassili N. Kouvelis and Georg Hausner for the invitation. We thank the following people for their help in sample collections: Xiaozhao Tang, Yang Cao, Yanchun Li, Gang Wu, Lei Zhang, Xin Zhang, Junfeng Liang, Zaochang Liu, Shanze Yoell, and Liam Yoell.
Supplementary Material
The Supplementary Material for this article can be found online at: https://www.frontiersin.org/articles/10.3389/fmicb.2021.699598/full#supplementary-material
Supplementary Table 1 | Average evolutionary divergence over tef-1 sequence pairs within and between groups (provincially adopted phylogenetic species) calculated by MEGA 6 using the K2P model.
Supplementary Table 2 | List of putative species, corresponding ST code and samples used in Figures 2, 4, tef-1 haplotype code, corresponding atp6 genotype and sample origins used in Figure 3.
Supplementary Table 3 | Intron numbers of mitochondrial genes in C. cibarius and related species.
References
Agapow, P. M., and Burt, A. (2001). Indices of multilocus linkage disequilibrium. Mol. Ecol. Resour. 1, 101–102. doi: 10.1046/j.1471-8278.2000.00014.x
An, D. Y., Liang, Z. Q., Jiang, S., Su, M. S., and Zeng, N. K. (2017). Cantharellus hainanensis, a new species with a smooth hymenophore from tropical China. Mycoscience 58, 438–444. doi: 10.1016/j.myc.2017.06.004
Antonin, V., Hofstetter, V., Ryoo, R., Ka, K. H., and Buyck, B. (2017). New Cantharellus species from the Republic of Korea. Mycol. Prog. 16, 753–759.
Barr, C. M., Neiman, M., and Taylor, D. R. (2005). Inheritance and recombination of mitochondrial genomes in plants, fungi and animals. New Phytol. 168, 39–50. doi: 10.1111/j.1469-8137.2005.01492.x
Basse, C. W. (2010). Mitochondrial inheritance in fungi. Curr. Opin. Microbiol. 13, 712–719. doi: 10.1016/j.mib.2010.09.003
Birky, C. W. Jr. (1995). Uniparental inheritance of mitochondrial and chloroplast genes: mechanisms and evolution. Proc. Natl. Acad. Sci. U.S.A. 92, 11331–11338. doi: 10.1073/pnas.92.25.11331
Birky, C. W. Jr. (2001). The inheritance of genes in mitochondria and chloroplasts: laws, mechanisms, and models. Annu. Rev. Genet. 35, 125–148. doi: 10.1146/annurev.genet.35.102401.090231
Brundrett, M. (2004). Diversity and classification of mycorrhizal associations. Biol. Rev. 79, 473–495. doi: 10.1017/S1464793103006316
Buyck, B., Antonín, V., Chakraborty, D., Baghela, A., Das, K., and Hofstetter, V. (2018). Cantharellus sect. Amethystini in Asia. Mycol. Prog. 17, 917–924. doi: 10.1007/s11557-018-1403-8
Buyck, B., Cruaud, C., Couloux, A., and Hofstetter, V. (2011). Cantharellus texensis sp. nov. from Texas, a southern lookalike of C. cinnabarinus revealed by tef-1 sequence data. Mycologia 103, 1037–1046. doi: 10.3852/10-261
Buyck, B., and Hofstetter, V. (2011). The contribution of tef-1 sequences to species delimitation in the Cantharellus cibarius complex in the southeastern USA. Fungal Divers. 49, 35–46. doi: 10.1007/s13225-011-0095-z
Buyck, B., Kauff, F., Eyssartier, G., Couloux, A., and Hofstetter, V. (2014). A multilocus phylogeny for worldwide Cantharellus (Cantharellales, Agaricomycetidae). Fungal Divers. 64, 101–121. doi: 10.1007/s13225-013-0272-3
Buyck, B., Valerie, H., Ryoo, R., Ka, K.-H., and Antonín, V. (2020). New Cantharellus species from South Korea. MycoKeys 76, 31–47. doi: 10.3897/mycokeys.76.58179
Cao, Y., Zhang, Y., Yu, Z., Mi, F., Liu, C., Tang, X., et al. (2013). Structure, gene flow, and recombination among geographic populations of a Russula virescens Ally from southwestern China. PLoS One 8:e73174. doi: 10.1371/journal.pone.0073174
Das, K., Hofstetter, V., Chakraborty, D., Baghela, A., Singh, S. K., and Buyck, B. (2015). Cantharellus sikkimensis sp nov. (Cantharellales, Agaricomycetes) from the Indian Himalayas. Phytotaxa 222, 267–275. doi: 10.11646/phytotaxa.222.4.4
de la Providencia, I. E., Nadimi, M., Beaudet, D., Morales, G. R., and Hijri, M. (2013). Detection of a transient mitochondrial DNA heteroplasmy in the progeny of crossed genetically divergent isolates of arbuscular mycorrhizal fungi. New Phytol. 200, 211–221. doi: 10.1111/nph.12372
Eyssartier, G., Stubbe, D., Walleyn, R., and Verbeken, A. (2009). New records of Cantharellus species (Basidiomycota, Cantharellaceae) from Malaysian dipterocarp rainforest. Fungal Divers. 36, 57–67. doi: 10.1002/yea.1667
Feng, B., and Yang, Z. L. (2018). Studies on diversity of higher fungi in Yunnan, southwestern China: a review. Plant Divers. 40, 165–171. doi: 10.1016/j.pld.2018.07.001
Foltz, M. J. (2011). Systematics and Molecular Phylogeny of Cantharellus spp. in Western Wisconsin. Ph. D. thesis. La Crosse, WI: University of Wisconsin-La Crosse.
Foltz, M. J., Perez, K. E., and Volk, T. J. (2013). Molecular phylogeny and morphology reveal three new species of Cantharellus within 20 m of one another in western Wisconsin, USA. Mycologia 105, 447–461. doi: 10.3852/12-181
Fourie, G., Van Der Merwe, N. A., Wingfield, B. D., Bogale, M., Wingfield, M. J., and Steenkamp, E. T. (2018). Mitochondrial introgression and interspecies recombination in the Fusarium fujikuroi species complex. IMA Fungus 9, 37–48. doi: 10.5598/imafungus.2018.09.01.04
Godornes, C., Leader, B., Molini, B., Centurion-Lara, A., and Lukehart, S. (2007). Quantitation of rabbit cytokine mRNA by real-time RT-PCR. Cytokine 38, 1–7. doi: 10.1016/j.cyto.2007.04.002
Hall, T. (1999). BioEdit: a user-friendly biological sequence alignment editor and analysis program for windows 95/98/NT. Nucleic Acids Symp. Ser. 41, 95–98. doi: 10.1021/bk-1999-0734.ch008
Hermann, G., and Shaw, J. (1998). Mitochondrial dynamics in Yeast. Annu. Rev. Cell Dev. Bio. 14, 265–303. doi: 10.1146/annurev.cellbio.14.1.265
Huelsenbeck, J. P., and Ronquist, F. (2001). MRBAYES: Bayesian inference of phylogenetic trees. Bioinformatics 17, 754–755. doi: 10.1093/bioinformatics/17.8.754
Jian, S., Dai, R., Gao, J., and Feng, B. (2020). Cantharellus albus, a striking new species from Southwest China. Phytotaxa 470, 133–144. doi: 10.11646/phytotaxa.470.2.2
Leong, D. T., Gupta, A., Bai, H. F., Wan, G., Yoong, L. F., Too, H. P., et al. (2007). Absolute quantification of gene expression in biomaterials research using real-time PCR. Biomaterials 28, 203–210. doi: 10.1016/j.biomaterials.2006.09.011
Li, Q., Liao, M., Yang, M., Xiong, C., Jin, X., Chen, Z., et al. (2018). Characterization of the mitochondrial genomes of three species in the ectomycorrhizal genus Cantharellus and phylogeny of Agaricomycetes. Int. J. Biol. Macromol. 118, 756–769. doi: 10.1016/j.ijbiomac.2018.06.129
Ling, F., Mikawa, T., and Shibata, T. (2011). Enlightenment of yeast mitochondrial homoplasmy: diversified roles of gene conversion. Genes 2, 169–190. doi: 10.3390/genes2010169
Liu, P., Wang, X., Yu, F., Chen, J., Tian, X., Deng, X., et al. (2009). Fungous kingdom: Yunnan of China and her Ectomycorrhizal macrofungal species diversity. Acta Botanica Yunnanica Suppl 31, 15–20.
Lynch, M. (1996). Mutation accumulation in transfer RNAs: molecular evidence for Muller’s ratchet in mitochondrial genomes. Mol. Biol. Evol. 13, 209–220. doi: 10.1093/oxfordjournals.molbev.a025557
Matsumoto, T., and Fukumasa-Nakai, Y. (1996). Mitochondrial DNA inheritance in sexual crosses of Pleurotus ostreatus. Curr. Genet. 30, 549–552. doi: 10.1007/s002940050168
Mendoza, H., Perlin, M., and Schirawski, J. (2020). Mitochondrial inheritance in phytopathogenic fungi-everything is known, or is it? Int. J. Mol. Sci. 21:3883. doi: 10.3390/ijms21113883
Misawa, K., Katoh, K., Kuma, K., and Miyata, T. (2002). MAFFT: a novel method for rapid multiple sequence alignment based on fast Fourier transform. Nucl. Acid. Res. 30, 3059–3066. doi: 10.1093/nar/gkf436
Myers, N., Mittermeier, R. A., Mittermeier, C. G., Da Fonseca, G. A., and Kent, J. (2000). Biodiversity hotspots for conservation priorities. Nature 403, 853–858. doi: 10.1038/35002501
Ogawa, W., Endo, N., Fukuda, M., and Yamada, A. (2018). Phylogenetic analyses of Japanese golden chanterelles and a new species description, Cantharellus anzutake sp nov. Mycoscience 59, 153–165. doi: 10.1016/j.myc.2017.08.014
Olariaga, I., Moreno, G., Manjon, J. L., Salcedo, I., Hofstetter, V., Rodriguez, D., et al. (2017). Cantharellus (Cantharellales, Basidiomycota) revisited in Europe through a multigene phylogeny. Fungal Divers. 83, 263–292. doi: 10.1007/s13225-016-0376-7
Olariaga, I., and Salcedo, I. (2008). Cantharellus ilicis sp. nov., a new species from the Mediterranean Basin collected in evergreen Quercus forests. Rev. Catalana Micología 30, 107–116.
Parad, G. A., Ghobad-Nejhad, M., Tabari, M., Yousefzadeh, H., Esmaeilzadeh, O., Tedersoo, L., et al. (2018). Cantharellus alborufescens and C. ferruginascens (Cantharellaceae, Basidiomycota) new to Iran. Crypto. Mycol. 39, 299–310. doi: 10.7872/crym/v39.iss3.2018.299
Peakall, R., and Smouse, P. E. (2012). GenAlEx 6.5: genetic analysis in Excel. population genetic software for teaching and research–an update. Bioinformatics 28, 2537–2539. doi: 10.1093/bioinformatics/bts460
Redhead, S., Norvell, L. L., and Danell, E. (1998). Cantharellus formosus and the Pacific golden chanterelle harvest in western North America. Mycotaxon (USA) 65, 285–322. doi: 10.1017/S0953756297003997
Sandor, S., Zhang, Y.-J., and Xu, J. (2018). Fungal mitochondrial genomes and genetic polymorphisms. Appl. Microb. Biotech. 102, 1–16. doi: 10.1007/s00253-018-9350-5
Sandor, S. R., Wang, H. C., Vaario, L. M., Trudell, S. A., and Xu, J. (2020). Mitochondrial multilocus DNA sequence analyses reveal limited genetic variability within and consistent differences Between species within the global matsutake species complex. Acta Edulis. Fungi. 27, 1–19. doi: 10.16488/j.cnki.1005-9873.2020.01.001
Sato, M., and Sato, K. (2011). Degradation of paternal mitochondria by fertilization-triggered autophagy in C. elegans embryos. Science 334, 1141–1144. doi: 10.1126/science.1210333
Saville, B. J., Kohli, Y., and Anderson, J. B. (1998). mtDNA recombination in a natural population. Proc. Natl. Acad. Sci. U.S.A. 95, 1331–1335. doi: 10.1073/pnas.95.3.1331
Shao, S. C., Buyck, B., Hofstettere, V., Tian, X. F., Geng, Y. H., Yu, F. Q., et al. (2014). Cantharellus hygrophorus, a new species in subgenus Afrocantharellus from tropical southwestern China. Crypto. Mycol. 35, 283–291. doi: 10.7872/crym.v35.iss3.2014.283
Shao, S. C., Buyck, B., Tian, X. F., Liu, P. G., and Geng, Y. H. (2016a). Cantharellus phloginus, a new pink-colored species from southwestern China. Mycoscience 57, 144–149. doi: 10.1016/j.myc.2015.12.004
Shao, S.-C., Liu, P.-G., Tian, X., Buyck, B., and Geng, Y.-H. (2016b). A new species of Cantharellus (Cantharellales, Basidiomycota, Fungi) from subalpine forest in Yunnan, China. Phytotaxa 252:273. doi: 10.11646/phytotaxa.252.4.3
Shao, S.-C., Liu, P.-G., Wei, T.-Z., and Herrera, M. (2021). New insights into the taxonomy of the genus Cantharellus in China: epityfication of C. yunnanensis W.F. Chiu and the first record of C. cibarius Fr. Cryptogam. Mycol. 42, 25–37. doi: 10.5252/cryptogamie-mycologie2021v42a3
Shao, S. C., Tian, X. F., and Liu, P. G. (2011). Cantharellus in southwestern China: a new species and a new record. Mycotaxon 116, 437–446. doi: 10.5248/116.437
Shibata, T., and Ling, F. (2007). DNA recombination protein-dependent mechanism of homoplasmy and its proposed functions. Mitochondrion 7, 17–23. doi: 10.1016/j.mito.2006.11.024
Stamatakis, A. (2006). RAxML-VI-HPC: maximum likelihood-based phylogenetic analyses with thousands of taxa and mixed models. Bioinformatics 22, 2688–2690. doi: 10.1093/bioinformatics/btl446
Stephens, M., and Donnelly, P. (2003). A comparison of bayesian methods for haplotype reconstruction from population genotype data. Am. J. Hum. Genet. 73, 1162–1169. doi: 10.1086/379378
Stojkovic, B., Sayadi, A., Dordevic, M., Jovic, J., Savkovic, U., and Arnqvist, G. (2017). Divergent evolution of life span associated with mitochondrial DNA evolution. Evolution 71, 160–166. doi: 10.1111/evo.13102
Suhara, H., and Kurogi, S. (2015). Cantharellus cyphelloides (Cantharellales), a new and unusual species from a Japanese evergreen broad-leaved forest. Mycol. Prog. 14:55. doi: 10.1007/s11557-015-1079-2
Sun, S., Fu, C., Ianiri, G., and Heitman, J. (2020). The pheromone and pheromone receptor mating-type locus is involved in controlling uniparental mitochondrial inheritance in cryptococcus. Genetics 214, 703–717. doi: 10.1534/genetics.119.302824
Thorn, R. G., Kim, J. I., Lebeuf, R., and Voitk, A. (2017). Golden chanterelles of newfoundland and labrador: a new species, a new record for north america, and a lost species rediscovered. Botany 95, 547–560. doi: 10.1139/cjb-2016-0213
Tian, X. F., Buyck, B., Shao, S. C., Liu, P. G., and Fang, Y. (2012). Cantharellus zangii, a new subalpine basidiomycete from southwestern China. Mycotaxon 120, 99–103. doi: 10.5248/120.99
Verma, S., Shakya, V. P. S., and Idnurm, A. (2018). Exploring and exploiting the connection between mitochondria and the virulence of human pathogenic fungi. Virulence 9, 426–446. doi: 10.1080/21505594.2017.1414133
Vielba-Fernandez, A., Bellon-Gomez, D., Tores, J. A., De Vicente, A., Perez-Garcia, A., and Fernandez-Ortuno, D. (2018). Heteroplasmy for the cytochrome b gene in Podosphaera xanthii and its role in resistance to QoI fungicides in spain. Plant Dis. 102, 1599–1605. doi: 10.1094/PDIS-12-17-1987-RE
Wang, L., Zhao, X., Li, X., and Li, L. (2009). Research status of the Cantharellus Population. Edible Fungi of China 28, 6–8.
Wang, P. F., Sha, T., Zhang, Y. R., Cao, Y., Mi, F., Liu, C. L., et al. (2017). Frequent heteroplasmy and recombination in the mitochondrial genomes of the basidiomycete mushroom Thelephora ganbajun. Sci. Rep. 7:1626. doi: 10.1038/s41598-017-01823-z
Wang, Y., and Hall, I. R. (2004). Edible ectomycorrhizal mushrooms: challenges and achievements. Can. J. Bot. 82, 1063–1073. doi: 10.1139/B04-051
Wang, Y., and Xu, J. (2020). Mitochondrial genome polymorphisms in the human pathogenic fungus Cryptococcus neoformans. Front. Microbiol. 11:706. doi: 10.3389/fmicb.2020.00706
Watling, R. (1997). Mycology–the business of fructification. Nature 385, 299–300. doi: 10.1038/385299a0
Wilson, A. J., and Xu, J. (2012). Mitochondrial inheritance: diverse patterns and mechanisms with an emphasis on fungi. Mycology 3, 158–166. doi: 10.1080/21501203.2012.684361
Xu, J. (2005). The inheritance of organelle genes and genomes: patterns and mechanisms. Genome 48, 951–958. doi: 10.1139/g05-082
Xu, J. (2007). “Origin, evolution, and extinction of asexual fungi,” in Sex in fungi, eds J. Heitman, J. W. Kronstad, J. W. Taylor, and L. A. Casselton (Washington, DC: ASM Press), 461–475.
Xu, J. (2020). Fungal species concepts in the genomics era. Genome 63, 459–468. doi: 10.1139/gen-2020-0022
Xu, J., Horgen, P. A., and Anderson, J. B. (1996). Somatic recombination in the cultivated mushroom Agaricus bisporus. Mycol. Res. 100, 188–192. doi: 10.1016/S0953-7562(96)80119-5
Xu, J., and Li, H. (2015). Current perspectives on mitochondrial inheritance in fungi. Cell Heal. Cytos. 7, 143–154. doi: 10.2147/Chc.S59508
Xu, J., and Wang, P. F. (2015). Mitochondrial inheritance in basidiomycete fungi. Fung. Biol. Rev. 29, 209–219. doi: 10.1016/j.fbr.2015.02.001
Xu, J., Yan, Z., and Guo, H. (2009). Divergence, hybridization, and recombination in the mitochondrial genome of the human pathogenic yeast Cryptococcus gattii. Mol. Ecol. 18, 2628–2642. doi: 10.1111/j.1365-294X.2009.04227.x
Xu, J., Zhang, Y., and Pun, N. (2013). Mitochondrial recombination in natural populations of the button mushroom Agaricus bisporus. Fung. Genet. Biol. 55, 92–97. doi: 10.1016/j.fgb.2012.09.004
Xue, B. T., Guo, J. L., Que, Y. X., Fu, Z. W., Wu, L. G., and Xu, L. P. (2014). Selection of suitable endogenous reference genes for relative copy number detection in sugarcane. Int. J. Mol. Sci. 15, 8846–8862. doi: 10.3390/ijms15058846
Yan, Z., Sun, S., Shahid, M., and Xu, J. (2007). Environment factors can influence mitochondrial inheritance in the fungus Cryptococcus neoformans. Fung. Genet. Biol. 44, 315–322. doi: 10.1016/j.fgb.2006.10.002
Yan, Z., and Xu, J. (2003). Mitochondria are inherited from the MATa parent in crosses of the basidiomycete fungus Cryptococcus neoformans. Genetics 163, 1315–1325. doi: 10.1017/S0016672303006141
Yang, Z. (2002). On wild mushroom resources and their utilization in Yunnan Province, Southwest China. J. Nat. Resour. 4, 463–469. doi: 10.1088/1009-1963/11/5/313
Zhang, Y., Mo, M., Yang, L., Mi, F., Cao, Y., Liu, C., et al. (2021). Exploring the species diversity of edible mushrooms in Yunnan, Southwestern China, by DNA barcoding. J. Fungi 7:310. doi: 10.3390/jof7040310
Zhang, Y., Yang, G. Z., Fang, M. L., Deng, C., Zhang, K. Q., Yu, Z. F., et al. (2020). Comparative analyses of mitochondrial genomes provide evolutionary insights into nematode-trapping fungi. Front. Microbiol. 11:617. doi: 10.3389/fmicb.2020.00617
Keywords: DNA barcoding, speciation, biogeography, heteroplasmy, nuclear-mitochondrial incongruence, yellow chanterelles
Citation: Zhang Y, Wang S, Li H, Liu C, Mi F, Wang R, Mo M and Xu J (2021) Evidence for Persistent Heteroplasmy and Ancient Recombination in the Mitochondrial Genomes of the Edible Yellow Chanterelles From Southwestern China and Europe. Front. Microbiol. 12:699598. doi: 10.3389/fmicb.2021.699598
Received: 23 April 2021; Accepted: 23 June 2021;
Published: 14 July 2021.
Edited by:
Vassili N. Kouvelis, National and Kapodistrian University of Athens, GreeceCopyright © 2021 Zhang, Wang, Li, Liu, Mi, Wang, Mo and Xu. This is an open-access article distributed under the terms of the Creative Commons Attribution License (CC BY). The use, distribution or reproduction in other forums is permitted, provided the original author(s) and the copyright owner(s) are credited and that the original publication in this journal is cited, in accordance with accepted academic practice. No use, distribution or reproduction is permitted which does not comply with these terms.
*Correspondence: Jianping Xu, jpxu@mcmaster.ca; Ying Zhang, yzh_1210@hotmail.com