- 1Programa de Doctorado en Ciencias Silvoagropecuarias y Veterinarias, Campus Sur Universidad de Chile, Santiago, Chile
- 2Laboratorio de Sistemática y Evolución, Departamento de Silvicultura y Conservación de la Naturaleza, Universidad de Chile, Santiago, Chile
- 3UMR 7205, Muséum National d’Histoire Naturelle, Centre National de la Recherche Scientifique, Ecole Pratique de Hautes Etudes, Institut de Systématique, Évolution, Biodiversité, Sorbonne Université, Université des Antilles, Paris, France
Recent reports indicate that the health of our planet is getting worse and that genuine transformative changes are pressing. So far, efforts to ameliorate Earth’s ecosystem crises have been insufficient, as these often depart from current knowledge of the underlying ecological processes. Nowadays, biodiversity loss and the alterations in biogeochemical cycles are reaching thresholds that put the survival of our species at risk. Biological interactions are fundamental for achieving biological conservation and restoration of ecological processes, especially those that contribute to nutrient cycles. Microorganism are recognized as key players in ecological interactions and nutrient cycling, both free-living and in symbiotic associations with multicellular organisms. This latter assemblage work as a functional ecological unit called “holobiont.” Here, we review the emergent ecosystem properties derived from holobionts, with special emphasis on detritivorous terrestrial arthropods and their symbiotic microorganisms. We revisit their relevance in the cycling of recalcitrant organic compounds (e.g., lignin and cellulose). Finally, based on the interconnection between biodiversity and nutrient cycling, we propose that a multicellular organism and its associates constitute an Ecosystem Holobiont (EH). This EH is the functional unit characterized by carrying out key ecosystem processes. We emphasize that in order to meet the challenge to restore the health of our planet it is critical to reduce anthropic pressures that may threaten not only individual entities (known as “bionts”) but also the stability of the associations that give rise to EH and their ecological functions.
Introduction
Recent reports from the United Nations’ Intergovernmental Science-Policy Platform on Biodiversity and Ecosystem Services (IPBES), declared that the Earth’s ecosystem health has become progressively worse at an unprecedented accelerating pace [Intergovernmental Science-Policy Platform on Biodiversity and Ecosystem Services (IPBES) and United Nations, 2020]. Our species has put at risk much of Earth’s biodiversity and ecosystem processes (Brooks et al., 2002; Pievani, 2014; Lade et al., 2019). The loss of biodiversity and damage to ecosystems is of such a magnitude that it is being directly linked to current global crises (i.e., sanitary, developmental, economic, security, and social). The diverse initiatives and efforts to biodiversity decline, such as the “Sustainable Development Goals” (Glossary), have had no real impact on biodiversity conservation and even less on ecological process restoration (Zeng et al., 2020). It has been proposed that only true “transformative changes,” aimed not only at protecting, but also at restoring nature’s critical condition will change the current biodiversity and environmental health decline [Intergovernmental Science-Policy Platform on Biodiversity and Ecosystem Services (IPBES) and United Nations, 2020]. Coherently, the decline of “genetic biodiversity” (i.e., biodiversity loss; Hooper et al., 2012) and alterations of the biogeochemical cycles (Glossary; Sabater, 2008; Pinder et al., 2013; Nielsen and Ball, 2015) have been considered among the most severely compromised Planetary Boundaries (Glossary; Rockström et al., 2009; Steffen et al., 2015).
These two Planetary Boundaries, biodiversity and biogeochemical cycles are not independent. Ecosystem processes such as nutrient cycles are highly dependent on biological diversity (Lacroix and Abbadie, 1998; Falkowski et al., 2008; Gamfeldt et al., 2008; Naeem et al., 2012; Bishop et al., 2020). Even the faculty of ecosystems to sustain multiple and simultaneous functions and services (i.e., ecosystems’ multifunctionality properties, see Glossary) are only possible when a highly diverse community is considered (Hector and Bagchi, 2007; Maestre et al., 2012). This calls into question the idea of function redundancy with “expendable” elements in biodiversity (Hector and Bagchi, 2007; Allan et al., 2015) and challenges us to develop new perspectives to apply this knowledge to conservation and the restoration of the environment.
A canonical example of the importance of community diversity for maintaining ecosystem health is the role of its above-mentioned multifunctional properties in relation to planet Earth’s nutrient cycles (Glossary; Crawford, 2005). To understand this case, it is necessary to review the role of microorganism communities as the basic elements connecting ecosystem processes with biodiversity. The history of life on our planet and the constancy of its ecosystems have depended largely on microorganism community functional diversity, allowing the Earth to be habitable by other (uni- or multi-cellular) life forms (Margulis, 1993; Kasting and Siefert, 2002; Hohmann-Marriott and Blankenship, 2011). These biogeochemical processes are until today essential for the maintenance of life on earth (Madsen, 2011). Microbial metabolism and biogeochemical cycles developed together in such a way that they shaped our planet atmosphere (Falkowski et al., 2008; Fenchel et al., 2012). The first forms of microbial life, more than 3.7 million years ago, were exposed to an environment full of toxic gases such as dinitrogen (N2), carbon dioxide (CO2), methane (CH4), and ammonia (NH3), among others (Margulis, 1993; Falkowski et al., 2008; Barton and Northup, 2011; Dodd et al., 2017). Microorganism communities gradually adapted to these conditions, using these gases as energy sources (Margulis, 1993; Kasting and Siefert, 2002; Hohmann-Marriott and Blankenship, 2011). Microorganism biodiversity relevance as the foundation of ecosystem dynamics is apparent even today in the cycles of the six mayor elements; H, C, N, O, S, and P (Falkowski et al., 2008). Particularly soil nitrogen cycle depends on microorganism communities; bacteria, fungi, and archaea and their diversity have been found associated with N in all its phases; fixation, mineralization, nitrification and denitrification (Hayatsu et al., 2008). Nitrogen is key to life on our planet, since it constitutes a prime part of the molecules that make up living organisms such as DNA, RNA, amino acids and proteins. However, it is currently thought that anthropic effects such as intensive agriculture, livestock and indiscriminate use of fertilizers are causing deep alterations to this cycle (Guiry et al., 2018; Wang et al., 2018), which is one of the above-mentioned global planetary boundaries exceeded (Steffen et al., 2015). Considering the interconnectedness and relevance of biodiversity for the protection and restoration of ecosystem health, it is key to understand the anthropic effects on microbial communities (Falkowski et al., 2008; Gillings and Paulsen, 2014). Thanks to methodological and epistemological advances in the study of biological interactions, novel venues have opened allowing to ponder the importance of microorganism communities (Krumbein, 1996; Barton and Northup, 2011). Therefore, in this work we propose it is necessary to incorporate this body of knowledge to biodiversity conservation in order to a more effective contribution to the maintenance of ecological processes that allow the Earth ecosystems’ functioning.
Microorganisms’ lifestyles can be broadly classified as free-living or associated with a multicellular host (Barton and Northup, 2011). In this work we review the relevance of current knowledge regarding this second major group (i.e., associated with a multicellular host, in an exo or endosymbiotic association, see Glossary) for the preservation and restoration of threatened nature. Specifically, our objectives are: (1) To review the ecosystem importance of the symbiosis between microorganisms and terrestrial arthropods in key processes for the maintenance of ecosystems (i.e., biogeochemical cycles), (2) To discuss how anthropic pressures (pollution and loss of habitat) can alter this symbiosis, and (3) debate the possibilities and challenges of incorporating this conceptual framework into conservation sciences in the current global scenario.
Multicellular Associations: the Ecosystem Holobiont
All multicellular organisms, e.g., animals and plants, have symbiotic interactions with microorganisms (Zilber-Rosenberg and Rosenberg, 2008; Bordenstein and Theis, 2015; Carthey et al., 2019). Microbiota and their hosts (either plants or animals) are capable of performing different ecosystem functions in a way that could not take place in the absence of these interactions (Koide, 2000; Selosse et al., 2004; Peirano, 2007; Tripp et al., 2017). In an evolutionary context, these phenomena have also been described as hologenomic adaptations (see Glossary), where new adaptive traits emerge as a result of the symbiosis between microorganisms and their multicellular hosts (Finlay et al., 1997; Kitade, 2004; Korb, 2007; Six, 2013; Dietrich et al., 2014; Zepeda Mendoza et al., 2018; Bredon et al., 2020; Suárez, 2020; Suárez and Triviño, 2020). These emergent properties are often associated to fundamental ecological processes that contribute to the maintenance of ecosystems, such as matter and energy flows (Figures 1, 2 and Supplementary Table 1). Ecologically sound examples are the dependence of plants on microorganisms in the rhizosphere (Mendes et al., 2013), the obligatory intracellular symbionts of vertebrates and invertebrates (Sabree et al., 2009; Tokuda et al., 2013; Ju et al., 2020) as well as macroalgae and their associated microbiota. In all these examples these associations’ emergent properties fulfill key ecosystem functions (van der Loos et al., 2019). Unfortunately, all of these processes are being strongly affected by anthropic activities (Marzinelli et al., 2018). These delicate key processes have been evidenced extensively in plants, for instance in the participation of plant microbiota in the biogeochemical cycles of nitrogen and methane, thanks to the interaction with microorganisms present in the rhizosphere (Turner et al., 2013). In this process human-derived perturbations have been found to arrest the contribution of this association in nutrient cycling (Turner et al., 2013). Likewise, the carbon and phosphorus cycles, fundamental in the ability of forests to store carbon dioxide, are carried out by trees in association with Rhizobium spp. (Batterman et al., 2013) and Rhizobacteria spp. (Granada et al., 2018).
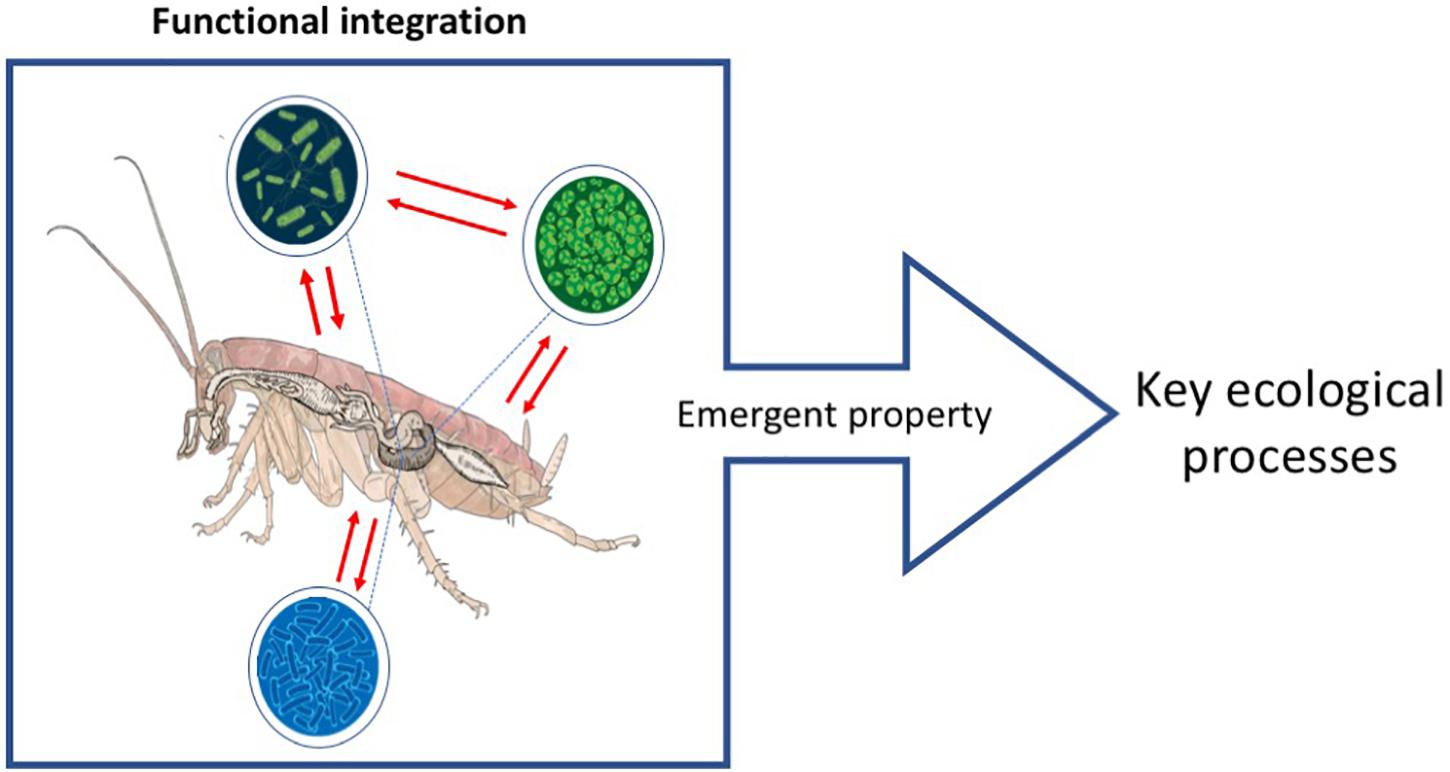
Figure 1. Ecosystem holobiont. The red lines show the functional integration between bionts resulting in the development of emergent properties with ecosystemic functions. For the case of the ecosystem holobiont, these properties are key ecosystem process such as the sustaining of nutrient cycling. Scheme based on the proposals of Catania et al. (2016) and Suárez (2020).
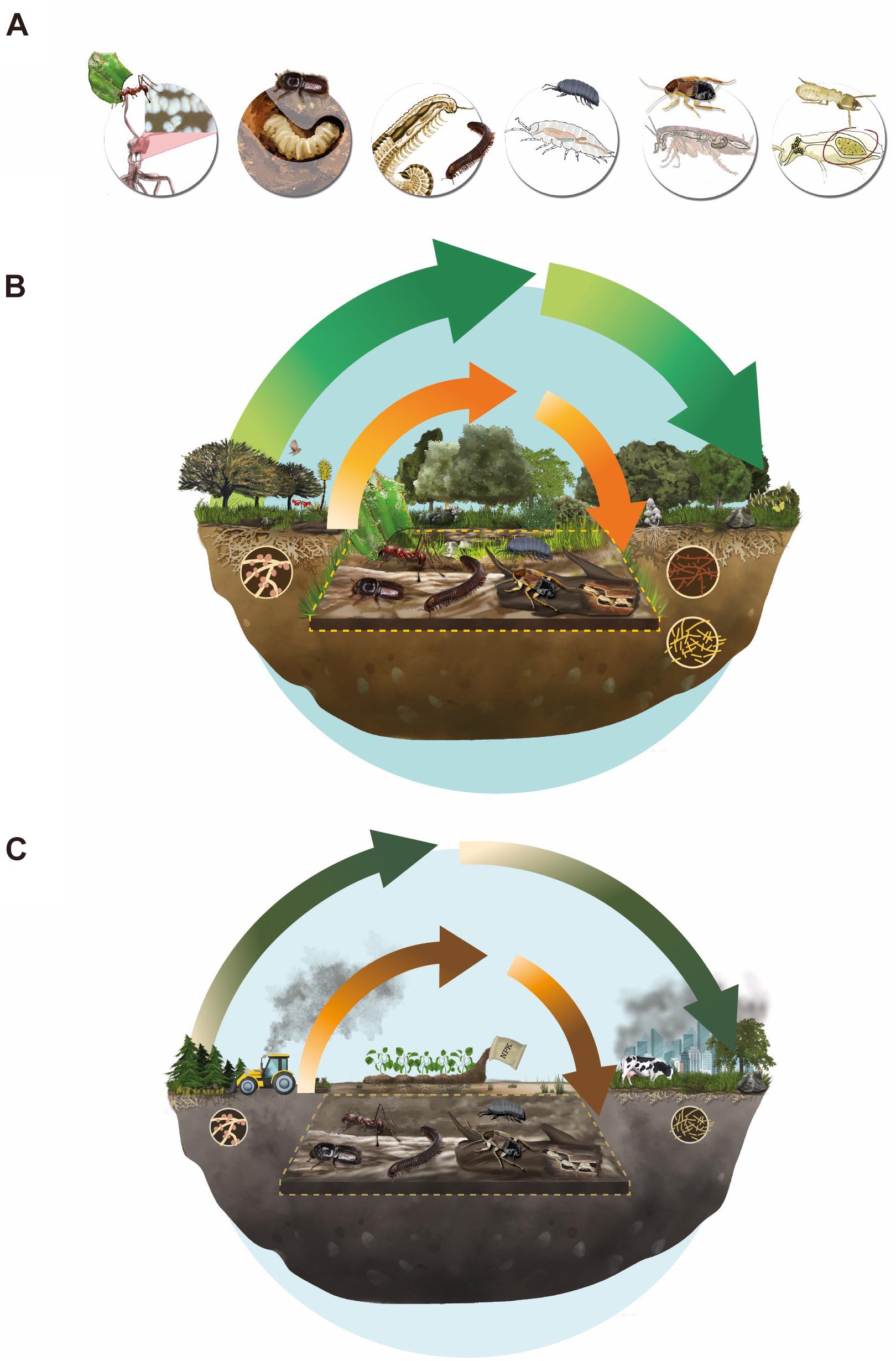
Figure 2. Emergent properties. (A) Examples of arthropods comprising Ecosystem Holobionts (EH) in conjunction with their associated microbiological bionts, from left to right: (i) ants, (ii) bark beetle, (iii) millipedes, (iv) woodlice, (v) cockroaches, and (vi) termites. These animal-microorganism associations are also represented in two contrasting scenarios: an undisturbed ecosystem (B) and a human-altered ecosystem (C). For (B,C) green arrows show nutrient cycles produced by the action of free-living microorganisms, and orange arrows the cycles in which EHs participate (within the dotted line). Arrows width illustrates the degree of anthropogenic disturbances impacting nutrient cycling processes, with thinner lines indicating greater degree of alteration.
Microbial associations have also been linked to the regulation of different ecological dynamics, for example the association between coral and dinoflagellate colonies, which form large reefs allowing the development of a miscellaneous community including fish and marine invertebrates, among others (Frade et al., 2008). There are also examples of the emergent benefits of microbial interactions with vertebrates, such as the case of carrion-feeding birds (Accipitriformes: Cathartidae). These have a strong adaptation to eat carrion thanks to the diversity of the microbial community of their skin. The processes of decomposition of organic matter of animal origin, and its further nutrient cycling, are accelerated through the action of these birds (Roggenbuck et al., 2014). Terrestrial invertebrates and their associated microorganisms have a fundamental role in the processes of plant-derived organic matter cycling and the subsequent nutrient flow (Hättenschwiler and Gasser, 2005; da Silva Correia et al., 2018). Such is the recurrence and value of these associations in nature that multicellular organisms, along with their community of microorganisms (microbiota), are currently being considered as an integrated and organized unit called “Holobiont” (see Glossary). This corresponds to a functional unit of life itself, capable of emergent properties that cannot be carried out by their parts or “bionts” (see Glossary) separately (Meyer-Abich, 1943; Margulis and Fester, 1991; Zilber-Rosenberg and Rosenberg, 2008; Guerrero et al., 2013; Six, 2013; Bordenstein and Theis, 2015; Theis et al., 2016; Carthey et al., 2019; Bredon et al., 2020). Although this definition has been criticized, mainly because it is still debated whether holobionts would constitute an evolutionary unit or unit of selection (Moran and Sloan, 2015; Douglas and Werren, 2016; Skillings, 2016; Stencel and Wloch-Salamon, 2018). Beyond that discussion, microbiota actively participating in biological processes such as reproduction and nutrition that have been described in all multicellular organisms (Moya and Ferrer, 2016; Gales et al., 2018; Ju et al., 2020; Supplementary Table 1). Alteration of the microorganism community leads to detrimental effects on their multicellular hosts in the vast majority of cases (Zhang et al., 2015; Warne et al., 2019).
However, beyond the evolutionary processes that underlie the existence of holobionts and further away, of understanding symbiosis as a mechanism of evolution (Klass et al., 2008; Zilber-Rosenberg and Rosenberg, 2008; Guerrero et al., 2013; Dietrich et al., 2014; Bordenstein and Theis, 2015; see symbiogenesis in Glossary), in this work we aim to incorporate and highlight the ecosystem aspects that make this integration a paramount phenomenon for the stability of key ecological processes. In addition of being relevant as selection units, holobionts are an ecosystemic unit with different elements integrated at a functional level (Catania et al., 2016; Doolittle and Booth, 2017; Lloyd and Wade, 2019; Suárez, 2020; Suárez and Stencel, 2020; Figure 1). This integrated unit is fundamental for ecosystems conservation as a whole. Thus, we propose the concept of “Ecosystem Holobiont” (hereafter “EH”) as a functional unit resulting from the integration of “bionts” composed by a multicellular organism together with its microorganism community, and the emergent properties that are generated as a result of the existence of this functional unit are indispensable for key ecological processes (Catania et al., 2016; Doolittle and Booth, 2017; Suárez, 2020; Suárez and Triviño, 2020; Glossary). EHs are fundamental for the adequate decomposition of recalcitrant organic compounds, e.g., lignin and cellulose, needed for nutrient cycling in soils (Guerrero et al., 2013; Six, 2013; Bredon et al., 2018, 2020; Supplementary Table 1).
In order to further illustrate the significance of the relation of EHs for biogeochemical cycles, we will review one milestone in Earth history that shows the relevance of microorganisms and multicellular integration in nutrient cycling. Between the Devonian and the Permian, 400 to 250 million years before present (MY), organic decomposition rates were increasing (Berner, 1990, 2001; Raymond et al., 2001). During the late Devonian, about 376–360 MY, the first trees appeared thanks to the development of evolutionary innovations such as lignin and cellulose (Algeo et al., 2001; Berner, 2001). These secondary plant compounds were difficult to digest for multicellular organisms that fed on plants living at that time (Martin, 1991; Calderón-Cortés et al., 2012). There is evidence of decomposition of wood material by lignolytic fungi from the late Carboniferous, 358.9–298.9 MY (Floudas et al., 2012). However, the activity of these fungi alone would not explain the increase in overall decomposition rates (Raymond et al., 2001). It has been then proposed that the radiation and evolution of detritivore invertebrates during the Carboniferous had a fundamental role in the decomposition of lignified plant litter (Nalepa et al., 2001; Kellogg and Taylor, 2004; Grimaldi and Engel, 2005). At first, detritivore invertebrates contributed by fractionating plant-derived materials mechanically, reducing them. At the same time, their foraging activity increased the contact surface with microorganism communities, facilitating decomposition (Shear and Kukalová-Peck, 1990; Crawford, 1992; Nalepa et al., 2001). This early exosymbiotic interaction (Glossary) between microorganisms and detritivores was only the beginning of an association that was slowly forged and cemented (Shear, 1991; Birkemoe et al., 2018). It has been suggested that this partnership was established through successive accidental intakes by invertebrates of microorganisms that were on the plant material (Ademolu et al., 2015). Then in the Mesozoic, 252 to 66 MY, different arthropod lineages, highly specialized in the decomposition of wood, developed complex interactions with a community of intestinal endosymbionts (see Glossary) and started to radiate (Grimaldi and Engel, 2005). There are fossil records of protists associated with cockroaches from the early Cretaceous (Poinar, 2009), as well as fossilized feces from these insects with leaves remnants, wood, cycad pollen and endosymbiotic protists (Hinkelman and Vršanská, 2020). The acquisition of flagellated symbionts during the late Jurassic period in lower termites allowed the hydrolysis of cellulose and fermentation products that were then absorbed by the host intestine (Bignell et al., 2010). Other forms of increasingly sophisticated symbiosis (Glossary) evolved by additional associations between arthropods and microorganisms, for example fungus-growing ants; where there is an intricate interaction between ants (Hymenoptera), fungi and actinomycete bacteria that probably originated during the Eocene (Schultz and Brady, 2008). Furthermore, today there are several cockroach lineages, such as Cryptocercidae, Blaberidae, Panesthinae and Zetoborinae (Blattodea), whose species show close intestinal endosymbionts interactions (Grandcolas and Deleporte, 1996; Guerrero et al., 2013) that allow this kind of EH to digest wood (Nalepa, 1984; Pellens et al., 2002; Brugerolle et al., 2003; Kitade, 2004; Korb, 2007; Bignell et al., 2010; Brune, 2014; Dietrich et al., 2014). Termites are also been considered “ecosystem engineers” (Glossary), since they play an irreplaceable role in nutrient cycling in arid and tropical ecosystems (Guerrero et al., 2013; Jouquet et al., 2016). For example, it has been found that termites contribute to soil physicochemical properties, soil turnover, water infiltration rate and soil microorganism diversity (Kaiser et al., 2017). However, this highly relevant function could not be carried out without the interaction between the multicellular (termite) organism and its complex intestinal endosymbiont assemblage (Kitade, 2004; Korb, 2007). The microorganism community associated with termites is composed of protists, bacteria and archaea (Brune, 2014). This EH organization is a necessary feature that confers the emergent properties that allow this entity to process lignified plant material, contributing to soil nutrient cycling as one of its emergent ecosystemic functions.
Considering these examples, it is also important to remind that the importance of the emergent properties resulting from the symbiosis between multicellular and microorganisms has been already emphasized by other authors (Catania et al., 2016; Suárez and Triviño, 2020). Therefore, there is both a corpus of evidences and rationale to contemplate the stability of the whole integration of bionts as the focus of conservation and restoration in order to preserve the associated processes they provide. A recent study developed in Burkina Faso illustrates the importance of EH; it showed that termite contribution to different aspects of ecosystem services depends on the species, and concomitantly on their obligatory association with intestinal endosymbionts (Kaiser et al., 2017). In the following section we develop further the relevance of terrestrial invertebrates along with their associated microorganisms and their ecosystem function.
Terrestrial Arthropods as a Case of Ecosystem Holobionts
Along with the battery of digestive enzymes that arthropods produce endogenously (Watanabe and Tokuda, 2010), multicellular biont’s association with microorganisms allows this association to exploit low nutrient food resources (Six, 2013; Brune, 2014). In many cases the partnership with symbiotic microorganisms is essential for terrestrial arthropods to complete their life cycle (Kukor and Martin, 1983; Klepzig et al., 2009). Here we provide some examples of arthropods and their microbial associates, that could be considered EH under our proposal scope, focusing on their role in nutrient cycling in terrestrial ecosystems (Figures 1, 2A and Supplementary Table 1).
Ants
These insects, belonging to the order Hymenoptera, family Formicidae (Ward, 2007), constitute the most abundant group of terrestrial arthropods, with an outstanding biomass of approximately 70 Mt of carbon (Tuma et al., 2020). To date, there are approximately 12,500 ant species described worldwide (Ward, 2010). Ants are widely recognized ecosystem engineers, mainly due to their influence on soil characteristics, nutrient cycling and resource availability (Jouquet et al., 2006; Tuma et al., 2020). For example, decomposition rates of cellulose-rich substrates have been shown to average 1.5 times higher and the net mineralization rates of N in Pogonomyrmex rugosus Emery, 1895 nests (Wagner and Jones, 2006). All these ecosystemic functions are carried out thanks to microbial partnerships. Ants have symbiotic relationships with multiple types of organisms, often involving more than two trophic levels (Ness et al., 2010), and are therefore considered multiparty symbiotic communities (Blatrix et al., 2009). Among the most remarkable and sophisticated examples are exosymbiotic relationships between leaf-cutter ants, fungi, yeasts and bacteria, where ants can set up farming systems thanks to these microorganisms (Schultz and Brady, 2008). These activities influence nutrient cycles by modifying primary productivity (Haines, 1975). It has been shown that N2 fixation exists in fungal gardens of at least eight species of leaf-cutter ants, as a result of nitrogen-fixing bacteria (Pinto-Tomás et al., 2009; Sapountzis et al., 2016). Two meta-analyses revealed that the refuse material from leaf-cutter ants nests show high levels of fertility, which constitute proper environments for plant growth (Farji-Brener and Werenkraut, 2015, 2017). Both the nests and the areas surrounding them, including refuse material areas, have a characteristic microbiota modulated by leaf-cutter ant activity; studies have suggested that these communities contribute to biodegradation and nutrient cycling processes (Lucas et al., 2017). Mound-building ants, from temperate and boreal forests, modify the properties of the soil where their mounds are located, generating soils with higher nitrogen and phosphorous content than the surrounding soil (Jurgensen et al., 2008). Studies have shown that the intestinal endosymbiont microbiota of leaf-cutter ants, which feed on fungal cultures, is composed of rhizobial N-fixing symbionts (Sapountzis et al., 2015). Ants have intestinal endosymbionts, for example intestinal bacterial communities have been found in Cephalotes spp. Latreille, 1802, that allow them to recycle nitrogenous residues from their diet (Hu et al., 2018). Diverse bacterial communities have been observed in the ileum of ant digestive system that may play a role in polysaccharide biodegradation (Bution and Caetano, 2008). This functional unit is strongly influencing nutrient cycling in terrestrial ecosystems; thus, it must be considered in the conservation actions that seek restoration. Once again while most efforts and resources in conservation policies are focused on charismatic vertebrates (Cardoso et al., 2011) relevant EH arthropods such as ants are being underestimated and unprotected. This must change in order to revert current global ecosystemic decline.
Bark Beetles
These coleopterans and their microorganism partners stand out as a case in which the resulting EH from this association is extremely important for the ecosystemic role of biodegradation, i.e., decomposition of recalcitrant plant material, such as cellulose and lignin. Therefore, this EH is very important for carbon cycling in its environment. Bark beetles belong to the Curculionidae family (subfamilies Scolytinae and Platypodinae). There are currently around 3,500 species that build and inhabit galleries under the bark of trees where they spend most of their life. It is also where they lay eggs; the larvae feed and develop in the same substrate (Kirkendall et al., 2015). These coleopteran bionts have symbiotic interaction with a variety of filamentous fungi and yeasts, mostly Ascomycetes of the genera; Ophthalmoma, Ceratocystiopsis, Grosmannia, and Ceratocystis that are capable of degrading the xylem, which is then eaten by beetles (Breznak, 1982; Spatafora, 2001; Six and Wingfield, 2011). It has been discovered that essential nutrients for the development of beetles are available thanks to the action of these fungi associates (Ayres et al., 2000). Most bark beetle species carry spores, either over the exoskeleton cuticle or in specialized structures called mycangia (Six and Paine, 1999). It is possible to suggest these bionts may also help catalyzing the action of their microorganism partners by increasing their vagility through the ecosystem. Thus, these evidences support that this association can be considered as an EH. The impact of bark beetles on biogeochemical cycles (i.e., Carbon and Nitrogen) has been demonstrated in pine forests. It has been observed that the C/N ratio varied between pine plots with and without beetles, and was lower in the patches where they are found (Morehouse et al., 2008). This is significant also because it favors other detritivores to thrive, for instance woodlice. It has been proven that soils with low C/N ratio are more palatable (Gerlach et al., 2014) and maintain stable woodlouse populations (Kautz et al., 2000). In spruce forests (Pinaceae) that had been wiped out by bark beetles it was observed an increase in soluble N (NH4-N, organic N) and in P in the upper soil horizons, in contrast with plots without them (Kaňa et al., 2013). So far, most studies about bark-eating insects and their associated microorganisms have focused on the harm that these EHs do to trees: “the main model postulates that fungal associates of tree-killing bark beetles are responsible for overwhelming tree defenses and incurring in host tree mortality” (Six and Wingfield, 2011). However, it is necessary to rethink the importance of bark beetles, shifting from the perspective of a pest to be able to visualize their contribution in the cycling of nutrients. In this way we will be able to manage and conserve the processes that underlie their life cycle.
Millipedes
Millipedes are arthropods of the Class Diplopoda. There are around 12,000 described species, but it is estimated that there could be around 80,000 and the vast majority are classified as detritivorous (Sierwald and Bond, 2007). They tend to be an important part of the litter arthropod biomass in different ecosystems (Crawford, 1976; Golovatch and Kime, 2009; Seeber et al., 2010). They are also essential in biodegradation of carbon and soil humification in terrestrial ecosystems (Alagesan, 2016; Pokhylenko et al., 2020; Supplementary Table 1). It has been found that the action of millipedes on leaf litter decreases the C/N ratio and improves some characteristics such as humidity and pH from acidic to neutral (Ashwini and Sridhar, 2006). There is also evidence that these invertebrates and their microbiota as a whole positively affect soil aggregation, levels of nitrogen and labile phosphorus (da Silva et al., 2017). The millipede gut is an ideal habitat for the development of symbiotic bacteria with enzymatic properties capable of cellulose and hemicellulose biodegradation (Taylor, 1982; Guzev and Byzov, 2006; Ramanathan and Alagesan, 2012). In the same fashion, yeast communities able to reuse uric acid have been found in millipedes’ hindgut, providing intestinal bacteria with compounds rich in nitrogen (Byzov et al., 1993). Millipedes can also influence the free microbial leaf litter community. It was observed that leaf litter fragmentation and the presence of the feces produced by this EH increased the CO2 release due to microbial metabolism, which has positive effects on decomposition rates (Suzuki et al., 2013). It has recently been observed that these arthropods are susceptible to urbanization, showing a reduction in species and functional richness in anthropized habitats (Tóth and Hornung, 2019).
Woodlice
Another astounding example are the terrestrial isopods (Oniscidea), also known as woodlice. These terrestrial crustaceans are among the indispensable and most abundant detritivore soil macrofauna for many temperate habitats, actively participating in nutrient cycling (Hedde et al., 2007; Vos et al., 2011; Sutton, 2013; Zuo et al., 2014; Chen and Shaner, 2018; Pokhylenko et al., 2020). Woodlice also constitute a fundamental fraction of the macroarthropod fauna in tropical forests and nearby fast-growing plantations (Pellens and Garay, 1999). As with the case of termites, the importance of terrestrial isopods for ecological restoration has been highlighted due to their role in litter and soil nutrient cycling processes, accelerating the biodegradation of plant material (Snyder and Hendrix, 2008). For example, the enzymatic battery of the Armadillidium vulgare (Latreille, 1804), that breaks down polysaccharides (e.g., lignocellulose), is produced endogenously by this arthropod in association with its microbiota (Zimmer and Topp, 1998; Dittmer et al., 2016; Bredon et al., 2018). These enzymes have recently been described in detail for at least four woodlouse species, suggesting that this trait was one of the keys in the success of the colonization of terrestrial environments by this group (Bredon et al., 2020). Experiments have shown the positive impact of these arthropods on microbial respiration and macronutrient availability in the soil (Teuben, 1991; Kautz and Topp, 1999). Considering these findings, woodlouse conservation should be a priority to protect and maintain biogeochemical cycles. The significance of these arthropods as bioindicators of ecosystems health status has been already emphasized (Paoletti and Hassall, 1999; Solomou et al., 2019), together with their ability to accumulate heavy metals in their hepatopancreas (midgut caeca) vesicles (Hopkin and Martin, 1982; Papp et al., 2019). However, there is no certainty on the impact that this could have on their performance as detritivores, since this tissue also harbors microorganisms related with cellulose and lignin biodegradation (Hobbelen et al., 2004; Dittmer et al., 2016; Supplementary Table 1).
Cockroaches
Cockroaches belong to the order Blattodea, and have more than 4,600 species described (Princis, 1962-1971; Beccaloni and Eggleton, 2013), which is likely to represent one fourth of their total diversity (Pellens and Grandcolas, 2007). Despite their bad reputation, only 20–30 (less than 1%) of these species are considered pests (Bell et al., 2007). The remaining cockroaches correspond to native species inhabiting diverse wild environments from deserts to tropical forests, where they are most diverse (e.g., 644 spp. in Brazil; 238 spp. in the Guiana Shield - Pellens and Grandcolas, 2007; Evangelista et al., 2017, respectively). Cockroaches can be found in a great diversity of microhabitats from the canopy to underground caves and tree holes, and often move across habitats in spite of habitat specialization (e.g., Grandcolas, 1993a; Weinstein, 1994; Pellens et al., 2007b, 2011). Some species are known to contribute to specific processes such as seed dispersal (Uehara and Sugiura, 2017), plant pollination (Nagamitsu and Inoue, 1997; Vlasáková, 2015; Suetsugu, 2019) and probably as scavengers in birds’ nests (Van Baaren et al., 2002). But their most generalized contribution is to the breakdown of organic debris (Nalepa et al., 2001; Sabo et al., 2005; Bell et al., 2007). One extreme specialization of cockroaches’ concerns wood-feeding, as it involves complex interactions with flagellates (Mastigophora) or ciliates (Ciliophora). This association conform an EH directly involved in the decomposition of cellulose and lignin (Grandcolas, 1995; Pellens et al., 2002; Brugerolle et al., 2003; Berlanga et al., 2016), and mechanisms that assure the transmission of endosymbionts among individuals and across generations. These interactions, related to life in a confined environment and to a nutrient-poor diet, have been hypothesized to be at the origin of sub-social behavior in Dictyoptera, which appeared independently in very different lineages (Pellens et al., 2007a; Klass et al., 2008; see also Grandcolas, 1993b and Murienne et al., 2008 for examples of xylophagy in other families). Although much remains to be studied about the role of cockroaches and their microorganism partners as EH involved in the decomposition of organic matter, available results suggest that their impact is not negligible. Thanks to the alliance between these insects their gut microbiota bionts, this EH is capable of digesting recalcitrant plant materials (Prins, 1991). When hosted in the cockroach gut, its symbiotic bacteria produce enzymes capable cellulose biodegradation, contributing with this emergent ecosystemic property (Cruden and Markovetz, 1987). Greater species richness was found in places in tropical forests with high phosphorus content, an interesting result since there may be a relationship between the action of cockroaches and the availability of this element (Tarli et al., 2014). Experimental studies have shown that the intestinal microbiota of different groups of cockroaches are usually quite stable, independent of their diet (Lampert et al., 2019). This presumably provides flexibility in the use of food resources, positioning these insects as an important element of soil trophic networks (Ardestani et al., 2020). Their ability to survive on diets poor in nitrogen thanks to the symbionts within their fat bodies (Blattabacterium spp.) allow them to develop in substrates with a high C/N ratio, which would put this cockroach- Blattabacterium interaction in the first line of recalcitrant organic compound biodegradation (Sabree et al., 2009; Tokuda et al., 2013). Although the ecosystem relevance of cockroaches and their associates have been recognized, the scientific literature regarding this group and its microbiota in wild environments is scarce, so the detritivore role of these EHs is still not fully understood. For example, cockroaches can harbor nitrogen-fixing bacteria in their gut microbiota (Cruden and Markovetz, 1987), therefore their participation in biogeochemical cycles is most likely being underestimated. Due to the fact that few research groups are currently studying this wild EH, i.e., most cockroach research is focused on pest species, their relevance has been largely underestimated. Although more research is needed to fully understand their contribution, it is desirable that these associations can be considered in further conservation plans involving the cycling of nutrients in diverse terrestrial ecosystems.
Termites
Termites (Blattodea: epifamily Termitoidea) are xylophagous organisms by excellence. Their global dry biomass is estimated to be around 50 Mt of carbon, which represents one fourth of total arthropod biomass (Tuma et al., 2020). They are capable of degrading cellulose and lignin thanks to the mutualistic interaction with their intestinal microbiota. This is composed mainly of prokaryotic organisms such as bacteria and archaea, and eukaryotes such as protists (Engel and Moran, 2013; Ni and Tokuda, 2013; Brune, 2014). Flagellates (Mastigophora) are also capable of breaking down cellulose, while prokaryotes contribute in the fermentation of soluble metabolites resulting from this breaking down (Brune and Stingl, 2005). It has been demonstrated that the association of termites and microorganisms is responsible for approximately 10% of the mineralization and biodegradation of carbon from soil litter in forests in Thailand (Yamada et al., 2005). In semi-arid ecosystems, such as the Australian Mulga (Acacia aneura F. Muell. ex Benth. – Fabaceae) forests, the partnership between termites and microorganisms’ contributes as the most relevant detritivorous function. Their gallery construction in the first 20 cm of the soil contributes to the degradation of organic matter, hence to plant and soil water retention capacity (Whitford et al., 1992). In tropical savannas, where termites are very abundant, it has been proposed that an important part of the CH4 balance is due to invertebrate-microorganism partnerships, since their activity contributes to the cycling of 21% of the methane produced by the soil (Jamali et al., 2011). Furthermore, in temperate ecosystems such as the forests of the southeastern United States, termites of the genus Reticulitermes Holmgren, 1913 (Rhinotermitidae) exert relevant influence on nutrient cycles, particularly increasing C and Ca in the soil (Myer and Forschler, 2019). In addition to intestinal endosymbionts, termites can have exosymbiotic (see Glossary) interactions with fungi, as is the case of Termitomyces spp. Heim, 1942 (Lyophyllaceae), which allows the degradation of recalcitrant materials from litter and wood before the ingestion by termites (Rouland-Lefèvre et al., 2006), this strategy reduces the C/N ratio of the substrate (Higashi et al., 1992). Nevertheless, despite their widely recognized importance in terrestrial ecosystems (Johnson and Whitford, 1975; Freymann et al., 2008; Jouquet et al., 2011; Harit et al., 2017), insufficient emphasis has been placed on studies of termite-microorganisms EH conservation worldwide, except for a few examples (see Davies et al., 2010, 2020; LeClare et al., 2020). Present-day evidences on their fundamental contribution to the stability of terrestrial ecosystems and thus the maintenance of Earth’s functioning should encourage their explicit inclusion in conservation and restoration programs and policy-making.
Threats to Ecological Processes Mediated by Ecosystem Holobionts
Several anthropic pressures are causing negative impacts on EHs and their associated ecological processes (Figures 2B,C). Here we will briefly mention those that are most relevant, according to the available scientific literature. These hazards can be broadly classified into two related categories: (i) Contamination and (ii) Land use change. Both alterations are considered direct drivers of biodiversity loss (IPBES, 2019). Below we review some of the most relevant factors reported in the literature.
Contamination
The presence of arthropods such as isopods and millipedes has long been considered a bioindicator of soil health status. However, the physiological state of these organisms is rarely considered, much less alterations in their microbiota and how this affects their performance. Evidence accumulated through decades points to the impact of chemical products, e.g., agrochemicals, pharmaceutical products and industrial and urban-derived contamination (e.g., sewage sludge production) on soil arthropod populations (Fox, 1964; Andrés et al., 2011; González-Alcaraz et al., 2020). There is a myriad of effects of chemical products on these organisms, such as weight loss, avoidance behavior and stress protein (hsp70) expression (but see van Gestel and Loureiro, 2018) for a study in which no negative consequences were reported). Nonetheless, there is a lack of studies evaluating the long-term and direct impacts of human-produced chemical products on terrestrial detritivore performance and subsequently on nutrient cycling. The closest is a recent study of gene expression against nickel exposure in Porcellionides pruinosus (Brandt, 1833) (Isopoda: Porcellionidae), showing a negative impact on oxidative stress, neurotoxicity and reproduction (Ferreira et al., 2019). Despite being a relevant contribution that explores the mechanisms behind the contamination effects on a soil arthropod, the microbiota dimension was not considered. Current evidence shows that antifungal and antibiotic chemical compounds released to the environment are affecting detritivorous invertebrates. For example, in livestock production, where antibiotic use is a common practice, there is evidence that it has negative consequences on dung beetle (Hammer et al., 2016) and springtail microbiota (Zhang et al., 2019), which can affect their performance and subsequently the nutrient cycles associated with their action. However, the mechanisms underlying this effect are still unclear (Lucas et al., 2019). Intensive agricultural management such as liming and fertilization can affect the intestinal microbiota of the edaphic fauna (Ding et al., 2019a,b). Recent work on soil collembolas showed that contamination with microplastics altered their gut microbiota and inhibited their growth (Zhu et al., 2018a). Other work showed that neonicotinoid and pyrethroid insecticides, widely used in agriculture, reduce the detritivore arthropods density (collembola, acari and diplopoda) as well as the rate of leaf litter decomposition (Pearsons and Tooker, 2021).
Land Use Change
Habitat loss and degradation due to land use change are some of the main threats to biodiversity (Caniani et al., 2016); their impact on biological communities is evident both compositionally and functionally (Allan et al., 2015). These ecosystem modifications often alter the interactions of the microbiota. There have been successful initiatives regarding agricultural management in relation to the activity of detritivorous arthropods such as “set-aside,” which consists of farmers leaving part of their land out of intensive production. Although this type of management has been efficient in allowing the establishment of millipedes and woodlice (Tóth et al., 2016), it must be accompanied by a production system change that tends toward agroecology in order to reduce exogenous input such as the use of nitrogenous fertilizers and pesticides that alter nutrient cycles (Vitousek et al., 1997; Galloway et al., 2008) and disrupt arthropod populations (Sánchez-Bayo, 2011; Sánchez-Bayo and Wyckhuys, 2019).
The presence of antibiotic resistance genes has been proven in the collembolan microbiome. This phenomenon is related to different uses of soil by this arthropod (arable and park) (Zhu et al., 2018b). Land use changes can influence the increase of EH populations such as forest plantations and bark beetles and their associated microbiome, which could also alter nutrient cycles (Kaňa et al., 2013).
Tolerance to human alterations has been reported for mound-building termites in savannah ecosystems, since these insect’s nests persist despite advance of human alterations. Yet, the same authors point out the need to understand how human activities impacts the processes carried out by this EH (Davies et al., 2020). In another savannah study, it is shown that at small spatial scales (1–2 km patch diameter) natural-agricultural landscape heterogeneity strongly promotes termite foraging activity. Nonetheless, the opposite effect is seen at a larger landscape scale (5 km), concluding that landscape heterogeneity can disrupt nutrient cycling functioning of termite-microorganism associations (LeClare et al., 2020). Therefore these dynamics are still in need of further research. For instance, it is also necessary to consider the type of perturbation and the specific attributes of this EH. A study comparing primary forest, grazing areas and agricultural fields found greater termite richness and abundance in primary forests and grazing areas than in agricultural fields, and the structure of termite feeding groups differed significantly among the three types of land use (Muvengwi et al., 2017).
Future Directions
Considering the conceptual framework proposed by IPBES for nature’s contribution to people (NCP) (see Glossary) the EH participates directly in at least two of them; NCP 1: habitat creation and maintenance; and NCP 8: formation, protection and decontamination of soils and sediments (Brauman et al., 2020) mainly through its participation in nutrient cycling and decomposition of recalcitrant materials (Supplementary Table 1). This means that this functional unit must be considered as an object of conservation.
We are facing difficult times when it comes to environmental conservation, but these are also times of great scientific discoveries; in recent decades we have seen a technological revolution that has allowed us a deeper understanding of the way in which organisms inhabit our planet (Madigan et al., 2015). Now we must take a further step toward understanding the biological interactions that make life on earth possible in order to restore and conserve them.
It is possible that the previous efforts to ameliorate Earth’s ecosystem crises have not been sufficient because canonically considered ecological included in these strategies, may not considered all key components underlying biodiversity’s ecosystem functions and their interrelationships. In order to develop strategies to cope with planetary boundaries such as biodiversity decline and biogeochemical cycles alterations cannot be regarded without considering EHs, because ecosystem processes such as nutrient cycles are highly dependent on biological diversity and its intricated interactions.
Conservation sciences must be nourished by updated methodological advances as well as epistemological discussions that seek to explain and understand the organization of life. In this way, the perspective of the EH’s functional integration provides us with a more complete view of nature as well as novel heuristic tools to face this challenge (Catania et al., 2016; Dupré, 2017; Suárez, 2020). Therefore, its incorporation into conservation policies and plans would provide concepts and methods to restore and preserve key processes (i.e., biogeochemical cycles) to guarantee ecosystems functioning in order to take actions that allow the restoration of key ecological processes for human survival.
Concluding Remarks
The scientific community has recently provided evidence indicating that arthropod populations are declining in many places in the world, and that the leading causes of this decline are of anthropic origin (Hallmann et al., 2017; Lister and Garcia, 2018; Sánchez-Bayo and Wyckhuys, 2019; Eggleton, 2020; Wagner, 2020). As shown in this essay, this biodiversity loss may most likely also involve the decline of symbiotic microorganism diversity associated to these arthropods, which often result of emergent hologenomic adaptations (see Glossary). This involves a myriad of mechanisms to transfer this association between individuals from one generation to another. It is becoming well established that the loss of all these partnership of organisms drives to the loss of the associated ecosystem functions, leading to further alterations in biogeochemical cycles (Eggleton, 2020). At present day, the nitrogen and phosphorus cycles are already altered beyond acceptable limits to preserve human well-being and ecosystem resilience (Steffen et al., 2015). We propose that the loss of EHs (Figure 1 and Supplementary Table 1) might lead to severe alterations in ecosystem functioning (Figure 2), because it involves the depletion of entire microorganisms’ communities integrated with multicellular units and their associated key ecosystem functions. Therefore, the focus of conservation and restoration must take into consideration the integrity of these functional units in order to preserve the emergent processes they carry out (Figure 2). Without these EHs, it will be impossible to ensure that safe continuity of nutrient cycles. Specifically, the probability that nitrogen and phosphorus cycles remain within boundaries that do not threaten the safe operating space for humanity may be under threat by the loss of EHs.
Detritivore arthropods and their microorganism partners constitute an important portion of the invertebrate-microbial biomass in multiple ecosystems and have a fundamental role as EHs in Earth’s nutrient cycling (Supplementary Table 1). Therefore, it is key to consider them in conservation and restoration efforts of ecological processes (Paoletti et al., 2007).
In order to synthesize our proposal, in this study we did not delve into other arthropods that could be considered as EHs, such as Siricidae wasps, oribatid mites, dung beetles, saproxylic beetles or springtails, among many others (Supplementary Table 1). For example, Sirex noctilio Fabricius, 1793 (Hymenoptera: Siricidae), inoculate the fungus Amylostereum areolatum (Amylostereaceae) in the wood, using it as an external digester of lignocellulose compounds (Breznak, 1982; Thompson et al., 2014) and bacterial symbiosis (Adams et al., 2011). It was discovered that Sirex cyaneus also has active enzymes of fungal origin in its guts (Kukor and Martin, 1983). However, to the best of our knowledge the role of Siricidae wasps and their influence on nutrient cycling has not been studied yet, therefore we have not treated this case in depth (see Supplementary Table 1). The same applies to the influences of bark beetle EHs. Although their contribution to nutrient cycles has been demonstrated (Kaňa et al., 2013), it is necessary to comprehend their effect on the ecosystems beyond the paradigms of forestry production (Six and Wingfield, 2011). Apart from the fact that these EHs can provoke a decrease in forest production, the increase in their density due to forest management can have an impact on biogeochemical cycles. Finally, it is necessary to consider the microbiota associated with arthropods as part of a joint epigenetic inheritance system (Villagra and Frías-Lasserre, 2020), which may allow the maintenance of lineages and the attributes associated with EHs. Finally, It is also necessary to explore into evolutionary processes such as horizontal transfer of genes from microorganisms to arthropods (Faddeeva-Vakhrusheva et al., 2017; Bredon et al., 2019), in order to understand the importance of this integration and thus make efforts that allow its conservation in order to secure as well as restore ecosystem functioning on our planet.
Author Contributions
All authors listed have made a substantial, direct and intellectual contribution to the work, and approved it for publication.
Funding
This work was funded by the “Beca Para Estudios de Doctorado Nacional” CONICYT-ANID No. 21180078 (to CS), Rufford Booster Grant No. 29177-B (to CS), and “Programa de Est mulo a la Excelencia Institucional (PEEI)” Universidad de Chile.
Conflict of Interest
The authors declare that the research was conducted in the absence of any commercial or financial relationships that could be construed as a potential conflict of interest.
Publisher’s Note
All claims expressed in this article are solely those of the authors and do not necessarily represent those of their affiliated organizations, or those of the publisher, the editors and the reviewers. Any product that may be evaluated in this article, or claim that may be made by its manufacturer, is not guaranteed or endorsed by the publisher.
Acknowledgments
We would like to thank Profs. Audrey Grez, César Marín, Luis Castañeda, Miguel Castillo, Juan Pablo Fuentes, and Cristian Villagra for their encouragement to write this article and insightful comments. We would also like to thank Prof. Lafayette Eaton for English review, Bruno Dastillung for format review, Margarita Muñoz (Fundación Planificable) for figures preparation, and two reviewers for the insightful comments.
Supplementary Material
The Supplementary Material for this article can be found online at: https://www.frontiersin.org/articles/10.3389/fmicb.2021.702763/full#supplementary-material
Glossary
Biogeochemical cycles: Cyclic flow of the biosphere elements, through a series of biochemical and geological processes (Jacobson et al., 2000).
Bionts: Organisms that make up the integration known as holobiont (Margulis and Fester, 1991).
Ecosystem engineers: Organisms whose actions modulate the availability of resources for other species (Jones et al., 1994).
Ecosystem Holobiont: Multicellular host and their associated microbiota participating in key ecological processes for the functioning of its ecosystems thanks to its holobiont-derived emergent properties (Figure 1, concept developed in this work).
Ecosystem multifunctionality: The ability of ecosystems to sustain multiple functions and services at the same time. This attribute is highly dependent on the biodiversity present in each ecosystem (Gamfeldt et al., 2008).
Endosymbiont: Symbiotic association where one of the associated organisms (bionts) inhabits inside the body of another biont (operational definition).
Exosymbiont or ectosymbiont: Symbiotic association where bionts inhabit different bodies (operational definition).
Holobiont: Functional symbiotic unit composed by the integration of multicellular and unicellular organisms. This holobiont occasionally generates a new features or attributes such as new morphological, physiological or immunological traits, among others. The holobiont concept has converged independently at different times possibly in the late 19th and early 20th century debates on the definition of symbiosis (Meyer-Abich, 1943; Margulis, 1990; Suárez, 2018; Baedke et al., 2020).
Hologenome: This correspond to multicellular host genome plus microbiome (Rosenberg et al., 2007; Zilber-Rosenberg and Rosenberg, 2008).
Hologenomic adaptation: Biological adaptations that have evolved in the holobiont or in a multispecies system (Suárez and Triviño, 2020).
Microbiome: Microbiota’s genome (Madigan et al., 2015).
Microbiota: Community of microorganisms that is normally located in multicellular host body (Madigan et al., 2015).
Multicellular/Multicellular organisms: Biont formed by more than one cell (Kaiser, 2001).
Nature’s contribution to people: Conceptual framework proposed by the Intergovernmental Science-Policy Platform on Biodiversity and Ecosystem Services (IPBES). This is based on the idea of ecosystem services (i.e., irreplaceable benefits for humanity provided by nature), but considering the interrelationship between humans and their ecosystems, and recognizing the role of local and indigenous people culture knowledge (Díaz et al., 2018).
Planetary Boundaries: Approach to global sustainability where safe operational limits for our species were defined. These limits include attributes of biodiversity, land use and biogeochemical cycles, among others (Rockström et al., 2009; Steffen et al., 2015).
Sustainable Development Goals (SDGs): Set of objectives developed at the summit of the United Nations Rio+20 in Brazil, 2012. These goals were proposed to reconcile environmental protection and socioeconomic development (Zeng et al., 2020).
Symbiogenesis: Mechanism by which evolution occurs as a result of symbiosis (Merezhkowsky, 1905; Sapp, 1994).
Symbiosis: In its most classical definition this correspond to biological interactions in which two entities differently named (different species) live together. Under this concept, cases of mutualism, commensalism and parasitism would be included under symbiosis conceptual umbrella (De Bary, 1879; Sapp, 1994; Oulhen et al., 2016; Suárez, 2018).
References
Adams, A. S., Jordan, M. S., Adams, S. M., Suen, G., Goodwin, L. A., Davenport, K. W., et al. (2011). Cellulose-degrading bacteria associated with the invasive woodwasp Sirex noctilio. ISME J. 5, 1323–1331. doi: 10.1038/ismej.2011.14
Ademolu, K. O., Idowu, A. B., Olabode, O. A., Olalonye, O., Adelabu, A. B., and Atayese, O. (2015). Effect of food plant sterilization on growth and nutritional value of adult variegated grasshopper Zonocerus variegatus (Linnaeus, 1758) (Orthoptera: Pyrgomorphidae). Braz. J. Biol. Sci. 2, 303–308.
Agamennone, V., Le, N. G., van Straalen, N. M., Brouwer, A., and Roelofs, D. (2019). Antimicrobial activity and carbohydrate metabolism in the bacterial metagenome of the soil-living invertebrate Folsomia candida. Sci. Rep. 9, 1–13. doi: 10.1038/s41598-019-43828-w
Alagesan, P. (2016). “Millipedes: diversity, distribution and ecology,” in Arthropod Diversity and Conservation in the Tropics and Sub-Tropics, eds A. K. Chakravarthy and S. Sridhara (Springer: Singapore), 119–137. doi: 10.1007/978-981-10-1518-2_7
Algeo, T. J., Scheckler, S. E., and Maynard, J. B. (2001). Effects of the Middle to Late Devonian Spread of Vascular Land Plants on Weathering Regimes, Marine Biotas, and Global Climate, in Plants Invade the Land: Evolutionary and Environmental Perspectives. New York, NY: Columbia University Press, 213–236. doi: 10.7312/gens11160-013
Allan, E., Manning, P., Alt, F., Binkenstein, J., Blaser, S., Blüthgen, N., et al. (2015). Land use intensification alters ecosystem multifunctionality via loss of biodiversity and changes to functional composition. Ecol. Lett. 18, 834–843. doi: 10.1111/ele.12469
Alonso-Pernas, P., Bartram, S., Arias-Cordero, E. M., Novoselov, A. L., Halty-deLeon, L., Shao, Y., et al. (2017). In vivo isotopic labeling of symbiotic bacteria involved in cellulose degradation and nitrogen recycling within the gut of the forest cockchafer (Melolontha hippocastani). Front. Microbiol. 8:1970. doi: 10.3389/fmicb.2017.01970
Andrés, P., Mateos, E., Tarrasón, D., Cabrera, C., and Figuerola, B. (2011). Effects of digested, composted, and thermally dried sewage sludge on soil microbiota and mesofauna. Appl. Soil Ecol. 48, 236–242. doi: 10.1016/j.apsoil.2011.03.001
Ardestani, M. M., Šustr, V., Hnilička, F., and Frouz, J. (2020). Food consumption of the cockroach species Blaptica dubia Serville (Blattodea: Blaberidae) using three leaf litter types in a microcosm design. Appl. Soil Ecol. 150:103460. doi: 10.1016/j.apsoil.2019.103460
Ashwini, K. M., and Sridhar, K. R. (2006). Breakdown of plantation residues by pill millipedes (Arthrosphaera magna) and assessment of compost quality. Curr. Sci. 90, 954–959.
Ayayee, P., Rosa, C., Ferry, J. G., Felton, G., Saunders, M., and Hoover, K. (2014). Gut microbes contribute to nitrogen provisioning in a wood-feeding cerambycid. Environ. Entomol. 43, 903–912. doi: 10.1603/EN14045
Ayayee, P. A., Kinney, G., Yarnes, C., Larsen, T., Custer, G. F., van Diepen, L. T., et al. (2020). Role of the gut microbiome in mediating standard metabolic rate after dietary shifts in the viviparous cockroach, Diploptera punctata. J. Exp. Biol. 223:jeb218271. doi: 10.1242/jeb.218271
Aylward, F. O., Burnum, K. E., Scott, J. J., Suen, G., Tringe, S. G., Adams, S. M., et al. (2012). Metagenomic and metaproteomic insights into bacterial communities in leaf-cutter ant fungus gardens. ISME J. 6, 1688–1701. doi: 10.1038/ismej.2012.10
Ayres, M. P., Wilkens, R. T., Ruel, J. J., Lombardero, M. J., and Vallery, E. (2000). Nitrogen budgets of phloem-feeding bark beetles with and without symbiotic fungi. Ecology 81, 2198–2210. doi: 10.2307/177108
Baedke, J., Fábregas-Tejeda, A., and Nieves Delgado, A. (2020). The holobiont concept before Margulis. J. Exp. Zool. Part B 334, 149–155. doi: 10.1002/jez.b.22931
Batterman, S. A., Hedin, L. O., Van Breugel, M., Ransijn, J., Craven, D. J., and Hall, J. S. (2013). Key role of symbiotic dinitrogen fixation in tropical forest secondary succession. Nature 502, 224–227. doi: 10.1038/nature12525
Beccaloni, G., and Eggleton, P. (2013). “Order Blattodea,” in Animal Biodiversity: An Outline of Higher-level Classification and Survey of Taxonomic Richness (Addenda 2013), Vol. 3703, ed. Z.-Q. Zhang (Auckland: Zootaxa), 46–48. doi: 10.11646/zootaxa.3703.1.10
Bell, W. J., Roth, L. M., and Nalepa, C. A. (2007). Cockroaches: Ecology, Behavior, and Natural History. Baltimore, MA: JHU Press.
Berlanga, M., Llorens, C., Comas, J., and Guerrero, R. (2016). Gut bacterial community of the xylophagous cockroaches Cryptocercus punctulatus and Parasphaeria boleiriana. PLoS One 11:e0152400. doi: 10.1371/journal.pone.0152400
Berner, R. A. (1990). Atmospheric carbon dioxide levels over Phanerozoic time. Science 249, 1382–1386. doi: 10.1126/science.249.4975.1382
Berner, R. A. (2001). “The effect of the rise of land plants on atmospheric CO2 during the Paleozoic,” in Plants Invade the Land: Evolutionary and Environmental Perspectives, eds P. G. Gensel and D. Edwards (New York, NY: Columbia University Press), 173–178. doi: 10.7312/gens11160-011
Bignell, D. E., Roisin, Y., and Lo, N. (eds) (2010). Biology of Termites: A Modern Synthesis. Berlin: Springer Science and Business Media, doi: 10.1007/978-90-481-3977-4
Birkemoe, T., Jacobsen, R. M., Sverdrup-Thygeson, A., and Biedermann, P. H. (2018). “Insect-fungus interactions in dead wood systems,” in Saproxylic Insects, ed. M. D. Ulyshen (Cham: Springer), 377–427. doi: 10.1007/978-3-319-75937-1_12
Bishop, T. R., Griffiths, H. M., Ashton, L. A., Eggleton, P., Woon, J. S., and Parr, C. L. (2020). Clarifying terrestrial recycling pathways. Trends Ecol. Evol. 36, 9–11. doi: 10.1016/j.tree.2020.09.005
Blatrix, R., Bouamer, S., Morand, S., and Selosse, M. A. (2009). Ant-plant mutualisms should be viewed as symbiotic communities. Plant Signal. Behav. 4, 554–556. doi: 10.4161/psb.4.6.8733
Bordenstein, S. R., and Theis, K. R. (2015). Host biology in light of the microbiome: ten principles of holobionts and hologenomes. PLoS Biol. 13:e1002226. doi: 10.1371/journal.pbio.1002226
Brauman, A., Majeed, M. Z., Buatois, B., Robert, A., Pablo, A. L., and Miambi, E. (2015). Nitrous oxide (N2O) emissions by termites: does the feeding guild matter? PLoS One 10:e0144340. doi: 10.1371/journal.pone.0144340
Brauman, K. A., Garibaldi, L. A., Polasky, S., Aumeeruddy-Thomas, Y., Brancalion, P. H., DeClerck, F., et al. (2020). Global trends in nature’s contributions to people. Proc. Natl. Acad. Sci. U.S.A. 117, 32799–32805. doi: 10.1073/pnas.2010473117
Bredon, M., Dittmer, J., Noël, C., Moumen, B., and Bouchon, D. (2018). Lignocellulose degradation at the holobiont level: teamwork in a keystone soil invertebrate. Microbiome 6:162.
Bredon, M., Herran, B., Bertaux, J., Grève, P., Moumen, B., and Bouchon, D. (2020). Isopod holobionts as promising models for lignocellulose degradation. Biotechnol. Biofuels 13, 1–14. doi: 10.1186/s13068-020-01683-2
Bredon, M., Herran, B., Lheraud, B., Bertaux, J., Grève, P., Moumen, B., et al. (2019). Lignocellulose degradation in isopods: new insights into the adaptation to terrestrial life. BMC Genomics 20:462. doi: 10.1186/s12864-019-5825-8
Breznak, J. A. (1982). Intestinal microbiota of termites and other xylophagous insects. Annu. Rev. Microbiol. 36, 323–323. doi: 10.1146/annurev.mi.36.100182.001543
Bridges, J. R. (1981). Nitrogen-fixing bacteria associated with bark beetles. Microb. Ecol. 7, 131–137. doi: 10.1007/BF02032495
Brooks, T. M., Mittermeier, R. A., Mittermeier, C. G., Da Fonseca, G. A., Rylands, A. B., Konstant, W. R., et al. (2002). Habitat loss and extinction in the hotspots of biodiversity. Conserv. Biol. 16, 909–923. doi: 10.1046/j.1523-1739.2002.00530.x
Brugerolle, G., Silva-Neto, I. D., Pellens, R., and Grandcolas, P. (2003). Electron microscopic identification of the intestinal protozoan flagellates of the xylophagous cockroach Parasphaeria boleiriana from Brazil. Parasitol. Res. 90, 249–256. doi: 10.1007/s00436-003-0832-7
Brune, A. (2014). Symbiotic digestion of lignocellulose in termite guts. Nat. Rev. Microbiol. 12, 168–180. doi: 10.1038/nrmicro3182
Brune, A., and Stingl, U. (2005). “Prokaryotic symbionts of termite gut flagellates: phylogenetic and metabolic implications of a tripartite symbiosis,” in Molecular Basis of Symbiosis, ed. J. Overmann (Berlin: Springer), 39–60. doi: 10.1007/3-540-28221-1_3
Bution, M. L., and Caetano, F. H. (2008). Ileum of the Cephalotes ants: a specialized structure to harbor symbionts microorganisms. Micron 39, 897–909. doi: 10.1016/j.micron.2007.11.008
Byzov, B. A., Thanh, V. N., and Babjeva, I. P. (1993). Interrelationships between yeasts and soil diplopods. Soil Biol. Biochem. 25, 1119–1126. doi: 10.1016/0038-0717(93)90160-D
Calderón-Cortés, N., Quesada, M., Watanabe, H., Cano-Camacho, H., and Oyama, K. (2012). Endogenous plant cell wall digestion: a key mechanism in insect evolution. Annu. Rev. Ecol. Evol. Syst. 43, 45–71. doi: 10.1146/annurev-ecolsys-110411-160312
Caniani, D., Labella, A., Lioi, D. S., Mancini, I. M., and Masi, S. (2016). Habitat ecological integrity and environmental impact assessment of anthropic activities: a GIS-based fuzzy logic model for sites of high biodiversity conservation interest. Ecol. Indic. 67, 238–249. doi: 10.1016/j.ecolind.2016.02.038
Cardoso, P., Erwin, T. L., Borges, P. A., and New, T. R. (2011). The seven impediments in invertebrate conservation and how to overcome them. Biol. Conserv. 144, 2647–2655. doi: 10.1016/j.biocon.2011.07.024
Carrasco, P., Pérez-Cobas, A. E., Van de Pol, C., Baixeras, J., Moya, A., and Latorre, A. (2014). Succession of the gut microbiota in the cockroach Blattella germanica. Int. Microbiol. 17, 99–109. doi: 10.2436/20.1501.01.212
Carthey, A. J., Blumstein, D. T., Gallagher, R. V., Tetu, S. G., and Gillings, M. R. (2019). Conserving the holobiont. Funct. Ecol. 34, 764–776. doi: 10.1111/1365-2435.13504
Catania, F., Krohs, U., Chittò, M., Ferro, D., Ferro, K., Leppennetier, G., et al. (2016). The hologenome concept: we need to incorporate function. Theory Biosci. 136, 89–98. doi: 10.1007/s12064-016-0240-z
Chen, C. L., and Shaner, P. J. L. (2018). Effects of greenfall on ground-dwelling arthropods in a subtropical forest. Zool. Stud. 57:e44. doi: 10.6620/ZS.2018.57-44
Crawford, C. S. (1976). Feeding-season production in the desert millipede Orthoporus ornatus (Girard)(Diplopoda). Oecologia 24, 265–276. doi: 10.1007/BF00345478
Crawford, C. S. (1992). Millipedes as model detritivores. Berichte des Naturwissenschaftlich-Medizinischen Verein Innsbruck. 10, 277–288.
Crawford, R. L. (2005). Microbial diversity and its relationship to planetary protection. Appl. Environ. Microbiol. 71, 4163–4168. doi: 10.1128/AEM.71.8.4163-4168.2005
Cruden, D. L., and Markovetz, A. J. (1987). Microbial ecology of the cockroach gut. Annu. Rev. Microbiol. 41, 617–643. doi: 10.1146/annurev.mi.41.100187.003153
da Silva, V. M., Antoniolli, Z. I., Jacques, R. J. S., Ott, R., da Silva Rodrigues, P. E., Andrade, F. V., et al. (2017). Influence of the tropical millipede, Glyphiulus granulatus (Gervais, 1847), on aggregation, enzymatic activity, and phosphorus fractions in the soil. Geoderma 289, 135–141. doi: 10.1016/j.geoderma.2016.11.031
da Silva Correia, D., Passos, S. R., Proença, D. N., Morais, P. V., Xavier, G. R., and Correia, M. E. F. (2018). Microbial diversity associated to the intestinal tract of soil invertebrates. Appl. Soil Ecol. 131, 38–46. doi: 10.1016/j.apsoil.2018.07.009
Davies, A. B., Brodrick, P. G., Parr, C. L., and Asner, G. P. (2020). Resistance of mound-building termites to anthropogenic land-use change. Environ. Res. Lett. 15:094038. doi: 10.1088/1748-9326/aba0ff
Davies, A. B., Parr, C. L., and Van Rensburg, B. J. (2010). Termites and fire: current understanding and future research directions for improved savanna conservation. Austr. Ecol. 35, 482–486. doi: 10.1111/j.1442-9993.2010.02124.x
De Bary, A. (1879). Die Erscheinung der Symbiose: Vortrag gehalten auf der Versammlung Deutscher Naturforscher und Aerzte zu Cassel. Strasbourg: K. J. Trübner.
Díaz, S., Pascual, U., Stenseke, M., Martín-López, B., Watson, R. T., Molnár, Z., et al. (2018). Assessing nature’s contributions to people. Science 359, 270–272. doi: 10.1126/science.aap8826
Dietrich, C., Köhler, T., and Brune, A. (2014). The cockroach origin of the termite gut microbiota: patterns in bacterial community structure reflect major evolutionary events. Appl. Environ. Microbiol. 80, 2261–2269. doi: 10.1128/AEM.04206-13
Ding, J., Zhu, D., Chen, Q. L., Zheng, F., Wang, H. T., and Zhu, Y. G. (2019a). Effects of long-term fertilization on the associated microbiota of soil collembolan. Soil Biol. Biochem. 130, 141–149. doi: 10.1016/j.soilbio.2018.12.015
Ding, J., Zhu, D., Li, H., Ding, K., Chen, Q. L., Lassen, S. B., et al. (2019b). The gut microbiota of soil organisms shows species-specific responses to liming. Sci. Total Environ. 659, 715–723. doi: 10.1016/j.scitotenv.2018.12.445
Dittmer, J., Lesobre, J., Moumen, B., and Bouchon, D. (2016). Host origin and tissue microhabitat shaping the microbiota of the terrestrial isopod Armadillidium vulgare. FEMS Microbiol. Ecol. 92:fiw063. doi: 10.1093/femsec/fiw063
Dodd, M. S., Papineau, D., Grenne, T., Slack, J. F., Rittner, M., Pirajno, F., et al. (2017). Evidence for early life in Earth’s oldest hydrothermal vent precipitates. Nature 543, 60–64. doi: 10.1038/nature21377
Doolittle, W. F., and Booth, A. (2017). It’s the song not the singer: an exploration of holobiosis and evolutionary theory. Biol. Philos. 32, 5–24. doi: 10.1007/s10539-016-9542-2
Douglas, A. E., and Werren, J. H. (2016). Holes in the hologenome: why host-microbe symbioses are not holobionts. mBio. 7:e02099-15. doi: 10.1128/mBio.02099-15
Dupré, J. (2017). The metaphysics of evolution. Interface Focus 7:20160148. doi: 10.1098/rsfs.2016.0148
Eggleton, P. (2020). The State of the World’s Insects. Annu. Rev. Environ. Resour. 45, 61–82. doi: 10.1146/annurev-environ-012420-050035
Engel, P., and Moran, N. A. (2013). The gut microbiota of insects–diversity in structure and function. FEMS Microbiol. Rev. 37, 699–735. doi: 10.1111/1574-6976.12025
Evangelista, D. A., Russell, G., Russell, K. N., Bourne, G., and Ware, J. L. (2017). Evidence that dispersal barriers influence blaberoid cockroach assemblages in a neotropical savanna–forest matrix. Insect Conserv. Divers. 10, 425–438. doi: 10.1111/icad.12246
Faddeeva-Vakhrusheva, A., Kraaijeveld, K., Derks, M. F., Anvar, S. Y., Agamennone, V., Suring, W., et al. (2017). Coping with living in the soil: the genome of the parthenogenetic springtail Folsomia candida. BMC Genomics 18:493. doi: 10.1186/s12864-017-3852-x
Falkowski, P. G., Fenchel, T., and Delong, E. F. (2008). The microbial engines that drive Earth’s biogeochemical cycles. Science 320, 1034–1039. doi: 10.1126/science.1153213
Farji-Brener, A. G., and Werenkraut, V. (2015). A meta-analysis of leaf-cutting ant nest effects on soil fertility and plant performance. Ecol. Entomol. 40, 150–158. doi: 10.1111/een.12169
Farji-Brener, A. G., and Werenkraut, V. (2017). The effects of ant nests on soil fertility and plant performance: a meta-analysis. J. Anim. Ecol. 86, 866–877. doi: 10.1111/1365-2656.12672
Fenchel, T., Blackburn, H., King, G. M., and Blackburn, T. H. (2012). Bacterial Biogeochemistry: The Ecophysiology of Mineral Cycling. Cambridge, MA: Academic press.
Ferreira, N. G., Morgado, R. G., Cunha, L., Novo, M., Soares, A. M., Morgan, A. J., et al. (2019). Unravelling the molecular mechanisms of nickel in woodlice. Environ. Res. 176:108507. doi: 10.1016/j.envres.2019.05.038
Finlay, B. J., Maberly, S. C., and Cooper, J. I. (1997). Microbial diversity and ecosystem function. Oikos 80, 209–213. doi: 10.2307/3546587
Floudas, D., Binder, M., Riley, R., Barry, K., Blanchette, R. A., Henrissat, B., et al. (2012). The Paleozoic origin of enzymatic lignin decomposition reconstructed from 31 fungal genomes. Science 336, 1715–1719. doi: 10.1126/science.1221748
Fox, C. J. S. (1964). The effects of five herbicides on the numbers of certain invertebrate animals in grassland soil. Can. J. Plant Sci. 44, 405–409. doi: 10.4141/cjps64-080
Frade, P. R., De Jongh, F., Vermeulen, F., Van Bleijswijk, J., and Bak, R. P. M. (2008). Variation in symbiont distribution between closely related coral species over large depth ranges. Mol. Ecol. 17, 691–703. doi: 10.1111/j.1365-294X.2007.03612.x
Freymann, B. P., Buitenwerf, R., Desouza, O., and Olff, H. (2008). The importance of termites (Isoptera) for the recycling of herbivore dung in tropical ecosystems: a review. Eur. J. Entomol. 105, 165–173. doi: 10.14411/eje.2008.025
Gales, A., Chatellard, L., Abadie, M., Bonnafous, A., Auer, L., Carrère, H., et al. (2018). Screening of phytophagous and xylophagous insects guts microbiota abilities to degrade lignocellulose in bioreactor. Front. Microbiol. 9:2222. doi: 10.3389/fmicb.2018.02222
Galloway, J. N., Townsend, A. R., Erisman, J. W., Bekunda, M., Cai, Z., Freney, J. R., et al. (2008). Transformation of the nitrogen cycle: recent trends, questions, and potential solutions. Science 320, 889–892. doi: 10.1126/science.1136674
Gamfeldt, L., Hillebrand, H., and Jonsson, P. R. (2008). Multiple functions increase the importance of biodiversity for overall ecosystem functioning. Ecology 89, 1223–1231. doi: 10.1890/06-2091.1
Gerlach, A., Russell, D. J., Jaeschke, B., and Römbke, J. (2014). Feeding preferences of native terrestrial isopod species (Oniscoidea, Isopoda) for native and introduced leaf litter. Appl. Soil Ecol. 83, 95–100. doi: 10.1016/j.apsoil.2014.02.006
Gillings, M. R., and Paulsen, I. T. (2014). Microbiology of the Anthropocene. Anthropocene 5, 1–8. doi: 10.1016/j.ancene.2014.06.004
Golovatch, S. I., and Kime, R. D. (2009). Millipede (Diplopoda) distributions: a review. Soil Org. 81, 565–597.
González-Alcaraz, M. N., Malheiro, C., Cardoso, D. N., Prodana, M., Morgado, R. G., van Gestel, C. A., et al. (2020). “Bioaccumulation and toxicity of organic chemicals in terrestrial invertebrates,” in Bioavailability of Organic Chemicals in Soil and Sediment, eds J. J. Ortega-Calvo and J. R. Parsons (Berlin: Springer), doi: 10.1007/698_2020_511
González-Escobar, J. L., Pereyra-Camacho, M. A., De Léon-Rodríguez, A., Grajales-Lagunes, A., Reyes-Agüero, A., and Chagolla-López, A. (2020). Biodegradation of recalcitrant compounds and phthalates by culturable bacteria isolated from Liometopum apiculatum microbiota. World J. Microbiol. Biotechnol. 36, 1–13. doi: 10.1007/s11274-020-02850-1
Granada, C. E., Passaglia, L. M., de Souza, E. M., and Sperotto, R. A. (2018). Is phosphate solubilization the forgotten child of plant growth-promoting rhizobacteria? Front. Microbiol. 9:2054. doi: 10.3389/fmicb.2018.02054
Grandcolas, P. (1993a). Habitats of solitary and gregarious species in the neotropical Zetoborinae (Insecta, Blattaria). Stud. Neotrop. Fauna Environ. 28, 179–190. doi: 10.1080/01650529309360902
Grandcolas, P. (1993b). Le genre Paramuzoa Roth, 1973 : sa répartition et un cas de xylographie chez les Nyctiborinae (Dictyoptera, Blattaria). Bull. Soc. Entomol. Fr. 98, 131–138.
Grandcolas, P. (1995). The appearance of xylophagy in cockroaches: two case studies with reference to phylogeny. J. Orthoptera Res. 4, 177–184. doi: 10.2307/3503473
Grandcolas, P., and Deleporte, P. (1996). The origin of Protistan symbionts in termites and cockroaches: a phylogenetic analysis. Cladistics 12, 93–98. doi: 10.1111/j.1096-0031.1996.tb00195.x
Grimaldi, D., and Engel, M. S. (eds) (2005). Evolution of the Insects. Cambridge, MA: Cambridge University Press.
Guerrero, R., Margulis, L., and Berlanga, M. (2013). Symbiogenesis: the holobiont as a unit of evolution. Int. Microbiol. 16, 133–143. doi: 10.2436/20.1501.01.188
Guiry, E., Beglane, F., Szpak, P., Schulting, R., McCormick, F., and Richards, M. P. (2018). Anthropogenic changes to the Holocene nitrogen cycle in Ireland. Sci. Adv. 4:eaas9383. doi: 10.1126/sciadv.aas9383
Guzev, V. S., and Byzov, B. A. (2006). Morphometric analysis of bacteria associated with soil millipedes. Microbiology 75, 219–225. doi: 10.1134/S0026261706020172
Haines, B. (1975). “Impact of leaf-cutting ants on vegetation development at barro colorado Island,” in Tropical Ecological Systems, eds F. B. Golley and E. Medina (Berlin: Springer), 99–111. doi: 10.1007/978-3-642-88533-4_8
Hallmann, C. A., Sorg, M., Jongejans, E., Siepel, H., Hofland, N., Schwan, H., et al. (2017). More than 75 percent decline over 27 years in total flying insect biomass in protected areas. PLoS One 12:e0185809. doi: 10.1371/journal.pone.0185809
Hammer, T. J., Fierer, N., Hardwick, B., Simojoki, A., Slade, E., Taponen, J., et al. (2016). Treating cattle with antibiotics affects greenhouse gas emissions, and microbiota in dung and dung beetles. Proc. R. Soc. B 283:20160150. doi: 10.1098/rspb.2016.0150
Harit, A., Shanbhag, R., Chaudhary, E., Cheik, S., and Jouquet, P. (2017). Properties and functional impact of termite sheetings. Biol. Fertil. Soils 53, 743–749. doi: 10.1007/s00374-017-1228-7
Hättenschwiler, S., and Gasser, P. (2005). Soil animals alter plant litter diversity effects on decomposition. Proc. Natl. Acad. Sc. U.S.A. 102, 1519–1524. doi: 10.1073/pnas.0404977102
Hayatsu, M., Tago, K., and Saito, M. (2008). Various players in the nitrogen cycle: diversity and functions of the microorganisms involved in nitrification and denitrification. J. Soil Sci. Plant Nutr. 54, 33–45. doi: 10.1111/j.1747-0765.2007.00195.x
Hector, A., and Bagchi, R. (2007). Biodiversity and ecosystem multifunctionality. Nature 448, 188–190. doi: 10.1038/nature05947
Hedde, M., Aubert, M., Bureau, F., Margerie, P., and Decaëns, T. (2007). Soil detritivore macro-invertebrate assemblages throughout a managed beech rotation. Ann. For. Sci. 64, 219–228. doi: 10.1051/forest:2006106
Hellemans, S., Marynowska, M., Drouet, T., Lepoint, G., Fournier, D., Calusinska, M., et al. (2019). Nest composition, stable isotope ratios and microbiota unravel the feeding behaviour of an inquiline termite. Oecologia 191, 541–553. doi: 10.1007/s00442-019-04514-w
Higashi, M., Abe, T., and Burns, T. P. (1992). Carbon—nitrogen balance and termite ecology. Proc. R. Soc. B 249, 303–308. doi: 10.1098/rspb.1992.0119
Hinkelman, J., and Vršanská, L. (2020). A Myanmar amber cockroach with protruding feces contains pollen and a rich microcenosis. Sci. Nat. 107, 1–19. doi: 10.1007/s00114-020-1669-y
Hobbelen, P. H. F., Koolhaas, J. E., and Van Gestel, C. A. M. (2004). Risk assessment of heavy metal pollution for detritivores in floodplain soils in the Biesbosch, The Netherlands, taking bioavailability into account. Environ. Pollut. 129, 409–419. doi: 10.1016/j.envpol.2003.11.010
Hohmann-Marriott, M. F., and Blankenship, R. E. (2011). Evolution of photosynthesis. Annu. Rev. Plant Biol. 62, 515–548. doi: 10.1146/annurev-arplant-042110-103811
Hooper, D. U., Adair, E. C., Cardinale, B. J., Byrnes, J. E., Hungate, B. A., Matulich, K. L., et al. (2012). A global synthesis reveals biodiversity loss as a major driver of ecosystem change. Nature 486, 105–108. doi: 10.1038/nature11118
Hopkin, S. P., and Martin, M. H. (1982). The distribution of zinc, cadmium, lead and copper within the hepatopancreas of a woodlouse. Tissue Cell 14, 703–715. doi: 10.1016/0040-8166(82)90060-X
Hu, Y., Sanders, J. G., Łukasik, P., D’Amelio, C. L., Millar, J. S., Vann, D. R., et al. (2018). Herbivorous turtle ants obtain essential nutrients from a conserved nitrogen-recycling gut microbiome. Nat. Commun. 9, 1–14. doi: 10.1038/s41467-018-03357-y
Intergovernmental Science-Policy Platform on Biodiversity and Ecosystem Services (IPBES) and United Nations (2020). Media Release: Nature’s Dangerous Decline ‘Unprecedented’; Species Extinction Rates ‘Accelerating’. New York, NY: United Nations.
IPBES (2019). Summary for Policymakers of the Global Assessment Report on Biodiversity and Ecosystem Services of the Intergovernmental Science-Policy Platform on Biodiversity and Ecosystem Services, eds S. Díaz, J. Settele, E. S. Brondízio, H. T. Ngo, M. Guèze, J. Agard, et al. (Bonn: IPBES secretariat), 56.
Jacobson, M., Charlson, R. J., Rodhe, H., and Orians, G. H. (2000). Earth System Science: From Biogeochemical Cycles to Global Changes. Cambridge, MA: Academic Press.
Jamali, H., Livesley, S. J., Grover, S. P., Dawes, T. Z., Hutley, L. B., Cook, G. D., et al. (2011). The importance of termites to the CH 4 balance of a tropical savanna woodland of northern Australia. Ecosystems 14, 698–709. doi: 10.1007/s10021-011-9439-5
Johnson, K. A., and Whitford, W. G. (1975). Foraging ecology and relative importance of subterranean termites in Chihuahuan desert ecosystems. Environ. Entomol. 4, 66–70. doi: 10.1093/ee/4.1.66
Jones, C. G., Lawton, J. H., and Shachak, M. (1994). “Organisms as ecosystem engineers,” in Ecosystem Management, eds F. B. Samson and F. L. Knopf (New York, NY: Springer), 130–147. doi: 10.2307/3545850
Jouquet, P., Bottinelli, N., Shanbhag, R. R., Bourguignon, T., Traoré, S., and Abbasi, S. A. (2016). Termites: the neglected soil engineers of tropical soils. Soil Sci. 181, 157–165. doi: 10.1097/SS.0000000000000119
Jouquet, P., Dauber, J., Lagerlöf, J., Lavelle, P., and Lepage, M. (2006). Soil invertebrates as ecosystem engineers : intended and accidental effects on soil and feedback loops. Appl. Soil Ecol. 32, 153–164. doi: 10.1016/j.apsoil.2005.07.004
Jouquet, P., Traoré, S., Choosai, C., Hartmann, C., and Bignell, D. (2011). Influence of termites on ecosystem functioning. Ecosystem services provided by termites. Eur. J. Soil Biol. 47, 215–222. doi: 10.1016/j.ejsobi.2011.05.005
Ju, J. F., Bing, X. L., Zhao, D. S., Guo, Y., Xi, Z., Hoffmann, A. A., et al. (2020). Wolbachia supplement biotin and riboflavin to enhance reproduction in planthoppers. ISME J. 14, 676–687. doi: 10.1038/s41396-019-0559-9
Jurgensen, M. F., Finér, L., Domisch, T., Kilpeläinen, J., Punttila, P., Ohashi, M., et al. (2008). Organic mound-building ants: their impact on soil properties in temperate and boreal forests. J. Appl. Entomol. 132, 266–275. doi: 10.1111/j.1439-0418.2008.01280.x
Kaiser, D. (2001). Building a multicellular organism. Annu. Rev. Genet. 35, 103–123. doi: 10.1146/annurev.genet.35.102401.090145
Kaiser, D., Lepage, M., Konaté, S., and Linsenmair, K. E. (2017). Ecosystem services of termites (Blattoidea: Termitoidae) in the traditional soil restoration and cropping system Zaï in northern Burkina Faso (West Africa). Agric. Ecosyst. Environ. 236, 198–211. doi: 10.1016/j.agee.2016.11.023
Kaňa, J., Tahovská, K., and Kopáček, J. (2013). Response of soil chemistry to forest dieback after bark beetle infestation. Biogeochemistry 113, 369–383. doi: 10.1007/s10533-012-9765-5
Kasting, J. F., and Siefert, J. L. (2002). Life and the evolution of Earth’s atmosphere. Science 296, 1066–1068. doi: 10.1016/B978-0-12-409548-9.11853-6
Kautz, G., and Topp, W. (1999). Acquisition of microbial communities and enhanced availability of soil nutrients by the isopod Porcellio scaber (Latr.)(Isopoda: Oniscidea). Biol. Fertil. Soils 31, 102–107. doi: 10.1007/s003740050631
Kautz, G., Zimmer, M., and Topp, W. (2000). Responses of the parthenogenetic isopod, Trichoniscus pusillus (Isopoda: Oniscidea), to changes in food quality. Pedobiologia 44, 75–85. doi: 10.1078/S0031-4056(04)70029-3
Kazuhira, Y., Hideaki, K., and Hirofumi, T. (1991a). Paracoprid dung beetles and gaseous loss of nitrogen from cow dung. Soil Biol. Biochem. 23, 643–647. doi: 10.1016/0038-0717(91)90077-W
Kazuhira, Y., Hdeaki, K., Takuro, K., and Toshiharu, A. (1991b). Nitrogen mineralization and microbial populations in cow dung, dung balls and underlying soil affected by paracoprid dung beetles. Soil Biol. Biochem. 23, 649–653. doi: 10.1016/0038-0717(91)90078-X
Kellogg, D. W., and Taylor, E. L. (2004). Evidence of oribatid mite detritivory in Antarctica during the late Paleozoic and Mesozoic. J. Paleontol. 78, 1146–1153. doi: 10.1017/S002233600004395X
Kirkendall, L. R., Biedermann, P. H., and Jordal, B. H. (2015). “Evolution and diversity of bark and ambrosia beetles,” in Bark Beetles, eds F. Vega and R. Hofstetter (Cambridge, MA: Academic Press), 85–156. doi: 10.1016/B978-0-12-417156-5.00003-4
Kitade, O. (2004). Comparison of symbiotic flagellate faunae between termites and a wood-feeding cockroach of the genus Cryptocercus. Microbes Environ. 19, 215–220. doi: 10.1264/jsme2.19.215
Klass, K. D., Nalepa, C., and Lo, N. (2008). Wood-feeding cockroaches as models for termite evolution (Insecta: Dictyoptera): Cryptocercus vs. Parasphaeria boleiriana. Mol. Phylogenetics Evol. 46, 809–817. doi: 10.1016/j.ympev.2007.11.028
Klepzig, K. D., Adams, A. S., Handelsman, J., and Raffa, K. F. (2009). Symbioses: a key driver of insect physiological processes, ecological interactions, evolutionary diversification, and impacts on humans. Environ. Entomol. 38, 67–77. doi: 10.1603/022.038.0109
Köhler, T., Dietrich, C., Scheffrahn, R. H., and Brune, A. (2012). High-resolution analysis of gut environment and bacterial microbiota reveals functional compartmentation of the gut in wood-feeding higher termites (Nasutitermes spp.). Appl. Environ. Microbiol. 78, 4691–4701. doi: 10.1128/AEM.00683-12
Koide, R. T. (2000). Functional complementarity in the arbuscular mycorrhizal symbiosis. New Phytol. 147, 233–235. doi: 10.1046/j.1469-8137.2000.00710.x
Krumbein, W. E. (1996). Geophysiology and parahistology of the interactions of organisms with the environment. Mar. Ecol. 17, 1–21. doi: 10.1111/j.1439-0485.1996.tb00486.x
Kukor, J. J., and Martin, M. M. (1983). Acquisition of digestive enzymes by siricid woodwasps from their fungal symbiont. Science 220, 1161–1163. doi: 10.1126/science.220.4602.1161
Lacroix, G., and Abbadie, L. (1998). Linking biodiversity and ecosystem function: an introduction. Acta Oecol. 19, 189–193. doi: 10.1016/S1146-609X(98)80023-4
Lade, S. J., Steffen, W., de Vries, W., Carpenter, S. R., Donges, J. F., Gerten, D., et al. (2019). Human impacts on planetary boundaries amplified by Earth system interactions. Nat. Sustain 3, 1–10. doi: 10.1038/s41893-019-0454-4
Lampert, N., Mikaelyan, A., and Brune, A. (2019). Diet is not the primary driver of bacterial community structure in the gut of litter-feeding cockroaches. BMC Microbiol. 19:238. doi: 10.1186/s12866-019-1601-9
LeClare, S. K., Mdluli, M., Wisely, S. M., and Stevens, N. (2020). Land-use diversity within an agricultural landscape promotes termite nutrient cycling services in a southern African savanna. Glob. Ecol. Conserv. 21:e00885. doi: 10.1016/j.gecco.2019.e00885
Lister, B. C., and Garcia, A. (2018). Climate-driven declines in arthropod abundance restructure a rainforest food web. PNAS 115, E10397–E10406. doi: 10.1073/pnas.1722477115
Liu, N., Li, H., Chevrette, M. G., Zhang, L., Cao, L., Zhou, H., et al. (2019). Functional metagenomics reveals abundant polysaccharide-degrading gene clusters and cellobiose utilization pathways within gut microbiota of a wood-feeding higher termite. ISME J. 13, 104–117. doi: 10.1038/s41396-018-0255-1
Liu, N., Zhang, L., Zhou, H., Zhang, M., Yan, X., Wang, Q., et al. (2013). Metagenomic insights into metabolic capacities of the gut microbiota in a fungus-cultivating termite (Odontotermes yunnanensis). PLoS One 8:e69184. doi: 10.1371/journal.pone.0069184
Lloyd, E., and Wade, M. J. (2019). Criteria for holobionts from community genetics. Biol. Theory 14, 151–170. doi: 10.1007/s13752-019-00322-w
Lucas, J., Bill, B., Stevenson, B., and Kaspari, M. (2017). The microbiome of the ant-built home: the microbial communities of a tropical arboreal ant and its nest. Ecosphere 8:e01639. doi: 10.1002/ecs2.1639
Lucas, J. M., Gora, E., Salzberg, A., and Kaspari, M. (2019). Antibiotics as chemical warfare across multiple taxonomic domains and trophic levels in brown food webs. Proc. R. S. B 286:20191536. doi: 10.1098/rspb.2019.1536
Luo, C., Li, Y., Chen, Y., Fu, C., Long, W., Xiao, X., et al. (2019). Bamboo lignocellulose degradation by gut symbiotic microbiota of the bamboo snout beetle Cyrtotrachelus buqueti. Biotechnol. Biofuels 12:70. doi: 10.1186/s13068-019-1411-1
Madigan, M., Martinko, J., Bender, K., Buckley, H., and Stahl, D. (2015). Brock Biology of Microorganisms, 14th Edn. London: Pearson Education Inc, 1006.
Madsen, E. L. (2011). Microorganisms and their roles in fundamental biogeochemical cycles. Curr. Opin. Biotechnol. 22, 456–464. doi: 10.1016/j.copbio.2011.01.008
Maestre, F. T., Quero, J. L., Gotelli, N. J., Escudero, A., Ochoa, V., Delgado-Baquerizo, M., et al. (2012). Plant species richness and ecosystem multifunctionality in global drylands. Science 335, 214–218. doi: 10.1126/science.1215442
Margulis, L. (1990). Words as battle cries: symbiogenesis and the new field of endocytobiology. Bioscience 40, 673–677.
Margulis, L. (1993). Symbiosis in cell Evolution: Microbial Communities in the Archean and Proterozoic Eons. New York, NY: W.H. Freeman and Co. Ltd, doi: 10.2307/1311435
Margulis, L., and Fester, R. (eds) (1991). Symbiosis as a Source of Evolutionary Innovation: Speciation and Morphogenesis. Cambridge, MA: MIT Press.
Martin, M. M. (1991). The evolution of cellulose digestion in insects. Phil. Trans. Biol Sci. 333, 281–288. doi: 10.1098/rstb.1991.0078
Marzinelli, E. M., Qiu, Z., Dafforn, K. A., Johnston, E. L., Steinberg, P. D., and Mayer-Pinto, M. (2018). Coastal urbanisation affects microbial communities on a dominant marine holobiont. npj Biofilms Microbiomes 4, 1–7. doi: 10.1038/s41522-017-0044-z
Matos, I. T. S. R., Assunção, E. N., Carmo, E. J. D., Soares, V. M., and Astolfi-Filho, S. (2017). Isolation and characterization of yeasts able to assimilate sugarcane bagasse hemicellulosic hydrolysate and produce xylitol associated with veturius transversus (Passalidae, Coleoptera, and Insecta). Int. J. Microbiol. 2017:5346741. doi: 10.1155/2017/5346741
Mendes, R., Garbeva, P., and Raaijmakers, J. M. (2013). The rhizosphere microbiome: significance of plant beneficial, plant pathogenic, and human pathogenic microorganisms. FEMS Microbiol. Rev. 37, 634–663. doi: 10.1111/1574-6976.12028
Merezhkowsky, C. (1905). Über natur und ursprung der chromatophoren imPflanzanreiche. Biologisches Centralblatt 25, 593–604.
Meyer-Abich, A. (1943). Beiträge zur Theorie der Evolution der Organismen. I. Das typologische Grundgesetz und seine Folgerungen für Phylogenie und Entwicklungsphysiologie [Contributions to the evolutionary theory of organisms: I. The basic typological law and its implications for phylogeny and developmental physiology]. Acta Biotheor. 7, 1–80. doi: 10.1007/BF01603792
Morales-Jiménez, J., de León, A. V. P., García-Domínguez, A., Martínez-Romero, E., Zúñiga, G., and Hernández-Rodríguez, C. (2013). Nitrogen-fixing and uricolytic bacteria associated with the gut of Dendroctonus rhizophagus and Dendroctonus valens (Curculionidae: Scolytinae). Microb. Ecol. 66, 200–210. doi: 10.1007/s00248-013-0206-3
Morales-Jiménez, J., Zúñiga, G., Villa-Tanaca, L., and Hernández-Rodríguez, C. (2009). Bacterial community and nitrogen fixation in the red turpentine beetle, Dendroctonus valens LeConte (Coleoptera: Curculionidae: Scolytinae). Microb. Ecol. 58, 879–891. doi: 10.1007/s00248-009-9548-2
Moran, N. A., and Sloan, D. B. (2015). The hologenome concept: helpful or hollow? PLoS Biol. 13:e1002311. doi: 10.1371/journal.pbio.1002311
Morehouse, K., Johns, T., Kaye, J., and Kaye, M. (2008). Carbon and nitrogen cycling immediately following bark beetle outbreaks in southwestern ponderosa pine forests. For. Ecol. Manag. 255, 2698–2708. doi: 10.1016/j.foreco.2008.01.050
Moya, A., and Ferrer, M. (2016). Functional redundancy-induced stability of gut microbiota subjected to disturbance. Trends Microbiol. 24, 402–413. doi: 10.1016/j.tim.2016.02.002
Murienne, J., Pellens, R., Budinoff, R. B., Wheeler, W. C., and Grandcolas, P. (2008). Phylogenetic analysis of the endemic new caledonian cockroach Lauraesilpha. Testing competing hypotheses of diversification. Cladistics 24, 802–812. doi: 10.1111/j.1096-0031.2008.00204.x
Muvengwi, J., Mbiba, M., Ndagurwa, H. G., Nyamadzawo, G., and Nhokovedzo, P. (2017). Termite diversity along a land use intensification gradient in a semi-arid savanna. J. Insect. Conserv. 21, 801–812. doi: 10.1007/s10841-017-0019-7
Myer, A., and Forschler, B. T. (2019). Evidence for the role of subterranean termites (Reticulitermes spp.) in temperate forest soil nutrient cycling. Ecosystems 22, 602–618. doi: 10.1007/s10021-018-0291-8
Naeem, S., Duffy, J. E., and Zavaleta, E. (2012). The functions of biological diversity in an age of extinction. Science 336, 1401–1406. doi: 10.1126/science.1215855
Nagamitsu, T., and Inoue, T. (1997). Cockroach pollination and breeding system of Uvaria elmeri (Annonaceae) in a lowland mixed-dipterocarp forest in Sarawak. Am. J. Bot. 84, 208–213. doi: 10.2307/2446082
Nalepa, C. A. (1984). Colony composition, protozoan transfer and some life history characteristics of the woodroach Cryptocercus punctulatus Scudder (Dictyoptera: Cryptocercidae). Behav. Ecol. Sociobiol. 14, 273–279. doi: 10.1007/BF00299498
Nalepa, C. A., Bignell, D. E., and Bandi, C. (2001). Detritivory, coprophagy, and the evolution of digestive mutualisms in Dictyoptera. Insectes Soc. 48, 194–201. doi: 10.1007/PL00001767
Ndiaye, D., Lepage, M., Sall, C. E., and Brauman, A. (2004). Nitrogen transformations associated with termite biogenic structures in a dry savanna ecosystem. Plant Soil 265, 189–196. doi: 10.1007/s11104-005-0892-9
Ness, J., Mooney, K., and Lach, L. (2010). Ants as Mutualists, Ant Ecology. Oxford: Oxford Press, 97–114. doi: 10.1093/acprof:oso/9780199544639.003.0006
Ni, J., and Tokuda, G. (2013). Lignocellulose-degrading enzymes from termites and their symbiotic microbiota. Biotechnol. Adv. 31, 838–850. doi: 10.1016/j.biotechadv.2013.04.005
Nielsen, U. N., and Ball, B. A. (2015). Impacts of altered precipitation regimes on soil communities and biogeochemistry in arid and semi-arid ecosystems. Glob. Change Biol. 21, 1407–1421. doi: 10.1111/gcb.12789
Odelson, D. A., and Breznak, J. A. (1983). Volatile fatty acid production by the hindgut microbiota of xylophagous termites. Appl Environ Microbiol. 45, 1602–1613. doi: 10.1128/AEM.45.5.1602-1613.1983
Oulhen, N., Schulz, B. J., and Carrier, T. J. (2016). English translation of Heinrich Anton de Bary’s 1878 speech,‘Die Erscheinung der Symbiose’(‘De la symbiose’). Symbiosis 69, 131–139. doi: 10.1007/s13199-016-0409-8
Paoletti, M. G., and Hassall, M. (1999). Woodlice (Isopoda: Oniscidea): their potential for assessing sustainability and use as bioindicators. Agric. Ecosyst. Environ. 74, 157–165. doi: 10.1016/S0167-8809(99)00035-3
Paoletti, M. G., Osler, G. H., Kinnear, A., Black, D. G., Thomson, L. J., Tsitilas, A., et al. (2007). Detritivores as indicators of landscape stress and soil degradation. Aust. J. Exp. Agric. 47, 412–423. doi: 10.1071/EA05297
Papp, D., Simon, E., Nagy, L., Mizser, S., and Tóthmérész, B. (2019). The effect of urbanization on trace element concentration and symmetry of woodlice (Armadillidium vulgare Latreille, 1804). Biol. Trace Elem. Res. 189, 251–258. doi: 10.1007/s12011-018-1454-3
Pearsons, K. A., and Tooker, J. F. (2021). Preventive insecticide use affects arthropod decomposers and decomposition in field crops. Appl. Soil Ecol. 157:103757. doi: 10.1016/j.apsoil.2020.103757
Peirano, A. (2007). In vivo measurements of the seasonal photosynthetic fluorescence of the Mediterranean coral Cladocora caespitosa (L.). Sci. Mar. 71, 629–635. doi: 10.3989/scimar
Pellens, R., D’Haese, C. A., Bellés, X., Piulachs, M. D., Legendre, F., Wheeler, W. C., et al. (2007a). The evolutionary transition from subsocial to eusocial behaviour in Dictyoptera: phylogenetic evidence for modification of the “shift-in-dependent-care” hypothesis with a new subsocial cockroach. Mol. Phylogenet. Evol. 43, 616–626. doi: 10.1016/j.ympev.2006.12.017
Pellens, R., Legendre, F., and Grandcolas, P. (2007b). Phylogenetic analysis of social behavior evolution in [Zetoborinae + Blaberinae + Gyninae + Diplopterinae] cockroaches: an update with the study of endemic radiations from the Atlantic forest, Stud. Neotrop. Fauna Environ 42, 25–31. doi: 10.1080/01650520600879538
Pellens, R., and Garay, I. (1999). Edaphic macroarthropod communities in fast-growing plantations of Eucalyptus grandis Hill ex Maid (Myrtaceae) and Acacia mangium Wild (Leguminosae) in Brazil. Eur. J. Soil Biol. 35, 77–89. doi: 10.1016/S1164-5563(99)00209-5
Pellens, R., Garay, I., and Grandcolas, P. (2011). “Biodiversity conservation and management in the Brazilian atlantic forest: every fragment must be considered,” in Biodiversity Hotspots, eds V. Rescigno and S. Maletta (New York, NY: Nova Publishers, Inc), 1–35.
Pellens, R., and Grandcolas, P. (2007). The conservation-refugium value of small and disturbed Brazilian Atlantic forest fragments for the endemic ovoviviparous cockroach Monastria biguttata (Insecta: Dictyoptera. Blaberidae, Blaberinae). Zool. Sci. 24, 11–19. doi: 10.2108/zsj.24.11
Pellens, R., Grandcolas, P., and Silva-Neto, I. D. D. (2002). A new and independently evolved case of xylophagy and the presence of intestinal flagellates in the cockroach Parasphaeria boleiriana (Dictyoptera, Blaberidae, Zetoborinae) from the remnants of the Brazilian Atlantic forest. Can. J. Zool. 80, 350–359. doi: 10.1139/z01-230
Pievani, T. (2014). The sixth mass extinction: anthropocene and the human impact on biodiversity. Rend. Lincei Sci. Fis. Nat. 25, 85–93. doi: 10.1007/s12210-013-0258-9
Pinder, R. W., Bettez, N. D., Bonan, G. B., Greaver, T. L., Wieder, W. R., Schlesinger, W. H., et al. (2013). Impacts of human alteration of the nitrogen cycle in the US on radiative forcing. Biogeochemistry 114, 25–40. doi: 10.1007/s10533-012-9787-z
Pinto-Tomás, A. A., Anderson, M. A., Suen, G., Stevenson, D. M., Chu, F. S., Cleland, W. W., et al. (2009). Symbiotic nitrogen fixation in the fungus gardens of leaf-cutter ants. Science 326, 1120–1123. doi: 10.1126/science.1173036
Poinar, G. Jr. (2009). Early cretaceous protist flagellates (Parabasalia: Hypermastigia: Oxymonada) of cockroaches (Insecta: Blattaria) in Burmese amber. Cretac. Res. 30, 1066–1072. doi: 10.1016/j.cretres.2009.03.008
Pokhylenko, A. P., Didur, O. O., Kulbachko, Y. L., Bandura, L. P., and Chernykh, S. A. (2020). Influence of saprophages (Isopoda, Diplopoda) on leaf litter decomposition under different levels of humidification and chemical loading. Biosyst. Diver. 28, 384–389. doi: 10.15421/012049
Princis, K., and Beier, M. (1962-1971). “Blattariae,” in Orthopterorum Catalogus, (The Hague: Junk’s-Gravenhage), 1224.
Prins, R. A., and Kreulen, D. A. (1991). Comparative aspects of plant cell wall digestion in insects. Anim. Feed Sci. Technol. 32, 101–118. doi: 10.1016/0377-8401(91)90013-I
Ramanathan, B., and Alagesan, P. (2012). Isolation, characterization and role of gut bacteria of three different millipede species. Indian J. Sci. Res. 3, 55–61.
Raymond, A., Cutlip, P., Sweet, M., Allmon, W. D., and Bottjer, D. J. (2001). “Rates and processes of terrestrial nutrient cycling in the Paleozoic: the world before beetles, termites, and flies,” in Evolutionary Paleoecology: The Ecological Context of Macroevolutionary Change, eds W. D. Allmon and D. J. Bottjer (New York, NY: Columbia University Press), 235–283. doi: 10.7312/allm10994-012
Rockström, J., Steffen, W., Noone, K., Persson, A., Chapin, F. S., Lambin, E. III, et al. (2009). Planetary boundaries: exploring the safe operating space for humanity. Ecol. Soc. 14:32.
Roggenbuck, M., Schnell, I. B., Blom, N., Bælum, J., Bertelsen, M. F., Sicheritz-Pontén, T., et al. (2014). The microbiome of New World vultures. Nat. Commun. 5, 1–8. doi: 10.1038/ncomms6498
Rosenberg, E., Koren, O., Reshef, L., Efrony, R., and Zilber-Rosenberg, I. (2007). The role of microorganisms in coral health, disease and evolution. Nat. Rev. Microbiol. 5, 355–362. doi: 10.1038/nrmicro1635
Rouland-Lefèvre, C., Inoue, T., and Johjima, T. (2006). “Termitomyces/termite interactions,” in Intestinal Microorganisms of Termites and Other Invertebrates, eds H. König and A. Varma (Berlin: Springer), 335–350. doi: 10.1007/3-540-28185-1_14
Sabater, S. (2008). Alterations of the global water cycle and their effects on river structure, function and services. Freshw. Rev. 1, 75–88. doi: 10.1608/FRJ-1.1.5
Sabo, J. L., Soykan, C. U., and Keller, A. (2005). “5.2 - Functional roles of leaf litter detritus in terrestrial food webs,” in Dynamic Food Webs, Multispecies Assemblages, Ecosystem Development and Environmental Change, Theoretical Ecology Series, Vol. 3, eds P. de Ruiter, J. C. Moore, V. Wolters, and K. Melville-Smith (Burlington, MA: Academic Press), 211–222. doi: 10.1016/B978-012088458-2/50021-7
Sabree, Z. L., Kambhampati, S., and Moran, N. A. (2009). Nitrogen recycling and nutritional provisioning by Blattabacterium, the cockroach endosymbiont. Proc. Natl. Acad. Sci. U.S.A. 106, 19521–19526. doi: 10.1073/pnas.0907504106
Sánchez-Bayo, F. (2011). Impacts of Agricultural Pesticides on Terrestrial Ecosystems. Ecological Impacts of Toxic Chemicals. Sharjah: Bentham Science Publishers Ltd, 63–87. doi: 10.2174/978160805121210063
Sánchez-Bayo, F., and Wyckhuys, K. A. (2019). Worldwide decline of the entomofauna: a review of its drivers. Biol. Conserv. 232, 8–27. doi: 10.1016/j.biocon.2019.01.020
Santana, R. H., Catão, E. C. P., Lopes, F. A. C., Constantino, R., Barreto, C. C., and Krüger, R. H. (2015). The gut microbiota of workers of the litter-feeding termite Syntermes wheeleri (Termitidae: Syntermitinae): archaeal, bacterial, and fungal communities. Microb. Ecol. 70, 545–556. doi: 10.1007/s00248-015-0581-z
Sapountzis, P., De Verges, J., Rousk, K., Cilliers, M., Vorster, B. J., and Poulsen, M. (2016). Potential for nitrogen fixation in the fungus-growing termite symbiosis. Front. Microbiol. 7:1993. doi: 10.3389/fmicb.2016.01993
Sapountzis, P., Nash, D. R., Schiøtt, M., and Boomsma, J. J. (2019). The evolution of abdominal microbiomes in fungus-growing ants. Mol. Ecol. 28, 879–899. doi: 10.1111/mec.14931
Sapountzis, P., Zhukova, M., Hansen, L. H., Sørensen, S. J., Schiøtt, M., and Boomsma, J. J. (2015). Acromyrmex leaf-cutting ants have simple gut microbiota with nitrogen-fixing potential. Appl. Environ. Microbiol. 81, 5527–5537. doi: 10.1128/AEM.00961-15
Sapountzis, P., Zhukova, M., Shik, J. Z., Schiott, M., and Boomsma, J. J. (2018). Reconstructing the functions of endosymbiotic Mollicutes in fungus-growing ants. eLife 7:e39209. doi: 10.7554/elife.39209
Sapp, J. (1994). Evolution by Association: A History of Symbiosis. Oxford: Oxford University Press On Demand.
Schultz, T. R., and Brady, S. G. (2008). Major evolutionary transitions in ant agriculture. Proc. Natl. Acad. Sci. U.S.A. 105, 5435–5440. doi: 10.1073/pnas.0711024105
Seeber, J., Rief, A., Seeber, G. U., Meyer, E., and Traugott, M. (2010). Molecular identification of detritivorous soil invertebrates from their faecal pellets. Soil Biol Biochem. 42, 1263–1267. doi: 10.1016/j.soilbio.2010.04.008
Selosse, M. A., Baudoin, E., and Vandenkoornhuyse, P. (2004). Symbiotic microorganisms, a key for ecological success and protection of plants. C. R. Biol. 327, 639–648. doi: 10.1016/j.crvi.2003.12.008
Shear, W. A. (1991). The early development of terrestrial ecosystems. Nature 351, 283–289. doi: 10.1038/351283a0
Shear, W. A., and Kukalová-Peck, J. (1990). The ecology of Paleozoic terrestrial arthropods: the fossil evidence. Can. J. Zool. 68, 1807–1834. doi: 10.1139/z90-262
Shukla, S. P., Plata, C., Reichelt, M., Steiger, S., Heckel, D. G., Kaltenpoth, M., et al. (2018). Microbiome-assisted carrion preservation aids larval development in a burying beetle. Proc. Natl. Acad. Sci. U.S.A. 115, 11274–11279. doi: 10.1073/pnas.1812808115
Sierwald, P., and Bond, J. E. (2007). Current status of the myriapod class Diplopoda (millipedes): taxonomic diversity and phylogeny. Annu. Rev. Entomol. 52, 401–420. doi: 10.1146/annurev.ento.52.111805.090210
Six, D. L. (2013). The bark beetle holobiont: why microbes matter. J. Chem. Ecol. 39, 989–1002. doi: 10.1007/s10886-013-0318-8
Six, D. L., and Paine, T. D. (1999). Phylogenetic comparison of ascomycete mycangial fungi and Dendroctonus bark beetles (Coleoptera: Scolytidae). Ann. Entomol. Soc. Am. 92, 159–166. doi: 10.1093/aesa/92.2.159
Six, D. L., and Wingfield, M. J. (2011). The role of phytopathogenicity in bark beetle–fungus symbioses: a challenge to the classic paradigm. Annu. Rev. Entomol. 56, 255–272. doi: 10.1146/annurev-ento-120709-144839
Skillings, D. (2016). Holobionts and the ecology of organisms: multi-species communities or integrated individuals? Biol Philos. 31, 875–892. doi: 10.1007/s10539-016-9544-0
Snyder, B. A., and Hendrix, P. F. (2008). Current and potential roles of soil macroinvertebrates (earthworms, millipedes, and isopods) in ecological restoration. Restor. Ecol. 16, 629–636. doi: 10.1111/j.1526-100X.2008.00484.x
Solomou, A. D., Sfougaris, A. I., and Sfenthourakis, S. (2019). Terrestrial isopods as bioindicators for environmental monitoring in olive groves and natural ecosystems. J. Nat. Hist. 53, 1721–1735. doi: 10.1080/00222933.2019.1658821
Spatafora, J. W. (2001). “Evolution of ascomycota-arthropoda symbioses,” in Symbiosis, ed. D. H. S. Richardson (Dordrecht: Springer), 589–609. doi: 10.1007/0-306-48173-1_37
Steffen, W., Richardson, K., Rockström, J., Cornell, S. E., Fetzer, I., Bennett, E. M., et al. (2015). Planetary boundaries: guiding human development on a changing planet. Science 347:1259855. doi: 10.1126/science.1259855
Stencel, A., and Wloch-Salamon, D. M. (2018). Some theoretical insights into the hologenome theory of evolution and the role of microbes in speciation. Theory Biosci. 137, 197–206. doi: 10.1007/s12064-018-0268-3
Suárez, J. (2018). The importance of symbiosis in philosophy of biology: an analysis of the current debate on biological individuality and its historical roots. Symbiosis 76, 77–96. doi: 10.1007/s13199-018-0556-1
Suárez, J. (2020). The stability of traits conception of the hologenome: an evolutionary account of holobiont individuality. HPLS 42:11. doi: 10.1007/s40656-020-00305-2
Suárez, J., and Stencel, A. (2020). A part-dependent account of biological individuality: why holobionts are individuals and ecosystems simultaneously. Biol. Rev. 95, 1308–1324. doi: 10.1111/brv.12610
Suárez, J., and Triviño, V. (2020). What is a hologenomic adaptation? Emergent individuality and inter-identity in multispecies systems. Front. Psychol. 11:187. doi: 10.3389/fpsyg.2020.00187
Suetsugu, K. (2019). Social wasps, crickets and cockroaches contribute to pollination of the holoparasitic plant Mitrastemon yamamotoi (Mitrastemonaceae) in southern Japan. Plant Biol. 21, 176–182. doi: 10.1111/plb.12889
Suzuki, Y., Grayston, S. J., and Prescott, C. E. (2013). Effects of leaf litter consumption by millipedes (Harpaphe haydeniana) on subsequent decomposition depends on litter type. Soil Biol. Biochem. 57, 116–123. doi: 10.1016/j.soilbio.2012.07.020
Takasuka, T. E., Book, A. J., Lewin, G. R., Currie, C. R., and Fox, B. G. (2013). Aerobic deconstruction of cellulosic biomass by an insect-associated Streptomyces. Sci. Rep. 3:1030. doi: 10.1038/srep01030
Tanahashi, M., Kubota, K., Matsushita, N., and Togashi, K. (2010). Discovery of mycangia and the associated xylose-fermenting yeasts in stag beetles (Coleoptera: Lucanidae). Naturwissenschaften 97, 311–317. doi: 10.1007/s00114-009-0643-5
Tarli, V. D., Pequeno, P. A., Franklin, E., de Morais, J. W., Souza, J. L., Oliveira, A. H., et al. (2014). Multiple environmental controls on cockroach assemblage structure in a tropical rain forest. Biotropica 46, 598–607. doi: 10.1111/btp.12138
Taylor, E. C. (1982). Role of aerobic microbial populations in cellulose digestion by desert millipedes. Appl. Environ. Microbiol. 44, 281–291. doi: 10.1128/AEM.44.2.281-291.1982
Teuben, A. (1991). Nutrient availability and interactions between soil arthropods and microorganisms during decomposition of coniferous litter: a mesocosm study. Biol. Fertil. Soils 10, 256–266. doi: 10.1007/BF00337376
Theis, K. R., Dheilly, N. M., Klassen, J. L., Brucker, R. M., Baines, J. F., Bosch, T. C., et al. (2016). Getting the hologenome concept right: an eco-evolutionary framework for hosts and their microbiomes. mSystems 1:e00028-16. doi: 10.1128/mSystems.00028-16
Thompson, B. M., Bodart, J., McEwen, C., and Gruner, D. S. (2014). Adaptations for symbiont-mediated external digestion in Sirex noctilio (Hymenoptera: Siricidae). Annal. Entomol. Soc. Am. 107, 453–460. doi: 10.1603/AN13128
Thompson, B. M., Grebenok, R. J., Behmer, S. T., and Gruner, D. S. (2013). Microbial symbionts shape the sterol profile of the xylem-feeding woodwasp, Sirex noctilio. J. Chem. Ecol. 39, 129–139. doi: 10.1007/s10886-012-0222-7
Tokuda, G., Elbourne, L. D., Kinjo, Y., Saitoh, S., Sabree, Z., Hojo, M., et al. (2013). Maintenance of essential amino acid synthesis pathways in the Blattabacterium cuenoti symbiont of a wood-feeding cockroach. Biol. Lett. 9:20121153. doi: 10.1098/rsbl.2012.1153
Tóth, Z., and Hornung, E. (2019). Taxonomic and functional response of millipedes (Diplopoda) to urban soil disturbance in a metropolitan area. Insects 11:25. doi: 10.3390/insects11010025
Tóth, Z., Hornung, E., Báldi, A., and Kovács-Hostyánszki, A. (2016). Effects of set-aside management on soil macrodecomposers in Hungary. Appl. Soil Ecol. 99, 89–97. doi: 10.1016/j.apsoil.2015.11.003
Tripp, E. A., Zhang, N., Schneider, H., Huang, Y., Mueller, G. M., Hu, Z., et al. (2017). Reshaping Darwin’s tree: impact of the symbiome. Trends Ecol. Evol. 32, 552–555. doi: 10.1016/j.tree.2017.05.002
Tuma, J., Eggleton, P., and Fayle, T. M. (2020). Ant-termite interactions: an important but under-explored ecological linkage. Biol. Rev. 95, 555–572. doi: 10.1111/brv.12577
Turner, T. R., James, E. K., and Poole, P. S. (2013). The plant microbiome. Genome Biol. 14, 1–10. doi: 10.1186/gb-2013-14-6-209
Uehara, Y., and Sugiura, N. (2017). Cockroach-mediated seed dispersal in Monotropastrum humile (Ericaceae): a new mutualistic mechanism. Bot. J. Linn. Soc. 185, 113–118. doi: 10.1093/BOTLINNEAN/BOX043
Urbina, H., Schuster, J., and Blackwell, M. (2013). The gut of Guatemalan passalid beetles: a habitat colonized by cellobiose-and xylose-fermenting yeasts. Fungal Ecol. 6, 339–355. doi: 10.1016/j.funeco.2013.06.005
Van Baaren, J., Deleporte, P., and Grandcolas, P. (2002). Cockroaches found in nests of Icteridae birds in French Guiana. Amazoniana 17, 243–248.
van der Loos, L. M., Eriksson, B. K., and Salles, J. F. (2019). The macroalgal holobiont in a changing sea. Trends Microbiol. 27, 635–650. doi: 10.1016/j.tim.2019.03.002
van Gestel, C. A., and Loureiro, S. (2018). Terrestrial isopods as model organisms in soil ecotoxicology: a review. ZooKeys 3, 127–162. doi: 10.3897/zookeys.801.21970
Vera-Ponce de León, A., Ormeño-Orrillo, E., Ramírez-Puebla, S. T., Rosenblueth, M., Degli Esposti, M., Martínez-Romero, J., et al. (2017). Candidatus Dactylopiibacterium carminicum, a nitrogen-fixing symbiont of Dactylopius cochineal insects (Hemiptera: Coccoidea: Dactylopiidae). Genome Biol. Evol. 9, 2237–2250. doi: 10.1093/gbe/evx156
Vesala, R., Arppe, L., and Rikkinen, J. (2019). Caste-specific nutritional differences define carbon and nitrogen fluxes within symbiotic food webs in African termite mounds. Sci. Rep. 9, 1–11. doi: 10.1038/s41598-019-53153-x
Villagra, C., and Frías-Lasserre, D. (2020). Epigenetic molecular mechanisms in insects. Neotrop. Entomol. 49, 615–642. doi: 10.1007/s13744-020-00777-8
Vitousek, P. M., Aber, J. D., Howarth, R. W., Likens, G. E., Matson, P. A., Schindler, D. W., et al. (1997). Human alteration of the global nitrogen cycle: sources and consequences. Ecol. Appl. 7, 737–750.
Vlasáková, B. (2015). Density dependence in flower visitation rates of cockroach-pollinated Clusia blattophila on the Nouragues inselberg. French Guiana. J. Trop. Ecol. 31, 95–98. doi: 10.1017/S0266467414000571
Vos, V. C., van Ruijven, J., Berg, M. P., Peeters, E. T., and Berendse, F. (2011). Macro-detritivore identity drives leaf litter diversity effects. Oikos 120, 1092–1098. doi: 10.1111/j.1600-0706.2010.18650.x
Wagner, D., and Jones, J. B. (2006). The impact of harvester ants on decomposition, N mineralization, litter quality, and the availability of N to plants in the Mojave Desert. Soil Biol. Biochem. 38, 2593–2601. doi: 10.1016/j.soilbio.2006.02.024
Wagner, D. L. (2020). Insect declines in the Anthropocene. Annu. Rev. Entomol. 65, 457–480. doi: 10.1146/annurev-ento-011019-025151
Wang, J., Chadwick, D. R., Cheng, Y., and Yan, X. (2018). Global analysis of agricultural soil denitrification in response to fertilizer nitrogen. Sci. Total Environ. 616, 908–917. doi: 10.1016/j.scitotenv.2017.10.229
Wang, Y., Ren, Y. C., Zhang, Z. T., Ke, T., and Hui, F. L. (2016). Spathaspora Allomyrinae sp. nov., a D-xylose-fermenting yeast species isolated from a scarabeid beetle Allomyrina dichotoma. Int. J. Syst. Evol. Microbiol. 66, 2008–2012.
Ward, P. S. (2007). Phylogeny, classification, and species-level taxonomy of ants (Hymenoptera: Formicidae). Zootaxa 1668, 549–563. doi: 10.11646/zootaxa.1668.1.26
Ward, P. S. (2010). “Taxonomy, phylogenetics, and evolution,” in Ant Ecology, Vol. 1, eds L. Lach, C. Parr, and C. Abbott (Oxford: Oxford Press), doi: 10.1093/acprof:oso/9780199544639.003.0001
Warne, R. W., Kirschman, L., and Zeglin, L. (2019). Manipulation of gut microbiota during critical developmental windows affects host physiological performance and disease susceptibility across ontogeny. J. Anim. Ecol. 88, 845–856. doi: 10.1111/1365-2656.12973
Watanabe, H., and Tokuda, G. (2010). Cellulolytic systems in insects. Annu. Rev. Entomol. 55, 609–632. doi: 10.1146/annurev-ento-112408-085319
Weinstein, P. (1994). Behavioural ecology of tropical cave cockroaches: preliminary field studies with evolutionary implications. Aust. J. Entomol. 33, 367–370. doi: 10.1111/j.1440-6055.1994.tb01249.x
Whitford, W. G., Ludwig, J. A., and Noble, J. C. (1992). The importance of subterranean termites in semi-arid ecosystems in south-eastern Australia. J. Arid Environ. 22, 87–91. doi: 10.1016/S0140-1963(18)30659-1
Xie, X., Anderson, A. B., Wran, L. J., Serrano, M. G., and Buck, G. A. (2017). Characterization of cellulose-degrading microbiota from the eastern subterranean termite and soil. F1000 Res. 6:2082. doi: 10.12688/f1000research.13148.1
Yamada, A., Inoue, T., Wiwatwitaya, D., Ohkuma, M., Kudo, T., Abe, T., et al. (2005). Carbon mineralization by termites in tropical forests, with emphasis on fungus combs. Ecol. Res. 20, 453–460. doi: 10.1007/s11284-005-0062-9
Zeng, Y., Maxwell, S., Runting, R. K., Venter, O., Watson, J. E., and Carrasco, L. R. (2020). Environmental destruction not avoided with the sustainable development goals. Nat. Sustain 3, 795–798. doi: 10.1038/s41893-020-0555-0
Zepeda Mendoza, M. L., Xiong, Z., Escalera-Zamudio, M., Runge, A. K., Thézé, J., Streicker, D., et al. (2018). Hologenomic adaptations underlying the evolution of sanguivory in the common vampire bat. Nat. Ecol. Evol. 2, 659–668. doi: 10.1038/s41559-018-0476-8
Zhang, L., Dong, D., Jiang, C., Li, Z., Wang, X., and Peng, Y. (2015). Insight into alteration of gut microbiota in Clostridium difficile infection and asymptomatic C. difficile colonization. Anaerobe 34, 1–7. doi: 10.1016/j.anaerobe.2015.03.008
Zhang, Q., Zhu, D., Ding, J., Zhou, S., Sun, L., and Qian, H. (2019). Species-specific response of the soil collembolan gut microbiome and resistome to soil oxytetracycline pollution. Sci. Total Environ. 668, 1183–1190. doi: 10.1016/j.scitotenv.2019.03.091
Zhu, D., Chen, Q. L., An, X. L., Yang, X. R., Christie, P., Ke, X., et al. (2018a). Exposure of soil collembolans to microplastics perturbs their gut microbiota and alters their isotopic composition. Soil Biol. Biochem. 116, 302–310. doi: 10.1016/j.soilbio.2017.10.027
Zhu, D., Chen, Q. L., Li, H., Yang, X. R., Christie, P., Ke, X., et al. (2018b). Land use influences antibiotic resistance in the microbiome of soil collembolans Orchesellides sinensis. Environ. Sci. Technol. 52, 14088–14098. doi: 10.1021/acs.est.8b05116
Zilber-Rosenberg, I., and Rosenberg, E. (2008). Role of microorganisms in the evolution of animals and plants: the hologenome theory of evolution. FEMS Microbiol. Rev. 32, 723–735. doi: 10.1111/j.1574-6976.2008.00123.x
Zimmer, M. (2002). Nutrition in terrestrial isopods (Isopoda: Oniscidea): an evolutionary-ecological approach. Biol. Rev. 77, 455–493. doi: 10.1017/S1464793102005912
Zimmer, M., and Topp, W. (1998). Microorganisms and cellulose digestion in the gut of the woodlouse Porcellio scaber. J. Chem. Ecol. 24, 1397–1408. doi: 10.1023/A:1021235001949
Keywords: mutualism, planetary boundaries, ecosystem engineers, ecosystem services, detritivore arthropods
Citation: Schapheer C, Pellens R and Scherson R (2021) Arthropod-Microbiota Integration: Its Importance for Ecosystem Conservation. Front. Microbiol. 12:702763. doi: 10.3389/fmicb.2021.702763
Received: 29 April 2021; Accepted: 02 July 2021;
Published: 02 August 2021.
Edited by:
Chih-Horng Kuo, Institute of Plant and Microbial Biology, Academia Sinica, TaiwanCopyright © 2021 Schapheer, Pellens and Scherson. This is an open-access article distributed under the terms of the Creative Commons Attribution License (CC BY). The use, distribution or reproduction in other forums is permitted, provided the original author(s) and the copyright owner(s) are credited and that the original publication in this journal is cited, in accordance with accepted academic practice. No use, distribution or reproduction is permitted which does not comply with these terms.
*Correspondence: Constanza Schapheer, Y3Auc2NoYXBoZWVyQGdtYWlsLmNvbQ==