- 1Research Division for Radiation Science, Korea Atomic Energy Research Institute, Jeongeup, South Korea
- 2Department of Biotechnology and Bioengineering, Chonnam National University, Gwangju, South Korea
- 3Department of Oral Microbiology and Immunology, School of Dentistry, Dental Research Institute, Seoul National University, Seoul, South Korea
- 4Department of Radiation Biotechnology and Applied Radioisotope Science, University of Science and Technology, Daejeon, South Korea
Deinococcus radiodurans is an extremely resistant bacterium against extracellular stress owing to on its unique physiological functions and the structure of its cellular constituents. Interestingly, it has been reported that the pattern of alteration in Deinococcus proportion on the skin is negatively correlated with skin inflammatory diseases, whereas the proportion of Staphylococcus aureus was increased in patients with chronic skin inflammatory diseases. However, the biological mechanisms of deinococcal interactions with other skin commensal bacteria have not been studied. In this study, we hypothesized that deinococcal cellular constituents play a pivotal role in preventing S. aureus colonization by inhibiting biofilm formation. To prove this, we first isolated cellular constituents, such as exopolysaccharide (DeinoPol), cell wall (DeinoWall), and cell membrane (DeinoMem), from D. radiodurans and investigated their inhibitory effects on S. aureus colonization and biofilm formation in vitro and in vivo. Among them, only DeinoPol exhibited an anti-biofilm effect without affecting bacterial growth and inhibiting staphylococcal colonization and inflammation in a mouse skin infection model. Moreover, the inhibitory effect was impaired in the Δdra0033 strain, a mutant that cannot produce DeinoPol. Remarkably, DeinoPol not only interfered with S. aureus biofilm formation at early and late stages but also disrupted a preexisting biofilm by inhibiting the production of poly-N-acetylglucosamine (PNAG), a key molecule required for S. aureus biofilm formation. Taken together, the present study suggests that DeinoPol is a key molecule in the negative regulation of S. aureus biofilm formation by D. radiodurans. Therefore, DeinoPol could be applied to prevent and/or treat infections or inflammatory diseases associated with S. aureus biofilms.
Introduction
Deinococcus radiodurans is a non-pathogenic bacterium extremely resistant to extracellular stresses, such as ionizing radiation, desiccation, UV radiation, and oxidizing agents (Fredrickson et al., 2008; Slade and Radman, 2011; Farci et al., 2016; Schmier et al., 2017). This exceptional resistance to multiple stresses results from the concerted actions of various physiological functions and well-regulated molecular mechanisms, including efficient DNA repair systems and enzymatic/non-enzymatic antioxidant systems (Makarova et al., 2001; Lim et al., 2019). In particular, D. radiodurans can protect against oxidative damage to important cellular components, such as proteins, nucleic acids, and lipids, via effective redox control and reactive oxygen species (ROS) scavenging (Chen et al., 2020). These features have been used in various industrial applications, such as decontamination of radioactive waste and development of cosmetic ingredients with the antioxidant and anti-aging functions (Xu et al., 2018; Lin et al., 2020).
Deinococcus has been reported as a commensal bacterium in various human tissues. Resident D. radiodurans has been found in healthy human skin (Chng et al., 2016). The Deinococcus-Thermus phylum has been reported in 23 gastric endoscopic biopsy samples and vaginal microbiota of healthy women (Bik et al., 2006; Diop et al., 2019). In addition, the beneficial role of Deinococcus in human skin has been speculated to be due to the quiet immune responses of host cells; however, its exact function has not been clarified (Ott et al., 2019). Interestingly, the proportion of Deinococcus is negatively correlated with skin inflammatory diseases, such as psoriasis or allergic skin inflammation, which may play a role in maintaining healthy skin (Yau et al., 2019; Guo et al., 2020). In contrast, other skin commensal bacteria, such as Staphylococcus aureus, Corynebacterium, and Actinobacteria, were detected at a high proportion in lesions in patients suffering from chronic skin inflammatory diseases, and it has been reported to be implicated in this etiology (Daly, 2009; Leyva-Castillo et al., 2020; Quan et al., 2020). However, the interactive function of D. radiodurans with other commensals and its effect on disease propensity has not been investigated.
Staphylococcus aureus is a Gram-positive opportunistic bacterium that commonly colonizes the skin, nose, and mucosal surfaces of healthy individuals (Schwartz et al., 2012; Truong-Bolduc et al., 2014; Mulcahy and McLoughlin, 2016). Approximately 20–30% of individuals are asymptomatically colonized by S. aureus, and 30% are intermittently colonized (Sakr et al., 2018). S. aureus is a leading cause of various infectious diseases, including pneumonia, sepsis, endocarditis, osteomyelitis, and skin and soft tissue infections (SSTIs). Skin tissue is the most common site for S. aureus colonization and infection, causing SSTI from minor, self-limiting, superficial infections to life-threatening diseases. S. aureus-associated SSTI may progress to invasive diseases, such as sepsis, endocarditis, and osteomyelitis (Olaniyi et al., 2017). S. aureus colonization and biofilm formation have been reported as universal behaviors that are significant risk factors for subsequent skin infections (Kwiecinski, 2015). When S. aureus forms a biofilm on the site of infection, it is 10–1,000 times more tolerant to antibiotics, antimicrobial peptides, and immune cell-mediated phagocytosis than the planktonic stage (Mah, 2012; Vestby et al., 2020).
There have been reports on the regulation of S. aureus biofilms via interactions with other commensal species. The negative regulatory effect of serine protease (ESP) from Staphylococcus epidermidis, a commensal bacterium, against S. aureus colonization has been shown in the nasal cavities of human volunteers (Iwase et al., 2010). We also previously reported that S. aureus biofilms were significantly inhibited by lipoteichoic acid derived from Lactobacillus plantarum, a probiotic commensal in the skin, gut, and oral cavity (Ahn et al., 2018). Understanding the interspecies interaction between commensals is important for understanding the pathology of inflammatory diseases or developing therapeutic strategies against these diseases. We hypothesized that D. radiodurans or its cellular constituents have beneficial effects on the host by playing a preventive role against S. aureus colonization and biofilm formation. Thus, we investigated the effect of D. radiodurans on S. aureus biofilm formation, including the underlying molecular mechanisms, and assessed its therapeutic potential to control S. aureus infection.
Materials and Methods
Bacteria and Reagents
The bacteria used in this study are listed in Supplementary Table 1. D. radiodurans strains were cultured at 30°C in tryptone glucose yeast extract (TGY) broth containing 0.5% tryptone, 0.3% yeast extract, and 0.1% glucose. S. aureus strains were cultured at 37°C in Luria-Bertani (LB) broth. All culture media used in this study were purchased from Difco (Franklin Lakes, NJ, United States). The LIVE/DEAD bacterial viability kit was purchased from Thermo Fisher Scientific (Waltham, MA, United States). The RNeasy Mini Kit was purchased from Qiagen GmbH (Hilden, Germany). The Primescript 1st strand cDNA synthesis kit was purchased from Takara Bio (Kusatsu, Japan). DNase I, RNase A, crystal violet solution, and Gram staining kit were purchased from Sigma-Aldrich (St. Louis, MO, United States). Wheat germ agglutinin conjugated with biotin (WGA-biotin) was purchased from GeneTex (Irvine, CA, United States). Horseradish peroxidase-conjugated streptavidin (streptavidin-HRP) was purchased from BD Biosciences (San Jose, CA, United States).
Purification of DeinoPol
DeinoPols were prepared from D. radiodurans R1, KCTC13953BP, KCTC13954BP, or KCTC13955BP, as previously described (Lin et al., 2020). Briefly, D. radiodurans was cultured in TGY broth at 30°C for 48 h under shaking conditions. After incubation, 0.1% deoxycholate was added to the bacteria to lyse the cell wall, and the bacterial suspension was then heated at 100°C for 10 min to inactivate the enzymes. The supernatant was harvested by centrifugation (10,000 × g, 30min, 4°C), concentrated, and dialyzed using a minimal tangential flow filtration system with 30K Minimate capsules (Pall Life Sciences, Port Washington, NY, United States). The concentrate was precipitated with four volumes of 95% ethanol (Daejungchem, Seoul, Korea) at 4°C for 12 h to yield the crude polysaccharide. To remove proteins, the precipitate was suspended in distilled water and mixed with three volumes of chloroform:n-butanol (4:1v/v) for 20 min, and the aqueous phase was collected, followed by precipitation with 80% ethanol. Finally, it was filtered with a 0.22-μm Millex-GP syringe filter unit (Merck Millipore, Burlington, MA, United States) and lyophilized. In some experiments, DeinoPol was further treated with proteinase K (50 μg/ml) or DNase I (50 μg/ml) at 37°C for 1 h, or heat at 100°C for 10 min.
Isolation of Deinococcus radiodurans Cell Wall Fraction (DeinoWall) and Cell Membrane Fraction (DeinoMem)
DeinoWall was isolated as previously described method (Wallinder and Neujahr, 1971), with some modifications. D. radiodurans was suspended in 1 M NaCl and disrupted using an ultrasonicator (Sanyo, Osaka, Japan), followed by removal of undisrupted cells or heavy cell debris from the bacterial lysates by centrifugation at 1,000 × g for 15 min. Next, the cytosolic proteins or light cell debris were removed by centrifugation at 18,800 × g for 15 min. The pellet containing DeinoWall was resuspended in 0.5% SDS in PBS and incubated at 60°C for 30 min to remove the cell membranous fraction. After centrifugation at 18,800 × g for 15 min, the pellets were resuspended in 1 M Tris-HCl and treated with 10 μg/ml DNase I and 50 μg/ml RNase A at 37°C for 2 h. After centrifugation at 18,800 × g for 15 min, the pellet was resuspended in 1 M Tris-HCl and incubated with 10 mM CaCl2 in the presence or absence of 200 μg/ml trypsin at 37°C for 18 h. After lyophilization, the quantity was determined by measuring the dry weight of DeinoWall and suspended in pyrogen-free water. To isolate DeinoMem, D. radiodurans was suspended in 0.1 M sodium citrate buffer (pH 4.7) and disrupted using an ultrasonicator. Bacterial lysates were vigorously mixed with an equal volume of n-butanol at RT for 30 min, and the aqueous phase was separated by centrifugation at 13,000 × g for 15 min. Butanol extraction was repeated three times. The aqueous phase was dialyzed against pyrogen-free water, followed by lyophilization and dissolution with pyrogen-free water.
Preparation of Culture Supernatant (DRsup) and Heat-Killed Bacteria (HKDR) of Deinococcus radiodurans
To prepare DRsup, D. radiodurans was cultured in TGY broth at 30°C for 16 h under shaking condition, followed by dilution to prepare OD600 of 1 corresponds to 108 CFU/ml. The culture was centrifuged at 10,410 × g for 10 min at 4°C. The culture supernatants were filtered through a 0.2 μm membrane filter to remove the remaining bacteria and debris, and then stored at −80°C. To prepare HKDR, the culture of D. radiodurans was centrifuged at 10,410 × g for 10 min at 4°C. After removal of the culture supernatant, the bacteria were washed and resuspended in PBS to a density of 109 CFU/ml. The bacteria were heat-killed at 70°C for 30 min, and then stored in aliquots at −80°C. Complete killing was examined by plating on an TGY-agar plate overnight.
Biofilm Assay With Crystal Violet Staining
S. aureus (5 × 107 CFU/ml) was grown in 96-well plates (SPL, Pocheon, Korea) at 37°C for 24 h in LB broth in the presence or absence of DeinoPol. In some experiments, to investigate the biofilm prevention effect of DeinoPol, DeinoPol suspended in PBS was pre-incubated onto 96-well plates for 12 h at RT. S. aureus (5 × 107 CFU/ml) was then grown in 96-well plates pre-coated with DeinoPol at 37°C for 24 h in LB broth. After incubation, planktonic bacteria were removed, and the biofilm was gently washed twice with PBS. The biofilms of S. aureus were stained with 0.1% crystal violet solution at RT for 30 min, followed by washing with PBS to remove non-specific stain. The adhered dye was dissolved in a solution (95% ethanol and 0.1% acetic acid), and absorbance was measured at 600 nm using a microplate reader (Biotec, Winooski, VT, United States).
Confocal Laser Scanning Microscopy
S. aureus (5 × 107 CFU/ml) was grown on sterile glass coverslips at 37°C for 24 h in LB broth in the presence or absence of DeinoPol. Planktonic bacteria were removed, and the biofilm was gently washed twice with PBS, followed by staining of the bacterial biofilm with SYTO9 and propidium iodide using the LIVE/DEAD bacterial viability kit according to the manufacturer’s instructions. After washing with PBS to remove non-specific stain, the biofilm was visualized using an LSM800 confocal laser scanning microscope (Zeiss, Jena, Germany). For quantification of the confocal microscopy data, 10 random independent fields of view per each group were selected and the mean fluorescence intensity (MFI) was analyzed using the ImageJ software.
Quantitative Real-Time Reverse Transcription Chain Reaction
S. aureus (1 × 108 CFU/ml) was grown on cell culture dishes (100 × 20 mm) for 12 h in LB broth in the presence or absence of DeinoPol (50 μg/ml). Bacteria were harvested, and total RNA was prepared using the RNeasy mini kit. Complementary DNA (cDNA) was synthesized from 5 μg of total RNA using the Primescript 1st strand cDNA synthesis kit. qRT-PCR was performed using SYBR Premix EX Taq (Takara Bio) in a real-time PCR system (Bio-Rad Laboratories, Hercules, CA, United States). The expression levels of these genes were normalized to the expression of gyrB. All primers were synthesized by Bionics (Seoul, Korea). The primer sequences are listed in Supplementary Table 2.
Poly-N-Acetylglucosamine Detection
A crude PNAG extract was prepared as described previously (Toledo-Arana et al., 2005). Briefly, S. aureus (1 × 108 CFU/ml) was grown in 1.7-ml microtubes at 37°C in LB broth in the presence or absence of DeinoPol, DeinoWall, or DeinoMem for 12 h. S. aureus was harvested by centrifugation at 10,000 × g for 5 min and washed five times with PBS. The pellets were then resuspended in 0.5 M EDTA (pH 8.0) and incubated at 100°C for 5 min. The supernatant was harvested by centrifugation at 10,000 × g for 10 min and treated with proteinase K (20 mg/ml) at 37°C for 30 min. The crude PNAG extracts were spotted onto a nitrocellulose membrane, and the blot was blocked with 5% skim milk in Tris-buffered saline with 0.1% Tween 20 (TBST) for 1 h. The membrane was then incubated overnight with 10 μg/ml WGA-biotin. After washing three times with TBST, PNAG was detected with streptavidin-HRP, followed by chemiluminescence detection using the ChemiDoc Touch Imaging System (Bio-Rad). The densities of PNAG were quantified using densitometry analysis in the ImageJ software.
Biofilm Formation on HaCaT Cells Monolayer
HaCaT cells (5 × 104 cells/well) were plated in a 96-well plate and grown until they reached confluence. S. aureus (5 × 105CFU/well) was added to the cell monolayer in the presence or absence of 30 μg/ml of DeinoPol. The cells were then incubated at 37°C for 8 h in a humidified 5% CO2 incubator to form biofilms. After incubation, the biofilm was washed twice with PBS, and the cells were lysed with 0.1% Triton X-100. The number of the bacterial colonies was then counted.
Staphylococcal Wound Infection Model
The animal experiments were approved by the Institutional Animal Care and Use Committee of the Korea Atomic Energy Research Institute (KAERI, IACUC-2019-03) and performed according to accepted veterinary standards by the KAERI Animal Care Center. Seven-week-old female BALB/c mice were obtained from Orient Bio (Seongnam, Korea). After 1 week of acclimatization, the mice were anesthetized by intraperitoneal administration of avertin (250 mg/kg), and a flat head of tack preheated in boiling water for 30 min was applied to the shaved dorsal of then mice for 30 s. S. aureus (1 × 107 CFU) was then applied locally to the site of the burn wound in the presence or absence of DeinoPol. At 48 h post-infection, the mouse skin tissues were collected and subjected to Gram staining or homogenization for counting the bacterial CFU.
Macrophage-Biofilm Interaction Assay
The experiment was performed as previously described (Ahn et al., 2018). Briefly, S. aureus (5 × 107 CFU/ml) was incubated in LB broth on sterile glass coverslips at 37°C for 24 h in the presence or absence of DeinoPol. The biofilm was washed with PBS to remove LB broth and the planktonic bacteria. RAW264.7 murine macrophage cell line in FBS (fetal bovine serum) free-DMEM medium was added at 1 × 106 cells/ml to the preformed biofilm and further incubated at 37°C for 2 h in a humidified incubator with 5% CO2, followed by washing with PBS to remove the left suspension bacteria and RAW 264.7 cells. Then, the remaining biofilm was detached, suspended in PBS, and plated onto LB agar plates. After 24 h of incubation, the number of bacterial colonies was determined.
Statistical Analysis
The mean value ± standard deviation (S.D.) was obtained from triplicate samples for each treatment group. Statistical significance was determined by one-way ANOVA and Tukey post-test. Asterisks indicate significant induction compared with the control group (*P < 0.05, **P < 0.01, and ***P < 0.001).
Results
Deinococcus radiodurans Inhibits Staphylococcus aureus Biofilm Formation
To determine whether D. radiodurans can inhibit staphylococcal biofilm formation, we compared the biofilm formation of S. aureus in the presence of the indicated concentrations of deinococcal culture supernatant (DRsup) or heat-killed D. radiodurans R1 (HKDR). As shown in Figures 1A,B, DRsup potently inhibited biofilm formation by S. aureus in a dose-dependent manner and HKDR significantly inhibited biofilm formation at 108 CFU/ml. To confirm, whether Deinococcus sp. inhibits staphylococcal biofilm formation, we tested three additional deinococcal strains, namely D. radiodurans KCTC13953BP, KCTC13954BP, and KCTC13955BP. All DRsup showed significant inhibitory effect on the biofilm production of S. aureus (Figure 1C). Next, we examined whether the anti-biofilm effect of D. radiodurans was due to its bactericidal effect on S. aureus. S. aureus was treated with DRsup or HKDR, and its growth and survival were measured. As shown in Figures 1D,E, neither DRsup nor HKDR altered S. aureus growth and viability. These data indicated that the inhibitory effect of D. radiodurans on staphylococcal biofilm formation was not due to a direct bactericidal effect, but may be caused by an indirect effect.
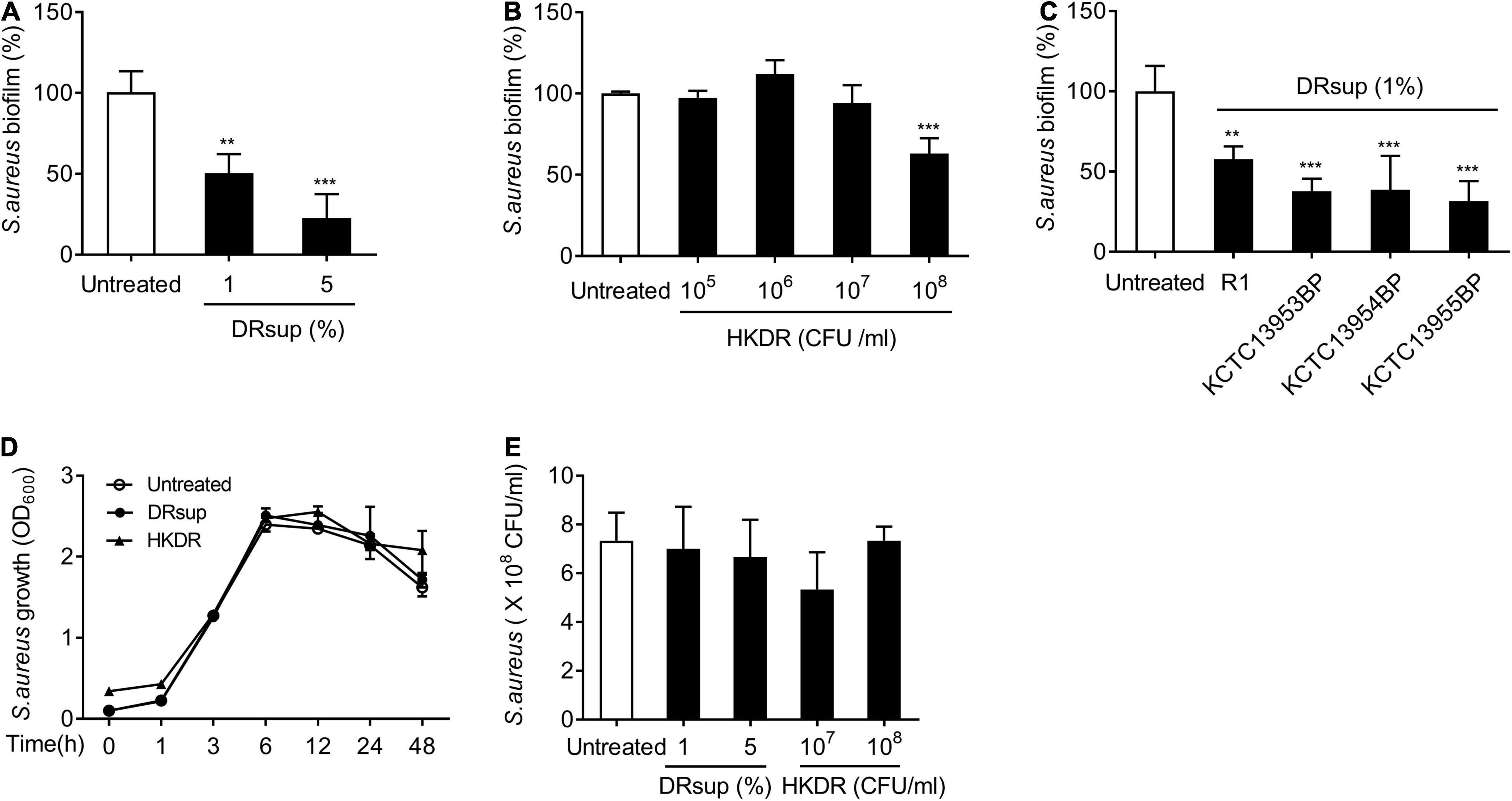
Figure 1. D. radiodurans inhibits S. aureus biofilm formation. (A–C) S. aureus (5 × 107 CFU/ml) was grown on polystyrene plates at 37°C for 24 h in the presence or absence of (A) culture supernatants (DRsup) (1 or 5%) or (B) Heat-killed cells (HKDR) (105, 106, 107, or 108 CFU/ml) of D. radiodurans R1, or (C) DRsup of D. radiodurans KCTC13953BP, KCTC13954BP, or KCTC13955BP strains. Biofilms were determined by crystal violet assay. (D) S. aureus (1 × 107 CFU/ml) was grown for 1, 3, 6, 12, 24, or 48 h in the presence or absence of HKDR (108CFU/ml) or DRsup (5%) under shaking culture conditions. The growth of S. aureus was determined by optical density (O.D.) at 600 nm. (E) S. aureus (5 × 107 CFU/ml) was grown at 37°C for 24 in the presence or absence of HKDR (107 or 108/ml) or DRsup (1 or 5%) under static culture conditions. Bacterial viability was measured by counting CFU. Data are the mean values ± S.D. of triplicate samples. Significant differences are indicated by asterisks (**P < 0.01 and ***P < 0.001).
DeinoPol Inhibits Staphylococcus aureus Biofilm Formation
Next, to identify the deinococcal component responsible for the inhibition of staphylococcal biofilm formation, major cell wall components were isolated, and their effects on biofilm formation were investigated. Three major representative cell wall components, namely exopolysaccharide (DeinoPol), cell wall (DeinoWall), and cell membrane (DeinoMem), were isolated and examined for their effects on S. aureus biofilm formation. DeinoPol markedly inhibited staphylococcal biofilm formation, whereas DeinoWall and DeinoMem did not (Figure 2A). As mentioned above, we found no direct bactericidal effect of DeinoPol on S. aureus (Figures 2C,D). To investigate whether DeinoPol is a major component of the cell wall fraction that is responsible for the inhibition of biofilm formation, we compared the biofilm inhibitory effect of WT and DeinoPol knockout mutants (Δdra0033) (Lin et al., 2020). As shown in Figure 2B, the inhibitory effect of the WT was significantly weakened in Δdra0033. Confocal microscopy analysis also showed that S. aureus biofilm formation was inhibited by DeinoPol in a dose-dependent manner; however, dead cells were rarely detected (Figure 2E). We previously demonstrated that DeinoPol is composed of 89.9% polysaccharides, 8.8% proteins and 1.3% DNA (Lin et al., 2020). To confirm polysaccharides are the major components of DeinoPol inhibiting biofilm formation, S. aureus biofilm formation was examined in the presence of proteinase K-treated DeinoPol, DNase I-treated DeinoPol, or heat-treated DeinoPol. As shown in Figure 2F, proteinase K, DNase I, and heat treatment did not significantly alter the inhibitory effects of DeinoPol on biofilm formation of S. aureus, indicating that the polysaccharides, the main component of DeinoPol, are predominantly responsible for the anti-biofilm effect of DeinoPol against S. aureus. Skin tissue is the most common site for S. aureus colonization and infection. To investigate whether DeinoPol interferes with staphylococcal colonization of skin cells, we used HaCaT cells, immortalized human keratinocytes, in a monolayer where S. aureus was grown to form biofilms in the presence or absence of DeinoPol. After 8 h of incubation, the biofilm bacteria were enumerated. As shown in Figure 2G, DeinoPol treatment considerably decreased biofilm bacterial burdens on HaCaT cell monolayers compared with the non-treatment group. Next, to examine whether the anti-biofilm effect of DeinoPol is a common characteristic of all D. radiodurans, DeinoPol was purified from various D. radiodurans strains, such as D. radiodurans KCTC13953BP, KCTC13954BP, and KCTC13955BP, and its effect on S. aureus biofilms was investigated. As expected, all tested DeinoPol significantly inhibited S. aureus biofilm formation at levels similar to those of DeinoPol isolated from the R1 strain (Figure 2H). Biofilm formation is considered an important mechanism in the pathogenesis of methicillin-resistant S. aureus (MRSA). Biofilms confer drug tolerance to broad-spectrum antibiotics, which contributes to the emergence of antibiotic-resistant bacteria, such as MRSA (Mirani and Jamil, 2011; Piechota et al., 2018). To confirm whether DeinoPol also inhibits the biofilm formation of MRSA, MRSA strains such as USA300, MW2, and Mu50 were treated with DeinoPol, and biofilm formation was examined. Figures 2I–K show that all tested MRSA strains showed significantly lower levels of biofilm formation after treatment with DeinoPol, indicating that DeinoPol might be a broad-spectrum inhibitor for various S. aureus strains including MRSA.
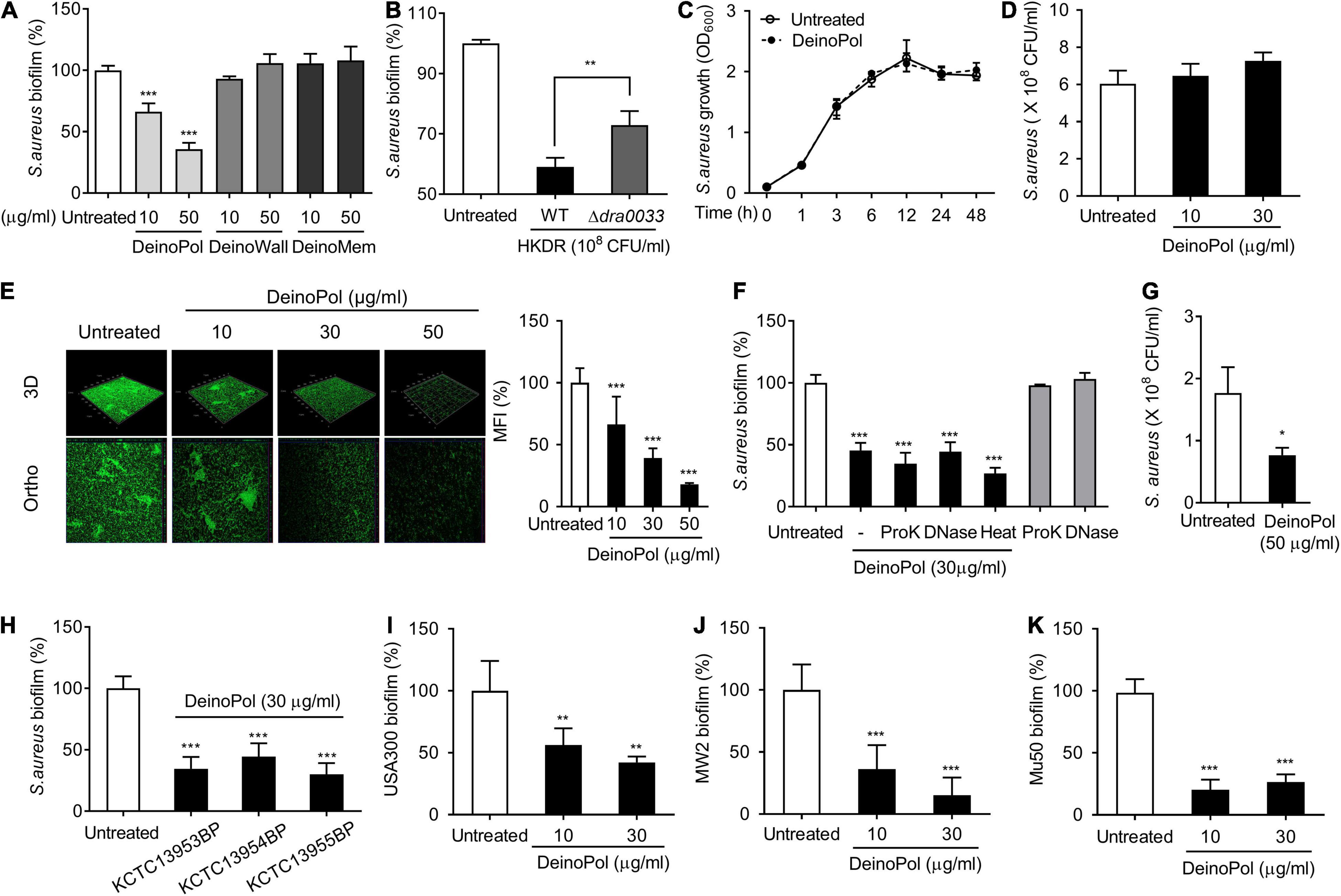
Figure 2. DeinoPol inhibits biofilm formation of S. aureus. (A,B) S. aureus (5 × 107 CFU/ml) was grown on polystyrene plates at 37°C for 24 h in the presence or absence of (A) DeinoPol (10 and 50 μg/ml), DeinoWall (10 and 50 μg/ml), or DeinoMem (10 and 50 μg/ml) isolated from D. radiodurans R1, or (B) HKDR (1 × 108 CFU/ml) of WT or Δdra0033 (DeinoPol-deficient strain) strains. Biofilms were determined by crystal violet assay. (C) S. aureus (1 × 107 CFU/ml) was grown for 1, 3, 6, 12, 24, or 48 h in the presence or absence of DeinoPol (30 μg/ml) under shaking conditions. The growth of S. aureus was determined by O.D. at 600 nm. (D) S. aureus (5 × 107 CFU/ml) was grown at 37°C for 24 h in the presence or absence of DeinoPol (10 or 30 μg/ml) under static culture condition. Bacterial viability was measured by counting CFU. Data are the mean values ± S.D. of triplicate samples. (E) S. aureus (5 × 107 CFU/ml) was grown on coverslips at 37°C for 24 h in the presence or absence of DeinoPol (10, 30 or 50 μg/ml) and DeinoPol-treated S. aureus biofilms were visualized by confocal laser scanning microscopy (green from SYTO9 and red from propidium iodide). One of three similar results is shown. In the right panel, the MFI values of each group were quantified by ImageJ software. (F) S. aureus (5 × 107 CFU/ml) was grown on polystyrene plates at 37°C for 24 h in the presence or absence of DeinoPol (30 μg/ml), 50 μg/ml of proteinase K (ProK)-treated DeinoPol (30 μg/ml), 50 μg/ml of DNase I (DNase)-treated DeinoPol (30 μg/ml), or heat-treated DeinoPol (30 μg/ml). Biofilms were determined by crystal violet assay. Data are the mean values ± S.D. of triplicate samples. (G) HaCaT cells monolayer was exposed to S. aureus (MOI = 10) at 37°C for 8 h in the presence or absence of 30 μg/ml DeinoPol. After removing the supernatant containing the planktonic bacteria, biofilms or bacteria adherent onto the cell monolayer were measured by counting CFU. (H) S. aureus (5 × 107 CFU/ml) was grown on polystyrene plates at 37°C for 24 h in the presence or absence of DeinoPol (30 μg/ml) purified from D. radiodurans KCTC13953BP, KCTC13954BP, or KCTC13955BP strains. (I) S. aureus USA300, (J) MV2, or (K) Mu50 (5 × 107 CFU/ml) were grown on polystyrene plates at 37°C for 24 h in the presence or absence of DeinoPol (10 or 30 μg/ml). Biofilms were determined by crystal violet assay. Data are the mean values ± S.D. of triplicate samples. Significant differences are indicated by asterisks (*P < 0.05, **P < 0.01, and ***P < 0.001).
DeinoPol Interferes With Biofilm Formation in the Early and Late Phases and Collapses Preexisting Biofilm
To determine the phase of biofilm development at which the inhibitory effect of DeinoPol occurs, S. aureus biofilms formed for 1, 3, 6, 12, 24, or 48 h in the presence or absence of DeinoPol were measured. The inhibitory effect of DeinoPol was observed from 3 h after biofilm formation and lasted up to 48 h (Figure 3A). Next, we determined whether DeinoPol has preventive or destructive effects on biofilms. First, the plates were pre-coated with DeinoPol at various concentrations, and their effects on the biofilm-forming ability of S. aureus were investigated. Figure 3B shows that biofilm formation was hampered by DeinoPol at concentrations from 8 to 200 μg/well in a dose-dependent manner, but was not inhibited by DeinoPol at 16 μg/ml. To examine the destructive effect of DeinoPol against S. aureus biofilms, preexisting biofilms for 24 h were treated with DeinoPol for 6 h, and the biofilm was measured. S. aureus biofilm was significantly destroyed by DeinoPol at 30 and 50 μg/ml, but not at 10 μg/ml (Figure 3C). These results suggest that DeinoPol can inhibit S. aureus biofilm formation at the early and late stages with prophylactic and destructive effects.
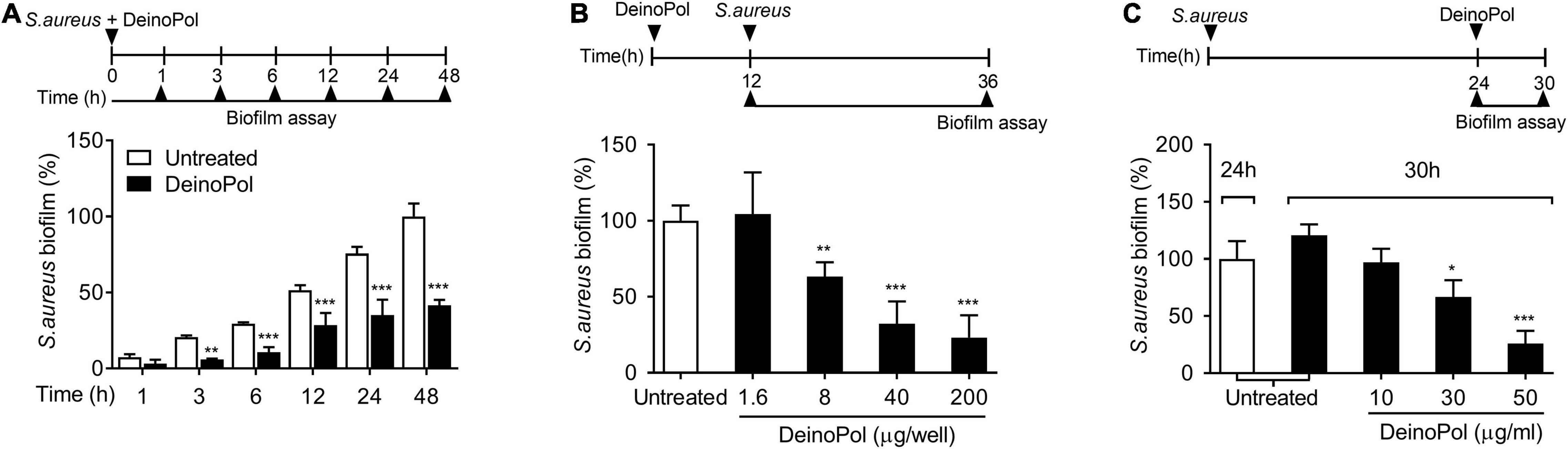
Figure 3. DeinoPol interferes with biofilm formation in the early and late phases and collapses preexisting biofilm. (A) S. aureus (5 × 107 CFU/ml) was grown on polystyrene plates at 37°C for 1, 3, 6, 12, 24, or 48 h in the presence of DeinoPol (30 μg/ml). (B) S. aureus (5 × 107 CFU/ml) was grown at 37°C for 24 h on polystyrene plates pre-coated with DeinoPol (1.6, 8, 40, or 200 μg/well) for 12 h. (C) Preexisting biofilm established with S. aureus (5 × 107 CFU/ml) at 37°C for 24 h on polystyrene plates was treated with DeinoPol (10, 30, or 50 μg/ml) and further incubated for 6 h. Biofilms were determined by crystal violet assay. Data are the mean values ± S.D. of triplicate samples. Significant differences are indicated by asterisks (*P < 0.05, **P < 0.01, and ***P < 0.001).
DeinoPol Regulates ica Gene Expression and Poly-N-Acetylglucosamine Production in Staphylococcus aureus
The intracellular adhesion (ica) locus is responsible for the production of poly-N-acetylglucosamine (PNAG), an essential component for staphylococcal biofilm formation (O’Gara, 2007). In addition, microbial surface components recognizing adhesive matrix molecules (MSCRAMMs) genes, such as fib (fibrinogen binding protein), cna (collagen binding protein), fnbA (fibronectin binding protein A), fnbB (fibronectin binding protein B), clfA (clumping factor A), clfB (clumping factor B), ebps (elastin-binding protein), and eno (laminin-binding protein), have been reported to be involved in the attachment of staphylococci. To identify the underlying mechanisms for biofilm inhibition by DeinoPol, the regulatory effect of DeinoPol on the expression of these staphylococcal biofilm-associated genes was examined. As shown in Figure 4A, when S. aureus was incubated with DeinoPol (50 μg/ml), the mRNA expression of icaABCD was significantly downregulated. Moreover, the mRNA expression of fib, cna, fnbA, fnbB, clfA, and clfB was suppressed by DeinoPol treatment, but ebps and eno expression was not affected (Figure 4B). Next, the production of PNAG was measured in S. aureus treated with DeinoPol, DeinoWall, or DeinoMem. Figures 4C–E show that PNAG production was inhibited by DeinoPol in a dose-dependent manner, whereas DeinoWall and DeinoMem did not affect to PNAG. These results suggest that DenoPol inhibits ica gene expression and PNAG production, contributing to the inhibition of S. aureus biofilm formation.
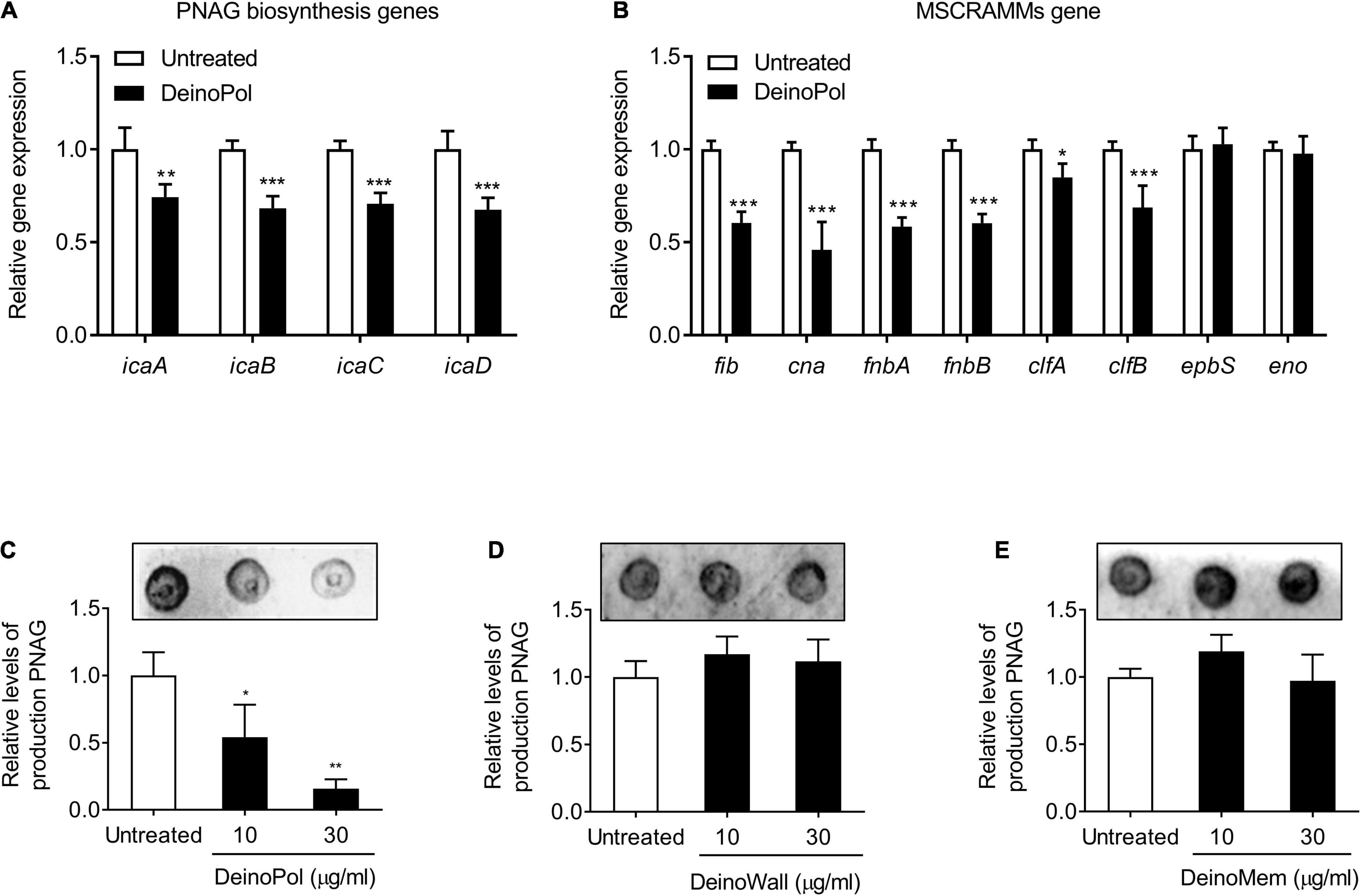
Figure 4. DeinoPol regulates ica gene expression and poly-N-acetylglucosamine production in S. aureus. (A,B) S. aureus (1 × 108 CFU/ml) was treated with DeinoPol (50 μg/ml) for 12 h. Total RNA was isolated, and the mRNA expression levels of (A) ica genes (icaA, icaB, icaC, and icaD) and (B) microbial surface components recognizing adhesive matrix molecules (MSCRAMMs) genes (fib, cna, fnbA, fnbB, clfA, clfB, ebpS, and eno) were examined by qRT-PCR analysis. The expression of gyrB was used for q-PCR normalization. Data are the mean values ± S.D. of triplicate samples. (C–E) S. aureus (1 × 108 CFU/ml) was treated with DeinoPol (10 or 30 μg/ml), DeinoWall (10 or 30 μg/ml), or DeinoMem (10 or 30 μg/ml) for 12 h. The crude extract of PNAG from S. aureus was blotted on nitrocellulose membrane and detected by WGA-biotin/HRP-streptavidin, followed by visualization with chemiluminescence detection. One of three similar results is shown. In the lower panel, the densities of PNAG were quantified by densitometry analysis. Data are the mean values ± S.D. of triplicate samples. Significant differences are indicated by asterisks (*P < 0.05, **P < 0.01, and ***P < 0.001).
DeinoPol Inhibits Staphylococcus aureus Burdens in Skin Wound Infection Model
The inhibition of S. aureus biofilm formation by DeinoPol in vivo was evaluated in a mouse model of burn wound biofilm. S. aureus was treated to the mouse dorsal burn wound in the presence or absence of DeinoPol for 48 h to allow biofilm formation and the amount of biofilm bacteria was calculated by counting colony-forming units. Figure 5A shows that the average bioburden on the mouse wound skin surface was 5.5 × 108 CFU/g tissue in the untreated group. However, this average of bioburden was decreased to 9.6 × 107 CFU/g and 7.6 × 107 CFU/g by treatment with 10 and 50 μg DeinoPol, respectively. Gram staining analysis of the skin tissue also showed that a dense bacterial community (deep violet) was observed below the epidermis of the skin in the S. aureus infection group, and it was substantially reduced in the DeinoPol treatment group (Figure 5B). These results suggest that DeinoPol exerts anti-biofilm effects in vivo, implying its potential use as a clinical treatment for infectious diseases associated with S. aureus biofilms.
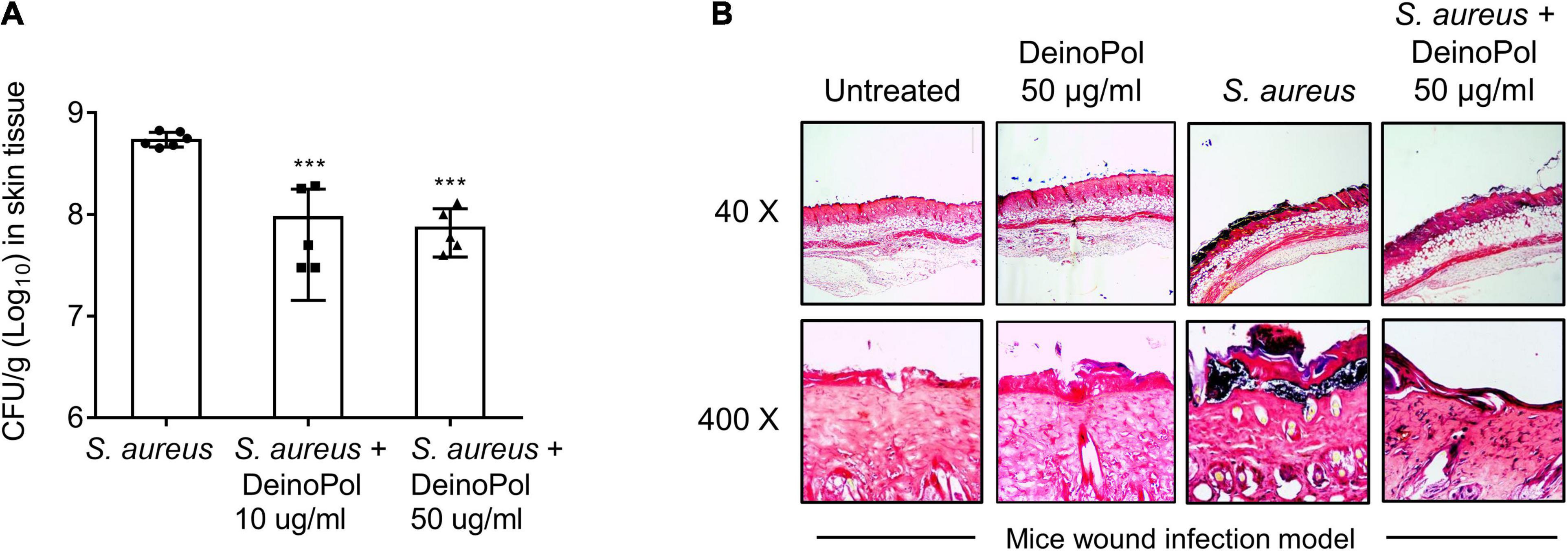
Figure 5. DeinoPol inhibits S. aureus burdens in skin wound infection model. (A,B) S. aureus (1 × 107CFU) was inoculated to the dorsal burn wound of mice in the presence or absence of DeinoPol (10 or 50 μg/wound) for 48 h to allow biofilm formation. (A) S. aureus infected-skin tissues were homogenized by using a 2-mm homogenizer bead, and bioburden was calculated by counting CFU. Six and five mice were used in the non-treatment and DeinoPol-treatment groups, respectively. Asterisks indicate significant induction compared with the non-treatment group (***P < 0.001). (B) The tissue sections were subjected to Gram-staining and imaged at 40 × or 400 × magnification. One of five similar results is shown.
DeinoPol Enhances the Antibacterial Susceptibility of Staphylococcus aureus in Biofilms
Biofilm resistance to antibiotics has been considered a public health concern owing to the improper use and overuse of antibiotics (Sharma et al., 2019). To examine if DeinoPol increases the inhibitory effect of antibiotics on S. aureus biofilm formation, S. aureus biofilm was treated with various antibiotics clinically used for S. aureus infection, such as penicillin, vancomycin, oxacillin, and cefazolin, in the presence or absence of DeinoPol, and then the biofilm was measured. As shown in Figures 6A–D, DeinoPol enhanced the capacity of all tested antibiotics to inhibit S. aureus biofilms. Although macrophages are major innate immune defenders against microbial infection, S. aureus biofilms have been reported to prevent macrophage phagocytosis and avoid immune responses (Thurlow et al., 2011). The effect of DeinoPol on macrophage function to eradicate S. aureus biofilms was examined. Pre-incubated S. aureus biofilm with DeinoPol was treated with RAW264.7 cells, and the degree of biofilm reduction and the cell viability were measured. Figure 6E shows that DeinoPol enhanced the removal effect of macrophages against S. aureus biofilm (left panel). The macrophage viability was not altered (right panel). These results indicate that DeinoPol can improve the susceptibility of S. aureus biofilms to antibiotics and macrophages.
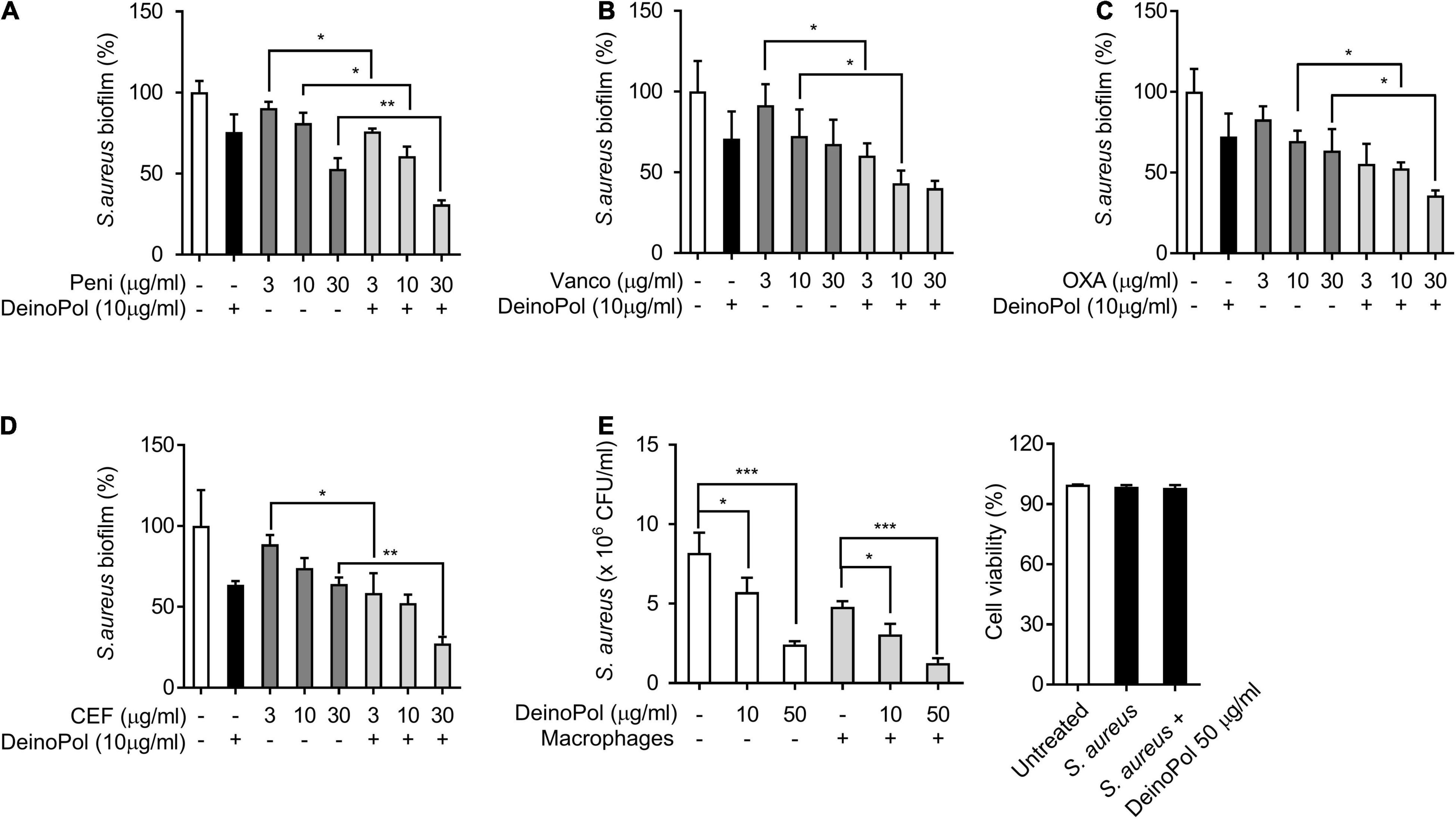
Figure 6. DeinoPol enhances the antibacterial susceptibility of S. aureus in biofilm. (A–D) S. aureus (5 × 107CFU/ml) was grown on polystyrene plates with DeinoPol (10 μg/ml) at 37°C for 24 h, and further incubated for 6 h in the presence or absence of (A) penicillin (3, 10, or 30 μg/ml), (B) vancomycin (3, 10, or 30 μg/ml), (C) oxacillin (3, 10, or 30 μg/ml), or (D) cefazolin (3, 10, or 30 μg/ml). Biofilms were determined by crystal violet assay. (E) S. aureus (5 × 107CFU/ml) was grown on coverslips at 37°C for 24 h with DeinoPol (10 or 50 μg/ml) and RAW 264.7 cells (1 × 106 cells/ml) were added to the preformed biofilm and further incubated at 37°C for 2 h. The remaining biofilms were measured by counting colony forming units (left panel) and the cell viability was assessed by flow cytometry after staining with propidium iodide (right panel). Data are the mean values ± S.D. of triplicate samples. Significant differences are indicated by asterisks (*P < 0.05, **P < 0.01, and ***P < 0.001).
Discussion
D. radiodurans, an extremophile bacterium, is a normal inhabitant flora of the human body, but its interactive role in the defense against pathogen infection has not been studied. In this study, we revealed that D. radiodurans inhibited S. aureus biofilm formation. Specifically, DeinoPol acted as a major component of D. radiodurans, inhibiting biofilm formation without affecting bacterial growth by abrogating the expression of the ica genes required for PNAG production. Furthermore, DeinoPol enhanced the susceptibility of biofilms to antibiotics and macrophages. These results indicate that D. radiodurans functions as a commensal that prevents S. aureus infection, and provide a compelling evidence that DeinoPol can be a potential antimicrobial agent for controlling S. aureus biofilm formation.
DeinoPol, not DeinoWall and DeinoMem, was identified as a functional constituent of D. radiodurans to inhibit biofilm formation by S. aureus in this study. Moreover, this inhibitory function was not confined to DeinoPol of the type strain, but was also possessed by the DeinoPol of D. radiodurans KCTC13953BP, KCTC13954BP, and KCTC13955BP strains isolated from Baekrokdam, Jeju, Republic of Korea. These results are consistent with previous reports showing the inhibitory function of extracellular galactan from Kingella kingae against biofilm formation by S. aureus, S. epidermidis, Candida albicans, and Aggregatibacter actinomycetemcomitans (Bendaoud et al., 2011), and inhibition of enterohemorrhagic Escherichia coli biofilm formation by the EPS released by Lactobacillus acidophilus (Kim et al., 2009). In contrast, EPS composes up to 40% of the dry weight of dental plaque, and glucan is essential for biofilm formation by Streptococcus mutans (Koo et al., 2010). An EPS-deficient strain of Bacillus subtilis, ΔepsH, formed smooth colonies and fragile pellicles compared with the WT strain (Epstein et al., 2011). This contradictory effect of EPS on bacterial biofilm formation is due to the diverse structures and compositions of each bacterial species (Joseph and Wright, 2004; Jiang et al., 2011). We previously showed that DeinoPol is composed of xylose, galactose, fucose, glucose, arabinose, and fructose in a molar ratio of 10.6:6.1:4.2:3.8:2.6:1.0, and 14.89% of unknown sugars (Lin et al., 2020). This oligosaccharide composition is similar to that of Lactobacillus plantarum WLPL04 EPS, which consists of xylose, glucose, and galactose at a molar ratio of 3.4:1.8:1 and exerts an anti-biofilm activity against Pseudomonas aeruginosa CMCC10104, Escherichia coli O157:H7, Salmonella typhimurium ATCC13311, and S. aureus CMCC26003 (Liu et al., 2017). In particular, DeinoPol contains fucose, which is considered a rare sugar contained in bacterial EPS and has been reported to have an inhibitory function on the biofilm of formation several bacteria (Khodse and Bhosle, 2010). L-Fucose inhibited the biofilm formation of Campylobacter jejuni NCTC11168, but not that of the fucose permease mutant strain (Dwivedi et al., 2016). L-Fucose and trithiotriazine-cored L-fucose cluster inhibited biofilm formation by P. aeruginosa through binding with LecB, a lectin of P. aeruginosa, and this effect was also observed in P. aeruginosa-infected cystic fibrosis patients (Hauber et al., 2008; Smadhi et al., 2014; Grishin et al., 2015). These previous reports support that the unique oligosaccharide composition and fucose content may play a crucial role in the anti-biofilm activities of DeinoPol.
Here, we showed that DeinoPol inhibited the biofilm formation of S. aureus in the early and late phases, and even destroyed preformed biofilms. This implies that the inhibition of biofilm formation by DeinoPol might be elicited by several distinct mechanisms. Carbohydrate, the major component of EPS, confers an anionic property to the bacterial EPS, which has been reported to interfere with the prerequisite for biofilm formation, such as cell-surface and cell-cell interactions, by electrostatic modifications (Valle et al., 2006; Jiang et al., 2011). The ability of DeinoPol to destroy the preformed biofilms is coincident with previous resports, such as dispersion of P. aeruginosa biofilm by A101 polysaccharide purified from Vibrio sp. QY101 (Jiang et al., 2011) and disruption of preformed biofilm of S. aureus and S. epidermidies by Kingella kingae exopolysaccharide (Bendaoud et al., 2011). However, the precise mechanisms by which the preformed biofilms are destroyed by bacterial polysaccharide are still unknown. It has been reported that bacterial polysaccharides act as a signaling molecule that can regulate gene expression of bacteria (Kim et al., 2009), which encourages speculation that DeinoPol induces the production of self-produced factors of S. aureus mediating biofilm disassembly, such as phenol soluble modulins (PSMs) or extracellular proteases (Boles and Horswill, 2008; Otto, 2013), via regulating gene expression in S. aureus. Further studies are required to elucidate the exact mechanisms of the potential receptor for DeinoPol and its signaling pathway modulating the expression of molecules known to degrade PNAG and S. aureus biofilm.
S. aureus in biofilms is 10–1,000 times more resistant to antibiotics and phagocytosis by macrophages and neutrophils than the planktonic bacteria, and this is associated with chronic inflammation (Mah, 2012). Hence, there have been recent attempts to use additives to enhance the susceptibility of bacterial biofilms to antibiotics or immune cells (Verma et al., 2009; Ferrer-Espada et al., 2020). As we found in this study, DeinoPol increased the anti-biofilm effect of antibiotics and macrophages. It is hypothesized that the biofilm matrix polymer is loosened by DeinoPol by regulating PNAG production, which eases the penetration of antibiotics. Inhibition of PNAG production by DeinoPol might be a key mechanism for enhancing the susceptibility of S. aureus biofilms to antibiotics, as PNAG has been reported to act as a physical barrier for S. aureus biofilms, conferring resistance to antibiotics and the immune system (Brooks and Jefferson, 2014). Molecules that can dissemble bacterial biofilms by degrading or regulating EPS synthesis are referred to as biofilm-dispersing agents, and they can be applied to various bacterial biofilms detected in the environment or clinics (Verderosa et al., 2019). For example, chitosan, D-amino acids, phenol-soluble modulins, and dispersin B are dispersing agents that enhance the susceptibility of biofilms to antibiotics by inducing the release of planktonic cells from biofilms (Alksne and Projan, 2000; Izano et al., 2007; Mu et al., 2014; Sanchez et al., 2014). This study confirms that DeinoPol is a potential biofilm-dispersing agent against S. aureus.
DeinoPol regulated the biofilm formation of S. aureus laboratory strains as well as MRSA isolates, suggesting that DeinoPol could be widely used to inhibit the biofilm formation of various S. aureus strains. Most natural biofilms are composed of multispecies bacteria (Yang et al., 2011). In particular, S. aureus has been reported to form a multispecies biofilm with P. aeruginosa, Salmonella spp., Enterococcus faecalis, Cutibacterium acnes, C. albicans, and S. epidermidis in various tissues (Citron et al., 2007; Pathak et al., 2012; Gannesen et al., 2018; Iniguez-Moreno et al., 2018; Trizna et al., 2020). Multispecies biofilms strengthen their protective effect against hostile environments via cell-cell communication by quorum sensing or diffusible signals, genetic exchanges, or physical interaction (Li and Tian, 2012; Rendueles and Ghigo, 2012; Hansen et al., 2017; Krzyzek and Gosciniak, 2018). Therefore, it is important to clarify the anti-biofilm effect of candidate molecules against single-species biofilms as well as multispecies biofilms. The regulatory effect of DeinoPol against multispecies biofilms containing S. aureus was not determined in this study, but should be investigated in future studies to advance the application of DeinoPol as a clinical treatment for S. aureus infectious diseases.
Although Δdra0033 strain, DeinoPol-deficient strain, was treated to S. aureus biofilm, approximately 30% S. aureus biofilm was reduced. This implies that unknown substances other than DeinoPol may be slightly involved in the residual effect of Δdra0033 strain on biofilm inhibition. Various substances derived from microorganism have been reported to inhibit S. aureus biofilm formation. For example, autoinducer-2 (AI-2), a quorum-sensing signaling molecule, is known to inhibit biofilm of S. aureus by down-regulating the transcription of icaA (Yu et al., 2012). AI-2 has also been reported as a major quorum-sensing molecule to regulate physiological functions in D. radiodurans (Lin et al., 2016), which may have acted as a substance responsible for residual inhibitory effect of Δdra0033 strain. D. radiodurans highly expresses carotenoids that play an important role in the antioxidant effects of the strain through resistance to ROS. Carotenoids have been reported to exhibit the anti-biofilm functions against various bacterial strains. Carotenoid zeaxanthin inhibited virulence gene expression and biofilm formation of P. aeruginosa via quorum sensing inhibition (Gokalsin et al., 2017). Biofilm formation of Gram-positive strains, such as S. aureus, Bacillus subtilis, and Listeria monocytogenes, were also inhibited by carotenoids isolated from skull and exterior covering body parts of prawn (Jeyachandran et al., 2020). These reports lead us to speculate that Δdra0033 strain-derived carotenoids were involved in its residual effects of biofilm inhibition. Besides, we previously demonstrated that single mutant Δdra0033 strain produced 79.8% less DeinoPol than that of WT strain. However, it still expressed DeinoPol in a detectable amount, which may function, at least in part, as an inhibitor against S. aureus biofilm. To date, there have been few studies on the anti-biofilm substance of D. radiodurans, so it can only be speculated. Further studies are needed to determine the existence and mechanisms of D. radiodurans-produced biofilm regulating molecules other than DeinoPol.
Live beneficial bacteria, such as probiotics, generally used as foods or dietary supplements rather than drugs due to its risk and safety issues (Venugopalan et al., 2010). However, various bacterial products, such as exopolysaccharides, have been approved for medical applications in human since the mid-nineteenth century (Moscovici, 2015). For example, dextran or hyaluronic acid/hyaluronan produced by bacteria are now applicated in chronic wound healing, osteoarthritis treatment, or plasma volume expansion for controlling wounds shock (Necas et al., 2008; Nwodo et al., 2012). DeinoPol contents and structure have already been described in our previous report (Lin et al., 2020) and its anti-biofilm efficacy in vitro and in vivo was also demonstrated in this study. Thereafter, if clinical trials demonstrate acceptable effects of DeinoPol, it could be used clinically as a standalone antimicrobial agent or an additive to antibiotics to treat biofilm-associated infections.
Taken together, the results of this study suggest that DeinoPol is a major constituent of D. radiodurans that inhibits S. aureus biofilm formation. DeinoPol can be used as a potential alternative or additive dispersing agent for the treatment of infectious diseases caused by S. aureus biofilms.
Data Availability Statement
The original contributions presented in the study are included in the article/Supplementary Material, further inquiries can be directed to the corresponding author/s.
Ethics Statement
The animal study was reviewed and approved by the Institutional Animal Care and Use Committee of Korea Atomic Energy Research Institute.
Author Contributions
KA and HS conceived the idea and contributed to the discussion of the results followed by writing and reviewing the manuscript. KA, FC, SH, and HS designed the experiments. FC, KA, JZ, and HJ performed the experiments, and/or interpreted the data. M-KK, KK, J-IC, SH, and SL provided critical comments and contributed to the discussion of the results followed by writing and reviewing the manuscript. All authors contributed to the article and approved the submitted version.
Funding
This study was supported by the grant from internal R&D program of KAERI (523210-21) funded by the Ministry of Science and ICT (MSIT) and the National Research Foundation of Korea grants NRF-2020M2D8A3094054 and NRF-2019M2D3A2060217.
Conflict of Interest
The authors declare that the research was conducted in the absence of any commercial or financial relationships that could be construed as a potential conflict of interest.
Publisher’s Note
All claims expressed in this article are solely those of the authors and do not necessarily represent those of their affiliated organizations, or those of the publisher, the editors and the reviewers. Any product that may be evaluated in this article, or claim that may be made by its manufacturer, is not guaranteed or endorsed by the publisher.
Supplementary Material
The Supplementary Material for this article can be found online at: https://www.frontiersin.org/articles/10.3389/fmicb.2021.712086/full#supplementary-material
References
Ahn, K. B., Baik, J. E., Yun, C. H., and Han, S. H. (2018). Lipoteichoic acid inhibits Staphylococcus aureus biofilm formation. Front. Microbiol. 9:327. doi: 10.3389/fmicb.2018.00327
Alksne, L. E., and Projan, S. J. (2000). Bacterial virulence as a target for antimicrobial chemotherapy. Curr. Opin. Biotechnol. 11, 625–636. doi: 10.1016/s0958-1669(00)00155-5
Bendaoud, M., Vinogradov, E., Balashova, N. V., Kadouri, D. E., Kachlany, S. C., and Kaplan, J. B. (2011). Broad-spectrum biofilm inhibition by Kingella kingae exopolysaccharide. J. Bacteriol. 193, 3879–3886. doi: 10.1128/JB.00311-11
Bik, E. M., Eckburg, P. B., Gill, S. R., Nelson, K. E., Purdom, E. A., Francois, F., et al. (2006). Molecular analysis of the bacterial microbiota in the human stomach. Proc. Natl. Acad. Sci. U.S.A. 103, 732–737. doi: 10.1073/pnas.0506655103
Boles, B. R., and Horswill, A. R. (2008). Agr-mediated dispersal of Staphylococcus aureus biofilms. PLoS Pathog. 4:e1000052. doi: 10.1371/journal.ppat.1000052
Brooks, J. L., and Jefferson, K. K. (2014). Phase variation of poly-N-acetylglucosamine expression in Staphylococcus aureus. PLoS Pathog. 10:e1004292. doi: 10.1371/journal.ppat.1004292
Chen, L., Li, J., Zhu, W., Kuang, Y., Liu, T., Zhang, W., et al. (2020). Skin and gut microbiome in psoriasis: gaining insight into the pathophysiology of it and finding novel therapeutic strategies. Front. Microbiol. 11:589726. doi: 10.3389/fmicb.2020.589726
Chng, K. R., Tay, A. S., Li, C., Ng, A. H., Wang, J., Suri, B. K., et al. (2016). Whole metagenome profiling reveals skin microbiome-dependent susceptibility to atopic dermatitis flare. Nat. Microbiol. 1:16106. doi: 10.1038/nmicrobiol.2016.106
Citron, D. M., Goldstein, E. J., Merriam, C. V., Lipsky, B. A., and Abramson, M. A. (2007). Bacteriology of moderate-to-severe diabetic foot infections and in vitro activity of antimicrobial agents. J. Clin. Microbiol. 45, 2819–2828. doi: 10.1128/JCM.00551-07
Daly, M. J. (2009). A new perspective on radiation resistance based on Deinococcus radiodurans. Nat. Rev. Microbiol. 7, 237–245. doi: 10.1038/nrmicro2073
Diop, K., Dufour, J. C., Levasseur, A., and Fenollar, F. (2019). Exhaustive repertoire of human vaginal microbiota. Hum. Microb. J. 11:100051. doi: 10.1016/j.humic.2018.11.002
Dwivedi, R., Nothaft, H., Garber, J., Xin Kin, L., Stahl, M., Flint, A., et al. (2016). L-fucose influences chemotaxis and biofilm formation in Campylobacter jejuni. Mol. Microbiol. 101, 575–589. doi: 10.1111/mmi.13409
Epstein, A. K., Pokroy, B., Seminara, A., and Aizenberg, J. (2011). Bacterial biofilm shows persistent resistance to liquid wetting and gas penetration. Proc. Natl. Acad. Sci. U.S.A. 108, 995–1000. doi: 10.1073/pnas.1011033108
Farci, D., Slavov, C., Tramontano, E., and Piano, D. (2016). The S-layer Protein DR_2577 binds deinoxanthin and under desiccation conditions protects against UV-radiation in Deinococcus radiodurans. Front. Microbiol. 7:155. doi: 10.3389/fmicb.2016.00155
Ferrer-Espada, R., Sanchez-Gomez, S., Pitts, B., Stewart, P. S., and Martinez-de-Tejada, G. (2020). Permeability enhancers sensitize beta-lactamase-expressing Enterobacteriaceae and Pseudomonas aeruginosa to beta-lactamase inhibitors, thereby restoring their beta-lactam susceptibility. Int. J. Antimicrob. Agents 56:105986. doi: 10.1016/j.ijantimicag.2020.105986
Fredrickson, J. K., Li, S. M., Gaidamakova, E. K., Matrosova, V. Y., Zhai, M., Sulloway, H. M., et al. (2008). Protein oxidation: key to bacterial desiccation resistance? ISME J. 2, 393–403. doi: 10.1038/ismej.2007.116
Gannesen, A. V., Lesouhaitier, O., Racine, P. J., Barreau, M., Netrusov, A. I., Plakunov, V. K., et al. (2018). Regulation of monospecies and mixed biofilms formation of skin Staphylococcus aureus and cutibacterium acnes by human natriuretic peptides. Front. Microbiol. 9:2912. doi: 10.3389/fmicb.2018.02912
Gokalsin, B., Aksoydan, B., Erman, B., and Sesal, N. C. (2017). Reducing virulence and biofilm of Pseudomonas aeruginosa by potential quorum sensing inhibitor Carotenoid: Zeaxanthin. Microb. Ecol. 74, 466–473. doi: 10.1007/s00248-017-0949-3
Grishin, A. V., Krivozubov, M. S., Karyagina, A. S., and Gintsburg, A. L. (2015). Pseudomonas Aeruginosa Lectins as targets for novel antibacterials. Acta Nat. 7, 29–41. doi: 10.32607/20758251-2015-7-2-29-41
Guo, J., Zhang, X., Saiganesh, A., Peacock, C., Chen, S., Dykes, G. A., et al. (2020). Linking the westernised oropharyngeal microbiome to the immune response in Chinese immigrants. Allerg. Asthma Clin. Immunol. 16:67. doi: 10.1186/s13223-020-00465-7
Hansen, L. B., Ren, D., Burmolle, M., and Sorensen, S. J. (2017). Distinct gene expression profile of Xanthomonas retroflexus engaged in synergistic multispecies biofilm formation. ISME J. 11, 300–303. doi: 10.1038/ismej.2016.107
Hauber, H. P., Schulz, M., Pforte, A., Mack, D., Zabel, P., and Schumacher, U. (2008). Inhalation with fucose and galactose for treatment of Pseudomonas aeruginosa in cystic fibrosis patients. Int. J. Med. Sci. 5, 371–376. doi: 10.7150/ijms.5.371
Iniguez-Moreno, M., Gutierrez-Lomeli, M., Guerrero-Medina, P. J., and Avila-Novoa, M. G. (2018). Biofilm formation by Staphylococcus aureus and Salmonella spp. under mono and dual-species conditions and their sensitivity to cetrimonium bromide, peracetic acid and sodium hypochlorite. Braz. J. Microbiol. 49, 310–319. doi: 10.1016/j.bjm.2017.08.002
Iwase, T., Uehara, Y., Shinji, H., Tajima, A., Seo, H., Takada, K., et al. (2010). Staphylococcus epidermidis Esp inhibits Staphylococcus aureus biofilm formation and nasal colonization. Nature 465, 346–349. doi: 10.1038/nature09074
Izano, E. A., Sadovskaya, I., Vinogradov, E., Mulks, M. H., Velliyagounder, K., Ragunath, C., et al. (2007). Poly-N-acetylglucosamine mediates biofilm formation and antibiotic resistance in Actinobacillus pleuropneumoniae. Microb. Pathog. 43, 1–9. doi: 10.1016/j.micpath.2007.02.004
Jeyachandran, S., Kiyun, P., Ihn-Sil, K., and Baskaralingam, V. (2020). Identification and characterization of bioactive pigment carotenoids from shrimps and their biofilm inhibition. J. Food Process. Preserv. 44:e14728. doi: 10.1111/jfpp.14728
Jiang, P., Li, J., Han, F., Duan, G., Lu, X., Gu, Y., et al. (2011). Antibiofilm activity of an exopolysaccharide from marine bacterium Vibrio sp, QY101. PLoS One 6:e18514. doi: 10.1371/journal.pone.0018514
Joseph, L. A., and Wright, A. C. (2004). Expression of Vibrio vulnificus capsular polysaccharide inhibits biofilm formation. J. Bacteriol. 186, 889–893. doi: 10.1128/jb.186.3.889-893.2004
Khodse, V. B., and Bhosle, N. B. (2010). Differences in carbohydrate profiles in batch culture grown planktonic and biofilm cells of Amphora rostrata Wm. Sm. Biofouling 26, 527–537. doi: 10.1080/08927014.2010.492468
Kim, Y., Oh, S., and Kim, S. H. (2009). Released exopolysaccharide (r-EPS) produced from probiotic bacteria reduce biofilm formation of enterohemorrhagic Escherichia coli O157:H7. Biochem. Biophys. Res. Commun. 379, 324–329. doi: 10.1016/j.bbrc.2008.12.053
Koo, H., Xiao, J., Klein, M. I., and Jeon, J. G. (2010). Exopolysaccharides produced by Streptococcus mutans glucosyltransferases modulate the establishment of microcolonies within multispecies biofilms. J. Bacteriol. 192, 3024–3032. doi: 10.1128/JB.01649-09
Krzyzek, P., and Gosciniak, G. (2018). A proposed role for diffusible signal factors in the biofilm formation and morphological transformation of Helicobacter pylori. Turk. J. Gastroenterol. 29, 7–13. doi: 10.5152/tjg.2017.17349
Kwiecinski, J. (2015). Biofilm formation by pathogenic Prototheca algae. Lett. Appl. Microbiol. 61, 511–517. doi: 10.1111/lam.12497
Leyva-Castillo, J. M., McGurk, A., and Geha, M. D. R. (2020). Allergic skin inflammation and S. aureus skin colonization are mutually reinforcing. Clin. Immunol. 218:108511. doi: 10.1016/j.clim.2020.108511
Li, Y. H., and Tian, X. (2012). Quorum sensing and bacterial social interactions in biofilms. Sensors (Basel) 12, 2519–2538. doi: 10.3390/s120302519
Lim, S., Jung, J. H., Blanchard, L., and de Groot, A. (2019). Conservation and diversity of radiation and oxidative stress resistance mechanisms in Deinococcus species. FEMS Microbiol. Rev. 43, 19–52. doi: 10.1093/femsre/fuy037
Lin, L., Li, T., Dai, S., Yu, J., Chen, X., Wang, L., et al. (2016). Autoinducer-2 signaling is involved in regulation of stress-related genes of Deinococcus radiodurans. Arch. Microbiol. 198, 43–51. doi: 10.1007/s00203-015-1163-7
Lin, S. M., Baek, C. Y., Jung, J. H., Kim, W. S., Song, H. Y., Lee, J. H., et al. (2020). Antioxidant activities of an exopolysaccharide (DeinoPol) produced by the extreme radiation-resistant bacterium Deinococcus radiodurans. Sci. Rep. 10:55. doi: 10.1038/s41598-019-56141-3
Liu, Z., Zhang, Z., Qiu, L., Zhang, F., Xu, X., Wei, H., et al. (2017). Characterization and bioactivities of the exopolysaccharide from a probiotic strain of Lactobacillus plantarum WLPL04. J. Dairy Sci. 100, 6895–6905. doi: 10.3168/jds.2016-11944
Mah, T. F. (2012). Biofilm-specific antibiotic resistance. Future Microbiol. 7, 1061–1072. doi: 10.2217/fmb.12.76
Makarova, K. S., Aravind, L., Wolf, Y. I., Tatusov, R. L., Minton, K. W., Koonin, E. V., et al. (2001). Genome of the extremely radiation-resistant bacterium Deinococcus radiodurans viewed from the perspective of comparative genomics. Microbiol. Mol. Biol. Rev. 65, 44–79. doi: 10.1128/MMBR.65.1.44-79.2001
Mirani, Z. A., and Jamil, N. (2011). Effect of sub-lethal doses of vancomycin and oxacillin on biofilm formation by vancomycin intermediate resistant Staphylococcus aureus. J. Basic Microbiol. 51, 191–195. doi: 10.1002/jobm.201000221
Moscovici, M. (2015). Present and future medical applications of microbial exopolysaccharides. Front. Microbiol. 6:1012. doi: 10.3389/fmicb.2015.01012
Mu, H., Guo, F., Niu, H., Liu, Q., Wang, S., and Duan, J. (2014). Chitosan improves anti-biofilm efficacy of gentamicin through facilitating antibiotic penetration. Int. J. Mol. Sci. 15, 22296–22308. doi: 10.3390/ijms151222296
Mulcahy, M. E., and McLoughlin, R. M. (2016). Host-Bacterial crosstalk determines Staphylococcus aureus nasal colonization. Trends Microbiol. 24, 872–886. doi: 10.1016/j.tim.2016.06.012
Necas, J., Bartosikova, L., Brauner, P., and Kolar, J. (2008). Hyaluronic acid (hyaluronan): a review. Vet. Med. 53, 397–411. doi: 10.17221/1930-vetmed
Nwodo, U. U., Green, E., and Okoh, A. I. (2012). Bacterial exopolysaccharides: functionality and prospects. Int. J. Mol. Sci. 13, 14002–14015. doi: 10.3390/ijms131114002
O’Gara, J. P. (2007). ica and beyond: biofilm mechanisms and regulation in Staphylococcus epidermidis and Staphylococcus aureus. FEMS Microbiol. Lett. 270, 179–188. doi: 10.1111/j.1574-6968.2007.00688.x
Olaniyi, R., Pozzi, C., Grimaldi, L., and Bagnoli, F. (2017). Staphylococcus aureus-associated skin and soft tissue infections: anatomical localization, epidemiology, therapy and potential prophylaxis. Curr. Top. Microbiol. Immunol. 409, 199–227. doi: 10.1007/82_2016_32
Ott, E., Fuchs, F. M., Moeller, R., Hemmersbach, R., Kawaguchi, Y., Yamagishi, A., et al. (2019). Molecular response of Deinococcus radiodurans to simulated microgravity explored by proteometabolomic approach. Sci. Rep. 9:18462. doi: 10.1038/s41598-019-54742-6
Otto, M. (2013). Staphylococcal infections: mechanisms of biofilm maturation and detachment as critical determinants of pathogenicity. Annu. Rev. Med. 64, 175–188. doi: 10.1146/annurev-med-042711-140023
Pathak, A. K., Sharma, S., and Shrivastva, P. (2012). Multi-species biofilm of Candida albicans and non-Candida albicans Candida species on acrylic substrate. J. Appl. Oral Sci. 20, 70–75. doi: 10.1590/s1678-77572012000100013
Piechota, M., Kot, B., Frankowska-Maciejewska, A., Gruzewska, A., and Wozniak-Kosek, A. (2018). Biofilm formation by methicillin-resistant and methicillin-sensitive Staphylococcus aureus strains from hospitalized patients in Poland. Biomed. Res. Int. 2018:4657396. doi: 10.1155/2018/4657396
Quan, C., Chen, X. Y., Li, X., Xue, F., Chen, L. H., Liu, N., et al. (2020). Psoriatic lesions are characterized by higher bacterial load and imbalance between Cutibacterium and Corynebacterium. J. Am. Acad. Dermatol. 82, 955–961. doi: 10.1016/j.jaad.2019.06.024
Rendueles, O., and Ghigo, J. M. (2012). Multi-species biofilms: how to avoid unfriendly neighbors. J. FEMS Microbiol. Rev. 36, 972–989. doi: 10.1111/j.1574-6976.2012.00328.x
Sakr, A., Bregeon, F., Mege, J. L., Rolain, J. M., and Blin, O. (2018). Staphylococcus aureus nasal colonization: an update on mechanisms, epidemiology, risk factors, and subsequent infections. Front. Microbiol. 9:2419. doi: 10.3389/fmicb.2018.02419
Sanchez, C. J. Jr., Akers, K. S., Romano, D. R., Woodbury, R. L., Hardy, S. K., Murray, C. K., et al. (2014). D-amino acids enhance the activity of antimicrobials against biofilms of clinical wound isolates of Staphylococcus aureus and Pseudomonas aeruginosa. Antimicrob. Agents Chemother. 58, 4353–4361. doi: 10.1128/AAC.02468-14
Schmier, B. J., Chen, X., Wolin, S., and Shuman, S. (2017). Deletion of the rnl gene encoding a nick-sealing RNA ligase sensitizes Deinococcus radiodurans to ionizing radiation. Nucleic Acids Res. 45, 3812–3821. doi: 10.1093/nar/gkx038
Schwartz, K., Syed, A. K., Stephenson, R. E., Rickard, A. H., and Boles, B. R. (2012). Functional amyloids composed of phenol soluble modulins stabilize Staphylococcus aureus biofilms. PLoS Pathog. 8:e1002744. doi: 10.1371/journal.ppat.1002744
Sharma, D., Misba, L., and Khan, A. U. (2019). Antibiotics versus biofilm: an emerging battleground in microbial communities. Antimicrob. Resist. Infect. Control 8:76. doi: 10.1186/s13756-019-0533-3
Slade, D., and Radman, M. (2011). Oxidative stress resistance in Deinococcus radiodurans. Microbiol. Mol. Biol. Rev. 75, 133–191. doi: 10.1128/MMBR.00015-10
Smadhi, M., de Bentzmann, S., Imberty, A., Gingras, M., Abderrahim, R., and Goekjian, P. G. (2014). Expeditive synthesis of trithiotriazine-cored glycoclusters and inhibition of Pseudomonas aeruginosa biofilm formation. Beilstein J. Org. Chem. 10, 1981–1990. doi: 10.3762/bjoc.10.206
Thurlow, L. R., Hanke, M. L., Fritz, T., Angle, A., Aldrich, A., Williams, S. H., et al. (2011). Staphylococcus aureus biofilms prevent macrophage phagocytosis and attenuate inflammation in vivo. J. Immunol. 186, 6585–6596. doi: 10.4049/jimmunol.1002794
Toledo-Arana, A., Merino, N., Vergara-Irigaray, M., Debarbouille, M., Penades, J. R., and Lasa, I. (2005). Staphylococcus aureus develops an alternative, ica-independent biofilm in the absence of the arlRS two-component system. J. Bacteriol. 187, 5318–5329. doi: 10.1128/JB.187.15.5318-5329.2005
Trizna, E. Y., Yarullina, M. N., Baidamshina, D. R., Mironova, A. V., Akhatova, F. S., Rozhina, E. V., et al. (2020). Bidirectional alterations in antibiotics susceptibility in Staphylococcus aureus-Pseudomonas aeruginosa dual-species biofilm. Sci. Rep. 10:14849. doi: 10.1038/s41598-020-71834-w
Truong-Bolduc, Q., Villet, R., Estabrooks, Z., and Hooper, D. C. (2014). Native efflux pumps contribute resistance to antimicrobials of skin and the ability of Staphylococcus aureus to colonize skin. J. Infect. Dis. 209, 1485–1493. doi: 10.1093/infdis/jit660
Valle, J., Da Re, S., Henry, N., Fontaine, T., Balestrino, D., Latour-Lambert, P., et al. (2006). Broad-spectrum biofilm inhibition by a secreted bacterial polysaccharide. Proc. Natl. Acad. Sci. U.S.A. 103, 12558–12563. doi: 10.1073/pnas.0605399103
Venugopalan, V., Shriner, K. A., and Wong-Beringer, A. (2010). Regulatory oversight and safety of probiotic use. Emerg. Infect. Dis. 16, 1661–1665. doi: 10.3201/eid1611.100574
Verderosa, A. D., Totsika, M., and Fairfull-Smith, K. E. (2019). Bacterial biofilm eradication agents: a current review. Front. Chem. 7:824. doi: 10.3389/fchem.2019.00824
Verma, V., Harjai, K., and Chhibber, S. (2009). Restricting ciprofloxacin-induced resistant variant formation in biofilm of Klebsiella pneumoniae B5055 by complementary bacteriophage treatment. J. Antimicrob. Chemother. 64, 1212–1218. doi: 10.1093/jac/dkp360
Vestby, L. K., Gronseth, T., Simm, R., and Nesse, L. L. (2020). Bacterial Biofilm and its role in the pathogenesis of disease. Antibiotics (Basel) 9:59. doi: 10.3390/antibiotics9020059
Wallinder, I. B., and Neujahr, H. Y. (1971). Cell wall and peptidoglycan from Lactobacillus fermenti. J. Bacteriol. 105, 918–926. doi: 10.1128/JB.105.3.918-926.1971
Xu, R., Wu, K., Han, H., Ling, Z., Chen, Z., Liu, P., et al. (2018). Co-expression of YieF and PhoN in Deinococcus radiodurans R1 improves uranium bioprecipitation by reducing chromium interference. Chemosphere 211, 1156–1165. doi: 10.1016/j.chemosphere.2018.08.061
Yang, L., Liu, Y., Wu, H., Hoiby, N., Molin, S., and Song, Z. J. (2011). Current understanding of multi-species biofilms. Int. J. Oral Sci. 3, 74–81. doi: 10.4248/IJOS11027
Yau, J. W., Hou, J., Tsui, S. K. W., Leung, T. F., Cheng, N. S., Yam, J. C., et al. (2019). Characterization of ocular and nasopharyngeal microbiome in allergic rhinoconjunctivitis. Pediatr. Allerg. Immunol. 30, 624–631. doi: 10.1111/pai.13088
Keywords: exopolysaccharide, Deinococcus radiodurans, Staphylococcus aureus, biofilm formation, infection
Citation: Chen F, Zhang J, Ji HJ, Kim M-K, Kim KW, Choi J-I, Han SH, Lim S, Seo HS and Ahn KB (2021) Deinococcus radiodurans Exopolysaccharide Inhibits Staphylococcus aureus Biofilm Formation. Front. Microbiol. 12:712086. doi: 10.3389/fmicb.2021.712086
Received: 19 May 2021; Accepted: 09 December 2021;
Published: 24 December 2021.
Edited by:
Lizziane Kretli Winkelströter, University of Western São Paulo, BrazilReviewed by:
Ricardo Oropeza, National Autonomous University of Mexico, MexicoChunlei Shi, Shanghai Jiao Tong University, China
Copyright © 2021 Chen, Zhang, Ji, Kim, Kim, Choi, Han, Lim, Seo and Ahn. This is an open-access article distributed under the terms of the Creative Commons Attribution License (CC BY). The use, distribution or reproduction in other forums is permitted, provided the original author(s) and the copyright owner(s) are credited and that the original publication in this journal is cited, in accordance with accepted academic practice. No use, distribution or reproduction is permitted which does not comply with these terms.
*Correspondence: Ho Seong Seo, aG9zZW9uZ3Nlb0BrYWVyaS5yZS5rcg==; Ki Bum Ahn, YWhua2JAa2FlcmkucmUua3I=