- 1College of Horticulture, Gansu Agricultural University, Lanzhou, China
- 2College of Food Science and Engineering, Gansu Agricultural University, Lanzhou, China
- 3Department of Postharvest Science of Fresh Produce, Agricultural Research Organization (ARO), Volcani Center, Rishon LeZion, Israel
As a multifunctional signaling molecule, hydrogen sulfide (H2S) has been reported to induce plant responses to a variety of abiotic stresses. However, there are no reports on H2S treatment inducing resistance in apples against Penicillium expansum, a biotic factor, and its possible mechanism of action. In this study, fumigating apples with 5 mM sodium hydrosulfide (NaHS), the exogenous donor of H2S, for 12 h reduced the diameter of lesions in fruit colonized by P. expansum. NaHS treatment markedly promoted the synthesis of endogenous H2S, hydrogen peroxide (H2O2), and nitrogen oxide (NO). In vivo NaHS treatment enhanced the activities of phenylalanine ammonia-lyase, cinnamate 4-hydroxylase, p-coumarate:coenzyme A ligase isoenzymes, caffeoyl-CoA-O-methyltransferase, caffeic acid-O-methyltransferase, ferulic acid-5-hydroxylase, cinnamyl-CoA reductase, and cinnamyl-alcohol dehydrogenase. The treatment also facilitated the production of specific phenolic acids, such as cinnamic acid, p-coumaric acid, caffeic acid, ferulic acid, and sinapic acid; total phenolic compounds; p-coumaryl alcohol; coniferyl alcohol; sinapyl alcohol; and lignin. NaHS treatment induced resistance against P. expansum in apples through H2O2- and NO-mediated activation of phenylpropanoid metabolism.
Introduction
Penicillium expansum is a critical pathogen that can cause blue mold in various temperate fruits (Errampalli, 2014), resulting not only in fruit rot but also in the accumulation of patulin in the fruit, which presents a potential safety hazard (Yu et al., 2020). Although P. expansum can be effectively controlled by the fungicides pyrimethanil and thiabendazole (Ghosh et al., 2006; Sugar and Basile, 2008), pesticide residues remain on the fruit after storage and pose a risk to human health and the pathogens can possible develop resistance to them. Moreover, there may be registration difficulties (Romanazzi et al., 2016). These reasons make it necessary to exploit new alternative strategies for preventing postharvest diseases.
Induced resistance is a novel, valid approach that has been applied to control postharvest diseases of fruit and vegetables by triggering natural resistance using elicitors and increasing host resistance against pathogens (Bi et al., 2020). Hydrogen sulfide (H2S) is a signaling molecule that is extensively found in plants and has a protective function in stress responses (Yamasaki and Cohen, 2016). Studies have shown that exogenous H2S and NaHS treatments potentially enhance plant resistance against various biological or abiotic stresses (Wang, 2012; Guo et al., 2016). NaHS treatment significantly inhibits the decay of fresh-cut pears caused by P. expansum and Aspergillus niger (Hu K. D. et al., 2014); alleviates fresh-cut sweet potato rot induced by Geotrichum candidum, Mucor rouxianus, and Rhizopus nigricans (Tang et al., 2014); and sustains a lower rot index in strawberry fruit (Hu et al., 2012). Other studies have indicated that NaHS treatment improves the activity of L-cysteine desulfhydrase (LCD) in Arabidopsis thaliana and promotes the generation of endogenous H2S, which regulates the downstream synthesis of salicylic acid, a signal molecule (Shi et al., 2015); reduces the activity of phenylalanine ammonia-lyase; enhances the activity of ascorbate peroxidase, catalase, and peroxidase; and eliminates excessive reactive oxygen species, all of which preserves the stability of the cell membrane (Hu K. D. et al., 2014). Previous molecular analyses revealed that the delayed postharvest senescence of apples caused by H2S was linked to the suppression of the genes involved in ethylene biosynthesis (MdACS1, MdACS3, MdACO1, and MdACO2) and signal transduction (MdETR1, MdERS1, MdERS2, MdERF3, MdERF4, and MdERF5); these results supported the proposed counteractive role of H2S in ethylene biosynthesis and signaling (Ziogas et al., 2018). However, H2S treatment in the present work induces the resistance of apples to P. expansum, and its mechanism of action has not been investigated yet.
The objectives of this study were to evaluate the effect of NaHS treatment on the colonization pattern of P. expansum in treated apples and analyze the possible mechanism of action by evaluating the levels of H2S, hydrogen peroxide (H2O2), and nitrogen oxide (NO) and the activation of the enzymatic products of phenylpropanoid metabolism.
Materials and Methods
Materials
Apples (Malus domestica Borkh cv. Delicious) were picked from a state-owned orchard in the Tiaoshan farm in Jingtai County, Gansu Province, China. The fruits selected were of similar size and without any defects. A total of 360 fruits were randomly divided into two groups. Each group included three replicates of 60 fruits. They were placed in transport boxes (45 apples per box), delivered to the laboratory on the day of harvesting, and maintained in cold storage (4°C ± 2°C; RH, 55–65%). P. expansum T01 was donated by Professor Tian Shipping of the Institute of Botany, Chinese Academy of Sciences, and preserved on potato dextrose agar at 25°C. NaHS (AR 68%) was bought from Shanghai Macklin Biochemical Technology Co., Ltd. HPLC-grade standards were purchased from Nanjing Yuanzhi Biotechnology Co., Ltd.
NaHS Treatment
Following previous screening study protocols (Li D. et al., 2016), a 5-mM solution of NaHS was prepared using distilled water. Accordingly, 50 ml of 5 mM NaHS solution was placed in a 90-mm-diameter container that was then placed in a 13-L desiccator. NaHS treatment was conducted following the method of Li D. et al. (2016) with appropriate modifications. The fruits were placed in ambient temperature (22°C ± 2°C; RH, 55–60%) for approximately 24 h and washed twice to remove dirt, after which they were dipped in 0.1% (v/v) sodium hypochlorite for 2 min. The fruits were washed again with tap water and dried at ambient temperature (22°C ± 2°C; RH, 55–60%) for 2 h. Then, the fruits were fumigated with NaHS solutions in a desiccator for 12 h with distilled water acting as control. All treated fruits were placed in a carton and stored at ambient temperature (22°C ± 2°C; RH, 55–60%). Overall, 48 fruits were randomly selected for each treatment with three replicates.
Inoculation
The spore suspension was prepared according to the method of Tahir et al. (2015). Herein, 5 ml of sterile water was added to a 5-day-old culture plate of P. expansum and the spores were scraped off and filtered through four layers of germ-free gauze to obtain a suspension of 1 × 106 spores/ml.
Inoculation and measurement of lesion diameters were conducted as per the method of Li S. E. et al. (2019). All fruits were disinfected using 75% ethanol, and four uniform piercings were made using a sterilized nail (3 mm × 3 mm). After 24 h of treatment, 20 μl of the prepared spore suspension was inoculated into the wound. The fruits were dried and then placed at ambient temperature (22°C ± 2°C; RH, 55–60%). Lesion diameters were measured at 3, 4, 5, 6, and 7 days after inoculation. The longest and shortest diameters of the lesion area were determined by the crossover method and the average was taken as the lesion diameter. Three replicates of 12 randomly selected fruits per treatment method were examined.
Sampling
Sampling was performed as per the method of Ge et al. (2017). After 0, 2, 4, 6, 8, and 10 days of treatment, the test and control fruits were peeled, and using a stainless steel knife, approximately 2.0 g of fresh tissue was collected from 2 to 3 mm below the epidermis at the equatorial part for each fruit. The samples were then pulverized into powder after adding liquid nitrogen and stored at −80°C in 50 ml centrifuge tubes.
Endogenous H2S, NO, and H2O2 Content Assays
Endogenous H2S content was determined following the method of Sekiya et al. (1982) with appropriate modifications. Frozen tissue powder (1 g) was homogenized in 1 ml of 50 mM PBS (pH = 6.8) extracting solution, including 100 mM EDTA-Na2 and 200 mM ascorbic acid. The homogenate (1 ml) was placed in 0.5 ml of 1 M HCl to release H2S, which was absorbed by 0.5 ml of 1% (w/v) zinc acetate. After allowing the reaction to occur for 30 min, 0.3 ml of 5 mM dimethyl-p-phenylenediamine dihydrochloride dissolved in 3.5 mM H2SO4 was added, followed by 0.3 ml of 50 mM NH4Fe(SO4)2 in 100 mM H2SO4. The absorbance was measured at 667 nm after incubating for 15 min at ambient temperature (22°C ± 2°C). Results are presented on a fresh weight basis, and the endogenous H2S content was represented as mol kg–1.
Nitrogen oxide content was determined using a kit from the Suzhou Keming Biotechnology Co., Ltd., following the protocols of the manufacturer. Frozen tissue powder (0.5 g) was placed in 1 ml of extracting solution, and then centrifuged at 10,000 × g for 15 min at 4°C. The reaction system included 100 μl of the supernatant and 50 μl of reagents 1 and 2, respectively. After incubating at ambient temperature (22°C ± 2°C; RH, 55–60%) for 15 min, the absorbance was measured at 550 nm, with extracting solution as a control. Results are expressed on a fresh weight basis, and the NO content was expressed as mmol kg–1.
H2O2 content was measured using the method of Prochazkova et al. (2001) with appropriate modifications. Frozen tissue powder (1 g) was homogenized in 1 ml of cooled acetone and centrifuged at 10,000 × g for 40 min at 4°C. The reaction system included 1 ml of the supernatant, 100 μl of 20% titanium tetrachloride solution (v/v), and 200 μl of ammonia solution. It was homogenized for 5 min and centrifuged at 10,000 × g for 15 min at 4°C. The sediment was washed four times with cooled acetone and dissolved in 1.5 ml of 1 mM H2SO4. Absorbance was measured at 410 nm. Results are represented on a fresh weight basis, and the H2O2 content was represented as mmol kg–1.
Measurement of Enzyme Activity
The activity of phenylalanine ammonia-lyase (PAL) was assayed following the method of Koukol and Conne (1961) with appropriate modifications. Frozen tissue powder (2 g) was homogenized in 2 ml of 0.1 M sodium borate buffer (pH = 8.8), including 5 mM β-mercaptoethanol (BME), 2 mM EDTA, and 4% polyvinylpyrrolidone (PVP) for PAL. The homogenates were centrifuged at 10,000 × g for 40 min at 4°C and the supernatant was used as a crude enzyme extract. The reaction system included 3 ml of buffer solution, 500 μl of L-phenylalanine, and 500 μl of crude enzyme extract. These were incubated for 1 h at 40°C, and the reaction was terminated by adding 200 μl of 6 M HCl. Absorbance was measured at 290 nm and PAL activity was represented as U mg–1 protein.
The activities of cinnamate 4-hydroxylase (C4H) and p-coumarate:coenzyme A ligase isoenzymes (4CL) were determined using the method of Voo et al. (1995) with appropriate modifications. Frozen tissue powder (2 g) was placed in 2 ml of extracting solution containing 5 mM BME, 4 mM MgCl2, 50 mM Tris–HCl, 10% glycerinum, 10 μM Leupeptin, 1 mM PMSF, 5 mM ascorbic acid, and 0.15% PVP to crude extracts of C4H and 4CL enzymes. The C4H reaction system included 2 ml of 0.05 M Tris–HCl (pH = 8.9) containing 2 μM trans-cinnamic acid, 2 μM NADPNa2, 5 μM D-glucose 6-phosphate disodium salt, and 800 μl of crude enzyme extract. The reaction system of 4CL included 150 μl of 75 mM MgCl2, 150 μl of 1 μM CoA, 150 μl of 80 M ATP, 150 μl of 20 mM p-coumaric acid, and 500 μl of crude enzymes extract. The absorbance of C4H and 4CL were measured at 340 and 333 nm, respectively, and the activities of C4H and 4CL were expressed as U mg–1 protein.
The activities of caffeoyl-CoA-O-methyltransferase (CCoAOMT), caffeic acid-O-methyltransferase (COMT), ferulic acid-5-hydroxylase (F5H), and cinnamyl-CoA reductase (CCR) were measured using the enzyme-linked immunosorbent assay (Shanghai Enzyme-Linked Biotechnology Co., Ltd.) according to the protocols of the manufacturer. Crude enzyme extractions containing frozen tissue powder (0.5 g) were homogenized in 4.5 ml of 0.01 M PBS (pH = 7.2) extracting solution for CCoAOMT, COMT, F5H, and CCR. The reaction system included 40 μl sample dilution and 10 μl crude enzymes were incubated for 30 min at 37°C, rinsed five times with washing liquid, and dried. Afterward, 50 μl of enzyme standard reagent was added, incubated for 30 min at 37°C, and rinsed five times again. Then, 50 μl chromogenic agent A and 50 μl chromogenic agent B were added, mixed, and incubated in the dark for 10 min at 25°C. Finally, 50 μl of termination solution was added. Absorbance was measured at 450 nm, and the amounts of CCoAOMT, COMT, F5H, and CCR were expressed as U mg–1 protein.
The activity of cinnamyl-alcohol dehydrogenase (CAD) was determined following the method of Goffner et al. (1992) with appropriate modifications. Frozen tissue powder (2 g) was placed in 2 ml of 0.2 M Tris–HCl (pH = 7.5) extracting solution for CAD crude enzyme extraction. The reaction system included 1 ml of 2 mM NADP, 1.4 ml of 1 mM trans-cinnamic acid, and 600 μl of crude enzyme. The mixture was incubated for 30 min at 37°C, and then the reaction was terminated by adding 200 μl of 1 M HCl. Absorbance was measured at 340 nm and the activity of CAD was expressed as U mg–1 protein.
Protein Content Assay
The protein content was determined following the method of Bradford (1976) using bovine serum albumin as standard.
Quantification of Phenolic Acid and Lignin Monomer Content
Phenolic acid and lignin monomer contents were determined using the method of Fuad and Imad (2015) with appropriate modifications. Frozen tissue powder (3 g) was homogenized in 2 ml of methanol (75%, v/v). Ultrasonic extraction was carried out for 1 h at ambient temperature (22°C ± 2°C; RH, 55–60%). The homogenates were centrifuged at 10,000 × g for 40 min, and 3 ml of the supernatant was evaporated for 5 h at 40°C in a low-temperature rotary evaporator. The concentrate was redissolved in 1 ml of mobile phase and filtered through a 0.22-μm microporous membrane for HPLC analysis. A Waters Symmetry® C18 (4.6 × 250 mm, 5 μm) column (Ultra-fast Liquid chromatography from ACQUITY Arc, Waters, United States) was used with a quadruple gradient. The elution velocity was 0.8 ml min–1 and the injection volume was 5 μl. Sinapic acid and caffeic acid were assayed at 325 nm. Ferulic acid, p-coumaric acid, cinnamic acid, p-coumaryl alcohol, sinapyl alcohol, and coniferyl alcohol were assayed at 322, 310, 276, 322, 273, and 263 nm, respectively. The content of phenolic acids and p-coumaryl alcohol, sinapyl alcohol, and coniferyl alcohol was calculated using peak time and peak area of the mixture of sample as a standard curve. Results are represented on a fresh weight basis, and the monomer content was expressed as mg kg–1.
Analysis of Total Phenolic Compounds and Lignin Contents
The amount of total phenolic compounds was assayed using the method of Chen et al. (2015) with appropriate modifications. Frozen tissue powder (1 g) was placed in 10 ml of 0.5% acetic acid 70% acetone extracting solution and incubated in the dark for 24 h. The homogenates were centrifuged at 10,000 × g for 40 min at 4°C. The reaction system included 1 ml of extracting solution, 2 ml of Folin–Ciocalteu reagent (1:10 dilution), and 2 ml of 7.5% (w/v) sodium carbonate. It was incubated for 5 min at 50°C with methanol as control. Absorbance was measured at 760 nm, and the content of total phenolic compounds was evaluated using a gallic acid standard curve. Results are represented on a fresh weight basis, and the total phenolic compound content was expressed as g kg–1.
The amount of lignin was measured following the method of Morrisson (1972) with appropriate modification. Frozen tissue powder (3 g) was placed in 5 ml of 95% ethanol and centrifuged at 10,000 × g for 10 min at 4°C. The sediment was rinsed thrice with 95% ethanol and thrice with ethanol:normal hexane = 1:2 (v/v) and then dried. The reaction system included the precipitate and 1 ml of 25% acetyl bromide glacial acetic acid solution, and it was incubated in a 70°C water bath for 30 min. The reaction was stopped by adding 1 ml of 2 M NaOH, after which 2 ml of glacial acetic acid and 200 μl of 7.5 M hydroxylamine hydrochloric acid were added. The homogenates were centrifuged at 10,000 × g for 25 min at 4°C. Glacial acetic acid was added to 20 μl of supernatant to bring the total volume to 5 ml. Absorbance was assayed at 280 nm. Lignin content was represented as OD280 kg–1.
Statistical Analysis
All experiments were performed at least thrice. Results were represented as mean ± standard error (Origin 8.5). The difference between treatments was analyzed using ANOVA and SPSS 18.0 (SPSS Inc., Chicago, IL, United States) for Duncan’s multiple range test at the 5% level.
Results
Effect of NaHS on P. expansum Colonization and Increased Endogenous H2S, NO, and H2O2 Content
The lesion diameter is an important indicator reflecting resistance of fruit. NaHS treatment reduced lesion diameter of apple fruit, which decreased by 32.25% compared with the control at 4 days of treatment (P < 0.05) (Figure 1).
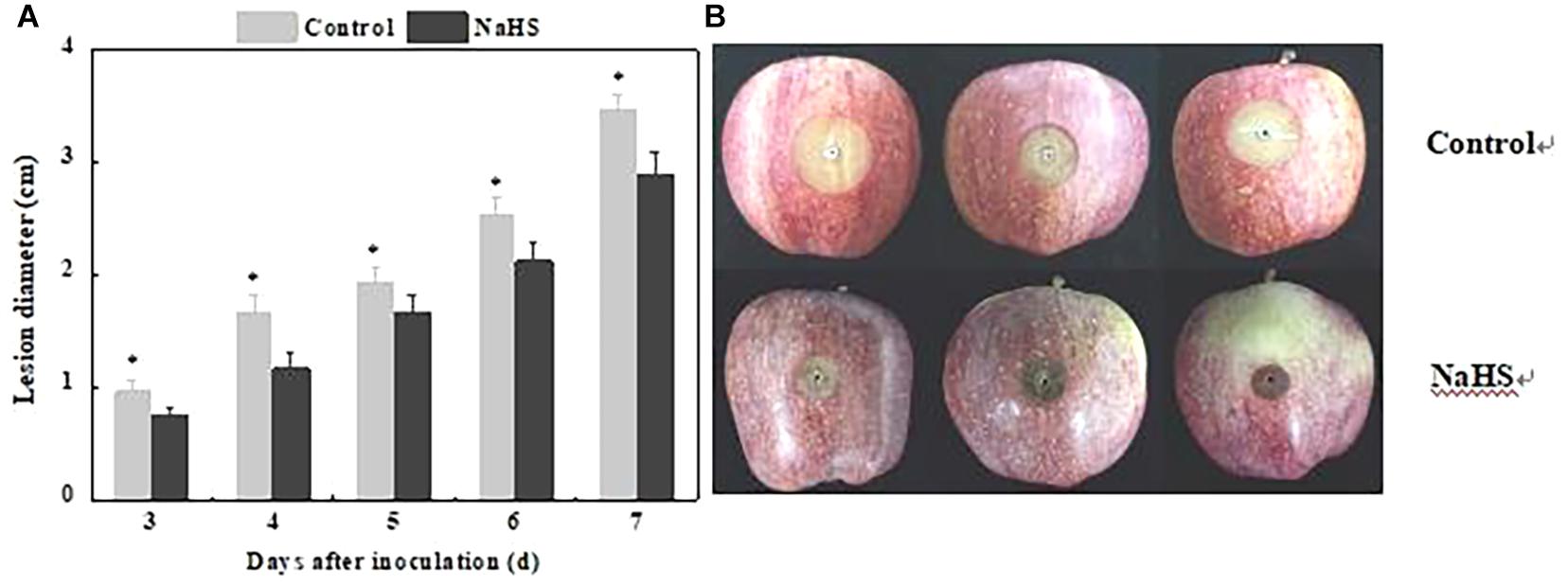
Figure 1. Inhibition of Penicillium expansum-induced decay in apples by NaHS. The bars represent the standard error of the mean (±SE). The lesion diameter (A) and representative photographs were taken after 5 days (B). ∗Significant differences at P < 0.05.
NaHS treatment enhanced the endogenous H2S level in fruit after 2 days of treatment (Figure 2A). Moreover, the level of endogenous NO in the NaHS-treated fruit increased after 2 days of treatment, which was 26.39 and 27.86% higher than that in the control at 2 and 6 days of treatment, respectively (Figure 2B). The H2O2 content increased in the NaHS-treated fruit after 2 days of treatment and peaked at 4 days of treatment, which was 74.29% higher than that of the control. At 6 days of treatment, compared with the control, the H2O2 content increased by 64.08% in the treated fruit (Figure 2C). Taken together, the results indicate that NaHS treatment of apples facilitated the generation of endogenous H2S, NO, and H2O2.
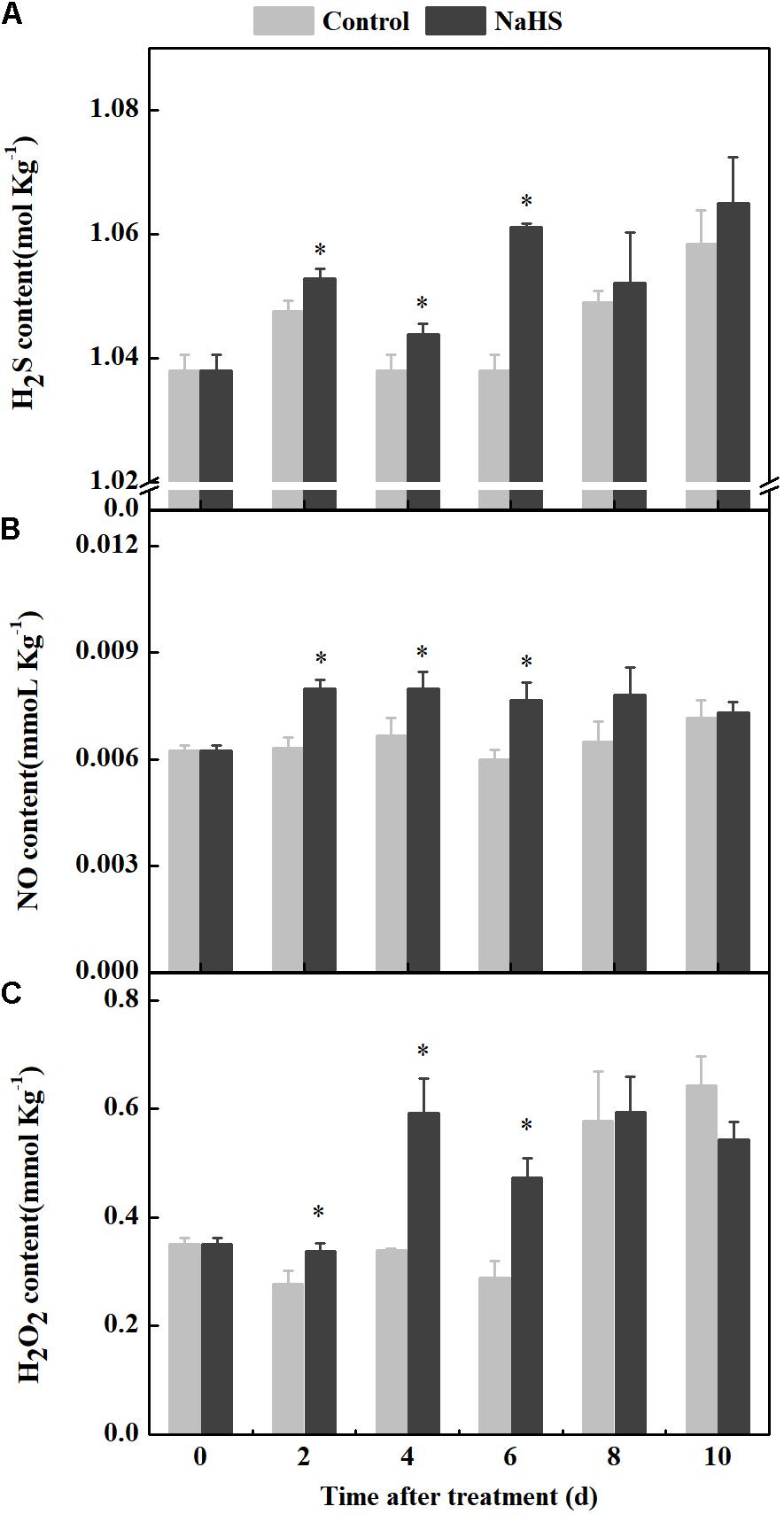
Figure 2. Changes in endogenous H2S, NO, and H2O2 contents in apples after NaHS treatment. H2S (A), NO (B), and H2O2 (C). The light gray and black bars represent the control and NaHS treatments, respectively. The bars represent the standard error of the mean (± SE). ∗Significant differences at P < 0.05.
NaHS Treatment Activated the Phenylpropanoid Pathway and Phenolic Compound Accumulation
The activities of key enzymes involved in phenylpropanoid metabolism are critical for regulating fungal colonization (Figure 3). NaHS treatment enhanced the activities of PAL, C4H, 4CL, COMT, F5H, CCoAOMT, CCR, and CAD in apples compared with the control. Among them, the maximum change was found in the activity of PAL, which was 158% higher in the NaHS-treated fruit compared with the control at 6 days of treatment (Figure 3A), while the minimum change was 4CL activity, which was 31.0% higher in the treated fruit compared with the control at 4 days of treatment(Figure 3C).
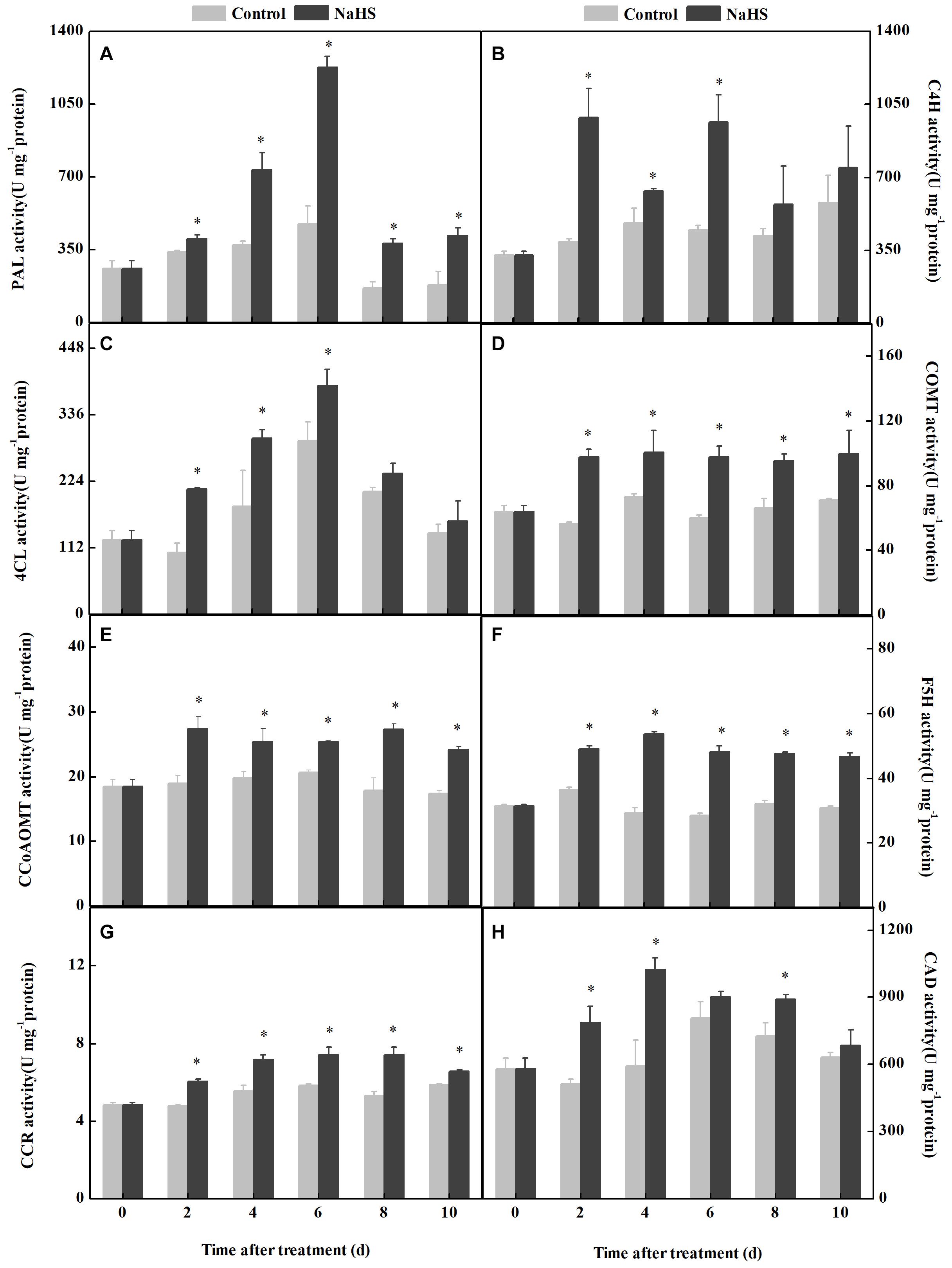
Figure 3. Changes in the activity of the key enzyme of the phenylpropanoid pathway in apples after NaHS treatment. PAL (A), C4H (B), 4CL (C), COMT (D), CCoAOMT (E), F5H (F), CCR (G), and CAD (H). The light gray and black bars represent the control and NaHS treatments, respectively. The bars represent the standard error of the mean (± SE). ∗Significant differences at P < 0.05.
Phenolic acids are mainly generated by the phenylpropanoid pathway. The contents of p-coumaric, sinapic, ferulic, and caffeic acid in fruits were increased by NaHS treatment after 2 days of treatment. The content of p-coumaric acid in the NaHS-treated fruit was 51.0% higher than that in the control at 8 days of treatment. Compared with the control, the range of changes in the sinapic, ferulic, and caffeic acid content of the treated fruit was around 15% during storage. In addition, the cinnamic acid content fluctuated in the two groups during storage. The total content of phenolic compounds in the treated fruits increased after 2 days of treatment compared with the control (Figure 4F). These results indicated that NaHS treatment affected phenylpropanoid pathway by altering the contents of five phenolic acids and the total phenolic compound content.
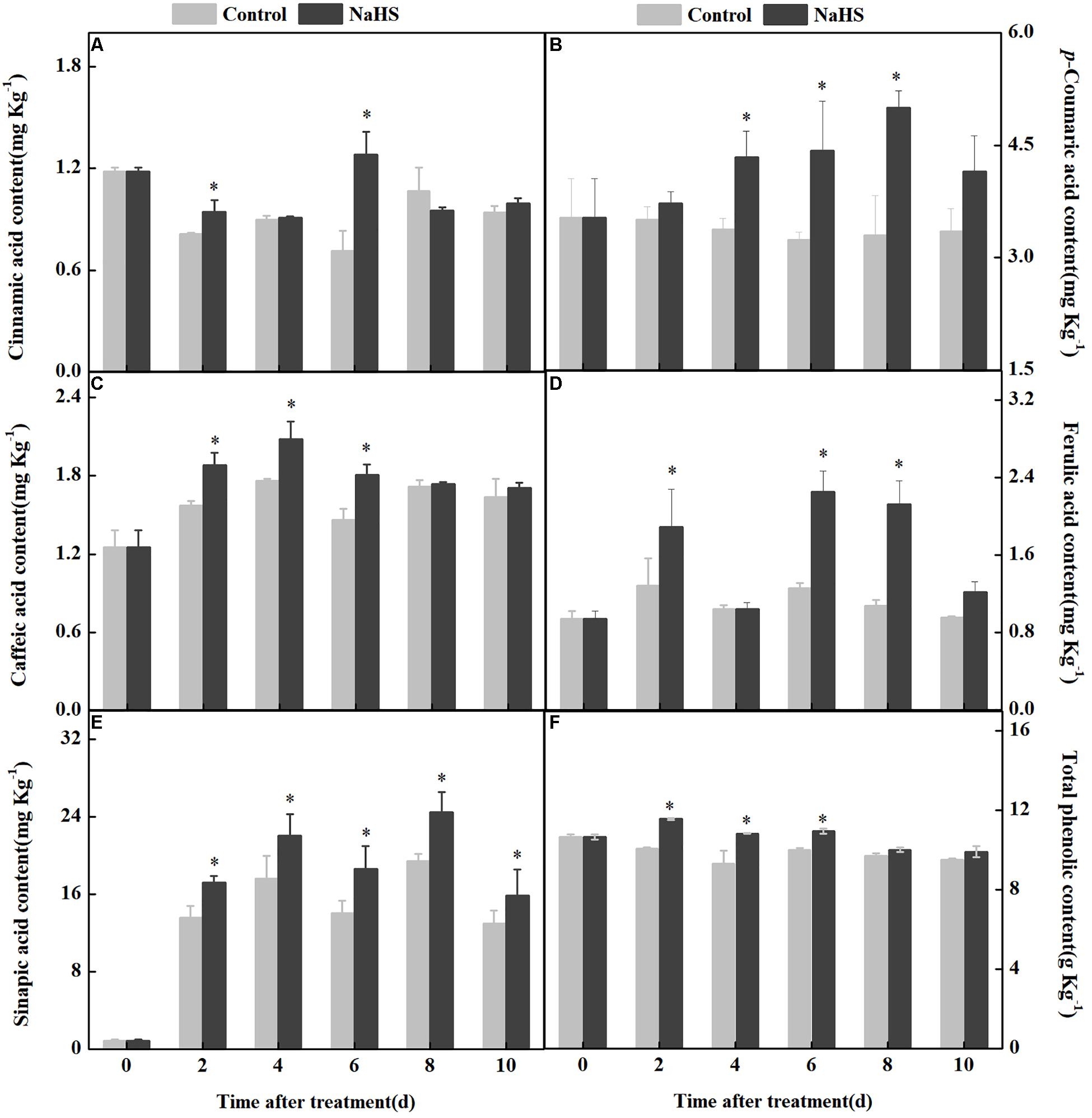
Figure 4. Changes in the content of five phenolic acids and total phenolic compounds in apples after NaHS treatment. Cinnamic acid (A), p-coumaric acid (B), caffeic acid (C), ferulic acid (D), sinapic acid (E), and total phenolic compounds (F). The light gray and black bars represent the control and NaHS treatments, respectively. The bars represent the standard error of the mean (± SE). ∗Significant differences at P < 0.05.
NaHS Treatment Promoted the Synthesis of Lignin Monomers and Lignin
Lignin is composed of p-coumaryl, sinapyl, and coniferyl alcohols, which all belonged to monolignols. The contents of p-coumaryl, sinapyl, and coniferyl alcohols in the fruit increased and consisted of the process of wound healing, while the NaHS treatment enhanced the accumulation of these compounds. At 6 days of treatment, the contents of p-coumaryl and sinapyl alcohols increased by 45 and 39% compared with the control, respectively (Figures 5A,C). At 8 days of treatment, the content of coniferyl alcohol in the NaHS-treated fruit was 25% higher than that in the control (Figure 5B). Moreover, the peak of lignin content was found at 4 days of treatment in the treated fruit, which was earlier and higher compared with the control (Figure 5D). These results indicated that the NaHS treatment enhanced the accumulation of p-coumaryl alcohol, sinapyl alcohol, coniferyl alcohol, and lignin in fruits.
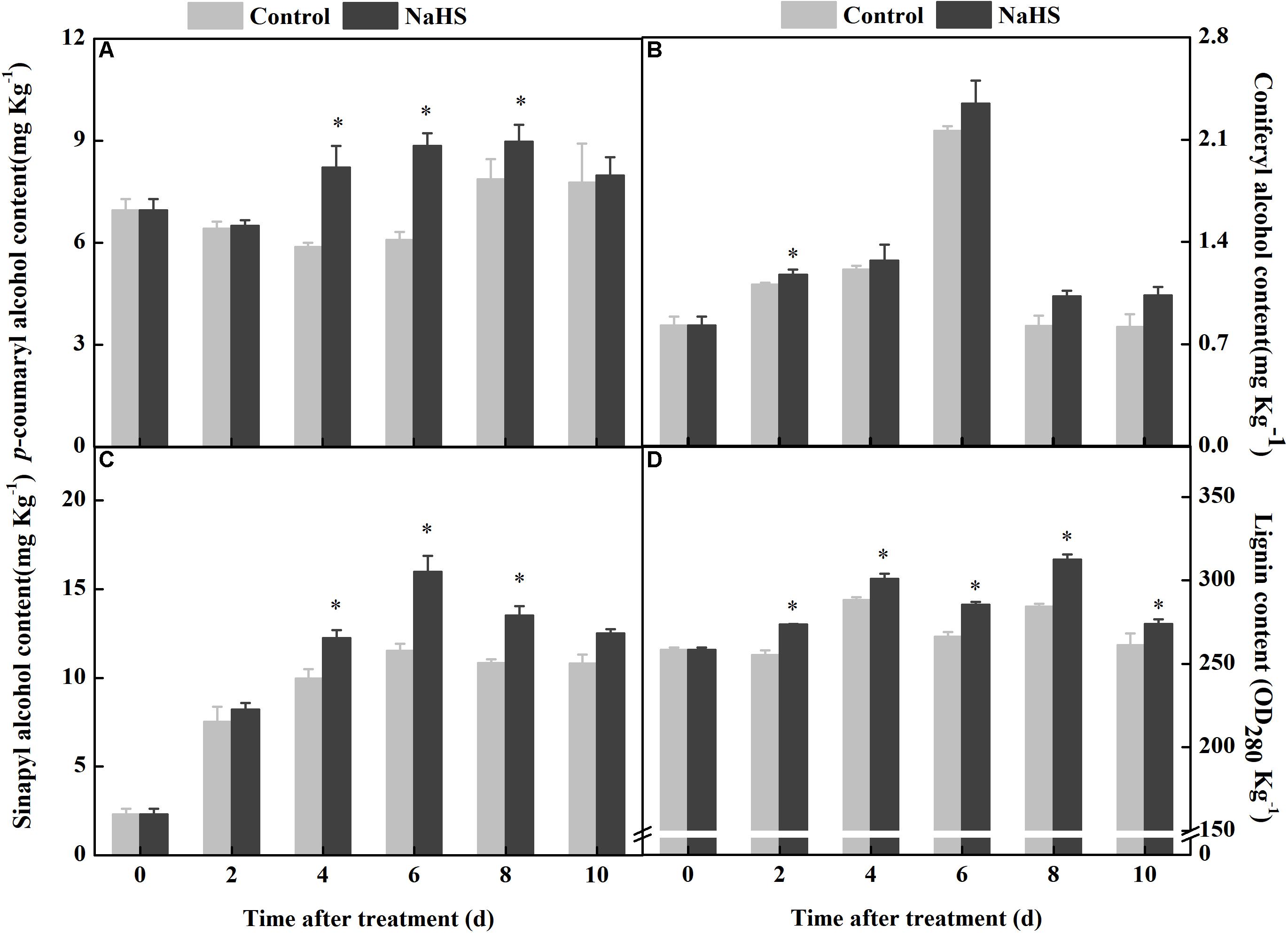
Figure 5. Changes in the content of p-coumaryl alcohol, coniferyl alcohol, sinapyl alcohol, and lignin in apples after NaHS treatment. p-Coumaryl alcohol (A), coniferyl alcohol (B), sinapyl alcohol (C), and lignin (D). The light gray and black bars represent the control and NaHS treatments, respectively. The bars represent the standard error of the mean (± SE). ∗Significant differences at P < 0.05.
Discussion
In this study, we have described a new mechanism by which NaHS treatment promotes the generation of endogenous H2S, NO, and H2O2 in apples, thus modulating the mechanism of host resistance (Figure 2). These results are similar to the observations that NaHS increases the contents of endogenous H2O2 and NO in wheat (Karpets et al., 2020) and H2S and NO in tomato seedlings (Liang et al., 2018). NaHS is used as a postharvest treatment to delay senescence in kiwifruits (Zhu et al., 2014), mulberries (Hu H. et al., 2014), and strawberries (Hu et al., 2012). At 1 ppm, H2S has no negative effects on human health, and the enzymes in the human body can detoxify H2S by oxidizing it to harmless sulfate (Wang, 2012).
NaHS is an exogenous H2S donor that dissociates into Na+ and HS– when dissolved in water; HS– then combines with H+ to generate H2S (Hosoki et al., 1997). L-cysteine and D-cysteine desulfhydrase are important enzymes for endogenous H2S generation and are activated by exogenous H2S in plants (Hu H. et al., 2014). L/D-CD can degrade cysteine into H2S, pyruvate, and ammonium (Wang, 2012). Endogenous H2S activates nitrate reductase (NR), which turns NAD(P)H and nitrate into NAD+ and H2O. Nitrite is unstable and rapidly decomposes to form NO (Besson-Bard et al., 2008; Liang et al., 2018). In addition, H2S can activate NADPH oxidase (NOX), which forms O2– by transferring electrons, which then produces H2O2 via superoxide dismutase (SOD) (Karpets et al., 2020). Therefore, we infer that NaHS promotes endogenous H2S production by activating L/D-CD activity. H2S accelerates the formation of H2O2 by activating NOX and SOD, resulting in induced resistance. As a signal molecule, H2O2 activates NR and facilitates the synthesis of endogenous NO. The potential mechanism of NaHS in promoting the production of endogenous H2S, NO, and H2O2 remains to be identified.
The possibility that NaHS modulates H2O2 raised the question of whether it will also modulate the phenylpropanoid pathway in apples. Phenylpropanoid metabolism, which generates phenolic acids and monolignols, plays a vital role in inducing stress resistance against biotic factors. Phenolic acids have shown antifungal and antioxidant activity (Romanazzi et al., 2016). PAL is a crucial enzyme in the phenylpropanoid metabolism, catalyzing the conversion of L-phenylalanine into cinnamic acid (Deng and Lu, 2017). C4H catalyzes the synthesis of p-coumaric acid from cinnamic acid and caffeic acid from p-coumaric acid. Sinapic acid is produced from caffeic acid by COMT and F5H (Arrieta-Baez and Stark, 2006; Vogt, 2010). In this study, we identified that NaHS treatment markedly enhanced the activities of PAL, C4H, 4CL, COMT, and F5H (Figures 3A–D,F) and accelerated the synthesis of cinnamic, p-coumaric, ferulic acid, and total phenolic compounds in apples (Figure 4). These results were similar to those where H2S treatment in Linum album roots increases PAL activity and accelerates the accumulation of ferulic acid and total phenolic compounds (Fakhari et al., 2019). Given that H2S promoted the synthesis of NO and H2O2, it is understandable that it also activated the phenylpropanoid pathway (Fakhari et al., 2019). A previous study showed that the gene expression and activity of PAL, C4H, and 4CL were upregulated by NO and that it elevated the generation of total phenolic and lignin in peach (Li G. J. et al., 2016). In addition, H2O2 activates PAL, C4H, and 4CL; promotes the synthesis of lignin; and accelerates the lignification process in bamboo shoot (Li D. et al., 2019). Therefore, we infer that H2S, NO, and H2O2 could serve as signal molecules to activate the phenylpropanoid pathway in the apple system.
p-Coumaric acid is a significant bridge connecting the phenylpropanoid and lignin pathways. Lignin is primarily composed of coniferyl, p-coumaroyl, and sinapyl alcohol, which are generated from p-coumaroyl-CoA, feruloyl-CoA, and sinapaldehyde, respectively, by an enzyme cascade of CCR and CAD or CCR (Vanholme et al., 2010). They undergo oxidative crosslinking to form guaiacyl lignin, syringyl lignin, and parahydroxyphenyl lignin, respectively (Zhao and Dixon, 2011; Chezem and Clay, 2016). The present study indicated that NaHS treatment improves the activities of CCoAOMT, F5H, COMT, CCR, and CAD (Figures 3D–H) and promotes the accumulation of p-coumaryl, coniferyl, sinapyl alcohols, and lignin in apples (Figure 5). It has been reported that NO not only activates PAL, C4H, and 4CL but also increases the content of lignin in muskmelon (Yan et al., 2019). In addition, H2O2 improves the synthesis of lignin in bamboo shoots (Li D. et al., 2019). Therefore, we speculate that H2S promotes the synthesis of phenolic acids, increases enzyme activity in lignin synthesis, and accelerates the accumulation of lignin and monolignols that could contribute to the enhanced resistance against the pathogen P. expansum.
Conclusion
Penicillium expansum is a wound pathogen, and enhanced phenylpropanoid metabolic activation in apples may contribute to the prevention of P. expansum colonization. NaHS treatment enabled the generation of endogenous H2S, NO, and H2O2 in apples. Moreover, NaHS supported the activities of PAL, C4H, 4CL, COMT, F5H, CCoAOMT, CCR, and CAD and induced the accumulation of cinnamic, p-coumaric, caffeic, ferulic, and sinapic acids and total phenolic compounds. NaHS treatment also accelerated the accumulation of p-coumaryl, coniferyl, and sinapyl alcohols and lignin. Given that NaHS fumigation on apples is safe for human consumption, this treatment could be considered as a possible postharvest treatment to induce resistance against P. expansum in apples.
Data Availability Statement
The raw data supporting the conclusions of this article will be made available by the authors, without undue reservation.
Author Contributions
HD wrote the manuscript. YB reviewed and edited the manuscript and was the project administrator and supervisor. BW carried out data curation and investigation. YL analyzed the data. LM provided experiment assistance. YZ and DP edited and organized the manuscript. All authors contributed to the article and approved the submitted version.
Funding
This work was supported by the National Natural Science Foundation of China (31861143046).
Conflict of Interest
The authors declare that the research was conducted in the absence of any commercial or financial relationships that could be construed as a potential conflict of interest.
Publisher’s Note
All claims expressed in this article are solely those of the authors and do not necessarily represent those of their affiliated organizations, or those of the publisher, the editors and the reviewers. Any product that may be evaluated in this article, or claim that may be made by its manufacturer, is not guaranteed or endorsed by the publisher.
Supplementary Material
The Supplementary Material for this article can be found online at: https://www.frontiersin.org/articles/10.3389/fmicb.2021.720372/full#supplementary-material
References
Arrieta-Baez, D., and Stark, R. E. (2006). Modeling suberization with peroxidase-catalyzed polymeriz-etion of hydroxy-cinnamic acids: Cross-coupling and dimerization reactions. Phytochemistry 67, 743–753. doi: 10.1016/j.phytochem.2006.01.026
Besson-Bard, A., Pugin, A., and Wendehenne, D. (2008). New insights into nitric oxide signaling in plants. Annu. Rev. Plant Biol. 59, 21–39. doi: 10.1146/annurev.arplant.59.032607.092
Bi, Y., Xue, H. L., and Wang, J. J. (2020). “Induced resistance in fruits and vegetables by elicitors to control postharvest diseases,” in Postharvest pathology of fresh horticultural produce, eds L. Palou and J. L. Smilanick (Dordrecht: CRC Press), 798–803.
Bradford, M. M. (1976). A rapid and sensitive method for the quantitation of microgram quantities of protein utilizing the principle of protein-dye binding.Anal. Biochem 72, 248–254. doi: 10.1016/0003-2697(76)90527-3
Chen, L. Y., Cheng, C. W., and Liang, J. Y. (2015). Effect of esterification condensation on the folin-ciocalteu method for the quantitative measurement of total phenols. Food Chem. 170, 10–15. doi: 10.1016/j.foodchem.2014.08.038
Chezem, W. R., and Clay, N. K. (2016). Regulation of plant secondary metabolism and associated specialized cell development by MYBs and bHLHs. Phytochemistry 131, 26–43. doi: 10.1016/j.phytochem.2016.08.006
Deng, Y., and Lu, S. (2017). Biosynthesis and Regulation of Phenylpropanoids in Plants. Crit. Rev. Plant Sci. 36, 257–290. doi: 10.1080/07352689.2017.1402852
Errampalli, D. (2014). Penicillium expansum (Blue Mold). Postharvest Decay 2014, 189–231. doi: 10.1016/B978-0-12-411552-1.00006-5
Fakhari, S., Sharifi, M., De, Michele, R., Ghanati, F., Safaie, N., et al. (2019). Hydrogen sulfide directs metabolic flux towards the lignan biosynthesis in linum album hairy roots. Plant Physiol. Biochem. 135, 359–371. doi: 10.1016/j.plaphy.2018.12.015
Fuad, A. R., and Imad, O. (2015). Development and validation of an HPLC-UV method for determination of eight phenolic compounds in date palms. J. AOAC Int. 98, 1335–1339. doi: 10.5740/jaoacint.15-010
Ge, Y. H., Wei, M. L., Li, C. Y., Chen, Y. R., Lv, J. Y., and Li, J. R. (2017). Effect of acibenzolar-S-methyl on energy metabolism and blue mould of ‘Nanguo’ pear fruit. Sci. Hortic. 225, 221–225. doi: 10.1016/j.scienta.2017.07.012
Ghosh, A., Pirgozliev, S., Errampalli, D., Wainman, L., and Stokes, S. (2006). Effect of preharvest application of pyrimethanil and calcium chloride for the control of postharvest blue mold and gray mold of apples.Can. J. Plant Pathol. 28, 350–350.
Goffner, D., Joffroy, I., Grima-Pettenati, J., Halpin, C., Knight, M. E., Schuch, W., et al. (1992). Purification and characterization of isoforms of cinnamyl alcohol denydrogenase from Eucalyptus xylem. Planta. 188, 48–53. doi: 10.1007/BF01160711
Guo, H. M., Xiao, T. Y., Zhou, H., Xie, Y. J., and Shen, W. B. (2016). Hydrogen sulfide: a versatile regulator of environmental stress in plants. Acta Physiol. Plant. 38, 1–13. doi: 10.1007/s11738-015-2038-x
Hosoki, R., Matsuki, N., and Kimura, H. (1997). The possible role of hydrogen sulfide as an endogenous smooth muscle relaxant in synergy with Nitric Oxide. Biochem. Biophys. Res. Commun. 237, 527–531. doi: 10.1006/bbrc.1997.6878
Hu, H., Shen, W., and Li, P. (2014). Effects of hydrogen sulphide on quality and antioxidant capacity of mulberry fruit. Int. J. Food Sci. Technol. 49, 399–409. doi: 10.1111/ijfs.12313
Hu, K. D., Wang, Q., Hu, L. Y., Gao, S. P., Wu, J., Li, Y. H., et al. (2014). Hydrogen sulfifide prolongs postharvest storage of fresh-cut pears (Pyrus pyrifolia) by alleviation of oxidative damage and inhibition of fungal growth. PLoS One 9:e85524. doi: 10.1371/journal.pone.0085524
Hu, L. Y., Hu, S. L., Wu, J., Li, Y. H., Zheng, J. L., Liu, J., et al. (2012). Hydrogen sulfide prolongs postharvest shelf life of strawberry and plays an antioxidative role in fruits. J. Agric. Food Chem. 60, 8684–8693. doi: 10.1021/jf300728h
Karpets, Y. V., Kolupaev, Y. E., Lugovaya, A. A., Shvidenko, N. V., and Yastreb, T. O. (2020). Functional interaction of ROS and nitric oxide during induction of heat resistance of wheat seedlings by hydrogen sulfide donor. Russ. J. Plant Physiol. 67, 653–660. doi: 10.1134/S1021443720030140
Koukol, J., and Conne, E. (1961). The metabolism of aromatic compounds in higher plants. IV. Purification and properties of the phenylalanine deaminase of Hordeum vulgare. J. Biol. Chem. 236, 2692–2698. doi: 10.1016/s0021-9258(18)69879-5
Li, D., Limwachiranon, J., Li, L., Du, R., and Luo, Z. S. (2016). Involvement of energy metabolism to chilling tolerance induced by hydrogen sulfide in cold-stored banana fruit. Food Chem. 208, 272–278. doi: 10.1016/j.foodchem.2016.03.113
Li, D., Limwachiranon, J., Li, L., Zhang, L., Xu, Y. Q., and Luo, Z. S. (2019). Hydrogen peroxide accelerated the lignification process of bamboo shoots by activating the phenylpropanoid pathway and programmed cell death in postharvest storage. Postharvest Biol. Technol. 153, 79–86. doi: 10.1016/j.postharvbio.2019.03.012
Li, G. J., Zhu, S. H., Wu, W. X., Zhang, C., Peng, Y., Wang, Q. G., et al. (2016). Exogenous nitric oxide induces disease resistance against monilinia fructicola through activating the phenylpropanoid pathway in peach fruit. J. Sci. Food Agric 2016:8146. doi: 10.1002/jsfa.8146
Li, S. E., Jiang, H., Wang, Y., Liang, L., Prusky, D., Yan, J., et al. (2019). Effect of benzothiadiazole treatment on improving the mitochondrial energy metabolism involved in induced resistance of apple fruit during postharvest storage. Food Chem. 302:125288. doi: 10.1016/j.foodchem.2019.125288
Liang, Y. L., Zheng, P., Li, S., Li, K. Z., and Xu, H. N. (2018). Nitrate reductase-dependent NO production is involved in H2S-induced nitrate stress tolerance in tomato via activation of antioxidant enzymes. Sci. Hort. 229, 207–214. doi: 10.1016/j.scienta.2017.10.044
Morrisson, I. M. (1972). A semi-micro method from the determination of lignin and its use in predicting the digestibility of forage crops. J. Agric. Food Chem. 23, 455–463. doi: 10.1002/jsfa.2740230405
Prochazkova, D., Sairam, R. K., Srivastava, G. C., and Singh, D. V. (2001). Oxidative stress and antioxidant activity as the basis of senescence in maize leaves. Plant Sci. 161, 765–771. doi: 10.1016/s0168-9452(01)00462-9
Romanazzi, G., Sanzani, S. M., Bi, Y., Tian, S. P., Martínez, P. G., and Alkan, N. (2016). Induced resistance to control postharvest decay of fruit and vegetables.Postharvest Biol. Technol. 122, 82–94. doi: 10.1016/j.postharvbio.2016.08.003
Sekiya, J., Schmidt, A., Wilson, L. G., and Filner, P. (1982). Emission of hydrogen-sulfifide by leaf tissue in response to L-cysteine. Plant Physiol. 70, 430–436. doi: 10.1104/pp.70.2.430
Shi, H. T., Ye, T. T., Han, N., Bian, H. W., Liu, X. D., and Chan, Z. L. (2015). Hydrogen sulfide regulates abiotic stress tolerance and biotic stress resistance in arabidopsis.J. Integr. Plant Biol. 57, 628–640. doi: 10.1111/jipb.12302
Sugar, D., and Basile, S. R. (2008). Timing and sequence of postharvest fungicide and biocontrol agent applications for control of pear decay. Postharvest Biol. Technol. 49, 107–112. doi: 10.1016/j.postharvbio.2007.12.008
Tahir, I. I., Nybom, H., Ahmadi-Afzadi, M., Røen, K., Sehic, J., and Røen, D. (2015). Susceptibility to blue mold caused by Penicillium expansum in apple cultivars adapted to a cool climate. Eur. J. Hortic. Sci. 80, 117–127. doi: 10.17660/eJHS.2015/80.3.4
Tang, J., Hu, K. D., Hu, L. Y., Li, Y. H., and Zhang, H. (2014). Hydrogen sulfide acts as a fungicide to alleviate senescence and decay in fresh-cut sweetpotato. HortScience 49, 938–943. doi: 10.21273/HORTSCI.49.7.938
Vanholme, R., Demedts, B., Morreel, K., Ralph, J., and Boerjan, W. (2010). Lignin biosynthesis and structure.J. Plant Biochem. Physiol. 153, 895–905. doi: 10.1104/pp.110.155119
Voo, K. S., Whetten, R. W., O’Malley, D. M., and Sederoff, R. R. (1995). 4-Coumarate: Coenzyme A ligase from loblolly pine xylem (isolation, characterization, and complementary DNA cloning). Plant Physiol. 108, 85–97. doi: 10.1104/pp.108.1.85
Wang, R. (2012). Physiological implications of hydrogen sulfide: a whiff exploration that blossomed. Physiol. Rev. 92, 791–896. doi: 10.1152/physrev.00017.2011
Yamasaki, H., and Cohen, M. F. (2016). Biological consilience of hydrogen sulfide and nitric oxide in plants: gases of primordial earth linking plant, microbial and animal physiologies. Nitric Oxide. 55, 91–100. doi: 10.1016/j.niox.2016.04.002
Yan, B. W., Zhang, Z., Zhang, P., Zhu, X., Jing, Y. Y., Wei, J., et al. (2019). Nitric oxide enhances resistance against black spot disease in muskmelon and the possible mechanisms involved. Sci. Hortic. 256:108650. doi: 10.1016/j.scienta.2019.108650
Yu, L. L., Qiao, N. Z., Zhao, J., Zhao, J. X., Zhang, H., Tian, F., et al. (2020). Postharvest control of Penicillium expansum in fruits: A review. Food Biosci. 36:100633. doi: 10.1016/j.fbio.2020.100633
Zhao, Q., and Dixon, R. A. (2011). Transcriptional networks for lignin biosynthesis: more complex than we thought? Trends Plant Sci. 16, 227–233. doi: 10.1016/j.tplants.2010.12.005
Zhu, L., Wang, W., Shi, J., Zhang, W., Shen, Y., Du, H., et al. (2014). Hydrogen sulfideextends the postharvest life and enhances antioxidant activity of kiwifruit duringstorage. J. Sci. Food Agr. 94, 2699–2704. doi: 10.1002/jsfa.6613
Keywords: apple fruit, NaHS, Penicillium expansum, induced resistance, signaling molecule, phenylpropanoid metabolism
Citation: Deng H, Wang B, Liu Y, Ma L, Zong Y, Prusky D and Bi Y (2021) Sodium Hydrosulfide Induces Resistance Against Penicillium expansum in Apples by Regulating Hydrogen Peroxide and Nitric Oxide Activation of Phenylpropanoid Metabolism. Front. Microbiol. 12:720372. doi: 10.3389/fmicb.2021.720372
Received: 04 June 2021; Accepted: 30 July 2021;
Published: 01 September 2021.
Edited by:
Khamis Youssef, Agricultural Research Center, EgyptReviewed by:
Kamal A. M. Abo-Elyousr, Assiut University, EgyptLintle Mohase, University of the Free State, South Africa
Copyright © 2021 Deng, Wang, Liu, Ma, Zong, Prusky and Bi. This is an open-access article distributed under the terms of the Creative Commons Attribution License (CC BY). The use, distribution or reproduction in other forums is permitted, provided the original author(s) and the copyright owner(s) are credited and that the original publication in this journal is cited, in accordance with accepted academic practice. No use, distribution or reproduction is permitted which does not comply with these terms.
*Correspondence: Yang Bi, Yml5YW5nQGdzYXUuZWR1LmNu