- 1Department of Respiratory and Critical Care Medicine, The First Affiliated Hospital, Zhejiang University School of Medicine, Hangzhou, China
- 2Department of Microbiology, University of Chicago, Chicago, IL, United States
- 3Department of Microbiology and Cell Science, University of Florida, Gainesville, FL, United States
Acinetobacter baumannii is an important pathogen of nosocomial infection. Recently, a group of genes, named “gig” (for Growth in Galleria), have been identified in a contemporary multi-drug resistant clinical isolate of A. baumannii—strain AB5075. Among these so-called gig genes, gigA and gigB were found to promote antibiotic resistance, stress survival, and virulence of AB5075 by interacting with the nitrogen phosphotransferase system (PTSNtr). This study aimed to investigate the roles of gigA/gigB, which appear to comprise a stress-signaling pathway (encoding for an atypical two-component system response regulator and a predicted anti-anti-sigma factor, respectively), and the involvement of ptsP (encoding the Enzyme I component of the PTSNtr) in the growth, stress resistance, and virulence of the widely studied A. baumannii strain ATCC 17978. Genetic analyses of strains harboring mutations of gigA and gigB were performed to investigate the roles of these genes in bacterial growth, stress resistance, evading macrophage defense, and killing of Galleria mellonella larva. In contrast with findings from strain AB5075 where gigA and gigB contribute to aminoglycoside resistance, the data presented herein indicate that the loss of gigA/gigB does not impact antibiotic resistance of strain ATCC 17978. Interestingly, however, we found that deletion of gigA/gigB in the ATCC 17978 background imparts a general growth in laboratory medium and also conferred growth and replication defects within murine macrophages and an inability to kill G. mellonella larvae. Importantly, studies as well as the loss of ptsP restored the phenotypes of the gigA/gigB mutant to that of the wild-type. The data presented herein indicate that in A. baumannii ATCC 17978, the gigA/gigB genes play a key role in both growth and virulence traits, but are dispensable for other stress-resistance survival phenotypes, including aminoglycoside resistance. Our findings thus highlight several similarities and also important differences between the gigA/gigB stress-signaling pathway in two commonly studied isolates of this troublesome pathogen.
Introduction
Acinetobacter baumannii is a Gram-negative bacterium responsible for approximately 20% of intensive care unit infections worldwide and is the top-ranking pathogen on the World Health Organization’s list of priority antibiotic-resistant pathogens (Lee et al., 2017; WHO, 2017; Karalewitz and Miller, 2018). Many circulating A. baumannii strains exhibit a multidrug-resistant phenotype due to a combination of intrinsic and acquired traits (Peleg et al., 2012; Gottig et al., 2014). Identification of virulence determinants and understanding of the mechanisms underlying the pathogenesis of A. baumannii are important for combating A. baumannii infection.
Recently, Gebhardt et al. (2015) have identified a group of genes, named “gig” (for Growth in Galleria), that are required for growth of the highly virulent and highly antibiotic resistant A. baumannii strain AB5075 in Galleria mellonella larvae. Among these genes, gigA and gigB were found to promote antibiotic resistance, stress survival, and virulence of AB5075 by interacting with the nitrogen phosphotransferase system (PTSNtr) (Gebhardt and Shuman, 2017). gigA encodes a protein phosphatase 2C-type phosphatase, and gigB encodes a putative anti-anti-sigma factor. GigA was shown to dephosphorylate GigB, which in turn regulates the phosphate level on NPr, a key component of the PTSNtr. Disruption of the GigA/GigB signaling pathway led to the altered expression of numerous stress response genes. Thus, the intersection of GigA/GigB with the PTSNtr promotes stress survival (Gebhardt and Shuman, 2017).
The ptsP gene encodes the enzyme I component of the PTSNtr. Mutations in ptsP increases tobramycin resistance (Schurek et al., 2008; Scribner et al., 2020; Abisado et al., 2021). In AB5075, deletion of ptsP in either a ΔgigA or ΔgigB background suppresses the gig mutant phenotypes to near-wild-type levels, including restoration of aminoglycoside resistance, stress survival, and growth in Galleria larvae (Gebhardt and Shuman, 2017). Our previous work has revealed that in A. baumannii AB5075 mutants lacking both gigA and gigB (i.e., a ΔgigAB double mutant), only concurrent complementation of both gigA and gigB can restore kanamycin resistance to wild-type levels, suggesting that gigA and gigB are inseparable in the pathogenesis of A. baumannii (Gebhardt and Shuman, 2017). However, the role played by ptsP in the survival and virulence of an A. baumannii ΔgigAB mutant strain remains unknown.
ATCC 17978 is among the best-studied strains of A. baumannii and is an ideal model for genetic manipulation compared with clinical isolates due to its sensitivity to most antibiotics and high genome homology to current A. baumannii isolates (Sahl et al., 2011; Jacobs et al., 2014a). In this study, we investigated the roles of gigA/gigB and the involvement of ptsP in the growth, stress response, and virulence of ATCC 17978. Our results may provide new information about the roles of gigA/gigB and the PTSNtr system in the pathogenesis of A. baumannii infection.
Materials and Methods
Bacterial Strains and Culture
A. baumannii ATCC 17978 was purchased from The American Type Culture Collection (Manassas, VA, United States). Escherichia coli DH5α was obtained from Invitrogen (Carlsbad, CA, United States). The tetracycline-resistant and sucrose-sensitive plasmid pMJG42, apramycin-resistant pMJG120, and gentamicin-resistant pMJG125 plasmids were kept in our laboratory at the University of Chicago (Chicago, IL, United States). The bacteria were cultured in lysogeny broth (LB) medium at 37°C. When required, the antibiotics added for selection were tetracycline (10 μg/mL), apramycin (50 μg/mL), and gentamicin (10 μg/mL).
Generation of Gene Deletion and Complementation Plasmids
Gene deletion and complementation plasmids were generated as previously described (Jacobs et al., 2014b; Gebhardt et al., 2015; Gebhardt and Shuman, 2017). Briefly, gene deletions were performed using allelic exchange plasmid pMJG42. The resulting plasmids (pMJG42-ΔgigAB, pMJG42-ΔptsP) were transformed into ATCC 17978 via electroporation to obtain ATCC 17978 ΔgigAB and ATCC 17978 ΔptsP mutants. After tetracycline selection and sucrose counterselection, the clones were subjected to colony PCR. Gene deletions were confirmed by sequencing. For complementation of the deleted gigA/gigB, the entire open reading frames of gigA/gigB were amplified by PCR, cloned into pMJG120 or pMJG125 to obtain pMJG120-gigAB or pMJG125-gigAB, and transformed into ATCC 17978 ΔgigAB via electroporation to obtain ATCC 17978 ΔgigAB pMJG120-gigAB or ATCC 17978 ΔgigAB pMJG125-gigAB.
ATCC 17978 ΔgigAB was transformed with pMJG42-gigA/gigB to generate ATCC 17978’ with in situ complementation of gigA and gigB. ATCC 17978 ΔptsP was transformed with pMJG42-gigAB to generate ATCC 17978 ΔptsPΔgigAB. All bacterial strains, plasmids, and primers in this study were summarized in Supplementary Tables 1–3.
Whole-Genome Sequencing
Eight strains of ATCC 17978 ΔgigAB were randomly selected from different batches for whole-genome sequencing. Genomic DNA was prepared using the QIAamp DNA Mini Kit (Qiagen, Germany) and then subjected to whole genome sequencing (WGS) using the Illumina Hiseq2500 platform (Illumina, CA, United States) following the 2 × 100 bp protocol. The average sequencing throughput was 1 Gb. Raw fastq reads were trimmed by Trimmomatic for quality control (Bolger et al., 2014) and subsequently mapped against the reference genome of ATCC 17978-mff (Accession No. CP012004) with Bowtie2 (Langmead and Salzberg, 2012). Variant calling was performed using the bcftools call function with the default parameters (Danecek et al., 2021). We had submitted all of these data to NCBI BioProject database under the BioProject ID PRJNA738724.1
Calculation of Gene Deletion Efficiency
After antibiotics selection and sucrose counterselection, 24 clones were randomly selected for colony PCR. Gene deletions were confirmed by sequencing. The gene deletion efficiency was calculated as (the number of the clones with successful deletion mutation)/24 × 100% The experiment was repeated three times, and data were expressed as the mean ± standard deviation (SD).
Efficiency of Plating Analysis
Overnight cultures of the indicated strains were back-diluted into fresh LB and grown for 2 h. After outgrowth, aliquots of the cultures were serially diluted. Then, a 10-μL aliquot was spotted onto LB agar plates with or without stressors as follows: HCl (medium adjusted to pH 5.5), ZnCl2 (final concentration = 1.25 mmol/L). Colony forming units (CFU) were counted at 12 h after incubation at 37 or 50°C. Efficiency of plating (EOP) was calculated as (CFU recovered on stress medium)/(CFU recovered on plain medium at 37°C).
Bacterial Growth Curves
ATCC 17978, ATCC 17978 ΔgigAB, ATCC 17978 ΔptsP, and ATCC 17978 ΔptsP ΔgigAB were cultured in LB medium without antibiotics. ATCC 17978 ΔgigAB pMJG120 and ATCC 17978 ΔgigAB pMJG120-gigAB were cultured in LB medium containing 50 mg/L apramycin. ATCC 17978 ΔgigAB pMJG125 and ATCC 17978 ΔgigAB pMJG125-gigAB was cultured in LB medium containing 10 mg/L gentamicin, in the presence or absence of 1% (w/v) arabinose.
Each strain was grown overnight on the appropriate LB agar plate, and a single colony was picked and expanded in 2 mL LB broth overnight. A 1 μL aliquot was diluted at 1:1,000, and the dilution was added into triplicate wells of a 96-well plate at 200 μL/well. LB medium without bacteria was used as a blank. The OD600 was determined every 15 min using a Biotek plate reader (Winooski, VT, United States). Growth curves were generated using GraphPad Prism 5 (San Diego, CA, United States). Each experiment was performed in triplicate and repeated three times. The mean was calculated for each experiment, and data were presented as the mean of three experiments.
Antibiotic Sensitivity Testing
Antibiotic sensitivity testing was performed as previously described (Gebhardt et al., 2015). The antibiotics used in this study are summarized in Table 1. Data were expressed as minimum inhibitory concentration (MIC).
Isolation of Mouse Bone Marrow-Derived Macrophages (BMDMs)
Bone marrow-derived macrophages (BMDMs) were obtained from 8 to 12 week old female C57BL/6J (Jackson Laboratories) mice as previously described (Toda et al., 2021). Briefly, bone marrow cells were collected from the femur and tibia of mice and maintained in RPMI 1640 medium (Gibco, Thermo Fisher Scientific, Waltham, MA, United States) supplemented with 10 ng/mL mouse macrophage colony-stimulating factor (mMCSF; Gibco), 10% fetal bovine serum (Gibco), and 1% Pen/Strep (Gibco) at 37°C in a humidified atmosphere of 5% CO2 for 7 days.
Bacterial Killing Assay
Mouse BMDMs were plated in a 96-well plate at a density of 50,000 cells/well and cultured overnight. Cells were infected with wild-type ATCC 17978, ATCC 17978 ΔgigAB, or ATCC 17978 ΔgigAB pMJG120-gigAB at 5 × 105 CFU/mL. The plate was centrifuged at 2,170 rpm for 30 min at room temperature, followed by incubation at 37°C for 30 min. After replacing the medium with RPMI 1640 containing 100 mg/L gentamicin to kill extracellular bacteria, the infected cells were incubated for an additional 1 h (t = 0 h). Then, the infected cells were cultured in RPMI 1640 supplemented with 25 mg/L gentamicin (wild type and ΔgigAB strains) or 1.5 mg/L polymyxin (ΔgigAB pMJG120-gigAB strain). Cell lysates were collected at 0, 2, and 6 h post infection using phosphate buffered saline (PBS) containing 1% Triton-X100, serially diluted, and plated on LB agar plates. CFU were enumerated after 18 h of growth at 37°C. Each experiment was performed in triplicate and repeated three times. The mean CFU of surviving bacteria was calculated for each experiment, and data were presented as the mean of three experiments.
G. mellonella Killing Assay
Infection of Galleria mellonella larvae (Knutson’s LiveBait, Brooklyn, MI) was performed as described previously (Jacobs et al., 2014a; Gebhardt and Shuman, 2017). Briefly, the bacteria were grown overnight in an orbital shaker (37°C, 200 rpm), and overnight cultures were resuspended in PBS to a final OD600 of 1.0. G. mellonella larvae were randomly divided into three groups (n = 10/group). A total of 10 μL cultures (5 × 106 CFU/mL) were inoculated into the last left proleg of each larva. After injection, larvae were incubated at 37°C. The number of dead larvae was recorded hourly. Each experiment was performed in triplicate and repeated three times. The mean larval survival was calculated for each experiment, and data were presented as the mean of three experiments.
Statistical Analysis
Data were expressed as the mean ± SD. Statistical analysis was performed using GraphPad Prism 5. Differences among groups were compared using one-way ANOVA followed by Dunnett’s post-hoc test. Killing curves were plotted using the Kaplan-Meier method. A P-value of < 0.05 was considered statistically significant.
Results
gigA/gigB Are Important for the Growth but Not Required for the Survival of ATCC 17978
In our preliminary study, we noticed that the gigA/gigB deletion efficiency in wild-type 17978 was only 4.2%, suggesting that loss of gigA/gigB inhibits the growth of 17978 (Table 2). To further explore how the genetic background affects the efficiency of gigA/gigB deletion, we assessed ΔgigAB mutation efficiency in various 17978 genetic backgrounds. All gene deletions and complementation were confirmed by sequencing. The results of these analyses are shown in Table 2, and indicate that strains harboring either a ptsP deletion or in trans-complementation of gigA/gigB greatly increased the frequency of isolating the gigA/gigB double deletion mutation, suggesting that ptsP deletion and gigA/gigB complementation can compensate for the apparent growth defect caused by loss of gigA/gigB.
In the arabinose-inducible pMJG125 vector-based complementation of gigA/gigB background, we observed that the ΔgigAB colonies were smaller than wild-type colonies in the absence of arabinose; this phenotype was eliminated by the supplementation with 1% arabinose (Table 2 and Supplementary Figure 1). This finding further confirms that gigA/gigB are important for the growth of 17978 and that complementation of gigA/gigB with arabinose supplementation promotes the growth of ΔgigAB mutant to the wild-type level.
To determine if the loss of gigA/gigB required the generation of suppressing mutations, we performed whole genome sequencing on eight 17978 ΔgigAB clones isolated from different batches of gene knockout experiments. We found that, other than the gigA/gigB deletion, the genome of each of the sequenced ΔgigAB clones was 100% identical to the genome of ATCC 17978-mff reference strain, suggesting that deletion of the gigA/gigB genes does not require suppressing/compensatory mutations and that ATCC 17978 can survive without gigA/gigB. Thus, gigA/gigB are important for the growth but not required for the survival of ATCC 17978 under our routine laboratory culturing conditions.
Loss of ptsP and/or gigAB Does Not Affect Antibiotic Resistance of ATCC 17978
To explore the roles of ptsP and gigA/gigB in the antibiotic resistance of ATCC 17978, we performed antibiotic susceptibility tests in the wild type and gene deletion strains. As shown in Table 1, although the MIC of apramycin, chloramphenicol, and kanamycin were decreased in at least two deletion mutation strains compared with those in wild-type ATCC 17978, the results did not reach statistical significance. These data suggest that, in contrast to our previous findings in the A. baumannii AB5075 strain background, ptsP and gigA/gigB are not required for antibiotic resistance of ATCC 17978.
Loss of ptsP Restores the Growth of 17978 ΔgigAB to the Wild-Type Level
To explore the involvement of ptsP in gigA/gigB-mediated growth of ATCC 17978, we performed growth curve analyses. As shown in Figure 1A, 17978 ΔgigAB exhibited remarkably suppressed growth compared with the wild-type, whereas 17978 ΔptsP exhibited a comparable growth rate to the wild-type, suggesting that gigA/gigB contribute to 17978 growth. Interestingly, 17978 ΔptsPΔgigAB showed comparable growth to the wild-type strain, indicating that loss of ptsP alleviates the growth defect associated with the loss of gigA/gigB. In addition, pMJG125-based complementation of gigA/gigB also restored the growth of 17978 ΔgigAB to the wild-type level in the presence of arabinose (Figure 1B).
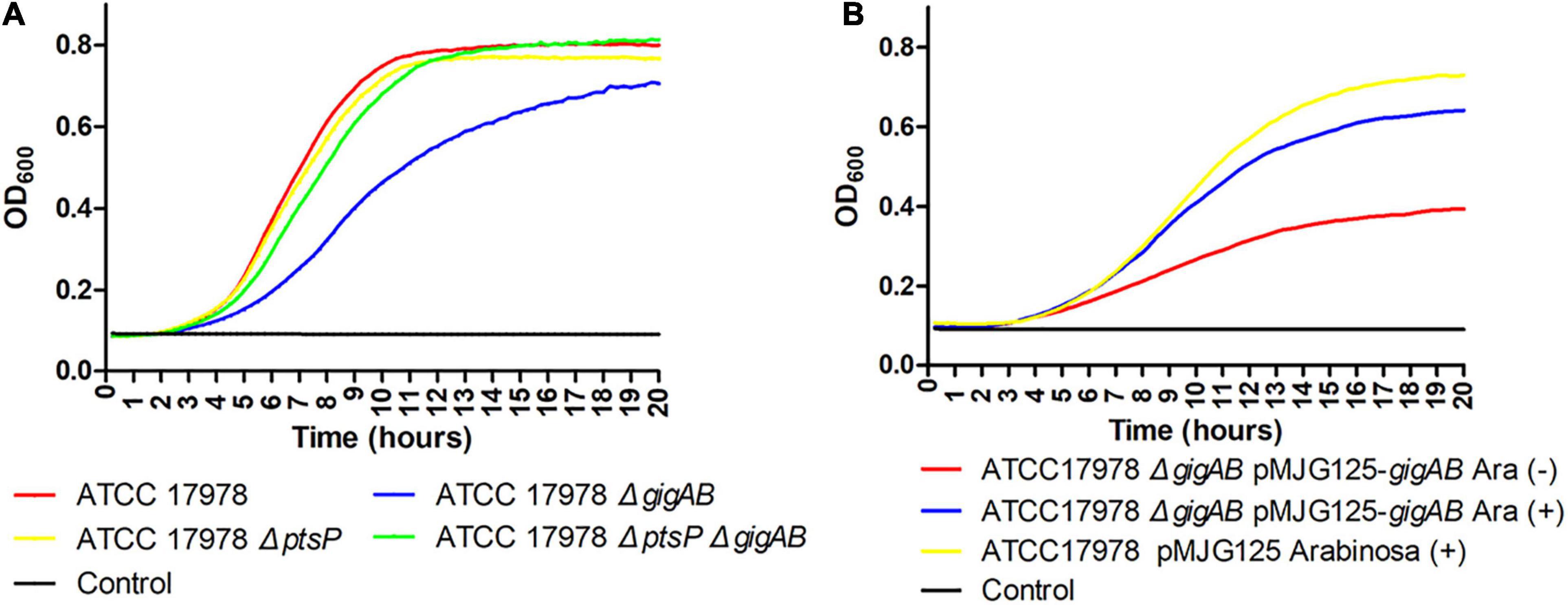
Figure 1. Growth curves of different ATCC 17978 stains. (A) The indicated strains were grown for 20 h at 37°C in LB. (B) The strains indicated were grown for 96 h at 37°C in LB with or without 1% arabinose. Growth was measured by determining the OD600 every 15 min. Each experiment was performed in triplicate and repeated three times, and the most representative curves were presented.
gigA/gigB Mediate in vitro High-Temperature Resistance of ATCC 17978
We next sought to explore any additional roles of gigA/gigB and ptsP in stress resistance of ATCC 17978. Although the wild-type 17978 grown at 50°C showed a moderately reduced colony size phenotype when compared with those grown at 37°C, no significant loss of CFU was observed (EOP = 1; Figure 2A, left panel). However, loss of both gigA and gigB resulted in a dramatic reduction in CFU at 50°C (EOP = 10–5; Figure 2A, right panel), suggesting that gigA and gigB contribute to high-temperature resistance of 17978. In the absence of arabinose, complementation of both gigA and gigB partially restored the growth of ΔgigAB mutant at 50°C (EOP = 10–3; Figure 2B, left panel). Importantly, arabinose supplementation further restored the growth of ΔgigAB mutant with gigA/gigB complementation to the wild-type level at 50 °C (EOP = 1; Figure 2B, right panel), despite the small sizes of the colonies. This finding suggests that gigA/gigB contribute to high-temperature resistance of 17978 on solid media. As observed in the growth curves described above, 17978 ΔptsP ΔgigAB and 17978 ΔptsP exhibited comparable growth at 50°C (EOP = 1; Figure 2C), suggesting that loss of ptsP restores the growth of ΔgigAB mutant under high-temperature stress. Taken together, these results suggest that gigA/gigB mediate high-temperature resistance of 17978 on LB agar plates, whereas ptsP negatively regulates this response.
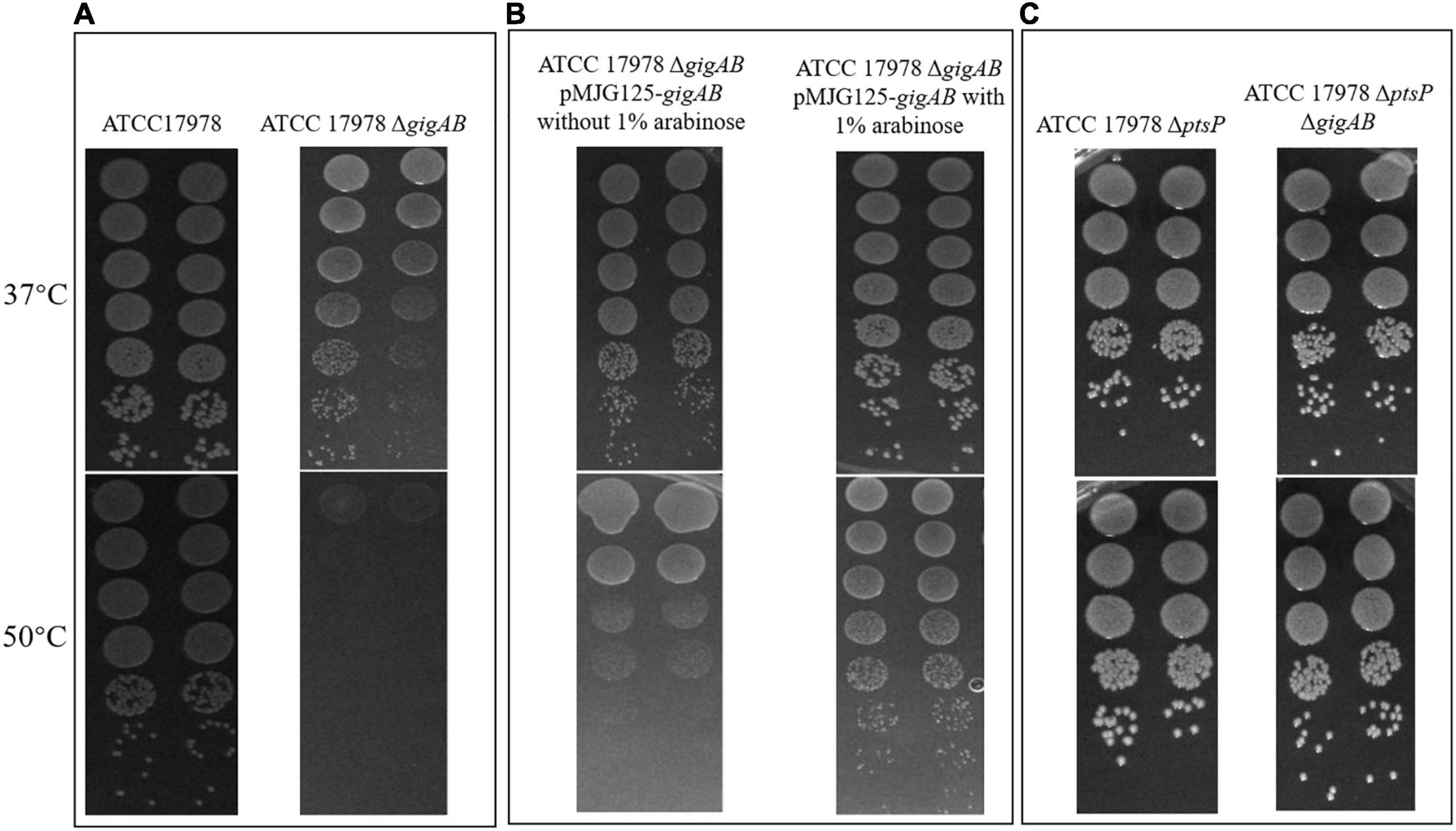
Figure 2. The roles of gigA/gigB in ATCC 17978 in response to high temperature. (A) Images of wild-type ATCC 17978 and ATCC 17978 ΔgigAB mutant grown on LB at 37 or 50°C. (B) Images of ATCC 17978 ΔgigAB pMJG125-gigAB grown on LB without or with 1% arabinose at 37 or 50°C. (C) Images of ATCC 17978 ΔptsP and ATCC 17978 ΔptsPΔgigAB grown on LB at 37 or 50°C.
When we examined the ability of the ΔgigAB strain to survive acid stress (pH = 5.5), we did not observe significant differences in the growth between the wild-type and ΔgigAB mutant strains (EOP = 1; Figure 3A), suggesting that the 17978 strain is not sensitive to pH stress as measured herein, and that the loss of gigA and gigB does not confer an acid stress sensitivity on the 17978 strain.
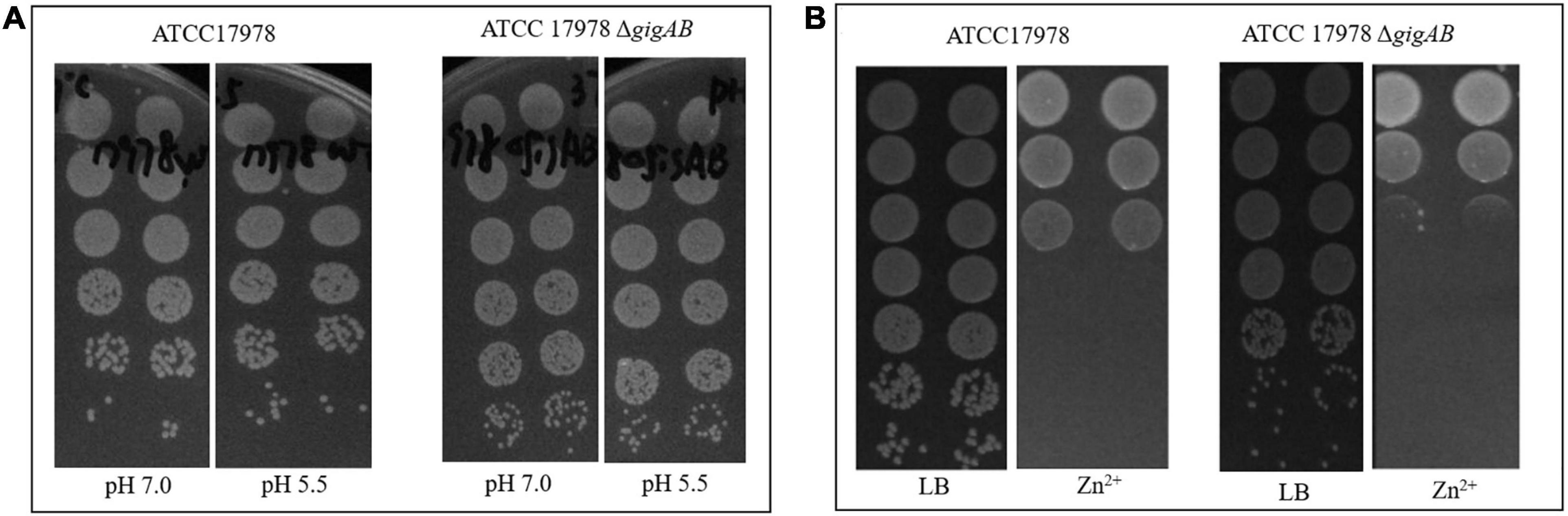
Figure 3. The roles of gigA/gigB in ATCC 17978 in response to acid or zinc. (A) Images of wild-type ATCC 17978 and ATCC 17978 ΔgigAB mutant grown on LB at pH 7.0 or pH 5.5. (B) Images of wild-type ATCC 17978 and ATCC 17978 ΔgigAB mutant grown on LB with or without 1.25 mmol/L Zn2+.
When cultured on LB containing Zn2+, both wild-type and ΔgigAB mutant demonstrated significantly suppressed growth compared with those cultured on LB without Zn2+ (EOP = 10–4, Figure 3B). No major difference was observed in the growth between the wild-type and ΔgigAB mutant. This finding suggests that factors other than gigA and gigB mediate zinc resistance of ATCC 17978.
gigA/gigB Protect ATCC 17978 From BMDM Killing
Evading macrophage phagocytosis is critical for the survival of pathogens in vivo (Rosales and Uribe-Querol, 2017). To investigate the roles of gigA/gigB in macrophage killing evasion of ATCC 17978, we infected murine BMDMs with the wild-type, ΔgigAB mutant, and gigAB complementation strains and monitored their survival and replication. When BMDMs were infected with wild-type 17978, we observed a 10-fold reduction of intracellular live bacteria at 2 h after infection (Figure 4). On the other hand, when BMDMs were infected with ΔgigAB mutant, we observed a 300-fold reduction of live bacteria in BMDMs at 2 h after infection (Figure 4), suggesting a decreased replication ability of the ΔgigAB mutant. Importantly, the ΔgigAB pMJG120-gigAB complementation strain exhibited a similar trend of survival and replication and comparable CFU at different time points to the wild-type 17978 (Figure 4). These results suggest that gigA and gigB promote macrophage killing evasion of ATCC 17978.
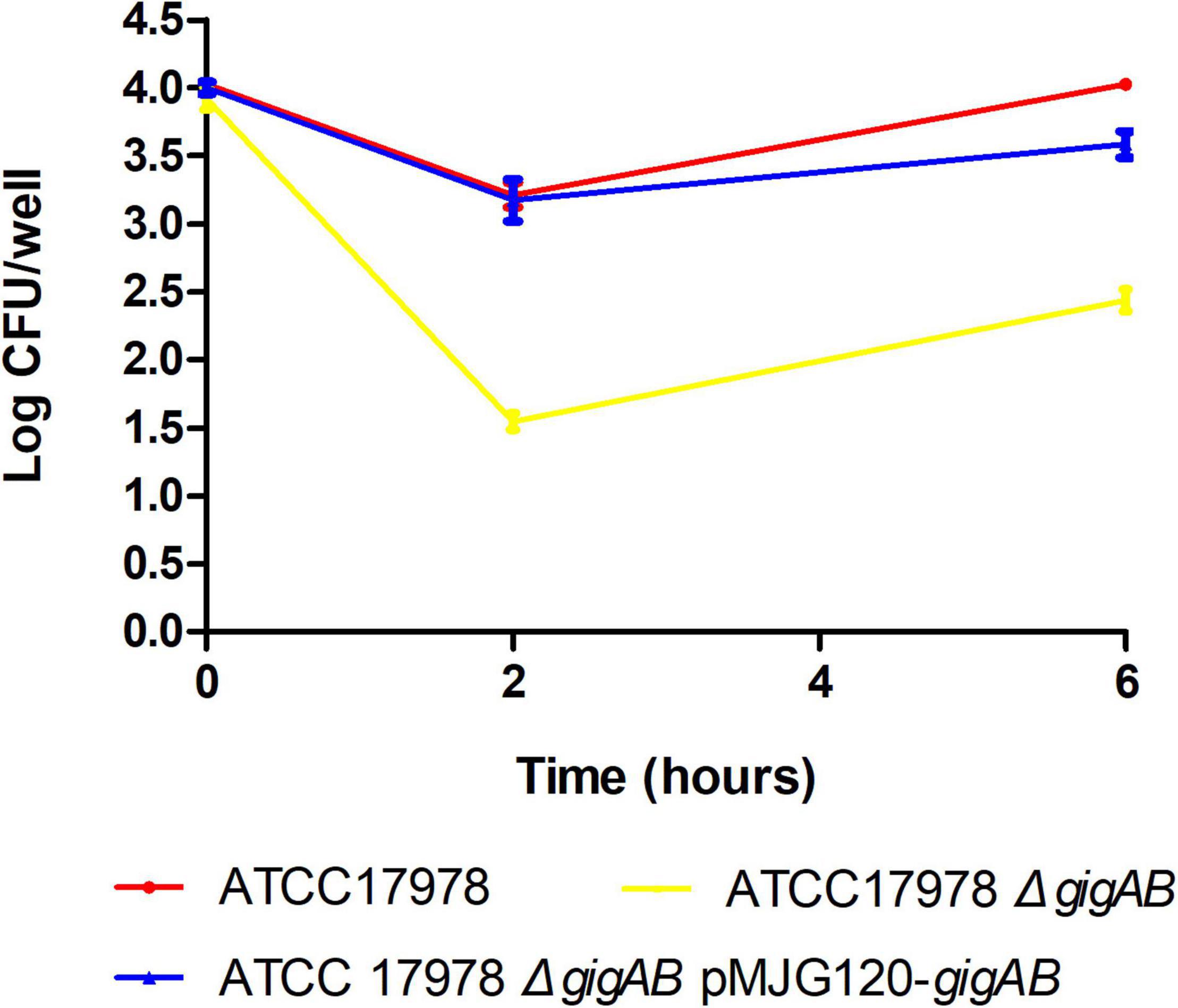
Figure 4. Bone marrow-derived macrophage killing of wild-type and mutant ATCC 17978. Murine bone marrow derived macrophage (BMDMs) were cultured for 24 h, then challenged with wild-type ATCC 17978, ATCC 17978 ΔgigAB, or ATCC 17978 ΔgigAB pMJG120-gigAB at 5 × 104 colony forming unit count (CFU)/mL in the presence of 1 mM IPTG. BMDMs were lysed at 0, 2, or 6 h after challenge and surviving bacteria were quantified via standard plate count method.
gigA/gigB Are Required for Killing G. mellonella
To examine the roles of gigA/gigB in the virulence of ATCC 17978, we performed a G. mellonella killing assay. As shown in Figure 5, inoculation of G. mellonella larvae with wild-type 17978 resulted in a rapid killing of the larvae starting 8 h after inoculation. No killing was observed in the larvae that received ΔgigAB mutant within 48 h after inoculation. Complementation of both gigA and gigB restored the virulence of bacteria to nearly wild-type level. Thus, gigA/gigB are required for the virulence of ATCC 17978. Much like for the growth and temperature studies described above, both the 17978 ΔpstP and 17978 ΔptsP ΔgigAB strains killed larvae with similar kinetics as the wild-type 17978 strain, suggesting that the loss of ptsP restores the virulence defect caused by the ΔgigAB deletion.
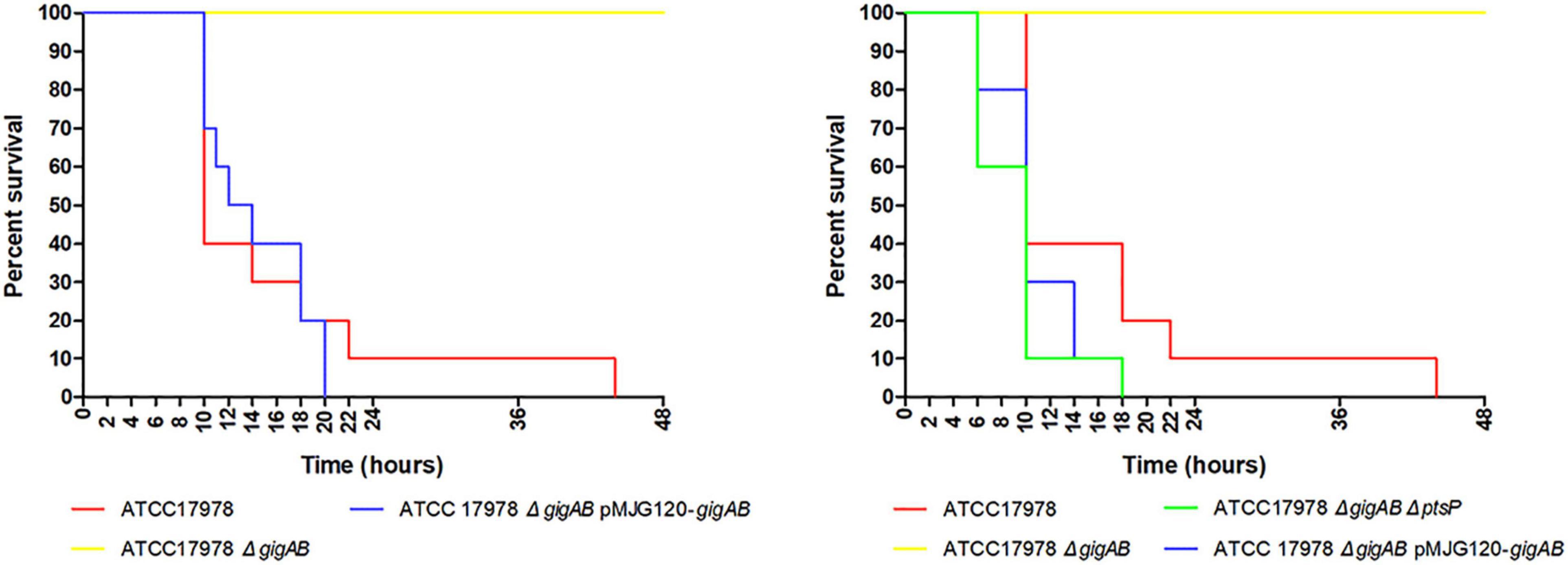
Figure 5. Killing of G. mellonella larvae. G. mellonella larvae were inoculated with 5 × 106 CFU/mL of wild-type ATCC 17978, ATCC 17978 ΔgigAB, or ATCC 17978 ΔgigAB pMJG120-gigAB (n = 10 larvae/group). After injection, larvae were incubated at 37°C. The number of dead larvae was recorded hourly.
Discussion
In this work, we sought to investigate the roles of gigA/gigB in the survival, stress resistance, macrophage killing evasion, and virulence of A. baumannii ATCC 17978 as well as the involvement of ptsP in gigA/gigB signaling. We found that gigA/gigB are important for growth of A. baumannii ATCC 17978, but are not explicitly required for survival of 17978. Indeed, the ΔgigAB mutant strain exhibited growth defects at both 37°C and 50°C compared with the wild-type strain, which was effectively restored by pMJG125-based gigA/gigB complementation in the presence of arabinose or loss of ptsP. Furthermore, gigA/gigB protected 17978 from murine BMDM killing and were required for the virulence of 17978 in G. mellonella.
Bacterial genetics remains an important and powerful tool for revealing the function(s) of specific genes. Efficient construction of gene knockouts or other types of mutations in bacteria often requires modifications of genetic background (Xu and Zhang, 2016). Our preliminary data have shown that the gigA/gigB deletion efficiency in wild-type 17978 was only 4.2%, suggesting that gigA/gigB are critical for the survival of ATCC 17978. We further found that gigA/gigB complementation or ptsP deletion significantly improved gigA/gigB deletion efficiency, suggesting that gigA/B complementation or ptsP deletion compensates for the lack of gigA/B in 17978. This is consistent with our previous study showing that loss of ptsP in the A. baumanniiΔgigA or ΔgigB mutant restores the growth of A. baumannii in G. mellonella larvae (Gebhardt and Shuman, 2017).
When studying genes essential for bacterial growth and/or survival, it is not uncommon to inadvertently isolate clones which harbor compensatory or suppressing mutations that alleviate the phenotype of the particular genes being studied (MacLean and Vogwill, 2014). To exclude the possibility that the ΔgigAB strain acquired such compensatory mutations, we performed whole genome sequencing in multiple independently derived ΔgigAB clones and found that, with the exception of the gigA/gigB deletion, the genome of ΔgigAB clones displayed 100% identity to the genome of the wild-type strain, suggesting that gigA/gigB deletion does not require subsequent compensatory mutations, further confirming that 17978 can survive without gigA/gigB.
When we knocked out gigA/gigB in the genetic background of pMJG125-gigAB conditional strain, we observed that in the absence of arabinose, the colonies of ΔgigAB mutants were smaller than those of the wild-type (Table 2 and Supplementary Figure 1). In addition, ΔgigAB mutant still showed growth defect even after the complementation of gigA/gigB in the absence of arabinose (Figure 1). Of note, arabinose supplementation effectively reversed these effects. We attribute these observations to leaky basal expression from the arabinose-promoter on the multi-copy pMJG125 plasmid.
In addition to the growth in LB medium, we also investigated the roles of gigA/gigB in ATCC 17978 in response to several environmental stresses, including antibiotics, high temperature, Zn2+, and acid. Neither the wild-type nor the ΔgigAB mutant showed significant growth defect to acid stress (Figure 3A). Additionally, we did not observe significant differences for MIC values for various antibiotics (Table 1) and colony formation in the presence of Zn2+ (Figure 3B) between the wild-type and the mutant strain lacking both gigA and gigB. These results suggest that factors other than gigA/gigB regulate the responses of ATCC 17978 to antibiotics and Zn2+ stresses, in contrast to what was previously observed in the more virulent AB5075 strain (Gebhardt et al., 2015; Gebhardt and Shuman, 2017; Blaschke et al., 2018). For example, it has previously been reported that the chromosomally-encoded efflux pump CraA, AdeAB efflux system, and incubation temperature regulate antibiotic resistance of ATCC 17978 (Adams et al., 2018; De Silva et al., 2018; Kroger et al., 2018). Additionally, transcriptional analyses have shown that zinc resistance efflux pumps are responsible for zinc stress response in ATCC 17978, including two cation diffusion facilitator transporters, one heavy metal efflux transporter, and one P-type ATPase (Hassan et al., 2017). That there are differential consequences of gigA/gigB deletion in the AB5075 background (i.e., aminoglycoside and zinc sensitivity) and the ATCC 17978 background (i.e., growth defect under routine culture conditions) suggests that some of the inputs and/or outputs of the GigA/GigB signaling pathway have diverged since the two strains separated; yet, other facets of the pathway, such as growth at elevated temperature and virulence, have remained intact. Further research will be required to understand the molecular mechanisms that underlie the different stress responses that are regulated by GigA/GigB amongst these two isolates.
Of note, our results showed that complementation of the ΔgigAB deletion strain with a plasmid-borne copy of gigA/gigB restored growth on agar plates at high temperature and that a subsequent deletion of ptsP in the ΔgigAB background also alleviated the high temperature growth defect caused by the loss of both gigA and gigB (Figure 2), consistent with previous observations in the AB5075 strain (Gebhardt and Shuman, 2017).
We finally examined the roles of gigA/gigB in evading macrophage phagocytosis and killing G. mellonella larvae. Our data indicate that gigA/gigB are required for 17978 in killing G. mellonella: no larvae died within 48 h after inoculation with ΔgigAB mutant. Additionally, we found that gigA/gigB contribute to the macrophage killing evasion of ATCC 17978, as evidenced by the decreased intracellular live bacteria and the suppressed bacterial replication in murine BMDMs infected with ΔgigAB mutant compared with those infected with the wild-type strain (Figure 5). As it has been reported that RNA chaperone Hfq and superoxide dismutase of ATCC 17978 also play important roles in evading macrophage phagocytosis (Heindorf et al., 2014; Kuo et al., 2017), it will be interesting to examine if the loss of gigA and/or gigB leads to altered expression of these virulence factors.
Conclusion
In this study, we demonstrate that gigA/gigB are important for the growth of A. baumannii strain ATCC 17978, although they are not explicitly required. The ΔgigAB mutant exhibits growth defects at both 37 and 50°C, which can be restored either through gigA/gigB complementation or by loss of ptsP. In contrast to findings in the A. baumannii AB5075 background (Gebhardt and Shuman, 2017), gigA/gigB do not appear to alter the response of strain 17978 to antibiotics or Zn2+ stress. Finally, like strain AB5075, the gigA/gigB genes are required for the virulence traits of strain ATCC 17978 in both resisting killing by macrophage and the G. mellonella infection model.
Data Availability Statement
The datasets presented in this study can be found in online repositories. The names of the repository/repositories and accession number(s) can be found below: http://www.ncbi.nlm.nih.gov/bioproject/, PRJNA738724.
Ethics Statement
The animal study was reviewed and approved by the Ethics Committee of the University of Chicago Medical Center.
Author Contributions
HZ performed the all stepss of experiment, analyzed the experimental data, and drafted the manuscript. MG helped construct A. baumannii ATCC17978 mutants. DC helped perform the BMDM isolation and bacterial killing experiments. YY analyzed whole genome sequences and helped to analyze data. MG and DC revised the manuscript. HS designed the study and revised the manuscript. All authors read and approved the final manuscript.
Funding
This work was supported by a research grant from the National Natural Science Foundation of China (81971897) and a research grant from the Natural Science Foundation of Zhejiang Province LQ20H0006). The funders had no role in the study design, data collection and interpretation, or the decision to submit the work for publication.
Conflict of Interest
The authors declare that the research was conducted in the absence of any commercial or financial relationships that could be construed as a potential conflict of interest.
Publisher’s Note
All claims expressed in this article are solely those of the authors and do not necessarily represent those of their affiliated organizations, or those of the publisher, the editors and the reviewers. Any product that may be evaluated in this article, or claim that may be made by its manufacturer, is not guaranteed or endorsed by the publisher.
Supplementary Material
The Supplementary Material for this article can be found online at: https://www.frontiersin.org/articles/10.3389/fmicb.2021.723949/full#supplementary-material
Footnotes
References
Abisado, R. G., Kimbrough, J. H., Mckee, B. M., Craddock, V. D., Smalley, N. E., Dandekar, A. A., et al. (2021). Tobramycin adaptation enhances policing of social cheaters in Pseudomonas aeruginosa. Appl. Environ. Microbiol. 87:e0002921.
Adams, F. G., Stroeher, U. H., Hassan, K. A., Marri, S., and Brown, M. H. (2018). Resistance to pentamidine is mediated by AdeAB, regulated by AdeRS, and influenced by growth conditions in Acinetobacter baumannii ATCC 17978. PLoS One 13:e0197412. doi: 10.1371/journal.pone.0197412
Blaschke, U., Suwono, B., Zafari, S., Ebersberger, I., Skiebe, E., Jeffries, C. M., et al. (2018). Recombinant production of A1S_0222 from Acinetobacter baumannii ATCC 17978 and confirmation of its DNA-(adenine N6)-methyltransferase activity. Protein Expr. Purif. 151, 78–85. doi: 10.1016/j.pep.2018.06.009
Bolger, A. M., Lohse, M., and Usadel, B. (2014). Trimmomatic: a flexible trimmer for Illumina sequence data. Bioinformatics 30, 2114–2120. doi: 10.1093/bioinformatics/btu170
Danecek, P., Bonfield, J. K., Liddle, J., Marshall, J., Ohan, V., Pollard, M. O., et al. (2021). Twelve years of SAMtools and BCFtools. Gigascience 10:giab008.
De Silva, P. M., Chong, P., Fernando, D. M., Westmacott, G., and Kumar, A. (2018). Effect of incubation temperature on antibiotic resistance and virulence factors of Acinetobacter baumannii ATCC 17978. Antimicrob. Agents Chemother. 62:e01514-17.
Gebhardt, M. J., Gallagher, L. A., Jacobson, R. K., Usacheva, E. A., Peterson, L. R., Zurawski, D. V., et al. (2015). Joint transcriptional control of virulence and resistance to antibiotic and environmental stress in Acinetobacter baumannii. mBio 6, e01660-15.
Gebhardt, M. J., and Shuman, H. A. (2017). GigA and GigB are master regulators of antibiotic resistance, stress responses, and virulence in Acinetobacter baumannii. J. Bacteriol. 199:e00066-17.
Gottig, S., Gruber, T. M., Higgins, P. G., Wachsmuth, M., Seifert, H., and Kempf, V. A. (2014). Detection of pan drug-resistant Acinetobacter baumannii in Germany. J. Antimicrob. Chemother. 69, 2578–2579.
Hassan, K. A., Pederick, V. G., Elbourne, L. D., Paulsen, I. T., Paton, J. C., Mcdevitt, C. A., et al. (2017). Zinc stress induces copper depletion in Acinetobacter baumannii. BMC Microbiol. 17:59. doi: 10.1186/s12866-017-0965-y
Heindorf, M., Kadari, M., Heider, C., Skiebe, E., and Wilharm, G. (2014). Impact of Acinetobacter baumannii superoxide dismutase on motility, virulence, oxidative stress resistance and susceptibility to antibiotics. PLoS One 9:e101033. doi: 10.1371/journal.pone.0101033
Jacobs, A. C., Thompson, M. G., Black, C. C., Kessler, J. L., Clark, L. P., Mcqueary, C. N., et al. (2014a). AB5075, a highly virulent isolate of Acinetobacter baumannii, as a model strain for the evaluation of pathogenesis and antimicrobial treatments. mBio 5:e01076-14.
Jacobs, A. C., Thompson, M. G., Gebhardt, M., Corey, B. W., Yildirim, S., Shuman, H. A., et al. (2014b). Genetic manipulation of Acinetobacter baumannii. Curr. Protoc. Microbiol. 35, 6G.2.1-11.
Karalewitz, A. P., and Miller, S. I. (2018). Multidrug-resistant Acinetobacter baumannii chloramphenicol resistance requires an inner membrane permease. Antimicrob. Agents Chemother. 62:e00513-18.
Kroger, C., Mackenzie, K. D., Alshabib, E. Y., Kirzinger, M. W. B., Suchan, D. M., Chao, T. C., et al. (2018). The primary transcriptome, small RNAs and regulation of antimicrobial resistance in Acinetobacter baumannii ATCC 17978. Nucleic Acids Res. 46, 9684–9698. doi: 10.1093/nar/gky603
Kuo, H. Y., Chao, H. H., Liao, P. C., Hsu, L., Chang, K. C., Tung, C. H., et al. (2017). Functional characterization of Acinetobacter baumannii Lacking the RNA Chaperone Hfq. Front. Microbiol. 8:2068. doi: 10.3389/fmicb.2017.02068
Langmead, B., and Salzberg, S. L. (2012). Fast gapped-read alignment with Bowtie 2. Nat. Methods 9, 357–359. doi: 10.1038/nmeth.1923
Lee, C. R., Lee, J. H., Park, M., Park, K. S., Bae, I. K., Kim, Y. B., et al. (2017). Biology of Acinetobacter baumannii: pathogenesis, antibiotic resistance mechanisms, and prospective treatment options. Front. Cell Infect. Microbiol. 7:55. doi: 10.3389/fcimb.2017.00055
MacLean, R. C., and Vogwill, T. (2014). Limits to compensatory adaptation and the persistence of antibiotic resistance in pathogenic bacteria. Evol. Med. Public Health 2015, 4–12. doi: 10.1093/emph/eou032
Peleg, A. Y., De Breij, A., Adams, M. D., Cerqueira, G. M., Mocali, S., Galardini, M., et al. (2012). The success of acinetobacter species; genetic, metabolic and virulence attributes. PLoS One 7:e46984. doi: 10.1371/journal.pone.0046984
Rosales, C., and Uribe-Querol, E. (2017). Phagocytosis: a fundamental process in immunity. Biomed. Res. Int. 2017:9042851.
Sahl, J. W., Johnson, J. K., Harris, A. D., Phillippy, A. M., Hsiao, W. W., Thom, K. A., et al. (2011). Genomic comparison of multi-drug resistant invasive and colonizing Acinetobacter baumannii isolated from diverse human body sites reveals genomic plasticity. BMC Genomics 12:291. doi: 10.1186/1471-2164-12-291
Schurek, K. N., Marr, A. K., Taylor, P. K., Wiegand, I., Semenec, L., Khaira, B. K., et al. (2008). Novel genetic determinants of low-level aminoglycoside resistance in Pseudomonas aeruginosa. Antimicrob. Agents Chemother. 52, 4213–4219. doi: 10.1128/aac.00507-08
Scribner, M. R., Santos-Lopez, A., Marshall, C. W., Deitrick, C., and Cooper, V. S. (2020). Parallel evolution of tobramycin resistance across species and environments. mBio 11:e00932-20.
Toda, G., Yamauchi, T., Kadowaki, T., and Ueki, K. (2021). Preparation and culture of bone marrow-derived macrophages from mice for functional analysis. STAR Protoc 2:100246. doi: 10.1016/j.xpro.2020.100246
WHO (2017). Global Priority List of Antibiotic-Resistant Bacteria to Guide Research, Discovery and Development of New Antibiotics. Geneva: World Health Organization.
Keywords: Acinetobacter baumannii, nitrogen phosphotransferase system, Galleria mellonella, gigA, gigB, ptsP
Citation: Zhou H, Gebhardt MJ, Czyz DM, Yao Y and Shuman HA (2021) The gigA/gigB Genes Regulate the Growth, Stress Response, and Virulence of Acinetobacter baumannii ATCC 17978 Strain. Front. Microbiol. 12:723949. doi: 10.3389/fmicb.2021.723949
Received: 11 June 2021; Accepted: 16 July 2021;
Published: 04 August 2021.
Edited by:
Remy A. Bonnin, Université Paris-Saclay, FranceReviewed by:
William T. Doerrler, Louisiana State University, United StatesPaul Stokes Hoffman, University of Virginia, United States
Copyright © 2021 Zhou, Gebhardt, Czyz, Yao and Shuman. This is an open-access article distributed under the terms of the Creative Commons Attribution License (CC BY). The use, distribution or reproduction in other forums is permitted, provided the original author(s) and the copyright owner(s) are credited and that the original publication in this journal is cited, in accordance with accepted academic practice. No use, distribution or reproduction is permitted which does not comply with these terms.
*Correspondence: Hua Zhou, emhvdWh1YTFAemp1LmVkdS5jbg==
†Present address: Michael J. Gebhardt, Division of Infectious Diseases, Boston Children’s Hospital, Harvard Medical School, Boston, MA, United States