- 1University of Reims Champagne-Ardenne, Résistance Induite et Bioprotection des Plantes Research Unit, EA 4707, INRAE USC 1488, SFR Condorcet FR CNRS 3417, Reims, France
- 2Instituto de Ciencias de la Vid y del Vino, Consejo Superior de Investigaciones Científicas, Universidad de la Rioja, Gobierno de La Rioja, Logroño, Spain
- 3Instituto Agroforestal Mediterráneo, Universitat Politècnica de València, Valencia, Spain
Grapevine trunk diseases (GTDs) are a big threat for global viticulture. Without effective chemicals, biocontrol strategies are developed as alternatives to better cope with environmental concerns. A combination of biological control agents (BCAs) could even improve sustainable disease management through complementary ways of protection. In this study, we evaluated the combination of Bacillus subtilis (Bs) PTA-271 and Trichoderma atroviride (Ta) SC1 for the protection of Chardonnay and Tempranillo rootlings against Neofusicoccum parvum Bt67, an aggressive pathogen associated to Botryosphaeria dieback (BD). Indirect benefits offered by each BCA and their combination were then characterized in planta, as well as their direct benefits in vitro. Results provide evidence that (1) the cultivar contributes to the beneficial effects of Bs PTA-271 and Ta SC1 against N. parvum, and that (2) the in vitro BCA mutual antagonism switches to the strongest fungistatic effect toward Np-Bt67 in a three-way confrontation test. We also report for the first time the beneficial potential of a combination of BCA against Np-Bt67 especially in Tempranillo. Our findings highlight a common feature for both cultivars: salicylic acid (SA)-dependent defenses were strongly decreased in plants protected by the BCA, in contrast with symptomatic ones. We thus suggest that (1) the high basal expression of SA-dependent defenses in Tempranillo explains its highest susceptibility to N. parvum, and that (2) the cultivar-specific responses to the beneficial Bs PTA-271 and Ta SC1 remain to be further investigated.
Introduction
Global environmental changes promote the incidence of plant diseases by increasing the pathogen pressure or make the plants more susceptible to them (O’Brien, 2017; Velásquez et al., 2018). Grapevine trunk diseases (GTDs) are among the most important groups of grapevine diseases all over the world, creating a big concern in all wine-producing countries (Mondello et al., 2018). Attacking the plant perennial part and leading inevitably to the short- or long-term death of vines, the pathogens responsible of GTDs are described as very injurious for the sustainability of the winemaking industry (Hofstetter et al., 2012). As main restrictors for viticulture, GTDs can lead to high economic losses, less table grape for consumers, and social and environmental disturbances (O’Brien, 2017). Both young and mature vines are affected by GTDs, even as nursery staged plants, reducing both productivity and longevity of the vineyard, thereby causing massive economic losses (Gramaje and Armengol, 2011).
Botryosphaeria dieback (BD) is one of the most significant GTDs, triggerable by more than 25 distinct species of Botryosphaeriaceae including the aggressive Neofusicoccum parvum (Úrbez-Torres, 2011; Billones-Baaijens and Savocchia, 2019; Reis et al., 2019; Larach et al., 2020). Symptomatic plants develop a low or apoplectic dieback phenotype, including a low budburst rate, a poor vegetative development, external canker, and internal longitudinal necrotic lesions that can lead to a full dead branch (Larignon et al., 2001, 2009; Larignon, 2004; Billones-Baaijens and Savocchia, 2019; Larach et al., 2020). Susceptibility to BD pathogens also differs between cultivars (Travadon et al., 2013; Fontaine et al., 2016a; Chacon et al., 2020; Claverie et al., 2020).
Due to the undetermined period of latency within the vines (asymptomatic state), early detection and management of GTDs remain presently a challenge in both nursery and vineyard, and only few preventives, but no curative methods, are available. Indeed, few chemicals are applied on pruning wounds in vineyards to prevent dissemination of the conidia of fungal pathogens (Sosnowski et al., 2004), preferring cultural practices in vineyard (Mondello et al., 2018) and sanitation methods (Gramaje and Armengol, 2011; Gramaje et al., 2018). However, these kinds of treatments cannot eradicate the pathogens once established in a vineyard (Calzarano et al., 2004; Gramaje and Armengol, 2011). An interesting alternative and complement to the previously cited GTDs control methods in vineyard is the use of biological control agents (BCAs) as reported by Mondello et al. (2018). For instance, several microorganisms have already been evaluated, both in vitro and in planta against BD pathogens (Hunt et al., 2001; Di Marco et al., 2004; John et al., 2004; Compant et al., 2013; Compant and Mathieu, 2017; Trotel-Aziz et al., 2019). Among them there are bacterial BCAs such as Pseudomonas, Bacillus, and Enterobacter species, and fungal BCAs such as several Fusarium and Trichoderma species (Mondello et al., 2018). Some of them, such as Trichoderma atroviride, Trichoderma harzianum, Bacillus subtilis, and Bacillus amyloliquefaciens, are already commercialized against some GTDs pathogens, or against other hemibiotrophic and necrotrophic pathogens including Botrytis cinerea, Fusarium oxysporum, or many others (Elad, 2000; Schmidt and Panstruga, 2011; Kuzmanovska et al., 2018; Thambugala et al., 2020; Alfiky and Weisskopf, 2021).
To date, Trichoderma species are the most used fungal-based BCA in viticulture (Harman, 2006; Muckherjee et al., 2012; Waghunde et al., 2016) and have been also widely investigated as BCA against GTDs (Di Marco et al., 2002, 2004; John et al., 2008; Halleen et al., 2010: Berbegal et al., 2020; Martínez-Diz et al., 2021a,b). Trichoderma spp. are described to directly antagonize GTD pathogen aggressiveness by competition for nutrients and space, mycoparasitism, cell-wall degrading enzymes, and antibiosis (Harman, 2006; Van Wees et al., 2008; Vinale et al., 2008; Pieterse et al., 2014; Waghunde et al., 2016). Trichoderma spp. have also been described as plant growth and defense stimulators (Harman, 2006; Van Wees et al., 2008; Vinale et al., 2008; Pieterse et al., 2014; Waghunde et al., 2016). Among Trichoderma spp., T. atroviride SC1 was shown to strongly reduce the infections caused by some GTD pathogens in nurseries and established vineyards at the registered dose rate of 2 g/L, equivalent to the density of 2 × 1010 conidia/L recommended by the commercial product (Pertot et al., 2016; Berbegal et al., 2020; Martínez-Diz et al., 2021a). Bacillus strains are another group of microorganisms extensively studied as BCA and reported to directly and indirectly protect plants against pathogens with different lifestyles (Magnin-Robert et al., 2007; Trotel-Aziz et al., 2008; Nguyen et al., 2020), including the GTD hemibiotrophic pathogens (Schmidt et al., 2001; Halleen et al., 2010; Rezgui et al., 2016; Kotze et al., 2019; Trotel-Aziz et al., 2019). A broad range of beneficial molecules are produced or encoded by the genome of Bacillus spp., both to induce or elicit plant defenses (as with phytohormones precursors, lipopolysaccharides, siderophores, etc.) and to directly compete, antagonize, or alter plant pathogens or their aggressiveness (Kloepper et al., 2004; Ongena and Jacques, 2008; Leal et al., 2021). Among Bacillus spp., B. subtilis is one of the most frequently tested against GTDs (Mondello et al., 2018), and B. subtilis PTA-271 has shown promising results in reducing infections caused by the aggressive strain N. parvum Bt67 (Trotel-Aziz et al., 2019). Literature also reports that the combination of two or more BCAs can improve the management of plant diseases (Weller, 1988; Guetsky et al., 2002; El-Tarabily, 2006; Yobo et al., 2011; Magnin-Robert et al., 2013), probably due to additive or synergistic effects of combined mechanisms in a complex changing environment (Meyer and Roberts, 2002).
Beneficial microbial interactions conferred by BCA lead to induced systemic resistance (ISR) in the plant, giving it greater protection to pathogens in spatially separated parts of the plant (Alström, 1991; Van Peer et al., 1991; Wei et al., 1991; De Vleesschauwer and Höfte, 2009). ISR is associated with an early, strong, and rapid activation of plant defenses upon pathogen infection, a phenomenon known as the priming state (Conrath et al., 2001, 2015; Pieterse et al., 2014). Among the BCA-induced defense responses, the most relevant are jasmonate (JA)- and ethylene (ET)-dependent ones, described as useful defenses against necrotrophs (Pieterse et al., 2001, 2014; Verhagen et al., 2004; Van der Ent et al., 2009; Niu et al., 2011; Nie et al., 2017). However, Niu et al. (2011) also reported that some BCAs may mediate ISR in a salicylic acid (SA)-dependent manner. In brief, the diversity of BCA ways of protection may depend on both the BCA and the pathogen, but also on the plant and even the cultivar (Mutawila et al., 2011; Pacifico et al., 2019; Nguyen et al., 2020; Stempien et al., 2020).
In this study, we evaluated the effect of combining a potential BCA, B. subtilis PTA-271 (thereafter Bs PTA-271), and a BCA-commercial product containing T. atroviride SC1 (thereafter Ta SC1), on the protection of two distinct grapevine cultivars, Chardonnay and Tempranillo, potentially showing distinct susceptibilities to GTDs. The pathogen selected was N. parvum Bt67 (thereafter Np-Bt67), described as a very aggressive pathogen associated to BD. As each BCA has already been recognized as beneficial to at least one cultivar (Trotel-Aziz et al., 2019; Berbegal et al., 2020; Martínez-Diz et al., 2021a), their beneficial effect was additionally investigated on the other cultivar, as single BCA and in dual combination of two BCAs. To compare the two BCAs, densities were aligned to the density optimized for Bs PTA-271 with Chardonnay rootlings. After looking for the protective capacity of BCAs in planta, their ways of action leading to protection were further explored. Thus, the indirect and direct benefits offered by each BCA and their combination were investigated, focusing on both grapevine immunity and the direct beneficial or detrimental physical interplays among the microorganisms in vitro (Bs PTA-271, Ta SC1, and Np-Bt67).
Materials and Methods
Plant Material and Growth Conditions
Three-node-long cuttings of grapevine Vitis vinifera L. cv. Tempranillo (clone RJ-26) and cv Chardonnay (clone 7535) were provided by Viveros Villanueva nursery (Navarra, Spain) and Pommery’s vineyards in Reims (France), respectively. Tempranillo cuttings were surface-sterilized for 6 h in a 0.05% cryptonol (8-hydroxyquinoline sulfate) solution, waxed and stored at 4°C in a cold chamber for 3 weeks, and then rehydrated with 0.05% cryptonol solution overnight. Chardonnay cuttings were directly surface-sterilized with 0.05% cryptonol solution overnight. Cuttings of both cv were then rooted as described by Lebon et al. (2005), using an indole-3-butyric acid (1 g/L) solution before being placed by 15 in 350-ml pots containing the soil Sorexto (horticultural soil M4600, Grenoble, France) in a culture chamber (24/20°C day/night, 55–65% relative humidity day/night, and 16-h photoperiod at 400 μmol/m2/s). They were watered three times a week. Only rootlings that have developed roots (30% rooting rate in 15 weeks) were kept for further experiments and transferred to individual 200-ml pots with the same culture conditions.
Biocontrol Agent’s Growth and Plant Treatments
Bacillus subtilis PTA-271
Bacillus subtilis PTA-271 (GenBank Nucleotide EMBL Accession No. AM293677 for 16S rRNA and DDBJ/ENA/GenBank Accession No. JACERQ000000000 for the whole genome) was isolated in 2001 from the rhizosphere of healthy Chardonnay grapevines (V. vinifera L. cv. Chardonnay) from a vineyard located in Champagne (Marne, France) (Trotel-Aziz et al., 2008; Leal et al., 2021). Bacterial growth started by adding 100 μl of glycerol stock suspension to sterile Luria Bertani (LB) medium and incubating at 28°C with agitation (100 rpm). Experiments were performed when the bacterial culture is at the exponential growth phase. After centrifugation (5,000 g, 10 min), the pellet was washed once with a sterile 10 mM MgSO4 medium and resuspended in a same MgSO4 medium. Bacterial density was measured by spectrophotometry at 450 and 650 nm, and the mean density was adjusted with a sterile MgSO4 medium before treatment according to Trotel-Aziz et al. (2019). The bacterial suspension was applied twice by drenching the soil at the root level of rootlings at a final density of 108 CFU/g soil. Inoculations were carried out when rootlings were 16 weeks old (considered as day 0) and 2 weeks later (day 15) as indicated in Figure 1. Control rootlings were similarly drenched twice with MgSO4 solution (Figure 1).
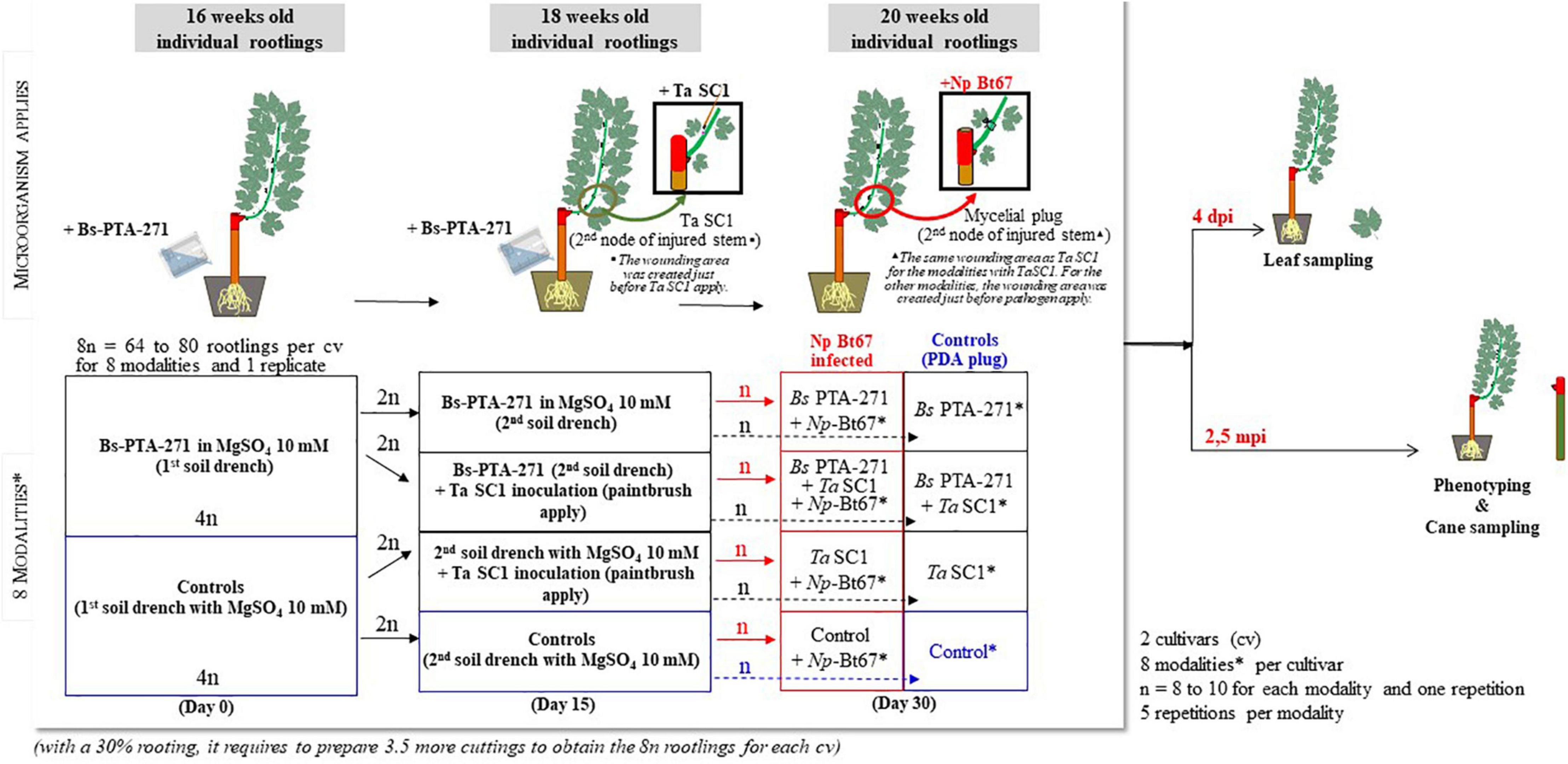
Figure 1. Diagram showing the Bacillus subtilis PTA-271 and Trichoderma atroviride SC1 inoculations, Neofusicoccum parvum strain Np-Bt67 infection, and sample collection process. *Modalities: Control, Bs PTA-271, Ta SC1, Bs PTA-271 + Ta SC1, Control + Np Bt67, Bs PTA-271 + Np Bt67, Ta SC1 + Np Bt67, Bs PTA-271 + Ta SC1 + Np Bt67.
Trichoderma atroviride SC1
Trichoderma atroviride SC1 (Vintec®, Belchim Crop Protection, Bi-PA; 1010 conidia per gram of formulated product) was suspended in water at 108 CFU/ml to compare the effect of each BCA at an equal density. In order to also take advantage of an eliciting effect, the Ta SC1 fungal suspension was applied once with a paintbrush to the second node of the previously wounded lignifying stem (5 mm diameter and 1 mm deep; made just before Ta SC1 apply) of 18-week-old rootlings (Figure 1). The inoculation site was immediately covered with parafilm (day 15).
Pathogen Strain and Growth
Neofusicoccum parvum strain Bt67 isolated from Portuguese vineyards (Estremadura region) is inscribed in the HIA collection (Lisbon University, Portugal). This fungus was maintained on potato dextrose agar (PDA, Sigma, Saint-Quentin-Fallavier, France) plates and stored at 4°C (Trotel-Aziz et al., 2019). The resulting mycelium was plated on PDA medium and incubated in the dark at 22°C for 7 days before inoculation.
Pathogen Inoculation to Plants, Quantification of Disease Symptoms, and Re-isolation of the Pathogen
Half of the 20-week-old rootlings that were treated with Bs PTA-271 (day 0 and day 15) and/or Ta SC1 (day 15) were further infected with the pathogen at the wounding area with a 3-mm-diameter mycelial plug from a 7-day-old culture of Np-Bt67 strain (day 30), thus at distinct time points (days 0–30) as summarized in Figure 1. The inoculation site was then covered with moist hydrophilic cotton before sealing with parafilm. The experiment was composed of five repetitions for each modality (treatment), with 8–10 replicate plants per treatment (Figure 1). To confirm that lesions were due to pathogen infection and not to the injury, the control plants were also injured and inoculated with sterile 3-mm PDA plugs (Figure 1). Rootlings, namely, “control,” are those that are neither treated with BCA nor infected with the pathogen. After inoculation, vines were kept in the same culture chamber and BD symptoms were assessed at 2.5 months post-inoculation (mpi) (Figure 1). Disease symptoms were evaluated as described by Trotel-Aziz et al. (2019) by quantifying the percentage of the full dead shoots from inoculated rootlings (Figure 2A, “Full dieback”) and by measuring both the external canker area and the internal necrosis length of the other lignified shoots (Figures 2B,C, respectively). To check the success of the infection and the lack of contaminations, re-isolation of pathogen was performed as described by Pinto et al. (2018), by quickly passing the infected stems onto the flame, then removing the top of the necrotic zone with a scalpel, before plating seven small pieces of tissue per plant onto PDA plates. Plates were then incubated at 28°C for at least 7 days. For every repetition of the experiments, re-isolations were performed with seven of the infected rootlings per infected combination (i.e., Np, Bs + Np, Ta + Np, and Bs + Ta + Np) and each replicate, and one negative control rootling per non-infected combination (i.e., Control, Bs, Ta, and Bs + Ta).
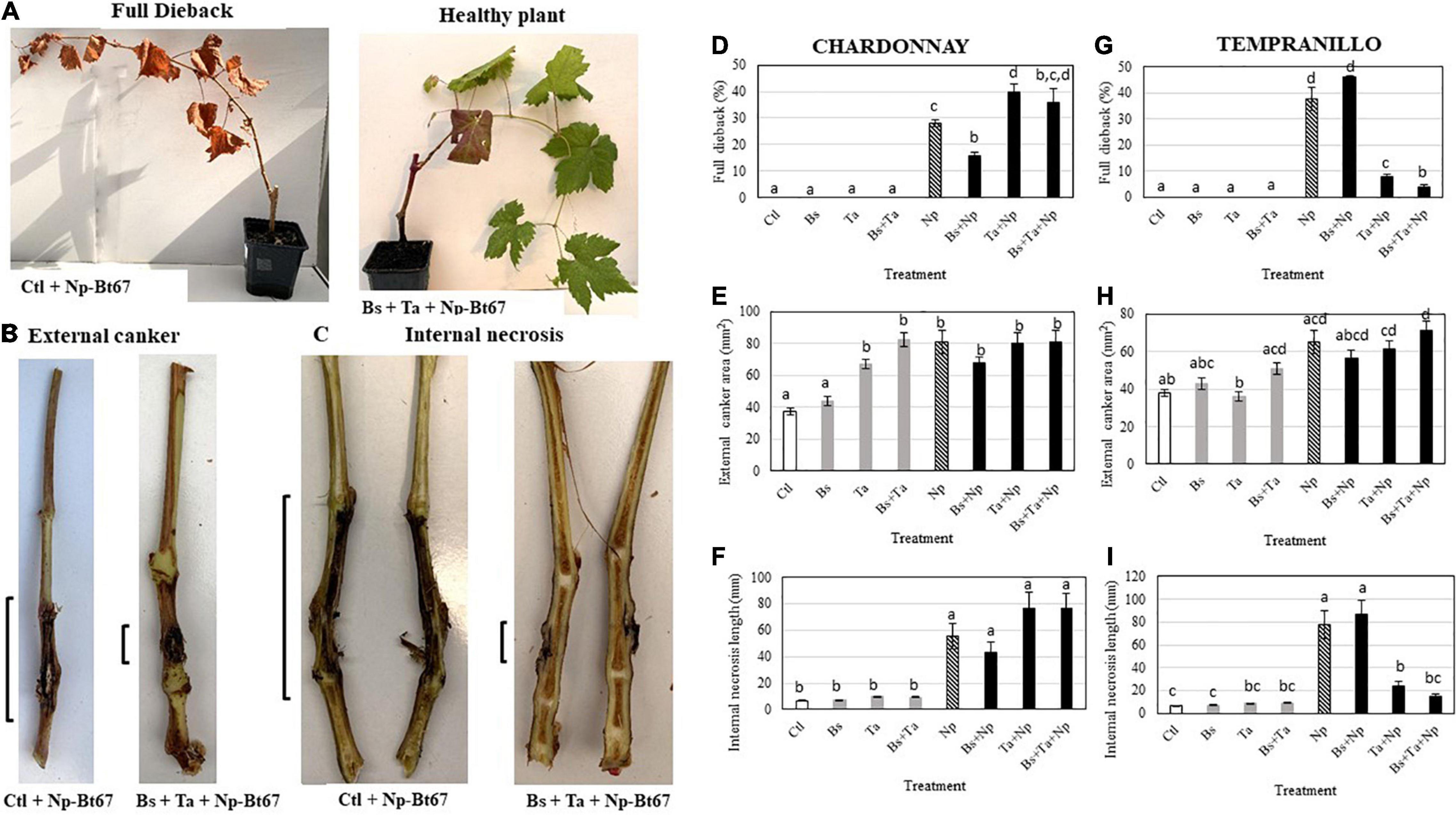
Figure 2. The beneficial combination of Bacillus subtilis PTA-271 and Trichoderma atroviride SC1 attenuates the Botryosphaeriaceae dieback symptoms induced in Chardonnay (D–F) and Tempranillo (G–I) rootlings by the Neofusicoccum parvum strain Np-Bt67. One-month pretreated grapevine rootlings with PTA-271, Ta SC1, and both BCA (Bs, Ta, and Bs + Ta) and non-treat plants (Ctl) were inoculated with pathogen mycelium (Np). Non-infected plants were inoculated with sterile medium without pathogen (Control). Compared to healthy asymptomatic rootlings (A, right), the infected symptomatic rootlings showed the typical Botryosphaeria dieback symptoms: full dieback percentage (A left, E), stem external canker (B,E,H) and stem internal necrosis (C,F,I) that were photographed (A–C) and quantified (D–I) at 2.5 months post-inoculation. Data are means ± standard deviation (SD) for at least three independent experiments with 10 biological replicates per treatment. Vertical bars with different letters are significantly different (multiple comparison procedures with Tukey’s test, p < 0.05).
Direct Confrontation Tests With Biocontrol Agents and Pathogen
Antagonism among microorganisms was checked in vitro, using B. subtilis PTA-271, T. atroviride SC1, and N. parvum Np-Bt67, in dual or three-way confrontation tests on PDA plates (9 mm diameter). Bs PTA-271 grown in LB medium and Ta SC1 resuspended in sterilized water were both used at 108 CFU/ml (5 μl drop), while a 7-day-old mycelium plug (3 mm) was used for the pathogen. Three types of direct confrontation were performed: (A) the pathogen/BCA combinations were plated at the same time, but at distinct areas (i.e., 5 cm away from each other); (B) one or two isolates (Bs PTA-271, Ta SC1, or Np-Bt67) were plated 48 h before the other(s), and at distinct areas; and (C) isolates were plated simultaneously and at the center of the plate. Controls containing one single isolate (Bs PTA-271, Ta SC1, or Np-Bt67) were also made, either on the side of the plate or at the center. All plates were incubated in the dark at 28°C for at least 11 days and photographed daily. Antagonistic effect was characterized by an inhibition zone around the BCA and/or the pathogen. Since the first kind of confrontation (A) added no more information compared to the others (B and C), it was not shown in this study. The experiment was conducted twice with three replicate plates per treatment, and the area occupied by each microorganism was measured daily using ImageJ software (Rueden et al., 2017), based on a reference distance common to all images.
RNA Extraction and Quantitative Reverse-Transcription Polymerase Chain Reaction Analysis
From the in planta assays with rootlings, leaf samples were collected 4 days post-inoculation of pathogen (dpi), ground in liquid nitrogen, and then stored at −80°C. RNA was extracted from powdered 40 leaves of 8 rootlings per replicate of each modality. Total RNA was extracted from 50 mg of leaf powder with Plant RNA Purification Reagent according to manufacturer instructions (Invitrogen, Pontoise, France), and DNase treated as described by the manufacturer’s instructions (RQ1 RNase-Free DNase, Promega). RNA quality was checked by agarose gel electrophoresis, and total RNA concentration was measured at 260 nm for each sample using the NanoDrop One spectrophotometer (Ozyme) and adjusted to 100 ng μl–1. First-strand cDNA was synthesized from 150 ng of total RNA using the Verso cDNA synthesis kit (Thermo Fisher Scientific, Inc., Waltham, MA, United States). Polymerase chain reaction (PCR) conditions were the ones described by Gruau et al. (2015). Quantitative reverse-transcription polymerase chain reaction (qRT-PCR) was performed with Absolute Blue qPCR SYBR Green ROX Mix according to manufacturer instructions (Thermo Fisher Scientific, Inc., Waltham, MA, United States), in a BioRad C1000 thermocycler using the Bio-Rad manager software CFX96 Real-Time PCR (BioRad, Hercules, CA, United States). A set of six defense-related genes, selected for their responsiveness to pathogen or priming state induced by beneficial microorganisms (Trotel-Aziz et al., 2019), was tracked by qRT-PCR using specific primers (Table 1). Quantitative RT-PCR reactions were carried out in duplicate in 96-well plates in a 15-μl final volume containing Absolute Blue SYBR Green ROX mix including Taq polymerase ThermoPrime, dNTPs, buffer, and MgCl2 (Thermo Fisher Scientific, Inc., Waltham, MA, United States), 280 nM forward and reverse primers, and 10-fold diluted cDNA according to the manufacturer’s protocol. Cycling parameters were 15 min of Taq polymerase activation at 95°C, followed by 40 two-step cycles composed of 10 s of denaturation at 95°C and 45 s of annealing and elongation at 60°C. Melting curve assays were performed from 65 to 95°C at 0.5°C s–1, and melting peaks were visualized to check amplification specificity. EF1 and 60SRP genes were used as references and experiments were repeated five times. Relative gene expression was determined with the formula fold induction: 2(−11Ct), where 11Ct = [Ct TG (US) − Ct RG (US)] − [Ct TG (RS) − Ct RG (RS)], where Ct is cycle threshold, Ct value is based on the threshold crossing point of individual fluorescence traces of each sample, TG is target gene, RG is reference gene, the US is an unknown sample, and RS is reference sample. Integration of the formula was performed by the CFX Manager 3.1 software (Bio-Rad). Although the genes analyzed were considered significantly up- or downregulated when changes in their expression were >2-fold or <0.5-fold, respectively, we still performed a statistical analysis. Control samples for the rootlings model are cDNA from leaves of rootlings untreated with BCA and inoculated with sterile PDA plugs (1× expression level).
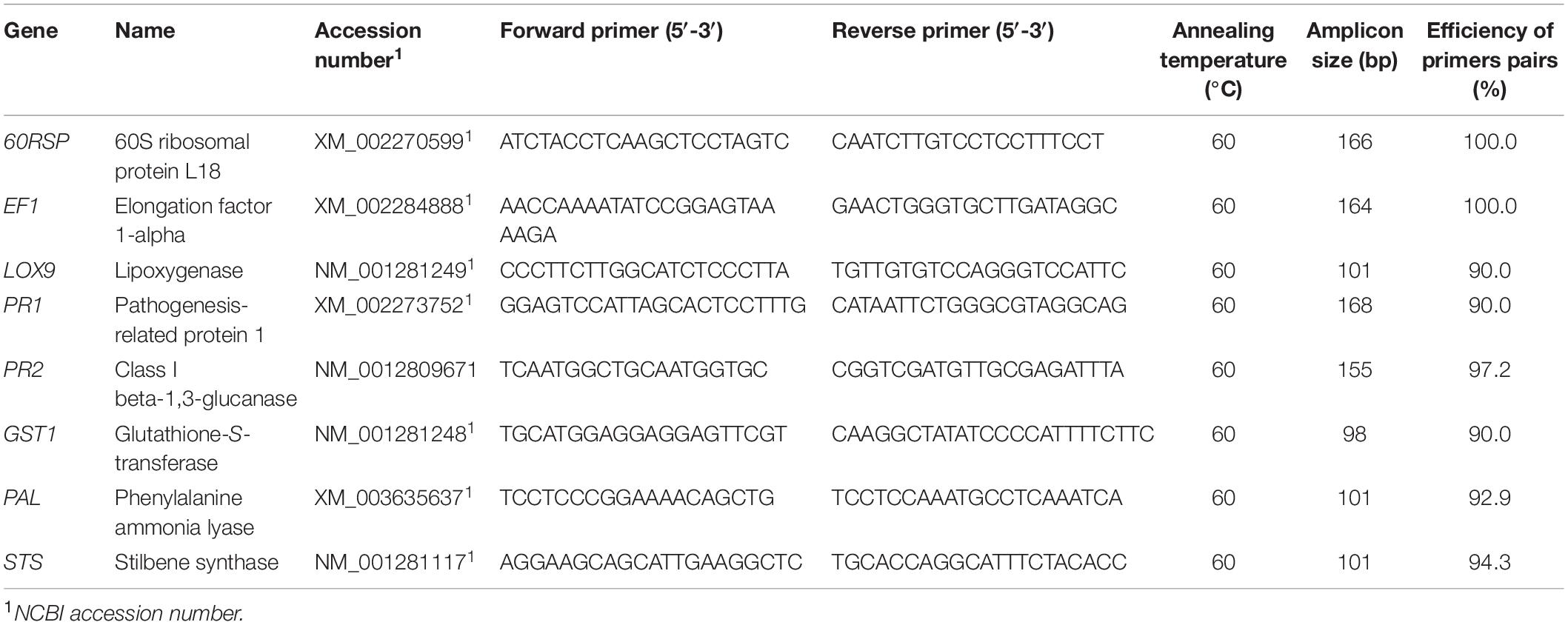
Table 1. Primer sequences used for qRT-PCR analysis of defense-related genes (Trotel-Aziz et al., 2019).
Statistical Analysis
Data of canker area and length of external and internal necrosis of the stems were obtained by the analysis of photos using ImageJ software (Rueden et al., 2017). Statistical analyses were carried out using all the vines of three replicates among five for each modality with RStudio software (Horton and Kleinman, 2015). For modality significance, mean values were compared by Tukey’s test (p < 0.05). Results of confrontation tests are from one representative repetition out of two showing the same trends. Statistical analyses were carried out using the SigmaStat 3.5 software. For treatment effect, mean values were compared by Tukey’s test (p < 0.05). Results of gene expression by qRT-PCR analysis correspond to means ± SEM deviation from three representative repetitions out of five showing the same trends. Statistical analyses were carried out using the XLSTAT 2021.1.1 5 software (Addinsoft, Paris, France). For treatment effect, mean values were analyzed using one-way analysis of variance (ANOVA). When differences in the means were significant, Fisher’s LSD post hoc test (α = 0.1) was applied to determine which treatments were significantly different from others.
Results
Effects of Bacillus subtilis PTA-271 and Trichoderma atroviride SC1 on Two Cultivars Infected With Neofusicoccum parvum Bt67
In control Chardonnay infected with the pathogen, the results of infection showed a rate of 28 ± 1.24% for dead shoot (full dieback), of 80.6 ± 7.35 mm2 for external canker size, and of 55.4 ± 9.44 mm for internal necrosis length (see Np-Bt67 in Figures 2D–F), while in Tempranillo, they reached 37.5 ± 4.56%, 65.2 ± 6.23 mm2, and of 77.8 ± 11.95 mm, respectively (see Np-Bt67 in Figures 2G–I).
In BCA-treated Chardonnay rootlings then infected with the pathogen, the results of the biocontrol assays showed that Bs-PTA-271-pretreated plants presented a significant lower number of plants with full dieback (by approximately 45%) than the infected control (Figure 2D). Infected plants pretreated with Ta SC1 did not reduce the full dieback development compared to infected control plants, while infected plants pretreated with both BCAs showed a great variability in the development of full dieback symptoms. Similarly, the external canker area (Figure 2E) and the internal necrosis length of infected Chardonnay (Figure 2F) were solely slightly reduced in Bs-PTA-271-pretreated rootlings (by 16 and 22%, respectively), but insignificantly (Figure 2E). In contrast, necrosis length was increased in Ta SC1 and both BCAs pretreated plants compared to infected control, although non-significant (Figure 2F).
In BCA-treated Tempranillo rootlings then infected with the pathogen, the results of the biocontrol assays showed that Ta-SC1- and combined-BCA-pretreated plants showed a significant lower number of full dieback (by approximately 80 and 91%, respectively) and length of stem internal necrosis (by approximately 70 and 81%, respectively) than the infected control (Figures 2G,I, respectively). In contrast, infected plants pretreated with Bs PTA-271 showed that neither full dieback development nor internal stem necrosis reduced, compared to infected control plants. Looking at the external canker area (Figure 2H), none of the treatments with Bs-PTA- 271-, Ta- SC1-, and combined-BCA-pretreated plants, consecutively infected, induced any significant difference with the infected control. Therefore, external canker may not appear as a relevant indicator for Np dieback with this experimental model, for both Tempranillo and Chardonnay.
Re-isolations of the pathogen confirmed that (1) there was no background infection elsewhere than in the artificially infected rootlings, satisfying thus the Koch’s postulates, and that (2) the pathogen was still alive in both dead and living stems of plants, as well as in infected plants pretreated or not with BCA. From all infected plants, the pathogen was successfully isolated with a percentage of success >90% that indicated no fungicidal effect from BCA toward Np-Bt67.
Effects of Biological Control Agents on the Basal Defense of Chardonnay and Tempranillo
The ability of Bs PTA-271 or Ta SC1 or both BCAs to enhance grapevine immunity was addressed in leaves of control rootlings. Six selected defense genes were targeted by qRT-PCR: the lipoxygenase LOX9 involved in oxylipin synthesis and described as dependent to JA/ET (Hamiduzzaman et al., 2005; Naznin et al., 2014); PR1 described to be regulated by SA(Dufour et al., 2013; Naznin et al., 2014; Caarls et al., 2015); the β-1,3-glucanase PR2 described to be regulated by various phytohormones such as SA, JA, and ET (Liu et al., 2010); the glutathione-S-transferase GST1 putatively involved in the detoxification process; the phenylalanine ammonia-lyase PAL catalyzing the first step in the phenylpropanoid pathway; and the stilbene synthase STS involved in the synthesis of phytoalexins. Since BCAs were not detected in leaves (not shown) where defenses were induced, the induction of plant defense by BCAs is systemic.
Data showed some differences in the level of expression of the basal defense genes between the greenhouse cultivars (Figure 3), despite the fact that rootlings all grew in the same chamber of the greenhouse with similar culture conditions. The cultivar Chardonnay exhibited a weak constitutive expression of targeted defense genes (Figure 3A) compared to Tempranillo (Figure 3B). In Chardonnay (Figure 4 and Supplementary Figure 1A), the application of Bs PTA-271 at root level induced a 2.8-fold increase of PR1 and PR2 expression in leaves, while the application of Ta SC1 at stem level did not induce any consistent changes in the expression of these same defense genes in leaves. Interestingly, the application of both BCAs induced the expression of the greatest number of targeted genes in the leaves: a 2.6-fold expression of LOX9 and a 6.6- or 6.8-fold expression of PR2 and STS, respectively. Bs PTA-271, alone or together with Ta SC1, may thus act as a priming stimulus for Chardonnay cultivar.
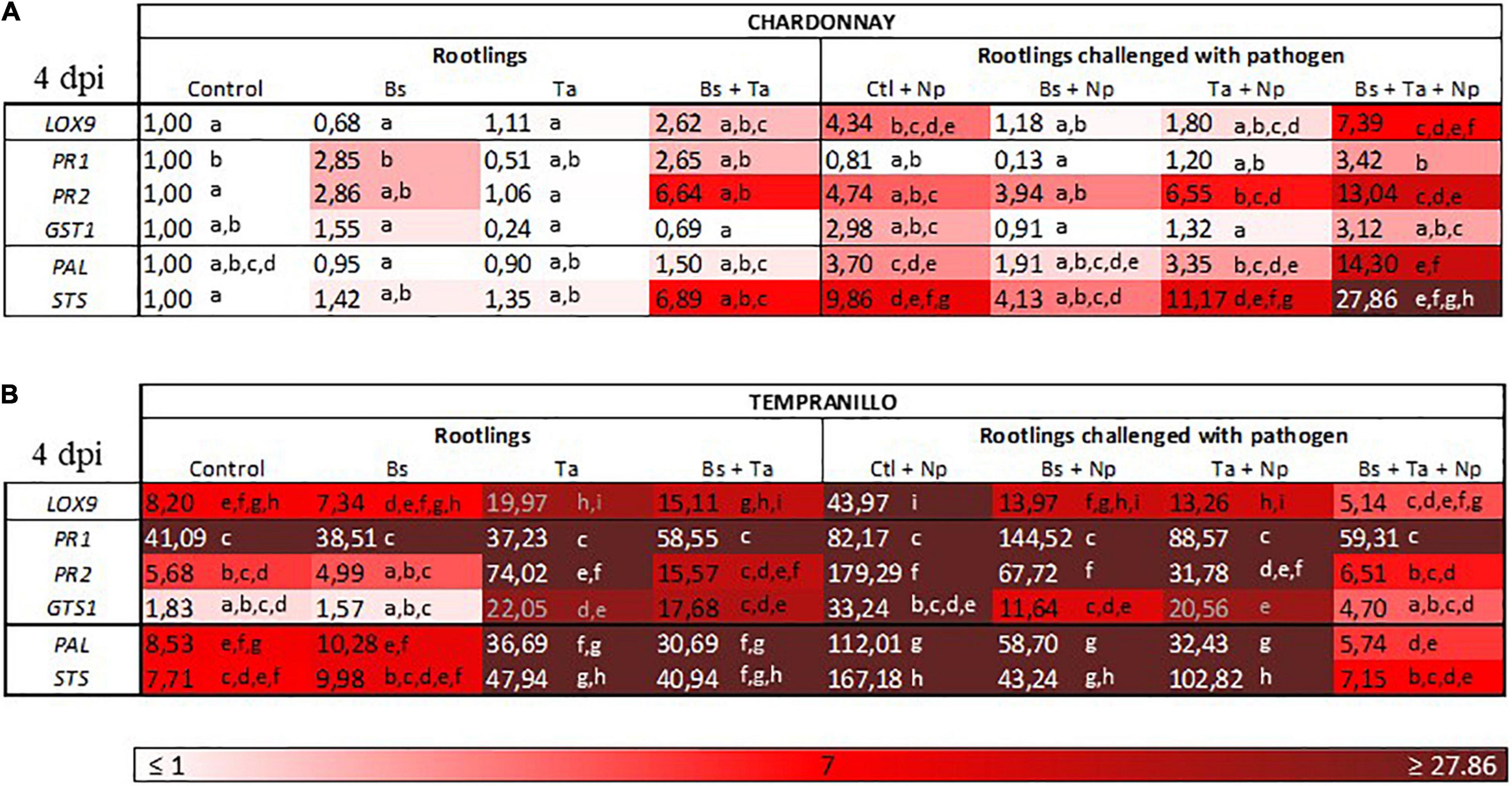
Figure 3. Bacillus subtilis PTA-271 and Trichoderma atroviride SC1 attenuates induced differential expression of defense-related genes in leaves of Chardonnay (A) and Tempranillo (B) rootlings before and after pathogen challenge. Twenty-week-old rootlings untreated or pretreated with PTA-271 or SC1 or both were further infected with sterile PDA plugs (Control, Bs, Ta, and Bs + Ta, respectively) or with mycelium plugs of Np-Bt67 (Ctl + Np, Bs + Np, Ta + Np, and Bs + Ta + Np, respectively). Transcript levels of defense-related genes monitored by qRT-PCR in plant leaves after 4 days of inoculation. Uninfected control of Chardonnay was considered as a reference sample (1× expression level) for both cultivars, and heatmaps represent changes in the transcript expression levels as indicated by the color shading. Data are the means from three representative replicates among five showing the same trends. Different letters indicate statistically significant differences between the treatments (ANOVA, Fisher’s LSD post hoc test, α = 0.1). Legends for genes are LOX9, lipoxygenase 9; PR1, pathogenesis-related protein 1; PR2, class I β-1,3-glucanase; GST1, glutathione-S-transferase 1; PAL, phenylalanine ammonia lyase; STS, stilbene synthase.
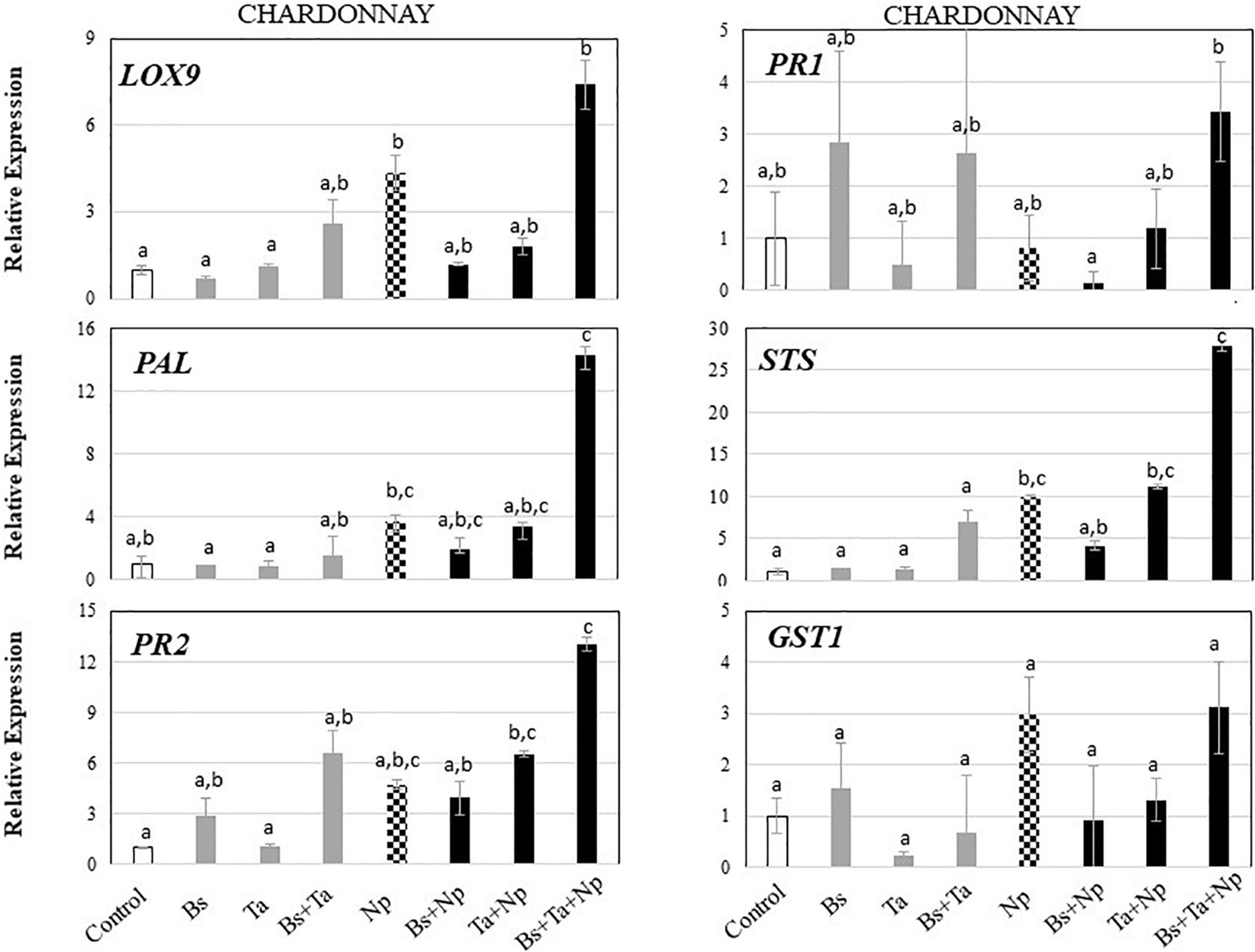
Figure 4. Bacillus subtilis PTA-271 and Trichoderma atroviride SC1 attenuates induced differential expression of defense-related genes in leaves of Chardonnay rootlings before and after pathogen challenge. Twenty-week-old rootlings untreated or pretreated with PTA-271 or SC1 or both were further infected with sterile PDA plugs (Control, Bs, Ta, and Bs + Ta, respectively) or with mycelium plugs of Np (Ctl + Np, Bs + Np, Ta + Np, and Bs + Ta + Np, respectively). Transcript levels of defense-related genes monitored by qRT-PCR in plant leaves after 4 days of inoculation. Uninfected control was considered as the reference sample (1× expression level). Data are the means from three representative replicates among five showing the same trends. Different letters indicate statistically significant differences between the treatments (ANOVA, Fisher’s LSD post hoc test, α = 0.1). Legends for genes are as in Figure 2.
Tempranillo (Figure 3B) showed a high basal expression of the targeted gene responsive to SA (i.e., PR1) compared to Chardonnay (Figure 3A). Interestingly, while the application of Bs PTA-271 (Figure 5 and Supplementary Figure 1B) did not induce any consistent changes of defense gene expression, that of Ta SC1 induced the expression of the greatest number of studied genes: by a factor of 2.4 for LOX9, 13.0 for PR2, 12.0 for GST1, 4.3 for PAL, and 6.2 for STS. To contrast with Chardonnay, no relevant number of targeted genes were overexpressed with the application of both BCAs (2.7-fold for PR2, 9.6-fold for GST1, 3.6-fold for PAL, and 5.3-fold for STS) compared to Ta SC1 alone. Ta SC1, alone or together with Bs PTA-271, may thus act as a priming stimulus for Tempranillo.
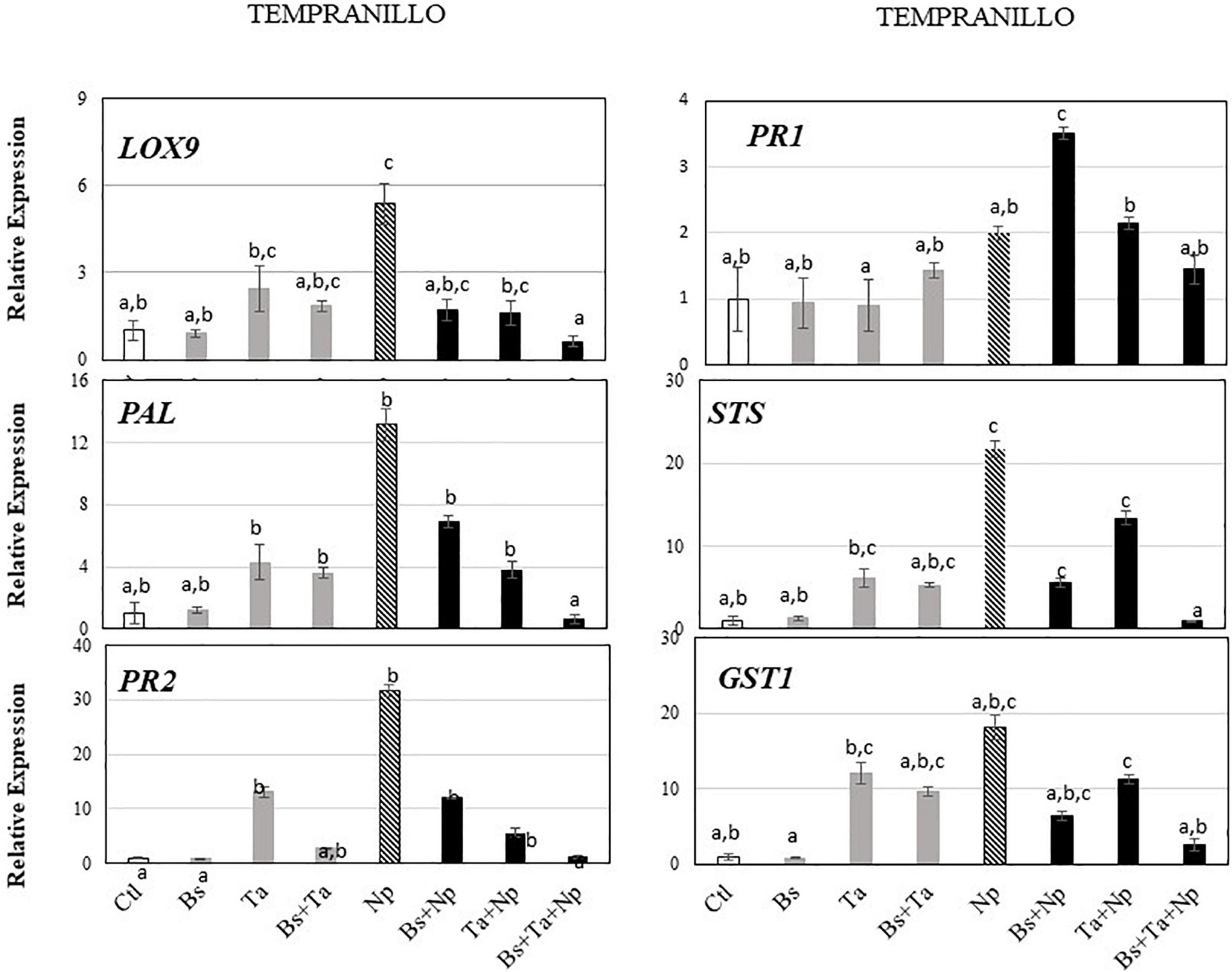
Figure 5. Bacillus subtilis PTA-271 and Trichoderma atroviride SC1 attenuates induced differential expression of defense-related genes in leaves of Tempranillo rootlings before and after pathogen challenge. Twenty-week-old rootlings untreated or pretreated with PTA-271 or SC1 or both were further infected with sterile PDA plugs (Control, Bs, Ta, and Bs + Ta, respectively) or with mycelium plugs of Np-Bt67 (Ctl + Np, Bs + Np, Ta + Np, and Bs + Ta + Np, respectively). Transcript levels of defense-related genes monitored by qRT-PCR in plant leaves after 4 days of inoculation. Uninfected control was considered as the reference sample (1× expression level). Data are the means from three representative replicates among five showing the same trends. Different letters indicate statistically significant differences between the treatments (ANOVA, Fisher’s LSD post hoc test, α = 0.1). Legends for genes are as in Figure 2.
Effects of Biological Control Agents on the Induced Defense of Chardonnay and Tempranillo Upon Pathogen Challenge
In leaves of Chardonnay rootlings infected with Np-Bt67, data from qRT-PCR showed that except for PR1 (0.8-fold expression), the expression of all targeted defense genes was consistently upregulated from 2.9- to 9.8-fold (4.3 for LOX9, 4.7 for PR2, 2.9 for GST1, 3.7 for PAL, and 9.8 for STS) (Figure 4 and Supplementary Figure 1A). As indicated before, in the absence of pathogen infection, Bs PTA-271 only induced a weak expression of PR2 and PR1 (2.8-fold increase) but was not significant according to ANOVA analysis (Figure 4 and Supplementary Figure 1A), suggesting that Bs PTA-271 may act as a priming stimulus in Chardonnay. However, upon pathogen challenge, no post-priming was observed in Bs-PTA-271-pretreated plants since it did not induce any stronger activation of the targeted plant immune defenses compared to Chardonnay-infected control at 4 dpi (Figure 3). In contrast, Ta SC1 showed no sign of priming stimulus in control Chardonnay, but it induced similar expression of PR2 (6.5-fold increase) and STS (11.1-fold increase) than infected control (4.7 and 9.8, respectively). Interestingly, the application of both BCAs enabled to reach the highest level of Chardonnay defense gene expression upon pathogen challenge (3.1–27.8) compared to infected control (0.8–9.8), a priming effect shown as consistent according to the discriminating capacity of the qRT-PCR technique, but still not yet significant according to the ANOVA analysis. Anyway, such a synergy at 4 dpi can result from a priming stimulus by Bs PTA-271, followed by a post-primed phase upon pathogen inoculation with a more rapid and strong activation of immune defenses due to interactions among each actor (Bs PTA-271, Ta SC1, Np-Bt67, and Chardonnay).
In leaves of Tempranillo infected with Np-Bt67, the expression of all targeted defense genes was consistently upregulated from 2.0- to 31.5-fold, and significantly for LOX9, PR2, PAL, and STS (Figure 5 and Supplementary Figure 1B). Compared to infected Chardonnay (Figure 3A), expression of the basal defense genes was significantly stronger in infected Tempranillo (Figure 3B), highlighting a higher basal defense level in Tempranillo toward Np-Bt67 than in Chardonnay at 4 dpi. The ability of each BCA or both to enhance Tempranillo immunity was also addressed (Figure 5 and Supplementary Figure 1B). As reported above, in the absence of pathogen infection, Ta SC1 alone induced a consistent expression of almost all the targeted defenses (2.44–13.03 for LOX9, PR2, GST1, PAL, and STS), suggesting that Ta SC1 may act as a priming stimulus for Tempranillo cultivar, but in a lesser extent when combined with Bs PTA-271 (1.84–9.66). However, upon pathogen challenge, no post-priming was observed in Ta SC1-pretreated rootlings since it did not induce any stronger expression of the targeted immune defenses than in the Tempranillo-infected control at 4 dpi, but lower (Figure 5 and Supplementary Figure 1B). Regarding Bs PTA-271 effect (i.e., Bs + Np), while it showed no sign of priming stimulus in control Tempranillo, it induced the expression of almost all targeted defenses, but similarly to Ta SC1, thus in a lower extent than the infected control (thus, no more priming in that condition with Bs PTA-271). Additionally, the pretreatment with both BCAs induced a lower expression level of Tempranillo defense genes upon pathogen challenge (0.63–2.57) than in infected control (2.0–31.56). However, such apparent non-expression of Tempranillo defenses at 4 dpi did not presume any useful induced defenses at other key times or among other defenses that were not targeted in this study.
Taking the uninfected control of Chardonnay as the reference sample for both cultivars, we can compare the immunity between Tempranillo and Chardonnay upon pathogen challenge (Figures 3A,B, respectively). As observed in control condition (i.e., high basal expression of PR1), infected Tempranillo showed once again a high expression of this targeted gene presumably responsive to SA, compared to Chardonnay. This suggests that Tempranillo would use SA-dependent defense pathways toward N. parvum, even when overexpressing PR2, GST1, PAL, and STS, unlike Chardonnay. These data thus highlight the possible role of SA signaling in Tempranillo, especially when infected and pretreated with Bs PTA-271 (i.e., PR1). Curiously, the JA/ET-responsive gene LOX9 was also highly upregulated in infected Tempranillo, while LOX9 was severely downregulated in infected Tempranillo pretreated with single or both BCAs, as well as PR2, PAL, and STS. Opposite trends were observed in Chardonnay: (i) no prominent role of SA signaling in infected Chardonnay, especially when pretreated with Bs PTA-271; and (ii) LOX9 was not so highly upregulated in infected Chardonnay (i.e., Ctl + Np), but LOX9 was upregulated in infected Chardonnay pretreated with both BCAs, and PR2, PAL, and STS with Ta SC1 or both BCAs. These data could suggest the prominent role of JA/ET signaling in Chardonnay, especially when infected and pretreated with BCA. Interestingly, PR2, PAL, and STS are common defenses induced by each BCA against Np-Bt67 for the two cultivars, prossibly through two distinct signaling pathways.
Direct Beneficial or Detrimental Interplays Between Bacillus subtilis PTA-271, Trichoderma atroviride SC1, and the Pathogen Neofusicoccum parvum-Bt67
Regarding the in vitro tests with Np-Bt67 (Figure 6), results showed that Bs PTA-271 and Ta SC1 antagonize Np-Bt67 when plated 48 h before the pathogen. As shown in Figures 6A,B, the growth of Np-Bt67 was consistently reduced by Ta SC1 or Bs PTA-271 in dual confrontation compared to the control. However, while the growth of Np-Bt67 was completely repressed from day 3 by Ta SC1 (Figure 6A), it was half-repressed by Bs PTA-271 (Figure 6B), enabling the pathogen to grow consistently less than the control over the same time period. Thus, Ta SC1 antagonistic effect was stronger than that of Bs PTA-271, although only a fungistatic effect was observed between them (since when transplanted, the pathogen grows back).
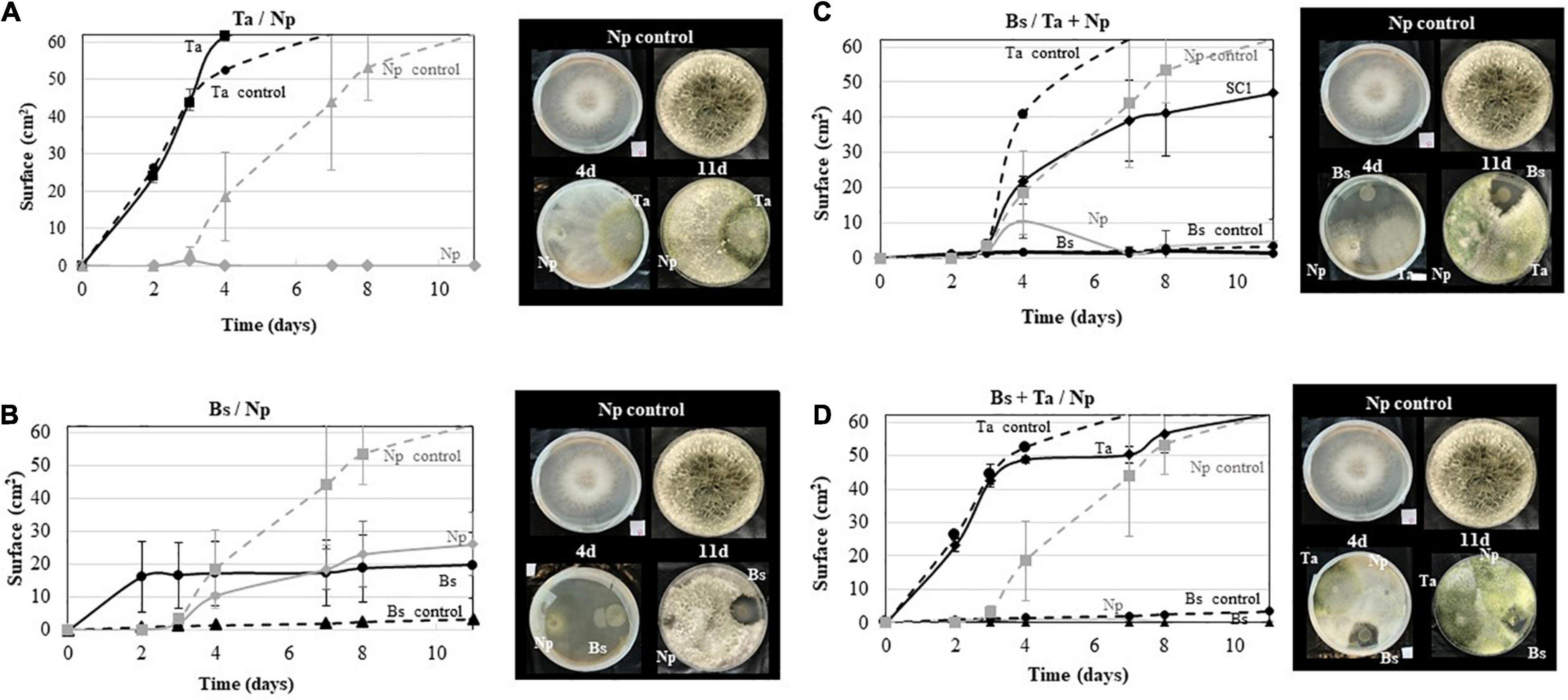
Figure 6. Antagonistic activity of Bacillus subtilis PTA-271 and Trichoderma atroviride SC1 against the Neofusicoccum parvum strain Bt67. (A) T. atroviride SC1 (Ta) was applied 48 h before N. parvum (Np) in the opposite sides of PDA plates. (B) B. subtilis PTA-271 (Bs) was applied 48 h before N. parvum (Np-Bt67) in the opposite sides of PDA plates. (C) B. subtilis PTA-271 (Bs) was applied 48 h before N. parvum (Np) and T. atroviride (Ta) at distinct areas of the PDA plates. (D) B. subtilis PTA-271 (Bs) and T. atroviride SC1 (Ta) were applied 48 h before N. parvum (Np) at distinct areas of the PDA plates. All plates were incubated at 28°C. Pictures of each plate condition were taken from day 1 to day 11 after the first inoculation. Photos on top are the control of Np-Bt67 and photos at the bottom indicate the confrontation assay at day 4 (left) and day 11 (right).
In three-way confrontations (Figures 6C,D), the antagonistic effect of Bs PTA-271 against Np-Bt67 was still reinforced in the presence of Ta SC1, even applied 48 h later (Figure 6C). Such benefit was yet reinforced when the two BCAs were both applied 48 h before the pathogen, in which the growth of Np-Bt67 was close to 5 mm2 (Figure 6D). However, it should be noted that Ta SC1 did not grow as fast when applied 48 h after Bs PTA-271, since Ta SC1 slopes are not parallel but weaker in Figure 6C than in Figure 6D.
To check the Ta SC1 capacity to keep its antagonistic effect when applied simultaneously with Bs PTA-271, dual confrontations were made between the two BCAs applied in the same area, with or without pathogen (Figure 7). As shown in Figure 7A, the growth of Ta SC1 was slowed down with Bs PTA-271, leading to a smaller Ta SC1 area than for the Ta SC1 control over the same time period. Interestingly, this detrimental effect of Bs PTA-271 on Ta SC1 disappeared in the three-way confrontation with the pathogen Np-Bt67 (Figure 7B), being all applied simultaneously at the same area. Thus, Ta SC1 and/or Bs PTA-271 may keep their strong antagonistic activity when facing a common adversary.
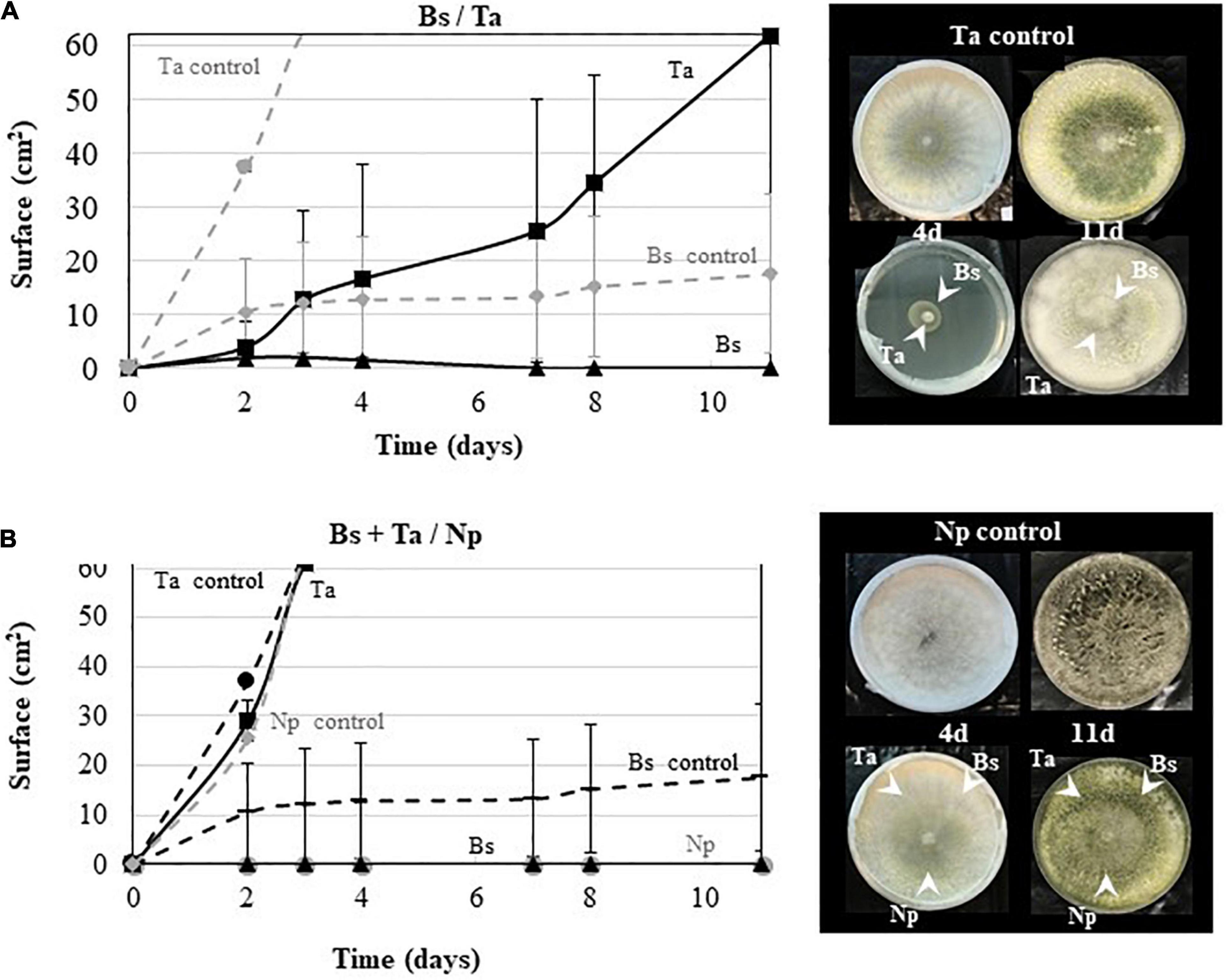
Figure 7. Antagonistic activity of Bacillus subtilis PTA-271 and Trichoderma atroviride SC1 against Neofusicoccum parvum Bt67. (A) B. subtilis PTA-271 (Bs) and T. atroviride SC1 (Ta) were applied simultaneously in the center of the PDA plates. (B) B. subtilis PTA-271 (Bs), T. atroviride SC1 (Ta), and N. parvum (Np) were applied simultaneously in the center of the PDA plates. All plates were incubated at 28°C. Pictures of each plate were taken from day 1 to day 11 after the first inoculation. Photos on top are SC1 control (A) and Np-Bt67 control (B), and pictures at the bottom indicate the confrontation assay at day 4 (left) and day 11 (right).
Discussion
In search of an effective protective BCA combination against N. parvum, we investigated a potential BCA and a BCA-commercial product, each already described as protectors against GTDs on distinct cultivars: B. subtilis PTA-271 with Chardonnay (Trotel-Aziz et al., 2019) and T. atroviride SC1 with different cultivars (Pertot et al., 2016; Berbegal et al., 2020; Martínez-Diz et al., 2021a). This study assessed the combined impact of these two BCAs on two cultivars artificially infected or not with one pathogen. N. parvum Bt67 was used, as a very aggressive pathogen associated to BD. Our investigation focused on the capacity of single and combined BCAs to counteract BD symptoms on both cultivars. To compare the two BCAs, densities were aligned to the density optimized for Bs PTA-271. After looking for the protective capacity of BCA in planta, their modes of action leading to protection were further explored. Thus, we investigated whether these BCAs could affect pathogen growth in vitro and cultivar immunity upon infection in planta.
Neofusicoccum parvum-Bt67 caused BD symptoms on the rootlings of the two grapevine cultivars, as shoot full dieback, canker external necrosis, and shoot internal necrosis. Interestingly, the full dieback symptoms were more severe on Tempranillo than on Chardonnay (i.e., 37.5 and 28%, respectively, Figures 2D,G), suggesting a greater susceptibility to BD for Tempranillo than Chardonnay, as already reported by Luque et al. (2009) and Cobos et al. (2019). Although there is a lack of comparative data between cultivars, the distinct susceptibility of some cultivars to GTDs has already been reported (Travadon et al., 2013; Fontaine et al., 2016b; Chacon et al., 2020; Reveglia et al., 2021), even within a same cultivar from one region to another or depending on the vintage (Mimiague and Le Gall, 1994). However, in Chardonnay and Tempranillo rootlings pretreated with one or both BCAs before inoculation of the pathogen, BD symptoms were significantly reduced with Bs PTA-271 or Ta SC1, respectively. Grapevine effective protection against Np-Bt67 has already been reported with Bs PTA-271 on Chardonnay rootlings (Trotel-Aziz et al., 2019), and with Ta SC1 on Tempranillo in nursery and vineyards conditions (Berbegal et al., 2020; Blundell et al., 2021). Additionally, in our experimental conditions, Chardonnay seems to favor the beneficial effect of Bs PTA-271, while Tempranillo favors that of Ta SC1 beneficial effect, highlighting the relationship between cultivar response and BCA effect. Manter et al. (2010) suggested that the differences between cultivars may result from minor changes in the composition of their endophyte community, with Trichoderma species being among the most common endophytic fungal isolates from Tempranillo (González and Tello, 2011). Therefore, Tempranillo could be subjected to Trichoderma’s influence. Similarly, the efficiency of Bs PTA-271 toward Chardonnay may be explained by its origin of sampling from an established Chardonnay vineyard, screened from healthy vines (Trotel-Aziz et al., 2008). In combination and according to our experimental conditions, despite cited as compatible strains (Kumar, 2013), Ta SC1 + Bs PTA-271 are less protective against Np-Bt67 in Chardonnay than Bs PTA-271 alone. The authors reported that Trichoderma spp. can interfere the plant signaling networks and secrete an arsenal of degrading enzymes (i.e., proteases) and secondary metabolites (Tiwari and Verma, 2019; Alfiky and Weisskopf, 2021), suggesting that Ta SC1 may alter both Bs PTA-271 integrity and beneficial effects in Chardonnay. However, the application of both BCAs enabled to reach the highest level of Chardonnay defense gene expression upon pathogen challenge (see Figure 3A), and the highest protection in Tempranillo cultivar (see Figure 2G), highlighting that Ta SC1 on its own would not interfere with the beneficial effects of Bs PTA-271.
Beneficial effects of combined BCAs have yet been reported in different pathosystems (Yobo et al., 2011; Magnin-Robert et al., 2013), and our study reports for the first time the biocontrol potential of the combination of Bs PTA-271 and Ta SC1 against Np-Bt67 in Tempranillo. Our results showed that Ta SC1 efficiently protects Tempranillo, and this protection is still observed in rootlings pretreated with both BCAs (see Figure 2G). Thus, a significant benefit is observed when using both BCAs in Tempranillo, despite the fact that they could antagonize each other. In this respect, Leal et al. (2021) reported that the genome of Bs PTA-271 encodes for the synthesis of bacillaene, a polyketide already described to antagonize Trichoderma spp. (Caulier et al., 2019). Therefore, the positive contribution of Bs PTA-271 and Ta SC1 to Tempranillo protection against Np-Bt67 suggests a fine-tuned orchestrated cooperation of BCAs when facing adversity, as supported by the Figure 7 results and highlighted by Alfiky and Weisskopf (2021). However, the application of BCAs to rootlings was spatially separated.
Empowered with aggressive molecules, Bacillus spp. and Trichoderma spp. can possibly exert direct beneficial or detrimental interplays within the host’s microbiome, and especially on the other BCA and pathogens such as GTDs fungi (Di Marco et al., 2002; Kloepper et al., 2004; Ongena and Jacques, 2008; Haidar et al., 2016; Trotel-Aziz et al., 2019; Yacoub et al., 2020; Úrbez-Torres et al., 2020; Blundell et al., 2021). In vitro dual confrontation tests confirmed the antagonistic activity of Bs PTA-271 or Ta SC1 toward Np-Bt67 since each of them significantly inhibits the mycelium growth of Np-Bt67 (see Figures 6A,B). This also prompts us the idea that the not-yet-convincing protection assay of Bs PTA-271 and Ta SC1 in infected Chardonnay would not result from a detrimental effect of Ta SC1 on some putative direct effects of Bs PTA-271. Indeed, the strong direct antagonistic activity of Bs PTA-271 and Ta SC1 toward Np-Bt67 also operates when the two BCAs were applied both 48 h before the pathogen (see Figure 6D). This antagonistic benefit is in accordance with the significant synergy of the protection in Tempranillo by both BCAs (see Figure 2G), and it confirms the benefit of using them in combination in Tempranillo to optimize the direct fight against Np-Bt67. A similar outcome was reported by Alexander and Phin (2014) using an effective combination of Bacillus spp. and Trichoderma spp. against Ganoderma spp. Such direct effects of BCA against pathogens are important life traits to protect grapevine from BD, still deprived of effective curative treatments nowadays (Mondello et al., 2018). In nursery too, healthy mother plants require a control of their sanitary status (Pertot et al., 2016), eventually provided by an early inoculation of such beneficial BCA with a strong antagonistic activity toward pathogens.
Direct beneficial or detrimental interplays between BCAs also condition their capacity to live together in symbiosis, even in planta as part of the holobiont (Bettenfeld et al., 2020). Dual confrontation was thus also performed between Bs PTA-271 and Ta SC1. As expected, Bs PTA-271 antagonized Ta SC1 (see Figure 7A; Caulier et al., 2019; Leal et al., 2021). However, this detrimental effect of Bs PTA-271 on Ta SC1 disappears in a three-way confrontation with Np-Bt67, when they were all applied simultaneously at the same area (see Figure 7B). These data confirm that Ta SC1 and Bs PTA-271 can positively interact to better confine Np-Bt67 and can lead to a direct positive contribution of this combination to the protection of Tempranillo against Np-Bt67. However, such a direct positive contribution of combined BCAs did not operate on the infected Chardonnay rootlings. Our experimental conditions could have altered the ability of Ta SC1 to exert its direct fungistatic effect (applied once at 108 CFU/ml with a paintbrush over a 5-mm2 area). This also strongly suggests that Chardonnay itself alters the fine-tuned orchestrated cooperation of BCAs, probably targeting the indirect Ta SC1 beneficial effect since BCAs are spatially separated. This prompts us to pursue our investigations further in order to decipher the indirect interactions driving to a beneficial outcome in grapevine control of Np-Bt67.
According to our previous works, a focus on grapevine systemic immunity was made by targeting six selected defense genes in leaves: the lipoxygenase LOX9 involved in oxylipin synthesis and described as dependent to JA/ET; PR1 described to be regulated by SA; the β-1,3-glucanase PR2 described to be regulated by various phytohormones such as SA, JA, and ET; the glutathione-S-transferase GST1 putatively involved in the detoxification process; the phenylalanine ammonia-lyase PAL catalyzing the first step in the phenylpropanoid pathway; and the stilbene synthase STS involved in the synthesis of phytoalexins (Trotel-Aziz et al., 2019). Interestingly, basal immunity results in a weak constitutive expression of the targeted defense genes in Chardonnay compared to Tempranillo (see Figure 3), despite the fact that literature described Chardonnay as less susceptible to BD than Tempranillo (Luque et al., 2009). Maybe our six targeted genes are not sufficiently exhaustive to presume at this preliminary stage of the susceptible versus tolerant status of both cultivars. However, looking at the specific red and white cultivar responses, the same studied genes are of interest: those specific to white grape cultivars include transcription factors from the ET pathway and lipid metabolism (e.g., lipoxygenase), while those specific to red grape cultivars are linked to the secondary metabolism in connection with the pathway of phenylpropanoids (e.g., PAL and its derivatives) and are expressed more strongly in the red cultivars, to distinguish them from the white ones (Lambert et al., 2012; Massonnet et al., 2017). Upon abiotic or biotic stress, other authors pointed out the highest synthesis of resveratrol in the most tolerant grapevine (Corso et al., 2015, Lakkis et al., 2019), but a gain of protection due to the BCA presence in susceptible cultivar (Lakkis et al., 2019). Considering that susceptible plants rather benefit from the help of BCA to induce their immunity, unlike resistant plants that already have high basal immunity, we examined the immunity induced by both cultivars studied.
In Tempranillo (see Figures 3B, 5 and Supplementary Figure 1B) under our experimental conditions, application of Bs-PTA-271 did not induce significant changes in plant defense responses compared to control, whether infected or not. Since Tempranillo basal immunity strongly upregulates PR1 as a marker of SA-dependent defenses, we can speculate that high basal SA content might contribute to prevent the beneficial effect of Bs PTA-271 on Tempranillo immunity. Our previous study showed that Bs PTA-271 primed the expression of the plant JA/ET-dependent defenses in Chardonnay rootlings (Trotel-Aziz et al., 2019), whereas it is reported that early activation of SA signaling could antagonize the expression of these JA/ET-dependent defenses (Pieterse et al., 2012; Van der Does et al., 2013). In this sense, Bs PTA-271 did not provide protection in Tempranillo against Np-Bt67. These results show therefore that cultivars differing in their basal immunity can condition the success of a BCA protection toward a pathogen. In contrast, Ta SC1 alone or together with Bs PTA-271 acts as a priming stimulus for Tempranillo, but no post-priming was observed with Ta SC1 alone and with Ta SC1 + Bs PTA-271 upon pathogen challenge. Looking at the defenses induced by these two protective modalities (Ta SC1 and Ta SC1 + Bs PTA-271) against Np-Bt67: the SA-dependent defenses (i.e., PR1, PAL, and STS) were rather strongly decreased in protected plants (i.e., asymptomatic despite infected), while they were the highest in symptomatic plants (Bs PTA-271). Since Botryosphaeriaceae are known to specifically metabolize grapevine phytoalexins (Stempien et al., 2017), which benefits pathogen fitness, we could suggest that the SA stimulation of the phenylpropanoid pathway and derivatives would wrongly serve the plant. In the case of Tempranillo exposed to Botryosphaeriaceae, the high constitutive expression of SA-dependent defense genes could thus appear as a disadvantage, confirming that Tempranillo would be less tolerant than Chardonnay to BD, as already reported by Luque et al. (2009) and Cobos et al. (2019). Fortunately, in the Tempranillo pretreated with both BCAs, the expressions of genes PR1, PAL, and STS were repressed, and in the Tempranillo pretreated with Ta SC1 alone, the expression of the genes PAL and STS were repressed. Therefore, the beneficial effect of Ta SC1 on Tempranillo to control Np-Bt67 could result from a repressive effect on detrimental SA-dependent defenses. Complementary approaches are in progress to screen the induced key levers able to trigger ISR in the whole plant. Additionally, the Ta SC1 beneficial effect on Tempranillo could also result from a direct antagonism toward Np-Bt67 since it is applied in the same area as Ta SC1.
In non-infected Chardonnay rootlings (see Figures 3A, 4 and Supplementary Figure 1A), Ta SC1 shoot application did not induce any significant changes of the selected targeted responses of plant defenses in leaves, while Bs PTA-271 root application upregulated the PR1 and PR2 gene expression and the combined application induced the expression of almost all targeted genes at a higher level than Bs PTA-271 alone. Bs PTA-271, alone or together with Ta SC1, may thus act as a priming stimulus in Chardonnay. However, upon pathogen challenge (4 dpi, designed as a relevant sampling time point for such experiment), Bs PTA-271 did not prime any of the targeted defenses, probably due to low to medium pathogen pressure compared to that reported in Trotel-Aziz et al. (2019). However, Bs PTA-271 beneficial effect on Chardonnay is supported by the phenotype of the Bs-PTA-271 treated rootlings, showing a significant protection for Chardonnay against Np-Bt67 at 2.5 mpi. This contrasts with the detected non-benefit provided by the combined application of both BCAs at 2.5 mpi, despite the fact that defenses were primed at 4 dpi, possibly due to a very high level of reactive oxygen species (ROS) that could amplify the plant defenses. It is interesting to note that GST1 (involved in the ROS detoxification process) is the only useful targeted gene that was weakly expressed in non-protected Chardonnay pre-treated with both BCAs and further infected with N. parvum (see Figure 3A). This may suggest that when a pathway with high induced defenses is not combined with sufficient ROS detoxification, a plant could potentially trigger many symptoms. In our experimental conditions, Chardonnay therefore favors Bs PTA-271’s beneficial effect, probably thanks to the different key levers including the induced grapevine immunity. Present at the root level, these results and those reported in Trotel-Aziz et al. (2019) suggest that systemic induced immunity conferred by Bs PTA-271 drove the plant ISR against Np-Bt67. Amazingly, the application of both BCAs enabled to reach the highest level of Chardonnay defense gene expression upon pathogen challenge (see Figure 3A), despite no protection at 2.5 mpi (see Figure 2D). Since Ta SC1 contributes to actively reducing the SA-dependent defenses in Tempranillo, one can hypothesize that Ta SC1 would also promote the Bs PTA-271 way of triggering immunity in Chardonnay. The authors also indicated that Trichoderma spp. may produce enzymes (i.e., ACC deaminase) able to shunt ET synthesis (Alfiky and Weisskopf, 2021), but in Tempranillo, the application of both BCAs enabled reaching the highest protection (see Figure 2G). Thus, it would be surprising that Ta SC1 on its own would succeed to interfere with the beneficial effects of Bs PTA-271 in Chardonnay. This opens the discussion of how the cultivar interacts with BCAs and the pathogen to condition the beneficial or detrimental outcome against Np-Bt67. Complementary approaches are already in progress to screen which are the induced key levers useful to control ISR in the whole plant.
Conclusion
Altogether, our results provide evidence that grapevine susceptibility to BD is cultivar-dependent, as well as the BCA beneficial effects. Bs PTA-271 was confirmed as an effective protector for Chardonnay against Np-Bt67, and Ta SC1 was shown for the first time as a good protector for Tempranillo. This study also reports for the first time the biocontrol potential of the combination of Bs PTA-271 and Ta SC1 against Np-Bt67 in Tempranillo. This is a promising result for an improved efficiency of sustainable biological control in a proven context of lack of effective chemicals to manage GTDs.
Endowed with aggressive molecules, Bs PTA-271 and Ta SC1 can antagonize each other, but Bs PTA-271 inhibits Np-Bt67 development with a greater efficiency in a three-way confrontation. This beneficial BCA collaboration against Np-Bt67 still operates in Tempranillo and confirms the interest of using both BCAs in combination to optimize the direct fight against Np-Bt67. These results are of great interest for effective curative treatments to obtain healthy mother plants in the nursery and to control BD in vineyard. However, the direct beneficial effect of combined BCAs did not operate to protect Chardonnay, suggesting that Chardonnay itself probably alters the fine-tuned orchestrated cooperation of BCAs that drives such direct beneficial effect.
Plant systemic immunity was also affected by each BCA. Our findings suggest a common feature for the two cultivars: the defenses that are greatly diminished in BCA-protected plants appear to be those that are responsive to SA, in contrast to symptomatic plants. For Tempranillo, the high basal expression of SA-dependent defenses may thus explain the highest susceptibility to BD and also the ineffectiveness of Bs PTA-271 in our experimental conditions. Complementary approaches are underway to further investigate the responses of each cultivar to both Bs PTA-271 and Ta SC1 under controlled conditions and upon pathogen challenge.
Data Availability Statement
The raw data supporting the conclusions of this article will be made available by the authors, without undue reservation.
Author Contributions
CL, PT-A, and FF designed and planned the research. CL performed most of the experiments, analyzed the data, and wrote the manuscript with input and discussion from PT-A, FF, JA, and DG. JA and DG validated the planned research and provided expertise for all stages of this work. J-FG ensured the quality of the mother cuttings and greenhouse conditions. NR assured the quality of the qRT-PCR analyses and data. All authors contributed to writing and revising the manuscript.
Funding
This work was supported by a French Grant from the Region GRAND-EST France and the City of GRAND-REIMS France through the BIOVIGNE Ph.D. program, whose functioning is supported by BELCHIM Crop Protection France. DG was supported by the Ramón y Cajal program, Spanish Government (RyC-2017-23098).
Conflict of Interest
The authors declare that the research was conducted in the absence of any commercial or financial relationships that could be construed as a potential conflict of interest.
Publisher’s Note
All claims expressed in this article are solely those of the authors and do not necessarily represent those of their affiliated organizations, or those of the publisher, the editors and the reviewers. Any product that may be evaluated in this article, or claim that may be made by its manufacturer, is not guaranteed or endorsed by the publisher.
Acknowledgments
We are grateful to Cecilia Rego (Higher Institute of Agronomy, Lisbon University, Portugal) for the pathogen gift. Thanks are also due to Laetitia Parent and Vincent Leclerc for their technical assistance.
Supplementary Material
The Supplementary Material for this article can be found online at: https://www.frontiersin.org/articles/10.3389/fmicb.2021.726132/full#supplementary-material
Supplementary Figure 1 | Bacillus subtilis PTA-271 and Trichoderma atroviride SC1 attenuates induce differential expression of defense-related genes in leaves of Chardonnay (A) and Tempranillo (B) rootlings before and after pathogen challenge. Twenty weeks old rootlings untreated or pretreated with PTA-271 or SC1 or both were further infected with sterile PDA plugs (Control, Bs, Ta, and Bs + Ta, respectively) or with mycelium plugs of Np-Bt67 (Ctl + Np, Bs + Np, Ta + Np, and Bs + Ta + Np, respectively). Transcript levels of defense-related genes monitored by qRT-PCR in plant leaf after 4 days of inoculation. Uninfected control of each cultivar was considered as a reference sample (1× expression level) for the same cultivar, and heatmaps represent changes in the transcript expression levels as indicated by the color shading. Data are the means from three representative replicates among five showing the same trends. Legends for genes are as in Figure 2.
References
Alexander, A., and Phin, C. K. (2014). Combination of biological agents in suppressing colonization of Ganoderma boninense of basal stem rot. Am. Eurasian J. Sustain Agric. 8, 1–7.
Alfiky, A., and Weisskopf, L. (2021). Deciphering Trichoderma–plant–pathogen interactions for better development of biocontrol applications. J. Fungus. 7:61. doi: 10.3390/jof7010061
Alström, S. (1991). Induction of disease resistance in common bean susceptible to halo blight bacterial pathogen after seed bacterization with rhizosphere pseudomonads. J. Gen. Appl. Microbiol. 37, 495–501. doi: 10.2323/jgam.37.495
Berbegal, M., Ramón-Albalat, A., León, M., and Armengol, J. (2020). Evaluation of long-term protection from nursery to vineyard provided by Trichoderma atroviride SC1 against fungal grapevine trunk pathogens. Pest Manag. Sci. 76, 967–977. doi: 10.1002/ps.5605
Bettenfeld, P., Fontaine, F., Trouvelot, S., Fernandez, O., and Courty, P. E. (2020). Woody plant declines. What’s wrong with the microbiome? Trends Plant Sci. 25, 381–394. doi: 10.1016/j.tplants.2019.12.024
Billones-Baaijens, R., and Savocchia, S. (2019). A review of Botryosphaeriaceae species associated with grapevine trunk diseases in Australia and New Zealand. Austr. Plant Dis. 48, 3–18. doi: 10.1007/s13313-018-0585-5
Blundell, R., Arreguin, M., and Eskalen, A. (2021). In vitro evaluation of grapevine endophytes, epiphytes and sap micro-organisms for potential use to control grapevine trunk disease pathogens. bioRxiv [Preprint]. doi: 10.1101/2021.02.09.430335
Caarls, L., Pieterse, C. M., and Van Wees, S. (2015). How salicylic acid takes transcriptional control over jasmonic acid signaling. Front. Plant Sci. 6:170. doi: 10.3389/fpls.2015.00170
Calzarano, F., Di Marco, S., and Cesari, A. (2004). Benefit of fungicide treatment after trunk renewal of vines with different types of esca necrosis. Phytopathol. Mediterr. 43, 116–123.
Caulier, S., Nannan, C., Gillis, A., Licciardi, F., Bragard, C., and Mahillon, J. (2019). Overview of the antimicrobial compounds produced by members of the Bacillus subtilis group. Front. Microbiol. 10:302. doi: 10.3389/fmicb.2019.00302
Chacon, J. L., Gramaje, D., Izquierdo, P. M., Martínez, J., and Mena, A. (2020). Evaluation of six red grapevine cultivars inoculated with Neofusicoccum parvum. Eur. J. Plant Pathol. 158, 811–815. doi: 10.1007/s10658-020-02111-9
Claverie, M., Notaro, M., Fontaine, F., and Wéry, J. (2020). Current knowledge on Grapevine Trunk Diseases with complex etiology: a systemic approach. Phytopathol. Mediterr. 59, 29–53. doi: 10.36253/phyto-11150
Cobos, R., Calvo-Peña, C., Álvarez-Pérez, J. M., Ibáñez, A., Diez-Galán, A., González-García, S., et al. (2019). Necrotic and cytolytic activity on grapevine leaves produced by Nep1-like proteins of Diplodia seriata. Front. Plant Sci. 10:1282. doi: 10.3389/fpls.2019.01282
Compant, S., Brader, G., Muzammil, S., Sessitsch, A., Lebrihi, A., and Mathieu, F. (2013). Use of beneficial bacteria and their secondary metabolites to control grapevine pathogen diseases. BioControl. 58, 435–455. doi: 10.1007/s10526-012-9479-6
Compant, S., and Mathieu, F. (2017). Biocontrol of Major Grapevine Diseases: Leading Research. Wallingford: CABI, 160–170.
Conrath, U., Beckers, G. J., Langenbach, C. J., and Jaskiewicz, M. R. (2015). Priming for enhanced defense. Annu. Rev. Phytopathol. 53, 97–119. doi: 10.1146/annurev-phyto-080614-120132
Conrath, U., Thulke, O., Katz, V., Schwindling, S., and Kohler, A. (2001). Priming as a mechanism in induced systemic resistance of plants. Eur. J. Plant Pathol. 107, 113–119.
Corso, M., Vannozzi, A., Maza, E., Vitulo, N., Meggio, F., Pitacco, A., et al. (2015). Comprehensive transcript profiling of two grapevine rootstock genotypes contrasting in drought susceptibility links the phenylpropanoid pathway to enhanced tolerance. J. Exp. Bot. 66, 5739–5752. doi: 10.1093/jxb/erv274
De Vleesschauwer, D., and Höfte, M. (2009). Rhizobacteria-induced systemic resistance. Adv. Bot. Res. 51, 223–281. doi: 10.1016/s0065-2296(09)51006-3
Di Marco, S., Osti, F., and Cesari, A. (2004). Experiments on the control of esca by Trichoderma. Phytopathol. Mediterr. 43, 108–115.
Di Marco, S., Osti, F., Roberti, R., Calzarano, F., and Cesari, A. (2002). Attivita‘ di specie di 412 Trichoderma nei confronti di Phaeomoniella chlamydospora, patogeno associato al mal delll’esca 413 della vite. Atti Soc. Ital. Sci. Natl. 2, 419–424.
Dufour, M. C., Lambert, C., Bouscaut, J., Mérillon, J. M., and Corio-Costet, M. F. (2013). Benzothiadiazole-primed defence responses and enhanced differential expression of defence genes in Vitis vinifera infected with biotrophic pathogens Erysiphe necator and Plasmopara viticola. Plant Pathol. 62, 370–382. doi: 10.1111/j.1365-3059.2012.02628.x
Elad, Y. (2000). Biological control of foliar pathogens by means of Trichoderma harzianum and potential modes of action. Crop Prot. 19, 709–714. doi: 10.1016/s0261-2194(00)00094-6
El-Tarabily, K. A. (2006). Rhizosphere-competent isolates of streptomycete and non-streptomycete actinomycetes capable of producing cell-wall-degrading enzymes to control Pythium aphanidermatum damping-off disease of cucumber. Botany 84, 211–222. doi: 10.1139/b05-153
Fontaine, F., Gramaje, D., Armengol, J., Smart, R., Nagy, Z. A., Borgo, M., et al. (2016a). Grapevine Trunk Diseases. A Review. Paris: OIV Publications. 24,979-10-91799-60-7.
Fontaine, F., Pinto, C., Vallet, J., Clément, C., Gomes, A. C., and Spagnolo, A. (2016b). The effects of grapevine trunk diseases (GTDs) on vine physiology. Eur. J. Plant Pathol. 144, 707–721. doi: 10.1007/s10658-015-0770-0
González, V., and Tello, M. L. (2011). The endophytic mycota associated with Vitis vinifera in central Spain. Fungal Divers. 47, 29–42. doi: 10.1007/s13225-010-0073-x
Gramaje, D., and Armengol, J. (2011). Fungal trunk pathogens in the grapevine propagation process: potential inoculum sources, detection, identification, and management strategies. Plant Dis. 95, 1040–1055. doi: 10.1094/pdis-01-11-0025
Gramaje, D., Urbez-Torres, J. R., and Sosnowski, M. R. (2018). Managing grapevine trunk diseases with respect to etiology and epidemiology: current strategies and future prospects. Plant Dis. 102, 12–39.
Gruau, C., Trotel-Aziz, P., Villaume, S., Rabenoelina, F., Clément, C., Baillieul, F., et al. (2015). Pseudomonas fluorescens PTA-CT2 triggers local and systemic immune response against Botrytis cinerea in grapevine. Mol. Plant Microbe Interact. 28, 1117–1129. doi: 10.1094/MPMI-04-15-0092-R
Guetsky, R., Shtienberg, D., Elad, Y., Fischer, E., and Dinoor, A. (2002). Improving biological control by combining biocontrol agents each with several mechanisms of disease suppression. Phytopathology 92, 976–985. doi: 10.1094/phyto.2002.92.9.976
Haidar, R., Deschamps, A., Roudet, J., Calvo-Garrido, C., Bruez, E., Rey, P., et al. (2016). 451 Multi-organ screening of efficient bacterial control agents against two major pathogens of 452 grapevine. Biol. Control 92, 55–65. doi: 10.1016/j.biocontrol.2015.09.003
Halleen, F., Fourie, P. H., and Lombard, P. J. (2010). Protection of grapevine pruning wounds against Eutypa lata by biological and chemical methods. South Afr. J. Enol. Vitic. 31, 125–132.
Hamiduzzaman, M. M., Jakab, G., Barnavon, L., Neuhaus, J. M., and Mauch-Mani, B. (2005). β-Aminobutyric acid-induced resistance against downy mildew in grapevine acts through the potentiation of callose formation and jasmonic acid signaling. Mol Plant Microbe Interact. 18, 819–829. doi: 10.1094/mpmi-18-0819
Harman, G. E. (2006). Overview of mechanisms and uses of Trichoderma spp. Phytopathology 96, 190–194. doi: 10.1094/phyto-96-0190
Hofstetter, V., Buyck, B., Croll, D., Viret, O., Couloux, A., and Gindro, K. (2012). What if esca disease of grapevine were not a fungal disease? Fungal Divers. 4, 51–67. doi: 10.1007/s13225-012-0171-z
Horton, N. J., and Kleinman, K. (2015). Using R and RStudio for Data Management, Statistical Analysis, and Graphics. Boca Raton, FL: CRC Press.
Hunt, J. S., Gale, D. S. J., and Harvey, I. C. (2001). Evaluation of Trichoderma as bio-control for protection against wood-invading fungi implicated in grapevine trunk diseases. Phytopathol. Mediterr. 40, S485–S486.
John, S., Scott, E. S., Wicks, T., and Hunt, J. (2004). Interactions between Eutypa lata and Trichoderma harzanium. Phytopathol. Mediterr. 43, 95–104.
John, S., Wicks, T. J., Hunt, J. S., and Scott, E. S. (2008). Colonisation of grapevine wood by Trichoderma harzianum and Eutypa lata. Aust. J. Grape Wine Res. 14, 18–24.
Kloepper, J. W., Ryu, M., and Zhang, S. (2004). Induced systemic resistance and promotion of plant growth by Bacillus spp. Phytopathology 94, 1259–1266. doi: 10.1094/phyto.2004.94.11.1259
Kotze, R. G., Van der Merwe, C. F., Crampton, B. G., and Kritzinger, Q. (2019). A histological assessment of the infection strategy of Exserohilum turcicum in maize. Plant Patho 68, 504–512. doi: 10.1111/ppa.12961
Kumar (2013). Trichoderma: a biological weapon for managing plant diseases and promoting sustainability. Int. J. Agrl. Sci. Vet. Med. 1, 106–121.
Kuzmanovska, B., Rusevski, R., Jankulovska, M., and Oreshkovikj, K. B. (2018). Antagonistic activity of Trichoderma asperellum and Trichoderma harzianum against genetically diverse Botrytis cinerea isolates. Chil. J. Agric. Res. 78, 391–399. doi: 10.4067/s0718-58392018000300391
Lakkis, S., Trotel-Aziz, P., Rabenoelina, F., Schwarzenberg, A., Nguema-Ona, E., Clément, C., et al. (2019). Strengthening grapevine resistance by Pseudomonas fluorescens PTA-CT2 relies on distinct defense pathways in susceptible and partially resistant genotypes to downy mildew and gray mold diseases. Front. Plant Sci. 10:1112. doi: 10.3389/fpls.2019.01112
Lambert, C., Bisson, J., Waffo-Téguo, P., Papastamoulis, Y., Richard, T., Corio-Costet, M. F., et al. (2012). Phenolics and their antifungal role in grapevine wood decay: focus on the Botryosphaeriaceae family. J. Agric. Food Chem. 60, 11859–11868. doi: 10.1021/jf303290g
Larach, A., Torres, C., Riquelme, N., Valenzuela, M., Salgado, E., Seeger, M., et al. (2020). Yield loss estimation and pathogen identification from Botryosphaeria dieback in vineyards of Central Chile over two growing seasons. Phytopathol. Mediterr. 59, 537–548.
Larignon, P., Fontaine, F., Farine, S., Clement, C., and Bertsch, C. (2009). Esca and black dead arm: two major actors of grapevine trunk diseases. C R Biol. 332, 765–783. doi: 10.1016/j.crvi.2009.05.005
Larignon, P., Fulchic, R., Cere, L., and Dubos, B. (2001). Observation on black dead arm in French vineyards. Phytopathol. Mediterr. 40(Suppl.), S336–S342.
Larignon, P. (2004). La constitution d’un groupe international de travail sur les maladies du bois et les premiers résultats des expérimentations menées par l’ITV en laboratoire et en pépinières. Les Maladies du Bois en Midi-Pyrénées 122, 24–27.
Leal, C., Fontaine, F., Aziz, A., Egas, C., Clément, C., and Trotel-Aziz, P. (2021). Genome sequence analysis of the beneficial Bacillus subtilis PTA-271 isolated from a Vitis vinifera (cv. Chardonnay) rhizospheric soil: assets for sustainable biocontrol. Environ. Microbiol. 16, 1–14.
Lebon, G., Duchêne, E., Brun, O., and Clément, C. (2005). Phenology of flowering and starch accumulation in grape (Vitis vinifera L.) cuttings and vines. Ann. Bot. 95, 943–948. doi: 10.1093/aob/mci108
Liu, B., Xue, X., Cui, S., Zhang, X., Han, Q., Zhu, L., et al. (2010). Cloning and characterization of a wheat β-1, 3-glucanase gene induced by the stripe rust pathogen Puccinia striiformis f. sp. Mol. Biol. Rep. 37, 1045–1052. doi: 10.1007/s11033-009-9823-9
Luque, J., Martos, S., Aroca, A., Raposo, R., and Garcia-Figueres, F. (2009). Symptoms and fungi associated with declining mature grapevine plants in northeast Spain. Plant Pathol. 91, 381–390.
Magnin-Robert, M., Quantinet, D., Couderchet, M., Aziz, A., and Trotel-Aziz, P. (2013). Differential induction of grapevine resistance and defense reactions against Botrytis cinerea by bacterial mixtures in vineyards. BioControl 58, 117–131. doi: 10.1007/s10526-012-9474-y
Magnin-Robert, M., Trotel-Aziz, P., Quantinet, D., Biagianti, S., and Aziz, A. (2007). Biological control of Botrytis cinerea by selected grapevine-associated bacteria and stimulation of chitinase and β-1, 3 glucanase activities under field conditions. Eur. J. Plant Pathol. 118, 43–57. doi: 10.1007/s10658-007-9111-2
Manter, D. K., Delgado, A., Holm, G., and Stong, A. (2010). Pyrosequencing reveals a highly diverse and cultivar-specific bacterial endophyte community in potato roots. Microb. Ecol. 60, 157–166.
Martínez-Diz, M. P., Díaz-Losada, E., Andrés-Sodupe, M., Bujanda, R., Maldonado-González, M. M., Ojeda, S., et al. (2021a). Field evaluation of biocontrol agents against black-foot and Petri diseases of grapevine. Pest Manag. Sci. 77, 697–708. doi: 10.1002/ps.6064
Martínez-Diz, M. P., Díaz-Losada, E., Díaz-Fernández, A., Bouzas-Cid, Y., and Gramaje, D. (2021b). Protection of grapevine pruning wounds against Phaeomoniella chlamydospora and Diplodia seriata by commercial biological and chemical methods. Crop Prot. 143:105465. doi: 10.1016/j.cropro.2020.105465
Massonnet, M., Fasoli, M., Tornielli, G. B., Altieri, M., Sandri, M., Zuccolotto, P., et al. (2017). Ripening transcriptomic program in red and white grapevine varieties correlates with berry skin anthocyanin accumulation. Plant physio 174, 2376–2396. doi: 10.1104/pp.17.00311
Meyer, S. L. F., and Roberts, D. P. (2002). Combinations of biocontrol agents for management of plant-parasitic nematodes and soilborne plant-pathogenic Fungi. J. Nematol. 34, 1–8.
Mimiague, F., and Le Gall, D. (1994). “Bilan sur les enquetes eutypiose dans le vignoble europeen,” in Quatrieme Conference Internationale sur les Maladies des Plante, Bordeaux: Annales ANPP, 1265–1276.
Mondello, V., Songy, A., Battiston, E., Pinto, C., Coppin, C., Trotel-Aziz, P., et al. (2018). Grapevine trunk diseases: a review of fifteen years of trials for their control with chemicals and biocontrol agents. Plant Dis. 102, 1189–1217. doi: 10.1094/pdis-08-17-1181-fe
Muckherjee, M., Muckherjee, P. K., Horwitz, A., Zachow, C., Berg, G., Zeilinger, S., et al. (2012). Trichoderma–plant–pathogen interactions: advances in genetics of biological control. Indian J Microbiol. 52, 522–529. doi: 10.1007/s12088-012-0308-5
Mutawila, C., Fourie, P. H., Halleen, F., and Mostert, L. (2011). Grapevine cultivar variation to pruning wound protection by Trichoderma species against trunk pathogens. Phytopathol. Mediterr. 50, S264–S276.
Naznin, H. A., Kiyohara, D., Kimura, M., Miyazawa, M., Shimizu, M., and Hyakumachi, M. (2014). Systemic resistance induced by volatile organic compounds emitted by plant growth-promoting fungi in Arabidopsis thaliana. PLoS One 9:e86882. doi: 10.1371/journal.pone.0086882
Nguyen, N. H., Trotel-Aziz, P., Villaume, S., Rabenoelina, F., Schwarzenberg, A., Nguema-Ona, E., et al. (2020). Bacillus subtilis and Pseudomonas fluorescens trigger common and distinct systemic immune responses in Arabidopsis thaliana depending on the pathogen lifestyle. Vaccines 8:503. doi: 10.3390/vaccines8030503
Nie, P., Li, X., Wang, S., Guo, J., Zhao, H., and Niu, D. (2017). Induced systemic resistance against Botrytis cinerea by Bacillus cereus AR156 through a JA/ET-and NPR1-dependent signaling pathway and activates PAMP-triggered immunity in Arabidopsis. Front. Plant Sci. 8:238. doi: 10.3389/fpls.2017.00238
Niu, D. D., Liu, H. X., Jiang, C. H., Wang, Y. P., Wang, Q. Y., Jin, H. L., et al. (2011). The plant growth–promoting rhizobacterium Bacillus cereus AR156 induces systemic resistance in Arabidopsis thaliana by simultaneously activating salicylate-and jasmonate/ethylene-dependent signaling pathways. Mol. Plant Microbe Interact. 24, 533–542. doi: 10.1094/mpmi-09-10-0213
Ongena, M., and Jacques, P. (2008). Bacillus lipopeptides: versatile weapons for plant disease biocontrol. Trends Microbiol. 16, 115–125. doi: 10.1016/j.tim.2007.12.009
Pacifico, D., Squartini, A., Crucitti, D., Barizza, E., Lo Schiavo, F., Muresu, R., et al. (2019). The role of the endophytic microbiome in the grapevine response to environmental triggers. Front. Plant Sci. 10:1256. doi: 10.3389/fpls.2019.01256
Pertot, I., Prodorutti, D., Colombini, A., and Pasini, L. (2016). Trichoderma atroviride SC1 prevents Phaeomoniella chlamydospora and Phaeoacremonium aleophilum infection of grapevine plants during the grafting process in nurseries. BioControl 61, 257–267. doi: 10.1007/s10526-016-9723-6
Pieterse, C. M., Van Pelt, J. A., Van Wees, S. C., Ton, J., Léon-Kloosterziel, K. M., Keurentjes, J. J., et al. (2001). Rhizobacteria-mediated induced systemic resistance: triggering, signalling and expression. Eur. J. Plant Pathol. 107, 51–61.
Pieterse, C. M. J., Van der Does, D., Zamioudis, C., Leon-Reyes, A., and Van Wees, S. C. M. (2012). Hormonal modulation of plant immunity. Annu. Rev. Cell Dev. Biol. 28, 489–521.
Pieterse, J., Zamioudis, C., Berendsen, L., Weller, M., Van Wees, M., and Bakker, M. (2014). Induced systemic resistance by beneficial microbes. Annu. Rev. Phytopathol. 52, 347–375.
Pinto, C., Sousa, S., Froufe, H., Egas, C., Clément, C., Fontaine, F., et al. (2018). Draft genome sequence of Bacillus amyloliquefaciens subsp. plantarum strain Fito_F321, an endophyte microorganism from Vitis vinifera with biocontrol potential. Stand Genomic Sci. 13, 1–12.
Reis, P., Pierron, R., Larignon, P., Lecomte, P., Abou-Mansour, E., Farine, S., et al. (2019). Vitis methods to understand and develop strategies for diagnosis and sustainable control of grapevine trunk diseases. Phytopathology 109, 916–931. doi: 10.1094/phyto-09-18-0349-rvw
Reveglia, P., Billones-Baaijens, R., Millera Niem, J., Masi, M., Cimmino, A., Evidente, A., et al. (2021). Production of phytotoxic metabolites by Botryosphaeriaceae in naturally infected and artificially inoculated grapevines. Plants 10:802. doi: 10.3390/plants10040802
Rezgui, A., Ben Ghnaya-Chakroun, A., Vallance, J., Bruez, E., Hajlaoui, M. R., Sadfi-Zouaoui, N., et al. (2016). Endophytic bacteria with antagonistic traits inhabit the wood tissues of grapevines from Tunisian vineyards. Biol. Control 99, 28–37. doi: 10.1016/j.biocontrol.2016.04.005
Rueden, C. T., Schindelin, J., Hiner, M. C., DeZonia, B. E., Walter, A. E., Arena, E. T., et al. (2017). ImageJ2: ImageJ for the next generation of scientific image data. BMC Bioinformatics 18:529. doi: 10.1186/s12859-017-1934-z
Schmidt, C. S., Lorenz, D., and Wolf, G. A. (2001). Biological control of the grapevine dieback fungus Eutypa lata I: screening of bacterial antagonists. Phytopathology 149, 427–435.
Schmidt, S. M., and Panstruga, R. (2011). Pathogenomics of fungal plant parasites: what have we learnt about pathogenesis? Curr. Opin. Plant Biol. 14, 392–399. doi: 10.1016/j.pbi.2011.03.006
Sosnowski, M. R., Creaser, M., and Wicks, T. (2004). Evaluating fungicides as pruning wound treatments to control Eutypa dieback. Aust. N.Z. Grapegrow. Winemak. 485, 51–53.
Stempien, E., Goddard, M. L., Wilhelm, K., Tarnus, C., Bertsch, C., and Chong, J. (2017). Grapevine Botryosphaeria dieback fungi have specific aggressiveness factor repertory involved in wood decay and stilbene metabolization. PLoS One 12:e0188766. doi: 10.1371/journal.pone.0188766
Stempien, E., Jean, R., Pierron, G., Adendorff, I., Van Jaarsveld, W. J., Halleen, F., et al. (2020). Host defence activation and root colonization of grapevine rootstocks by the biological control fungus Trichoderma atroviride. Phytopathol. Mediterr. 59, 615–626.
Thambugala, K. M., Daranagama, D. A., Phillips, A. J., Kannangara, S. D., and Promputtha, I. (2020). Fungi vs. fungi in biocontrol: an overview of fungal antagonists applied against fungal plant pathogens. Front. Cell Infect. Microbiol. 10:604923. doi: 10.3389/fcimb.2020.604923
Tiwari, S., and Verma, T. (2019). “Cellulose as a potential feedstock for cellulose enzyme production,” in Approaches to Enhance Industrial Production of Fungal Cellulases, eds M. Srivastava, N. Srivastava, P. W. Ramteke, and P. K. Mishra (Berlin: Springer), 89–116. doi: 10.1007/978-3-030-14726-6_6
Travadon, R., Rolshausen, P. E., Gubler, W. D., Cadle-Davidson, L., and Baumgartner, K. (2013). Susceptibility of cultivated and wild Vitis spp. to wood infection by fungal trunk pathogens. Plant Dis. 97, 1529–1536. doi: 10.1094/pdis-05-13-0525-re
Trotel-Aziz, P., Abou-Mansour, E., Courteaux, B., Rabenoelina, F., Clément, C., Fontaine, F., et al. (2019). Bacillus subtilis PTA-271 counteracts Botryosphaeria dieback in grapevine, triggering immune responses and detoxification of fungal phytotoxins. Front. Plant Sci. 10:25. doi: 10.3389/fpls.2019.00025
Trotel-Aziz, P., Couderchet, M., Biagianti, S., and Aziz, A. (2008). Characterization of new bacterial biocontrol agents Acinetobacter, Bacillus, Pantoea and Pseudomonas spp. mediating grapevine resistance against Botrytis cinerea. Environ. Exp. Bot. 64, 21–32. doi: 10.1016/j.envexpbot.2007.12.009
Úrbez-Torres, J. R. (2011). The status of Botryosphaeriaceae species infecting grapevines. Phytopathol. Mediterr. 50, 5–45.
Úrbez-Torres, J. R., Tomaselli, E., Pollard-Flamand, J., Boulé, J., Gerin, D., and Pollastro, S. (2020). Characterization of Trichoderma isolates from southern Italy, and their potential biocontrol activity against grapevine trunk disease fungi. Phytopathol. Mediterr. 59, 425–439.
Van der Does, D., Leon-Reyes, A., Koornneef, A., Van Verk, M. C., Rodenburg, N., Pauwels, L., et al. (2013). Salicylic acid suppresses jasmonic acid signaling downstream of SCFCOI1-JAZ by targeting GCC promoter motifs via transcription factor ORA59. Plant Cell 25, 744–761. doi: 10.1105/tpc.112.108548
Van der Ent, S., Van Wees, S. C., and Pieterse, C. M. (2009). Jasmonate signaling in plant interactions with resistance-inducing beneficial microbes. Phytochemistry 70, 1581–1588. doi: 10.1016/j.phytochem.2009.06.009
Van Peer, R., Niemann, G. J., and Schippers, B. (1991). Induced resistance and phytoalexin accumulation in biological control of Fusarium wilt of carnation by Pseudomonas sp. strain WCS 417 r. Phytopathology. 81, 728–734. doi: 10.1094/phyto-81-728
Van Wees, S. C., Van der Ent, S., and Pieterse, C. M. (2008). Plant immune responses triggered by beneficial microbes. Curr Opin Plant Biol. 11, 443–448. doi: 10.1016/j.pbi.2008.05.005
Velásquez, A. C., Castroverde, C. D. M., and He, S. Y. (2018). Plant–pathogen warfare under changing climate conditions. Curr. Biol. 28, R619–R634.
Verhagen, B. W., Glazebrook, J., Zhu, T., Chang, H. S., Van Loon, L. C., and Pieterse, C. M. (2004). The transcriptome of rhizobacteria-induced systemic resistance in Arabidopsis. Mol Plant Microbe Interact. 17, 895–908. doi: 10.1094/mpmi.2004.17.8.895
Vinale, F., Sivasithamparam, K., Ghisalberti, E. L., Marra, R., Barbetti, M. J., Li, H., et al. (2008). A novel role for Trichoderma secondary metabolites in the interactions with plants. Physiol Mol Plant Path. 72, 80–86. doi: 10.1016/j.pmpp.2008.05.005
Waghunde, R. R., Shelake, R. M., and Sabalpara, A. N. (2016). Trichoderma: a significant fungus for agriculture and environment. Afr J Agric Res. 11, 1952–1965. doi: 10.5897/ajar2015.10584
Wei, G., Kloepper, J. W., and Tuzun, S. (1991). Induction of systemic resistance of cucumber to Colletotrichum orbiculare by select strains of plant growth-promoting rhizobacteria. Phytopathology 81, 1508–1512. doi: 10.1094/phyto-81-1508
Weller, D. M. (1988). Biological control of soilborne plant pathogens in the rhizosphere with bacteria. Annu. Rev. Phytopathol. 26, 379–407. doi: 10.1146/annurev.py.26.090188.002115
Yacoub, A., Magnin, N., Gerbore, J., Haidar, R., Bruez, E., Compant, S., et al. (2020). The biocontrol root-oomycete, Pythium Oligandrum, triggers grapevine resistance and shifts in the transcriptome of the trunk pathogenic fungus, Phaeomoniella chlamydospora. Int. J. Mol. Sci. 21:6876. doi: 10.3390/ijms21186876
Keywords: Chardonnay, Tempranillo, biocontrol, Neofusicoccum parvum, plant immunity, synergistic effect
Citation: Leal C, Richet N, Guise J-F, Gramaje D, Armengol J, Fontaine F and Trotel-Aziz P (2021) Cultivar Contributes to the Beneficial Effects of Bacillus subtilis PTA-271 and Trichoderma atroviride SC1 to Protect Grapevine Against Neofusicoccum parvum. Front. Microbiol. 12:726132. doi: 10.3389/fmicb.2021.726132
Received: 16 June 2021; Accepted: 08 September 2021;
Published: 14 October 2021.
Edited by:
Fred Asiegbu, University of Helsinki, FinlandReviewed by:
Pierluigi Reveglia, University of Foggia, ItalyDanai Gkizi, Agricultural University of Athens, Greece
Regina Billones-Baaijens, Charles Sturt University, Australia
Copyright © 2021 Leal, Richet, Guise, Gramaje, Armengol, Fontaine and Trotel-Aziz. This is an open-access article distributed under the terms of the Creative Commons Attribution License (CC BY). The use, distribution or reproduction in other forums is permitted, provided the original author(s) and the copyright owner(s) are credited and that the original publication in this journal is cited, in accordance with accepted academic practice. No use, distribution or reproduction is permitted which does not comply with these terms.
*Correspondence: Patricia Trotel-Aziz, patricia.trotel-aziz@univ-reims.fr