- Department of Food Science and Biotechnology, Sungkyunkwan University, Suwon, South Korea
Effects of Latilactobacillus sakei ADM14 on changes in lipid metabolism and fecal microbiota composition were studied in high-fat diet (HFD) mouse model. The mice were divided into three groups: normal diet (ND), high-fat diet (HD), and HFD plus L. sakei ADM14 (HDA). Oral administration of L. sakei ADM14 daily for 10weeks decreased body weight gain, fat tissue mass, and liver weight in mice and reduced the size of histologically stained liver adipocytes. In addition, serum total cholesterol, triglycerides, and blood glucose decreased significantly. Latilactobacillus sakei ADM14 regulated the expression of genes related to lipid metabolism in epididymal adipose tissue and liver and induced changes in the composition of fecal microbiota, thereby improving energy harvests and changing metabolic disorder-related taxa. A significant decrease (p<0.05) in the Firmicutes to Bacteroidetes ratio was found in the HDA group compared to the HD group, particularly due to the difference in the relative abundance of the Bacteroidetes between the two groups over 10weeks. Differences in proportions of some taxa reported to have correlation with obesity were also found between HD and HDA groups. These results suggest that L. sakei ADM14 can have a positive effect on metabolic disorders such as obesity and fatty liver through effective regulation of host lipid metabolism and gut microbiota.
Introduction
Obesity has emerged as an important health problem through a rapid increase in incidence worldwide. Excessive body weight gain and body fat accumulation pose a serious threat, leading to the onset of obesity and metabolic disorders such as diabetes and cardiovascular disease (Bastien et al., 2014). A high-fat diet (HFD) affects elevated serum cholesterol and triglycerides (TG) and increases the risk of chronic low-grade inflammation from obesity, along with adipose tissue enlargement (Dewulf et al., 2011). The influence of metabolic disorders in combination with the HFD can also damage the liver. Recently, the association and risk of obesity with nonalcoholic fatty liver disease (NAFLD) has received a lot of attention. NAFLD includes a variety of symptoms ranging from simple steatosis to nonalcoholic fatty hepatitis, cirrhosis, or hepatocellular carcinoma (Wong et al., 2015), and more than 80% of obese individuals in western countries are affected by NAFLD (Younossi, 2019). Recently, several studies have focused on gut microbiota as a new environmental factor contributing to the relationship between obesity and NAFLD (Le Roy et al., 2013; Tilg et al., 2016; Da Silva et al., 2018). The gut microbial community consists of thousands of bacterial species, coexisting with the host, and has a significant influence on the physiology and metabolism of the host (Turnbaugh et al., 2006; Ridaura et al., 2013). Recently, a link between the effects of choline deficiency on fatty liver development and changes in the human gut microbiota was confirmed (Spencer et al., 2012), and it was revealed that changes in the gut microbiota regulate the progression of NAFLD (Buzzetti et al., 2016). Thus, the regulation of gut microbial communities may suggest new therapeutic strategies in the management of metabolic diseases such as obesity and NAFLD. Probiotics regulate the gut microbiome and have proven beneficial effects on metabolic symptoms. They are also known to be effective in improving lipid profiles and hyperlipidemia, while affecting lipid metabolism (Roller et al., 2004; Sun and Buys, 2015).
In our previous study, a lactic acid bacterium, designated ADM14, was found to have anti-adipogenic effect by reducing significantly intracellular triglyceride content on 3T3-L1 adipocytes and by decreasing the expression of five adipogenic marker genes (Won et al., 2020a). The strain ADM14 was identified as a member of Lactobacillus sakei in the study of Won et al. (2020a), but L. sakei has been recently reclassified as L. sakei (Zheng et al., 2020). The aim of this study was to investigate the effect of L. sakei ADM14 on host lipid metabolism, fatty liver, and metabolic problems in HFD mouse model. In addition, changes in gut microbiota structure on the host were analyzed through fecal microbiome analysis the relationship between the gut-liver axis was evaluated.
Materials and Methods
Bacterial Strain Preparation and Growth Conditions
Latilactobacillus sakei (L. sakei) ADM14 was cultured using De Man-Rogosa-Sharpe (MRS, BD Difco, Sparks, MD, United States) agar at 30°C for 24h and was stored at −80°C in 20% glycerol (v/v; Georgiachem, GA, United States) for cryopreservation until it was used in experiments.
Animals, Diets, and Experimental Design
The animal care and studies were conducted in accordance with the Animal Care and Use Committee of the College of Biotechnology at Sungkyunkwan University (approval date: 07-09-2019, approval number: SKKUIACUC-18-04-14-3). Male C57BL/6J mice aged 5weeks were purchased from RaonBio Inc. (Yongin, Republic of Korea) and maintained under controlled temperature and humidity (24±2°C, 50±10%) with a 12h light/dark cycle. After a 1-week acclimation period, 6-week-old mice were randomly divided into three groups (n=8): normal diet (ND), high-fat diet (HD), and high-fat diet plus L. sakei ADM14 (HDA, 108–109CFU per 200μl 0.85% saline). The ND group received a normal chow diet (10% of energy from fat, 16.1kJ, RaonBio Inc.) for 10weeks and the HD group received a HFD (60% of energy from fat, 21.9kJ, RaonBio Inc.) for 10weeks (Supplementary Table S1). The HDA group received a HFD for 10weeks and L. sakei ADM14 by daily oral administration. Food and water were fed ad libitum. Live L. sakei ADM14 was administered by oral gavage at a concentration of 108–109CFU per 200μl 0.85% saline daily as recommended by the WHO and the Korea Food and Drug Administration. During the experiment, food intake and body weight were measured weekly. Fecal samples were collected weekly and stored at −80°C. The food efficiency ratio (FER) was expressed as total body weight gained from the diet divided by total diet consumed during the animal experiments, and total calorie intake was calculated as the total amount of diet consumed during the animal experiment multiplied by the caloric value of the diet. The mice that died during the experiment of 10weeks were excluded, and the mice with the maximum and minimum values in weight were also excluded. Finally, 4–6 mice per group were used in further analyses. At the end of the experiment, mice were fasted for 16h and sacrificed under anesthesia. After sacrifice, visceral organs (liver, spleen, and kidney) and epididymal and subcutaneous fat pad were collected and weighed. Epididymal fat pads and liver were preserved by freezing in liquid nitrogen for genetic analysis. A portion of the liver was frozen and stained with Oil Red O (Sigma-Aldrich, St. Louis, MO, United States) for histological study. Blood was collected via cardiac puncture and was centrifuged for 10min at 3,000rpm for serum separation.
Serum Analysis
Alanine transaminase (ALT), aspartate transaminase (AST), total cholesterol, glucose, triglyceride (TG), high-density lipoprotein (HDL), and low-density lipoprotein (LDL) levels were measured using a biochemical automatic analyzer (AU480, Beckman Coulter Inc., Brea, CA, United States) according to the manufacturer’s instructions.
RNA Extraction and Quantitative Real-Time PCR
Total RNA was extracted from epididymal fat tissue and liver using an RNeasy Mini Kit (Qiagen, Hilden, Germany) and TRIzol (Invitrogen, Carlsbad, CA, United States) according to the manufacturer’s protocol. First-strand complementary DNA was synthesized using a Veriti™ 96-Well Thermal Cycler machine (Thermo Scientific, Waltham, MA, United States) by mixing the extracted total RNA and ReverTra Ace Master Mix (Toyobo, Osaka, Japan). A mixture of Power SYBR Premix ExTaq (RP041A; Takara, Shiga, Japan), primers and cDNA was used for amplification using a thermal cycler machine (Takara). Gene expression was normalized by a housekeeping gene, 36B4. The primer sequences for the genes are shown in Supplementary Table S2.
16S rRNA Gene Sequence Analysis of Gut Microbiota and Bioinformatics
For microbiome analysis, total genomic DNA was extracted from fecal samples using a QIAamp DNA Stool Mini Kit (Qiagen) according to the manufacturer’s protocol. The first amplification from the total genomic DNA was performed in the V3 to V4 regions with primer sequences of 16S rRNA gene as shown in Supplementary Table S3 (Fadrosh et al., 2014). A second amplification was performed by attaching an Illumina NexTera barcode to the first amplification product. Sequencing was performed according to the method of Chunlab Inc. (Seoul, Republic of Korea) using an Illumina MiSeq sequencing system (Illumina, San Diego, CA, United States). Taxonomic profiling and sequencing data analysis were performed using the Illumina platform (Chunlab Inc.). Alpha diversity was calculated using OTU information and expressed as the Chao 1 and Shannon index. The microbiota structure between groups was measured to principal coordinate analysis (PCoA) at the genus level using the beta diversity index. The linear discriminant analysis effect size (LEfSe) method was performed using latent Dirichlet allocation (LDA) score 3.0 cutoff and value of p<0.05.
Statistical Analysis
Statistical analyses were conducted using SPSS ver. 19.0 (SPSS Inc., Chicago, IL, United States). Data are presented as mean±SEM. Significant differences in the gene expression of tissue between animal experimental groups were determined by unpaired Student’s t-test. For relative abundance analysis of the gut microbiome, significant differences between groups were determined using the Wilcoxon rank-sum test. Values were considered statistically significant when p<0.05.
Results
Effects of L. sakei ADM14 on Body, Liver, and Fat Tissue Weight
Changes in weight between the groups of mice were observed for 10weeks. The weight of group fed the HFD increased more rapidly (Figure 1A). After 10weeks, the average weight gains in the ND, HD, and HDA groups were 6.31±0.56, 15.03±1.25, and 11.30±1.01g, respectively. Compared with the HD group, the weight gain of the HDA group decreased significantly by 24.7% (p<0.05). While the total caloric intake was significantly different (ND vs. HD, p<0.01; ND vs. HDA, p<0.05) between the ND and HFD intake groups, there was no significant difference between the HD and HDA groups (Figure 1B). The FER increased significantly (p<0.01) in HD compared to ND, but there was no significant difference despite a 12.9% decrease in HDA compared to HD (Figure 1C). No significant weight changes were observed in the kidneys and spleen, of each group (Figure 1D). There was a significant increase (p<0.01) in the mass of epididymal fat and subcutaneous fat in HD compared to ND (Figure 1D). In contrast, there was a significant decrease of 44.5% in epididymal fat (p<0.01) and 33.8% in subcutaneous fat (p<0.05) in HDA compared to HD (Figure 1D). The liver weight was not significantly different between ND and HD, but a significant decrease (p<0.05) was observed in HDA compared to HD (Figure 1D). In the histological analysis of the liver using Oil Red O staining, it was observed that the size of stained adipocytes decreased in HDA compared to HD (Figure 1E).
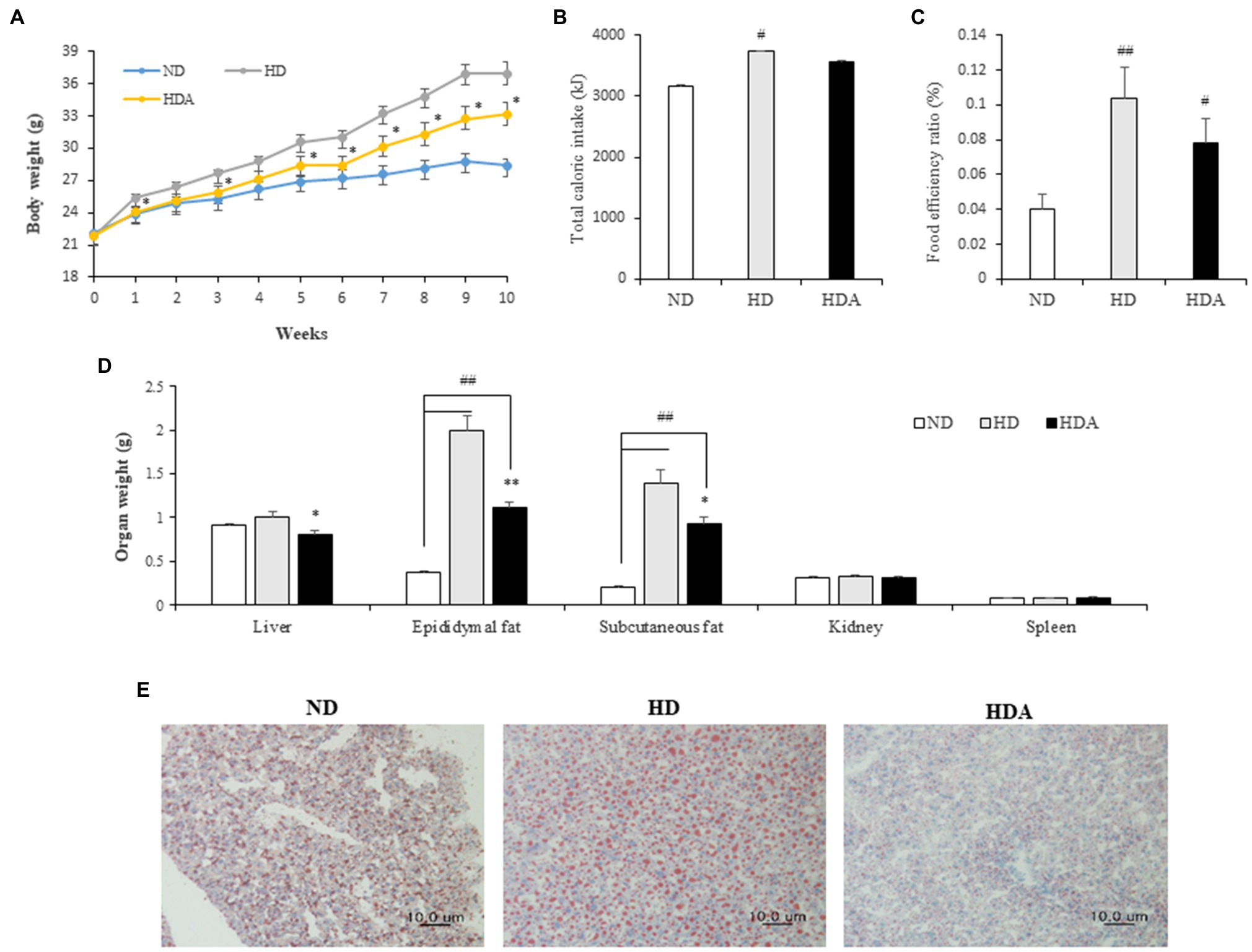
Figure 1. Effect of administering Lactobacillus sakei ADM14 on body indicators in high-fat diet (HFD) mouse model. (A) Weight change of mice groups. (B) Total caloric intake for 10weeks. (C) Food efficiency ratio (FER) for 10weeks. (D) Organs weights of mice after sacrifice. (E) Histological analysis of liver stained with Oil Red O. ND, normal diet group; HD, high-fat diet group; and HDA, high-fat diet plus L. sakei ADM14 group. The results are shown as mean±SEM (n=5–7). Significant differences between HD and ND are indicated as #p<0.05 and ##p<0.01. Significant differences between HDA and ND are indicated as #p<0.05 and ##p<0.01. Significant differences between HD and HDA are indicated as *p<0.05 and **p<0.01.
Effects of L. sakei ADM14 on Serum Biochemical Parameters
The concentrations of total cholesterol, glucose, TG, and HDL of the serum in the HD group were significantly (p<0.01) higher than those of the ND group (Table 1). Compared with the HD group, total cholesterol, glucose, and TG of the serum decreased in the HDA group administered with L. sakei ADM14 (Table 1). Total cholesterol decreased by 17.0% (p<0.05), glucose decreased by 29.9% (p<0.05), and TG decreased by 34.2% (p<0.01). Compared to the HD group, the HDA group showed a significant increase (p<0.05) in HDL, and the LDL decreased by 9.0% but there was no significant difference between them. ALT and AST were analyzed and there was no significant difference among the groups (Supplementary Figure S1).
Effects of L. sakei ADM14 on Genes of Lipid Metabolism in Epididymal Fat Tissue and Liver
The results for the mRNA expression of genes for lipid metabolism in epididymal fat tissue are shown in Figure 2. The expressions of genes related to lipid metabolism in epididymal fat tissue of HD group increased when compared to the ND group (Figure 2). Compared to the HD group, the HDA group administered with L. sakei ADM14 significantly downregulated (p<0.01) peroxisome proliferator-activated receptor gamma (Pparγ), sterol regulatory element-binding protein-1C (Srebp1C), and fatty acid synthetase (Fas), of four genes related to fatty acid synthesis (Figure 2B). In addition, the expression of adipocyte protein 2 (aP2) was also significantly decreased (p<0.01) in the HDA compared to the HD (Figure 2A). Likewise, the expressions of Pparγ-activated genes, lipoprotein-lipase (Lpl), and cluster of differentiation 36 (CD36) were significantly decreased (p<0.05) in the HDA compared to the HD (Figure 2A). The expressions of tumor necrosis factor-alpha (TNFα; p<0.01), interleukin 6 (IL-6; p<0.01), and monocyte chemoattractant protein-1 (MCP-1; p<0.05) related to pro-inflammatory cytokines in the HDA were significantly decreased compared to the HD group (Figure 2C).
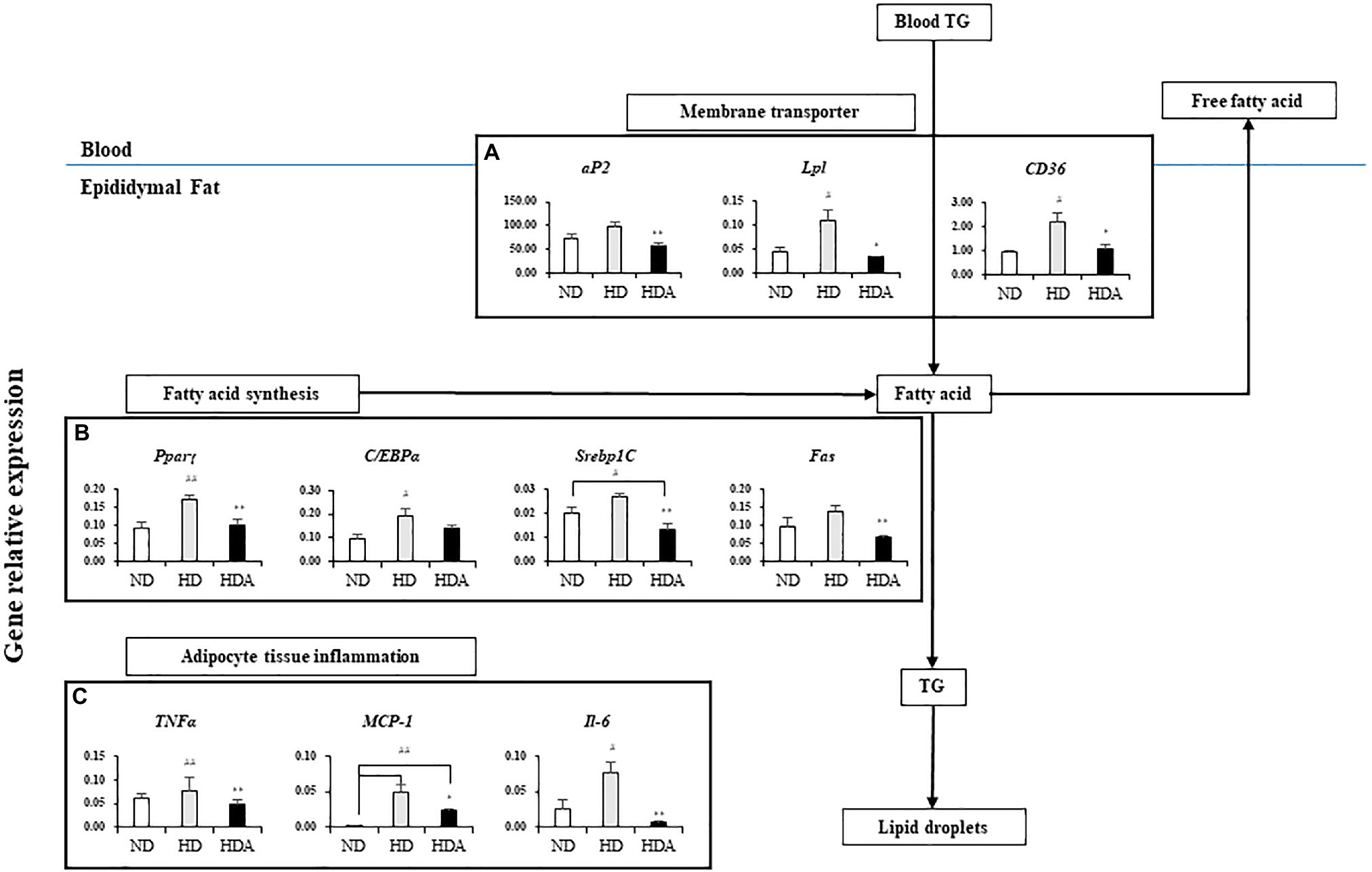
Figure 2. Effects of administering L. sakei ADM14 on the transcription of genes related to lipid metabolism in epididymal fat tissue. The results are shown as mean±SEM (n=5). Significant differences between HD and ND are indicated as #p<0.05 and ##p<0.01. Significant differences between HDA and ND are indicated as #p<0.05 and ##p<0.01. Significant differences between HD and HDA are indicated as *p<0.05 and **p<0.01.
To investigate the effect of L. sakei ADM14 on lipid metabolism in the liver, expression of overall related genes was investigated (Figure 3). There were also differences in the expressions of genes related to lipid metabolism in the liver between ND and HDA groups (Figure 3). Compared with HD, the lipid production-related genes, Pparγ (p<0.05), Srebp1C (p<0.01), Fas (p<0.05), and acetyl-CoA carboxylase 1 (Acc1; p<0.05), were significantly decreased in HDA (Figure 3D). In addition, the significantly increased expressions (p<0.05) of β-oxidation-related genes, carnitine palmitoyltransferase1 alpha (Cpt1α) and peroxisome proliferator-activated receptor alpha (Pparα), were found in HDA (Figure 3B). Carbohydrate-response element-binding proteins, ChREBPα (p<0.01) and ChREBPβ (p<0.05), which are major transcriptional regulatory factors that are activated by glucose catabolism in the liver and regulates lipogenesis, were observed to be significantly downregulated in HDA (Figure 3A). There was no significant change in the level of Lpl expression, but CD36 expression showed a significant decrease (p<0.05) in HDA (Figure 3C). As a result of observing the expression of diglyceride acyltransferase (Dgat) that catalyzes TG formation, it was found that both Dgat1 (p<0.05) and Dgat2 (p<0.01) were significantly decreased (Figure 3E).
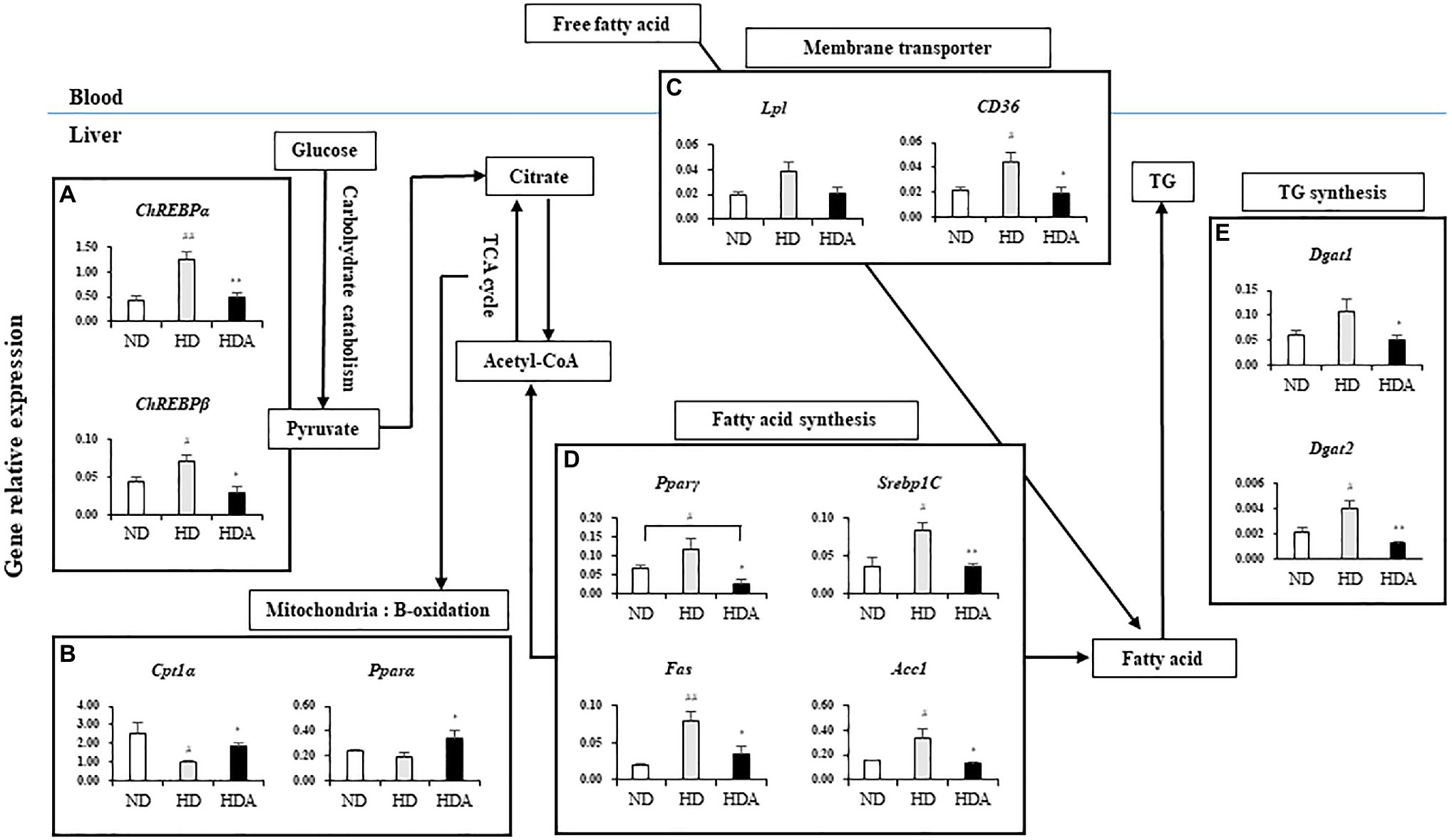
Figure 3. Effects of administered L. sakei ADM14 on the transcription of genes related to glucose and lipid metabolism in the liver. The results are shown as mean±SEM (n=5). Significant differences between HD and ND are indicated as #p<0.05 and ##p<0.01. Significant differences between HDA and ND are indicated as #p<0.05 and ##p<0.01. Significant differences between HD and HDA are indicated as *p<0.05 and **p<0.01.
Effects of L. sakei ADM14 on Changes in Ratio and Composition of Fecal Microbiota
On analyzing alpha diversity, there was no significant difference in the Chao1 index of each group, but there was a significant increase (p<0.05) in the HDA group in the Shannon index compared to the ND group (Supplementary Figure S2). On the other hand, there was no significant change between the HD group and the HDA group.
Changes of fecal microbiota in the phylum level were assessed at 1, 6, and 10weeks using each group (Figure 4A). An increase in Firmicutes and a decrease in Bacteroidetes from 1 to 10weeks were observed in both HD and HDA groups, but each showed a difference in the range of variation. The Firmicutes ratios at 1, 6, and 10weeks were 49.02, 70.55, and 74.98%, respectively, in the HD group and 43.58, 70.02, and 74.04%, respectively, in the HDA group. There was no significant difference in the Firmicutes ratio between the two groups at 6 and 10weeks. The Bacteroidetes ratios at 1, 6, and 10weeks were 37.14, 22.37, and 3.56%, respectively, in the HD group and 38.49, 19.20, and 16.72%, respectively, in the HDA group. At 10weeks, the Bacteroidetes ratios of the two groups showed a significant difference (p<0.05). In addition, the Proteobacteria ratios at 1, 6, and 10weeks were 2.95, 5.28, and 14.16%, respectively, in the HD group and 2.01, 9.50, and 7.21% at 1, 6, and 10weeks, respectively, in the HDA group. The HD group showed a steady increase in the Proteobacteria ratios, but the HDA group decreased again from 6 to 10weeks in the Proteobacteria ratios. The proportions of the three major phyla, Firmicutes, Bacteroidetes, and Proteobacteria, at 10weeks of HD and HDA groups were similar with those of the two groups shown in cecal microbiota from our previous study (Won et al., 2020b), even though the proportion of the Proteobacteria in HDA group is higher than that of Bacteroidetes in the study of Won et al. (2020b). However, the Verrucomicrobia, which was the second major phylum in the cecal microbiota of HD group, was found only as minor phylum at 6 and 10weeks in fecal microbiota of this study (Won et al., 2020b; Figure 4A).
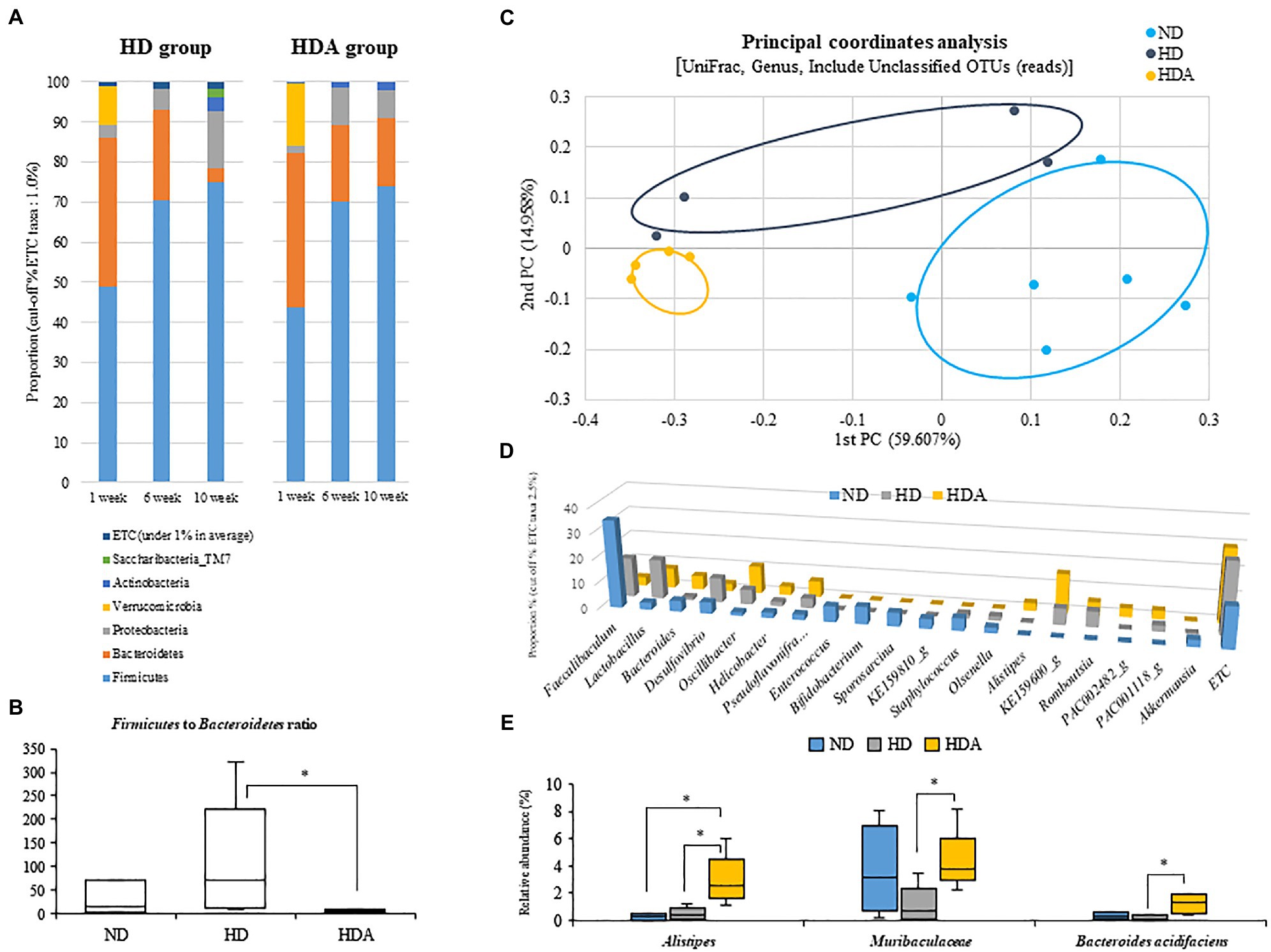
Figure 4. Effect of administered L sakei ADM14 on fecal microbiome composition. (A) Changes in fecal microbiota in HD and HDA groups at the phylum level in 1, 6, and 10weeks. (B) Firmicutes to Bacteroidetes ratios between groups at 10weeks. (C) Fecal microbiota composition of groups at 10weeks is shown by the genus level UniFrac principal coordinates analysis (PCoA). (D) Main fecal microbiota composition of genus level (cut off taxa 2.5%) at 10weeks. (E) The relative abundance of specific taxa in fecal microbiota at 10weeks. The results are shown as mean±SEM (n=4–6). Significant differences between groups are indicated as *p<0.05.
Two dominant phyla, Firmicutes and Bacteroidetes, were compared between the three groups at 10weeks (Figure 4B). The Firmicutes to Bacteroidetes ratio was significantly decreased (p<0.05) in the HDA group compared to the HD group as well as ND group. At the genus level, UniFrac PCoA showed a tendency to separate among the three groups (Figure 4C). The percentage of major genus composition in the ND, HD, and HDA groups were investigated (Figure 4D). The proportions of genera Bacteroides, Oscillibacter, Helicobacter, Pseudoflavonifractor, Alistipes, KE159600_g, PAC002482_g, and PAC001118_g were higher in the HDA group than in other two groups. On the other hand, the proportions of genera Faecalibaculum, Lactobacillus, Desulfovibrio, Staphylococcus, Olsenella, and Romboutsia were lower in the HDA group compared to the HD group. Significant increases (p<0.05) in levels of family Muribaculaceae and Bacteroides acidifaciens were found between the HDA and HD groups (Figure 4E). Significant increase (p<0.05) in genus Alistipes was found in the HDA group compared to the ND and HD groups (Figure 4E). LEfSe analysis was calculated to identify specific bacterial taxa dominant in the HD and HDA groups (Figure 5). Pseudomonas and PAC000677_g were the core genus microbiota of the HD group, and Bacteroides, PAC002482_g, Alistipes, PAC001063_g, PAC000198_g, and PAC001066_g were identified as the dominant genus microbiota of the HDA group.
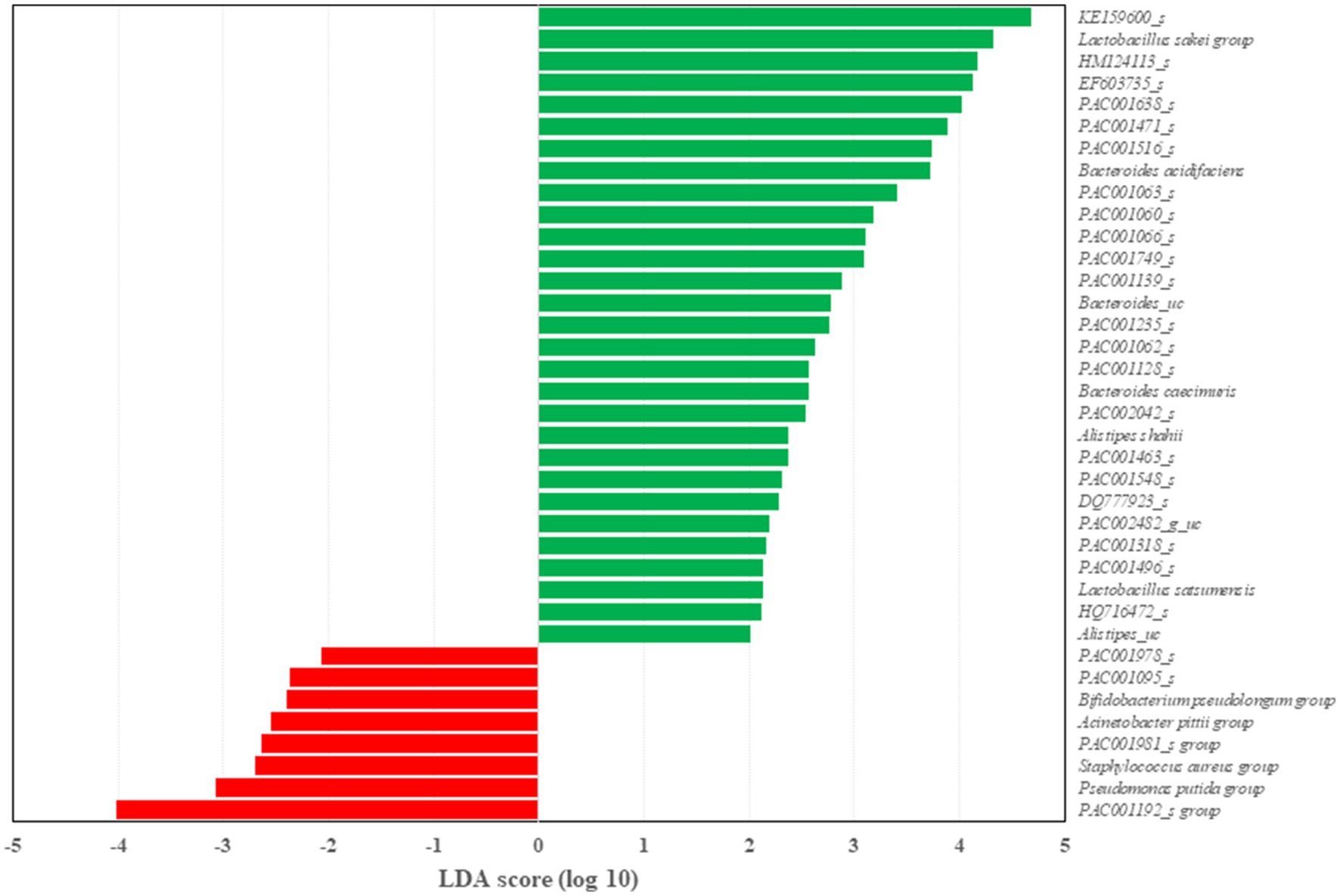
Figure 5. Differentially represented genus between HD and HDA group through linear discriminant analysis effect size (LEfSe) analysis. Latent Dirichlet allocation (LDA) score indicates the effect size. n=4–6 per group.
Discussion
The incidence and severity of obesity and metabolic complications are constantly rising, and drug therapy has the potential to cause adverse effects (Sonnenburg and Bäckhed, 2016; Dahiya et al., 2017). The necessity for alternative therapy is increasing, and attention has been drawn by the effectiveness and utilization of probiotic lactobacilli (Aryana and Olson, 2017). Animal studies and clinical studies have reported the anti-obesity effects of probiotic lactic acid bacteria and improvements in metabolic disorders (Dahiya et al., 2017). In this study, the administration of L. sakei AMD14 suppressed weight gain and reduced body fat mass in a high-fat mouse model as shown in our previous study (Won et al., 2020b). Although, there is no significant difference in total caloric consumption, the difference in physical indicators is confirmed to be an inhibitory effect by L. sakei ADM14 (Figure 1). The reduction in the weight of subcutaneous fat tissue, as well as reduction in the weight of the epididymal fat tissue also observed previous study (Won et al., 2020b), appears to induce the overall decrease in lipid accumulation in mice administered with L. sakei AMD14. In this study, it was found that the weight of the liver was decreased, and as a result of microscopic observation through Oil Red O staining of the liver tissue, a decrease in the size of the adipocytes was observed (Figures 1D,E). This is seen as evidence that the expansion of adipocytes and accumulation of lipids in the liver were inhibited. Cardiovascular disease and lipidemia are major metabolic complications that occur and intensify because of obesity (Cani and Delzenne, 2009). These metabolic disorders are mainly caused by an increase in blood cholesterol and TGs (Yoo and Kim, 2016). The significant increases in total cholesterol, TG, and glucose in serum of the HD group were consistent with the predicted characteristics of obesity. Administration of L. sakei ADM14 suppressed and improved these indicators and confirmed the possibility of preventing symptoms related to lipidemia. The improvement in blood lipids after administration of L. sakei ADM14 could be evaluated on the basis of another case that the probiotic lactic acid bacteria effectively improved the serum markers of high-fat diet mice (Wang et al., 2012).
To confirm the potential mechanism by which L. sakei ADM14 inhibits obesity in a high-fat diet mouse model, genetic expression analysis of epididymal adipose tissue was performed (Figure 2). Adipose tissue is one of the important organs that regulate lipid metabolism (Schneeberger et al., 2015). Adipocyte transcription factors Pparγ and C/EBPα play an important role in the development of adipocytes (Rosen et al., 1999; Jeong et al., 2008), and our results showed a tendency to decrease in the expression of both genes. It has been reported that these transcription factors have a positive correlation with lipid accumulation rates (Park et al., 2009). Additionally, the decreased expression of Srebp1C, which is involved in fatty acid synthesis, and Fas, which is involved in regulating lipid production, suggests that the accumulation of lipids in adipocytes was inhibited. Likewise, aP2, which is related to adipose production, and CD36 and Lpl, which are related to lipid storage showed decreased expression and through this, it could be confirmed that a change occurred in adipose tissue metabolism. The expression of Pparγ, C/EBPα, Fas, aP2, and CD36 in epididymal fat was confirmed to decrease also in this study as shown previously (Won et al., 2020b). From these results, it could be confirmed that administration of L. sakei ADM14 may cause changes in adipose tissue metabolism by affecting transcription factors, lipid production, or lipid storage. Low-grade chronic inflammation of adipose tissue is one of the major factors in obesity-induced insulin resistance and metabolic disorders (Gregor and Hotamisligil, 2011). As the expression of inflammation-related genes in adipose tissue was decreased, it was also confirmed that low-grade inflammation was alleviated (Figure 2; Won et al., 2020b).
Nonalcoholic fatty liver disease is representative of the symptoms induced by obesity (Canfora et al., 2019). The balance of lipid metabolism in the liver is disrupted, causing excessive lipogenesis and hepatic lipid accumulation (Rotman and Sanyal, 2017; Friedman et al., 2018). NAFLD can lead to liver damage and develop into fatty hepatitis, cirrhosis, or hepatocellular carcinoma (Le Roy et al., 2013). Fundamentally, the increase in the expression of major genes related to lipogenesis in liver tissue seems to be one of the biggest causes. Glucose absorbed in the intestine regulates the transcription of lipogenic genes through ChREBP (Kawaguchi et al., 2001). In our results, it appears that the decreased expression of ChREBPα/β resulted in the decreased expression of adipogenic genes, thus affecting the reduction of hepatic lipid accumulation in the liver. The expression of fatty acid synthesis-related genes such as Pparγ, Fas, and Acc1 decreased with the inhibition of glucose catabolism. The expression of Srebp1C targeting Fas and Acc1 also decreased. The main characteristic of NAFLD is the accumulation of TG in the liver (Eslamparast et al., 2013). In a state of excess energy such as obesity, the activity of Dgat, which catalyzes TG synthesis, increases (Choi and Diehl, 2008). The increase in free fatty acid delivered to the liver also upregulates the synthesis of TG and ultimately increases the likelihood of developing NAFLD (Choi and Diehl, 2008). The results of our study showed that the expression of Dgat1 and Dgat2 was reduced in the final stage of TG synthesis. Likewise, a reduction in the uptake of free fatty acid through the reduction of Lpl and CD36 expression seems to affect the process of TG synthesis. In our results, it was confirmed that the expression of Dgat1 and Dgat2, which catalyzes TG synthesis, was decreased. Likewise, it appears that a reduction in the uptake of free fatty acid to the liver through a decrease in Lpl and CD36 expression influenced the TG synthesis. β-oxidation is one of the key pathways for fatty acid metabolism in the liver (Lu et al., 2014). Pparα and Cpt1α were upregulated in the HDA group compared to the HD group. This has been shown to upregulate lipolysis and fatty acid oxidation. In this study, although NAFLD is shown not to be caused by high-fat diet, genetic analysis in the mechanisms of lipid metabolism in the liver suggests that L. sakei ADM14 downregulates lipid production and upregulates fatty acid oxidation-related genes, while regulating hepatocytes lipid accumulation and a reduction in TG synthesis (Figure 3).
A high-fat diet can contribute to changes in the gut microbiome and can lead to metabolic imbalances caused by gut microbiota dysbiosis (Wang et al., 2011; Wu et al., 2017). In addition, gut microbiota dysbiosis has recently been reported to be associated with liver diseases as well as metabolic diseases such as obesity (Da Silva et al., 2018; Kong et al., 2018). Therefore, for the prevention and treatment of metabolic diseases, an analysis targeting the gut microbiota must be conducted (Li et al., 2020). As a result of analyzing the fecal microbiota, we observed a significant decrease (p<0.05) in the Firmicutes to Bacteroidetes ratio in the HDA group compared to the HD group at the phylum level as also shown in cecal microbiota from our previous study (Won et al., 2020b; Figure 2). The main cause was the difference in the relative abundance of the Bacteroidetes between HDA and HD groups over 10weeks. In particular, relative abundance of the Bacteroidetes after 10weeks from the HDA group was also similar with that of cecum shown in the study of Won et al. (2020b). In the analyses of the fecal microbiota at three different times (1, 6, and 10weeks), the increase in Firmicutes ratios from 1 to 6weeks was much greater than that from 6 to 10weeks in both the HD and HDA groups, whereas the decrease in Bacteroidetes ratios was greater from 6 to 10weeks in the HD group but was greater from 1 to 6weeks in the HDA group (Figure 4A). The Proteobacteria ratios from 6 to 10weeks were decreased in the HDA group unlike in the HD group (Figure 4A). The administration of L. sakei ADM14 from 1 to 10weeks does not affect Firmicutes ratios between the HD and HDA groups but affect the ratios of Bacteroidetes and Proteobacteria in the HDA group. In the study of Won et al. (2020b), administration of L. sakei ADM14 was shown to increase Firmicutes ratios in the cecal microbiota. These results suggest that the failure to increase the Firmicutes ratio in fecal microbiota despite the administration of L. sakei might be due to colonization of L. sakei ADM14 in the intestine. The Firmicutes can produce more harvestable energy than Bacteroidetes, and when the relative abundance of Firmicutes increases and Bacteroidetes decreases, the absorption of calories in the digestive tract increases (Turnbaugh et al., 2006; Komaroff, 2017). This difference in terms of energy harvesting promotes metabolic diseases such as obesity. In our results, the administration of L. sakei ADM14 induces a change in the proportion of the dominant taxa at the phylum level and suggests that differences in energy resources may occur. At the genus level, Bacteroides, Oscillibacter, and Alistipes, which have higher proportions in the HDA group compared to the HD group, were reported to have a negative correlation with obesity (Dahiya et al., 2017; Thingholm et al., 2019). Bacteroides and Alistipes were also observed to have higher proportions in cecal sample of the HDA group in previous study by Won et al. (2020b). Bacteroides and Alistipes are known to be the major producers of short chain fatty acids such as acetic acid and butyric acid (Borton et al., 2017; Yin et al., 2018). In fecal microbiota, members of genus Alistipes, family Muribaculaceae and species Bacteroides acidifaciens were notable bacteria whose relative abundance was significantly changed by the administration of L. sakei ADM14 (Figure 4E). The genus Alistipes is found at low levels in the intestines of patients with hepatocellular carcinoma, colitis, and NAFLD and has been reported to have protective effects against some diseases including liver fibrosis, colitis, and cardiovascular disease (Parker et al., 2020). Muribaculaceae, previously known as family S24-7, was reported as representative bacteria with low abundance in mice fed a high-fat diet (Lagkouvardos et al., 2016). Bacteroides acidifaciens has been reported to be effective in preventing metabolic disorders such as obesity by controlling the host lipid metabolism (Yang et al., 2017). Overall, L. sakei ADM14 changed the composition of gut microbiota, suggesting the possibility of preventing metabolic disorders caused by dysbiosis. In particular, an increase in the abundance of Alistipes through gut microbiota modification helps to prevent diseases such as fatty liver through gut-liver axis modulation.
Conclusion
In this study, Latilactobacillus sakei ADM14 was found to inhibit adipogenesis by regulating the expression level of lipid metabolism in adipose tissue and liver tissue in a high-fat diet mouse model. In addition, fecal microbial community analysis suggested that the composition and ratio of gut microbiota were modified to induce changes in energy harvest, and the possibility of preventing metabolic disorders through modulation of the gut-liver axis. To our knowledge, there are few studies to evaluate the relationships among obesity, lipid metabolism in both epididymal fat and liver, and fecal microbiomes at different times by probiotic strain, particularly lactic acid bacteria isolated from kimchi. Further research and clinical studies are needed to evaluate their applicability and effectiveness in human.
Data Availability Statement
The datasets presented in this study can be found in online repositories. The names of the repository/repositories and accession number(s) can be found at: https://www.ncbi.nlm.nih.gov/ (PRJNA751416, PRJNA751419, PRJNA751420, PRJNA751421, PRJNA751431, and PRJNA751433).
Ethics Statement
The animal study was reviewed and approved by Animal Care and Use Committee of the College of Biotechnology at Sungkyunkwan University (approval number: SKKUIACUC-18-04-14-3).
Author Contributions
S-MW and MS performed the experiments of this study and reviewed the manuscript. MK supported some experiments of this study. KP discussed the results of this study. J-HY designed this study and wrote manuscript. All authors contributed to the article and approved the submitted version.
Funding
This work was supported by the “Cooperative Research Program for Agriculture Science and Technology Development (project no. PJ015247)” of the Rural Development Administration, Republic of Korea and a Research Initiative Program of Sungkyunkwan University, Republic of Korea.
Conflict of Interest
The authors declare that the research was conducted in the absence of any commercial or financial relationships that could be construed as a potential conflict of interest.
Publisher’s Note
All claims expressed in this article are solely those of the authors and do not necessarily represent those of their affiliated organizations, or those of the publisher, the editors and the reviewers. Any product that may be evaluated in this article, or claim that may be made by its manufacturer, is not guaranteed or endorsed by the publisher.
Supplementary Material
The Supplementary Material for this article can be found online at: https://www.frontiersin.org/articles/10.3389/fmicb.2021.746601/full#supplementary-material
References
Aryana, K. J., and Olson, D. W. (2017). A 100-year review: yogurt and other cultured dairy products. J. Dairy Sci. 100, 9987–10013. doi: 10.3168/jds.2017-12981
Bastien, M., Poirier, P., Lemieux, I., and Després, J. P. (2014). Overview of epidemiology and contribution of obesity to cardiovascular disease. Prog. Cardiovasc. Dis. 56, 369–381. doi: 10.1016/j.pcad.2013.10.016
Borton, M. A., Sabag-Daigle, A., Wu, J., Solden, L. M., and Wrighton, K. C. (2017). Chemical and pathogen-induced inflammation disrupt the murine intestinal microbiome. Microbiome 5, 1–15. doi: 10.1186/s40168-017-0264-8
Buzzetti, E., Pinzani, M., and Tsochatzis, E. A. (2016). The multiple-hit pathogenesis of non-alcoholic fatty liver disease (NAFLD). Metabolism 65, 1038–1048. doi: 10.1016/j.metabol.2015.12.012
Canfora, E. E., Meex, R. C. R., Venema, K., and Blaak, E. E. (2019). Gut microbial metabolites in obesity, NAFLD and T2DM. Nat. Rev. Endocrinol. 15, 261–273. doi: 10.1038/s41574-019-0156-z
Cani, P., and Delzenne, N. (2009). The role of the gut microbiota in energy metabolism and metabolic disease. Curr. Pharm. Des. 15, 1546–1558. doi: 10.2174/138161209788168164
Choi, S. S., and Diehl, A. M. (2008). Hepatic triglyceride synthesis and nonalcoholic fatty liver disease. Curr. Opin. Lipidol. 19, 295–300. doi: 10.1097/MOL.0b013e3282ff5e55
Da Silva, H. E., Teterina, A., Comelli, E. M., Taibi, A., Arendt, B. M., and Allard, J. P. (2018). Nonalcoholic fatty liver disease is associated with dysbiosis independent of body mass index and insulin resistance. Sci. Rep. 8:1466. doi: 10.1038/s41598-018-19753-9
Dahiya, D. K., Renuka, Puniya, M., Shandilya, U. K., Dhewa, T., and Shukla, P. (2017). Gut microbiota modulation and its relationship with obesity using prebiotic fibers and probiotics: a review. Front. Microbiol. 8:563. doi: 10.3389/fmicb.2017.00563
Dewulf, E. M., Cani, P. D., Neyrinck, A. M., Possemiers, S., and Delzenne, N. M. (2011). Inulin-type fructans with prebiotic properties counteract GPR43 overexpression and PPARγ-related adipogenesis in the white adipose tissue of high-fat diet-fed mice. J. Nutr. Biochem. 22, 712–722. doi: 10.1016/j.jnutbio.2010.05.009
Eslamparast, T., Eghtesad, S., Hekmatdoost, A., and Poustchi, H. (2013). Probiotics and nonalcoholic fatty liver disease. Middle East J. Dig. Dis. 5, 129–136.
Fadrosh, D. W., Ma, B., Gajer, P., Sengamalay, N., Ott, S., Brotman, R. M., et al. (2014). An improved dual-indexing approach for multiplexed 16S rRNA gene sequencing on the Illumina MiSeq platform. Microbiome 2:6. doi: 10.1186/2049-2618-2-6
Friedman, S. L., Neuschwander-Tetri, B. A., Rinella, M., and Sanyal, A. J. (2018). Mechanisms of NAFLD development and therapeutic strategies. Nat. Med. 24, 908–922. doi: 10.1038/s41591-018-0104-9
Gregor, M. F., and Hotamisligil, G. S. (2011). Inflammatory mechanisms in obesity. Annu. Rev. Immunol. 29, 415–445. doi: 10.1146/annurev-immunol-031210-101322
Jeong, H. J., Yoon, S. J., and Pyun, Y. R. (2008). Polysaccharides from edible mushroom Hinmogi (Tremella fuciformis) inhibit differentiation of 3T3-L1 adipocytes by reducing mRNA expression of PPARγ, C/EBPα, and leptin. Food Sci. Biotechnol. 17, 267–273. doi: 10.1016/j.bbrc.2011.05.086
Kawaguchi, T., Takenoshita, M., Kabashima, T., and Uyeda, K. (2001). Glucose and cAMP regulate the L-type pyruvate kinase gene by phosphorylation/dephosphorylation of the carbohydrate response element binding protein. Proc. Natl. Acad. Sci. U. S. A. 98, 13710–13715. doi: 10.1073/pnas.231370798
Komaroff, A. L. (2017). The microbiome and risk for obesity and diabetes. JAMA 317, 355–356. doi: 10.1001/jama.2016.20099
Kong, C., Gao, R., Yan, X., Huang, L., and Qin, H. (2018). Probiotics improve gut microbiota dysbiosis in obese mice fed a high-fat or high-sucrose diet. Nutrition 60, 175–184. doi: 10.1016/j.nut.2018.10.002
Lagkouvardos, I., Pukall, R., Abt, B., Foesel, B. U., and Clavel, T. (2016). The mouse intestinal bacterial collection (miBC) provides host-specific insight into cultured diversity and functional potential of the gut microbiota. Nat. Microbiol. 1, 1–16. doi: 10.1038/nmicrobiol.2016.131
Le Roy, T., Llopis, M., Lepage, P., Bruneau, A., Rabot, S., and Gérard, P. (2013). Intestinal microbiota determines development of non-alcoholic fatty liver disease in mice. Gut 62, 1787–1794. doi: 10.1136/gutjnl-2012-303816
Li, H., Liu, F., Lu, J., Shi, J., Guan, J., Yan, F., et al. (2020). Probiotic mixture of lactobacillus plantarum strains improves lipid metabolism and gut microbiota structure in high fat diet-fed mice. Front. Microbiol. 11:512. doi: 10.3389/fmicb.2020.00512
Lu, H.-J., Tzeng, T.-F., Liou, S.-S., Chang, C. J., Yang, C., Wu, M.-C., et al. (2014). Ruscogenin ameliorates experimental nonalcoholic steatohepatitis via suppressing lipogenesis and inflammatory pathway. Biomed Res. Int. 2014:652680. doi: 10.1155/2014/652680
Park, H. J., Della-Fera, M. A., Hausman, D. B., Rayalam, S., and Baile, C. A. (2009). Genistein inhibits differentiation of primary human adipocytes. J. Nutr. Biochem. 20, 140–148. doi: 10.1016/j.jnutbio.2008.01.006
Parker, B. J., Wearsch, P. A., Veloo, A. C. M., and Rodriguez-Palacios, A. (2020). The genus Alistipes: gut bacteria with emerging implications to inflammation, cancer, and mental health. Front. Immunol. 11:906. doi: 10.3389/fimmu.2020.00906
Ridaura, V. K., Faith, J. J., Rey, F. E., Cheng, J., Duncan, A. E., and Gordon, J. I. (2013). Gut microbiota from twins discordant for obesity modulate metabolism in mice. Science 341:1241214. doi: 10.1126/science.1241214
Roller, M., Rechkemmer, G., and Watzl, B. (2004). Prebiotic inulin enriched with oligofructose in combination with the probiotics Lactobacillus rhamnosus and Bifidobacterium lactis modulates intestinal immune functions in rats. J. Nutr. 134, 153–156. doi: 10.1093/jn/134.1.153
Rosen, E. D., Sarraf, P., Troy, A. E., Bradwin, G., Moore, K., and Mortensen, R. M. (1999). PPARγ is required for the differentiation of adipose tissue in vivo and in vitro. Mol. Cell 4, 611–617. doi: 10.1016/S1097-2765(00)80211-7
Rotman, Y., and Sanyal, A. J. (2017). Current and upcoming pharmacotherapy for non-alcoholic fatty liver disease. Gut 66, 180–190. doi: 10.1136/gutjnl-2016-312431
Schneeberger, M., Everard, A., Gómez-Valadés, A. G., and Cani, P. D. (2015). Akkermansia muciniphila inversely correlates with the onset of inflammation, altered adipose tissue metabolism and metabolic disorders during obesity in mice. Sci. Rep. 5:16643. doi: 10.1038/srep16643
Sonnenburg, J. L., and Bäckhed, F. (2016). Diet-microbiota interactions as moderators of human metabolism. Nature 535, 56–64. doi: 10.1038/nature18846
Spencer, M. D., Hamp, T. J., Reid, R. W., Fischer, L. M., Steven, H., and Fodor, A. A. (2012). Association between composition of the human gastrointestinal microbiome and development of fatty liver with choline deficiency. Gastroenterology 140, 976–986. doi: 10.1053/j.gastro.2010.11.049
Sun, J., and Buys, N. (2015). Effects of probiotics consumption on lowering lipids and CVD risk factors: a systematic review and meta-analysis of randomized controlled trials. Ann. Med. 47, 430–440. doi: 10.3109/07853890.2015.1071872
Thingholm, L. B., Rühlemann, M. C., Koch, M., Fuqua, B., and Huttenhower, C. (2019). Obese individuals with and without type 2 diabetes show different gut microbial functional capacity and composition. Cell Host Microbe 26, 252.e10–264.e10. doi: 10.1016/j.chom.2019.07.004
Tilg, H., Cani, P. D., and Mayer, E. A. (2016). Gut microbiome and liver diseases. Gut 65, 2035–2044. doi: 10.1136/gutjnl-2016-312729
Turnbaugh, P. J., Ley, R. E., Mahowald, M. A., Magrini, V., Mardis, E. R., and Gordon, J. I. (2006). An obesity-associated gut microbiome with increased capacity for energy harvest. Nature 444, 1027–1031. doi: 10.1038/nature05414
Wang, Z., Klipfell, E., Bennett, B. J., Koeth, R., Levison, B. S., and Hazen, S. L. (2011). Gut flora metabolism of phosphatidylcholine promotes cardiovascular disease. Nature 472, 57–65. doi: 10.1038/nature09922
Wang, J., Zhang, H., Chen, X., Chen, Y., Menghebilige, and Bao, Q. (2012). Selection of potential probiotic lactobacilli for cholesterol-lowering properties and their effect on cholesterol metabolism in rats fed a high-lipid diet. J. Dairy Sci. 95, 1645–1654. doi: 10.3168/jds.2011-4768
Won, S. M., Chen, S., Park, K. W., and Yoon, J.-H. (2020a). Isolation of lactic acid bacteria from kimchi and screening of Lactobacillus sakei ADM14 with anti-adipogenic effect and potential probiotic properties. LWT 126:109296. doi: 10.1016/j.lwt.2020.109296
Won, S. M., Chen, S., Lee, S. Y., Lee, K. E., Park, K. W., and Yoon, J.-H. (2020b). Lactobacillus sakei ADM14 induces anti-obesity effects and changes in gut microbiome in high-fat diet-induced obese mice. Nutrients 12:3703. doi: 10.3390/nu12123703
Wong, R. J., Aguilar, M., Cheung, R., Perumpail, R. B., Harrison, S. A., and Ahmed, A. (2015). Nonalcoholic steatohepatitis is the second leading etiology of liver disease among adults awaiting liver transplantation in the United States. Gastroenterology 148, 547–555. doi: 10.1053/j.gastro.2014.11.039
Wu, H., Esteve, E., Tremaroli, V., Khan, M. T., Caesar, R., and Bäckhed, F. (2017). Metformin alters the gut microbiome of individuals with treatment-naive type 2 diabetes, contributing to the therapeutic effects of the drug. Nat. Med. 23, 850–858. doi: 10.1038/nm.4345
Yang, J.-Y., Lee, Y.-S., Kim, Y., Lee, S.-H., Ryu, S., Fukuda, S., et al. (2017). Gut commensal Bacteroides acidifaciens prevents obesity and improves insulin sensitivity in mice. Mucosal Immunol. 10, 104–116. doi: 10.1038/mi.2016.42
Yin, J., Li, Y., Han, H., Chen, S., Gao, J., Liu, G., et al. (2018). Melatonin reprogramming of gut microbiota improves lipid dysmetabolism in high-fat diet-fed mice. J. Pineal Res. 65, 1–18. doi: 10.1111/jpi.12524
Yoo, J., and Kim, S. (2016). Probiotics and prebiotics: present status and future perspectives on metabolic disorders. Nutrients 8:173. doi: 10.3390/nu8030173
Younossi, Z. M. (2019). Non-alcoholic fatty liver disease: a global public health perspective. J. Hepatol. 70, 531–544. doi: 10.1016/j.jhep.2018.10.033
Zheng, J., Wittouck, S., Salvetti, E., Franz, C. M. A. P., Harris, H. M. B., Mattarelli, P., et al. (2020). A taxonomic note on the genus Lactobacillus: description of 23 novel genera, emended description of the genus Lactobacillus Beijerinck 1901, and union of Lactobacillaceae and Leuconostocaceae. Int. J. Syst. Evol. Microbiol. 70, 2782–2858. doi: 10.1099/ijsem.0.004107
Keywords: Latilactobacillus sakei, probiotics, obesity, lipid metabolism, microbiome
Citation: Won S-M, Seo MJ, Kwon MJ, Park KW and Yoon J-H (2021) Oral Administration of Latilactobacillus sakei ADM14 Improves Lipid Metabolism and Fecal Microbiota Profile Associated With Metabolic Dysfunction in a High-Fat Diet Mouse Model. Front. Microbiol. 12:746601. doi: 10.3389/fmicb.2021.746601
Edited by:
Sabina Górska, Hirszfeld Institute of Immunology and Experimental Therapy, Polish Academy of Sciences (PAN), PolandReviewed by:
Filippo Rossi, Catholic University of the Sacred Heart, ItalyAleksandr G. Bulaev, Federal Center Research Fundamentals of Biotechnology, Russian Academy of Sciences (RAS), Russia
Copyright © 2021 Won, Seo, Kwon, Park and Yoon. This is an open-access article distributed under the terms of the Creative Commons Attribution License (CC BY). The use, distribution or reproduction in other forums is permitted, provided the original author(s) and the copyright owner(s) are credited and that the original publication in this journal is cited, in accordance with accepted academic practice. No use, distribution or reproduction is permitted which does not comply with these terms.
*Correspondence: Jung-Hoon Yoon, amh5b29uNjlAc2trdS5lZHU=
†These authors have contributed equally to this work