- 1Botánica, Instituto Cavanilles de Biodiversidad y Biología Evolutiva (ICBIBE), Fac. CC. Biológicas, Universitat de València, Valencia, Spain
- 2Department of Botany, Faculty of Science, Charles University, Prague, Czechia
- 3Departamento de Farmacología, Farmacognosia y Botánica, Facultad de Farmacia, Universidad Complutense de Madrid, Madrid, Spain
- 4Departamento de Biología, Geología, Física y Química Inorgánica, Escuela Superior de Ciencias Experimentales y Tecnología (ESCET), Universidad Rey Juan Carlos, Madrid, Spain
The worldwide, ecologically relevant lichen-forming genus Parmelia currently includes 41 accepted species, of which the Parmelia sulcata group (PSULgp) and the Parmelia saxatilis group (PSAXgp) have received considerable attention over recent decades; however, phycobiont diversity is poorly known in Parmelia s. lat. Here, we studied the diversity of Trebouxia microalgae associated with 159 thalli collected from 30 locations, including nine Parmelia spp.: P. barrenoae, P. encryptata, P. ernstiae, P. mayi, P. omphalodes, P. saxatilis, P. serrana, P. submontana, and P. sulcata. The mycobionts were studied by carrying out phylogenetic analyses of the nrITS. Microalgae genetic diversity was examined by using both nrITS and LSU rDNA markers. To evaluate putative species boundaries, three DNA species delimitation analyses were performed on Trebouxia and Parmelia. All analyses clustered the mycobionts into two main groups: PSULgp and PSAXgp. Species delimitation identified 13 fungal and 15 algal species-level lineages. To identify patterns in specificity and selectivity, the diversity and abundance of the phycobionts were identified for each Parmelia species. High specificity of each Parmelia group for a given Trebouxia clade was observed; PSULgp associated only with clade I and PSAXgp with clade S. However, the degree of specificity is different within each group, since the PSAXgp mycobionts were less specific and associated with 12 Trebouxia spp., meanwhile those of PSULgp interacted only with three Trebouxia spp. Variation-partitioning analyses were conducted to detect the relative contributions of climate, geography, and symbiotic partner to phycobiont and mycobiont distribution patterns. Both analyses explained unexpectedly high portions of variability (99 and 98%) and revealed strong correlations between the fungal and algal diversity. Network analysis discriminated seven ecological clusters. Even though climatic conditions explained the largest proportion of the variation among these clusters, they seemed to show indifference relative to climatic parameters. However, the cluster formed by P. saxatilis A/P. saxatilis B/Trebouxia sp. 2/Trebouxia sp. S02/Trebouxia sp. 3A was identified to prefer cold-temperate as well as humid summer environments.
Introduction
Lichens are complex systems individualized from cyclical symbiotic associations (Khakhina et al., 1993) which represent the best example of symbiotic associations between organisms belonging to several taxonomically and phylogenetically distinct groups. Lichens are composed of a fungus, or mycobiont, and an internal population of photosynthetic organisms, or photobionts, which may be green microalgae (phycobionts) and/or cyanobacteria (cyanobionts) (Hawksworth and Honegger, 1994). Many lichen-forming fungal species are widely distributed and occupy ca. 8% of the surface of the planet (Nash, 2008). However, phycobiont distribution patterns seem to show their own ecological preferences, independently of the lichen forming fungus (Peksa and Škaloud, 2011; Řídká et al., 2014; Rolshausen et al., 2020; Moya et al., 2021). In fact, both restricted and globally distributed phycobionts have been recorded (Muggia et al., 2014; Vančurová et al., 2018; Garrido-Benavent et al., 2021).
Success in the development of the lichen thallus across micro and macro ecological scales is clearly influenced by the presence of the two main symbionts in the environment, and by the degree of selectivity and specificity that either symbiont shows for each other (Beck et al., 2002; Yahr et al., 2004, 2006). Terms such as specificity and selectivity have been defined by several authors (Muggia and Grube, 2018); however, these concepts are mainly determined by sampling bias and should be constantly revised. In general, mycobionts which show a high specificity for algal partners tend to accept only single algal lineages (Piercey-Normore and DePriest, 2001; O’Brien et al., 2013; Leavitt et al., 2015; Magain et al., 2017; Kosecka et al., 2020; Mark et al., 2020; Pino-Bodas and Stenroos, 2020; Garrido-Benavent et al., 2021), while mycobionts which are generalist can associate with many different algal lineages (Wirtz et al., 2003; Muggia et al., 2013; Sadowska-Deś et al., 2014); this has also been reported for photobionts and their preference toward fungal partners (Peksa and Škaloud, 2011). Specific or generalist associations among lichen symbionts are not random, but rather are modulated by ecological, environmental and evolutionary factors (Beck et al., 2002; Miadlikowska et al., 2006; Leavitt et al., 2015; Grube and Wedin, 2016; Chagnon et al., 2019), which have significant impacts on the structure of lichen communities and species distribution (Muggia et al., 2014; Sork and Werth, 2014; Steinová et al., 2019). Many analyses have pointed out that climatic conditions are the most important factor shaping phycobiont distribution patterns (Kroken and Taylor, 2000; Helms et al., 2003; Fernández-Mendoza et al., 2011; Peksa and Škaloud, 2011; Řídká et al., 2014); however, the influence of factors such as phylogenetic and evolutionary specialization, reproductive strategy, the presence of compatible phycobionts as well as ecological factors should not be overlooked (Yahr et al., 2006; Muggia et al., 2013; Printzen et al., 2013; Singh et al., 2017; Ertz et al., 2018; Pardo-De la Hoz et al., 2018; Molins et al., 2021).
Trebouxia Puymaly Tschermak-Woess (Trebouxiales, Trebouxiaceae) is one of the most frequent lichen symbionts, associating with over 7,000 species of lichen-forming fungi from across a wide range of fungal classes (Lücking et al., 2017). Trebouxia is among the most widespread phycobionts, and it associates with a broad range of lichen-forming fungi; estimated to be 80% in temperate regions and more than 20% worldwide (Muggia et al., 2020; Nelsen, 2021). To date, 29 Trebouxia species have been described based on a combination of morphological traits and genetic diversity (Muggia et al., 2018). More recently, four major clades have been confirmed within Trebouxia in a multi-locus phylogeny (Muggia et al., 2020). These includes clades A (arboricola/gigantea-type), C (corticola-type), I (impressa/gelatinosa-type), and S (simplex/jamesii-type). Some Trebouxia algae of clade S, specifically associating with the lichen-forming fungus Cetrariella delisei, were provisionally segregated into a new clade, D (Xu et al., 2020).
The ecologically relevant lichen-forming genus Parmelia has received considerable attention over recent decades. This genus is widely distributed in cold-temperate Europe, North America, and eastern Asia (Hale, 1987; Hawksworth et al., 2008, 2011; Crespo et al., 2010). The genus Parmelia s. str. includes 41 currently accepted species which belong to the parmelioid crown of Parmeliaceae. Within Parmelia s. str., the Parmelia sulcata group (PSULgp) includes sorediate species; 6 spp and the Parmelia saxatilis group (PSAXgp) mainly isidiate species; 12 spp (Divakar et al., 2016; Molina et al., 2017). Both isidia and soredia are dispersal packages of both fungus and algae (vegetative reproduction). Several molecular studies on PSAXgp and PSULgp have been published and have revealed the presence of cryptic species (Crespo et al., 1997, 1999, 2002). Over the last two decades’ molecular investigations focusing on PSAXgp and PSULgp from the Iberian Peninsula have been carried out. These studies allowed us to described Parmelia serrana (Molina et al., 2004) and Parmelia encryptata (Molina et al., 2011a). Also, Divakar et al. (2005) detected additional Parmelia barrenoae species in the PSULgp from the Iberian Peninsula, North America and Africa. More recently, Parmelia rojoi was segregated from PSAXgp from Spain (Crespo et al., 2020). Apart from these, Parmelia imbricaria, Parmelia mayi, and Parmelia sulymae were segregated from PSAXgp in North America (Molina et al., 2011b,2017). The species delimitation in Parmelia discordans/Parmelia omphalodes, and Parmelia hygrophila/Parmelia submontana complexes remains unclear (Molina et al., 2017; Ossowska et al., 2019).
Contrasting patterns of worldwide distributions in some Parmelia spp., and geographically restricted distributions in other species of this genus, have been documented (Molina et al., 2011a; Crespo et al., 2020). However, photobiont diversity associated with these lichen-forming fungal species remains almost unknown. Leavitt et al. (2015) analyzed the algal nrITS region from 11 mycobiont genera of Parmeliaceae and Lecanoreceae (but they did not include Parmelia). They explored the interactions patterns in these species based on habitat preferences and mycobiont phylogeny, and concluded that: “fungal specificity and selectivity for algal partners played a major role in determining lichen partnerships.” Recently, Ossowska et al. (2019) evaluated the genetic variability of phycobionts within the Parmelia omphalodes group, which include P. omphalodes, P. discordans, and P. pinnatifida. All taxa investigated in this study showed moderate selectivity in their phycobiont choice and associated only with Trebouxia from clade S. Dumitru (2019) performed a preliminary study of phycobiont diversity in Parmelia spp. distributed in the Iberian Peninsula. The results of this study were the starting point of the present analyses. Furthermore, we do not know the distribution pattern of phycobiont species associated with the remaining Parmelia lichen taxa, and this may play a crucial role in speciation of this lichen forming fungal species.
Thus, as ecological requirements of phycobionts could be directly related with the host distribution, in the present study we focused on Trebouxia biodiversity in the lichen-forming genus Parmelia to better understand their global spatial distribution and ecological preferences.
Materials and Methods
Lichens Sampled
In this study 159 thalli were sampled from 30 locations (Supplementary Table 1) including nine formally described Parmelia spp., viz. Parmelia barrenoae, Parmelia encryptata, Parmelia ernstiae, Parmelia mayi, Parmelia omphalodes, Parmelia saxatilis, Parmelia serrana, Parmelia submontana, and Parmelia sulcata. Both fresh (n = 133) and herbarium samples (n = 26) were included in the analyses. Fresh specimens were stored at −20°C after sampling.
DNA Extraction, PCR Amplification and Sanger Sequencing
Lichen thalli were processed as described in Moya et al. (2021), in brief: specimens were examined under a stereo microscope to remove surface contamination (mainly tree bark). Selected fragments from different parts of each thallus were randomly excised and pooled together. These fragments were superficially sterilized following Arnold et al. (2009).
Total genomic DNA was isolated and purified using the DNeasy Plant Mini kit (Qiagen, Hilden, 121 Germany) following the manufacturer’s instructions. The mycobionts and the phycobionts were identified by Sanger sequencing. The fungal nuclear ribosomal internal transcribed spacer (nrITS) was amplified using the primer pair ITS1F (Gardes and Bruns, 1993) and ITS4 (White et al., 1990). Two algal loci were amplified to confirm the identity of the main phycobiont in the 159 lichen thalli; a region of the chloroplast LSU rDNA gene, using the algal specific primers 23SU1 and 23SU2 (del Campo et al., 2010) and the nrITS using the primer pair nr-SSU-1780 (Piercey-Normore and DePriest, 2001) and ITS4 (White et al., 1990).
All PCR reactions were performed following Moya et al. (2018). PCR products were visualized on 2% agarose gels and purified using the Gel Band Purification Kit (GE Healthcare Life Science, Buckinghamshire, United Kingdom). Amplified PCR products were sequenced with an ABI 3730XL using the BigDye Terminator 3.1 Cycle Sequencing Kit (Applied Biosystems, Foster City, CA, United States). Sanger sequences were visualized and manually evaluated with Chromas v 2.6.6.0.1 Taxonomic assignment of Sanger sequenced strains was checked by conducting phylogenetic analyses.
Mycobiont Phylogenetic Analyses
Fungal sequence identity was evaluated using the mega-BLAST search function in GenBank (Sayers et al., 2011). Newly obtained data set (n = 159) were aligned with an nrITS dataset published in our previous DNA barcoding study (Divakar et al., 2016). A multiple sequence alignment was performed using the MAFFT v 7.0 program (Katoh and Toh, 2008) implementing the G-INS-I alignment algorithm, “1PAM/K = 2” scoring matrix, with an offset value of 0.0, and the remaining parameters set to default values. Phylogenetic analysis was carried out using the maximum likelihood (ML) approach. The ML analysis was performed using the RAxML v 8.2.6 program (Stamatakis, 2014) as implemented on the CIPRES Web Portal with the GTRGAMMA substitution model. Nodal support was evaluated using the “rapid bootstrapping” option with 1,000 replicates (Stamatakis et al., 2008). Only clades that received bootstrap support of greater than, or equal to, 70% (Hillis and Bull, 1993) were considered as well supported. The phylogenetic tree was drawn in FigTree v 1.4.1 (Rambaut, 2012).
Phycobiont Phylogenetic Analyses
Trebouxia phycobiont identity was further checked by phylogenetic assignment. For the nrITS, a multiple alignment was constructed including the Trebouxia data set obtained by Sanger sequencing (n = 159) and 10 selected sequences from clades S and I (Muggia et al., 2020). Asterochloris mediterranea (KP257398) was included as an outgroup. A multiple alignment was built using MAFFT v 7.0 (Katoh et al., 2002; Katoh and Toh, 2008) using default parameters. The substitution model TVM + G was the most accurate for the nrITS region according to the Akaike information criterion (AIC) using JModelTest v 2.1.4 (Darriba et al., 2012). The phylogenetic trees were inferred by Bayesian inference (BI) and maximum likelihood (ML) approaches. ML analysis was implemented in RAxML v 8.1.11 (Stamatakis, 2006) using the GTRGAMMA substitution model. Bootstrap support (BS) was calculated based on 1,000 pseudoreplicates (Stamatakis et al., 2008). BI was carried out in MrBAYES v 3.2 (Ronquist et al., 2012). Settings included two parallel runs with six chains over 20 million generations, starting with a random tree and sampling after every 200th step. We discarded the first 25% of data as burn-in, and the corresponding posterior probabilities (PPs) were calculated from the remaining trees. Estimated sampled sized (ESS) values above 200, and potential scale reduction factor values approaching 1,000, were considered indicators of chain convergence. All analyses were performed with the CIPRES Science Gateway v 3.3 (Miller et al., 2011). Phylogenetic trees were visualized in FigTree v 1.4.1 (Rambaut, 2012).
In the case of the chloroplast genome marker, a multiple alignment was built including the Trebouxia obtained (n = 129) and a selection of Trebouxia type species belonging to clades S and I available from the Culture Collection of Algae at Göttingen University (SAG) and from the Culture Collection of Algae at the University of Texas (UTEX). Asterochloris mediterranea (KP257332) was included as an outgroup. The alignment and phylogenetic analysis were carried out as previously described for the nrITS. The best-fit substitution model for this region was GTR + I + G.
Delimitation of Mycobiont and Phycobiont Species
To estimate putative species boundaries in the concatenated Trebouxia (nrITS + LSU rDNA phycobiont) and Parmelia (nrITS mycobiont) data sets, we performed three species delimitation analyses (GMYC, bPTP, ABGD). All analyses were performed following Vančurová et al. (2018). Summarizing, the Bayesian analyses with BEAST v 1.8.2 were performed (Drummond et al., 2012) to obtain ultrametric trees under the assumption of uncorrelated log normal relaxed molecular clocks. For each of the alignment partitions, the most appropriate substitution model was estimated using the Bayesian information criterion (BIC) as implemented in JModelTest2 (Guindon and Gascuel, 2003; Darriba et al., 2012). The models were selected as follows: GTR + G + I for both algal and fungal ITS1 and ITS2 partitions, HKY for both algal and fungal 5.8 rDNA partitions, and GTR + G + I for the LSU rDNA partition. The analyses were performed under the constant population size coalescent, as the tree prior and Ucld mean prior were set to exponential distribution with mean 10 and initial value 1. Five MCMC analyses were run for 30 million generations, sampling every 10,000 generations. The outputs were diagnosed for convergence using Tracer v. 1.7 (Rambaut et al., 2018), and the five tree files were merged using the burn−in set to 3 million generations (all ESS values of the merged data set were above 900). A consensus tree was generated using TreeAnnotator v 1.8.2.2 The GMYC analysis was performed on the ultrametric consensus tree under the single−threshold model, using the SPLITS package (Monaghan et al., 2009) in R v 4.0.5 (R Core Team, 2013). The bPTP analysis was also performed on the ultrametric consensus tree, using the bPTP web Server3. The analysis was run for 200,000 generations, using 0.3 burn−in and 100 thinning. Both ML and Bayesian solutions were examined. Finally, the ABGD analysis was performed on the concatenated algal and ITS rDNA fungal alignments, using the ABGD web server4. Genetic distances were calculated using the K80 model, and the model parameters were set to Pmin 0.001, Pmax 0.01, Steps 10 and Nb bins 20. Separate analyses were run under varying relative gap width values (X set to 0.1, 0.3, 0.5, 0.8, 1.0) to assess the consistency of the inferred groups. The final OTU delimitation was selected as a consensus between all three delimitation approaches described above. To assign names to each recovered lineage of both Trebouxia and Parmelia, we used either the names of previously described species or the clade names of yet undescribed lineages, following the labeling system of previous studies.
Mycobiont and Phycobiont Interaction Patterns
To identify patterns in specificity and selectivity, the diversity and abundance of phycobionts were identified for each Parmelia species. A mycobiont-phycobiont interaction network was implemented, where Trebouxia and Parmelia were grouped at species level. Bipartite networks were constructed using the bipartite package in R (R Core Team, 2013). The topological properties of the network were determined by modularity analyses using Gephi v 0.9.2 (Bastian et al., 2009). The default algorithms were used and the analysis was performed under the following settings: undirected graph type, force atlas with 0.1 inertia, repulsion 4,000, strength, attraction strength 5, and maximum displacement 1. We applied the auto stabilize function with strength 100, sensibility 0.2, and gravity 50. To improve readability and esthetics, a no-overlap algorithm to spread nodes apart was used. The weighted average degree was calculated, and this criterion was applied to adjust the nodes by sizes. The modularity analysis was performed using the Louvain method and the modules were visualized by colors.
Variation Partitioning
Two variation partitioning analyses were performed following Vančurová et al. (2018). Summarizing, first the relative effects of geography, climate, substrate chemistry and the symbiotic partner on the variance in photobiont as well as mycobiont diversity were evaluated. Second, we tested whether the modules identified by the modularity analyses (see above) can be differentiated by geography, climate and substrate chemistry. From the entire data set of 159 specimens, 14 were excluded due to insufficient substrate/habitat data, resulting in a data set of 145 samples. The relative effects of climate, substrate/habitat, geographical distance and the symbiotic partner on the variance in photobiont as well as mycobiont diversity were analyzed by variation partitioning in redundancy analysis, using the varpart function in the vegan package (Oksanen et al., 2017). The phylogenetic distances of phycobionts, or mycobionts, were used as a response variable, coded as the first 10 PCoA axes. Climatic data were obtained from the CHELSA Bioclim database (Karger et al., 2017) at a resolution of 2.5 arc minutes. At each sampling site, climatic data were obtained by applying a 5 km buffer to limit the effects of spatial bias. Geographical distance values (latitude and longitude) were transformed to the principal coordinates of neighbor matrices (PCNM) vectors representing the geographical distances at various spatial scales (Borcard et al., 2004). PCNM vectors were calculated based on the pairwise geographical distances obtained by the distGPS function in the BoSSA package (Lefeuvre, 2018). The first 100 PCNM were used for the analysis. All response variables (19 environmental variables, 5 substrates, 8 PCNM scores) were condensed into principal component variables (PCs). The Broken−stick distribution (Jackson, 1993) was used to select which principal components to include in variation partitioning analysis, using the bstick function in the vegan package (Oksanen et al., 2017). Accordingly, the first three PCA axes were selected in all response variables, with the exception of geography where only two axes were retained. To detect and visualize the differential ordination (tendency or strategies) of samples in the hyperspace, we performed Principal component analyses (PCA) according to climatic factors (BIO1–BIO19) and the grouped results depending on the distribution patterns of lichen species and the genus of the mycobiont host. The differences were visualized by box plots. The significance of a difference between groups was tested by Wilcox tests using the ggsignif package (Ahlmann-Eltze and Patil, 2021). All analyses were performed in R v 4.0.5 (R Core Team, 2013), using RStudio v 1.2.133583.
Results
Phylogenetic Analyses and Delimitation of Species
Mycobiont
The tree topology obtained (Figure 1) was fully congruent with previously described Parmelia phylogenies (Molina et al., 2017). The phylogeny showed three major clades: P. fertilis, PSULgp and PSAXgp. PSAXgp included seven clades, viz. P. ernstiae, P. imbricaria, P. mayi, P. omphalodes, P. saxatilis, P. serrana, and P. submontana. PSULgp included four clades: P. sulcata s. str., P. barrenoae, P. encryptata, and P. fraudans.
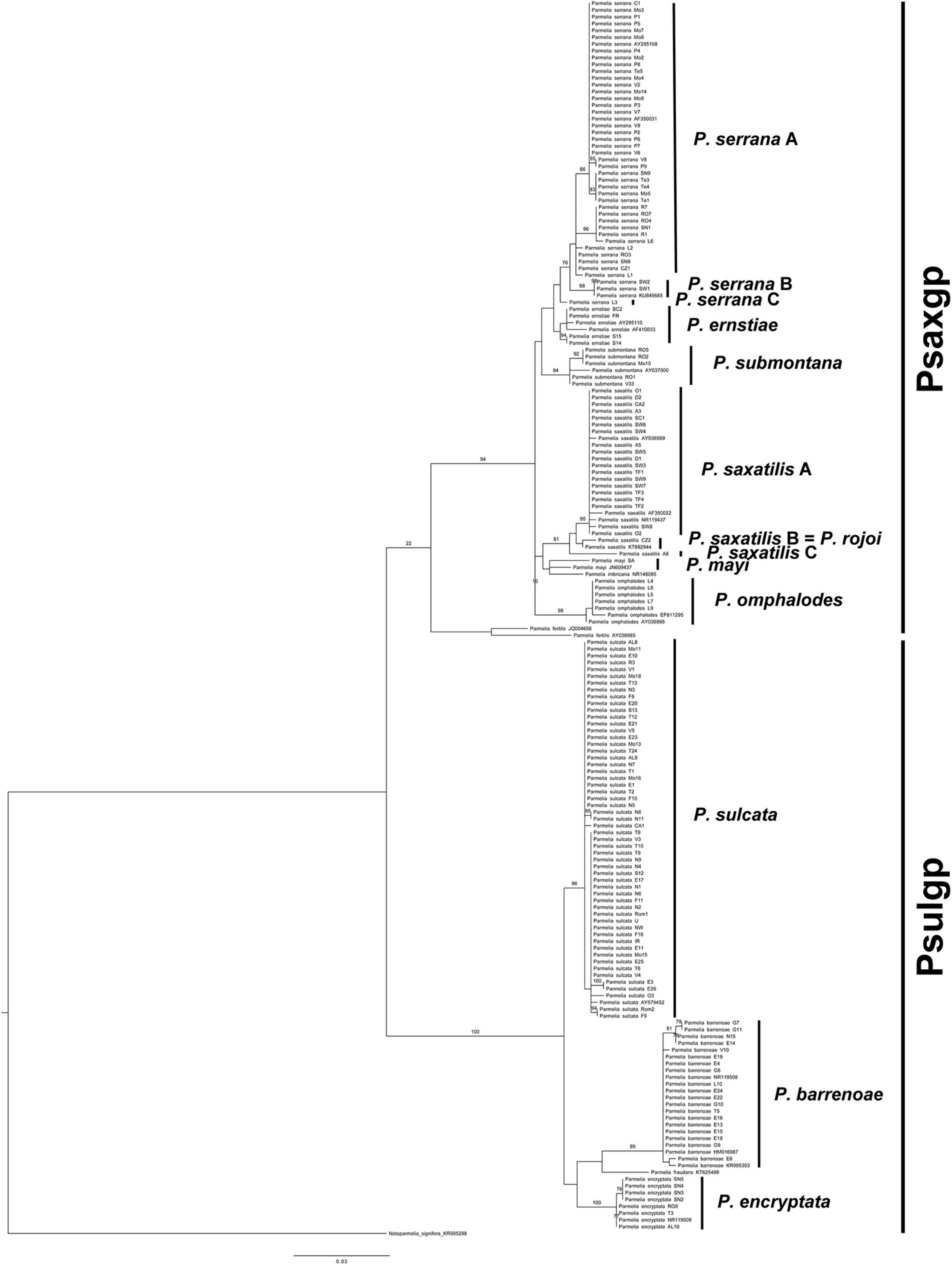
Figure 1. Maximum likelihood (ML) internal transcribed spacer (nrITS) tree of the Parmelia specimens. (ML) bootstrap values ≥ 70% from the RAxML analysis are indicated above branches.
To evaluate putative species boundaries, three species delimitation analyses (GMYC, bPTP, and ABGD) were performed in the Parmelia data set (n = 159). These analyses delimited 13 species clusters (Supplementary Figure 1), viz. P. sulcata, P. encryptata, P. barrenoae, P. serrana A, P. serrana B, P. serrana C, P. ernstiae, P. submontana, P. omphalodes, P. saxatilis A, P. saxatilis B = P. rojoi, P. saxatilis C, and P. mayi. Ten of the lineages could be placed in formally described species, whereas P. serrana B (samples SW1, SW2 from Sweden and KU845685 from Poland), P. serrana C (specimen L3 from Ávila, Spain), and P. saxatilis C (specimen A6 from Lleida, Spain) are undescribed lineages in Parmelia.
Phycobiont
A total of 15 Trebouxia microalgae species were detected in the 159 specimens analyzed (Figure 2). As mentioned in the introduction, Trebouxia spp. tended to form five well-supported monophyletic clades: A, C, I, S, and D. According to this accepted Trebouxia clade code (Leavitt et al., 2015; Moya et al., 2017, 2021; Muggia et al., 2020; Xu et al., 2020), all sequences generated in this study belong exclusively to clades S and I. LSU rDNA phylogeny corroborated the Trebouxia assignment at clade level (S and I), but showed less resolution at a species level (Supplementary Figure 2).
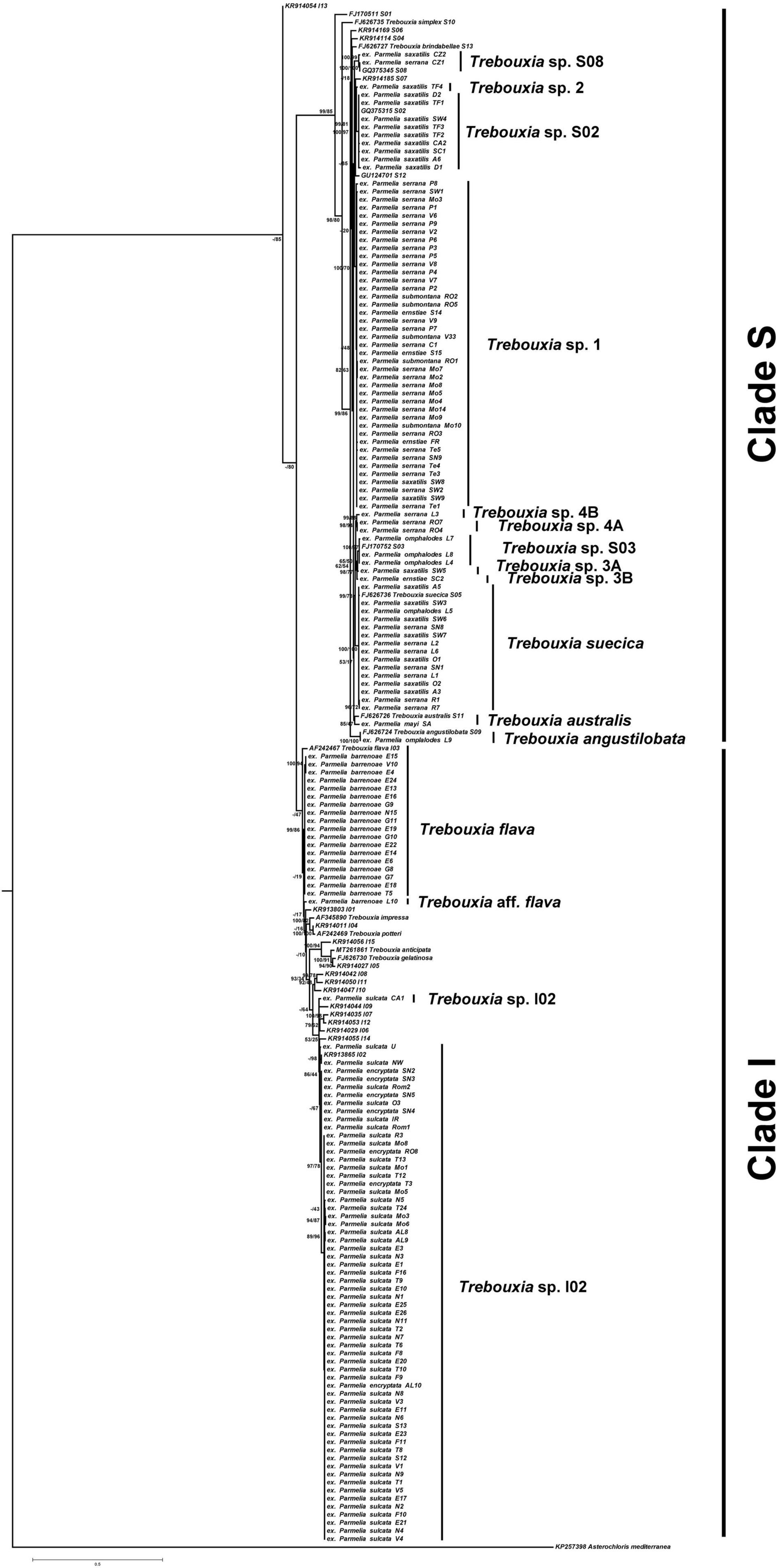
Figure 2. Trebouxia phylogenetic analysis. Rooted nrITS gene tree representing 192 Trebouxia sequences, including 10 well–accepted Trebouxia species from SAG and UTEX retrieved from the GenBank. Newly generated sequences are marked as ex Parmelia spp._locality-code_number. Fifteen Trebouxia species detected were indicated. Values at nodes indicate statistical support estimated by two methods: bootstrap support (BS, RAxML analysis) and posterior probabilities (PP, MrBayes analysis). Scale bar shows the estimated number of substitutions per site.
To estimate putative species boundaries GMYC, bPTP, and ABGD species delimitation analyses were performed on the Trebouxia concatenated nrITS and LSU rDNA data set (n = 159). These three analyses delimited 15 species clusters (Supplementary Figure 3), viz. Trebouxia sp. I02, T. flava, Trebouxia aff. flava, Trebouxia sp. 1, Trebouxia sp. S08, Trebouxia sp. S02, Trebouxia sp. 2, T. suecia, Trebouxia sp. S03, Trebouxia sp. 3A and 3B, Trebouxia sp. 4A and B, T. australis and T. angustilobata. Eight of the lineages could be placed in formally Trebouxia described species, whereas Trebouxia aff. flava (ex P. barrenoae from Ávila, Spain), Trebouxia sp. 1 (ex P. saxatilis A, P. serrana A and B, P. submontana, and P. ernstiae), Trebouxia sp. 2 (ex P. saxatilis A from Chile), Trebouxia sp. 3A (ex P. saxatilis A from Sweden) and B (ex P. ernstiae from Scotland), Trebouxia sp. 4A (ex P. serrana A from Ronda, Spain) and B (ex P. serrana C from Ávila, Spain) were newly detected.
The Associations Among Phycobiont, Mycobiont, and Environmental Conditions
Patterns in specificity and selectivity were identified for each Parmelia species (Figure 3). High specificity of each Parmelia group for a given Trebouxia clade level was observed; PSULgp (P. sulcata, P. barrenoae, P. encryptata) associated only with clade I, and PSAXgp (P. serrana A, P. serrana B, P. serrana C, P. ernstiae, P. submontana, P. omphalodes, P. saxatilis A, P. saxatilis B = P. rojoi, P. saxatilis C, and P. mayi) with clade S. However, the degree of specificity is different within each group, since the PSAXgp mycobionts were less specific and associate with 12 Trebouxia spp. (Trebouxia sp. 1, Trebouxia sp. S08, Trebouxia sp. S02, Trebouxia sp. 2, T. suecia, Trebouxia sp. S03, Trebouxia sp. 3A and 3B, Trebouxia sp. 4A and 4B, T. australis and T. angustilobata), meanwhile those of PSULgp interacted with only three Trebouxia spp. (Trebouxia sp. I02, T. flava and Trebouxia aff. flava). Twelve Trebouxia lineages showed specificity toward a single Parmelia spp. (i.e., T. australis-P. mayi or Trebouxia sp. 4B-P. serrana C). Others were not specific toward a single mycobiont, but associated mainly with one fungal species-level lineage. For example, Trebouxia sp. 1 accepts five fungal species-level lineages, but prefers P. serrana.
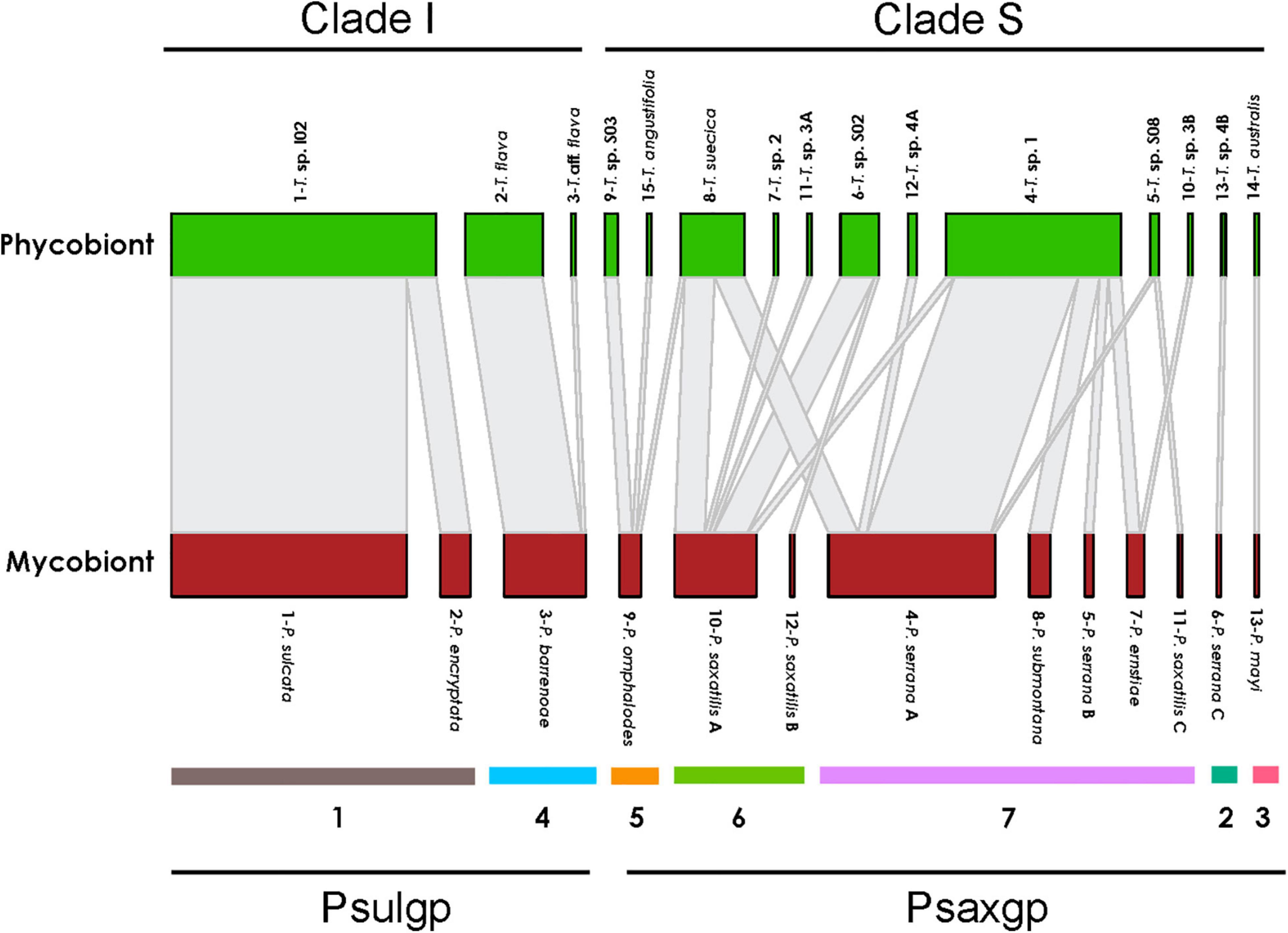
Figure 3. Interaction network structure between lichen mycobiont species-level lineages in the genus Parmelia and phycobiont species-level lineages. The width of the links is proportional to the number of specimens forming the association. Seven modules determined by modularity analysis were indicated. PSAXgp and PSULgp mycobionts and Trebouxia Clade I and Clade S are highlighted.
Variation-partitioning analyses were performed to detect the relative contributions of climate, geography and symbiotic partner to phycobiont and mycobiont distribution patterns (Figure 4). Both analyses explained unexpectedly high portions of the variability.
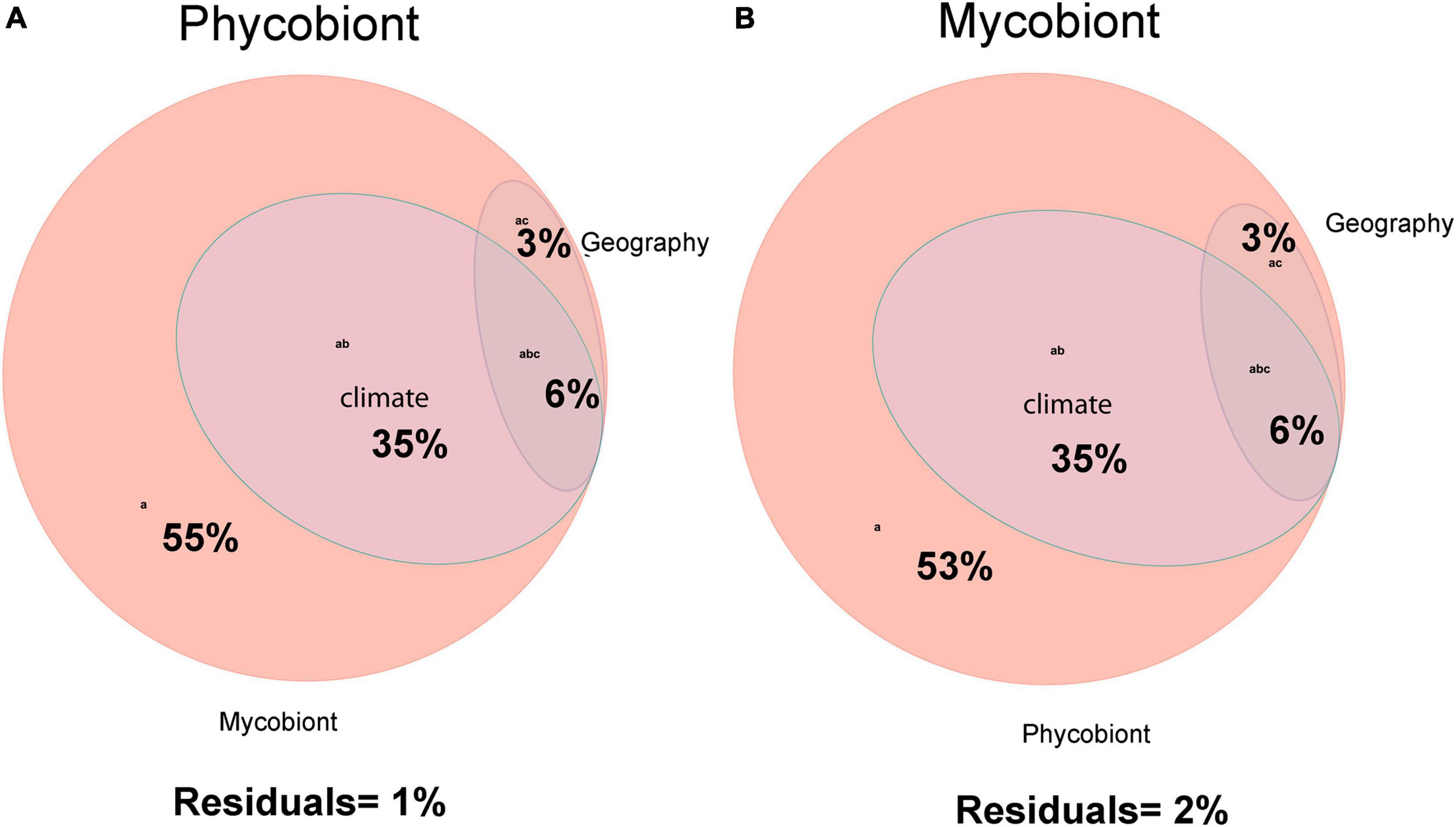
Figure 4. (A) Venn’s diagram showing the variation in distribution of phycobionts associated with the lichen-forming fungal genus Parmelia explained by effects of climate, geography and the mycobiont. (B) Venn’s diagram showing the variation in distribution of Parmelia mycobionts associated with the lichen-forming fungal genus Parmelia explained by effects of climate, geography and the phycobiont.
Among the phycobionts, climatic conditions, geography and the mycobiont explained 99% of the variation (Figure 4A). The largest proportion of the variation was explained by the symbiotic partner (55% independent effect and 99% in combination with other variables). Climatic conditions explained 42% of the variability, but explained nothing independently. The variables associated with geography explained 9% of the variability shared with other variables.
In the phylogeny of the mycobionts, climatic conditions, geography and the phycobiont explained 98% of the variation (Figure 4B). The greatest proportion of the variation (53% independent effect and 98% in combination with other variables) was explained by the symbiotic partner. The same proportions as in the phycobionts were detected for the climatic conditions and geography.
Correlation Between Bioclimatic Variables and Symbiont Association Pattern
Network analysis has been applied to investigate the topological properties of the mycobiont-phycobiont interactions. Our network analysis discriminated seven ecological clusters (Supplementary Figure 4A and listed below), four of them including 96% of all taxa. These four main ecological clusters included 62 (Cluster 1), 11 (Cluster 4), 17 (Cluster 6), and 31 (Cluster 7) taxa. The remaining three clusters comprised only a single taxon (Clusters 2 and 3) and three taxa (Cluster 5), respectively.
1—P. sulcata/P. encryptata/Trebouxia sp. I02
2—P. serrana C/Trebouxia sp. 4B
3—P. mayi/T. australis
4—P. barrenoae/T. flava/Trebouxia aff. flava
5—P. omphalodes/T. angustilobata/Trebouxia sp. S03
6—P. saxatilis A/P. saxatilis B/Trebouxia sp. 2/Trebouxia sp. S02/Trebouxia sp. 3A
7—P. serrana A/P. saxatilis C/P. submontana/P. serrana B/P. ernstiae/Trebouxia sp. S08/Trebouxia sp. 4A/Trebouxia sp. 1/Trebouxia sp. 3B
To identify the factors that shape these seven identified clusters, we performed a second variation-partitioning analysis (Supplementary Figure 4B). We analyzed the relative contributions of climate, geography and substrate to the module associations. The response variables explained 58% of the total variation. The largest proportion of the variation was elucidated by the climatic conditions (27% independent effect and 39% in combination with other variables). The second most important variable was the substrate, which explained 13% of the variability independently, despite 7% being shared with other variables. The third variable, geography, explained only 6% of the variation (independent effect, 7% in combination with other variables).
PCA analyses were performed to identify which clusters were represented as a function of climate (Supplementary Figure 5). PCA ordination of all analyzed species resulted in a 69.5% cumulative variance, explained on the first 2 axes (first-40.8%, second-28.7%). Even though climatic conditions explained the largest proportion of the variation among these clusters, they seemed to show indifference relative to climatic parameters (Supplementary Figure 5B). However, slight differences among the groups were observed when only the major clusters were analyzed by PCA, with Cluster 6 (P. saxatilis A/P. saxatilis B/Trebouxia sp. 2/Trebouxia sp. S02/Trebouxia sp. 3A) being climatically the most distinct (Figure 5A).
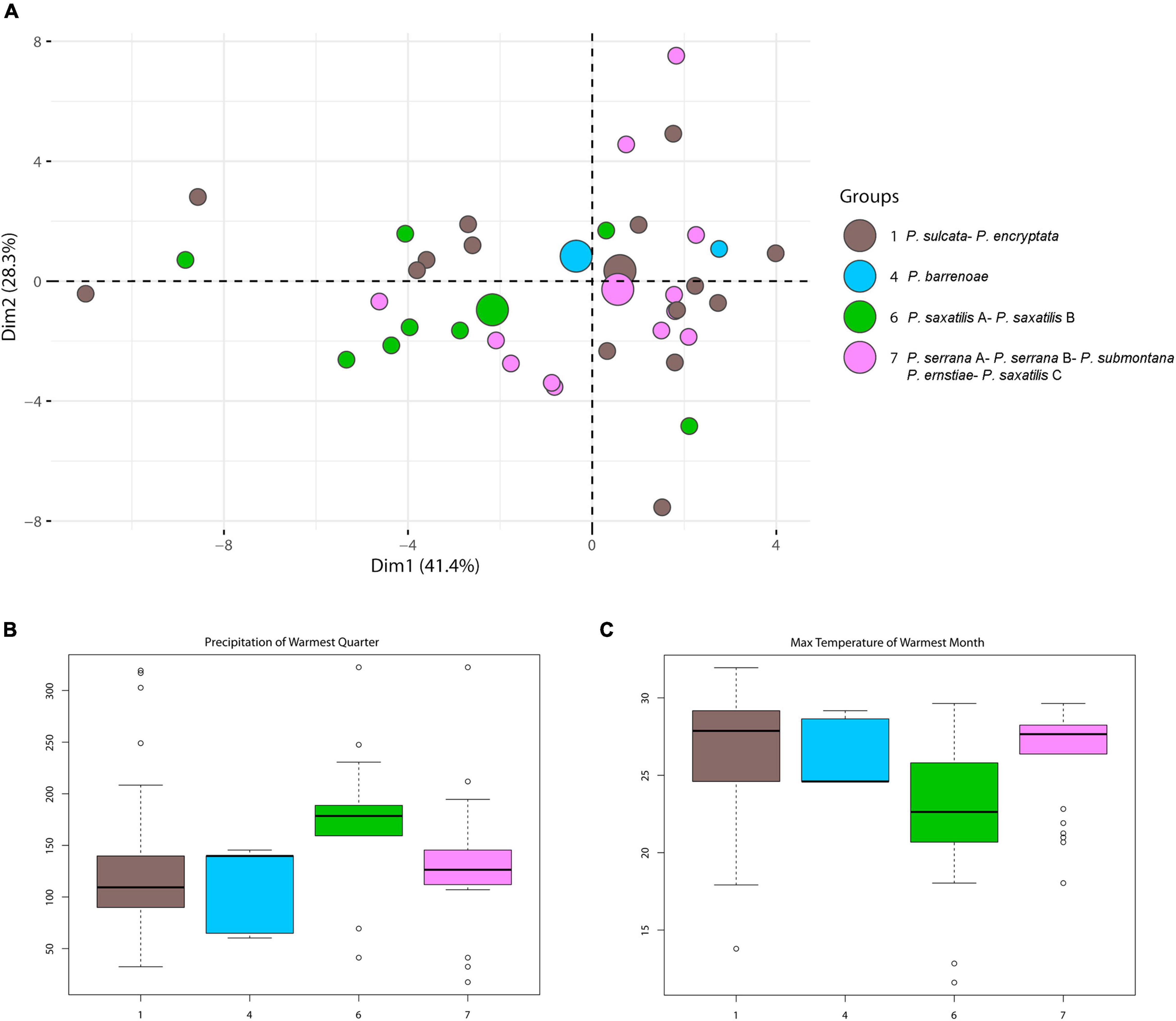
Figure 5. (A) PCA result for modules 1, 4, 6, and 7 distributions depending on climatic factors. Large circles represent group centroids. (B,C) Box-plot diagram representing differences in climate preferences in selected modules 1, 4, 6, and 7. Climatic data were obtained from the Global Climate Data – WorldClim. (B) BIO5 = Max temperature of warmest month (°C) and (C) BIO18 = precipitation of warmest quarter (°C).
Indeed, this cluster clearly differed by preferring cold-temperate, humid-in-summer environments (BIO5, 18, Wilcox tests p < 0.001 for all comparisons; Figures 5B,C), according to Rivas-Martínez et al. (2017) under non-Mediterranean bioclimates. The climatic differentiation of clusters 1, 4, and 7 was not significant (Wilcox tests, p < 0.05), but cluster 4 showed the least variance.
Discussion
Although lichens are among the best examples of symbiotic associations, so far research has mainly been directed toward the fungal partner (mycobiont) and our knowledge about phycobiont diversity is still scarce. In fact, even in an ecologically relevant lichen genus, such as Parmelia, microalgae diversity is poorly known (Dumitru, 2019; Ossowska et al., 2019). The present study analyzed the diversity of Trebouxia associated with nine Parmelia spp., to arrive at a starting point regarding information about the myco-phycobiont interaction patterns, and provides the first insights into the ecological requirements of phycobionts associated with this genus, mainly in the Iberian Peninsula.
In general, lichen forming fungi associate with several phycobiont species belonging to a single microalgae genus. In Parmelia, the main phycobiont genus is Trebouxia. In this study, the genetic diversity of Trebouxia spp. was checked by using a nuclear region (nrITS barcode) and a chloroplast marker. In the case of the nrITS region, 15 Trebouxia microalgae species were detected in 159 specimens examined. According to the clade code mentioned for Trebouxia, all the sequences generated in this study belong exclusively to clade S and clade I. Chloroplast marker phylogeny corroborated the Trebouxia assignment at a clade level (S and I), but showed less resolution at a species level. These results agree with previous results (Ossowska et al., 2019) which pointed out a high diversity of Trebouxia (Trebouxia sp. S02, Trebouxia sp. S04, Trebouxia sp. S05, Trebouxia sp. Sun1, Trebouxia sp. Sun2) associated with three Parmelia spp. Some lineages are reported for the first time (Trebouxia aff. flava, Trebouxia sp. 1, Trebouxia sp. 2, Trebouxia sp. 3A and B, Trebouxia sp. 4A and B), while other already-known ones (Trebouxia sp. I02, T. flava, Trebouxia sp. S08, T. suecica, Trebouxia sp. S03, T. australis, and T. angustilobata) had never been found associated with Parmelia (only Trebouxia sp. S02 were previously described in Parmelia). The most frequent lineages of Trebouxia in the Parmelia spp. analyzed were Trebouxia sp. I02 and the new Trebouxia sp. 1. Previous studies had already found Trebouxia sp. I02 phycobionts in other genera, such as Oropogon, Melanelixia, Melanohalea, Rhizoplaca, and Montanelia (Leavitt et al., 2015). Other common lineages found are T. flava and T. suecica. For them, the range of associated host species has widened. Even though several authors focus their studies on the phycobiont distribution ranges (Blaha et al., 2006; Leavitt et al., 2016), our knowledge in this area is still incomplete. Our study helped us to expand the information concerning the distribution of different Trebouxia species. For example, Trebouxia sp. 3B or 4B were only found in Scotland and Spain, respectively. In contrast, Trebouxia sp. I02 were distributed among Spain, Romania, Norway, Ireland, the United States, and Canada. The richness in species of Parmelia added to the contrasting patterns of worldwide distributions in some Parmelia spp., and geographically restricted distributions in other species of this genus, could favor the high Trebouxia diversity found in this genus.
Climatic conditions are considered one of the most decisive factors influencing range species distribution (Currie et al., 2004). In fact, several authors have proven climatic variables to be good predictors of phycobiont diversity (Nelsen and Gargas, 2009; Fernández-Mendoza et al., 2011; Leavitt et al., 2016; Magain et al., 2017). In the present study, the distribution of both phycobionts and mycobionts of Parmelia lichens was almost completely explained by the symbiotic partner, climatic conditions and habitat (geography), which is really unexceptional. Climate explains part of the genetic variation of the phycobionts associated with Parmelia; however, most of the variation explained by climate is shared with other factors (Figure 4 and Supplementary Figure 4). These results suggest that the biodiversity patterns of the photobionts associated with the lichen forming genus Parmelia are multifactorial, with a mixture of factors acting together, as found in other genera (Vančurová et al., 2018; Jüriado et al., 2019). The identity of the partnership was clearly the main factor determining photobiont genetic variation, which agrees with the results found in many other lichens (Leavitt et al., 2015; Vančurová et al., 2018; Jüriado et al., 2019). This is expected in lichens with dominant asexual reproduction, such as the Parmelia spp. included in this study (Fernández-Mendoza et al., 2011; Steinová et al., 2019); the Parmelia sulcata group includes sorediate species, and the Parmelia saxatilis group mainly isidiate species, and both isidia and soredia are dispersal packages of both fungi and algae.
In the present work, mycobionts were studied by carrying out phylogenetic analyses of the nrITS region. This analysis was key to corroborate the accurate species identification and to segregate cryptic species. We estimated the delimitation of 13 species clusters. Ten of the lineages were placed in formally described species, whereas P. serrana B (which included samples from Sweden and Poland), P. serrana C and P. saxatilis C (from Spain) are undescribed lineages in Parmelia, and they are probably new cryptic species. The recent description of P. rojoi (= P. saxatilis B) in the Iberian Peninsula, and the fact that all species of PSAXgp distributed in Europe also occur in Spain, allowed Crespo et al. (2020) to hypothesized this area as a refugium during Pleistocene glaciations. The discovery of the new lineages (P. serrana C and P. saxatilis C) corroborates this hypothesis. Parmelia serrana and Parmelia saxatilis in PSAXgp are the most widespread taxon, and the most successful in colonizing diverse habitats in the Iberian Peninsula. Crespo et al. (2020) hypothesized that “the appearance of a Mediterranean climatic rhythm played a crucial role in the diversification of P. serrana in the Iberian Peninsula,” which could also have affected P. saxatilis. The presence of cryptic species inside P. serrana and P. saxatilis should be revised.
Finally, patterns in specificity and selectivity at a species level were analyzed for the 13 species of Parmelia identified. The mycobiont genus Parmelia varied its specificity toward the phycobiont depending on the level (group or species). Surprisingly, high specificity of each Parmelia group for a given Trebouxia clade level was observed: PSULgp associated only with clade I and PSAXgp with clade S. Differences in the presence of vegetative structures between both groups were previously highlighted (PSAXgp-isidiate vs. PSULgp-sorediate), thus supporting the idea proposed by Steinová et al. (2019) that ‘in Cladonia the specificity of the mycobiont toward the photobiont could be attributed to differences in the size of the vegetative propagules and the amount of them’. However, the degree of specificity is different within each group, since the PSAXgp mycobionts were less specific and associate with 12 Trebouxia spp., meanwhile those of PSULgp interacted only with three Trebouxia spp. In detail, as described by Smith et al. (2009), isidia contain both microalgae and fungal hyphae, and this cortex provide a tough outer surface. On other hand, soredium are produced from exposed medulla tissue and they are not enclosed in this protective outer cortical layer. These differences between isidia and soredia may influence phycobiont choice as, for example, sun irradiance, humidity and light exposure will be different in both structures. This very marked specificity of isidiates for clade S of Trebouxia and sorediate for clade I was probably already established when the divergence between isidiate and sorediate Parmelia occurred. Moreover, the new lineages, which have diversified, maintained the specificity for their respective clade.
Eight Parmelia species included in this study associate with only one Trebouxia lineage and could be considered specialist species. However, in some of these species, such as P. mayi and P. serrana C, a low number of specimens were sampled, thus making it difficult to establish if they are specialists or not. Five of the species studied cooperate with more than one Trebouxia (Figure 4); ranging from 2 to 5. The most generalist species are P. saxatilis A and P. serrana A, which associate with 5 and 4 Trebouxia spp., respectively. Several studies have reported the capability of mycobionts to associate with several phycobiont species instead of being specific toward one single microalgae lineage (Otálora et al., 2010). This is commonly considered as an adaptive strategy of the mycobiont to colonize a wider range of environmental conditions; selecting the best adapted phycobiont (Yahr et al., 2004, 2006; Casano et al., 2011; Muggia et al., 2013). In the case of lichen with asexual reproduction, association with more than one Trebouxia spp. could be related to the fact that the joint dispersal of fungal and algal does not always imply the maintenance of the same species of microalgae, but that once established the mycobiont can associate with other species of phycobionts (Dal Grande et al., 2018).
In general, it has been shown that species with worldwide distributions associate with a wide phycobiont range (Blaha et al., 2006; Muggia et al., 2014; Moya et al., 2017), while species growing in restricted areas are more specific (Otálora et al., 2010, 2013; Leavitt et al., 2016; Magain et al., 2017). However, there are exceptions to this rule, such as gypsum lichen species which colonized very restricted areas, but showed high flexibility in their phycobiont choice (Moya et al., 2021). Some species of Parmelia included in this study have broad geographical and ecological distribution ranges. Therefore, in these species (P. sulcata and P. saxatilis) the association with several Trebouxia species was expected, while for P. encryptata, with a more restricted distribution, lower diversity was to be assumed. However, since widely distributed P. sulcata associate with only one Trebouxia lineage, the differences in distribution ranges do not completely explain the differences found in specificity and selectivity patterns.
Moreover, colonization strategy and thallus morphology were described as key parameters in phycobiont choice and the range of associated phycobionts (Piercey-Normore, 2004; Bačkor et al., 2010; Moya et al., 2020). Piercey-Normore (2004) pointed out these factors to explain the association myco/phycobiont patterns in Cladonia. More recently, Moya et al. (2017, 2021) and Molins et al. (2021) underlined that different thallus parts of crustose or fruticulose lichen species could harbor different microalgal compositions. Also, Leavitt et al. (2015) highlighted evidence of the correlation between thalli growth forms and selectivity in Parmeliaceae mycobionts. In the present study, all the species included show similar substrata i.e., rocks and barks (although PSULgp spp. are epiphytes) and foliaceous patterns. Summarizing, our results show that interaction patterns in Parmelia may be influenced by a mixture of factors, including the type of vegetative structure, mycobiont distribution and habitat, with Cluster 6 (P. saxatilis A/P. saxatilis B/Trebouxia sp. 2/Trebouxia sp. S02/Trebouxia sp. 3A) being climatically the most distinct by preferring cold-temperate, humid-in-summer environments (non- Mediterranean bioclimates).
Data Availability Statement
The data presented in the study are deposited in the GenBank repository, and the accession number is provided in Supplementary Table 1.
Author Contributions
PM, AM, SC, and EB conceptualized the study. AM, SC, and CD performed the experiments and generated the data. PM, AM, PŠ, and PD performed by the formal analysis. EB, AC, MM, PŠ, and PD provided resources, funding, and supervision. EB provided project administration. All authors contributed to writing, reviewing and editing.
Funding
This work was supported by the Prometeo Excellence in Research Program (Generalitat Valenciana, Spain) (PROMETEOII/2013/021 and PROMETEO/2017/039), the Ministerio de Economía y Competitividad (MINECO and FEDER, Spain) (CGL2016-79158-P), the Primus Research Programme of Charles University SCI/13 and Spanish Ministerio de Ciencia e Innovación PID2019-105312GB-I00.
Conflict of Interest
The authors declare that the research was conducted in the absence of any commercial or financial relationships that could be construed as a potential conflict of interest.
Publisher’s Note
All claims expressed in this article are solely those of the authors and do not necessarily represent those of their affiliated organizations, or those of the publisher, the editors and the reviewers. Any product that may be evaluated in this article, or claim that may be made by its manufacturer, is not guaranteed or endorsed by the publisher.
Acknowledgments
Daniel Sheerin who revised the English manuscript.
Supplementary Material
The Supplementary Material for this article can be found online at: https://www.frontiersin.org/articles/10.3389/fmicb.2021.765310/full#supplementary-material
Supplementary Figure 1 | Summary of the three species delimitation analyses (GMYC, bPTP, and ABGD) using mycobiont nrITS sequences. The topology shown corresponds to the Bayesian ultrametric tree obtained in BEAST. Each color corresponds to the different fungal species delimited.
Supplementary Figure 2 | Trebouxia phylogenetic analysis. Rooted LSU rDNA gene tree representing 139 Trebouxia sequences, including 9 well−accepted Trebouxia species from SAG and UTEX retrieved from the GenBank. Newly generated sequences are marked as ex Parmelia spp._locality-code number. Seven Trebouxia species detected were indicated. Values at nodes indicate statistical support estimated by two methods: bootstrap support (BS, RAxML analysis) and posterior probabilities (PP, MrBayes analysis). Scale bar shows the estimated number of substitutions per site.
Supplementary Figure 3 | Summary of the three species delimitation analyses (GMYC, bPTP, and ABGD) using concatenated phycobiont nrITS and LSU DNA dataset. The topology shown corresponds to the Bayesian ultrametric tree obtained in BEAST. Each color corresponds to the different algal species delimited.
Supplementary Figure 4 | (A) Network diagram showing the location of the seven clusters (1–7) (B) Venn’s diagram showing the variation in distribution of the clusters explained by effects of climate, geography and substrate.
Supplementary Figure 5 | PCA result of seven clusters distribution depending on climatic factors. Large circles represent group centroids. Climatic data were obtained from the Global Climate Data—WorldClim. BIO1, Annual Mean Temperature; BIO2, Mean Diurnal Range [Mean of monthly (max temp—min temp)]; BIO3, Isothermality (BIO2/BIO7) (× 100); BIO4, Temperature Seasonality (standard deviation × 100); BIO5, Max Temperature of Warmest Month; BIO6, Min Temperature of Coldest Month; BIO7, Temperature Annual Range (BIO5-BIO6); BIO8, Mean Temperature of Wettest Quarter; BIO9, Mean Temperature of Driest Quarter; BIO10, Mean Temperature of Warmest Quarter; BIO11, Mean Temperature of Coldest Quarter; BIO12, Annual Precipitation; BIO13, Precipitation of Wettest Month; BIO14, Precipitation of Driest Month; BIO15, Precipitation Seasonality (Coefficient of Variation); BIO16, Precipitation of Wettest Quarter; BIO17, Precipitation of Driest Quarter; BIO18, Precipitation of Warmest Quarter; BIO19, Precipitation of Coldest Quarter.
Supplementary Table 1 | Samples used in this study, with details on collection data, herbarium codes and the GenBank accession numbers for the sequences generated for the nrITS (mycobiont and microalgae) and LSU rDNA.
Footnotes
- ^ http://technelysium.com.au/wp/chromas/
- ^ http://beast.bio.ed.ac.uk
- ^ http://species.h-its.org/ptp/
- ^ https://bioinfo.mnhn.fr/abi/public/abgd/abgdweb.html
References
Ahlmann-Eltze, C., and Patil, I. (2021). Ggsignif: R package for displaying significance brackets for ‘gplot2. PsyArXiv [Preprint] doi: 10.31234/osf.io/7awm6
Arnold, A. E., Miadlikowska, J., Higgins, K. L., Sarvate, S. D., Gugger, P., Way, A., et al. (2009). A phylogenetic estimation of trophic transition networks for ascomycetous fungi: are lichens cradles of symbiotrophic fungal diversification? Syst. Biol. 58, 283–297. doi: 10.1093/sysbio/syp001
Bačkor, M., Peksa, O., Škaloud, P., and Backorová, M. (2010). Photobiont diversity in lichens from metal-rich substrata based on ITS rDNA sequences. Ecotoxicol. Environ. Safe. 73, 603–612. doi: 10.1016/j.ecoenv.2009.11.002
Bastian, M., Heymann, S., and Jacomy, M. (2009). “Gephi: an open source software for exploring and manipulating networks,” in Proceedings of the International AAAI Conference on Weblogs and Social Media, San Jose, CA.
Beck, A., Kasalicky, T., and Rambold, G. (2002). Myco-photobiontal selection in a Mediterranean cryptogam community with Fulgensia fulgida. New Phytol. 153, 317–326.
Blaha, J., Baloch, E., and Grube, M. (2006). High photobiont diversity associated with the euryoecious lichen-forming ascomycete Lecanora rupicola (Lecanoraceae, Ascomycota). Biol. J. Linn. Soc. 88, 283–293. doi: 10.1111/j.1095-8312.2006.00640.x
Borcard, D., Legendre, P., Avois-Jacquet, C., and Tuomisto, H. (2004). Dissecting the spatial structure of ecological data at multiple scales. Ecology 85, 1826–1832.
Casano, L. M., del Campo, E., García-Breijo, F., Reig-Armiñana, J., Gasulla, F., del Hoyo, A., et al. (2011). Two Trebouxia algae with different physiological performances are ever present in lichen thalli of Ramalina farinacea. Coexistence versus competition? Appl. Environ. Microbiol. 13, 806–818. doi: 10.1111/j.1462-2920.2010.02386.x
Chagnon, P. L., Magain, N., Miadlikowska, J., and Lutzoni, F. (2019). Species diversification and phylogenetically constrained symbiont switching generated high modularity in the lichen genus Peltigera. J. Ecol. 107, 1645–1661. doi: 10.1111/1365-2745.13207
Crespo, A., Bridge, P. D., Cubero, O. F., and Hawksworth, D. L. (1997). Determination of genotypic variability in the lichen-forming fungus Parmelia sulcata. Bibl. Lichenol. 68, 73–79.
Crespo, A., Bridge, P. D., Hawksworth, D. L., Grube, M., and Cubero, O. F. (1999). Comparison of rRNA genotype frequencies of Parmelia sulcata from long established and recolonizing sites following sulphur dioxide amelioration. Plant Syst. Evol. 217, 177–183.
Crespo, A., Kauff, F., Divakar, P. K., del Prado, R., Pérez-Ortega, S., de Paz, G., et al. (2010). Phylogenetic generic classification of parmelioid lichens (Parmeliaceae, Ascomycota) based on molecular, morphological and chemical evidence. Taxon 59, 1735–1753. doi: 10.1002/tax.596008
Crespo, A., Molina, M. C., Blanco, O., Schroeter, B., Sancho, L. G., and Hawksworth, D. L. (2002). rDNA ITS and beta-tubulin gene sequence analyses reveal two monophyletic groups within the cosmopolitan lichen Parmelia saxatilis. Mycol. Res. 106, 788–795. doi: 10.1017/s095375620200610x
Crespo, A., Rico, V. J., Garrido, E., Lumbsch, H. T., and Divakar, P. K. (2020). A revision of species of the Parmelia saxatilis complex in the Iberian Peninsula with the description of P. rojoi, a new potentially relict species. Lichenologist 52, 365–376.
Currie, D. J., Mittelbach, G. G., Cornell, H. V., Field, R., Guégan, J. F., Hawkins, B. A., et al. (2004). Predictions and tests of climate-based hypotheses of broad-scale variation in taxonomic richness. Ecol. Lett. 7, 1121–1134. doi: 10.1111/j.1461-0248.2004.00671.x
Dal Grande, F., Rolshausen, G., Divakar, P. K., Crespo, A., Otte, J., Schleuning, M., et al. (2018). Environment and host identity structure communities of green algal symbionts in lichens. New Phytol. 217, 277–289. doi: 10.1111/nph.14770
Darriba, D., Taboada, G. L., Doallo, R., and Posada, D. (2012). jModelTest 2, more models, new heuristics and parallel computing. Nat. Methods 9:772. doi: 10.1038/nmeth.2109
del Campo, E. M., Gimeno, J., De Nova, J. P. G., Casano, L. M., Gasulla, F., García-Breijo, F., et al. (2010). South European populations of Ramalina farinacea (L) Ach share different Trebouxia algae. Bibl. Lichenol. 105, 247–256.
Divakar, P. K., Leavitt, S. D., Molina, M. C., Del-Prado, R., Lumbsch, H. T., and Crespo, A. (2016). A DNA barcoding approach for identification of hidden diversity in Parmeliaceae (Ascomycota): Parmelia sensu stricto as a case study. Bot. J. Linn. Soc. 180, 21–29.
Divakar, P. K., Molina, M. C., Lumbsch, H. T., and Crespo, A. (2005). Parmelia barrenoae, a new lichen species related to Parmelia sulcata (Parmeliaceae) based on molecular and morphological data. Lichenologist 37, 37–46.
Drummond, A. J., Suchard, M. A., Xie, D., and Rambaut, A. (2012). Bayesian phylogenetics with BEAUti and the BEAST 1.7. Mol. Biol. Evol. 29, 1969–1973. doi: 10.1093/molbev/mss075
Dumitru, C. (2019). Las Simbiosis Liquénicas Como Sistemas Mutualistas Complejos: Diversidad y Coexistencia De Microalgas En El Género Parmelia. Trabajo Fin De Máster, Facultad De Ciencias Biológicas. Valencia: Universitat de València, 1–65.
Ertz, D., Guzow-Krzemińska, B., Thor, G., Łubek, A., and Kukwa, M. (2018). Photobiont switching causes changes in the reproduction strategy and phenotypic dimorphism in the Arthoniomycetes. Sci. Rep. 8, 1–14. doi: 10.1038/s41598-018-23219-3
Fernández-Mendoza, F., Domaschke, S., García, M. A., Jordan, P., Martín, M. P., and Printzen, C. (2011). Population structure of mycobionts and photobionts of the widespread lichen Cetraria aculeata. Mol. Ecol. 20, 1208–1232. doi: 10.1111/j.1365-294X.2010.04993.x
Gardes, M., and Bruns, T. D. (1993). ITS primers with enhanced specificity for basidiomycetes-application to the identification of mycorrhizae and rusts. Mol. Ecol. 2, 113–118. doi: 10.1111/j.1365-294x.1993.tb00005.x
Garrido-Benavent, I., Molins, A., and Barreno, E. (2021). Genetic variation of the symbiont partners in the endangered macrolichen Seirophora villosa (Teloschistaceae, Ascomycota). Bot. J. Linn. Soc. doi: 10.1093/botlinnean/boab100
Grube, M., and Wedin, M. (2016). Lichenized fungi and the evolution of symbiotic organization. Microbiol. Spectr. 4, 4–6. doi: 10.1128/microbiolspec.FUNK-0011-2016
Guindon, S., and Gascuel, O. (2003). A simple, fast, and accurate algorithm to estimate large phylogenies by maximum likelihood. Syst. Biol. 52, 696–704. doi: 10.1080/10635150390235520
Hale, M. E. Jr. (1987). A monograph of the lichen genus Parmelia Acharius sensu stricto (Ascomycotina: Parmeliaceae). Smithsonian Contr. Bot. 66, 1–55. doi: 10.5479/si.0081024x.66
Hawksworth, D. L., Blanco, O., Divakar, P. K., Ahti, T., and Crespo, A. (2008). A first checklist of parmelioid and similar lichens in Europe and some adjacent territories, adopting revised generic circumscriptions and with indications of species distributions. Lichenologist 40, 1–21. doi: 10.1017/s0024282908007329
Hawksworth, D. L., Divakar, P. K., Crespo, A., and Ahti, T. (2011). The checklist of parmelioid and similar lichens in Europe and some adjacent territories: additions and corrections. Lichenologist 43, 639–645. doi: 10.1017/s0024282911000454
Hawksworth, D. L., and Honegger, R. (1994). “The lichen thallus: a symbiotic phenotype of nutritionally specialized fungi and its response to gall producers,” in Plant Galls, ed. M. A. J. Williams (Oxford: Clarendon Press), 77–98.
Helms, G., Friedl, T., and Rambold, G. (2003). Phylogenetic relationships of the physciaceae inferred from rDNA sequence data and selected phenotypic characters. Mycologia 95, 1078–1099. doi: 10.1080/15572536.2004.11833022
Hillis, D. M., and Bull, J. J. (1993). An empirical test of bootstrapping as a method for assessing confidence in phylogenetic analysis. Syst. Biol. 42, 182–192.
Jackson, D. A. (1993). Stopping rules in principal components analysis: a comparison of heuristical and statistical approaches. Ecology 74, 2204–2214. doi: 10.2307/1939574
Jüriado, I., Kaasalainen, U., Jylhä, M., and Rikkinen, J. (2019). Relationships between mycobiont identity, photobiont specificity and ecological preferences in the lichen genus Peltigera (Ascomycota) in Estonia (northeastern Europe). Fungal Ecol. 39, 45–54. doi: 10.1016/j.funeco.2018.11.005
Karger, D. N., Conrad, O., Böhner, J., Kawohl, T., Kreft, H., Soria-Auza, R. W., et al. (2017). Climatologies at high resolution for the earth’s land surface areas. Sci. Data 4, 1–20.
Katoh, K., Misawa, K., Kuma, K., and Miyata, T. (2002). MAFFT: a novel method for rapid multiple sequence alignment based on fast Fourier transform. Nucleic Acids Res. 30, 3059–3066. doi: 10.1093/nar/gkf436
Katoh, K., and Toh, H. (2008). Recent developments in the MAFFT multiple sequence alignment program. Brief. Bioinform. 9, 286–298. doi: 10.1093/bib/bbn013
Khakhina, L. N., Margulis, L., and McMenamin, M. (1993). Concepts of Symbiogenesis: History of Symbiosis as an Evolutionary Mechanism. New Haven: Yale University Press.
Kosecka, M., Jabłońska, A., Flakus, A., Rodriguez-Flakus, P., Kukwa, M., and Guzow-Krzemińska, B. (2020). Trentepohlialean algae (Trentepohliales, Ulvophyceae) show preference to selected mycobiont lineages in lichen symbioses. J. Phycol. 56, 979–993. doi: 10.1111/jpy.12994
Kroken, S., and Taylor, J. W. (2000). Phylogenetic species, reproductive mode, and specificity of the green alga Trebouxia forming lichens with the fungal genus Letharia. Bryologist 103, 645–660. doi: 10.1639/0007-2745(2000)103[0645:psrmas]2.0.co;2
Leavitt, S. D., Kraichak, E., Nelsen, M. P., Altermann, S., Divakar, P. K., Alors, D., et al. (2015). Fungal specificity and selectivity for algae play a major role in determining lichen partnerships across diverse ecogeographic regions in the lichen-forming family Parmeliaceae (Ascomycota). Mol. Ecol. 24, 3779–3797. doi: 10.1111/mec.13271
Leavitt, S. D., Kraichak, E., Vondrak, J., Nelsen, M. P., Sohrabi, M., Pérez-Ortega, S., et al. (2016). Cryptic diversity and symbiont interactions in rock-posy lichens. Mol. Phylogenet. Evol. 99, 261–274. doi: 10.1016/j.ympev.2016.03.030
Lefeuvre, P. (2018). BoSSA: A Bunch of Structure and Sequence Analysis. R package version, 1. Available online at: http://cran.r-project.org/web/packages/BoSSA/index.html (accessed October 20, 2020)
Lücking, R., Hodkinson, B. P., and Leavitt, S. D. (2017). The 2016 classification of lichenized fungi in the Ascomycota and Basidiomycota – approaching one thousand genera. Bryologist 119, 361–416.
Magain, N., Miadlikowska, J., Goffinet, B., Sérusiaux, E., and Lutzoni, F. (2017). Macroevolution of specificity in cyanolichens of the genus Peltigera section polydactylon (Lecanoromycetes, Ascomycota). Syst. Biol. 66, 74–99. doi: 10.1093/sysbio/syw065
Mark, K., Laanisto, L., Bueno, C. G., Niinemets, Ü, Keller, C., and Scheidegger, C. (2020). Contrasting co-occurrence patterns of photobiont and cystobasidiomycete yeast associated with common epiphytic lichen species. New Phytol. 227, 1362–1375. doi: 10.1111/nph.16475
Miadlikowska, J., Kauff, F., Hofstetter, V., Fraker, E., Grube, M., Hafellner, J., et al. (2006). New insights into classification and evolution of the Lecanoromycetes (Pezizomycotina, Ascomycota) from phylogenetic analyses of three ribosomal RNA-and two protein-coding genes. Mycologia 98, 1088–1103. doi: 10.3852/mycologia.98.6.1088
Miller, M., Pfeiffer, W., and Schwartz, T. (2011). “CIPRES science gateway: a community resource for phylogenetic analyses,” in Proceedings of the 2011 TeraGrid Conference: Extreme Digital Discovery, July 18- 21, Salt Lake City, UT.
Molina, M. C., Divakar, P. K., Millanes, A. M., Sanchez, E., Del-Prado, R., Hawksworth, D. L., et al. (2011a). Parmelia sulcata (Ascomycota: Parmeliaceae), a sympatric monophyletic species complex. Lichenologist 43, 585–601. doi: 10.1017/s0024282911000521
Molina, M. C., Del-Prado, R., Divakar, P. K., Sánchez-Mata, D., and Crespo, A. (2011b). Another example of cryptic diversity in lichen-forming fungi: the new species Parmelia mayi (Ascomycota: Parmeliaceae). Org. Divers. Evol. 11, 331–342. doi: 10.1007/s13127-011-0060-4
Molina, M. C., Divakar, P. K., Goward, T., Millanes, A. M., Lumbsch, H. T., and Crespo, A. (2017). Neogene diversification in the temperate lichen-forming fungal genus Parmelia (Parmeliaceae, Ascomycota). System. Biodivers. 15, 166–181. doi: 10.1080/14772000.2016.1226977
Molina, M. D., Crespo, A., Blanco, O., Lumbsch, H. T., and Hawksworth, D. L. (2004). Phylogenetic relationships and species concepts in Parmelia s. str. (Parmeliaceae) inferred from nuclear ITS rDNA and beta-tubulin sequences. Lichenologist 36, 37–54.
Molins, A., Moya, P., Muggia, L., and Barreno, E. (2021). Thallus growth stage and geographic origin shape microalgal diversity in Ramalina farinacea lichen holobionts. J. Phycol. 57, 975–987. doi: 10.1111/jpy.13140
Monaghan, M. T., Wild, R., Elliot, M., Fujisawa, T., Balke, M., Inward, D. J. G., et al. (2009). Accelerated species inventory on Madagascar using coalescent-based models of species delineation. Syst. Biol. 58, 298–311. doi: 10.1093/sysbio/syp027
Moya, P., Chiva, S., Molins, A., Garrido-Benavent, I., and Barreno, E. (2021). Unravelling the symbiotic microalgal diversity in Buellia zoharyi (lichenized Ascomicota) from the Iberian Peninsula and Balearic Islands using DNA Metabarcoding. Diversity 13:220. doi: 10.3390/d13060220
Moya, P., Chiva, S., Molins, A., Jadrná, I., Škaloud, P., Peksa, O., et al. (2018). Myrmecia israeliensis as the primary symbiotic microalga in squamulose lichens growing in European and Canary Island terricolous communities. Fottea 18, 72–85. doi: 10.5507/fot.2017.022
Moya, P., Molins, A., Chiva, S., Bastida, J., and Barreno, E. (2020). Symbiotic microalgal diversity within lichenicolous lichens and crustose hosts on Iberian Peninsula gypsum biocrusts. Sci. Rep. 10:14060. doi: 10.1038/s41598-020-71046-2
Moya, P., Molins, A., Martínez-Alberola, F., Muggia, L., and Barreno, E. (2017). Unexpected associated microalgal diversity in the lichen Ramalina farinacea is uncovered by pyrosequencing analyses. PLoS One 12:e0175091. doi: 10.1371/journal.pone.0175091
Muggia, L., and Grube, M. (2018). Fungal diversity in lichens: from extremotolerance to interactions with algae. Life 8:15. doi: 10.3390/life8020015
Muggia, L., Leavitt, S., and Barreno, E. (2018). The hidden diversity of lichenised Trebouxiophyceae (Chlorophyta). Phycologia, 57, 503–524. doi: 10.2216/17-134.1
Muggia, L., Nelsen, M., Kirika, P. M., Barreno, E., Beck, A., Lindgren, H., et al. (2020). Formally described species woefully underrepresent phylogenetic diversity in the common lichen photobiont genus Trebouxia (Trebouxiophyceae, Chlorophyta): an impetus for developing an integrated taxonomy. Mol. Phylogenet. Evol. 149:106821. doi: 10.1016/j.ympev.2020.106821
Muggia, L., Pérez-Ortega, S., Fryday, A., Spribille, T., and Grube, M. (2014). Global assessment of genetic variation and phenotypic plasticity in the lichen-forming species Tephromela atra. Fungal Divers. 64, 233–251. doi: 10.1007/s13225-013-0271-4
Muggia, L., Vančurová, L., Škaloud, P., Peksa, O., Wedin, M., and Grube, M. (2013). The symbiotic playground of lichen thalli – a highly flexible photobiont association in rock-inhabiting lichens. FEMS Microbiol. Ecol. 85, 313–323. doi: 10.1111/1574-6941.12120
Nelsen, M. P. (2021). Sharing and double-dating in the lichen world. Mol. Ecol. 30, 1751–1754. doi: 10.1111/mec.15884
Nelsen, M. P., and Gargas, A. (2009). Symbiont flexibility in Thamnolia vermicularis (Pertusariales: Icmadophilaceae). Bryologist 112, 404–417. doi: 10.1639/0007-2745-112.2.404
O’Brien, H. E., Miadlikowska, J., and Lutzoni, F. (2013). Assessing population structure and host specialization in lichenized cyanobacteria. New Phytol. 198, 557–566. doi: 10.1111/nph.12165
Oksanen, J., Blanchet, F. G., Friendly, M., Kindt, R., Legendre, P., Mcglinn, D., et al. (2017). Community Ecology Package ‘vegan’. Version 2.4-4. Available online at: https://cran.r-project.org/web/packages/vegan/vegan.pdf (accessed November 28, 2020).
Ossowska, E., Guzow-Krzemińska, B., Kolanowska, M., Szczepańska, K., and Kukwa, M. (2019). Morphology and secondary chemistry in species recognition of Parmelia omphalodes group – evidence from molecular data with notes on the ecological niche modelling and genetic variability of photobionts. Mycokeys 61, 39–74. doi: 10.3897/mycokeys.61.38175
Otálora, M. A., Aragón, G., Martínez, I., and Wedin, M. (2013). Cardinal characters on a slippery slope – a re-evaluation of phylogeny, character evolution, and evolutionary rates in the jelly lichens (Collemataceae s. str). Mol. Phylogenet. Evol. 68, 185–198. doi: 10.1016/j.ympev.2013.04.004
Otálora, M. A., Martínez, I., O’Brien, H., Molina, M. C., Aragón, G., and Lutzoni, F. (2010). Multiple origins of high reciprocal symbiotic specificity at an intercontinental spatial scale among gelatinous lichens (Collemataceae, Lecanoromycetes). Mol. Phyl. Evol. 56, 1089–1095. doi: 10.1016/j.ympev.2010.05.013
Pardo-De la Hoz, C. J., Magain, N., Lutzoni, F., Goward, T., Restrepo, S., and Miadlikowska, J. (2018). Contrasting symbiotic patterns in two closely related lineages of trimembered lichens of the genus Peltigera. Front. Microbiol. 9:2770. doi: 10.3389/fmicb.2018.02770
Peksa, O., and Škaloud, P. (2011). Do photobionts influence the ecology of lichens? A case study of environmental preferences in symbiotic green alga Asterochloris (Trebouxiophyceae). Mol. Ecol. 20, 3936–3948. doi: 10.1111/j.1365-294X.2011.05168.x
Piercey-Normore, M. D. (2004). Selection of algal genotypes by three species of lichen fungi in the genus Cladonia. Can. J. Bot. 82, 947–961.
Piercey-Normore, M. D., and DePriest, P. T. (2001). Algal switching among lichen symbioses. Am. J. Bot. 88, 1490–1498. doi: 10.2307/3558457
Pino-Bodas, R., and Stenroos, S. (2020). Global biodiversity patterns of the photobionts associated with the genus Cladonia (Lecanorales, Ascomycota). Microb. Ecol. 82, 1–15. doi: 10.1007/s00248-020-01633-3
Printzen, C., Domaschke, S., Fernández-Mendoza, F., and Pérez-Ortega, S. (2013). Biogeography and ecology of Cetraria aculeata, a widely distributed lichen with a bipolar distribution. Mycokeys 6, 33–53. doi: 10.3897/mycokeys.6.3185
R Core Team (2013). R: A Language and Environment for Statistical Computing. Vienna: R Foundation for Statistical Computing.
Rambaut, A. (2012). FigTree Version 1.4.0. Available online at: http://tree.bio.ed.ac.uk/software/figtree (accessed December 1, 2021).
Rambaut, A., Drummond, A. J., Xie, D., Baele, G., and Suchard, M. A. (2018). Posterior summarization in Bayesian phylogenetics using Tracer 1.7. Syst. Biol. 67, 901–904. doi: 10.1093/sysbio/syy032
Řídká, T., Peksa, O., Rai, H., Upreti, D. K., and Škaloud, P. (2014). “Photobiont diversity in Indian Cladonia lichens, with special emphasis on the geographical patterns,” in Terricolous Lichens in India, eds H. Rai and D. K. Upreti (New York, NY: Springer), 53–71. doi: 10.1007/978-1-4614-8736-4_4
Rivas-Martínez, S., Penas, Á, del Río, S., González, T. E. D., and Rivas-Sáenz, S. (2017). “Bioclimatology of the Iberian Peninsula and the Balearic Islands,” in The Vegetation of the Iberian Peninsula, ed. J. Lloidi (Cham: Springer), 29–80. doi: 10.1007/978-3-319-54784-8_2
Rolshausen, G., Hallman, U., Grande, F. D., Otte, J., Knudsen, K., and Schmitt, I. (2020). Expanding the mutualistic niche: parallel symbiont turnover along climatic gradients. Proc. R. Soc. B 287:20192311. doi: 10.1098/rspb.2019.2311
Ronquist, F., Teslenko, M., van der Mark, P., Ayres, D. L., Darling, A., Höhna, S., et al. (2012). MrBayes 3 2, efficient Bayesian phylogenetic inference and model choice across a large model space. Syst. Biol. 61, 539–542. doi: 10.1093/sysbio/sys029
Sadowska-Deś, A. D., Dal Grande, F., Lumbsch, H. T., Beck, A., Otte, J., Hur, J. S., et al. (2014). Integrating coalescent and phylogenetic approaches to delimit species in the lichen photobiont Trebouxia. Mol. Phylogenet. Evol. 76, 202–210. doi: 10.1016/j.ympev.2014.03.020
Sayers, E. W., Barrett, T., Benson, D. A., Bolton, E., Bryant, S. H., Canese, K., et al. (2011). Database resources of the national center for biotechnology information. Nucleic Acids Res. 39, 38–51.
Singh, G., Dal Grande, F., Divakar, P. K., Otte, J., Crespo, A., and Schmitt, I. (2017). Fungal–algal association patterns in lichen symbiosis linked to macroclimate. New Phytol. 214, 317–329. doi: 10.1111/nph.14366
Smith, C. W., Aptroot, A., Coppins, B. J., Fletcher, A., Gilbert, O. L., James, P. W., et al. (2009). The lichens of Great Britain and Ireland, 2nd Edn. London: British Lichen Society.
Sork, V. L., and Werth, S. (2014). Phylogeography of Ramalina menziesii, a widely distributed lichen-forming fungus in western North America. Mol. Ecol. 23, 2326–2339. doi: 10.1111/mec.12735
Stamatakis, A. (2006). RAxML-VI-HPC, maximum likelihood-based phylogenetic analyses with thousands of taxa and mixed models. Bioinformatics 22, 2688–2690. doi: 10.1093/bioinformatics/btl446
Stamatakis, A. (2014). RAxML version 8: a tool for phylogenetic analysis and post-analysis of large phylogenies. Bioinformatics 30, 1312–1313. doi: 10.1093/bioinformatics/btu033
Stamatakis, A., Hoover, P., and Rougemont, J. (2008). A rapid bootstrap algorithm for the RAxML web servers. Syst. Biol. 57, 758–771. doi: 10.1080/10635150802429642
Steinová, J., Škaloud, P., Yahr, R., Bestová, H., and Muggia, L. (2019). Reproductive and dispersal strategies shape the diversity of mycobiont-photobiont association in Cladonia lichens. Mol. Phylogenet. Evol. 134, 226–237. doi: 10.1016/j.ympev.2019.02.014
Vančurová, L., Muggia, L., Peksa, O., Řídká, T., and Škaloud, P. (2018). The complexity of symbiotic interactions influences the ecological amplitude of the host: a case study in Stereocaulon (lichenized Ascomycota). Mol. Ecol. 27, 3016–3033. doi: 10.1111/mec.14764
White, T. J., Bruns, T., Lee, S., and Taylor, J. (1990). “Amplification and direct sequencing of fungal ribosomal RNA genes for phylogenetics,” in PCR Protocols: A Guide to Methods and Applications, Vol. 18, eds M. A. Innis, D. H. Gelfand, J. J. Snisky, and T. J. White (San Diego, CA: Academic Press), 315–322. doi: 10.1016/b978-0-12-372180-8.50042-1
Wirtz, N., Lumbsch, H. T., Green, T. A., Türk, R., Pintado, A., Sancho, L., et al. (2003). Lichen fungi have low cyanobiont selectivity in maritime Antarctica. New Phytol. 160, 177–183. doi: 10.1046/j.1469-8137.2003.00859.x
Xu, M., De Boer, H., Olafsdottir, E. S., Omarsdottir, S., and Heidmarsson, S. (2020). Phylogenetic diversity of the lichenized algal genus Trebouxia (Trebouxiophyceae, Chlorophyta): a new lineage and novel insights from fungal-algal association patterns of Icelandic cetrarioid lichens (Parmeliaceae, Ascomycota). Bot. J. Linn. Soc. 194, 460–468. doi: 10.1093/botlinnean/boaa050
Yahr, R., Vilgalys, R., and Depriest, P. T. (2004). Strong fungal specificity and selectivity for algal symbionts in Florida scrub Cladonia lichens. Mol. Ecol. 13, 3367–3378. doi: 10.1111/j.1365-294X.2004.02350.x
Keywords: distribution, habitat, microalgae, phycobiont, symbiosis, Trebouxia
Citation: Moya P, Molins A, Škaloud P, Divakar PK, Chiva S, Dumitru C, Molina MC, Crespo A and Barreno E (2021) Biodiversity Patterns and Ecological Preferences of the Photobionts Associated With the Lichen-Forming Genus Parmelia. Front. Microbiol. 12:765310. doi: 10.3389/fmicb.2021.765310
Received: 26 August 2021; Accepted: 26 November 2021;
Published: 24 December 2021.
Edited by:
Martin Kukwa, University of Gdańsk, PolandReviewed by:
Samantha Chandranath Karunarathna, Key Laboratory for Plant Diversity and Biogeography of East Asia, Kunming Institute of Botany, Chinese Academy of Sciences (CAS), ChinaXin-Zhan Liu, Institute of Microbiology, Chinese Academy of Sciences (CAS), China
Copyright © 2021 Moya, Molins, Škaloud, Divakar, Chiva, Dumitru, Molina, Crespo and Barreno. This is an open-access article distributed under the terms of the Creative Commons Attribution License (CC BY). The use, distribution or reproduction in other forums is permitted, provided the original author(s) and the copyright owner(s) are credited and that the original publication in this journal is cited, in accordance with accepted academic practice. No use, distribution or reproduction is permitted which does not comply with these terms.
*Correspondence: Patricia Moya, patricia.moya@uv.es
†ORCID: Patricia Moya, orcid.org/0000-0003-0397-863X; Arantzazu Molins, orcid.org/0000-0003-3449-1023; Pavel Škaloud, orcid.org/0000-0003-1201-3290; Pradeep K. Divakar, orcid.org/0000-0002-0300-0124; Salvador Chiva, orcid.org/0000-0002-1615-6443; Cristina Dumitru, orcid.org/0000-0003-1479-6057; Maria Carmen Molina, orcid.org/0000-0002-8397-5887; Ana Crespo, orcid.org/0000-0002-5271-0157; Eva Barreno, orcid.org/0000-0002-2622-4550
‡These authors have contributed equally to this work
§Present address: Arantzazu Molins, Research Group on Plant Biology under Mediterranean Conditions, Departament de Biologia, INAGEA, Universitat de les Illes Balears, Illes Balears, Spain