- 1Programa de Microbiología y Micología, Facultad de Medicina, Instituto de Ciencias Biomédicas, Universidad de Chile, Santiago, Chile
- 2Programa Disciplinario de Inmunología, Facultad de Medicina, Instituto de Ciencias Biomédicas, Universidad de Chile, Santiago, Chile
- 3C+, Research Center in Technologies for Society, School of Engineering, Universidad del Desarrollo, Santiago, Chile
- 4Millennium Initiative for Collaborative Research on Bacterial Resistance, Santiago, Chile
- 5Departamento de Estudios Científicos, Instituto de Salud Pública de Chile, Santiago, Chile
- 6Escuela de Tecnología Médica, Facultad de Medicina, Universidad de Chile, Santiago, Chile
- 7Integramédica-BUPA, Santiago, Chile
Shigellosis is an enteric infectious disease in which antibiotic treatment is effective, shortening the duration of symptoms and reducing the excretion of the pathogen into the environment. Shigella spp., the etiologic agent, are considered emerging pathogens with a high public health impact due to the increase and global spread of multidrug-resistant (MDR) strains. Since Shigella resistance phenotype varies worldwide, we present an overview of the resistance phenotypes and associated genetic determinants present in 349 Chilean S. sonnei strains isolated during the periods 1995–1997, 2002–2004, 2008–2009, and 2010–2013. We detected a great variability in antibiotic susceptibility patterns, finding 300 (86%) MDR strains. Mobile genetic elements (MGE), such as plasmids, integrons, and genomic islands, have been associated with the MDR phenotypes. The Shigella resistance locus pathogenicity island (SRL PAI), which encodes for ampicillin, streptomycin, chloramphenicol, and tetracycline resistance genes, was detected by PCR in 100% of the strains isolated in 2008–2009 but was less frequent in isolates from other periods. The presence or absence of SRL PAI was also differentiated by pulsed-field gel electrophoresis. An atypical class 1 integron which harbors the blaOXA–1-aadA1-IS1 organization was detected as part of SRL PAI. The dfrA14 gene conferring trimethoprim resistance was present in 98.8% of the 2008–2009 isolates, distinguishing them from the SRL-positive strains isolated before that. Thus, it seems an SRL-dfrA14 S. sonnei clone spread during the 2008–2009 period and declined thereafter. Besides these, SRL-negative strains harboring class 2 integrons with or without resistance to nalidixic acid were detected from 2011 onward, suggesting the circulation of another clone. Whole-genome sequencing of selected strains confirmed the results obtained by PCR and phenotypic analysis. It is highlighted that 70.8% of the MDR strains harbored one or more of the MGE evaluated, while 15.2% lacked both SRL PAI and integrons. These results underscore the temporal dynamics of antimicrobial resistance in S. sonnei strains circulating in Chile, mainly determined by the spread of MGE conferring MDR phenotypes. Since shigellosis is endemic in Chile, constant surveillance of antimicrobial resistance phenotypes and their genetic basis is a priority to contribute to public health policies.
Introduction
Shigellosis is one of the most common diarrheal diseases in children under 5 years old, especially in developing countries, although it occurs in people of all ages worldwide (Kotloff et al., 2018). Shigella spp., the etiologic agent, are considered emerging pathogens with a high public health impact due to the increase and global spread of multidrug-resistant (MDR) strains (Chu et al., 1998; Ashkenazi et al., 2003; Vrints et al., 2009; Shiferaw et al., 2012; Gu et al., 2017; Puzari et al., 2018). This situation is particularly worrying because shigellosis is one of the few enteric infections for which antibiotics are recommended as therapeutic management (Williams and Berkley, 2016, 2018). Antibiotics shorten the severity and duration of symptoms, reducing the excretion time of the pathogen and potential complications of the infection (Chu et al., 1998).
Shigella resistance phenotype varies worldwide. The emergence of Shigella spp. resistant to the most commonly used antibiotics for treating this disease is reported in Latin America, North America, Europe, and South Asia (Chu et al., 1998; Oh et al., 2003; Gu et al., 2012; Shiferaw et al., 2012; Marcoleta et al., 2013; Puzari et al., 2018; Chung The et al., 2019, 2021; Sati et al., 2019; Bardsley et al., 2020).
The genetic determinants of MDR phenotypes in Shigella spp. have been associated with the presence of different plasmids, integrons, and genomic islands (Muñoz et al., 2003; Turner et al., 2003; Toro et al., 2005; Barrantes and Achí, 2016; Miranda et al., 2016; Kang et al., 2019; Ranjbar and Farahani, 2019). The Shigella resistance locus pathogenicity island (SRL PAI) is a 66-kb genomic region inserted into the serX gene (which codifies for tRNASer), conferring resistance to ampicillin, streptomycin, chloramphenicol, and tetracycline. A ferric dicitrate transport system and genes involved in D-aspartate metabolism are also encoded in SRL PAI, described firstly in Shigella flexneri 2a YSH6000 (SRLYSH6000; Luck et al., 2001; Turner et al., 2003; Henríquez et al., 2020). Additionally, integrons have played an essential role in the spread of antimicrobial resistance genes since their discovery in 1989. Integrons are composed of three elements: the intI gene that encodes a tyrosine recombinase (integrase), the adjacent recombination site (attI) recognized by the integrase, and the promoter (Pc) necessary for the efficient transcription and expression of gene cassettes present in the integron. They have the ability to capture and express foreign genes, such as resistance gene cassettes, and facilitate their horizontal transfer to a wide range of pathogens (Stokes and Hall, 1989; Recchia and Hall, 1995). Class 1 and class 2 integrons have widely disseminated among species of the family Enterobacteriaceae and other Gram-negative bacteria. In Shigella spp., antimicrobial resistance is often due to both classes of integrons (Pan et al., 2006; Chang et al., 2011; Kaushik et al., 2018; Shariati et al., 2018; Kang et al., 2019).
The heterogeneous geographical distribution of antimicrobial-resistant strains and mobile genetic elements (MGE) underscores the importance of keeping the local antimicrobial resistance surveillance. Hence, the objective of this study was to characterize the phenotypic and genotypic diversity of Chilean S. sonnei that have circulated for almost two decades from 1995 to 2013, focusing on the MDR strains.
Materials and Methods
Bacterial Strains and Culture Conditions
A total of 349 Shigella sonnei strains were obtained from a bacterial collection belonging to the Programa de Microbiología y Micología, ICBM, Universidad de Chile, isolated from stool samples of patients suffering from acute diarrhea (n = 190; 1995–2004) and from the Instituto de Salud Pública de Chile [ISP], 2019 (Public Health Institute of Chile) (n = 159; 2010–2013). These strains were grouped into five time periods according to the isolation date: period A, between 1995 and 1997 (n = 60); period B, between 2002 and 2004 (n = 50); period C, between 2008 and 2009 (n = 80); period D, between 2010 and 2011 (n = 80); and period E, between 2012 and 2013 (n = 79) (Figures 1B,C). S. sonnei strains from periods A and B were isolated from patients living in Región Metropolitana. Period C is composed of strains mostly isolated from Región Metropolitana (61/80) and Antofagasta (15/80), a region located 1,000 km north of Región Metropolitana. Periods D and E include representative strains from Región Metropolitana (40 in each period) and northern and southern areas of the country (Figures 1B,C). The isolates were stored at −80°C in trypticase soy broth with 30% glycerol. The Shigella strains were identified by conventional and automated biochemical methods (VITEK-2, bioMérieux) and serotyped by agglutination with type-specific antisera (phase I and II, Denka Seiken, Tokyo, Japan). All strains were routinely cultured at 37°C on LB broth or agar.
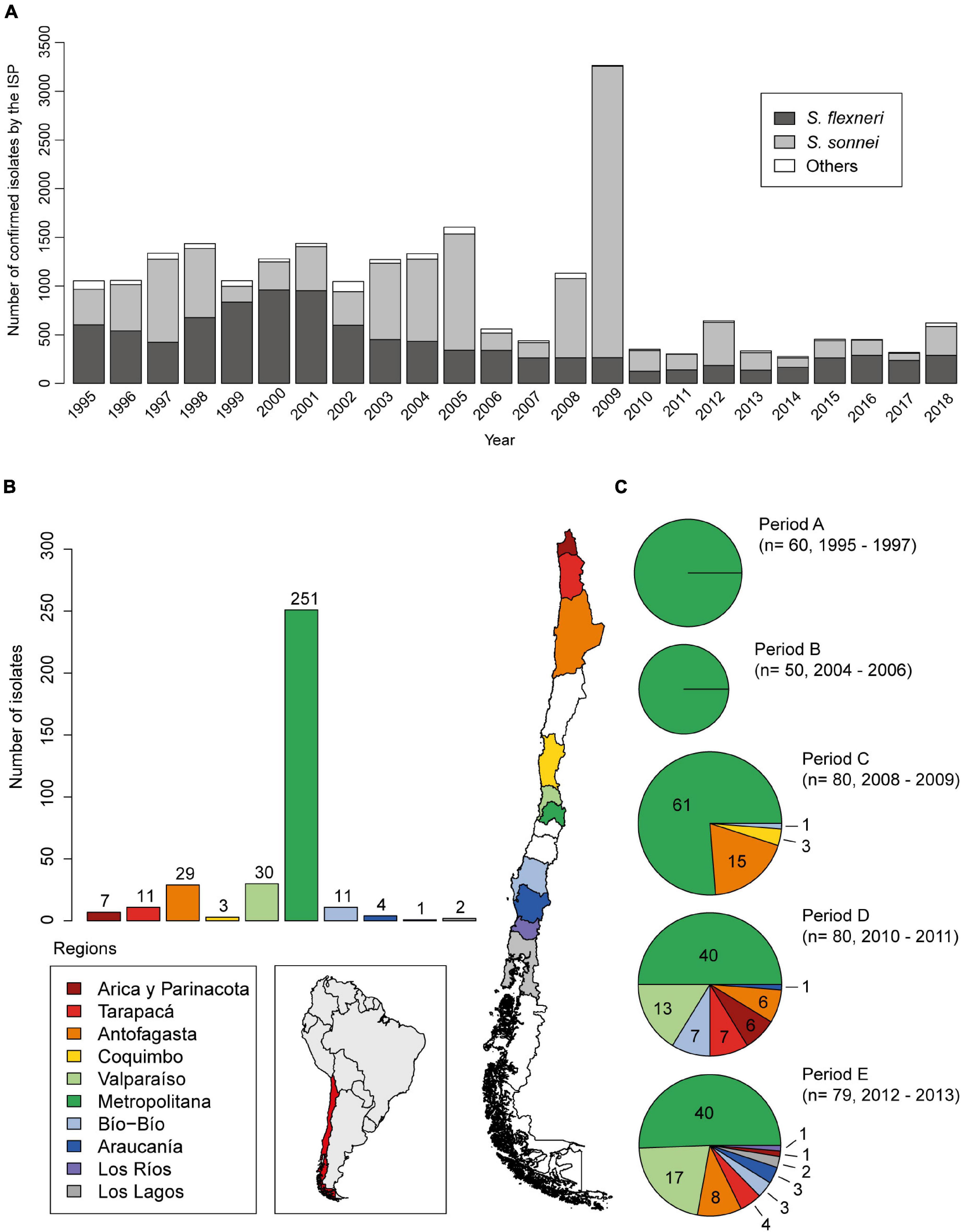
Figure 1. Epidemiological distribution of Shigella sonnei in Chile. (A) Nationwide detection of Shigella spp. strains confirmed by Instituto de Salud Pública de Chile, ISP from 1995 to 2018. (B) Geographical distribution of 349 Shigella sonnei strains analyzed in this work. (C) Characterization of groups defined by time of isolation and geographical distribution. S. sonnei strains from periods A and B were isolated from patients living in Region Metropolitana. Period C is composed by strains mostly isolated from the Region Metropolitana (61/80) and from Antofagasta (15/80), a region 1,000 km north from Santiago (Región Metropolitana, Chile). Periods D and E include representative strains from Región Metropolitana (40 in each period) and from the northern and southern regions of the country, which were kindly provided by the ISP (B,C).
Antimicrobial Susceptibility
The antibiotic susceptibility of S. sonnei strains was determined by disk diffusion assay tests, performed according to the Clinical and Laboratory Standards Institute guidelines (CLSI, 2018). The antibiotics included the following: ampicillin (AMP), 10 μg; cefotaxime (CTX), 30 μg; chloramphenicol (CHL), 30 μg; tetracycline (TET), 30 μg; streptomycin (STR), 10 μg; sulfamethoxazole/trimethoprim (SXT), 23.75/1.25 μg; trimethoprim (TMP), 30 μg; nalidixic acid (NAL), 30 μg; and ciprofloxacin (CIP), 5 μg. Escherichia coli ATCC 25922 was included as control. For purposes of the current analysis, both intermediate and resistant isolates were considered resistant.
Detection of Integrons and Shigella Resistance Locus Pathogenicity Island
The presence of the MGE associated with antibiotic resistance was detected by conventional PCR. DNA templates were obtained from bacterial lysates of individual colonies or genomic DNA preparation (E.Z.N.A. Bacterial DNA kit, Omega, Bio-Tek, Atlanta, United States). Specific primers for class 1 and class 2 integrases were designed (Supplementary Table 1). Two microliters of the sample were added to 20 μl of the reaction mix containing 3 mM MgCl2, 400 μM (each) dNTPs (Invitrogen, United States), and primers (25 nM) together with 1 U of Paq polymerase (Agilent Technologies, United States). PCR reactions were performed as follows: an initial 2-min denaturation cycle at 95°C, followed by 30 cycles at 95°C for 1 min, 56°C for 30 s, and 72°C for 30 s, with a final extension at 72°C for 10 min. The PCR products were analyzed by electrophoresis in 1% agarose gels and stained with ethidium bromide.
Primers for the detection of SRL PAI were designed using the sequences of the flanking regions of the serX gene and the SRL island of S. flexneri YSH6000 (GenBank accession number: AF326777.3; Luck et al., 2001). The presence of SRL PAI was defined by three PCR reactions; the presence of SRL PAI (SRL-positive) was defined by the following pattern: SRL I (−), SRL II (+), and SRL III (+). When the PCR pattern was SRL I (+), SRL II (−), and SRL III (−), strains were classified as SRL-negative. Any other amplification pattern was considered also as SRL-negative. The PCR reactions were performed with an initial 2-min denaturation cycle at 95°C, followed by 30 cycles at 95°C for 1 min, 50°C for 30 s, and 72°C for 1 min, with a final extension at 72°C for 10 min. The PCR products were analyzed by electrophoresis in 1.5% agarose gels and stained with ethidium bromide.
Detection of Antimicrobial Resistance Genetic Markers
DNA templates were obtained as mentioned before. Two microliters of the sample was added to 20 μl of the reaction mix containing 3 mM MgCl2, 400 μM (each) dNTPs (Invitrogen, United States), and primers together with 1 U of Paq polymerase (Agilent Technologies, United States). Specific primers for trimethoprim resistance genes (dfrA1, dfrA8, and dfrA14), blaOXA–1 for ampicillin, cat for chloramphenicol, and qacEΔ1 for quaternary ammonium salts resistance were previously described (Supplementary Table 1; Di Conza et al., 2002; Toro et al., 2005; Miranda et al., 2016). The PCR reactions were performed as follows: an initial 2-min denaturation cycle at 95°C, followed by 30 cycles at 95°C for 1 min, 56°C for 30 s, and 72°C for 1 min, with a final extension at 72°C for 10 min. The PCR products were analyzed by agarose gel electrophoresis and stained with ethidium bromide.
Pulsed-Field Gel Electrophoresis
According to PulseNet protocol (CDC)1, bacterial suspensions of 276 S. sonnei strains were embedded in agarose plugs, lysed, and then digested with endonuclease XbaI (Thermo Fischer Scientific, United States) at 37°C for 2 h. The macrorestriction of genomic DNA fragments was separated by pulsed-field gel electrophoresis on a CHEF-DRIII Chiller system (Bio-Rad Laboratories, Richmond, CA, United States) in 1% agarose gel using 0.5X TBE buffer at 6 V/cm and 14°C, with ramped pulse times of 2.2–54.2 s for 21 h. Salmonella enterica serovar Braenderup strain H9812 was included as molecular size standard three times on each gel to normalize the images and to compare the fingerprints among several gels. The DNA band profiles were analyzed with GelCompar software (version 3.0; Applied Maths, Sint-Martens-Latem, Belgium). A similarity dendrogram was constructed using the unweighted-pair group method with arithmetic mean (UPGMA) with the Dice similarity coefficient and a band tolerance of 1.5%. Pulsegroups were defined by sharing 73% similarity and more than 93.5% for pulsetypes.
Genome Sequencing and Data Processing
Illumina sequencing was performed on 29 selected Chilean S. sonnei strains by Microbes NG (Birmingham, United Kingdom) using the Nextera XT library protocol. Sequencing was done on the Illumina MiSeq platform (Illumina, San Diego, CA, United States). Reads were processed with Trimmomatic 0.30 to remove adapters and for quality trimming. For de novo genome assembly, reads were processed using Shovill2 with Spades 3.14.0 (Nurk et al., 2013)3, with a Kmer range from 31 to 127 (31, 55, 79, 103, and 127). For SNP identification, the trimmed reads were mapped to the reference genome of S. sonnei SS046 using Snippy with default parameters4. The core SNPs were identified from the alignment, and a final phylogenetic tree was generated using FastTree (Price et al., 2010). Resfinder was used to predict the presence of known acquired resistance genes and chromosomal mutations associated with resistance to quinolones (Camacho et al., 2009; Bortolaia et al., 2020; Zankari et al., 2020)5. MLST typing using sequences was done by https://cge.cbs.dtu.dk/services/MLST/ (Larsen et al., 2012). All the assembled genomes from this study are available on NCBI under the BioProject accession PRJNA602693.
Comparative Genomic Analysis
Draft genome sequences were managed using the Geneious software (v11.0.5; Biomatters, Ltd., New Zealand). The assembled contig sequence was mapped against the reference sequence of the SRL PAI carried by S. flexneri 2a str YSH6000 using BlastN in Geneious. Comparisons of the genetic structure of SRL PAIs were performed using EasyFig v2.1 (Sullivan et al., 2011).
Statistical Analysis
Evaluation of pairwise association between the timeframe of isolation of the strains and the specific genes that they carry was performed using contingency tables by odds ratios. The statistical significance of these associations was determined by Pearson’s chi-square test or Fisher’s exact test (when frequencies were less than 5) (R Core Team, 2014; Csardi, 2015; Wei et al., 2017). When any of the cell values of the contingency table was zero, Haldane correction (Haldane, 1940) was used by adding 0.5 to all cells.
Results
Antibiotic Resistance Phenotypes of Chilean S. sonnei Strains
To characterize the molecular and phenotypic resistance profile of Chilean S. sonnei strains, we analyzed 349 available strains isolated between the years 1995 and 2013. To better understand the temporal dynamics of antibiotic resistance of these strains, they were grouped according to the date of isolation in periods A–E. This collection included a total of 80 strains isolated in 2008–2009, which corresponds to period C, when an increase of S. sonnei frequency was registered (Figure 1A). The geographical origin of the strains is shown in Figures 1B,C.
Antimicrobial susceptibility test (AST) for all these 349 strains showed that 100% were sensitive to ciprofloxacin, while 3 (0.9%) and 33 (9.5%) isolates were resistant to cefotaxime and nalidixic acid, respectively. In contrast, these strains displayed high rates of antibiotic resistance to streptomycin (305, 87.4%), trimethoprim (264, 75.6%), ampicillin (257, 73.6%), tetracycline (257, 73.6%), sulfamethoxazole/trimethoprim (256, 73.3%), and chloramphenicol (195, 55.9%). The resistant phenotype to the last five antibiotics in period C is particularly noteworthy (Table 1).
Isolates resistant to at least one antibiotic in three or more drug classes were considered MDR6. Consequently, 300 out of 349 strains (86%) presented an MDR phenotype. Furthermore, the most frequent MDR phenotype was AMP-CHL-TET-STR-SXT-TMP, displayed by 123 strains (35.2%), followed by AMP-CHL-TET-STR (36, 10.3%), AMP-STR-SXT-TMP (32, 9.2%), TET-STR-SXT-TMP (25, 7.2%), and AMP-CHL-TET-SXT-TMP (20, 6.7%) (Supplementary Table 2).
Figure 2 shows the temporal distribution of the resistance phenotypes by antibiotic (concentric circles) and MDR phenotypes simultaneously. It should be noted that all the strains of period C displayed resistance to six antibiotics (AMP-CHL-TET-STR-SXT-TMP). As mentioned above, this was the most frequent MDR profile in the present study. Interestingly, this MDR phenotype was observed with a lower frequency not only before period C (5/349, 1.4%) but also after this (38/349, 10.9%). On the contrary, in the other periods, heterogeneous MDR phenotypes were observed. Analyzing each period, the most frequent MDR phenotypes in period A were AMP-CHL-TET-STR (20/60, 33%) and AMP-STR-SXT-TMP (19/60, 32%). In period B, AMP-STR-SXT-TMP (12/50, 24%) and AMP-TET-STR-SXT-TMP (10/50, 20%) were detected. Meanwhile, these were AMP-CHL-TET-STR-SXT-TMP (19/80, 24%) and AMP-CHL-TET-SXT-TMP (15/80, 19%) in period D and TET-STR-SXT-TMP-NAL (25/79, 32%) and AMP-CHL-TET-STR-SXT-TMP (19/79, 24%) in period E. Notably, 32 out of 33 NALR strains were isolated in period E, and 25 of them displayed the TET-STR-SXT-TMP-NAL MDR phenotype, suggesting the emergence of a new clone of S. sonnei at that time.
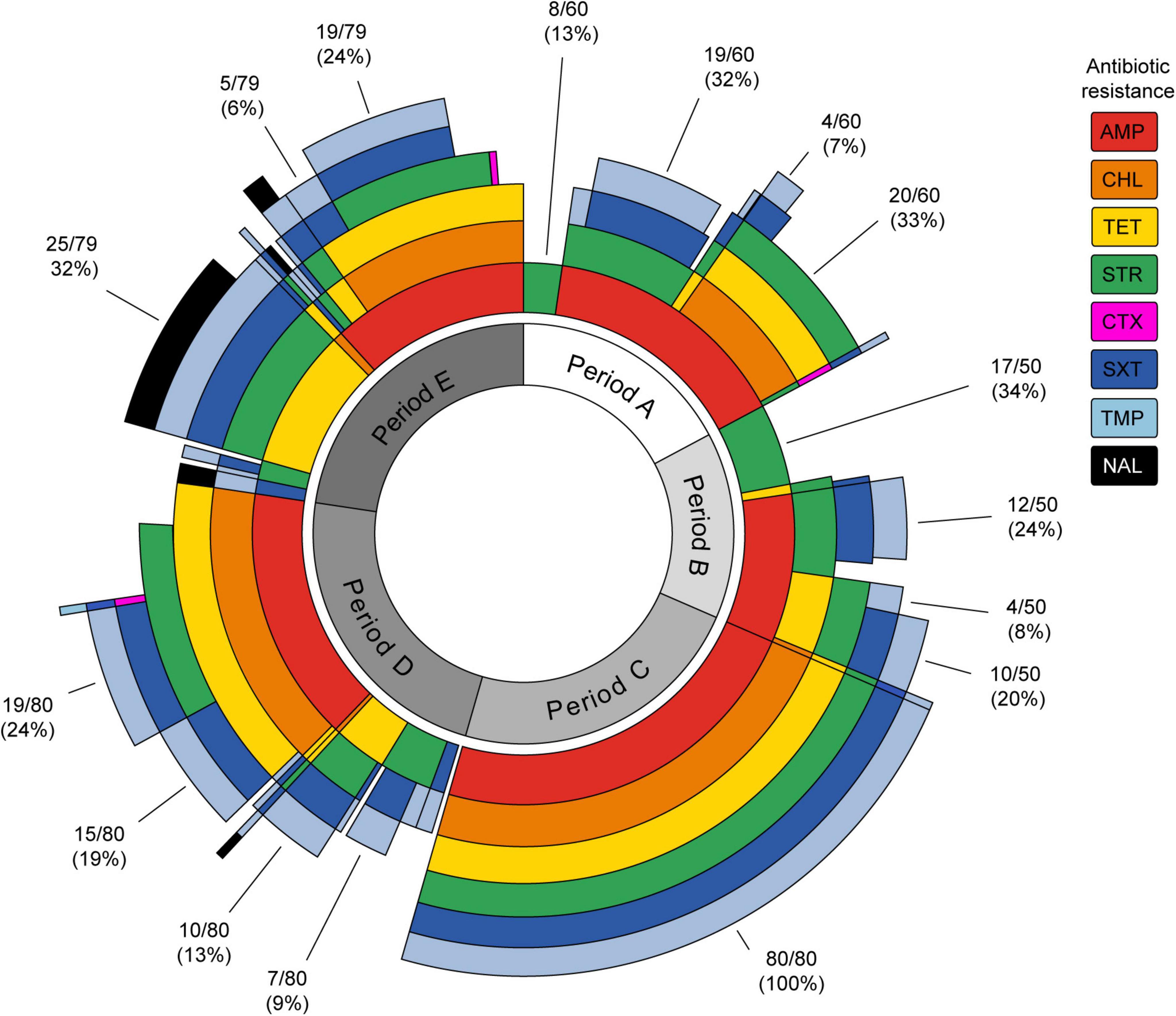
Figure 2. Sunburst plot showing the antibiotic resistance and multi-drug resistance phenotypes of Shigella sonnei strains isolated in different time periods. Each antibiotic is represented by a concentric circle of a different color. The sum of the concentric circle represents the resistance phenotype pattern for each strain. The percentages of most frequent profiles for each period are shown. AMP, ampicillin; CHL, chloramphenicol; TET, tetracycline; STR, streptomycin; CTX, cefotaxime; SXT, sulfamethoxazole/trimethoprim; TMP, trimethoprim; NAL, nalidixic acid. The figure was prepared using the sunburstR (Bostock et al., 2019) package in R (R Core Team, 2014).
Distribution of Genetic Elements Associated to Multidrug-Resistant in Chilean S. sonnei Strains
The simultaneously resistant phenotype to ampicillin, streptomycin, chloramphenicol, and tetracycline in some S. sonnei strains suggested the presence of the SRL PAI. To determine whether these strains harbor this genetic element, three PCR assays were designed. A combination of SRL I, SRL II, and SRL III PCR amplification patterns allowed us to detect the insertion of SRL PAI within the serX gene. The SRL I (−), SRL II (+), and SRL III (+) pattern was expected for SRL-positive strains. Conversely, the SRL I (+), SRL II (−), and SRL III (−) pattern was expected for no insertion at serX, indicating the absence of SRL PAI.
Of the 349 strains tested, 192 (55%) were classified as SRL-positive and 157 (45%) as SRL-negative. The 192 SRL-positive strains were distributed as follows: 24/60 (40%) from period A, 2/50 (4%) from period B, 80/80 (100%) from period C, 52/80 (65%) from period D, and 34/79 (43%) from period E (Figure 3A). The detailed SRL PAI distribution in the strains is described in Supplementary Table 2. The group of SRL-positive strains includes five strains that should have been classified as SRL-negative due to the PCR pattern. Nonetheless, because their antibiotic profile corresponded to the SRL-positive phenotype, we further evaluated the presence of pathogenicity island integrase and orf58 genes as well as antibiotic resistance determinant genes in these strains (Supplementary Table 1). The results allowed us to confirm the presence of SRL PAI probably at another insertion site (SRL + out) (data not shown). Any other PCR pattern was considered negative for the presence of SRL, which was obtained for 14 strains (Supplementary Table 2).
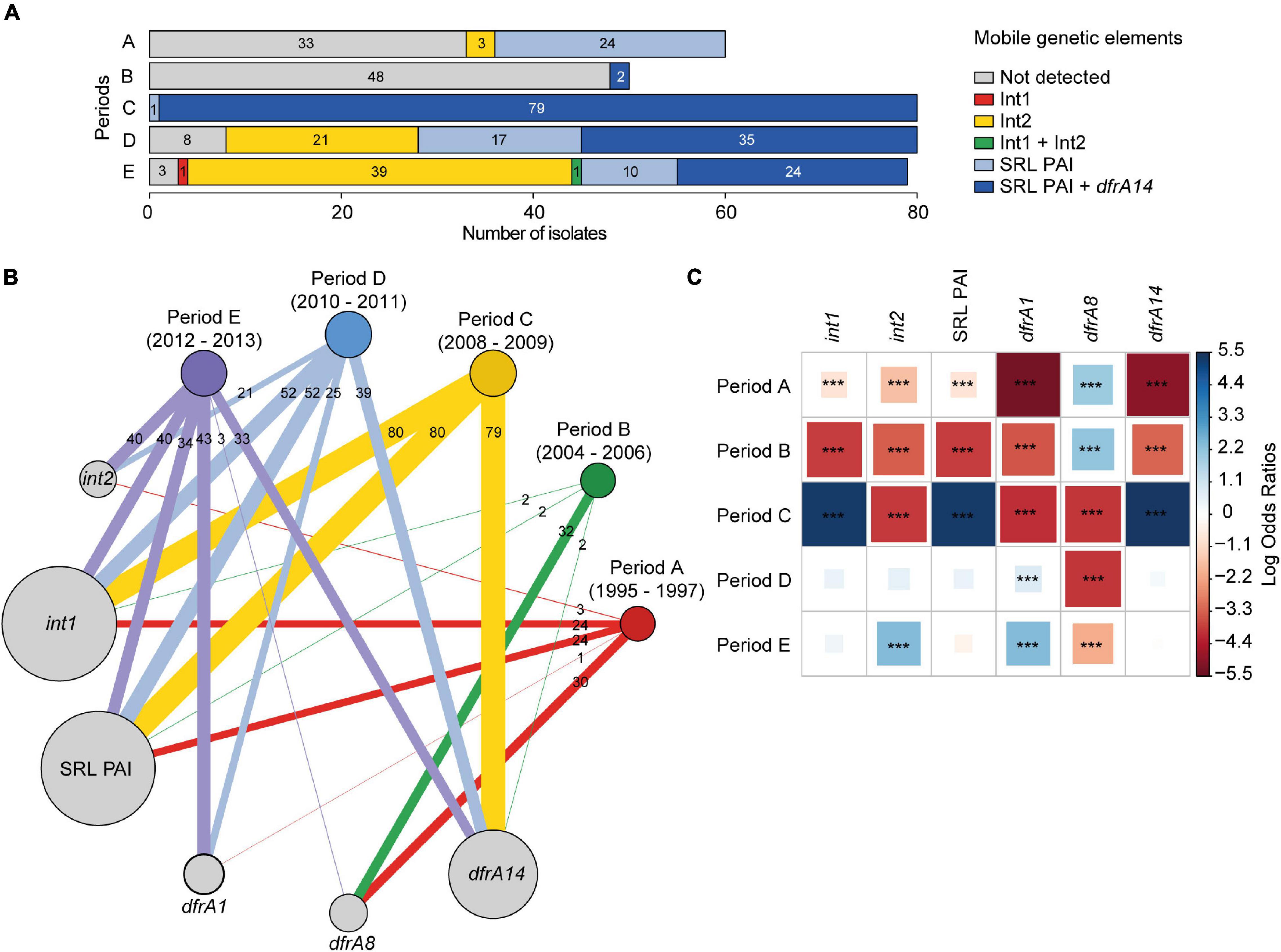
Figure 3. Patterns of association between the Shigella sonnei isolation date (periods A–E) and genetic elements related to antimicrobial resistance. (A) Distribution of class 1, class 2 integrons, SRL PAI, and dfrA14 gene. (B) Graph of modules showing the interactions between periods of isolation and specific genetic determinants. Modules are weighted by the number of strains. Besides this, links are weighted by both the number of strains linked between modules and the number of strains within modules. The figure was prepared using the igraph (Csardi, 2015) package in R (R Core Team, 2014). (C) Pairwise association plot for periods of isolation and specific genetic elements. Red and blue squares represent negative and positive associations, respectively. The color scale represents the magnitude of the association determined by odds ratios. ***p < 0.001 determined by Pearson’s chi-square test or Fisher’s exact test. The figure was prepared using the package corrplot (Wei et al., 2017) in R (R Core Team, 2014).
In addition, class 1 and class 2 integrons were searched by PCR, using specific primers for integrases (Supplementary Table 1). Class 1 integron was detected in 194/349 (55.6%) strains, including 100% of the SRL-positive strains (Figure 3B). This result is consistent with the presence of an atypical class 1 integron within SRL PAI, which harbors the blaOXA–1-aadA1-IS1 gene organization. Indeed, we assessed and detected the adjacent blaOXA–1 and aadA1 genes by PCR for at least 70 SRL-positive strains (data not shown).
Of the 157 SRL-negative strains, only one SRL-negative strain from period E harbored a typical class 1 integron (0.3%) (Figure 3A), defined by the dfrA1-aadA1-qacEΔ1-sul1 gene cluster and further confirmed by PCR and whole-genome sequencing (WGS) of the strain.
On the other hand, class 2 integron was detected in 64/349 (18.3%) strains; all of them were SRL-negative isolates. They belong to the following periods: 3 to period A, 21 to period D, and 40 to period E, with one of them harboring class 1 and class 2 integrons simultaneously (Figure 3A). It should be noted that class 2 integron was neither detected among strains from period B nor C (Figure 3B). In addition, 92 isolates (26.4%) were negative for MGE, SRL PAI, and integrons. Of this group, 53 isolates (15.2%) were MDR.
It is interesting to remark that 144 out of 192 (75%) SRL-positive strains displayed an MDR phenotype to AMP-CHL-TET-STR and also to SXT and TMP. Therefore, we searched for the presence of previously detected dfrA1, dfrA8, and dfrA14 genes, which confer resistance to TMP (TMPR) (Miranda et al., 2016). Overall, dfrA1, dfrA8, and dfrA14 genes were detected in 69 (19.7%), 65 (18.6%), and 153 (43.8%) strains, respectively (Figure 3B). Focusing on the 264 TMPR strains, we detected dfrA1 in 54 strains (20.5%), dfrA8 in 51 (19.3%) strains, and dfrA14 in 142 (53.8%) strains as sole TMPR genetic marker. The combinations dfrA1-dfrA8, dfrA1-dfrA14, and dfrA8-dfrA14 were scarcely present in 1.1% (3), 3.8% (10), and 0.4% (1), respectively. Three TMPR strains did not carry any of these TMP gene markers, while two TMP-sensitive strains harbored dfrA1 cassette, and 10 carried dfrA8 (Supplementary Table 2).
Notably, 140 out of 144 (97.2%) SRL-positive TMPR strains harbored the dfrA14 gene, distributed as follows: 2 strains in period B, 79 in C, 35 in D, and 24 in E. From the four remaining strains, two of them harbored dfrA8, and two lacked the tested TMPR genes. On the other hand, there were 120 SRL-negative TMPR strains that harbored a unique TMPR gene, distributed as follows: 54 with dfrA1, 49 with dfrA8, and 4 with dfrA14. The presence of dfrA1-dfrA8 or dfrA1-dfrA14 pairs was detected in 3 and 9 strains, respectively, and one strain was negative for these markers. In addition, the presence of dfrA1 gene was detected among 62 out of 64 class 2 integron-positive strains.
All these associations are illustrated in Figure 3B, where it can be seen that the distribution of these genetic determinants depends on the isolation periods. In this context, strains from periods A and B were significantly associated with the presence of the dfrA8 gene; the presence of the SRL PAI, the atypical class 1 integron, and the dfrA14 gene was associated with strains from period C, while the presence of the class 2 integron and the dfrA1 gene was associated with strains from period E (Figure 3C).
In summary, we detected 300 (86%) MDR strains out of 349. They were distributed in 247 (70.8%) containing one or more of the MGE evaluated in this work. In contrast, only 53 (15.2%) were negative for SRL PAI and class 1 and 2 integrons.
Pulsed-Field Gel Electrophoresis Analysis of Chilean S. sonnei Strains
To assess the clonality of the strains in this study, we performed a pulsed-field gel electrophoresis analysis of 276 S. sonnei isolates using XbaI digestion. The samples covering all the periods were randomly selected, including 56 from period A, 36 from period B, 71 from period C, 48 from period D, and 65 from period E. The obtained tree was split into two major pulsegroups with 69.1% of similarity (Figure 4). Pulsegroup A comprises 62 strains with similarities greater than 73.7% and whose common feature was the absence of SRL PAI. In addition, 55 isolates out of 62 belonged to periods A and B, i.e., strains isolated between 1995 and 2004 (88.7%), and 42 of them (67.7%) carried the genetic marker dfrA8.
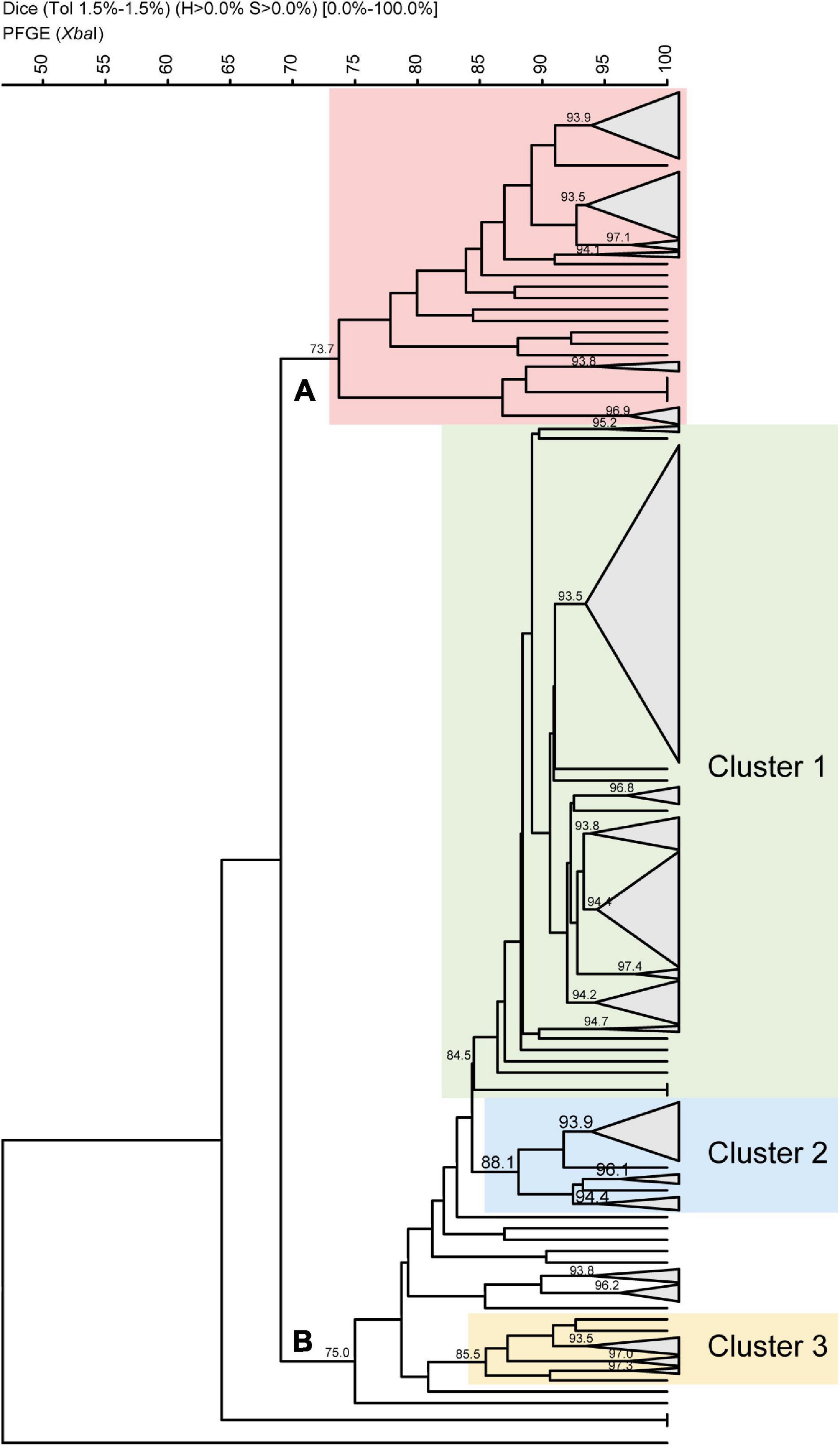
Figure 4. Dendrogram based on Dice coefficients of similarity for 276 Chilean Shigella sonnei strains by pulsed-field gel electrophoresis patterns. The dendrogram was constructed by UPGMA (GelCompar II). Shown are two pulsegroups (A,B) with a similarity of 69.1%. Twenty-two pulsetypes were defined with a similarity of >93.5%. Within pulsegroup A, all the strains belong to periods A and B, and most of them harbored dfrA8 as TMP resistance marker. Pulsegroup B comprises 214 strains divided in three clusters. Within cluster 1, there are 144 SRL and SRL/dfrA14 strains. Cluster 2 contains 25 strains, of which 21 are NAL-resistant strains harboring class 2 integron. Cluster 3 is composed of 13 strains, of which 11 carry class 2 integron, but they are NAL-sensitive strains.
Pulsegroup B includes 214 strains sharing 75% similarity, and it represents a more heterogeneous group with 3 subgroups or clusters (Figure 4). The largest subgroup, named cluster 1, includes 158 strains sharing 84.5% of similarity. Here 144 out of the 163 tested SRL-positive strains were grouped, with 108 of them carrying SRL/dfrA14 and 36 just SRL. Cluster 1, the largest pulsetype defined at 93.5% similarity, included 84 strains: 70 isolates carried SRL/dfrA14, 10 strains harbored only SRL PAI, and 4 strains were without the island, regardless of the collection period. A subgroup composed of 25 strains (cluster 2) includes 23 isolates harboring the class 2 integron, 21 of which are simultaneously resistant to nalidixic acid. Finally, there is a subgroup composed of 13 strains (cluster 3), from period E, 11 of which have a class 2 integron. Hence, both clusters B2 and B3 include 75.6% of class 2 integron-positive strains and 84% of nalidixic acid-resistant strains of this analysis.
Whole-Genome Sequence Analysis From Chilean S. sonnei Strains
A total of 29 isolates representing different patterns of resistant phenotypes were selected for sequencing. Strains from all periods, positive and negative for SRL PAI and class 1 or 2 integrons, were included. Genetic determinants of resistance were obtained by Resfinder (Bortolaia et al., 2020) and were concordant with phenotypical AST and PCR results (Table 2). Seventeen out of 29 sequenced strains harbored SRL PAI: 11 were SRL/dfrA14-positive, and the remaining 6 strains carried only SRL PAI. The presence of the blaOXA–1, aadA1, cat, and tetB genes in those strains was confirmed. In those SRL/dfrA14-positive strains, this gene was harbored in the pABC-3 plasmid. In all cases, disruption of the strA gene by the insertion of dfrA14, included in the sul2/strA::dfrA14/strB genes cluster, was identified. In addition, the dfrA15 gene was detected as another determinant of TMPR through sequencing of one of the three TMPR strains negative for the other alleles (Table 2).
The lineage of the strains was determined according to the location of the genome in the full-core SNP phylogeny, comparing 470 publicly available S. sonnei sequences (Figure 5). This analysis allowed categorizing 20 out of 29 Chilean S. sonnei strains as sublineage IIIa. In fact, all SRL-positive strains sequenced in this study were sublineage IIIa. The seven sequenced strains harboring the class 2 integron were more heterogeneous, being part of sublineages IIa, IIIa, and IIIb and global. Three class 2 integron-positive strains were classified in sublineage IIIb, sharing this classification with most of the Argentinian strains. Two NALR-sequenced strains, displaying a D87Y mutation in gyrA and class 2 integron, belonged to the global lineage (Table 2 and Figure 5).
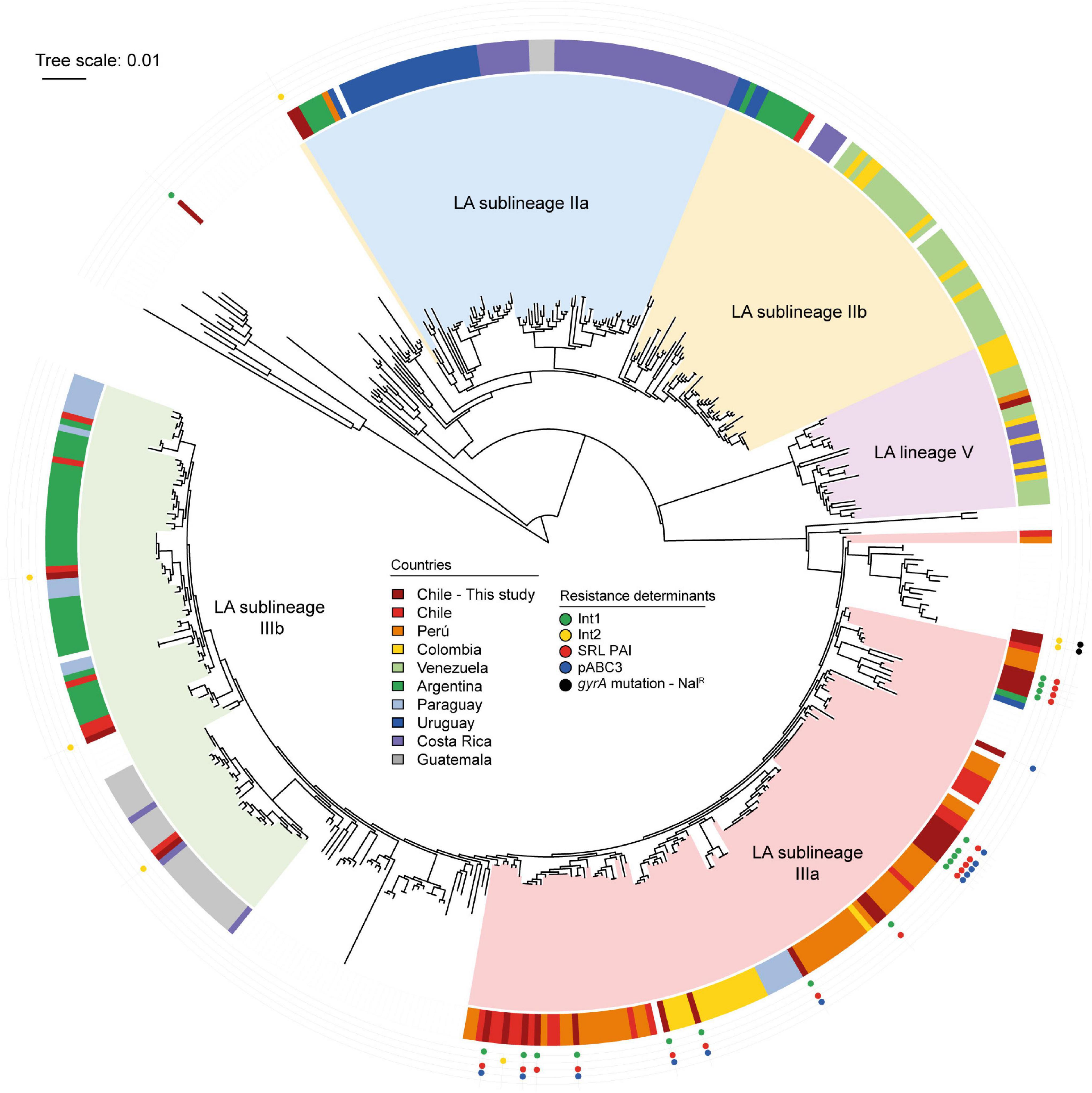
Figure 5. Phylogenetic analysis of Shigella sonnei genomes sequenced in this study and publicly available S. sonnei genomes. Maximum likelihood phylogenetic tree (midpoint rooted) based on core genome SNPs showing phylogenetic relationships among 470 S. sonnei strains. The Latin-American (LA) lineages are based in the study by Baker et al. (2017). The country of origin of LA strains is shown in the colored ring, according to the legend. The global reference strains in the tree do not have country labels. The presence of antimicrobial resistance determinants of Chilean S. sonnei strains sequenced in this study is shown in the outer tracks, according to the legend. The tree was edited using iTOL (Letunic and Bork, 2016).
Using the MLST 2.0 tool, we found that all but one of the sequenced strains had sequence type ST-152 (Table 2). The exception was a strain isolated in period E, whose sequence type was ST-1504. This strain belonged to the global sublineage and was confirmed to be the only one harboring the typical class 1 integron mentioned above.
Genomic Organization of Shigella Resistance Locus Pathogenicity Island in Shigella
Since the SRL PAI was widely distributed among Chilean S. sonnei strains isolated over a period of 18 years, we investigated the genetic conservation of this PAI. For this, we performed alignments between the prototypical SRL PAI harbored by the S. flexneri 2a str. YSH6000 (SRLYSH6000) and five draft genomes of S. sonnei representative strains of each period (see section “Materials and Methods”). As a result, we found several contigs containing DNA regions with >90% identity with SRLYSH6000. In silico analyses allowed us to map these homologous sequences against the genetic structure of SRLYSH6000, assembling the complete genetic structure of this PAI in those five analyzed genomes (Figure 6). The SRL PAIs harbored by the S. sonnei strains comprise approximately 75 kb. As expected, in all five draft genomes, there is a high degree of conservation compared with the one in the S. flexneri YSH6000 strain. Among the genes identified in these PAIs, we found the antibiotic resistance genes (blaOXA–1, aadA1, cat, and tet), a ferric dicitrate transport system, and the recently described orfs 8 and 9 that participate in the metabolism of D-aspartate (Henríquez et al., 2020). However, a significant difference observed in the SRL PAI identified in the S. sonnei strains corresponds to a deletion of a gene that encodes an autotransporter protein of the Ag43 allele family. One possible explanation is that this deletion originates from the insertion of a DNA fragment of about 10 kb, containing genes encoding for integrases and hypothetical proteins.
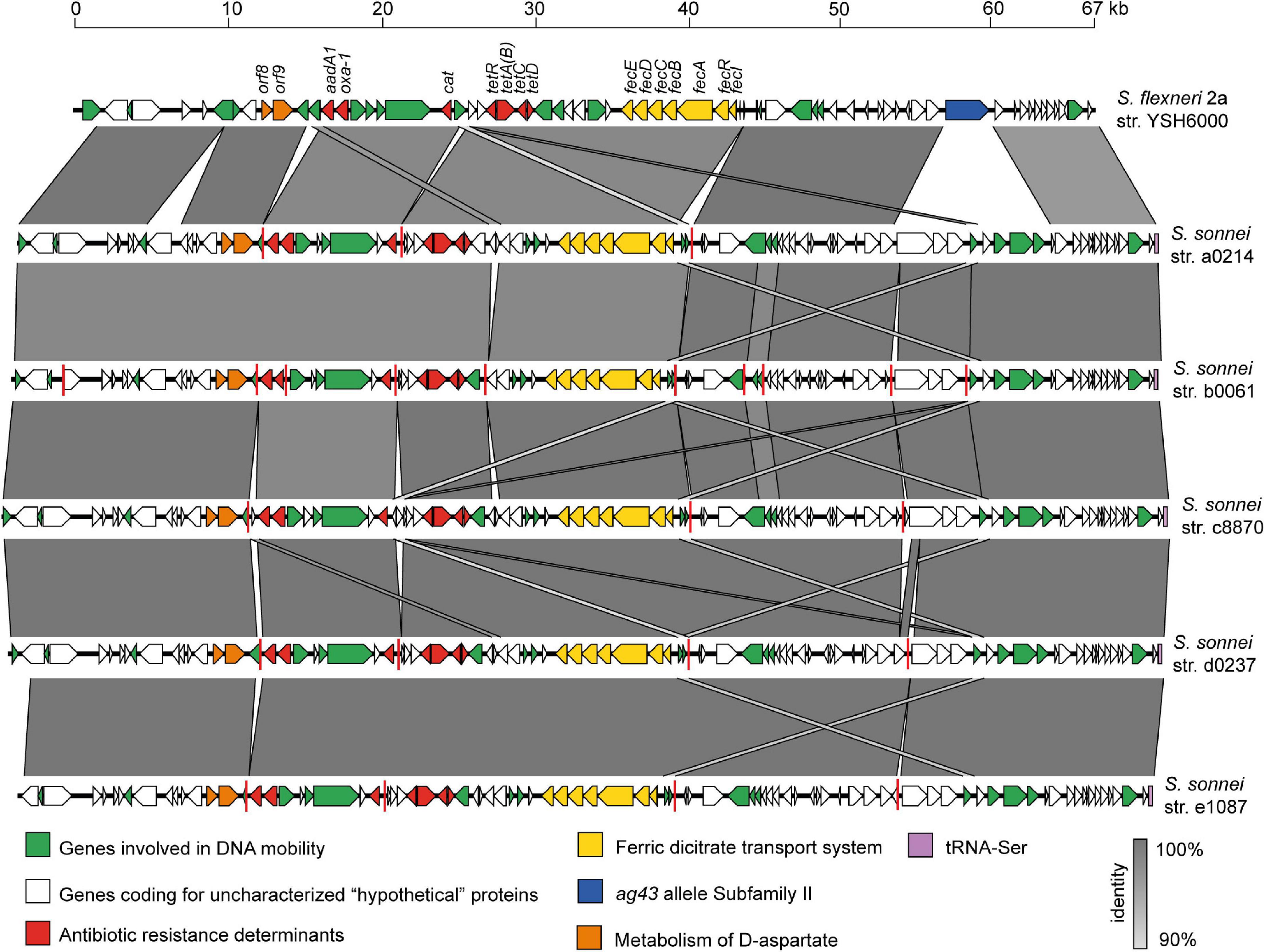
Figure 6. Comparison of the genetic structure of SRL pathogenicity islands carried by the Shigella flexneri 2a YSH6000 strain and Chilean S. sonnei strains. The predicted genes and the direction of transcription are represented as block arrows. The genes are color-coded according to gene function, as indicated in the legends at the bottom. The names of some genes are indicated. The conserved regions are shaded in gray, and the intensity of the color indicates the nucleotide identity levels, from 88 to 100%. Contig boundaries are shown as red lines. The GenBank accession numbers are indicated in Table 2. The figure was prepared using EasyFig (Sullivan et al., 2011).
Discussion
According to data from ISP, the epidemiology of shigellosis in Chile registered around 1,000 Shigella isolates per year between 1995 and 2008, from which 47% corresponded to S. flexneri and 47% were S. sonnei. In contrast, in 2009, there was an increase of up to 3,000 isolates, when a S. sonnei peak was observed rising up to about 90%. Furthermore, a sharp decline to less than 500 isolates during the years 2010–2018 was registered. Nevertheless, S. sonnei and S. flexneri frequency remained near to 50% (ISP, Boletín Vigilancia de Laboratorio, Shigella 2014–2018; Figure 1A).
The ISP reports also showed the antibiotic resistance profiles. However, the genetic determinants of resistance in our country have barely been studied. In this context, we described SRL PAI as one of the MGE present in Chilean S. sonnei strains. This genetic element was first described in S. flexneri 2a YH6000 (Luck et al., 2001). The 66-kb SRL PAI includes an SRL locus encoding resistance determinants to streptomycin (aadA1), ampicillin (blaOXA–1), chloramphenicol (cat), and tetracycline (tetB). The SRL PAI is likely acquired due to horizontal gene transfer underscoring the role of this mechanism to disseminate Shigella MDR phenotype (Turner et al., 2003, 2004). It has also been described in S. dysenterieae serotype 1 and S. flexneri serotypes 3a and 3b (Baker et al., 2015; Njamkepo et al., 2016; Parajuli et al., 2019). On the other hand, we described the presence of the dfrA14 gene in 79 out of 80 strains isolated in 2008–2009, differentiating from strains isolated before that period. This marker is coded in the pABC-3 plasmid in the sul2/strA::dfrA14/strB gene cluster (Miranda et al., 2016).
Based on the results of this study, we considered CHL resistance in MDR strains as a predictive phenotypic marker of the presence of SRL PAI, which pointed us to look at this marker more closely. The 2000–2010 report from Foodnet at CDC highlighted a high level of MDR S. sonnei strains in United States (Shiferaw et al., 2012). It is noteworthy that, from the 954 S. sonnei strains analyzed in that period, only 10 isolates (1%) included the CHL resistance phenotype, suggesting a low frequency of SRL-positive strains.
The S. sonnei strains circulating in Europe have diverse MDR patterns. Surprisingly, in the 18 years of surveillance in Belgium (Vrints et al., 2009), non-CHL resistance was detected in almost 5,000 isolates, suggesting the absence of SRL-positive strains. On the other hand, a study conducted in England and Wales characterized 341 S. sonnei isolated during 2015 by WGS (Sadouki et al., 2017). From that collection, only 8 strains (2.3%) appeared to carry both elements, the SRL PAI and the pABC-3 plasmid, based on the presence of sequence-predicted resistance genes. These strains could have been imported by travelers (Kotloff et al., 2018). Besides these, studies conducted by Baker et al. (2018) also demonstrated the presence of SRL and plasmid pABC-3 not only in S. sonnei but also in S. flexneri 2a strains.
In China, it is challenging to realize the presence of the SRL PAI in S. sonnei strains since CHL is not included in the AST. However, in a 10-year surveillance in Jiangsu (2002–2011), atypical class 1 integron was detected in 168 of 340 S. sonnei isolates (49.4%) harboring blaOXA–1-aadA1 gene cassettes (Gu et al., 2017; Kang et al., 2019). As mentioned, this integron is part of the SRL locus, suggesting the presence of SRL PAI in strains isolated from this region. Indeed Zhang et al. (2014) described the presence of SRL in an epidemic clone of S. flexneri MDR strain, carrying genes for resistance against streptomycin, ampicillin, chloramphenicol, and tetracycline.
A completely different picture was observed with the Chilean S. sonnei strains. Since 1998, a high resistance to CHL has been reported (Fullá et al., 2005; Toro et al., 2005; Marcoleta et al., 2013). The present study detected 55.9% (195/349) of CHL-resistant strains among isolates from the 1995–2013 period, 191 of which possess the SRL PAI, thus correlating it as the most critical genetic element associated with CHL resistance.
The landscape of S. sonnei in Latin America was recently described by Baker et al. (2017). From WGS studies, it is possible to infer the circulation of SRL PAI in this region. Based on supplementary data analysis, we confirmed that 79 out of the 323 strains characterized by the PulseNet Latin American group at least harbored the SRL locus. The distribution of predicted SRL-positive strains was 46/48 Peruvian strains, 16/31 Colombian strains, and 17/27 Chilean S. sonnei isolates. All these strains belonged to sublineage IIIa. The sequence analysis of Peruvian S. sonnei strains showed that 40 out of the 48 strains harbor the SRL locus and the dfrA14 gene probably as part of pABC-3 plasmid (Miranda et al., 2016), while 6 out of the 48 seem to carry only the SRL locus. In that dataset, the oldest Peruvian isolates were obtained in 1999, indicating the simultaneous presence of SRL PAI and the pABC-3 plasmid in those strains. In Colombia, 2 and 14 strains were predicted to harbor SRL PAI and SRL/pABC-3 genetic elements, respectively. For the Chilean strains analyzed in that work, the authors had access to strains isolated in the period 2010–2011 from different areas of the country, and the presence of SRL and SRL/pABC-3 was predicted in 3 and 14 of them, respectively (Baker et al., 2017). It is very likely that more than one of these strains coincide with those included here in period D, where we described 21% SRL-positive strains and 43.8% of SRL/pABC-3 strains. Our results show the close phylogenetic relationship in sublineage IIIa of all the Chilean strains harboring the SRL PAI to be nearly related with the Peruvian and Colombian isolates, suggesting the interchange of strains among these countries (Figure 5).
The results of the current study show that SRL-positive strains have been circulating in Chile from 1995 onward, and dfrA14 was not present until 2006 when a SRL/pABC-3-positive strain was isolated for the first time. After that, all the strains included in period C (2008–2009 period) harbored SRL/pABC-3 genetic elements. It should be noted that this group is formed by 61 strains from different areas of Región Metropolitana, and 15 were isolated in Antofagasta, showing the dissemination to a much wider geographical area of the country. It is not known why these strains spread massively in our country in those years. Even the diminished prevalence of Shigella after 2009 and the co-circulation of different clones later have not been resolved (Figure 1A).
Interestingly, the S. sonnei strains circulating in Brazil appear to be different clones. A study of 72 Brazilian strains isolated over 31 years from 1983 to 2014 suggests two prevalent subtypes that differ little; however, neither of them displays the MDR associated with the presence of SRL PAI (Seribelli et al., 2016). Although a more recent study showed that most S. sonnei strains isolated in northeastern Brazil were associated with azithromycin resistance, it seems that the SRL element is circulating as 20% of them were resistant to CHL (Medeiros et al., 2018).
Surveillance for MDR S. sonnei infections acquired from domestic and international sources allowed the isolation of two S. sonnei strains in Pennsylvania from patients infected during a international travel to Peru (Abelman et al., 2019), showing the spread of SRL-positive strains to North America. The predicted antimicrobial gene resistance markers harbored by those strains strongly suggest the presence of the same Latin American clone, including SRL PAI (blaOXA–1, aadA1, tetB, and cat1) and the plasmid pABC-3 (strA, strB, sul2, and dfrA14). The key marker is again CHL resistance; only these strains had the appropriate phenotype and the genetic markers for the presence of SRL PAI.
The increase in Shigella spp. MDR strains has been a cause for concern worldwide over the past two decades. The spread of class 1 and class 2 integrons has also been documented in Latin America (Barrantes and Achí, 2016). The emergence and successful spread of a particular MDR strain of S. sonnei biotype g carrying a class 2 integron, resistant to STR, SXT, and TET, was reported in several countries, including Australia, Senegal, Taiwan, Japan, Korea, and Iran (McIver et al., 2002; Oh et al., 2003; Gassama-Sowa et al., 2006; Seol et al., 2006; Ranjbar et al., 2007; Izumiya et al., 2009; Chang et al., 2011). Among the Chilean S. sonnei strains, we could detect the presence of class 2 integron to be circulating steadily just since 2011.
The World Health Organization has recommended fluoroquinolones and cephalosporins as the preferred drugs for the treatment of Shigella infections (Williams and Berkley, 2016, 2018). However, resistance to these antibiotics has been described. During 1998–2009, the nalidixic acid resistance rates in S. sonnei isolated from Asia and Africa have increased to 44%, whereas in Europe and America they were less than 3%. Consequently, the spread of ciprofloxacin-resistant S. sonnei has been increasing globally, apparently from South Asia (Gu et al., 2012; Chung The et al., 2016, 2019, 2021). In Chile, ciprofloxacin resistance was not detected during the period 1995–2013, but the presence of 9.5% of NALR strains among these 349 isolates, concentrated in a short time between 2011 to 2013, is of great concern. Two of these Chilean NALR strains were sequenced, thus finding the D87Y mutation in gyrA. Moreover, the latest report of ISP showed the emergence of CIPR in Chilean S. sonnei strains to be at 2–3% (ISP, Boletín Vigilancia de Laboratorio, Shigella 2014–2018).
Our results regarding the emergence of NALR strains agree with reports from Latin American (Sati et al., 2019). Such surveillance provides evidence of the increasing prevalence of NALR S. sonnei in this region since 2011. This information supports the presence of class 2 integron and the MDR phenotype to TET, STR, SXT, TMP, and NAL, suggesting that the entry of NALR strains of S. sonnei is more likely due to global world trade and human travel (Chung The et al., 2016, 2021).
In summary, this work shows an overview of the resistance phenotypes associated with genetic determinants, emphasizing the MDR molecular mechanisms that have circulated in Chile during the period 1995–2013. We were able to demonstrate the presence of a clone or variant of S. sonnei SRL-dfrA14, harboring the pABC-3 plasmid, which spread during the 2008–2009 period. After that, different clones harboring class 2 integron have emerged. Therefore, our results underscore the role of the SRL PAI and integrons as genetic elements associated with the MDR phenotype. On the other hand, the high similarity of the SRL sequences from representative strains of different periods would rule out that this element has affected by itself the spread of S. sonnei isolated during period C, 2008–2009. Even considering the limitations of the current study, the data presented in this work revealed the temporal dynamics of antimicrobial resistance in S. sonnei strains circulating in Chile, mainly determined by the widespread dissemination of MGE conferring MDR phenotypes. Since shigellosis is endemic in Chile, constant surveillance of antimicrobial resistance phenotypes and their genetic basis is a priority in order to contribute to the orientation of public health policies.
Data Availability Statement
The datasets presented in this study can be found in online repositories. The names of the repository/repositories and accession number(s) can be found in the article/Supplementary Material.
Author Contributions
CT and JS contributed to study design, data analysis, data interpretation, manuscript writing, and revision of the manuscript. DM contributed to data analysis, data interpretation, manuscript writing, figures design, and revision of the manuscript. JU contributed to data analysis, data interpretation, and revision of the manuscript. JD contributed to data analysis and revision of the manuscript. LC and RC contributed to data acquisition and data analysis. TH and CG contributed to data acquisition, data analysis, and data interpretation. GH and MU contributed to study design, data analysis, data interpretation, and revision of the manuscript. CT was the principal investigator at the FONDECYT grant that funded this work. All authors contributed to the article and approved the submitted version.
Funding
This work was supported by the Fondo Nacional de Desarrollo Científico y Tecnológico, FONDECYT 1130394.
Conflict of Interest
The authors declare that the research was conducted in the absence of any commercial or financial relationships that could be construed as a potential conflict of interest.
Publisher’s Note
All claims expressed in this article are solely those of the authors and do not necessarily represent those of their affiliated organizations, or those of the publisher, the editors and the reviewers. Any product that may be evaluated in this article, or claim that may be made by its manufacturer, is not guaranteed or endorsed by the publisher.
Acknowledgments
We thank Juan Silva, from Universidad de Antofagasta, for providing some of the strains that belonged to the 2008–2009 outbreaks. We also thank Instituto de Salud Pública de Chile, ISP Chile, for giving S. sonnei strains isolated during the 2010–2013 period. We are grateful to “LÍNEAS DE APOYO A LA INVESTIGACIÓN FINANCIADAS POR EL ICBM (2021).”
Supplementary Material
The Supplementary Material for this article can be found online at: https://www.frontiersin.org/articles/10.3389/fmicb.2021.794470/full#supplementary-material
Footnotes
- ^ https://www.cdc.gov/pulsenet/pathogens/protocols.html
- ^ https://github.com/tseemann/shovill
- ^ https://link.springer.com/chapter/10.1007%2F978-3-642-37195-0_13
- ^ https://github.com/tseemann/snippy
- ^ https://cge.cbs.dtu.dk/services/ResFinder/
- ^ https://www.cdc.gov/narms/resources/glossary.html
References
Abelman, R. L., M’ikanatha, N. M., Figler, H. M., and Dudley, E. G. (2019). Use of whole genome sequencing in surveillance for antimicrobial-resistant Shigella sonnei infections acquired from domestic and international sources. Microb. Genom. 5:e000270. doi: 10.1099/mgen.0.000270
Ashkenazi, S., Levy, I., Kazaronovski, V., and Samra, Z. (2003). Growing antimicrobial resistance of Shigella isolates. J. Antimicrob. Chemother. 51, 427–429. doi: 10.1093/jac/dkg080
Baker, K. S., Campos, J., Pichel, M., Della Gaspera, A., Duarte-Martínez, F., Campos-Chacón, E., et al. (2017). Whole genome sequencing of Shigella sonnei through PulseNet Latin America and Caribbean: advancing global surveillance of foodborne illnesses. Clin. Microbiol. Infect. 23, 845–853. doi: 10.1016/j.cmi.2017.03.021
Baker, K. S., Dallman, T. J., Ashton, P. M., Day, M., Hughes, G., Crook, P. D., et al. (2015). Intercontinental dissemination of azithromycin-resistant shigellosis through sexual transmission: a cross-sectional study. Lancet Infect. Dis. 15, 913–921. doi: 10.1016/S1473-3099(15)00002-X
Baker, K. S., Dallman, T. J., Field, N., Childs, T., Mitchell, H., Day, M., et al. (2018). Genomic epidemiology of Shigella in the United Kingdom shows transmission of pathogen sublineages and determinants of antimicrobial resistance. Sci. Rep. 8:7389. doi: 10.1038/s41598-018-25764-3
Bardsley, M., Jenkins, C., Mitchell, H. D., Mikhail, A. F. W., Baker, K. S., Foster, K., et al. (2020). Persistent transmission of shigellosis in England is associated with a recently emerged multidrug-resistant strain of Shigella sonnei. J. Clin. Microbiol. 58, e01692–19. doi: 10.1128/JCM.01692-19
Barrantes, K., and Achí, R. (2016). The importance of integrons for development and propagation of resistance in Shigella: the case of Latin America. Braz. J. Microbiol. 47, 800–806. doi: 10.1016/j.bjm.2016.07.019
Bortolaia, V., Kaas, R. S., Ruppe, E., Roberts, M. C., Schwarz, S., Cattoir, V., et al. (2020). ResFinder 4.0 for predictions of phenotypes from genotypes. J. Antimicrob. Chemother. 75, 3491–3500. doi: 10.1093/jac/dkaa345
Bostock, M., Rodden, K., Warne, K., Russell, K., Breitwieser, F., and Yetman, C. J. (2019). Package ‘sunburstR’. Available Online at: https://cran.r-project.org/web/packages/sunburstR/sunburstR.pdf (accessed April 20, 2020).
Camacho, C., Coulouris, G., Avagyan, V., Ma, N., Papadopoulos, J., Bealer, K., et al. (2009). BLAST+: architecture and applications. BMC Bioinformatics 10:421. doi: 10.1186/1471-2105-10-421
Chang, C. Y., Lu, P. L., Lin, C. C., Lee, T. M., Tsai, M. Y., and Chang, L. L. (2011). Integron types, gene cassettes, antimicrobial resistance genes and plasmids of Shigella sonnei isolates from outbreaks and sporadic cases in Taiwan. J. Med. Microbiol. 60, 197–204. doi: 10.1099/jmm.0.022517-0
Chu, Y., Houang, E. T. S., Lyon, D. J., Ling, J. M., Ng, T., and Cheng, A. F. B. (1998). Antimicrobial resistance in Shigella flexneri and Shigella sonnei in Hong Kong, 1986 to 1995. Antimicrob. Agents Chemother. 42, 440–443. doi: 10.1128/AAC.42.2.440
Chung The, H., Bodhidatta, L., Pham, D. T., Mason, C. J., Ha Thanh, T., Voong Vinh, P., et al. (2021). Evolutionary histories and antimicrobial resistance in Shigella flexneri and Shigella sonnei in Southeast Asia. Commun. Biol. 4:353. doi: 10.1038/s42003-021-01905-9
Chung The, H., Boinett, C., Pham Thanh, D., Jenkins, C., Weill, F. X., Howden, B. P., et al. (2019). Dissecting the molecular evolution of fluoroquinolone-resistant Shigella sonnei. Nat. Commun. 10:4828. doi: 10.1038/s41467-019-12823-0
Chung The, H., Rabaa, M. A., Pham Thanh, D., De Lappe, N., Cormican, M., Valcanis, M., et al. (2016). South Asia as a reservoir for the global spread of ciprofloxacin-resistant Shigella sonnei: a cross-sectional study. PLoS Med. 13:e1002055. doi: 10.1371/journal.pmed.1002055
CLSI (2018). M100. Performance Standards For Antimicrobial Susceptibility Testing, 28th Edn. Wayne: Clinical and Laboratory Standards Institute.
Csardi, G. (2015). R Package ‘igraph’. Available Online at: http://igraph.org (accessed January 22, 2020).
Di Conza, J., Ayala, J. A., Power, P., Mollerach, M., and Gutkind, G. (2002). Novel class 1 integron (InS21) carrying blaCTX-M-2 in Salmonella enterica serovar Infantis. Antimicrob. Agents Chemother. 46, 2257–2261. doi: 10.1128/AAC.46.7.2257-2261.2002
Fullá, N., Prado, V., Durán, C., Lagos, R., and Levine, M. M. (2005). Surveillance for antimicrobial resistance profiles among Shigella species isolated from a semirural community in the northern administrative area of Santiago, Chile. Am. J. Trop. Med. Hyg. 72, 851–854. doi: 10.4269/ajtmh.2005.72.851
Gassama-Sowa, A., Diallo, M. H., Boye, C. S., Garin, B., Sire, J. M., Sow, A. I., et al. (2006). Class 2 integron-associated antibiotic resistance in Shigella sonnei isolates in Dakar, Senegal. Int. J. Antimicrob. Agents 27, 267–270. doi: 10.1016/j.ijantimicag.2005.10.016
Gu, B., Cao, Y., Pan, S., Zhuang, L., Yu, R., Peng, Z., et al. (2012). Comparison of the prevalence and changing resistance to nalidixic acid and ciprofloxacin of Shigella between Europe–America and Asia–Africa from 1998 to 2009. Int. J. Antimicrob. Agents 40, 9–17. doi: 10.1016/j.ijantimicag.2012.02.005
Gu, B., Xu, T., Kang, H., Xu, Y., Liu, G., Pan, S., et al. (2017). A 10-year surveillance of antimicrobial susceptibility patterns in Shigella sonnei isolates circulating in Jiangsu Province, China. J. Glob. Antimicrob. Resist. 10, 29–34. doi: 10.1016/j.jgar.2017.03.009
Haldane, J. B. S. (1940). The mean and variance of | chi 2, when used as a test of homogeneity, when expectations are small. Biometrika 31, 346–355. doi: 10.2307/2332614
Henríquez, T., Salazar, J. C., Marvasi, M., Shah, A., Corsini, G., and Toro, C. S. (2020). SRL pathogenicity island contributes to the metabolism of D-aspartate via an aspartate racemase in Shigella flexneri YSH6000. PLoS One 15:e0228178. doi: 10.1371/journal.pone.0228178
Instituto de Salud Pública de Chile [ISP] (2019). Boletín Vigilancia de Laboratorio. Vigilancia de Laboratorio de Shigella spp. Santiago: Instituto de Salud Pública de Chile.
Izumiya, H., Tada, Y., Ito, K., Morita-Ishihara, T., Ohnishi, M., Terajima, J., et al. (2009). Characterization of Shigella sonnei isolates from travel-associated cases in Japan. J. Med. Microbiol. 58, 1486–1491. doi: 10.1099/jmm.0.011809-0
Kang, H., Wang, L., Li, Y., Lu, Y., Fan, W., Bi, R., et al. (2019). Dissemination of multidrug-resistant Shigella flexneri and Shigella sonnei with Class 1, Class 2, and atypical Class 1 integrons in China. Microb. Drug Resist. 25, 1465–1474. doi: 10.1089/mdr.2018.0229
Kaushik, M., Kumar, S., Kapoor, R. K., Virdi, J. S., and Gulati, P. (2018). Integrons in Enterobacteriaceae: diversity, distribution and epidemiology. Int. J. Antimicrob. Agents 51, 167–176. doi: 10.1016/j.ijantimicag.2017.10.004
Kotloff, K. L., Riddle, M. S., Platts-Mills, J. A., Pavlinac, P., and Zaidi, A. K. M. (2018). Shigellosis. Lancet 391, 801–812. doi: 10.1016/S0140-6736(17)33296-8
Larsen, M. V., Cosentino, S., Rasmussen, S., Friis, C., Hasman, H., Marvig, R. L., et al. (2012). Multilocus sequence typing of total genome sequenced bacteria. J. Clin. Micobiol. 50, 1355–1361. doi: 10.1128/JCM.06094-11
Letunic, I., and Bork, P. (2016). Interactive tree of life (iTOL) v3: an online tool for the display and annotation of phylogenetic and other trees. Nucleic Acids Res. 44, W242–W245. doi: 10.1093/nar/gkw290
Luck, S. N., Turner, S. A., Rajakumar, K., Sakellaris, H., and Adler, B. (2001). Ferric dicitrate transport system (Fec) of Shigella flexneri 2a YSH6000 is encoded on a novel pathogenicity island carrying multiple antibiotic resistance genes. Infect. Immun. 69, 6012–6021. doi: 10.1128/IAI.69.10.6012-6021.2001
Marcoleta, A., Toro, C., Prado, V., Serrano, M., Fernández, P., Benadof, D., et al. (2013). Antibiotic susceptibility patterns among Shigella sonnei, isolated during three different periods in Región Metropolitana. Chile. Rev. Chilena Infectol. 30, 616–621. doi: 10.4067/S0716-10182013000600007
McIver, C. J., White, P. A., Jones, L. A., Karagiannis, T., Harkness, J., Marriott, D., et al. (2002). Epidemic strains of Shigella sonnei biotype g carrying integrons. J. Clin. Microbiol. 40, 1538–1540. doi: 10.1128/JCM.40.4.1538-1540.2002
Medeiros, P. H. Q. S., Lima, A. ÂM., Guedes, M. M., Havt, A., Bona, M. D., Rey, L. C., et al. (2018). Molecular characterization of virulence and antimicrobial resistance profile of Shigella species isolated from children with moderate to severe diarrhea in northeastern Brazil. Diagn. Microbiol. Infect. Dis. 90, 198–205. doi: 10.1016/j.diagmicrobio.2017.11.002
Miranda, A., Ávila, B., Díaz, P., Rivas, L., Bravo, K., Astudillo, J., et al. (2016). Emergence of plasmid-borne dfrA14 trimethoprim resistance gene in Shigella sonnei. Front. Cell Infect. Microbiol. 6:77. doi: 10.3389/fcimb.2016.00077
Muñoz, J., Bello, H., Domínguez, M., Mella, S., Zemelman, R., and González, G. (2003). Integrons and antimicrobial resistance gene cassettes in Shigella flexneri strains. Rev. Med. Chil. 131, 727–733.
Njamkepo, E., Fawal, N., Tran-Dien, A., Hawkey, J., Strockbine, N., Jenkins, C., et al. (2016). Global phylogeography and evolutionary history of Shigella dysenteriae type 1. Nat. Microbiol. 1:16027. doi: 10.1038/nmicrobiol.2016.27
Nurk, S., Bankevich, A., Antipov, D., Gurevich, A., Korobeynikov, A., Lapidus, A., et al. (2013). “Assembling genomes and mini-metagenomes from highly chimeric reads,” in Research in Computational Molecular Biology. RECOMB 2013. Lecture Notes in Computer Science, Vol. 7821, eds M. Deng, R. Jiang, F. Sun, and X. Zhang (Berlin: Springer), 158–170. doi: 10.1007/978-3-642-37195-0_13
Oh, J. Y., Yu, H. S., Kim, S. K., Seol, S. Y., Cho, D. T., and Lee, J. C. (2003). Changes in patterns of antimicrobial susceptibility and integron carriage among Shigella sonnei isolates from Southwestern Korea during epidemic periods. J. Clin. Microbiol. 41, 421–423. doi: 10.1128/JCM.41.1.421-423.2003
Pan, J. C., Ye, R., Meng, D. M., Zhang, W., Wang, H. Q., and Liu, K. Z. (2006). Molecular characteristics of class 1 and class 2 integrons and their relationships to antibiotic resistance in clinical isolates of Shigella sonnei and Shigella flexneri. J. Antimicrob. Chemother. 58, 288–296. doi: 10.1093/jac/dkl228
Parajuli, P., Deimel, L. P., and Verma, N. K. (2019). Genome analysis of Shigella flexneri serotype 3b strain SFL1520 reveals significant horizontal gene acquisitions including a multidrug resistance cassette. Genome Biol. Evol. 11, 776–785. doi: 10.1093/gbe/evz026
Price, M. N., Dehal, P. S., and Arkin, A. P. (2010). FastTree 2 - Approximately maximum-likelihood trees for large alignments. PLoS One 5:e9490. doi: 10.1371/journal.pone.0009490
Puzari, M., Sharma, M., and Chetia, P. (2018). Emergence of antibiotic resistant Shigella species: a matter of concern. J. Infect. Public Health 11, 451–454. doi: 10.1016/j.jiph.2017.09.025
R Core Team (2014). R: A Language and Environment for Statistical Computing. Vienna: R Foundation for Statistical Computing.
Ranjbar, R., Aleo, A., Giammanco, G., Dionisi, A. M., Sadeghifard, N., and Mammina, C. (2007). Genetic relatedness among isolates of Shigella sonnei carrying class 2 integrons in Tehran, Iran, 2002–2003. BMC Infect. Dis. 7:62. doi: 10.1186/1471-2334-7-62
Ranjbar, R., and Farahani, A. (2019). Shigella: antibiotic-resistance mechanisms and new horizons for treatment. Infect. Drug Resist. 12, 3137–3167. doi: 10.2147/IDR.S219755
Recchia, G. D., and Hall, R. M. (1995). Gene cassettes: a new class of mobile element. Microbiol. 141, 3015–3027. doi: 10.1099/13500872-141-12-3015
Sadouki, Z., Day, M. R., Doumith, M., Chattaway, M. A., Dallman, T. J., Hopkins, K. L., et al. (2017). Comparison of phenotypic and WGS-derived antimicrobial resistance profiles of Shigella sonnei isolated from cases of diarrhoeal disease in England and Wales, 2015. J. Antimicrob. Chemother. 72, 2496–2502. doi: 10.1093/jac/dkx170
Sati, H. F., Bruinsma, N., Galas, M., Hsieh, J., Sanhueza, A., Ramon Pardo, P., et al. (2019). Characterizing Shigella species distribution and antimicrobial susceptibility to ciprofloxacin and nalidixic acid in Latin America between 2000-2015. PLoS One 14:e0220445. doi: 10.1371/journal.pone.0220445
Seol, S. Y., Kim, Y. T., Jeong, Y. S., Oh, J. Y., Kang, H. Y., Moon, D. C., et al. (2006). Molecular characterization of antimicrobial resistance in Shigella sonnei isolates in Korea. J. Med. Microbiol. 55, 871–877. doi: 10.1099/jmm.0.46441-0
Seribelli, A. A., Frazão, M. R., Medeiros, M. I., and Falcão, J. P. (2016). Molecular and phenotypic characterization of Shigella sonnei isolated over 31 years suggest the circulation of two prevalent subtypes in the São Paulo State in Brazil. J. Med. Microbiol. 65, 666–677. doi: 10.1099/jmm.0.000290
Shariati, A., Sabzehali, F., Goudarzi, M., and Azimi, H. (2018). Integrons and antimicrobial resistance in bacteria: a systematic review. Arch. Adv. Biosci. 9, 39–48. doi: 10.22037/jps.v9i2.18726
Shiferaw, B., Solghan, S., Palmer, A., Joyce, K., Barzilay, E. J., Krueger, A., et al. (2012). Antimicrobial susceptibility patterns of Shigella isolates in foodborne diseases active surveillance network (FoodNet) sites, 2000-2010. Clin. Infect. Dis. 54, S458–S463. doi: 10.1093/cid/cis230
Stokes, H. W., and Hall, R. M. (1989). A novel family of potentially mobile DNA elements encoding site-specific gene-integration functions: integrons. Mol. Microbiol. 3, 1669–1683. doi: 10.1111/j.1365-2958.1989.tb00153.x
Sullivan, M. J., Petty, N. K., and Beatson, S. A. (2011). Easyfig: a genome comparison visualizer. Bioinformatics 27, 1009–1010. doi: 10.1093/bioinformatics/btr039
Toro, C. S., Farfán, M., Contreras, I., Flores, O., Navarro, N., Mora, G. C., et al. (2005). Genetic analysis of antibiotic-resistance determinants in multidrug-resistant Shigella strains isolated from Chilean children. Epidemiol. Infect. 133, 81–86. doi: 10.1017/S0950268804003048
Turner, S. A., Luck, S. N., Sakellaris, H., Rajakumar, K., and Adler, B. (2003). Molecular epidemiology of the SRL pathogenicity island. Antimicrob. Agents Chemother. 47, 727–734. doi: 10.1128/AAC.47.2.727-734.2003
Turner, S. A., Luck, S. N., Sakellaris, H., Rajakumar, K., and Adler, B. (2004). Role of attP in integrase-mediated integration of the Shigella resistance locus pathogenicity island of Shigella flexneri. Antimicrob. Agents Chemother. 48, 1028–1031. doi: 10.1128/AAC.48.3.1028-1031.2004
Vrints, M., Mairiaux, E., Van Meervenne, E., Collard, J. M., and Bertrand, S. (2009). Surveillance of antibiotic susceptibility patterns among Shigella sonnei strains isolated in Belgium during the 18-year period 1990 to 2007. J. Clin. Microbiol. 47, 1379–1385. doi: 10.1128/JCM.02460-08
Wei, T., Simko, V., Levy, M., Xie, Y., Jin, Y., Zemla, J., et al. (2017). Package “corrplot”. Available Online at: https://cran.r-project.org/web/packages/corrplot/corrplot.pdf (accessed April 20, 2020).
Williams, P., and Berkley, J. (2016). Current WHO guidelines and the WHO esssential medicine list for children. Available Online at: https://www.who.int/selection_medicines/committees/expert/21/applications/s6_paed_antibiotics_appendix5_dysentery.pdf?ua=1 (accessed April 4, 2019).
Williams, P., and Berkley, J. (2018). Guidelines for the treatment of dysentery (shigellosis): a systematic review of the evidence. Paediatr. Int. Child Health 38, 50–65. doi: 10.1080/20469047.2017.1409454
Zankari, E., Allesøe, R., Joensen, K. G., Cavaco, L. M., Lund, O., and Aarestrup, F. M. (2020). PointFinder: a novel web tool for WGS-based detection of antimicrobial resistance associated with chromosomal point mutations in bacterial pathogens. J. Antimicrob. Chemother. 72, 2764–2768. doi: 10.1093/jac/dkx217
Keywords: Shigella sonnei, antibiotic resistance, mobile genetic elements (MGE), integrons, SRL pathogenicity island, multidrug-resistant (MDR) bacteria, dfrA14, class 2 integron
Citation: Toro CS, Salazar JC, Montero DA, Ugalde JA, Díaz J, Cádiz LA, Henríquez T, García C, Díaz P, Camponovo R, Hermosilla G and Ulloa MT (2022) Antimicrobial Resistance Dynamics in Chilean Shigella sonnei Strains Within Two Decades: Role of Shigella Resistance Locus Pathogenicity Island and Class 1 and Class 2 Integrons. Front. Microbiol. 12:794470. doi: 10.3389/fmicb.2021.794470
Received: 13 October 2021; Accepted: 22 December 2021;
Published: 04 February 2022.
Edited by:
Jing Wang, Yangzhou University, ChinaReviewed by:
Ashima Kushwaha Bhardwaj, Independent Researcher, Gurugram, IndiaFarzad Badmasti, Microbiology Research Center, Pasteur Institute of Iran, Iran
Thandavarayan Ramamurthy, National Institute of Cholera and Enteric Diseases (ICMR), India
Copyright © 2022 Toro, Salazar, Montero, Ugalde, Díaz, Cádiz, Henríquez, García, Díaz, Camponovo, Hermosilla and Ulloa. This is an open-access article distributed under the terms of the Creative Commons Attribution License (CC BY). The use, distribution or reproduction in other forums is permitted, provided the original author(s) and the copyright owner(s) are credited and that the original publication in this journal is cited, in accordance with accepted academic practice. No use, distribution or reproduction is permitted which does not comply with these terms.
*Correspondence: Cecilia S. Toro, Y2V0b3JvQHUudWNoaWxlLmNs
‡Present address: Leandro A. Cádiz, Departamento de Patología Animal, Facultad de Ciencias Veterinarias y Pecuarias, Universidad de Chile, Santiago, Chile; Tania Henríquez, Biozentrum, Ludwig-Maximilians-Universität München, Martinsried, Germany Camila; García, Laboratorio de Microbiología, Clínica Las Condes, Santiago, Chile; Patricia Díaz, Departamento de Salud Pública, Escuela de Medicina, Pontificia Universidad Católica de Chile, Santiago, Chile
†These authors have contributed equally to this work and share first authorship