- 1Doctorado en Ciencias Aplicadas mención Sistemas Marinos Costeros, Universidad de Antofagasta, Antofagasta, Chile
- 2Laboratorio de Complejidad Microbiana y Ecología Funcional, Instituto Antofagasta and Centro de Bioingeniería y Biotecnología (CeBiB), Universidad de Antofagasta, Antofagasta, Chile
- 3Laboratorio de Biotecnología en Ambientes Extremos, Centro de Excelencia en Medicina Traslacional, Universidad de la Frontera, Temuco, Chile
- 4Departamento de Ciencias y Geografía, Facultad de Ciencias Naturales y Exactas y HUB Ambiental UPLA, Universidad de Playa Ancha, Valparaíso, Chile
- 5Centro de Investigación Oceanográfica COPAS COASTAL, Universidad de Concepción, Concepción, Chile
- 6Laboratorio de Sedimentología y Paleoambientes, Facultad de Ciencias del Mar y de Recursos Biológicos, Instituto de Ciencias Naturales A. von Humboldt, Universidad de Antofagasta, Antofagasta, Chile
- 7Independent Researcher, Talca, Chile
- 8Centro de Investigación y Estudios Avanzados del Maule, Vicerrectoría de Investigación de Investigación y Posgrado, Universidad Católica del Maule, Campus San Miguel, Talca, Chile
- 9J’EAI CHARISMA (IRD-France, UMNG-Colombia, UA-Chile, UCM-Chile, UCH-Chile, IGP-Peru, UPCH-Peru) and Nucleo Milenio UPWELL, Concepción, Chile
- 10Center for Bioinformatics and Integrative Biology, Facultad de Ciencias de la Vida, Universidad Andrés Bello, Santiago, Chile
- 11Departamento de Biotecnología, Facultad de Ciencias del Mar y Recursos Biológicos, Universidad de Antofagasta, Antofagasta, Chile
Taxonomic and functional microbial communities may respond differently to anthropogenic coastal impacts, but ecological quality monitoring assessments using environmental DNA and RNA (eDNA/eRNA) in response to pollution are poorly understood. In the present study, we investigated the utility of the co-occurrence network approach’s to comprehensively explore both structure and potential functions of benthic marine microbial communities and their responses to Cu and Fe fractioning from two sediment deposition coastal zones of northern Chile via 16S rRNA gene metabarcoding. The results revealed substantial differences in the microbial communities, with the predominance of two distinct module hubs based on study zone. This indicates that habitat influences microbial co-occurrence networks. Indeed, the discriminant analysis allowed us to identify keystone taxa with significant differences in eDNA and eRNA comparison between sampled zones, revealing that Beggiatoaceae, Carnobacteriaceae, and Nitrosococcaceae were the primary representatives from Off Loa, whereas Enterobacteriaceae, Corynebacteriaceae, Latescibacteraceae, and Clostridiaceae were the families responsible for the observed changes in Mejillones Bay. The quantitative evidence from the multivariate analyses supports that the benthic microbial assemblages’ features were linked to specific environments associated with Cu and Fe fractions, mainly in the Bay. Furthermore, the predicted functional microbial structure suggested that transporters and DNA repair allow the communities to respond to metals and endure the interacting variable environmental factors like dissolved oxygen, temperature, and salinity. Moreover, some active taxa recovered are associated with anthropogenic impact, potentially harboring antibiotic resistance and other threats in the coastal zone. Overall, the method of scoping eRNA in parallel with eDNA applied here has the capacity to significantly enhance the spatial and functional understanding of real-time microbial assemblages and, in turn, would have the potential to increase the acuity of biomonitoring programs key to responding to immediate management needs for the marine environment.
1. Introduction
High abundance, diversity, and activity of microorganisms in marine sediments play vital roles in global biogeochemical cycles (Prokopenko et al., 2013; Hoshino et al., 2020), partially because they are involved in the transforming and degrading organic matter (Koho et al., 2013), these processes, release nitrogen and phosphorus nutrients into the water column, thus facilitating the growth of primary producers and contributing to climate regulation (Cavicchioli et al., 2019). However, most previous studies that consider the composition and spatial distribution of microbial communities in coastal areas both worldwide (Jayakumar et al., 2012; Glass et al., 2015) and in Chile have focused on the water column (e.g., Molina et al., 2010, 2020, 2021; Stewart et al., 2012; Ulloa et al., 2012) and little is known about benthic microbial structure, activity and interactions in marine sediments that experience fluctuating redox conditions due to the oxygen-depleted waters and trace metal (TM) gradients (Farías et al., 2004; Broman et al., 2017; Rasigraf et al., 2017; Miranda et al., 2020).
In general, these conditions are generated by vertical geochemical zonation that results from the thermodynamically driven succession of organic matter (OM) availability and high-potential electron acceptors (e.g., oxygen and nitrate; Prokopenko et al., 2013; Broman et al., 2017; Rasigraf et al., 2017). Therefore, marine sediments are considered both a sink and a source for OM as well as other nutrients and pollutants, contributing substantially to climate regulation processes via carbon burial (Koho et al., 2013; Sander et al., 2015). In fact, previous research carried out in northern Chilean coastal sediments, indicates that OM accumulation governs the biogeochemistry of the sediments bio-essential TMs such as Cu and Fe closely associated with nutrient-rich but oxygen-depleted waters (Valdés et al., 2005, 2014; Plass et al., 2020) via atmospheric deposition from the Atacama Desert and continental inputs, primarily the Loa River (Romero et al., 2003; Flores-Aqueveque et al., 2014). Moreover, although the TMs are important redox-active elements, they persist and accumulate, influencing the selection, distribution and dynamics of particular microbial guilds associated with metalloenzyme-involved metabolisms, such as nitrification or denitrification besides other processes related with sulfur cycling (Glass et al., 2015; Rasigraf et al., 2020), and therefore, establishing specific metabolic networks and geochemical profiles in marine zones associated with oxygen-depleted waters (Glass et al., 2015; Rasigraf et al., 2017).
Hence, the relevant question is whether microbial benthic communities and their control mechanisms will be varied in the marine sediments with different depositional histories and fluctuating redox conditions due to the influence of oxygen-depleted waters and TMs gradients off northern Chile. We hypothesized that the fractionation of Cu and Fe acts as a driving forces in modulating the structure of benthic microbial assemblages and could be directly linked to the responses of active and resident communities. To test the hypothesis, we combined the analysis of DNA- and RNA-derived microbial 16S rRNA gene sequences from sediments of two contrasting coastal zones in northern Chile, one from the semi-enclosed Mejillones Bay, and the other that we referred to as the Off-Loa zone, as it has river input from the hyperarid Atacama Desert. We investigated the spatial microbial community characteristics, assembly patterns, gene prediction and environmental driving factors focusing on changes in response to Cu and Fe within 10–60 m water-depth gradient. Furthermore, we performed various network and statistical analyses in order to identify the significance of co-occurrence of microbial communities between contrasting study zones.
Correlation-based network analysis provides a good way to determine the potential interplays between microbes as well as their structuring and mediating ecological functions in various environments (Chaffron et al., 2010; Banerjee et al., 2018; Felipe-Lucia et al., 2020), including the Mejillones Bay and Loa River (Zárate et al., 2020, 2021). Therefore, the results of the present study highlights important targets to better understand microbial responses to the bioavailability of TMs in low-oxygen sedimentary environments. This knowledge is indispensable for understanding and predicting how ecosystems will respond to many of today’s biggest challenges, from climate change (hypoxia and warming) to the propagation of metal and antibiotic resistance mechanisms between microbes (Nogales et al., 2011; Hernando-Amado et al., 2019).
2. Materials and methods
2.1. Study area
Fieldwork was conducted in two upwelling coastal sites off northern Chile (Supplementary Figure S1) in late autumn 2017: named Mejillones Bay (MB; 23°20′ S, 70°30′ W) and Off Loa Zone (OL; 21°20′ S, 71°05′ W). These sites were chosen as they fall located in a natural laboratory area.
MB is located in the southeastern Pacific on the coast of the Atacama Desert and has been considered a semi-enclosed system with high biological production (Marín et al., 2003), strongly anthropized via the development of multiple mineral exporting harbors and a thermoelectric plant, leading to significant implications on the heavy metal (HM) accumulation in sediments (Valdés, 2012; Castillo et al., 2019; Zárate et al., 2021). OL is located approximately 210 km north of MB near the city of Iquique (Isla et al., 2010). This area, though less studied than MB, has been described as a spawning and nursery area for small pelagic fish with high planktonic production (Santander et al., 2017), supporting world’s largest fisheries (Herrera and Escribano, 2006; Palma et al., 2006). In addition, is exposed to the discharge of the Loa River, which extends east to west through the Atacama Desert and to the Pacific Ocean, carrying different pollutants originating from industrial mining (e.g., the Chuquicamata copper mine), agriculture and domestic wastes. However, due to the water usage the Loa River discharge to the coastal zone is mainly sporadic owing to precipitation increases and flood (up to four orders of magnitude) mainly during austral summer (i.e., December and March; Romero et al., 2003).
2.1.1. Sample collection
Three sediment cores 1–5 cm long and with 2.5 cm inner diameters were collected at each field site using an Ekman Box-core (Bottom sampler Ekman-Birge, Hydro-Bios) at depths of 20 and 60 m in the middle of bay (MB), and at 20, 30, and 60 m depth Off OL between July and November 2017, respectively. The sediment for DNA and RNA were immediately preserved at 4°C during transport to the laboratory. Subsequently, the uppermost 3 cm of the sediment cores were cut into slices every 1 cm and stored at −20°C for DNA and −80°C for RNA, the latter preserved with RNAlater® solution (Ambion, United States) until the extraction process. Sediment subsamples from each of the same core fraction used in the nucleic acid analyses were used for measuring Cu and Fe concentration, total carbon (TC), nitrogen (TN), Sulfur content (TS) and grain size distribution. Briefly, the content of TC, TN, and TS was measured by analyzing ~3 mg of dry sediment on a LECO CHNS elemental analyzer (2400 series II, Perkin Elmer). Then the analytical procedure was verified with the analysis of sulfanilic acid (C6H7NO3S) as a reference material (Castillo et al., 2019). At the collection sites, pH, salinity, conductivity, dissolved oxygen (DO), and temperature (°C) of the water column were measured using a CTD-O profiler (SBE 19 plus V2 Sea cat, SeaBird).
2.2. eDNA/RNA extraction and sequence-data processing
Total environmental DNA and RNA (eDNA/eRNA) was extracted in triplicate from approximately 0.5 g (wet weight) of the samples at each depth (i.e., 20, 30, and 60 m) for a total of 66 samples from two sites (see Figures 1, 2) across the Atacama Desert Coastline, employing the MoBio PowerSoil® DNA Isolation Kit (MO BIO Laboratories, Inc.) according to the manufacturer’s instructions. We supplemented with three 5-min rounds of bead beating. The yield and quality of the extracted DNA samples were reviewed on 0.8% agarose gel. The extracted genomic DNA was quantified and checked for purity at A260/280 nm by a NanoDrop spectrophotometer (Thermo Fisher 150 Scientific, United States). The DNA was stored at −20°C until further sequence processing.
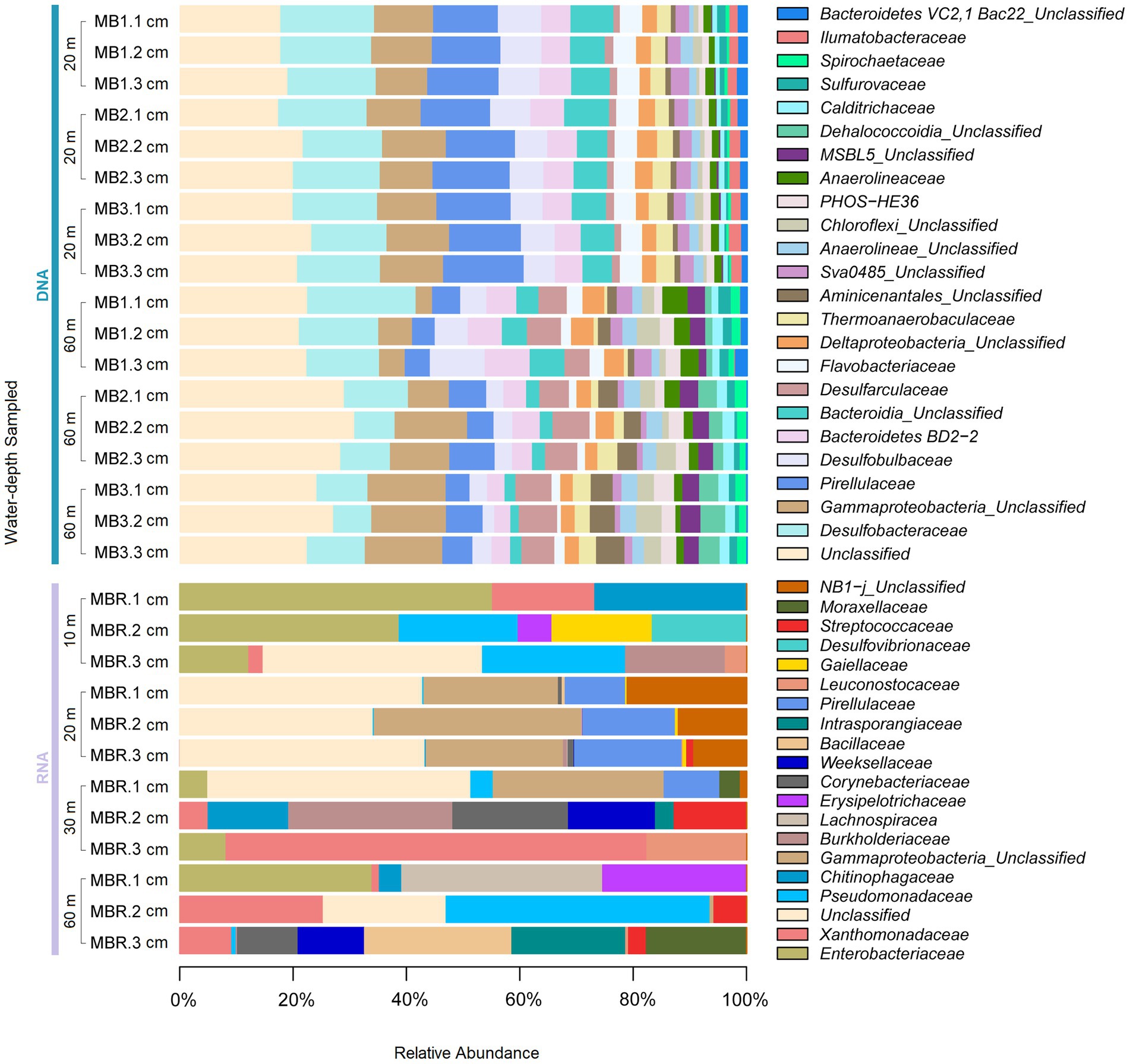
Figure 1. The specific composition of the benthic microbial community at family level in DNA and RNA samples across the water depths sampled in Mejillones Bay.
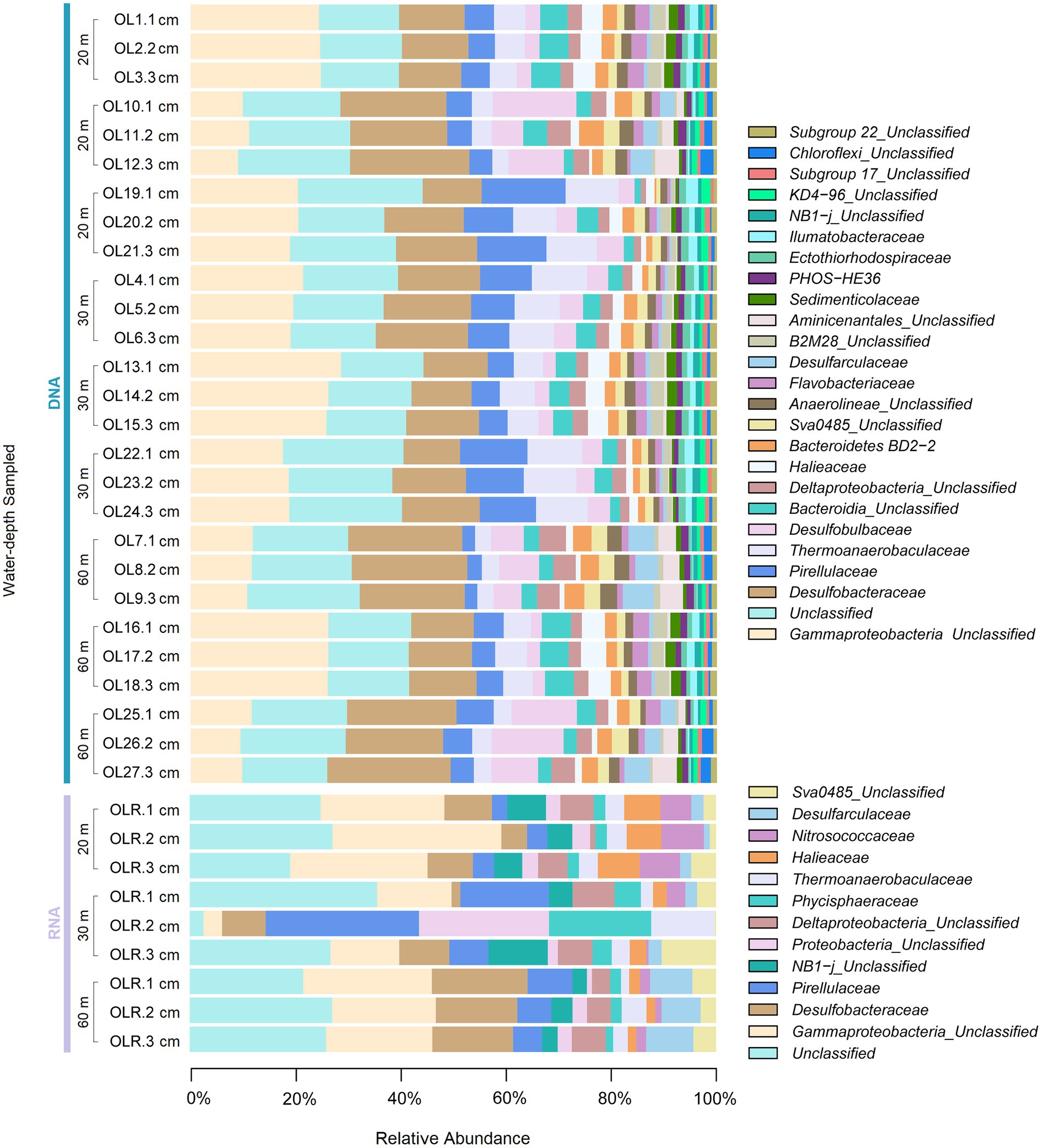
Figure 2. The specific composition of the benthic microbial community at the family level in DNA and RNA samples across the water depths sampled in Off-Loa River zone.
eRNA samples were thawed on ice, and the remaining RNAlater® was carefully removed and discarded after initiating the RNA extraction from 2 g of sediment (wet weight) using mirVana™ miRNA Isolation Kit (Ambion, United States) following the manufacturer’s specifications with the addition of a step of mechanical cell disruptions (200-μm-diameter zirconium beads, Low Binding Zirconium Beads, OPS Diagnostics) for three rounds of 30 s (∼3,000 rpm) using a Mini-Beadbeater-8 (Biospec Products). DNA traces were removed using the Turbo DNA-free kit (Ambion, United States) and RNA concentrations were verified using the Qubit® RNA Assay Kit (Thermo Fisher Scientific, United States). Quality was ensured via agarose gel electrophoresis, following manufacturer’s instructions and immediately reverse transcribed to complementary DNA (cDNA) using 20 ng of total RNA with random primers provided by the ImProm-II™ Reverse Transcription System (Promega Corp, United States). Synthesized cDNA was further used for amplification according to the protocol.
The DNA samples were sequenced using next-generation Illumina MiSeq platform at Macrogen Corporation (South Korea). Sequencing of amplicons encompassing the V3–V4 hypervariable regions of 16S rRNA gene (~460 bp) of the prokaryotes were amplified using the 515 F/806 R primer pair (Caporaso et al., 2011). Meanwhile, cDNA sample analyses were performed using an Illumina MiSeq platform (pair ends 2 × 126 bp) technology MrDNA (Shallowater, Texas, United States). Illumina-generated rRNA-amplicon sequences (iTags) were further processed through bioinformatics analysis.
2.3. Microbial community sequence and biostatistics analyses
Sequencing analyses to assess the structure and composition of the benthic microbial communities from two field sampling coastal zones (MB and OL). Raw sequences were demultiplexed, joined paired reads, quality-filtered, chimera checked, curated and analyzed using the DADA2 pipeline (Callahan et al., 2016).
All sequences were filtered to truncate the paired reads to 150 nt and eliminate reads with quality values of <2. Error rates were estimated and corrected by pooling all the reads from the sequencing run, with default parameters. Taxonomy was assigned using the SILVA release v132 references alignment (Quast et al., 2013). Resulting amplicon sequence variants (ASVs) were analyzed using the Phyloseq package in R (McMurdie and Holmes, 2013). Noteworthy, taxonomic data were analyzed by both order and family, levels that were chosen based on the number of classified ASVs (Supplementary Table S1). For ASVs that were not assigned taxonomies of these categories, we used the last level classification (ending in “_Unclassified”).
Within-sample diversity (alpha diversity) was determined by calculating the Shannon and Simpson diversity indices (evenness) using the total number of observed ASV and Chao1 (richness; Shannon and Weaver, 1949; Oksanen et al., 2020) in the vegan R packages (R Core Team, 2015). Beta-diversities of communities were compared using the non-Metric Multidimensional Scaling (nMDS) to visualize the levels of dissimilarity of communities with Bray-Curtis distance between samples. Analysis of similarity (ANOSIM) was used to test the significance of differences between groups of sampling zones (MB and OL) of the NMDS analyses. Venn diagrams were also constructed using the InteractiVenn (Heberle et al., 2015) based on the distribution of families showing specific and shared ASVs between eDNA/eRNA and sampling zones.
Benthic microbial communities and their correlation with environmental variables were explored by Spearman’s correlation and permutational multivariate analysis of variance using distance matrices (PERMANOVA) to test the significance of variables using R statistical software. In addition, redundancy analysis (RDA) was performed for a better understanding of the correlation among abundant bacterial families and physicochemical parameters in different sampling zones using CANOCO v4.5 for Windows via Monte Carlo permutation testing with 999 permutations (Ter Braak and Smilauer, 2002). Variables with a variance inflation factor (VIF) of >20 were removed from the dataset one at a time in order to control for correlated explanatory variables which could inflate the variance of the canonical coefficients.
To highlight the substantial shifts in benthic microbial community assemblage, linear discriminant analysis (LDA) effect size (LEfSe; Segata et al., 2011) was used to identify taxa with significant differences between DNA and RNA at various taxonomic levels. In addition, a differential abundance analysis was performed with the DESeq2 (Love et al., 2014) of the R library to identify the ASVs at the family level that have significant fold changes between the sampling zones (MB vs. OL) and water-depths. The log2 fold change plot was made with the significant ASVs at a value of p < 0.01. Finally, microbial community functional potential profiles were predicted based on 16S rRNA gene data using PICRUSt and the functional terms stored in the Kyoto Encyclopedia of Genes and Genomes (KEGG) database (Langille et al., 2013). The heatmap was created based on Bray–Curtis dissimilarity dendrogram of the functional capabilities detected.
A co-occurrence network was applied to reveal the interaction between microbial communities. The network inference was estimated based on co-occurrence patterns based on Spearman’s significant correlations (R > 0.75; p ≤ 0.001) at the family level using Fruchtermann–Reingold layout (Fruchterman and Reingold, 1991). In order to describe the topology of the resulting microbial network from the study zones (Supplementary Table S2), a set of metrics including edges, nodes, degree, betweenness centrality (BC), clustering coefficient (CC), average path length, modularity and network diameter was calculated (Newman, 2003). Nodes with highest BC value, representing the relevance of a node as capable of holding together with communicating nodes, were recognized as possible “keystone taxa” in co-occurrence networks (Brandes et al., 2001). The network visualization and modular analysis were conducted in Gephi v0.9.2 software (Bastian and Heymann, 2009).
2.4. Chemical procedures for sequential extraction of Fe and Cu in sediment cores
The exchangeable fraction of Fe and Cu in sediments was determined using the BCR three step sequential extraction procedure described by Rauret et al. (1999) plus the pseudo-total fraction, which was obtained after digestion with aqua regia.
Details of the experimental protocol for sequential extraction and the reagents applied in this study have been described in a previous study carried out by Pardo et al. (2004). Briefly, all extractions were drained into 50 ml metal-free polypropylene tubes (VWR), where the ground sediment samples (1 g) were mixed for 16 h (overnight) at room temperature, using a mechanical shaker. The extract was then separated from the solid residue by centrifugation at 3,000 rpm for 15 min, and each resultant supernatant liquid (each extraction step was evaporated to near dryness) was transferred into a new vial, then sealed and stored at −20°C until chemical analyses. Subsequently, the residue sediment was washed twice by adding 10 ml of deionized water (Mili-Q quality), shaken for 15 min on the end-over-end shaker and centrifuged for 20 min at 3,000 rpm. The supernatant was decanted, taking care not to lose the solid residue to use in the next step, testing visually the clear solution indicating the total digestion (no residual) of the sediment.
Three independent replicates were performed for each sample (1 g sediment) and blanks were measured in parallel for each set of analyses using aqua regia extraction and the BCR procedure, respectively. Laboratory blanks and replicates were analyzed during each batch of extractions. Finally, the residual fraction (RF) was determined separately by digestion with aqua regia for 12 h, using a CEM-MARS 5 brand microwave digestion system with closed Teflon vessels and covers used for acid digestion of the sediment samples. Vessels containing 1 g of the sediment and 12 ml of acid (15 ml HNO3 + 5 ml HCl) were heated at 60°C for 2 h and then at 100°C for 2 h. After cooling, digests were filtered through Whatman filter paper into 100 ml volumetric flasks.
Fe and Cu concentrations in the different extracted solutions were quantified by inductively coupled plasma optical emission spectrometry (ICP-OES), using a PerkinElmer Optima 8300DV spectrometer. All dilutions were prepared with deionized water. The plastic and glassware used were soaked in 5% HNO3 solution overnight and rinsed with distilled water prior to use. To evaluate the accuracy and precision of the BCR extraction in all samples, the marine sediment MESS-3 standards (National Research Council, Canada) were used following all the lab procedures. Measured concentrations in BCR-701 included in the analysis were in good agreement against their certified values, and the standard deviation of replicated samples was on average ± 7%.
3. Results
3.1. Environmental characteristics of water and trace metals in surface sediments
Water-depths ranged from 20 to 60 m. The variations of temperature (T°C) and DO (Supplementary Table S3) distinguished the two sampling zones, MB and OL. The DO levels in the overlying bottom water were found to vary from 1.11 to 0.160 mL/L in MB, while 0.078 to 0.065 mL/L was the observed range across the depth in OL. The salinity in the study locations varied slightly from 34.83 to 34.86 PSU. Meanwhile, the temperature decreased with depth in MB (15.03°C–14.07°C between 20 and 60 m), whereas it remained stable at both depths in OL with a range of 12.53–12.78°C.
TC and TS concentrations in the sediments of both sampling localities increase with depth and were much higher in MB than OL; however, only TC showed a significant difference between sampling zones (p = 0.003). Sediment values of TN in MB were also slightly higher than those in OL (p = 0.005), ranging from 3.43 to 3.82 and 0.26 to 0.46, respectively (Supplementary Table S3).
Cu and Fe were measured to establish their exchangeable fractions and assess their potential bioavailability in surface sediments of MB and OL. Analysis of marine sediment standard reference material showed satisfactory accuracy, with the recoveries for Fe and Cu being up to 100%. The average pseudo-total elemental (total extraction) concentration in the sediment of MB for Fe and Cu were observed as 4351.09–5132.34 mg kg−1 and, 46.79–50.54 mg kg−1 at 20 and 60 m depth, respectively. Meanwhile, OL sediments presented pseudo-total contents of Fe and Cu to be 7568.95–7133.01 mg kg−1 and 49.57–53.61 mg kg−1 at 20 and 60 m, respectively (Table 1). As well as most Fe and Cu content was bound within the crystal structure of the minerals as a residual fraction (RF), being 43% and 46% in MB and 57% and 58% in the OL at 20 and 60 m, respectively. Results indicated that, the fractions in the sediments of both sampling localities followed a trend of TMs magnitude concentration from RF > oxidizable > reducible > exchangeable with slight differences (see Supplementary Figure S2). The exchangeable fraction of Fe and Cu was higher at 20 m (52%) than 60 m (51%) in both sampling localities. The reducible and oxidizable fractions showed similar percentages with 60% and 75% at 20 and 60 m depths.
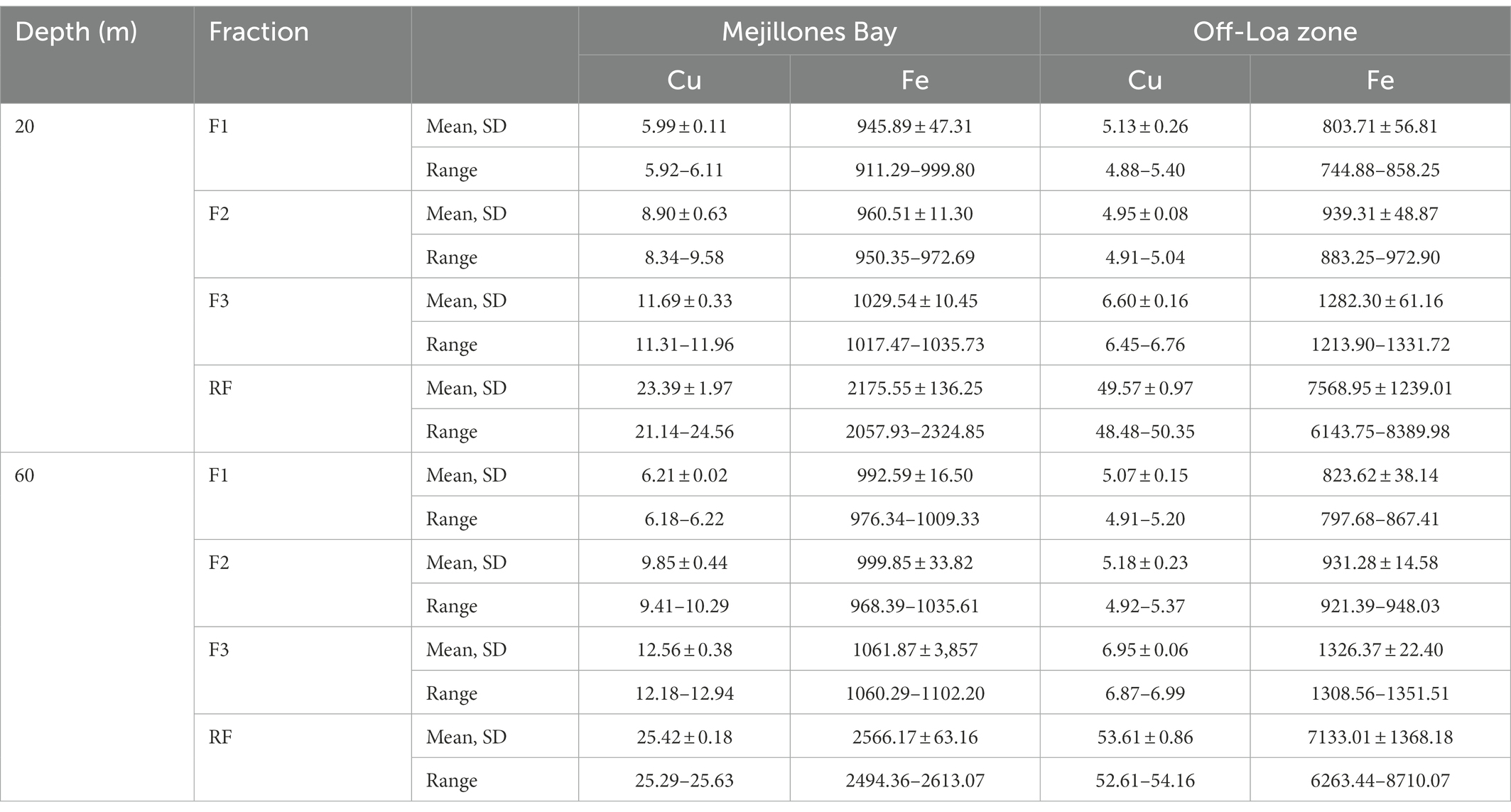
Table 1. Concentrations of Cu and Fe in each fraction of surface sediments of Mejillones Bay and Off-Loa River zone (mg.kg−1; n = 3).
3.2. Diversity and spatial patterns of benthic microbial community structure
DNA sequencing yielded 1,309,920 and 1,083,719 microbial raw reads in MB and OL, respectively. After denoising and chimera remotion, a total of 1,287,708 in MB and 951,723 in OL from 16S rRNA gene sequences were employed for downstream analyses. RNA sequencing yielded 727,041 and 391,476 microbial raw reads to sediment samples in MB and OL, respectively. After the same quality control, a total of 593,410 and 315,869 high-quality sequences remained in MB and OL, respectively (see Supplementary Table S4).
To compare the diversity and richness of benthic microbial communities in the different sampling localities across water-depth, Chao1, ACE, Simpson, and the Shannon diversity indices were calculated based on the generated ASVs (Supplementary Figure S3). The richness, estimated by the number of ACE and Chao1, were significantly different (p < 0.001) among the eDNA and eRNA from MB and OL, yet had no significant correlation with the sampled water-depth gradients. In general, these parameters indicate that the resident (eDNA) microbial abundance and diversity in both study zones were higher than that in active (eRNA) fraction of the communities studied.
Both benthic sampling zones harbored diverse microbial communities, which were identified from eDNA and eRNA (Figures 1, 2). The classification of 16S rRNA gene fragment sequences from MB sediment samples were assigned to 48 bacterial phyla and 6 Archaea groups and further classified into 123 classes, 264 orders, and 366 families of prokaryotes. In OL, a total 47 bacterial phyla and 6 Archaea groups were observed, which included 122 classes, 265 orders and 385 families. Microbial taxa exhibiting a relative abundance >1% in each sediment sequences at various taxonomic levels were designated as dominant. The benthic microbial communities at both sampling zones consisted mainly of the same microbial communities, yet their distributions differed (Figure 1). Proteobacteria accounted for 28.5% and 43.4% of the relative abundance in MB and OL, respectively. Within this phylum, the sequences mainly consisted of Deltaproteobacteria (18.6% and 21.7%), Gammaproteobacteria (9.3% and 21.1%), and Alphaproteobacteria (0.4% at both locations).
However, differences in the relative contribution of dominating classes were found in the different templates, for example, Gammaproteobacteria was higher (23.0%) in eRNA than in eDNA samples (9.3%), whereas Deltaproteobacteria (18.6%), Bacteroidia (10.0%) and Planctomycetacia (6.3%) were higher in eDNA compared with eRNA templates (3.5, 5.3 and 1.0%, respectively). Also, eDNA recorded higher proportions of Deltaproteobacteria (21.7%), Gammaproteobacteria (21.1%), Bacteroidia (6.6%), and Planctomycetacia (5.3%) compared to the RNA fraction (7.5, 9.8, 1.3 and 2.4%, respectively). The retrieved archaeal in the sequencing were detected at very low frequencies (always <0.5%). The archaeal groups were affiliated with Thermoplasmata, Bathyarchaeia, Lokiarchaeia, Nitrososphaeria, Nanohaloarchaeia, Woesearchaeia, Methanomicrobia, Micrarchaeia, Odinarchaeia, and Heimdallarchaeia. The rest of the groups were rare and represented by <0.04% of relative abundance at both sampling localities.
3.3. Differential distribution of ASVs at family level in the sampling zones across sampled water-depth
At both sites, the most abundant microbial families by far (>3% relative abundance) in eDNA samples were Desulfobacteraceae, Pirellulaceae, Desulfobulbaceae, and Thermoanaerobaculaceae. However, in eRNA samples, Enterobacteriaceae, Xanthomonadaceae, Pirellulaceae, and Pseudomonadaceae were the most abundantly active families (Figures 1, 2). Additionally, although many validated reads were classified at the phylum level, the number of reads affiliated with unclassified taxa increased from the phylum to the genus level (see Supplementary Figure S4), recording 25.7 and 16.7 from DNA and RNA, respectively, at the class level.
Dissimilarity analysis based in nMDS plots through the ANOSIM test using study areas and depth categories are shown in Figure 3A. These analyses indicate that the microbial community in the eDNA fractions from sediment samples was significantly different (p < 0.001) from the eRNA fractions with a clear separation of the assemblages with the depth (Supplementary Figure S5). Moreover, the Venn diagram (Figures 3B, 4A) showed that 302 ASVs at level family (66.2%) were shared among the two sampling zones, 73 and 107 ASVs were exclusively recorded in MB and OL, representing 13.7% and 20.1% of the microbial community.
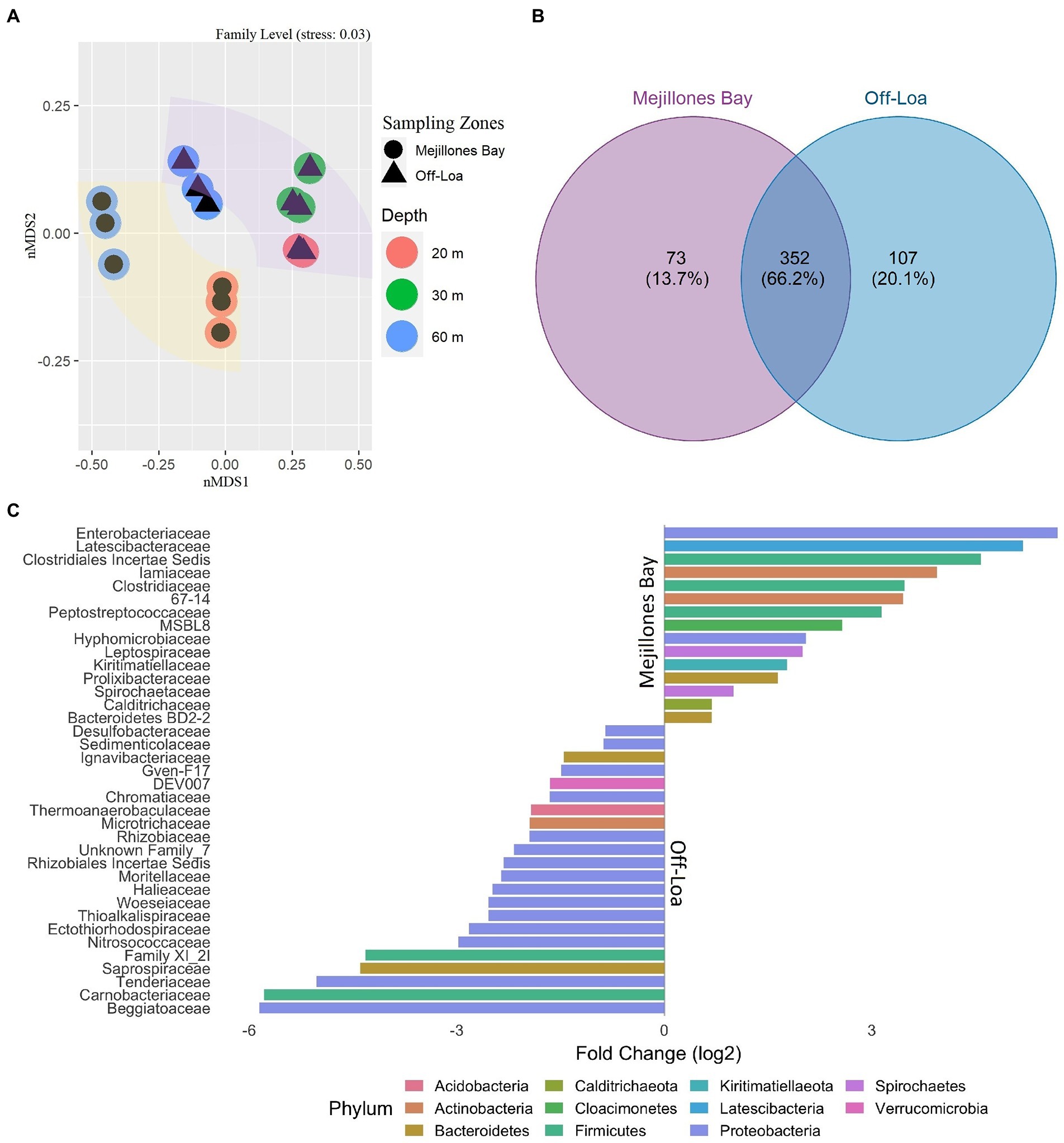
Figure 3. Microbial assemblage structure in Mejillones Bay and Off-Loa River zone. (A) Non-metric multidimensional scaling (nMDS) analysis of different microbial communities at different water-depths. (B) Venn diagram showing the degree of overlap of ASVs at the family level in the study zones. (C) DESeq2 analysis showing the family differential abundance based on sampling zones (positive fold change values more abundant in MB and negative fold change values more abundant in OL).
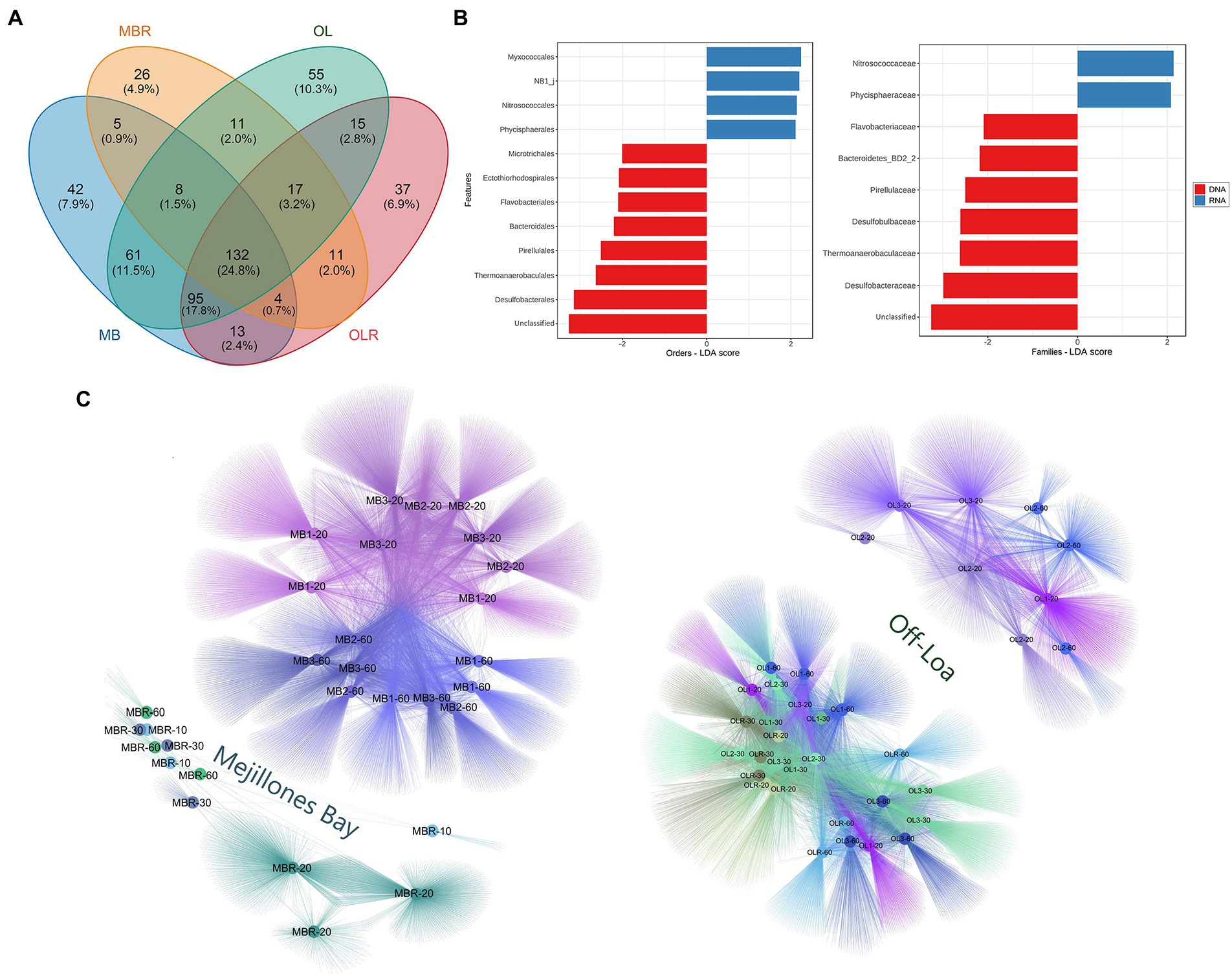
Figure 4. Microbial assemblage structure in Mejillones Bay and Off-Loa River zone. (A) Venn diagram with degree of overlap of families in eDNA (MB-OL) and eRNA samples (MBR-OLR). (B) Cladogram of linear discriminant analysis (LDA) score showing the significantly different orders and families in the DNA (red) and RNA (blue). (C) Microbial patterns of presence/absence of communities in eDNA and eRNA from the study zones.
Overall, the communities from the two sampling localities presented a 32.56% dissimilarity when compared to each other. DESeq2 analysis showed that the six major families responsible for the significant spatial variations in MB were Enterobacteriaceae, Latescibacteraceae, Clostridiales Incertae Sedis, Iamiaceae, Clostridiaceae, and Solirubrobacterales_67–14. Beggiatoaceae, Carnobacteriaceae, Tenderiaceae, Saprospiraceae, Family XI_2I, and Nitrosococcaceae were the corresponding representatives in OL (Figure 3C). Indeed, Nitrosococcaceae and Phycisphaeraceae were the families differentially active in RNA compared with DNA samples (Figure 4B). Such differences were also noticeable in the presence and absence network of microbial assemblage both eDNA and eRNA (Figure 4C).
Across the depths sampled, major shifts in the relative abundance of families were also observed; At 20 m in MB, the major differences consisted of Microtrichaceae, Unknown Family_7 (Gammaproteobacteria), DEV007, Rhizobiaceae and Nitrosococcaceae. In OL at the same depth, however, Saprospiraceae, Eel-36e1D6n (Myxococcales), Desulfomicrobiaceae, Tenderiaceae, and Marinifilaceae were differentially abundant (Supplementary Figure S6A). The families that characterize the differences at 60 m consisted of VHS-B3-70 (Myxococcales), Latescibacteraceae, Omnitrophaceae, Desulfuromonadaceae and Thermoflexaceae in MB. In OL at 60 m, AB-539-J10 (Dehalococcoidia), Beggiatoaceae, TG3 (Fibrobacterales), Carnobacteriaceae, Bacillaceae and UBA12409 (Babeliales) were responsible for the differences observed (Supplementary Figure S6B).
3.4. Linking microbial assemblages to metal fractioning and physico-chemical factors
RDA analysis of the benthic microbial community structure variability in the study area is shown in Figure 5. The first two axes of the RDA account for 93.0 and 87.7% of the total variation in MB and OL, respectively, with the RDA axis 1 accounting for 52.4% and 72.2% of the variation of each. The overlying environmental variables associated with TM and other physicochemical parameters indicates the relationship of factors with the greater impact in the benthic microbial community structuring variability.
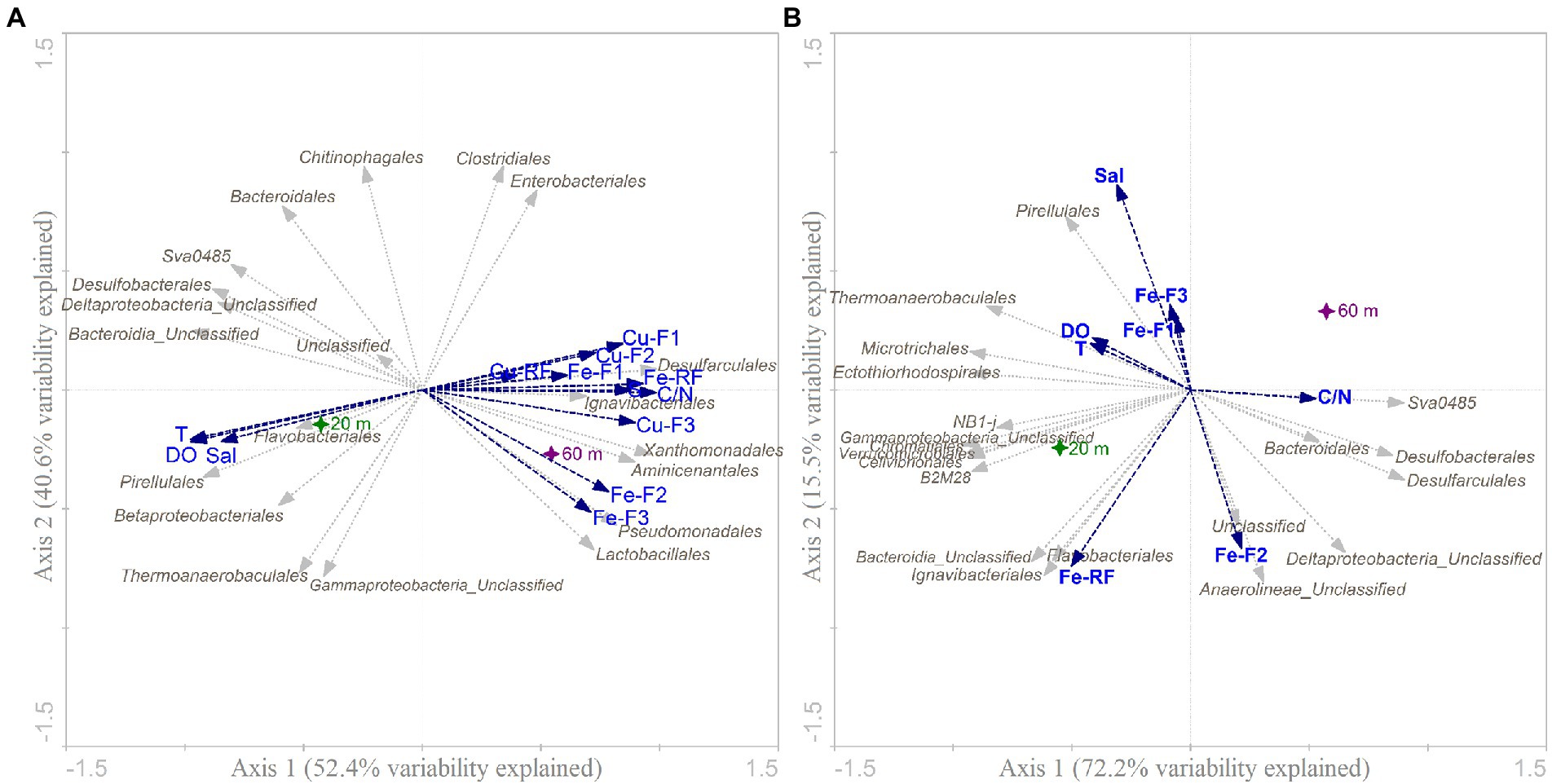
Figure 5. RDA ordination plot for the first two principal dimensions of the most significant physicochemical variables shaping benthic microbial assemblage composition and structure in the sediments of (A) Mejillones Bay and (B) Off-Loa River zone across water depth sampled. The symbols indicate the sampling sites, and the arrows indicate the environmental variables and their relative effects on the community.
In MB (Figure 5A), all TM fractions (F1, F2, F3, and RF) combined with the C/N ratio showed a positive relationship with the microbial assemblage at 60 m depth, mainly composed by members of the Desulfarculales, Ignavibacteriales, Xanthomonadales, Aminicenantales, Pseudomonadales, and Lactobacillales. In addition, temperature, DO, and salinity correlated negatively with Flavobacteriales, Pirellulales, Betaproteobacteriales, and Thermoanaerobaculales orders at 20 m.
Conversely, a different result was observed in OL where the metal fractioning was not clearly associated with the microbial communities across the depth sampled (Figure 5B). The ASVs were grouped with the shallow zone sampled (20 m). Salinity, DO, temperature, and the F1, F3 and RF of Fe fraction were associated with Flavobacteriales, Pirellulales, Thermoanaerobaculales, Microtrichales, Ectothiorhodospirales, and Ignavibacteriales, whereas Fe-F2 and C/N accounted for the distribution of Sva0485, Desulfarculales, Desulfobacterales, Anaerolineae, and Deltaproteobacteria Unclassified.
The Mantel test analysis, recorded no significant correlation between microbial structure and the measured environmental variables. However, a combination with PERMANOVA testing of significance highlighted that Cu-F1 (p = 0.021*), Cu-F2 (p = 0.01**), Cu-F3 (p = 0.005**), Fe-F3 (p = 0.037*), C/N ratio (p = 0.011*), temperature (p = 0.027*), TC (p = 0.003**), and TN (p = 0.005**), explained a high proportion of microbial community variation in both sampling locations. The relative importance of each physicochemical parameter and bioavailable metal(loid) to diversity indices (Chao1, Observed and Shannon) was also tested using PERMANOVA. Furthermore, Spearman’s rank-order correlations were determined between environmental variables and microbial communities at both locations, which then corroborated that microbial communities were significantly associated both positively and negatively with salinity, temperature, DO, TN, TS, TC, with Cu and Fe fractioning in their distinct variations (Supplementary Figure S7).
3.5. Co-occurrence networks and predicted metabolic potential of benthic microbial communities
Network constructed based on the co-occurrence patterns of microbes in surface sediments from MB and OL are shown in Figure 6. The analysis revealed that both study zones had unique co-occurrence patterns, composed by two large central module hubs. Nevertheless, OL had the highest values of modularity (i.e., higher complexity) and degree centrality (0.521 and 15.91, respectively) compared to the MB (0.461 and 15.21, respectively). Moreover, the average clustering coefficient, which was used to describe how well nodes related to their neighbors, indicated marginally lower modularity in MB (0.324) than in OL (0.338).
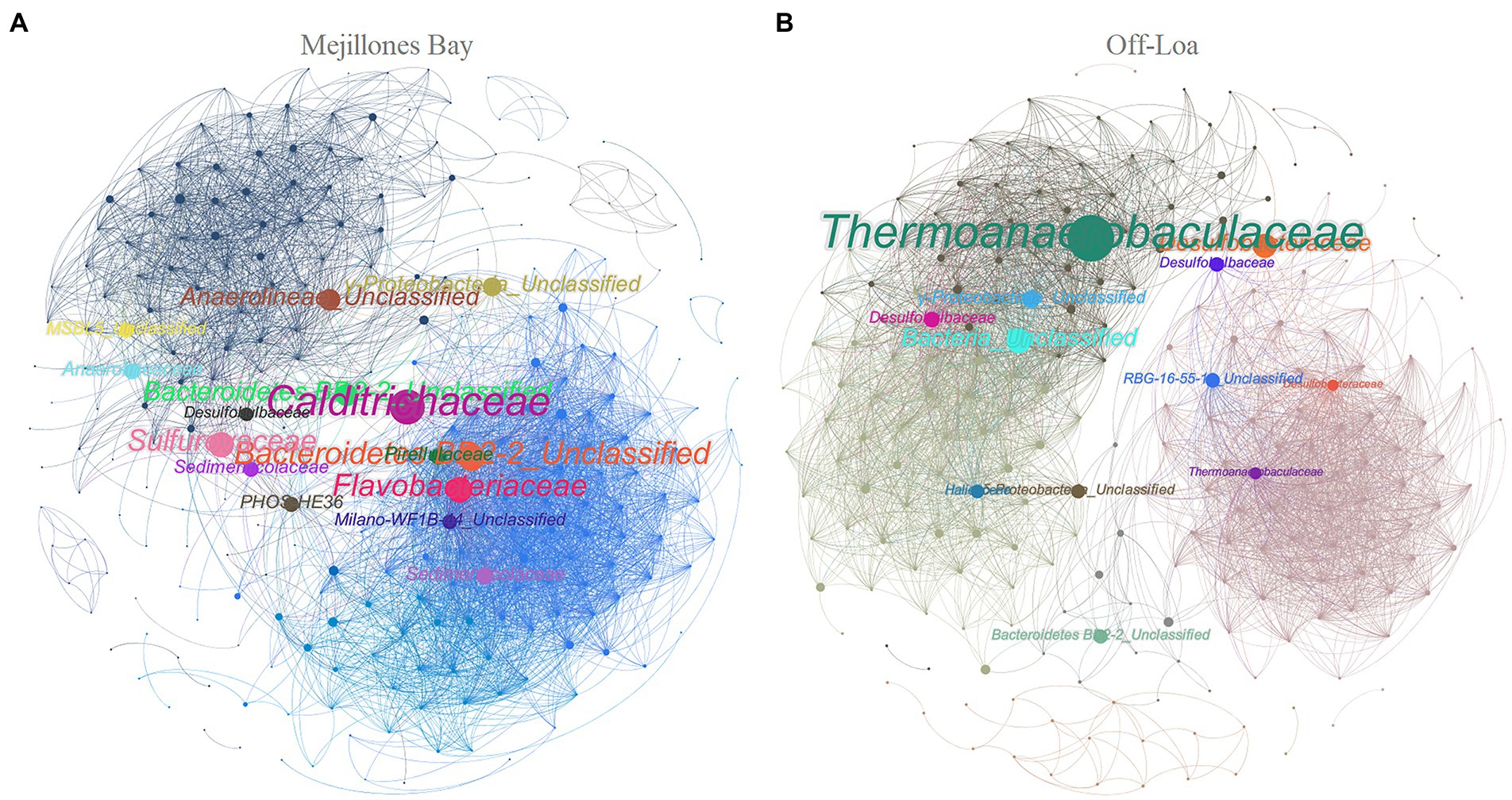
Figure 6. Sedimentary network of the microbial co-occurrence patterns in (A) Mejillones Bay and (B) Off-Loa River zone. Nodes (bubbles) represent ASVs at the family level and edges (lines) represent the strong (Spearman’s p > 0.75) and statistically significant (FDR adjusted p < 0.01) correlations. Module hubs were colored according to modularity class and the size of each node is proportional to the value of BCC.
The network indices (BC and CC), along with a large number of nodes (264) and edges (3,918) between taxa, suggest that the Calditrichaceae, Bacteroidetes BD2-2_Unclassified, Flavobacteriaceae, Sulfurovaceae, Anaerolineae_Unclassified, γ-Proteobacteria_Unclassified, Sedimenticolaceae, Pirellulaceae, Anaerolineaceae, PHOS-HE36, MSBL5_Unclassified, Milano-WF1B-44_Unclassified and Desulfobulbaceae members play a key role in the benthic environment of MB (Figure 6A). In OL zone, the network consisted of 223 nodes and 3,548 edges. Here, Thermoanaerobaculaceae, Bacteria_Unclassified, Desulfobacteraceae, γ-Proteobacteria_Unclassified, Desulfobulbaceae, RBG-16-55-12_Unclassified, Bacteroidetes BD2-2_Unclassified, δ-Proteobacteria_Unclassified and Halieaceae were significantly correlated with each other (Figure 6B).
PICRUST analyses considering KEGG level 3 categories and, a relative abundance greater than >1.0% of the total detected pathways was defined as a dominant pathway. With this criterion, 10 and 4 dominant pathways were stablished in MB and OL, respectively (Supplementary Figure S7). Most of the genes were involved in transporters (average 4.9%), unclassified/poorly characterized (3.6%), ABC transporters (2.9%), DNA repair and recombination proteins (2.4%), signal transduction (2.2%), Purine metabolism (1.9%), bacterial motility proteins (1.9%), Secretion system (1.8%), translation_ribosome (1.8%), and function unknown (1.7%). Among them, transporters, unclassified/poorly characterized, ABC transporter and DNA repair and recombination proteins were remarkably higher in MB (Supplementary Figure S8).
4. Discussion
The northern Chilean coast is especially sensitive to the oxygen decline and pollution increase due to both raw sewage input from urban areas and intensive maritime traffic closely linked with mining transport, leading to significant implications on the accumulation of pollutants in marine sediments (Valdés et al., 2014; Castillo et al., 2019). Which in turn could substantially affect microbial communities in the biogeochemical transformation of TMs, leading to modifications to ecosystem processes and functions (Glass et al., 2015; Coclet et al., 2021). To unravel the specific implications for microbial assemblages and their driving forces in the benthic environment, this work goes a step further using 16S rRNA gene sequencing at DNA and RNA (cDNA) molecular levels to comprehensively explore both structure and potential functions as well as provide a first insight into the prokaryotic ecology from two contrasting coastal zones of northern Chile, both of which are characterized by major upwelling or riverine inputs.
4.1. Integrated influence of the environmental heterogeneity-driven benthic microbial assemblages
To understand the patterns of microbial assemblages, determining the environmental driving factors is needed, which may play an important role in shaping the community (Tamburini et al., 2020). To the best of our knowledge, there are few studies that have addressed the cumulative effects of oxygen-deficient waters on geochemical metal fractioning in Chilean benthic coastal environments. Thus, this study makes an effort to understand the distributions of Cu and Fe fractions from marine sediments, which is essential in determining the mobility and potential toxicity of TMs.
Though the variation in fraction distributions was observed, recording higher concentrations the MB than in OL, the RF (considered an inert, immobile metal phase) was dominant in both TMs of the sampled zones. Chakraborty et al. (2016) reported that concentrations of Cu associated with F3 and F4 in the Arabian Sea sediments were higher than their concentrations in the F1 and F2. As such, their actual bioavailability is likely to be rather low (chemically stable) and less harmful to the quality of the benthic environment (Cyriac et al., 2021). In addition, the lability of Cu in sediment gradually decreased with decreasing DO levels of the bottom water, which in turn could increase their association with sedimentary OM (Sander et al., 2015). Therefore, we considered that the lower hydrodynamics of MB likely explained the higher levels of TMs when compared to OL, which is physically closer to the discharge of the Loa River.
Besaury et al. (2013) also reported decrease in the ratio of available/total copper concentration alongside an increase of total organic carbon in marine sediments of Chañaral Bay in northern Chile. Thus, it is possible that the low-oxygen levels recorded coupled with the high quantity of OM previously reported both MB and OL zones (Isla et al., 2010; Castillo et al., 2019) increase the geochemical sequestration of TMs and therefore controls bioavailable concentrations due to formation of non-labile complexes in the sediments (Strom et al., 2011; Cyriac et al., 2021). Nevertheless, naturally occurring processes linked to changes in pH, salinity, decomposition and mineralization can favor the release of mobile metal fractions from the sediment to the overlying water, facilitating bioaccumulation processes within the benthic food webs (Chakraborty et al., 2016). In this context, regular assessment to control metal pollution in the coastal environment is recommended (Cyriac et al., 2021). Moreover, marine sediments under high anthropogenic pressure constantly increase HM concentrations, which may lead to significant additional pollution (Jacquiod et al., 2018; Jroundi et al., 2020).
The strategy of using eDNA and eRNA in parallel, revealed the previously unappreciated potential that both active and total microbial communities displayed contrasting patterns and respond differently to environmental heterogeneity gradient. In MB, both Cu and Fe fractions induced significant microbial communities shifts of Desulfarculales, Ignavibacteriales, Xanthomonadales, Aminicenantales, Pseudomonales, and Lactobacillales, whereas Pirellulales, Betaproteobacteriales, and Thermoanaerobaculales were closely related to changes in DO, salinity, and temperature. In contrast, only these two latter factors showed a significant correlation with Pirellulales and Thermoanaerobaculales in OL, implying that multiple interacting factors in similar environments could have a specific response at the microbial level. This is consistent with previous studies that have demonstrated the clear structuring effect of DO, salinity and temperature in different environments, therefore explaining a significant portion of global microbial community distribution patterns (Zinger et al., 2011; Rodríguez et al., 2018; Hoshino et al., 2020). In the northwestern part of the Gulf of Mexico, the observed pattern was driven primarily by environmental gradients associated with local oceanographic parameters closely linked to water column depth, nutrients, temperature, salinity, OM, DO, and HMs (Jiménez et al., 2018; Rodríguez et al., 2021). Such spatially structured environmental gradients allow micro niches development and promote the coexistence of microbial taxa that are considered regulators of ocean ecosystem services (Fortunato et al., 2012; Cavicchioli et al., 2019).
While environmental gradients clearly affect microbial abundance and activity, much of the spatial variation in microbial activity is linked to pollutant distribution and mobility (Quero et al., 2015; Jacquiod et al., 2018). In this study, Spearman’s rank correlation and RDA analyses, suggested that MB’s microbial structuration seemed to be mainly driven by metal fractioning of Cu and Fe, whereas the major environmental determinants in OL were Fe fractioning, though statistically insignificant (Figure 5; Supplementary Figure S6). It is noteworthy that Coclet et al. (2021) found that Cu, Mn, and Zn modulated the predicted functional profiles of biofilm communities in an anthropized coastal area. These findings further support previous observations of the structural role of metals in other coastal microbial patterns (Jacquiod et al., 2018; Coclet et al., 2019), and even complimented via network analysis in different aquatic environments.
Regarding the TMs effects observed in OL, the high water-sediment flow from the Loa River during altiplanic rain could have resulted in an increase in the mass effect and homogenization of sedimentary environmental conditions on the coastal, resulting in decrease in the differential of microbial response. Overall, quantitative evidence supported that the majority of each locale’s (MB and OL) taxa is strongly associated with specific environmental features, suggesting that spatial heterogeneity linked to specific variables of study areas could affect the microbial community assemblage (Zhang et al., 2021). A possible explanation is that the different microbial assemblages have developed distinct mechanisms to adapt to environmental heterogeneity of study zones, driving stabilization of the functional structure and maintenance of ecological services (Zinger et al., 2011; Cavicchioli et al., 2019; Hoshino et al., 2020).
Indeed, the major functional pathways at both zones were involved in transporters, BC transporters, DNA repair, and recombination proteins, and signal transduction, which were previously found in an aquatic system affected by acid mine drainage. Such a finding highlights that transporters are involved in metal tolerance and detoxification (Coclet et al., 2021), particularly multiple efflux systems that allow microbes to sense and respond to variable environmental conditions and adapt to the environment (Chen et al., 2015). These results are fundamental value because understanding how different local regimes of environmental variability modify the spatial structure of benthic communities may provide a starting point to consider how communities could respond to future climate changes and metal pollution scenarios in reduced oxygen environments (Cavicchioli et al., 2019).
However, these results must be interpreted carefully because it should be recognized that, while microbial community structure could be influenced by variables measured within this study, additional variables that were not measured (e.g., nitrogen, sulfur content) could also affect microbial community structure (Farías et al., 2004; Hoshino et al., 2020). Therefore, future work should aim to employ an integrated metatranscriptomic and metagenomic approach to functional inferences. The combination of these methods can concurrently investigate the transcriptional patterns of specific microbes, revealing their overall functional and metabolic activities within the whole community (Stewart et al., 2012).
4.2. Spatial co-occurrence patterns of benthic microbial assemblages
The benthic microbial assemblages from two contrasting coastal study zones revealed strong spatial variation patterns with a large fluctuation in microbial communities in terms of taxonomic composition. This contributed to the keystone taxa groups that formed, distinguishing two strong module hubs from each study area. The taxa that are defined as module hubs and connectors of the network are regarded as keystone taxa due to their role in maintaining network structures, and they may play a role in maintaining ecosystem stability (Cui et al., 2019).
Overall, the keystone taxa we observed in the benthic microbial community may expand the range of possible metabolic roles into sediments, elucidating their ecological niches. A clear example of this was the identification of Calditrichaceae (phylum Calditrichaeota) in the sediments of MB, which probably pointed to a stronger line of evidence for the importance of this family in marine sediments, as it has been previously reported as an unrecognized but significant player in geochemical element cycling that is able to degrade detrital proteins (Marshall et al., 2017). Many members of the family Flavobacteriaceae have been isolated from marine environments and also have the capacity to degrade high-molecular-weight organic polymers, including cellulose, chitosan, and proteins (Yang et al., 2020). Moreover, this particular family has the ability to tolerate toxic effects of certain metals (Quero et al., 2015; Tamburini et al., 2020).
The reconstruction of metagenome-assembled genomes in the microbial-mediated sulfur cycle determined that Sedimenticolaceae possess near-complete, encoded pathways for denitrification (Vavourakis et al., 2019). Indeed, along with Desulfobulbaceae, Sedimenticolaceae was the most abundant family reported in association with marine sediments from Chilean salmonid farms (Miranda et al., 2020). Furthermore, some of these families including Desulfobacteraceae were observed in the permanent anoxic/hypoxic sediment in the deepest part of the Baltic Sea, which highlighted active functional traits related to OM degradation, reduction of sulfate, and methanogenesis (Broman et al., 2017).
Network analysis also facilitated further identification of Thermoanaerobaculaceae as the major representative of OL, which have been previously described as thermophilic in the sediments of Boka Kotorska Bay (Montenegro, Croatia; Jokanović et al., 2021). Interestingly, some prominent keystone taxa in our study belonged to the bacteria rare subcommunity, which indicated that these microbes may compose the hidden backbone of microbial communities. Furthermore, the beta-diversity analysis showed a clear distinction between the microbial assemblages in MB and OL with significantly higher richness (Chao1; p < 0.05) in the DNA samples compared to the RNA samples across sampled water-depths.
In accordance with discriminant analysis for the spatial variation between the sampled zones revealed that Beggiatoaceae, Carnobacteriaceae, Tenderiaceae, Saprospiraceae, Family XI_2I and Nitrosococcaceae were representative of OL. For MB, Enterobacteriaceae, Corynebacteriaceae, Latescibacteraceae, Clostridiales Incertae Sedis, Iamiaceae, Clostridiaceae and Solirubrobacterales_67-14 were the families responsible for the observed changes, indicating the dispersal of fecal bacteria from the anthropogenically affected coastal area and therefore representing a reservoir of pathogenicity potentially harmful to human health (Nogales et al., 2011). Indeed, feco–oral route is the most important route of transmission especially for resistant pathogens of the family Enterobacteriaceae (Aslam et al., 2018).
Based on several active bacteria detected, such as Burkholderia, Campylobacter, Clostridium, Enterobacter and Pseudomonas groups, which are resistant to various antibiotics, the influence of MB environment is also of concern Mainly due to pose a serious threat to global human, animal, and environmental health, a concern recognized by numerous important organizations such as the World Health Organization (Aslam et al., 2018; Miranda et al., 2018). However, the above differential assemblage compositions among study zones also suggest the cooperation between all organisms, potentially translating into success of the whole ecosystem (Cardoso et al., 2017), because they reveal the performed functions that are either similar or complementary to each other and may co-occur due to shared and preferred environmental conditions (Jroundi et al., 2020).
Previous studies that have analyzed eRNA in parallel with eDNA also reported significantly higher values in the overall community. What is more, DNA sampling (living, dead, eaten, and recently moved through the sediments) is logistically simpler than sampling RNA or the active community, which usually contains fewer communities (review Birrer et al., 2018; Pawlowski et al., 2022 for more details); it should be noted that this is not always the case because eRNA is usually more sensitive, particularly for biosecurity applications, where detecting viable non-indigenous organisms is critical. Therefore, highlighting new opportunities for integrating functional genomics into environmental monitoring programs that may inform management actions in order to represent real-time ecological change and impacts could serve as a predictive tool for identifying at-risk habitats (Bowers et al., 2021), especially as sequencing costs decline. Therefore, the findings of this study help to lay the groundwork for scoping eRNA in parallel with eDNA applied to significantly enhance the spatial and functional understanding of real-time microbial assemblages and, in turn, to increase the acuity of biomonitoring programs key to responding to immediate management needs for the marine environment.
Campbell and Kirchman (2012) explain the decoupling between abundance and microbial activity as a result of grazing and virus lysis. Interestingly, sediment chemistry can affect the efficiency of nucleic acid extraction and PCR amplification, especially in polluted sediments like those of MB (Valdés et al., 2005; Valdés, 2012; Castillo et al., 2019), where organic and inorganic compounds bind to the sediment (Sander et al., 2015). It is also important to note that the potential bias in the differential nucleic acids extraction and amplification could generate an underestimation of some groups diversity (Cardoso et al., 2017; Birrer et al., 2018). For this reason, the libraries were normalized by an equal number of sequences before performing calculations or statistical tests.
When exploring the spatial co-occurrence patterns of microbial assemblages between study zones, two distinct module hubs (regions densely connected) were clearly observed in both MB and OL networks, indicating that habitat significantly influenced the microbial co-occurrence networks (Cui et al., 2019; Felipe-Lucia et al., 2020). In fact, the habitat preference of microorganisms may play a vital role in determining their co-occurrence patterns (Chaffron et al., 2010; Jroundi et al., 2020). It was found that the microbial network in the OL benthic zone showed higher complexity (given the value of modularity) compared to the MB (as described in the results). The lower modularity network in MB, could mean that its communities are more vulnerable and sensitive to various disturbances, demonstrating that, in high-stress environments, their communities could break down (Banerjee et al., 2018; Philippot et al., 2021).
That said, the high modularity in OL mainly indicates diverse micro-niches and structured microbial communities (Zhang et al., 2021), characteristic of large complex systems that imply a higher ecological degree of stability under perturbations. This improves efficiency in transferring information, energy, and resources of microbial communities, regard to sediment nutrient conversion and pollutant biodegradation (Santolini and Barabási, 2018; Philippot et al., 2021). One explanation for the high spatial connectivity and the selection of the microbe pool in the coastal area surrounding the OL may also be attributed to the Loa River, which acts as a true green/life corridor that runs across the hyper-arid core of the Atacama Desert also known as the dry limit for life on Earth, helping to establish specific networks (Zárate et al., 2020). In this way, the input may support a distinct set of microbial processes that remain unstudied (Fortunato et al., 2012; Fazi et al., 2020; Jroundi et al., 2020).
For instance, in the coastal wetlands of both the Yellow River Delta (China) and the northern Adriatic Sea (Italy), it has been emphasized that river input altered microbial structure patterns (Fazi et al., 2020; Zhao et al., 2020). Thus, the results of this study are consistent with the environmental microbiomes that highlight associations between prokaryotic consortia, representing potential metabolic interactions with habitat transition (Chaffron et al., 2010; Petro et al., 2017) and local heterogeneity that is usually characterized by highly productive systems. In addition, in the Yap Trench (Western Pacific Ocean), the formation of multiple modules that respond to the environmental heterogeneity in sediments was also reported, providing evidence for ecological niche differentiation (Zhang et al., 2021). This study also highlights the relevance of the 66.2% of the resident microbial communities that were shared in the study areas, suggesting that they harbor a persistent functional pool of ecological potential that may be temporally consistent and regionally transferable (Clark et al., 2020). Therefore, it is possible to propose that these common taxa could be more useful for the development of coastal health assessment and biological monitoring tools (Sun et al., 2013; Quero et al., 2015). It is crucial to clarify the processes underlying changes microbial communities and ecosystems in response to environmental perturbations (Nogales et al., 2011; Cavicchioli et al., 2019).
5. Conclusion
This study demonstrated that microbial assemblages from two contrasting benthic coastal zones in northern Chile were highly diverse and strongly regulated by a combination of different environmental factors including Cu, Fe, dissolved oxygen, salinity, and temperature, Such conditions led to heterogeneous distribution with rare communities that contributed to keystone taxa in networks similar to large complex systems, which potentially serve a key role in ecosystem function, though the temporal dynamics of which requires further description and understanding. Additionally, we highlight the possibility that the keystone taxa identified could serve as a basis for future monitoring programs of contaminated environments. This is especially true for the microbial processes occurring in the sediment of Mejillones Bay, whose bacteria could play an important role in the region exchange of antibiotic resistance. Therefore, the eRNA in parallel with eDNA approach utilized here has the capacity to significantly enhance the spatial and functional understanding of real-time microbial assemblages and, in turn, would have the potential to increase the acuity of biomonitoring programs that are key to addressing the global concern of antimicrobial resistance from the coastal environment. Hence, future work should aim to further experimental validation and cultivation-based approaches will be required to identify consistent responses of such indicator taxa, employing an integrated metagenomic and metatranscriptomic approach to reveal functional and metabolic activities within the whole microbial benthic community.
Data availability statement
The datasets presented in this study can be found in online repositories. The names of the repository/repositories and accession number(s) can be found in the article/Supplementary material.
Author contributions
AZ, CD, and JV proposed and designed the study. AZ organized and performed field sampling. SV and AZ analyzed the samples. GI and JU performed the bioinformatic analyses. VM, AC, and AZ analyzed and interpreted the results and participated in manuscript writing. All authors contributed to the article and approved the submitted version.
Funding
This work was supported by a Scientific and Technological Research Scholarship for Doctoral Studies (CONICYT-PCHA/Doctorado Nacional/2015–21150407) and Centro de Bioingeniería y Biotecnología (CeBiB) FB0001. The funders had no role in study design, data collection and analysis, decision to publish, or preparation of the manuscript.
Acknowledgments
We would like to thank Katalin Plummer for editing the English version of the manuscript and Ana Garcia-Cegarra for the study zones map construction. Special thanks also go to Luis Aguilar for diving and sampling assistance. We also thank the National Commission for Scientific and Technological Research (FONDECYT 1181773; Center CeBiB FB 0001) of Cristina Dorador.
Conflict of interest
The authors declare that the research was conducted in the absence of any commercial or financial relationships that could be construed as a potential conflict of interest.
Publisher’s note
All claims expressed in this article are solely those of the authors and do not necessarily represent those of their affiliated organizations, or those of the publisher, the editors and the reviewers. Any product that may be evaluated in this article, or claim that may be made by its manufacturer, is not guaranteed or endorsed by the publisher.
Supplementary material
The Supplementary material for this article can be found online at: https://www.frontiersin.org/articles/10.3389/fmicb.2022.1020491/full#supplementary-material
References
Aslam, B., Wang, W., Arshad, M. I., Khurshid, M., Muzammil, S., Rasool, M. H., et al. (2018). Antibiotic resistance: a rundown of a global crisis. Infect. Drug Resist. 11, 1645–1658. doi: 10.2147/IDR.S173867
Banerjee, S., Schlaeppi, K., and van der Heijden, M. G. A. (2018). Keystone taxa as drivers of microbiome structure and functioning. Nat. Rev. Microbiol. 16, 567–576. doi: 10.1038/s41579-018-0024-1
Bastian, M., and Heymann, S. (2009). Gephi: An open source software for exploring and manipulating networks. ICWSM 8, 361–362.
Besaury, L., Marty, F., Buquet, S., Mesnage, V., Muyzer, G., and Quillet, L. (2013). Culture-dependent and independent studies of microbial diversity in highly copper-contaminated Chilean marine sediments. Microb. Ecol. 65, 311–324. doi: 10.1007/s00248-012-0120-0
Birrer, S. C., Dafforn, K. A., Simpson, S. L., Kelaher, B. P., Potts, J., Scanes, P., et al. (2018). Interactive effects of multiple stressors revealed by sequencing total (DNA) and active (RNA) components of experimental sediment microbial communities. Sci. Total Environ. 637-638, 1383–1394. doi: 10.1016/j.scitotenv.2018.05.065
Bowers, H. A., Pochon, X., Ammon, U., Gemmell, N., Stanton, J. L., Jeunen, G., et al. (2021). Towards the optimization of eDNA/eRNA sampling Technologies for Marine Biosecurity Surveillance. Water 13, 1–24. doi: 10.3390/w13081113
Brandes, U., Raab, J., and Wagner, D. (2001). Exploratory network visualization simultaneous display of actor status and connections. J. Soc. Struct. 2, 1–28.
Broman, E., Sachpazidou, V., Pinhassi, J., and Dopson, M. (2017). Oxygenation of hypoxic coastal Baltic Sea sediments impacts on chemistry, microbial community composition, and metabolism. Front. Microbiol. 8, 1–15. doi: 10.3389/fmicb.2017.02453
Callahan, B. J., McMurdie, P. J., Rosen, M. J., Han, A. W., Johnson, A. J. A., and Holmes, S. P. (2016). DADA2: high-resolution sample inference from Illumina amplicon data. Nat. Methods 13, 581–583. doi: 10.1038/nmeth.3869
Campbell, B. J., and Kirchman, D. L. (2012). Bacterial diversity, community structure and potential growth rates along an estuarine salinity gradient. ISME J. 7, 210–220. doi: 10.1038/ismej.2012.93
Caporaso, J. G., Lauber, C. L., Walters, W. A., Berg-lyons, D., Lozupone, C. A., Turnbaugh, P. J., et al. (2011). Global patterns of 16S rRNA diversity at a depth of millions of sequences per sample. Proc. Natl. Acad. Sci. 108, 4516–4522. doi: 10.1073/pnas.1000080107
Cardoso, D. C., Sandionigi, A., Cretoiu, M. S., Casiraghi, M., Stal, L., and Bolhuis, H. (2017). Comparison of the active and resident community of a coastal microbial mat. Sci. Rep. 7, 2969–2910. doi: 10.1038/s41598-017-03095-z
Castillo, A., Valdés, J., Sifeddine, A., Vega, S. E., Díaz-Ochoa, J., and Marambio, Y. (2019). Evaluation of redox-sensitive metals in marine surface sediments influenced by the oxygen minimum zone of the Humboldt current system, Northern Chile. Int. J. Sediment Res. 34, 178–190. doi: 10.1016/j.ijsrc.2018.08.005
Cavicchioli, R., Bakken, L. R., Baylis, M., Foreman, C. M., Karl, D. M., Koskella, B., et al. (2019). Scientists’ warning to humanity: microorganisms and climate change. Nat. Rev. Microbiol. 17, 569–586. doi: 10.1038/s41579-019-0222-5
Chaffron, S., Rehrauer, H., Pernthaler, J., and Von Mering, C. (2010). A global network of coexisting microbes from environmental and whole-genome sequence data. Genome Res. 20, 947–959. doi: 10.1101/gr.104521.109
Chakraborty, P., Chakraborty, S., Jayachandran, S., Madan, R., Sarkar, A., Linsy, P., et al. (2016). Effects of bottom water dissolved oxygen variability on copper and lead fractionation in the sediments across the oxygen minimum zone, western continental margin of India. Sci. Total Environ. 566-567, 1052–1061. doi: 10.1016/j.scitotenv.2016.05.125
Chen, L. X., Hu, M., Huang, L. N., Hua, Z. S., Kuang, J. L., Li, S. J., et al. (2015). Comparative metagenomic and metatranscriptomic analyses of microbial communities in acid mine drainage. ISME J. 9, 1579–1592. doi: 10.1038/ismej.2014.245
Clark, D. E., Pilditch, C. A., Pearman, J. K., Ellis, J. I., and Zaiko, A. (2020). Environmental DNA metabarcoding reveals estuarine benthic community response to nutrient enrichment – evidence from an in-situ experiment. Environ. Pollut. 267, 115472–115412. doi: 10.1016/j.envpol.2020.115472
Coclet, C., Garnier, C., D’Onofrio, S., Durrieu, G., Pasero, E., Le Poupon, C., et al. (2021). Trace metal contamination impacts predicted functions more than structure of marine prokaryotic biofilm communities in an Anthropized coastal area. Front. Microbiol. 12, 1–16. doi: 10.3389/fmicb.2021.589948
Coclet, C., Garnier, C., Durrieu, G., Omanović, D., D’Onofrio, S., Le Poupon, C., et al. (2019). Changes in bacterioplankton communities resulting from direct and indirect interactions with trace metal gradients in an urbanized marine coastal area. Front. Microbiol. 10:257. doi: 10.3389/fmicb.2019.00257
Cui, Y., Chun, S. J., Baek, S. H., Lee, M., Kim, Y., Lee, H. G., et al. (2019). The water depth-dependent co-occurrence patterns of marine bacteria in shallow and dynamic southern coast. Korea. Sci. Rep. 9, 1–13. doi: 10.1038/s41598-019-45512-5
Cyriac, M., Gireeshkumar, T. R., Furtado, C. M., Fathin, K. P. F., Shameem, K., Shaik, A., et al. (2021). Distribution, contamination status and bioavailability of trace metals in surface sediments along the southwest coast of India. Mar. Pollut. Bull. 164, 112042–112013. doi: 10.1016/j.marpolbul.2021.112042
Farías, L., Graco, M., and Ulloa, O. (2004). Temporal variability of nitrogen cycling in continental-shelf sediments of the upwelling ecosystem off Central Chile. Deep. Res. Part II Top. Stud. Oceanogr. 51, 2491–2505. doi: 10.1016/j.dsr2.2004.07.029
Fazi, S., Baldassarre, L., Cassin, D., Quero, G. M., Pizzetti, I., Cibic, T., et al. (2020). Prokaryotic community composition and distribution in coastal sediments following a Po river flood event (northern Adriatic Sea, Italy). Estuar. Coast. Shelf Sci. 233, 106547–106512. doi: 10.1016/j.ecss.2019.106547
Felipe-Lucia, M. R., Soliveres, S., Penone, C., Fischer, M., Ammer, C., Boch, S., et al. (2020). Land-use intensity alters networks between biodiversity, ecosystem functions, and services. Proc. Natl. Acad. Sci. U. S. A. 117, 28140–28149. doi: 10.1073/pnas.2016210117
Flores-Aqueveque, V., Vargas, G., Alfaro, S., Caquineau, S., and Valdes, J. (2014). Assessing the origin and variability of Eolian lithic material for high-resolution Paleoceanographic reconstructions off northern Chile (23 °S). J. Sediment. Res. 84, 897–909. doi: 10.2110/jsr.2014.72
Fortunato, C. S., Herfort, L., Zuber, P., Baptista, A. M., and Crump, B. C. (2012). Spatial variability overwhelms seasonal patterns in bacterioplankton communities across a river to ocean gradient. ISME J. 6, 554–563. doi: 10.1038/ismej.2011.135
Fruchterman, T. M. J., and Reingold, E. M. (1991). Graph drawing by force-directed placement. Software—Practice Exp. 21, 1129–1164.
Glass, J. B., Kretz, C. B., Ganesh, S., Ranjan, P., Seston, S. L., Buck, K. N., et al. (2015). Meta-omic signatures of microbial metal and nitrogen cycling in marine oxygen minimum zones. Front. Microbiol. 6, 1–13. doi: 10.3389/fmicb.2015.00998
Heberle, H., Meirelles, V. G., da Silva, F. R., Telles, J. I., and Minghim, R. (2015). InteractiVenn: A web-based tool for the analysis of sets through Venn diagrams. BMC Bioinformatics 16:169. doi: 10.1186/s12859-015-0611-3
Hernando-Amado, S., Coque, T. M., Baquero, F., and Martínez, J. L. (2019). Defining and combating antibiotic resistance from one health and Global Health perspectives. Nat. Microbiol. 4, 1432–1442. doi: 10.1038/s41564-019-0503-9
Herrera, L., and Escribano, R. (2006). Factors structuring the phytoplankton community in the upwelling site off El Loa River in northern Chile. J. Mar. Syst. 61, 13–38. doi: 10.1016/j.jmarsys.2005.11.010
Hoshino, T., Doi, H., Uramoto, G. I., Wörmer, L., Adhikari, R. R., Xiao, N., et al. (2020). Global diversity of microbial communities in marine sediment. Proc. Natl. Acad. Sci. 117, 27587–27597. doi: 10.1073/pnas.1919139117
Isla, E., Homs, P., Sañé, E., Escribano, R., Claramunt, G., and Teixidó, N. (2010). Biochemical composition of seston in two upwelling sites within the Humboldt current system (21 °S to 23 °S): summer conditions. J. Mar. Syst. 82, 61–71. doi: 10.1016/j.jmarsys.2010.03.004
Jacquiod, S., Cyriaque, V., Riber, L., Al-soud, W. A., Gillan, D. C., Wattiez, R., et al. (2018). Long-term industrial metal contamination unexpectedly shaped diversity and activity response of sediment microbiome. J. Hazard. Mater. 344, 299–307. doi: 10.1016/j.jhazmat.2017.09.046
Jayakumar, A., Al-Rshaidat, M. M. D., Ward, B. B., and Mulholland, M. R. (2012). Diversity, distribution, and expression of diazotroph nifH genes in oxygen-deficient waters of the Arabian Sea. FEMS Microbiol. Ecol. 82, 597–606. doi: 10.1111/j.1574-6941.2012.01430.x
Jiménez, M. F. S.-S., Cerqueda-García, D., Montero-Muñoz, J. L., Aguirre-Macedo, M. L., and García-Maldonado, J. Q. (2018). Assessment of the bacterial community structure in shallow and deep sediments of the Perdido Fold Belt region in the Gulf of Mexico. PeerJ 6:e5583. doi: 10.7717/peerj.5583
Jokanović, S., Kajan, K., Perović, S., Ivanić, M., Mačić, V., and Orlić, S. (2021). Anthropogenic influence on the environmental health along Montenegro coast based on the bacterial and chemical. Environ. Pollut. 271, 1–13. doi: 10.1016/j.envpol.2020.116383
Jroundi, F., Martinez-Ruiz, F., Merroun, M. L., and Gonzalez-Muñoz, M. T. (2020). Exploring bacterial community composition in Mediterranean deep-sea sediments and their role in heavy metal accumulation. Sci. Total Environ. 712, 135660–135619. doi: 10.1016/j.scitotenv.2019.135660
Koho, K. A., Nierop, K. G. J., Moodley, L., Middelburg, J. J., Pozzato, L., Soetaert, K., et al. (2013). Microbial bioavailability regulates organic matter preservation in marine sediments. Biogeosciences 10, 1131–1141. doi: 10.5194/bg-10-1131-2013
Langille, M. G. I., Zaneveld, J., Caporaso, J. G., McDonald, D., Knights, D., Reyes, J. A., et al. (2013). Predictive functional profiling of microbial communities using 16S rRNA marker gene sequences. Nat. Biotechnol. 31, 814–821. doi: 10.1038/nbt.2676
Love, M. I., Huber, W., and Anders, S. (2014). Moderated estimation of fold change and dispersion for RNA-seq data with DESeq2. Genome Biol. 15, 550–521. doi: 10.1186/s13059-014-0550-8
Marín, V. H., Delgado, L. E., and Escribano, R. (2003). Upwelling shadows at Mejillones Bay (northern Chilean coast): a remote sensing in situ analysis. Investig. Mar. 31, 47–55. doi: 10.4067/S0717-71782003000200005
Marshall, I. P. G., Starnawski, P., Cupit, C., Fernández Cáceres, E., Ettema, T. J. G., Schramm, A., et al. (2017). The novel bacterial phylum Calditrichaeota is diverse, widespread and abundant in marine sediments and has the capacity to degrade detrital proteins. Environ. Microbiol. Rep. 9, 397–403. doi: 10.1111/1758-2229.12544
McMurdie, P. J., and Holmes, S. (2013). Phyloseq: an R package for reproducible interactive analysis and graphics of microbiome census data. PLoS One 8, 1–11. doi: 10.1371/journal.pone.0061217
Miranda, R. M., Aguila-Torres, P., Aranda, C. P., Maldonado, J., and Casado, A. (2020). Taxonomy and diversity of bacterial communities associated with marine sediments from Chilean salmonid farms. Aquacult. Res. 52, 1605–1620. doi: 10.1111/are.15014
Miranda, C. D., Godoy, F. A., and Lee, M. R. (2018). Current status of the use of antibiotics and the antimicrobial resistance in the chilean salmon farms. Front. Microbiol. 9, 1–14. doi: 10.3389/fmicb.2018.01284
Molina, V., Belmar, L., Levipan, H. A., Ramírez-Flandes, S., Anguita, C., Galán, A., et al. (2020). Spatiotemporal distribution of key pelagic microbes in a seasonal oxygen-deficient coastal upwelling system of the eastern South Pacific Ocean. Front. Mar. Sci. 7, 1–17. doi: 10.3389/fmars.2020.561597
Molina, V., Belmar, L., and Ulloa, O. (2010). High diversity of ammonia-oxidizing archaea in permanent and seasonal oxygen-deficient waters of the eastern South Pacific. Environ. Microbiol. 12, 2450–2465. doi: 10.1111/j.1462-2920.2010.02218.x
Molina, V., Cornejo-D’Ottone, M., Soto, E. H., Quiroga, E., Alarcón, G., Silva, D., et al. (2021). Biogeochemical responses and seasonal dynamics of the benthic boundary layer microbial communities during the El Niño 2015 in an eastern boundary upwelling system. Water 13, 1–18. doi: 10.3390/w13020180
Newman, M. (2003). The structure and function of complex networks. SIAM Rev. 45, 167–256. doi: 10.1137/S003614450342480
Nogales, B., Lanfranconi, M. P., Piña-Villalonga, J. M., and Bosch, R. (2011). Anthropogenic perturbations in marine microbial communities. FEMS Microbiol. Rev. 35, 275–298. doi: 10.1111/j.1574-6976.2010.00248.x
Oksanen, J. A. R. I., Blanchet, F. G., Friendly, M., Kindt, R., Legendre, P., McGlinn, D., et al. (2020).Community Ecology Package. R Packag. version 2, 5–7. Available at: https://cran.r-project.org/web/packages/vegan/index.html
Palma, W., Escribano, R., and Rosales, S. A. (2006). Modeling study of seasonal and inter-annual variability of circulation in the coastal upwelling site of the El Loa River off northern Chile. Estuar. Coast. Shelf Sci. 67, 93–107. doi: 10.1016/j.ecss.2005.11.011
Pardo, R., Helena, B. A., Cazurro, C., Guerra, C., Debán, L., Guerra, C. M., et al. (2004). Application of two- and three-way principal component analysis to the interpretation of chemical fractionation results obtained by the use of the B.C.R. procedure. Anal. Chim. Acta 523, 125–132. doi: 10.1016/j.aca.2004.07.015
Pawlowski, J., Bruce, K., Panksep, K., Aguirre, F. I., Amalfitano, S., Apothéloz-Perret-Gentil, L., et al. (2022). Environmental DNA metabarcoding for benthic monitoring: a review of sediment sampling and DNA extraction methods. Sci. Total Environ. J. 818, 151783–151717. doi: 10.1016/j.scitotenv.2021.151783
Petro, C., Starnawski, P., Schramm, A., and Kjeldsen, K. U. (2017). Microbial community assembly in marine sediments. Aquat. Microb. Ecol. 79, 177–195. doi: 10.3354/ame01826
Philippot, L., Griffiths, B. S., and Langenheder, S. (2021). Microbial community resilience across ecosystems and multiple disturbances. Microbiol. Mol. Biol. Rev. 85, 1–24. doi: 10.1128/MMBR.00026-20
Plass, A., Schlosser, C., Sommer, S., Dale, A. W., Achterberg, E. P., and Scholz, F. (2020). The control of hydrogen sulfide on benthic iron and cadmium fluxes in the oxygen minimum zone off Peru. Biogeosciences 17, 3685–3704. doi: 10.5194/bg-17-3685-2020
Prokopenko, M. G., Hirst, M. B., De Brabandere, L., Lawrence, D. J., Berelson, W. M., Granger, J., et al. (2013). Nitrogen losses in anoxic marine sediments driven by Thioploca-anammox bacterial consortia. Nature 500, 194–198. doi: 10.1038/nature12365
Quast, M. G., Pruesse, M. B., Yilmaz, L., Gerken, D. J., Schweer, W. M., Yarza, J., et al. (2013). The SILVA ribosomal RNA gene database project: Improved data processing and web-based tools. Nucleic Acids Res. 41, 590–596. doi: 10.1093/nar/gks1219
Quero, G. M., Cassin, D., Botter, M., Perini, L., and Luna, G. M. (2015). Patterns of benthic bacterial diversity in coastal areas contaminated by heavy metals, polycyclic aromatic hydrocarbons (PAHs) and polychlorinated biphenyls (PCBs). Front. Microbiol. 6:1053. doi: 10.3389/fmicb.2015.01053
Rasigraf, O., Schmitt, J., Jetten, M. S. M., and Lüke, C. (2017). Metagenomic potential for and diversity of N-cycle driving microorganisms in the Bothnian Sea sediment. Microbiology 6:e00475. doi: 10.1002/mbo3.475
Rasigraf, O., van Helmond, N. A. G. M., Frank, J., Lenstra, W. K., Egger, M., Slomp, C. P., et al. (2020). Microbial community composition and functional potential in Bothnian Sea sediments is linked to Fe and S dynamics and the quality of organic matter. Limnol. Oceanogr. 65, S113–S133. doi: 10.1002/lno.11371
Rauret, G., López-Sánchez, J. F., Sahuquillo, A., Rubio, R., Davidson, C., Ure, A., et al. (1999). Improvement of the BCR three step sequential extraction procedure prior to the certification of new sediment and soil reference materials. J. Environ. Monit. 1, 57–61. doi: 10.1039/A807854H
R Core Team. (2015). R: A Language and Environment for Statistical Computing [computer program]. Vienna: R Foundation for Statistical Computing.
Rodríguez, J., Gallampois, C. M. J., Haglund, P., Timonen, S., and Rowe, O. (2021). Bacterial communities as indicators of environmental pollution by POPs in marine sediments. Environ. Pollut. 268, 115690–115611. doi: 10.1016/j.envpol.2020.115690
Rodríguez, J., Gallampois, C. M. J., Timonen, S., Andersson, A., Sinkko, H., Haglund, P., et al. (2018). Effects of organic pollutants on bacterial communities under future climate change scenarios. Front. Microbiol. 9, 1–21. doi: 10.3389/fmicb.2018.02926
Romero, L., Alonso, H., Campano, P., Fanfani, L., Cidu, R., Dadea, C., et al. (2003). Arsenic enrichment in waters and sediments of the Rio Loa (second region, Chile). Appl. Geochem. 18, 1399–1416. doi: 10.1016/S0883-2927(03)00059-3
Sander, S. G., Buck, K. N., and Wells, M. (2015). The effect of natural organic ligands on trace metal speciation in San Francisco Bay: implications for water quality criteria. Mar. Chem. 173, 269–281. doi: 10.1016/j.marchem.2014.09.015
Santander, E., Rojas, E., Moraga, R., and Salinas, P. (2017). Response of the abundance and size structure of bacterioplankton to different oceanographic conditions in Chipana bay (21°20‘s). Rev. Biol. Mar. Oceanogr. 52, 451–465. doi: 10.4067/s0718-19572017000300004
Santolini, M., and Barabási, A.-L. (2018). Predicting perturbation patterns from the topology of biological networks. Proc. Natl. Acad. Sci. 115, E6375–E6383. doi: 10.1073/pnas.1720589115
Segata, N., Izard, J., Waldron, L., Gevers, D., Miropolsky, L., Garrett, W. S., et al. (2011). Metagenomic biomarker discovery and explanation. Genome Biol. 12, R60–R18. doi: 10.1186/gb-2011-12-6-r60
Shannon, C. E., and Weaver, W. (1949). The Mathematical Theory of Communication, By CE Shannon (And Recent Contributions to the Mathematical Theory of Communication), W. Weaver. Champaign, IL, USA: University of illinois Press.
Stewart, F. J., Ulloa, O., and DeLong, E. F. (2012). Microbial metatranscriptomics in a permanent marine oxygen minimum zone. Environ. Microbiol. 14, 23–40. doi: 10.1111/j.1462-2920.2010.02400.x
Strom, D., Simpson, S. L., Batley, G. E., and Jolley, D. F. (2011). The influence of sediment particle size and organic carbon on toxicity of copper to benthic invertebrates in oxic/suboxic surface sediments. Environ. Toxicol. Chem. 30, 1599–1610. doi: 10.1002/etc.531
Sun, M. Y., Dafforn, K. A., Johnston, E. L., and Brown, M. V. (2013). Core sediment bacteria drive community response to anthropogenic contamination over multiple environmental gradients. Environ. Microbiol. 15, 2517–2531. doi: 10.1111/1462-2920.12133
Tamburini, E., Doni, L., Lussu, R., Meloni, F., Cappai, G., Carucci, A., et al. (2020). Impacts of anthropogenic pollutants on benthic prokaryotic communities in Mediterranean touristic ports. Front. Microbiol. 11:1234. doi: 10.3389/fmicb.2020.01234
Ter Braak, C., and Smilauer, P. (2002). CANOCO Reference Manual and CanoDraw for Windows User’s Guide: Software for Canonical Community Ordination (Version 4.5). Power, Ithaca, NY Microcomput.
Ulloa, O., Delong, E. F., Letelier, R. M., and Stewart, F. J. (2012). Microbial oceanography of anoxic oxygen minimum zones. Proc. Natl. Acad. Sci. 109, 15996–16003. doi: 10.1073/pnas.1205009109
Valdés, J. (2012). Heavy metal distribution and enrichment in sediments of Mejillones Bay (23° S), Chile: a spatial and temporal approach. Environ. Monit. Assess. 184, 5283–5294. doi: 10.1007/s10661-011-2339-5
Valdés, J., Sifeddine, A., Boussafir, M., and Ortlieb, L. (2014). Redox conditions in a coastal zone of the Humboldt system (Mejillones, 23° S). Influence on the preservation of redox-sensitive metals. J. Geochem. Explor. 140, 1–10. doi: 10.1016/j.gexplo.2014.01.002
Valdés, J., Vargas, G., Sifeddine, A., Ortlieb, L., and Guíñez, M. (2005). Distribution and enrichment evaluation of heavy metals in Mejillones Bay (23oS), northern Chile: geochemical and statistical approach. Mar. Pollut. Bull. 50, 1558–1568. doi: 10.1016/j.marpolbul.2005.06.024
Vavourakis, C. D., Mehrshad, M., Balkema, C., Van Hall, R., Andrei, A.-Ş., Ghai, R., et al. (2019). Metagenomes and metatranscriptomes shed new light on the microbial-mediated sulfur cycle in a Siberian soda lake. BMC Biol. 17, 69–20. doi: 10.1186/s12915-019-0688-7
Yang, Z., Wang, Y. Z., Wu, Z. C., Ren, L. R., Xiong, C. J., and Ma, Y. (2020). Microbial community structure and seasonal variations in mudflat sediments of Sansha bay, China. Appl. Ecol. Environ. Res. 18, 987–1000. doi: 10.15666/aeer/1801_9871000
Zárate, A., Dorador, C., Araya, R., Guajardo, M., Florez, J. Z., Icaza, G., et al. (2020). Connectivity of bacterial assemblages along the Loa River in the Atacama Desert, Chile. PeerJ 8:e9927. doi: 10.7717/peerj.9927
Zárate, A., Dorador, C., Valdés, J., Molina, V., Icaza, G., Pacheco, A. S., et al. (2021). Benthic microbial diversity trends in response to heavy metals in an oxygen-deficient eutrophic bay of the Humboldt current system offshore the Atacama Desert. Environ. Pollut. 286:117281. doi: 10.1016/j.envpol.2021.117281
Zhang, C., Liu, Q., Li, X., Wang, M., Liu, X., Yang, J., et al. (2021). Spatial patterns and co-occurrence networks of microbial communities related to environmental heterogeneity in deep-sea surface sediments around yap trench. Western Pacific Ocean. Sci. Total Environ. 759, 1–14. doi: 10.1016/j.scitotenv.2020.143799
Zhao, Q., Zhao, H., Gao, Y., Zheng, L., Wang, J., and Bai, J. (2020). Alterations of bacterial and archaeal communities by freshwater input in coastal wetlands of the Yellow River Delta. China. Appl. Soil Ecol. 153, 103581–103512. doi: 10.1016/j.apsoil.2020.103581
Keywords: sediments microbial gradient, hyperarid coast, trace metals, microbial networks, predicted functions, biomonitoring
Citation: Zárate A, Molina V, Valdés J, Icaza G, Vega SE, Castillo A, Ugalde JA and Dorador C (2023) Spatial co-occurrence patterns of benthic microbial assemblage in response to trace metals in the Atacama Desert Coastline. Front. Microbiol. 13:1020491. doi: 10.3389/fmicb.2022.1020491
Edited by:
Monica Sharma, Babasaheb Bhimrao Ambedkar University, IndiaReviewed by:
Nnanake-Abasi Offiong, Topfaith University, NigeriaDigvijay Verma, Babasaheb Bhimrao Ambedkar University, India
Copyright © 2023 Zárate, Molina, Valdés, Icaza, Vega, Castillo, Ugalde and Dorador. This is an open-access article distributed under the terms of the Creative Commons Attribution License (CC BY). The use, distribution or reproduction in other forums is permitted, provided the original author(s) and the copyright owner(s) are credited and that the original publication in this journal is cited, in accordance with accepted academic practice. No use, distribution or reproduction is permitted which does not comply with these terms.
*Correspondence: Ana Zárate, ✉ YW5hLnphcmF0ZUB1ZnJvbnRlcmEuY2w=; Verónica Molina, ✉ dmVyb25pY2EubW9saW5hQHVwbGEuY2w=; Cristina Dorador, ✉ Y3Jpc3RpbmEuZG9yYWRvckB1YW50b2YuY2w=