- 1Key Laboratory of Mollisols Agroecology, Northeast Institute of Geography and Agroecology, Chinese Academy of Sciences, Harbin, China
- 2Institute of Pratacultural Science, Heilongjiang Academy of Agricultural Science, Harbin, China
- 3University of Chinese Academy of Sciences, Beijing, China
Root-knot nematodes (RKNs; Meloidogyne spp.), one of the most economically important plant-parasitic nematodes (PPNs), cause severe yield and quality losses in agriculture annually. The application of biological control agents is an environmentally safe and effective approach to control RKNs. Here, we report the genomic characteristics of a Bacillus velezensis strain YS-AT-DS1 (Bv-DS1) isolated from the tidal soil, revealing that it has a 4.73 Mb circular chromosome with an average GC-content of 46.43%, 3,977 genes, 86 tRNAs, and 27 rRNAs, and contains secondary metabolite clusters for producing antimicrobial compounds. In vitro assays indicated that Bv-DS1 has not only antagonistic activities against fungal pathogens, but also shows nematicidal activity, with a mortality rate of 71.62% mortality rates in second-stage juvenile (J2s) Meloidogyne incognita. We then focused on the biocontrol efficiency of Bv-DS1 against M. incognita in pot assays. Preinoculation with Bv-DS1 enhanced tomato growth, and significantly reduced the infection rate of J2s, and the number of galls and egg masses on tomato roots. The underlying mechanism in Bv-DS1-induced resistance to M. incognita was further investigated through split-root experiments, and analysing the expression of the genes related to jasmonic acid (JA), salicylic acid (SA), and the tonoplast intrinsic protein (TIP). The results indicated that Bv-DS1 could not activate host systemic-induced resistance (ISR) in the split-root system of tomatoes. Additionally, the expression of JA- (LOX D and MC) and SA- (PAL2 and PR) responsive genes did not change in Bv-DS1-pretreated plants at 3 and 14 days after nematode inoculation. The presented data showed that JA-and SA-dependent pathways were not required for the biocontrol action of the Bv-DS1 against RKN. The TIP genes, responsible for transport of water and small substrates in plants, have previously been shown to negatively regulate the parasitism of PPNs. Surprisingly, Bv-DS1 compromised the downregulation of TIP1.1 and TIP1.3 by M. incognita. Together, our data suggest that Bv-DS1 exhibits a dual effect on plant growth promotion and protection against RKN, possibly related to the regulation of water and solute transport via TIPs. Thus, the Bv-DS1 strain could be used as a biocontrol agent for RKN control in sustainable agriculture.
Introduction
Root-knot nematodes (RKNs; Meloidogyne spp.) are the most economically important plant-parasitic nematodes (PPNs) that cause severe yield losses of at least 100 billion dollars annually (Elling, 2013). As obligate biotrophs, RKNs have a broad range of host plants and are parasitic to more than 5,000 plant species, including field crops, vegetables, grass shrubs, and even fruit trees (Blok et al., 2008). RKNs have a very short life cycle, high reproductive capacity, and mainly attack the roots of growing plants. In addition, RKN infection, in combination with other fungal and bacterial pathogens in the soil, can cause secondary damage to host roots, which further exacerbates crop loss (Jones et al., 2013). In China, RKNs have also become a major yield-limiting factor in protected agriculture due to intensive production, continuous monoculture, and the maintenance of a stable microclimate (Jin et al., 2017, 2022). For example, RKNs can often be found during the off-season in polyhouse cultivation of vegetables in the northeast and northwest China due to favourable conditions such as moisture, temperature, and continuous availability of hosts, causing severe economic losses (Li K. et al., 2015, 2017; Liang, 2017; Li, 2020). Common management methods for RKNs include the utilisation of synthetic chemical nematicides and RKN-resistant cultivars (Giannakou and Anastasiadis, 2005; Jordan, 2018). However, breeding resistant cultivars requires lengthy procedures and heavy manpower, and there are limited genetic resources to develop resistance to RKNs (Davies and Elling, 2015). Frequent and excessive application of the synthetic chemical nematicides has caused high toxicity to soil ecosystems and humans, resulting in severe restriction or outright bans (Aktar et al., 2009; Coyne et al., 2018). Thus, there is an urgent need to explore environmentally safe and effective alternatives to control RKNs.
Biological control has emerged as an environmentally-friendly alternative to suppress various soil-borne pathogens, including PPNs (Mhatre et al., 2019; Lahlali et al., 2022). Numerous microorganisms, including fungi, bacteria, and actinomycetes, have been identified as potential biocontrol agents for the efficient management of RKNs in many crops, especially vegetables (Silva et al., 2017; Kim et al., 2018; Jin et al., 2019; Park et al., 2020; Pocurull et al., 2020; Sharma et al., 2020; Shahid et al., 2022; Wang et al., 2022). Some microbial antagonists of RKNs are able to directly parasitize nematode eggs or other developmental stages, such as Streptomyces rubrogriseus, Pasteuria penetrans (Davies and Curtis, 2011; Jin et al., 2017; Topalović et al., 2019). Some fungal and bacterial species were reported to produce metabolites which indirectly reduce RKN density by inhibiting egg hatching, repelling, immobilizing and/or killing J2s (Cheng et al., 2017; Park et al., 2020; Khoja et al., 2021; Sun et al., 2021). In addition, induction of resistance in plants by these biocontrol microorganisms is another indirect strategy for controlling RKNs (Dehghanian et al., 2020; Pocurull et al., 2020; Sharma et al., 2020). Other microbial species have often shown versatility in the mechanisms of control of RKNs. For instance, several species of Pasteuria spp. and Pochonia spp. exhibited parasitism against eggs and sedentary stages of RKNs, and can also produce secondary metabolites with nematicidal activity or activate plant defences against RKNs (Selim et al., 2014; Giné et al., 2016; Ghahremani et al., 2019).
Rhizosphere bacteria belonging to the Bacillus genus have been widely described to effectively reduce RKNs in both greenhouse and field experiments, such as B. firmus (Terefe et al., 2009), B. pumilus (Lee and Kim, 2016), B. amyloliquefaciens (Jamal et al., 2017), B. subtilis (Cao et al., 2019; Das et al., 2021), B. atrophaeus (Ayaz et al., 2021), B. cereus (Yin et al., 2021b) and B. altitudinis (Ye et al., 2022). As Bacillus species can rapidly colonise and reproduce in the plant rhizosphere and exhibit strong resistance to various environmental stresses, biocontrol agents based on Bacillus have shown greater advantages in production, storage, and reliability of RKN biocontrol efficiency compared with other bacterial antagonists of RKN (Lalloo et al., 2010). Moreover, Bacillus species can enhance plant growth and improve plant health. Some Bacillus strains have been commercially approved in many countries to control PPNs in agriculture, such as B. subtilis GB03 (Kloepper et al., 2004), B. firmus GB-126 (Wilson and Jackson, 2013) and B. firmus I-1582 (European Commission, 2019). The Bacillus genus achieve their biocontrol effectiveness against RKN through different mechanisms, including parasitism, production of nematicidal chemicals, intoxication, induction of plant systemic resistance, and regulation of water and nutrient uptake. For example, B. firmus I-1582 was proved to colonize eggs of M. incognita and degrade eggshells (Ghahremani et al., 2020). Microbial community analysis of infected J2s of RKNs showed a dominance of the Bacillus genera in suppressive soil against RKNs (Adam et al., 2014), suggesting some Bacillus species might parasitize J2s of RKN. B. thuringiensis has been found to produce Cry proteins that result in lysis of the intestine and nematode death (Wei et al., 2003). Other Bacillus isolates are reported to produce volatile organic compounds to prevent plant roots from RKN invasion by increasing mortality, reducing motility, or inhibiting hatching of J2s from the eggs (Huang et al., 2010; Gao et al., 2016; Du et al., 2017; Chen et al., 2021; Yin et al., 2021a; Ye et al., 2022). Previous studies have also provided evidence that the induced systemic resistance (ISR) in host plants by Bacillus species contributes to their antagonistic effects against RKNs (Ayaz et al., 2021; Yin et al., 2021b; Tian et al., 2022). It is worth noting that the activation of specific plant signalling pathways during ISR by Bacillus spp. varies depending on the species of bacterial isolates, host plant, and nematode. Additionally, El-Hadad et al. (2011) demonstrated that the inoculation of B. megaterium can suppress the population of RKN in the soil through regulation of phosphate solubilisation and mineralisation capacity. B. cereus BCM2 was verified to have excellent nematicidal activity against RKNs by secreting proteases (Hu et al., 2020). Thus, the identification of more antagonists from Bacillus. spp. is essential for their mass production and application in integrated strategies for RKNs control. While a comprehensive understanding of nematode biocontrol mechanisms using the genera Bacillus is a pre-requisite for further improving biocontrol efficiency of RKNs in agriculture.
Here, the B. velezensis strain YS-AT-DS1 (Bv-DS1) strain was previously isolated from tidal soil in Dongying city (Shandong province, China), and displayed promoted growth and antagonistic effects on pathogenic fungi. Thus we speculated it might have nematicidal activities against M. incognita. The main objective of this study was to investigate the biocontrol activity of Bv-DS1 against M. incognita in pot assays. The capability of Bv-DS1 to induce systemic resistance against M. incognita was determined in split-root system of tomato. To better understand the potential biocontrol mechanism, the expression of defence-related genes involved in jasmonic acid (JA), salicylic acid (SA), and ion-transport pathways were analysed in Bv-DS1-inoculated roots of tomato after nematode infection. In addition, tomato SA and JA mutant lines were used to assess the importance of the hormone-mediated defence pathways in biocontrol effects.
Materials and methods
Plant materials and nematode culture
Wild-type tomato (Solanum lycopersicum) cultivars “Castlemart” and “Moneymaker”, the JA biosynthetic mutant spr2 in the “Castlemart” cultivar (Li et al., 2003), and the SA-deficient NahG transgenic line in the “Moneymaker” cultivar were kindly provided by Prof. Zhao Jiuhai (Northeast Institute of Geography and Agroecology, Chinese Academy of Sciences). The susceptible tomato cultivar “Zhongshu-4” was used in all nematode inoculation assays, unless indicated otherwise. Tomato seeds were surface sterilised in 1% NaClO for 5 min and then rinsed thoroughly with sterile water three times. The seeds were germinated in sterile vermiculite for 5–7 days at 26°C and then maintained in a growth chamber with a photoperiod of 16-h light (26°C) and 8-h dark (21°C). After 3 weeks of growth, tomato plants were used for nematode inoculation. All plants were watered daily and fertilised twice per week with Hoagland solution.
The population of Meloidogyne incognita used in this study was cultivated on the tomato cultivar “Zhongshu-4” (susceptible to M. incognita) in a greenhouse with a 16/8-h light/dark cycle at 21–26°C. Egg masses were extracted from tomato roots on the 42nd day after inoculation. Eggs were collected on a 25 μm sieve and placed in an incubator at 28°C for hatching second-stage juveniles (J2s). Fresh J2s were collected daily and used as inoculums for testing nematode mortality and infection assays.
Strain isolation, identification, and genomic of DNA extraction
Bv-DS1 was isolated from a tidal soil sample collected in Dongying, Shandong Province, China, using the 10-fold dilution method on lysogeny broth (LB) medium. The complete 16S rRNA was sequenced in BGI (Shenzhen, China) and blasted using EzBioCloud (https://www.ezbiocloud.net/). The purified strain was stored in the China centre for type culture collection (CCTCC) with the accession number CCTCC M 2021239. Genomic DNA was extracted using the blood and cell culture DNA midi Kit (Cat. No. 13343, Qiagen, United States) according to the manufacturer’s protocol. Briefly, an appropriate volume of cultured bacteria was pelleted by centrifugation at 4,000 × g for 10 min and the supernatant was discarded. The bacteria pellet was then resuspended in 3.5 ml of Buffer B1 (with RNase A) by vortexing at top speed. A stock solution of 20 μl lysozyme stock solution (100 mg/ml) and 100 μl QIAGEN Protease or QIAGEN Proteinase K was added and incubated at 37°C for at least 30 min. Next, 1.2 ml of Buffer B2 was added and mixed by vortexing for a few seconds, followed by incubation at 50°C for 30 min. Then, the sample was vortexed for 10 s at maximum speed and applied to the equilibrated QIAGEN Genomic-tip. The QIAGEN Genomic-tip was washed with 2 × 7.5 ml of Buffer QC, followed by the precipitation, purification and dissolving of DNA. DNA concentration and purity were determined using a Qubit fluorometer and NanoDrop 2000 spectrophotometer (Thermo Fisher Scientific, Carlsbad, CA, United States). DNA integrity was assessed using 0.5% agarose gel electrophoresis.
Library construction and sequencing of the Bv-DS1 genome
Whole genome sequencing was performed using the MGISEQ-2000 platform and Oxford Nanopore Technologies (ONT) PromethION P24 device at BGI (Shenzhen, China). For the MGI sequencing library, the insert size was 350 bp with a pair-end sequencing length of 150 bp. Briefly, 1 μg of genomic DNA was randomly fragmented using a g-TUBE device (Covaris, Inc., Woburn, MA, United States) according to the manufacturer’s instructions. The DNA fragments with an average size of 200–400 bp were selected using magnetic beads. The selected fragments were 3′-adenylated through end-repair and adapter-ligation; PCR products were purified using the magnetic beads. The double-stranded PCR products were heat denatured and circularised using the splint oligo sequence. The single-strand circular DNA (ssCirDNA) was formatted as the final library and qualified using FastQC. For ONT sequencing, genomic DNA was used to construct a library using a ligation sequencing kit (SQK-LSK109) and native barcoding kit (EXP-NBD114) according to the standard 1D native barcoding protocol provided by the manufacturer (Oxford Nanopore, Oxford, UK). Briefly, 48 μl of genomic DNA was mixed with 3.5 μl NEBNext FFPE DNA repair buffer (New England BioLabs, Ipswich, MA, United States), 2 μl NEBNext FFPE DNA repair mix (NEB), 3 μl ultra II end-prep enzyme mix (NEB), and 3.5 μl ultra II end-prep reaction buffer (NEB) in a 200 μl PCR tube. The mixture was incubated at 20°C for 5 min followed by 65°C for 5 min. Next, 500 ng end-prepped samples were mixed with 2.5 μl native barcode (one barcode per sample) and 25 μl blunt/TA ligase master mix. The mixtures were incubated at 28°C for 10 min. A total of 700 ng pooled and barcoded DNA was used to perform adapter-ligation by adding 20 μl NEB next quick ligation reaction buffer (5×), 5 μl adapter mix II and 10 μl quick T4 DNA ligase. The mixture was incubated for 10 min at room temperature. The constructed library was quantified using a Qubit DNA HS assay kit in a 4.0 Fluorometer (Invitrogen, San Diego, CA, United States) and then loaded into the flow cell R9.4.1 of a PromethION P24 device (BGI-ShenZhen, China).
Genomic data analysis of Bv-DS1
All the raw data were trimmed using SOAPnuke v.1.5.2 (Li D. et al., 2015). High-quality reads were assembled de novo using Megahit software (Chen et al., 2018). Assembled contigs with lengths less than 300 bp were discarded in the subsequent analysis. The prediction of coding genes (CDS) was analysed with Glimmer (version 3.02) and the annotation was done by alignment against the COG, Gene Ontology (GO), and Kyoto Encyclopedia of Genes and Genomes (KEGG) databases. Secondary metabolites analysis was performed using antiSMASH 5.0 software (Blin et al., 2019).
Inhibition of pathogenic fungi
The ability of Bv-DS1 to inhibit Rhizoctonia solani, Fusarium avenaceum and Fusarium graminearum were investigated using a plate confrontation method according to the description by Gao et al. (2021). Briefly, the pathogenic fungi were inoculated in the centre of the PDA plate and 100 μl Bv-DS1 (OD600 = 1) were inoculated 2.5 cm from the centre containing pathogenic fungi, with plates not inoculated with Bv-DS1 used as a control. All plates were incubated at 27°C for several days until the pathogenic fungi on the control plates grew all over the petri dish. Then the growth diameter of the pathogen was measured.
Screening the ability of IAA production
The measurement of IAA production was conducted by using a modified quantification method based on Bric et al. (1991). Briefly, Bv-DS1 was cultured for 24 h in 1 ml of LB liquid medium. Then 10 μl of bacterial inoculums were transferred into the same medium supplemented with 100 μg mL−1 of L-tryptophan (Sigma-Aldrich) followed by 7 days of incubation at 28°C on a shaking incubator (200 rpm/min). Then, bacterial cells were removed from the culture medium by centrifugation (4,000 × g, 5 min). The supernatant was then transferred with Salkowski reagent (49 ml of 35% HClO4, 1 ml of 0.5 M FeCl3) to an ELISA plate in a 1:1 the ratio, which was incubated at room temperature for 35 min. The absorbency was then read at 490 nm by using a multi-functional enzyme labeller (CLARIOstar Plus, BMG, Germany). The uninoculated tryptophan-containing medium mixed with Salkowski reagent was used as a blank. Three independent cultivations were used as triplicated replicates. A standard curve was generated from serial dilutions of IAA stock solution.
Effect of Bv-DS1 culture filtrate on J2s mortality of Meloidogyne incognita
Bv-DS1 was cultured in 100 ml of LB liquid medium for 48 h at 28°C on a shaking incubator (200 rpm/min). The fermented bacteria were centrifuged at 2,500 × g for 10 min, and the bacterial pellet was resuspended and adjusted to a density of 1.0 × 108 colony-forming units (CFUs) per millilitre with sterile water, which was used further for inoculation. Additionally, the supernatant of the Bv-DS1 strain was collected and filtered using a 0.22-μM Millipore filter. The prepared culture filtrate was used to test nematocidal efficacy in vitro.
For inoculation with Bv-DS1, the 3-week-old tomato plants were transplanted to individual pots (14 cm in height and 12 cm in diameter) filled with sterilized sand-soil medium (2:1, vol/vol) for another 3 days until the initiation of the experiment. Each transplanted plant was inoculated with Bv-DS1 by adding 20 ml of bacterial suspension into 2-cm-deep holes. Subsequently, the plants were put back in the growth chamber and used for nematode inoculation after 3 days of growth.
For nematode mortality assay, 10 μl of nematode suspension (approximately 100 J2s) and 490 μl of Bv-DS1 culture filtrate (100 and 10%, respectively) were added to each well of the 24-well culture plate (Corning, United States), and sterile water was used as the control. The plates were incubated in darkness for 24 h at room temperature, and then the number of living and dead nematodes were counted under a stereomicroscope (Olympus, Japan). J2s were considered to be dead if their body was straight and immobile after the Na2CO3 stimulus for 30 s (Hu et al., 2019). The experiment was carried out twice with 10 replicates. Corrected J2s mortality was calculated using the following equation:
[(mortality rate of J2s treated with Bv-DS1 - mortality rate of J2s treated using the sterile water)/(1 - mortality rate of J2s treated using sterile water)] × 100.
Nematode inoculation
To investigate the effects of Bv-DS1 on the nematode-invasion ability, gall formation, and host defence, a pot experiment was conducted at different time points using four treatments: (1) roots treated with sterile water, (2) roots pre-inoculated with Bv-DS1 alone, (3) roots inoculated with nematodes alone, (4) the roots preinoculated with Bv-DS1 and then infected with nematodes. Three-week-old tomato plants were transplanted to pots filled with sand-soil medium and were grown for 6 days. Two holes were opened on the surface of pots, and plants were inoculated with 500 J2s per plant in one pot. At 3, 7, and 14 days after nematode inoculation (dai), roots were collected and used for RNA extraction and gene expression analysis. At 35 dai, plant height, fresh weight of root and shoot, and stem thickness were measured, and the disease severity was assessed by counting gall numbers on roots. Each treatment was performed with six replicates, and three independent experiments were conducted for each treatment.
To further evaluate the control efficacy of Bv-DS1 against RKN in the greenhouse condition, the soil collected from the M. incognita-infested tomato field (Shenyang, Liaoning province, China) was used in the pot experiment. The population densities of M. incognita were 1.5 nematodes per cm3 of soil. Three-week-old tomato plants were transplanted into pots (20.5 cm deep × 14 cm diameter) filled with soil media containing 20% sterilized sand and 80% diseased soil. After 3 days of transplantation, 20 ml of the Bv-DS1 suspension (108 CFU/ml) was drenched into the rhizosphere of the plant in each pot; the plants were irrigated with the same volume of sterile water that served as the control. All plants were grown in the greenhouse under a completely randomized design for 42 days. Afterwards, the roots of tomato plants were collected, and fresh root weight was measured. Root galls per plant were counted and the number of egg masses was determined using 0.01% erioglaucine (Sigma, St. Louis, MO, United States) staining (Omwega et al., 1988). Finally, eggs were extracted from the roots according to the previously described method (Hussey and Barker, 1973). Each treatment had eight replicates, and the experiment was repeated twice.
Split-root assay
The split-root system of tomato was used to evaluate the ability of Bv-DS1 to induce systemic plant resistance against M. incognita as described by Martínez-Medina et al. (2017). Three-week-old tomato plants were transferred to the split-root system by splitting the root system into two halves that were planted into two adjacent pots (14 cm in height and 12 cm in diameter) containing a sterilized sand-soil mixture (2:1, v/v) (Figure 1A). A total of three treatments were used in this experiment with eight replicates for each treatment. The treatments included (1) half of the root system being inoculated with 500 J2s of M. incognita (RKN/−) and another half of the roots with sterile water, (2) half of the roots were pre-inoculated with the Bv-DS1 suspension and then infected with nematodes (RKN + Bv-DS1/−), and (3) half of the root system was pre-inoculated with Bv-DS1 and another half was infected with nematodes (RKN/Bv-DS1). For treatments of Bv-DS1, the plants in the split-root set-up were inoculated with 20 ml of suspension of Bv-DS1 (108 CFU/ml) 6 days after transplantation. The pots were placed in the greenhouse under the same condition for another 3 days.
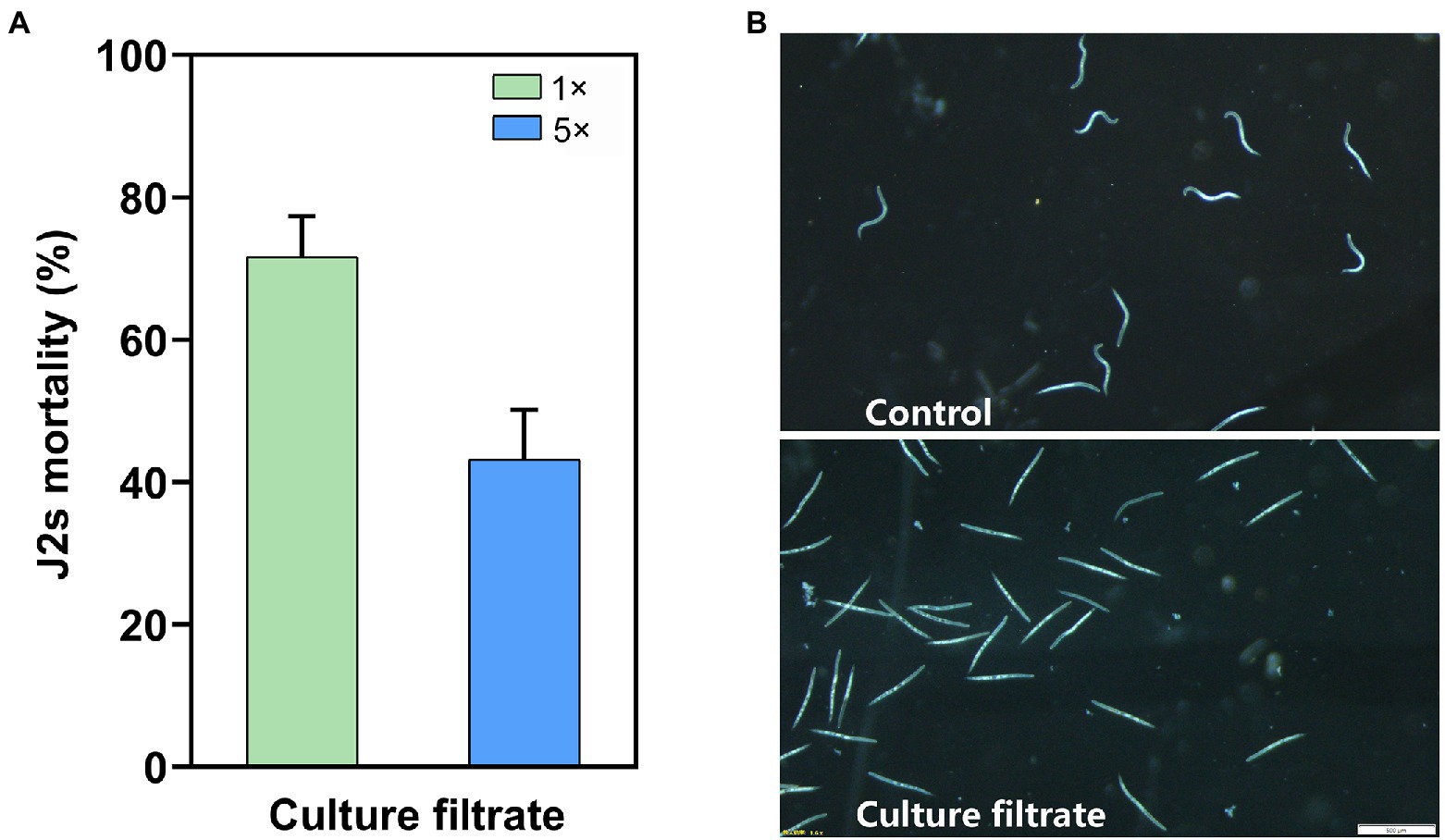
Figure 1. Filtrate culture of Bacillus velezensis YS-AT-DS1 (Bv-DS1) affected the J2s mortality of M. incognita. (A) Corrected J2s mortalities of M. incognita were analysed after incubation with the 1× and 5× Bv-DS1 filtrate cultures for 24 h at room temperature. The error bars represent the mean ± SE of the data from 10 replicates. (B) Microscopic observation of J2s body immersed in Bv-DS1 filtrate culture or the sterilized distilled water after 48 h incubation. Scale bar = 500 μm.
RNA extraction and quantitative real time-PCR analysis
Tomato roots were frozen in liquid nitrogen and ground to a fine powder in a pestle and mortar. RNA was extracted using an RNAprep pure plant kit (TianGen Biotech, Beijing, China) according to the manufacturer’s instructions. One microgram of total RNA was used to synthesize cDNA using FastKing gDNA dispelling RT SuperMix FastKing Kit (TianGen Biotech, Beijing, China). qRT-PCR analysis was performed in the LightCycler® 480 System with SYBR green master mix (Vazyme, Nanjing, China). The target gene primers used for qRT-PCR are listed in Supplementary Table S1. Reaction conditions were as follows: 95°C for 5 min, and then 40 two-step cycles of 10 s at 95°C and 30 s at 60°C. The relative expression levels of the defence-related tomato genes and the actin gene from M. incognita were normalized and calculated using the reference gene expression of SIEF1α using the 2-∆∆Ct method.
Statistical analysis
Data analysis was performed using SPSS version 17.0 software (SPSS Inc., Chicago, United States). The statistically significant differences were analysed using one-way ANOVA (multiple comparisons) or Student’s t-test (unpaired comparisons), as shown in the figure legends. The error bars in the figures indicated the standard error (SE) of means, and the significance level was set at p < 0.05.
Results
Taxonomic identification and genomic features of Bv-DS1
Analysing the complete 16S rRNA sequence (1,471 bp) of the stain using EzBioCloud revealed that it showed 100% similarity with B. velezensis CR-502. Phylogenetic analysis indicated that it grouped with the B. velezensis strain CR-502 T (AY603658), thus confirming its classification as B. velezensis (Supplementary Figure 1). Further analysis of genome characteristics revealed that the genome of Bv-DS1 comprised a circular chromosome of 4.73 Mb (Figure 2A), and was deposited in NCBI with the accession number CP102866. The chromosome of Bv-DS1 included 4,007,438 bp, with an average GC-content of 46.43%, 3,977 protein-coding genes (CDS), 86 tRNAs, and 27 rRNAs. A total of 4,334 (82.2%) CDSs were classified into Cluster of Orthologous Groups of proteins (COG) families composed of 25 categories (Figure 2B). Among the categories, amino acid transport and metabolism (306 genes), transcription (288 genes), and cell wall/membrane/envelope biogenesis (207 genes) were the top three functional categories. However, there was a high proportion of function unknown genes (206), and general function prediction-only genes (330 genes) were poorly characterized (Supplementary Table S2).
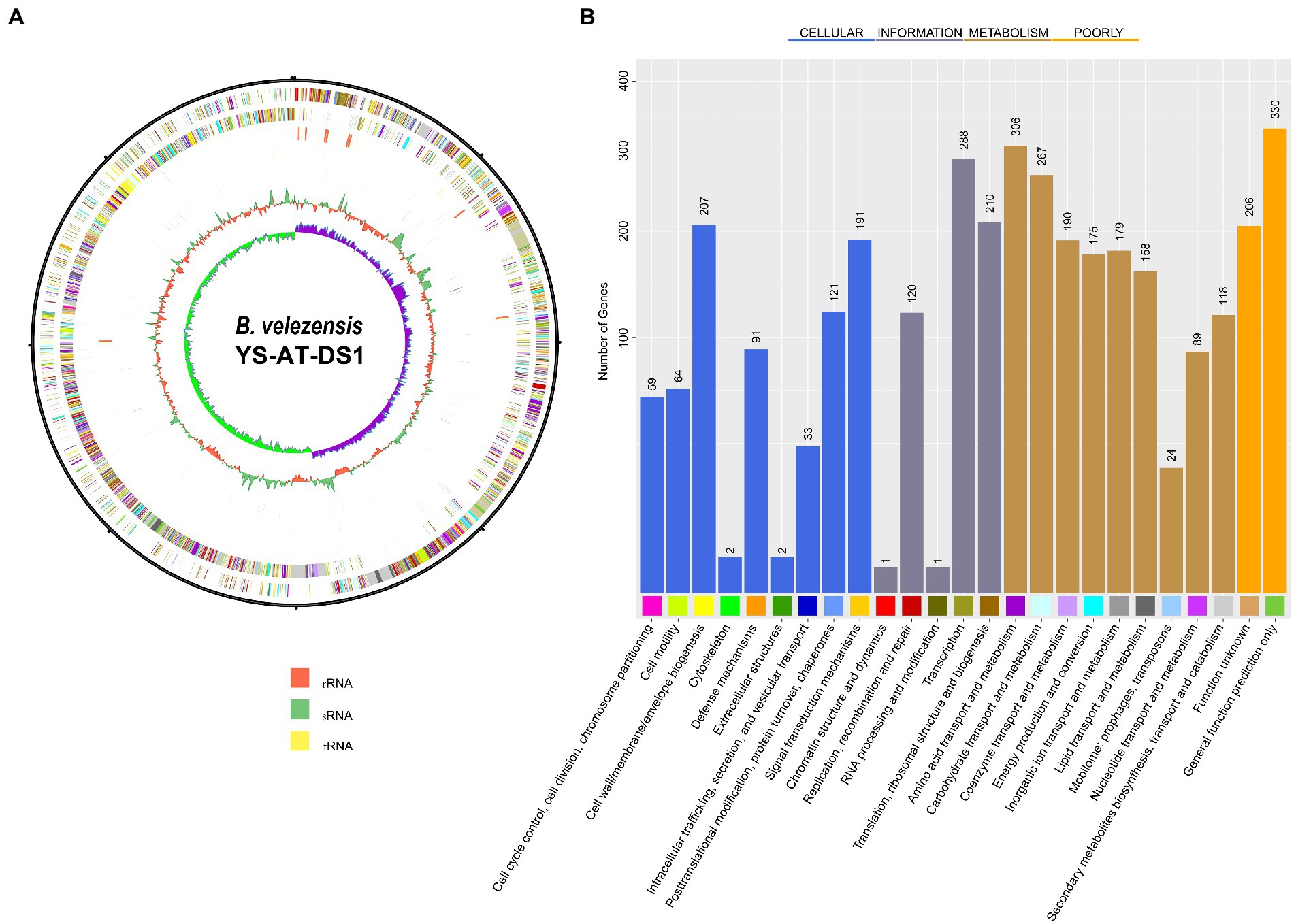
Figure 2. (A) Graphical circular map of Bacillus velezensis YS-AT-DS1 chromosome. From outer circle to the centre: CDS on forward strand (coloured according to COG categories), all CDS and RNA genes on forward strand, all CDS and RNA genes on reverse strand, CDS on reverse strand (coloured according to COG categories). The map was generated using Bacterial Annotation System, BASys. (B) COG functional classification.
Genetic basis for the anti-pathogen activity of Bv-DS1
Using the antiSMASH genome analysis tool, the detection of secondary metabolite clusters of Bv-DS1 were detected (Supplementary Table S3). Three trans ATPKS, two NRPS, and one other (bacilysin) cluster showed 100% similarity to the known biosynthetic gene clusters. Several clusters related to surfactin, aurantinin B/aurantinin C/aurantinin D, and butirosin A/butirosin B saccharides were also detected in the Bv-DS1 genome (Supplementary Table S3).
IAA production, in vitro biocontrol activity of strain Bv-DS1 against fungal pathogens and root-knot nematodes
As Bv-DS1 promoted the growth of tomatoes plants, we hypothesized that it might be also able to produce IAA. Bv-DS1 produced 3.07 μg mL−1 IAA and showed antifungal activity against three soybean pathogens (Rhizoctonia solani, Fusarium avenaceum, and Fusarium graminearum) that cause root rot disease, with the inhibition zones of 1.04 ± 0.18 cm, 1.04 ± 0.15 cm and 1.70 ± 0.20 cm, respectively (Supplementary Figure 2). The nematicidal activity of Bv-DS1 was assessed in a 24-cell plate by analysing the mortality rates of M. incognita J2s after treatment with Bv-DS1 culture. After incubation for 24 h, the 1 × Bv-DS1 and 5 × filtrates resulted in 71.62 and 43.16% corrected J2s mortality rates of M. incognita, respectively (Figure 1A). The microscopic observation indicated that most J2s were straight and immobile in the filtrate culture of Bv-DS1 after 48 h treatment. In comparison, the untreated J2s displayed the normal ‘S’ bend shape and were much more active (Figure 1B). These results demonstrated that Bv-DS1 metabolites had nematicidal activity against M. incognita in vitro.
Promotion of tomato growth and suppression of Meloidogyne incognita infection induced by Bv-DS1
The efficacy of Bv-DS1 against M. incognita in tomatoes was evaluated in the pot assay. After 5 weeks of transplantation, Bv-DS1 treatments caused a significant increase in the plant height and root and shoot weight of tomato plants, inoculated or non-inoculated with nematodes, suggesting that Bv-DS1 had a positive effect on plant growth (Figure 3A; Table 1). The expression of the actin gene of M. incognita was determined in tomato roots at 3 and 7 dai to investigate the effect of Bv-DS1 on M. incognita infection (Martínez-Medina et al., 2017). Although the actin gene of M. incognita showed low expression levels in both Bv-DS1-treated and untreated plants at 3 dai, actin gene expression in Bv-DS1-treated roots was significantly lower than that in the non-inoculation roots (Figure 3B). At 7 dai, pre-inoculation with Bv-DS1 led to a 1.5-fold reduction in expression levels of actin compared to the roots without Bv-DS1 treatments (Figure 3B). These results showed that the application of Bv-DS1 inhibited early infection of M. incognita. Additionally, the number of root galls on tomato roots pre-inoculated with Bv-DS1 (100 ± 11 per plant) was significantly lower than that of the non-inoculated control roots (200 ± 11 per plant). These results indicated that Bv-DS1 had plant growth promoting potential and could efficiently control M. incognita (Figure 3C).
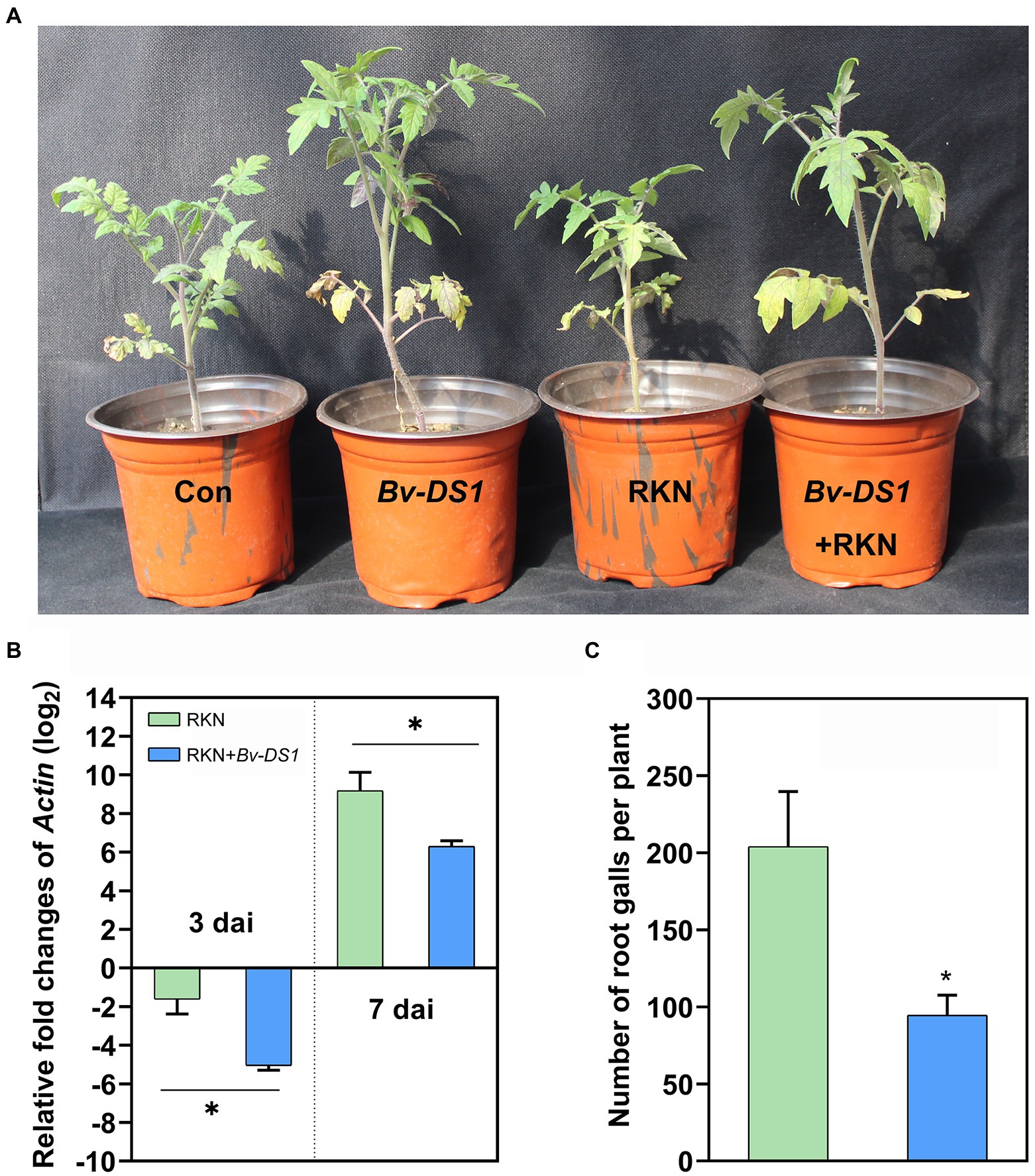
Figure 3. The control efficacy of Bacillus velezensis YS-AT-DS1 (Bv-DS1) against M. incognita in pots. (A) Plant growth promotion was observed in the Bv-DS1-pre-inoculated tomato plants on the 35th day after inoculation (dai) with M. incognita. (B) The relative expression folds of the actin gene from M. incognita were evaluated inside the roots of Bv-DS1-pre-inoculated or non-inoculated tomato plants at 3 and 7 dai. Each value is presented as mean ± SE of three biological replicates for all three plant roots. Asterisks indicate significant differences between treatments (p < 0.05) according to Student’s t test. (C) The number of galls was counted in roots of Bv-DS1-pre-inoculated or non-inoculated tomato plants at 35 dai. Data are presented as mean ± SE of eight plants for each treatment. Asterisk indicates statistically significant differences between treatments (p < 0.05) according to Student’s t test.
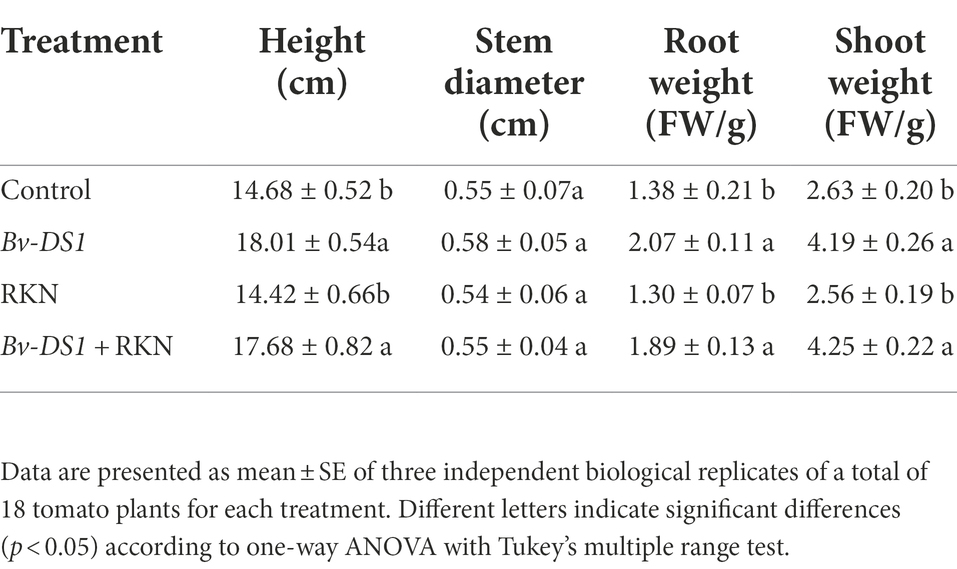
Table 1. Effect of Bacillus velezensis YS-AT-DS1 on plant growth parameters after inoculation with RKN.
Efficiency of strain Bv-DS1 against Meloidogyne incognita in the disease soil
The control efficacy of Bv-DS1 against RKN was tested further in the soil collected from the M. incognita-infested tomato field. Irrigation of Bv-DS1 into the rhizosphere significantly increased the fresh weight of the tomato plants by 35.5% compared to the control group at 42 days (Figure 4A). The number of galls and egg masses were lower on the tomato roots after treatment with Bv-DS1 (Figure 4B), which decreased by 29.3 and 33.8%, respectively (Figure 4C). Eggs per egg mass from the root system in the soil of Bv-DS1 drenching was also markedly lower than those collected from the non-inoculated plants (Figure 4D). These results suggested that Bv-DS1 could enhance the resistance of tomatoes to suppress M. incognita reproduction.
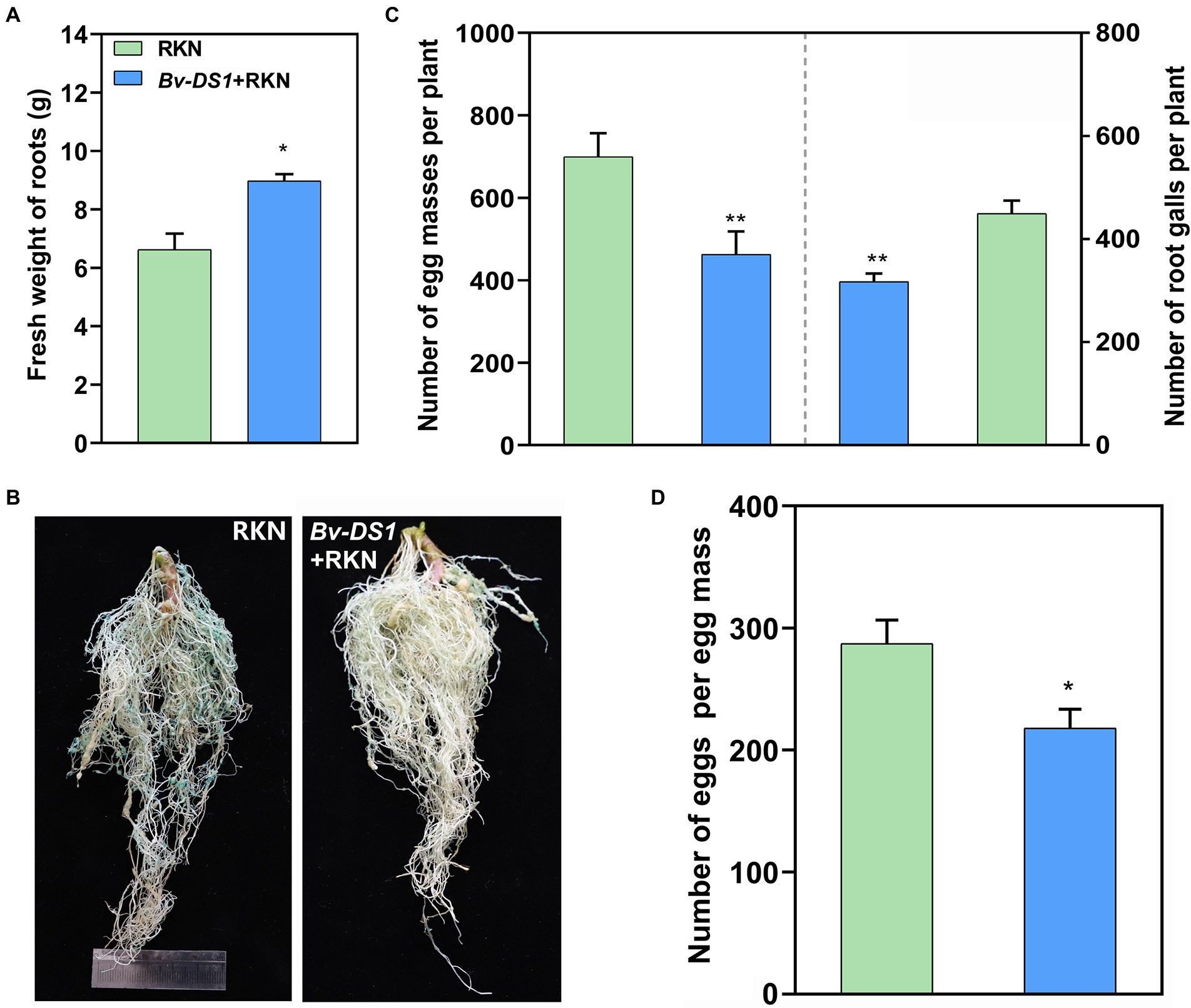
Figure 4. Bacillus velezensis YS-AT-DS1 (Bv-DS1) reduced M. incognita infection when tomato plants were grown in diseased soil. (A) Fresh weight of tomato roots pre-inoculated or non-inoculated with Bv-DS1 growing in the RKN-diseased soil for 42 days. (B) Root galling and egg masses on tomato plant pre-inoculated or non-inoculated with Bv-DS1. (C) Number of galls or egg masses per tomato plant. Data are presented as mean ± SE of 10 plants for each treatment. Asterisk indicates significant differences between treatments (p < 0.05) according to Student’s t test. (D) Number of eggs per egg mass. Data are shown as mean ± SE of eight plants for each treatment. Asterisk indicates significant differences between treatments (p < 0.05) according to Student’s t test.
Bv-DS1 induced the local resistance of tomato against Meloidogyne incognita
To assess whether the Bv-DS1-mediated plant resistance to RKN occurred in the systemic root tissue of tomato, a split-root system of tomato (Martínez-Medina et al., 2017) was used (Figure 5A). Compared to the control roots (only inoculated with RKN), pre-inoculation with Bv-DS1 caused a reduction in the galling of the local root system (Bv + RKN/−) (Figure 5B). The number of galls and egg masses in the local root system of the Bv-DS1-treated plants significantly decreased by 48.42 and 64.81%, respectively (Figures 5C,D). Although there was a slight reduction in the number of galls and egg masses in the systematic root tissue after Bv-DS1 treatments, no statistical significance was found in the Bv-DS1-induced systemic protective effects when compared to the control group (Figures 5C,D). These results indicated that Bv-DS1 could not elicit systemic resistance to RKN in tomatoes.
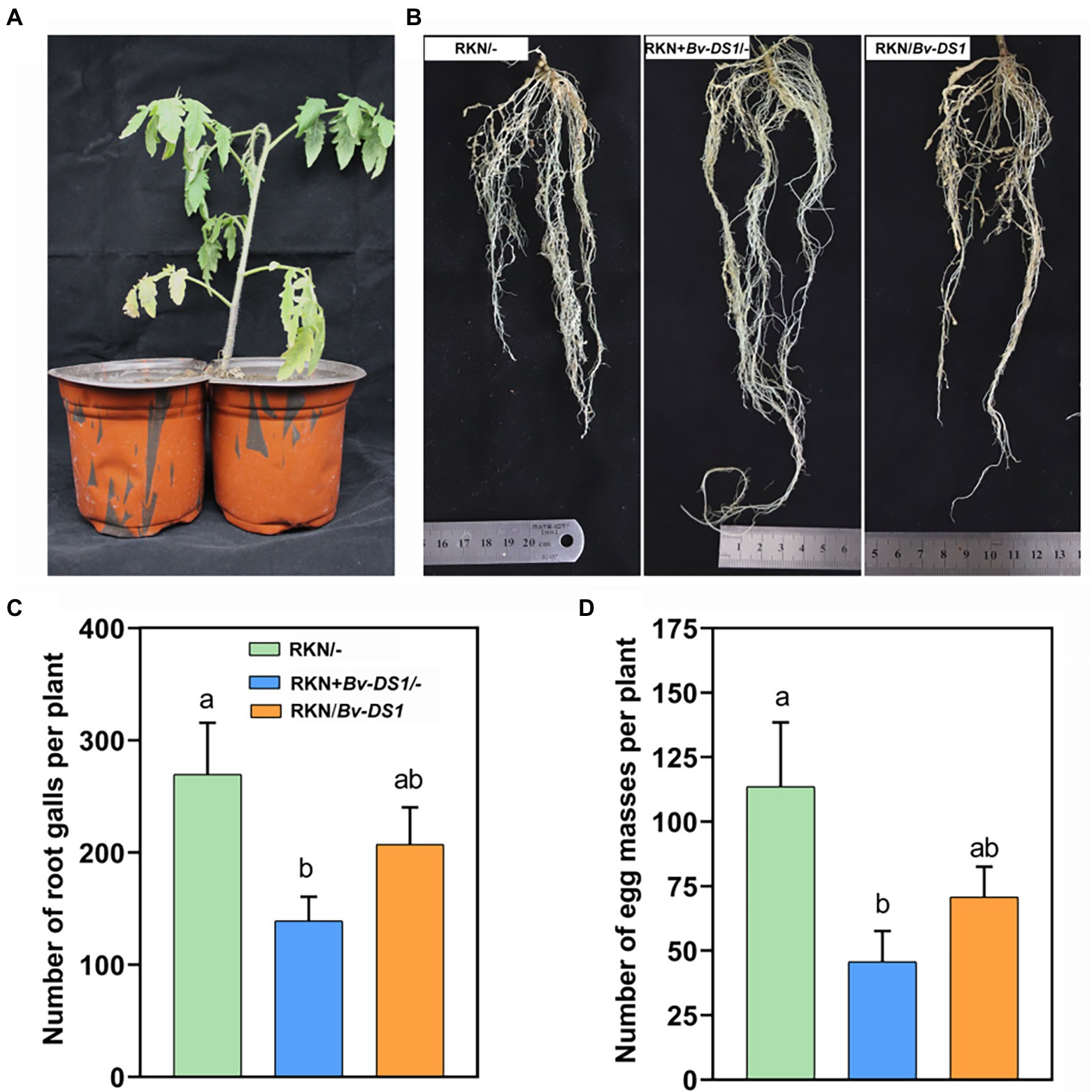
Figure 5. Bacillus velezensis YS-AT-DS1 (Bv-DS1) induced the local resistance of tomato plants to M. incognita in the split-root assay. (A) The split-root system of tomato growth in two adjacent pots. (B) Less root galls were observed in tomato plants with half of roots inoculated with Bv-DS1 and RKN together (RKN + Bv-DS1/−) compared to other treatments (RKN/−; RKN/Bv-DS1). (C) The number of galls was counted at 35 dai in the split-root system. Data are shown as mean ± SE of six plants for each treatment. Different letters indicate significant differences between treatments (p < 0.05) according to Tukey’s multiple comparisons test following one-way ANOVA. (D) Number of eggs per egg mass. Data are presented as mean ± SE of six plants for each treatment. Different letters indicate significant differences between treatments (p < 0.05) according to Tukey’s multiple comparisons test following one-way ANOVA.
Effects of Bv-DS1 on the defence-responsive gene expression in tomato roots
SA and JA are two important plant hormones and play crucial roles in plant defence response to nematode infection. To examine whether SA or JA-dependent signalling contributed to the Bv-DS1-mediated tomato resistance to RKN, we analysed the detailed transcript abundance of the SA and JA marker genes in Bv-DS1-preinoculated tomato roots under RKN stress (Figure 6). The expression of JA-related genes LOX D and MC in the roots of the RKN-untreated plants was significantly upregulated via Bv-DS1 preinoculation. RKN infection also resulted in the upregulation of transcript levels of MC at 7 and 14 dai. No significant effect of Bv-DS1 on the expression of MC in nematode-infected roots was found at 3, 7, and 14 dai. However, co-inoculation of RKN and Bv-DS1 caused a transiently significant downregulation of LOX D expression at 7 dai (Figure 6). Expression levels of SA-responsive genes PAL2 and PR in the roots of Bv-DS1 pretreated plants were similar to that of the non-inoculated tomato roots at 3 and 7 dai. Similar to the changes in JA marker genes, PAL2 and PR transcripts were significantly upregulated via Bv-DS1 preinoculation or RKN infection alone, but this activation of RKN-induced PAL2 and PR expression was not observed in Bv-DS1 preinoculated roots at 14 dai.
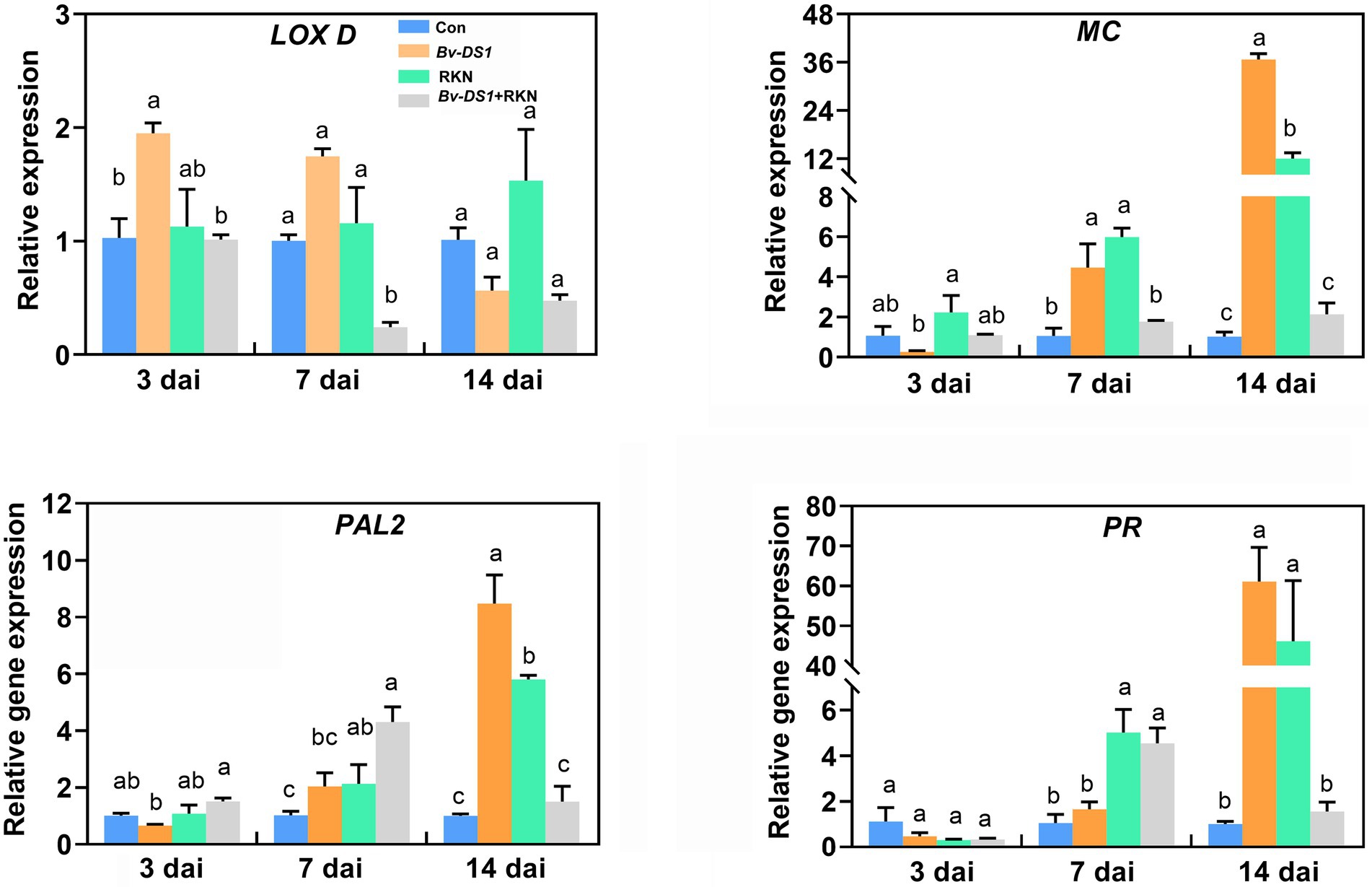
Figure 6. Expression levels of defence-related genes were determined in tomato roots non-inoculated or pre-inoculated with Bacillus velezensis YS-AT-DS1 at 3, 7, and 14 days after M. incognita inoculation. Relative gene expression level was normalized to the tomato reference gene SIEF. Error bars represent the SE of the mean of three biological replicates for three plant roots for each treatment. Different letters indicate significant differences between treatments (p < 0.05) according to Tukey’s multiple comparisons test following one-way ANOVA.
The SA-deficient transgenic NahG tomato line, the JA-deficient mutant spr2 and their corresponding background wild-type tomato lines ‘Castlemart’ and ‘Moneymaker’ were used to further assess the roles of SA and JA pathways in the biocontrol effects of Bv-DS1 in tomatoes against RKN. A significant reduction in root galls was observed in both Bv-DS1-treated ‘Castlemart’ and JA-deficient mutant spr2 when compared to the Bv-DS1 non-inoculation roots. Similarly, Bv-DS1 preinoculation also resulted in a reduction in the number of root galls in both the NahG tomato line and the wild-type ‘Moneymaker’ at 21 dai (Supplementary Figure 3). These findings indicated that Bv-DS1-induced tomato resistance against RKN was not dependent on the SA or JA pathways.
Bv-DS1 reverses the suppression of TIP genes in tomato By RKN infection
Tonoplast intrinsic proteins (TIPs), localised in vacuoles, play a key role in plant defences against PPNs through the regulation of water and ion transport (Baranowski et al., 2019). Therefore, three TIP genes (TIP1.1, TIP1.2, TIP1.3), which displayed significant downregulation in the RKN-infected susceptible tomato roots (Shukla et al., 2018), were selected to study the effects of Bv-DS1 on their expression in the RKN-inoculated tomato roots. The expression of TIP1.1 and TIP1.2 was significantly upregulated by Bv-DS1 at 24 and 72 h, respectively. TIP1.3 transcript levels reached the peak at 24 h and then declined at 72 h but were still higher than that of untreated control roots (Figure 7A). The expression levels of three TIP genes were significantly downregulated at 3 dai, this suppression of RKN-induced TIP1.1 and TIP1.3 expression was alleviated in tomato roots through Bv-DS1 preinoculation (Figure 7B).
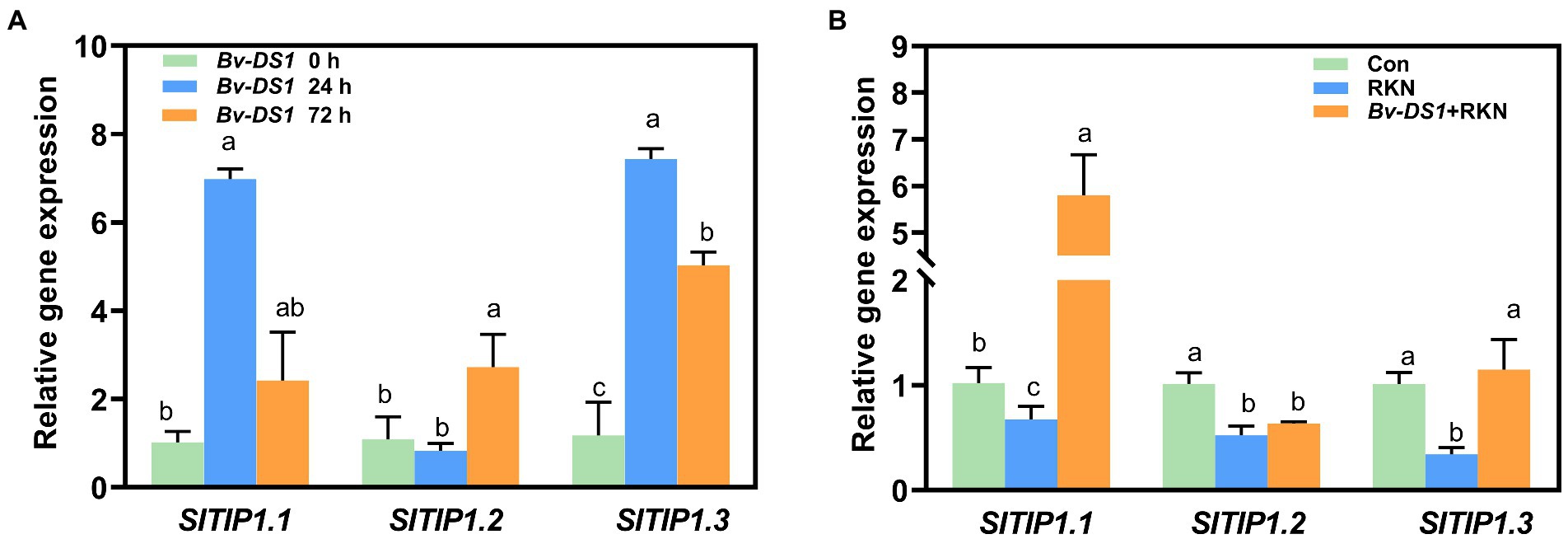
Figure 7. Bacillus velezensis YS-AT-DS1 (Bv-DS1) activated the expression levels of SITIPs in tomato roots. (A) Expression levels of SITIPs in tomato roots after Bv-DS1 pre-inoculation for 0, 24, and 72 h. (B) Bv-DS1 treatments reversed the suppression of SITIPs in tomato roots induced by M. incognita at 3 dai. Relative gene expression level was normalised to the tomato reference gene SIEF. Each value is presented as mean ± SE of three biological replicates for three plant roots for each treatment. Different letters indicate significant differences between treatments (p < 0.05) according to Tukey’s multiple comparisons test following one-way ANOVA.
Discussion
Bacillus velezensis is an important member of plant growth-promoting rhizobacteria (PGPR) and are extensively studied for their potential to promote plant growth and to control soil-borne diseases (Jiang et al., 2018; Wang et al., 2020; Ding et al., 2021; Han et al., 2022). However, information about their effectivity against PPNs, including RKNs, remains limited (Xiang et al., 2017; Tian et al., 2022). This study provides evidence for the ability of a novel B. velezensis strain Bv-DS1, isolated from a tidal soil sample, to enhance tomato growth and reduce M. incognita infection of tomato roots. In addition, this strain also exhibited inhibitory activity against three fungal pathogens in vitro. Hence, the results of this study may provide valuable information to optimize the use of Bv-DS1 as a PGPR resource for controlling a broad range of soil-borne diseases and increasing crop yield.
Numerous studies employing comparative genomic analysis have revealed that the genomes of B. velezensis harboured multiple gene clusters related to secondary metabolites, which are involved in plant growth promotion, biofilm formation, and antimicrobial activity (Grady et al., 2019; Xu et al., 2020; Liu et al., 2021; Zaid et al., 2022). Some reports have shown the capacity of B. velezensis species to form sessile communities (biofilms) (Ding et al., 2021), promoting plant growth (Xiang et al., 2017; Fan et al., 2018), and biocontrol efficacy (Wang et al., 2020; Han et al., 2022) under different experimental conditions, from in vitro studies to field experiments on different crops. In the present study, we found that tomato inoculation with Bv-DS1 increased the plant height and biomass compared to untreated controls, and this PGPR trait may be related to the ability of Bv-DS1 to produce IAA activity. The in vitro assay in this study suggested that Bv-DS1 had a similar antifungal activity against soybean pathogenic fungi R. solani and F. graminearum and the genome contains several gene clusters that were predicted to be responsible for the biosynthesis of antimicrobial (surfactin, bacilysin, macrolactin, fengycin, and bacillibactin) (Supplementary Table S3). It has been shown that antibiotic substances secreted by B. velezensis, including surfactin, bacillomycin D, fengycin, and bacillibactin, have significant antagonistic activity against plant pathogens (Yamamoto et al., 2015; Gao et al., 2017; Chen et al., 2020). Since most of these gene clusters associated with antimicrobial activity are conserved in all B. velezensis strains, their antagonistic activities against pathogenic fungi and bacteria have been verified by many studies. Our research objective was focused on the biocontrol efficacy of the RKN M. incognita.
The results of the pot experiments demonstrated that application of Bv-DS1 significantly reduced M. incognita invasion and nematode reproduction,suggesting the biocontrol potential of Bv-DS1 in controlling PPNs. It is well known that Bacillus spp. can act as nematode antagonists through inhibiting J2 hatching from eggs, motility, and viability (Ghahremani et al., 2020; Chen et al., 2021; Yin et al., 2021a; Ye et al., 2022). Similar antagonism was observed in some B. velezensis stains, which displayed biocontrol activities against PPNs. Xiang et al. (2017) found that B. velezensis Bve12 can directly kill H. glycines J2s in vitro, and consistently reduced H. glycines population density in greenhouse and field conditions. B. velezensis GJ-7 can significantly suppress the hatching of M. hapla eggs, and the mortality rate of J2s in 100% fermentation broth of B. velezensis GJ-7 was 87% after 24 h treatment (Wu et al., 2022). Recently, Tian et al. (2022) isolated a PGPR strain B. velezensis Bv-25 from cucumber rhizosphere, which is able to disrupt the chemosensory function of M. incognita J2s by suppressing the expression of ord-1 and flp-18. This research also pointed out that Bv-25 can inhibit egg hatching and cause J2s mortality (Tian et al., 2022). Bv-DS1 fermentation filtrate also showed significant J2-killing activity, with the mortality rate of M. incognita J2s at 71.62% within 24 h treatment. We noted that almost all J2s were dead after 48 h treatment with Bv-DS1 filtrate. This effect may contribute to the suppression of nematode infection at early stages, as well as the reduction in the number of galls and egg masses per root system in the Bv-DS1-inoculated pots. These studies suggested that B. velezensis culture filtrate may contain similar nematicidal metabolites, which are toxic to PPNs. In recent years, a large number of volatile organic compounds (VOCs) with strong nematicidal activity were identified from Bacillus strains (Du et al., 2017; Chen et al., 2021; Yin et al., 2021a; Ye et al., 2022). Future studies are needed to identify the VOCs with nematicidal activity from Bv-DS1, in order to elucidate the specific mechanism of the Bv-DS1 strain biocontrol against M. incognita.
Induced systemic resistance (ISR) of the host plant is an important strategy of biocontrol microorganisms against plant pathogens. Previous studies revealed that Bacillus strains, including B.velezensis, were able to trigger ISR in nematode-infected plants that effectively reduced the disease progression (Ayaz et al., 2021; Yin et al., 2021b; Tian et al., 2022). In the current study, the split-root experiments demonstrated that pre-treatment with Bv-DS1 in half of the tomato roots failed to significantly reduce the number of galls and egg masses on the other half of the roots inoculated with RKN. This indicates that Bv-DS1 does not induce ISR, only local plant defences against RKN in split-root system of tomato. Our data are not in agreement with previous observations revealing the ability of B.velezensis Bv-25 to suppress RKN infection through the ISR mechanism in split-root system of cucumbers (Tian et al., 2022). Interestingly, the findings of Ghahremani et al. (2020) indicated that B. firmus can induce ISR against M. incognita in tomatoes but not in cucumbers in a split-root system. These observations imply that ISR in host plants by Bacillus spp. is dependent on the bacterial strain or plant species. It is therefore possible that the ability of Bv-DS1 to activate the ISR would be observed in other crops when exposed to infection by other PPNs. In plant-RKN interactions, the functions of phytohormones JA and SA have been well documented for their contribution to host plant defence (Molinari et al., 2014; Kammerhofer et al., 2015). Some Bacillus species were able to induce plant resistance against RKN by activating JA-and/or SA signalling. For example, the expression of PR1 and PR3 associated with SA signalling in nematode-infected cucumber roots was induced by Bv-25 (Tian et al., 2022). Wu et al. (2022) reported that the inoculation with B. velezensis GJ-7 strains induced the expression of PnPR1, a SA marker gene, in Panax notoginseng roots, suggesting that SA pathway may contribute to the GJ-7-mediated P. notoginseng resistance against RKN. Bc-cm103 (B. cereus strain) promoted the expression of LOX1 genes related to JA in cucumbers following RKN inoculation for just 6 h (Yin et al., 2021a). The upregulation of JA related genes by B. firmus was observed at 7 days and 40 days after RKN inoculation in tomatoes but no effect was found in cucumbers (Ghahremani et al., 2020). Nematicidal volatiles (MIV and 2-UD) from B. atrophaeus have been reported to upregulate the expression of JA-and SA-related genes (PR1, PR5, and LOX1) in tomato roots (Ayaz et al., 2021). Our results from qRT-PCR showed that individual Bv-DS1 can trigger the upregulation of LOX D and MC expression related to JA genes at 3, 7, 14 days after preinoculation, and the SA marker genes PAL2 and PR were induced from 7 to 14 days. However, the activation of SA and JA marker genes by Bv-DS1 was not observed in tomato roots during nematode infection, suggesting that Bv-DS1-induced resistance against RKN is independent of the JA and SA signalling pathways. Previously, Martínez-Medina et al. (2017) used JA-and SA-impaired tomato plants to confirm the role and timing of SA-and JA pathways in Trichoderma-induced resistance to RKN. These transgenic and mutant tomato plants were used in this study. There was no difference in the development of RKN on SA-or JA mutants with or without Bv-DS1 inoculation. This finding was in line with our observation of the changes in the expression of the SA and JA marker genes induced by Bv-DS1 and RKN, suggesting that JA-and SA-dependent defences were not required for Bv-DS1-mediated protection against RKN in tomatoes.
The tonoplast intrinsic proteins (TIPs) have been described as the most abundant aquaporin proteins localized in the plant tonoplast (Maurel et al., 2009) and play an important role in plant growth and development by regulating the transport of small substrates, such as water, glycerol, ammonia, H2O2, and urea (Höfte et al., 1992; Gerbeau et al., 1999; Soto et al., 2008; Lindahl et al., 2018). TIPs have also been found to regulate plant responses to PPN infection (Szakasits et al., 2009; Xue et al., 2013; Baranowski et al., 2019). In Arabidopsis, the feeding-site (syncytia) formation by cyst nematode is accompanied by reduced expression of several TIP genes (Szakasits et al., 2009; Baranowski et al., 2019). Among them, the characteristic downregulation of TIP1;1 gene was further validated by observing the reduction in accumulation of γ-TIP1; 1-YFP fusion protein in nematode feeding sites (Baranowski et al., 2019), whereas Arabidopsis mutants TIP1;1 exhibited increased susceptibility to Heterodera schachtii (Baranowski et al., 2019), suggesting that TIP1;1 negatively regulated the parasitism of cyst nematodes. In tomatoes, transcriptome data revealed the downregulation of multiple plant aquaporins including TIPs upon RKN infection (Ji et al., 2013; Shukla et al., 2018). Similarly, we found that inoculation with M. incognita resulted in significant downregulation of TIP1.1, TIP1.2, and TIP1.3 genes in tomato. These results indicate there is a tight relationship between TIPs expression and PPN parasitism in plant. Intriguingly, tomato seedlings inoculated with Bv-DS1 had significantly upregulated expression of three TIPs (TIP1.1, TIP1.2, and TIP1.3). More significantly, the suppression of TIP1.1 and TIP1.3 expression by RKNs could be reversed using Bv-DS1 pre-treatment, suggesting that TIPs participate in Bv-DS1-mediated resistance of tomatoes against RKNs. Although a B. megaterium strain was previously reported to regulate aquaporin proteins (ZmPIPs) in maize under salt stress (Marulanda et al., 2010), this was the first report that revealed the putative function of TIPs, the subfamily members of aquaporin, in plant resistance against RKN by Bacillus strains. In addition, Xue et al. (2013) reported that tomato TIP2;3 protein was hijacked by the Mi8D05 effector of M. incognita, which eventually might promote the giant cell development via control of water and solute transport. It has been proposed that the maintenance of turgor pressure in feeding cells of PPNs is very important for the nutrient sink function of nematodes (Böckenhoff and Grundler, 1994; Hofmann and Grundler, 2007). Thus, we hypothesized that the modification of tomato TIP aquaporin expression by Bv-DS1 may interfere with the hydraulic and turgor pressure of giant cells by regulating the flux of water and solute metabolites, resulting in a suppression of RKN development. Based on our data, it is worth clarifying the role of TIPs in Bv-DS1-induced resistance against RKN by using a genetic method to construct TIP mutants in tomatoes.
Conclusion
In summary, the B.velezensis strain YS-AT-DS1 exhibited IAA production, antifungal, and nematicidal activities against M. incognita in tomatoes. The mechanisms details of its resistance to M. incognita were related with neither the ISR nor the JA-and SA-dependent pathways, but might be closely related with the regulation of water and solute transport via activating the expression of TIP1.1 and TIP1.3, under the described conditions. Further studies are required to understand the function of aquaporin protein TIPs involved in Bv-DS1-mediated resistance against M. incognita. In addition, genome analysis illustrated that it encodes several potential genes implicated in biocontrol effects. Thus, this study provided a theoretical reference for B. velezensis strain commercialization as a potential candidate for eco-friendly biofertilizer. Its biocontrol effects on field crops and its potential plant growth promoting activities, and the mechanisms by which they occur, merit further investigation.
Data availability statement
The datasets presented in this study can be found in online repositories. The names of the repository/repositories and accession number(s) can be found at: https://www.ncbi.nlm.nih.gov/, CP102866.
Author contributions
YH and ZY conceived and designed the experiments, analyzed the data, and wrote the manuscript. YH, ZY, JY, YW, YL, SW, and FP performed the experiments. All authors have read and approved the final manuscript.
Funding
This work was supported by the National Key R & D Program of China (2021YFD1500803), Youth Innovation Promotion Association of CAS (Nos. 2019233 and 2020236).
Acknowledgments
We appreciate Zhihuang Xie for his help with organizing the figures.
Conflict of interest
The authors declare that the research was conducted in the absence of any commercial or financial relationships that could be construed as a potential conflict of interest.
Publisher’s note
All claims expressed in this article are solely those of the authors and do not necessarily represent those of their affiliated organizations, or those of the publisher, the editors and the reviewers. Any product that may be evaluated in this article, or claim that may be made by its manufacturer, is not guaranteed or endorsed by the publisher.
Supplementary material
The Supplementary material for this article can be found online at: https://www.frontiersin.org/articles/10.3389/fmicb.2022.1035748/full#supplementary-material
References
Adam, M., Westphal, A., Hallmann, J., and Heuer, H. (2014). Specific microbial attachment to root knot nematodes in suppressive soil. Appl. Environ. Microbiol. 80, 2679–2686. doi: 10.1128/AEM.03905-13
Aktar, M. W., Sengupta, D., and Chowdhury, A. (2009). Impact of pesticides use in agriculture: their benefits and hazards. Interdiscip. Toxicol. 2, 1–12. doi: 10.2478/v10102-009-0001-7
Ayaz, M., Ali, Q., Farzand, A., Khan, A. R., Ling, H., and Gao, X. (2021). Nematicidal volatiles from bacillus atrophaeus GBSC56 promote growth and stimulate induced systemic resistance in tomato against Meloidogyne incognita. Int. J. Mol. Sci. 22:5049. doi: 10.3390/ijms22095049
Baranowski, Ł., Różańska, E., Sańko-Sawczenko, I., Matuszkiewicz, M., Znojek, E., Filipecki, M., et al. (2019). Arabidopsis tonoplast intrinsic protein and vacuolar H+-adenosinetriphosphatase reflect vacuole dynamics during development of syncytia induced by the beet cyst nematode Heterodera schachtii. Protoplasma 256, 419–429. doi: 10.1007/s00709-018-1303-4
Blin, K., Shaw, S., Steinke, K., Villebro, R., Ziemert, N., Lee, S. Y., et al. (2019). AntiSMASH 5.0: updates to the secondary metabolite genome mining pipeline. Nucleic Acids Res. 47, W81–W87. doi: 10.1093/nar/gkz310
Blok, V. C., Jones, J. T., Phillips, M. S., and Trudgill, D. L. (2008). Parasitism genes and host range disparities in biotrophic nematodes: the conundrum of polyphagy versus specialisation. BioEssays 30, 249–259. doi: 10.1002/bies.20717
Böckenhoff, A., and Grundler, F. M. W. (1994). Studies on the nutrient-uptake by the beet cyst-nematode Heterodera schachtii by in situ microinjection of fluorescent-probes into the feeding structures in Arabidopsis thaliana. Parasitology 109, 249–255. doi: 10.1017/S003118200007637X
Bric, J. M., Bostock, R. M., and Silverstone, S. E. (1991). Rapid in situ assay for indoleacetic acid production by bacteria immobilized on a nitrocellulose membrane. Appl. Environ. Microbiol. 57, 535–538. doi: 10.1128/AEM.57.2.535-538.1991
Cao, H., Jiao, Y., Yin, N., Li, Y., Ling, J., Mao, Z., et al. (2019). Analysis of the activity and biological control efficacy of the Bacillus subtilis strain Bs-1 against Meloidogyne incognita. Crop Prot. 122, 125–135. doi: 10.1016/j.cropro.2019.04.021
Chen, Y. X., Chen, Y. S., Shi, C. M., Huang, Z. B., Zhang, Z., Li, S. K., et al. (2018). SOAPnuke: a map reduce acceleration supported software for integrated quality control and preprocessing of high-throughput sequencing data. Gigascience 7, 1–6. doi: 10.1093/gigascience/gix120
Chen, W., Wang, J., Huang, D., Cheng, W., Shao, Z., Cai, M., et al. (2021). Volatile organic compounds from bacillus aryabhattai MCCC 1K02966 with multiple modes against Meloidogyne incognita. Molecules 27:103. doi: 10.3390/molecules27010103
Chen, M., Wang, J., Liu, B., Zhu, Y., Xiao, R., Yang, W., et al. (2020). Biocontrol of tomato bacterial wilt by the new strain bacillus velezensis FJAT-46737 and its lipopeptides. BMC Microbiol. 20, 160–112. doi: 10.1186/s12866-020-01851-2
Cheng, W., Yang, J., Nie, Q., Huang, D., Yu, C., and Zheng, L. (2017). Volatile organic compounds from Paenibacillus polymyxa KM2501-1 control Meloidogyne incognita by multiple strategies. Sci. Rep. 7:16213. doi: 10.1038/s41598-017-16631-8
Coyne, D. L., Cortada, L., Dalzell, J. J., Claudius-cole, A. O., Haukeland, S., Luambano, N., et al. (2018). Plant-parasitic nematodes and food security in sub-Saharan Africa. Annu. Rev. Phytopathol. 56, 381–403. doi: 10.1146/annurev-phyto-080417-045833
Das, S., Abdul Wadud, M., and Khokon, A. M. (2021). Functional evaluation of culture filtrates of Bacillus subtilis and Pseudomonas fluorescens on the mortality and hatching of Meloidogyne javanica. Saudi J. Biol. Sci. 28, 1318–1323. doi: 10.1016/j.sjbs.2020.11.055
Davies, K. G., and Curtis, R. (2011). Cuticle surface coat of plant-parasitic nematodes. Annu. Rev. Phytopathol. 49, 135–156. doi: 10.1146/annurev-phyto-121310-111406
Davies, L. J., and Elling, A. A. (2015). Resistance genes against plant-parasitic nematodes: a durable control strategy? Nematology 17, 249–263. doi: 10.1163/15685411-00002877
Dehghanian, S. Z., Abdollahi, M., Charehgani, H., and Niazi, A. (2020). Combined of salicylic acid and Pseudomonas fluorescens CHA0 on the expression of PR1 gene and control of Meloidogyne javanica in tomato. Biol. Control 141:104134. doi: 10.1016/j.biocontrol.2019.104134
Ding, H. X., Mo, W. D., Yu, S., Cheng, H. H., Peng, L. J., and Liu, Z. Y. (2021). Whole genome sequence of bacillus velezensis strain GUMT319: a potential biocontrol agent against tobacco black shank disease. Front. Microbiol. 12:658113. doi: 10.3389/fmicb.2021.658113
Du, C. Y., Cao, S. Y., Shi, X. Y., Nie, X. T., Zheng, J. S., Deng, Y., et al. (2017). Genetic and biochemical characterization of a gene operon for trans-aconitic acid, a novel nematicide from bacillus thuringiensis. J. Biol. Chem. 292, 3517–3530. doi: 10.1074/jbc.M116.762666
El-Hadad, M. E., Mustafa, M. I., Selim, S. M., El-Tayeb, T. S., Mahgoob, A. E. A., and Aziz, N. H. A. (2011). The nematicidal effect of some bacterial biofertilizers on Meloidogyne incognita in sandy soil. Braz. J. Microbiol. 42, 105–113. doi: 10.1590/S1517-83822011000100014
Elling, A. A. (2013). Major emerging problems with minor Meloidogyne species. Phytopathology 103, 1092–1102. doi: 10.1094/PHYTO-01-13-0019-RVW
Fan, B., Wang, C., Song, X., Ding, X., Wu, L., Wu, H., et al. (2018). Bacillus velezensis FZB42 in 2018: the gram-positive model strain for plant growth promotion and biocontrol. Front. Microbiol. 9:2491. doi: 10.3389/fmicb.2018.02491
Gao, L., Ma, J., Liu, Y., Huang, Y., Mohamad, O. A. A., and Jiang, H. C. (2021). Diversity and biocontrol potential of cultivable endophytic bacteria associated with halophytes from the West Aral Sea basin. Microorganisms 9:1448. doi: 10.3390/microorganisms9071448
Gao, H. J., Qi, G. F., Yin, R., Zhang, H. C., Li, C. G., and Zhao, X. Y. (2016). Bacillus cereus strain S2 shows high nematicidal activity against Meloidogyne incognita by producing sphingosine. Sci. Rep. 6:28756. doi: 10.1038/srep28756
Gao, Z. F., Zhang, B. J., Liu, H. P., Han, J. C., and Zhang, Y. J. (2017). Identification of endophytic bacillus velezensis ZSY-1 strain and antifungal activity of its volatile compounds against Alternaria solani and Botrytis cinerea. Biol. Control 105, 27–39. doi: 10.1016/j.biocontrol.2016.11.007
Gerbeau, P., Güçlü, J., Ripoche, P., and Maurel, C. (1999). Aquaporin Nt-TIPa can account for the high permeability of tobacco cell vacuolar membrane to small neutral solutes. Plant J. 18, 577–587. doi: 10.1046/j.1365-313x.1999.00481.x
Ghahremani, Z., Escudero, N., Beltrán-Anadón, D., Saus, E., Cunquero, M., Andilla, J., et al. (2020). Bacillus firmus strain I-1582, a nematode antagonist by itself and through the plant. Front. Plant Sci. 11:796. doi: 10.3389/fpls.2020.00796
Ghahremani, Z., Escudero, N., Saus, E., Gabaldón, T., and Sorribas, F. J. (2019). Pochonia chlamydosporia induces plant-dependent systemic resistance to Meloidogyne incognita. Front. Plant Sci. 10:945. doi: 10.3389/fpls.2019.00945
Giannakou, I. O., and Anastasiadis, I. (2005). Evaluation of chemical strategies as alternatives to methyl bromide for the control of root-knot nematodes in greenhouse cultivated crops. Crop Prot. 24, 499–506. doi: 10.1016/j.cropro.2004.09.007
Giné, A., Carrasquilla, M., Martínez-Alonso, M., Gaju, N., and Sorribas, F. J. (2016). Characterization of soil suppressiveness to root-knot nematodes in organic horticulture in plastic greenhouse. Front. Plant Sci. 7:164. doi: 10.3389/fpls.2016.00164
Grady, E. N., MacDonald, J., Ho, M. T., Weselowski, B., McDowell, T., Solomon, O., et al. (2019). Characterization and complete genome analysis of the surfactin-producing, plant-protecting bacterium bacillus velezensis. 9D-6. BMC Microbiol. 19:5. doi: 10.1186/s12866-018-1380-8
Han, V. C., Yu, N. H., Yoon, H., Ahn, N. H., Son, Y. K., Lee, B. H., et al. (2022). Identification, characterization, and efficacy evaluation of bacillus velezensis for shot-hole disease biocontrol in flowering cherry. Plant Pathol. J. 38, 115–130. doi: 10.5423/PPJ.OA.01.2022.0004
Hofmann, J., and Grundler, F. M. W. (2007). How do nematodes get their sweets? Solute supply to sedentary plant-parasitic nematodes. Nematology 9, 451–458. doi: 10.1163/156854107781487305
Höfte, H., Hubbard, L., Reizer, J., Ludevid, D., Herman, E. M., and Chrispeels, M. J. (1992). Vegetative and seed-specific forms of tonoplast intrinsic protein in the vacuolar membrane of Arabidopsis thaliana. Plant Physiol. 99, 561–570. doi: 10.1104/pp.99.2.561
Hu, H. J., Gao, Y., Li, X., Chen, S., Yan, S., and Tian, X. (2020). Identification and nematicidal characterization of proteases secreted by endophytic bacteria Bacillus cereus BCM2. Phytopathology 110, 336–344. doi: 10.1094/PHYTO-05-19-0164-R
Hu, Y. F., You, J., Li, C. J., Pan, F. J., and Wang, C. L. (2019). Assessing the effects of water extract of Narcissus tazetta bulb on hatching, mortality, chemotaxis and reproduction of the soybean cyst nematode, Heterodera glycines. Nematology 22, 53–62. doi: 10.1163/15685411-00003280
Huang, Y., Xu, C. K., Ma, L., Zhang, K. Q., Duan, C. Q., and Mo, M. H. (2010). Characterisation of volatiles produced from bacillus megaterium YFM3. 25 and their nematicidal activity against Meloidogyne incognita. Eur. J. Plant Pathol. 126, 417–422. dio: 10.1007/s10658-009-9550-z. doi: 10.1007/s10658-009-9550-z
Hussey, R. S., and Barker, K. R. (1973). A comparison of methods of collecting inocula of Meloidogyne spp. including a new technique. Plant Dis. Rep. 57, 1025–1028.
Jamal, Q., Cho, J. Y., Moon, J. H., Munir, S., Anees, M., and Kim, K. Y. (2017). Identification for the first time of Cyclo(d-pro-l-Leu) produced by bacillus amyloliquefaciens Y1 as a Nematocide for control of Meloidogyne incognita. Molecules 22:1839. doi: 10.3390/molecules22111839
Ji, H., Gheysen, G., Denil, S., Lindsey, K., Topping, J. F., Nahar, K., et al. (2013). Transcriptional analysis through RNA sequencing of giant cells induced by Meloidogyne graminicola in rice roots. J. Exp. Bot. 64, 3885–3898. doi: 10.1093/jxb/ert219
Jiang, C. H., Liao, M. J., Wang, H. K., Zheng, M. Z., Xu, J. J., and Guo, J. H. (2018). Bacillus velezensis, a potential and efficient biocontrol agent in control of pepper gray mold caused by botrytis cinerea. Biol. Control 126, 147–157. doi: 10.1016/j.biocontrol.2018.07.017
Jin, N., Chen, Y. P., Liu, Q., and Jian, H. (2022). Research progresses in occurrence, diagnoses, pathogenic mechanisms and integrated management of vegetable root-knot nematodes in China. J. Plant. Dis. Protect. 49, 424–438. doi: 10.13802/j.cnki.zwbhxb.2022.2022828
Jin, N., Liu, S. M., Peng, H., Huang, W. K., Kong, L. A., Wu, Y. H., et al. (2019). Isolation and characterization of aspergillus Niger NBC001 underlying suppression against Heterodera glycines. Sci. Rep. 9:591. doi: 10.1038/s41598-018-37827-6
Jin, N., Xue, H., Li, W. J., Wang, X. Y., Liu, Q., Liu, S. S., et al. (2017). Field evaluation of streptomyces rubrogriseus hdz-9-47 for biocontrol of Meloidogyne incognita on tomato. J. Integr. Agr. 16, 1347–1357. doi: 10.1016/S2095-3119(16)61553-8
Jones, J. T., Haegeman, A., Danchin, E. G. J., Gaur, H. S., Helder, J., Jones, M. G., et al. (2013). Top 10 plant-parasitic nematodes in molecular plant pathology. Mol. Plant Pathol. 14, 946–961. doi: 10.1111/mpp.12057
Jordan, S. (2018). Yield to the resistance: the impact of nematode resistant varieties on alfalfa yield. Nat. Resour. Model. 31:e12150. doi: 10.1111/NRM.12150
Kammerhofer, N., Radakovic, Z., Regis, J. M. A., Dobrev, P., Vankova, R., Grundler, F. M. W., et al. (2015). Role of stress-related hormones in plant defence during early infection of the cyst nematode Heterodera schachtii in Arabidopsis. New Phytol. 207, 778–789. doi: 10.1111/nph.13395
Khoja, S., Eltayef, K. M., Baxter, I., Myrta, A., Bull, J. C., and Butt, T. (2021). Volatiles of the entomopathogenic fungus, Metarhizium brunneum, attract and kill plant parasitic nematodes. Biol. Control 152:104472. doi: 10.1016/j.biocontrol.2020.104472
Kim, T. Y., Jang, J. Y., Yu, N. H., Chi, W. J., Bae, C. H., Yeo, J. H., et al. (2018). Nematicidal activity of grammicin produced by Xylaria grammica KCTC 13121BP against Meloidogyne incognita. Pest Manag. Sci. 74, 384–391. doi: 10.1002/ps.4717
Kloepper, J. W., Ryu, C. M., and Zhang, S. A. (2004). Induced systemic resistance and promotion of plant growth by bacillus spp. Phytopathology 94, 1259–1266. doi: 10.1094/PHYTO.2004.94.11.1259
Lahlali, R., Ezrari, S., Radouane, N., Kenfaoui, J., Esmaeel, Q., El Hamss, H., et al. (2022). Biological control of plant pathogens: a global perspective. Microorganisms 10:596. doi: 10.3390/microorganisms10030596
Lalloo, R., Maharajh, D., Görgens, J., and Gardiner, N. (2010). A downstream process for production of a viable and stable Bacillus cereus aquaculture biological agent. Appl. Microbiol. Biot. 86, 499–508. doi: 10.1007/s00253-009-2294-z
Lee, Y. S., and Kim, K. Y. (2016). Antagonistic potential of Bacillus pumilus L1 against root-knot nematode, Meloidogyne arenaria. J. Phytopathol. 164, 29–39. doi: 10.1111/jph.12421
Li, T. Y. (2020). Current situation, species identification and toxicity variation of root-knot nematode in Handan protected vegetables. Master thesis. Handan: Hebei University of Engineering (in Chinese).
Li, M., Bai, Q. J., Xi, X. M., Li, Y. M., and Jian, H. (2017). Identification of the root-knot nematodes on vegetables in greenhouses in Chifeng City of Inner Mongolia. Acta Phytopathol. Sin. 47, 286–288. doi: 10.13926/j.cnki.apps.000011
Li, K. M., Dong, Y. Q., Cao, X. S., and Bayier, (2015). Occurrence and identification of root-knot nematode on greenhouse vegetables in Xinjiang. Plant Prot. 41, 191–194.
Li, D. H., Liu, C. M., Luo, R. B., Sadakane, J., and Lam, T. (2015). MEGAHIT: an ultra-fast single-node solution for large and complex metagenomics assembly via succinct de Bruijn graph. Bioinformatics 31, 1674–1676. doi: 10.1093/bioinformatics/btv033
Li, C. Y., Liu, G. H., Xu, C. C., Lee, G. I., Bauer, P., Ling, H. Q., et al. (2003). The tomato suppressor of prosystemin-mediated responses2 gene encodes a fatty acid desaturase required for the biosynthesis of jasmonic acid and the productiosn of a systemic wound signal for defense gene expression. Plant Cell 15, 1646–1661. doi: 10.1105/tpc.012237
Liang, Y. H. (2017). Investigation and identification of root knot nematode in greenhouse vegetable of Northeast China Master thesis Shenyang: Shenyang Agricultural University (in Chinese)
Lindahl, V., Gourdon, P., Andersson, M., and Hess, B. (2018). Permeability and ammonia selectivity in aquaporin TIP2; 1: linking structure to function. Sci. Rep. 8:2995. doi: 10.1038/s41598-018-21357-2
Liu, H. Y., Zeng, Q. C., Yalimaimaiti, N., Wang, W., Zhang, R. F., and Yao, J. (2021). Comprehensive genomic analysis of bacillus velezensis AL7 reveals its biocontrol potential against Verticillium wilt of cotton. Mol. Gen. Genomics. 296, 1287–1298. doi: 10.1007/S00438-021-01816-8
Martínez-Medina, A., Fernandez, I., Lok, G. B., Pozo, M. J., Pieterse, C. M., and Van Wees, S. C. (2017). Shifting from priming of salicylic acid-to jasmonic acid-regulated defences by Trichoderma protects tomato against the root knot nematode Meloidogyne incognita. New Phytol. 213, 1363–1377. doi: 10.1111/nph.14251
Marulanda, A., Azcón, R., Chaumont, F., Ruiz-Lozano, J. M., and Aroca, R. (2010). Regulation of plasma membrane aquaporins by inoculation with a bacillus megaterium strain in maize (Zea mays L.) plants under unstressed and salt-stressed conditions. Planta 232, 533–543. doi: 10.1007/s00425-010-1196-8
Maurel, C., Santoni, V., Luu, D. T., Wudick, M. M., and Verdoucq, L. (2009). The cellular dynamics of plant aquaporin expression and functions. Curr. Opin. Plant Biol. 12, 690–698. doi: 10.1016/j.pbi.2009.09.002
Mhatre, P. H., Karthik, C., Kadirvelu, K., Divya, K. L., and Shanmuganathan, R. (2019). Plant growth promoting rhizobacteria (PGPR): a potential alternative tool for nematodes bio-control. Biocatal. Agr. Biotech. 17, 119–128. doi: 10.1016/j.bcab.2018.11.009
Molinari, S., Fanelli, E., and Leonetti, P. (2014). Expression of tomato salicylic acid (SA)-responsive pathogenesis-related genes in Mi-1-mediated and SA-induced resistance to root-knot nematodes. Mol. Plant Pathol. 15, 255–264. doi: 10.1111/mpp.12085
Omwega, C. O., Thomason, I. J., and Roberts, P. A. (1988). A non-destructive technique for screening bean germplasm for resistance to Meloidogyne incognita. Plant Dis. 72, 970–972. doi: 10.1094/PD-72-0970
Park, E. J., Jang, H. J., Park, C. S., Lee, S. J., Lee, S., Kim, K. H., et al. (2020). Evaluation of nematicidal activity of Streptomyces yatensis KRA-28 against Meloidogyne incognita. J. Microbiol. Biotechnol. 30, 700–707. doi: 10.4014/jmb.1908.08038
Pocurull, M., Fullana, A. M., Ferro, M., Valero, P., Escudero, N., et al. (2020). Commercial formulates of Trichoderma induce systemic plant resistance to Meloidogyne incognita in tomato and the effect is additive to that of the Mi-1.2 resistance gene. Front. Microbiol. 10:3042. doi: 10.3389/fmicb.2019.03042
Selim, M. E., Mahdy, M. E., Sorial, M. E., Dababat, A. A., and Sikora, R. A. (2014). Biological and chemical dependent systemic resistance and their significance for the control of root-knot nematodes. Nematology 16, 917–927. doi: 10.1163/15685411-00002818
Shahid, M., Gowen, S. R., and Burhan, M. (2022). Studies on the possible role of plant host on the development of root-knot nematode, Meloidogyne javanica and Pasteuria penetrans as affected by different harvesting dates. Plant Prot. 6, 133–141. doi: 10.33804/pp.006.02.4207
Sharma, N., Khanna, K., Manhas, R. K., Bhardwaj, R., Ohri, P., Alkahtani, J., et al. (2020). Insights into the role of Streptomyces hydrogenans as the plant growth promoter, photosynthetic pigment enhancer and biocontrol agent against Meloidogyne incognita in Solanum lycopersicum seedlings. Plants (Basel). 9:1109. doi: 10.3390/plants9091109
Shukla, N., Yadav, R., Kaur, P., Rasmussen, S., Goel, S., Agarwal, M., et al. (2018). Transcriptome analysis of root-knot nematode (Meloidogyne incognita) infected tomato (Solanum lycopersicum) roots reveals complex gene expression profiles and metabolic networks of both host and nematode during susceptible and resistance responses. Mol. Plant Pathol. 19, 615–633. doi: 10.1111/mpp.12547
Silva, S. D., Carneiro, R. M. D. G., Faria, M., Souza, D. A., Monnerat, R. G., and Lopes, R. B. (2017). Evaluation of Pochonia chlamydosporia and Purpureocillium lilacinum for suppression of Meloidogyne enterolobii on tomato and banana. J. Nematol. 49, 77–85. doi: 10.21307/jofnem-2017-047
Soto, G., Alleva, K., Mazzella, M. A., Amodeo, G., and Muschietti, J. P. (2008). AtTIP1;3 and AtTIP5;1, the only highly expressed Arabidopsis pollen-specific aquaporins, transport water and urea. FEBS Lett. 582, 4077–4082. doi: 10.1016/j.febslet.2008.11.002
Sun, X., Zhang, R., Ding, M., Liu, Y., and Li, L. (2021). Biocontrol of the root-knot nematode Meloidogyne incognita by a nematicidal bacterium pseudomonas simiae MB751 with cyclic dipeptide. Pest Manag. Sci. 77, 4365–4374. doi: 10.1002/ps.6470
Szakasits, D., Heinen, P., Wieczorek, K., Hofmann, J., Wagner, F., Kreil, D. P., et al. (2009). The transcriptome of syncytia induced by the cyst nematode Heterodera schachtii in Arabidopsis roots. Plant J. 57, 771–784. doi: 10.1111/j.1365-313X.2008.03727.x
Terefe, M., Tefera, T., and Sakhuja, P. K. (2009). Effect of a formulation of Bacillus firmus on root-knot nematode Meloidogyne incognita infestation and the growth of tomato plants in the greenhouse and nursery. J. Invertebr. Pathol. 100, 94–99. doi: 10.1016/j.jip.2008.11.004
Tian, X. L., Zhao, X. M., Zhao, S. Y., Zhao, J. L., and Mao, Z. C. (2022). The biocontrol functions of bacillus velezensis strain Bv-25 against Meloidogyne incognita. Front. Microbiol. 13:843041. doi: 10.3389/FMICB.2022.843041
Topalović, O., Elhady, A., Hallmann, J., Richert-Pöggeler, K. R., and Heuer, H. (2019). Bacteria isolated from the cuticle of plant-parasitic nematodes attached to and antagonized the root-knot nematode Meloidogyne hapla. Sci. Rep. 9:11477. doi: 10.1038/s41598-019-47942-7
Wang, G. F., Meng, J. F., Tian, T., Xiao, X. Q., Zhang, B., and Xiao, Y. N. (2020). Endophytic bacillus velezensis strain B-36 is a potential biocontrol agent against lotus rot caused by fusarium oxysporum. J. Appl. Microbiol. 128, 1153–1162. doi: 10.1111/jam.14542
Wang, D., Wang, J., Su, P., Dai, J., Tan, X., Zhang, D., et al. (2022). Effects of dazomet combined with Rhodopsesudomonas palustris PSB-06 on root-knot nematode, Meloidogyne incognita infecting ginger and soil microorganisms diversity. Front. Microbiol. 13:1021445. doi: 10.3389/fmicb.2022.1021445
Wei, J. Z., Hale, K., Carta, L., Platzer, E., Wong, C., Fang, S. C., et al. (2003). Bacillus thuringiensis crystal proteins that target nematodes. Proc. Natl. Acad. Sci. U. S. A. 100, 2760–2765. doi: 10.1073/pnas.0538072100
Wilson, M. J., and Jackson, T. A. (2013). Progress in the commercialisation of bionematicides. BioControl 58, 715–722. doi: 10.1007/s10526-013-9511-5
Wu, W. T., Wang, J. J., Wang, Z. H., Guo, L. W., Zhu, S. S., Zhu, Y. Y., et al. (2022). Rhizosphere bacteria from Panax notoginseng against Meloidogyne hapla by rapid colonization and mediated resistance. Front. Microbiol. 13:877082. doi: 10.3389/fmicb.2022.877082
Xiang, N., Lawrence, K. S., Kloepper, J. W., Donald, P. A., and McInroy, J. A. (2017). Biological control of Heterodera glycines by spore-forming plant growth-promoting rhizobacteria (PGPR) on soybean. PLoS One 12:e0181201. doi: 10.1371/journal.pone.0181201
Xu, P., Xie, S., Liu, W., Jin, P., Wei, D., Yaseen, D. G., et al. (2020). Comparative genomics analysis provides new strategies for bacteriostatic ability of bacillus velezensis HAB-2. Front. Microbiol. 11:594079. doi: 10.3389/fmicb.2020.594079
Xue, B. Y., Hamamouch, N., Li, C. Y., Huang, G. Z., Hussey, R. S., Baum, T. J., et al. (2013). The 8D05 parasitism gene of Meloidogyne incognita is required for successful infection of host roots. Phytopathology 103, 175–181. doi: 10.1094/PHYTO-07-12-0173-R
Yamamoto, S., Shiraishi, S., and Suzuki, S. (2015). Are cyclic lipopeptides produced by bacillus amyloliquefaciens S13-3 responsible for the plant defence response in strawberry against Colletotrichum gloeosporioides? Lett. Appl. Microbiol. 60, 379–386. doi: 10.1111/lam.12382
Ye, L., Wang, J. Y., Liu, X. F., Guan, Q., Dou, N. X., Li, J., et al. (2022). Nematicidal activity of volatile organic compounds produced by Bacillus altitudinis AMCC 1040 against Meloidogyne incognita. Arch. Microbiol. 204:521. doi: 10.1007/s00203-022-03024-3
Yin, N., Liu, R., Zhao, J. L., Khan, R. A. A., Li, Y., Ling, J., et al. (2021a). Volatile organic compounds of Bacillus cereus strain Bc-cm103 exhibit fumigation activity against Meloidogyne incognita. Plant Dis. 105, 904–911. doi: 10.1094/PDIS-04-20-0783-RE
Yin, N., Zhao, J. L., Liu, R., Li, Y., Ling, J., Yang, Y. H., et al. (2021b). Biocontrol efficacy of Bacillus cereus strain Bc-cm103 against Meloidogyne incognita. Plant Dis. 105, 2061–2070. doi: 10.1094/PDIS-03-20-0648-RE
Keywords: Bacillus velezensis, biocontrol agent, induced systemic resistance, root-knot nematodes, plant-parasitic nematodes, Meloidogyne incognita
Citation: Hu Y, You J, Wang Y, Long Y, Wang S, Pan F and Yu Z (2022) Biocontrol efficacy of Bacillus velezensis strain YS-AT-DS1 against the root-knot nematode Meloidogyne incognita in tomato plants. Front. Microbiol. 13:1035748. doi: 10.3389/fmicb.2022.1035748
Edited by:
Xiancan Zhu, Anhui Normal University, ChinaReviewed by:
Tariq Mukhtar, Pir Mehr Ali Shah Arid Agriculture University, PakistanShailendra Singh, Invertis University, Bareilly, India
Copyright © 2022 Hu, You, Wang, Long, Wang, Pan and Yu. This is an open-access article distributed under the terms of the Creative Commons Attribution License (CC BY). The use, distribution or reproduction in other forums is permitted, provided the original author(s) and the copyright owner(s) are credited and that the original publication in this journal is cited, in accordance with accepted academic practice. No use, distribution or reproduction is permitted which does not comply with these terms.
*Correspondence: Zhenhua Yu, eXV6aGVuaHVhQGlnYS5hYy5jbg==