- College of Life Sciences and Shandong Engineering Research Center of Plant-Microbia Restoration for Saline-alkali Land and Shandong Key Laboratory of Agricultural Microbiology and National Engineering Laboratory for Efficient Utilization of Soil and Fertilizer Resources, Shandong Agricultural University, Tai’an, China
The multiple-sugar metabolism regulator (MsmR), a transcription factor belonging to the AraC/XylS family, participates in polysaccharide metabolism and virulence. However, the transcriptional regulatory mechanisms of MsmR1 in Paenibacillus polymyxa remain unclear. In this study, knocking out msmR1 was found to reduce polymyxin synthesis by the SC2-M1 strain. Chromatin immunoprecipitation assay with sequencing (ChIP-seq) revealed that most enriched pathway was that of carbohydrate metabolism. Additionally, electromobility shift assays (EMSA) confirmed the direct interaction between MsmR1 and the promoter regions of oppC3, sucA, sdr3, pepF, yycN, PPSC2_23180, pppL, and ydfp. MsmR1 stimulates polymyxin biosynthesis by directly binding to the promoter regions of oppC3 and sdr3, while also directly regulating sucA and influencing the citrate cycle (TCA cycle). In addition, MsmR1 directly activates pepF and was beneficial for spore and biofilm formation. These results indicated that MsmR1 could regulate carbohydrate and amino acid metabolism, and indirectly affect biological processes such as polymyxin synthesis, biofilm formation, and motility. Moreover, MsmR1 could be autoregulated. Hence, this study expand the current knowledge of MsmR1 and will be beneficial for the application of P. polymyxa SC2 in the biological control against the certain pathogens in pepper.
Introduction
Paenibacillus polymyxa, proposed by Ash et al. (1993), is a plant growth-promoting rhizobacteria (PGPR) that contributes to disease prevention and growth promotion by inducing plant systemic resistance, resulting in generation of secondary metabolites (Li et al., 2019; Liu et al., 2021; Yin et al., 2022). Paenibacillus polymyxa is an important strain resource in microbial medicine, microbial environmental remediation, microbial pesticides, and fertilizer research (Timmusk et al., 2005; Jeong et al., 2019). Paenibacillus polymyxa synthesizes polymyxin—an important antibiotic against gram-negative pathogens—via a multienzyme non-ribosomal peptide synthetase system (NRPS; Supplementary Figure S1A; Yu et al., 2015, 2018). Polymyxin is composed of 10 orderly assembled amino acid residues, five of which are L-2,4-diaminobutyric acid (L-Dabs) biosynthesized by 2,4-diaminobutyrate aminotransferase (EctB) (Choi et al., 2009). Moreover, polymyxins are primarily divided into seven types: A, B, C, D, E, M, and P, composed of a tripeptide side chain acylated by a fatty acid at the amino-terminus, and a cyclic heptapeptide (Supplementary Figure S1B). Indeed, carbon sources significantly impact the rate of polymyxin production and biosynthesis, for example, starch can promote polymyxin synthesis (Yu et al., 2018).
Carbohydrates exist in various forms, including monosaccharides, disaccharides, and polysaccharides. Energy released from carbohydrate catabolism is required for metabolic activity and growth (Warda et al., 2016). Pathways of carbohydrate catabolism involve complex networks that regulate gene expression. In bacteria, carbohydrate utilization is regulated by transcription factors (TFs) regulating the expression of genes which encodes transporters and enzymes (Khoroshkin et al., 2016). Efficient utilization of carbohydrates by bacteria is determined by various utilization systems and carbohydrate transporters (Warda et al., 2016).
Indeed, TFs have essential roles in regulating gene expression in all domains of life (Mejía-Almonte et al., 2020; Cortés-Avalos et al., 2021). More specifically, TFs can activate or repress gene transcription by binding to DNA in a manner dependent on environmental factors (Cortés-Avalos et al., 2021). In bacteria, TFs can be classified into at least 19 families based on their amino acid sequences. The largest TFs families are LysR, TetR/ArcR, and AraC/XylS, which together account for 30% of the total number of TFs identified in bacteria (Kotecka et al., 2021). The AraC/XylS family of TFs was originally described by Henikoff et al. (1990); members of this family act as positive transcriptional regulators or as both activators and repressors. Previous studies focusing on the TFs of RhaR (Kolin et al., 2008; Kettle, 2013), AraC (Schleif, 2010), UreR (Parra and Collins, 2012), and MelR (Belyaeva et al., 2000) in Escherichia coli, MsmR (Nakata et al., 2005) in Streptococcus, XylS (Zwick et al., 2013) in Pseudomonas putida, and Toxt (Lowden et al., 2010) in Vibrio cholerae revealed that they contain a highly conserved C-terminal DNA binding domain (DBD) and a variable ligand-binding domain (LBD) at the N-terminal (Bustos and Schleif, 1993; Cortés-Avalos et al., 2021). The DBD contains approximately 100 amino acids, with approximately 20% sequence identity in bacteria, and seven α-helices, forming two helix-turn-helix (HTH) DNA-binding motifs (Egan, 2002; Gahlot et al., 2021). While the DBD is in contact with RNA polymerase (RNAP) for DNA binding and transcription (Ibarra et al., 2008), the LBD is involved in dimerization and effector/signal recognition (Egan, 2002). The AraC/XylS family transcriptional regulators control multiple functions, such as carbon metabolism, amino acid metabolism, stress response (e. g. Rob, SoxS, PliA, and OpiA were involved in stress response in Erwinia amylovora; Pletzer et al., 2014), and quorum sensing (Cortés-Avalos et al., 2021; Kotecka et al., 2021).
The MsmR of the AraC/XylS family is involved in polysaccharide metabolism (Russell et al., 1992). In Streptococcus mutants, MsmR positively regulates an operon for the uptake and catabolism of carbon sources (Russell et al., 1992). In Streptococcus pyogenes, MsmR positively regulates fibronectin-and collagen-binding T-antigen (FCT) region genes, including nra, which inhibit the expression of crucial virulence factors (Kreikemeyer et al., 2007). Meanwhile, the transcriptional regulatory mechanisms of MsmR1 in P. polymyxa remain unclear.
Paenibacillus polymyxa SC2 was isolated from the rhizosphere of pepper plants in Guizhou province, China (Ma et al., 2011). Paenibacillus polymyxa SC2-M1 is spontaneously mutated from P. polymyxa SC2 during strain cultivation, making it suitable for molecular manipulations (Hou et al., 2016). A previous study reported that the msmR1 gene is upregulated by 2.17-fold during the interaction between P. polymyxa SC2 and pepper (Liu et al., 2021), indicating that msmR1 may play an important role in this interaction. In the present study, we aimed to explore the regulatory mechanism of the transcription factor MsmR1 in polymyxin synthesis and related biological processes in P. polymyxa SC2. To this end, chromatin immunoprecipitation with sequencing (ChIP-seq) was performed and the results were verified by electrophoretic mobility shift assay (EMSA). The oppC3, sucA, sdr3, pepF, yycN, PPSC2_23180, pppL, and ydfp genes were found to be directly regulated by the global regulator MsmR1; the functions of these genes were further explored to delineate the regulatory mechanism of MsmR1. The results of this study provide a theoretical basis for the improved application of P. polymyxa SC2 in the biological control against the certain pathogens.
Materials and methods
Bacterial strains and culture conditions
The strains and plasmids used in this work are presented in Supplementary Table S1. Paenibacillus polymyxa SC2-M1 and its mutants, Escherichia coli DH5α, E. coli BL21, and Trans 110 were cultured in Luria-Bertani (LB) broth at 37°C. When plasmids were presented in cells, antibiotics were added to LB medium as follows: chloramphenicol (6 μg/ml for strain SC2-M1 and its mutants), kanamycin (50 μg/ml), tetracycline (20 μg/ml), ampicillin (100 μg/ml), and erythromycin (12.5 μg/ml). To measure carbon source utilization by the strains, basal medium (MgSO4 0.02%, NaCl 0.02%, (NH4)2SO4 0.8%, K2HPO4 0.368%, KH2PO4 0.132%) with a 1% sole carbon source was used.
Competent cells of E. coli were prepared using the transformation and storage solution (TSS) method and transformed by the heat pulse method (Chung et al., 1989). Competent cells were prepared and P. polymyxa SC2-M1 were electrotransformed as previously described (Hou et al., 2016).
Plasmid and strain construction
To construct knockout SC2-M1 strain mutants, 650–1,000 bp of homologous arm-flanking target genes were cloned from genomic DNA using PCR. The cat gene, encoding the chloramphenicol resistance cassette, was cloned from pDG1661 (Guérout-Fleury et al., 1996) using PCR to replace the target knockout genes. Two homologous arms and cat cassettes were fused by fusion PCR (Cha-Aim et al., 2012), and the fusion DNA fragments were ligated into the thermo-sensitive elimination plasmid pRN5101 to construct knockout vectors. The plasmid was demethylated by being further transformed into Trans 110. The demethylated knockout vectors were transferred into the SC2-M1 strain. Double-crossover recombinants were detected as previously reported (Hou et al., 2016), and the knockout strains were confirmed by PCR using the corresponding primers (Supplementary Table S2). For in situ complementation of the knockout genes, 650–1,000 bp of homologous arms fused with the corresponding complete target genes were cloned by PCR from genomic DNA (not involved in the resistance cassette), and ligated into pRN5101 to construct the complement vectors. Demethylated complementary vectors were introduced into the mutant strains. Double-crossover recombinants were detected as described above, and the complemented strains were confirmed by PCR using the corresponding primers (Supplementary Table S2). For ChIP-seq analysis, we obtained a Flag-tagged strain using homologous recombination. The Flag-tag was added in the front of termination codon of msmR1 and a chloramphenicol resistance cassette was also added after the Flag tag using overlapping PCR. The homologous arms (650–1,000 bp) were cloned by PCR amplification, and ligated to pRN5101 to construct the plasmid pRN5101-msmR1-Flag. Demethylated pRN5101-msmR1-Flag were introduced into SC2-M1 and double-crossover recombinants were detected as described above, designated as strain SC2-M1-Flag. For overexpression of target genes, fragments carrying complete target genes were cloned from genomic DNA, fused with the promoter fragments, and ligated into pHY300PLK between BglII and SalI sites by ClonExpress MultiS One Step Cloning Kit (Vazyme Biotech, Nanjing, China) for overexpression in SC2-M1. To evaluate the promoter activity of target DNA fragments, the fragments were cloned from genomic DNA, and the promoterless green fluorescent protein (gfp) fragment from pGFP4412 was cloned (Li et al., 2019). The two fragments were fused using PCR and ligated into pHY300PLK between BglII and SalI sites as described above, thus generating the pHY300PLK-promoter-gfp plasmid.
Chromatin immunoprecipitation assay with sequencing (ChIP-seq)
The SC2-M1 and SC2-M1-Flag strains were cultured in 50 ml of LB medium at 37°C with shaking at 180 rpm for 10 h, inoculated with an initial OD600 of 0.8 in 50 ml of LB broth by 2% (v/v), and cultured at 37°C with shaking at 180 rpm for 7 h. The two strains were then fixed with 1% formaldehyde for 10 min at 28°C and the reaction was stopped with 125 mM glycine for 5 min. The cells of the two strains were washed thrice with phosphate buffer solution (PBS) at 8000 rpm for 5 min at 4°C and stored at-80°C. ChIP was performed by Wuhan IGENEBOOK Biotechnology Co. Ltd., China. The manufacturer’s instructions of Illumina TruSeq ChIP Sample Prep Set A were followed to construct sequencing libraries using chromatin immunoprecipitated DNA which was then sequenced on an Illumina Xten using the PE 150 method (Chen et al., 2021). The obtained reads were filtered by Trimmomatic (v 0.30). Clean reads were then mapped to the P. polymyxa SC2 genome using Bwa (v 0.7.15), which allowed for up to two mismatches (Pjanic, 2017). MsmR1 peaks were produced and visualized using MACS software and Integrative Genomics Viewer (IGV, v 2.3.91), respectively (Zhang et al., 2008; Thorvaldsdóttir et al., 2013). To understand the potential roles of genes, Kyoto Encyclopedia of Genes and Genomes (KEGG) and multiple EM for motif elicitation (MEME) were used for pathway enrichment analysis and motif analysis, respectively (Chen et al., 2021; Wang et al., 2022).
Quantitative real-time PCR
SteadyPure Universal RNA Extraction Kit (Accurate Biology, Hunan, China) was used to purify the total RNA from the target bacteria. cDNA was obtained from 1 μg of total RNA using the Evo M-MLV RT Kit with gDNA clean for qPCR II (Accurate Biology, Hunan, China). qRT-PCR analysis was performed on a Roter-Gene Q PCR system using the SYBR Green Pro Taq HS qPCR Kit (Accurate Biology, Hunan, China). The relative expression of target genes was calculated using the 2−ΔΔCt method (Liu et al., 2021). The housekeeping gene glyceraldehyde-3-phosphate dehydrogenase (gapdh) was used as an internal control.
Purification of MsmR1 protein and electromobility shift assays
An 828 bp fragment (275 amino acids) of msmR1 was cloned from the genomic DNA of strain SC2-M1 using primers MsmR1F and MsmR1R (Supplementary Table S2). The fragments of msmR1 and plasmid pET-28b(+) were simultaneously cleaved with XhoI and SalI, purified, ligated using T4 DNA ligase, and transferred into E. coli DH5α to obtain pET28b(+)-msmR1.
To overexpress the MsmR1 protein, the plasmid pET28b(+)-msmR1 was transferred into E. coli BL21. The transformants were cultured in kanamycin-resistant LB broth at 37°C with shaking at 180 rpm for 10 h. Next, the cells were inoculated into 50 ml of kanamycin-resistant LB broth at 2% (v/v) and cultured at 37°C with shaking at 180 rpm for approximately 1.2 h until the OD600 reached 0.5–0.6. Then the cells were induced by 0.4 mM IPTG at 16°C for 20 h, harvested, washed, resuspended in PBS, and sonicated on ice. MsmR1-His6 protein was purified using BeyoGold™ His-tag Purification Resin (Beyotime, China) following the manufacturer’s instructions. The purified protein was added to a final concentration of 10% (v/v) glycerol and stored at-80°C. EMSA was performed using a chemiluminescent EMSA kit (Beyotime, China; Zhang et al., 2021). The concentration of labeled probes and competitive probes were about 0.4 and 4 μM, respectively. The gradient concentration of protein were set up for the EMSA experiment.
Detection of the fluorescence intensity of promotors
Promoter reporter plasmids were transformed into E. coli DH5α cells. The strains were inoculated in LB plate containing 20 μg/ml tetracycline and fluorescence was observed with an OLYMPUS fluorescence microscope at 1000× (Li et al., 2019). The fluorescence intensity of the promoters was detected at different time points as previously reported (Li et al., 2019).
Antibacterial activity analysis
An Oxford cup assay was used to evaluate polymyxin production by the SC2-M1 strain, as previously described (Liu et al., 2021). Bacteria were inoculated in liquid LB medium and cultured at 37°C with shaking at 180 rpm for 10 h. Bacteria were then inoculated with an initial OD600 of 0.8 in 50 ml fermentation medium (sucrose 40 g/l, (NH4)2SO4 8 g/l, CaCO3 5 g/l, KH2PO4 0.2 g/l, MgSO4 0.2 g/l, NaCl 0.2 g/l) at 2% (v/v) and cultured at 37°C with shaking at 180 rpm for 48 h.
Motility assay
A motility assay was performed as previously reported (Guo et al., 2019). Bacteria were cultured on LB medium for approximately 12 h, after which the OD600 was adjusted to 0.6. Diluted cell solutions (2 μl) were added to the center of the plate containing 0.5% glucose, 0.22% NaCl, 0.13% yeast extract, 0.45% tryptone, and 0.5% agar.
Growth curve assay
Bacteria were cultured overnight in liquid LB medium and adjusted to an OD600 of 0.8. Diluted strain solutions were inoculated at 2% in 50 ml of fresh LB medium. The bacteria were cultured at 37°C with shaking at 180 rpm. The OD600 and number of live bacterial cells were measured every 3 h. A total of 100 μl of the strain solution was serially diluted with 900 μl of sterile water; 100 μl of the dilution was then spread on an LB plate. The plates were subsequently incubated for 24 h at 37°C.
The growth curves of bacteria on the carbon sources were determined using a basal medium. Bacteria were cultured overnight in LB medium. The cultures were centrifuged, washed thrice with basal medium, and resuspended to inoculate 50 ml of fresh basal medium with 1% sole carbon source; the original OD600 value was approximately 0.1. Cultures were incubated at 37°C with shaking at 180 rpm, and growth curves were determined according to OD600 every 3 h.
Statistical analysis
For data analysis, the analysis of variance (ANOVA) and Least Significant Difference (LSD) test (p ≤ 0.05) were performed using statistical software SPSS (v 19.0, SPSS Inc., Chicago, IL, USA; Wang et al., 2020).
Results
Overview of the transcription factor MsmR1 from Paenibacillus polymyxa SC2
The MsmR1 of strain SC2, which is classified as an AraC/XylS family transcriptional regulator, contains two domains: an N-terminal heterologous dimerization domain and a C-terminal DNA-binding domain (Figure 1A). The C-terminus of MsmR1 shows high similarities to that of AraC family members: RhaR, AraC, UreR, MelR, MsmR, XylS, and Toxt. Seven α-helix were identified in the C-terminal domain of MsmR1, which combined to form two helix-turn-helix (HTH) motifs (Figure 1A).
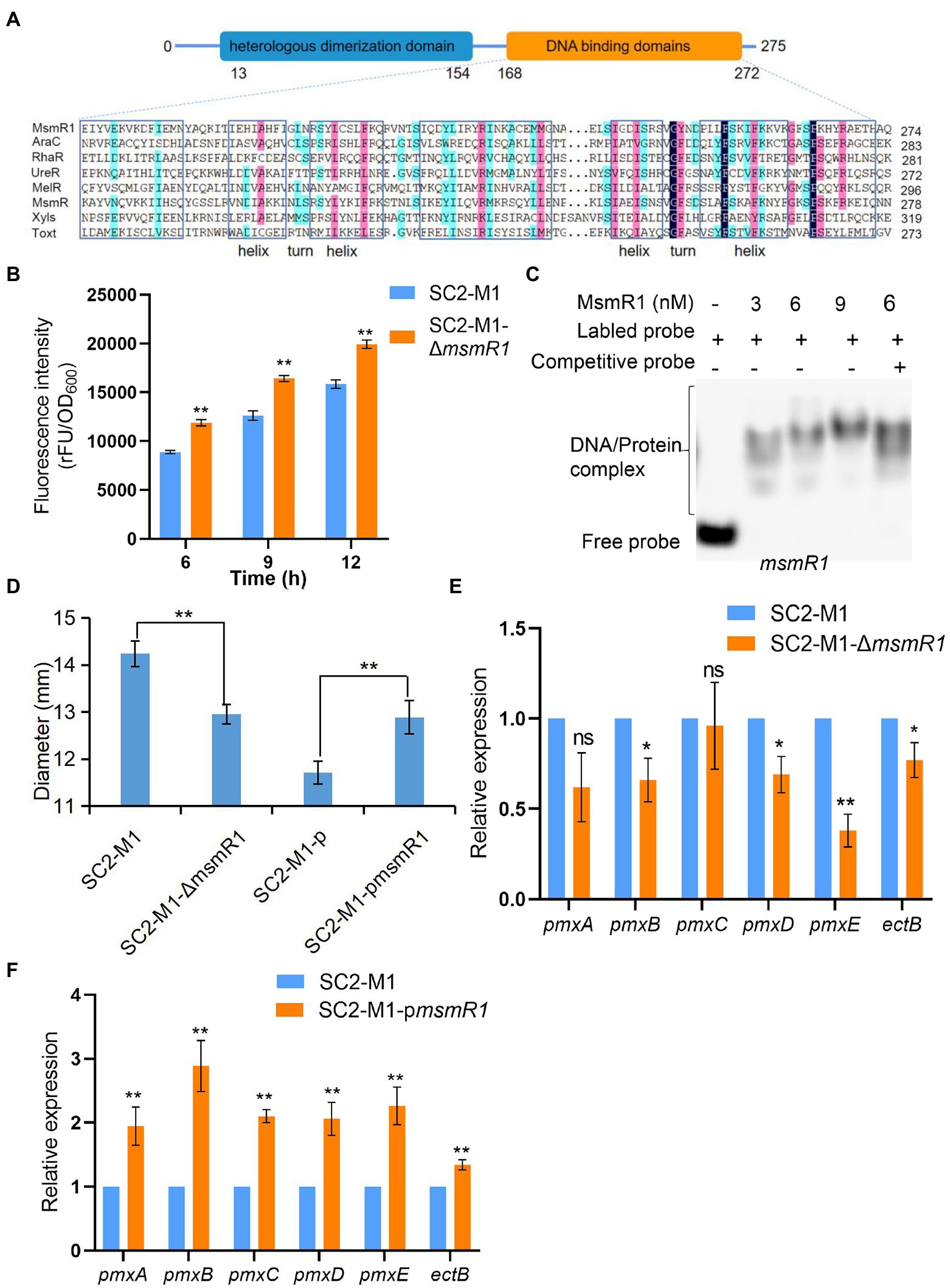
Figure 1. Characteristics of MsmR1 protein in Paenibacillus polymyxa SC2-M1. (A) Homologous alignment of MsmR1 protein with several AraC family members: AraC (UniProtKB/Swiss-Prot: P0A9E0), RhaR (UniProtKB/Swiss-Prot: P09378), UreR (UniProtKB/Swiss-Prot: P32326), MelR (UniProtKB/Swiss-Prot: P0ACH8), MsmR (UniProtKB/Swiss-Prot: Q00753), XylS (UniProtKB/Swiss-Prot: P07859), and Toxt (UniProtKB/Swiss-Prot: A5F384). Sequences were aligned by DNAMAN software. (B) Fluorescence intensity of msmR1 promoter in strains SC2-M1 and SC2-M1-ΔmsmR1 detected at different time points. (C) EMSA results depicting MsmR1 binding to its own promoter. (D) Size of the inhibition zone between the SC2-M1, SC2-M1-ΔmsmR1, SC2-M1-p, and SC2-M1-pmsmR1 strains was determined using the Oxford cup method. (E) Relative expression of genes related to polymyxin synthesis gene cluster in strain SC2-M1 and SC2-M1-ΔmsmR1. (F) Relative expression of genes related to polymyxin synthesis gene cluster in the control strain SC2-M1-p and the overexpression strain SC2-M1-pmsmR1. *p < 0.05 and **p < 0.01; ns, p > 0.05.
AraC family TFs are often auto-regulated (Sun et al., 2016). Hence, the promoter activity of msmR1 was evaluated using the pHY300PLK-msmR1-gfp plasmid. This plasmid was found to possess promoter activity in E. coli DH5α cells. In contrast, the control (pHY300PLK-gfp) showed no fluorescence (Supplementary Figure S2A). The pHY300PLK-msmR1-gfp plasmid was introduced into the strains SC2-M1 and SC2-M1-ΔmsmR1 which was a msmR1 knock out strain obtained via homologous recombination (Supplementary Figure S2B). The fluorescence intensity of the strain SC2-M1-ΔmsmR1 was significantly higher than that of the SC2-M1 strain (Figure 1B), revealing that MsmR1 could auto-regulate its transcription. EMSA was further performed to determine whether MsmR1 is capable of direct auto-regulation. MsmR1 was found to specifically bind to its own promoter resulting in a retarded band (Figure 1C), indicating that MsmR1 auto-regulates its expression.
To investigate the function of MsmR1, msmR1 knock out strain SC2-M1-ΔmsmR1 and overexpression strain SC2-M1-pmsmR1 formed by ligation into pHY300PLK plasmid (Supplementary Figure S2C), were used. Meanwhile, SC2-M1-p was set as the control. Notably, the amount of polymyxin in the mutant SC2-M1-ΔmsmR1 was significantly less than that in the SC2-M1 strain; the diameter of the inhibitory zone was decreased by 9.0% (Figure 1D; Supplementary Figure S2D). The qRT-PCR results revealed that the polymyxin synthetase genes were downregulated in the knockout strain compared with SC2-M1 (Figure 1E). In SC2-M1-pmsmR1, the inhibitory zone diameter was increased by 10.03% compared with SC2-M1-p (Figure 1D; Supplementary Figure S2D). The results were consistent with the qRT-PCR results which presented a significant increase in expression of polymyxin synthetase genes in SC2-M1-pmsmR1 when compared with that in SC2-M1-p (Figure 1F).
Given that AraC family members are involved in carbohydrate metabolism (Cortés-Avalos et al., 2021), the growth curves of the SC2-M1 and SC2-M1-ΔmsmR1 strains were determined using LB and basal media with sole carbon sources. Although phenotypic differences were noted between the two strains using glucose, xylose, galactose, fructose, and lactose, the differences were not significant (Supplementary Figure S2E).
Identification of MsmR1 binding genes
To determine the target genes directly regulated by MsmR1 in P. polymyxa SC2, ChIP-seq experiments were performed using C-terminal FLAG-tagged msmR1 in SC2-M1. The qRT-PCR results demonstrated that the expression of msmR1 was high during the early stage and low during the decline phase (Supplementary Figure S3A). Based on the qRT-PCR results and the growth curve, a culture time of 7 h (mid-logarithmic phase) was selected for subsequent ChIP-seq analysis (Supplementary Figure S3).
Approximately 3.83–4.86 million reads in the ChIP-seq results were derived from the six samples, which were mapped to the P. polymyxa SC2 genome (Supplementary Table S3). Overall, 649 peaks were identified between the two strains, SC2-M1 and SC2-M1-Flag. Among these, 96.15% were located in the coding sequence (CDS) and 3.85% were located in the intergenic regions. To categorize the functions of MsmR1 target genes, KEGG enrichment analysis was performed. The target genes were enriched in various functions, including cellular processes (1.56%), environmental information processing (6.25%), genetic information processing (10.94%), and metabolism (81.25%; Supplementary Table S4). In the metabolism category, carbohydrate metabolism was the most enriched pathway, while amino acid metabolism, metabolism of cofactors and vitamins, lipid metabolism, energy metabolism, and nucleotide metabolism were also significantly enriched (Figure 2). Hence, MsmR1 primarily regulates basal metabolism in SC2 cells. According to the predicted results of MEME, we selected the fragments related to primary metabolism for further verification (Supplementary Table S5).
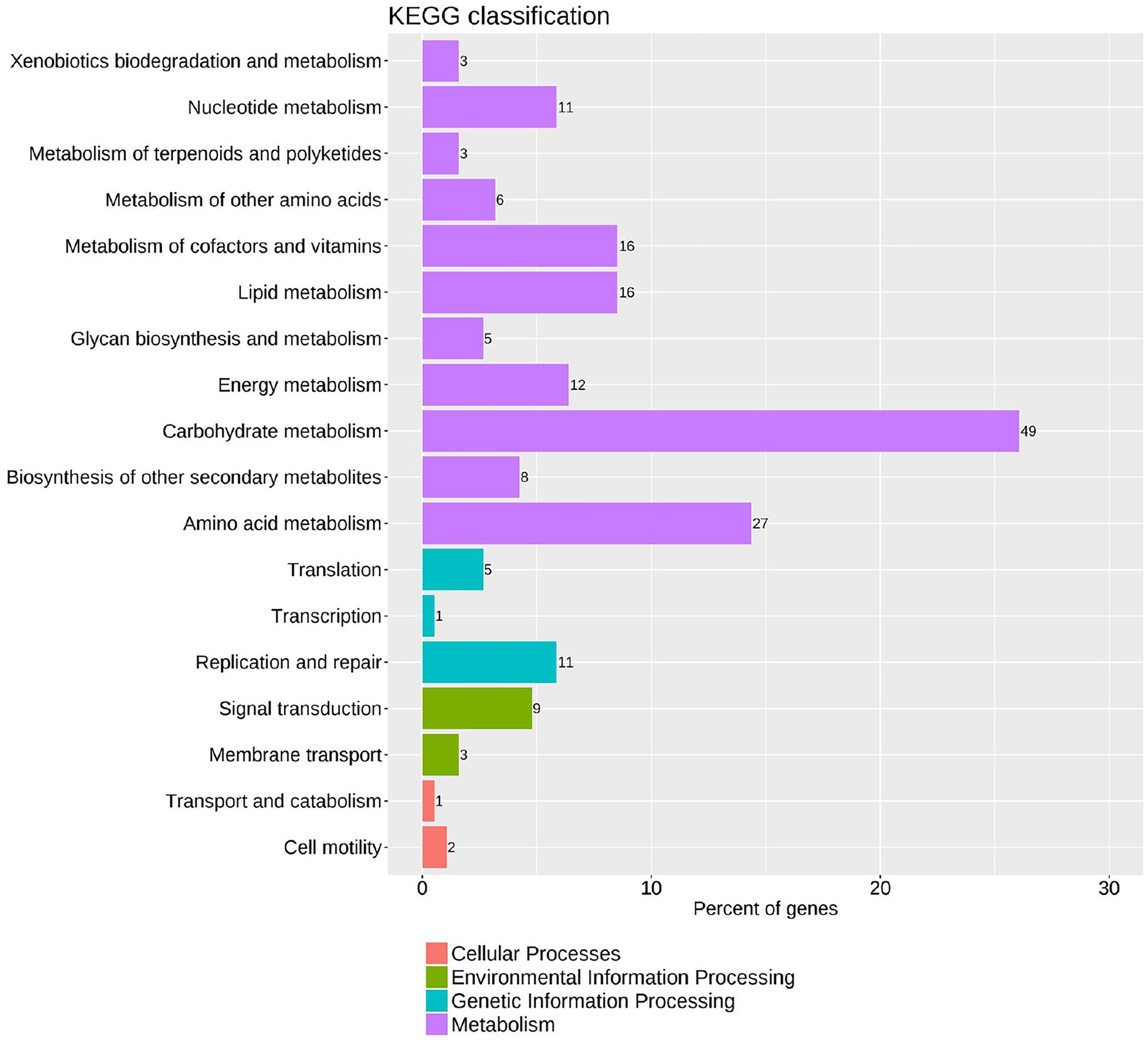
Figure 2. KEGG enrichment analysis of MsmR1 binding DNA according to ChIP-seq data. The fragments obtained from ChIP-seq were analyzed by KEGG, and were enriched in cellular process, environmental information process, genetic information process, and metabolism.
EMSA was performed to further validate the potential direct binding fragments with MsmR1. As shown in Figures 3A–K, MsmR1 could bind to the upstream fragments of oppC3, sucA, sdr3, yycN, pepF, pppL, ydfp, PPSC2_23180, gabD, araA, and bglX3. The qRT-PCR results revealed that compared with strain SC2-M1, the relative expression of genes oppC3, sucA, sdr3, yycN, pepF, pppL, ydfp, and PPSC2_23180 were significantly decreased in msmR1 mutant (p < 0.05, Figure 3L), which suggested that MsmR1 could positively regulate these genes. While no significant difference was observed in the expression of gabD, araA, and bglX3 between SC2-M1 and SC2-M1-ΔmsmR1 (p < 0.05, Figure 3L). These results indicated that MsmR1 might not regulate the expression of gabD, araA, and bglX3, even though MsmR1 could bind to them. Additionally, we also determined two MsmR1-binding motif by MEME (Figure 3M).
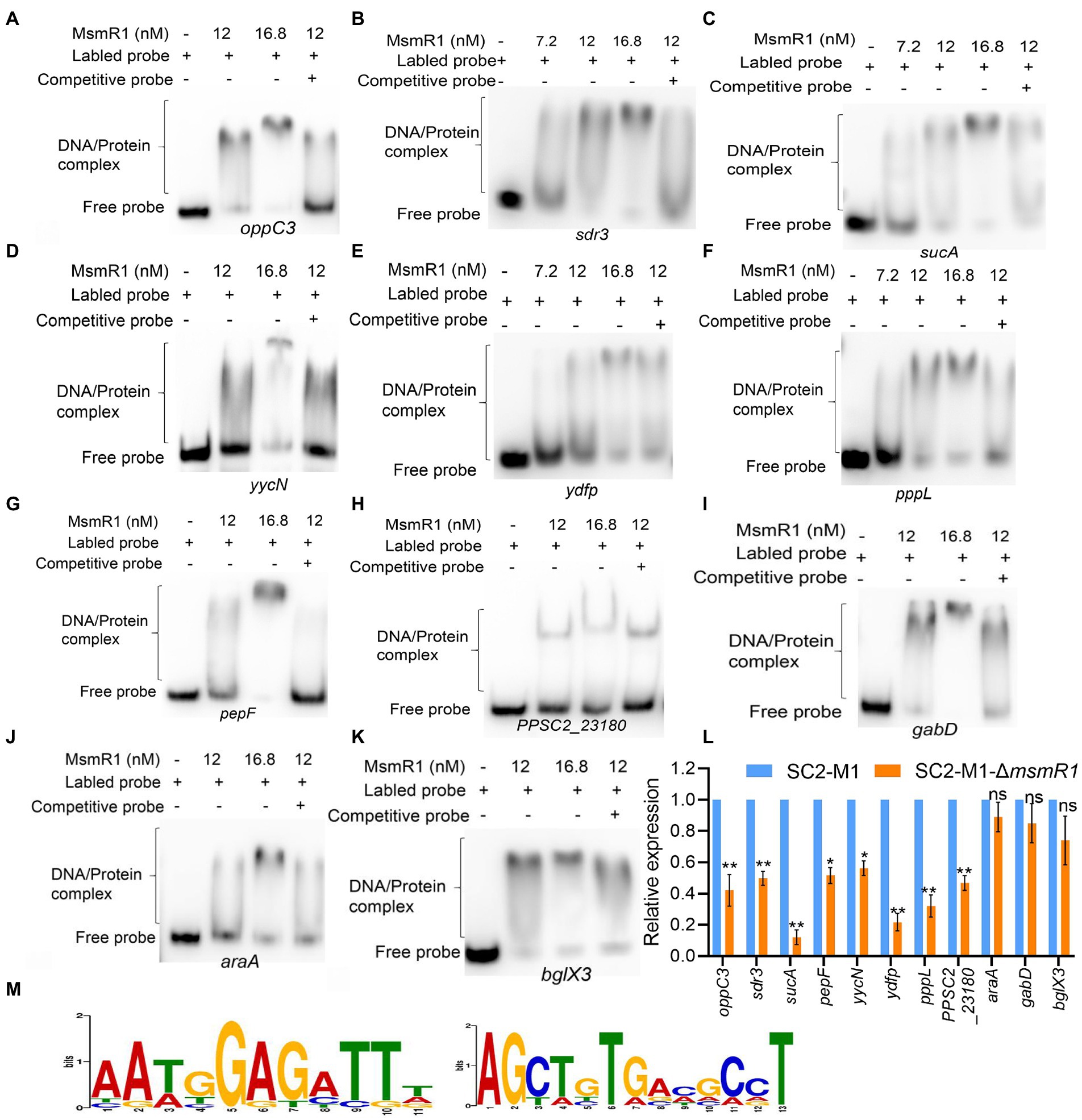
Figure 3. Identification of MsmR1 binding genes. EMSA results revealed that MsmR1 could bind directly to the upstream fragment of (A) oppC3, (B) sdr3, (C) sucA, (D) yycN, (E) ydfp, (F) pppL, (G) pepF, (H) PPSC2_23180, (I) gabD, (J) araA and (K) bglX3. (L) The relative expression of oppC3, sdr3, sucA, pepF, yycN, PPSC2_23180, pppL, ydfp, gabD, araA and bglX3 determined by qRT-PCR. (M) MsmR1 binding motifs identified by MEME analysis. *p < 0.05, **p < 0.01 and ns, p > 0.05.
MsmR1 positively regulates polymyxin synthesis by directly binding to the oppC3 and sdr3 promoters
As described previously, carbohydrate and amino acid could affect the biosynthesis of polymyxin (Yu et al., 2018). The oppC3 gene encodes a peptide ABC transporter together with the OppB protein forming a transmembrane channel that can transport and release peptides and nickel into cells (Ge et al., 2018; Slamti and Lereclus, 2019). The gene sdr3 whose predicted product belongs to the short-chain dehydrogenase/reductase (SDR) family is important for carbohydrate, amino acid, lipid metabolism, and redox sensor mechanisms (Kavanagh et al., 2008). oppC3 and sdr3 affected the growth state of strain SC2-M1 in LB medium (Figures 4A,B). Therefore, we determined the expression levels of primary metabolism-related genes, such as those involved in carbohydrate, amino acid, and fatty acid metabolism (Supplementary Table S2), and all of these genes were downregulated in the mutant strains compared with SC2-M1 (Figures 4C,D). The growth state of SC2-M1-ΔoppC3 and SC2-M1-Δsdr3 in basal medium with the sole carbon source supported their roles in carbohydrate metabolism (Supplementary Figures S4, S5). In the present study, polymyxin synthesis was significantly reduced in the knockout strains SC2-M1-ΔoppC3 and SC2-M1-Δsdr3 compared with SC2-M1, and was recovered in the complement/overexpression strain (Figures 4E,F). Moreover, the qRT-PCR results confirmed that polymyxin synthetase genes were downregulated in the knockout strain compared with SC2-M1 (Figures 4C,D). The oppC3 and sdr3 genes indirectly influence polymyxin synthesis by reducing the rate of primary metabolism and nutrient uptake, and further reduce precursors and energy for polymyxin synthesis.
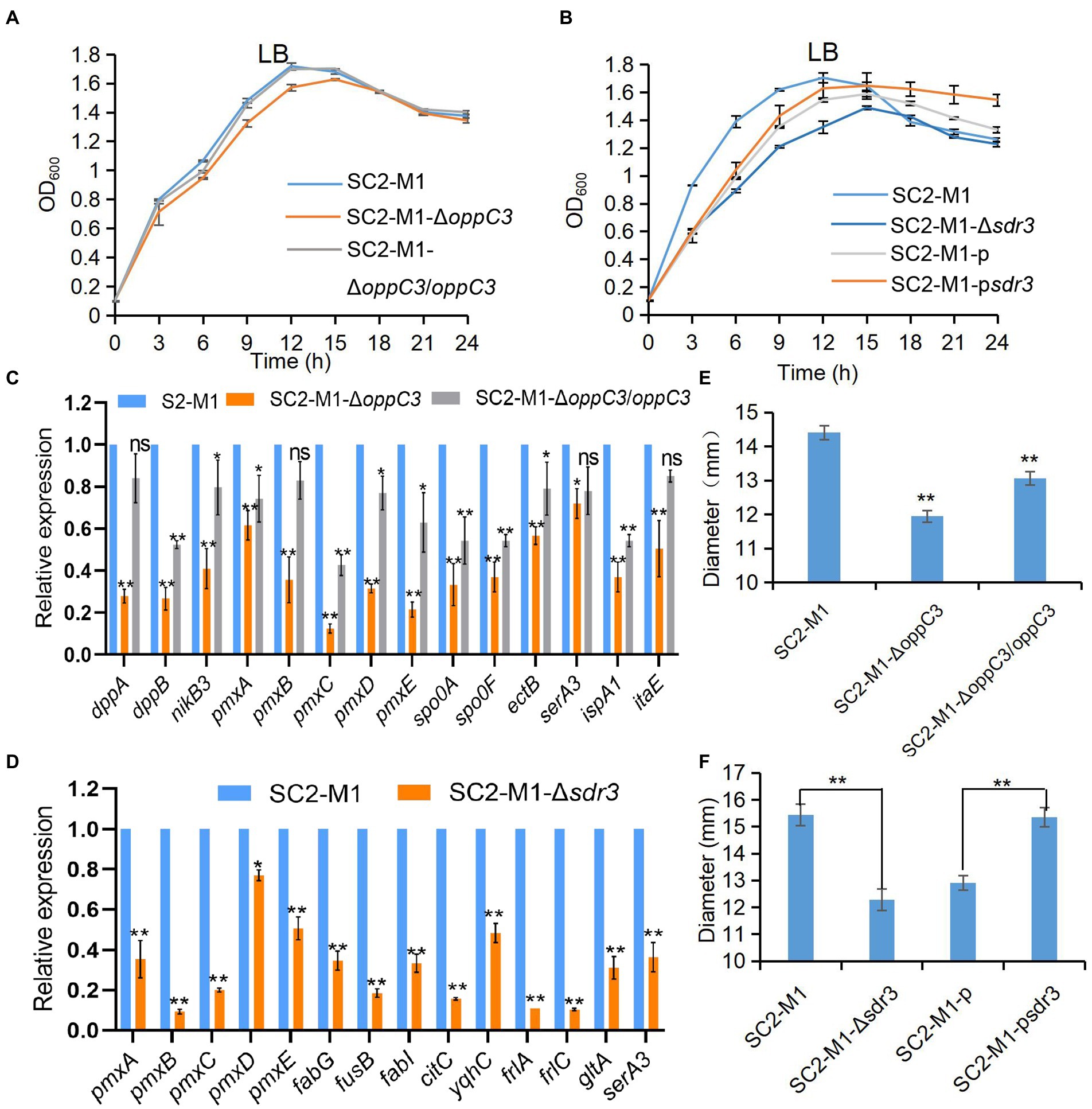
Figure 4. MsmR1 regulates polymyxin synthesis by directly binding to oppC3 and sdr3 promoters. (A) Growth curves of SC2-M1, SC2-M1-ΔoppC3, and SC2-M1-ΔoppC3/oppC3 in LB medium at a wavelength of 600 nm. (B) Growth curves of SC2-M1, SC2-M1-Δsdr3, SC2-M1-p, and SC2-M1-psdr3 in LB medium detected at a wavelength of 600 nm. (C) Relative expression of genes related to polymyxin synthesis gene cluster and amino acid transport and metabolism (dppA, dppB, ectB, serA3, ispA1, and itaE) in strain SC2-M1 and SC2-M1-ΔoppC3 by qRT-PCR assay. (D) Gene expression related to polymyxin synthesis, amino acid metabolism (gltA, serA3), fatty acid metabolism (fabG, fabI, fusB) in strains SC2-M1 and SC2-M1-Δsdr3. (E) Inhibition zone of the SC2-M1, SC2-M1-ΔoppC3, and SC2-M1-ΔoppC3/oppC3 strains. (F) Inhibition zone of the SC2-M1, SC2-M1-Δsdr3, SC2-M1-p, and SC2-M1-psdr3 strains. *p < 0.05, **p < 0.01 and ns, p > 0.05.
MsmR1 positively regulates the TCA cycle by directly binding to the sucA promoter
The sucA gene encodes 2-oxoglutarate dehydrogenase (E1), part of the 2-oxoglutarate dehydrogenase complex (Gayán et al., 2019). In the TCA cycle, α-ketoglutarate is converted to succinate, which requires the catalytic action of the 2-oxoglutarate dehydrogenase complex (sucAB) and succinate dehydrogenase (sucCD; Walshaw et al., 1997). To assess the function of sucA in SC2, a knockout mutant strain SC2-M1-ΔsucA was generated. The colony morphology on LB plates appeared flat and transparent following sucA knockout, whereas the original morphology was recovered in the complemented strain SC2-M1-ΔsucA/sucA (Figure 5A).
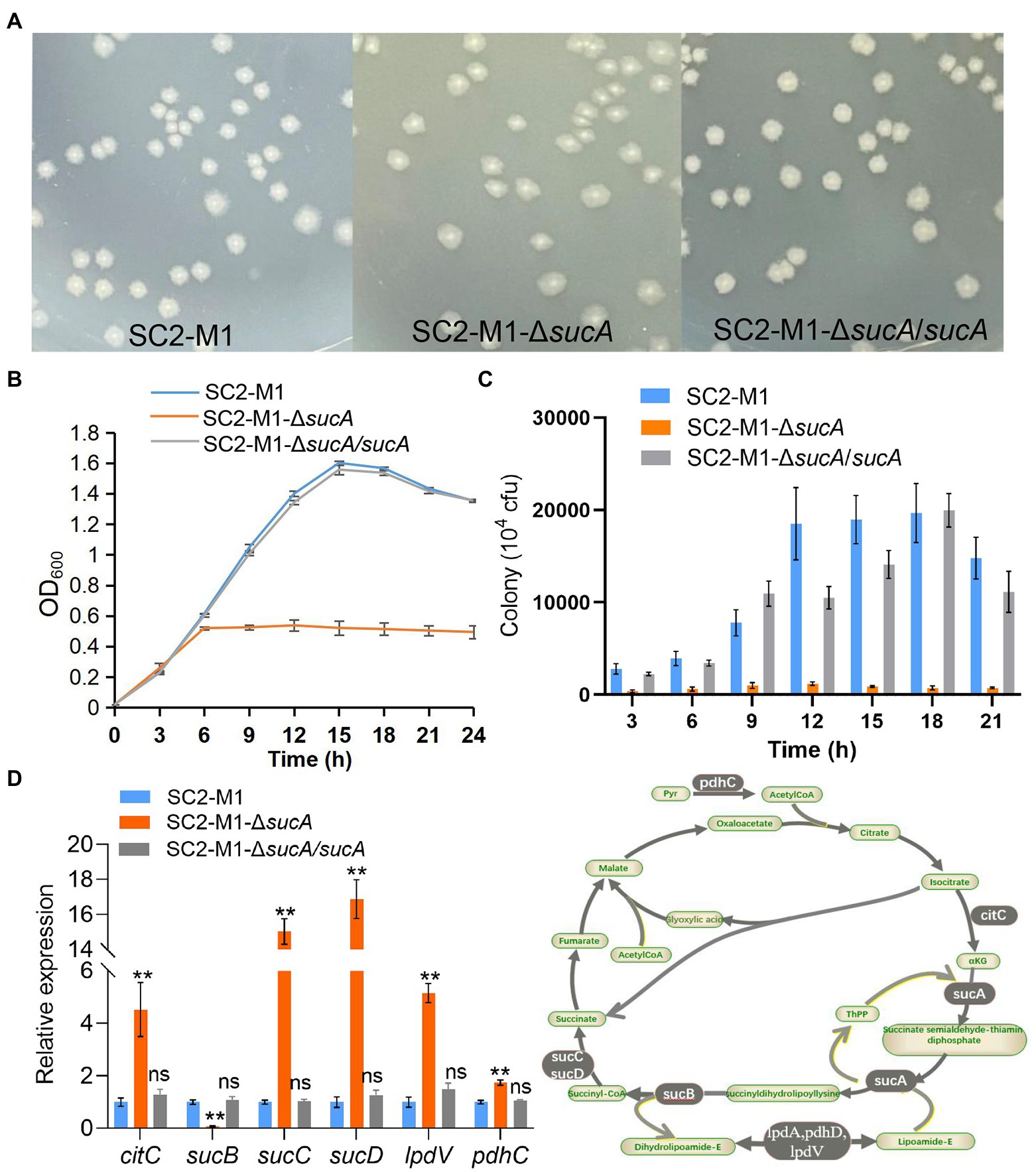
Figure 5. MsmR1 regulates carbohydrate metabolism by directly binding to the sucA promoter. (A) Colony morphology of the SC2-M1, SC2-M1-ΔsucA, and SC2-M1-ΔsucA/sucA strains in LB plates. (B) Growth curves of SC2-M1, SC2-M1-ΔsucA, and SC2-M1-ΔsucA/sucA in LB broth determined by OD600. (C) SC2-M1, SC2-M1-ΔsucA, and SC2-M1-ΔsucA/sucA colonies in LB broth determined by the live bacterial count method every 3 h. (D) Relative expression of genes related to the TCA cycle determined by qRT-PCR assay. **p < 0.01 and ns, p > 0.05.
The growth curve in LB medium was determined to evaluate the growth status, and showed that growth of the mutant strain SC2-M1-ΔsucA was markedly reduced compared to SC2-M1 and SC2-M1-ΔsucA/sucA (Figure 5B). Simultaneously, live bacterial counts were determined every 3 h; those of SC2-M1-ΔsucA were greatly reduced compared with those of SC2-M1 and SC2-M1-ΔsucA/sucA, which was consistent with the OD600 results (Figure 5C). Moreover, expression of the related gene sucB was markedly downregulated, while other genes involved in the TCA cycle, such as sucC, sucD, citC, lpdV, and pdhC (Supplementary Table S2) were markedly upregulated (Figure 5D). Due to disruption of the TCA cycle, bacteria may metabolize carbohydrate sources through the pentose phosphate pathway and glyoxylate cycle. Therefore, the energy decreased and the bacterial mass was further reduced.
During carbon source (xylose, arabinose, glucose, galactose, fructose, mannose, sucrose, maltose, trehalose, lactose and raffinose) utilization, the SC2-M1-ΔsucA strain presented a longer lag phase compared with the SC2-M1 strain. For the utilization of xylose, galactose, fructose, lactose, and raffinose, SC2-M1-ΔsucA presented a markedly lower OD600 value during the stationary phase compared with SC2-M1 (Supplementary Figure S6). Hence, sucA have essential roles in carbon source utilization.
MsmR1 regulates motility, biofilm, and spore synthesis, as well as other processes
The pepF gene encodes an oligoendopeptidase F. In Bacillus subtilis, the pepF homolog phrA can regulate spore formation (Kanamaru et al., 2002), and in Aeromonas hydrophila, pepF can regulate biofilm formation (Du et al., 2016). qRT-PCR results revealed that pepF regulated spore and biofilm formation. In other words, the relative expressions of genes related to biofilm and spore formation were downregulated (Figure 6A). The relative expressions of these genes were also downregulated in strain SC2-M1-ΔmsmR1 compared with SC2-M1 (Supplementary Figure S7). Overall, MsmR1 could positively regulate spore and biofilm formation.
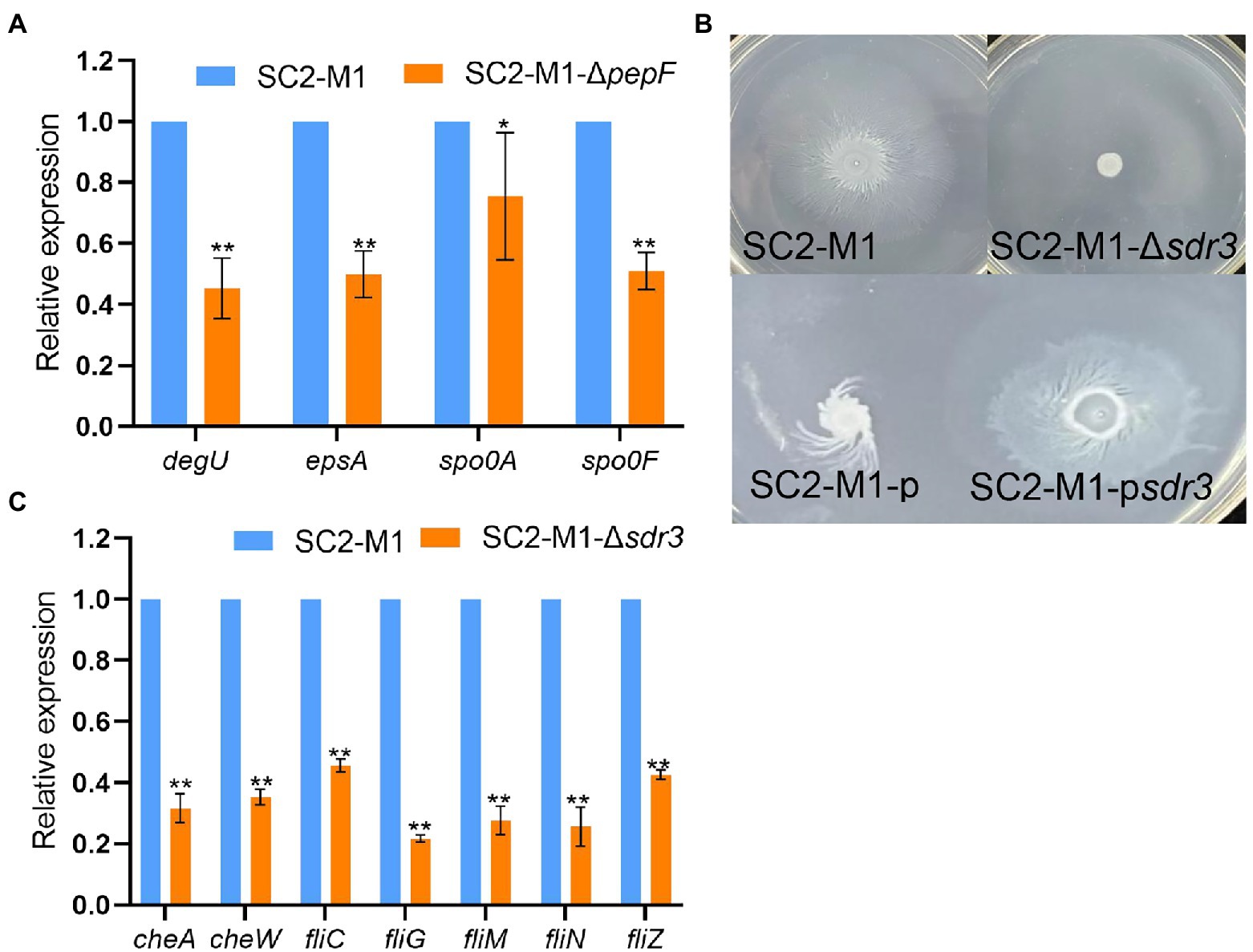
Figure 6. MsmR1 positively regulates motility, biofilm, and spore synthesis. (A) The expression of genes related to spore and biofilm formation in strain SC2-M1-ΔpepF and SC2-M1. (B) Motility of the SC2-M1, SC2-M1-Δsdr3, SC2-M1-p, and SC2-M1-psdr3 strains in a specific plate. (C) The expression of genes related to chemotaxis and flagellum (cheA, cheW, fliC, fliG, fliM, fliN, fliZ) determined by qRT-PCR. *p < 0.05 and **p < 0.01.
Chemotaxis enables microbes to sense environmental signals and move to more favorable environments, which is important for bacterial colonization (Ud-Din and Roujeinikova, 2017). We found that motility was lost following sdr3 deletion and was increased in the SC2-M1-psdr3 strain compared with that in the SC2-M1-p strain (Figure 6B). Moreover, the relative expression levels of genes encoding chemotaxis and flagellum (Supplementary Table S2) were downregulated in the SC2-M1-Δsdr3 strain compared with those in the SC2-M1 strain (Figure 6C). Taken together, these results indicate that MsmR1 may positively regulate cell motility by directly binding to the sdr3 promoter.
The yycN gene encodes GNAT family acetyltransferase. The ydfp gene acts as a DoxX family protein, combined with SodA and SseA, forming a membrane-associated oxidoreductase complex (Nambi et al., 2015). The pppL gene encodes a PPM family protein phosphatase, which participates in environmental stresses (Shi et al., 1998). Hence, MsmR1 in P. polymyxa SC2 may also be involved in other biological processes.
Discussion
The purpose of this study was to elucidate the transcriptional regulatory mechanism of MsmR1 in P. polymyxa SC2. Sequence analysis revealed that MsmR1 comprises seven α-helices forming two HTH motifs at the C-terminal domain, which is the DNA binding domain, as well as a sensing or oligomerization domain of approximately 140 amino acids at the N-terminal domain. MsmR1 is a typical AraC/XylS family transcriptional regulator (Egan, 2002), which can act as activators, repressors, or both, and are involved in various biological processes ranging from metabolism to stress response and virulence (Gahlot et al., 2021). In this study, a ChIP-seq experiment combined with EMSA was performed, the regulatory network of the global regulator MsmR1 was proposed (Figure 7), and the role of MsmR1 in P. polymyxa was described.
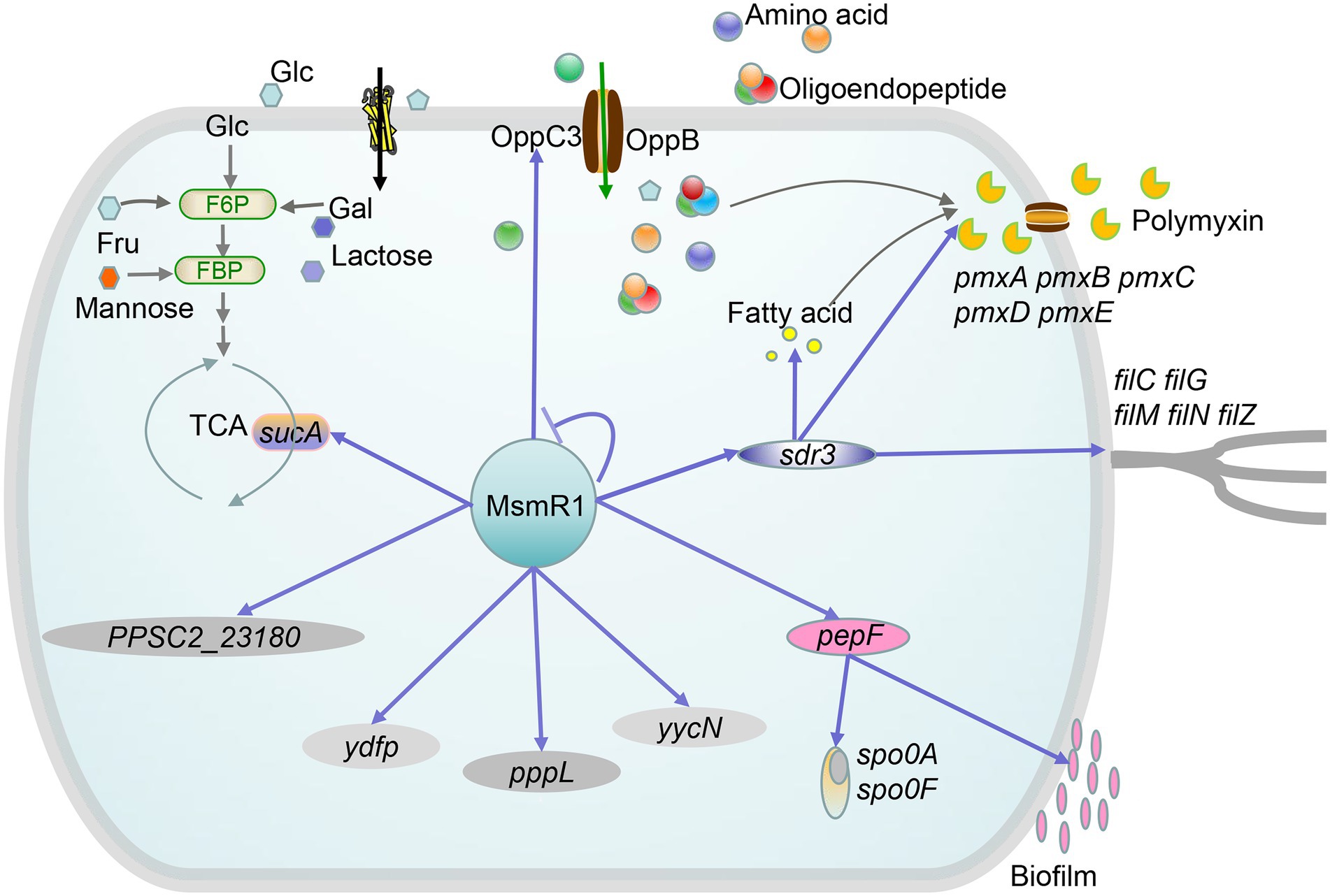
Figure 7. Proposed regulatory network of MsmR1 in P. polymyxa SC2. MsmR1 plays a regulatory role in the TCA cycle (sucA), amino acid metabolism, polymyxin synthesis (oppC3, sdr3), motility (sdr3), and biofilm and spore formation (pepF). Blue arrows: activation; bar-ended lines: repression; green arrows: metabolism process.
MsmR1 directly and positively regulates the expression of oppC3, sucA, sdr3, pepF, yycN, PPSC2_23180, pppL, and ydfp. In particular, MsmR1 directly regulates sucA and influences the TCA cycle. OppC3, an ABC transporter, transports extracellular oligopeptides and oligosaccharides into cells, affecting primary metabolism and polymyxin synthesis. Meanwhile, as a member of the short-chain dehydrogenases/reductases (SDRs) family, Sdr3 can influence fatty acid, carbohydrate, amino acid metabolism, and motility, which in turn reduces energy and precursors for polymyxin synthesis. PepF affects spore and biofilm formation. Due to the fact that spontaneous mutant strain SC2-M1 lost the ability to form endospores and biofilm (Hou et al., 2016), we could not detect the physiological or biochemical characteristics of endospores and biofilm in the test strains. However, since the genes were not successfully knocked out, the functions of PPSC2_23180, ydfp, pppL, and yycN required further investigation. Nevertheless, the results of this study revealed the mechanism through which MsmR1 regulates polymyxin synthesis, carbohydrate metabolism, and other biological processes.
We obtained 649 peaks by ChIP-seq. KEGG enrichment analysis of these sequences revealed that the genes were involved in various functions, including cellular processes, environmental information processing, genetic information processing, and metabolism. Among these, the proportion of genes enriched in metabolism was the highest, reaching 81.25%, of which carbohydrate metabolism was the most enriched, followed by amino acid metabolism. These results support previous findings indicating that MsmR is primarily involved in polysaccharide metabolism (Russell et al., 1992). In addition, only 3.85% of the identified fragments were located in intergenic regions, whereas 96.15% were located in coding regions. This may be due to differences in the DNA-binding properties of MsmR1 in vivo and in vitro. Meanwhile, in B. subtilis, 643 binding sites for AbrB and 411 binding sites for Abh were identified, and approximately half of these binding sites were located in the gene coding region. Although half of all binding sites are located in intergenic regions, this does not affect transcription, even with strong binding intensities (Chumsakul et al., 2011). RutR is a regulator of pyrimidine catabolism in E. coli, and possesses 20 binding sites primarily bound to the gene coding regions with minimal effects on transcription levels (Shimada et al., 2008). Hence, MsmR1 may be a transcriptional regulator with binding site preferences that are similar to those of RutR.
Additionally, EMSA experiments revealed that MsmR1 could also bind its own promoter, however, this was not observed in the ChIP-seq results. In fact, most genes from the ChIP-seq results were not found to bind to MsmR1. There are several possible reasons for this. First, strains in the middle of the logarithmic growth phase were selected for ChIP-seq experiments; MsmR1 may interact weakly with these genes, and some binding sites may not have been detected (Galagan et al., 2013). Second, other cofactors may be required for MsmR1 to bind target genes. Transcription factors are typically coordinated by two or more transcription factors (Zhang et al., 2021). Third, adding a Flag tag to the C-terminus of MsmR1 with a cat resistance gene in the genome of SC2-M1 may interfere with the DNA binding ability and result in false-positive or -negative results. ChIP results reported for Salmonella enterica did not reveal previously described OmpR-binding targets but revealed that binding to some OmpR-regulated genes was affected by the C-terminal Flag tag (Perkins et al., 2013; Zhang et al., 2021). Finally, the EMSA conditions may influence the binding process. In the EMSA experiment, some retarded bands were relatively clear at low or high protein concentrations, while other DNA-MsmR1 binding processes presented clearer retarded bands only at high protein concentrations and smeared bands at low concentrations. This might be due to the different binding abilities of MsmR1 to DNA or the stability of the MsmR1-DNA complex in vitro during electrophoresis. As reported previously, a weak interaction occurs between the regulator VjbR and the virB (PvirB) promoter; however, no retarded signal was observed in the EMSA experiment, indicating that VjbR and PvirB cannot withstand the electrophoretic conditions of EMSA (Arocena et al., 2010). Therefore, in ChIP-seq, some DNA may have bound weakly to MsmR1 causing it to not be detected during EMSA electrophoresis.
MsmR is a regulator of polysaccharide metabolism, hence the growth curve of the msmR1 knockout strain was determined. The results revealed no significant change in the LB medium or minimal medium with different carbon sources (Supplementary Figure S2E). However, its role in carbohydrate metabolism cannot be ruled out, as most of the identified target genes are related to carbon source metabolism. We hypothesized that this may be due to the functional redundancy of MsmR1 homologs in the SC2 strain. For genome sequence analysis, three genes were annotated as msmR (msmR5, msmR7, and msmR9), and 44 AraC family transcription factors were identified in the genome of P. polymyxa SC2, most of which were related to carbon source metabolism, such as arabinose metabolism and L-rhamnose metabolism (Supplementary Table S6). This possibility was verified using qRT-PCR, which revealed that msmR5, msmR7, and msmR9 were all upregulated after msmR1 knockout (Supplementary Figures S8A–C). Since msmR5 was the most upregulated, we then assessed the impact of knocking out msmR5. The results showed no significant difference in SC2-M1, single mutant of msmR5 (SC2-M1-ΔmsmR5) and double mutant (SC2-M1-ΔmsmR1/msmR5; Supplementary Figure S8D). In Pseudomonas aeruginosa PAO1161, PA3027 is an AraC-type transcriptional regulator with no effect on growth or carbon source utilization due to the functional redundancy of glpD homologs (Kotecka et al., 2021). Moreover, in Ustilaginoidea virens, due to the functional redundancy of other cutinase genes, cutinase activity was not affected after knockout of the cutinase G-box-binding protein UvCGBP1 (Chen et al., 2021). As a result of gene functional redundancy, growth status and carbon source utilization could not be considered the main markers of the msmR1 knockout mutant.
AraC family transcription factors often form symmetric dimers and bind to palindromic DNA sequences (Kato et al., 2005; Sun et al., 2016). A 5-nt inverted repeat was identified by binding site analysis of the AraC family transcription factor SAV742, separated by 10 or 12 nt (GCCGA-n10/n12-TCGGC). Mutating the probe revealed that the 5-nt inverted repeat was essential for SAV742 binding (Sun et al., 2016). In E. coli, the TF RhaS could bind to the promoter region of rhaI1: ATCTTTCCCTGGTTGCC (Egan and Schleif, 1994). In Pseudomonas putida, Xyls could bind to two 15 bp direct repeats (TGCA-N6-GGNTA; Domínguez-Cuevas et al., 2008). In Vibrio cholerae, Toxt binds to a “toxbox” (yrTTTTwwTwAww). In the tcpA, ctxAB, and tcpI-2 promoters, two “toxboxes” are direct repeats, while in the acfD/acfA, tagA, and tcpI-1 promoters, two “toxboxes” are inverted repeats (Weber and Klose, 2011). In Streptomyces griseus, the AdpA regulon consensus binding sequence is 5’-TGGCSNGWWY-3′, serving as inverted repeats at both ends, separated by 13–14 bp loops (Yamazaki et al., 2004). In contrast, Streptococcus pyogenes possesses two identical MsmR-binding motifs, ACTGTGACCATAA and GAATAGCTATTC, without palindromic sequences or inverted repeats (Nakata et al., 2005). In a study on the binding sequences (emm, mrp, arp, enn, and scpA) of Mga in Streptococcus pyogenes, a 45-bp binding site was identified by DNase I protection experiments, containing three conserved nucleotide regions and 29 nucleotides, separated by several unrelated nucleotides (Almengor and McIver, 2004). This consensus sequence failed to identify the location of the Mga-binding sites in the mga-promoter (McIver et al., 1999), suggesting that Mga can bind with different sequences (Almengor and McIver, 2004). In a study on the DNA-binding site of MsmR1 in the SC2 strain, two motifs were preliminary identified, while no palindromic sequences or inverted repeats were found. At the same time, no similar motifs were identified with those of other homologue MsmR1 proteins.
Oligopeptide permease (Opp) is primarily involved in importing short-chain peptides, such as dipeptide and tripeptide, from the extracellular environment (Solomon et al., 2003; Ge et al., 2018). The Opp system play an important role in the uptake of limiting nutrients like nickel, proline and betaine, especially under starvation and stress conditions (Alloing et al., 2006; Ge et al., 2018). Additionally, the Opp system plays a crucial role in various processes controlled by signal peptides, including sporulation, virulence, and binding (Slamti and Lereclus, 2019). In fact, OppC is required for peptide uptake but not for cell viability (Solomon et al., 2003). In this study, construction of an oppC3-knockout mutant revealed that oppC3 also has an important role in amino acid metabolism. Simultaneously, the expression levels of genes related to nickel and sporulation were significantly downregulated following oppC3 knockout. Polymyxin synthesis requires various amino acids as precursors. Owing to the downregulation of amino acid metabolism-related genes, precursor substances for polymyxin biosynthesis were reduced, further inhibiting the synthesis of polymyxin. In addition, oppC3 may play an important role in oligosaccharide uptake by detecting the utilization of a sole carbon source. More specifically, oppC3 plays a crucial role in the uptake of lactose and sucrose, but not maltose and trehalose. Meanwhile, with raffinose, a trisaccharide composed of glucose, galactose, and fructose, strains with oppC3 knockout did not exhibit significant growth, suggesting that oppC3 is critical for raffinose uptake. Hence, given that the role of oppC in the uptake of oligosaccharides has not yet been reported, the findings of this study report an important novel role of oppC3 in the uptake of oligosaccharides.
During the interaction between P. polymyxa SC2 and pepper, the biosynthetic genes of polymyxin and fusaricidin were upregulated, which induced systemic resistance in pepper and enhanced its resistance to certain pathogens. Simultaneously, the upregulation of genes related to chemotaxis, motility, and biofilm formation is beneficial for the colonization of strains in the rhizosphere of plants (Liu et al., 2021). MsmR1, a global transcriptional regulator, regulates biological processes, including polymyxin synthesis, carbohydrate metabolism, biofilm formation, chemotaxis, and motility, which are beneficial for the interaction between P. polymyxa SC2 and pepper. The findings of this study provide a theoretical basis for polymyxin production and the application of strain P. polymyxa SC2.
Conclusion
In the study, we investigated the transcriptional regulatory mechanism of MsmR1 in P. polymyxa SC2. MsmR1, a AraC/XylS family transcriptional regulator, is involved in polymyxin synthesis. In particular, MsmR1 was found to be highly associated with carbohydrate metabolism pathways. Indeed, MsmR1 affects polymyxin synthesis by directly regulating oppC3 and sdr3, affecting basal metabolism and precursors for polymyxin synthesis, while also directly regulating sucA and influencing the TCA cycle. In addition, MsmR1 could influence spore and biofilm formation and other biological processes. These results provide a theoretical basis for the application of P. polymyxa SC2 in the biological control of various pathogenic bacteria in pepper.
Data availability statement
The data presented in the study are deposited in the NCBI Sequence Read Archive (SRA) repository, accession number PRJNA885964.
Author contributions
DZ performed the laboratory work, analyzed the data, and wrote the manuscript. HL, YC, and ST analyzed the data. CW, BD, and YD provided insights on the manuscript. YD and BD supported the study. All authors contributed to the article and approved the submitted version.
Funding
This work was funded by the National Natural Science Foundation of China (31770115), Key Research and Development Program of Shandong Province (2021CXGC010804), and Tai-Shan Scholar Program from the Shandong Provincial Government.
Conflict of interest
The authors declare that the research was conducted in the absence of any commercial or financial relationships that could be construed as a potential conflict of interest.
Publisher’s note
All claims expressed in this article are solely those of the authors and do not necessarily represent those of their affiliated organizations, or those of the publisher, the editors and the reviewers. Any product that may be evaluated in this article, or claim that may be made by its manufacturer, is not guaranteed or endorsed by the publisher.
Supplementary material
The Supplementary material for this article can be found online at: https://www.frontiersin.org/articles/10.3389/fmicb.2022.1039806/full#supplementary-material
References
Alloing, G., Travers, I., Sagot, B., Le Rudulier, D., and Dupont, L. (2006). Proline betaine uptake in Sinorhizobium meliloti: characterization of Prb, an Opp-like ABC transporter regulated by both proline betaine and salinity stress. J. Bacteriol. 188, 6308–6317. doi: 10.1128/JB.00585-06
Almengor, A. C., and McIver, K. S. (2004). Transcriptional activation of sclA by Mga requires a distal binding site in Streptococcus pyogenes. J. Bacteriol. 186, 7847–7857. doi: 10.1128/JB.186.23.7847-7857.2004
Arocena, G. M., Sieira, R., Comerci, D. J., and Ugalde, R. A. (2010). Identification of the quorum-sensing target DNA sequence and N-Acyl homoserine lactone responsiveness of the Brucella abortus virB promoter. J. Bacteriol. 192, 3434–3440. doi: 10.1128/JB.00232-10
Ash, C., Priest, F., and Collins, M. (1993). Molecular identification of rRNA group 3 bacilli using a PCR probe test. Anton. Leeuw. Int. J. G. 64, 253–260. doi: 10.1007/BF00873085
Belyaeva, T. A., Wade, J. T., Webster, C. L., Howard, V. J., Thomas, M. S., Hyde, E. I., et al. (2000). Transcription activation at the Escherichia coli melAB promoter: the role of MelR and the cyclic AMP receptor protein. Mol. Microbiol. 36, 211–222. doi: 10.1046/j.1365-2958.2000.01849.x
Bustos, S. A., and Schleif, R. F. (1993). Functional domains of the AraC protein. Proc. Natl. Acad. Sci. U. S. A. 90, 5638–5642. doi: 10.1073/pnas.90.12.5638
Cha-Aim, K., Hoshida, H., Fukunaga, T., and Akada, R. (2012). Fusion PCR via novel overlap sequences. Methods Mol. Biol. 852, 97–110. doi: 10.1007/978-1-61779-564-0_8
Chen, X., Li, P., Liu, H., Chen, X., Huang, J., Luo, C., et al. (2021). A novel transcription factor UvCGBP1 regulates development and virulence of rice false smut fungus Ustilaginoidea virens. Virulence 12, 1563–1579. doi: 10.1080/21505594.2021.1936768
Choi, S.-K., Park, S.-Y., Kim, R., Kim, S.-B., Lee, C.-H., Kim, J. F., et al. (2009). Identification of a polymyxin synthetase gene cluster of Paenibacillus polymyxa and heterologous expression of the gene in Bacillus subtilis. J. Bacteriol. 191, 3350–3358. doi: 10.1128/JB.01728-08
Chumsakul, O., Takahashi, H., Oshima, T., Hishimoto, T., Kanaya, S., Ogasawara, N., et al. (2011). Genome-wide binding profiles of the Bacillus subtilis transition state regulator AbrB and its homolog Abh reveals their interactive role in transcriptional regulation. Nucleic Acids Res. 39, 414–428. doi: 10.1093/nar/gkq780
Chung, C., Niemela, S. L., and Miller, R. H. (1989). One-step preparation of competent Escherichia coli: transformation and storage of bacterial cells in the same solution. Proc. Natl. Acad. Sci. U. S. A. 86, 2172–2175. doi: 10.1073/pnas.86.7.2172
Cortés-Avalos, D., Martínez-Pérez, N., Ortiz-Moncada, M. A., Juárez-González, A., Baños-Vargas, A. A., Estrada-de Los Santos, P., et al. (2021). An update of the unceasingly growing and diverse AraC/XylS family of transcriptional activators. FEMS Microbiol. Rev. 45:fuab020. doi: 10.1093/femsre/fuab020
Domínguez-Cuevas, P., Marín, P., Marqués, S., and Ramos, J. L. (2008). XylS–Pm promoter interactions through two helix–turn–helix motifs: identifying XylS residues important for DNA binding and activation. J. Mol. Biol. 375, 59–69. doi: 10.1016/j.jmb.2007.10.047
Du, H., Pang, M., Dong, Y., Wu, Y., Wang, N., Liu, J., et al. (2016). Identification and characterization of an Aeromonas hydrophila oligopeptidase gene pepF negatively related to biofilm formation. Front. Microbiol. 7:1497. doi: 10.3389/fmicb.2016.01497
Egan, S. M. (2002). Growing repertoire of AraC/XylS activators. J. Bacteriol. 184, 5529–5532. doi: 10.1128/JB.184.20.5529-5532.2002
Egan, S. M., and Schleif, R. F. (1994). DNA-dependent renaturation of an insoluble DNA binding protein: identification of the RhaS binding site at rhaBAD. J. Mol. Biol. 243, 821–829. doi: 10.1006/jmbi.1994.1684
Gahlot, D. K., Ifill, G., and MacIntyre, S. (2021). Optimised heterologous expression and functional analysis of the Yersinia pestis F1-Capsular antigen regulator Caf1R. Int. J. Mol. Sci. 22:9805. doi: 10.3390/ijms22189805
Galagan, J. E., Minch, K., Peterson, M., Lyubetskaya, A., Azizi, E., Sweet, L., et al. (2013). The Mycobacterium tuberculosis regulatory network and hypoxia. Nature 499, 178–183. doi: 10.1038/nature12337
Gayán, E., Rutten, N., Van Impe, J., Michiels, C. W., and Aertsen, A. (2019). Identification of novel genes involved in high hydrostatic pressure resistance of Escherichia coli. Food Microbiol. 78, 171–178. doi: 10.1016/j.fm.2018.10.007
Ge, Y., Lee, J. H., Hu, B., and Zhao, Y. (2018). Loss-of-function mutations in the Dpp and Opp permeases render Erwinia amylovora resistant to kasugamycin and blasticidin S. Mol. Plant Microbe Interact. 31, 823–832. doi: 10.1094/MPMI-01-18-0007-R
Guérout-Fleury, A.-M., Frandsen, N., and Stragier, P. (1996). Plasmids for ectopic integration in Bacillus subtilis. Gene 180, 57–61. doi: 10.1016/s0378-1119(96)00404-0
Guo, Q.-Q., Zhang, W.-B., Zhang, C., Song, Y.-L., Liao, Y.-L., Ma, J.-C., et al. (2019). Characterization of 3-oxacyl-acyl carrier protein reductase homolog genes in Pseudomonas aeruginosa PAO1. Front. Microbiol. 10:1028. doi: 10.3389/fmicb.2019.01028
Henikoff, S., Wallace, J. C., and Brown, J. P. (1990). Finding protein similarities with nucleotide sequence databases. Methods Enzymol. 183, 111–132. doi: 10.1016/0076-6879(90)83009-x
Hou, X., Yu, X., Du, B., Liu, K., Yao, L., Zhang, S., et al. (2016). A single amino acid mutation in Spo0A results in sporulation deficiency of Paenibacillus polymyxa SC2. Res. Microbiol. 167, 472–479. doi: 10.1016/j.resmic.2016.05.002
Ibarra, J. A., Pérez-Rueda, E., Segovia, L., and Puente, J. L. (2008). The DNA-binding domain as a functional indicator: the case of the AraC/XylS family of transcription factors. Genetica 133, 65–76. doi: 10.1007/s10709-007-9185-y
Jeong, H., Choi, S.-K., Ryu, C.-M., and Park, S.-H. (2019). Chronicle of a soil bacterium: Paenibacillus polymyxa E681 as a tiny guardian of plant and human health. Front. Microbiol. 10:467. doi: 10.3389/fmicb.2019.00467
Kanamaru, K., Stephenson, S., and Perego, M. (2002). Overexpression of the PepF oligopeptidase inhibits sporulation initiation in Bacillus subtilis. J. Bacteriol. 184, 43–50. doi: 10.1128/JB.184.1.43-50.2002
Kato, J.-y., Ohnishi, Y., and Horinouchi, S. (2005). Autorepression of AdpA of the AraC/XylS family, a key transcriptional activator in the A-factor regulatory cascade in Streptomyces griseus. J. Mol. Biol. 350, 12–26. doi: 10.1016/j.jmb.2005.04.058
Kavanagh, K., Jörnvall, H., Persson, B., and Oppermann, U. (2008). Medium-and short-chain dehydrogenase/reductase gene and protein families: the SDR superfamily: functional and structural diversity within a family of metabolic and regulatory enzymes. Cell. Mol. Life Sci. 65, 3895–3906. doi: 10.1007/s00018-008-8588-y
Kettle, B. C. (2013). Genetic Analysis of allosteric signaling in RhaR from Escherichia coli and characterization of the VirF protein from Shigella flexneri. [Doctoral dissertation thesis]. Kansas (IL): University of Kansas.
Khoroshkin, M. S., Leyn, S. A., Van Sinderen, D., and Rodionov, D. A. (2016). Transcriptional regulation of carbohydrate utilization pathways in the Bifidobacterium genus. Front. Microbiol. 7:120. doi: 10.3389/fmicb.2016.00120
Kolin, A., Balasubramaniam, V., Skredenske, J. M., Wickstrum, J. R., and Egan, S. M. (2008). Differences in the mechanism of the allosteric l-rhamnose responses of the AraC/XylS family transcription activators RhaS and RhaR. Mol. Microbiol. 68, 448–461. doi: 10.1111/j.1365-2958.2008.06164.x
Kotecka, K., Kawalek, A., Kobylecki, K., and Bartosik, A. A. (2021). The AraC-type transcriptional regulator GliR (PA3027) activates genes of glycerolipid metabolism in Pseudomonas aeruginosa. Int. J. Mol. Sci. 22:5066. doi: 10.3390/ijms22105066
Kreikemeyer, B., Nakata, M., Köller, T., Hildisch, H., Kourakos, V., Standar, K., et al. (2007). The Streptococcus pyogene serotype M49 Nra-Ralp3 transcriptional regulatory network and its control of virulence factor expression from the novel eno ralp3 epf sagA pathogenicity region. Infect. Immun. 75, 5698–5710. doi: 10.1128/IAI.00175-07
Li, H., Ding, Y., Zhao, J., Ge, R., Qiu, B., Yang, X., et al. (2019). Identification of a native promoter PLH-77 for gene expression in Paenibacillus polymyxa. J. Biotechnol. 295, 19–27. doi: 10.1016/j.jbiotec.2019.02.002
Liu, H., Li, Y., Ge, K., Du, B., Liu, K., Wang, C., et al. (2021). Interactional mechanisms of Paenibacillus polymyxa SC2 and pepper (Capsicum annuum L.) suggested by transcriptomics. BMC Microbiol. 21, 70–16. doi: 10.1186/s12866-021-02132-2
Lowden, M. J., Skorupski, K., Pellegrini, M., Chiorazzo, M. G., Taylor, R. K., and Kull, F. J. (2010). Structure of Vibrio cholerae ToxT reveals a mechanism for fatty acid regulation of virulence genes. Proc. Natl. Acad. Sci. U. S. A. 107, 2860–2865. doi: 10.1073/pnas.0915021107
Ma, M., Wang, C., Ding, Y., Li, L., Shen, D., Jiang, X., et al. (2011). Complete genome sequence of Paenibacillus polymyxa SC2, a strain of plant growth-promoting rhizobacterium with broad-spectrum antimicrobial activity. J. Bacteriol. 193, 311–312. doi: 10.1128/JB.01234-10
McIver, K. S., Thurman, A. S., and Scott, J. R. (1999). Regulation of mga transcription in the group A streptococcus: specific binding of Mga within its own promoter and evidence for a negative regulator. J. Bacteriol. 181, 5373–5383. doi: 10.1128/JB.181.17.5373-5383.1999
Mejía-Almonte, C., Busby, S. J., Wade, J. T., van Helden, J., Arkin, A. P., Stormo, G. D., et al. (2020). Redefining fundamental concepts of transcription initiation in bacteria. Nat. Rev. Genet. 21, 699–714. doi: 10.1038/s41576-020-0254-8
Nakata, M., Podbielski, A., and Kreikemeyer, B. (2005). MsmR, a specific positive regulator of the Streptococcus pyogenes FCT pathogenicity region and cytolysin-mediated translocation system genes. Mol. Microbiol. 57, 786–803. doi: 10.1111/j.1365-2958.2005.04730.x
Nambi, S., Long, J. E., Mishra, B. B., Baker, R., Murphy, K. C., Olive, A. J., et al. (2015). The oxidative stress network of Mycobacterium tuberculosis reveals coordination between radical detoxification systems. Cell Host Microbe 17, 829–837. doi: 10.1016/j.chom.2015.05.008
Parra, M. C., and Collins, C. M. (2012). Mutational analysis of the N-terminal domain of UreR, the positive transcriptional regulator of urease gene expression. Microbiol. Res. 167, 433–444. doi: 10.1016/j.micres.2012.03.005
Perkins, T. T., Davies, M. R., Klemm, E. J., Rowley, G., Wileman, T., James, K., et al. (2013). ChIP-seq and transcriptome analysis of the OmpR regulon of Salmonella enterica serovars Typhi and Typhimurium reveals accessory genes implicated in host colonization. Mol. Microbiol. 87, 526–538. doi: 10.1111/mmi.12111
Pjanic, M. (2017). ChIPSeqFPro, a pipeline for sequential processing of ChIP-Seq fastq to bigwig files. bioRxiv :118281. doi: 10.1101/118281
Pletzer, D., Schweizer, G., and Weingart, H. (2014). AraC/XylS family stress response regulators Rob, SoxS, PliA, and OpiA in the fire blight pathogen Erwinia amylovora. J. Bacteriol. 196, 3098–3110. doi: 10.1128/JB.01838-14
Russell, R. R., Aduse-Opoku, J., Sutcliffe, I. C., Tao, L., and Ferretti, J. J. (1992). A binding protein-dependent transport system in Streptococcus mutans responsible for multiple sugar metabolism. J. Biol. Chem. 267, 4631–4637. doi: 10.1016/S0021-9258(18)42880-3
Schleif, R. (2010). AraC protein, regulation of the l-arabinose operon in Escherichia coli, and the light switch mechanism of AraC action. FEMS Microbiol. Rev. 34, 779–796. doi: 10.1111/j.1574-6976.2010.00226.x
Shi, L., Potts, M., and Kennelly, P. J. (1998). The serine, threonine, and/or tyrosine-specific protein kinases and protein phosphatases of prokaryotic organisms: a family portrait. FEMS Microbiol. Rev. 22, 229–253. doi: 10.1111/j.1574-6976.1998.tb00369.x
Shimada, T., Ishihama, A., Busby, S. J., and Grainger, D. C. (2008). The Escherichia coli RutR transcription factor binds at targets within genes as well as intergenic regions. Nucleic Acids Res. 36, 3950–3955. doi: 10.1093/nar/gkn339
Slamti, L., and Lereclus, D. (2019). The oligopeptide ABC-importers are essential communication channels in Gram-positive bacteria. Res. Microbiol. 170, 338–344. doi: 10.1016/j.resmic.2019.07.004
Solomon, J., Su, L., Shyn, S., and Grossman, A. D. (2003). Isolation and characterization of mutants of the Bacillus subtilis oligopeptide permease with altered specificity of oligopeptide transport. J. Bacteriol. 185, 6425–6433. doi: 10.1128/JB.185.21.6425-6433.2003
Sun, D., Zhu, J., Chen, Z., Li, J., and Wen, Y. (2016). SAV742, a novel AraC-family regulator from Streptomyces avermitilis, controls avermectin biosynthesis, cell growth and development. Sci. Rep.. 6, 1–14. doi: 10.1038/srep36915
Thorvaldsdóttir, H., Robinson, J. T., and Mesirov, J. P. (2013). Integrative genomics viewer (IGV): high-performance genomics data visualization and exploration. Brief. Bioinform. 14, 178–192. doi: 10.1093/bib/bbs017
Timmusk, S., Grantcharova, N., and Wagner, E. G. H. (2005). Paenibacillus polymyxa invades plant roots and forms biofilms. Appl. Environ. Microb. 71, 7292–7300. doi: 10.1128/AEM.71.11.7292-7300.2005
Ud-Din, A. I. M. S., and Roujeinikova, A. (2017). Methyl-accepting chemotaxis proteins: a core sensing element in prokaryotes and archaea. Cell. Mol. Life Sci. 74, 3293–3303. doi: 10.1007/s00018-017-2514-0
Walshaw, D. L., Wilkinson, A., Mundy, M., Smith, M., and Poole, P. S. (1997). Regulation of the TCA cycle and the general amino acid permease by overflow metabolism in Rhizobium leguminosarum. Microbiology 143, 2209–2221. doi: 10.1099/00221287-143-7-2209
Wang, P.-H., Sai, W., NIE, W.-H., Yan, W., Iftikhar, A., Ayizekeranmu, Y., et al. (2022). A transferred regulator that contributes to Xanthomonas oryzae pv. Oryzicola oxidative stress adaptation and virulence by regulating the expression of cytochrome bd oxidase genes. J. Integr. Agric. 21, 1673–1682. doi: 10.1016/S2095-3119(21)63801-7
Wang, C., Zhao, D., Qi, G., Mao, Z., Hu, X., Du, B., et al. (2020). Effects of Bacillus velezensis FKM10 for promoting the growth of Malus hupehensis Rehd and inhibiting Fusarium verticillioides. Front. Microbiol. 10:2889. doi: 10.3389/fmicb.2019.02889
Warda, A. K., Siezen, R. J., Boekhorst, J., Wells-Bennik, M. H., de Jong, A., Kuipers, O. P., et al. (2016). Linking Bacillus cereus genotypes and carbohydrate utilization capacity. PLoS One 11:e0156796. doi: 10.1371/journal.pone.0156796
Weber, G. G., and Klose, K. E. (2011). The complexity of ToxT-dependent transcription in Vibrio cholerae. Indian J. Med. Res. 133:201.
Yamazaki, H., Tomono, A., Ohnishi, Y., and Horinouchi, S. (2004). DNA-binding specificity of AdpA, a transcriptional activator in the A-factor regulatory cascade in Streptomyces griseus. Mol. Microbiol. 53, 555–572. doi: 10.1111/j.1365-2958.2004.04153.x
Yin, Z., Wang, X., Hu, Y., Zhang, J., Li, H., Cui, Y., et al. (2022). Metabacillus dongyingensis sp. nov. is represented by the plant growth-promoting bacterium BY2G20 isolated from saline-alkaline soil and enhances the growth of Zea mays L. under salt stress. mSystems 7:e01426-21. doi: 10.1128/msystems.01426-21
Yu, Z., Guo, C., and Qiu, J. (2015). Precursor amino acids inhibit polymyxin E biosynthesis in Paenibacillus polymyxa, probably by affecting the expression of polymyxin E biosynthesis-associated genes. Biomed. Res. Int. 2015, 1–11. doi: 10.1155/2015/690830
Yu, Z., Sun, Z., Yin, J., and Qiu, J. (2018). Enhanced production of polymyxin E in Paenibacillus polymyxa by replacement of glucose by starch. Biomed. Res. Int. 2018, 1–10. doi: 10.1155/2018/1934309
Zhang, Y., Liu, T., Meyer, C. A., Eeckhoute, J., Johnson, D. S., Bernstein, B. E., et al. (2008). Model-based analysis of ChIP-Seq (MACS). Genome Biol. 9, R137–R139. doi: 10.1186/gb-2008-9-9-r137
Zhang, Q., Xing, C., Kong, X., Wang, C., and Chen, X. (2021). ChIP-seq analysis of the global regulator Vfr reveals novel insights into the biocontrol agent Pseudomonas protegens FD6. Front. Microbiol. 12:1156. doi: 10.3389/fmicb.2021.667637
Keywords: Paenibacillus polymyxa, MsmR1, polymyxin, carbohydrate metabolism, ChIP-seq
Citation: Zhao D, Li H, Cui Y, Tang S, Wang C, Du B and Ding Y (2022) MsmR1, a global transcription factor, regulates polymyxin synthesis and carbohydrate metabolism in Paenibacillus polymyxa SC2. Front. Microbiol. 13:1039806. doi: 10.3389/fmicb.2022.1039806
Edited by:
Bin Liu, University of Minnesota Twin Cities, United StatesReviewed by:
Yangbo Hu, Wuhan Institute of Virology (CAS), ChinaNing Xu, Tianjin Institute of Industrial Biotechnology (CAS), China
Copyright © 2022 Zhao, Li, Cui, Tang, Wang, Du and Ding. This is an open-access article distributed under the terms of the Creative Commons Attribution License (CC BY). The use, distribution or reproduction in other forums is permitted, provided the original author(s) and the copyright owner(s) are credited and that the original publication in this journal is cited, in accordance with accepted academic practice. No use, distribution or reproduction is permitted which does not comply with these terms.
*Correspondence: Binghai Du, du_binghai@163.com; Yanqin Ding, dyq@sdau.edu.cn