- 1Beijing Laboratory for Food Quality and Safety, College of Food Science and Nutritional Engineering, China Agricultural University, Beijing, China
- 2Tianjin Key Laboratory of Aqua-Ecology and Aquaculture College of Fisheries, Tianjin Agricultural University, Tianjin, China
- 3College of Engineering, China Agricultural University, Beijing, China
- 4Key Laboratory of Precision Nutrition and Food Quality, Department of Nutrition and Health, China Agricultural University, Beijing, China
- 5School of Life Science and Engineering, Southwest University of Science and Technology, Mianyang, China
Plantaricin E/F (PlnEF) is a pair of two-component class IIb bacteriocin produced by lactic acid bacteria. PlnEF commonly displays potent antimicrobial activity against certain Gram-positive organisms. In this study, we investigated the synergistic activity of PlnEF combined with lactic acid against Gram-negative food and aquaculture potential pathogen Aeromonas hydrophila LPL-1, which is naturally resistant to PlnEF. We applied SDS-PAGE, wavelength-scanning, laser confocal microscopy, flow cytometer, scanning electron microscopy (SEM), transmission electron microscopy (TEM), and two-dimensional electrophoresis to investigate their synergistic inhibitory activities. The results showed that L-lactic acid drove the release of LPS from A. hydrophila, making it possible for PlnEF to contact the inner cell membrane of A. hydrophila. Besides, co-treatment of lactic acid and PlnEF caused severe morphological and intracellular changes of A. hydrophila, including blebs on the cell surface, abnormal cell elongation, inner membrane disruption, pore-forming through the outer and inner membrane, coagulation of the cytoplasm, and structural transformation of DNA. Protein profile analysis revealed that combined treatment of lactic acid and PlnEF inhibited the energy metabolism, protein synthesis, protein folding, and DNA replication in A. hydrophila. These findings proved that PlnEF combined with lactic acid was efficient against A. hydrophila and shed light on bacteriocin’s potential and a new inhibition mechanism against A. hydrophila.
Importance: Bacteriocins and their producing strains are increasingly used to substitute artificial preservatives and antibiotics in the food and aquaculture industries. However, the bacteriocins produced by lactic acid bacteria are efficient to mainly Gram-positive bacteria. Our paper had demonstrated the antimicrobial activity of class IIb bacteriocin against potential Gram-negative pathogen, A. hydrophila LPL-1, when combined with lactic acid. The results could refresh our knowledge about the potential of class IIb bacteriocins produced by lactic acid bacteria.
Introduction
Lactic acid bacteria (LAB) are the most commonly used probiotics that can inhibit or kill various Gram-positive and Gram-negative pathogens. The main antibacterial substances produced by LAB include organic acid (Özcelik et al., 2016), bacteriocin (Mokoena, 2017), H2O2 (Dashe et al., 2020), enzymes, acetoin, acetaldehyde, etc. (Moradi et al., 2020).
Bacteriocins produced by lactic acid bacteria are ribosomally synthesized cationic peptides with or without post-translational modification, which can be grouped into different classes (Cotter et al., 2005; García et al., 2010; Alvarez-Sieiro et al., 2016). Among them, the well-studied class I and class II bacteriocins have been used in the food industry (nisin and pediocin PA-1) (Gharsallaoui et al., 2016; Santos et al., 2018; Ibarra-Sánchez et al., 2020; Anumudu et al., 2021) and shown a great potential as an alternative to antibiotics (Bonhi and Imran, 2019; Lopetuso et al., 2019; Fu and Kapila, 2021). The mode of action for class I bacteriocins (e.g., nisin) is inhibition of peptidoglycan synthesis and forming pores in the cell membrane. Class II bacteriocins can form pores in the cell membrane (Cotter et al., 2013). The majority of class I and class II bacteriocins produced by lactic acid bacteria are commonly known to inhibit Gram-positive pathogens but have limited inhibitory effects against Gram-negative bacteria (Cotter et al., 2005; García et al., 2010; Cui et al., 2021). However, studies have found several bacteriocins produced by LAB strains that display a stronger antagonism to Gram-negative bacteria (Riley and Wertz, 2002; Todorov and Dicks, 2005; Rumjuankiat et al., 2015; Sahoo et al., 2015; Yang et al., 2015; Kumariya et al., 2019; Sheoran and Tiwari, 2019; Haghighatafshar et al., 2021). Gram-negative bacteria are resistant to many antimicrobial substances due to the impermeability of their outer membrane (OM) (Christaki et al., 2020). Disruption of the OM barrier allows for the entry of otherwise inactive antimicrobials into Gram-negative pathogens, thus sensitizing the bacteria (Savage, 2001; Ciepluch et al., 2019; MacNair and Brown, 2020). It is proposed that the OM perturbants or permeabilizers such as lactic acid (Kalchayanand et al., 1992; Nykänen et al., 1998), chelating agent (Alakomi et al., 2003; Martin-Visscher et al., 2011), polycations, hydrophobic antibiotics, detergents, lysozyme, polyanionic polyethyleneimine (Helander et al., 1997), polymyxin B (Chi and Holo, 2018), protamine, etc. (Johansen et al., 1997), and some bacteriocins (Sheoran and Tiwari, 2021) could broaden the antibacterial spectrum of bacteriocins, thus potentiating Gram-negative bacteria inhibition. Among the reported OM permeabilizers, lactic acid is a promising metabolite. As the most common metabolite of LAB, lactic acid has been reported to be an outer membrane permeability agent leading to the release of outer membrane LPS in Gram-negative bacteria (Alakomi et al., 2000; Wang et al., 2020), thus increasing the sensitivity of A. hydrophila to class IIa bacteriocin, pediocin PA-1 (Wang et al., 2020). The lipopolysaccharide (LPS) is enriched in the outer membrane of Gram-negative bacteria, providing a natural barrier against many antibotics (Cetuk et al., 2021) and the bacteriocins that are mainly effective against Gram-positive bacteria (Kalchayanand et al., 1992; Gao et al., 1999; Osmanağaoğlu, 2005). Due to the LPS barrier’s damage, lactic acid-treated Gram-negative bacteria could be more sensitive to bacteriocins. Nykänen et al. has elucidated the synergistic potential of class I bacteriocin-nisin and lactic acid against Gram-negative pathogen Pseudomonas fluorescens and Pseudomonas aeruginosa ATCC9721 (Nykänen et al., 1998). Kalchayanand et al. found that acid stress (40% lactic acid, 16% propionic acid, and 16% acetic acid in water) increases the sensitivity of Yersinia enterocolitica Y7P and Pseudomonas fluorescens PF2 to nisin and pediocin AcH (Kalchayanand et al., 1992). We have proved the synergistic activity of class IIa bacteriocin-pediocin PA-1 and lactic acid against A. hydrophila and provided a potential mechanism of their synergistic inhibitory mechanism. L-lactic acid released the outer membrane LPS, making it possible for pediocin PA-1 to contact the plasma membrane of A. hydrophila, resulting in the dissipation of proton-motive force in the inner membrane and cell death (Wang et al., 2020). However, there were no reports about the combined use of class IIb bacteriocins with lactic acid. Class IIb bacteriocins possess a different antibacterial spectrum (Wu et al., 2021), stability, receptors, and action of mode compared with class I and class IIa bacteriocins (Cotter et al., 2013). They are reported to be alternative antimicrobial peptides in food preservation (Abdulhussain Kareem and Razavi, 2020). Besides, their producing strains, including Lactiplantibacillus plantarum (former Lactobacillus plantarum), Enterococcus faecalis (Maldonado-Barragán et al., 2009), Lactococcus lactis (Zendo et al., 2006), identified as potential probiotics, are promising for the-insuit application of bacteriocin producing strains in food, agriculture or pharmaceutical field (Yin et al., 2018). Therefore, it is urgent to figure out the synergistic activity of lactic acid and class IIb bacteriocin and its underlying mechanism for better application of class IIb bacteriocin and their producing strains in Gram-negative pathogen control. Class IIb bacteriocins are ribosomally synthesized, unmodified, two-peptide bacteriocins. The antimicrobial activities of class IIb bacteriocin rely on the complementary action of the two peptides (Ekblad et al., 2016). So far, more than 15 pairs of two peptide bacteriocins have been identified (Nissen-Meyer et al., 2010). Among them, plantaricin E/F showed efficient inhibitory activity on Gram-positive bacteria by causing cell membrane damage, resulting in the dissipation of cell proton motive force and finally causing cell death (Zhang et al., 2016).
Aeromonas hydrophila is an important Gram-negative pathogen associated with various human diseases (Ottaviani et al., 2011; Igbinosa et al., 2012; Kali et al., 2016) and aquatic animal diseases (Mzula et al., 2019; Anjur et al., 2021). Furthermore, A. hydrophlia are reported to be resistant to many antibiotics (Stratev and Odeyemi, 2016). And they are causing significant economic loss worldwide and are considerable threats to food safety and aquaculture (Praveen et al., 2016). Therefore, controlling A. hydrophila is necessary for aquaculture and food safety (Daskalov, 2006; Pal, 2018). The potential pathogen A. hydrophila LPL-1 was originally isolated from the spoiled sturgeon flesh sample in Beijing in 2013. The spoilage role of strain A. hydrophila LPL-1 was confirmed with fresh sturgeon fish flesh. With inoculation of 106 CFU/g of A. hydrophila LPL-1, the fish flesh was seriously spoiled after two days of refrigerator storage. Meanwhile, A. hydrophila LPL-1 also caused 100% mortality in zebrafish after one day of the soaking challenge with 1 × 109CFU/mL viable bacteria (unpublished research).
In this study, we investigated the synergistic inhibitory effect of PlnEF and lactic acid against A. hydrophila and its underlying mechanisms. A potential pathogenic strain A. hydrophila LPL-1 was used to evaluate the inhibition effect, the damage of cell structure, and alternation in cellular protein profile.
Materials and Methods
Strains and Growth Conditions
The strain A. hydrophila LPL-1 was originally isolated from spoiled sturgeon in our lab and identified its species by 16S ribosomal RNA gene sequencing and whole-genome sequencing (WGS) (GenBank, BioProject ID PRJNA767215)1. A. hydrophila LPL-1 shares 3,795 genes, or 94.44% genes with the representative of Aeromonas hydrophila ATCC 7966 (Supplementary Figure 1). The bacteria cultured in Luria-Bertani (LB) liquid medium for 24 h was collected by centrifugation (1600 g, 5 min, 4°C), resuspended with sterile skim milk, and freeze-dried. The bacterial powder was stored at −80°C and was routinely cultured in LB broth at 30°C under aerobic conditions.
Bacteriocin Synthesis and Fluorescent Label
The mature peptides of plantaricin E (PlnE: FNRDGY NFGKSVRHVVDAIGSVAGIRGILKSIR) and plantaricin F (PlnF: VFHAYSARGVRNNYKSAVGPADWVISAVRGFIHG) were synthesized using the solid-phase synthesis method by Gill biochemical Shanghai Co., LTD., China (purity (HPLC) > 95%, Supplementary Figure 2). PlnE was fluorescently labeled by fluorescein isothiocyanate (FITC) as follows: FITC (2 eq.) (resolved in Pyridine (2 eq.) and N, N-Diisopropylethylamine (DIPEA 2 eq.) was mixed with the crude peptide for 1 h. FITC-labeled PlnE was subsequently purified to over 95% chromatographic homogeneity by reverse-phase high-performance liquid chromatography and confirmed by mass spectrometry analysis.
Antibacterial Activity of PlnEF Against Aeromonas hydrophila
About 1.0 × 107 CFU/mL (confirmed by plate count method) A. hydrophila LPL-1 was inoculated in LB broth with 10 mM L-lactic acid, 10 mM L-lactic acid with different levels of PlnEF (0.5, 2, 5, 10, 25 μM), and an equal volume of distilled water (as control). All the samples were cultured at 30°C for 12 h in a 96-well plate and subsequently analyzed for OD600 using a Multi-function microplate reader (Thermo Scientific Varioskan Flash). The minimum inhibitory concentration (MIC90) values were defined as the lowest concentration of PlnEF at which the growth of bacteria in 90% of the microplates was inhibited.
The bactericidal activity was measured by propidium iodide (PI) staining. Briefly, A. hydrophila (0.8-1.0 × 108 CFU/mL, confirmed by plate count method) were collected and resuspended in saline (as control), saline with 10 mM L-lactic acid, saline with 10 mM L-lactic acid combined with 25 μM PlnEF, respectively, and incubated at 30°C for 2, 4, 6, 8 h. After that, the cells were washed with sterile saline to remove the antimicrobial substances, and other substances interfered with PI. Bacterial cells resuspended with saline were incubated with 10 μM PI for 1 h at 30°C. The proportion of dead cells (PI stained cells) was determined using Flow cytometry (BD Calibur, BD Co., Franklin Lakes, NJ, United States). The total number of counting cells was 20,000. The minimum bactericidal concentration (MBC) is identified by determining the lowest concentration of PlnEF (when combined with 10 mM lactic acid) that totally reduces the viability of 1.0 × 105CFU/mL A. hydrophila after 24 h under 4°C, and no colony forms on the plate after 48 h incubation at 30°C using plate counting methods.
Lipopolysaccharide Release and Iinteraction With PlnEF Assay
The released LPS were detected by SDS-PAGE according to the previous study with little modifications (Fomsgaard et al., 1990). Briefly, the A. hyrophila LPL-1 cells were treated with L-lactic acid (5, 10, and 12 mM) for 0.5 to 5 h. All culture supernatants were collected, freeze-dried, and then dissolved in 100 μL of SDS-PAGE sample buffer (Novex), heated at 100°C for 10 min, and then added with proteinase K (to a final concentration of 0.25 mg/mL) and kept at 60°C for 1 h. Each sample was then evaluated by SDS-PAGE in 12% acrylamide gels; 10 μL of each sample was applied to the gel. The gels were stained with silver (0.2% AgNO3). The LPS released from A. hyrophila LPL-1 were incubated with 0, 2.5, 5, 10 μM PlnEF in sterile distilled water for 1 h at 30°C and then scanned from 190 to 210 nm using a spectrophotometer (UV2000 Unocal Shanghai instrument Co., LTD., China).
Distribution of PlnEF on Aeromonas hydrophila
Aeromonas hydrophila cells were collected at the end of the logarithmic phase (10 h), washed twice with sterile saline, and resuspended in saline containing 10 mmol/L glucose, the final concentration of A. hydrophila was about 1 × 109 CFU/mL (confirmed by plate count method). Part of the cells was incubated with 10 mM L-lactic acid at 4°C and collected after 2, 4, and 8 h of processing, washed twice immediately to remove the lactic acid. The rest of A. hydrophila cells were collected at 2, 4, and 8 h, and washed twice as the control cells. FITC-labeled PlnE and an equal amount of PlnF were added in different collected cells to a final concentration of 25 μM. About 10 μL cell suspension was dropped immediately on a clean slide, gently covered with a coverslip, and immediately observed under a laser confocal microscope (Zeiss 710 META, Germany Zeiss Company, Germany). Meanwhile, the proportion of PlnEF distributed cells (the number of cells with typical fluorescence of FITC out of the total cell number) was determined using Flow cytometry (BD Calibur, BD Co., Franklin Lakes, NJ, United States). The total number of counting cells was set as 20,000.
Scanning and Transmission Electron Microscopy
Aeromonas hydrophila cells (∼1 × 109 CFU/mL, confirmed by plate count method) in saline containing 10 mmol/L glucose were added with 10 mM L-lactic acid, 25 μM PlnEF, 10 mM L-lactic acid combined with 25 μM PlnEF and incubated at 30°C for 2, 4, and 8 h. The cells without PlnEF or lactic acid were set as control. Cells for scanning electron microscopy (SEM) analysis were collected by centrifugation and fixed in 2.5% glutaraldehyde at 4°C for 2-4 h. After that, the cells were dehydrated with gradient alcohol solutions and further freeze-dried. The powder of dry cells was distributed on a conductive adhesive, coated with gold, and imaged using a versatile scanning electron microscope (SEM, FEI Quanta 200, Netherlands). Part of cells treated for 8 h was fixed with 2.5% glutaraldehyde, then be prepared to ultrathin slices according to the reference (Yamanaka et al., 2005) for further observation using a transmission electron microscope (TEM, Hitachi H-7650B, Japan).
Proteomics Analysis
Protein Extraction and Purification
Aeromonas hydrophila LPL-1 was collected by centrifugation (2,000 g, 4 min, 4°C) after 5 h incubation in LB broth and washed three times in sterile saline. The collection of bacterial cells was resuspended with (1) LB broth, (2) LB broth with 10 mM L-lactic acid, (3) LB broth with 10 mM L-lactic acid + 25 μM PlnEF, the viable count of A. hydrophila was equal in each sample as 1-3 × 109 CFU/ml. All the samples were cultured aerobically at 30°C for 8 h and then collected by centrifugation (3,000 g, 5 min, 4°C) and washed three times with cold, sterile saline. The total protein of A. hydrophila cells was extracted using a Bacterial Total Protein Extraction Kit BB-3182-50T (BestBio, Shanghai, China). The extracted protein was immediately purified three times by TCA-acetone precipitation. The purified protein was dissolved in Hydration Loading Buffer I (without DTT or Bio-Lyte), and quantified by the Bradford method, and stored at −20°C for the following experiments.
Two-Dimensional Electrophoresis
The separation of proteins was performed by two-dimensional electrophoresis (2DE) according to the two-dimensional electrophoresis step-by-step user instructions (BioRad, Hercules, CA, United States). The specific steps are as follows: 700 μg of total protein from each sample was diluted to up to 300 μL with Hydration loading buffer I (containing Dithiothreitol (DTT) and Bio-Lyte). Each mixture was loaded onto a 17 cm precast immobilized pH gradient (IPG) gel strip (pH gradient 4-7). The first-dimension separation-isoelectric focusing (IEF)-was then carried out in the Protean IEF Cell (Bio-Rad, Hercules, CA, United States). IPG DryStrips were equilibrated in a reducing agent followed by an alkylating agent. The second dimension was performed by placing the strips on 12% acrylamide gels (Bio-Rad, Hercules, CA, United States) to allow protein separation by electrophoresis in a Criterion™ Vertical Electrophoresis Cell (Bio-Rad, Hercules, CA, United States). The analytical gels were visualized with Bio-Rad Laboratories GS-710 Calibrated Imaging Densitometer Scanner after Coomassie Brilliant Blue G-250 staining. The digitalized 2-DE gel images were studied (protein spot detection, spot matching, and semi-quantitative statistical analysis) using PDQuest 2-D Analysis Software (Bio-Rad, Hercules, CA, United States).
MALDI-TOF/TOF Mass Spectrometry Analysis
Spots present in only one of the conditions or displayed quantitative abundance changes of more than 1.5-fold were selected for identification by MALDI-TOF/TOF. Protein spots of interest were picked from the stained gel and were then washed and digested. The samples were mixed with a matrix solution CCA (α-cyano-4-hydroxycinnamic acid), spotted on a MALDI plate (Applied Biosystems, Foster City, CA, United States), and allowed to air-dry. To obtain a peptide mass fingerprint (PMF), lists of peak intensities and mass-to-charge (m/z) values were analyzed with a 4,800 Proteomics Analyzer MALDI-TOF/TOF Mass Spectrometer (Applied Biosystems, Foster City, CA, United States).
Quantitative Real-Time PCR (RT-qPCR)
RT-qPCR was performed to confirm the mRNA level of identified proteins. The reactions were prepared using TriPure reagent, 2 × SYBR Green qPCR Mix, PC48-miRNA First-strand synthesis kit (Aidlab Biotechnologies Co., Ltd., Beijing, China), according to the manufacturer’s instructions. Fifteen genes were analyzed: acnB, sdhA, pckA, prpD, gyrB, gap, glpk, purA, rspA, turf1, turf2, tyrB, pnp, hptG, ligA. 16S rDNA was used as a control to normalize the values. Primers for qRT-PCR were designed using Primer3Plus (Untergasser et al., 2007). The sequences of the primers are presented in Supplementary Table 1. All statistical comparisons were performed using Student’s t-test (p < 0.05).
Statistics
All data are presented as Mean ± (SD) of 3 independent experiments. Data were analyzed using a one-way analysis of variance (ANOVA) with Dunnett’s test for comparisons to control. The SPSS 12.0 statistical software (IBM, CA, United States) was used for the analysis. p < 0.05 or p < 0.1 (two-dimensional electrophoresis) was considered significant.
Results
PlnEF Combined With Lactic Acid Showed Bacteriostatic and Bactericidal Activity Against Aeromonas hydrophila LPL-1
As shown in Figure 1, the combination of PlnEF and lactic acid showed greater bacteriostatic and bactericidal activity against A. hydrophila LPL-1. Introducing 10 mM lactic acid significantly increased the inhibitory activity of PlnEF against A. hydrophila LPL-1 (Figure 1A). In addition, with the presence of lactic acid (10 mM), the bacteriostatic activity of PlnEF showed a dose-dependent manner. The MIC of PlnEF was 25 μM that completely inhibited the growth of A. hydrophila LPL-1 within 12 h, shown as a low OD600 (0.08) as the absorption of LB broth. Besides, the co-treatment of lactic acid also improved the bactericidal activity of PlnEF against A. hydrophila LPL-1 (Figure 1B). The proportion of dead cells treated with 10 mM lactic acid and 25 μM PlnEF significantly increased in a time-dependent manner. After 8 h treatment, ∼40% of A. hydrophila LPL-1 cells were dead induced by lactic acid and PlnEF together, while only ∼20% were killed by lactic acid alone, and none were killed by PlnEF alone (The flow cytometry assay results were shown in Supplementary Figure 3). The MBC of PlnEF combined with 10 mM lactic acid against A. hydrophila LPL-1 was 75 μM, as determined by plate counting method (as shown in Supplementary Table 2).
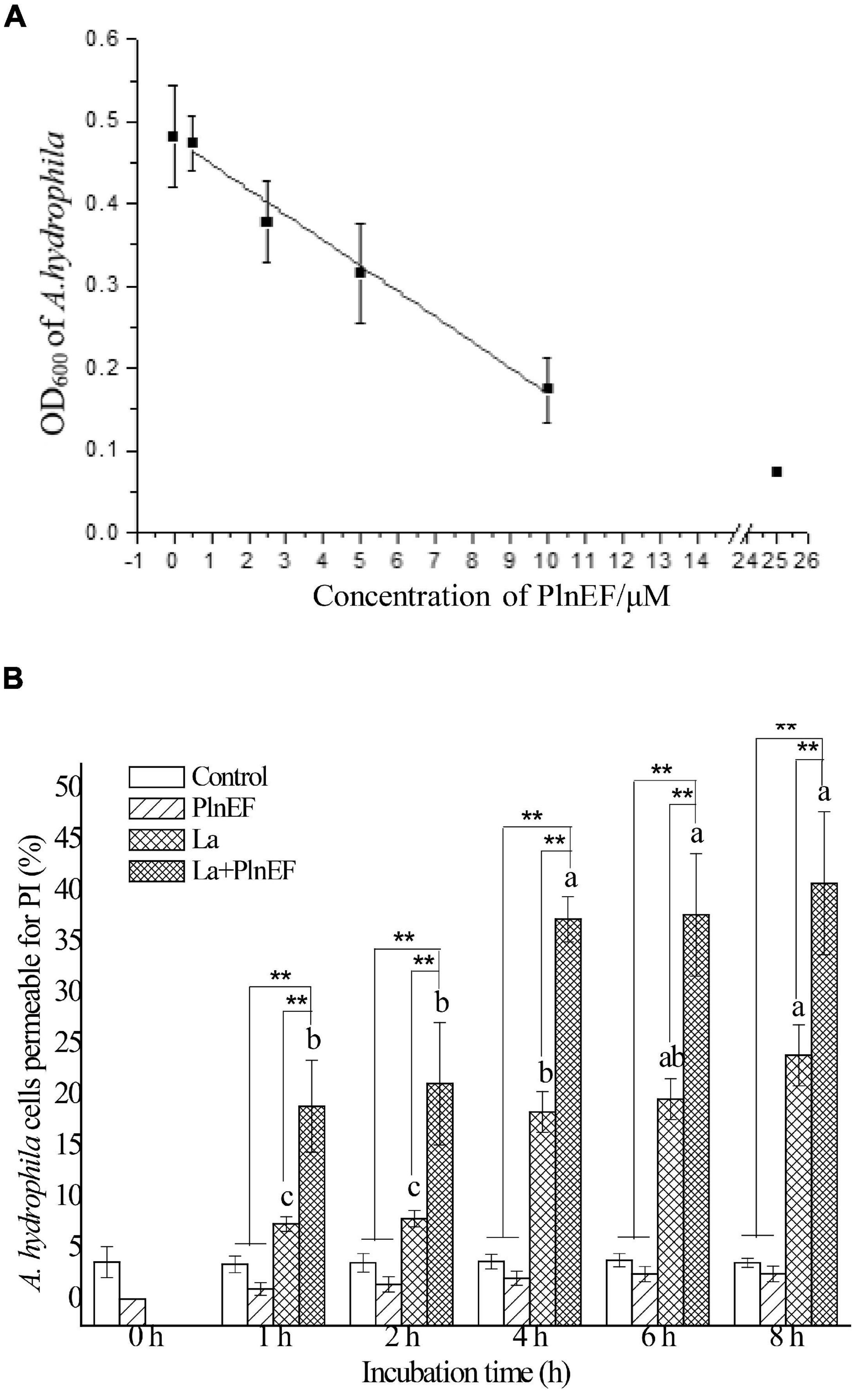
Figure 1. Effects of bacteriocin PlnEF and lactic acid co-treatment on inhibiting the growth and promoting lethality of A. hydrophila LPL-1. (A) Aeromonas hydrophila was cultured with lactic acid (10 mM) and different concentrations of PlnEF (0.5-25 μM) in 96-well cell culture plates for 12 h. The co-treatment of PlnEF and lactic acid significantly inhibited the growth of A. hydrophila. The results are representative of three independent experiments expressed as Means ± SD. (B) Aeromonas hyrophila cells were collected and treated with 10 mM lactic acid, 25 μM PlnEF and 10 mM lactic acid combined with 25 μM PlnEF at 30°C for 2, 4, 6, and 8 h. The co-treatment of PlnEF and lactic acid significantly promoted the proportion of dead cells. The results are representative of three independent experiments expressed as Means ± SD. Mean without a common letter indicated p < 0.05. Different letters of a–c indicated a significant difference between statistics of lactic acid and combined treatment at different time, ** indicated p < 0.01.
Lactic Acid Caused the Release of LPS From Aeromonas hydrophila LPL-1 Outer Membrane
The release of LPS by lactic acid and the interaction between LPS with PlnEF was investigated in A. hydrophila LPL-1. In our study, lactic acid treatment (5, 10, 12 mM) significantly induced the LPS release from A. hydrophila LPL-1 (Figure 2A). With the increase of concentration and incubation time, a significant increase of released LPS was observed, indicating a stimulatory effect of lactic acid on LPS release. The released LPS showed a characteristic absorption peak at 195 ~196 nm, while a significant red shift was observed after PlnEF treatment, suggesting a binding between PlnEF and LPS (Figure 2B). Notably, the peak values increased in a concentration-dependent manner of PlnEF, indicating an interaction between LPS and PlnEF.
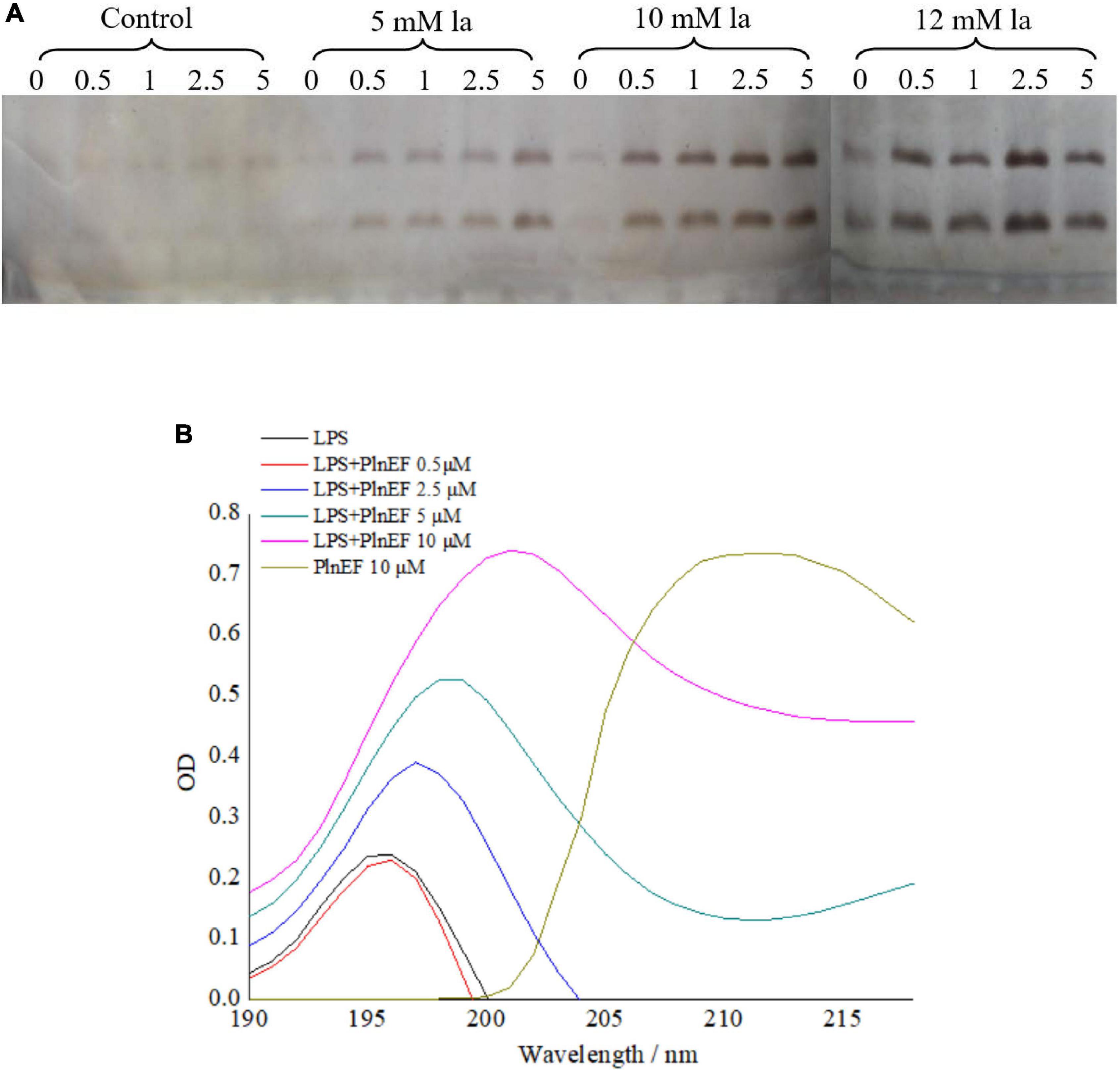
Figure 2. Interaction between bacteriocin PlnEF and LPS released from A. hydrophila LPL-1 (A) and effects of lactic acid on increasing LPS released from A. hydrophila LPL-1 (B). LPS released from A. hydrophila LPL-1 was treated with different concentrations of PlnEF (0.5, 2.5, 5, and 10 μM) showed a red shift in absorption peak. Aeromonas hyrophila LPL-1 cells were treated with different concentrations of lactic acid (5, 10, and 12 mM) for 0, 0.5, 1, 2.5, and 5 h. The release of LPS from A. hyrophila LPL-1 significantly increased by lactic acid in a dose-dependent and time-dependent manner.
Pre-Treatment With Lactic Acid Allowed the Accumulation of PlnEF on/in Aeromonas hydrophila LPL-1 Cells
The penetration and accumulation of PlnEF on/in A. hydrophila LPL-1 cells were measured with laser confocal microscopy. Without lactic acid pre-treatment, there was no FITC-labeled PlnE observed on/in A. hydrophila LPL-1 (Figure 3A). In comparison, there was an obvious accumulation of green fluorescence produced by FITC-labeled PlnE on/in A. hydrophila LPL-1 cells after pre-treated with lactic acid. Besides, the proportion of the green-fluorescent cells increased with the time prolongation of lactic acid pre-treatment (Figure 3A). The results of flow cytometry further showed a precise increasing proportion of FITC-positive cells (Figure 3B). After 6 h pre-treating with 10 mM lactic acid, up to 50% of the bacterial cells showed the fluorescent signal of FITC-labeled PlnE (Figure 3B). These results suggested that pre-treatment with lactic acid allowed the accumulation of PlnEF on/in A. hydrophila LPL-1 cells.
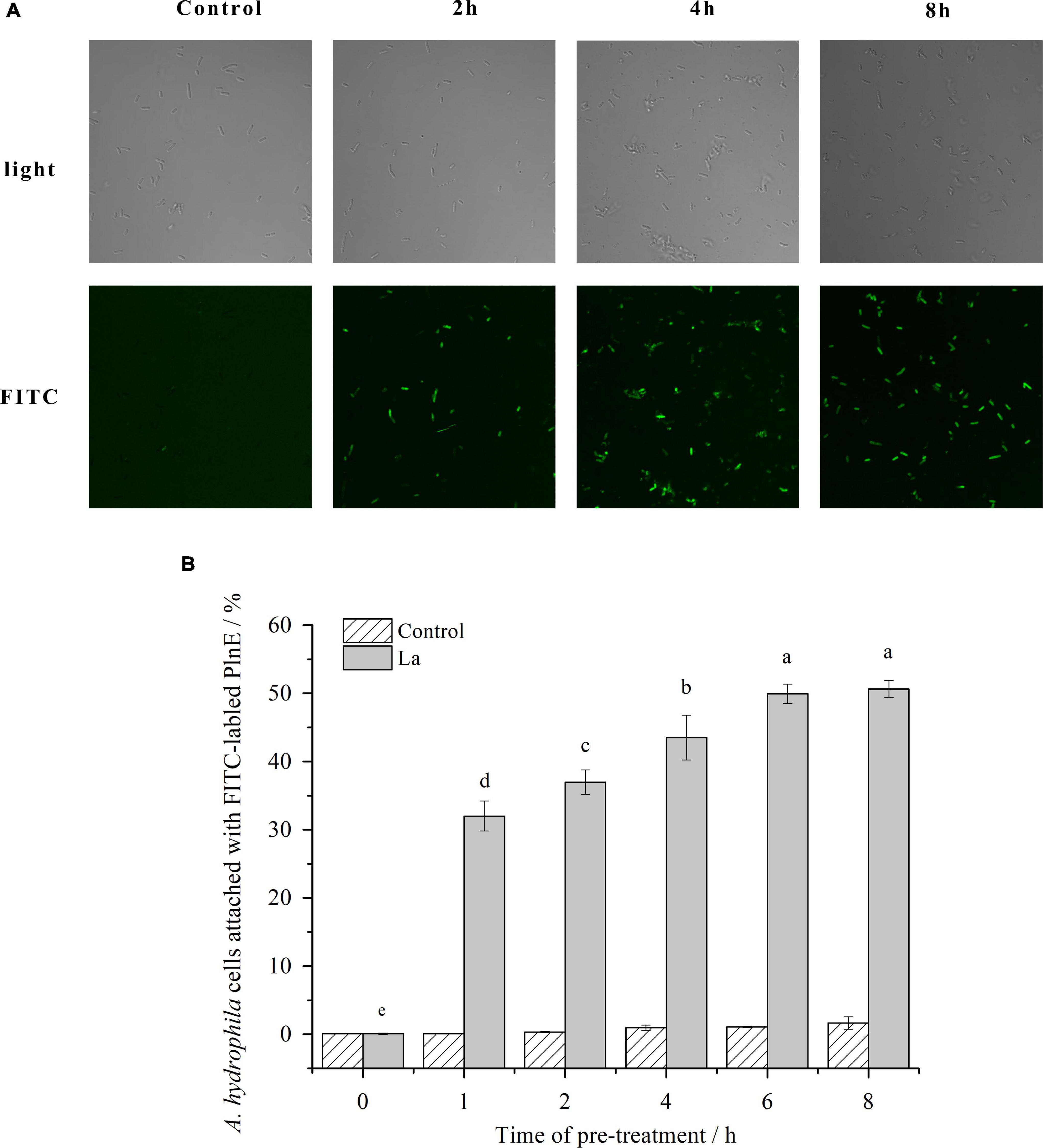
Figure 3. Effects of lactic acid on promoting the accumulation of FITC labeled PlnEF in A. hydophila LPL-1. Aeromonas hyrophila LPL-1 cells were treated with FITC labeled PlnEF and lactic acid (25 μM) for 2, 4, and 8h. The co-treatment significantly increased the number of FITC labeled PlnEF in A. hyrophila LPL-1. (A) The FITC positive cells under a laser confocal microscope. (B) The proportions of FITC-positive cells in A. hyrophila LPL-1. The results are representative of three independent experiments expressed as Means ± SD. Means without a common letter are significantly different (p < 0.05).
PlnEF Combined With Lactic Acid-Induced Significant Damage and Deterioration of Aeromonas hydrophila LPL-1 Cellular Structure
The effect of PlnEF and lactic acid either alone or in combination on cell morphological and structural change of A. hydrophila LPL-1 was studied using scanning electron microscopy (SEM) (Figure 4) and transmission electron microscopy (TEM) (Figure 5). Under the normal cultural condition, the control cells of A. hydrophila LPL-1 appeared as rod shapes with a blunt circle at both ends, with a smooth surface and intact morphology (Figure 4A). With increasing incubation time, moderate outer membrane damage (green arrows), cellular deformation, and shrinkage (blue arrow) were observed in a small portion of bacterial cells (Figure 4C). Meanwhile, some nanometer vesicles (yellow arrows) appeared on the surface of A. hydrophila LPL-1 control cells (Figure 4B). A. hydrophila LPL-1 treated with PlnEF alone had a similar morphology with the control cells (Figures 4D–E). During 8 h incubation with PlnEF, most of A. hydrophila LPL-1 cells remained typical smooth surface and rod shape with a few nanometer vesicles around (Figure 4E). However, the length of the A. hydrophila LPL-1 cells increased (purple arrows) when cultured with PlnEF compared against the control, and several cells had two suspected splitting points (pink arrows) (Figures 4D,F). Besides, apical surface protrusion (cyan arrow) was detected on a small proportion of bacterial cells (Figures 4D,F). Compared to PlnEF treatment alone, lactic acid treatment alone induced severer shrinkage (blue arrow) and apical surface protrusion (cyan arrow), and outer membrane damage (green arrow) in A. hydrophila LPL-1 cells (Figures 4H–J), indicating a strong disruption effect of lactic acid on the cell morphology. The strongest disruption was found when A. hydrophila LPL-1 was treated with lactic acid and PlnEF together. Serious surface deformation, shrinkage, collapse was observed in A. hydrophila LPL-1 cells (Figures 4K,L). Some of the cells even had visible holes (red arrow), and visible fragments of cracking bacteria (orange arrow). Furthermore, the combination of lactic acid and PlnEF also caused multiple splits (pink arrow) in several cells, and more nanometer vesicles (yellow arrow) appeared on the surface of the cells after 2 h treatment. Corresponding changes were found in the internal structures of A. hydrophila LPL-1 (Figure 5). The control cells of A. hydrophila LPL-1 were rod-shaped in the longitudinal section and elliptical-shaped in the cross-section (Figures 5A1–A4). All cells had clear edges of outer membranes (OM), cytoplasmic membrane (CM), and uniform periplasmic space (PS, the inner space between OM and CM). The cytoplasm was evenly distributed, shown as unanimous electron density. The DNA was distributed randomly in the cell, with some dark filamentous and dots in the middle of the DNA, possibly a supercoiled DNA (SCDNA). The PlnEF treated cells did not show any noticeable change in inter-structure compared to the control sample, indicating a limited effect of PlnEF on A. hydrophila LPL-1 (Figures 5B1–B4). Lactic acid-treated cells had visible deformation, such as irregular protrusion (cyan arrows), and lengthen (purple arrows) as well as evident outer membrane damage (red solid line arrows) (Figures 5C1–C4). Besides, a separation of the outer and inner membrane (white arrow) was observed. Moreover, the area of DNA was brighter (red dashed-line arrow) than control, and the content of the high electron density substance of DNA remarkably decreased. Similar to the SEM results, A. hydrophila LPL-1 treated with lactic acid combined with PlnEF showed more severe deformation on the inner structure (Figures 5D1–D8). The separation of the outer and inner membrane (white arrows) was observed. Cell inner membranes damaged (red dotted arrows) showing an incomplete and blurred shape. Protruding vesicles (yellow arrows) were captured. Cytoplasm loss was indicated by decreased electron density in most of the cells. An abnormal cytoplasm condense was revealed by deepening color through the center of the cell (brown arrows). Some cells were elongated (purple arrows). Besides, individual cells inflated at damaged parts of the outer membrane, forming a protruding part (cyan box) without the outer membrane’s surroundings. There were electron condensed particles, shown as dark granules (black solid line arrows), distributed between isolated cell walls and cell membranes.
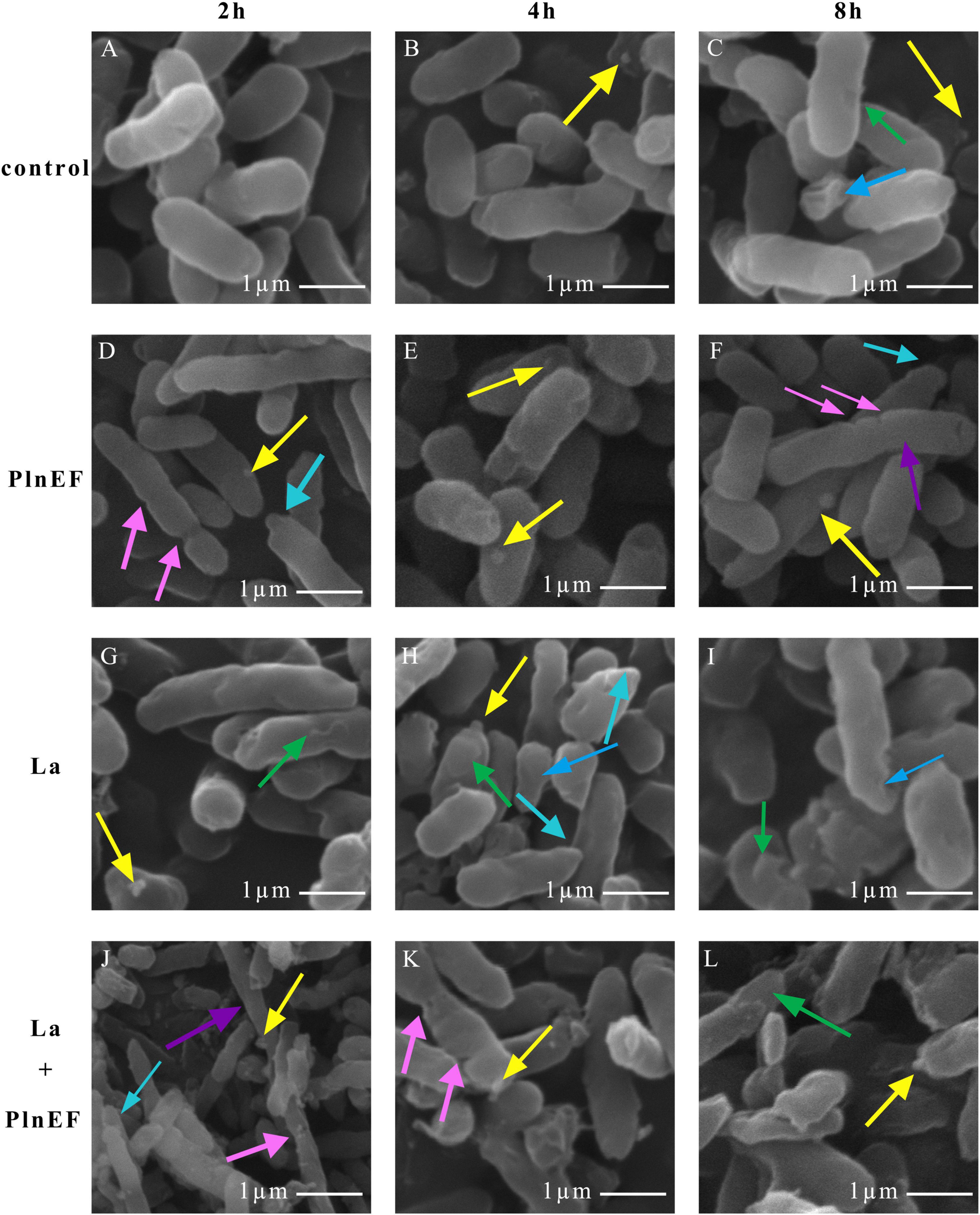
Figure 4. Effects of bacteriocin PlnEF and lactic acid co-treatment on changing cell morphology of A. hyrophila LPL-1. Aeromonas hyrophila LPL-1 cells were treated with lactic acid (10 mM) and/or PlnEF (25 μM) at 4°C for 2, 4, and 8 h. Images were observed using a scanning electron microscope (SEM). The scale bar indicates length 1 μm, HV = 15-20 kV, direct mag (20,000-50,000). (A–C) the control cells, (D–F) the PlnEF treated cells, (G–I) lactic acid treated cells, (J–L) PlnEF combined lactic acid treated cells. Lactic acid treatment induced outer membrane damage (green arrows), deformation and shrinkage (blue arrows), and apical surface protrusion (cyan arrow). The co-treatment of PlnEF and lactic acid induced extra small vesicles (yellow arrows), multiple splitting points (pink arrows), holes (red arrows), increase in length (purple arrows) and fragmentation of cracking bacteria (orange arrows).
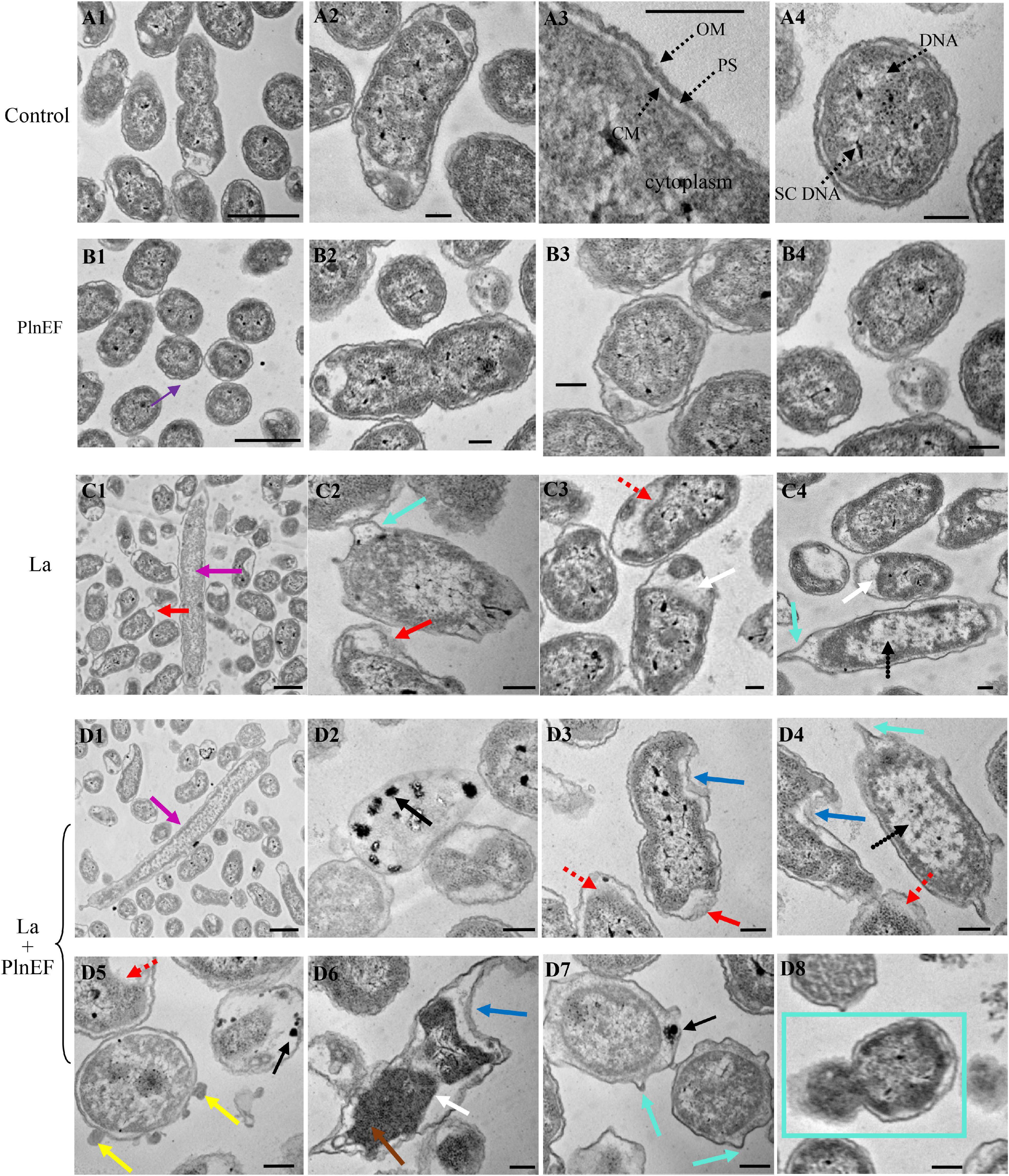
Figure 5. Effects of bacteriocin PlnEF and lactic acid co-treatment on affecting internal structural deformation of A. hydrophila. Aeromonas hyrophila LPL-1 cells were treated lactic acid (10 mM) and/or PlnEF (25 μM) at 4°C for 8 h. Images were observed using a Hitachi H-7650B transmission electron microscope (TEM). The scale bar indicates length 1 μm, HV = 80 kV, direct mag (20,000-1,00,000). (A1–A4) the control cells, (B1–B4) the PlnEF treated cells, (C1–C4) lactic acid treated cells, (D1–D8) PlnEF and lactic acid combined treated cells. The binary fission (BF), outer membranes (OM), cytoplasmic membrane (CM), periplasmic space (PS), supercoiled DNA (SCDNA) are visible. Lactic acid treatment induced protrusion (cyan arrows), en (purple arrows), outer membrane damage (red solid line arrows), cell inner membranes damaged (red dotted arrows), the outer and inner membrane separation (white arrows), and reduced electron density region (black dotted arrows). Co-treatment of PlnEF and lactic acid induced extra dark granules (black solid line arrows), small vesicles (yellow arrows), deepening (brown arrows), protruding part (cyan box), sag (blue arrows).
The Combination of Lactic Acid and PlnEF Leads to an Alternation of Proteomic Profile in Aeromonas hydrophila LPL-1.
A two-dimensional electrophoresis (2-DE) separation of total proteins, using the same concentrations of prepared proteins from A. hydrophila cells cultured in LB broth, and treated with lactic acid, plantaricin E/F either solely or in combination, were shown in Figures 6A–D. The differentially expressed protein spots based on the comparison of the control sample were pointed out in the reference map Figure 6E. The number of over-expressed protein numbers was 3, 12, and 8 for single PlnEF, single lactic acid, and their combination treatment, respectively. The down-expressed number of protein was 5, 49, and 30 in turn (Supplementary Tables 3–5). We identified 27 differentially expressed proteins in A. hydrophila cells (Data are available via ProteomeXchange with identifier PXD029702), and further tested the results by q-PCR (Figure 6F). The relative mRNA level of acnB, prpD, glpk, turf1/2 in the combined treated sample was consistent with the protein level. However, there was a discrepancy between mRNA and protein abundance of tyrB, pnp, htpG and ligA. The differently expressed proteins participated in several pathways (Table 1), including energy metabolism (TCA, glycolysis, pyruvate metabolism, gluconeogenesis, glycerophospholipid synthesis), amino and protein metabolism, purine, and pyrimidine metabolism, DNA replication, transcription and repair, peptide transport, and stress response. In terms of energy metabolism, AcnB and ADSS were significantly (p < 0.1) over-expressed, GAPDH was significantly (p < 0.1) down-expressed in the single lactic acid-treated sample. In the combined treated sample, there was a significant (p < 0.1) down-expression of AcnB, PrpD, and GK, suggesting an inhibition in energy metabolism by the combined treatment. In terms of protein synthesis, in the single lactic acid-treated sample, the levels of two detected elongation factor Tu (5,422 and 7,407) were significantly down-regulated, but the level of point 5,438 that also stands for elongation factor Tu significantly increased; the aromatic amino acid aminotransferase and threonyl-tRNA synthetase decreased statistically (p < 0.1). In combined treated samples, the levels of elongation factor Tu, threonyl-tRNA synthetase (5,422 and 5,438), and threonyl-tRNA synthetase were significantly (p < 0.1) higher than the control group. As for nucleotide synthesis, both single lactic acid treatment and combined treatment significantly (p < 0.1) reduced the level of polynucleotide phosphorylase/polyadenylase. Interestingly, single lactic acid treatment reduced the content of DNA gyrase subunit B, but combined treatment increased its level. The combined treatment also reduced the level of NAD-dependent DNA ligase. Hsp90 (heat shock protein 90) is a chaperone protein that assists other proteins in folding correctly. Combined treatment significantly (p < 0.1) reduced the level of heat shock protein 90, indicating a potential interference in protein folding and function.
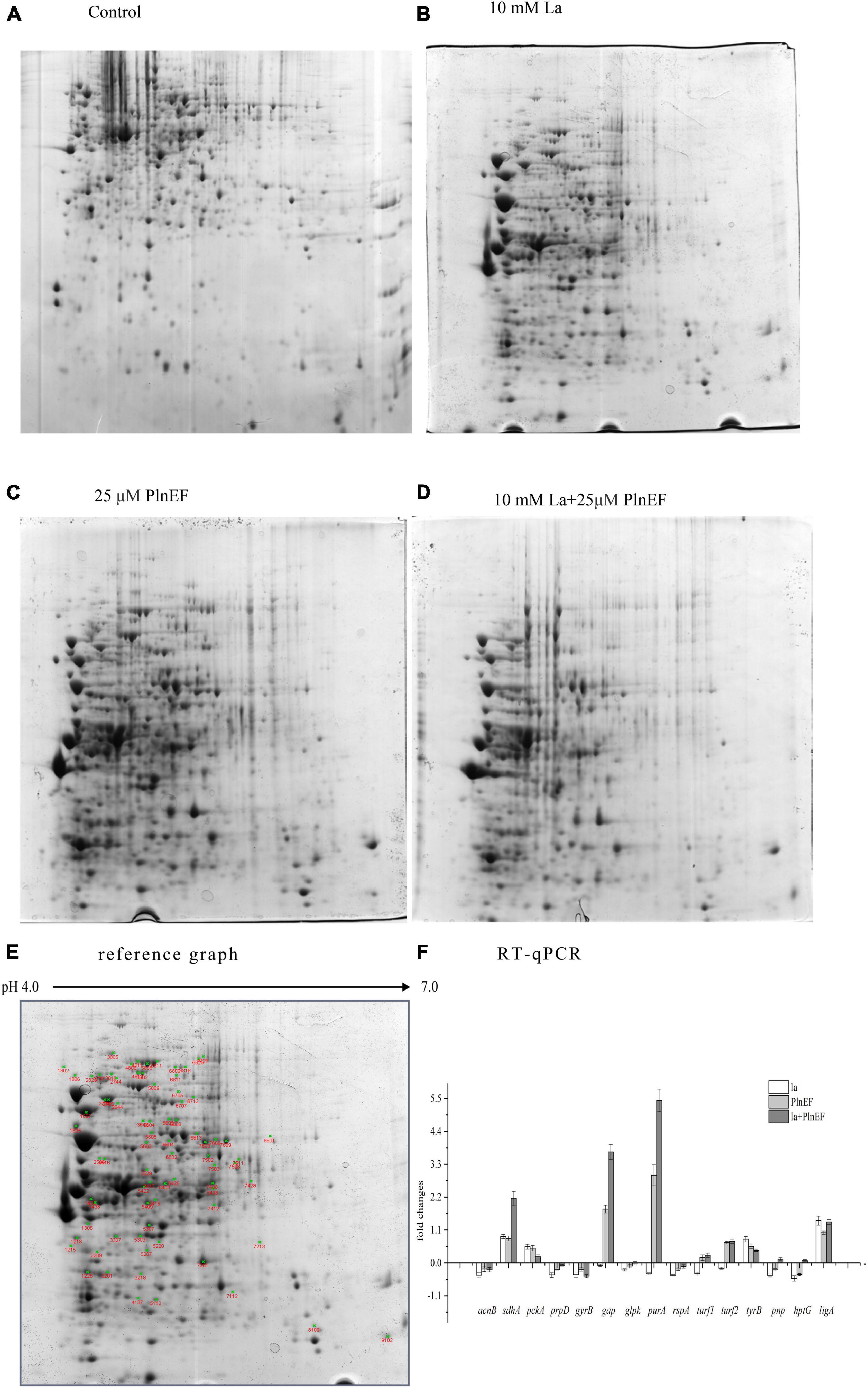
Figure 6. Effects of bacteriocin PlnEF and lactic acid co-treatment on the proteomic profile of A. hydrophila. (A–D) A two-dimensional electrophoresis (2-DE) separation of total proteins from A. hydrophila. The cells of A. hydrophila LPL-1 (1–3 × 109 CFU/ml) were cultured in LB broth (control), LB broth with 25 μM PlnEF; 10 mM L-lactic acid; and 10 mM L-lactic acid + 25μM PlnEF aerobically at 30°C for 8 h. The first dimension comprised an 17-cm non-linear pH 4–7 immobilized pH gradient (IPG) subjected to isoelectric focusing. The second dimension was a 21-cm 12% SDS-PAGE (sodium dodecyl sulfate polyacrylamide gel electrophoresis) gel. Proteins were detected by Coomassie Brilliant Blue G-250 staining. The non-linear pH range of the first-dimension IPG strip is indicated along the top of the gel, acidic pH to the left. The Mr (relative molecular mass) scale can estimate the molecular weights of the separated proteins. (E) Two-dimensional polyacrylamide gel electrophoresis (PAGE) reference map of the whole proteins from A. hydrophila LPL-1. Using the PDQuest software (Bio-Rad), the average gel of each population was compared with the reference map gel to identify the differentially expressed protein spots (green cross). (F) The relative mRNA levels of several genes in A. hydrophila LPL-1 treated by la-10mM lactic acid, PlnEF-25 μM PlnEF, la + EF-10 mM lactic acid + 25 μM PlnEF for 8 h revealed by Quantitative RT-PCR. acnB: bifunctional aconitate hydratase 2/2-methylisocitrate; sdhA: succinate dehydrogenase flavoprotein subunit; pckA: phosphoenolpyruvate carboxykinase; prpD: 2-methylcitrate dehydratase; gap: glyceraldehyde-3-phosphate dehydrogenase (GAPDH); glpk: glycerol kinase (GK); purA: adenylosuccinate synthetase (ADSS); rspA: 30S ribosomal subunit protein S1; turf1, turf2: elongation factor Tu; aromatic amino acid aminotransferase (AAA-ATs) gyrB: DNA gyrase subunit B; ligA. NAD-dependent DNA ligase; tyrB: threonyl-tRNA synthetase; pnp: polynucleotide phosphorylase/polyadenylase; hptG: heat shock protein 90.
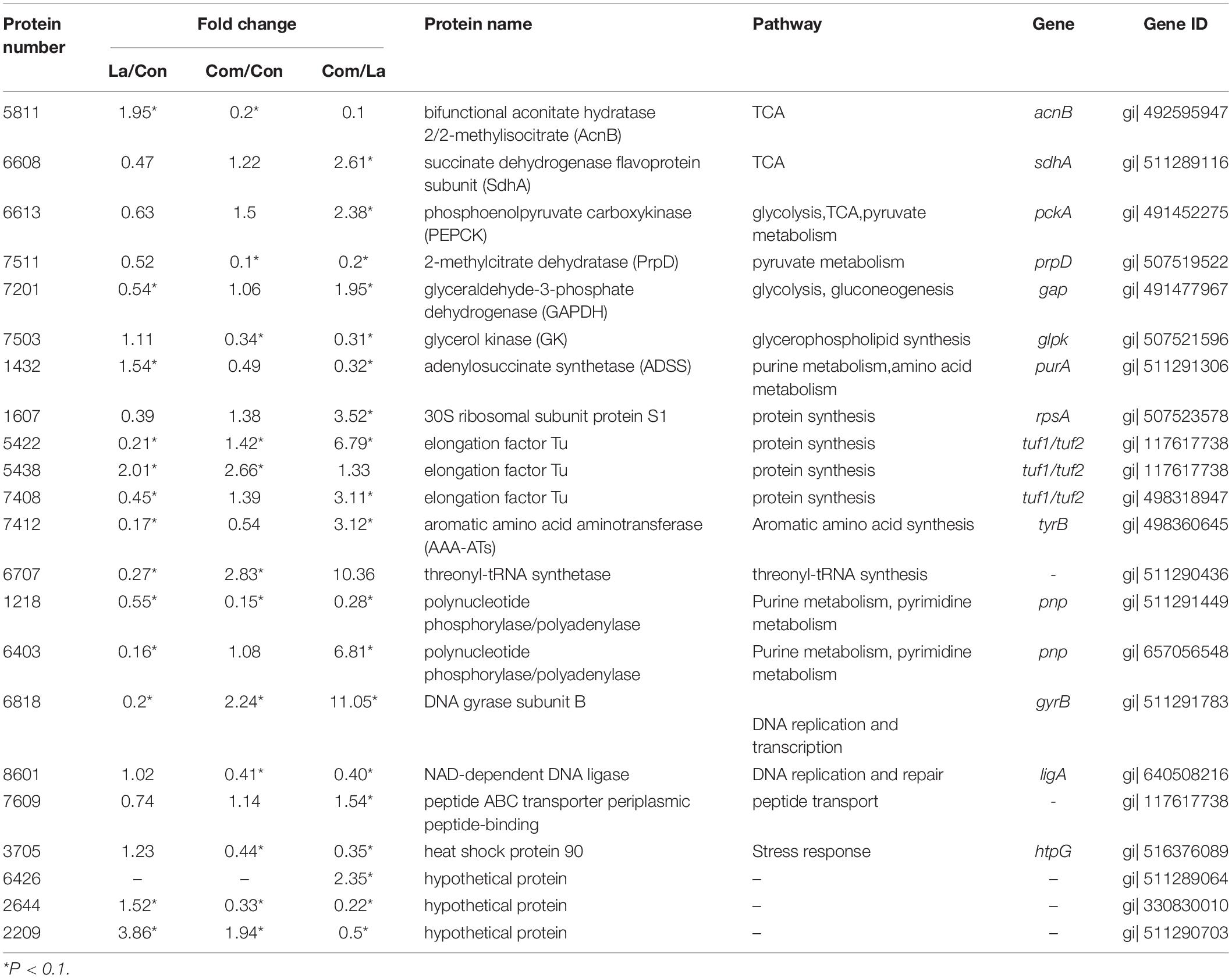
Table 1. The differently expressed proteins of A. hydrophila LPL-1 treated with lactic acid and PlnEF combined with lactic acid against control cells.
Discussion
Lactic acid and bacteriocin are two important metabolites of lactic acid bacteria, which have been reported for their antimicrobial activities (Barbosa et al., 2017; Komesu et al., 2017; Mokoena, 2017; Gao et al., 2019; Vieco-Saiz et al., 2019). However, there is less study on the synergistic inhibitory mechanism of bacteriocin and lactic acid. The present study investigated the synergistic inhibitory activity and mechanism of IIb bacteriocin PlnEF and lactic acid on potential Gram-negative pathogen A. hydrophila LPL-1.
In the present study, we found that combining class IIb bacteriocin-PlnEF with lactic acid significantly enhanced the inhibition ability against potential Gram-negative pathogen A. hydrophila LPL-1. Besides, we also found PlnEF and lactic acid had synergistic inhibition against several Gram-negative pathogens. Thus the inhibition activity against Gram-negative pathogen of PlnEF may be universal but not specific against A. hydrophila LPL-1 (Supplementary Figure 4). The result was an essential addition to the universal synergistic action of lactic acid and different bacteriocins, given that the synergistic inhibitory effects were confirmed between class I bacteriocin-nisin (Nykänen et al., 1998), class IIa pediocin AcH (Kalchayanand et al., 1992), and pediocin PA-1 (Wang et al., 2020). In addition, the PlnEF are cationic peptides and effective in micromolar-level. According to our previous study, the MIC (minimum inhibitory concentration) and the MBC (minimum bactericidal concentration) of PlnEF against Lactiplantibacillus plantarum (former Lactobacillus plantarum) were 8 μM and 16 μM, respectively (Zhang et al., 2016). In this study, the MIC and MBC of PlnEF against A. hydrophila were 25 and 75 μM when combined with 10 mM lactic acid. These results suggested a comparable but lower antibacterial efficiency of PlnEF against A. hydrophila to Gram-positive bacteria when lactic acid was incorporated. This is similar to our previous work, where the MIC of class IIa bacteriocin pediocin PA-1 in combination with lactic acid against A. hydrophila ATCC 35654 (50 μM) and CICC 10500 (30 μM) was also higher than its MIC (5 μM) against a sensitive Gram-positive bacteria L. plantarum (Wang et al., 2020).
The outer membrane (OM) of A. hydrophila works as an efficient permeability barrier to protect them against bacteriocin, such as PlnEF. L-lactic acid has been reported to be an efficient OM permeabilizer (Vaara, 1992), as well as exert the activity to released LPS from Gram-negative bacteria, such as Salmonella enterica serovar Typhimurium (Alakomi et al., 2000) and A. hydrophila ATCC 35654 (Wang et al., 2020). Our study observed the release of LPS by lactic acid in A. hydrophila LPL-1. Besides, the interaction, most likely the electrostatic interaction between PlnEF and LPS was found in this study, indicating the protective effect of LPS against bacteriocin in Gram-negative bacteria. However, with the treatment of lactic acid, the LPS barrier was damaged. And further transportation and accumulation of FITC-labeled PlnEF in A. hydrophila were revealed in the present study. Generally, to exert biological activity, antimicrobial peptides are required to interact and/or communicate with cells first (Hancock and Rozek, 2002). As PlnEF shows hydrophobic properties, there is a high possibility that the specific location of PlnEF is in the inner membrane by directly sticking into the membrane, which is believed to be the primary mechanism of membrane-leaking bacteriocin (Nissen-Meyer et al., 2009; Moghal et al., 2020). The loss of membrane integrity caused by the combination of PlnEF and lactic acid shown by SEM and TEM supports the idea that PlnEF anchor and cause injuries on the inner membrane. A similar result has been reported in our previous research on the synergistic inhibitory effect of lactic acid and class IIa bacteriocin pediocin PA-1 against A. hydrophila (Wang et al., 2020). As reported, class IIb bacteriocin used UppP (undecaprenyl pyrophosphate phosphatase) (Kjos et al., 2014), streptococcal membrane protein LsrS (Biswas and Biswas, 2014), or a Zn-dependent metallopeptidase (Uzelac et al., 2013) as their receptors. However, either the location of PlnEF or PlnEF-associated receptor was not identified in the present study yet. Further studies are needed to determine the receptor in A. hydrophila for PlnEF.
The A. hydrophila cells treated with lactic acid and PlnEF also exhibited morphological and size changes in our study. It has been claimed that environmental stress (Typas et al., 2012; Mueller and Levin, 2020), or substance interfering dividing related genes that affect the bacteria size and/or morphology (Domínguez-Cuevas et al., 2013; Vedyaykin et al., 2019; Di Somma et al., 2020). Combined treatment with PlnEF and lactic acid caused stress to cells, which could further affect the expression of genes and proteins related to the cell size and morphology. Besides, the PlnEF and lactic acid-treated cells showed a lot of vesicles, which were similar to the outer membrane vesicles (OMVs) reported from other studies, such as E. coli treated with sericin (Xue et al., 2016). Gram-negative bacteria could produce such OMVs, consisting of protein, lipid, and lipopolysaccharide enclosed by a lipid bilayer (Jan, 2017; Avila-Calderón et al., 2021), during growth or under pressure (Schwechheimer and Kuehn, 2015; Toyofuku et al., 2019). Our previous research also found OMVs induced by the synergistic treatment of lactic acid and class IIa bacteriocin pediocin PA-1 in A. hydrophila (Wang et al., 2020). These OMVs are believed to be toxic to other organisms and benefit the survival of their producing bacteria (Avila-Calderón et al., 2015). Supportively, we also found reduced cytotoxicity when A. hydrophila was treated with PlnEF and lactic acid (unpublished data). However, the involvement of OMVs and cytotoxicity reduction remains unclear. DNA is the most important molecule for cellular activity. In our study, the original DNA region became electron-light after treated with lactic acid, indicating an abnormal change in DNA conformation. This will result in a failure of cell division or other DNA-involved cellular activity. The lightening of electron density in the DNA region had been reported in A. hydrophila treated with lactic acid and PA-1 (Wang et al., 2020), Escherichia coli and Salmonella cells treated with lactic acid (Wang et al., 2015), Cronobacter sakazakii treated with Chrysanthemum buds crude extract (Chang et al., 2021), E. coli cells treated with polyhexamethylene (Zhou et al., 2010). Meanwhile, apparent condensation granules, supposed to be condensed DNA or protein, were visible in cells treated with lactic acid and PlnEF together in our study.
These results indicated a complicated and cooperative mechanism between PlnEF and lactic acid to inhibit A. hydrophila. As vesicles induction and loss of membrane integrity were typical in PlnEF sensitive cells after being treated with PlnEF (Zhang et al., 2016), we believe that one possible mechanism of PlnEF against A. hydrophila, under the assistance of lactic acid, was membrane disruption. Moreover, the treatments induced condense of cytoplasm, relaxed DNA, and failure of dividing might be an inspiring mechanism that contributes to the synergistic effect of PlnEF and lactic acid. The complex cellular morphological changes suggested that in addition to the enhanced OM permeabilizing action of PlnEF induced by lactic acid, the presence of PlnEF could stimulate the activity of lactic acid in return.
To further elucidate the mechanism involved in the inhibition of A. hydrophila, we had analyzed the differentially expressed proteins aroused by co-treatment of PlnEF and lactic acid. We found significant down-regulation of key proteins in TCA, suggesting inhibition of energy metabolism. Hotshock protein Hsp90 was down-regulated, but DNA gyrase GraB was up-regulated by co-treatment of PlnEF and lactic acid, suggesting abnormal protein folding and DNA status, which was in accordance with the emerging of outer membrane vesicles and reduced DNA electron density proved in SEM and TEM.
In conclusion, the present study investigated the synergistic bacteriostatic and bactericidal activity of bacteriocin PlnEF and lactic acid against potential Gram-negative pathogen, A. hydrophila LPL-1. The overall mechanism of the synergistic activity of PlnEF and lactic acid against A. hydrophila was shown in Figure 7. The LPS is acting as a barrier against PlnEF. Upon the release of LPS as induced by lactic acid, PlnEF integrates and causes damage in the inner membrane of A. hydrophila. In the center of the cell, there is a reduction of energy metabolism, down-regulation of Hsp90, and up-regulation of DNA gyrase GraB, indicating abnormal protein folding and DNA status, consistent with the outer membrane vesicles and reduced DNA electron density shown in SEM and TEM. Thereby, lactic acid and PlnEF synergistically inhibit bacterial growth and cause cell death.
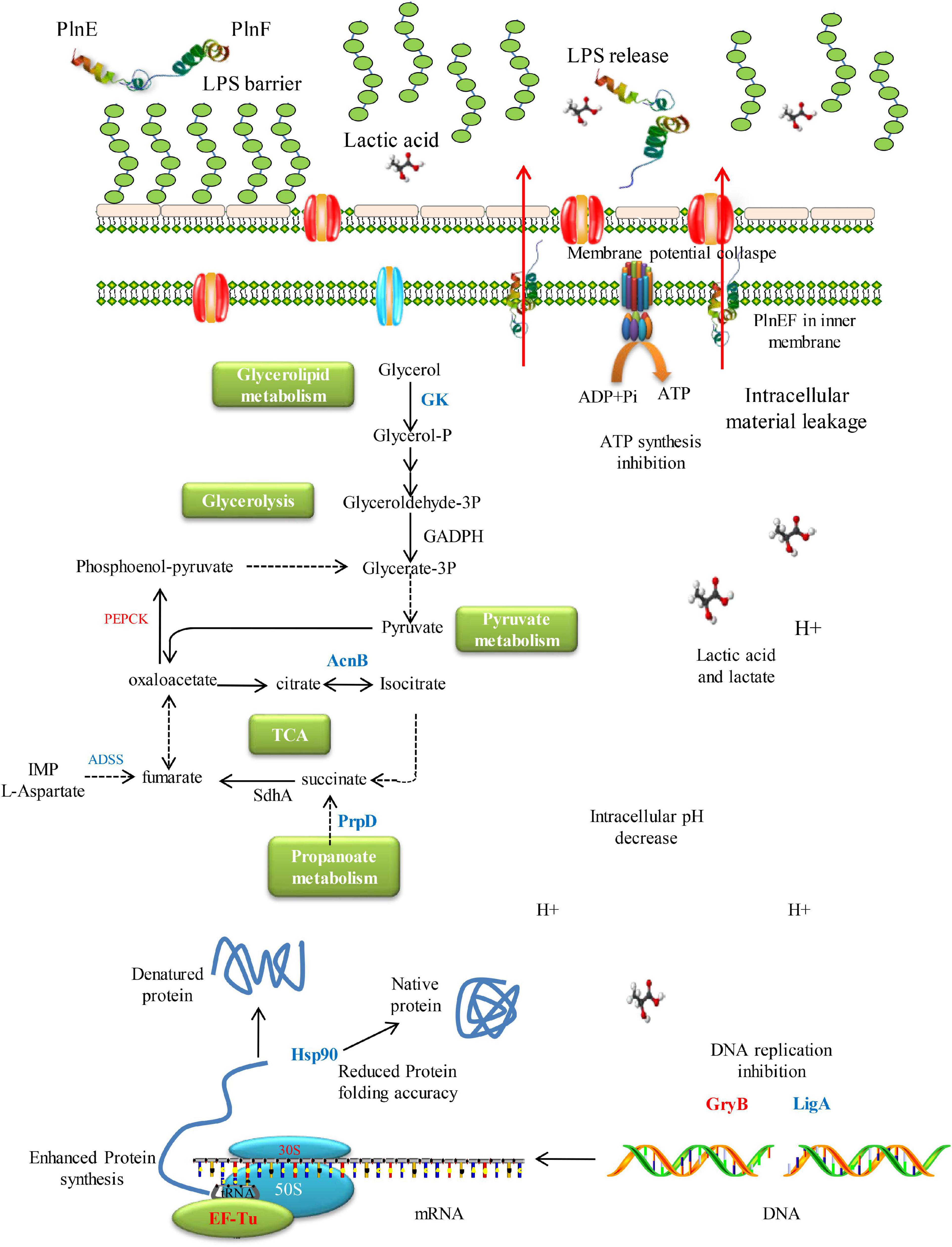
Figure 7. Schematic mechanism of inhibition of synergistic inhibition of PlnEF and lactic acid on A. hydrophila. The LPS works as a barrier against PlnEF. After the release of LPS induced by lactic acid, PlnEF insert into the inner membrane of A. hydrophila, causing the collapse of membrane potential. In the center of the cell, reduction of energy metabolism, down-regulation of Hsp90 but up-regulation of DNA gyrase GraB, suggesting abnormal protein folding and DNA status, which was in accordance with the outer membrane vesicles and reduced DNA electron density proved in SEM and TEM.
Data Availability Statement
The whole-genome sequencing data of A. hydrophila LPL-1 are deposited in GenBank, accession number PRJNA767215. The proteome profiling data are deposited in ProteomeXchange, accession number PXD029702.
Author Contributions
All authors listed have made a substantial, direct, and intellectual contribution to the work, and approved it for publication.
Funding
This work was supported by the National Natural Science Foundation of China (NSFC, Grant Nos. 32172172, 31671831, and 31702360) and Beijing Innovation Team Project of Sturgeon and Trout (BAIC08-2021).
Conflict of Interest
The authors declare that the research was conducted in the absence of any commercial or financial relationships that could be construed as a potential conflict of interest.
Publisher’s Note
All claims expressed in this article are solely those of the authors and do not necessarily represent those of their affiliated organizations, or those of the publisher, the editors and the reviewers. Any product that may be evaluated in this article, or claim that may be made by its manufacturer, is not guaranteed or endorsed by the publisher.
Supplementary Material
The Supplementary Material for this article can be found online at: https://www.frontiersin.org/articles/10.3389/fmicb.2022.774184/full#supplementary-material
Footnote
References
Abdulhussain Kareem, R., and Razavi, S. H. (2020). Plantaricin bacteriocins: as safe alternative antimicrobial peptides in food preservation—A review. J. Food Saf. 40:e12735. doi: 10.1111/jfs.12735
Alakomi, H.-L., Saarela, M., and Helander, I. M. (2003). Effect of EDTA on Salmonella enterica serovar Typhimurium involves a component not assignable to lipopolysaccharide release. Microbiology 149, 2015–2021. doi: 10.1099/mic.0.26312-0
Alakomi, H.-L., Skyttä, E., Saarela, M., Mattila-Sandholm, T., Latva-Kala, K., and Helander, I. M. (2000). Lactic acid permeabilizes Gram-negative bacteria by disrupting the outer membrane. Appl. Environ. Microbiol. 66, 2001–2005. doi: 10.1128/AEM.66.5.2001-2005.2000
Alvarez-Sieiro, P., Montalbán-López, M., Mu, D., and Kuipers, O. P. (2016). Bacteriocins of lactic acid bacteria: extending the family. Appl. Microbiol. Biotechnol. 100, 2939–2951. doi: 10.1007/s00253-016-7343-9
Anjur, N., Sabran, S. F., Daud, H. M., and Othman, N. Z. (2021). An update on the ornamental fish industry in Malaysia: Aeromonas hydrophila-associated disease and its treatment control. Vet. World 14, 1143–1152. doi: 10.14202/vetworld.2021.1143-1152
Anumudu, C., Hart, A., Miri, T., and Onyeaka, H. (2021). Recent advances in the application of the antimicrobial peptide nisin in the inactivation of spore-forming bacteria in foods. Molecules 26:5552. doi: 10.3390/molecules26185552
Avila-Calderón, E. D., Araiza-Villanueva, M. G., Cancino-Diaz, J. C., López-Villegas, E. O., Sriranganathan, N., Boyle, S. M., et al. (2015). Roles of bacterial membrane vesicles. Arch. Microbiol. 197, 1–10. doi: 10.1007/s00203-014-1042-7
Avila-Calderón, E. D., Ruiz-Palma, M. D. S., Aguilera-Arreola, M., Velázquez-Guadarrama, N., Ruiz, E. A., Gomez-Lunar, Z., et al. (2021). Outer membrane vesicles of Gram-negative bacteria: an outlook on biogenesis. Front. Microbiol. 12:557902. doi: 10.3389/fmicb.2021.557902
Barbosa, A. A. T., Mantovani, H. C., and Jain, S. (2017). Bacteriocins from lactic acid bacteria and their potential in the preservation of fruit products. Crit. Rev. Biotechnol. 37, 852–864. doi: 10.1080/07388551.2016.1262323
Biswas, S., and Biswas, I. (2014). A conserved streptococcal membrane protein, LsrS, exhibits a receptor-like function for lantibiotics. J. Bacteriol. 196, 1578–1587. doi: 10.1128/JB.00028-14
Bonhi, K., and Imran, S. (2019). Role of bacteriocin in tackling the global problem of multi-drug resistance: an updated review. Biosci. Biotechnol. Res. Commun. 12, 601–608. doi: 10.21786/bbrc/12.3/8
Cetuk, H., Anishkin, A., Scott, A. J., Rempe, S. B., Ernst, R. K., and Sukharev, S. (2021). Partitioning of seven different classes of antibiotics into LPS monolayers supports three different permeation mechanisms through the outer bacterial membrane. Langmuir 37, 1372–1385. doi: 10.1021/acs.langmuir.0c02652
Chang, Y., Xing, M., Hu, X., Feng, H., Wang, Y., Guo, B., et al. (2021). Antibacterial activity of Chrysanthemum buds crude extract against Cronobacter sakazakii and its application as a natural disinfectant. Front. Microbiol. 11:632177. doi: 10.3389/fmicb.2020.632177
Chi, H., and Holo, H. (2018). Synergistic antimicrobial activity between the broad spectrum bacteriocin garvicin KS and nisin, farnesol and polymyxin B against Gram-positive and Gram-negative bacteria. Curr. Microbiol. 75, 272–277. doi: 10.1007/s00284-017-1375-y
Christaki, E., Marcou, M., and Tofarides, A. (2020). Antimicrobial resistance in bacteria: mechanisms, evolution, and persistence. J. Mol. Evol. 88, 26–40. doi: 10.1007/s00239-019-09914-3
Ciepluch, K., Skrzyniarz, K., Barrios-Gumiel, A., Quintana, S., and Arabski, M. (2019). Dendronized silver nanoparticles as bacterial membrane permeabilizers and their interactions with P. aeruginosa lipopolysaccharides, lysozymes, and phage-derived endolysins. Front. Microbiol. 10:2771. doi: 10.3389/fmicb.2019.02771
Cotter, P. D., Hill, C., and Ross, R. P. (2005). Bacteriocins: developing innate immunity for food. Nat. Rev. Microbiol. 3, 777–788. doi: 10.1038/nrmicro1273
Cotter, P. D., Ross, R. P., and Hill, C. (2013). Bacteriocins—a viable alternative to antibiotics? Nat. Rev. Microbiol. 11, 95–105. doi: 10.1038/nrmicro2937
Cui, Y., Luo, L., Wang, X., Lu, Y., Yi, Y., Shan, Y., et al. (2021). Mining, heterologous expression, purification, antibactericidal mechanism, and application of bacteriocins: a review. Compr. Rev. Food Sci. Food Saf. 20, 863–899. doi: 10.1111/1541-4337.12658
Dashe, D., Hansen, E. B., Kurtu, M. Y., Berhe, T., Eshetu, M., Hailu, Y., et al. (2020). Preservation of raw camel milk by lactoperoxidase system using hydrogen peroxide producing lactic acid bacteria. Open J. Anim. Sci. 10, 387–401. doi: 10.4236/ojas.2020.103024
Daskalov, H. (2006). The importance of Aeromonas hydrophila in food safety. Food Control. 17, 474–483. doi: 10.1016/j.foodcont.2005.02.009
Di Somma, A., Avitabile, C., Cirillo, A., Moretta, A., Merlino, A., Paduano, L., et al. (2020). The antimicrobial peptide Temporin L impairs E. coli cell division by interacting with FtsZ and the divisome complex. Biochim. Biophys. Acta Gen. Subj. 1864:129606. doi: 10.1016/j.bbagen.2020.129606
Domínguez-Cuevas, P., Porcelli, I., Daniel, R. A., and Errington, J. (2013). Differentiated roles for MreB-actin isologues and autolytic enzymes in Bacillus subtilis morphogenesis. Mol. Microbiol. 89, 1084–1098. doi: 10.1111/mmi.12335
Ekblad, B., Kyriakou, P. K., Oppegard, C., Nissen-Meyer, J., Kaznessis, Y. N., and Kristiansen, P. E. (2016). Structure–function analysis of the two-peptide bacteriocin plantaricin EF. Biochemistry 55, 5106–5116. doi: 10.1021/acs.biochem.6b00588
Fomsgaard, A., Freudenberg, M., and Galanos, C. (1990). Modification of the silver staining technique to detect lipopolysaccharide in polyacrylamide gels. J. Clin. Microbiol. 28, 2627–2631. doi: 10.1128/jcm.28.12.2627-2631.1990
Fu, W., and Kapila, Y. L. (2021). Nisin, a probiotic bacteriocin, combined with limited chemoradiation therapy in head and neck cancer. Arch. Clin. Med. Case Rep. 5, 531–536. doi: 10.26502/acmcr.96550389
Gao, Y., Van Belkum, M. J., and Stiles, M. E. (1999). The outer membrane of Gram-negative bacteria inhibits antibacterial activity of brochocin-C. Appl. Environ. Microbiol. 65, 4329–4333. doi: 10.1128/AEM.65.10.4329-4333.1999
Gao, Z., Daliri, E. B.-M., Wang, J., Liu, D., Chen, S., Ye, X., et al. (2019). Inhibitory effect of lactic acid bacteria on foodborne pathogens: a review. J. Food Prot. 82, 441–453. doi: 10.4315/0362-028X.JFP-18-303
García, P., Rodríguez, L., Rodríguez, A., and Martínez, B. (2010). Food biopreservation: promising strategies using bacteriocins, bacteriophages and endolysins. Trends Food Sci. Technol. 21, 373–382. doi: 10.1016/j.tifs.2010.04.010
Gharsallaoui, A., Oulahal, N., Joly, C., and Degraeve, P. (2016). Nisin as a food preservative: part 1: physicochemical properties, antimicrobial activity, and main uses. Crit. Rev. Food Sci. Nutr. 56, 1262–1274.
Haghighatafshar, H., Talebi, R., and Tukmechi, A. (2021). The effect of bacteriocin isolated from Lactobacillus rhamnosus on Pseudomonas aeruginosa lipopolysaccharides. Avicenna J. Clin. Microbiol. Infect. 8, 45–50. doi: 10.34172/ajcmi.2021.09
Hancock, R. E. W., and Rozek, A. (2002). Role of membranes in the activities of antimicrobial cationic peptides. FEMS Microbiol. Lett. 206, 143–149. doi: 10.1111/j.1574-6968.2002.tb11000.x
Helander, I. M., Alakomi, H.-L., Latva-Kala, K., and Koski, P. (1997). Polyethyleneimine is an effective permeabilizer of Gram-negative bacteria. Microbiology 143, 3193–3199. doi: 10.1099/00221287-143-10-3193
Ibarra-Sánchez, L. A., El-Haddad, N., Mahmoud, D., Miller, M. J., and Karam, L. (2020). Invited review: advances in nisin use for preservation of dairy products. J. Dairy Sci. 103, 2041–2052. doi: 10.3168/jds.2019-17498
Igbinosa, I. H., Igumbor, E. U., Aghdasi, F., Tom, M., and Okoh, A. I. (2012). Emerging Aeromonas species infections and their significance in public health. Sci. World J. 2012, 1–13. doi: 10.1100/2012/625023
Jan, A. T. (2017). Outer membrane vesicles (OMVs) of Gram-negative bacteria: a perspective update. Front. Microbiol. 8:1053. doi: 10.3389/fmicb.2017.01053
Johansen, C., Verheul, A., Gram, L., Gill, T., and Abee, T. (1997). Protamine-induced permeabilization of cell envelopes of Gram-positive and Gram-negative bacteria. Appl. Environ. Microbiol. 63, 1155–1159. doi: 10.1128/aem.63.3.1155-1159.1997
Kalchayanand, N., Hanlin, M., and Ray, B. (1992). Sublethal injury makes Gram-negative and resistant Gram-positive bacteria sensitive to the bacteriocins, pediocin AcH and nisin. Lett. Appl. Microbiol. 15, 239–243. doi: 10.1111/j.1472-765X.1992.tb00773.x
Kali, A., Kalaivani, R., Charles, P., and Seetha, K. (2016). Aeromonas hydrophila meningitis and fulminant sepsis in preterm newborn: a case report and review of literature. Indian J. Med. Microbiol. 34, 544–557. doi: 10.4103/0255-0857.195383
Kjos, M., Oppegård, C., Diep, D. B., Nes, I. F., Veening, J. W., Nissen-Meyer, J., et al. (2014). Sensitivity to the two-peptide bacteriocin lactococcin G is dependent on UppP, an enzyme involved in cell-wall synthesis. Mol. Microbiol. 92, 1177–1187. doi: 10.1111/mmi.12632
Komesu, A., de Oliveira, J. A. R., da Silva Martins, L. H., Maciel, M. R. W., and Maciel Filho, R. (2017). Lactic acid production to purification: a review. BioResources 12, 4364–4383. doi: 10.15376/biores.12.2.komesu
Kumariya, R., Garsa, A. K., Rajput, Y. S., Sood, S. K., Akhtar, N., and Patel, S. (2019). Bacteriocins: classification, synthesis, mechanism of action and resistance development in food spoilage causing bacteria. Microb. Pathog. 128, 171–177. doi: 10.1016/j.micpath.2019.01.002
Lopetuso, L. R., Giorgio, M. E., Saviano, A., Scaldaferri, F., Gasbarrini, A., and Cammarota, G. (2019). Bacteriocins and bacteriophages: therapeutic weapons for gastrointestinal diseases? Int. J. Mol. Sci. 20:183. doi: 10.3390/ijms20010183
MacNair, C. R., and Brown, E. D. (2020). Outer membrane disruption overcomes intrinsic, acquired, and spontaneous antibiotic resistance. mBio 11:e01615-20. doi: 10.1128/mBio.01615-20
Maldonado-Barragán, A., Caballero-Guerrero, B., Jiménez, E., Jiménez-Díaz, R., Ruiz-Barba, J. L., and Rodríguez, J. M. (2009). Enterocin C, a class IIb bacteriocin produced by E. faecalis C901, a strain isolated from human colostrum. Int. J. Food Microbiol. 133, 105–112. doi: 10.1016/j.ijfoodmicro.2009.05.008
Martin-Visscher, L. A., Yoganathan, S., Sit, C. S., Lohans, C. T., and Vederas, J. C. (2011). The activity of bacteriocins from Carnobacterium maltaromaticum UAL307 against Gram-negative bacteria in combination with EDTA treatment. FEMS Microbiol. Lett. 317, 152–159. doi: 10.1111/j.1574-6968.2011.02223.x
Moghal, M. M. R., Hossain, F., and Yamazaki, M. (2020). Action of antimicrobial peptides and cell-penetrating peptides on membrane potential revealed by the single GUV method. Biophys. Rev. 12, 339–348. doi: 10.1007/s12551-020-00662-z
Mokoena, M. P. (2017). Lactic acid bacteria and their bacteriocins: classification, biosynthesis and applications against uropathogens: a mini-review. Molecules 22:1255. doi: 10.3390/molecules22081255
Moradi, M., Kousheh, S. A., Almasi, H., Alizadeh, A., Guimarães, J. T., Yılmaz, N., et al. (2020). Postbiotics produced by lactic acid bacteria: the next frontier in food safety. Compr. Rev. Food Sci. Food Saf. 19, 3390–3415. doi: 10.1111/1541-4337.12613
Mueller, E. A., and Levin, P. A. (2020). Bacterial cell wall quality control during environmental stress. mBio 11:e2456-20. doi: 10.1128/mbio.02456-20
Mzula, A., Wambura, P. N., Mdegela, R. H., and Shirima, G. M. (2019). Current state of modern biotechnological-based Aeromonas hydrophila vaccines for aquaculture: a systematic review. BioMed Res. Int. 2019, 1–11. doi: 10.1155/2019/3768948
Nissen-Meyer, J., Oppegård, C., Rogne, P., Haugen, H. S., and Kristiansen, P. E. (2010). Structure and mode-of-action of the two-peptide (Class-IIb) bacteriocins. Probiot. Antimicrob. Proteins 2, 52–60. doi: 10.1007/s12602-009-9021-z
Nissen-Meyer, J., Rogne, P., Oppegard, C., Haugen, H. S., and Kristiansen, P. E. (2009). Structure-function relationships of the non-lanthionine-containing peptide (class II) bacteriocins produced by Gram-positive bacteria. Curr. Pharm. Biotechnol. 10, 19–37. doi: 10.2174/138920109787048661
Nykänen, A., Vesanen, S., and Kallio, H. (1998). Synergistic antimicrobial effect of nisin whey permeate and lactic acid on microbes isolated from fish. Lett. Appl. Microbiol. 27, 345–348. doi: 10.1046/j.1472-765X.1998.00450.x
Osmanağaoğlu, Ö (2005). Sensitivity of sublethally injured Gram-negative bacteria to pediocin P. J. Food Saf. 25, 266–275. doi: 10.1111/j.1745-4565.2005.00022.x
Ottaviani, D., Parlani, C., Citterio, B., Masini, L., Leoni, F., Canonico, C., et al. (2011). Putative virulence properties of Aeromonas strains isolated from food, environmental and clinical sources in Italy: a comparative study. Int. J. Food Microbiol. 144, 538–545. doi: 10.1016/j.ijfoodmicro.2010.11.020
Özcelik, S., Kuley, E., and Özogul, F. (2016). Formation of lactic, acetic, succinic, propionic, formic and butyric acid by lactic acid bacteria. LWT 73, 536–542. doi: 10.1016/j.lwt.2016.06.066
Pal, M. (2018). Is Aeromonas hydrophila a potential pathogen of food safety concern. J. Food Microbiol. 2, 1–2.
Praveen, P. K., Debnath, C., Shekhar, S., Dalai, N., and Ganguly, S. (2016). Incidence of Aeromonas spp. infection in fish and chicken meat and its related public health hazards: a review. Vet. World 9, 6–11. doi: 10.14202/vetworld.2016.6-11
Riley, M. A., and Wertz, J. E. (2002). Bacteriocins: evolution, ecology, and application. Annu. Rev. Microbiol. 56, 117–137. doi: 10.1146/annurev.micro.56.012302.161024
Rumjuankiat, K., Perez, R. H., Pilasombut, K., Keawsompong, S., Zendo, T., Sonomoto, K., et al. (2015). Purification and characterization of a novel plantaricin, KL-1Y, from Lactobacillus plantarum KL-1. World J. Microbiol. Biotechnol. 31, 983–994. doi: 10.1007/s11274-015-1851-0
Sahoo, T. K., Jena, P. K., Patel, A. K., and Seshadri, S. (2015). Purification and molecular characterization of the novel highly potent bacteriocin TSU4 produced by Lactobacillus animalis TSU4. Appl. Biochem. Biotechnol. 177, 90–104. doi: 10.1007/s12010-015-1730-z
Santos, J., Sousa, R., Otoni, C., Moraes, A., Souza, V., Medeiros, E., et al. (2018). Nisin and other antimicrobial peptides: production, mechanisms of action, and application in active food packaging. Innov. Food Sci. Emerg. Technol. 48, 179–194. doi: 10.1016/j.ifset.2018.06.008
Savage, P. B. (2001). Multidrug-resistant bacteria: overcoming antibiotic permeability barriers of Gram-negative bacteria. Ann. Med. 33, 167–171. doi: 10.3109/07853890109002073
Schwechheimer, C., and Kuehn, M. J. (2015). Outer-membrane vesicles from Gram-negative bacteria: biogenesis and functions. Nat. Rev. Microbiol. 13, 605–619. doi: 10.1038/nrmicro3525
Sheoran, P., and Tiwari, S. K. (2019). Enterocin LD3 from Enterococcus hirae LD3 causing efflux of intracellular ions and UV-absorbing materials in Gram-negative bacteria. J. Appl. Microbiol. 126, 1059–1069. doi: 10.1111/jam.14203
Sheoran, P., and Tiwari, S. K. (2021). Synergistically-acting enterocin LD3 and plantaricin LD4 against Gram-positive and Gram-negative pathogenic bacteria. Probiot. Antimicrob. Proteins 13, 542–554. doi: 10.1007/s12602-020-09708-w
Stratev, D., and Odeyemi, O. A. (2016). Antimicrobial resistance of Aeromonas hydrophila isolated from different food sources: a mini-review. J. Infect. Public Health 9, 535–544. doi: 10.1016/j.jiph.2015.10.006
Todorov, S. D., and Dicks, L. M. T. (2005). Lactobacillus plantarum isolated from molasses produces bacteriocins active against Gram-negative bacteria. Enzyme Microb. Technol. 36, 318–326. doi: 10.1016/j.enzmictec.2004.09.009
Toyofuku, M., Nomura, N., and Eberl, L. (2019). Types and origins of bacterial membrane vesicles. Nat. Rev. Microbiol. 17, 13–24. doi: 10.1038/s41579-018-0112-2
Typas, A., Banzhaf, M., Gross, C. A., and Vollmer, W. (2012). From the regulation of peptidoglycan synthesis to bacterial growth and morphology. Nat. Rev. Microbiol. 10, 123–136. doi: 10.1038/nrmicro2677
Untergasser, A., Nijveen, H., Rao, X., Bisseling, T., Geurts, R., and Leunissen, J. A. (2007). Primer3Plus, an enhanced web interface to Primer3. Nucleic Acids Res. 35, W71–W74. doi: 10.1093/nar/gkm306
Uzelac, G., Kojic, M., Lozo, J., Aleksandrzak-Piekarczyk, T., Gabrielsen, C., Kristensen, T., et al. (2013). A Zn-dependent metallopeptidase is responsible for sensitivity to LsbB, a Class II leaderless bacteriocin of Lactococcus lactis subsp. lactis BGMN1-5. J. Bacteriol. 195, 5614–5621. doi: 10.1128/jb.00859-13
Vaara, M. (1992). Agents that increase the permeability of the outer membrane. Microbiol. Rev. 56, 395–411. doi: 10.1128/mr.56.3.395-411.1992
Vedyaykin, A. D., Ponomareva, E. V., Khodorkovskii, M. A., Borchsenius, S. N., and Vishnyakov, I. E. (2019). Mechanisms of bacterial cell division. Microbiology 88, 245–260. doi: 10.1134/s0026261719030159
Vieco-Saiz, N., Belguesmia, Y., Raspoet, R., Auclair, E., Gancel, F., Kempf, I., et al. (2019). Benefits and inputs from lactic acid bacteria and their bacteriocins as alternatives to antibiotic growth promoters during food-animal production. Front. Microbiol. 10:57. doi: 10.3389/fmicb.2019.00057
Wang, C., Chang, T., Yang, H., and Cui, M. (2015). Antibacterial mechanism of lactic acid on physiological and morphological properties of Salmonella Enteritidis, Escherichia coli and Listeria monocytogenes. Food Control 47, 231–236. doi: 10.1016/j.foodcont.2014.06.034
Wang, Y., Wang, J. R., Bai, D. Q., Wei, Y. L., Sun, J. F., Luo, Y. L., et al. (2020). Synergistic inhibition mechanism of pediocin PA-1 and L-lactic acid against Aeromonas hydrophila. Biochim. Biophys. Acta Biomembr. 1862:183346. doi: 10.1016/j.bbamem.2020.183346
Wu, A., Fu, Y., Kong, L., Shen, Q., Liu, M., Zeng, X., et al. (2021). Production of a class IIb bacteriocin with broad-spectrum antimicrobial activity in Lactiplantibacillus plantarum RUB1. Probiot. Antimicrob. Proteins 13, 1820–1832. doi: 10.1007/s12602-021-09815-2
Xue, R., Liu, Y., Zhang, Q., Liang, C., Qin, H., Liu, P., et al. (2016). Shape changes and interaction mechanism of Escherichia coli cells treated with sericin and use of a sericin-based hydrogel for wound healing. Appl. Environ. Microbiol. 82, 4663–4672. doi: 10.1128/AEM.00643-16
Yamanaka, M., Hara, K., and Kudo, J. (2005). Bactericidal actions of a silver ion solution on Escherichia coli, studied by energy-filtering transmission electron microscopy and proteomic analysis. Appl. Environ. Microbiol. 71, 7589–7593. doi: 10.1128/AEM.71.11.7589-7593.2005
Yang, W., Ying, S., Xu, Z., Zhang, Z., Song, J., Meng, G., et al. (2015). Bacteriocin-producing probiotics enhance the safety and functionality of sturgeon sausage. Food Control. 50, 729–735. doi: 10.1016/j.foodcont.2014.09.045
Yin, X., Heeney, D., Srisengfa, Y., Golomb, B., Griffey, S., and Marco, M. (2018). Bacteriocin biosynthesis contributes to the anti-inflammatory capacities of probiotic Lactobacillus plantarum. Beneficial Microbes 9, 333–344. doi: 10.3920/bm2017.0096
Zendo, T., Koga, S., Shigeri, Y., Nakayama, J., and Sonomoto, K. (2006). Lactococcin Q, a novel two-peptide bacteriocin produced by Lactococcus lactis QU 4. Appl. Environ. Microbiol. 72, 3383–3389. doi: 10.1128/AEM.72.5.3383-3389.2006
Zhang, X., Wang, Y., Liu, L., Wei, Y., Shang, N., Zhang, X., et al. (2016). Two-peptide bacteriocin PlnEF causes cell membrane damage to Lactobacillus plantarum. BBA Biomembr. 1858, 274–280. doi: 10.1016/j.bbamem.2015.11.018
Keywords: lactic acid, bacteriocin, Gram-negative bacteria, lipopolysaccharide, inhibition
Citation: Wang Y, Wei Y, Shang N and Li P (2022) Synergistic Inhibition of Plantaricin E/F and Lactic Acid Against Aeromonas hydrophila LPL-1 Reveals the Novel Potential of Class IIb Bacteriocin. Front. Microbiol. 13:774184. doi: 10.3389/fmicb.2022.774184
Received: 11 September 2021; Accepted: 04 January 2022;
Published: 15 February 2022.
Edited by:
Giovanna Batoni, University of Pisa, ItalyReviewed by:
Debendra K. Sahoo, Institute of Microbial Technology (CSIR), IndiaZhaoxin Lu, Nanjing Agricultural University, China
Copyright © 2022 Wang, Wei, Shang and Li. This is an open-access article distributed under the terms of the Creative Commons Attribution License (CC BY). The use, distribution or reproduction in other forums is permitted, provided the original author(s) and the copyright owner(s) are credited and that the original publication in this journal is cited, in accordance with accepted academic practice. No use, distribution or reproduction is permitted which does not comply with these terms.
*Correspondence: Nan Shang, bnNoYW5nQGNhdS5lZHUuY24=; Pinglan Li, bGlwaW5nbGFuQGNhdS5lZHUuY24=.