- 1Department of Clinical Sciences, Institute of Tropical Medicine Antwerp, Antwerp, Belgium
- 2Department of Medicine, University of Cape Town, Cape Town, South Africa
Antimicrobial resistance in Neisseria gonorrhoeae is an important global health concern. The genetically related commensal Neisseria act as a reservoir of resistance genes, and horizontal gene transfer (HGT) has been shown to play an important role in the genesis of resistance to cephalosporins and macrolides in N. gonorrhoeae. In this study, we evaluated if there was evidence of HGT in the genes gyrA/gyrB and parC/parE responsible for fluoroquinolone resistance. Even though the role of gyrB and parE in quinolone resistance is unclear, the subunits gyrB and parE were included as zoliflodacin, a promising new drug to treat N. gonorrhoeae targets the gyrB subunit. We analyzed a collection of 20,047 isolates; 18,800 N. gonorrhoeae, 1,238 commensal Neisseria spp., and nine Neisseria meningitidis. Comparative genomic analyses identified HGT events in genes, gyrA, gyrB, parC, and parE. Recombination events were predicted in N. gonorrhoeae and Neisseria commensals. Neisseria lactamica, Neisseria macacae, and Neisseria mucosa were identified as likely progenitors of the HGT events in gyrA, gyrB, and parE, respectively.
Introduction
Neisseria gonorrhoeae, a gram-negative diplococcus, is an obligate human pathogen that causes the sexually transmitted infection gonorrhea (Ligon, 2005; Tapsall et al., 2009). Neisseria gonorrhoeae has been remarkably adept at developing resistance to antimicrobials, including β-lactams, macrolides, and fluoroquinolones. This has resulted in N. gonorrhoeae being included as a high priority pathogen by the WHO (Willcox, 1970; CDC, 1985; Unemo and Shafer, 2011; Chesson et al., 2014; Blair et al., 2015; Tacconelli and Magrini, 2017). Current treatment guidelines typically advocate using only ceftriaxone or in combination with azithromycin (Unemo et al., 2020). Increasing incidence of resistance to both ceftriaxone and azithromycin have led to novel treatment strategies. A particularly promising novel agent, zoliflodacin, a spiropyrimidinetrione, whose target site is gyrB is currently being evaluated in phase III studies following successful phase II studies (Alm et al., 2015; Taylor et al., 2018; Bradford et al., 2020). Recent trials have also established that ciprofloxacin (CIP) can be used to treat gonorrhea if genotyping confirms the absence of gyrA mutations (Allan-Blitz et al., 2017). This renewed interest in agents targeting the DNA gyrases led to the current study to assess if gyrA/B and parC/E mutations in N. gonorrhoeae can be acquired by horizontal gene transfer (HGT).
The first-generation quinolone, nalidixic acid, was first used in 1962 (Lesher et al., 1962). The second-generation fluoroquinolone, CIP, was first used clinically in the mid-1980s, and from 1993, it was used to treat uncomplicated gonorrhea (CDC, 1993; Davis et al., 1996). Fluoroquinolones target DNA gyrase (topoisomerase II) and topoisomerase IV, the enzymes involved in the DNA replication process (Drlica and Zhao, 1997; Larkin et al., 2007). During DNA replication, DNA gyrase catalyzes the unwinding of DNA molecules and topoisomerase IV decatenates daughter chromosomes following DNA replication (Reece and Maxwell, 1991; Kato et al., 1992; Zechiedrich and Cozzarelli, 1995; Zechiedrich et al., 1997). DNA gyrase is comprised of two subunits, GyrA and GyrB (molecular mass ≈96 and 88 kDa, respectively), which are homologous to the C and E subunits of topoisomerase IV, designated as ParC (molecular mass ≈88 kDa), and ParE (molecular mass ≈70 kDa), respectively. Ciprofloxacin resistance in N. gonorrhoeae is caused by point mutations in the quinolone resistance-determining region (QRDR) of gyrA and parC, which results in amino acid substitutions that alter the target protein structure, thereby reducing the fluoroquinolone-target enzyme binding affinity, leading to resistance (Belland et al., 1994; Piddock, 1999; Alcalá et al., 2003; Bodoev and Il’ina, 2015; Yahara et al., 2020). The majority of the resistance mutations in gonococci are located in the QRDR regions, in codons 67–106 in GyrA and 56–108 in ParC (Belland et al., 1994). The known resistant-associated mutations (RAMs) include substitutions at amino acid positions S91 and D95 in GyrA and G85, D86, S87, S88, Q91, and R116 in ParC (Piddock, 1999; Yang et al., 2006; Bodoev and Il’ina, 2015). Studies have shown that GyrA is the primary target and that ParC is the secondary target for fluoroquinolones in N. gonorrhoeae (Wittmann et al., 1973; Belland et al., 1994; Trees et al., 1999; Tanaka et al., 2000; Shultz et al., 2001; Lindbäck et al., 2002).
The genus Neisseria includes several commensals and two pathogens—N. gonorrhoeae and Neisseria meningitidis. Neisseria species are naturally competent and transfer DNA to one another (Catlin, 1960; Higashi et al., 2011). HGT of penA, mtrCDE, rpsE, and rplD from commensal Neisseria has been shown to be important in the genesis of resistance to β-lactams and macrolides in the pathogenic Neisseria (Bowler et al., 1994; Shafer, 2018; Wadsworth et al., 2018; Yahara et al., 2018; Fiore et al., 2020; Manoharan-Basil et al., 2021). A study from Shanghai has similarly demonstrated that HGT from gyrA in commensal Neisseria played a critical role in the genesis of fluoroquinolone resistance in N. meningitidis (Chen et al., 2020). HGT from commensal streptococci has also been shown to have played a role in the genesis of fluoroquinolone resistance in Streptococcus pneumoniae (Irene et al., 1998; Janoir et al., 1999; José et al., 2000). Recently, Yahara et al., 2021 suggested that fluoroquinolone resistance substitutions at GyrA and ParC in N. gonorrhoeae isolates have arisen from independent mutations and evidence of HGT in gyrA/B and parC/E were not available for N. gonorrhoeae. It is unknown but essential to establish if HGT has played a role in fluoroquinolone resistance in N. gonorrhoeae. If commensal Neisseria can serve as a reservoir of resistance for N. gonorrhoeae, then addressing the resistance determinants in commensals is important (de Korne-Elenbaas et al., 2021; Kenyon et al., 2021). The above considerations led to the current study, where we used a dataset of 20,047 globally sourced N. gonorrhoeae (1928–2020), N. meningitidis and Neisseria commensals to assess if there was evidence of HGT in the four fluoroquinolone resistance-conferring genes (gyrA/B and parC/E). This led to the identification of HGT in the QRDR regions of gyrA, gyrB, and parE in N. gonorrhoeae.
Materials and Methods
Dataset for Analysis
The isolates for the analyses were downloaded from the Pathosystems Resource Integration Center V3.6.9 (PATRIC) database (Davis et al., 2020), GenBank,1 pubMLST (Jolley et al., 2018), and pathogenwatch.2 Whole-genome sequence (WGS) data from our previous study with an additional 8,388 isolates were used in the current study (Manoharan-Basil et al., 2021). WGS data comprised 20,047 isolates, of which 18,800 were N. gonorrhoeae, nine were N. meningitidis, and 1,238 were commensal Neisseria isolates. Supplementary Table 1 in Data Sheet 1 summarizes the organisms used in this study. The European Committee on Antimicrobial Susceptibility Testing breakpoints was used to define ciprofloxacin susceptibility.3 The minimum inhibitory concentration (MIC) to ciprofloxacin (CIP) ≥16 mg/L, >0.06 mg/L, 0.03 ≤ 0.06, and <0.03 mg/L are classified as being high-level resistant (HLR), resistant (R), intermediate (I), and susceptible (S) to CIP, respectively (Sánchez-Busó et al., 2021). The isolates were categorized into three distinct eras: pre-antibiotic (pre-1950s), golden (1950–1970s), and post-modern (1980-20-first century) following the convention of Golparian et al. (2020).
Gene-By-Gene Analysis, Allelic Profiling, and Recombination Analysis
The analysis was carried out as described in Manoharan-Basil et al. (2021). In brief, WGS (n = 20,047) were analyzed using chewBBACA version 2.8.5 (Silva et al., 2018), followed by recombination analysis using Recombination Detection Program (RDP4) program version 4.100 (Martin et al., 2015). Firstly, a training file was created from the complete genome of N. gonorrhoeae FA1090 using Prodigal and was used in subsequent steps (Hyatt et al., 2010). Secondly, a study-specific Neisseria scheme was created from two complete Neisseria gonorrhoeae genomes (FA1090 and MS11). Thirdly, a FASTA file for each coding sequence (CDS) was generated, followed by the creation of whole-genome (wg) multi-locus sequence typing (MLST) loci. The core-genome (cg) MLST loci were then extracted from the wgMLST loci and visualized using a grape tree (Zhou et al., 2018). GrapeTree facilitates the clustering of the isolates based on their allelic profiles using a minimum spanning algorithm. The SchemaEvaluator option implemented in chewBBACCA uses MAFFT that allows multiple sequence alignments of the alleles of each locus and constructs neighbor-joining (NJ) tree using ClustalW2 (Katoh et al., 2002; Larkin et al., 2007). Finally, UniProtFinder4 was used to retrieve the functional information of the CDSs. GyrA, gyrB, parC, and parE genes were identified based on the UniProt identifier. The multiple sequence alignments and NJ trees of the above genes were extracted from the schema evaluator. The gene-by-gene analysis was carried out on a server with Intel(R) Xeon(R) Silver 4114 CPU @ 2.20 GHz with 125 Gb RAM and HDD distributed RAID-5 using 20 CPU. The analysis took ~15 days to complete.
The multiple sequence alignment files were imported in MEGAX version 10.1.7, and the CDSs were translated (Kumar et al., 2018). The presence or absence of non-synonymous substitutions in the QRDR regions for each amino acid position was denoted as “0” and “1,” respectively. The NJ trees and the corresponding metadata were visualized using Interactive tree of life (iTOL) v6 (Letunic and Bork, 2021) and microreact (Argimón et al., 2016).
Whole-genome sequence of commensal Neisseria spp., (n = 1,247) along with N. meningitidis (n = 9) and N. gonorrhoeae (n = 18,800) were used in the recombination analysis (Supplementary Table 1 in Data Sheet 1). The nucleotide alignments of each gene were screened for recombination events using the Recombination Detection Program (RDP4) program (Martin et al., 2015). Recombinant events supported by at least two of the seven algorithms available in RDP software: RDP, GENECONV, Bootscan, Maxchi, Chimera, SiSscan, and 3Seq were used with default settings, except the window size was increased to 60 nt in RDP, 120 nt in MaxChi and Chimera, and to 500 in BootScan and SiScan (Lemey et al., 2009; Gomez-Valero et al., 2011; Manoharan-Basil et al., 2021). NJ trees were constructed using 1,000 bootstrap replicates (Salminen et al., 1995). According to the definition in RDP4, the minor parent is the parental sequence that contributes the smaller fraction of the recombinant sequence, while the major parent is the parental sequence that contributes the larger fraction (Martin et al., 2015). Additionally, for the sequences which had the same recombination event, the percentage similarities of the nucleotide were determined between donor and recipient sequences. Furthermore, to identify the RAM harboring donors of the gyrA and parC in N. gonorrhoeae and Neisseria commensal isolates, RDP4 analysis was carried out as above and was limited to all Neisseria commensal and N. gonorrhoeae alleles with known RAMs.
Statistical Analysis
Statistical analyses were performed using JMP®, version 14.0.0 (SAS Institute Inc., Cary, NC, 1989–2021). To evaluate the correlation between multiple pairs of variables (SNPs) and to assess the correlations between phenotypic and genotypic patterns of resistance to ciprofloxacin, Spearman’s rank correlation was used (Elhadidy et al., 2020). Geometric mean (GM) MICs were calculated for each allele. A value of p of <0.001 was considered statistically significant.
Results
Characteristics of Isolates Used in the Study
A total of 20,047 isolates from 1928 to 2020 were used in the study and included 18,800 (93.7%) N. gonorrhoeae, nine (0.47%) N. meningitidis, and 1,247 (6.2%) Neisseria commensal isolates. Neisseria commensal included the human nasopharyngeal commensals N. polysaccharea (n = 74), N. bergeri (n = 66), N. lactamica (n = 699), N. cinerea (n = 52), N. subflava (n = 98), N. oralis (n = 8), N. mucosa (n = 38), N. elongata (n = 32), and N. bacilliformis (n = 8), along with several species isolated from animals and animal-related source (n = 163; Supplementary Table 1 in Data Sheet 1; Figure 1).
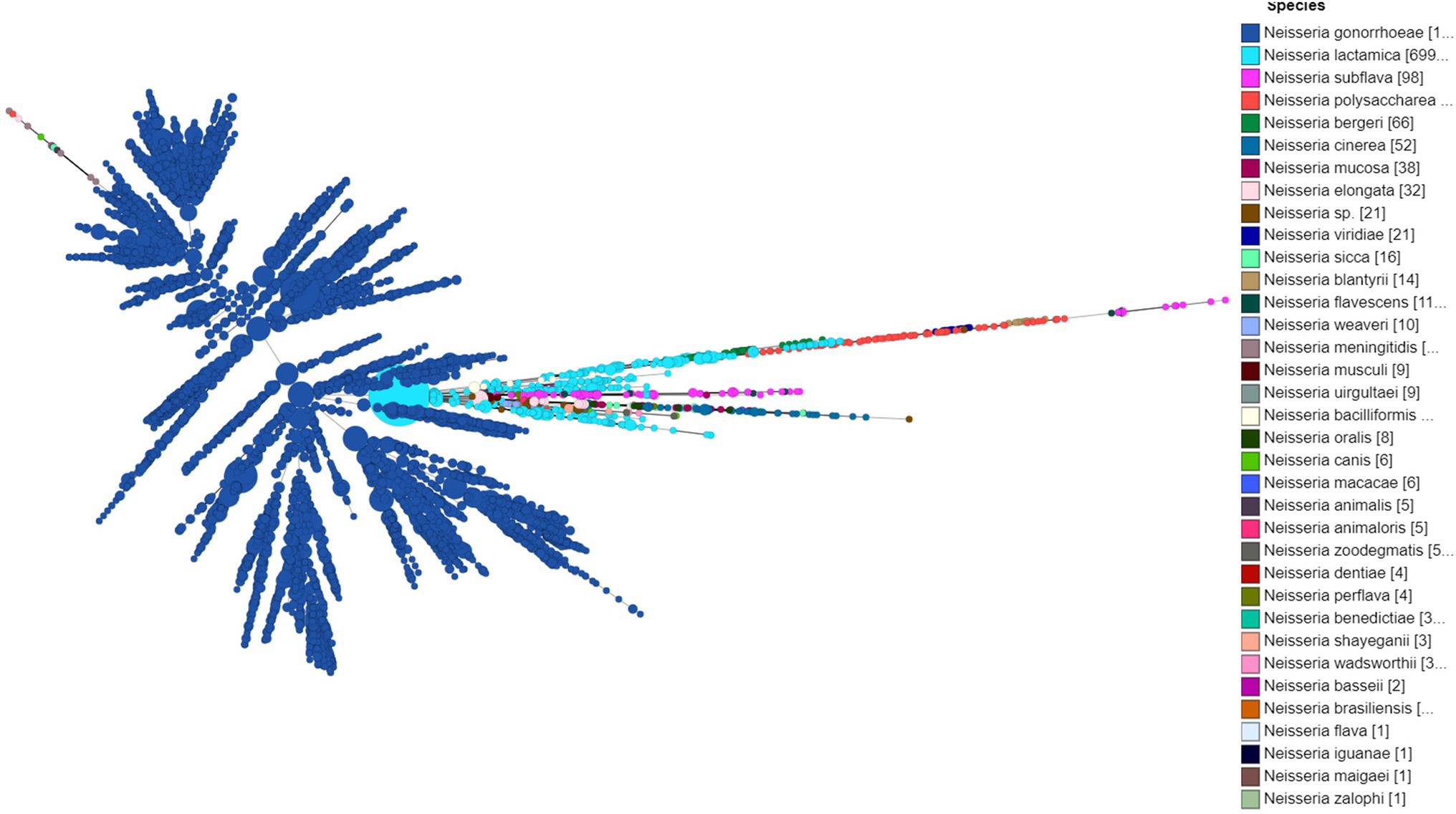
Figure 1. Minimum spanning tree comparing core-genome allelic profiles in association with the Neisseria species used in the study. Numbers in brackets refer to the number of isolates. Isolates are displayed as circles. The size of each circle indicates the number of isolates of this particular type.
Out of the 20,047 isolates, 9,796 (49.8%) samples were from 31 European countries, 3,399 (16.9%) from North America, 3,485 (17.3%) from Oceania, 1,750 from Asia (8.7%), 477 (2.37%) from Africa, and 162 (0.8%) from South America (Figure 2; Supplementary Table 2 in Data Sheet 1). The country of isolation was not available for 978 (4.8%) isolates.
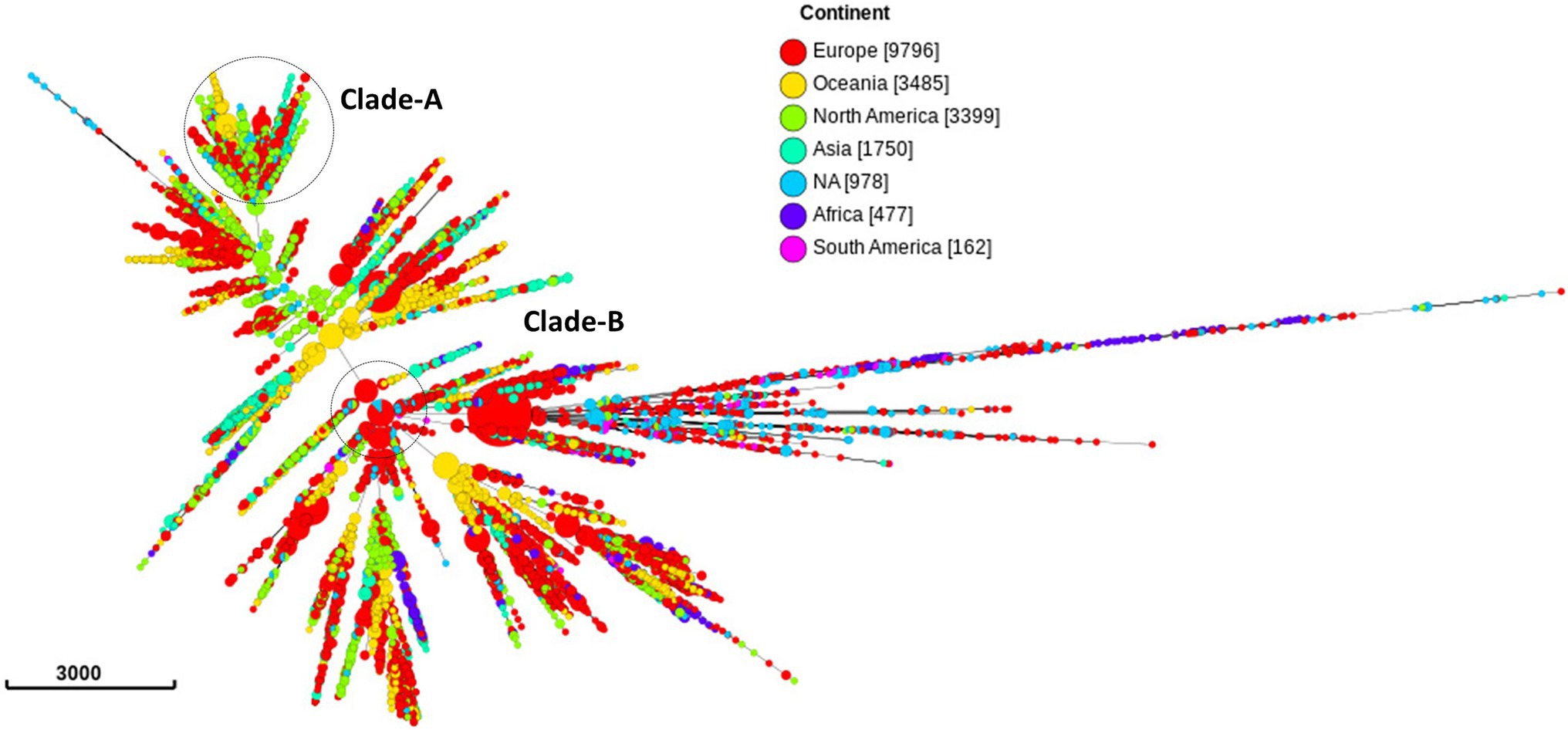
Figure 2. Minimum spanning tree comparing core-genome allelic profiles in association with continents. Numbers in brackets refer to the number of isolates. Isolates are displayed as circles. The size of each circle indicates the number of isolates of this particular type. Clade A, B of ST1901 are represented as dotted circles.
Antimicrobial Susceptibility of Neisseria Isolates
Among the 18,800 N. gonorrhoeae isolates, the CIP MIC was available for 12,942 (68.8%) isolates. Out of these isolates, 6,192 (47.8%) of the isolates were CIP resistant (R), 45 (0.34%) isolates were CIP intermediate (I), and 6,705 (51.8%) isolates were susceptible to CIP (Supplementary Table 2 in Table 2). Out of the 1,247 Neisseria commensal species, CIP MIC was available for only 14 isolates. Seven of these isolates had MICs >0.06 mg/L.
Characterization of Resistance-Associated Mutations in QRDR Regions of gyrA, gyrB, parC, and parE Genes in Neisseria gonorrhoeae
Strong positive correlations between CIP MICs and substitutions in GyrA at amino acid positions 91 (S91F, 45.4%) and 95 (D95A, 13.82%; D95G, 28.2%; D95N, 3.04%) and ParC at 85 (G85C, 0.5%; G85D, 0.2%), 86 (D86N, 6.8%), 87 (S87I, 1%; S87N, 3.4%; S87R, 25.7%), 88 (S88P, 2.1%), and 91 (E91G, 6.3%; E91K, 0.7%; E91Q, 0.9%) were observed (Supplementary Table 3 in Data Sheet 1).
In vitro generated zoliflodacin-resistant mutants containing substitutions in GyrB (D429, K450, and S467N) have been found to be associated with increased MICs of zoliflodacin (Alm et al., 2015; Foerster et al., 2015, 2019). In the current study, substitutions in GyrB-D429V were present in a single Japanese isolate (PubMLST ID-31741; Pathogenwatch ID-ERR363582; Adamson et al., 2021) along with GyrA-S91F and ParC-S87R substitutions. Fifty isolates had the GyrB-S467N substitution [allele 293 (n = 12) and allele-446 (n = 38); Pathogenwatch (n = 24) and PubMLST (n = 26)] along with mutations in gyrA and or parC. These isolates were from China (n = 1, ST7367), Japan (n = 2, ST7363), Norway [n = 11; ST1925 (n = 10), ST7363 (n = 1)], and Vietnam [n = 36; ST7373 (n = 34), ST1925 (n = 2)]. None of the isolates had the GyrB-K450 substitution.
Except 27 isolates, all high-level fluoroquinolone (CIP MICs ≥16 mg/L) resistant gonococcal isolates, n = 2,384 (12.7%) had known substitutions at GyrA-91 (S91F), GyrA-92 (A92P), GyrA-95 (D95A/G/N), and ParC-85 (G85C), 86 (D86N), 87 (S87I/N/R/Y), 88 (S88P), and 91 (E91G/K) positions (Figures 3–5; Supplementary Table 2 in Table 2). Twenty-seven isolates (1.1%; GM MIC = 22.3 mg/L), 14 (0.5%; GM MIC = 27.5 mg/L), 2,040 (85%; GM MIC = 21.1 mg/L), 273 (11.4%), and 3 (0.1%) had one, two, three, four, and five RAMs, respectively (Supplementary Table 1 in Table 1). A total of 3,808 (20.2%) N. gonorrhoeae isolates had low level fluoroquinolone resistance (CIP MICs >0.06 and <16 mg/L). Out of these isolates, 79 isolates (2%, GM MIC = 1.53 mg/L) had no known RAMs (Figures 3–5; Supplementary Table 2 in Table 2). Eighty-nine (2.3%; GM MIC = 0.64 mg/L), 302 (7.9%; GM MIC = 27.5 mg/L), 3,017 (79.2%; GM MIC = 21.18 mg/L), 326 (8.5%), and 5 (0.1%) isolates had one, two, three, four, and five RAMs, respectively (Supplementary Table 1 in Table 1). Out of 6,705 (35.7%) susceptible gonococcal isolates, 6,620 (98.7%) had no GyrA or ParC substitutions, whereas 46, 9, 33, and 2 isolates had one, two, three, and four RAMs, respectively (Supplementary Table 1 in Table 1). Seven Neisseria commensals (0.08%) with ciprofloxacin MICs >0.06 mg/L, had GyrA-S91I/T/V or GyrA-D95N substitutions.
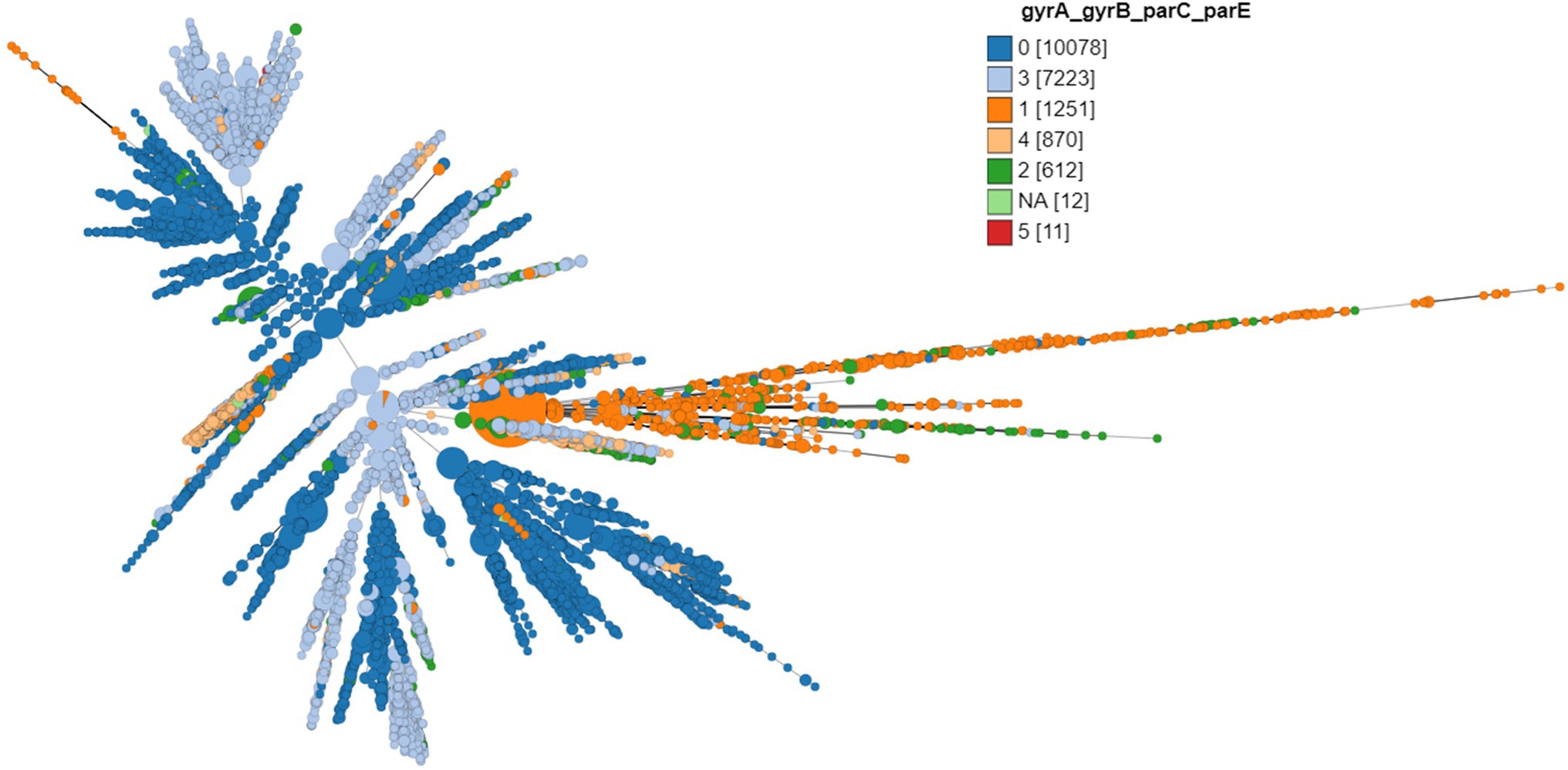
Figure 3. Minimum spanning tree comparing core-genome allelic profiles with the number of substitutions in GyrA, GyrB, ParC, and ParE. Numbers in brackets refer to the number of isolates. Isolates are displayed as circles. The size of each circle indicates the number of isolates of this particular type.
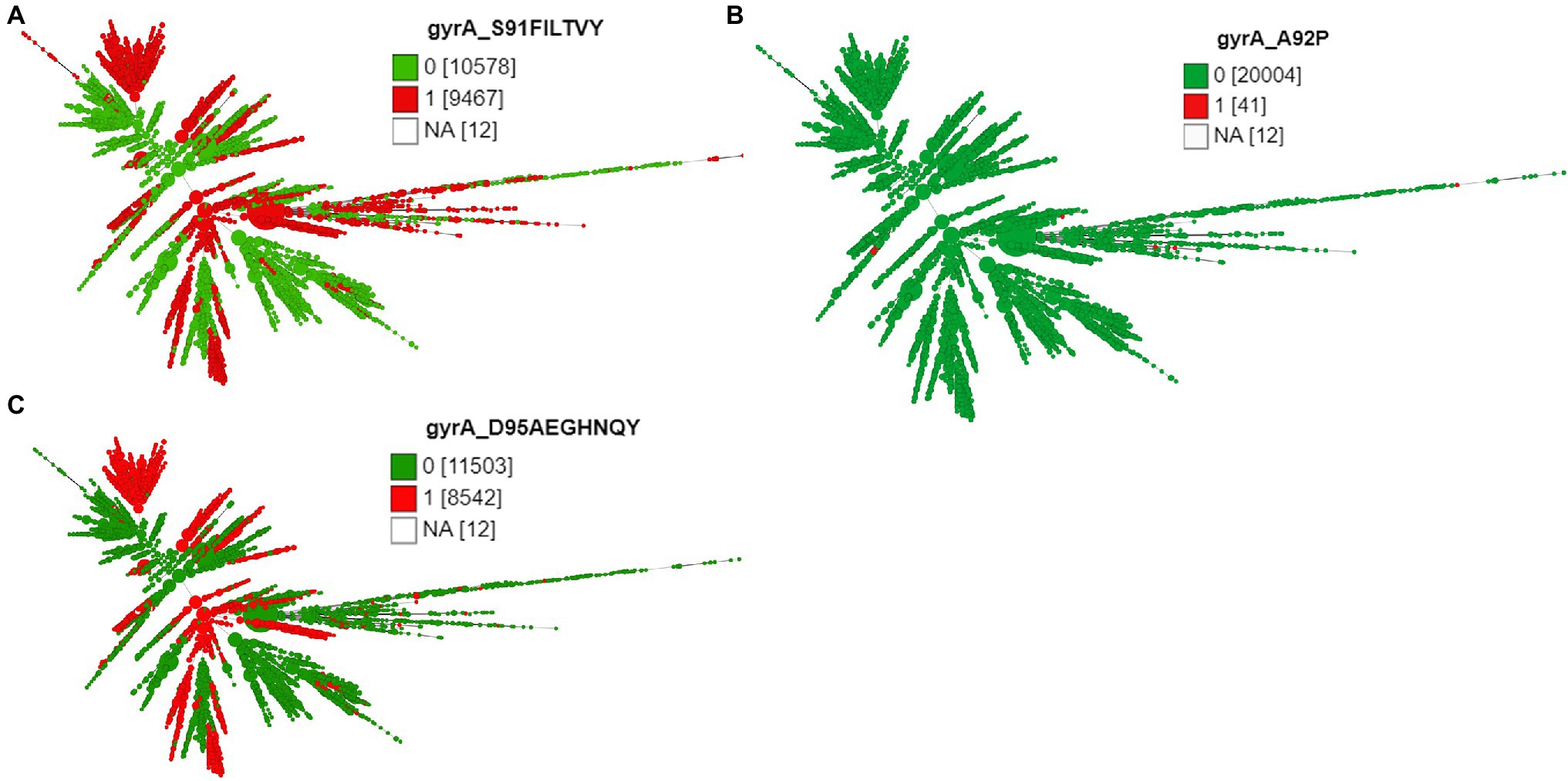
Figure 4. Minimum spanning tree comparing core-genome allelic profiles with GyrA substitutions at amino acid (A) position 91, (B) position 92, and (C) position 95. Numbers in brackets refer to the number of isolates. 1 and 0 denotes the presence and absence of RAMs, respectively. Isolates are displayed as circles. The size of each circle indicates the number of isolates of this particular type.
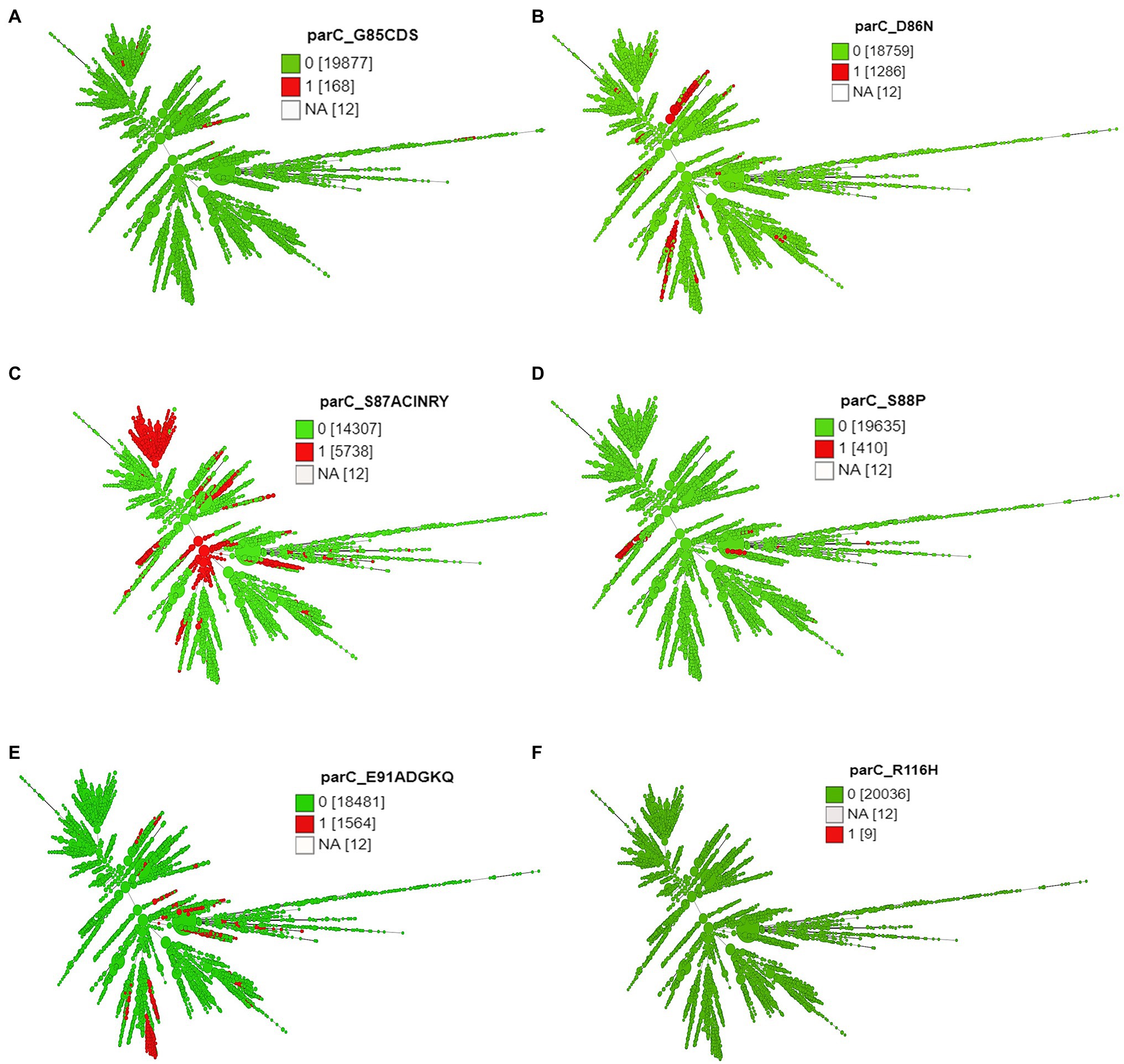
Figure 5. Minimum spanning tree comparing core-genome allelic profiles with ParC substitutions at amino acid (A) position 85, (B) position 86, (C) position 87, (D) position 88, (E) position 91, and (F) position 116. Numbers in brackets refer to the number of isolates. 1 and 0 denotes the presence and absence of RAMs, respectively. Isolates are displayed as circles. The size of each circle indicates the number of isolates of this particular type.
The different combinations of RAMs and the number of isolates are presented in Figures 3–5 and Supplementary Figure 1.
Genetic Structure and Geographical Dispersal of Fluoroquinolone Resistance in Neisseria gonorrhoeae
A total of 1,323 loci were defined as core to the Neisseria genome in this dataset. Allelic designations were defined for approximately 95% of the core-genome. A total of 583 different sequence types (STs) characterized the available population. The most frequent ST was ST1901 (n = 2,677) followed by ST9363 (n = 1,627), ST7363 (n = 1,054), ST1579 (n = 743), and ST7359 (n = 653; Figure 6; Supplementary Table 2 in Table 2). MLST types were not available for 2,424 isolates, and 93 isolates were classified as new ST types. Of the 2,384 CIP HLR isolates detected, half of the isolates [1,201 (50.4%)] belonged to ST1901, followed by ST7363 (11.49%) and ST1579 (2.5%). ST types were not available for 231 (9.6%) isolates. The total number of HLR, I, R, and S isolates and their prevalence in each continent is listed in Supplementary Table 4 in Data Sheet 1.
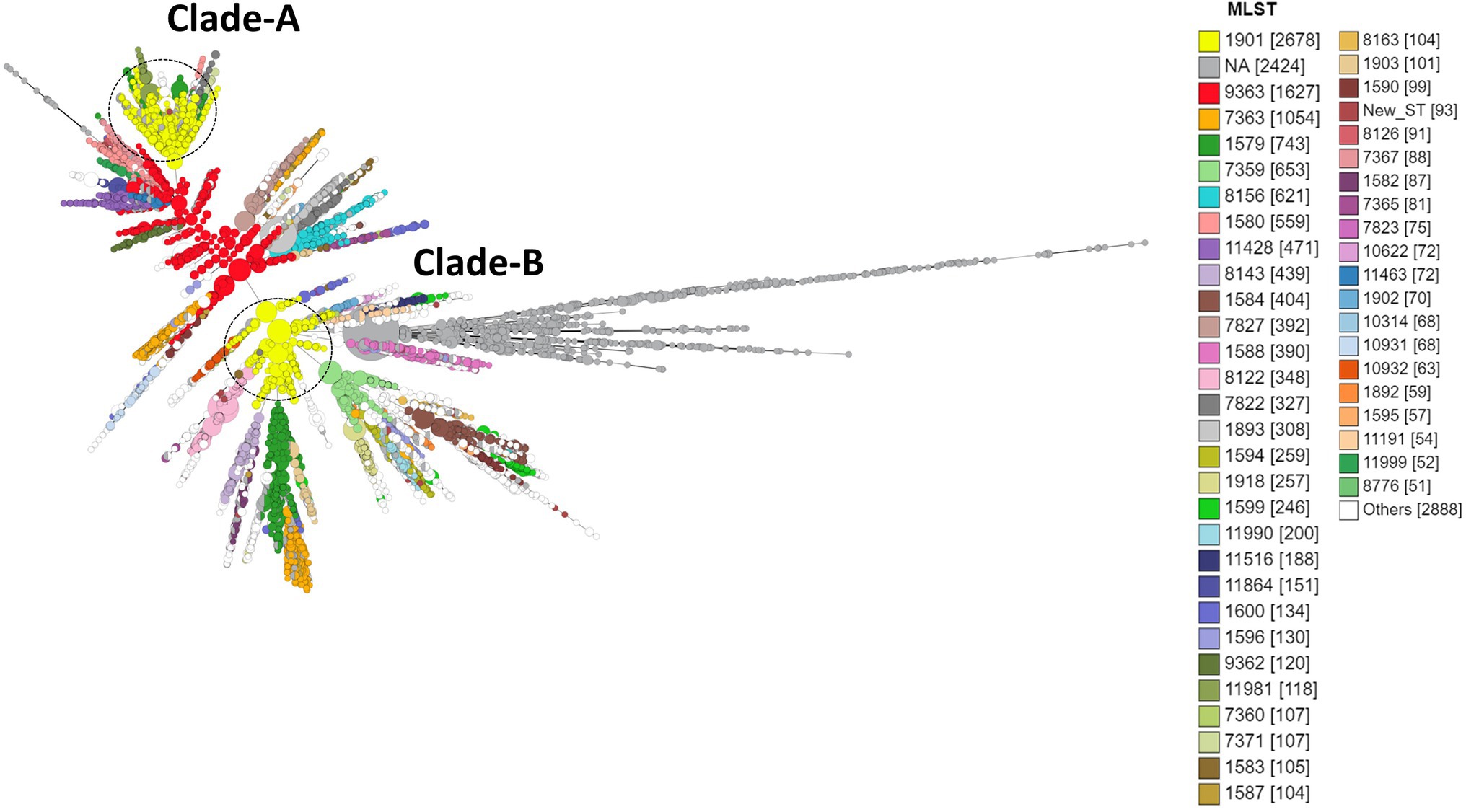
Figure 6. Minimum spanning tree comparing core-genome allelic profiles with MLST resulting in isolates with similar allelic profiles forming clusters. Isolates are displayed as circles. The size of each circle indicates the number of isolates of this particular type. Numbers in brackets refer to the number of isolates. Clade A, B of ST1901 are represented as dotted circles.
The three most prevalent ST types (>1,000 isolates) were further characterized. ST1901 was split into two clades, referred to as Clade A and Clade B (Figure 6). Isolates of clade A were predominately from North America, while clade B isolates were predominately from Europe (Figure 2). Both clades had substitutions at GyrA-S91F (n = 2,616, Figure 4A; Supplementary Table 2 in Table 2), GyrA-D95G (n = 2,585, Figure 4C), and ParC-S87R (n = 2,663, Figure 5C). Around 0.4, 20.5, and 44% of isolates belonging to ST1901 were S, R, and HLR, respectively. About 34% of isolates had no MIC information (Table 1).
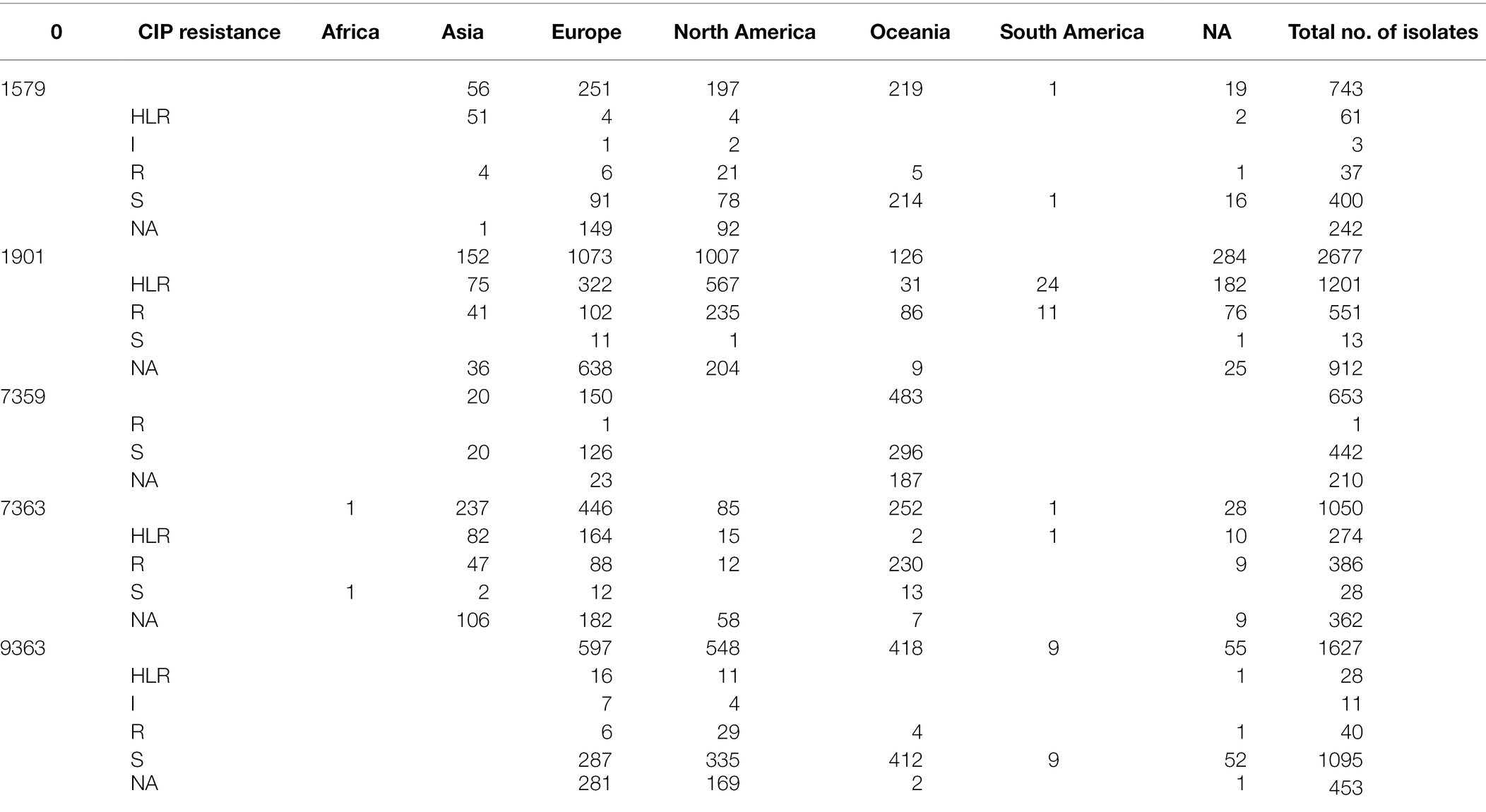
Table 1. Distribution of 5 predominant ST-types of N. gonorrhoeae isolates, country of isolation and their known resistance-associated mutations.
ST9363 isolates were distributed across Europe (36.69%), North America (33.68%), and Oceania (25.69%; Table 1; Figure 2). The first HLR isolate was found in North America in 2011. About 67.3, 0.6, 2.4, and 1.7% of isolates were S, I, R, and HLR, respectively. About 27.8% of isolates had no MIC information. The resistant isolates had one or more of the following substitutions: GyrA-S91F (n = 78, Figure 4A), GyrA-D95A/G (n = 76/2, Figure 4C), ParC-D86N (n = 71, Figure 5B), and the ParC-S87R (n = 10, Figure 5C).
At least three clades for ST7363 were observed (Figure 6). Isolates that belonged to ST7359 were mostly from Oceania (Figure 2). Out of the 653 isolates, 442 were susceptible, and MIC was not available for 210 isolates. Only one isolate from Europe (Ireland) was CIP resistant (MIC-2 mg/L) with no known RAMs. Further details pertaining to the distribution of MLST, CIP MICs, and the prevalence in each continent are provided in Table 1 and Supplementary Table 4 in Data Sheet 1.
The Time of Emergence of Fluoroquinolone Resistance-Associated Mutations in Neisseria gonorrhoeae
Substitution at position 91 (S91T) of GyrA was first observed in one of two of the oldest isolates in the collection obtained in 1928. The MICs were not available for the pre-antibiotic and the golden era isolates. GyrA S91F, which leads to high-level resistance to CIP, was first observed in 1992 (post-modern era). Four isolates were available from the same year. Two isolates were from the Philippines and had GyrA-S91F along with additional substitutions, and two isolates had no RAMs. Out of the two isolates with RAMs, one isolate belonged to ST1901 (D95G; ParC-S87R), and the other to ST7367 (D95G). Substitutions at position 95 of GyrA (D95G/N) and positions 86, 87, 88, and 91 of ParC were first observed in 1996. A substitution in position 85 of ParC was first observed in 1998 (Figure 7; Supplementary Table 6 in Data Sheet 1).
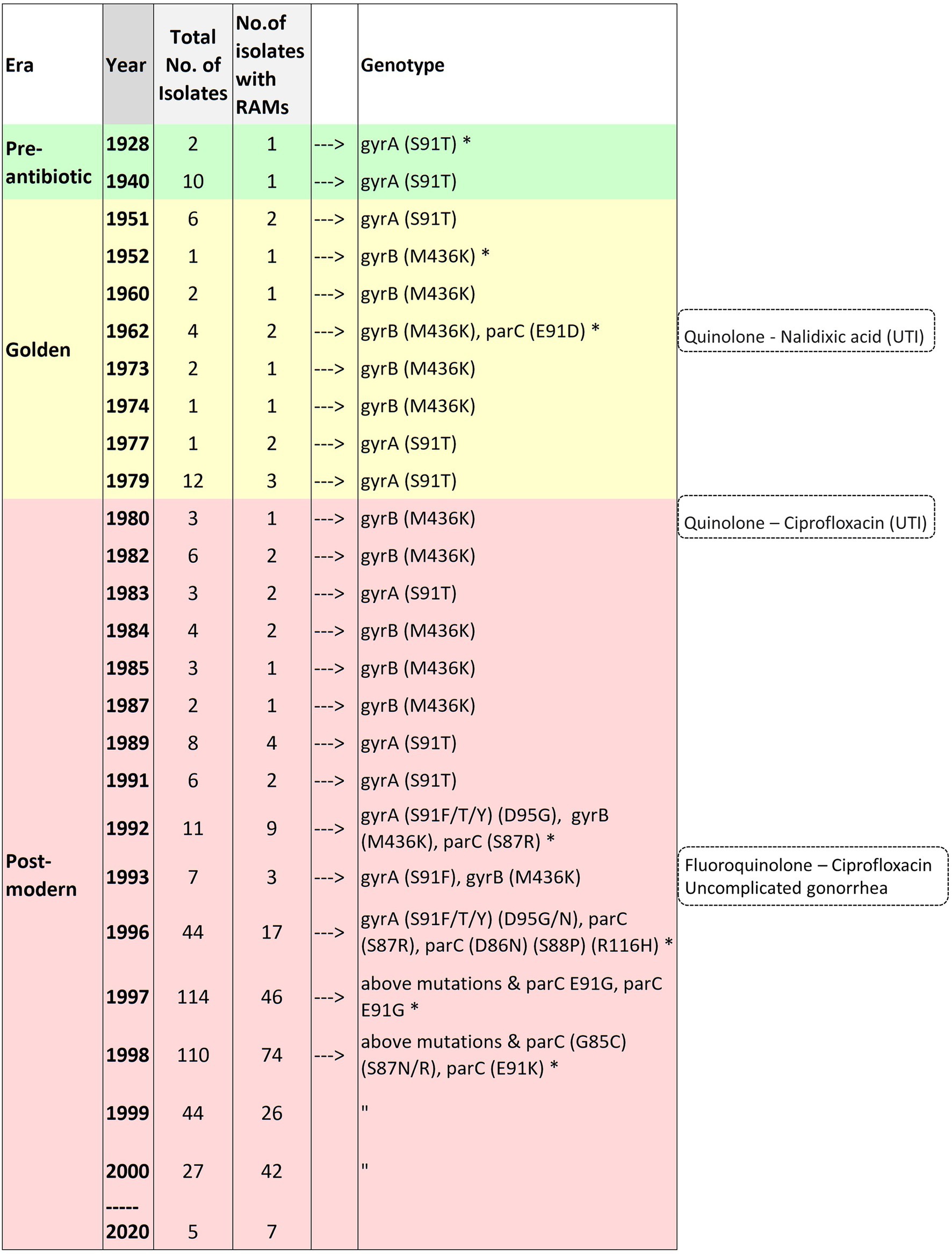
Figure 7. Time of emergence of ciprofloxacin resistance-associated mutations. Asterisks denote the first occurrence of the mutation.
Prevalence of HGT in QRDR Region of gyrA, gyrB, parC, and parE
The lengths of gyrA, gyrB, parC, and parE of the reference isolate FA1090 were 2,751, 2,391, 2,307, and 1,986 bp, respectively. The length of the alleles ranged between 2,454–3,054 bp for gyrA, 2,388–2,644 bp for gyrB, 2088–2,538 bp for parC, and 1,629–2,151 bp for parC. Multiple alignment files of each gene were trimmed to the gene length of the FA1090 isolate and were used in the recombination analysis.
About 688, 631, 898, and 595 alleles were found for gyrA, gyrB, parC, and parE genes, respectively (Figures 8A–D; Supplementary Tables 6A–D in Data Sheet 1). Putative HGT events were predicted in all the genes (Figures 9A–D). Unique recombination events were supported by at least two out of seven detection methods. A total of 63, 8, 80, and 67 recombination events were identified for gyrA, gyrB, parC, and parE, respectively. For the sequences that included only the RAMs in gyrA and parC, a total of 58 recombination events were identified for gyrA (Supplementary Table 7E in Data Sheet 1) and 16 for parC gene (Supplementary Table 7F in Data Sheet 1). Each recombination event was checked manually. Examples of recombinant events with known RAMs in QRDR regions of gyrA and parC and in QRDR regions of gyrB and parE are presented below.
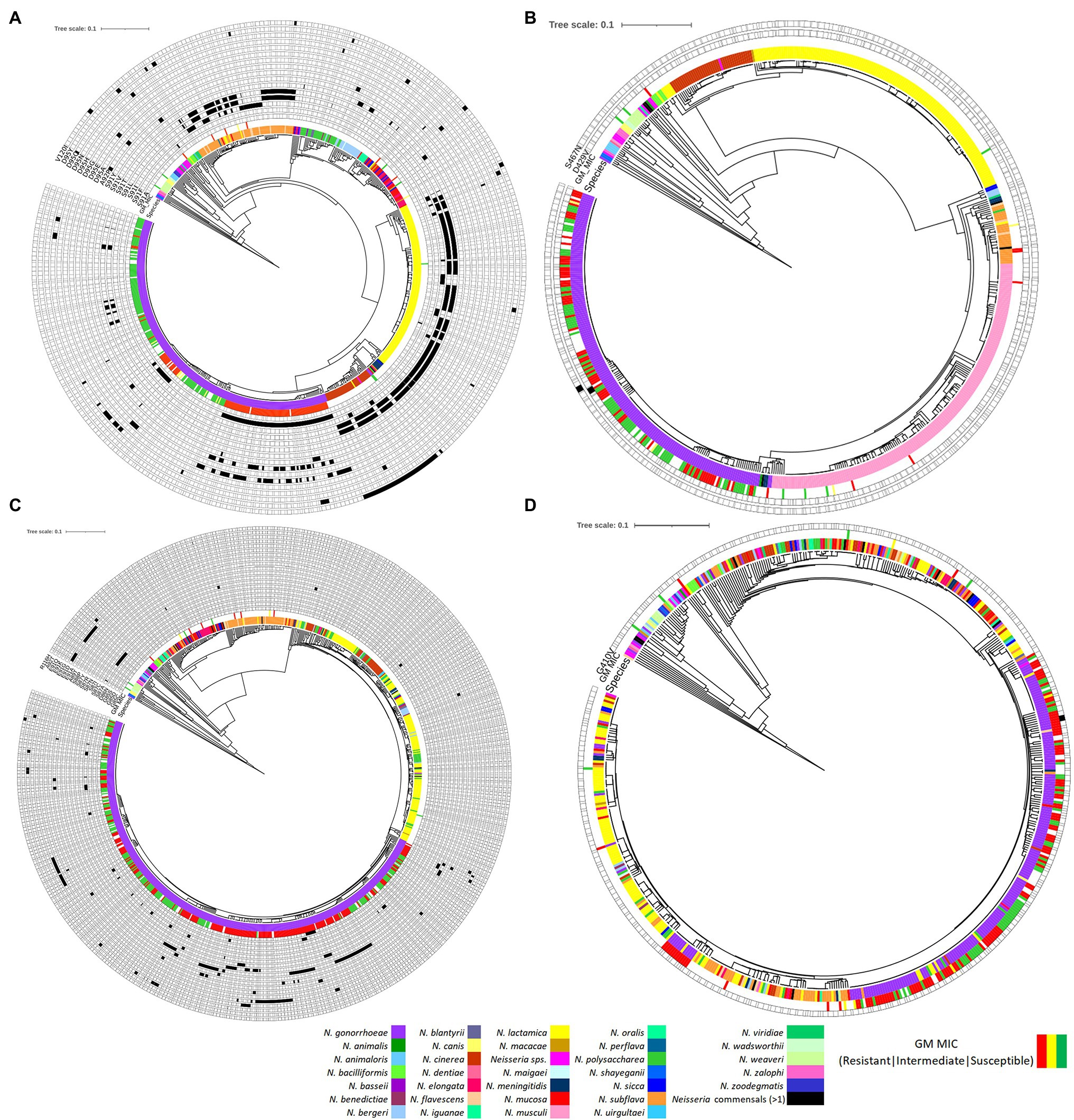
Figure 8. Phylogenetic trees of (A) gyrA, (B) gyrB, (C) parC, and (D) parE based on Neisseria spp. Figure was generated by iTOL using cgMLST profile data determined by the chewBACCA software. Purple node denotes N. gonorrhoeae, and the rest denotes Neisseria commensals. Filled and outlined black rectangle denotes the presence and absence of non-synonymous SNPs, respectively. The geometric mean (GM) ciprofloxacin MIC SIR profiles are denoted as green, yellow, and red, respectively.
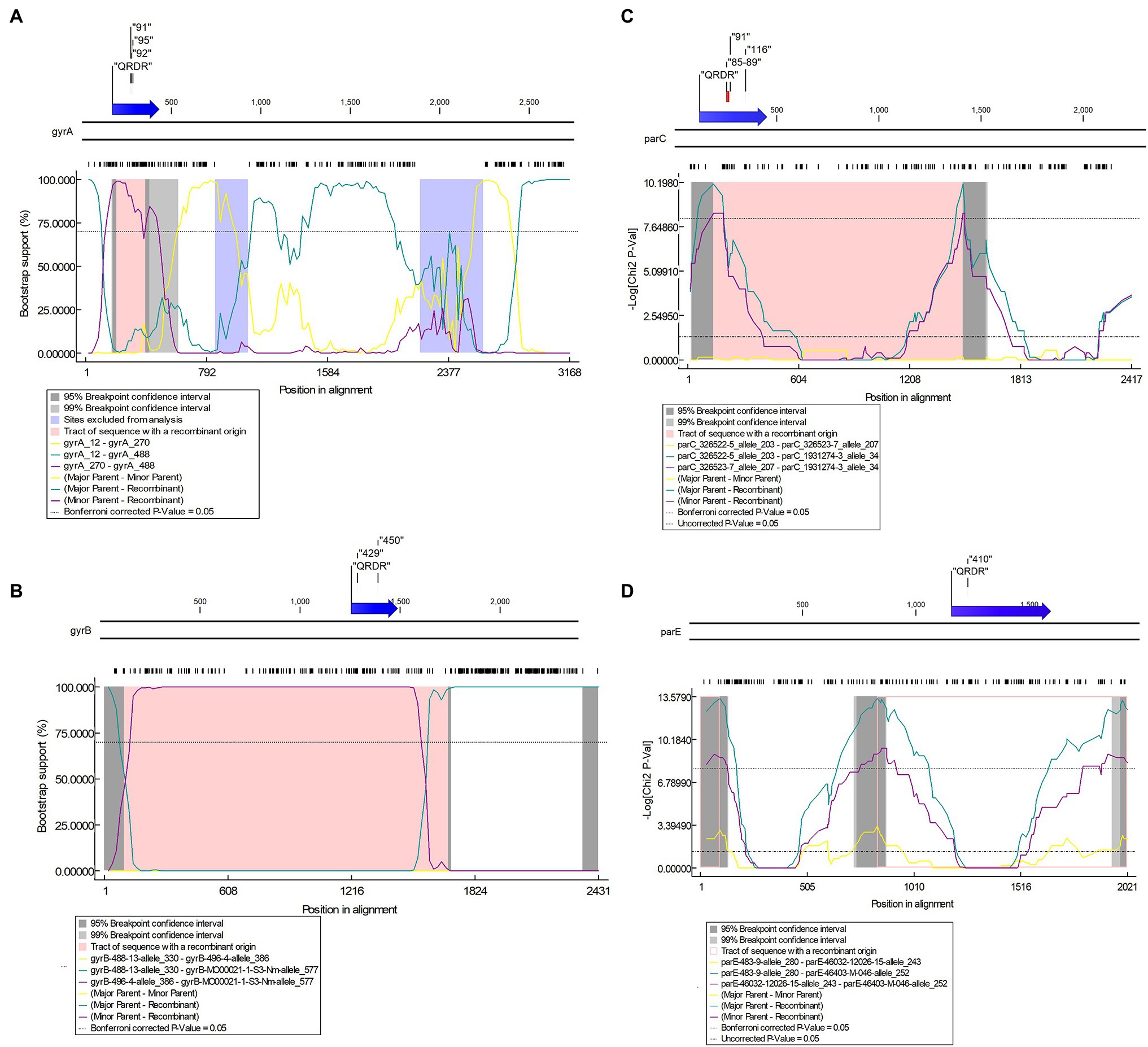
Figure 9. Potential putative recombination events between Neisseria strains. (A) Recombination event 3 in gyrA between N. gonorrhoeae (recipient) and N. lactamica (donor); (B) Recombination event 3 in gyrB between N. gonorrhoeae/N. meningitidis (recipient) and N. macacae (donor); (C) Recombination event 21 in parC between Neisseria sp. (recipient) and N. zoodegmatis (donor); (D) Recombination event 19 in parE between N. gonorrhoeae (recipient) and N. mucosa (donor). Top—The functional map of N. gonorrhoeae reference strain FA1090 with QRDR and known resistance-associated mutations (RAMs) are shown within inverted commas. Bottom—Bootscan (A,B) and maxchi (C,D) plots generated using RDP4 software are depicted.
gyrA
For gyrA, the QRDR region is present between 169 and 432 bp. Putative recombination event 32 in the QRDR region with N. gonorrhoeae as a recombinant was identified. The event was supported by five methods (RDP, GENECONV, Bootscan, Maxchi, and Chimaera). Neisseria gonorrhoeae (allele—488; n = 2, GM MIC-0.02 mg/L, ST11702, Year-1940) was the recombinant, and evidence of the same recombinant event was present in four other alleles (allele-93, n = 3, ST1175, Year-2010–2012; allele-491; n = 2, ST1688, Year-1928; allele-496, n = 2, ST11703, Year-1951; and allele-508, n = 2, ST11708, Year-1951). The corresponding minor parent was N. lactamica (allele-270; n = 1; recombinant region—197–396 nt), and major parent was N. cinerea (allele-12; n = 1; recombinant region—1–199 and 397–3,168 nt; Figure 9A; Supplementary Table 7A in Data Sheet 1; Supplementary Figure 2). The minor parent N. lactamica and the recombinants had the S91T substitution (Supplementary Table 6A in Data Sheet 1).
Recombination events were also found in the commensal Neisseria. In event 5, for example, N. polysaccharea (allele—361; n = 1) was the recombinant, and the sequences with the same recombinant event were present in 10 isolates belonging to five alleles; N. bergeri (alleles- 28, 214, 215, and 223; n = 9) and N. oralis (allele-29, n = 1). The corresponding minor parent was N. cinerea (allele-61; n = 1; recombinant region—1–172 and 2,946–3,168 nt) and major parent was N. elongata (allele-637; n = 2; GM MIC-0.25 mg/L) recombinant region—173–2,945 nt (Supplementary Table 7A in Data Sheet 1). The major parent N. elongata (allele-637), the recombinant N. polysaccharea (allele—361), and isolates with the same recombinant (n = 10) had the S91V substitution (Supplementary Table 6A in Data Sheet 1). The event was supported by all seven methods.
gyrB
The QRDR region of gyrB is between 1,255 and 1,488 bp, which is also the zoliflodacin resistance-conferring region (Figure 9B). Recombination event 3 was supported by all seven methods (RDP, GENECONV, Bootscan, Maxchi, Chimera, SiSscan, and 3Seq; Figure 9B) and N. meningitidis (n = 1; allele-577) was the recombinant. The same recombination event was present in five alleles, including N. gonorrhoeae (n = 13, allele-472, 518, and 562) and N. meningitidis (n = 2; allele-544 and 567). The minor and major parents were N. macacae (allele-386; n = 1; region—96–1,690 nt) and N. mucosa (allele-330; n = 1; region—1–95 and 1,691–2,431 nt), respectively (Figure 9B; Supplementary Tables 5, 7B in Data Sheet 1). The recipient and the donor had no known RAMs (Supplementary Table 6B in Data Sheet 1).
parC
The QRDR region lies between 121 and 452 bp. Recombination event 21 took place in the QRDR region and involved the transmission of known RAMs between commensal Neisseria. The recombinant was Neisseria sp. (allele—34; n = 2). Neisseria animaloris (allele—203; n = 1; recombinant region—1–141 and 1,499–2,417 nt) and N. zoodegmatis. (allele—207; n = 1; recombinant region—142–1,498 nt) were the major and minor parents, respectively (Figure 9C). The same event was seen in another allele (allele-35, n = 2). The minor parent and the recombinant had the S87A and E91A RAMs (Supplementary Table 6C in Data Sheet 1). The event was supported by three methods (Maxchi, Chimera, and 3Seq).
parE
The QRDR region lies between ~1,140 and 1,600 bp. Recombinant event 19 for parE was supported by three methods (Maxchi, Chimera, and 3Seq). The recombinant was N. gonorrhoeae (allele-252; n = 1). The corresponding major parent was N. cinerea (allele 280, n = 1; recombinant region—92–837 nt), and the unknown parent was inferred from N. mucosa (allele-243; n = 3; recombinant region—1–91 and 838–2,021 nt; Figure 9D). The recipient and the donor had no known RAMs (Supplementary Table 6D in Data Sheet 1).
Discussion
The large and diverse collection of Neisseria isolates used in this study enabled us to investigate the temporal and spatial patterning of fluoroquinolone RAMs as well as the evidence of HGT in gyrA, gyrB, parC, and parE genes in N. gonorrhoeae and related Neisseria spp.
In accordance with previous studies, we found that the global spread of ciprofloxacin-resistant N. gonorrhoeae is associated with three STs 1,901, 9,363, and 7,363 being responsible for 65% of all high-level resistance to ciprofloxacin (Osnes et al., 2020; Reimche et al., 2021). ST1901, with substitutions in GyrA-S91F, D95G, and ParC-S87R, was by itself responsible for over half of all high-level resistance. Osnes et al. have recently demonstrated that this ST emerged in East Asia before spreading globally between 1987 and 1996 (Osnes et al., 2021). Its emergence and spread were facilitated by the increasing use of fluoroquinolones as a treatment for gonorrhea at this time (Osnes et al., 2020). The widespread use of fluoroquinolones in the general population may also have played a role (Kenyon et al., 2018a). Notably, no global distribution of ST1901 clones with substitutions in GyrA-S91Y and ParC-R116H during the same period from East Asia was observed.
The GyrA-S91T substitution was first reported in 1928 in N. gonorrhoeae and cannot be plausibly linked with antimicrobial pressure. GyrA-S91T is not associated with reduced susceptibility to ciprofloxacin (Golparian et al., 2020). Notably, two isolates from 1928 had different amino acid substitutions at position 91. One isolate belonging to ST11688 had the threonine (T) amino acid at position 91, whereas the other isolate from ST11680 had the serine (S) at position 91. Interestingly, the meningococci have the threonine (T) substitution at position 91 (Hong et al., 2013). Resolving the parental isolate of gonococci could resolve the ST types of gonococci, especially the two clades of ST1901. Further analysis pertaining to the above was not carried out as this was beyond the scope of the present study. The next mutation to occur in the four genes under consideration was a substitution in ParC-E91D in 1962 in N. canis. This occurred at the time when the first-generation quinolone, nalidixic acid, was introduced (Lesher et al., 1962).
Our analyses suggest that 199 bp of the QRDR region in gyrA with GyrA-S91T substitution was acquired from N. lactamica. Fourteen isolates of N. gonorrhoeae belonging to five alleles had obtained this section of the QRDR region, including the GyrA-S91T. All the isolates belonged to the pre-antibiotic/golden era except three isolates from allele-93 that belonged to the post-modern era with a GM MIC of 0.006 mg/L. Evidence of HGT in QRDR regions of Neisseria spp. and mutation harboring commensals with substitutions in known amino acid positions, GyrA-S91, ParC-S87, and ParC-E91 were identified. The recombinants with GyrA-S91V and ParC-S87A, ParC-E91A were N. polysaccharea and Neisseria sp., respectively. The mutation harboring commensal of GyrA-S91V in the recombinant N. polysaccharea (n = 1, MIC-NA) was N. elongata (n = 2, GM MIC—0.25 mg/L) and ParC-S87A, E91A in the recombinant Neisseria sp. (n = 2, MIC-NA) was N. zoodegmatis (n = 1, MIC-NA). Twenty-one Neisseria commensal isolates had the GyrA-S91V substitution, but only one isolate, N. elongata had the data for ciprofloxacin susceptibility (MIC 0.25 mg/L). It was therefore not possible to determine if the mutation is associated with reduced susceptibility to ciprofloxacin. The same implies to the substitutions in ParC.
The analysis of whole-genome sequences identified non-synonymous mutations in the GyrB-D429V and S467N, that is, where zoliflodacin first- or second-step resistance mutations have been previously selected in vitro (Alm et al., 2015; Foerster et al., 2015, 2019). Substitutions in GyrB-D429V was present in one isolate belonging to ST7363 (Adamson et al., 2021; Unemo et al., 2021). GyrB-S467N substitution was present in 50 isolates and belonged to ST7367 (n = 1), ST7363 (n = 37), and ST1925 (n = 12). Evidence of HGT in the QRDR region of gyrB was identified, and the commensal donor was N. macacae.
Caveats of our study include that only a few early isolates were available, and very few isolates from some continents, such as Africa and South America, were available. The study included only nine N. meningitidis isolates. The probability of detecting HGT events could have been maximized using a greater number of N. meningitidis. Moreover, MIC values were not available for many commensal isolates. Some of the Pathogenwatch MICs are estimated based on genotype using 485.toml.5 These estimated MICs may differ slightly from MICs obtained via phenotypic testing. We were also not able to identify the commensal donors for parC RAMs. This could be due to the paucity of commensal isolates with known RAMs. We did not include other genetic mutations, such as the norM promoter mutation, which can lead to increased fluoroquinolone resistance (Rouquette-Loughlin et al., 2003). Finally, the HGT events were not confirmed experimentally.
Studies have shown that chromosomal gyrA and parC mutations from commensal Neisseria have been responsible for most fluoroquinolone resistance in meningococci (Chen et al., 2020; Shen and Chen, 2020). With the current dataset, we showed evidence of HGT in genes gyrA/B and parC/E and found that HGT played a lesser role in acquiring fluoroquinolone resistance mutations in gonococci. Nevertheless, our findings add to the growing evidence base that commensal Neisseria species act as a reservoir of antimicrobial resistance that can be taken up by the pathogenic Neisseria (Bowler et al., 1994; Shafer, 2018; Yahara et al., 2018; Fiore et al., 2020; Goytia et al., 2021; Manoharan-Basil et al., 2021). The study highlights the significant role that HGT can play in transferring QRDR regions to N. gonorrhoeae. Zoliflodacin is a promising new drug to treat N. gonorrhoeae (Foerster et al., 2019; Bradford et al., 2020; Adamson et al., 2021; Kenyon et al., 2021). Our finding that zoliflodacin resistance-conferring region of gyrB in N. gonorrhoeae can be taken up from N. macacae has important consequences. Extensive use of zoliflodacin could lead to resistance in commensal Neisseria, which could then be transferred to N. gonorrhoeae via transformation. This provides an additional rationale to conduct surveillance of antimicrobial susceptibility in Neisseria commensals to prevent the emergence of AMR in the pathogenic Neisseria (Kenyon et al., 2018b; Dong et al., 2020; Fiore et al., 2020).
Data Availability Statement
The original contributions presented in the study are included in the article/Supplementary Material, further inquiries can be directed to the corresponding author.
Author Contributions
SM-B and CK conceptualized the study, interpreted the data, performed the statistical analysis, and wrote the first draft. SM-B was responsible for data collection and bioinformatic analysis. All authors read the final draft and approved the submitted version.
Funding
The study was funded by SOFI 2021 grant—“PReventing the Emergence of untreatable STIs via radical Prevention” (PRESTIP).
Conflict of Interest
The authors declare that the research was conducted in the absence of any commercial or financial relationships that could be construed as a potential conflict of interest.
Publisher’s Note
All claims expressed in this article are solely those of the authors and do not necessarily represent those of their affiliated organizations, or those of the publisher, the editors and the reviewers. Any product that may be evaluated in this article, or claim that may be made by its manufacturer, is not guaranteed or endorsed by the publisher.
Supplementary Material
The Supplementary Material for this article can be found online at: https://www.frontiersin.org/articles/10.3389/fmicb.2022.793612/full#supplementary-material
Supplementary Presentation 2 | Alignment of GyrA alleles-1 (reference, FA1090), 3, 496, 488, 491, 508 from N. gonorrhoeae and allele-270 from N. lactamica (donor). The quinolone resistance determining region (QRDR), the 199 bp of the recombinant region and the resistance associated mutations (RAM) are depicted in red, green and blue arrows, respectively. Amino acid substitutions are indicated as grey shading.
Footnotes
1. ^https://www.ncbi.nlm.nih.gov/genbank/
2. ^https://pathogen.watch/ngonorrhoeae
3. ^http://www.eucast.org/clinical_breakpoints/
5. ^https://gitlab.com/cgps/pathogenwatch/amr-libraries/blob/0.0.17/485.toml
References
Adamson, P. C., Lin, E. Y., Ha, S.-M., and Klausner, J. D. (2021). Using a public database of Neisseria gonorrhoeae genomes to detect mutations associated with zoliflodacin resistance. J. Antimicrob. Chemother. 76, 2847–2849. doi: 10.1093/jac/dkab262
Alcalá, B., Arreaza, L., Salcedo, C., Antolín, I., Borrell, N., Cacho, J., et al. (2003). Molecular characterization of ciprofloxacin resistance of gonococcal strains in Spain. Sex. Transm. Dis. 30, 395–398. doi: 10.1097/00007435-200305000-00004
Allan-Blitz, L.-T., Humphries, R. M., Hemarajata, P., Bhatti, A., Pandori, M. W., Siedner, M. J., et al. (2017). Implementation of a rapid genotypic assay to promote targeted ciprofloxacin therapy of Neisseria gonorrhoeae in a large health system. Clin. Infect. Dis. 64, 1268–1270. doi: 10.1093/cid/ciw864
Alm, R. A., Lahiri, S. D., Kutschke, A., Otterson, L. G., McLaughlin, R. E., Whiteaker, J. D., et al. (2015). Characterization of the novel DNA gyrase inhibitor AZD0914: low resistance potential and lack of cross-resistance in Neisseria gonorrhoeae. Antimicrob. Agents Chemother. 59, 1478–1486. doi: 10.1128/AAC.04456-14
Argimón, S., Abudahab, K., Goater, R. J. E., Fedosejev, A., Bhai, J., Glasner, C., et al. (2016). Microreact: visualizing and sharing data for genomic epidemiology and phylogeography. Microb. Genom. 2:e000093. doi: 10.1099/mgen.0.000093
Belland, R. J., Morrison, S. G., Ison, C., and Huang, W. M. (1994). Neisseria gonorrhoeae acquires mutations in analogous regions of gyrA and parC in fluoroquinolone-resistant isolates. Mol. Microbiol. 14, 371–380. doi: 10.1111/j.1365-2958.1994.tb01297.x
Blair, J. M. A., Webber, M. A., Baylay, A. J., Ogbolu, D. O., and Piddock, L. J. V. (2015). Molecular mechanisms of antibiotic resistance. Nat. Rev. Microbiol. 13, 42–51. doi: 10.1038/nrmicro3380
Bodoev, I. N., and Il’ina, E. N. (2015). Molecular mechanisms of formation of drug resistance in Neisseria gonorrhoeae: history and prospects. Mol. Genet. Microbiol. Virol. 30, 132–140. doi: 10.3103/S0891416815030027
Bowler, L. D., Zhang, Q. Y., Riou, J. Y., and Spratt, B. G. (1994). Interspecies recombination between the penA genes of Neisseria meningitidis and commensal Neisseria species during the emergence of penicillin resistance in N. meningitidis: natural events and laboratory simulation. J. Bacteriol. 176, 333–337. doi: 10.1128/jb.176.2.333-337.1994
Bradford, P. A., Miller, A. A., O’Donnell, J., and Mueller, J. P. (2020). Zoliflodacin: an oral spiropyrimidinetrione antibiotic for the treatment of Neisseria gonorrheae, including multi-drug-resistant isolates. ACS Infect. Dis. 6, 1332–1345. doi: 10.1021/acsinfecdis.0c00021
Catlin, B. W. (1960). Interspecific transformation of Neisseria by culture slime containing deoxyribonucleate. Science 131, 608–610. doi: 10.1126/science.131.3400.608-a
CDC (1985). Tetracycline-resistant Neisseria gonorrhoeae—Georgia, Pennsylvania, New Hampshire. MMWR Morb. Mortal. Wkly Rep. 34, 563–564.
CDC (1993). CDC sexually transmitted diseases treatment guidelines. Centers for Disease Control and Prevention. MMWR Recomm. Rep. 42, 1–102.
Chen, M., Zhang, C., Zhang, X., and Chen, M. (2020). Meningococcal quinolone resistance originated from several commensal Neisseria species. Antimicrob. Agents Chemother. 64, e01494–e01419. doi: 10.1128/AAC.01494-19
Chesson, H. W., Kirkcaldy, R. D., Gift, T. L., Owusu-Edusei, K. Jr., and Weinstock, H. S. (2014). Ciprofloxacin resistance and gonorrhea incidence rates in 17 cities, United States, 1991-2006. Emerg. Infect. Dis. 20, 612–619. doi: 10.3201/eid2004.131288
Davis, R., Markham, A., and Balfour, J. A. (1996). Ciprofloxacin. an updated review of its pharmacology, therapeutic efficacy and tolerability. Drugs 51, 1019–1074. doi: 10.2165/00003495-199651060-00010
Davis, J. J., Wattam, A. R., Aziz, R. K., Brettin, T., Butler, R., Butler, R. M., et al. (2020). The PATRIC bioinformatics resource center: expanding data and analysis capabilities. Nucleic Acids Res. 48, D606–D612. doi: 10.1093/nar/gkz943
de Korne-Elenbaas, J., Bruisten, S. M., de Vries, H. J. C., and Van Dam, A. P. (2021). Emergence of a Neisseria gonorrhoeae clone with reduced cephalosporin susceptibility between 2014 and 2019 in Amsterdam, Netherlands, revealed by genomic population analysis. J. Antimicrob. Chemother. 76, 1759–1768. doi: 10.1093/jac/dkab082
Dong, H. V., Pham, L. Q., Nguyen, H. T., Nguyen, M. X. B., Nguyen, T. V., May, F., et al. (2020). Decreased cephalosporin susceptibility of oropharyngeal Neisseria species in antibiotic-using men who have sex with men in Hanoi. Clin. Infect. Dis. 70, 1169–1175. doi: 10.1093/cid/ciz365
Drlica, K., and Zhao, X. (1997). DNA gyrase, topoisomerase IV, and the 4-quinolones. Microbiol. Mol. Biol. Rev. 61, 377–392. doi: 10.1128/mmbr.61.3.377-392.1997
Elhadidy, M., Ali, M. M., El-Shibiny, A., Miller, W. G., Elkhatib, W. F., Botteldoorn, N., et al. (2020). Antimicrobial resistance patterns and molecular resistance markers of campylobacter jejuni isolates from human diarrheal cases. PLoS One 15:e0227833. doi: 10.1371/journal.pone.0227833
Fiore, M. A., Raisman, J. C., Wong, N. H., Hudson, A. O., and Wadsworth, C. B. (2020). Exploration of the neisseria resistome reveals resistance mechanisms in commensals that may be acquired by N. gonorrhoeae through horizontal gene transfer. Antibiotics 9, 1–12. doi: 10.3390/antibiotics9100656
Foerster, S., Drusano, G., Golparian, D., Neely, M., Piddock, L. J. V., Alirol, E., et al. (2019). In vitro antimicrobial combination testing of and evolution of resistance to the first-in-class spiropyrimidinetrione zoliflodacin combined with six therapeutically relevant antimicrobials for Neisseria gonorrhoeae. J. Antimicrob. Chemother. 74, 3521–3529. doi: 10.1093/jac/dkz376
Foerster, S., Golparian, D., Jacobsson, S., Hathaway, L. J., Low, N., Shafer, W. M., et al. (2015). Genetic resistance determinants, in vitro time-kill curve analysis and pharmacodynamic functions for the novel topoisomerase II inhibitor ETX0914 (AZD0914) in Neisseria gonorrhoeae. Front. Microbiol. 6:1377. doi: 10.3389/fmicb.2015.01377
Golparian, D., Harris, S. R., Sánchez-Busó, L., Hoffmann, S., Shafer, W. M., Bentley, S. D., et al. (2020). Genomic evolution of Neisseria gonorrhoeae since the preantibiotic era (1928-2013): antimicrobial use/misuse selects for resistance and drives evolution. BMC Genomics 21:116. doi: 10.1186/s12864-020-6511-6
Gomez-Valero, L., Rusniok, C., Jarraud, S., Vacherie, B., Rouy, Z., Barbe, V., et al. (2011). Extensive recombination events and horizontal gene transfer shaped the Legionella pneumophila genomes. BMC Genomics. doi: 10.1186/1471-2164-12-536
Goytia, M., Thompson, S. T., Jordan, S. V. L., and King, K. A. (2021). Antimicrobial resistance profiles of human commensal Neisseria species. Antibiotics 10:538. doi: 10.3390/antibiotics10050538
Higashi, D. L., Biais, N., Weyand, N. J., Agellon, A., Sisko, J. L., Brown, L. M., et al. (2011). N. elongata produces type IV pili that mediate interspecies gene transfer with N. gonorrhoeae. PLoS One 6:e21373. doi: 10.1371/journal.pone.0021373
Hong, E., Thulin Hedberg, S., Abad, R., Fazio, C., Enríquez, R., Deghmane, A. E., et al. (2013). Target gene sequencing to define the susceptibility of Neisseria meningitidis to ciprofloxacin. Antimicrob. Agents Chemother. 57, 1961–1964. doi: 10.1128/AAC.02184-12
Hyatt, D., Chen, G. L., LoCascio, P. F., Land, M. L., Larimer, F. W., and Hauser, L. J. (2010). Prodigal: prokaryotic gene recognition and translation initiation site identification. BMC Bioinformatics 11:119. doi: 10.1186/1471-2105-11-119
Irene, G., Marios, G., Fernando, A., Delia, B., Josefina, L., and de la Campa, A. G. (1998). Fluoroquinolone resistance mutations in the parC, parE, and gyrA genes of clinical isolates of viridans group Streptococci. Antimicrob. Agents Chemother. 42, 2792–2798. doi: 10.1128/AAC.42.11.2792
Janoir, C., Podglajen, I., Kitzis, M.-D., Poyart, C., and Gutmann, L. (1999). In vitro exchange of fluoroquinolone resistance determinants between Streptococcus pneumoniae and Viridans streptococci and genomic organization of the parE-parC region in S. mitis. J. Infect. Dis. 180, 555–558. doi: 10.1086/314888
Jolley, K. A., Bray, J. E., and Maiden, M. C. J. (2018). Open-access bacterial population genomics: BIGSdb software, the PubMLST.org website and their applications. Wellcome Open Res. 3:124. doi: 10.12688/wellcomeopenres.14826.1
José, F. M., Asunción, F., Josefina, L., and De La Campa, A. G. (2000). Horizontal transfer of parC and gyrA in fluoroquinolone-resistant clinical isolates of Streptococcus pneumoniae. Antimicrob. Agents Chemother. 44, 840–847. doi: 10.1128/AAC.44.4.840-847.2000
Kato, J., Suzuki, H., and Ikeda, H. (1992). Purification and characterization of DNA topoisomerase IV in Escherichia coli. J. Biol. Chem. 267, 25676–25684. doi: 10.1016/S0021-9258(18)35660-6
Katoh, K., Misawa, K., Kuma, K. I., and Miyata, T. (2002). MAFFT: a novel method for rapid multiple sequence alignment based on fast Fourier transform. Nucleic Acids Res. 30, 3059–3066. doi: 10.1093/nar/gkf436
Kenyon, C., Buyze, J., and Wi, T. (2018a). Antimicrobial consumption and susceptibility of Neisseria gonorrhoeae: a global ecological analysis. Front. Med. 5:329. doi: 10.3389/fmed.2018.00329
Kenyon, C., Laumen, J., and Manoharan-Basil, S. (2021). Choosing new therapies for gonorrhoea: we need to consider the impact on the pan-neisseria genome. a viewpoint. Antibiotics 10:515. doi: 10.3390/antibiotics10050515
Kenyon, C. R., Schwartz, I. S., Chris, R., and ISS, K. (2018b). Effects of sexual network connectivity and antimicrobial drug use on antimicrobial resistance in Neisseria gonorrhoeae. Emerg. Infect. Dis. 24, 1195–1203. doi: 10.3201/eid2407.172104
Kumar, S., Stecher, G., Li, M., Knyaz, C., and Tamura, K. (2018). MEGA X: molecular evolutionary genetics analysis across computing platforms. Mol. Biol. Evol. 35, 1547–1549. doi: 10.1093/molbev/msy096
Larkin, M. A., Blackshields, G., Brown, N. P., Chenna, R., McGettigan, P. A., McWilliam, H., et al. (2007). Clustal W and Clustal X version 2.0. Bioinformatics 23, 2947–2948. doi: 10.1093/bioinformatics/btm404
Lemey, P., Salemi, M., and Vandamme, A. (eds.) (2009). The Phylogenetic handbook: a practical approach to phylogenetic analysis and hypothesis testing. 142–160.
Lesher, G. Y., Froelich, E. J., Gruett, M. D., Bailey, J. H., and Brundage, R. P. (1962). 1,8-naphthyridine derivatives. A new class of chemotherapeutic agents. J. Med. Pharm. Chem. 91, 1063–1065. doi: 10.1021/jm01240a021
Letunic, I., and Bork, P. (2021). Interactive tree of life (iTOL) v5: an online tool for phylogenetic tree display and annotation. Nucleic Acids Res. 49, W293–W296. doi: 10.1093/nar/gkab301
Ligon, B. L. (2005). Albert Ludwig Sigesmund Neisser: discoverer of the cause of gonorrhea. Semin. Pediatr. Infect. Dis. 16, 336–341. doi: 10.1053/j.spid.2005.07.001
Lindbäck, E., Rahman, M., Jalal, S., and Wretlind, B. (2002). Mutations in gyrA, gyrB, parC, and parE in quinolone-resistant strains of Neisseria gonorrhoeae. APMIS 110, 651–657. doi: 10.1034/j.1600-0463.2002.1100909.x
Manoharan-Basil, S. S., Laumen, J. G. E., Van Dijck, C., De Block, T., De Baetselier, I., Kenyon, C., et al. (2021). Evidence of horizontal gene transfer of 50S ribosomal genes rplB, rplD, and rplY in Neisseria gonorrhoeae. Front. Microbiol. 12:683901. doi: 10.3389/fmicb.2021.683901
Martin, D. P., Murrell, B., Golden, M., Khoosal, A., and Muhire, B. (2015). RDP4: detection and analysis of recombination patterns in virus genomes. Virus Evol. 1:vev003. doi: 10.1093/ve/vev003
Osnes, M., Didelot, X., de Korne-Elenbaas, J., Alfsnes, K., Brynildsrud, O., Syversen, G., et al. (2020). The sudden emergence of a Neisseria gonorrhoeae strain with reduced susceptibility to extended-spectrum cephalosporins, Norway. bioRxiv [Preprint]. doi: 10.1101/2020.02.07.935825
Osnes, M. N., van Dorp, L., Brynildsrud, O. B., Alfsnes, K., Schneiders, T., Templeton, K. E., et al. (2021). Antibiotic treatment regimes as a driver of the global population dynamics of a major gonorrhea lineage. Mol. Biol. Evol. 38, 1249–1261. doi: 10.1093/molbev/msaa282
Piddock, L. J. (1999). Mechanisms of fluoroquinolone resistance: an update 1994-1998. Drugs 58, 11–18. doi: 10.2165/00003495-199958002-00003
Reece, R. J., and Maxwell, A. (1991). DNA gyrase: structure and function. Crit. Rev. Biochem. Mol. Biol. 26, 335–375. doi: 10.3109/10409239109114072
Reimche, J. L., Chivukula, V. L., Schmerer, M. W., Joseph, S. J., Pham, C. D., Schlanger, K., et al. (2021). Genomic analysis of the predominant strains and antimicrobial resistance determinants Within 1479 Neisseria gonorrhoeae isolates from the US gonococcal isolate surveillance project in 2018. Sex. Transm. Dis. 48, S78–S87. doi: 10.1097/OLQ.0000000000001471
Rouquette-Loughlin, C., Dunham, S. A., Kuhn, M., Balthazar, J. T., and Shafer, W. M. (2003). The NorM efflux pump of Neisseria gonorrhoeae and Neisseria meningitidis recognizes antimicrobial cationic compounds. J. Bacteriol. 185, 1101–1106. doi: 10.1128/JB.185.3.1101-1106.2003
Sánchez-Busó, L., Yeats, C. A., Taylor, B., Goater, R. J., Underwood, A., Abudahab, K., et al. (2021). A community-driven resource for genomic epidemiology and antimicrobial resistance prediction of Neisseria gonorrhoeae at Pathogenwatch. Genome Med. 13:61. doi: 10.1186/s13073-021-00858-2
Salminen, M. O., Carr, J. K., Burke, D. S., and Mccutchan, F. E. (1995). Identification of Breakpoints in Intergenotypic Recombinants of HIV Type 1 by Bootscanning. AIDS Res. Hum. Retroviruses. doi: 10.1089/aid.1995.11.1423
Shafer, W. M. (2018). Mosaic drug efflux gene sequences from commensal neisseria can lead to low-level azithromycin resistance expressed by Neisseria gonorrhoeae clinical isolates. MBio 9, e01747–e01718. doi: 10.1128/mBio.01747-18
Shen, Y., and Chen, M. (2020). Prevalence, sequence type, and quinolone resistance of Neisseria lactamica carried in children younger than 15 years in Shanghai, China. J. Inf. Secur. 80, 61–68. doi: 10.1016/j.jinf.2019.08.020
Shultz, T. R., Tapsall, J. W., and White, P. A. (2001). Correlation of in vitro susceptibilities to newer quinolones of naturally occurring quinolone-resistant Neisseria gonorrhoeae strains with changes in GyrA and ParC. Antimicrob. Agents Chemother. 45, 734–738. doi: 10.1128/AAC.45.3.734-738.2001
Silva, M., Machado, M. P., Silva, D. N., Rossi, M., Moran-Gilad, J., Santos, S., et al. (2018). chewBBACA: a complete suite for gene-by-gene schema creation and strain identification. Microb. Genom. 4:e000166. doi: 10.1099/mgen.0.000166
Tacconelli, E., and Magrini, N. (2017). Global Priority List of Antibiotic-Resistant Bacteria to Guide Research, Discovery, and Development of New Antibiotics. Organ Mund la Salud.
Tanaka, M., Nakayama, H., Haraoka, M., and Saika, T. (2000). Antimicrobial resistance of Neisseria gonorrhoeae and high prevalence of ciprofloxacin-resistant isolates in Japan, 1993 to 1998. J. Clin. Microbiol. 38, 521–525. doi: 10.1128/JCM.38.2.521-525.2000
Tapsall, J. W., Ndowa, F., Lewis, D. A., and Unemo, M. (2009). Meeting the public health challenge of multidrug- and extensively drug-resistant Neisseria gonorrhoeae. Expert Rev. Anti-Infect. Ther. 7, 821–834. doi: 10.1586/eri.09.63
Taylor, S. N., Marrazzo, J., Batteiger, B. E., Hook, E. W. III, Seña, A. C., Long, J., et al. (2018). Single-dose zoliflodacin (ETX0914) for treatment of urogenital gonorrhea. N. Engl. J. Med. 379, 1835–1845. doi: 10.1056/NEJMoa1706988
Trees, D. L., Sandul, A. L., Peto-Mesola, V., Aplasca, M. R., Bun Leng, H., Whittington, W. L., et al. (1999). Alterations within the quinolone resistance-determining regions of GyrA and ParC of Neisseria gonorrhoeae isolated in the Far East and the United States. Int. J. Antimicrob. Agents 12, 325–332. doi: 10.1016/s0924-8579(99)00081-3
Unemo, M., Ahlstrand, J., Sánchez-Busó, L., Day, M., Aanensen, D., Golparian, D., et al. (2021). High susceptibility to zoliflodacin and conserved target (GyrB) for zoliflodacin among 1209 consecutive clinical Neisseria gonorrhoeae isolates from 25 European countries, 2018. J. Antimicrob. Chemother. 76, 1221–1228. doi: 10.1093/jac/dkab024
Unemo, M., Ross, J., Serwin, A., Gomberg, M., Cusini, M., and Jensen, J. S. (2020). 2020 European guideline for the diagnosis and treatment of gonorrhoea in adults. Int. J. STD AIDS doi: 10.1177/0956462420949126 [Epub ahead of print].
Unemo, M., and Shafer, W. M. (2011). Antibiotic resistance in Neisseria gonorrhoeae: origin, evolution, and lessons learned for the future. Ann. N. Y. Acad. Sci. 1230, E19–E28. doi: 10.1111/j.1749-6632.2011.06215.x
Wadsworth, C. B., Arnold, B. J., Sater, M. R. A., and Grad, Y. H. (2018). Azithromycin resistance through interspecific acquisition of an epistasis-dependent efflux pump component and transcriptional regulator in Neisseria gonorrhoeae. MBio 9, e01419–e01418. doi: 10.1128/mBio.01419-18
Willcox, R. R. (1970). A survey of problems in the antibiotic treatment of gonorrhoea. With special reference to South-East Asia. Br. J. Vener. Dis. 46, 217–242. doi: 10.1136/sti.46.3.217
Wittmann, H. G., Stöffler, G., Apirion, D., Rosen, L., Tanaka, K., Tamaki, M., et al. (1973). Biochemical and genetic studies on two different types of erythromycin resistant mutants of Escherichia coli with altered ribosomal proteins. Mol. Gen. Genet. 127, 175–189. doi: 10.1007/BF00333665
Yahara, K., Ma, K. C., Mortimer, T. D., Shimuta, K., Nakayama, S. I., Hirabayashi, A., et al. (2021). Emergence and evolution of antimicrobial resistance genes and mutations in Neisseria gonorrhoeae. Genome Med. 13:51. doi: 10.1186/s13073-021-00860-8
Yahara, K., Ma, K. C., Mortimer, T. D., Shimuta, K., Nakayama, S. I., Hirabayashi, A., et al. (2020). Emergence and evolution of antimicrobial resistance genes and mutations in Neisseria gonorrhoeae. bioRxiv [Preprint]. doi: 10.1101/2020.10.26.354993
Yahara, K., Nakayama, S. I., Shimuta, K., Lee, K. I., Morita, M., Kawahata, T., et al. (2018). Genomic surveillance of neisseria gonorrhoeae to investigate the distribution and evolution of antimicrobial-resistance determinants and lineages. Microb. Genomics 4:e000205. doi: 10.1099/mgen.0.000205
Yang, Y., Liao, M., Gu, W.-M., Bell, K., Wu, L., Eng, N. F., et al. (2006). Antimicrobial susceptibility and molecular determinants of quinolone resistance in Neisseria gonorrhoeae isolates from Shanghai. J. Antimicrob. Chemother. 58, 868–872. doi: 10.1093/jac/dkl301
Zechiedrich, E. L., and Cozzarelli, N. R. (1995). Roles of topoisomerase IV and DNA gyrase in DNA unlinking during replication in Escherichia coli. Genes Dev. 9, 2859–2869. doi: 10.1101/gad.9.22.2859
Zechiedrich, E. L., Khodursky, A. B., and Cozzarelli, N. R. (1997). Topoisomerase IV, not gyrase, decatenates products of site-specific recombination in Escherichia coli. Genes Dev. 11, 2580–2592. doi: 10.1101/gad.11.19.2580
Keywords: HGT, gyrA, gyrB, parC, parE, Neisseria gonorrhoeae, commensal Neisseria
Citation: Manoharan-Basil SS, González N, Laumen JGE and Kenyon C (2022) Horizontal Gene Transfer of Fluoroquinolone Resistance-Conferring Genes From Commensal Neisseria to Neisseria gonorrhoeae: A Global Phylogenetic Analysis of 20,047 Isolates. Front. Microbiol. 13:793612. doi: 10.3389/fmicb.2022.793612
Edited by:
Baolei Jia, Chung-Ang University, South KoreaReviewed by:
Oscar Q. Pich, Parc Taulí Foundation, SpainDmitry Gryadunov, Engelhardt Institute of Molecular Biology (RAS), Russia
Leshan Xiu, Shanghai Jiao Tong University, China
Copyright © 2022 Manoharan-Basil, González, Laumen and Kenyon. This is an open-access article distributed under the terms of the Creative Commons Attribution License (CC BY). The use, distribution or reproduction in other forums is permitted, provided the original author(s) and the copyright owner(s) are credited and that the original publication in this journal is cited, in accordance with accepted academic practice. No use, distribution or reproduction is permitted which does not comply with these terms.
*Correspondence: Sheeba Santhini Manoharan-Basil, sbasil@itg.be