- 1Clinical and Translational Research Institute, The Medical City, Pasig, Philippines
- 2Institute of Biology, College of Science, University of the Philippines Diliman, Quezon City, Philippines
- 3Department of Biological Sciences, College of Science, University of Santo Tomas, Manila, Philippines
- 4Research Center for Natural and Applied Sciences, University of Santo Tomas, Manila, Philippines
- 5The Graduate School, University of Santo Tomas, Manila, Philippines
- 6National Institutes of Health, University of the Philippines Manila, Manila, Philippines
Southeast Asia (SEA) can be considered a hotspot of antimicrobial resistance (AMR) worldwide. As recent surveillance efforts in the region reported the emergence of multidrug-resistant (MDR) pathogens, the pursuit of therapeutic alternatives against AMR becomes a matter of utmost importance. Phage therapy, or the use of bacterial viruses called bacteriophages to kill bacterial pathogens, is among the standout therapeutic prospects. This narrative review highlights the current understanding of phages and strategies for a phage revolution in SEA. We define phage revolution as the radical use of phage therapy in infectious disease treatment against MDR infections, considering the scientific and regulatory standpoints of the region. We present a three-phase strategy to encourage a phage revolution in the SEA clinical setting, which involves: (1) enhancing phage discovery and characterization efforts, (2) creating and implementing laboratory protocols and clinical guidelines for the evaluation of phage activity, and (3) adapting regulatory standards for therapeutic phage formulations. We hope that this review will open avenues for scientific and policy-based discussions on phage therapy in SEA and eventually lead the way to its fullest potential in countering the threat of MDR pathogens in the region and worldwide.
Introduction
A recent report identified Southeast Asia (SEA) as a global epicenter of antimicrobial resistance and emerging infectious diseases evolution (Chua et al., 2021). In the report by Chua et al. (2021) and in our paper, SEA is defined as the region that is home to the member states of the Association of Southeast Asian Nations (ASEAN; composed of Brunei, Cambodia, Indonesia, Laos, Malaysia, Myanmar, Philippines, Singapore, Thailand, and Vietnam). The issue of multidrug resistance (MDR) remains one of the region’s substantial challenges. Over the last 10 years, clinicians and researchers reported the rise of critical MDR organisms in SEA (Table 1). Alarming reports from the region also point to the emergence of resistance to colistin, a last-resource antibiotic, making MDR a concern of global interest and high priority. The current burden of AMR in the region remains unknown. Still, country-specific reports indicated high percentages of carbapenem-resistant Acinetobacter baumannii (39–68%), Pseudomonas aeruginosa (15–44%), and Klebsiella pneumoniae (4–29%) in Malaysia, Philippines, Thailand, and Vietnam (Center for Disease Dynamics, Economics, and Policy, 2021). Unregulated access to antibiotics, weak antibiotic stewardship programs, and low-quality antibiotics are the primary culprits of rampant MDR in SEA (Zellweger et al., 2017). Ultimately, the decreasing efficacy of our existing antibiotics and the shortage of newly discovered antimicrobials in the global pipeline will exacerbate the current situation of MDR. The World Health Organization estimates that antibiotic resistance will cause 10 million deaths in 2050, nearly half of which coming from Asia-Pacific, including SEA (World Health organization, 2019).
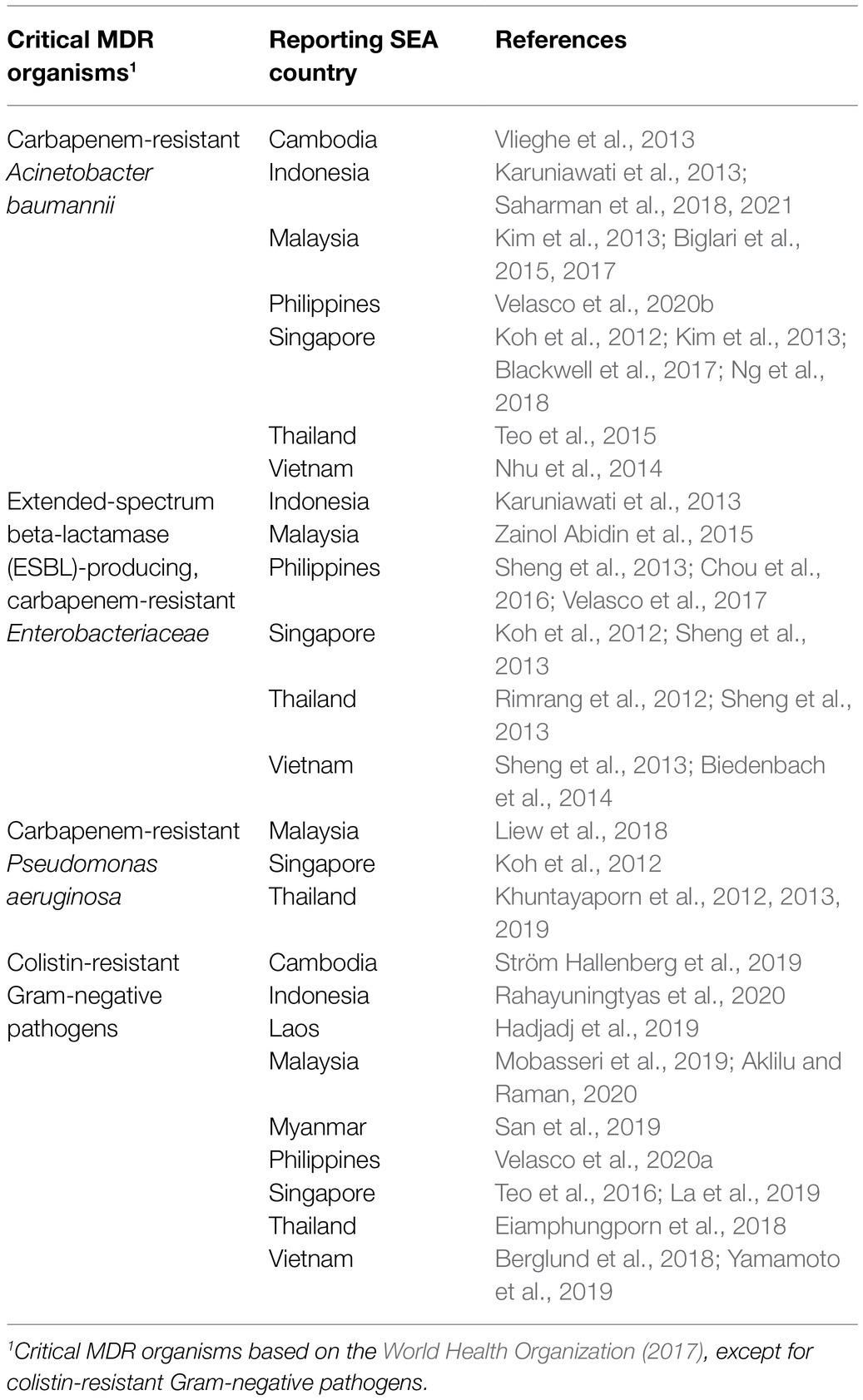
Table 1. Reports of critical multidrug-resistant (MDR) organisms from clinical isolates in Southeast Asia (SEA) from 2011 to 2021.
The threat of an imminent “post-antibiotic” era pushed scientists to identify novel agents and therapeutic approaches to combat MDR organisms. Harper and Enright (2011) described this situation where researchers progressively look for new antimicrobial agents or revisit traditional therapeutic options as a “perfect storm.” One of the therapeutic prospects to mitigate the AMR crisis is phage therapy. Phage therapy refers to the use of bacterial viruses, called bacteriophages (or simply phages), to treat and control infections. Phage therapy harnesses the capabilities of the virus to specifically bind and inject their genetic material into their target bacteria, produce progeny virions, and eventually lyse their host. Although the pioneering works of d’Herelle (1917) and Twort (1915) on phage therapy sparked the scientific community’s interest in its prospects for therapeutic application against human and animal infections, it was quickly overshadowed by the discovery and extensive use of penicillin. Members of the medical community continued using phages to manage common infections until the late 1940s, with applications reported in Europe and the United States (Harper and Enright, 2011; Ghannad and Mohammadi, 2012; Jin et al., 2012; Barbu et al., 2016). In recent years, a rekindled interest in phage therapy for treating MDR organisms led to remarkable breakthroughs. While several recent review articles highlight the advances of phage therapy (Khalid et al., 2021; Ng et al., 2021; Wu and Zhu, 2021), none of these papers focus on the ongoing research and practical applicability in SEA, particularly in the clinical setting. A recent perspective paper discussed phage applications in SEA, focusing on livestock, aquaculture, and agriculture sectors (Rajandas et al., 2021). The potential of phage therapy for clinical applications, however, is not examined. In this narrative review of the published literature, we assess the current understanding of phages, challenges in phage therapy, and strategies that will pave the way for a phage revolution, which we define as the use of phage therapy in infectious disease treatment in humans, considering the scientific and regulatory standpoint in SEA. Asavarut and Hajitou (2014) loosely mentioned “phage revolution” in a book review on phage therapy. In our article, we expound on the concept of phage revolution by proposing a three-phase strategy that can guide member states of the ASEAN region. The proposed roadmap is essential for realizing phage therapy’s promise in countering the threat of MDR clinical pathogens in the region and potentially in the world.
Phages in SEA
Phages are viral particles composed of genetic material enclosed in a capsid or head, and in most cases, a proteinaceous tail. The term “bacteriophage” literally translates to “bacteria-eater.” Biologists describe them as natural parasites (or predators) of bacteria and associate them with maintaining the balance of microorganisms on Earth (Wittebole et al., 2014). Tailed phages of the class Caudoviricetes including podovirus, myovirus, and siphovirus (Liu et al., 2021), and the polyhedral Microviridae family are usually associated with phage therapy applications (Supplementary Figure 1; Harper and Enright, 2011; Lin et al., 2017). Scientists attribute the phages’ antibacterial activity to their well-established life cycle (lytic or lysogenic; Supplementary Figure 2). In some cases, phages can adapt both lysogenic and lytic strategies (i.e., pseudolysogeny) as alternative infection steps in response to different host strains, host physiology, and environmental changes (Mäntynen et al., 2021). Since phages are ubiquitous, it is not surprising that they may be isolated from an extensive range of sampling sites like feces, seawater, sewage, soil, sludge, and anywhere bacteria may grow (Khawaja et al., 2016; Bhetwal et al., 2017).
In the past decade, scientists reported the isolation of diverse phages in SEA from different sample types, with potential activities against human pathogens (Table 2). Among the phages discovered in the region with notable activity against MDR organisms are: (1) phages vB_AbaM_PhT2 (Styles et al., 2020) and AB1801 (Wintachai et al., 2019), which exhibited anti-MDR A. baumannii activity, in vitro and in vivo, respectively, (2) phage UPM2146 (Assafiri et al., 2021), which exhibited activity against carbapenem-resistant K. pneumoniae, in vitro and in vivo, (3) phage C34 (Guang-Han et al., 2016), which controlled naturally resistant Burkholderia pseudomallei bacterial load, in vivo, (4) phage ΦHN10 (Phothichaisri et al., 2018), which exhibited the highest breadth of activity against various Clostridiodes difficile strains, in vitro, (5) phage ΦKAZ14 (Ahmad et al., 2015), which induced lysis of extended-spectrum beta-lactamase (ESBL)-producing Escherichia coli, in vitro, (6) phages UPMK_1 and 2 (Dakheel et al., 2019) and ΦNUSA-1 and 10 (Tan et al., 2020), which all exhibited lytic activity against methicillin-resistant Staphylococcus aureus (MRSA), and anti-biofilm activity for the first two phages in in vitro experiments, (7) cocktail of phages vB_SenS_WP109, WP110, and WP128 (Pelyuntha et al., 2021), which induced lysis of multiple MDR Salmonella serovars, in vitro, and (8) phages KP1, 2 (Cornista et al., 2019), and KP1801 (Wintachai et al., 2020), which were shown to infect ESBL-producing K. pneumoniae, in vitro. Although the actual diversity of phages remains understudied in SEA, we believe that there is a significant richness of phage strains in this region, a renowned biodiversity hotspot not only in terms of fauna and flora but also in microbiota. This hypothesis is supported by the growing number of genome data entries for phage in the ASEAN Microbial Database (AMIBASE), with more than 200 recorded distinct phage entries, with each entry containing one or more phage strains or genomes (https://www.amibase.org/index.php, accessed on 20 December 2021). The majority of the discovered phages belong to the class Caudoviricetes (188 records), dominated by siphovirus (80 records) and myovirus (60 records). Microviridae phages were also reported, although infrequently (four records). Clinically important host ranges of the reported phages include Acinetobacter, Aeromonas, Bacillus, Bordetella, Brucella, Burkholderia, Campylobacter, Caulobacter, Citrobacter, Clostridioides, Corynebacterium, Edwardsiella, Enterobacter, Enterococcus, Erysipelothrix, Escherichia, Helicobacter, Leptospira, Listeria, Mycobacterium, Nocardia, Propionibacterium, Pseudomonas, Raoultella, Salmonella, Serratia, Shewanella, Shigella, Staphylococcus, Stenotrophomonas, Streptococcus, Vibrio, Weissella, and Yersinia. However, most of the phage’s actual activities against these hosts have yet to be described. Phage sources listed in AMIBASE include freshwater, wastewater, sludge, marine water and sediment, soil, hot spring, bioreactor, humans, plants, invertebrates, and air, although the isolation procedures were not specified. Singapore contributed most of the records (191), followed by Malaysia (187) and Thailand (171). Brunei, Laos, and Myanmar have no phage records in AMIBASE as of this writing, indicating potential avenues for discovering novel phages in these countries. We hope that through this initial report, we can increase the number of phages and phage genomes annotated in the region so more data on phages can be shared and studied by SEA researchers.
Phage Research in SEA
Our understanding of phages and phage therapy relies on the research of basic and applied scientists worldwide. To determine the extent and coverage of biomedical phage research in SEA, we conducted a preliminary original article search in PubMed (https://www.pubmed.ncbi.nlm.nih.gov/, accessed 20 December 2021) using the keywords “phage” AND “[ASEAN country]” from 2011 to 2021. We then screened the articles and only included original research conducted in SEA countries or those which used samples coming from SEA. We attempted a localized biomedical literature search but failed due to the unavailability of a health research database in most SEA countries. In the Philippines (HERDIN, https://www.registry.healthresearch.ph, accessed 01 July 2021), Malaysia (Ministry of Health Virtual Library, https://www.vlib.moh.gov.my, accessed 01 July 2021), and Indonesia (https://www.neliti.com, accessed 01 July 2021), the available health research registries reported no local biomedical publications on phages. We summarized our literature search result in Figure 1. The current literature search we conducted is limited only to PubMed, but it can help determine the region’s strengths and priorities on biomedical phage research. Thailand, Malaysia, and Singapore were the top three contributors to biomedical-related phage research in SEA. In the latest economic report, these countries have high gross domestic product (Vu, 2020) which may reflect their capability to perform high-end scientific explorations for potential high-value therapeutics, such as phage therapy. Hence, it is not surprising to see these countries as the frontrunners for phage research in the region. We believe that the studies on phages in SEA can still be increased, particularly by focusing on its applications against MDR organisms. By leveraging the existing phage expertise in the region, a research consortium on phages could be created to further address the research gaps on phages in SEA. We will expound on this concept later on in this review.
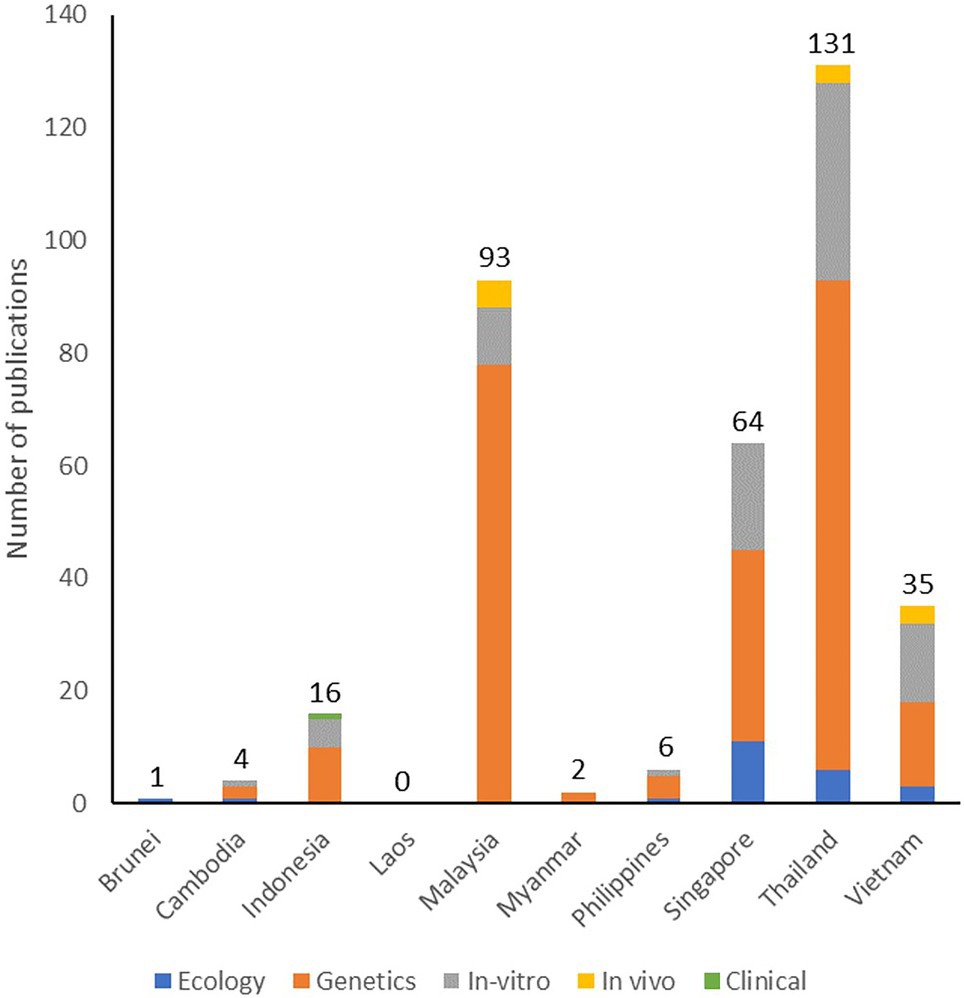
Figure 1. Number and types of original research articles on phages published in SEA and indexed in PubMed from 2011 to 2021.
Most research on phages in SEA consists of genetic studies, including genomic characterization of phages and bioengineering applications, such as phage typing and phage display. In terms of bioengineering phages for therapeutic applications, SEA scientists reported the use of phages for targeted gene therapy (Namdee et al., 2018; Przystal et al., 2019; Chongchai et al., 2021) and adaptation of nanomaterial-based phage delivery systems (Adamu Ahmad et al., 2016; Kaikabo et al., 2017). There is also a notable amount of research on in vitro testing of phages, including the testing for the phage’s host range, biocontrol assays against pathogens, and phage formulation techniques. We retrieved a few publications on in vivo testing of phages using invertebrates, such as moth (Wintachai et al., 2019; Nale et al., 2021) and oysters (Le et al., 2020); in vivo vertebrate model systems included zebrafish (Assafiri et al., 2021), catfish (Le et al., 2018; Dang et al., 2021), tilapia (Dela Cruz-Papa et al., 2014, 2017), chicken (Wong et al., 2014; Kaikabo et al., 2017), and mice (Guang-Han et al., 2016). Lastly, a few publications focused on the applications of phages in combating MDR organisms. For instance, SEA researchers discovered and characterized phages with activity against MRSA (Dakheel et al., 2019; Tan et al., 2020), ESBL-producing E. coli (Adamu Ahmad et al., 2016), MDR K. pneumoniae (Cornista et al., 2019; Assafiri et al., 2021), MDR A. baumannii (Wintachai et al., 2019; Styles et al., 2020), MDR Salmonella species (Pelyuntha et al., 2021), and Clostridioides difficile (Phothichaisri et al., 2018). The efficacy of these phages has yet to be confirmed clinically. Currently, global case reports lean towards phage therapy applications for bone, surgical, and other local infections, with several cases also reporting usage in critical and systemic infections, especially those caused by MDR organisms. With the region’s current strength in basic and genetic characterization of phages and growing expertise in in vitro and in vivo testing of localized external infections in animals, we expect that the future clinical applications of phage therapy in the region would also lean towards the treatment of surgical and skin infections of known etiology. Unfortunately, at the time of writing our review, the region had no published case report on any phage therapy applications in humans.
Considerations in Phage Therapy
Not all phages are ideal for therapeutic applications. Therapeutic phages should be obligately lytic to ensure killing of the target pathogen (Harper and Enright, 2011; Wittebole et al., 2014; Lehman et al., 2019). The phage should have a high rate of adsorption to the target pathogen, a short generation time (Drulis-Kawa et al., 2015), and species-specific activity (Khawaja et al., 2016). As in the case with typical antimicrobial agents, scientists also recommend profiling the phages in a phagogram or a phage equivalent of an antibiogram (Barbu et al., 2016). A phagogram refers to the continuous testing of the phage efficacy against a defined collection of pathogens called a pathogen library or diversity panel (Deresinski, 2009; Casey et al., 2018). Phagogram profiling will ensure that the therapeutic phages are specific to their target pathogen. Standardized phagogram profiling is not yet incorporated in most in vitro and in vivo testing of phages discovered in SEA, although specificity testing in multiple strains of target pathogens have been done (Abdulamir et al., 2015; Phothichaisri et al., 2018; Dakheel et al., 2019; Tan et al., 2020).
Therapeutic phages are usually combined with storage media to produce a phage formulation. As with other therapeutics, phage formulations should be prepared according to current good manufacturing practices (GMP) and established quality assurance standards. Phage formulations should neither have impurities nor contaminants (i.e., endotoxins and host cell proteins; Jennes et al., 2017; Furfaro et al., 2018; Lehman et al., 2019). Since the delivery route influences the efficacy of phage therapy, various studies have explored different ways of phage administration for therapeutic purposes. One common way to administer phage therapy is through topical applications for skin and burn infections (Morozova et al., 2018). Oral phage administration is also a common scheme (Kakasis and Panitsa, 2019). Considering that the phages will be taken internally, they should be stable at human body temperature (35–39°C) and physiological pH (4–9; Jin et al., 2012). Other factors, such as stability in low gastric pH and bile salts, should also be considered (Letkiewicz et al., 2010). Hence, phage formulations can include bicarbonate water or be enclosed in a gel (Kakasis and Panitsa, 2019). In a study in Malaysia, researchers constructed chitosan nanoparticles loaded with phage ΦKAZ14 as an alternative oral delivery platform in chickens. They indicated that the chitosan-phage nanoparticle is more stable, in vivo, compared to standalone phage (Adamu Ahmad et al., 2016). An extension of this study showed that the same nanoparticle controlled the bacterial colonization of avian pathogenic E. coli in chicken and decreased the symptoms of colibacillosis (Kaikabo et al., 2017). The applicability of using this nanoparticle-based oral delivery method in clinical applications can be explored in SEA since the technology and knowledge for creating it is already being explored in Malaysia. Other administration routes for therapeutic phages include the respiratory route through intranasal spray (Cao et al., 2015; Casey et al., 2018), systemic route through intraperitoneal or intramuscular injection (Criscuolo et al., 2017; Jennes et al., 2017), or rectal route through fecal microbiota transplant (Letkiewicz et al., 2010; Broecker et al., 2013). These administration routes are yet to be explored in SEA, in vivo or clinically.
Similar to antibiotic therapies, the pharmacodynamic properties of phages must be determined to establish an effective dosing regimen for the successful treatment of severe infections. Proper phage dosage ensures that an appropriate amount of the virus will encounter the target pathogen in the infection site (Zhang et al., 2018). In an inundative therapy (passive treatment), a high titer (108–1010) of phages is administered to the patients (usually in single-dose regimen) to kill a large number of the target pathogen (Danis-Wlodarczyk et al., 2021). Although some studies aligned with this strategy (Zhang et al., 2018), opposing views caution that a too high number of phages may result in “lysis from without,” or the non-specific lysis of cells caused by phages (Barbu et al., 2016; Cieplak et al., 2018). More studies are needed to confirm this phenomenon. Meanwhile, an active therapy depends on the in situ amplification of phages to achieve the inundative concentration for bacterial clearance (Danis-Wlodarczyk et al., 2021). No formal study on phage pharmacodynamics has been done in the SEA population.
Biofilm formation is an important virulence factor in most MDR pathogens. Remarkably, phages have been studied for their activity against bacterial biofilms. In vitro studies in SEA showed that certain bacteriophages degrade biofilms of A. baumannii (Wintachai et al., 2019), E. coli (Jassim et al., 2012), K. pneumoniae (Wintachai et al., 2020), and S. aureus (Abdulamir et al., 2015; Dakheel et al., 2019). This activity can be attributed to the production of various hydrolases (i.e., extracellular polymeric substance depolymerases) in the capsid that can effectively disrupt and disperse bacterial biofilms (Lin et al., 2017). Some phages would also have tail fibers with inherent depolymerase activities (Dufour et al., 2016). A deeper understanding of the role and mechanisms of phages in disrupting biofilms remains to be gained, as in vivo studies and reports in the clinical setting have yet to be described.
Finally, phage resistance is another aspect that should be considered in phage therapy. Researchers believe that the evolutionary pressure exerted by the phage greatly exceeds any resistance mechanisms acquired by the target bacteria (El-Shibiny and El-Sahhar, 2017; Harper, 2018). In addition, the rapid evolution of phages helps improve their virulence towards the target bacteria, making adaptive bacterial resistance ineffective to phages (Golkar et al., 2014). However, the occurrence of phage-resistant bacteria is inevitable and should be considered when designing phage therapy treatment (Cairns and Payne, 2008; Casey et al., 2018). For instance, a P. aeruginosa small colony variant resistant to phage PB1 has been described in Singapore (Lim et al., 2016), but its susceptibility to other phages has not been explored. To prevent phage resistance, one should be on the lookout for bacterial mechanisms against phages after administering phage therapy. These mechanisms may include the CRISPR-Cas system, superinfection exclusion, and abortive infection. Phage cocktails could also be explored as an option to help prevent the rise of phage resistance, as we will describe later. In summary, the considerations in phage therapy should be observed starting from the characterization of the isolated phages, their preparation, and the administration to the patients (Supplementary Figure 3).
Perspectives of Phage Therapy in SEA
Frederick Twort and Félix d’Hérelle independently observed the bactericidal activity of phages in 1915 and 1917, respectively. In 1919, d’Hérelle used phages to treat bacterial infections, marking the start of non-randomized trials worldwide and launching the term “phage therapy” (Wittebole et al., 2014; Criscuolo et al., 2017). In SEA, the earliest documented phage therapy application was from the expeditions of d’Herelle and colleagues in Laos and Vietnam from 1916 to 1930 (Chanishvili, 2012). According to the historical accounts, d’Herelle used phages isolated from plague-infected rats to treat plague victims and introduced phages from cholera patients into village wells to decrease mortality rates from cholera in the region (Vandamme and Mortelmans, 2019).
Despite the decline in the medical field’s enthusiasm on phage therapy (i.e., due to the discovery of penicillin and lack of experimental support for phage) in the late 1940s, phage therapy centers were continuously built in the USSR, Georgia (George Eliava Institute, 1916), Poland (Hirtszfeld Institute; Institute of Immunology and Experimental Therapy of Polish Academy of Sciences, 1952), and France (Pasteur Institute in Lyon and Paris, 1917). In SEA, the Phage Directory (https://www.phage.directory/, accessed on 20 December 2021), an online directory to find and track phage laboratories worldwide, conceptualized and maintained by an independent, two-person organization, recorded several phage laboratories and phage collections, but no registered phage therapy center. The listed phage laboratories are found in Indonesia (University of Jember-KeRis Terapi Bakteriofag), the Philippines (University of Santo Tomas-Papa Lab), and Thailand (Silpakorn University-Akoy Lab, Kasetsart University-Kasetsart Phages). In Malaysia, the Center for Excellence for Omics-Driven Computational Biodiscovery (COMBio) of the Asian Institute of Medicine, Science and Technology also explores phages isolated from rainforests against key human pathogens (Rajandas et al., 2021). Meanwhile, the listed phage collections are found in Indonesia (University of Jember-KeRis Terapi Bakteriofag Phage Collection) and Thailand (Silpakorn University-Nasanit/Akoy Lab Phage Collection) only. Some institutions and phage laboratories not listed in the Phage Directory, but whose members contributed to recent biomedical and pre-clinical research literature on phages, can be found in Indonesia (Atmajaya Catholic University, Brawijaya University, Universitas Airlangga), Malaysia (International Medical University, Monash University Malaysia, Universiti Putra Malaysia, Universiti Sains Malaysia, University of Malaya), Philippines (University of the Philippines), Singapore (Nanyang Technological University, National University Singapore), Thailand (Chulabhorn Research Institute, Chulabhorn Royal Academy, Chulalongkorn University, Mae Fah Luang University, Mahidol University, Prince of Songkla University, Srinakharinwirot University, University of Phayao, Walailak University), and Vietnam (Ho Chi Minh City University of Technology, Vietnam National University). Although the list of institutions and laboratories presented in this review is based only on what is retrievable in PubMed (https://www.pubmed.ncbi.nlm.nih.gov/, accessed 20 December 2021) and may not be complete, this report shows that there is an interest among SEA academic laboratories in studying phages.
In 2009, the Nestlé Research Center (Switzerland) started the first randomized, double-blind, placebo-controlled phase I trial on the safety of phage therapy in Bangladesh. The study reported that orally administered phages do not affect the normal flora of the participants, particularly the native E. coli populations (Sarker et al., 2016). In SEA, a clinical trial in Singapore evaluated phage therapy in wound care for critical limb ischemia. This trial, registered as “ongoing” at the clinical trial registry of the Singapore Health Sciences Authority (HSA, https://www.eservice.hsa.gov.sg, accessed 20 December 2021) in 2015, was sponsored by D&D Pharma (Pte.) Ltd. (Protocol number SGH001). The Singapore General Hospital and Mount Elizabeth Novena Hospital & Specialist Centre served as the trial sites. However, the trial’s interim data or publications were unavailable when writing this review. There is currently no registered clinical trial that explicitly targets multidrug-resistant pathogens in the region (based on www.clinicaltrials.gov as of 20 December 2021).
Although clinical studies on phage therapy are lacking in SEA, in vivo pre-clinical studies have been reported. For instance, phages have been shown to successfully control A. baumannii (Wintachai et al., 2019) and Salmonella spp. (Nale et al., 2021) in Galleria mellonella larvae. These findings are initial evidence of phage efficacy in reducing surface colonization of pathogens in animals, including humans. Researchers in SEA also showed the safety of using phages in controlling K. pneumoniae infection in Danio rerio larvae (Assafiri et al., 2021). Another study of lung infections caused by B. pseudomallei in mice indicated that phages administered peritoneally successfully protected the animals from disease progression and mortality (Guang-Han et al., 2016). Other in vivo phage therapy studies focused on treating infections in poultry (Wong et al., 2014; Kaikabo et al., 2017) and aquaculture (Dela Cruz-Papa et al., 2014, 2017; Le et al., 2018; Dang et al., 2021). The implications for clinical use, however, may not be apparent especially if the bacterial pathogen is specific only to the animal (i.e., A. hydrophila in freshwater fishes, avian pathogenic E. coli in birds, and Vibrio alginolyticus in oysters). However, with the potential zoonotic transmission of infections, the expanded use of specific phages for treating both animals and humans could be possible.
The lack of actual clinical experience, established phage collections, and phage consortium in SEA are gaps of knowledge that need to be filled, thus providing an opportunity to build the foundation for shifting the paradigm of treatment. In the next section, we propose systematic strategies to start a phage revolution in SEA and invite more biologists, biomedical scientists, and clinical decision-makers in the region to explore phage therapy against MDR organisms.
Strategies for Phage Revolution in SEA
A structured research and implementation approach is necessary to encourage phage therapy against multidrug-resistant infections in SEA (Figure 2). The first phase involves the extensive isolation, characterization, and matching of phages to their target pathogen, guided by the existing knowledge on phage biology and the mechanisms of phage infection. The second phase involves designing appropriate models, both laboratory-based and clinical-based, to implement phage therapy protocols. Lastly, establishing regulatory guidelines for standardized phage therapy will be the peak phase of the revolution, which could hopefully pave the way to create appropriate regulations for phage therapy in SEA.
Phase One: Extensive Phage Isolation and Characterization
Starting the phage revolution entails mastery of phage isolation and characterization. As discussed earlier, isolation and characterization of phages involve determining their morphology, lytic activity, specificity, generation time, and adsorption rates using traditionally accepted methods. These methods remain to be useful but present certain limitations. We summarized these limitations in Supplementary Table 1, but a thorough discussion on this topic is presented by Hyman (2019). With the advancements in instrumentation and understanding phage biology, other non-traditional methods can be explored to improve phage isolation and characterization further. For instance, specific samples could be explored in isolating phages with targeted activities. As discussed earlier, phages can be isolated practically in all types of samples. However, to acquire phages with activities against MDR clinical pathogens, we propose exploring the sites heavily contaminated with clinical samples and hospital sewage, as indicated in some studies (Kakasis and Panitsa, 2019). Hospital wastewater systems are the most readily accessible sites for this purpose. Meanwhile, clinical samples, such as blood, stool, and urine, can also be used as the primary source of phages (Letkiewicz et al., 2010; García-Quintanilla et al., 2013). We believe that this approach is more straightforward in isolating specific phages, given that the clinical samples contain the actual bacterial target. Working on the ecological principle that predators are present where the prey is, phages can also be isolated from infectious samples (Chibani-Chennoufi et al., 2004). Despite this proposal, we do not discourage exploring other potential sources of phages for clinical applications. As proposed by Rajandas et al. (2021), other biodiverse habitats, such as the rainforests, which are commonly found in SEA, can be explored as sources of clinically relevant phages. This notion is supported by the isolation of phages with activities against clinical pathogens from farms (Wongsuntornpoj et al., 2014; Sellvam et al., 2018), freshwater (Al-Fendi et al., 2014; Wangkahad et al., 2015), marine environment (Guang-Han et al., 2016), and soil (Shan et al., 2014; Withatanung et al., 2016) in SEA. There is now also increasing availability of widely studied phages with known activities against clinical infections. These phages are curated or produced by several phage organizations as listed in Phage Directory (https://phage.directory/orgs, accessed on 20 December 2021) and The Bacteriophage Ecology Group (http://companies.phage.org/, accessed on 20 December 2021). To promote access to these phages, a mechanism to collaborate with these external phage resources should be established in SEA.
With the advancement in molecular biology, additional phage characterization steps before therapeutic use are now possible. Some scientists suggest structural protein analysis for each phage using sodium dodecyl sulfate polyacrylamide gel electrophoresis (SDS-PAGE), although without suggested practical reason (Kwiatek et al., 2015). Phage protein profiling can aid in phage classification, bioengineering, and identifying potential immune reactions; however, more studies are needed to establish these applications. With the movement to pursue genome-based phage classification (Turner et al., 2021), we would also recommend whole-genome sequencing (WGS) for the characterization of newly discovered phages. WGS can be used as a crude screening method for choosing the ideal therapeutic strain. In SEA, novel phages have been screened using WGS for their lysogenic and endotoxin production potentials (Gan et al., 2013; Lal et al., 2016a; Wirjon et al., 2016; Thanh et al., 2020; Dewanggana et al., 2021; Nuidate et al., 2021), which are important properties to avoid in choosing therapeutic phages. WGS can also aid in the more detailed classification and identification of the phages, as also applied in SEA strains with promising therapeutic potentials (Gan et al., 2013; Hoai et al., 2016; Lal et al., 2016a,b; Wirjon et al., 2016; Sellvam et al., 2018; Handoko et al., 2019; Thanh et al., 2020; Tu et al., 2020; Nuidate et al., 2021). Metagenomic sequencing of phages in the prospect samples will be helpful in the initial screening of phage genomes even before the isolation of the actual virions. This strategy has been used in studying phages found in Brunei and Malaysia (Kerfahi et al., 2019).
In terms of preparing the phage formulations, extensive optimization and characterization are also warranted. For phage delivery platforms, different methods can be studied to optimize phage stability and activity. For instance, researchers in Thailand used novel microencapsulation of phage cocktails against S. typhimurium via freeze-drying (Petsong et al., 2021). The researchers also showed that microencapsulated phages are more stable and elicit similar efficacy against the target bacteria in an in vitro surface contamination experiment (Petsong et al., 2019). To deliver phages via systemic routes, one should consider phage clearance by the immune system. In an in vivo study in Malaysia, an intraperitoneal phage administration was found to be effective in reducing B. pseudomallei infection in mice (Guang-Han et al., 2016). Although the formulation appears tolerable among mice, the appropriate concentration to be used and the immune interaction has yet to be formally described, more so if administered to humans. Scientists recommended optimizing the phage titer in preparations for every strain and target (Górski et al., 2015). However, the kinetics of phages in human peripheral blood is still not established (Schooley et al., 2017). We believe it will be beneficial to consider a personalized pharmacokinetics study of the specific phages for systemic infections. As different patients may have different rates of phage clearance, trials of multiple phage dosages may be necessary (Lin et al., 2017). As mentioned earlier, an appropriate phagogram profiling should also be conducted for each new phage. The phagogram profiling should be done on all potential strains of the target pathogens.
In addition to understanding phage formulation components and qualities, phage banks should also be established in SEA. Although university-based phage collections are present in the region as described earlier, the phage revolution could benefit from maintained and monitored phage repositories that could act as the central source of viruses for therapy. Like any other biobank, the creation and maintenance of phage banks require equipment and process investments. Although they are not widespread, ultralow-temperature freezers (−80°C) are already present in SEA and could serve as a starting point for the long-term storage of promising specimens. Otherwise, efficient preservation methods, such as the use of pH-neutral solutions with low ionic contents, or lyophilization with sugar stabilizers can also be explored for long-term storage with less equipment requirements (Duyvejonck et al., 2021). The quality assurance systems and protocols also need further attention; these are currently non-existent for the biobanking of phages in the region. For instance, the proper delineation and characterization of the banked phage (master seed lot), phage products (working seed lots), and therapy-ready preparations need to be considered (Pirnay et al., 2015; Pelfrene et al., 2016).
Phase Two: Laboratory- and Clinic-Based Phage Therapy Applications
The second revolutionary phase entails the appropriate modeling and implementation of phage therapy. Modern applications of phage therapy not only include the traditional use of phage formulations but also the use of combination therapies, phage products, and other phage-related interventions. Krylov and colleagues differentiate these modern applications into three generations of phage therapy products (Krylov et al., 2015). In our current review, we enriched the previous definitions by referring to the first-generation phage therapy as the traditional phage-based therapy, the second-generation as the use of phage-derived biologics and combination therapy, and the third-generation as the use of bioengineered phages. We summarized these applications in Table 3.
The first-generation phage therapy is the most studied. Traditionally, phage therapy can be done using a monoculture of a single specific phage against uncomplicated infections. However, as scientists began to address complex infections, they considered the use of phage cocktails. Phage cocktails refer to the combination of various phage strains or clones in a single preparation (Burrowes et al., 2011). In SEA, cocktails have been used for in vitro and in vivo therapeutic applications. For instance, Phothaworn et al. (2020) used a two-component phage cocktail (SE-W109 and ST-W77) to increase the efficacy and breadth of Salmonella serovars killed by the formulation. Similarly, Dela Cruz-Papa et al. (2017) used a two-component phage cocktail (UP87, B614) from the Philippines to increase the efficacy of A. hydrophila clearance in Oreochromis niloticus compared to monotherapy. These properties could be beneficial in treating complex infections caused by different bacterial strains. Others indicate that phage cocktails may help prevent or combat phage resistance among the targets. In a recent study by Nale et al. (2021), a three-component cocktail (with the same two phages as used previously by Phothaworn et al., 2020) exhibited effective clearance of phage-resistant Salmonella, in vitro, possibly due to complementary activities of the phages in the formulation. This property could further be explored in justifying the utility of phages for long-term therapeutic applications without worrying about phage resistance. Banking on the high specificity of phage cocktails, it can be customized based on the pathogen profile of the infection from patient sample cultures (Lin et al., 2017; Furfaro et al., 2018). Logistically, phage cocktail preparations may be more costly and time-consuming since the characteristics (i.e., life cycle) and properties (i.e., stability in preparations and in the human body) of the phage must be matched and complementary with each other (Cairns and Payne, 2008; Krylov et al., 2015; Lin et al., 2017). This characterization is vital to ensure the component phages’ orchestrated modes of action (Cairns and Payne, 2008).
Second-generation phage therapy involves the use of phage-derived products, such as lytic enzymes. Phages use lytic enzymes to hydrolyze the cell wall of their host bacteria, allowing for the release of viral progenies. The enzymes, including holins and endolysins, are possible phage-based pharmaceuticals in the future (Lin et al., 2017). In particular, these proteins are seen as potential antimicrobials because of their low-dosage potency and high target specificity similar to phages. Mass production of lytic enzymes is also easier using traditional recombinant techniques (Lin et al., 2017). In recent years, engineered lytic enzymes have been explored to induce highly specific or broad-spectrum cell wall cleavage. In Singapore, chimeric lysins composed of different segments from LysEF-P10 (targets Enterococcus faecalis) and PlyV12 (targets Streptococcus sp., Staphylococcus sp., and Enterococcus sp.) have been successfully engineered. The researchers showed that the engineered lysin called P10N-V12C (with cell wall-binding domain of PlyV12) have both a broader lytic activity against enterococci, streptococci, and staphylococci, and a specific activity against E. faecalis, in vitro (Binte Muhammad Jai et al., 2020). In the future, the actual application of lytic enzymes to treat infection warrants additional in vitro and in vivo studies.
Some researchers believe that antibiotic and phage activities do not interfere with each other because of their different modes of action. Antibiotic resistance may not affect phage therapy efficacy (Zhang et al., 2018). One hypothesis is that a more substantial antimicrobial effect can be achieved if clinicians combine antibiotics and phages into one therapeutic regimen (Viertel et al., 2014; Torres-Barceló, 2018). First observed in 2007, researchers reported that a minimal dose of an antibiotic could stimulate the therapeutic activity of phage in E. coli, a phenomenon described as the Phage-Antibiotic Synergy (PAS; Comeau et al., 2007). Scientists in Indonesia investigated PAS in reducing the load of E. coli and Salmonella spp., in vitro. Their results showed that phage фPT1b combined with amoxicillin and tetracycline at specific ratios successfully increased the lysis of different E. coli strains. These findings indicate a reduced level of resistance to the antibiotics (Narulita et al., 2020). Similar observations have been reported with the combination of phages ϕSZIP1, ϕSZIP2, and ϕSZUT plus cefadroxil against Salmonella sp. Escherichia sp. and S. aureus (Iqbal et al., 2020) and with phage P22 plus ciprofloxacin against S. typhimurium (Petsong et al., 2018). Scientists hypothesized that the initial antibiotic dose elongates the bacteria, making it easier for phages to bind to them due to the increased surface area of the pathogen (Torres-Barceló, 2018). Using this “combination therapy,” the lifespan of exhausted, early generation antibiotics can be prolonged since only its initial effects on the pathogens (not necessarily the bactericidal activity) are needed to achieve the therapeutic effect desired (Iqbal et al., 2020; Narulita et al., 2020). In addition, it could help increase the activity of the antibiotics against polymicrobial interactions like biofilms, although no investigations in SEA have been made to confirm this activity. The actual clinical performance of combined therapy using antibiotics and phages has yet to be investigated in SEA.
The genetic characteristics of phages make them ideal for bioengineering, eventually giving rise to third-generation phage therapies. The phage display technique involves fusing antibody variants or peptides to proteins of the phage coat proteins; iterative rounds of screening then enhance the phage coat’s affinity for target (Criscuolo et al., 2017). DotBio, a start-up biotechnology company in Singapore, utilizes the phage display technique to produce DotBody technology proposed to be used in multi-functional therapies (https://www.dotbio.com/en/technology, accessed 20 December 2021). Meanwhile, scientists from SEA reported bioengineered phages with applications as vectors for selective delivery of transgenes in mammalian cells via phage surface modification (Namdee et al., 2018; Chongchai et al., 2021) and dual tumor targeting by hybridization of an adeno-associated virus with the phage capsid (Przystal et al., 2019). However, no specific modifications have been done to create phages specifically targeting clinically relevant bacteria. Hence, although scientists in SEA engineer phages for clinical applications, they still have to explore engineered phages in combating MDR organisms. Among the avenues that can be explored in the region is the genetic programming of phages to deliver CRISPR/Cas system to the target multidrug-resistant bacteria. This technique can be useful in disrupting the expression of antibiotic resistance genes of the host, making them more susceptible to antibiotics, and preventing the spread of antibiotic resistance genes (Lin et al., 2017). Aside from CRISPR/Cas, phages can also be engineered to kill the host by delivering other lethal genes encoding for lytic enzymes, such as endonucleases, lytic enzymes, and toxins (Viertel et al., 2014). Finally, a whole phage particle can also be constructed, eliminating the need to isolate and purify bacteriophages. These “designer phages” can be used to incorporate all positive traits of bacteriophage for therapeutic use. Constructing designer phages is still an uncharted niche in SEA.
To address the need for rigorous testing of phages before clinical use, extensive laboratory testing for safety and efficacy is necessary (Nale and Clokie, 2021). Laboratory testing of phages should be conducted at three levels – in vitro, ex vivo, and in vivo (Supplementary Figure 4). For in vitro testing, the characterization of phages and optimization of phage formulations should be given priority as described in the previous section of this review. This level of testing should also include the production of phage formulations that will be used in the succeeding testing stages. We believe that the first level of testing phages will not be a problem in SEA since the academic institutions and existing phage laboratories regularly conduct phage testing and characterization. However, we propose to develop standard laboratory protocols for the use of different phage laboratories to ensure that the manufacturing process can be standardized, monitored, and regulated in the future. These protocols should also follow the current GMP principles (Moelling et al., 2018). The second and third levels of testing will be more challenging since it involves using live cell cultures, tissue models, and live animals. Researchers recently used human cell lines in studying the dynamics and interaction of phages and mammalian cells infected with the target bacteria (Møller-Olsen et al., 2018; Shan et al., 2018). However, a more sophisticated method of testing the efficacy of phages is to mimic the human organ environment (ex vivo) affected by the bacteria by using infection models. A recent study reported this approach to infer that an enterococci phage cocktail is effective in a collagen wound infection model (Melo et al., 2019). Another ex vivo approach used controlled and maintained batch fermentation setups to simulate the complex internal environment of the animals (or humans) when studying phages to be administered internally (Rivas et al., 2010; Nale and Clokie, 2021). Ex vivo approaches in studying phage efficacy and safety have yet to be done in SEA. The use of animal models (in vivo) in testing phage efficacy remains an accepted method in the scientific community. Animal models ensure that proper phage delivery methods in living systems and immune response dynamics will be considered when phage therapy is administered (Nale and Clokie, 2021). The choice of animal is critical in ensuring that the human infection pathogenesis and environment can be mimicked accurately. As discussed earlier, in vivo phage therapy studies have been conducted in SEA, but the transition from doing pre-clinical to clinical research in phages has yet to be done.
The past five years saw the advent of three-dimensional cellular constructs mirroring the functions of human tissues and organs (a general term called tissue engineering and regenerative medicine or TERM) in SEA, primarily by groups in Singapore (Han et al., 2020). With TERM, the option of using bioprinted infection models for phage therapy arises. Bioprinting refers to fabricating three-dimensional tissue constructs using biomaterials and living cells (Gungor-Ozkerim et al., 2018). We believe that exploring this option would be beneficial in a personalized therapeutic approach by providing an avenue for laboratories to test the dynamics and efficacy of the phage formulations in patient-derived cells bioprinted to form tissues or organoids and infected with a clinical isolate from the same patient. Using this approach, researchers may reduce the amount of animal testing required, while the ex vivo environment may reflect the actual infection environment in humans more accurately than in animal models. In addition, patient cells and patient-derived bacterial pathogens will be used for testing. We believe that this approach is also complementary to the refined in vivo approach (i.e., use of invertebrate models), as suggested in other studies (Nale et al., 2015, 2021; Brix et al., 2020).
Phase Three: Adaptive Regulatory Mechanisms
The third phase of the phage revolution involves creating standards and guidelines for the therapeutic use of phages. Currently, regulatory agencies in most parts of the world, including SEA, have not officially approved any phage-based products for use in humans. Experts attributed this situation to the fact that no standardized definitions, regulations, and guidelines are available for phage formulations (Wittebole et al., 2014; Krylov et al., 2015; Morozova et al., 2018). In addition, other regulatory standards in the SEA and gaps for implementation have been observed, potentially affecting the progress of phage-based therapeutics as an accepted therapeutic option (Table 4). Although combating antimicrobial resistance is among the top priorities of the ASEAN health cluster for 2016–2020, phage therapy is not among the strategies listed (Association of Southeast Asian Nation, 2021). Given that there are no formal regulatory discussions on phage therapy applications, we hope that this paper could start a dialogue between the different stakeholders in the region. The initial movement towards this goal has been started by the implementation of the inaugural symposium on phage and phage-derived technologies in Singapore last December 2021, organized by Cellexus. Other activities, such as the first Virtual SEA Phage Workshop to be administered by Phages for Global Health in 2022 and the Protein Engineering and Phage Display Conference to be launched in Malaysia in 2023, are also on the way. We are optimistic that additional regional activities towards phage use and appreciation can be done in the next few years once discussions continue to progress.
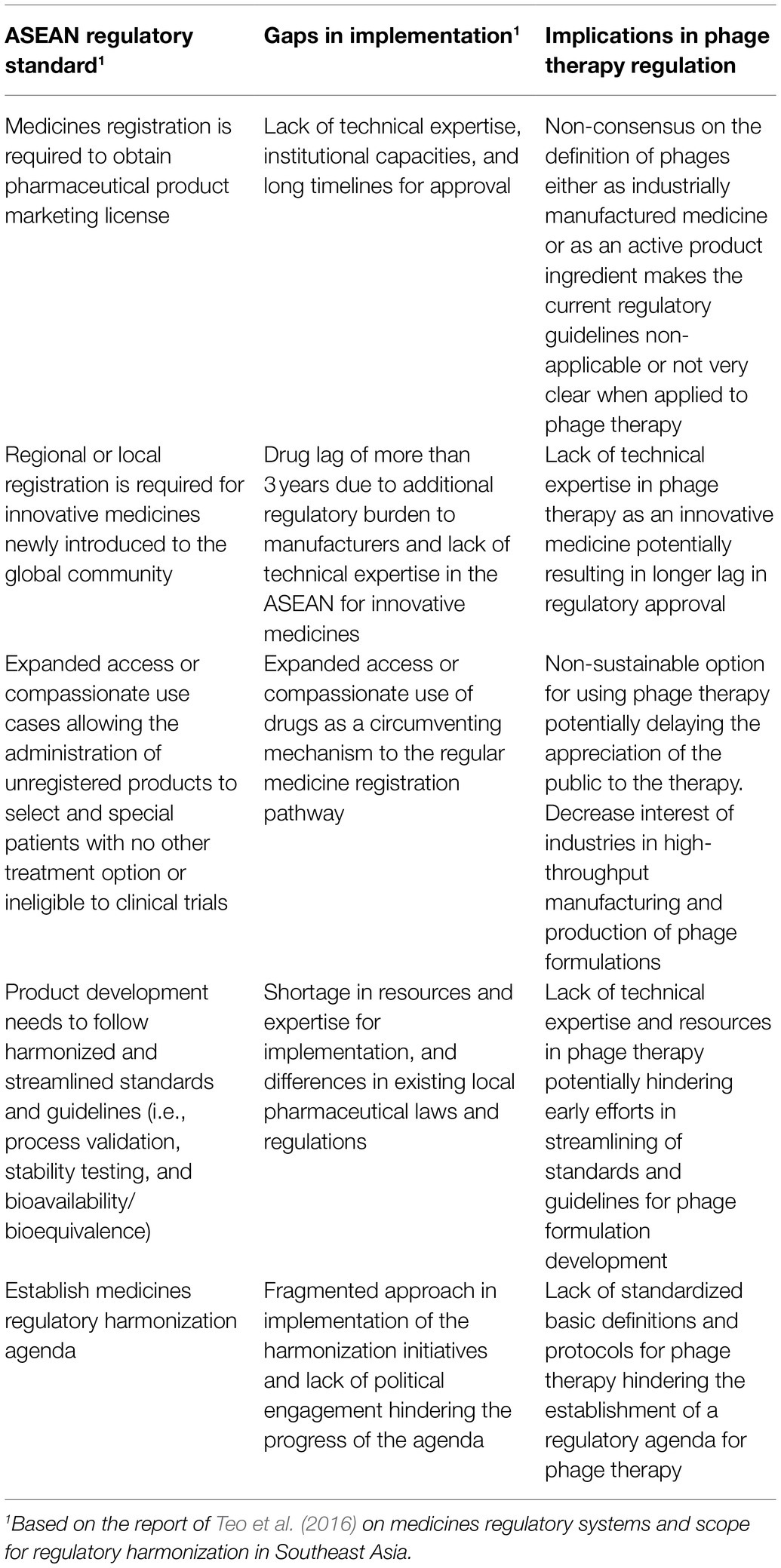
Table 4. Existing regulatory standards with potential implications in phage therapy implementation in SEA.
Despite the current situation in SEA, global regulatory agencies like the US-Food and Drug Administration (US-FDA) and the European Medicines Agency (EMA) provide avenues for the compassionate use of phages as expanded access investigational (i.e., unregulated) new medicines. In these cases, phage formulations can be administered to patients on a per case basis, but the actual scheme of facilitating compassionate phage therapy remains highly variable (McCallin et al., 2019). For patients with financial resources and capacity to travel, phage therapy can also be accessed by traveling to countries where phage therapy is an approved therapeutic option (i.e., Georgia). It is important to note that the regulatory agencies in these regions consider phages as industrially manufactured medicines. Therefore, phages can be treated like traditional drugs, which should follow GMP standards for manufacturing, undergo efficacy testing in clinical trials, and obtain marketing clearance (Brives and Pourraz, 2020). In ASEAN countries, expanded access or compassionate use of unregistered treatment options, including phage therapy, has also been a viable option (Teo et al., 2016). ASEAN countries reported high rates of compassionate use of unregistered medicines, but the use of phage therapy even in critical cases has not been reported. Although compassionate use exists as a safety net, we believe that this workaround is not a sustainable regulatory solution in the long run because: (1) it limits the use of phage therapy for special or emergency cases only, and (2) it creates an impression that phage therapy is only a last-line treatment option, preventing progression in terms of acceptability to the pharmaceutical industries and the general public.
Pirnay et al. (2018) described a magistral approach as a model of regulating phage therapy, where phages are considered active product ingredients instead of industrially manufactured medicines. This approach, currently applied in Belgium, involves the collaboration of phage formulation manufacturers (can be individual hospital pharmacies) and Belgian-Approved Laboratories in ensuring the production of high-quality phages for human use. In this approach, individual hospitals can access phages from biobanks that underwent quality control measures by the Belgian-Approved Laboratories (Moelling et al., 2018). The manufacturers have leeway on the actual production of the phage formulation, as long as it follows the standards of current GMP or the like. This attribute opens the possibility of personalized phage formulations for individual patient management. The Belgian-Approved Laboratories serve as the quality control body that assesses and certifies the constitution of phages before they can be given to the manufacturers for magistral preparations. In SEA, the magistral approach can be considered as soon as the following requirements are satisfied: (1) establishment of well-maintained and monitored phage banks (which will be the source of phages or active pharmaceutical ingredient), (2) appointment of “ASEAN-Approved Laboratories,” and (3) training of pharmacies and independent manufacturers in producing magistral preparations of phages following GMP. For the first requirement, existing phage banks in academic institutions could be tapped, or a central phage biobank could be established, accessible to SEA countries. For the second requirement, each ASEAN member could delegate existing public agencies or private institutions with capabilities to conduct quality control testing for phages. Virology institutes with access to advanced phage characterization equipment like electron microscopes and sequencing platforms would be ideal for this purpose. For the last requirement, hospitals and interested manufacturers should be trained and certified for GMP standards and phage formulation protocols. A consortium of experts in the SEA could act as a preliminary certifying body for this purpose.
Given the current regulatory hurdles, one thing is clear: for the phage revolution to proceed, a more adaptable regulatory pipeline must be pursued in SEA (Jennes et al., 2017; Lin et al., 2017). Independent international organizations, such as Phages for Human Applications Group Europe (P.H.A.G.E), aim to do this by getting support from phage biologists, physicians, and policymakers in recognizing that phage therapy is a valid and promising option for treating bacterial infections in humans (http://www.p-h-a-g-e.org/, accessed on 20 December 2021). A similar organization or consortium can be made in the SEA to educate stakeholders, organize scientific fora, and exchange technical and regulatory experience on phage therapy applications in the region. Lastly, a research and biotechnology business model can be adapted to promote the sustainability of a phage revolution in SEA. Although phage therapy has weak support in terms of patent and market distribution, biotechnology companies and healthcare facilities could benefit from the phage industry by serving as niche or specialty providers (Fauconnier et al., 2020). These companies could use the phage specialization as leverage in creating spinoffs for phage therapy in SEA. To form these initiatives, the ASEAN could tap the WHO for resource capacity-building, and resource mobilization, especially for member states considered as low- to middle-income countries (LMICs; Fauconnier et al., 2020). The current business environment is not ideal for pharmaceutical companies to support phage formulations’ widespread production and marketing (Verbeken et al., 2014). Despite this drawbacks, we are optimistic that the utilization and ultimately market utilization of phage formulations and phage therapy will increase over the following years, with an estimated compound annual growth rate of 8.1% from 2021 to 2028 (Accurize Market Research, https://www.accurizemarketresearch.com/report/global-phage-therapy-market/, accessed on 15 July 2021). As of this writing, the Phage Directory (https://phage.directory/orgs, accessed 20 December 2021) and The Bacteriophage Ecology Group (http://companies.phage.org/, accessed on 20 December 2021) listed up to 60 biotechnology companies specializing in phages, none of which are SEA-based. This situation creates an opportunity for the SEA countries to establish their own regional spinoff company specializing in phages and phage therapy.
Final Thoughts on Phage Revolution
Aside from being a global biodiversity hotspot, SEA can also be considered a global hotspot for antimicrobial resistance (Zellweger et al., 2017). Hence, the region must have effective therapeutic strategies to combat the growing resistance against antibiotics in the clinical setting. We view phage therapy as one of the most viable options in achieving this goal, given the opportunities present in the region. The historical data and the strategies we presented in this review point to the consideration of three things that would potentially spark the upcoming phage revolution in SEA: (1) increase the interest and knowledge of scientists in SEA about phages, (2) invest in virology facilities and systems, and (3) follow adaptable regulatory pipeline that will allow the use of phage therapy in clinics without hampering the quality of phage formulations.
The involvement of dedicated scientists is vital because they will become the pioneers of phage therapy in the region. They will also serve as the foundation in applying phage therapy knowledge in the clinics. Without these experts, the call for phage therapy in the region will be futile. To increase the interest in the field, the promotion of phage research by regional experts is critical. Creating a phage consortium or organization in SEA can help achieve this goal. As discussed in this review, the consortium could act as a technical expert for ASEAN-Approved Laboratories, a source of continuing education for the region, and provide an avenue where exchanges of ideas and practices about phage therapy could be discussed. As more experts in phages develop, we expect an increase in the number of scientific publications covering the understanding of phage biology, systematics, and anti-MDR organism potential, and eventually the rise of clinical trials focusing on the clinical applications of phages against MDR organisms. We also expect increased capacity-building activities to hone complementary skills for phage therapy implementation, such as electron microscopy, WGS, phage formulation techniques, phage administration, and bioprinting. The inaugural symposium on phage and phage-derived technologies in Singapore (2021), and the first virtual SEA phage workshop by Phages for Global Health in Malaysia (2022) are good starting points in increasing scientific interest on phage in the region. Meanwhile, to promote phage therapy appreciation among the public, citizen science programs that focus on accessible and appreciable activities involving phages can be done in schools following the model of the Science Education Alliance-Phage Hunters Advancing Genomics and Evolutionary Science (SEA-PHAGE, https://seaphages.org/, accessed last 20 December 2021). With educational activities, the concept of phage and phage therapy can be integrated within the public knowledge, hopefully making the phage revolution easier to implement and encourage.
As with all research-based endeavors, facilities and protocols are also essential to spark the phage revolution in SEA. But this aspect may not be an added economic burden to the region. As we have mentioned in this review, the creation of critical phage facilities like phage banks and phage testing centers could stem from existing facilities like the university-based phage collections or existing virological institutes, respectively. However, we believe that establishing a dedicated facility accessible to the SEA region would be more beneficial in creating a specific niche that could address concerns about phage therapy. The facility would be critical later on when regulations and standards on phage therapy have been created.
Lastly, following an adaptable model for the regulation and standardization of phage therapy would be a critical undertaking for all the countries within the ASEAN. We believe that the existing collaborations in the region regarding pharmaceutical and biological products regulations are vital starting points for creating adaptable guidelines applicable to SEA. These existing collaborations can be revisited and modified using the regulatory models from other countries; after these changes, regulatory agencies can accommodate more investigational and personalized therapeutic options like phage therapy. We accept that the regulatory aspect will take time. Still, the sooner the region starts its discussion on the matter, the sooner decision-makers can implement phage therapy for MDR organism-infected patients, who may have no other hope of survival other than exploring phage therapy.
In summary, a phage revolution that targets MDR organisms in SEA is an ambitious yet beneficial concept that should be opened to the scientists, clinical decision-makers, regulators, administrators, and other stakeholders of the region. In this review, we presented the details of preparing for this promising endeavor, starting from the basic understanding of the phages, the knowledge of phage therapy requirements, its historical use in the world and region, and the strategies to move forward. We hope that this review will open avenues for scientific and policy-based discussions on phage therapy and eventually lead the way to its fullest potential in combating MDR organisms in SEA.
Author Contributions
MCa and RD conceptualized the topic and contents of the manuscript. MCa wrote and formatted the texts. MCr helped in formatting the texts. DdC-P, RR, and RD reviewed and provided expert opinion on the manuscript. All authors read and approved the final version of the manuscript.
Funding
The authors received publication support from The Medical City.
Conflict of Interest
The authors declare that the research was conducted in the absence of any commercial or financial relationships that could be construed as a potential conflict of interest.
Publisher’s Note
All claims expressed in this article are solely those of the authors and do not necessarily represent those of their affiliated organizations, or those of the publisher, the editors and the reviewers. Any product that may be evaluated in this article, or claim that may be made by its manufacturer, is not guaranteed or endorsed by the publisher.
Acknowledgments
The authors acknowledge the support of the Clinical and Translational Research Institute and Eugenio Jose F. Ramos of The Medical City Ortigas, and Marvin Belen for their encouragement in the completion of this review article.
Supplementary Material
The Supplementary Material for this article can be found online at: https://www.frontiersin.org/articles/10.3389/fmicb.2022.820572/full#supplementary-material
References
Abdulamir, A. S., Jassim, S. A., Hafidh, R. R., and Bakar, F. A. (2015). The potential of bacteriophage cocktail in eliminating methicillin-resistant Staphylococcus aureus biofilms in terms of different extracellular matrices expressed by PIA, ciaA-D and FnBPA genes. Ann. Clin. Microbiol. Antimicrob. 14:49. doi: 10.1186/s12941-015-0106-0
Adamu Ahmad, K., Sabo Mohammed, A., and Abas, F. (2016). Chitosan nanoparticles as carriers for the delivery of ΦKAZ14 bacteriophage for oral biological control of colibacillosis in chickens. Molecules 21:256. doi: 10.3390/molecules21030256
Ahmad, K. A., Mohanmmed, A. S., Abas, F., and Chin, S. C. (2015). T4-like coliphage ΦKAZ14 virulent to pathogenic and extended spectrum β-lactamase-producing Escherichia coli of poultry origin. Virol. Sin. 30, 73–75. doi: 10.1007/s12250-014-3541-8
Aklilu, E., and Raman, K. (2020). MCR-1 gene encoded colistin-resistant Escherichia coli in raw chicken meat and bean sprouts in Malaysia. Int. J. Microbiol. 2020:8853582. doi: 10.1155/2020/8853582
Al-Fendi, A., Shueb, R. H., Ravichandran, M., and Yean, C. Y. (2014). Isolation and characterization of lytic vibriophage against Vibrio cholerae O1 from environmental water samples in Kelantan, Malaysia. J. Basic Microbiol. 54, 1036–1043. doi: 10.1002/jobm.201300458
Asavarut, P., and Hajitou, A. (2014). The phage revolution against antibiotic resistance. Lancet Infect. Dis. 14:686. doi: 10.1016/S1473-3099(14)70867-9
Assafiri, O., Song, A. A. L., Tan, G. H., Hanish, I., Hashim, A. M., and Yusoff, K. (2021). Klebsiella virus UPM2146 lyses multiple drug-resistant Klebsiella pneumoniae in vitro and in vivo. PLoS One 16:e0245354. doi: 10.1371/journal.pone.0245354
Association of Southeast Asian Nation (2021). ASEAN Health Cluster 2 Work Programme for 2016 to 2020. Available at: https://asean.org/wp-content/uploads/2021/01/ASEAN-Health-Cluster-2-Work-Programme_FINAL-ENDORSED.pdf (Accessed December 20, 2021).
Barbu, E. M., Cady, K. C., and Hubby, B. (2016). Phage therapy in the era of synthetic biology. Cold Spring Harb. Perspect. Biol. 8:a023879. doi: 10.1101/cshperspect.a023879
Berglund, B., Hoang, N. T. B., Tärnberg, M., Le, N. K., Svartström, O., Khu, D. T. K., et al. (2018). Insertion sequence transpositions and point mutations in mgrB causing colistin resistance in a clinical strain of carbapenem-resistant Klebsiella pneumoniae from Vietnam. Int. J. Antimicrob. Agents 51, 789–793. doi: 10.1016/j.ijantimicag.2017.11.012
Bhetwal, A., Maharjan, A., Shakya, S., Satyal, D., Ghimire, S., Khanal, P. R., et al. (2017). Isolation of potential phages against multidrug-resistant bacterial isolates: promising agents in the rivers of Kathmandu, Nepal. Biomed. Res. Int. 2017:3723254. doi: 10.1155/2017/3723254
Biedenbach, D. J., Bouchillon, S. K., Hoban, D. J., Hackel, M., Phuong, D. M., Nga, T. T. T., et al. (2014). Antimicrobial susceptibility and extended-spectrum beta-lactamase rates in aerobic gram-negative bacteria causing intra-abdominal infections in Vietnam: report from the study for monitoring antimicrobial resistance trends (SMART 2009–2011). Diagn. Microbiol. Infect. Dis. 79, 463–467. doi: 10.1016/j.diagmicrobio.2014.05.009
Biglari, S., Alfizah, H., Ramliza, R., and Rahman, M. M. (2015). Molecular characterization of carbapenemase and cephalosporinase genes among clinical isolates of Acinetobacter baumannii in a tertiary medical Centre in Malaysia. J. Med. Microbiol. 64, 53–58. doi: 10.1099/jmm.0.082263-0
Biglari, S., Hanafiah, A., Mohd Puzi, S., Ramli, R., Rahman, M., and Lopes, B. S. (2017). Antimicrobial resistance mechanisms and genetic diversity of multidrug-resistant Acinetobacter baumannii isolated from a teaching hospital in Malaysia. Microb. Drug Resist. 23, 545–555. doi: 10.1089/mdr.2016.0130
Binte Muhammad Jai, H. S., Dam, L. C., Tay, L. S., Koh, J., Loo, H. L., Kline, K. A., et al. (2020). Engineered lysins with customized lytic activities against enterococci and staphylococci. Front. Microbiol. 11:574739. doi: 10.3389/fmicb.2020.574739
Blackwell, G. A., Holt, K. E., Bentley, S. D., Hsu, L. Y., and Hall, R. M. (2017). Variants of AbGRI3 carrying the armA gene in extensively antibiotic-resistant Acinetobacter baumannii from Singapore. J. Antimicrob. Chemother. 72, 1031–1039. doi: 10.1093/jac/dkw542
Brives, C., and Pourraz, J. (2020). Phage therapy as a potential solution in the fight against AMR: obstacles and possible futures. Palgrave Commun. 6, 1–11. doi: 10.1057/s41599-020-0478-4
Brix, A., Cafora, M., Aureli, M., and Pistocchi, A. (2020). Animal models to translate phage therapy to human medicine. Int. J. Mol. Sci. 21:3715. doi: 10.3390/ijms21103715
Broecker, F., Kube, M., Klumpp, J., Schuppler, M., Biedermann, L., Hecht, J., et al. (2013). Analysis of the intestinal microbiome of a recovered Clostridium difficile patient after fecal transplantation. Digestion 88, 243–251. doi: 10.1159/000355955
Burrowes, B., Harper, D. R., Anderson, J., McConville, M., and Enright, M. C. (2011). Bacteriophage therapy: potential uses in the control of antibiotic-resistant pathogens. Expert Rev. Anti-Infect. Ther. 9, 775–785. doi: 10.1586/eri.11.90
Cairns, B. J., and Payne, R. J. (2008). Bacteriophage therapy and the mutant selection window. Antimicrob. Agents Chemother. 52, 4344–4350. doi: 10.1128/AAC.00574-08
Cao, F., Wang, X., Wang, L., Li, Z., Che, J., Wang, L., et al. (2015). Evaluation of the efficacy of a bacteriophage in the treatment of pneumonia induced by multidrug resistance Klebsiella pneumoniae in mice. Biomed. Res. Int. 2015:752930. doi: 10.1155/2015/752930
Casey, E., Van Sinderen, D., and Mahony, J. (2018). In vitro characteristics of phages to guide ‘real life’ phage therapy suitability. Viruses 10:163. doi: 10.3390/v10040163
Center for Disease Dynamics, Economics and Policy (2021). Resistance Map. Available at: https://resistancemap.cddep.org/AntibioticResistance.php (Accessed December 20, 2021).
Chanishvili, N. (2012). Phage therapy—history from Twort and d'Herelle through soviet experience to current approaches. Adv. Virus Res. 83, 3–40. doi: 10.1016/B978-0-12-394438-2.00001-3
Chibani-Chennoufi, S., Bruttin, A., Dillmann, M. L., and Brüssow, H. (2004). Phage-host interaction: an ecological perspective. J. Bacteriol. 186, 3677–3686. doi: 10.1128/JB.186.12.3677-3686.2004
Chongchai, A., Waramit, S., Suwan, K., Al-Bahrani, M., Udomruk, S., Phitak, T., et al. (2021). Bacteriophage-mediated therapy of chondrosarcoma by selective delivery of the tumor necrosis factor alpha (TNFα) gene. FASEB J. 35:e21487. doi: 10.1096/fj.202002539R
Chou, A., Roa, M., Evangelista, M. A., Sulit, A. K., Lagamayo, E., Torres, B. C., et al. (2016). Emergence of Klebsiella pneumoniae ST273 carrying Bla NDM-7 and ST656 carrying Bla NDM-1 in Manila, Philippines. Microb. Drug Resist. 22, 585–588. doi: 10.1089/mdr.2015.0205
Chua, A. Q., Verma, M., Hsu, L. Y., and Legido-Quigley, H. (2021). An analysis of national action plans on antimicrobial resistance in Southeast Asia using a governance framework approach. Lancet Reg. Health West. Pac. 7:100084. doi: 10.1016/j.lanwpc.2020.100084
Chyerochana, N., Kongprajug, A., Somnark, P., Kamphaengthong, P. L., Mongkolsuk, S., and Sirikanchana, K. (2020). Distributions of enterococci and human-specific bacteriophages of enterococci in a tropical watershed. Int. J. Hyg. Environ. Health 226:113482. doi: 10.1016/j.ijheh.2020.113482
Cieplak, T., Soffer, N., Sulakvelidze, A., and Nielsen, D. S. (2018). A bacteriophage cocktail targeting Escherichia coli reduces E. coli in simulated gut conditions, while preserving a non-targeted representative commensal normal microbiota. Gut Microbes 9, 391–399. doi: 10.1080/19490976.2018.1447291
Comeau, A. M., Tétart, F., Trojet, S. N., Prere, M. F., and Krisch, H. M. (2007). Phage-antibiotic synergy (PAS): β-lactam and quinolone antibiotics stimulate virulent phage growth. PLoS One 2:e799. doi: 10.1371/journal.pone.0000799
Cornista, J. C., Martin, J. L., Monzales, J. M., and Balolong, M. P. (2019). Screening of bacteriophages against different genotypes of extended spectrum b-lactamase (ESBL)-producing Klebsiella pneumoniae isolated from five hospitals in Cavite and metro Manila, Philippines. Philipp. J. Health Res. Dev. 23, 25–37.
Criscuolo, E., Spadini, S., Lamanna, J., Ferro, M., and Burioni, R. (2017). Bacteriophages and their immunological applications against infectious threats. J. Immunol. Res. 2017:3780697. doi: 10.1155/2017/3780697
Dakheel, K. H., Rahim, R. A., Neela, V. K., Al-Obaidi, J. R., Hun, T. G., Isa, M. N. M., et al. (2019). Genomic analyses of two novel biofilm-degrading methicillin-resistant Staphylococcus aureus phages. BMC Microbiol. 19, 1–23. doi: 10.1186/s12866-019-1484-9
Dang, T. H. O., Xuan, T. T., Duyen, L. T., Le, N. P., and Hoang, H. A. (2021). Protective efficacy of phage PVN02 against haemorrhagic septicaemia in striped catfish Pangasianodon hypophthalmus via oral administration. J. Fish Dis. 44, 1255–1263. doi: 10.1111/jfd.13387
Danis-Wlodarczyk, K., Dąbrowska, K., and Abedon, S. T. (2021). Phage therapy: the pharmacology of antibacterial viruses. Curr. Issues Mol. Biol. 40, 81–164. doi: 10.21775/cimb.040.081
Dela Cruz-Papa, D. M. A., Baquiran, J. M. R., Pineda, C. J., Susi, L. C., and Papa, R. D. S. (2017). Treatment of motile aeromonad septicemia in Nile tilapia (Oreochromis niloticus) using phage cocktail therapy with notes on the isolation and description of a novel phage B614. Philipp. Agric. Sci. 100, 324–331.
Dela Cruz-Papa, D. M. A. D., Candare, C. M. G., Cometa, G. L. S., Gudez, D., Guevara, A. M. I. T., Relova, M. B. T. G., et al. (2014). Aeromonas hydrophila bacteriophage UP87: an alternative to antibiotic treatment for motile aeromonas septicemia in Nile tilapia (Oreochromis niloticus). Philipp. Agric. Sci. 97, 96–101.
Deresinski, S. (2009). Bacteriophage therapy: exploiting smaller fleas. Clin. Infect. Dis. 48, 1096–1101. doi: 10.1086/597405
Dewanggana, M. N., Waturangi, D. E., and Yogiara, (2021). Genomic characterization of bacteriophage BI-EHEC infecting strains of enterohemorrhagic Escherichia coli. BMC Res. Notes 14:459. doi: 10.1186/s13104-021-05881-5
d’Herelle, M. F. (1917). Sur un microbe invisible antagonistic des bacilles dysenterique. C. R. Acad. Sci. Paris 165, 373–375.
Drulis-Kawa, Z., Majkowska-Skrobek, G., and Maciejewska, B. (2015). Bacteriophages and phage-derived proteins–application approaches. Curr. Med. Chem. 22, 1757–1773. doi: 10.2174/0929867322666150209152851
Dufour, N., Clermont, O., La Combe, B., Messika, J., Dion, S., Khanna, V., et al. (2016). Bacteriophage LM33_P1, a fast-acting weapon against the pandemic ST131-O25b: H4 Escherichia coli clonal complex. J. Antimicrob. Chemother. 71, 3072–3080. doi: 10.1093/jac/dkw253
Duyvejonck, H., Merabishvili, M., Vaneechoutte, M., de Soir, S., Wright, R., Friman, V. P., et al. (2021). Evaluation of the stability of bacteriophages in different solutions suitable for the production of Magistral preparations in Belgium. Viruses 13:865. doi: 10.3390/v13050865
Eiamphungporn, W., Yainoy, S., Jumderm, C., Tan-Arsuwongkul, R., Tiengrim, S., and Thamlikitkul, V. (2018). Prevalence of the colistin resistance gene mcr-1 in colistin-resistant Escherichia coli and Klebsiella pneumoniae isolated from humans in Thailand. J. Glob. Antimicrob. Resist. 15, 32–35. doi: 10.1016/j.jgar.2018.06.007
El-Shibiny, A., and El-Sahhar, S. (2017). Bacteriophages: the possible solution to treat infections caused by pathogenic bacteria. Can. J. Microbiol. 63, 865–879. doi: 10.1139/cjm-2017-0030
Fauconnier, A., Nagel, T. E., Fauconnier, C., Verbeken, G., De Vos, D., Merabishvili, M., et al. (2020). The unique role that WHO could play in implementing phage therapy to combat the global antibiotic resistance crisis. Front. Microbiol. 11:1982. doi: 10.3389/fmicb.2020.01982
Furfaro, L. L., Payne, M. S., and Chang, B. J. (2018). Bacteriophage therapy: clinical trials and regulatory hurdles. Front. Cell. Infect. Microbiol. 8:376. doi: 10.3389/fcimb.2018.00376
Gan, H. M., Sieo, C. C., Tang, S. G. H., Omar, A. R., and Ho, Y. W. (2013). The complete genome sequence of EC1-UPM, a novel N4-like bacteriophage that infects Escherichia coli O78: K80. Virol. J. 10, 1–8. doi: 10.1186/1743-422X-10-308
García-Quintanilla, M., Pulido, M. R., López-Rojas, R., Pachón, J., and McConnell, M. J. (2013). Emerging therapies for multidrug resistant Acinetobacter baumannii. Trends Microbiol. 21, 157–163. doi: 10.1016/j.tim.2012.12.002
Ghannad, M. S., and Mohammadi, A. (2012). Bacteriophage: time to re-evaluate the potential of phage therapy as a promising agent to control multidrug-resistant bacteria. Iran. J. Basic Med. Sci. 15:693.
Golkar, Z., Bagasra, O., and Pace, D. G. (2014). Bacteriophage therapy: a potential solution for the antibiotic resistance crisis. J. Infect. Dev. Ctries. 8, 129–136. doi: 10.3855/jidc.3573
Górski, A., Dąbrowska, K., Hodyra-Stefaniak, K., Borysowski, J., Międzybrodzki, R., and Weber-Dąbrowska, B. (2015). Phages targeting infected tissues: novel approach to phage therapy. Future Microbiol. 10, 199–204. doi: 10.2217/fmb.14.126
Guang-Han, O., Leang-Chung, C., Vellasamy, K. M., Mariappan, V., Li-Yen, C., and Vadivelu, J. (2016). Experimental phage therapy for Burkholderia pseudomallei infection. PLoS One 11:e0158213. doi: 10.1371/journal.pone.0158213
Gungor-Ozkerim, P. S., Inci, I., Zhang, Y. S., Khademhosseini, A., and Dokmeci, M. R. (2018). Bioinks for 3D bioprinting: an overview. Biomater. Sci. 6, 915–946. doi: 10.1039/C7BM00765E
Hadjadj, L., Baron, S. A., Olaitan, A. O., Morand, S., and Rolain, J. M. (2019). Co-occurrence of variants of mcr-3 and mcr-8 genes in a Klebsiella pneumoniae isolate from Laos. Front. Microbiol. 10:2720. doi: 10.3389/fmicb.2019.02720
Han, F., Wang, J., Ding, L., Hu, Y., Li, W., Yuan, Z., et al. (2020). Tissue engineering and regenerative medicine: achievements, future, and sustainability in Asia. Front. Bioeng. Biotechnol. 8:83. doi: 10.3389/fbioe.2020.00083
Handoko, Y. A., Wardani, A. K., Sutrisno, A., Widjanarko, S. B., Thurgood, T. L., Thompson, D. W., et al. (2019). Genome sequences of two Bacillus phages isolated from Indonesia. Microbiol. Resour. Announc. 8, e01058–e01119. doi: 10.1128/MRA.01058-19
Harper, D. R. (2018). Criteria for selecting suitable infectious diseases for phage therapy. Viruses 10:177. doi: 10.3390/v10040177
Harper, D. R., and Enright, M. C. (2011). Bacteriophages for the treatment of Pseudomonas aeruginosa infections. J. Appl. Microbiol. 111, 1–7. doi: 10.1111/j.1365-2672.2011.05003.x
Hoai, T. D., Mitomi, K., Nishiki, I., and Yoshida, T. (2018). A lytic bacteriophage of the newly emerging rainbow trout pathogen Weissella ceti. Virus Res. 247, 34–39. doi: 10.1016/j.virusres.2018.01.016
Hoai, T. D., Nishiki, I., Fujiwara, A., Yoshida, T., and Nakai, T. (2019). Comparative genomic analysis of three lytic Lactococcus garvieae phages, novel phages with genome architecture linking the 936 phage species of Lactococcus lactis. Mar. Genomics 48:100696. doi: 10.1016/j.margen.2019.100696
Hoai, T. D., Nishiki, I., and Yoshida, T. (2016). Properties and genomic analysis of Lactococcus garvieae lysogenic bacteriophage PLgT-1, a new member of Siphoviridae, with homology to Lactococcus lactis phages. Virus Res. 222, 13–23. doi: 10.1016/j.virusres.2016.05.021
Hoang, A. H., Tran, T. T. X., Le, P. N., and Dang, T. H. O. (2019). Selection of phages to control Aeromonas hydrophila: an infectious agent in striped catfish. Biocontrol Sci. 24, 23–28. doi: 10.4265/bio.24.23
Hoang, H. A., Yen, M. H., Ngoan, V. T., Nga, L. P., and Oanh, D. T. (2018). Virulent bacteriophage of Edwardsiella ictaluri isolated from kidney and liver of striped catfish Pangasianodon hypophthalmus in Vietnam. Dis. Aquat. Org. 132, 49–56. doi: 10.3354/dao03302
Hyman, P. (2019). Phages for phage therapy: isolation, characterization, and host range breadth. Pharmaceuticals (Basel) 12:35. doi: 10.3390/ph12010035
Iqbal, M., Narulita, E., Zahra, F., and Murdiyah, S. (2020). Effect of phage-antibiotic synergism (PAS) in increasing antibiotic inhibition of bacteria caused of foodborne diseases. J. Infect. Dev. Ctries. 14, 488–493. doi: 10.3855/jidc.12094
Jassim, S. A., Abdulamir, A. S., and Abu Bakar, F. (2012). Novel phage-based bio-processing of pathogenic Escherichia coli and its biofilms. World J. Microbiol. Biotechnol. 28, 47–60. doi: 10.1007/s11274-011-0791-6
Jennes, S., Merabishvili, M., Soentjens, P., Pang, K. W., Rose, T., Keersebilck, E., et al. (2017). Use of bacteriophages in the treatment of colistin-only-sensitive Pseudomonas aeruginosa septicaemia in a patient with acute kidney injury: a case report. Crit. Care 21, 1–3. doi: 10.1186/s13054-017-1709-y
Jin, J., Li, Z. J., Wang, S. W., Wang, S. M., Huang, D. H., Li, Y. H., et al. (2012). Isolation and characterization of ZZ1, a novel lytic phage that infects Acinetobacter baumannii clinical isolates. BMC Microbiol. 12, 1–8. doi: 10.1186/1471-2180-12-156
Kaikabo, A. A., AbdulKarim, S. M., and Abas, F. (2017). Evaluation of the efficacy of chitosan nanoparticles loaded ΦKAZ14 bacteriophage in the biological control of colibacillosis in chickens. Poult. Sci. 96, 295–302. doi: 10.3382/ps/pew255
Kakasis, A., and Panitsa, G. (2019). Bacteriophage therapy as an alternative treatment for human infections. A comprehensive review. Int. J. Antimicrob. Agents 53, 16–21. doi: 10.1016/j.ijantimicag.2018.09.004
Karuniawati, A., Saharman, Y. R., and Lestari, D. C. (2013). Detection of carbapenemase encoding genes in Enterobacteriaceae, Pseudomonas aeruginosa, and Acinetobacter baumanii isolated from patients at intensive care unit Cipto Mangunkusumo hospital in 2011. Acta Med. Indones. 45, 101–106.
Kerfahi, D., Tripathi, B. M., Dong, K., Kim, M., Kim, H., Ferry Slik, J. W., et al. (2019). From the high arctic to the equator: do soil metagenomes differ according to our expectations? Microb. Ecol. 77, 168–185. doi: 10.1007/s00248-018-1215-z
Khalid, A., Lin, R. C., and Iredell, J. R. (2021). A phage therapy guide for clinicians and basic scientists: background and highlighting applications for developing countries. Front. Microbiol. 11:599906. doi: 10.3389/fmicb.2020.599906
Khawaja, K. A., Rauf, M., Abbas, Z., and Rehman, S. U. (2016). A virulent phage JHP against Pseudomonas aeruginosa showed infectivity against multiple genera. J. Basic Microbiol. 56, 1090–1097. doi: 10.1002/jobm.201500764
Khuntayaporn, P., Montakantikul, P., Mootsikapun, P., Thamlikitkul, V., and Chomnawang, M. T. (2012). Prevalence and genotypic relatedness of carbapenem resistance among multidrug-resistant P. aeruginosa in tertiary hospitals across Thailand. Ann. Clin. Microbiol. Antimicrob. 11, 1–7. doi: 10.1186/1476-0711-11-25
Khuntayaporn, P., Montakantikul, P., Santanirand, P., Kiratisin, P., and Chomnawang, M. T. (2013). Molecular investigation of carbapenem resistance among multidrug-resistant Pseudomonas aeruginosa isolated clinically in Thailand. Microbiol. Immunol. 57, 170–178. doi: 10.1111/1348-0421.12021
Khuntayaporn, P., Yamprayoonswat, W., Yasawong, M., and Chomnawang, M. T. (2019). Dissemination of carbapenem-resistance among multidrug resistant Pseudomonas aeruginosa carrying metallo-beta-lactamase genes, including the novel blaIMP-65 gene in Thailand. Infect. Chemother. 51, 107–118. doi: 10.3947/ic.2019.51.2.107
Kim, D. H., Choi, J. Y., Kim, H. W., Kim, S. H., Chung, D. R., Peck, K. R., et al. (2013). Spread of carbapenem-resistant Acinetobacter baumannii global clone 2 in Asia and AbaR-type resistance islands. Antimicrob. Agents Chemother. 57, 5239–5246. doi: 10.1128/AAC.00633-13
Koh, T. H., Tan, T. T., Khoo, C. T., Ng, S. Y., Tan, T. Y., Hsu, L. Y., et al. (2012). Acinetobacter calcoaceticus-Acinetobacter baumannii complex species in clinical specimens in Singapore. Epidemiol. Infect. 140, 535–538. doi: 10.1017/S0950268811001129
Krylov, V., Shaburova, O., Pleteneva, E., Krylov, S., Kaplan, A., Burkaltseva, M., et al. (2015). Selection of phages and conditions for the safe phage therapy against Pseudomonas aeruginosa infections. Virol. Sin. 30, 33–44. doi: 10.1007/s12250-014-3546-3
Kwiatek, M., Mizak, L., Parasion, S., Gryko, R., Olender, A., and Niemcewicz, M. (2015). Characterization of five newly isolated bacteriophages active against Pseudomonas aeruginosa clinical strains. Folia Microbiol. 60, 7–14. doi: 10.1007/s12223-014-0333-3
La, M. V., Lee, B., Hong, B. Z., Yah, J. Y., Koo, S. H., Jiang, B., et al. (2019). Prevalence and antibiotic susceptibility of colistin-resistance gene (mcr-1) positive enterobacteriaceae in stool specimens of patients attending a tertiary care hospital in Singapore. Int. J. Infect. Dis. 85, 124–126. doi: 10.1016/j.ijid.2019.05.029
Lal, T. M., Sano, M., Hatai, K., and Ransangan, J. (2016a). Complete genome sequence of a giant Vibrio phage ValKK3 infecting Vibrio alginolyticus. Genom. Data 8, 37–38. doi: 10.1002/jobm.201500611
Lal, T. M., Sano, M., and Ransangan, J. (2016b). Genome characterization of a novel vibriophage VpKK5 (Siphoviridae) specific to fish pathogenic strain of Vibrio parahaemolyticus. J. Basic Microbiol. 56, 872–888. doi: 10.1016/j.gdata.2016.03.002
Lal, T. M., Sano, M., and Ransangan, J. (2017). Isolation and characterization of large marine bacteriophage (Myoviridae), VhKM4 infecting Vibrio harveyi. J. Aquat. Anim. Health 29, 26–30. doi: 10.1080/08997659.2016.1249578
Lau, G. L., Sieo, C. C., Tan, W. S., and Ho, Y. W. (2012). Characteristics of a phage effective for colibacillosis control in poultry. J. Sci. Food Agric. 92, 2657–2663. doi: 10.1002/jsfa.5683
Le, T. S., Nguyen, T. H., Vo, H. P., Doan, V. C., Nguyen, H. L., Tran, M. T., et al. (2018). Protective effects of bacteriophages against Aeromonas hydrophila causing motile Aeromonas septicemia (MAS) in striped catfish. Antibiotics 7:16. doi: 10.3390/antibiotics7010016
Le, T. S., Southgate, P. C., O’Connor, W., Vu, S. V., and Kurtböke, D. İ. (2020). Application of bacteriophages to control Vibrio alginolyticus contamination in oyster (Saccostrea glomerata) larvae. Antibiotics 9:415. doi: 10.3390/antibiotics9070415
Lehman, S. M., Mearns, G., Rankin, D., Cole, R. A., Smrekar, F., Branston, S. D., et al. (2019). Design and preclinical development of a phage product for the treatment of antibiotic-resistant Staphylococcus aureus infections. Viruses 11:88. doi: 10.3390/v11010088
Letkiewicz, S., Międzybrodzki, R., Kłak, M., Jończyk, E., Weber-Dąbrowska, B., and Górski, A. (2010). The perspectives of the application of phage therapy in chronic bacterial prostatitis. FEMS Immunol. Med. Microbiol. 60, 99–112. doi: 10.1111/j.1574-695X.2010.00723.x
Liew, S. M., Rajasekaram, G., Puthucheary, S. D., and Chua, K. H. (2018). Detection of VIM-2-, IMP-1-and NDM-1-producing multidrug-resistant Pseudomonas aeruginosa in Malaysia. J. Glob. Antimicrob. Resist. 13, 271–273. doi: 10.1016/j.jgar.2018.01.026
Lim, W. S., Phang, K. K., Tan, A. H., Li, S. F., and Ow, D. S. (2016). Small colony variants and single nucleotide variations in Pf1 region of PB1 phage-resistant Pseudomonas aeruginosa. Front. Microbiol. 7:282. doi: 10.3389/fmicb.2016.00282
Lin, D. M., Koskella, B., and Lin, H. C. (2017). Phage therapy: an alternative to antibiotics in the age of multi-drug resistance. World J. Gastrointest. Pharmacol. Ther. 8:162. doi: 10.4292/wjgpt.v8.i3.162
Liu, Y., Demina, T. A., Roux, S., Aiewsakun, P., Kazlauskas, D., Simmonds, P., et al. (2021). Diversity, taxonomy, and evolution of archaeal viruses of the class Caudoviricetes. PLoS Biol. 19:e3001442. doi: 10.1371/journal.pbio.3001442
Lukman, C., Yonathan, C., Magdalena, S., and Waturangi, D. E. (2020). Isolation and characterization of pathogenic Escherichia coli bacteriophages from chicken and beef offal. BMC Res. Notes 13:8. doi: 10.1186/s13104-019-4859-y
Mäntynen, S., Laanto, E., Oksanen, H. M., Poranen, M. M., and Díaz-Muñoz, S. L. (2021). Black box of phage-bacterium interactions: exploring alternative phage infection strategies. Open Biol. 11:210188. doi: 10.1098/rsob.210188
McCallin, S., Sacher, J. C., Zheng, J., and Chan, B. K. (2019). Current state of compassionate phage therapy. Viruses 11:343. doi: 10.3390/v11040343
Melo, L. D., Ferreira, R., Costa, A. R., Oliveira, H., and Azeredo, J. (2019). Efficacy and safety assessment of two enterococci phages in an in vitro biofilm wound model. Sci. Rep. 9, 1–12. doi: 10.1038/s41598-019-43115-8
Mobasseri, G., Teh, C. S. J., Ooi, P. T., and Thong, K. L. (2019). The emergence of colistin-resistant Klebsiella pneumoniae strains from swine in Malaysia. J. Glob. Antimicrob. Resist. 17, 227–232. doi: 10.1016/j.jgar.2018.12.015
Moelling, K., Broecker, F., and Willy, C. (2018). A wake-up call: we need phage therapy now. Viruses 10:688. doi: 10.3390/v10120688
Møller-Olsen, C., Ho, S. F. S., Shukla, R. D., Feher, T., and Sagona, A. P. (2018). Engineered K1F bacteriophages kill intracellular Escherichia coli K1 in human epithelial cells. Sci. Rep. 8, 1–18. doi: 10.1038/s41598-018-35859-6
Morozova, V. V., Vlassov, V. V., and Tikunova, N. V. (2018). Applications of bacteriophages in the treatment of localized infections in humans. Front. Microbiol. 9:1696. doi: 10.3389/fmicb.2018.01696
Nale, J. Y., and Clokie, M. R. (2021). Preclinical data and safety assessment of phage therapy in humans. Curr. Opin. Biotechnol. 68, 310–317. doi: 10.1016/j.copbio.2021.03.002
Nale, J. Y., Spencer, J., Hargreaves, K. R., Buckley, A. M., Trzepiński, P., Douce, G. R., et al. (2015). Bacteriophage combinations significantly reduce Clostridium difficile growth in vitro and proliferation in vivo. Antimicrob. Agents Chemother. 60, 968–981. doi: 10.1128/AAC.01774-15
Nale, J. Y., Vinner, G. K., Lopez, V. C., Thanki, A. M., Phothaworn, P., Thiennimitr, P., et al. (2021). An optimized bacteriophage cocktail can effectively control Salmonella in vitro and in Galleria mellonella. Front. Microbiol. 11:609955. doi: 10.3389/fmicb.2020.609955
Namdee, K., Khongkow, M., Boonrungsiman, S., Nittayasut, N., Asavarut, P., Temisak, S., et al. (2018). Thermoresponsive bacteriophage nanocarrier as a gene delivery vector targeted to the gastrointestinal tract. Mol. Ther. Nucleic Acids 12, 33–44. doi: 10.1016/j.omtn.2018.04.012
Narulita, E., Aji, G. A., Wahono, B., Murdiyah, S., and Yulian, R. (2020). Synergism of phage фPT1b and antibiotics for reducing infection of Escherichia coli. Biogenesis 8, 22–28. doi: 10.24252/biov8il.11280
Ng, D. H., Marimuthu, K., Lee, J. J., Khong, W. X., Ng, O. T., Zhang, W., et al. (2018). Environmental colonization and onward clonal transmission of carbapenem-resistant Acinetobacter baumannii (CRAB) in a medical intensive care unit: the case for environmental hygiene. Antimicrob. Resist. Infect. Control 7, 1–8. doi: 10.1186/s13756-018-0343-z
Ng, R. N., Tai, A. S., Chang, B. J., Stick, S. M., and Kicic, A. (2021). Overcoming challenges to make bacteriophage therapy standard clinical treatment practice for cystic fibrosis. Front. Microbiol. 11:593988. doi: 10.3389/fmicb.2020.593988
Nhu, N. T. K., Lan, N. P. H., Campbell, J. I., Parry, C. M., Thompson, C., Tuyen, H. T., et al. (2014). Emergence of carbapenem-resistant Acinetobacter baumannii as the major cause of ventilator-associated pneumonia in intensive care unit patients at an infectious disease hospital in southern Vietnam. J. Med. Microbiol. 63:1386. doi: 10.1099/jmm.0.076646-0
Nuidate, T., Kuaphiriyakul, A., Surachat, K., and Mittraparp-Arthorn, P. (2021). Induction and genome analysis of HY01, a newly reported prophage from an emerging shrimp pathogen Vibrio campbellii. Microorganisms 9:400. doi: 10.3390/microorganisms9020400
Pelfrene, E., Willebrand, E., Cavaleiro Sanches, A., Sebris, Z., and Cavaleri, M. (2016). Bacteriophage therapy: a regulatory perspective. J. Antimicrob. Chemother. 71, 2071–2074. doi: 10.1093/jac/dkw083
Pelyuntha, W., Ngasaman, R., Yingkajorn, M., Chukiatsiri, K., Benjakul, S., and Vongkamjan, K. (2021). Isolation and characterization of potential Salmonella phages targeting multidrug-resistant and major serovars of Salmonella derived from broiler production chain in Thailand. Front. Microbiol. 12:662461. doi: 10.3389/fmicb.2021.662461
Petsong, K., Benjakul, S., and Vongkamjan, K. (2019). Evaluation of storage conditions and efficiency of a novel microencapsulated Salmonella phage cocktail for controlling S. enteritidis and S. typhimurium in-vitro and in fresh foods. Food Microbiol. 83, 167–174. doi: 10.1016/j.fm.2019.05.008
Petsong, K., Benjakul, S., and Vongkamjan, K. (2021). Optimization of wall material for phage encapsulation via freeze-drying and antimicrobial efficacy of microencapsulated phage against Salmonella. J. Food Sci. Technol. 58, 1937–1946. doi: 10.1007/s13197-020-04705-x
Petsong, K., Uddin, M. J., Vongkamjan, K., and Ahn, J. (2018). Combined effect of bacteriophage and antibiotic on the inhibition of the development of antibiotic resistance in Salmonella typhimurium. Food Sci. Biotechnol. 27, 1239–1244. doi: 10.1007/s10068-018-0351-z
Phothaworn, P., Dunne, M., Supokaivanich, R., Ong, C., Lim, J., Taharnklaew, R., et al. (2019). Characterization of flagellotropic, Chi-Like Salmonella phages isolated from Thai poultry farms. Viruses 11:520. doi: 10.3390/v11060520
Phothaworn, P., Supokaivanich, R., Lim, J., Klumpp, J., Imam, M., Kutter, E., et al. (2020). Development of a broad-spectrum Salmonella phage cocktail containing Viunalike and Jerseylike viruses isolated from Thailand. Food Microbiol. 92:103586. doi: 10.1016/j.fm.2020.103586
Phothichaisri, W., Ounjai, P., Phetruen, T., Janvilisri, T., Khunrae, P., Singhakaew, S., et al. (2018). Characterization of bacteriophages infecting clinical isolates of Clostridium difficile. Front. Microbiol. 9:1701. doi: 10.3389/fmicb.2018.01701
Pirnay, J. P., Blasdel, B. G., Bretaudeau, L., Buckling, A., Chanishvili, N., Clark, J. R., et al. (2015). Quality and safety requirements for sustainable phage therapy products. Pharm. Res. 32, 2173–2179. doi: 10.1007/s11095-014-1617-7
Pirnay, J. P., Verbeken, G., Ceyssens, P. J., Huys, I., De Vos, D., Ameloot, C., et al. (2018). The magistral phage. Viruses 10:64. doi: 10.3390/v10020064
Pringsulaka, O., Patarasinpaiboon, N., Suwannasai, N., Atthakor, W., and Rangsiruji, A. (2011). Isolation and characterisation of a novel podoviridae-phage infecting Weissella cibaria N 22 from Nham, a Thai fermented pork sausage. Food Microbiol. 28, 518–525. doi: 10.1016/j.fm.2010.10.011
Przystal, J. M., Waramit, S., Pranjol, M. Z. I., Yan, W., Chu, G., Chongchai, A., et al. (2019). Efficacy of systemic temozolomide-activated phage-targeted gene therapy in human glioblastoma. EMBO Mol. Med. 11:e8492. doi: 10.15252/emmm.201708492
Rahayuningtyas, I., Indrawati, A., Wibawan, I. W. T., Palupi, M. F., and Istiyaningsih, I. (2020). Phylogenetic group determination and plasmid virulence gene profiles of colistin-resistant Escherichia coli originated from the broiler meat supply chain in Bogor, Indonesia. Vet. World 13:1807. doi: 10.14202/vetworld.2020.1807-1814
Rajandas, H., Parimannan, S., Sicheritz-Pontén, T., and Clokie, M. R. J. (2021). inPhocus: the diverse landscape of phage studies in the association of Southeast Asian nations region. Phage 2, 94–99. doi: 10.1089/phage.2021.29020.hra
Rezaeinejad, S., Vergara, G. G. R. V., Woo, C. H., Lim, T. T., Sobsey, M. D., and Gin, K. Y. H. (2014). Surveillance of enteric viruses and coliphages in a tropical urban catchment. Water Res. 58, 122–131. doi: 10.1016/j.watres.2014.03.051
Rimrang, B., Chanawong, A., Lulitanond, A., Wilailuckana, C., Charoensri, N., Sribenjalux, P., et al. (2012). Emergence of NDM-1-and IMP-14a-producing enterobacteriaceae in Thailand. J. Antimicrob. Chemother. 67, 2626–2630. doi: 10.1093/jac/dks267
Rivas, L., Coffey, B., McDonnell, M. J., Burgess, C. M., Coffey, A., Ross, R. P., et al. (2010). In vivo and ex vivo evaluations of bacteriophages e11/2 and e4/1c for use in the control of Escherichia coli O157:H7. Appl. Environ. Microbiol. 76, 7210–7216. doi: 10.1128/AEM.01530-10
Saharman, Y. R., Karuniawati, A., Sedono, R., Aditianingsih, D., Qi, H., Verbrugh, H. A., et al. (2021). Multimodal intervention to reduce acquisition of carbapenem-non-susceptible Gram-negative bacteria in intensive care units in the National Referral Hospital of Indonesia: an interrupted time series study. J. Crit. Care 64, 237–244. doi: 10.1016/j.jcrc.2021.04.016
Saharman, Y. R., Karuniawati, A., Sedono, R., Aditianingsih, D., Sudarmono, P., Goessens, W. H., et al. (2018). Endemic carbapenem-nonsusceptible Acinetobacter baumannii-calcoaceticus complex in intensive care units of the national referral hospital in Jakarta, Indonesia. Antimicrob. Resist. Infect. Control 7, 1–12. doi: 10.1186/s13756-017-0296-7
San, N., Aung, M. S., Thu, P. P., Myint, Y. Y., Aung, M. T., San, T., et al. (2019). First detection of the mcr-1 colistin resistance gene in Escherichia coli from a patient with urinary tract infection in Myanmar. New Microbes New Infect. 30:100550. doi: 10.1016/j.nmni.2019.100550
Sarker, S. A., Sultana, S., Reuteler, G., Moine, D., Descombes, P., Charton, F., et al. (2016). Oral phage therapy of acute bacterial diarrhea with two coliphage formulations: a randomized trial in children from Bangladesh. EBioMedicine 4, 124–137. doi: 10.1016/j.ebiom.2015.12.023
Schooley, R. T., Biswas, B., Gill, J. J., Hernandez-Morales, A., Lancaster, J., Lessor, L., et al. (2017). Development and use of personalized bacteriophage-based therapeutic cocktails to treat a patient with a disseminated resistant Acinetobacter baumannii infection. Antimicrob. Agents Chemother. 61, e00954–e01117. doi: 10.1128/AAC.00954-17
Sellvam, D., Lau, N. S., and Arip, Y. M. (2018). Genome organization of Escherichia phage YD-2008. S: a new entry to siphoviridae family. Trop. Life Sci. Res. 29:37. doi: 10.21315/tlsr2018.29.1.3
Shan, J., Korbsrisate, S., Withatanung, P., Adler, N. L., Clokie, M. R., and Galyov, E. E. (2014). Temperature dependent bacteriophages of a tropical bacterial pathogen. Front. Microbiol. 5:599. doi: 10.3389/fmicb.2014.00599
Shan, J., Ramachandran, A., Thanki, A. M., Vukusic, F., Barylski, J., and Clokie, M. (2018). Bacteriophages are more virulent to bacteria with human cells than they are in bacterial culture; insights from HT-29 cells. Sci. Reports 8:5091. doi: 10.1038/s41598-018-23418-y
Sheng, W. H., Badal, R. E., and Hsueh, P. R. (2013). Distribution of extended-spectrum β-lactamases, AmpC β-lactamases, and carbapenemases among enterobacteriaceae isolates causing intra-abdominal infections in the Asia-Pacific region: results of the study for monitoring antimicrobial resistance trends (SMART). Antimicrob. Agents Chemother. 57, 2981–2988. doi: 10.1128/AAC.00971-12
Sjahriani, T., Wasito, E. B., and Tyasningsih, W. (2021). Isolation and identification of Escherichia coli O157:H7 lytic bacteriophage from environment sewage. Int. J. Food Sci. 2021:7383121. doi: 10.1155/2021/7383121
Ström Hallenberg, G., Börjesson, S., Sokerya, S., Sothyra, T., and Magnusson, U. (2019). Detection of mcr-mediated colistin resistance in Escherichia coli isolates from pigs in small-scale farms in Cambodia. Antimicrob. Agents Chemother. 63, e02241–e02318. doi: 10.1128/AAC.02241-18
Styles, K. M., Thummeepak, R., Leungtongkam, U., Smith, S. E., Christie, G. S., Millard, A., et al. (2020). Investigating bacteriophages targeting the opportunistic pathogen Acinetobacter baumannii. Antibiotics 9:200. doi: 10.3390/antibiotics9040200
Tan, C. S., Aqiludeen, N. A., Tan, R., Gowbei, A., Mijen, A. B., Santhana Raj, L., et al. (2020). Could bacteriophages isolated from the sewage be the solution to methicillin-resistant Staphylococcus aureus? Med. J. Malaysia 75, 110–116.
Teo, J. W., Chew, K. L., and Lin, R. T. (2016). Transmissible colistin resistance encoded by mcr-1 detected in clinical enterobacteriaceae isolates in Singapore. Emerg. Microbes Infect. 5, 1–12. doi: 10.1038/emi.2016.85
Teo, J., Lim, T. P., Hsu, L. Y., Tan, T. Y., Sasikala, S., Hon, P. Y., et al. (2015). Extensively drug-resistant Acinetobacter baumannii in a Thai hospital: a molecular epidemiologic analysis and identification of bactericidal polymyxin B-based combinations. Antimicrob. Resist. Infect. Control 4:2. doi: 10.1038/emi.2016.85
Thammatinna, K., Egan, M. E., Htoo, H. H., Khanna, K., Sugie, J., Nideffer, J. F., et al. (2020). A novel vibriophage exhibits inhibitory activity against host protein synthesis machinery. Sci. Rep. 10, 1–14. doi: 10.1038/s41598-020-59396-3
Thanh, N. C., Nagayoshi, Y., Fujino, Y., Iiyama, K., Furuya, N., Hiromasa, Y., et al. (2020). Characterization and genome structure of virulent phage EspM4VN to control Enterobacter sp. M4 isolated from plant soft rot. Front. Microbiol. 11:885. doi: 10.3389/fmicb.2020.00885
Torres-Barceló, C. (2018). The disparate effects of bacteriophages on antibiotic-resistant bacteria. Emerg. Microbes Infect. 7, 1–12. doi: 10.1038/s41426-018-0169-z
Tu, V. Q., Nguyen, T. T., Tran, X. T., Millard, A. D., Phan, H. T., Le, N. P., et al. (2020). Complete genome sequence of a novel lytic phage infecting Aeromonas hydrophila, an infectious agent in striped catfish (Pangasianodon hypophthalmus). Arch. Virol. 165, 2973–2977. doi: 10.1007/s00705-020-04793-2
Turner, D., Kropinski, A. M., and Adriaenssens, E. M. (2021). A roadmap for genome-based phage taxonomy. Viruses 13:506. doi: 10.3390/v13030506
Twort, F. W. (1915). An investigation on the nature of ultra-microscopic viruses. Lancet 186, 1241–1243. doi: 10.1016/s0140-6736(01)20383-3
Vandamme, E. J., and Mortelmans, K. (2019). A century of bacteriophage research and applications: impacts on biotechnology, health, ecology and the economy! J. Chem. Technol. Biotechnol. 94, 323–342. doi: 10.1002/jctb.5810
Velasco, J. M., Valderama, M. T., Margulieux, K., Diones, P. C., Peacock, T., Navarro, F. C., et al. (2020b). Comparison of carbapenem-resistant microbial pathogens in combat and non-combat wounds of military and civilian patients seen at a tertiary military hospital, Philippines (2013–2017). Mil. Med. 185, e197–e202. doi: 10.1093/milmed/usz148
Velasco, J. M. S., Valderama, M. T. G., Margulieux, K. R., Diones, P. C. S., Reyes, A. M. B., Leonardia, S. G., et al. (2020a). First report of the mcr-1 colistin resistance gene identified in two Escherichia coli isolates from clinical samples, Philippines, 2018. J. Glob. Antimicrob. Resist. 21, 291–293. doi: 10.1093/milmed/usz148
Velasco, J. M., Valderama, M. T., Peacock, T., Warawadee, N., Nogrado, K., Navarro, F. C., et al. (2017). Carbapenemase-producing enterobacteriaceae and nonfermentative bacteria, the Philippines, 2013–2016. Emerg. Infect. Dis. 23:1597. doi: 10.3201/eid2309.161237
Verbeken, G., Huys, I., Pirnay, J. P., Jennes, S., Chanishvili, N., Scheres, J., et al. (2014). Taking bacteriophage therapy seriously: a moral argument. Biomed. Res. Int. 2014:621316. doi: 10.1155/2014/621316
Vergara, G. G. R. V., Goh, S. G., Rezaeinejad, S., Chang, S. Y., Sobsey, M. D., and Gin, K. Y. H. (2015). Evaluation of FRNA coliphages as indicators of human enteric viruses in a tropical urban freshwater catchment. Water Res. 79, 39–47. doi: 10.1016/j.watres.2015.04.022
Viertel, T. M., Ritter, K., and Horz, H. P. (2014). Viruses versus bacteria—novel approaches to phage therapy as a tool against multidrug-resistant pathogens. J. Antimicrob. Chemother. 69, 2326–2336. doi: 10.1093/jac/dku173
Vlieghe, E. R., Phe, T., De Smet, B., Veng, H. C., Kham, C., Lim, K., et al. (2013). Bloodstream infection among adults in Phnom Penh, Cambodia: key pathogens and resistance patterns. PLoS One 8:e59775. doi: 10.1371/journal.pone.0059775
Vongkamjan, K., Benjakul, S., Vu, H. T. K., and Vuddhakul, V. (2017). Longitudinal monitoring of Listeria monocytogenes and Listeria phages in seafood processing environments in Thailand. Food Microbiol. 66, 11–19. doi: 10.1016/j.fm.2017.03.014
Vu, K. (2020). ASEAN Economic Prospects Amid Emerging Turbulence: Development Challenges and Implications for Reform. Washington: Brookings Institution.
Vu, H. T. K., Stasiewicz, M. J., Benjakul, S., and Vongkamjan, K. (2021). Genomic analysis of prophages recovered from Listeria monocytogenes lysogens found in seafood and seafood-related environment. Microorganisms 9:1354. doi: 10.3390/microorganisms9071354
Wangkahad, B., Bosup, S., Mongkolsuk, S., and Sirikanchana, K. (2015). Occurrence of bacteriophages infecting Aeromonas, Enterobacter, and Klebsiella in water and association with contamination sources in Thailand. J. Water Health 13, 613–624. doi: 10.2166/wh.2014.204
Waturangi, D. E., Kasriady, C. P., Guntama, G., Sahulata, A. M., Lestari, D., and Magdalena, S. (2021). Application of bacteriophage as food preservative to control enteropathogenic Escherichia coli (EPEC). BMC Res. Notes 14:336. doi: 10.1186/s13104-021-05756-9
Wintachai, P., Naknaen, A., Pomwised, R., Voravuthikunchai, S. P., and Smith, D. R. (2019). Isolation and characterization of Siphoviridae phage infecting extensively drug-resistant Acinetobacter baumannii and evaluation of therapeutic efficacy in vitro and in vivo. J. Med. Microbiol. 68, 1096–1108. doi: 10.1099/jmm.0.001002
Wintachai, P., Naknaen, A., Thammaphet, J., Pomwised, R., Phaonakrop, N., Roytrakul, S., et al. (2020). Characterization of extended-spectrum-β-lactamase producing Klebsiella pneumoniae phage KP1801 and evaluation of therapeutic efficacy in vitro and in vivo. Sci. Rep. 10:11803. doi: 10.1038/s41598-020-68702-y
Wirjon, I. A., Lau, N. S., and Arip, Y. M. (2016). Complete genome sequence of Proteus mirabilis phage pPM_01 isolated from raw sewage. Intervirology 59, 243–253. doi: 10.1159/000468987
Withatanung, P., Chantratita, N., Muangsombut, V., Saiprom, N., Lertmemongkolchai, G., Klumpp, J., et al. (2016). Analyses of the distribution patterns of Burkholderia pseudomallei and associated phages in soil samples in Thailand suggest that phage presence reduces the frequency of bacterial isolation. PLoS Negl. Trop. Dis. 10:e0005005. doi: 10.1371/journal.pntd.0005005
Wittebole, X., De Roock, S., and Opal, S. M. (2014). A historical overview of bacteriophage therapy as an alternative to antibiotics for the treatment of bacterial pathogens. Virulence 5, 226–235. doi: 10.4161/viru.25991
Wong, C. L., Sieo, C. C., Tan, W. S., Abdullah, N., Hair-Bejo, M., Abu, J., et al. (2014). Evaluation of a lytic bacteriophage, Φ st1, for biocontrol of Salmonella enterica serovar Typhimurium in chickens. Int. J. Food Microbiol. 172, 92–101. doi: 10.1016/j.ijfoodmicro.2013.11.034
Wongsuntornpoj, S., Switt, A. I. M., Bergholz, P., Wiedmann, M., and Chaturongakul, S. (2014). Salmonella phages isolated from dairy farms in Thailand show wider host range than a comparable set of phages isolated from US dairy farms. Vet. Microbiol. 172, 345–352. doi: 10.1016/j.vetmic.2014.05.023
World Health Organization (2017). Global Priority List of Antibiotic-Resistant Bacteria to Guide Research, Discovery, and Development of New Antibiotics. WHO Press, 1–7. Available at: https://www.who.int/medicines/publications/WHO-PPL-Short_Summary_25Feb-ET_NM_WHO.pdf (Accessed January 15, 2020).
World Health organization (2019). New Report Calls for Urgent Action to Avert Antimicrobial Resistance Crisis. Available at: https://www.who.int/news/item/29-04-2019-new-report-calls-for-urgent-action-to-avert-antimicrobial-resistance-crisis (Accessed January 15, 2020).
Wu, N., and Zhu, T. (2021). Potential of therapeutic bacteriophages in nosocomial infection management. Front. Microbiol. 12:638094. doi: 10.3389/fmicb.2021.638094
Yamamoto, Y., Kawahara, R., Fujiya, Y., Sasaki, T., Hirai, I., Khong, D. T., et al. (2019). Wide dissemination of colistin-resistant Escherichia coli with the mobile resistance gene mcr in healthy residents in Vietnam. J. Antimicrob. Chemother. 74, 523–524. doi: 10.1093/jac/dky435
Yingkajorn, M., Sermwitayawong, N., Palittapongarnpimp, P., Nishibuchi, M., Robins, W. P., Mekalanos, J. J., et al. (2014). Vibrio parahaemolyticus and its specific bacteriophages as an indicator in cockles (Anadara granosa) for the risk of V. parahaemolyticus infection in southern Thailand. Microb. Ecol. 67, 849–856. doi: 10.1007/s00248-014-0382-9
Zainol Abidin, N. Z., Sulong, A., Alfizah, H., Muttaqillah, N. A., and Ding, C. H. (2015). Molecular detection of the New Delhi metallo-β-lactamase-1 gene in enterobacteriaceae isolates in a tertiary medical Centre. Malays. J. Pathol. 37, 227–232.
Zellweger, R. M., Carrique-Mas, J., Limmathurotsakul, D., Day, N. P. J., Thwaites, G. E., Baker, S., et al. (2017). A current perspective on antimicrobial resistance in Southeast Asia. J. Antimicrob. Chemother. 72, 2963–2972. doi: 10.1093/jac/dkx260
Keywords: bacteriophage, multidrug resistance, phage revolution, phage therapy, Southeast Asia
Citation: Carascal MB, dela Cruz-Papa DM, Remenyi R, Cruz MCB and Destura RV (2022) Phage Revolution Against Multidrug-Resistant Clinical Pathogens in Southeast Asia. Front. Microbiol. 13:820572. doi: 10.3389/fmicb.2022.820572
Edited by:
Hany Anany, Agriculture and Agri-Food Canada (AAFC), CanadaReviewed by:
Michela Gambino, University of Copenhagen, Denmark Sabrina Ingrid Green, Baylor College of Medicine, United StatesCopyright © 2022 Carascal, dela Cruz-Papa, Remenyi, Cruz and Destura. This is an open-access article distributed under the terms of the Creative Commons Attribution License (CC BY). The use, distribution or reproduction in other forums is permitted, provided the original author(s) and the copyright owner(s) are credited and that the original publication in this journal is cited, in accordance with accepted academic practice. No use, distribution or reproduction is permitted which does not comply with these terms.
*Correspondence: Mark B. Carascal, bWJjYXJhc2NhbEB0aGVtZWRpY2FsY2l0eS5jb20=