- 1Eco-Evo-Devo Laboratory, Guangxi Key Laboratory in Forest Ecology and Conservation, College of Forestry, Guangxi University, Nanning, China
- 2Key Laboratory of Environment Change and Resources Use in Beibu Gulf, Nanning Normal University, Nanning, China
The symbiosis between a host and its microbiome is essential for host fitness, and this association is a consequence of the host’s physiology and habitat. Sinocyclocheilus, the largest cavefish diversification of the world, an emerging multi-species model system for evolutionary novelty, provides an excellent opportunity for examining correlates of host evolutionary history, habitat, and gut-microbial community diversity. From the diversification-scale patterns of habitat occupation, major phylogenetic clades (A–D), geographic distribution, and knowledge from captive-maintained Sinocyclocheilus populations, we hypothesize habitat to be the major determinant of microbiome diversity, with phylogeny playing a lesser role. For this, we subject environmental water samples and fecal samples (representative of gut-microbiome) from 24 Sinocyclocheilus species, both from the wild and after being in captivity for 6 months, to bacterial 16S rRNA gene profiling using Illumina sequencing. We see significant differences in the gut microbiota structure of Sinocyclocheilus, reflective of the three habitat types; gut microbiomes too, were influenced by host-related factors. There is no significant association between the gut microbiomes and host phylogeny. However, there is some microbiome related structure at the clade level, with the most geographically distant clades (A and D) being the most distinct, and the two overlapping clades (B and C) showing similarities. Microbes inhabiting water were not a cause for significant differences in fish-gut microbiota, but water quality parameters were. Transferring from wild to captivity, the fish microbiomes changed significantly and became homogenized, signifying plastic changes and highlighting the importance of environmental factors (habitat) in microbiome community assembly. The core microbiome of this group, at higher taxonomic scale, resembled that of other teleost fishes. Our results suggest that divergent natural environments giving rise to evolutionary novelties underlying host adaptations, also includes the microbiome of these fishes.
Introduction
The gastrointestinal tract of an animal is occupied by a microbiome, a staggering diversity of microbial colonies that are in a symbiotic association with the host (Hooper and Gordon, 2001). These commensal gut-bacterial relationships influence many vital aspects of the host, such as immune function, nutrient absorption, development, and behavior (Mazmanian et al., 2005; Nicholson et al., 2005; Mcfall-Ngai et al., 2013). Hence, the gut microbiome is considered an extension of the host genome, and these microbes are thought to have served as a “bridge” between the host and the external environment during evolution (Shapira, 2016). Therefore, the microbiome of an organism is the result of an interplay between the host’s phylogeny and its environment (Gill et al., 2006). Hence, the microbiome of a host is dynamic system (Talwar et al., 2018), where microbe communities change depending on the environment and host physiology (Nayak, 2010).
The phylogenetic relationships of hosts is associated with the diversity of their microbiota (Brucker and Bordenstein, 2012). The term “phylosymbiosis” has been used to encapsulate this idea (Lim and Bordenstein, 2020), which has been supported through controlled laboratory experimentation and analyses of wild populations (Kohl et al., 2017; Ingala et al., 2018), but a pattern of phylosymbiosis is still weakly supported or absent in some host systems (Wong et al., 2013; Brooks et al., 2016; Grond et al., 2020). However, large diversification-scale studies addressing correlates of host evolutionary history and microbial community diversity are few (Ross et al., 2018).
A related idea extending from phylosymbiosis is that of the “core-microbiome,” which was initially introduced in a narrower sense in human host studies (Turnbaugh et al., 2009, 2010; Qin et al., 2010). Since then, a plethora of core-microbiomes, ranging from single species to higher taxonomic groups, has manifested, reinforcing the association between host phylogeny and gut microbiome composition. The selective-mechanisms that contribute to the establishment of a core-microbiome in hosts, however, are unclear. Differential selection pressures in the form of host-physiology (linked to host phylogeny) and environment on microbiota in large diversifications occupying contrastingly different habitats, can shed light on gut-microbe and host associations. Such a system exists in species that have evolved to occupy caves.
Various vertebrate groups have occupied caves by adapting to conditions of low availability of food, oxygen, and light, leading to repeated evolution of troglomorphic adaptations such as lowered metabolism, specialized behaviors, specialized sensory systems, and loss of eyes and pigmentation (Zhao and Zhang, 2006; Gross et al., 2008). This accelerated enhancement or regression of certain traits in troglomorphic forms is a useful model system to study the process of natural selection in response to cave-associated selective regimes (Yang et al., 2016; Hart et al., 2020; Zhao et al., 2021). Though rarely tested, cave systems provide opportunities to test hypotheses pertaining to microbiome community assembly in response to phylogeny and environment. Although large troglomorphic diversifications are rare, one such radiation exists in a group of freshwater fishes in China. Sinocyclocheilus (Cyprinidae, Barbinae), the largest cavefish diversification in the world, represents an emerging model system for evolutionary novelty (Jiang et al., 2019; Mao et al., 2021, 2022; Zhao et al., 2021), provides an invaluable opportunity for examining the associations between host evolutionary history, environment and gut-microbial community diversity. With almost 75 extant species, Sinocyclocheilus is a monophyletic group of cyprinid cavefishes endemic to the expansive southwestern karstic region of China, the largest limestone area in the world, with an area ca. 620,000 km2 (Jiang et al., 2019). Having first evolved during the late-Miocene, these fishes show morphology-to-habitat correlation in a staggering array of adaptations to subterranean life. Interestingly, they also show several independent events of cave occupation. The microbiome of Sinocyclocheilus has not been subjected to a diversification-scale study so far.
Recent mt-DNA based phylogenies of Sinocyclocheilus suggest four major clades, Clades A – D, each with distinct morphologies, habitat-occupation strategies and geographic ranges (Zhao and Zhang, 2009; Jiang et al., 2019; Mao et al., 2021). They extend from the Eastern Guangxi autonomous region to the South-Eastern Guizhou and Eastern Yunnan Provinces of China. The earliest emerging clade (Clade A) is restricted to Guangxi, at the Eastern fringes of the distribution of the genus. Clades B and C, which have overlapping distributions, are restricted to the middle of the range of the genus (Guizhou, North-Central and North-Western Guangxi), and species of Clade D are found mostly in lotic habitats associated with hills to the west. They are classified into three major habitat types as Troglobitic (exclusively cave species, Stygobitic), Troglophilic (associated with caves, Stygophilic), and Surface (non-cave dependent species). Exceptionally, some of the Normal-Eyed surface fishes living in Guangxi and Guizhou can be found in both caves and surface rivers depending on the season (personal observations) and are therefore considered to be seasonal surface dwellers, while others living in the Yunnan region are considered to be permanent surface dwellers. These habitat types are correlated with their morphological adaptations, especially of their eye condition: Normal-Eyed (Surface), Micro-eyed (Troglophilic) and Blind (Troglobitic) (Mao et al., 2021).
While earlier gastrointestinal tract related microbiome studies focused mostly on fishes of economic importance, recent studies have focused on species of evolutionary, ecological or conservation significance (Sullam et al., 2015; Talwar et al., 2018; Rennison et al., 2019; Sevellec et al., 2019; Harer et al., 2020). Sinocyclocheilus is identified with the later (recent) group of studies. However, very little is known of the feeding ecology and the microbiome of Sinocyclocheilus. Cave-inhabiting species feed on cave insects, bat excrement and debris carried by the water flow (Zhang et al., 2015). A previous study on the gut microbiota of Sinocyclocheilus showed abundance of cellulose-degrading bacteria such as Bacillus, Clostridium, and Planctomyces, in the cave dwelling species compared to the surface species (Chen et al., 2019).
Water chemistry has been associated with the gut microbiome of hosts in a different cavefish system. The dissolved oxygen content in cave water was shown to alter the beta-diversity of gut microbiota in Astyanax mexicanus, the well-known single-species cavefish model system (Ornelas-Garcia et al., 2018). This suggests that cavefish species and populations living in different aquatic environments have distinct microbial communities. However, such data do not exist for Sinocyclocheilus.
Furthermore, the changes of the bacterial microbiome when cavefish populations are transferred from various wild habitat types to captivity has not been documented so far. This can be used to study the changes in microbiome community in the short run, helping further understand the acquired microbiome for the life of these fish and the factors that may influence such microbiome assembly. Knowledge of the changes in the microbiome is also useful for conservation breeding, reintroduction and study of these rare and threatened cavefishes. As a part of our explorations into cavefishes, we have maintained 40 Sinocyclocheilus species in captivity from 1 to 3 years, with many of them still thriving (personal observations). Since the microbiome is dependent on external factors, such as water conditions and diet, we presume, given proper care, that they are adaptable, together with plastic changes to their gut microbiota.
Here we carry out a diversification-scale analysis of Sinocyclocheilus using 24 representative species of the phylogeny (phylogeny has a strong association with geography) and habitat representation to understand the host-microbiome relationships of these fishes, including that in captivity. We formulate our hypothesis based mainly on the following observations: (i) these fishes have repeatedly evolved to occupy caves, independent of the phylogeny (ii) given appropriate water conditions and diet, these fishes can survive in captivity over long periods, hence they are adaptable with changes to their gut microbiome. From these observations, we hypothesize that the microbiome of Sinocyclocheilus is dependent primarily on habitat associations (Troglobitic – Blind; Troglophilic – Micro-Eyed; Surface – Normal), with phylogeny playing a secondary role. We test this hypothesis through the following predictions: (i) That the microbiomes of troglobitic species are more similar to one another than to surface species, with the troglophilic species in between. Since there are independently derived blind (troglobitic) species, we predict that they will have similar microbiomes. (ii) That the microbiomes of the four clades will be dissimilar, except Clades B and C as they overlap in geographic distribution. (iii) Since Sinocyclocheilus is monophyletic, that there will be a core microbiome characteristic of the group. (iv) Since we have maintained these fishes in captivity for more than 3 years, we predict that they can survive on an acquired microbiome that is different from the wild one.
Materials and Methods
Sample Collection, Captive Care and Animal Ethics
To address the questions of phylosymbiosis, influence of the environment, captive altered and the core microbiome in this cavefish system, we inventoried the gut microbial communities of 24 species of Sinocyclocheilus from provenance to domestication, as well as collecting bacterial samples from cave water. Sinocyclocheilus species (N = 24) were collected from Guangxi Zhuang Autonomous Region, Guizhou Province and Yunnan Provinces during the period of May, 2020 – December, 2020 (Figure 1). Majority of fishes were collected from caves and few from surface rivers, depending on the species. For each species, live specimens were sampled (N = 3–5) using umbrella nets and placed in sterile container and water until a fresh fecal sample was collected (wild-sample). Fecal samples were collected using disposable sterile pipettes and placed in sterile vials. These were immediately frozen and stored in liquid nitrogen at −80°C until DNA extraction. The collection time for the fecal sample was less than 10 min as the excitement after being caught made the fish defecate quickly. Then the fish were placed in polythene bags containing water from the habitat, filled with medical grade oxygen, and transported to laboratory in heat-proof boxes. Due to the rarity of these fishes, note that we used fecal samples as a surrogate for the gut samples to facilitate subsequent captive studies as well.
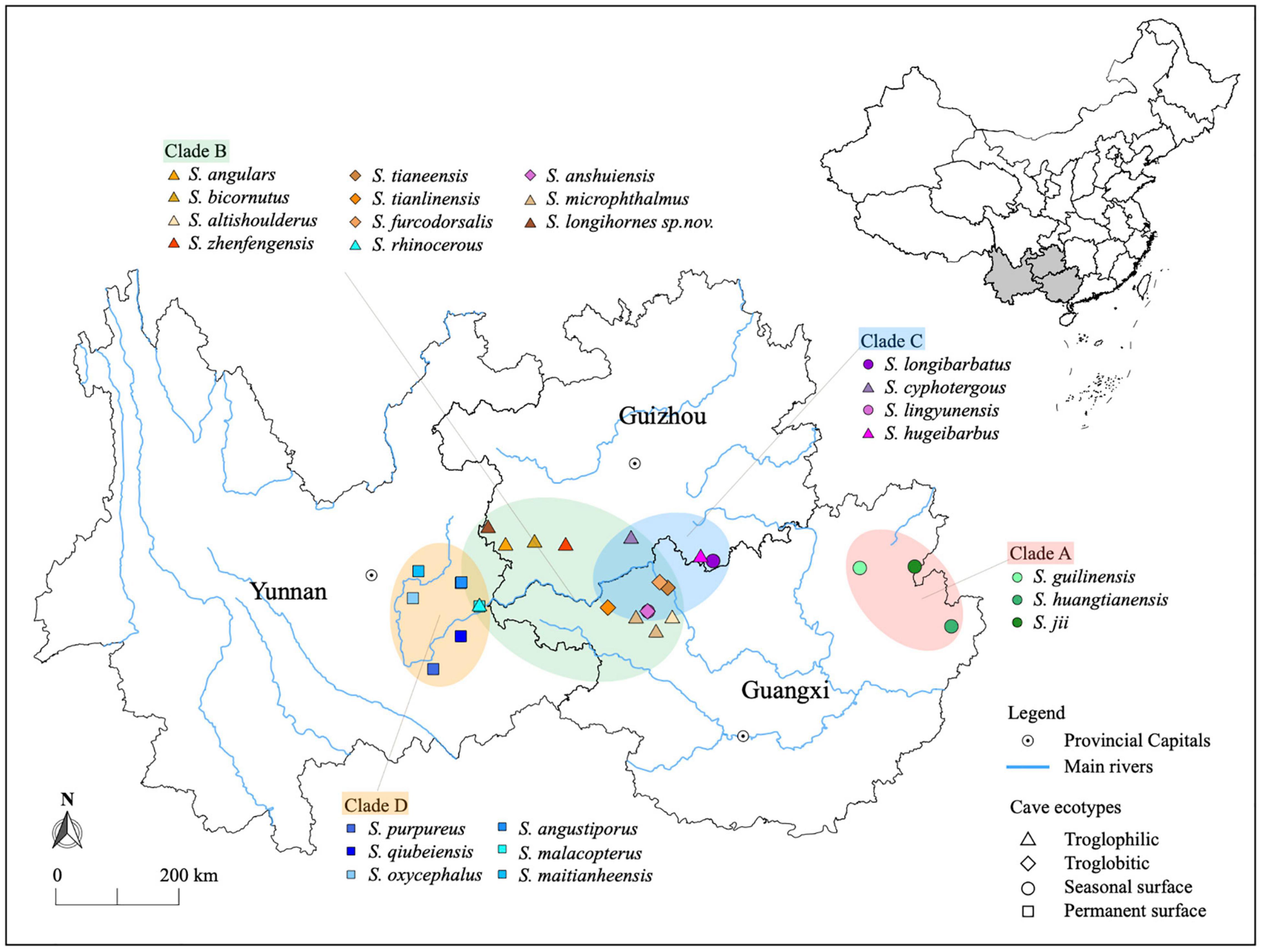
Figure 1. Distribution of 24 Sinocyclocheilus species and geographic context of phylogenetic clades. Colored dots represent the species. The four phylogenetic clades are represented by the colored ellipses. Clades A (East) and D (West) are geographically disjunct and Clades B and C are overlapping.
Water samples from 13 caves were collected from the areas where fishes inhabit. Water samples from seven caves were not collected due to complex (often deep) habitats. One liter of water sample was collected from three sites of a particular area (three liters in total) and stored in sterile bottles. Upon collection, the water samples were filtered through 0.22 μm filter membranes using a portable vacuum pump and immediately frozen and stored in liquid nitrogen at −80°C until DNA extraction. A portable multi-parameter water quality analyzer (MACH HQ30d) was used to measure conductivity, dissolved oxygen (DO), pH and water temperature, directly from the cave water (ca. 50 cm below the surface).
Fishes collected from field were brought to laboratory and were conditioned for long term captive rearing. They were placed in independent isolation tanks for 2 weeks before being introduced into the main system. Fish that were injured and whose condition deteriorated over the 2 weeks were not introduced into the main system. The test subjects whose fecal samples were collected were placed in an ESEN aquarium system, a recirculating filtered water system with separate fish rearing tanks. Each species of Sinocyclocheilus were kept in separate box, with a common water circulation system. All captive fishes were kept at 18–20°C, fed ad libitum by a diet based on protein (about 45%) and fat (about 10%). Fecal samples in captivity were collected after 6 months after being captured. For this, each fish was placed in a sterile plastic box and the fecal sample collected using a sterile dropper as soon as the fishes defecated. Samples collected were again placed in a sterile vial and immediately frozen at −80°C until DNA extraction.
The present study was conducted in accordance with the recommendations of Institutional Animal Care and Use Committee of Guangxi University (GXU), Nanning-China. All fish were cared for at a fish-room of the Eco.Evo.Devo Group in Forestry college (GXU2019-071).
DNA Extraction, PCR Amplification, and Sequencing
Bacterial genomic DNA was extracted from fecal and water samples according to the protocol set by Novogene Corporation (Beijing, China). Total genomic DNA from samples was extracted using SDS (Sodium Dodecyl Sulfate) method. Concentration of DNA and its purity was monitored on 1% agarose gels. DNA was further diluted to 1 ng/μL using sterile water.
Hyper-variable regions (V3–V4) of the 16S rRNA gene were PCR-amplified from genomic DNA, by using the bacteria-specific universal barcode-primers 341F (5′-CCTAYGGGRBGCASCAG-3′) and 806R (5′-GGACTACNN GGGTATCTAAT-3′). All polymerase chain reactions were performed using 15 μL of Phusion® High-Fidelity PCR Master Mix (New England Biolabs, Ipswich, MA, United States), 0.2 μM of each forward and reverse primer and 10 ng of DNA template. Thermal cycling conditions were as follows: initial denaturation at 98°C for 1 min, followed by 30 cycles of denaturation at 98°C for 10 s, annealing at 50°C for 30 s, elongation at 72°C for 30 s and final extension at 72°C for 5 min.
PCR products were mixed in equal volumes of 1× loading buffer containing SYB green and further electrophoresis was operated on 2% agarose gel for detection. PCR products were mixed in equal ratios. The PCR products were further purified using Qiagen Gel Extraction Kits (Qiagen, Germany). Sequencing libraries were generated with NEBNext® Ultra™ IIDNA Library Preparation Kit (Cat No. E7645). Quality of library generated was evaluated on a Qubit@ 2.0 Flurometer (Thermo Scientific, Waltham, MA, United States) and Agilent Bioanalyzer 2100 system. The library was sequenced on an Illumina NovaSeq platform and 250 bp paired-end reads were generated. Paired-end reads were assigned to samples based on their unique barcodes and were truncated by cutting off the barcodes and primer sequences. Paired-end reads were merged using FLASH (Version 1.2.11) which resulted in raw tags (Magoc and Salzberg, 2011). To obtain high quality clean tags, quality filtering on raw tags was performed using fastp software (Version 0.20.0). To detect the chimera sequences, the clean tags were compared with Silva database using Vsearch (Version 2.15.0). The chimera sequences were further removed to obtain effective tags. Raw sequences generated were submitted to the National Center for Biotechnology Information (NCBI) BioProject database (accession number PRJNA772569).
Amplicon Sequence Variants Denoise and Taxonomic Annotation
To obtain initial ASVs (Amplicon Sequence Variants) denoise was performed using DADA2 in QIIME2 software (Version 2021.4). ASVs with abundance less than five were filtered out. Taxonomic annotation was performed within Silva Database using QIIME2 software (Version 2021.4). Further, to examine the phylogenetic relationship of individual ASV and the differences of the dominant species among different samples, multiple sequence alignment was performed using QIIME2 software (Version 2021.4). The absolute abundance of ASVs was further normalized using standard sequence number corresponding to the samples with least sequences. The standard sequence numbers of the three ASV tables used sequentially in this paper are 6,240, 8,799, and 7,437. Subsequently the alpha and beta diversity analysis were performed based on the output of the normalized data.
Data Analysis and Visualization
Alpha diversity indices and beta diversity distances were calculated using QIIME2 software (Version 2021.4). To analyze the diversity, richness and uniformity of communities in each groups, we used box plots to show the Shannon index in R (4.1.1, amplicon package) (Liu et al., 2021). The Tukey’s honestly significant difference test (Tukey’s HSD), Analysis of Variance (ANOVA) test were used for significance testing. To evaluate the effect of different factors on the assembly of bacterial communities, we compared beta diversity using Bray–Curtis distances and performed Canonical Analysis of Principal Coordinates (CAP) in R (4.1.1, amplicon package). To explain the effect of environmental factors on the structure of gut microbial communities Canonical Correspondence Analysis (CCA) was carried out using Canoco software. Venn and Upset diagrams were plotted using TBtools (Chen et al., 2020). Manhattan plot were used to display the ASVs enriched or reduced between the wild group and captive group in R (4.1.1, edgeR and ggplot2 packages). To determine the biomarker, Linear discriminant analysis effect size (LEfSe) was implied using LEfSe software (Version 1.0). The taxa with a log Linear discriminant analysis score (LDA) more than four orders of magnitude were considered. To evaluate the functions of communities in the samples and different groups, PICRUSt2 software (Version 2.4.1) was used for the function annotation analysis.
Extent of phylosymbiosis for these fishes can be tested using the association between host phylogenies and metagenomic relationships of the gut bacterial microbiome. For this we used data from the most recent phylogeny for Sinocyclocheilus. We aligned cytochrome b (cytb) and NADH dehydrogenase subunit 4 (ND4) gene fragments independently in MUSCLE, implemented in MEGA-X. Then both gene fragments were also concatenated in MEGA-X. We inferred the maximum likelihood phylogenetic tree with IQTREE software by using Model Finder with the TIM3 + F + I + G4 substitution model and 1,000 rapid bootstrap replicates. We estimated genetic distance matrices for cytb and ND4 gene fragments using Mega X software for 24 species of Sinocyclocheilus. Also, some data was derived from a published paper from our laboratory (Mao et al., 2021). Phylogenetic distance matrix was used to perform Mantel tests (R package – vegan 2.5.7) with the host microbial similarity distance matrix. To generate UPGMA cluster trees, Unifrac distance across each microbial sample (Unweighed Pair Group Method with Arithmetic Mean) was used (base R function “hclust”). R package phangorn (Version 2.7.1) was used to test phylogenetic congruence between the host phylogeny and microbiome structure, using Robinson Foulds metric to estimate topological similarity. Finally, using the data of standard eye diameter (sED) and gut microbial alpha diversity, we performed a phylogenetic independent contrasts analysis (PIC, Felsenstein, 1985), which enabled us to understand how microbiome diversity divergence is linked to the three habitat types by removing the effect of species phylogenetic relationships in trait analysis.
Results
We collected 24 species of Sinocyclocheilus from the wild, together with their fecal samples and water samples from their habitat, they were then raised in captivity (Figure 1). They represented the four main phylogenetic clades with the following clade associations: Clade A (3 species – Seasonal Surface); Clade B (4 species – Troglobitic, 7 species – Troglophilic); Clade C (2 species – Seasonal Surface, 2 species – Troglophilic); Clade D (6 species – Permanent Surface). After 6 months in captivity, we sampled their feces again (17 species). Our sequence library contained microbiomes from 75 wild fecal samples, 46 captive fecal samples, and 19 water samples (Supplementary Table 1). According to the rarefaction curves, the estimations of species richness were steady and unbiased for all samples (Supplementary Figure 1).
Composition of Gut Microbiomes in Sinocyclocheilus
We used wild populations to demonstrate the natural gut microbial community structure of cavefish (Supplementary Table 2). The predominant phyla composing the gut microbes of Sinocyclocheilus were Proteobacteria, Fusobacteria, Firmicutes, Bacteroidetes, and Verrucomicrobiota, with relative abundances of 57.4, 31.6, 6.1, 2.7, and 0.4%, respectively. As expected, there are differences between Sinocyclocheilus species, mainly in the abundance of Proteobacteria and the Fusobacteria (Figure 2). Individual species in the same clade (Clade A, Clade C, and Clade D) had similar gut microbiota, while individual species in Clade B had a diverse gut microbiota (Figure 2). At level of taxonomic order, considering all wild-samples, the most abundant taxa were Fusobacteriales (31.6%), Aeromonadales (32.7%), Enterobacterales (10.9%), Burkholderiales (5.4%), Pseudomonadales (2.9%) and Bacteroidales (1.8%) (Supplementary Figure 2A). In addition, the gut microbiota of all these cavefish were dominated by eight genera (Supplementary Figure 2B), Cetobacterium (31.6%), Aeromonas (31.2%), Acinetobacter (2.2%), Shewanella (1.5%), ZOR0006 (1.4%), Deefgea (1.3%), Enterobacter (1.0%), and Crenothrix (0.9%).
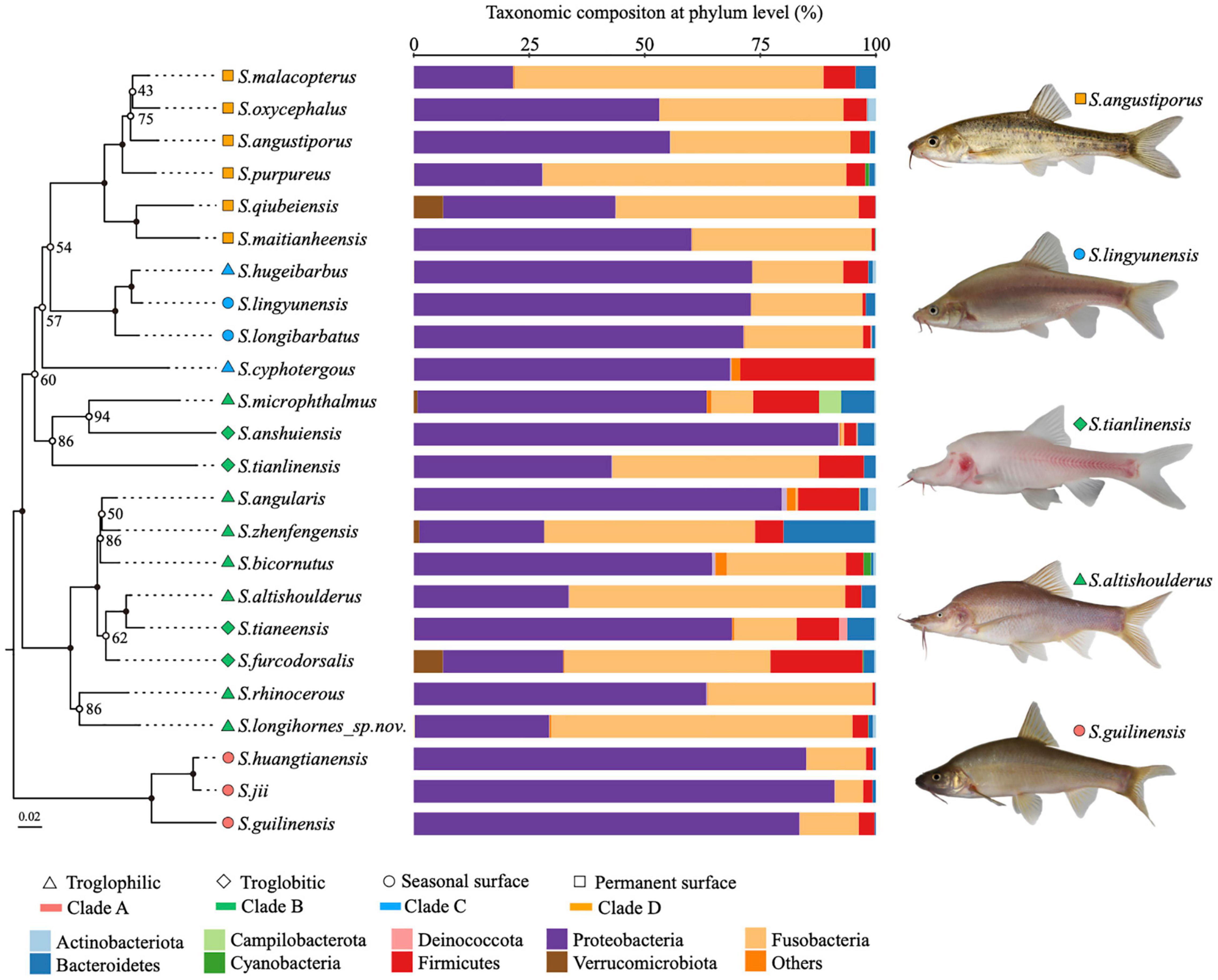
Figure 2. Phylogenetic relationships and microbial affinities of Sinocyclocheilus species (N = 24). (Left) The maximum likelihood phylogenetic tree based on concatenated cytb and ND4 gene fragments of 24 Sinocyclocheilus species, with 1,000 bootstrap replicates. Filled circles on the node represents bootstrap support is 100; (Middle) phylum level composition of the gut microbiome. The microbial community for each species is displayed as the sum of all individuals within a given species; (Right) images of representative species from the four main phylogenetic clades.
The Association of Gut Bacterial Communities With the Host Phylogeny
We used Mantel tests to assess whether between-species gut microbial distance is correlated with between-species genetic and geographic distances. There was no significant correlation between-species gut microbiota and geographical distance (Bray–Curtis Mantel R = −0.035, P = 0.568; unweighted UniFrac R = 0.065, P = 0.23; weighted R = 0.065, P = 0.263). And the gut microbiota and Sinocyclocheilus genetic divergence were insignificant irrespective of geographical distance (Bray–Curtis Mantel R = −0.119, P = 0.823; unweighted UniFrac R = −0.038, P = 0.601; weighted R = −0.195, P = 0.895). To test for signatures of host phylogeny on metagenomic community composition, we first constructed phylogenetic trees using the maximum likelihood estimation for cytb and ND4 gene fragments from 24 species of Sinocyclocheilus, and then used unweighted Unifrac distance matrices to construct UPGMA trees. We found that the phylogenetic tree and the similarity distance clustering tree appeared to be unrelated (Bray–Curtis, Robinson–Foulds distance = 36.0; unweighted UniFrac, Robinson–Foulds distance = 38.0; weighted UniFrac, Robinson–Foulds distance = 38.0).
Next, we investigated the variation of intestinal flora at a lower scale, i.e., under different phylogenetic clades and habitats. We classified wild populations of Sinocyclocheilus into four groups based on their clades and three groups based on their habitats, which have been reported by other authors (Yang et al., 2016; Mao et al., 2021) (Supplementary Table 1). Simpson index revealed that within-sample diversity (alpha diversity) of Clade D differs from those of Clade A and Clade B, while Clade C was not significantly dissimilar from the other groups (Figure 3A and Supplementary Table 3). Overall, the gut microbiota of Clade A and Clade B had higher diversity than that of Clade D. Furthermore, a Constrained Principal Coordinate Analysis (CPCoA) of the full dataset revealed that the host phylogeny has a significant impact on bacterial communities, accounting for 11.5% of the variation (Figure 3B). We found a clear separation between Clade A and Clade D from the first component, as well as an overlap between Clade B and Clade C (Figure 3B). It indicated that Clade A and Clade D were each inhabited by a distinctive bacterial community, while the Clade B and Clade C had similar microbiomes. Further, the metagenomic relationships of four groups inferred by computing unweighted Unifrac distance matrices indicated that the microbial composition of the four clades were consistent with phylogenetic relationships (unweighted UniFrac, Robinson–Foulds distance = 0.0) (Figure 3C). Bacterial composition varied across clades at the phylum level, as evidenced by a decrease in Proteobacteria and an increase in Fusobacteria from A to D (Figure 3C). However, this change was not linear, as pattern for Clade C changed before Clade B (Figure 3C). Moreover, we identified 29 ASVs using the LDA Effect Size (LEfSe) method, which are specific bacteria that play an important role in the four phylogenetic clades (Biomaker). Six ASVs were found in the Clade A group, thirteen in the Clade B group, five in the Clade C group, and five in the Clade D group (FDR adjusted P < 0.05, LDA score > 4.0; Supplementary Figure 3).
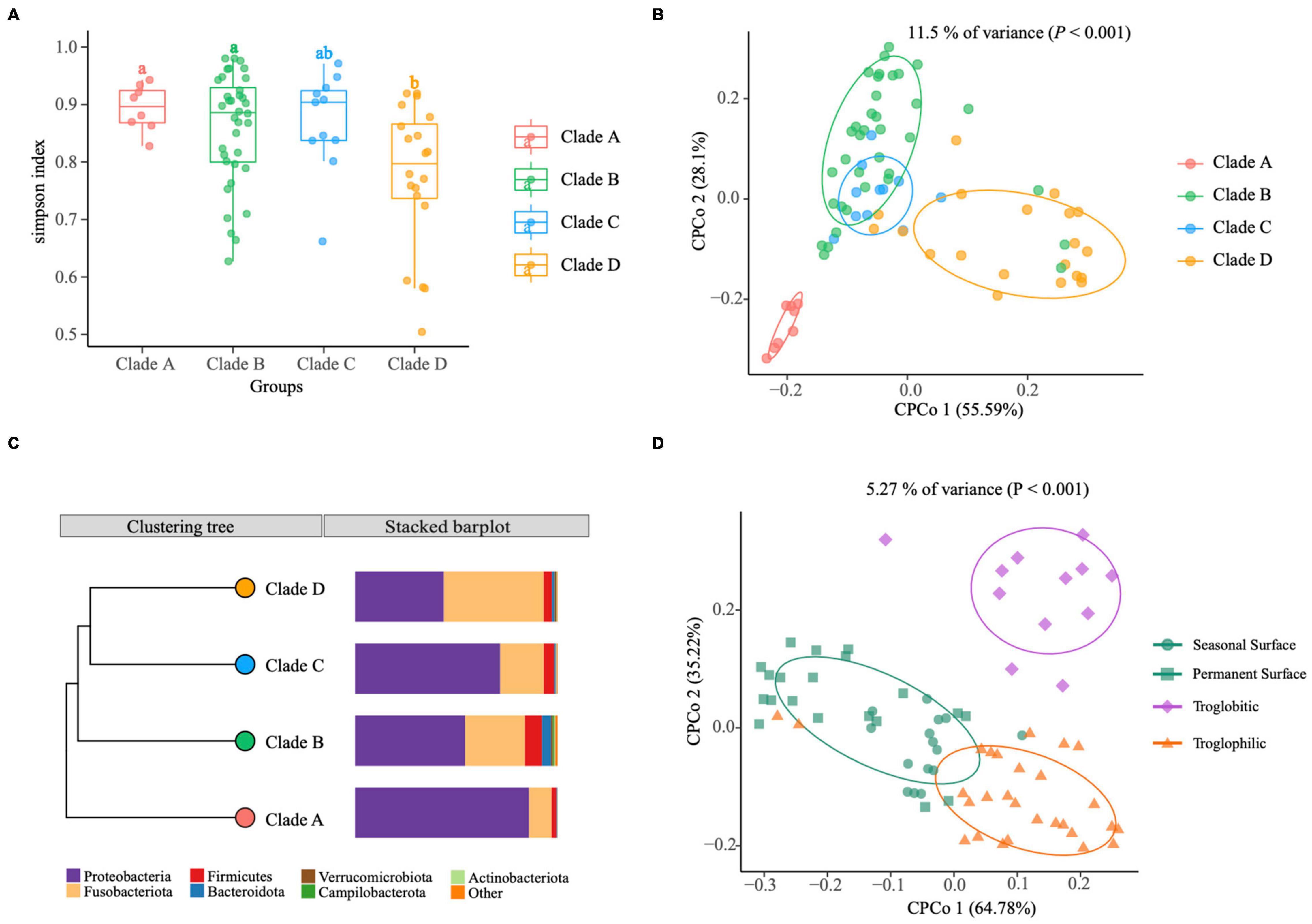
Figure 3. The gut microbiomes of Sinocyclocheilus species (N = 24) in context of phylogenetic clades and habitats. (A) Simpson index. The bottom and top of the box are the first and third quartiles, the band inside the box is the median, and the ends of the whiskers represent the minimum and maximum. Different letters indicate significant difference between groups (ANOVA, Tukey HSD test). (B) CPCoA of Bray–Curtis dissimilarity showing host phylogeny has significant effect on gut microbiome (11.5% of total variance was explained by the phylogenetic clade, P < 0.001). Total number of fishes used: clade A (N = 8), clade B (N = 36), clade C (N = 11) and clade D (N = 20). The ellipses include 68% of samples from each group. (C) The UPGMA tree with unweighted Unifrac distance matrices representing bacterial community composition of phylogenetic clades at phylum level indicated with bar plots. (D) CPCoA of Bray–Curtis dissimilarity showing living habitat has significant effect on gut microbiome (5.27% of total variance, explained by the phylogenetic clade, P < 0.001). Total number of fishes analyzed: Surface (N = 35), Troglobitic (N = 12), Troglophilic (N = 28). The ellipses include 68% of samples from each group.
Microbiome Community Structure Associations With Host Habitat
According to the degree of connection with caves, cavefish living habitats were classified as surface, troglophilic, or troglobitic. We evaluated beta diversity (diversity among samples) using Bray–Curtis distances and did a Canonical Analysis of Principal Coordinates (CAP) to examine the influence of different living habitats on the assembly of Sinocyclocheilus cavefish bacterial communities. This analysis revealed that significant differences between samples came from the Surface, Troglobitic and Troglophilic environments, explaining 5.27% of the variation in wild populations (Figure 3D, P < 0.001). Troglobitic group is clearly separated from Troglophilic group and the Surface group on the second axis (35.22% variance), while the Surface group is clearly separated from Troglophilic group on the first axis (64.78% variance). CAP analysis showed that the gut microbiota of seasonal surface species (green squares) are more similar to the Troglophilic group compared to Permanent Surface species (green circles). It demonstrates that seasonal Surface species from Clade A and Clade C are similar in gut microbiome structure to Troglophilic species despite their similarity in appearance to permanently Surface species from Yunnan province.
As the habitat type reflects the eye condition of each species, we used standard eye diameter (sED) in a phylogenetic comparative analysis to infer the correlation between habitat type (sED as a proxy for habitat type) and the fish gut microbial community alpha diversity. The Pearson correlation among these two variables were statistically insignificant (Chao1 index: r2 = 0.032, p = 0.198; Shannon index: r2 = −0.017, p = 0.448). To ascertain the effect of phylogeny on microbial community diversity, we performed a phylogenetic independent contrasts (PIC) analysis. This enabled us to account for potential confounding effects by the Sinocyclocheilus phylogeny on the result. The PIC results also only showed a weak negative correlation between Chao1 index and sED (r2 = −0.027, p = 0.537), as well as a weak positive correlation between Shannon index and sED (r2 = −0.025, p = 0.523), which were statistically insignificant (Supplementary Figure 4). This further suggests that the connection between fish habitat and gut microbial diversity may be only a random pattern.
Influence of Surrounding Water on the Host Microbiome
We quantified the contribution of variations in cave water-associated microbiota to the composition of the cavefish gut microbiota. This could signal the effect of a major environmental factor on the assembly of cavefish microbiome.
We analyzed the 19 cave water samples with their corresponding fish samples (Supplementary Table 4). There were 9,350 ASVs in the water samples and 4,224 ASVs in the fecal samples. The gut microbial ASVs shared with the cave water microbial community accounted for 22.1% (Figure 4A). Next, we used fast expectation-maximization microbial source tracking (FEAST) (Shenhav et al., 2019) to estimate how much of the Sinocyclocheilus cavefish gut microbiota derives from cave water. This shows that 52.13% of fish gut microbes came from water sources, while 47.87% came from unknown sources (Figure 4B). Furthermore, the water microbial communities in the 19 caves were also found to be different (Figure 4C). A corollary question is whether the differences in fish gut flora we discovered are attributable to varied microbial exposures in the environment. We assume the causal relationship between these two discrepancies and find two approaches to verify this prediction. First, we focus on whether the cavefish gut microbiota is more similar to the water microbiota of their location, compared to the water microbiota from other locations. The one-tailed t-test, did not show similarity between Sinocyclocheilus cavefish gut microbiota and local water flora (Bray–Curtis t = −0.094, P = 0.5378). Second, we assessed whether the cavefish gut microbiota distance matrix of (18 species, 19 locations) correlated with the distance matrix of the water microbiota between sites. We observed no link between cavefish gut microbiota distance and water microbiota distance using the partial Mantel test, which was adjusted for geographic distance as a confounding variable (Bray–Curtis R = 0.066, P = 0.275). In conclusion, microbes in cave water serve as a source of gut microbiota for cavefish, but they are not significant enough to explain the differences in gut microbiota among Sinocyclocheilus populations in different caves.
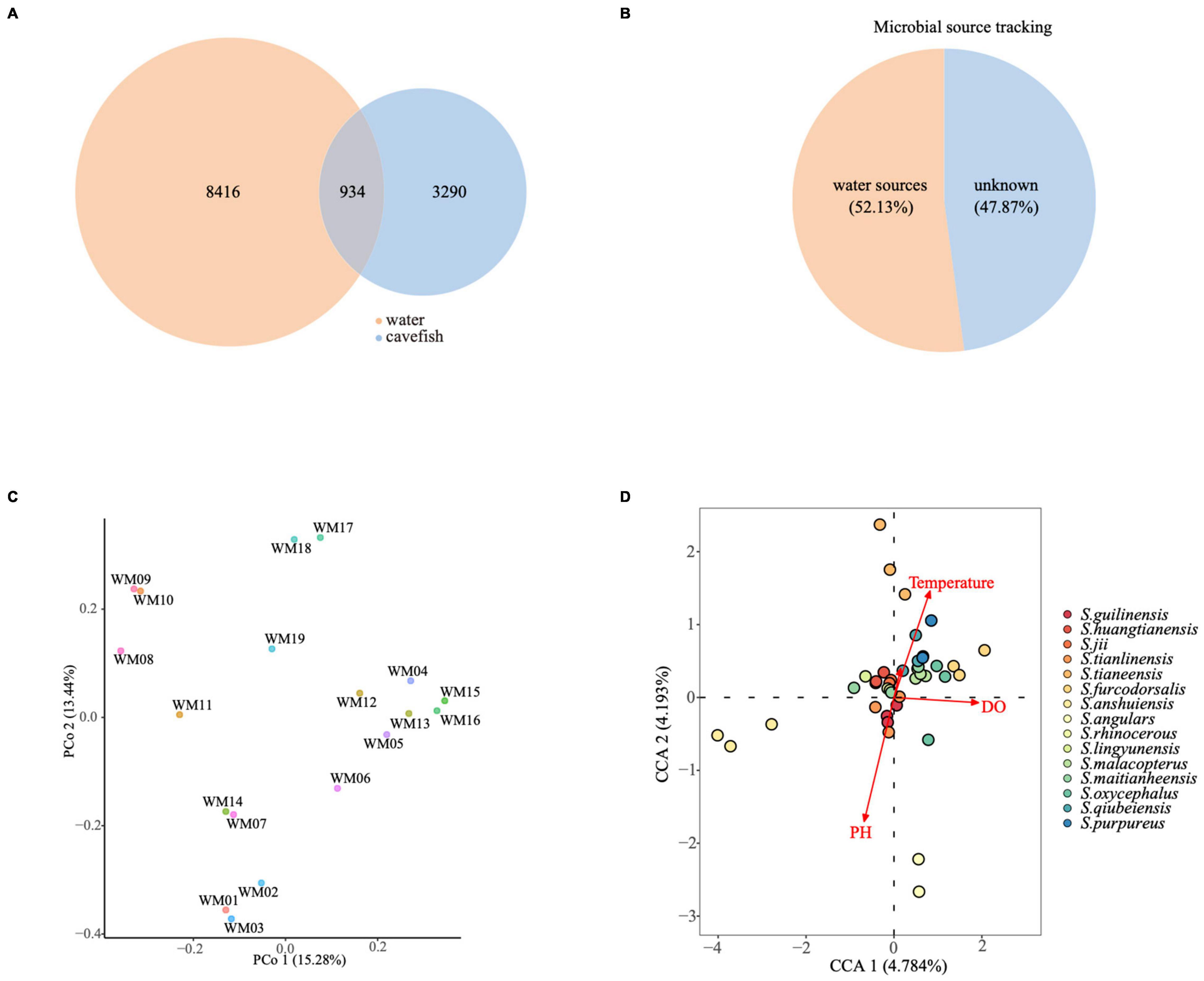
Figure 4. Influence of cave water on the gut microbiome in Sinocyclocheilus species. (A) Number of ASVs shared among cavefishes and water microbiotas. (B) Fast expectation-maximization microbial source tracking. Colors within the circle and area occupied indicate different source in the fecal sample. (C) Unconstrained Principal Coordinate Analysis (Unconstrained PCoA- for principal coordinates PCo1 and PCo2) with Bray–Curtis distance, showing difference between the cave water microbiotas (N = 19). (D) Water chemistry related changes in the microbial community explained using Canonical correlation analysis (CCA).
In addition to the microbes in water, water chemistry also may affect the variation of cavefish gut microbiota. We next evaluated the association between physiochemical variables of water and fish gut flora diversity by CCA (Figure 4D). In the CCA analysis, dissolved oxygen (DO), pH and temperature were significantly correlated to cavefish gut microbiota, while conductivity was not (Figure 4D and Supplementary Table 5). In this analysis, the individual samples show greater separation in the first axis, while DO too, has a higher correlation with the first axis. However, although three physicochemical indicators were related to the shaping of the cavefish gut microbiota, they had a low interpretation rate of 4.3% for DO, 4.2% for pH and 3.2% for Temperature (Supplementary Table 5).
Changes in the Bacterial Assemblages From Wild to Captivity
We collected 24 species of Sinocyclocheilus from the wild. Fecal samples were collected from each specimen prior to transfer to captivity. After 6 months in captivity, fecal samples were again collected from the 17 species that survived over this period. To find changes in bacterial assemblages from wild to captivity, fecal samples from both groups were analyzed (Supplementary Table 6). Measurement of within-sample diversity (alpha diversity) revealed a significant difference between wild and captive populations (P < 0.001, Tukey HSD test) (Figure 5A and Supplementary Table 7). The fecal microbiota of the captive group, except for three species, had lower richness and diversity than those of the wild group (Figure 5A), indicating that cavefish lost some bacterial species while in captivity. Further, we used non-metric multidimensional scaling (NMDS) analysis with Bray–Curtis distance to investigate the effect of captivity on the intestinal flora of cavefish (Figure 5B and Supplementary Figure 5). The results revealed that the intestinal microbiota of the wild and captive groups divided along the first MDS axis into two distinct clusters: the cluster of captives was more cohesive (Figure 5B), indicating that captivity altered cavefish gut microbiota and, to a certain extent, homogenized the gut bacteria across species.
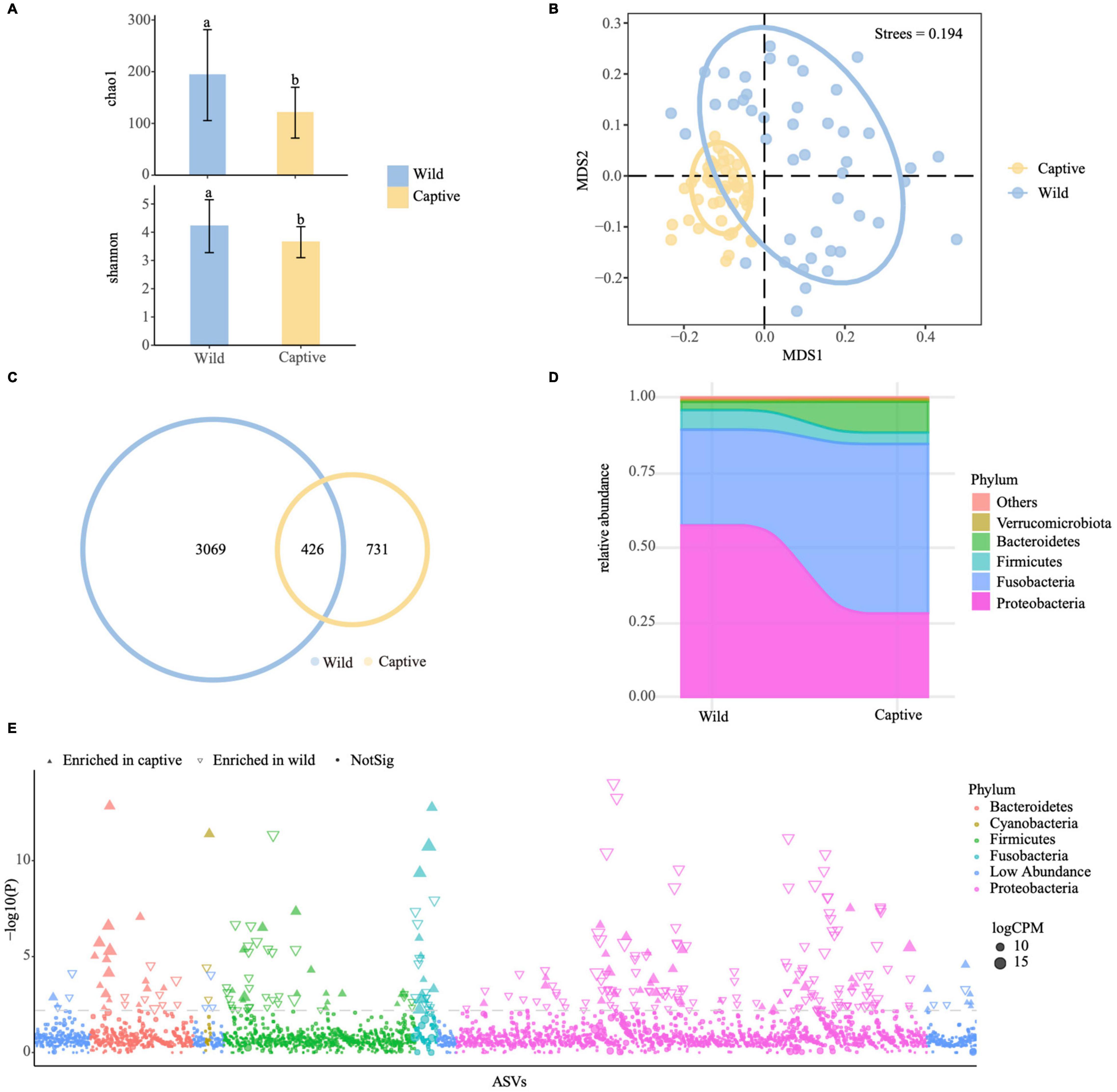
Figure 5. Effect of captivity on the gut microbiota of Sinocyclocheilus species (N = 24). (A) Chao1 and Shannon indices for gut samples from wild and captive populations. Letters represent significant difference between the groups (P < 0.05, ANOVA, Tukey HSD test). Data bars represent mean and error bars represent the standard error of the mean. (B) Non-metric multidimensional scaling (NMDS) analysis with Bray–Curtis distances, showing gut bacterial composition across the captive and wild cavefishes (P < 0.001, ANOSIM test). The ellipses cover 80% of data for each fish sample. (C) Venn diagram showing unique and shared ASVs between the captive and wild fish populations. (D) Distribution of gut bacterial taxa in captive and wild fishes at phylum level. (E) Manhattan plot showing ASVs enriched in the gut of captive and wild fish populations. Each dot or triangle represents a single ASV. ASVs enriched in captive and wild groups are represented by filled or empty triangles, respectively [false discovery rate (FDR) adjusted P < 0.05, Wilcoxon rank-sum test]. ASVs are arranged in taxonomic order and colored according to the phylum; counts per million reads mapped. Replicated samples are as follows: wild group (N = 46), captive group (N = 46). A total of 17 species of Sinocyclocheilus.
We discovered not only that captive populations generally had fewer ASVs than wild populations, but also that more than one-third of their ASVs overlapped with wild populations. Hence, in captivity, species of Sinocyclocheilus lost a significant portion of their wild-microbiome, but acquired some new ASVs also over the period of 6 months (Figure 5C and Supplementary Table 7). The total sequences of the captive cavefish group were classified into five major phyla, Proteobacteria, Fusobacteria, Firmicutes, Bacteroidetes, and Verrucomicrobiota (Figure 5D). The number of Fusobacteria and Bacteroidetes increased, while the number of Proteobacteria and Firmicutes decreased when compared to the field populations (Figure 5D and Supplementary Table 7). Next, using Manhattan plots, we analyzed the enrichment of ASVs in the wild and in captivity according to their taxonomy (Figure 5E and Supplementary Table 7). ASVs enriched in the captive group belonged to a wide range of bacterial phyla, including Bacteroidetes, Cyanobacteria, Fusobacteria, Spirochaetota, and Verrucomicrobiota (FDR adjusted P < 0.05, Wilcoxon rank sum test; Figure 5E and Supplementary Table 7). The wild group had a high abundance of enriched ASVs belonging to Actinobacteriota, Firmicutes, and Proteobacteria (FDR adjusted P < 0.05, Wilcoxon rank sum test; Figure 5E and Supplementary Table 7). These results suggested that captivity might change the structure of microbial communities in two ways: by removing some ASVs and changing the abundance of shared ASVs. In order to find fecal microbiota biomarkers that could be used to distinguish wild and captive cavefish, we used the LDA Effect Size (LEfSe) to create a model to test the correlations of wild and captive populations with fecal microbiota data at the phylum, class, order, family, genus, and ASV levels (Supplementary Figure 6). Finally, we also considered the variation of gut bacteria in species from the four phylogenetic clades following captivity. In these fish, there was no significant variability in alpha diversity (mainly Chao1 index and Shannon index) of intestinal microbes. PCoA analysis using unweighted_unifrac distance showed that Clade D is separated from clade A and Clade C, however, the weighted_unifrac distance shows that the four groups are clustered together (Supplementary Figure 7 and Supplementary Table 8).
Functional Prediction of Intestinal Microbiota and Core Bacteria in Sinocyclocheilus
We used PICRUSt2 software to perform microbiome functional analysis on 75 fecal samples from wild populations and annotated the MetaCyc Metabolic Pathway Database to gain pathway information for 24 species of gut bacteria from provenances (Supplementary Table 9). We further showed the top 35 pathways in relative abundance using a heatmap plot (Figure 6A). These were involved in three types of biological metabolic pathways: generation of precursor metabolites and energy, biosynthesis and degradation/utilization/assimilation (Figure 6A and Supplementary Table 9). Surprisingly, gut microbiota in different Sinocyclocheilus species appeared to have diverse metabolic pathways, but species from the same phylogenetic clade except for Clade B showed similar pathway abundance (Figure 5A). Among them, Clade A was comparable to Clade C group, whereas Clade D group was distinct from the other clades.
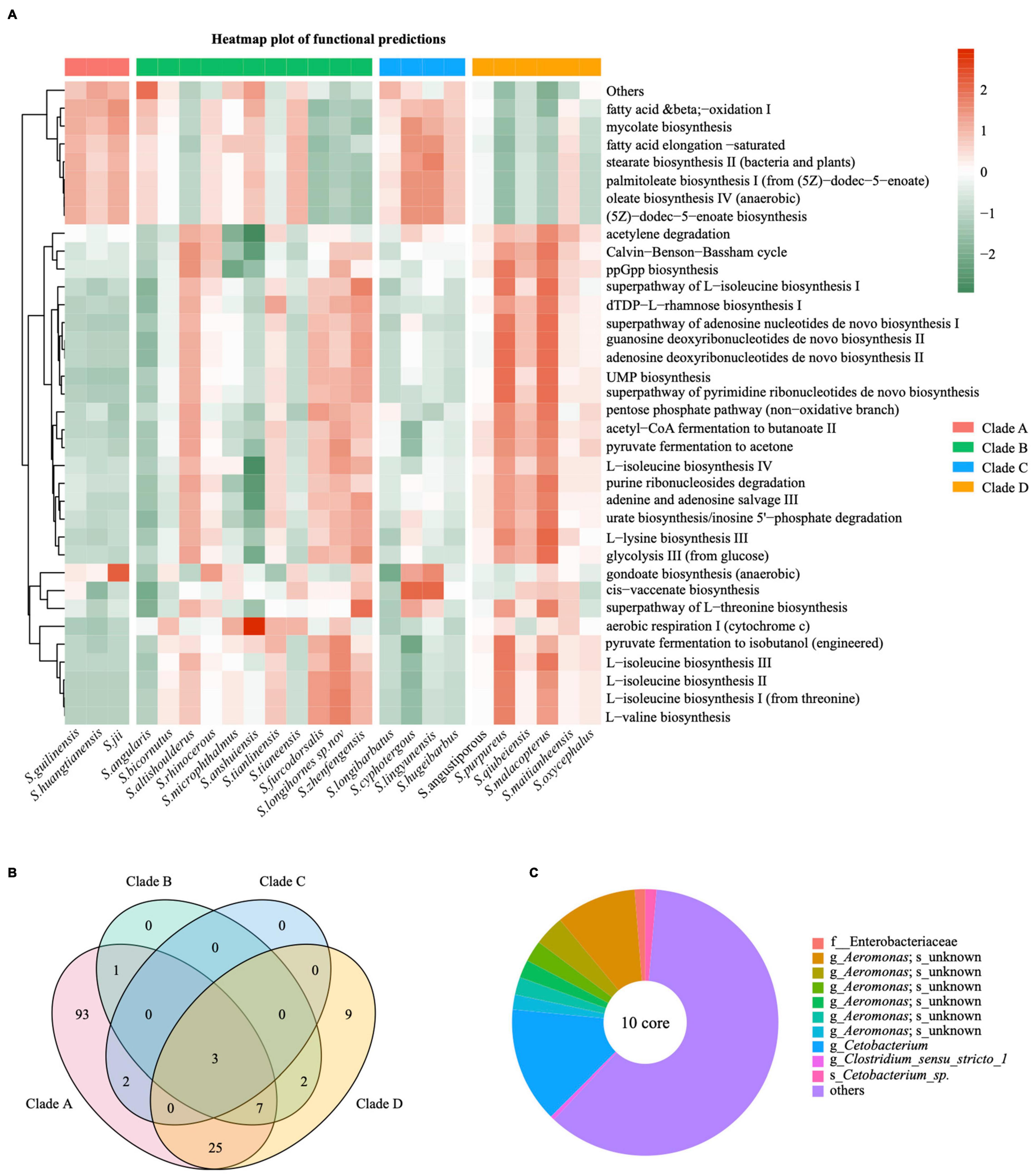
Figure 6. Functional characteristics of main bacterial taxa and the core microbiome in Sinocyclocheilus species (N = 24). (A) Heatmap illustrating the metabolic and ecological functions of gut bacteria in different species of cavefishes based on MetaCyc database. The color scale of higher (red) and lower (blue) shows the functional characteristics in the fish species. (B) Venn diagram showing the number of shared and unique ASVs among the Sinocyclocheilus species (N = 24) belonging to four clades. (C) Diagram representing the core microbiome in Sinocyclocheilus species (N = 24).
A follow-up question is whether each phylogenetic clade had a unique microbiota resulting in functional differences. We first identified specific bacteria (Biomaker) important in distinguishing among four phylogenetic clades using LDA Effect Size (LEfSe) method (Supplementary Figure 3). Next, microbiota common to species within each phylogenetic clade are of interest. Species in Clade A shared 131 ASVs, Clade B shared 13 ASVs, Clade C shared 5 ASVs and Clade D shared 46 ASVs (Figure 6B). Combined Figure 6A with Figure 6B, functional prediction of the gut flora showed that the more shared microbiomes the group had the more similar functional prediction of the samples within the group. Note that each phylogenetic clade has an uneven number of species, which may contribute to differences in the number of shared bacteria.
Despite the fact that groups of core microbes have been shown in a variety of vertebrates, especially at a higher taxonomic scale, it is worthwhile to ascertain whether specific microbial communities exists in guts of multiple species at a lower taxonomic scale, such as within a genus. To ascertain the core gut microbes of Sinocyclocheilus, we evaluated the shared ASVs across 22 Sinocyclocheilus species. Here, we did not use the single samples from Sinocyclocheilus cyphotergous and S. lugdunensis as they would have caused us to overlook several microorganisms important to Sinocyclocheilus in general. Using the Upset plot, we discovered 10 ASVs across all 22 species, and classifier analysis revealed that 6 ASVs belong to the genus Aeromonas, while the other 4 ASVs are f_Enterobacteriaceae, g_Cetobacterium, g_Clostridium_sensu_stricto_1 and s_Cetobacterium_sp. (Supplementary Figure 8 and Supplementary Table 10). These shared microbes might constitute the ‘core microbiota’ of the Sinocyclocheilus intestine. We next plotted the relative abundance of these 10 ASVs according to their occurrence for the 22 Sinocyclocheilus species (Figure 6C and Supplementary Table 10). We found that although several of these 10 ASVs were discovered in low abundance, in combination, they accounted for around one-third of all gut microbial content (Figure 6C).
Discussion
We considered the influence of phylogenetic, habitat and environmental factors, including transfer to captivity in shaping the microbiome in Sinocyclocheilus cavefishes. From these results, we evaluated the ecological and evolutionary correlates and the plasticity of the microbiome assemblages across the Sinocyclocheilus diversification. We hypothesized that the microbiome of Sinocyclocheilus is dependent primarily on habitat associations (Troglobitic – Blind; Troglophilic – Micro-Eyed; and Surface – Normal) and that phylogeny plays a secondary role. The knowledge from this study is foundational, as a diversification scale analysis of microbiome associations, in which hosts share a MRCA but diverge into multiple novel habitat types, has not been carried out so far.
Despite being a monophyletic and deeply divergent genus of freshwater fishes, the microbiome of Sinocyclocheilus showed similarities to the microbiomes of other known fish taxa at a higher taxonomic level. The Sinocyclocheilus cavefish gut microbes at the phylum level consisted mainly of Proteobacteria, Fusobacteria, and Firmicutes, representing 90% of the community. Others included smaller percentages of Bacteroidetes, Actinobacteria, Verrucomicrobia, Cyanobacteria, and Campilobacterota. Our results shows that composition and proportions of the gut microbiota of Sinocyclocheilus are similar to those of other Teleost fishes (Llewellyn et al., 2014). Despite the obvious differences in composition among Sinocyclocheilus species, the major phyla are still Proteobacteria, Fusobacteria, and Firmicutes. This is not unexpected, since the Fusobacteria phyla have been demonstrated to be the dominant amongst most freshwater and marine fish studied to date (Qian et al., 2021; Xin et al., 2021; Zhang et al., 2021).
It has been shown that host phylogeny influences microbial community structure (Brucker and Bordenstein, 2012). Previous studies have demonstrated the existence of “phylosymbiosis” in some species under laboratory or wild conditions (Brooks et al., 2016; Kohl et al., 2017; Ingala et al., 2018). Yet, in these studies, the habitats were not markedly different from each other. For example, genetic differences between populations of three-spine sticklebacks (Gasterosteus aculeatus) living in different lakes were positively correlated with differences in their gut microbiota (Smith et al., 2015). As predicted, differences in gut microbiota among Sinocyclocheilus cavefish species appear to be independent of host genetic differences. We have evaluated several possible explanations for this result: (i) In Sinocyclocheilus, even sister species occupy different habitats; hence, it appears that the ecological signature overrides the phylogenetic signal of microbiome diversity. (ii) “phylosymbiosis,” as understood at present, is explained at a higher taxonomic level of the host species, or in different populations of the same species (Phillips et al., 2012). At these two extreme scales, especially under similar habitat conditions among the species considered, it is expected to see a pattern of phylosymbiosis. (iii) Microbes that are in a close association to host phylogeny maybe absent or rarely present in fecal collections. For example, a study comparing fecal and intestinal sampling methods noted that the topology of the host phylogenetic tree and the microbiota similarity clustering tree were identical in the gut contents samples and diverged in the fecal samples (Ingala et al., 2018). Taken as a group, there is only weak support for “phylosymbiosis” among Sinocyclocheilus cavefish species, but we found that species within the same phylogenetic clade share a similar microbiome and the phylogenetic relationships between phylogenetic clades were consistent with metagenomic microbial similarity clustering relationships. However, these clades also have a distinct geographic distribution context. Of the four clades, A is at the eastern end of the Sinocyclocheilus distribution and D is at the Western end, with B and C overlapping each other in the center of the distribution. If the microbiomes of clades A and D are very different from each other, and clades B and C are similar, one would expect that distribution of the clade is important for the determination of the microbiome, rather than the phylogeny per se. And this is indeed what we observed. That is, from east to west, Proteobacteria decreases and Fusobacteria increases. Thus, the “phylosymbiosis” exists between phylogenetic clades of Sinocyclocheilus cavefish rather than species, and the geographic distribution rather than phylogeny seems to explain abundance of microbial composition.
Habitat occupation is a significant determinant of the microbiome of the cavefishes, and it seems to override the phylogeny and geographic distribution. Previous studies show that habitat type is reflected in eye condition, but with a clear distinction, where one group of Surface fish (Clade D) are permanently surface dwelling (due to lack of caves in the habitats they live in), but in the others (Clade A) where caves are available, they enter caves seasonally when the surface water dries out. Sinocyclocheilus have access to more resources as they progress from Troglobitic to Surface (Clade D); hence, the cave environment is thought to have a direct impact on the abundance of food sources available to Sinocyclocheilus species. The previous study on the relationship between gut microbial diversity and feeding habits in Sinocyclocheilus showed that the gut microbiota of cave dwelling (Troglobitic) species is more conducive to protein digestion and bile secretion, while Surface species has a preference for plant food sources (Chen et al., 2019). Our results demonstrate that there are significant differences in the gut microbiota structure of Sinocyclocheilus reflective of the three habitats, but patterns of diversity in cavefish microbiomes may not mirror habitat diversity seen in independent cave-dwelling populations. As we hypothesized, Troglobitic species have a different gut microbiome structure compared to Troglophilic and Surface species, and there is a continuous weak association in the differentiation of the microbiota from obligate Surface to Troglophilic to Troglobitic species, which is independent of the Sinocyclocheilus phylogeny. However, we point out that representative taxon sampling for the few Troglobitic species from Clade – D is lacking. When these independently derived Troglobitic taxa are added in future analyses, we presume that the PIC will show a greater independence of the microbiome diversity patterns from the phylogeny. Hence, the unique gut microbiota of the troglobitic forms can be considered as another line of evidence of their adaptation to the cave environment.
Water may have an impact on the assembly of the fish gut microbial community in two ways: (i) colonization of the fish gut by aquatic microbes (Giatsis et al., 2015) and (ii) the immediate influence of physicochemical parameters of water (Sylvain et al., 2016). Our results suggest that effects from both pathways may exist between cave water and cavefish gut microbiota differences. Because the feeding behavior of Sinocyclocheilus cavefish has not been recorded, our study did not analyze food as a source of microbiota of other potential environmental sources (for example, sediment and bat guano). Surprisingly, our results suggest that about half of the cavefish gut microbiota is formed by colonization with water-associated microbes, and demonstrate the existence of interactions between cave water-associated microorganisms and cavefish-associated microorganisms. A previous study using Bayesian community-level source tracking to estimate the source of gut microbiota in sticklebacks revealed that an average of 12.6% of fish gut microbes were of aquatic origin (Smith et al., 2015), which is markedly lower than our results. The use of fish fecal samples may be one of the reasons for the discrepancy, and it cannot be denied that microbes in fish excreta differ from those in the whole fish intestine (Nielsen et al., 2017). It is worth considering whether fact that more water-derived microbes were present in the feces than water-derived microbes in the gut suggests that some water-derived microbes have not successfully colonized in the fish gut and were excreted (evidence of rejection). Dissolved oxygen (DO), pH and Temperature were demonstrated to influence the assembly of Sinocyclocheilus cavefish gut microbes in our data. Coincidentally, DO was also an influential factor in the difference in gut microbiota between the cave population and the surface population of Astyanax mexicanus (Ornelas-Garcia et al., 2018). That is, gut microbial community diversity of cavefish appears to be sensitive to water DO concentration.
We considered captivity as an extension of the habitat where changes in the microbiome took place as an adjustment to captivity. By comparing wild and captive populations, we found that not only the observed ASV and diversity of the fish gut microbiota were significantly reduced after captivity, but also that the microbiota structure was altered. A plausible explanation is that the fish gut microbiota responded to the environmental change from complex and diverse provenances to a homogeneous environment of the laboratory. We also found that each of the 17 captive species still had a distinct, yet diminished microbiota, despite being in the same recirculating water system and fed on a similar diet. However, the differences in gut microbiota are less evident in laboratory fishes than in wild fishes. For example, NMDS analysis revealed a more similar gut microbial community structure in laboratory fishes, while in the wild fishes the microbial community structure was relatively dispersed. Since 17 species of Sinocyclocheilus cavefishes thrived under laboratory conditions, acquiring a similar microbiome over a long period of time, we conclude that these fishes are adaptable.
On the other hand, differences in the biodiversity of gut microbiota from species of the four phylogenetic clades seem to disappear following captivity (Supplementary Figure 7). Only beta diversity analysis using unweighted_unifrac distances showed that Clade D was clearly separated from Clade A and C. It should be highlighted that the number of captive species from each of the four phylogenetic clades utilized in this study is unbalanced, which could lead to bias in the results. A previous study suggested that the effects of captivity on the alpha diversity of fish gut microbes may be species-dependent, such that some species did not change after captivity (Eichmiller et al., 2016). Our results found a decrease in alpha diversity (Chao1 and Shannon indexes) for most species, but an increase in S. tianlinensis, S. lingyunensis and S. maitianheensis. We observed that there is an approximate alpha diversity index range in captive species: the Chao1 index from 100 to 150 and the Shannon index from 3 to 3.5 (Supplementary Figure 9).
A closer observation of the bacterial phyla also suggests a functional adjustment toward captivity related changes of the microbiome. In captivity, the diversity of Fusobacteria and Bacteroidetes increased, while Proteobacteria and Firmicutes decreased; in fact, Fusobacteria became the most abundant phylum of microorganisms in laboratory fish. Bacteroidetes has been shown to produce digestive enzymes and members of Bacteroidetes have a greater competitive advantage in the gut during periods of relative food deprivation (Xia et al., 2014). Captive fishes were offered food that consisted mainly of protein and fat; this may be responsible for the increase of Bacteroidetes in the intestine. Several studies of the gut microbiota in Cyprinidae have again observed that Fusobacteria is more prevalent in captive fish (Ni et al., 2014; Eichmiller et al., 2016), so the various microorganisms belonging to Fusobacteria may be more suited to the homogeneous environment of the laboratory. Thus, we concur with the opinion that not only the fish but also their gut microbiota can be domesticated.
In addition to the diverse gut microbiota of Sinocyclocheilus cavefish, our fecal bacteria related data from 22 species (nearly one-third of recorded species) point toward 10 ASVs that are shared across Sinocyclocheilus cavefish species. Functional roles of these 10 ASVs suggests that they may be important for cavefish to extract essential vitamins and maintain homeostasis. For example, Cetobacterium is a symbiotic bacterium that occupies an important ecological niche in the intestinal tract of freshwater fish (Tsuchiya et al., 2008; Ramirez et al., 2018). Members of the genus Cetobacterium have been shown to be associated with vitamin B12 synthesis in freshwater fish, as well as in aiding carbohydrate utilization by modulating glucose homeostasis (Wang et al., 2021; Xie et al., 2021). Aeromonas is a major colonizer in the gastrointestinal tract in freshwater fish (Trust et al., 1979; Haruo et al., 1985) and these release numerous proteases, thus aiding in digestion (Pemberton et al., 1997). However, some members of the genus Aeromonas, such as Aeromonas hydrophila, are conditionally pathogenic bacteria (Muduli et al., 2021). Although our results did not indicate harmful species of Aeromonas, we found a significant reduction of Aeromonas abundance in the cavefish gut after captivity.
The bacteria represented by the core microbiome constituting of 10 ASVs did not disappear following captivity, but their compositional abundance increased or decreased. Furthermore, by comparing to a study on the intestinal flora of Sinocyclocheilus cavefish, it was found that the bacteria represented by these 10ASVs were also found in the intestines of three species from a previous study (Sinocyclocheilus qujingensis, Sinocyclocheilus aluensis, and Sinocyclocheilus lateristritus) that were not used in our study (Chen et al., 2019). Therefore, a member of family Enterobacteriaceae, six members of the genus Aeromonas, two members of the genus Cetobacterium and a member of the genus Clostridium_sensu_stricto_1 represent a “core microbiota” in Sinocyclocheilus cavefish. Given that different species of Sinocyclocheilus cavefish contain these glucose homeostasis-regulating and protein-degrading microbes, there may be strong selective pressure to maintain these “core microbes.” Although Sinocyclocheilus is monophyletic, its core-microbiome does not seem to be specialized at a higher taxonomic level, contrary to what we expected.
Sinocyclocheilus species are difficult to sample due to inaccessibility of their habitats, made worse by their small population sizes. Many of the species are known only from their type locality, from a few specimens or drawings from when they were first discovered. Hence an ethical way of sampling adequate numbers of these fishes is through fecal sampling, which we resorted to here. Though fecal sampling does not capture the entire gut microbiome, it allows repeated sampling of the same population. Since Sinocyclocheilus fishes are extremely sensitive to stress, we did not attempt abdominal-stroking to force collect feces as some studies of larger and more robust fishes have done (Eichmiller et al., 2016).
Sinocyclocheilus allowed us to study microbiome community in the largest diversification of freshwater cavefishes in the world. We hypothesized habitat to be the most important determinant of microbiome diversity for this group of fish. Our results show that habitat is indeed important, as is, to a lesser extent, the phylogeny, in determining their gut microbiome. Phylogeny has a geographic context, and we showed that the most divergent clades (A and D) at the Eastern and the Western ends of the Sinocyclocheilus distribution, respectively, show the most divergent microbiomes; the two clades that overlap (B and C) have a similar microbiome. We also show that they acquire a common microbiome in captivity, irrespective of their phylogenetic position, region of origin and habitat, indicating that they are plastic in the context of microbe related changes in their environment. This further reinforces the importance of habitat as a determinant of the gut microbiome. Finally, the core microbiome of Sinocyclocheilus at a higher taxonomic level is remarkably similar to the other teleost fishes, indicating that freshwater fishes maintain a similar microbiome to achieve their physiological needs related to the aquatic realm.
Data Availability Statement
All raw data have been deposited to the NCBI database with BioProject ID PRJNA772569.
Ethics Statement
The animal study was reviewed and approved by Institutional Animal Care and Use Committee of Guangxi University (GXU), Nanning-China (#GXU2019-071).
Author Contributions
SZ and MM: conceptualization. SZ, AR, TM, YL, and JY: data curation. SZ, AR, TM, and GE: formal analysis. JY and MM: funding acquisition. SZ, AR, TM, YL, GE, JH, JY, and MM: investigation. SZ, AR, TM, YL, GE, and MM: methodology. MM: project administration. JY and MM: resources. MM: supervision. SZ, AR, TM, YL, GE, JH, JY, and MM: validation. SZ and TM: visualization. SZ, TM, AR, JH, and MM: writing original draft. SZ, AR, TM, YL, GE, JH, JY, and MM: writing review and editing.
Funding
(1) Startup funding from Guangxi University to MM for fieldwork, aquarium facilities, and lab work, (2) National Natural Science Foundation of China (#31860600) to JY for fieldwork and (3) Innovation Project of Guangxi Graduate Education (#YCBZ2021008) to SZ, TM, and YL for research work. These funding bodies played no role in the design of the study and collection, analysis, and interpretation of data and in writing the manuscript.
Conflict of Interest
The authors declare that the research was conducted in the absence of any commercial or financial relationships that could be construed as a potential conflict of interest.
Publisher’s Note
All claims expressed in this article are solely those of the authors and do not necessarily represent those of their affiliated organizations, or those of the publisher, the editors and the reviewers. Any product that may be evaluated in this article, or claim that may be made by its manufacturer, is not guaranteed or endorsed by the publisher.
Acknowledgments
We acknowledge the following individuals and organizations: Chenghai Fu, Jiajun Zhou, Yining Chen, and Tao Luo for assistance with fish sampling; Hongfu Yang (fisheries workstation in Qiubei County, Yunnan Province) for providing us with support and knowledge; Dan Sun (Guangxi University) for assistance with data analysis; and Rohan Pethiyagoda (Australian Museum) for suggestions to improve the manuscript.
Supplementary Material
The Supplementary Material for this article can be found online at: https://www.frontiersin.org/articles/10.3389/fmicb.2022.823254/full#supplementary-material
Supplementary Figure 1 | Rarefaction curves of each sample. (A) Fecal samples from 24 species of wild Sinocyclocheilus. (B) Fecal samples from 17 species of captive Sinocyclocheilus. (C) Water microbial samples from 19 caves. Different colors of the lines indicate different sample, respectively.
Supplementary Figure 2 | Microbial composition of Sinocyclocheilus species. (A) Order level. (B) Genus level. Different colors in the figures indicate the different groups, and details are shown on the right sides of each figure, respectively.
Supplementary Figure 3 | LDA Effect Size (LEfSe) method to find out microbiota biomarkers between four Phylogenetic clades.
Supplementary Figure 4 | The phylogenetic independent contrasts between standard eye diameter (sED) and gut microbial alpha diversity index.
Supplementary Figure 5 | Analysis of similarities (Anosim) test based on Bray–Curtis distance for wild group and captive group.
Supplementary Figure 6 | LDA Effect Size (LEfSe) method to find out microbiota biomarkers in wild and captive cavefish.
Supplementary Figure 7 | The biodiversity of gut microbiota in species from the four phylogenetic clades after captivity.
Supplementary Figure 8 | Ten shared ASVs among 22 species of Sinocyclocheilus.
Supplementary Figure 9 | Comparison of alpha diversity indices of Sinocyclocheilus (N = 17) before and after captivity.
Supplementary Table 1 | Sample collection information form.
Supplementary Table 2 | ASV table, Taxonomic annotation, and metadata table of wild samples.
Supplementary Table 3 | ANOVA, Tukey HSD test of phylogenetic clades.
Supplementary Table 4 | ASV table, Taxonomic annotation and metadata table of water and fish microbiota.
Supplementary Table 5 | Water chemistry with the results of its analysis.
Supplementary Table 6 | ASV table, Taxonomic annotation and metadata table of Captive population.
Supplementary Table 7 | Changes in the bacterial assemblages from wild to captivity. (A) ANOVA, Tukey HSD test of wild and captive groups; (B) overlapped 426 ASVs in Wild and Captive populations; (C) relative abundance of the two groups at the Phylum level; (D) differential abundance of ASVs between Wild and Captive populations.
Supplementary Table 8 | Statistical test results of the biodiversity of gut microbiota in species from the four phylogenetic clades after captivity.
Supplementary Table 9 | Pathway information annotated the MetaCyc Metabolic Pathway Database.
Supplementary Table 10 | Ten core microbiome of Sinocyclocheilus and their abundance.
References
Brooks, A. W., Kohl, K. D., Brucker, R. M., Opstal, E., and Bordenstein, S. R. (2016). Phylosymbiosis: relationships and functional effects of microbial communities across host evolutionary history. PLoS Biol. 14:e2000225. doi: 10.1371/journal.pbio.2000225
Brucker, R. M., and Bordenstein, S. R. (2012). The roles of host evolutionary relationships (genus: Nasonia) and development in structuring microbial communities. Evolution 66, 349–362. doi: 10.1111/j.1558-5646.2011.01454.x
Chen, C., Chen, H., Zhang, Y., Thomas, H. R., and Xia, R. (2020). TBtools: an Integrative Toolkit Developed for Interactive Analyses of Big Biological Data. Mol. Plant 2020:13. doi: 10.1016/j.molp.2020.06.009
Chen, H., Li, C., Liu, T., Chen, S., and Xiao, H. (2019). A metagenomic study of intestinal microbial diversity in relation to feeding habits of surface and cave-dwelling Sinocyclocheilus Species. Microb. Ecol. 79, 299–311. doi: 10.1007/s00248-019-01409-4
Eichmiller, J. J., Hamilton, M. J., Staley, C., Sadowsky, M. J., and Sorensen, P. W. (2016). Environment shapes the fecal microbiome of invasive carp species. Microbiome 4:44. doi: 10.1186/s40168-016-0190-1
Felsenstein, J. (1985). Phylogenies and the Comparative Method. Am. Natural. 125, 1–15. doi: 10.1086/284325
Giatsis, C., Sipkema, D., Smidt, H., Heilig, H., Benvenuti, G., Verreth, J., et al. (2015). The impact of rearing environment on the development of gut microbiota in tilapia larvae. Sci. Rep. 5:18206. doi: 10.1038/srep18206
Gill, S. R., Pop, M., DeBoy, R. T., Eckburg, P. B., Turnbaugh, P. J., Samuel, B. S., et al. (2006). Metagenomic analysis of the human distal gut microbiome. Science 312, 1355–1359. doi: 10.1126/science.1124234
Grond, K., Bell, K. C., Demboski, J. R., Santos, M., Sullivan, J. M., and Hird, S. M. (2020). No evidence for phylosymbiosis in western chipmunk species. Fems Microbiol. Ecol. 96:1. doi: 10.1093/femsec/fiz182
Gross, J. B., Protas, M., Conrad, M., Scheid, P. E., Vidal, O., Jeffery, W. R., et al. (2008). Synteny and candidate gene prediction using an anchored linkage map of Astyanax mexicanus. Proc. Natl. Acad. Sci. U.S.A. 105, 20106–20111. doi: 10.1073/pnas.0806238105
Harer, A., Torres-Dowdall, J., Rometsch, S. J., Yohannes, E., Machado-Schiaffino, G., and Meyer, A. (2020). Parallel and non-parallel changes of the gut microbiota during trophic diversification in repeated young adaptive radiations of sympatric cichlid fish. Microbiome 8:1. doi: 10.1186/s40168-020-00897-8
Hart, P. B., Niemiller, M. L., Burress, E. D., Armbruster, J. W., Ludt, W. B., and Chakrabarty, P. (2020). Cave-adapted evolution in the North American amblyopsid fishes inferred using phylogenomics and geometric morphometrics. Evolution 74, 936–949. doi: 10.1111/evo.13958
Haruo, S., Tokuyama, K., and Deguchi, Y. (1985). The intestinal microflora of carp Cyprinus carpio, crass carp Ctenopharyngodon idella and Tilapia Sarotherodon niloticus. Bull. Japan Soc. Sci. Fish. 51, 1325–1329. doi: 10.2331/suisan.51.1325
Hooper, L. V., and Gordon, J. I. (2001). Commensal host-bacterial relationships in the gut. Science 292, 1115–1118. doi: 10.1126/science.1058709
Ingala, M. R., Simmons, N. B., Claudia, W., Konstantinos, K., Speer, K. A., and Perkins, S. L. (2018). Comparing microbiome sampling methods in a wild mammal: Fecal and intestinal samples record different signals of host ecology, evolution. Front. Microbiol. 9:803. doi: 10.3389/fmicb.2018.00803
Jiang, W. S., Li, J., Lei, X. Z., Wen, Z. R., Han, Y. Z., Yang, J. X., et al. (2019). Sinocyclocheilus sanxiaensis, a new blind fish from the three Gorges of Yangtze river provides insights into speciation of Chinese cavefish. Zool. Res. 40, 552–557. doi: 10.24272/j.issn.2095-8137.2019.065
Kohl, K. D., Varner, J., Wilkening, J. L., and Dearing, M. (2017). Gut microbial communities of American pikas (Ochotona princeps): evidence for phylosymbiosis and adaptations to novel diets. J. Anim. Ecol. 87, 323–330. doi: 10.1111/1365-2656.12692
Lim, S. J., and Bordenstein, S. R. (2020). An introduction to phylosymbiosis. Proc. R. Soc. B-Biol. Sci. 287:1922. doi: 10.1098/rspb.2019.2900
Liu, Y. X., Qin, Y., Chen, T., Lu, M., and Bai, Y. (2021). A practical guide to amplicon and metagenomic analysis of microbiome data. Protein Cell 315–330. doi: 10.1007/s13238-020-00724-8
Llewellyn, M. S., Boutin, S., Hoseinifar, S. H., and Derome, N. (2014). Teleost microbiomes: The state of the art in their characterization, manipulation and importance in aquaculture and fisheries. Front. Microbiol. 5:207. doi: 10.3389/fmicb.2014.00207
Magoc, T., and Salzberg, S. L. (2011). FLASH: fast length adjustment of short reads to improve genome assemblies. Bioinformatics 27, 2957–2963. doi: 10.1093/bioinformatics/btr507
Mao, T. R., Liu, Y. W., Meegaskumbura, M., Yang, J., Ellepola, G., Senevirathne, G., et al. (2021). Evolution in Sinocyclocheilus cavefish is marked by rate shifts, reversals, and origin of novel traits. BMC Ecol. Evol. 21:45. doi: 10.1186/s12862-021-01776-y
Mao, T. R., Liu, Y. W., Vasconcellos, M. M., Pie, M. R., Ellepola, G., Fu, C. H., et al. (2022). Evolving in the darkness: phylogenomics of Sinocyclocheilus cavefishes highlights recent diversification and cryptic diversity. Mole. Phyl. Evol. 168, 1055–7903. doi: 10.1016/j.ympev.2022.107400
Mazmanian, S. K., Liu, C. H., Tzianabos, A. O., and Kasper, D. L. (2005). An immunomodulatory molecule of symbiotic bacteria directs maturation of the host immune system. Cell 122, 107–118. doi: 10.1016/j.cell.2005.05.007
Mcfall-Ngai, M., Hadfield, M. G., Bosch, T., Carey, H. V., and Wernegreen, J. J. (2013). Animals in a bacterial world, a new imperative for the life sciences. Proc. Natl. Acad. Sci. U.S.A. 110, 3229–3236. doi: 10.1073/pnas.1218525110
Muduli, C., Tripathi, G., Paniprasad, K., Kumar, K., Singh, R. K., and Rathore, G. (2021). Virulence potential of Aeromonas hydrophila isolated from apparently healthy freshwater food fish. Biologia 76, 1005–1015. doi: 10.2478/s11756-020-00639-z
Nayak, S. K. (2010). Role of gastrointestinal microbiota in fish. Aquac. Res. 41, 1553–1573. doi: 10.1111/j.1365-2109.2010.02546.x
Ni, J., Yan, Q., Yu, Y., and Zhang, T. (2014). Factors influencing the grass carp gut microbiome and its effect on metabolism. FEMS Microbiol. Ecol. 87, 704–714. doi: 10.1111/1574-6941.12256
Nicholson, J. K., Holmes, E., and Wilson, I. D. (2005). Gut microorganisms, mammalian metabolism and personalized health care. Nat. Rev. Microbiol. 3, 431–438. doi: 10.1038/nrmicro1152
Nielsen, S., Walburn, J. W., Verges, A., Thomas, T., and Egan, S. (2017). Microbiome patterns across the gastrointestinal tract of the rabbitfish Siganus fuscescens. PeerJ 2017:5. doi: 10.7717/peerj.3317
Ornelas-Garcia, P., Pajares, S., Sosa-Jimenez, V. M., Retaux, S., and Miranda-Gamboa, R. A. (2018). Microbiome differences between river-dwelling and cave-adapted populations of the fish Astyanax mexicanus (De Filippi, 1853). PeerJ 6:e5906. doi: 10.7717/peerj.5906
Pemberton, J. M., Kidd, S. P., and Schmidt, R. (1997). Secreted enzymes of Aeromonas. FEMS Microbiol. Lett. 152, 1–10. doi: 10.1111/j.1574-6968.1997.tb10401.x
Phillips, C. D., Phelan, G., Dowd, S. E., Mcdonough, M. M., Ferguson, A. W., Hanson, J. D., et al. (2012). Microbiome analysis among bats describes influences of host phylogeny, life history, physiology and geography. Mol. Ecol. 21, 2617–2627. doi: 10.1111/j.1365-294X.2012.05568.x
Qian, M. R., Wang, J. M., Ji, X. F., Yang, H., Tang, B., Zhang, H., et al. (2021). Sub-chronic exposure to antibiotics doxycycline, oxytetracycline or florfenicol impacts gut barrier and induces gut microbiota dysbiosis in adult zebrafish (Daino rerio). Ecotoxicol. Environm. Saf. 221:12. doi: 10.1016/j.ecoenv.2021.112464
Qin, J. J., Li, R. Q., Raes, J., Arumugam, M., Burgdorf, K. S., Manichanh, C., et al. (2010). A human gut microbial gene catalogue established by metagenomic sequencing. Nature 464, 59–U70. doi: 10.1038/nature08821
Ramirez, C., Coronado, J., Silva, A., and Romero, J. (2018). Cetobacterium is a major component of the microbiome of Giant Amazonian Fish (Arapaima gigas) in Ecuador. Animals 8:189. doi: 10.3390/ani8110189
Rennison, D. J., Rudman, S. M., and Schluter, D. (2019). Parallel changes in gut microbiome composition and function during colonization, local adaptation and ecological speciation. Proc. R. Soc. B-Biol. Sci. 286:1916. doi: 10.1098/rspb.2019.1911
Ross, A. A., Muller, K. M., Weese, J. S., and Neufeld, J. D. (2018). Comprehensive skin microbiome analysis reveals the uniqueness of human skin and evidence for phylosymbiosis within the class Mammalia. Proc. Natl. Acad. Sci. U S A. 115, E5786–E5795. doi: 10.1073/pnas.1801302115
Sevellec, M., Laporte, M., Bernatchez, A., Derome, N., and Bernatchez, L. (2019). Evidence for host effect on the intestinal microbiota of whitefish (Coregonus sp.) species pairs and their hybrids. Ecol. Evol. 9, 11762–11774. doi: 10.1002/ece3.5676
Shapira, M. (2016). Gut microbiotas and host evolution: scaling up symbiosis. Trends Ecol. Evol. 31, 539–549. doi: 10.1016/j.tree.2016.03.006
Shenhav, L., Thompson, M., Joseph, T. A., Briscoe, L., Furman, O., Bogumil, D., et al. (2019). FEAST: fast expectation-maximization for microbial source tracking. Nat. Methods 16, 627–632. doi: 10.1038/s41592-019-0431-x
Smith, C. C. R., Snowberg, L. K., Caporaso, J. G., Knight, R., and Bolnick, D. I. (2015). Dietary input of microbes and host genetic variation shape among-population differences in stickleback gut microbiota. ISME J. 9, 2515–2526. doi: 10.1038/ismej.2015.64
Sullam, K. E., Rubin, B. E. R., Dalton, C. M., Kilham, S. S., Flecker, A. S., and Russell, J. A. (2015). Divergence across diet, time and populations rules out parallel evolution in the gut microbiomes of Trinidadian guppies. ISME J. 9, 1508–1522. doi: 10.1038/ismej.2014.231
Sylvain, F. E., Cheaib, B., Llewellyn, M., Correia, T. G., Fagundes, D. B., Val, A. L., et al. (2016). pH drop impacts differentially skin and gut microbiota of the Amazonian fish tambaqui (Colossoma macropomum). Sci. Rep. 2016:6. doi: 10.1038/srep32032
Talwar, C., Nagar, S., Lal, R., and Negi, R. K. (2018). Fish gut microbiome: current approaches and future perspectives. Indian J. Microbiol. 58, 397–414. doi: 10.1007/s12088-018-0760-y
Trust, T. J., Bull, L. M., Currie, B. R., and Buckley, J. T. (1979). Obligate anaerobic bacteria in the gastrointestinal microflora of the Grass carp (Ctenopharyngodon idella). Goldfish (Carassius auratus), and Rainbow trout (Salmo gairdneri). J. Fish. Res. Board Can. 36, 1174–1179. doi: 10.1139/f79-169
Tsuchiya, C., Sakata, T., and Sugita, H. (2008). Novel ecological niche of Cetobacterium somerae, an anaerobic bacterium in the intestinal tracts of freshwater fish. Lett. Appl. Microbiol. 46, 43–48. doi: 10.1111/j.1472-765X.2007.02258.x
Turnbaugh, P. J., Hamady, M., Yatsunenko, T., Cantarel, B. L., Duncan, A., Ley, R. E., et al. (2009). A core gut microbiome in obese and lean twins. Nature 457, 480–484. doi: 10.1038/nature07540
Turnbaugh, P. J., Quince, C., Faith, J. J., Mchardy, A. C., Yatsunenko, T., Niazi, F., et al. (2010). Organismal, genetic, and transcriptional variation in the deeply sequenced gut microbiomes of identical twins. Proc. Natl. Acad. Sci. U.S.A. 107, 7503–7508. doi: 10.1073/pnas.1002355107
Wang, A., Zhang, Z., Ding, Q., Yang, Y., Bindelle, J., Ran, C., et al. (2021). Intestinal Cetobacterium and acetate modify glucose homeostasis via parasympathetic activation in zebrafish. Gut Microbes 13:e1900996. doi: 10.1080/19490976.2021.1900996
Wong, A. C. N., Chaston, J. M., and Douglas, A. E. (2013). The inconstant gut microbiota of Drosophila species revealed by 16S rRNA gene analysis. ISME J. 7, 1922–1932. doi: 10.1038/ismej.2013.86
Xia, J., Lin, G., Fu, G., Wan, Z., Lee, M., Wang, L., et al. (2014). The intestinal microbiome of fish under starvation. BMC Genom. 15:266. doi: 10.1186/1471-2164-15-266
Xie, M. X., Zhou, W., Xie, Y. D., Li, Y., Zhang, Z., Yang, Y. L., et al. (2021). Effects of Cetobacterium somerae fermentation product on gut and liver health of common carp (Cyprinus carpio) fed diet supplemented with ultra-micro ground mixed plant proteins. Aquaculture 543, 736943. doi: 10.1016/j.aquaculture.2021.736943
Xin, J. J., Yan, S. H., Hong, X. S., Zhang, H., and Zha, J. M. (2021). Environmentally relevant concentrations of clozapine induced lipotoxicity and gut microbiota dysbiosis in Chinese rare minnow (Gobiocypris rarus). Environ. Pollut. 286:117298. doi: 10.1016/j.envpol.2021.117298
Yang, J., Chen, X., Bai, J., Fang, D., Qiu, Y., Jiang, W., et al. (2016). The cavefish genome provides insights into cave adaptation. BMC Biol. 2016, 1–13.
Zhang, G., Yang, W., Liang, Y., Wenze, F. U., Zhang, R., and Zhou, X. (2015). Correlation study between cave animal community and the cavern environment in dolomite karst area, Guizhou province. Sich. J. Zool. 34, 375–382.
Zhang, X., Wen, K., Ding, D. X., Liu, J. T., Lei, Z., Chen, X. X., et al. (2021). Size-dependent adverse effects of microplastics on intestinal microbiota and metabolic homeostasis in the marine medaka (Oryzias melastigma). Environ. Int. 151:14. doi: 10.1016/j.envint.2021.106452
Zhao, Y., and Zhang, C. (2006). Cavefishes: concept, diversity and research progress. Biodiv. Sci. 14, 451–460. doi: 10.1360/biodiv.050226
Zhao, Y., and Zhang, C. (2009). Endemic fshes of Sinocyclocheilus (Cypriniformes: Cyprinidae) in China-species diversity, cave adaptation, systematics and zoogeography. Beijing: Science Press.
Keywords: fecal microbiome, Sinocyclocheilus, 16S rRNA, phylosymbiosis, core-microbiome, habitat, captive, wild
Citation: Zhou S, Rajput AP, Mao T, Liu Y, Ellepola G, Herath J, Yang J and Meegaskumbura M (2022) Adapting to Novel Environments Together: Evolutionary and Ecological Correlates of the Bacterial Microbiome of the World’s Largest Cavefish Diversification (Cyprinidae, Sinocyclocheilus). Front. Microbiol. 13:823254. doi: 10.3389/fmicb.2022.823254
Received: 27 November 2021; Accepted: 09 February 2022;
Published: 14 March 2022.
Edited by:
Franck Carbonero, Washington State University Health Sciences Spokane, United StatesReviewed by:
Seth Rudman, Washington State University Vancouver, United StatesTongtong Li, Zhejiang University of Technology, China
Libby Wilson, Kansas State University, United States
Copyright © 2022 Zhou, Rajput, Mao, Liu, Ellepola, Herath, Yang and Meegaskumbura. This is an open-access article distributed under the terms of the Creative Commons Attribution License (CC BY). The use, distribution or reproduction in other forums is permitted, provided the original author(s) and the copyright owner(s) are credited and that the original publication in this journal is cited, in accordance with accepted academic practice. No use, distribution or reproduction is permitted which does not comply with these terms.
*Correspondence: Madhava Meegaskumbura, bWFkaGF2YV9tQG1hYy5jb20=