- 1Unité de Recherche Physiologie, Pathologie, et Génétique Végétales (PPGV), INP PURPAN, Université de Toulouse, Toulouse, France
- 2Laboratoire de Recherche en Sciences Végétales, CNRS, UPS, Toulouse INP, Université de Toulouse, Toulouse, France
- 3Metatoul-AgromiX Platform, MetaboHUB, National Infrastructure for Metabolomics and Fluxomics, LRSV, CNRS, UPS, Toulouse INP, Université de Toulouse, Toulouse, France
- 4MetaboHUB-MetaToul, National Infrastructure of Metabolomics and Fluxomics, Toulouse, France
Given their well-known antifungal abilities, species of the genus Trichoderma are of significant interest in modern agriculture. Recent studies have shown that Trichoderma species can induce plant resistance against different phytopathogens. To further extend this line of investigation, we investigate herein the transcriptomic response of grapevine trunk to Vintec®, which is a Trichoderma atroviride SC1-based commercial formulation for biological control of grapevine trunk diseases and which reduces wood colonization. The aim of the study is to understand whether the biocontrol agent Vintec® modifies the trunk response to Phaeoacremonium minimum and Phaeomoniella chlamydospora, which are two esca-associated fungal pathogens. An analysis of transcriptional regulation identifies clusters of co-regulated genes whose transcriptomic reprogramming in response to infection depends on the absence or presence of Vintec®. On one hand, the results show that Vintec® differentially modulates the expression of putative genes involved in hormonal signaling, especially those involved in auxin signaling. On the other hand, most significant gene expression modifications occur among secondary-metabolism-related genes, especially regarding phenylpropanoid metabolism and stilbene biosynthesis. Taken together, these results suggest that the biocontrol agent Vintec® induces wood responses that counteract disease development.
Introduction
Crops worldwide face multiple diseases that cause huge economic loss (Savary et al., 2019). Over the past decades, pest management has relied mostly on chemical control, which has caused serious harm to environmental and human health. An alternative to these conventional phytosanitary products is the use of living organisms or natural substances; a strategy known as biological control (Eilenberg et al., 2001). Although biological control offers numerous advantages over chemical pesticides, its main advantages are that it is environmentally friendly, cost-effective, and human safe technology (Cook, 1988; Bhat et al., 2013; Brodeur et al., 2018; Ram et al., 2018).
The main approach to managing plant disease via biological control consists in using antagonistic fungi or bacteria to combat plant pathogens (Thambugala et al., 2020). In this regard, the genus Trichoderma is of great interest (Vinale et al., 2008; Zin and Badaluddin, 2020; Ferreira and Musumeci, 2021). Trichoderma is a genus of fungi inhabiting a large spectrum of soils and its species are opportunistic plant symbionts (Vinale et al., 2008) that attack or compete with other fungi by using various direct mechanisms, such as the production of antimicrobial compounds or lytic enzymes or the competition for resources (Kubicek et al., 2001; Benítez et al., 2004). To date, 25 Trichoderma species have been identified as potential biological control agents (BCAs) and several commercial formulations based on different strains of Trichoderma are available (Ferreira and Musumeci, 2021).
Trichoderma spp. also act indirectly by reprogramming gene expression in the host (Shaw et al., 2016). The associations between Trichoderma spp. and the host plant often improve plant resistance to disease, augment abiotic stress tolerance, and enhance other developmental processes. For instance, Zhao et al. (2021) reported increased growth of tomato plants upon inoculation by T. afroharzianum. In addition, T. longibrachiatum reportedly enhances the salt tolerance of wheat (Zhang et al., 2016) and the water-stress response on tomato (De Palma et al., 2019). T. harzianum enhances the antioxidant response (Mastouri et al., 2012) and also stimulates the production of jasmonic acid and salicylic acid, which reduces the incidence of root nematodes on tomato seedlings (Yan et al., 2021).
In viticulture, biological control has attracted significant interest, especially against pathogens associated with grapevine trunk diseases (GTDs). GTDs are a growing concern because of their increasing incidence in vineyards worldwide, which reduces yield and productivity, degrading significantly the economics of the viticulture industry (Fontaine et al., 2016; Mondello et al., 2018a,b). Esca, Botryosphaeria dieback, and Eutypa dieback are currently the three main GTDs in European vineyards. Esca is a complex disease with symptoms that vary depending on the age of the vine and the infecting pathogen (Mugnai et al., 1999; Graniti et al., 2000; Bertsch et al., 2013). Numerous pathogenic agents are associated with Esca; the principal causing agents are Phaeomoniella chlamydospora, Phaeoacremonium minimum (previously Phaeoacremonium aleophylum) (Larignon et al., 2000), Fomitiporia mediterranea, and other Phaeoacremonium spp. (Gramaje et al., 2015). Since the ban of sodium arsenate and benzimidazoles in the early 2000s, no effective treatment has been found to eradicate these diseases. Instead, an integrated pest-management strategy has been established that consists of different prophylactic treatments both in nurseries and in vineyards (Mondello et al., 2018a,b).
In recent years, the use of various strains of Trichoderma has produced good results against pathogens associated with these diseases. In grapevine nurseries, the application of T. harzianum during propagation proved as effective in combating these diseases as the use of conventional phytosanitary products (Fourie et al., 2001). Similar results were obtained by Di Marco et al. (2004); Di Marco and Osti (2007), who reported a reduced incidence of esca-associated pathogens and improved morphological features. In vineyards, the application of various Trichoderma spp. for protecting pruning wounds reduced the presence of pathogens (Kotze et al., 2011), decreasing the percentage of infected plants and reducing the severity of symptoms (Bigot et al., 2020).
In France, three Trichoderma-based biocontrol commercial solutions certified against GTDs are currently available. One is a combination of T. asperellum ICC012 and T. gamsi ICC080 (Escalator®, De Sangosse); the other two are formulations of T. atroviride I1237 (Esquive WP®, Agrauxine by Lesaffre) and T. atroviride SC1 (Vintec®, Belchim Crop Protection). Recent studies designed to evaluate the efficacy of Vintec® showed promising results in the battle against GTDs. Pertot et al. (2016) reported that the application of the BCA Vintec® at different stages of the propagation process decreases vine infections by P. minimum and P. chlamydospora. In addition, Berbegal et al. (2020) confirmed that the application of the BCA Vintec® in the nursery reduces the incidence of diverse GTDs and showed that subsequent treatments in vineyards provide long-term protection against GTDs.
The results of a previous study show that the application of the BCA Vintec® on grapevine wood triggers significant metabolic changes upon infection with P. chlamydospora and P. minimum. Three weeks after infection, the different classes of stilbenoid and flavonoid compounds, known for their antifungal properties, are differently produced in plants pre-inoculated with Vintec® compared with non-pre-inoculated plants (Chervin et al., 2022). In the present work, we investigate rapid transcriptomic reprogramming in grapevine trunk in response to inoculation with the BCA Vintec®, which is a commercial T. atroviride SC1-based solution. The aims of this study are thus (i) to investigate the plant molecular mechanisms modified by the presence of the BCA Vintec® and (ii) to determine whether the Vintec® treatment enhances plant response against the two esca-associated pathogens P. chlamydospora and P. minimum.
Materials and Methods
Fungal Material
This study used the fungal strains P. minimum CBS 100398 and P. chlamydospora CBS 239.74 from the Westerdijk Fungal Biodiversity Institute (Utrecht, Netherlands). Both fungi were grown in Malt Extract-Agar (MEA) at 26°C in the dark. The BCA Vintec® (T. atroviride SC1, 2 × 1010 conidia per gram of formulated product) was provided by Belchim Crop Protection, and the Vintec® suspension was prepared at 2 g/L in autoclaved distilled water.
Plant Material
One-year-old canes of Cabernet Sauvignon clone 15 were collected from Daydé Nurseries (Pépinières Daydé, Montans, Occitanie, France) in January 2019 and divided into two-node dormant cuttings. The cuttings were surface-disinfected in a 10 L bath of 0.05% bleach (2.6% active chloride) for 30 s, then in a 10 L bath of 0.05% 8-hydroxyquinoline sulfate (Beltanol®, Syngenta) for 12 h in a cold chamber (4°C). After the bleach disinfection, the cuttings were rinsed twice with clear water, and after the 8-hydroxyquinoline sulfate disinfection, the cuttings were rinsed three times with clear water. Cuttings were first planted in autoclaved (121°C, 15 min) mineral wool to allow rooting and budding. Three weeks after the emergence of the first leaf, cuttings were transferred to individual pots filled with potting substrate (PAM2, PROVEEN Substrates). The plants were then grown in plant growth tents (photoperiod 12 h/12 h, 45% humidity, 25°C).
Plant Inoculation
The plants were drilled at the internode and inoculated with 20 μL of Vintec® suspension or 20 μL of sterile water. Five days after Vintec® inoculation, the plants were infected with two plugs of colonized agar, one of each fungus. The injuries were protected with parafilm (American National Can, Chicago, United States). After the infection, five different conditions were studied: non-injured/non-infected plants (NINi, n = 25), injured/non-infected plants (INi, n = 25), inoculated with Vintec® (IV, n = 25), infected with both fungi (IPP, n = 25), and inoculated with Vintec® and infected with both fungi (IVPP, n = 25). Wood samples of 1 cm above and below the inoculation site were taken at 48 h post-infection (hpi) and 6 weeks post-infection (wpi). Four biological replicates consisting of a pool of four plants were collected for each condition at 48 hpi. At the latest time (i.e., 6 wpi), only three pools of three plants were taken. Immediately after collection, the samples were frozen in liquid nitrogen and stored at –80°C. Before RNA and DNA extraction, the samples were lyophilized and ground. The samples collected 6 wpi were used to confirm pathogen infection via qPCR quantification of the Pm/Pch β-tubulin gene as per Pouzoulet et al. (2013).
RNA Extraction, DNAse Treatment, and Reverse Transcription
One milliliter of RNA extraction buffer (CTAB 2%, PVPP 2%, Tris 300 mM, EDTA 25 mM, NaCl 2 M, pH 8, 2% β-mercaptoethanol) was added to one hundred milligrams of wood powder and the mix was incubated 10 min at 65°C under continuous agitation (9,000 rpm). The samples were then centrifuged for 15 min at 10,000 rpm, 4°C. One volume of chloroform: isoamyl alcohol (24/1, v/v) solution was added to the supernatant, following which the solution was centrifuged for 15 min at 10,000 rpm, 4°C to form two phases. The upper aqueous phase contains nucleic acids. This stage was repeated twice.
To allow the nucleic acids to precipitate, 0.6 volume of isopropanol and 0.1 volume of CH3COONa 3M were added to the upper phase, following which the samples were stored overnight at –80°C. The next day, the samples were centrifuged for 30 min at 10,000 rpm, 4°C. The resulting pellet was redissolved in 300 μL of SSTE buffer (NaCl 1 M, SDS 0.5%, Tris 10 mM, EDTA 1 mM, pH 7) and 600 μL of pure ethanol. The mixture was transferred to a mini spin column (RNeasy mini kit, Qiagen, United States). The successive steps were done as described in the kit handbook. RNA was eluted in 30 μL of RNase-free water, aliquoted in 6 μL samples, and stored at –80°C. RQ1 RNase-Free DNase kit (Promega, United States) was used to eliminate the possible co-purified genomic DNA.
RNA Sequencing and Data Analysis
cDNA library preparation and sequencing were done by using the genomic platform GeT-Plage (GenoToul, INRA, Toulouse, France). The cDNA libraries were prepared with the help of TruSeq Stranded mRNA (Illumina) and then sequenced by using an Illumina HiSeq 3000 sequencer as paired-end reads of 150 bp (Illumina, CA, United States). Quality control was done by using fastqc (v0.11.9; Andrews, 2010). If necessary, quality trimming was done by using sickle-trim (v1.33; Joshi and Fass, 2011) or cutadapt (v2.10; Martin, 2011) to remove adapters. Mapping to the reference genome 12X (Canaguier et al., 2017) was done by using STAR (v2.7.5a; Dobin et al., 2013), and mapped counts were extracted by using featureCounts (subread v2.0.1; samtools v1.9; Liao et al., 2014). Differential expression analysis was performed using the R package SARTools1. All conditions were compared with the NINi control (i.e., INi vs NINi, IV vs NINi, IPP vs NINi, IVPP vs NINi) and differentially expressed genes (DEGs; p-value < 0.05) were extracted. DEGs induced by the injury (INi vs NINi) were discarded for the remainder of the analysis. DEG functional annotations were assessed by using the VitisNet functional annotation database (Grimplet et al., 2009). Clustering was analyzed by using R with the pheatmap package, and functional enrichment was calculated in R by using Fisher’s exact test.
RT-qPCR
Five genes were tested by RT-qPCR to validate RNA seq results. Gene candidates (listed in Table 1) satisfied the following criteria: (i) they were differentially expressed compared with the control condition, (ii) they were specific to a single condition, and (iii) they served an interesting putative biological function. For RT-qPCR analysis, cDNA was first synthesized from RNA samples by reverse transcriptase reaction using the GoScript® reverse transcriptase Kit (Promega, United States). RT-qPCR reactions were done in an ABI 7500 Real-Time PCR cycler (Applied Biosystems, Foster City, United States) using GoTaq RT-qPCR systems (Promega, United States) in a final volume of 10 μL. Primers were designed by using Primer Blast (Ye et al., 2012; Optimal Tm = 62°C, 50–60% GC) and were used at a concentration of 0.5 μM. The cycling program consisted of 95°C for 5 min (initial denaturation); 40 cycles of 15 s at 95°C (denaturation) followed by 45 s at 62°C (annealing and extension); and an additional melting analysis of 40 min from 60 to 95°C. The ABI SDS software v.1.4 (Applied Biosystems, Foster City, United States) was used to collect the amplification data. Gene expression was calculated by using the 2–ddCt method (Zhang et al., 2020).
Results
Transcriptomic Woody Response to BCA Vintec®, Pathogens, and Wound
We performed RNA sequencing on wood samples inoculated with the BCA Vintec® (IV), the two pathogens (IPP), or the BCA Vintec® in combination with pathogens (IVPP). An injured control (INi) was added to the analysis to select DEGs affected only by the treatment and not by the injury. We obtained an average of 20.4 M reads per condition and pool. The percentage of reads aligned to the reference genome of Vitis vinifera (12X.0 version, cv PN40024) varies depending on the condition (see Supplementary Table 1). The transcriptome of reference (Vitis_vinifera.12X.48.chr.gtf.gz, from Ensembl plants genomes) was used for gene annotation. Differential analysis was applied to transcript normalized counts. All test conditions were compared with the non-injured/non-inoculated control (i.e., INi vs NINi; IPP vs NINi; IV vs NINi; and IVPP vs NINi).
The injury alone modified the expression of 1,869 genes (p-value < 0.05), whereas the number of DEGs induced by the BCA Vintec® alone, the two pathogens, and the BCA Vintec® in combination with the two pathogens was 2,114, 3,748, and 3,519, respectively. From these sets of DEGs, we discarded those that were in response to injury, which left 1,442 DEGs in response to the BCA Vintec®, 3204 DEGs in response to the two pathogens, and 2,739 DEGs in response to the two pathogens in the presence of the BCAVintec® (Figure 1A). The pattern expression of five candidate genes was confirmed by qRT-PCR analysis (Supplementary Figures 1, 2) as validation of the RNAseq analysis.
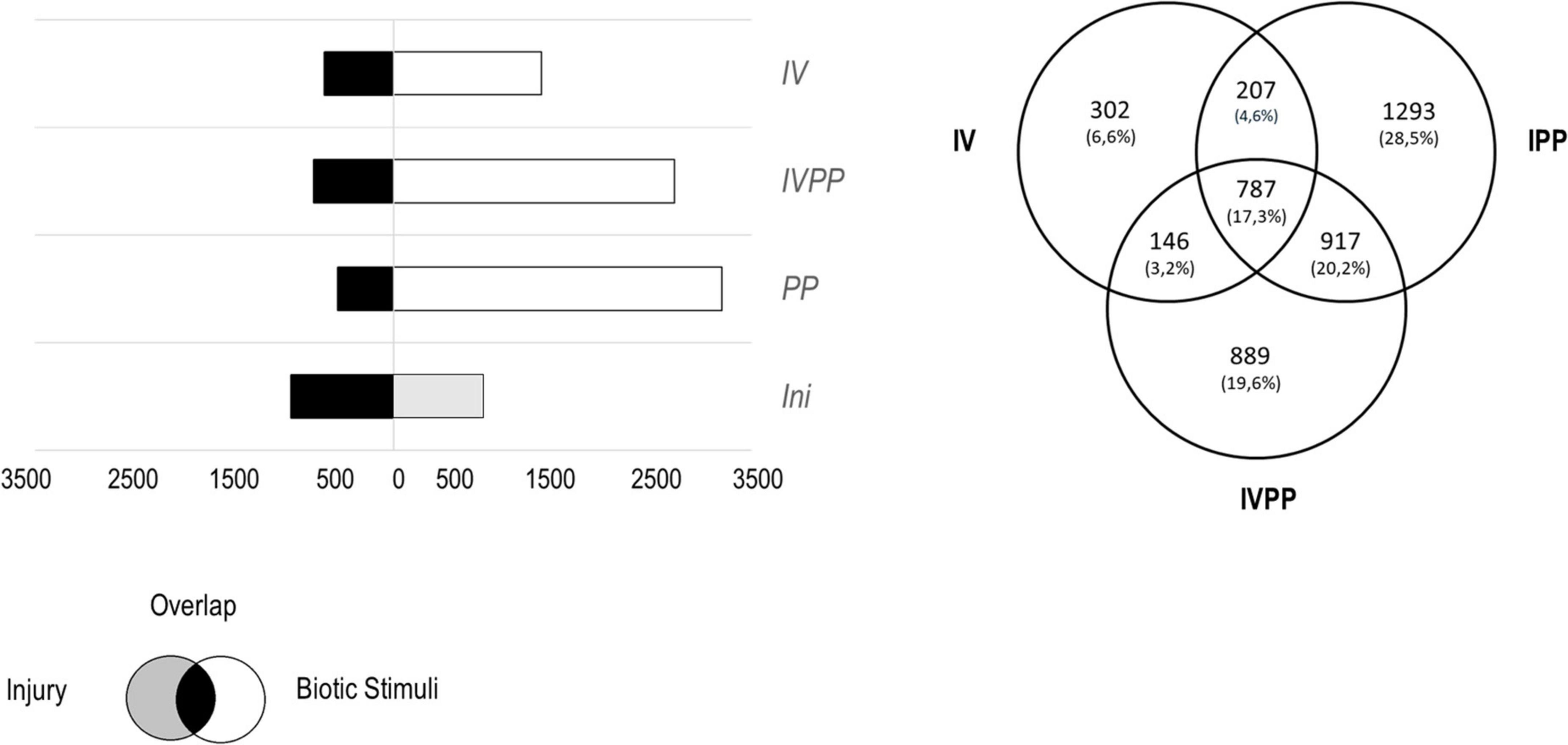
Figure 1. (A) Number of the total differentially expressed genes (DEGs, p-value < 0.05) by the injury, in the presence of Vintec® (IV), during the infection (IPP) or during the infection in plants pre-inoculated with Vintec® (IVPP). In black, DEGs induced by the injury and the biotic stimulus; in gray, DEGs induced by the injury alone; in white, DEGs induced by each of the three biological stimuli. (B) Venn diagram representing specific and common DEGs for all the three biotic stimuli.
The comparison of the three sets of DEGs revealed that only 787 genes were differentially expressed in all three conditions, which represents 17.3% of the total DEGs. The presence of Vintec® specifically induced the differential expression of 448 genes, from which 146 genes were also differentially expressed in the presence of the two pathogens. Infection with the two pathogens modified the expression of 2,210 genes, of which 917 genes overlapped with infection with the two pathogens in the presence of the BCA Vintec®. Interestingly, the combination of the two pathogens with Vintec® modifies the expression of 889 genes, which represents 19.6% of the total DEGs (Figure 1B). In other words, 889 genes were differentially expressed when the plants were inoculated with BCA Vintec® prior to pathogen infection.
Gene Clustering Leads to Identification of Three Groups of Co-regulated Genes
For further analysis, the DEGs were separated into three groups based on their expression pattern (Figure 2). Each group was composed of two clusters of co-regulated genes showing patterns of expression. Group I contained genes whose expression was modified by one of the three biotic stimuli, but more strongly by the two pathogens (Cluster 1 and 3). Group II contained genes whose expression was modified by the presence of the two pathogens in plants pre-inoculated with the BCA Vintec® (Clusters 4 and 5), and Group III contained genes whose expression was modified by the presence of the BCA Vintec® (Clusters 6 and 2).
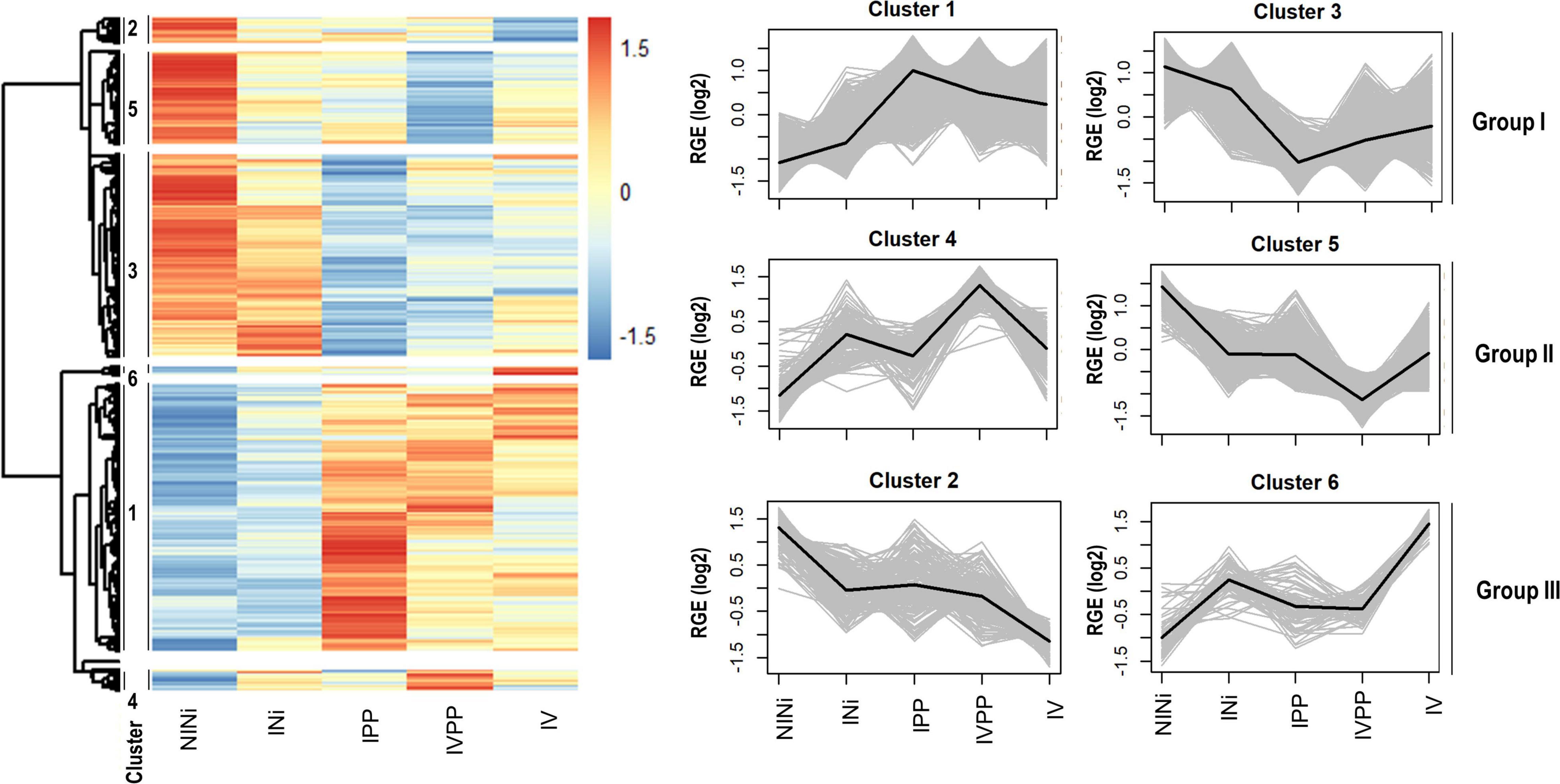
Figure 2. Cluster analysis of the total DEGs associated to the different biotic stimuli. The heatmap shows the different gene expression patterns. Each line represents one gene, and each column represents a biological condition. The color key indicates log2 relative gene expression (RGE) values. The numbers on the left of the heatmap indicated the different clusters. DEGs were arranged in six different clusters depending on their pattern profile. Dendrograms represent the hierarchical distance between genes and clusters. On the right, the expression pattern for each cluster is showed. Clusters were rearranged in three groups: Clusters 1 and 3 (Group I), responding to the three biotic stimuli but specially to the infection; clusters 2 and 6 (Group III), responding to the BCA Vintec®; and clusters 4 and 5 (Group II), responding to the infection and the BCA Vintec®.
Group I contains the majority of DEGs (76.5%). The expression pattern of the DEGs in this group is thus very heterogeneous. Group III regroups the smallest portion of total DEGs (5%). Based on their expression pattern, the induction or repression of these genes seem to specifically respond to the presence of Vintec®. The rest of the DEGs (18.5%) appears in clusters 4 and 5 (Group II). The expression of these genes is up- or downregulated by the presence of the two pathogens in plants pre-inoculated with the BCA Vintec®. The greater percentages of annotated DEGs in the three groups (23.6, 18.0, and 20.5%, respectively) were associated with “Primary Metabolism” functions, followed by “Signaling” (7.6%) and “Regulation” (7.4%) in Group I, “Transport elements” (10.6%) and “Regulation” (8.8%) in Group II, and “Transport elements” (12%) and “Cellular process” (8.1%) in Group III.
Functional Enrichment Gives Insights Into Different Perception of Vintec® and Pathogen Infection
Functional enrichment analysis (Fisher’s exact test, p-value < 0.05) was applied to the three groups (see results in Tables 2–4). The “Glutathione metabolism” (p-value = 3.291 × 10–10), “Phenylpropanoid biosynthesis” (p-value = 2.481 × 10–5), and “Tyrosine metabolism” (p-value = 2.53 × 10–4) categories are enriched for upregulated DEGs in Group I. Among upregulated functions, “ABA biosynthesis” and “ethylene-mediated signaling pathway” are also enriched (p-value = 1.49 × 10–3 and 3 × 10–3, respectively), whereas “Cytokinin inactivation” (p-value = 4.92 × 10–2) joins the downregulated categories in Group I. No significant enrichment for categories associated with “Hormone signaling” occurs among DEG functions of Group II. In contrast, in Group III, auxin-mediated signaling appears to be repressed because auxin-responsive elements (VIT_03s0038g01130, VIT_03s0038g00950, VIT_19s0085g00010) are strongly downregulated compared with the NINi control, and auxin inactivation-related genes are upregulated.
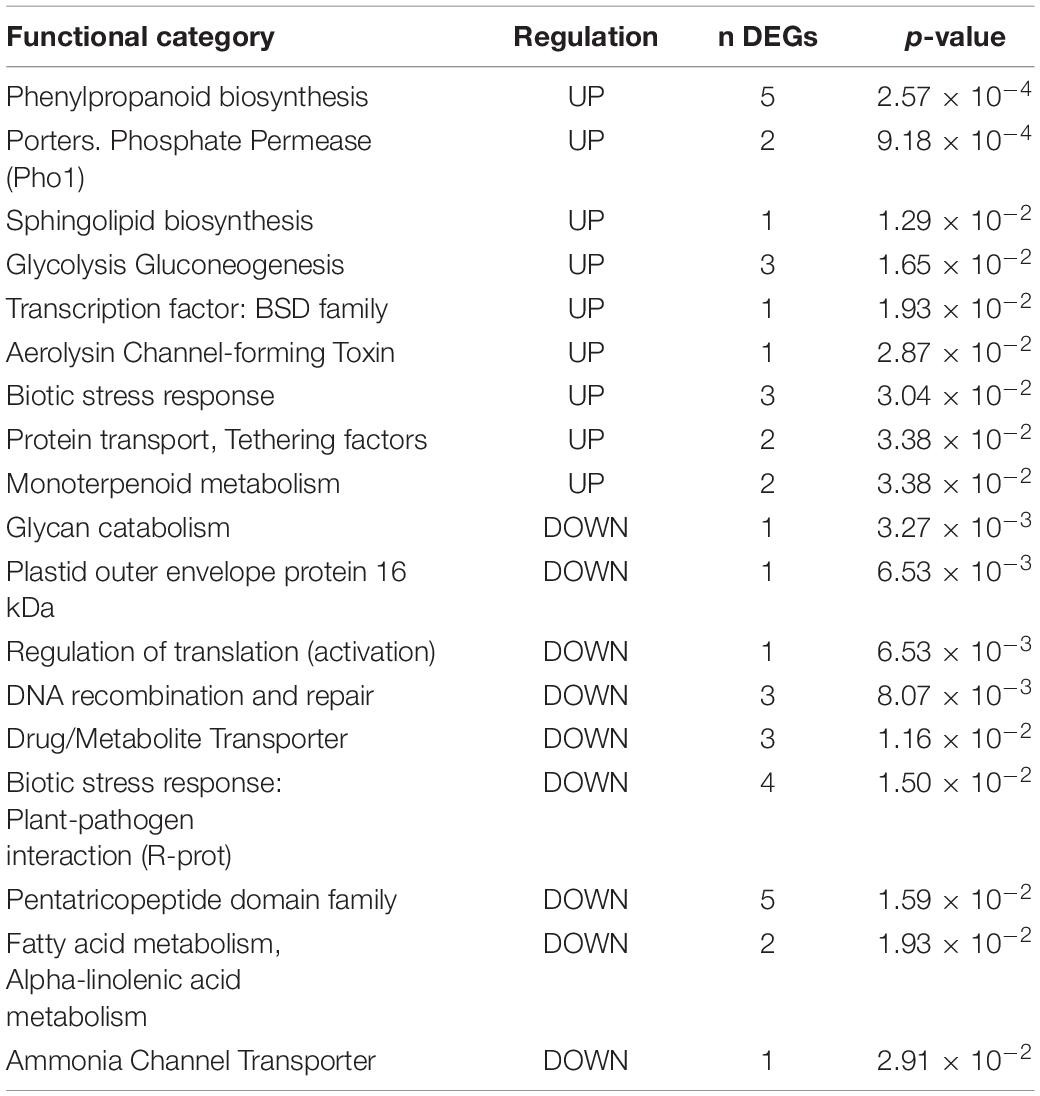
Table 3. Functional enrichment in Group II. DEGs up- and down-regulated by the two pathogens and Vintec® (IVPP).
Auxins, cytokinins, and gibberellins control plant vegetative development and growth by coordinating cell proliferation and differentiation (Vanstraelen and Benkov, 2012). No DEGs appear related to gibberellin signaling. However, in addition to auxins, differential regulation of genes is associated with cytokinin biosynthesis and signaling (Figure 3). The analysis of gene expression associated with “auxin-mediated signaling pathways” in the presence of Vintec® reveals an inverse gene expression pattern compared with the NINi control. Most auxin-responsive putative genes (ARFs) are repressed by the presence of Vintec®, and this repression is more significant under the IPPV condition. In addition, auxin inactivation elements seem to be over-expressed in IV and IVPP. Altogether, these results suggest that auxin signaling pathways are repressed by the presence of Vintec®. These statements are also valid for cytokinin-mediated signaling pathways. Cytokinin type-A responsive elements, which negatively regulate type-B responsive elements, are induced in both IV and IVPP conditions, as well as cytokinin catabolic pathways. Furthermore, the category “cell wall organization and biogenesis” is enriched among upregulated genes of Group III (p-value = 8.01 × 10–4) but downregulated in Group I (p-value = 6.12 × 10–5), which could be linked to these differences in hormonal signaling.
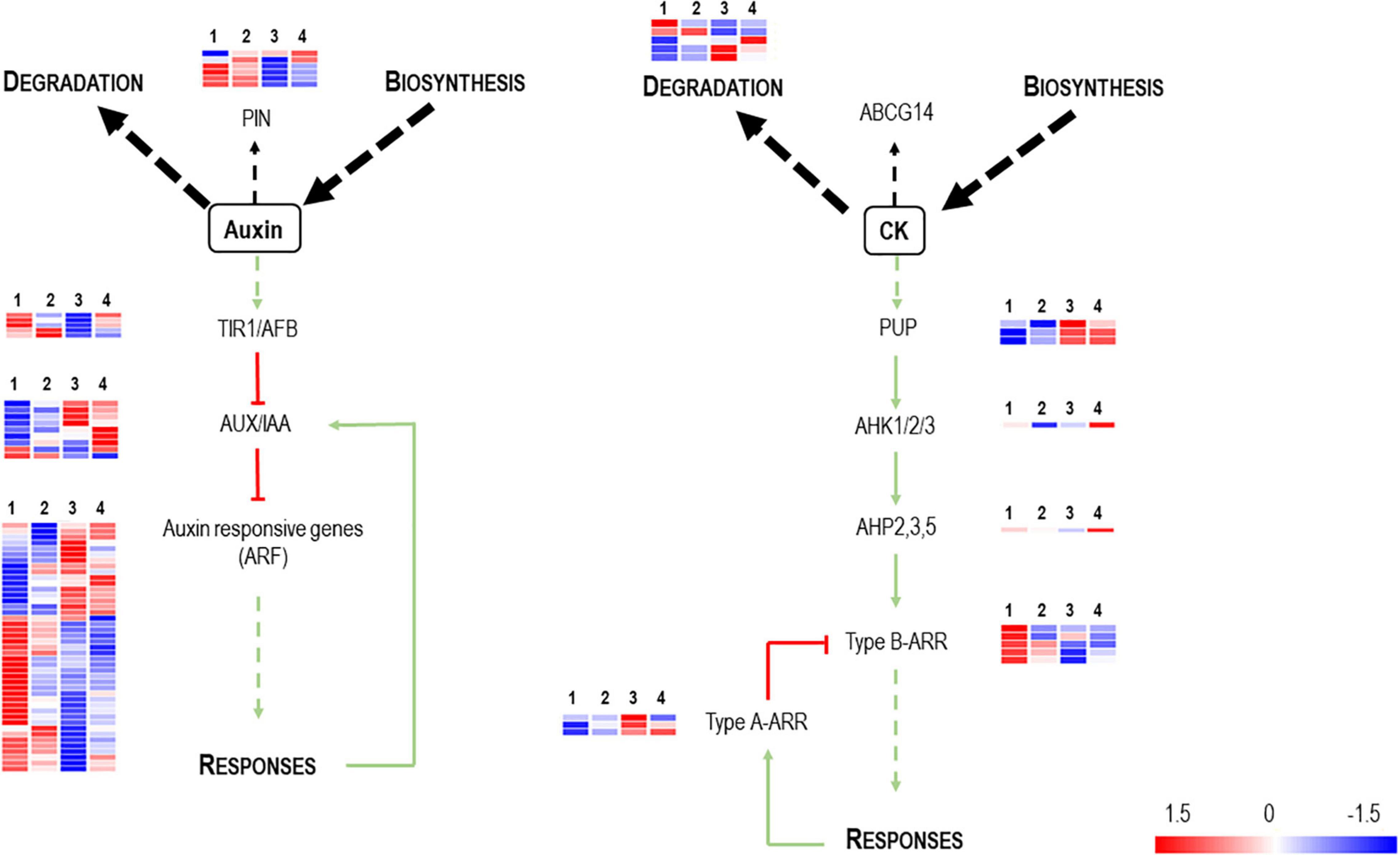
Figure 3. Schematic representation of auxin and cytokinin metabolism, transport and signaling pathways (adapted from Schaller et al., 2015). PIN and ABCG14 are efflux carriers which transport auxin and cytokinin, respectively, throughout the plant. Auxins binds TIR1 receptors with induces the degradation of the AUX/IAA complex, which are transcriptional repressors of the Auxin Response Factors (ARFs). This induces the relieve of the repression of the ARFs and thus the activation of the expression of auxin related genes. In the case of cytokinin signaling, three components are involved: cytokinin receptors, AHKs; their downstream targets, AHPs; which activates the ARRs. There exist two categories of ARR (types A and B). Type-B ARRs positively control the response to cytokinin and type-A ARRs negatively regulate cytokinin signaling. The heatmaps represent the expression profiles of the identified DEGs associated to these gene functions. The color key represents the log2 relative gene expression values, red for upregulated genes and blue for downregulated genes. Each line represents one gene, and each column represents one condition (1st column, NINi; 2nd column, INi; 3rd column, IVPP; 4th column, V).
BCA Vintec® Enhances Reprogramming of Secondary Metabolism Gene Expression During Pathogen Infection
Among the up-regulated DEGs in Group II, the category “Phenylpropanoid biosynthesis” is significantly enriched (p-value = 2.57 × 10–4), along with “Monoterpenoid metabolism” (p-value = 3.38 × 10–2), which is also related to “Secondary metabolism.” Interestingly, when studying the expression pattern of DEGs associated with “Flavonoid biosynthesis,” “Terpenoid biosynthesis,” or “Phenylpropanoid biosynthesis,” we observe specific signatures (Figure 4). Some genes are up-regulated in the presence of the two pathogens, but their expression remains unchanged in plants pre-inoculated with Vintec®. In contrast, we observe another set of genes responding specifically to the infection only in the presence of Vintec®.
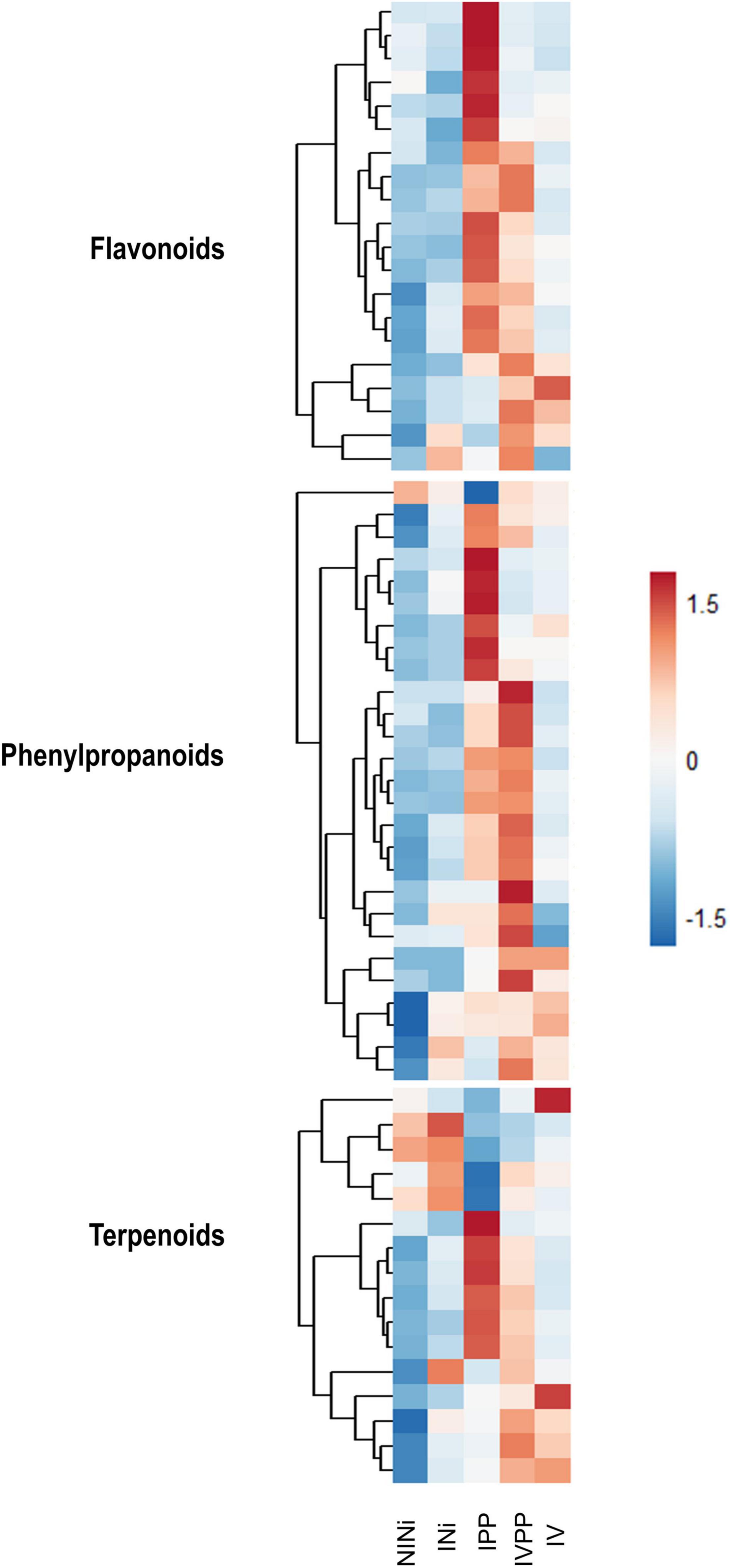
Figure 4. Heatmap representing the detected DEGs associated to flavonoids, phenylpropanoids and terpenoids biosynthesis. The color key represents the log2 relative gene expression values. Each line represents one gene, and each column represents one condition.
Secondary metabolites such as flavonoids, terpenoids, or stilbenes are known to play important roles in plant protection against biotic and abiotic stresses (Samanta et al., 2011). Interestingly, the upregulation of some of these genes is exclusive to the IVPP condition. The results show that genes VIT_10s0042g00920 and VIT_16s0100g00940 (ENTREZ ID 100266562 and 100853526, respectively) are strongly upregulated in plants inoculated with Vintec® before infection. These genes code for two putative stilbene synthases, which are enzymes associated with the biosynthesis of resveratrol and pterostilbene. In contrast, VIT_110037g00440 (ENTREZ ID 100250337), another gene associated with this same metabolic pathway, is significantly repressed during the infection in the presence of the BCA. The first two genes code for the stilbene synthase, which is implicated in the conversion of coumaroyl-CoA into trans-resveratrol, whereas the third gene codes for a shikimate O-hydroxycinnamoyltransferase, which catalyzes the interconversion of coumaroyl-CoA into caffeoyl-CoA (Figure 5).
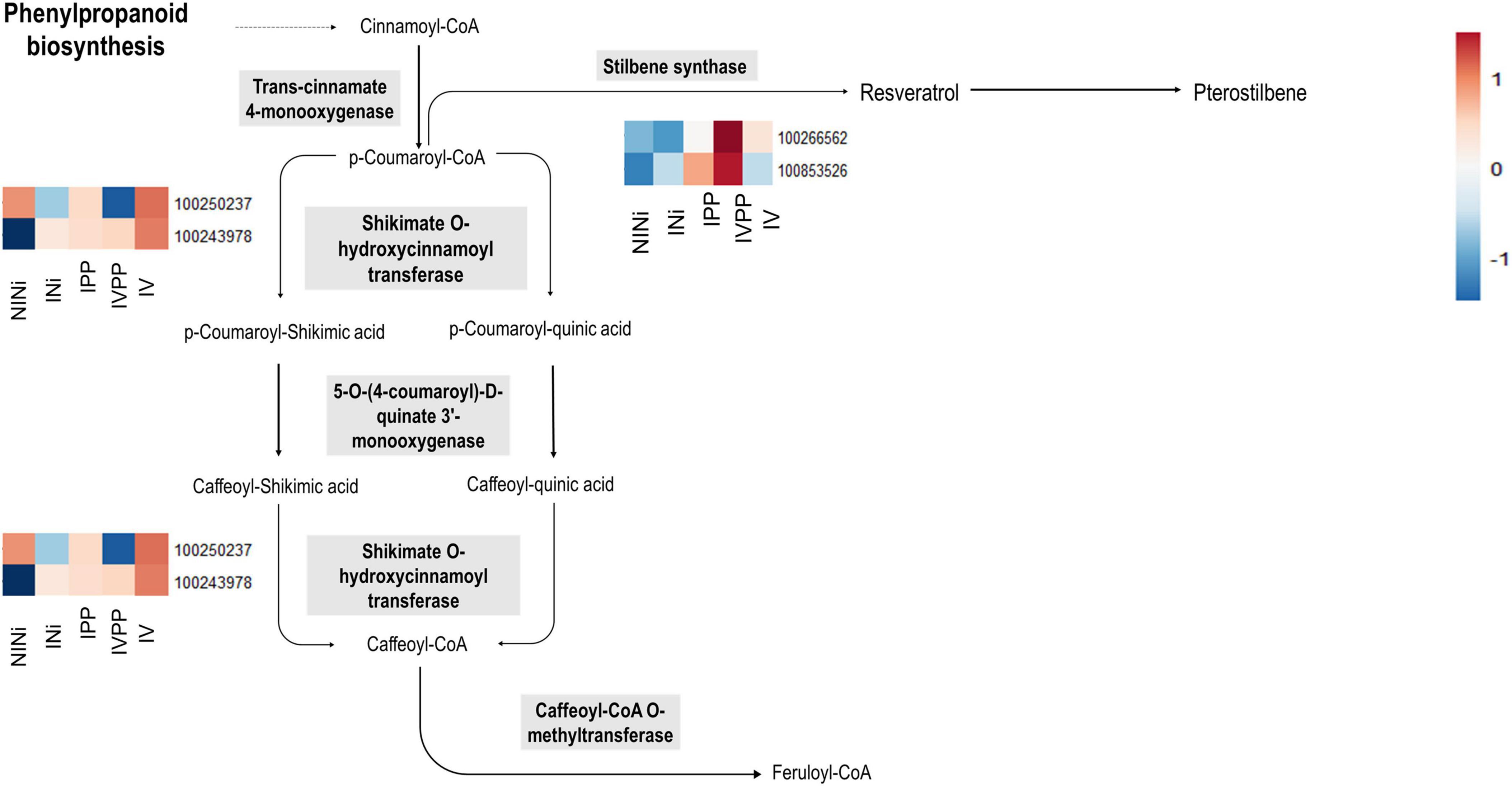
Figure 5. Schematic representation of stilbene biosynthesis (Adapted from KEGG Pathways). The heatmap represent the expression profile of the identified DEGs associated to the ENTREZ IDs of the enzymes implicated in this biosynthesis pathway. The color key represents the log2 relative gene expression values. Each line represents one gene, and each column represents one condition.
Discussion
Trichoderma spp. have been known since the 1920s for their ability to compete with other fungi via direct mechanisms such as competition for nutrients and space, mycoparasitism, or antibiosis (Harman, 2006). However, the ability of Trichoderma spp. to trigger modifications in plant response was not studied until the 1990s (De Meyer et al., 1998). Since then, the way in which Trichoderma spp. affect plant species in response to diverse abiotic and biotic stimuli has been widely studied (Tucci et al., 2011; De Palma et al., 2019; Wang et al., 2020; Yan et al., 2021). For instance, several studies report that Trichoderma spp. modulates growth promotion while enhancing salt and water stress tolerance via plant transcriptome reprogramming in Arabidopsis thaliana (Brotman et al., 2013), tomato (Mastouri et al., 2012; De Palma et al., 2019; Zhao et al., 2021), wheat (Zhang et al., 2016), and cacao (Bae et al., 2009). In another example involving tomatoes, the application of T. harzianum helps activate plant defense reactions against root-knot nematodes and stink bugs (Alınç et al., 2021) by enhancing secondary metabolite production and the activation of defense-related enzymes (Yan et al., 2021). Defense induction by Trichoderma spp. is also reported in cucumber (Yedidia et al., 2003; Shoresh et al., 2005), A. thaliana (Mathys et al., 2012), rice, and cotton (Djonović et al., 2006). In the present work, we study the transcriptome reprogramming in grapevine trunk triggered by the commercial biocontrol solution Vintec® (Trichoderma atroviride SC1) both alone and during infection with P. chlamydospora and P. minimum, the two main pathogens associated with esca disease.
Recent studies have shown that pathogen infection triggers the reprogramming of gene expression in grapevine trunk (Pierron et al., 2016; Massonnet et al., 2017; Gonçalves et al., 2019; Labois et al., 2020). Here, we confirm that the combined infection of P. chlamydospora and P. minimum leads to the modification of a large set of genes. The presence of the BCA Vintec® alone also modifies gene expression of grapevine trunk but to a lesser extent than pathogen infection, suggesting that grapevine trunk perceives and differentially responds to the BCA or the pathogens. Functional enrichment analysis also shows dissimilarities between the BCA Vintec® and the two pathogens.
Hormone signaling depends on the biotic stimuli. Plant hormones orchestrate plant development over different stages, are key actors in virtually every physiological plant process, and are vital for maintaining the balance between defense and growth when a plant is stressed (Pieterse et al., 2012; Naseem et al., 2015). Several studies show that the establishment of the plant-Trichoderma interaction and the ability to activate systemic resistance depends on different mechanisms employing jasmonic acid (JA) and ethylene (ET) signaling pathways (Shoresh et al., 2005, 2010; De Palma et al., 2019). The role of auxin signaling has been widely studied in connection with growth promotion induced by Trichoderma. Initially, Trichoderma was thought to modulate plant growth via the production of hormone-like compounds (Gravel et al., 2007; Contreras-Cornejo et al., 2009; Wang et al., 2020). Recent studies reveal that Trichoderma may activate the internal molecular mechanisms of plants, thereby altering hormonal signaling (Martínez-Medina et al., 2017; Patel et al., 2019; Wang et al., 2020). Wang et al. (2020) report that, in AUXR1, overexpressing poplar plantlets in which auxin is constitutively overproduced the level of indole-3-acetic acid decreases upon inoculation by T. asperellum.
The present results showed that the BCA Vintec® alone negatively regulates auxin signaling and differentially regulates cytokinin signaling in grapevine trunk. The cross-talk between auxin and cytokinin signaling reportedly plays a major role in coordinating cell enlargement and cell division in meristem tissues (Aloni et al., 2006; Immanen et al., 2016). These studies show that auxin and cytokinin signaling act in an antagonistic but partially overlapping way (Schaller et al., 2015). Studies done on poplar provide insight into the distribution profiles of these two phytohormones during the activity of the vascular cambium, which is the lateral meristem where wood formation starts. Immanen et al. (2016) show that cell division in poplar vascular cambium increases significantly in transgenic trees, overexpressing a cytokinin biosynthesis-related gene from Arabidopsis thaliana (AtIP7). They also report that the levels of auxin and auxin-related genes also increase, which suggests that cytokinin functions as a major regulator of cambial activity.
During infection, no hormone-signaling-related functional category is enriched among genes up- or downregulated in plants pre-inoculated with Vintec®. On the contrary, the categories “Ethylene-signaling pathway” and “ABA biosynthesis” are enriched among DEGs in the presence of the two pathogens. Ethylene intervenes in many physiological processes; for instance, its cross-talk with auxin signaling regulates diverse plant developmental processes (Zemlyanskaya et al., 2018) and its interaction with jasmonic acid signaling modulates plant defense responses (Pieterse et al., 2012). Abscisic acid (ABA) is associated with abiotic stress responses, mainly drought and water stresses (Xie et al., 2005; Chen et al., 2020), but can play a role in the biotic stress response, which, in most cases, is considered to be a negative regulation of plant response (Mauch-Mani and Mauch, 2005; Asselbergh et al., 2008; Ueno et al., 2015). Various studies demonstrate that ABA interacts negatively with SA and JA/ET signaling (Anderson et al., 2004; Mauch-Mani and Mauch, 2005; Cao et al., 2011; Ueno et al., 2015). Altogether, this evidence combined with the present results suggests that both the pathogens and the BCA Vintec® may interact with hormone signaling, altering hormone homeostasis in the trunk. Pathogens might use this mechanism to interfere with plant immunity, whereas the presence of Vintec® could counteract this strategy.
Conversely, numerous studies report that Trichoderma spp. stimulate plant natural defenses before or during pathogen infection (Shoresh et al., 2010; Shaw et al., 2016). Mathys et al. (2012) show that T. hamatum T382 activates Induced Systemic Resistance (ISR) in A. thaliana, resulting in accelerated activation of the defense response to Botrytis cinerea. They postulated that the stimulation of the phenylpropanoid pathway by T. hamatum plays an essential role in ISR. Yan et al. (2021) also report that another species of Trichoderma, T. harzianum, enhances the accumulation of secondary metabolites such as phenols and flavonoids and suppresses root-knot nematode infections in tomato. Pratap Singh et al. (2021) also report a higher total phenolic content in plants pre-inoculated with T. harzianum and T. asperellum after Sclerotinia sclerotium infection in brinjal. The present study also reveals an enrichment in the secondary metabolism biosynthesis pathways during the infection, mostly associated with phenylpropanoids, flavonoids, and phenols.
Several studies highlight the accumulation of this type of metabolite in diseased grapevine wood (Amalfitano et al., 2000; Lima et al., 2012; Rusjan et al., 2017). Remarkably, the pattern of expression of genes associated with the biosynthesis of phenylpropanoids, flavonoids, or phenols depends on the presence or the absence of the BCA Vintec® during the infection. Our results suggest that the presence of the BCA Vintec® enhances the accumulation of stilbenes, which have antifungal properties, in accordance with parallel metabolomic work on the same pathosystem (Chervin et al., 2022). Stilbenes are described as phytoalexins in grapevine, so they are implicated in plant defense (Langcake and Pryce, 1977). For instance, the protective role of a number of stilbenes against Plasmopara viticola, the causal agent of downy mildew in grapevine, is described by Pezet et al. (2015) and Gabaston et al. (2017). In addition, a number of studies investigate how elicitation with chitosan affects grapevine gray mold (Iriti et al., 2004) and powdery mildew (Ait Barka et al., 2004; Romanazzi et al., 2021). The mechanism underlying the protection of V. vinifera by chitosan elicitation is related to the enhanced production of stilbenes in grapes (Lucini et al., 2018). Recently, a study comparing tolerant V. vinifera subspecies (V. vinifera subs. Sylvestris) and susceptible V. vinifera subs. vinifera showed that, after infection by Neofusicoccum parvum, tolerant subspecies contained more resveratrol and its derivates than susceptible subspecies (Labois et al., 2020). Although the role of these compounds in esca disease has not yet been completely elucidated, all this evidence suggests that stilbenes play a key role in the protection of grapevine against trunk diseases.
Conclusion
This study shows that the application of the BCA Vintec®—a Trichoderma atroviride SC1 commercial solution—enhances modifications in the gene response to grapevine trunk diseases, both alone and during pathogen infection with two pathogens associated with esca disease. The BCA Vintec® seems to alter in different ways the hormone homeostasis both alone and during infection with P. minimum and P. chlamydospora. Only during the infection does the BCA Vintec® promote the expression of genes related to the biosynthesis of stilbenes, phenols, and flavonoids, which are metabolites known for their antifungal properties. Altogether, these results suggest that the BCA Vintec® interferes in the plant response to esca-associated pathogens by enhancing the primary defense response.
Data Availability Statement
The datasets presented in this study]can be found in online repositories. The name of the repository and accession number can be found below: NCBI; PRJNA817518.
Author Contributions
AR-O and AJ conceived the experimental plan. AJ coordinated the study. AR-O conducted the experiments (plant production, RNA and DNA extractions, qRT-PCR, and qPCR reactions), conducted RNA sequencing bioinformatics analysis, and wrote the manuscript. CB assisted with plant production and qRT-PCR reactions. AJ, TL, JC, and JD contributed to the data analysis and interpretation. All co-authors revised and contributed modifications to the final manuscript.
Funding
We are grateful to the French Public Bank of Investment (BPI France) for funding this work within the framework of the PSPC Project “SOLSTICE” (SOLutionS pour des Traitements Intégrés dans une Conduite Environnementale).
Conflict of Interest
The authors declare that the research was conducted in the absence of any commercial or financial relationships that could be construed as a potential conflict of interest.
Publisher’s Note
All claims expressed in this article are solely those of the authors and do not necessarily represent those of their affiliated organizations, or those of the publisher, the editors and the reviewers. Any product that may be evaluated in this article, or claim that may be made by its manufacturer, is not guaranteed or endorsed by the publisher.
Acknowledgments
We thank Belchim Crop Protection for providing the BCA Vintec® and especially Wilfried Remus-Borel, Thomas Besnier, and Gabriel D’Enjoy for their expertise and fruitful discussions related to this work. Finally, we thank the GeT-Plage platform for their work and the high-quality sequences that they generated.
Supplementary Material
The Supplementary Material for this article can be found online at: https://www.frontiersin.org/articles/10.3389/fmicb.2022.898356/full#supplementary-material
Footnotes
References
Ait Barka, E., Eullaffroy, P., Clément, C., and Vernet, G. (2004). Chitosan improves development, and protects Vitis vinifera L. against Botrytis cinerea. Plant Cell Rep. 22, 608–614. doi: 10.1007/s00299-003-0733-3
Alınç, T., Cusumano, A., Peri, E., Torta, L., and Colazza, S. (2021). Trichoderma harzianum Strain T22 modulates direct defense of tomato plants in response to nezara viridula feeding activity. J. Chem. Ecol. 47:455. doi: 10.1007/S10886-021-01260-3
Aloni, R., Aloni, E., Langhans, M., and Ullrich, C. I. (2006). Role of cytokinin and auxin in shaping root architecture: regulating vascular differentiation, lateral root initiation, root apical dominance and root gravitropism. Ann. Bot. 97, 883–893. doi: 10.1093/AOB/MCL027
Amalfitano, C., Evidente, A., Mugnai, L., Tegli, S., Bertelli, E., and Surico, G. (2000). Phenols and stilbene polyphenols in the wood of Esca-diseased grapevines. Phytopathol. Mediterr. 39, 178–183. doi: 10.1400/57841
Anderson, J. P., Badruzsaufari, E., Schenk, P. M., Manners, J. M., Desmond, O. J., Ehlert, C., et al. (2004). Antagonistic interaction between abscisic acid and jasmonate-ethylene signaling pathways modulates defense gene expression and disease resistance in arabidopsis. Plant Cell 16, 3460–3479. doi: 10.1105/tpc.104.025833
Andrews, S. (2010). Babraham bioinformatics – FastQC a quality control tool for high throughput sequence data. Soil 5, 47–81. doi: 10.1016/0038-0717(73)90093-X
Asselbergh, B., De Vleesschauwer, D., and Höfte, M. (2008). Global switches and fine-tuning—ABA modulates plant pathogen defense. Mol. Plant Microbe Interact. 21, 709–719. doi: 10.1094/MPMI-21-6-0709
Bae, H., Sicher, R. C., Kim, M. S., Kim, S. H., Strem, M. D., Melnick, R. L., et al. (2009). The beneficial endophyte Trichoderma hamatum isolate DIS 219b promotes growth and delays the onset of the drought response in Theobroma cacao. J. Exp. Bot. 60, 3279–3295. doi: 10.1093/JXB/ERP165
Benítez, T., Rincón, A. M., Limón, M. C., and Codón, A. C. (2004). Biocontrol mechanisms of Trichoderma strains. Int. Microbiol. 7, 249–260. doi: 10.2436/im.v7i4.9480
Berbegal, M., Ramón-Albalat, A., León, M., and Armengol, J. (2020). Evaluation of long-term protection from nursery to vineyard provided by Trichoderma atroviride SC1 against fungal grapevine trunk pathogens. Pest Manag. Sci. 76, 967–977. doi: 10.1002/ps.5605
Bertsch, C., Ramírez-Suero, M., Magnin-Robert, M., Larignon, P., Chong, J., Abou-Mansour, E., et al. (2013). Grapevine trunk diseases: complex and still poorly understood. Plant Pathol. 62, 243–265. doi: 10.1111/j.1365-3059.2012.02674.x
Bhat, A., Junaid, J. M., Dar, N. A., Bhat, T. A., Hussain Bhat, A., and Bhat, M. A. (2013). Commercial biocontrol agents and their mechanism of action in the management of plant pathogens. Int. J. Mod. Plant Anim. Sci. 1, 39–57.
Bigot, G., Sivilotti, P., Stecchina, M., Lujan, C., Freccero, A., and Mosetti, D. (2020). Long-term effects of Trichoderma asperellum and Trichoderma gamsii on the prevention of esca in different vineyards of Northeastern Italy. Crop. Prot. 137:105264. doi: 10.1016/j.cropro.2020.105264
Brodeur, J., Abram, P. K., Heimpel, G. E., and Messing, R. H. (2018). Trends in biological control: public interest, international networking and research direction. BioControl 63, 11–26. doi: 10.1007/s10526-017-9850-8
Brotman, Y., Landau, U., Cuadros-Inostroza, Á, Takayuki, T., Fernie, A. R., Chet, I., et al. (2013). Trichoderma-plant root colonization: escaping early plant defense responses and activation of the antioxidant machinery for saline stress tolerance. PLoS Pathog. 9:e1003221. doi: 10.1371/JOURNAL.PPAT.1003221
Canaguier, A., Grimplet, J., Di Gaspero, G., Scalabrin, S., Duchêne, E., Choisne, N., et al. (2017). A new version of the grapevine reference genome assembly (12X.v2) and of its annotation (VCost.v3). Genomics Data 14, 56–62. doi: 10.1016/j.gdata.2017.09.002
Cao, F. Y., Yoshioka, K., and Desveaux, D. (2011). The roles of ABA in plant-pathogen interactions. J. Plant Res. 124, 489–499. doi: 10.1007/s10265-011-0409-y
Chen, K., Li, G. J., Bressan, R. A., Song, C. P., Zhu, J. K., and Zhao, Y. (2020). Abscisic acid dynamics, signaling, and functions in plants. J. Integr. Plant Biol. 62, 25–54. doi: 10.1111/JIPB.12899
Chervin, J., Romeo-Oliván, A., Fournier, S., Puech-Pages, V., Dumas, B., Jacques, A., et al. (2022). Modification of early response of Vitis vinifera to pathogens relating to esca disease and biocontrol agent vintec® revealed by untargeted metabolomics on woody tissues. Front. Microbiol. 13:234. doi: 10.3389/fmicb.2022.835463
Contreras-Cornejo, H. A., Macías-Rodriíguez, L., Cortés-Penagos, C., and López-Bucio, J. (2009). Trichoderma virens, a plant beneficial fungus, enhances biomass production and promotes lateral root growth through an auxin-dependent mechanism in Arabidopsis. Plant Physiol. 149, 1579–1592. doi: 10.1104/PP.108.130369
Cook, R. J. (1988). Biological control and holistic plant-health care in agriculture. Am. J. Altern. Agric. 3, 51–62. doi: 10.1017/S0889189300002186
De Meyer, G., Bigirimana, J., Elad, Y., and Höfte, M. (1998). Induced systemic resistance in Trichoderma harzianum T39 biocontrol of Botrytis cinerea Geert. Eur. J. Plant Pathol. 104, 279–286.
De Palma, M., Salzano, M., Villano, C., Aversano, R., Lorito, M., Ruocco, M., et al. (2019). Transcriptome reprogramming, epigenetic modifications and alternative splicing orchestrate the tomato root response to the beneficial fungus Trichoderma harzianum. Hortic. Res. 61:5. doi: 10.1038/s41438-018-0079-1
Di Marco, S., Cesari, A., and Osti, F. (2004). Experiments on the control of Esca by “Thricoderma”. Phytopathol. Mediterr. 43, 1000–1008. doi: 10.1400/14580
Di Marco, S., and Osti, F. (2007). Applications of Trichoderma to prevent Phaeomoniella chlamydospora infections in organic nurseries. Phytopathol. Mediterr. 46, 73–83.
Djonović, S., Pozo, M. J., Dangott, L. J., Howell, C. R., and Kenerley, C. M. (2006). Sm1, a proteinaceous elicitor secreted by the biocontrol fungus Trichoderma virens induces plant defense responses and systemic resistance. Mol. Plant Microbe Interact. 19, 838–853. doi: 10.1094/MPMI-19-0838
Dobin, A., Davis, C. A., Schlesinger, F., Drenkow, J., Zaleski, C., Jha, S., et al. (2013). STAR: ultrafast universal RNA-seq aligner. Bioinformatics 29, 15–21. doi: 10.1093/BIOINFORMATICS/BTS635
Eilenberg, J., Hajek, A., and Lomer, C. (2001). Suggestions for unifying the terminology in biological control. BioControl 46, 387–400.
Ferreira, F. V., and Musumeci, M. A. (2021). Trichoderma as biological control agent: scope and prospects to improve efficacy. World J. Microbiol. Biotechnol. 37:90. doi: 10.1007/s11274-021-03058-7
Fontaine, F., Gramaje, D., Armengol, J., Smart, R., Nagy, Z. A., Borgo, M., et al. (2016). Grapevine Trunk Diseases. A review. Available online at: https://www.oiv.int/public/medias/4650/trunk-diseases-oiv-2016 (accessed 1 April, 2019).
Fourie, P. H., Halleen, F., Van der Vyver, J., and Schreuder, W. (2001). Effect of Trichoderma treatments on the occurrence of decline pathogens in the roots and rootstocks of nursery grapevines. Phytopathol. Mediterr. 40, 473–478. doi: 10.14601/PHYTOPATHOL_MEDITERR-1619
Gabaston, J., Cantos-Villar, E., Biais, B., Waffo-Teguo, P., Renouf, E., Corio-Costet, M. F., et al. (2017). Stilbenes from Vitis vinifera L. Waste: a sustainable tool for controlling Plasmopara viticola. J. Agric. Food Chem. 65, 2711–2718. doi: 10.1021/acs.jafc.7b00241
Gonçalves, M. F. M., Nunes, R. B., Tilleman, L., Van De Peer, Y., Deforce, D., Van Nieuwerburgh, F., et al. (2019). Dual RNA sequencing of Vitis vinifera during Lasiodiplodia theobromae infection Unveils host–pathogen interactions. Int. J. Mol. Sci. 20:6083. doi: 10.3390/IJMS20236083
Gramaje, D., Mostert, L., Groenewald, J. Z., and Crous, P. W. (2015). Phaeoacremonium: from esca disease to phaeohyphomycosis. Fungal Biol. 119, 759–783. doi: 10.1016/j.funbio.2015.06.004
Graniti, A., Surico, G., and Mugnai, L. (2000). Esca of grapevine: a disease complex or a complex of diseases? Phytopathol. Mediterr. 39, 16–20. doi: 10.14601/Phytopathol_Mediterr-1539
Gravel, V., Antoun, H., and Tweddell, R. J. (2007). Growth stimulation and fruit yield improvement of greenhouse tomato plants by inoculation with Pseudomonas putida or Trichoderma atroviride: possible role of indole acetic acid (IAA). Soil Biol. Biochem. 39, 1968–1977. doi: 10.1016/J.SOILBIO.2007.02.015
Grimplet, J., Cramer, G. R., Dickerson, J. A., Mathiason, K., van Hemert, J., and Fennell, A. Y. (2009). Vitisnet: “Omics” integration through grapevine molecular networks. PLoS One 4:e8365. doi: 10.1371/journal.pone.0008365
Harman, G. E. (2006). Overview of mechanisms and uses of Trichoderma spp. Phytopathology 96, 190–194. doi: 10.1094/PHYTO-96-0190
Immanen, J., Nieminen, K., Smolander, O. P., Kojima, M., Alonso Serra, J., Koskinen, P., et al. (2016). Cytokinin and auxin display distinct but interconnected distribution and signaling profiles to stimulate cambial activity. Curr. Biol. 26, 1990–1997. doi: 10.1016/j.cub.2016.05.053
Iriti, M., Rossoni, M., Borgo, M., and Faoro, F. (2004). Benzothiadiazole enhances resveratrol and anthocyanin biosynthesis in grapevine, meanwhile improving resistance to Botrytis cinerea. J. Agric. Food Chem. 52, 4406–4413. doi: 10.1021/JF049487B
Joshi, N. A., and Fass, J. N. (2011). Sickle: A Sliding-Window, Adaptive, Quality-based Trimming Tool for FastQ Files (Version 1.33) [Software]. Available online at https://github.com/najoshi/sickle
Kotze, C., Van Niekerk, J., Mostert, L., Halleen, F., and Fourie, P. (2011). Evaluation of Biocontrol Agents for Grapevine Pruning Wound Protection against Trunk Pathogen Infection. Firenze: Firenze University Press Mediterranean Phytopathological Union. doi: 10.2307/26458725
Kubicek, C. P., Mach, R. L., Peterbauer, C. K., and Lorito, M. (2001). Trichoderma: from genes to biocontrol. J. Plant Pathol. 83, 11–23.
Labois, C., Wilhelm, K., Laloue, H., Tarnus, C., Bertsch, C., Goddard, M. L., et al. (2020). Wood metabolomic responses of wild and cultivated grapevine to infection with Neofusicoccum parvum, a trunk disease pathogen. Metabolites 10:232. doi: 10.3390/METABO10060232
Langcake, P., and Pryce, R. J. (1977). A new class of phytoalexins from grapevines. Experientia 33, 151–152. doi: 10.1007/BF02124034
Larignon, P., Dupont, J., and Dubos, B. (2000). Esca disease. The biological background of two agents of the disease, Phaeoacremonium aleophilum and Phaeomoniella chlamydospora. Phytoma 527, 30–35.
Liao, Y., Smyth, G. K., and Shi, W. (2014). featureCounts: an efficient general purpose program for assigning sequence reads to genomic features. Bioinformatics 30, 923–930.
Lima, M. R. M., Ferreres, F., and Dias, A. C. P. (2012). Response of Vitis vinifera cell cultures to Phaeomoniella chlamydospora: changes in phenolic production, oxidative state and expression of defence-related genes. Eur. J. Plant Pathol. 132, 133–146. doi: 10.1007/s10658-011-9857-4
Lucini, L., Baccolo, G., Rouphael, Y., Colla, G., Bavaresco, L., and Trevisan, M. (2018). Chitosan treatment elicited defence mechanisms, pentacyclic triterpenoids and stilbene accumulation in grape (Vitis vinifera L.) bunches. Phytochemistry 156, 1–8. doi: 10.1016/j.phytochem.2018.08.011
Martin, M. (2011). Cutadapt removes adapter sequences from high-throughput sequencing reads. EMBnet. journal 17, 10–12. doi: 10.14806/EJ.17.1.200
Martínez-Medina, A., Fernandez, I., Lok, G., Pozo, M., Pieterse, C., and Van Wees, S. (2017). Shifting from priming of salicylic acid- to jasmonic acid-regulated defences by Trichoderma protects tomato against the root knot nematode Meloidogyne incognita. New Phytol. 213, 1363–1377. doi: 10.1111/NPH.14251
Massonnet, M., Figueroa-Balderas, R., Galarneau, E. R. A. A., Miki, S., Lawrence, D. P., Sun, Q., et al. (2017). Neofusicoccum parvum colonization of the grapevine woody stem triggers asynchronous host responses at the site of infection and in the leaves. Front. Plant Sci. 8:1117. doi: 10.3389/fpls.2017.01117
Mastouri, F., Björkman, T., and Harman, G. (2012). Trichoderma harzianum enhances antioxidant defense of tomato seedlings and resistance to water deficit. Mol. Plant. Microbe Interact. 25, 1264–1271. doi: 10.1094/MPMI-09-11-0240
Mathys, J., De Cremer, K., Timmermans, P., Van Kerckhove, S., Lievens, B., Vanhaecke, M., et al. (2012). Genome-wide characterization of ISR induced in Arabidopsis thaliana by Trichoderma hamatum T382 against Botrytis cinerea infection. Front. Plant Sci. 3:108. doi: 10.3389/fpls.2012.00108
Mauch-Mani, B., and Mauch, F. (2005). The role of abscisic acid in plant–pathogen interactions. Curr. Opin. Plant Biol. 8, 409–414. doi: 10.1016/J.PBI.2005.05.015
Mondello, V., Larignon, P., Armengol, J., Kortekamp, A., Vaczy, K., Prezman, F., et al. (2018a). Management of grapevine trunk diseases: knowledge transfer, current strategies and innovative strategies adopted in Europe. Phytopathol. Mediterr. 57, 369–383. doi: 10.14601/Phytopathol_Mediterr-23942
Mondello, V., Songy, A., Battiston, E., Pinto, C., Coppin, C., Trotel-Aziz, P., et al. (2018b). Grapevine trunk diseases: a review of fifteen years of trials for their control with chemicals and biocontrol agents. Plant Dis. 102, 1189–1217. doi: 10.1094/PDIS-08-17-1181-FE
Mugnai, L., Graniti, A., and Surico, G. (1999). Esca (Black measles) and brown wood-streaking: two old and elusive diseases of grapevines. Plant Dis. 83, 404–418. doi: 10.1094/PDIS.1999.83.5.404
Naseem, M., Kaltdorf, M., and Dandekar, T. (2015). The nexus between growth and defence signalling: auxin and cytokinin modulate plant immune response pathways. J. Exp. Bot. 66, 4885–4896. doi: 10.1093/JXB/ERV297
Patel, J., Teli, B., Bajpai, R., Meher, J., Rashid, M., Mukherjee, A., et al. (2019). Trichoderma-Mediated Biocontrol and Growth Promotion in Plants: An Endophytic Approach. Amsterdam: Elsevier Inc., doi: 10.1016/b978-0-12-817004-5.00013-0
Pertot, I., Prodorutti, D., Colombini, A., and Pasini, L. (2016). Trichoderma atroviride SC1 prevents Phaeomoniella chlamydospora and Phaeoacremonium aleophilum infection of grapevine plants during the grafting process in nurseries. BioControl 61, 257–267. doi: 10.1007/s10526-016-9723-6
Pezet, R., Gindro, K., Viret, O., and Richter, H. (2015). Effects of resveratrol, viniferins and pterostilbene on Plasmopara viticola zoospore mobility and disease development. Vitis J. Grapevine Res. 43, 145–145. doi: 10.5073/VITIS.2004.43.145-148
Pierron, R. J. G., Pouzoulet, J., Couderc, C., Judic, E., Compant, S., and Jacques, A. (2016). Variations in early response of grapevine wood depending on wound and inoculation combinations with Phaeoacremonium aleophilum and Phaeomoniella chlamydospora. Front. Plant Sci. 7:268. doi: 10.3389/fpls.2016.00268
Pieterse, C. M. J., Van Der Does, D., Zamioudis, C., Leon-Reyes, A., and Van Wees, S. C. M. (2012). Hormonal modulation of plant immunity. Annu. Rev. Cell Dev. Biol. 28, 489–521. doi: 10.1146/ANNUREV-CELLBIO-092910-154055
Pouzoulet, J., Mailhac, N., Couderc, C., Besson, X., Daydé, J., Lummerzheim, M., et al. (2013). A method to detect and quantify Phaeomoniella chlamydospora and Phaeoacremonium aleophilum DNA in grapevine-wood samples. Appl. Microbiol. Biotechnol. 97, 10163–10175. doi: 10.1007/s00253-013-5299-6
Pratap Singh, S. S., Keswani, C., Pratap Singh, S. S., Sansinenea, E., Xuan Hoat, T., Singh, S. P., et al. (2021). Trichoderma spp. mediated induction of systemic defense response in brinjal against Sclerotinia sclerotiorum. Curr. Res. Microb. Sci. 2:100051. doi: 10.1016/J.CRMICR.2021.100051
Ram, R. M., Keswani, C., Bisen, K., Tripathi, R., Singh, S. P., and Singh, H. B. (2018). “Biocontrol technology: eco-friendly approaches for sustainable agriculture,” in Omics Technologies and Bio-Engineering: Towards Improving Quality of Life, Vol. Volume 2, eds D. Barh and V. Azevedo (Amsterdam: Elsevier), 177–190. doi: 10.1016/B978-0-12-815870-8.00010-3
Romanazzi, G., Mancini, V., Foglia, R., Marcolini, D., Kavari, M., and Piancatelli, S. (2021). Use of chitosan and other natural compounds alone or in different strategies with copper hydroxide for control of grapevine downy mildew. Plant Dis. 105, 3261–3268. doi: 10.1094/PDIS-06-20-1268-RE
Rusjan, D., Persic, M., Likar, M., Biniari, K., and Mikulic-Petkovsek, M. (2017). Phenolic responses to Esca-associated fungi in differently decayed grapevine woods from different trunk parts of “Cabernet Sauvignon”. J. Agric. Food Chem. 65, 6615–6624. doi: 10.1021/acs.jafc.7b02188
Savary, S., Willocquet, L., Pethybridge, S. J., Esker, P., McRoberts, N., and Nelson, A. (2019). The global burden of pathogens and pests on major food crops. Nat. Ecol. Evol. 33, 430–439. doi: 10.1038/s41559-018-0793-y
Schaller, G. E., Bishopp, A., and Kieber, J. J. (2015). The Yin-Yang of hormones: cytokinin and auxin interactions in plant development. Plant Cell 27, 44–63. doi: 10.1105/TPC.114.133595
Shaw, S., Le Cocq, K., Paszkiewicz, K., Moore, K., Winsbury, R., de Torres Zabala, M., et al. (2016). Transcriptional reprogramming underpins enhanced plant growth promotion by the biocontrol fungus Trichoderma hamatum GD12 during antagonistic interactions with Sclerotinia sclerotiorum in soil. Mol. Plant Pathol. 17, 1425–1441. doi: 10.1111/MPP.12429
Shoresh, M., Harman, G. E., and Mastouri, F. (2010). Induced systemic resistance and plant responses to fungal biocontrol agents. Annu. Rev. Phytopathol. 48, 21–43. doi: 10.1146/ANNUREV-PHYTO-073009-114450
Shoresh, M., Yedidia, I., and Chet, I. (2005). Involvement of jasmonic acid/ethylene signaling pathway in the systemic resistance induced in cucumber by Trichoderma asperellum T203. Phytopathology 95, 76–84. doi: 10.1094/PHYTO-95-0076
Thambugala, K. M., Daranagama, D. A., Phillips, A. J. L. L., Kannangara, S. D., and Promputtha, I. (2020). Fungi vs. fungi in biocontrol: an overview of fungal antagonists applied against fungal plant pathogens. Front. Cell. Infect. Microbiol. 10:604923. doi: 10.3389/fcimb.2020.604923
Tucci, M., Ruocco, M., De Masi, L., De Palma, M., and Lorito, M. (2011). The beneficial effect of Trichoderma spp. on tomato is modulated by the plant genotype. Mol. Plant Pathol. 12, 341–354. doi: 10.1111/J.1364-3703.2010.00674.X
Ueno, Y., Yoshida, R., Kishi-Kaboshi, M., Matsushita, A., Jiang, C. J., Goto, S., et al. (2015). Abiotic stresses antagonize the rice defence pathway through the tyrosine-dephosphorylation of OsMPK6. PLoS Pathog. 11:e1005231. doi: 10.1371/JOURNAL.PPAT.1005231
Vanstraelen, M., and Benkov, E. (2012). Hormonal interactions in the regulation of plant development. Annu. Rev. Cell Dev. Biol. 28, 463–487. doi: 10.1146/ANNUREV-CELLBIO-101011-155741
Vinale, F., Sivasithamparam, K., Ghisalberti, E. L., Marra, R., Woo, S. L., and Lorito, M. (2008). Trichoderma-plant-pathogen interactions. Soil Biol. Biochem. 40, 1–10. doi: 10.1016/j.soilbio.2007.07.002
Wang, Y., Hou, X., Deng, J., Yao, Z., Lyu, M., and Zhang, R. (2020). AUXIN RESPONSE FACTOR 1 acts as a positive regulator in the response of poplar to Trichoderma asperellum inoculation in overexpressing plants. Plants (Basel) 9:272. doi: 10.3390/PLANTS9020272
Xie, Z., Ruas, P., and Shen, Q. J. (2005). Regulatory networks of the phytohormone abscisic acid. Vitam. Horm. 72, 235–269. doi: 10.1016/S0083-6729(05)72007-0
Yan, Y., Mao, Q., Wang, Y., Zhao, J., Fu, Y., Yang, Z., et al. (2021). Trichoderma harzianum induces resistance to root-knot nematodes by increasing secondary metabolite synthesis and defense-related enzyme activity in Solanum lycopersicum L. Biol. Control 158:104609. doi: 10.1016/J.BIOCONTROL.2021.104609
Ye, J., Coulouris, G., Zaretskaya, I., Cutcutache, I., Rozen, S., and Madden, T. L. (2012). Primer-BLAST: a tool to design target-specific primers for polymerase chain reaction. BMC Bioinformatics 13:134. doi: 10.1186/1471-2105-13-134
Yedidia, I., Shoresh, M., Kerem, Z., Benhamou, N., Kapulnik, Y., and Chet, I. (2003). Concomitant Induction of systemic resistance to Pseudomonas syringae pv. lachrymans in cucumber by Trichoderma asperellum (T-203) and accumulation of phytoalexins. Appl. Environ. Microbiol. 69, 7343–7353. doi: 10.1128/AEM.69.12.7343-7353.2003
Zemlyanskaya, E. V., Omelyanchuk, N. A., Ubogoeva, E. V., and Mironova, V. V. (2018). Deciphering auxin-ethylene crosstalk at a systems level. Int. J. Mol. Sci. 19:4060. doi: 10.3390/IJMS19124060
Zhang, S., Gan, Y., and Xu, B. (2016). Application of plant-growth-promoting fungi Trichoderma longibrachiatum T6 enhances tolerance of wheat to salt stress through improvement of antioxidative defense system and gene expression. Front. Plant Sci. 7:1405. doi: 10.3389/FPLS.2016.01405
Zhao, J., Liu, T., Liu, W. C., Zhang, D. P., Dong, D., Wu, H. L., et al. (2021). Transcriptomic insights into growth promotion effect of Trichoderma afroharzianum TM2-4 microbial agent on tomato plants. J. Integr. Agric. 20, 1266–1276. doi: 10.1016/S2095-3119(20)63415-3
Keywords: biocontrol, Trichoderma atroviride SC1, Phaeomoniella chlamydospora, Phaeoacremonium minimum, esca, grapevine trunk diseases
Citation: Romeo-Oliván A, Chervin J, Breton C, Lagravère T, Daydé J, Dumas B and Jacques A (2022) Comparative Transcriptomics Suggests Early Modifications by Vintec® in Grapevine Trunk of Hormonal Signaling and Secondary Metabolism Biosynthesis in Response to Phaeomoniella chlamydospora and Phaeoacremonium minimum. Front. Microbiol. 13:898356. doi: 10.3389/fmicb.2022.898356
Received: 17 March 2022; Accepted: 08 April 2022;
Published: 17 May 2022.
Edited by:
Rajarshi Kumar Gaur, Deen Dayal Upadhyay Gorakhpur University, IndiaReviewed by:
Vivek Sharma, Chandigarh University, IndiaArup Kumar Mukherjee, National Rice Research Institute (ICAR), India
Copyright © 2022 Romeo-Oliván, Chervin, Breton, Lagravère, Daydé, Dumas and Jacques. This is an open-access article distributed under the terms of the Creative Commons Attribution License (CC BY). The use, distribution or reproduction in other forums is permitted, provided the original author(s) and the copyright owner(s) are credited and that the original publication in this journal is cited, in accordance with accepted academic practice. No use, distribution or reproduction is permitted which does not comply with these terms.
*Correspondence: Alban Jacques, YWxiYW4uamFjcXVlc0BwdXJwYW4uZnI=