- 1Department of Earth, Atmospheric, and Planetary Sciences, Massachusetts Institute of Technology, Cambridge, MA, United States
- 2Life Sciences Department, Natural History Museum, London, United Kingdom
- 3School of Earth and Environmental Sciences, Cardiff University, Cardiff, United Kingdom
- 4Institute of Geosciences, Christian-Albrechts-University of Kiel, Kiel, Germany
- 5Marine Geochemistry, Alfred Wegener Institute, Helmholtz Centre for Polar and Marine Research, Bremerhaven, Germany
- 6Coastal Marine Field Station, University of Waikato, Tauranga, New Zealand
Persistent cold temperatures, a paucity of nutrients, freeze-thaw cycles, and the strongly seasonal light regime make Antarctica one of Earth’s least hospitable surface environments for complex life. Cyanobacteria, however, are well-adapted to such conditions and are often the dominant primary producers in Antarctic inland water environments. In particular, the network of meltwater ponds on the ‘dirty ice’ of the McMurdo Ice Shelf is an ecosystem with extensive cyanobacteria-dominated microbial mat accumulations. This study investigated intact polar lipids (IPLs), heterocyte glycolipids (HGs), and bacteriohopanepolyols (BHPs) in combination with 16S and 18S rRNA gene diversity in microbial mats of twelve ponds in this unique polar ecosystem. To constrain the effects of nutrient availability, temperature and freeze-thaw cycles on the lipid membrane composition, lipids were compared to stromatolite-forming cyanobacterial mats from ice-covered lakes in the McMurdo Dry Valleys as well as from (sub)tropical regions and hot springs. The 16S rRNA gene compositions of the McMurdo Ice Shelf mats confirm the dominance of Cyanobacteria and Proteobacteria while the 18S rRNA gene composition indicates the presence of Ochrophyta, Chlorophyta, Ciliophora, and other microfauna. IPL analyses revealed a predominantly bacterial community in the meltwater ponds, with archaeal lipids being barely detectable. IPLs are dominated by glycolipids and phospholipids, followed by aminolipids. The high abundance of sugar-bound lipids accords with a predominance of cyanobacterial primary producers. The phosphate-limited samples from the (sub)tropical, hot spring, and Lake Vanda sites revealed a higher abundance of aminolipids compared to those of the nitrogen-limited meltwater ponds, affirming the direct affects that N and P availability have on IPL compositions. The high abundance of polyunsaturated IPLs in the Antarctic microbial mats suggests that these lipids provide an important mechanism to maintain membrane fluidity in cold environments. High abundances of HG keto-ols and HG keto-diols, produced by heterocytous cyanobacteria, further support these findings and reveal a unique distribution compared to those from warmer climates.
Introduction
Antarctica is replete with environments where complex life is excluded. Sub-zero temperatures, the scarcity of liquid water, nutrient limitation (Sorrell et al., 2013), and strong seasonality with prolonged periods of darkness followed by exposure to high UV radiation present challenges for most organisms (Vincent and Quesada, 1994). While macroscopic eukaryotic life struggles with such extreme conditions, bacteria have been shown to withstand low temperatures and to be well adapted to variable light, nutrient, and osmotic conditions (Jungblut and Neilan, 2010). Cyanobacteria, especially, can proliferate to form dense populations in many aquatic Antarctic environments (Vincent and Quesada, 1994). Notably, the ‘dirty ice’ located on the McMurdo Ice Shelf, located between the Ross Sea and Transantarctic Mountains, supports one of the largest networks of meltwater ponds in Antarctica and these are rich in biomass dominated by mm-cm thick benthic cyanobacteria-based microbial communities, as initially described by Scott in 1905 during his first Antarctic expedition aboard the RRS Discovery (Scott, 1907).
The ‘dirty ice’ region of the McMurdo Ice Shelf is an ablation zone located southeast of Bratina Island and northwest of Southern Victoria Land and comprises an area in excess of 1,500 km2 (Howard-Williams et al., 1990). The ice is covered with pockets of dark sediment and other debris derived from the seafloor, which is conveyed to the surface as ice ablates, driven by relatively warm and dry katabatic winds originating from the polar plateau (Debenham, 1919; Glasser et al., 2006). During summer, this area hosts a network of shallow meltwater ponds, varying in size and shape and ranging from 10s to several 10,000s m2 (Howard-Williams et al., 1990). Although the water in the ponds share similar sources from combinations of precipitation and melting sea ice, they are characterized by contrasting physicochemical conditions, such as variable salt concentrations, pH-values, and water temperatures (Howard-Williams et al., 1990).
Metagenomic and small subunit rRNA gene studies have confirmed microscopy surveys identifying diverse and highly productive cyanobacterial communities in the ‘dirty ice’ of the McMurdo Ice Shelf (Varin et al., 2012; Jackson et al., 2021). Major taxa comprise the heterocyte forming genera Anabaena, Nostoc, and Nodularia and filamentous non-heterocyte forming genera including Oscillatoria, Phormidium, Pseudanabaena, and Leptolyngbya (Jungblut et al., 2005; Varin et al., 2012; Jackson et al., 2021). Accumulation of photosynthetic biomass is reflected by high chlorophyll concentrations of up to 400 mg m–2 (Howard-Williams et al., 1990), being in the same range as active and organic-rich intertidal zones of the Wadden Sea (North Sea) (Stal et al., 1985). Other bacterial representatives detected by 16S rRNA gene clone libraries or successfully cultivated from Antarctic microbial mats and sediments include Firmicutes, Actinobacteria, Bacteroidetes, and Proteobacteria (e.g., Sjöling and Cowan, 2003; Varin et al., 2012; Archer et al., 2015). Besides bacterial assemblages, various eukaryotes, such as algae, fungi, and microscopic metazoa, have been reported from Antarctic meltwater ponds (Khan et al., 2011; Jungblut et al., 2012). Due to the high biomass and diverse microbial communities, the McMurdo meltwater ponds are often recognized as microbial oases in an environment that is otherwise hostile to complex life.
These oases are of particular paleobiological interest as they serve as analogs for conditions that prevailed during the Cryogenian Period (∼720 – 636 Ma) when Earth experienced two long-lasting glaciations interspersed with periodic ‘hothouse’ conditions as articulated in the ‘Snowball Earth’ hypothesis (Hoffman et al., 1998, 2017; Rooney et al., 2015). During ice-house times, sea-ice extended to low latitudes, perhaps, completely enveloping the planet. This would have severely limited photosynthesis in the oceans leading to pervasively anoxic water columns (Lechte et al., 2019). Fossil evidence, lipid biomarkers, and geochemical data, however, indicate that biogeochemical cycles were active and that major lineages thrived and diversified during the Cryogenian epoch (Corsetti et al., 2006; Moczydłowska, 2008; Brocks et al., 2017; Hoffman et al., 2017; Johnson et al., 2017). This suggests a prevalence of microbial refugia despite challenging surface conditions. Meltwater pond systems, similar to those of McMurdo Sound, cryoconites, and environments at the edges of the ice shelves could have supplied oxygen-rich meltwaters to the oceans and provided habitable conditions for more complex biota during this period (Hoffman, 2016; Hawes et al., 2018; Lechte et al., 2019).
Lipid biomarkers, such as intact polar lipids (IPLs), heterocyte glycolipids (HGs), and bacteriohopanepolyols (BHPs), have been extensively applied to investigate the chemotaxonomic composition of metabolically active microbial communities. IPLs comprising a polar head group bound to a glycerol backbone and linked to a hydrophobic hydrocarbon tail, encode valuable chemotaxonomic information (Rütters et al., 2002; Sturt et al., 2004). HGs comprise a sugar head group attached to an alkyl chain with 26 – 32 carbon atoms (e.g., Gambacorta et al., 1999; Bauersachs et al., 2009a) and are used to evaluate communities of diazotrophic cyanobacteria (Bauersachs et al., 2011). BHPs are composed of a pentacyclic triterpenoid core with functional groups along a ribose-derived side chain. They are used as biomarkers for bacteria and their ecological niches due to the high diversity of chemical structures and characteristic distribution patterns (e.g., Rashby et al., 2007; Matys et al., 2019).
The high biomass and low anthropogenic interference in these meltwater ponds, coupled with climatic conditions unique to the Antarctic, constitute an ideal natural laboratory to study homeoviscous adaption strategies of the microbial cell membranes. However, to date, only one study has investigated the lipid distribution of actively growing microbial mats in three McMurdo Ice Shelf meltwater ponds (Jungblut et al., 2009), while another recent study investigated lipid biomarkers in ancient microbial mats from the McMurdo Ice Shelf (Lezcano et al., 2022). Here, we expand on this to include comprehensive biomarker analyses combined with 16S and 18S rRNA gene composition data to further characterize the microbial communities that thrive in this unusual setting. We also compare the lipid distribution of the ‘dirty ice’ samples with microbial communities in the ice-covered Antarctic lakes from the McMurdo Dry Valleys and some well-documented (sub)tropical and hot spring environments to assess adaptions of the lipid membrane to changes in nutrient supply, temperature, and irradiance regimes. These findings offer a new perspective on biosignatures of the cryosphere and the Cryogenian Period.
Materials and Methods
Sample Collection and Study Sites
Microbial mat samples were collected from twelve meltwater ponds on the McMurdo Ice Shelf, located adjacent to Bratina Island (Figures 1A,B, 78.00’S, 165.30’E) in January 2018. Clean stainless-steel spatulas were used to excise slices of submerged, intact mats from the pond edges, avoiding the underlying sediment as much as possible. In addition, one semi-dried mat located above the water line was collected from Skua Pond. Layers of different colors were evident in some cases, but not all. However, no delamination was attempted since we were interested to sample the living community throughout. In addition, we collected ‘Nostoc spheres’ and a ‘sheet,’ which lay on top of the microbial mats from four ponds (Table 1). The ‘Nostoc spheres’ had a size of 5 – 15 mm in diameter, a brownish color, and consisted of a community of Nostoc and grazers. They were neutrally buoyant could be scooped up with an aquarium net, thereby avoiding any material from the mats below. They were collected from the littoral zone of the ponds in a water depth, ranging from 10 to 30 cm. All samples were frozen immediately after sample collection in combusted glass jars and returned to New Zealand (NZ), where they were lyophilized, shipped to Cambridge (United States), and then maintained at −20°C until lipid extraction. The physicochemical conditions of the meltwater ponds (Table 1) were measured at each site with a HA40d portable multimeter (Hach, Loveland, CO, United States) (Hawes et al., 1993). Small subsamples of intact mats for DNA analysis were taken with a sterilized scalpel, transferred to sterile Eppendorf tubes, and frozen, on-site, in liquid nitrogen. These samples were initially air-lifted to NZ before being shipped frozen to the Natural History Museum London (United Kingdom), where they were stored at −20°C until further processing.
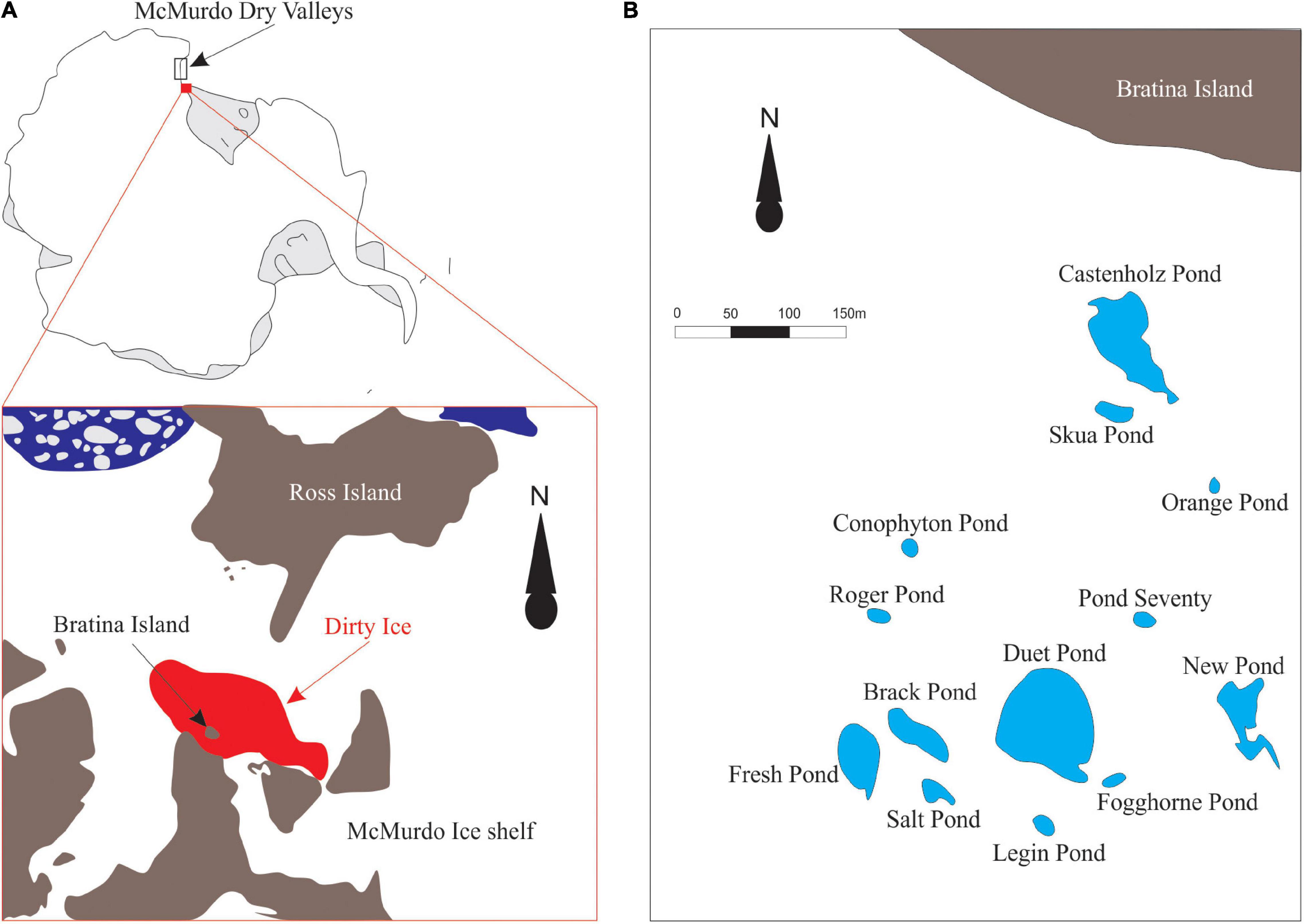
Figure 1. (A) The schematic figure of the sampling location on the McMurdo Ice Shelf (78.00’S, 165.30’E). Microbial mats were collected from meltwater ponds on the ‘dirty ice’ marked in red. The figure was modified from Hawes et al. (2018). (B) Schematic map of the investigated meltwater ponds in the ‘dirty ice’ (modified from Jackson et al., 2021).
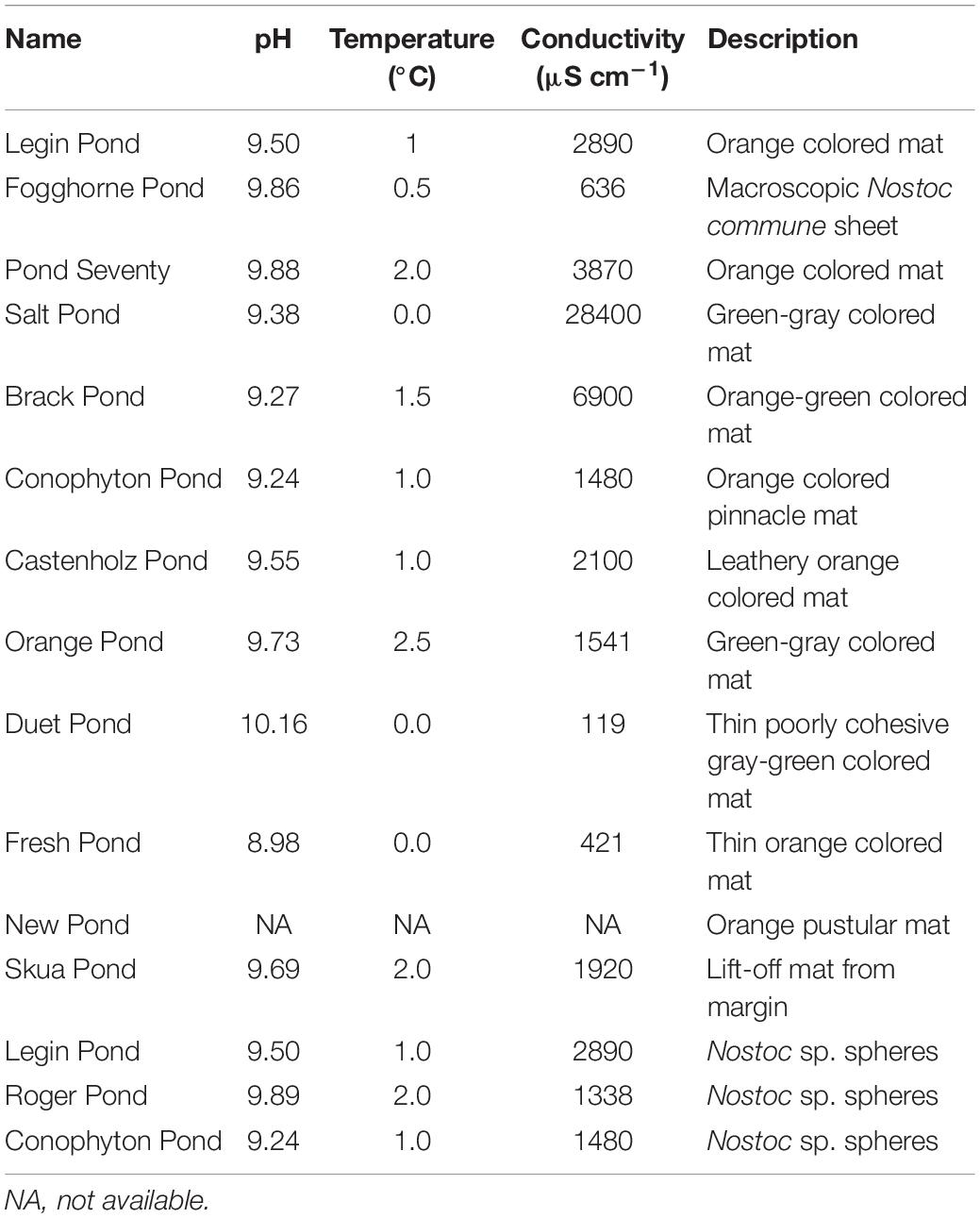
Table 1. Investigated meltwater ponds, their physicochemical properties, and the description of the microbial mats in the ponds.
Sampling of Microbial Mats From Antarctic Lakes
To evaluate the effect of irradiance on lipid composition, additional mat samples from three ice-covered Antarctic lakes were investigated: Lake Joyce (77.72°S, 161.62°E), Lake Fryxell (77.36°S, 162.6°E) and Lake Vanda (77.52°S, 161.67°E, Supplementary Figure 1). The physicochemical conditions and incident photosynthetically active radiation (PAR) from sample sites are shown in Table 2. All lakes are located in the McMurdo Dry Valleys and are perennially covered with a 4–7 m thick layer of ice that attenuates the photosynthetically active radiation. Samples were collected from depths below the ice-water interface by divers. Samples were either taken using push core or cut from the surrounding mat with a spatula and delaminated from the underlying unconsolidated mat and sediment to maintain mat layering and orientation. Two samples were collected from Lake Joyce: the sample from 20 m depth was sampled from the outer surface of a columnar stromatolite in November 2009 (PAR 0.1%) (Mackey et al., 2018), and the sample from 12 m depth from a flat mat adjacent to a pinnacle stromatolite in November 2014 (PAR 0.7%) (Mackey et al., 2017b). Lake Fryxell samples were collected in November 2012 from 9.1 (PAR 0.5%) and 9.7 (PAR 0.3%) m depth along a benthic transect (Jungblut et al., 2016). Lake Vanda samples (PAR 0.9 – 14%) were collected at 9 m depth in December 2013, where microbial mats grew on former lakeshore deposits, including loose boulders that partially shade underlying mats (Mackey et al., 2017a). For samples from Lakes Joyce, Fryxell, and Vanda, PAR was measured within 1 m of the lakebed by divers using a diver-deployed LI-1400 data logger with an LI-192 cosine corrected underwater quantum detector (LI-COR Biosciences, Lincoln NE, United States). For the partially shaded mats from Lake Vanda, PAR variation across mat samples was modeled using Structure from Motion reconstructions of the lake bottom combined with measurements of the angular light field at the sampling depth (Mackey et al., 2017a). Samples from Lake Vanda were collected across variations in modeled PAR following Matys et al. (2019). A figure of modeled incident PAR and sampling sites is available in Supplementary Figure 2.
Sampling of Microbial Mats From (Sub)tropical and Hot Spring Environments
To compare the lipid composition of the meltwater ponds with microbial mats from (sub)tropical and hot spring environments, additional samples were investigated from Highborne Cay (24.72°N, 76.82°W, Bahamas), Hamelin Pool in Shark Bay (26.26°S, 114.21°E, Australia), and Yellowstone National Park (44.55°N, 110.84°W, “Spent Kleenex-spring,” Lower Geyser Basin). The mats from Highborne Cay were collected in 2010 from a tidal flat and included some encrusted algae. The Hamelin Pool samples were taken from a stromatolite-forming cyanobacterial mat (colloform mat, structures with moderately laminated carbonate fabrics) in June 2011. The Yellowstone sample was collected in June 2012. All samples were stored in sterile Whirl-Paks immediately after sample collection at −20°C and freeze-dried before extraction.
DNA Extraction and Polymerase Chain Reaction
DNA was extracted using the PowerBiofilm DNA isolation Kit (MO BIO Laboratories). Archaeal and bacterial 16S small ribosomal subunit rRNA (260 bp) was sequenced by the primers utilizing 515 F (5′-TGGCCAGCMGCCGCGGTAA -3′) and 806R (5′- GGACTACNVGGGTWTCTAAT -3′) (Caporaso et al., 2011, 2012). Eukaryotic 18S rRNA gene (130 bp) was amplified using the primers 1391F (5′- GTACACACCGCCCGTC -3′) and EukBr (5′- TGATCCTTCTGCAGGTTCACCTAC -3′) (Amaral-Zettler et al., 2009; Caporaso et al., 2011). Triplicate PCR reactions were prepared in a sterilized laminar hood with a final volume of 20 μL. Different DNA volumes (0.5, 1.0, and 1.5 μL) were used for each replicate to compensate for amplification biases. The reaction mix contained: 9.84 μL sterile PCR-grade water, 0.8 μL of bovine serum albumin (20 mg mL–1), 4 μL of 5X GoTaq reaction buffer (Promega), 2 μL of MgCl2, 0.16 μL of 200 μM dNTP, 0.2 μL GoTaq G2 DNA polymerase (Promega) and 1 μL of each primer (10 μM). DNA was amplified using the following thermocycler program: 94°C for 3 min, followed by 30 cycles of 94°C for 45 s, 50°C (16S rRNA gene amplification) or 56°C (18S rRNA gene amplification) for 60 s, 72°C for 90 s and a final extension at 72°C for 10 min. In the following, PCR products were purified using AxyPrep Mag PCR Clean-up Kits (Axygen), and triplicate products were combined. The concentration of the purified PCR products was measured in duplicate with a Qubit 2.0 Fluorometer (Thermo Fisher Scientific), and 16S and 18S rRNA gene amplicons were pooled separately at equimolar concentrations for high throughput sequencing. The samples were sequenced (2 × 250 cycles) on an Illumina Miseq platform at the Natural History Museum sequencing facility (United Kingdom). The sequencing data has been deposited at SRA (BioProject ID: PRJNA 758785).
16S and 18S rRNA Gene Sequence Analyses
16S and 18S rRNA gene sequences were analyzed using the QIIME 2 bioinformatics platform, version 2019.4 (Bolyen et al., 2019). The demultiplexed paired-end reads were joined and denoised using DADA2 processing (Callahan et al., 2016). Chimeras and low-quality sequences were removed during this step. Low-frequency amplicon sequence variants (ASVs), which appeared fewer than 3 times, were removed. ASVs were assigned taxonomy using the SILVA 132 database (Quast et al., 2013; Yilmaz et al., 2014). Chloroplast and mitochondrial sequences were also removed, and the datasets were then rarefied to compare community compositions across samples.
Lipid Extraction
Before lipid extraction, lyophilized mats and ‘Nostoc spheres’ were homogenized. IPLs, HGs, and BHPs were extracted four times following a modified Bligh and Dyer protocol (Sturt et al., 2004) with methanol (MeOH), dichloromethane (DCM), and an aqueous solution (2:1:0.8, vol/vol/vol). For the first two extraction steps, the aqueous solution was prepared from monopotassium phosphate (8.7 g L–1, pH 7.4), while in steps three and four, trichloroacetic acid (50 g L–1, pH 2) was added to the aqueous phase. The sediments were extracted two additional times with DCM and MeOH (9:1, vol/vol) to enhance the extraction efficiency of less polar compounds. The samples were sonicated for 20 min in an ultrasonic bath during each extraction step. The sonicated sediment was then centrifuged at 175 relative centrifugal force (rcf) for 10 min, and the supernatants were combined in a separatory funnel. Deionized Milli-Q water was added to the funnel to allow phase separation. The aqueous solution was extracted three times with DCM. Finally, the organic phase was washed three times with deionized Milli-Q water, and the total lipid extract (TLE) was gently dried under a stream of N2 and stored at −20°C until further processing.
Intact Polar Lipids and Heterocyte Glycolipids Analyses
The IPLs and HGs were measured in aliquots of the TLE using a 6520 accurate mass quadrupole time-of-flight mass spectrometer (Agilent Technologies) with an electrospray ionization source coupled to a 1200 series high-performance liquid chromatograph (HPLC, Agilent Technologies). IPLs and HGs were separated using a Waters Acquity amide column (150 mm × 2.1 mm, 1.7 μm particle size) used in hydrophilic interaction mode, as described previously (Wörmer et al., 2013). Eluent A consisted of acetonitrile, DCM, formic acid, and ammonium hydroxide (75:25:0.01:0.01, vol/vol/vol/vol), and eluent B was prepared from HPLC-grade water, MeOH, formic acid, and ammonium hydroxide (50:50:0.4:04, vol/vol/vol/vol). The eluent flow and temperature remained constant at 0.4 mL min–1 and 40°C throughout the analysis. The gradient started with an isocratic flow of 5% B for 4 min. The proportion of eluent B gradually increased to 25% at 22.5 min and 40% at 26.5 min. Eluent B was held at 40% for 1 min, and then the column was re-equilibrated with 5% B for 8 min. The mass spectrometer was operated in positive ionization mode, scanning 200 – 2,000 daltons. IPLs and HGs were identified based on their exact mass, retention time, and characteristic fragmentation patterns (Sturt et al., 2004; Bauersachs et al., 2009b; Wörmer et al., 2012; Bale et al., 2018). IPL and HG abundances were estimated semi-quantitatively by comparing the parent ion relative to a known amount of injection standard (phosphocholine archaeol, 10 ng injected). For IPLs, response factors were determined from commercially available standards. No standards were available for betaine lipids, ornithine lipids, sulfoquinovosyl diacylglycerol (SQ-DAG), phosphoinositol diacylglycerol (PI-DAG) and HGs, so a relative response factor of unity was chosen for these compounds. Response factors were determined in triplicates with standard variations between 1 and 17% (mean 5%). The lipid measurements are based on singular injections; however, an analytical error of <17% can be applied based on the response factor triplicates. All detected IPLs and HGs were in the linearity range of the instrument.
Analysis of Bacteriohopanepolyols
An aliquot of each TLE was acetylated with 40 μL of a mixture of pyridine and acetic anhydride (1:1, vol/vol) at 70°C for 1 h and left overnight at room temperature prior to the analysis of BHPs. The analysis was performed using the same HPLC-MS system and methodology as described by Matys et al. (2017). BHPs were separated by an Agilent Poroshell 120 EC-C19 column (2.1 mm × 150 mm, 2.7 μm particle size), maintained at 30°C, and a solvent flow rate of 0.19 mL min–1. Eluent A contained a mixture of MeOH and HPLC-grade water (95:5, vol/vol) and eluent B consisted of isopropanol (100%). The gradient started with 0% B for 2 min, increased to 20% at 20 min, stayed constant for 10 min, and further increased from 20% B (30 min) to 30% B at 40 min. Thereafter, the column was reconditioned for 15 min with 0% B. BHPs were identified based on their accurate mass, retention time, and characteristic fragments (Talbot et al., 2003, 2007, 2008). BHPs were quantified using 3α,12α-dihydroxy-5β-pregnan-20-one 3,12-diacetate (Matys et al., 2017). Due to the highly complex mixture of BHPs in the mat samples and the lack of available standards, BHPs were not corrected for response factors. BHPs were detected in singular injections and were in the linearity range of the instrument. The lipid data has been deposited at the AHED repository (https://doi.org/10.48667/r1zs-v785).
Calculations
Variation of the microbial community between the different ponds were analyzed by a principal coordinates analysis (PCoA). PCoA analysis for Bacteria, Archaea, Eukaryotes and IPLs was calculated by Bray-Curtis dissimilarities matrices using the “vegan” package in R v. 4.0.4 (Dixon, 2003; R Core Team, 2021) and projected on a 2D-plot.
To evaluate the membrane properties, the double bond index (DBI) and the average chain length (ACL) of IPLs were calculated as described by Evans et al. (2017). CX:Y in the DBI refers to the peak area of the compound with x carbon atoms and y unsaturation. The peak area of the compound was multiplied by the number of unsaturation and divided by the total peak area of all compounds (Eq. 1). The ACL was calculated by multiplying the number of x C-atoms in the side chain with the peak area of the compound, divided by the total summed peak area of all compounds (Cx:y, Eq. 2). To account for varying chain lengths of the different IPL species (e.g., PE-DAG C27 – 38:Y and DPG-DAG C57 – 73:Y), the number of unsaturations was normalized according to their average chain length (unsaturation per carbon atom, U/C, Eq. 3).
Results and Discussion
This study extends our knowledge of bacterial and eukaryotic microbial communities on the McMurdo Ice Shelf by combining lipid biomarker and molecular analyses of microbial mats from twelve meltwater ponds and three separately collected ‘Nostoc spheres.’ Prior lipid analysis in these environments screened for hydrocarbons, sterols, and fatty acids (Jungblut et al., 2009; Lezcano et al., 2022). This study focuses on IPLs, HGs, and BHPs to provide insights into how microbes may have shaped their lipid membranes to adapt to the local ice-shelf conditions and, by extension, to environments that may have existed during the Cryogenian glacial epoch.
16S and 18S rRNA Gene Diversity in the Meltwater Ponds
The 16S rRNA gene analysis revealed a dominance of cyanobacterial gene sequences (40–70%, Figure 2), highlighting the predominance of phototrophic bacteria in the microbial mats in the meltwater ponds. Our analysis confirms that cyanobacterial genera, such as Nostoc, Oscillatoria, Leptolyngbya, Phormidium, and Pseudanabaena (Supplementary Table 1), are important primary producers in Antarctic inland water environments and that they are well adapted to the persistently cold settings (e.g., Jungblut and Neilan, 2010; Jackson et al., 2021). Accordingly, cyanobacteria could have played a major role in sustaining life during the glaciations of the Cryogenian period, consistent with the detection of microbialites preserved in a Cryogenian sequence in Yukon, Canada (Macdonald et al., 2018). Moreover, carbonates from Svalbard, deposited during the Marinoan pan-glaciation (∼650 – 635 Ma ago), show textures consistent with microbial mat accumulation associated with glacial sedimentation (Fairchild et al., 2016), thus, providing strong parallels between Cryogenian sediments and modern deposits from the McMurdo Dry Valley region.
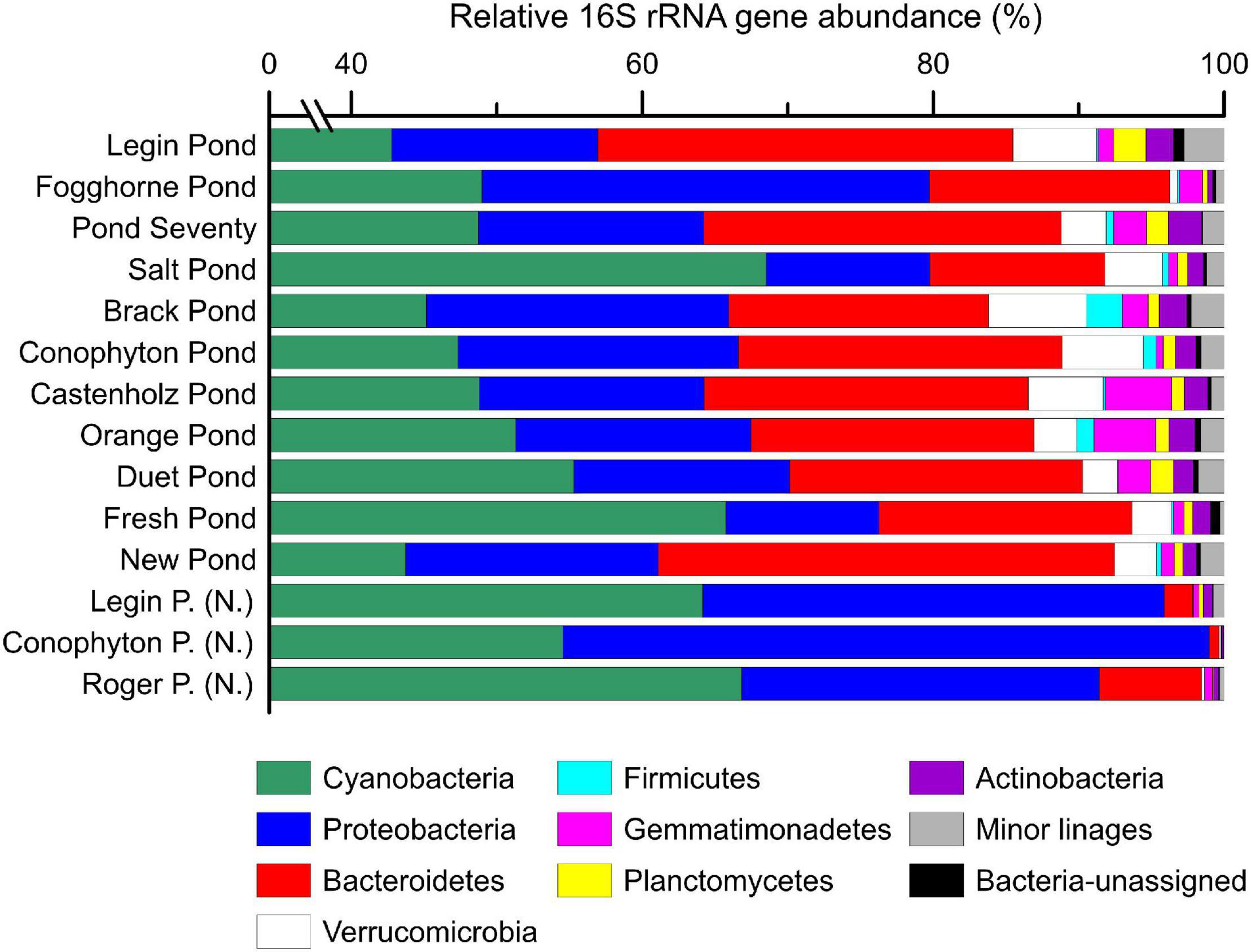
Figure 2. The relative abundance (%) of bacterial 16S rRNA genes in microbial mat communities. Shown are the major bacterial phyla. Samples with sub-fix (N.) indicate ‘Nostoc spheres,’ which were collected in three different meltwater ponds. Detailed results from the 16S rRNA gene sequencing are provided in the supporting information. Note the broken axis between 1 and 39%.
A principal coordinate analysis, based on the Bray–Curtis dissimilarity of 16S rRNA gene bacterial community, revealed distinct assemblages across the different meltwater ponds (Figure 3). The ‘Nostoc spheres’ and Nostoc commune sheet from Fogghorne Pond formed one cluster, which predominantly contains Nostoc spp. (i.e., N. punctiforme PCC 73102, Supplementary Table 1) (Rippka et al., 1979). The low conductivity cluster (Figure 3), on the contrary, revealed a higher diversity of cyanobacteria (Supplementary Table 1). The 16S rRNA gene survey from Brack and, in particular, Salt Pond showed variations of the microbial community composition correlating with increasing conductivity (Figure 3) (Jackson et al., 2021). Both ponds showed a high abundance of Oscillatoria spp. (Supplementary Table 1), suggesting that these filamentous non-heterocytous cyanobacteria are tolerant of brackish conditions. Moreover, the microbial mats from Salt Pond revealed a prevalence of the heterocytous cyanobacterium Nodularia, which is well adapted to intermediate salt concentrations (Möke et al., 2013). High abundances of these cyanobacteria in Salt Pond have also been observed recently by Jackson et al. (2021).
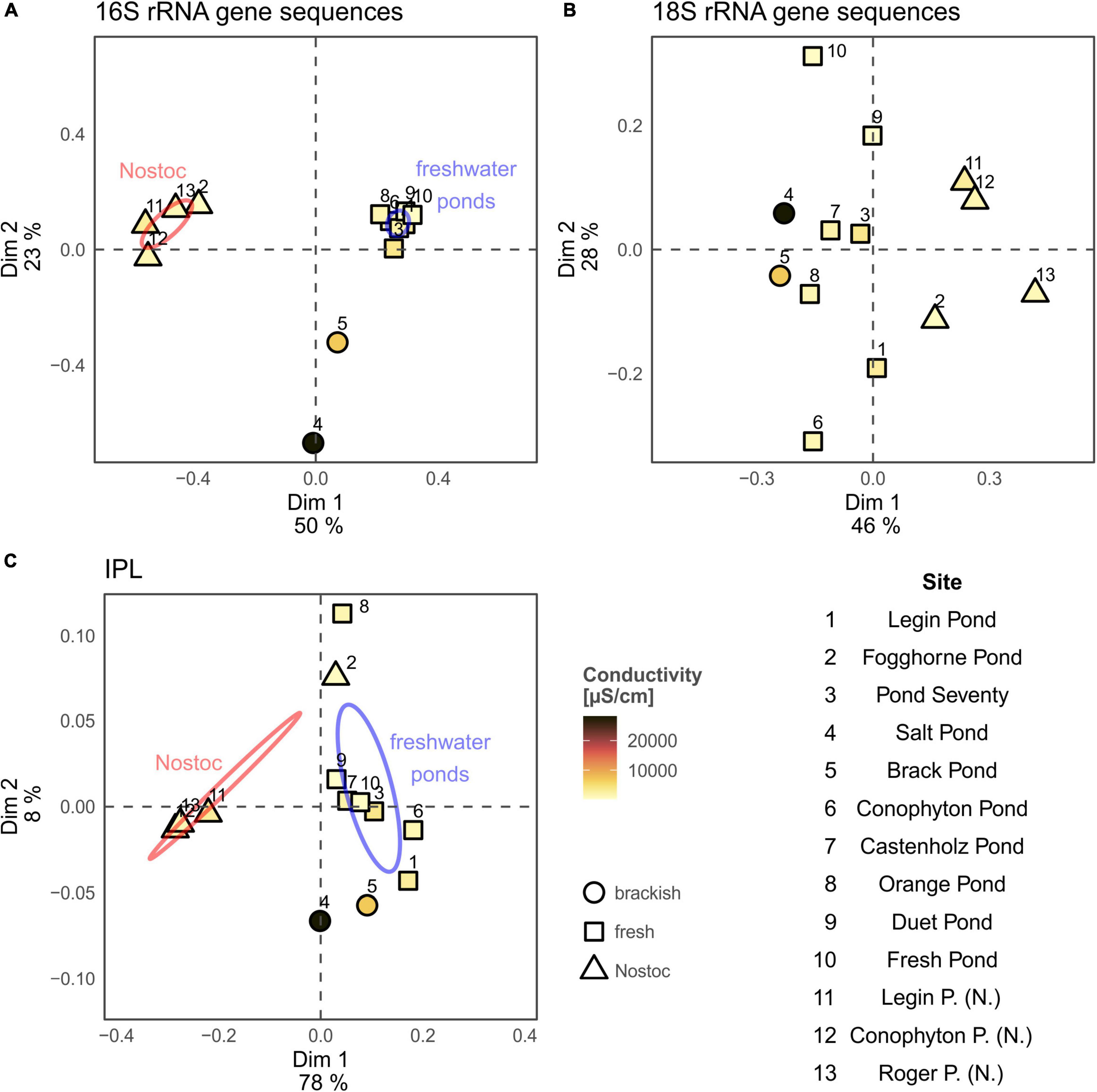
Figure 3. Principal coordinates analysis (PCoA) of the 16S rRNA gene (A) and 18S rRNA gene community composition (B) and intact polar lipid analysis (C) from the microbial mats collected at the McMurdo Ice Shelf. Ellipses for Nostoc type samples (samples 2, 11, 13, and 14; red; see Table 1) and freshwater samples (blue; see Table 1) represent the 95% confidence interval and were calculated using build in function of the “vegan” package. Conductivity results are shown in Table 1. Results from New Pond and Skua Pond were excluded from the PCoA analysis due to missing environmental (New Pond) and rRNA gene sequence data (Skua Pond).
Other important phyla in the microbial mats are Proteobacteria (10–45%) and Bacteroidetes (1–30%) based on 16S rRNA gene sequences (Figure 2). Proteobacteria revealed a high abundance of α- and β-Proteobacteria, and Bacteroidetes are dominated by Sphingobacteriales and Cytophagales (Supplementary Tables 2, 3). A predominance of these microbes has also been previously found in Antarctic meltwater ponds (Varin et al., 2012; Jackson et al., 2021). Minor groups (<10%) include representatives of Verrucomicrobia, Firmicutes, Gemmatimonadetes, Planctomycetes, and Actinobacteria (Figure 2). This agrees with a previous study that has detected these phyla in the microbial mats from the McMurdo Ice Shelf (Varin et al., 2012). While similar 16S rRNA gene communities were identified for the microbial mats on the McMurdo Ice Shelf and Lake Untersee, we are also aware that PCR can lead to amplification biases (Greco et al., 2020; Jackson et al., 2021). Further evaluation of the composition will benefit from metagenomic approaches that cover all DNA, including ribosomal and protein-coding genes, without PCR.
Archaeal 16S rRNA gene sequences were only detected in Pond Seventy and Salt Pond and represented less than 0.01% of 16S rRNA gene sequences (Supplementary Table 4). This suggests that archaea are only a minor component of microbial mats in meltwater ponds on the McMurdo Ice Shelf, which is in line with previous studies (Varin et al., 2012; Jackson et al., 2021). However, while neither amplicon nor metagenomic analyses of microbial mats on the McMurdo Ice Shelf (Varin et al., 2012; Jackson et al., 2021) have been able to detect high abundances of archaea, it cannot be excluded that the universal 16S rRNA gene primers used here (Caporaso et al., 2012) are less efficient to cover all archaeal groups, as suggested earlier (Parada et al., 2016).
The meltwater ponds contained several eukaryotic groups including, microalgae, amoebae, metazoa, and fungi (Figure 4 and Supplementary Tables 5–9). The ‘Nostoc sphere’ samples revealed a high relative abundance of rotifers compared to the other samples, suggesting that these microscopic animals are grazing on the cyanobacteria. In addition, these samples had a lower proportion of photosynthetic eukaryotes, which were primarily of the families Chlorophyceae and Bacillariophyceae. The other microbial mats from the meltwater ponds revealed a more heterogeneous distribution of eukaryotes, additionally featuring a higher proportion of protists such as the Amoebozoa (Discosea and Tubulinea) and ciliates. PCoA of the 18S rRNA gene sequences revealed no distinct eukaryal assemblages in the microbial mats from the meltwater ponds, consistent with previous observations that eukarya on the McMurdo Ice Shelf have a broad tolerance to the local environmental conditions (Jackson et al., 2021). The genetic diversity of phototrophic and heterotrophic eukaryotes in the microbial mats highlights that the meltwater ponds are a habitat for a diverse eukaryotic community. Protists, fungi, and microfauna vary in size and range from uni- to multicellular organisms. Therefore, it cannot be excluded that some methodological biases occur in the documented relative 18S rRNA gene abundance in the community composition.
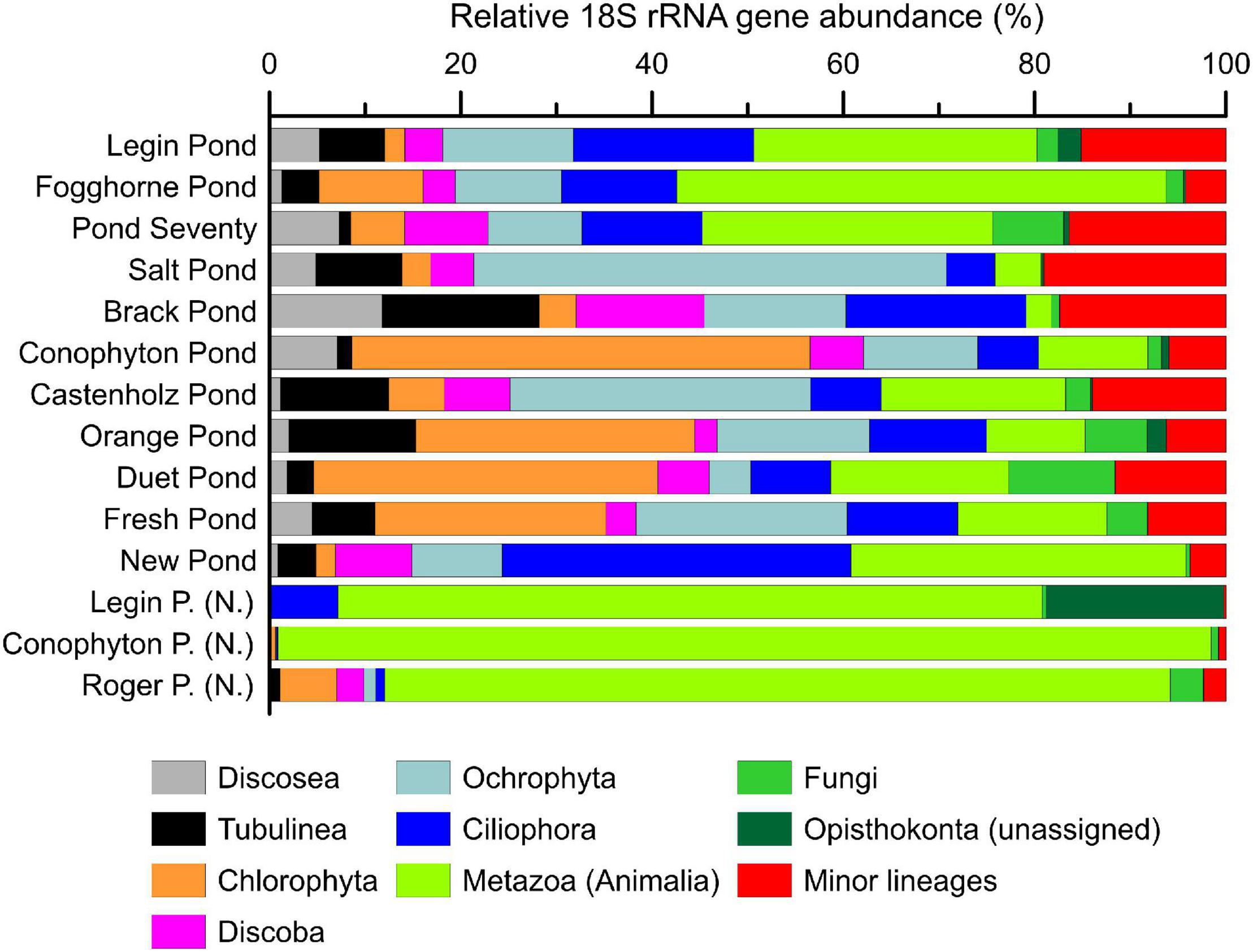
Figure 4. The relative abundance (%) of eukaryotic 18S rRNA genes in microbial mat communities. Shown are the major eukaryotic phyla. Detailed results from the 18S rRNA sequencing can be found in the supporting information. Samples with (N.) indicate ‘Nostoc spheres,’ which were collected in three different meltwater ponds.
Source Assignments of Intact Polar Lipids in Meltwater Ponds
A variety of bacterial and eukaryal IPLs, comprising glycosidic, phosphatidic, and nitrogen-bearing head groups linked via ester bonds to the hydrophobic tail were detected in the meltwater ponds (Figure 5, for structures, see Supplementary Figure 4). In line with the molecular data (Supplementary Table 4), the results revealed a predominance of IPLs associated with cyanobacteria. Thus, glycolipids (G-DAGs), which are the major lipid class in cyanobacteria (Wada and Murata, 1998), showed the highest relative abundance (45 – 60%, Figure 5A). PG-DAG/DPG-DAG (di)-phosphoglycerol-DAG and SQ-DAG are also commonly detected in cyanobacteria (Wada and Murata, 1998) represented 14–25% and 1–3% of the total IPLs in the microbial mats from the McMurdo Ice Shelf (Figure 5A). Other detected IPLs, such as PC-DAG (2–11%), PE-DAG (phosphoethanolamine-DAG, 6–14%), and PI-DAG (∼1%), are rarely present in cyanobacteria (e.g., Wada and Murata, 1998), suggesting that these lipids are derived from other classes of bacteria or eukarya. This is also indicated by the 16S rRNA gene results, which showed that Proteobacteria and Bacteroidetes play an important role in the meltwater pond communities (Figure 2). PE-DAGs are ubiquitous in various bacterial groups (e.g., Goldfine and Hagen, 1968; Sturt et al., 2004), while PC-DAGs are commonly associated with eukarya (Raetz, 1986). Nevertheless, up to 15% of bacteria produce PC-DAGs (Geiger et al., 2013) – complicating the assignment of this lipid class to a specific biological source. Aminolipids were the least abundant lipids in the microbial mats from the meltwater ponds (2–15%, Figure 5A). Ornithine lipids, representing up to 8% of the total IPLs, are widespread in bacteria (Geiger et al., 2010), whereas betaine lipids (2–14%) are typically found in eukaryotic algae, fungi, and amoebae (Kato et al., 1996; Sohlenkamp et al., 2003), although they have been reported in cyanobacteria too (Rezanka et al., 2003). Archaeal IPLs were not detected in any mats from the meltwater ponds.
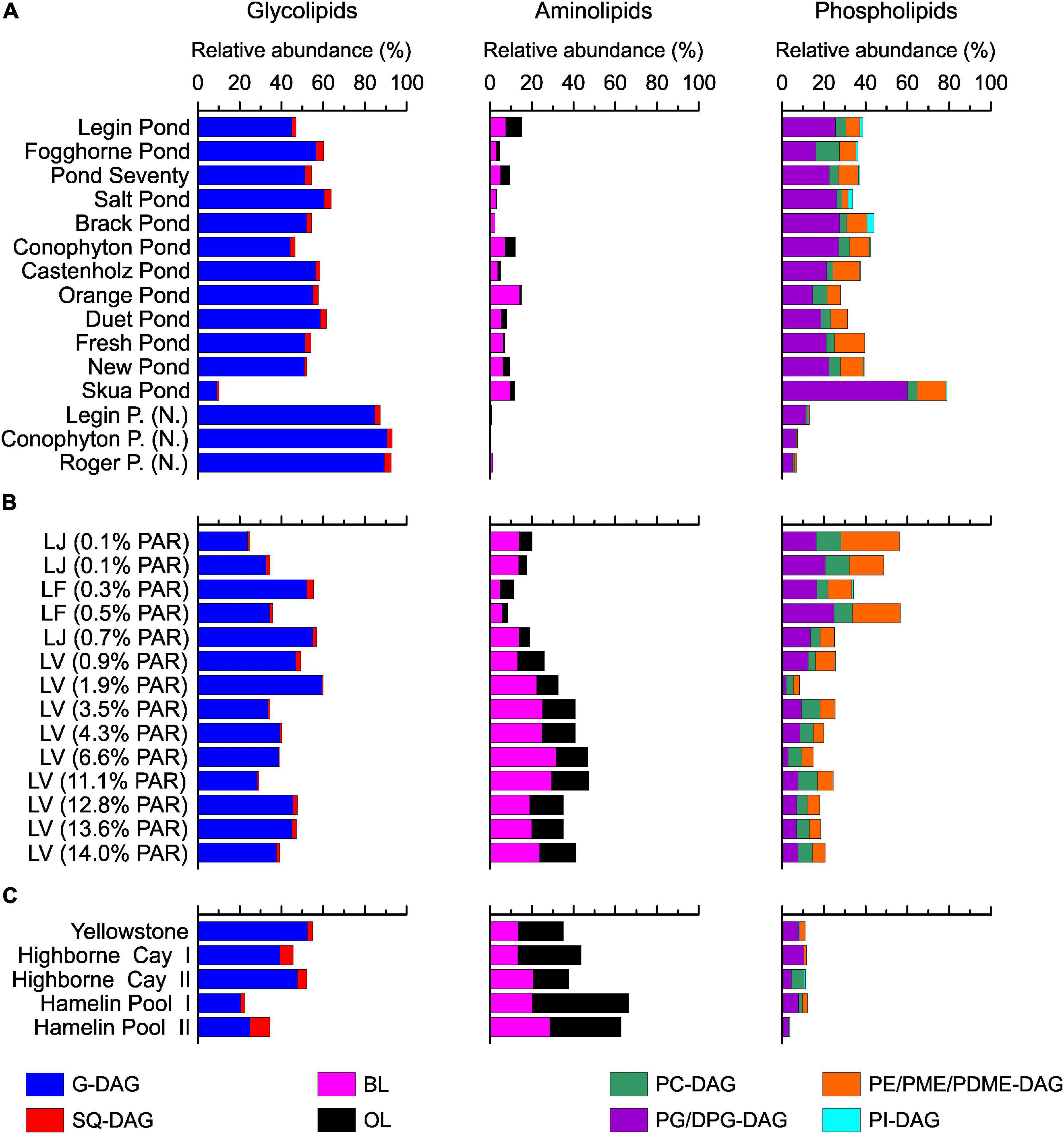
Figure 5. Intact polar lipids head group distribution in the meltwater ponds from the McMurdo Ice Shelf (A). Samples with (N.) indicate ‘Nostoc spheres’, which were collected in three different meltwater ponds. Panel (B) shows the results from the three different Antarctic lakes: Lake Joyce (LJ), Lake Fryxell (LF), and Lake Vanda (LV). The brackets show the percentage of photosynthetically active radiation (PAR) that reached the microbial mats. The PAR was adopted from previous studies (Jungblut et al., 2016; Mackey et al., 2017b,2018; Matys et al., 2019). Panel (C) shows the relative abundance of IPLs in the microbial mats from the Yellowstone National Park (United States), Highborne Cay (Bahamas), and Hamelin Pool (Australia).
Principal coordinates analysis of the IPLs showed separate clusters of the ‘Nostoc spheres’ and the microbial mats (Figure 3). The IPL distribution in the ‘Nostoc spheres’ revealed a high abundance of G-DAGs (85 – 90%), while other IPLs were only present in trace amounts (Figure 5A). The IPL pattern agrees with previous results from Nostoc cultures (Bauersachs et al., 2009a; Schouten et al., 2013). Surprisingly, the Nostoc commune sheet in Fogghorne Pond did not cluster with the ‘Nostoc spheres’ as observed for the 16S rRNA gene analysis. Heterogenic distributions of the bacterial community in this mat may have resulted in variations between the DNA and IPL analysis because individual samples were collected for both types of analyses. An additional explanation may be that the different growth patterns, either as spheres (∼90% G-DAGs) or as sheets (∼55% G-DAGs), could affect the lipid composition in Nostoc communities. Future research is needed to investigate whether different growth patterns might affect the lipid composition in these microbes. Moreover, the PCoA showed no pronounced differences in the IPL distribution between fresh and brackish meltwater. This implies that the low to medium salt concentrations have little effect on the lipid composition of microbes in Antarctic meltwater ponds.
The microbial mat from the Skua Pond showed an IPL distribution distinct from the communities from the other meltwater ponds (Figure 5A). Here, phospholipids were predominant, in particular DPG-DAGs (47%) were abundant. The name ‘Skua’ arose from the presence of nesting skuas in the vicinity. Bird guano is possibly the source of elevated phosphorous in Skua Pond (Hawes et al., 2014). Alternatively, a distinct microbial community, which synthesizes high abundances of DPG-DAG seems also plausible given that this was the only sample that was collected as a broken and partially desiccated mat at the water line, while all other samples were subaqueous. Unfortunately, the Skua DNA samples were compromised during shipping, and thus we were unable to investigate 16S and 18S rRNA genes for this pond.
Heterocyte Glycolipid Composition in McMurdo Ice Shelf Meltwater Ponds
Seven different types of HGs were detected in the meltwater ponds (Figure 6, for structures, see Supplementary Figure 5). HG26 diols and keto-ols were most abundant and constituted between 70 and 100% of all HGs. Particularly, mats dominated by Nostoc spp. (‘Nostoc spheres’ and Fogghorne Pond) and Nodularia spp. (Salt Pond, Supplementary Table 1) showed a strong dominance of HG26 diols and keto-ols. This is in line with previous culture studies, in which both HGs were exclusively detected in these two species (e.g., Gambacorta et al., 1999; Bauersachs et al., 2009a). In addition, the predominance of HG26 diols and keto-ols in microbial mats from Pond Seventy suggests that the dominant but based on the 16S rRNA gene analysis enigmatic cyanobacterial species (Supplementary Table 1B), may be found with the Nostocaceae. The microbial mats from the other meltwater ponds, which showed a more heterogeneous distribution of cyanobacteria (Supplementary Table 1), contained 0 – 28% of HG28 diols, keto-diols, and triols. These HGs have been described as minor compounds in some Nostocaceae, such as Anabaena and Aphanizomenon spp. (Wörmer et al., 2012; Bauersachs et al., 2017), while they are predominant in representatives of Rivulariaceae, including Calothrix spp. (Bauersachs et al., 2009a).
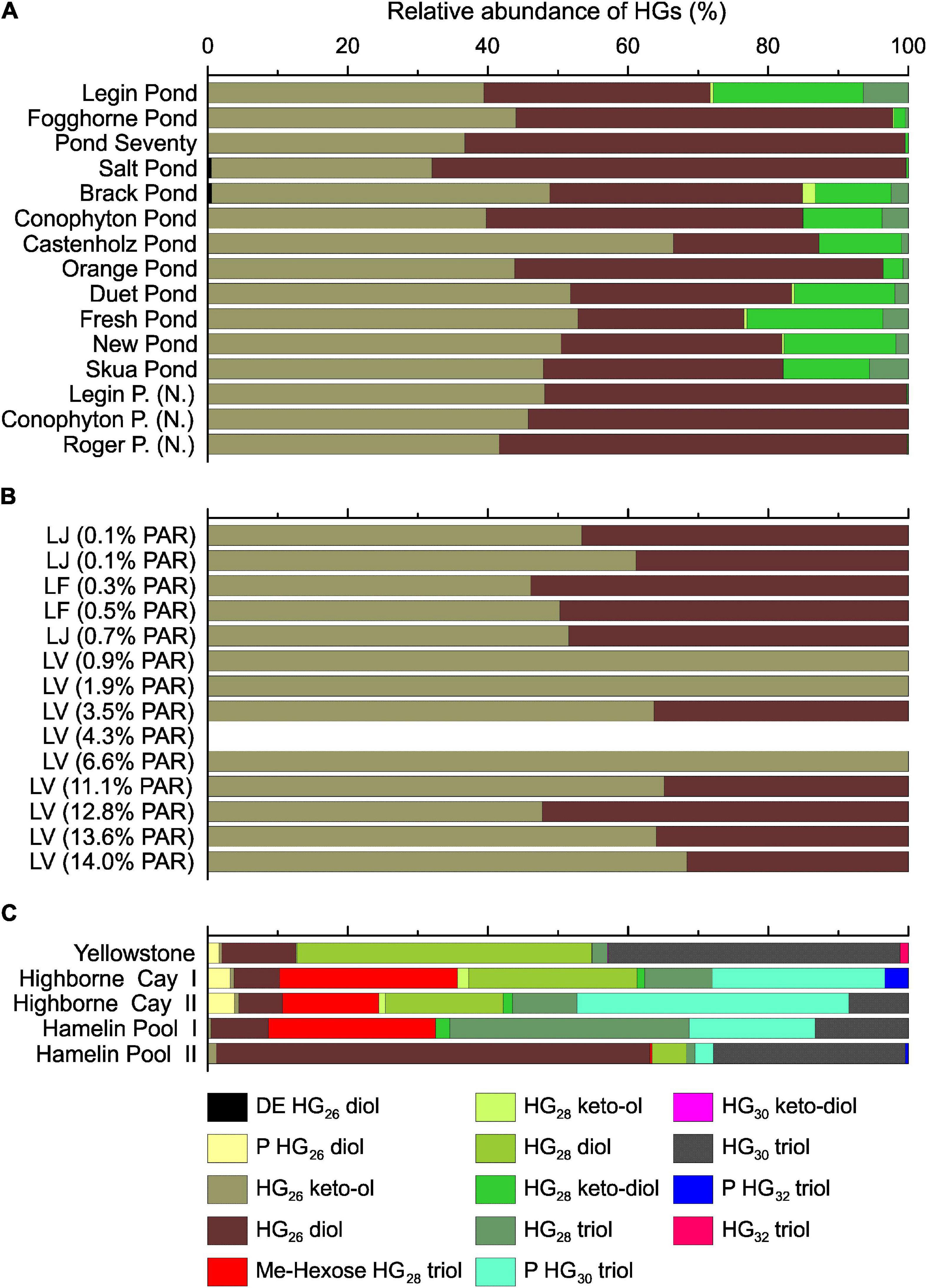
Figure 6. Heterocyte glycolipid distribution in the meltwater ponds from the McMurdo Ice Shelf (A). Samples with (N.) indicate ‘Nostoc spheres,’ which were collected in three different meltwater ponds. Panel (B) shows the results from the three different Antarctic lakes: Lake Joyce (LJ), Lake Fryxell (LF) and Lake Vanda (LV). The brackets show the percentage of photosynthetically active radiation (PAR) that reached the microbial mats. The PAR was adopted from previous studies (Jungblut et al., 2016; Mackey et al., 2017b,2018; Matys et al., 2019). HGs in LV 4.3% PAR were below detection limit. Panel (C) shows the relative abundance of HGs in the microbial mats from the Yellowstone National Park (United States), Highborne Cay (Bahamas), and Hamelin Pool (Australia). DE, deoxyhexose; P, pentose; Me-hexose, methyl-hexose.
Bacteriohopanepolyol Composition in McMurdo Ice Shelf Meltwater Ponds
The BHPs showed a heterogeneous distribution across the microbial mats and ‘Nostoc spheres’ (Figure 7, for structures, see Supplementary Figure 6). Accordingly, no significant connection between the microbial compositions and BHP distributions was visible. Bacteriohopanetetrol (BHT) was the predominant BHP in most samples, followed by aminotriol and BHT cyclitol ether. Microbial mats from Castenholz Pond, Fresh Pond, and Skua Pond, on the contrary, are dominated by BHT cyclitol ether over aminotriol, and BHT. All three components are detected in a wide range of bacteria, which precludes a clear biological assignment of these lipids (Talbot et al., 2008; Van Winden et al., 2012). Aminopentol, predominant in New Pond, has been suggested as a biomarker for methanotrophic bacteria type I (Van Winden et al., 2012), which is surprising given that these bacteria were not detected in this pond based on the 16S rRNA gene analysis. However, a recent study showed that nitrite-oxidizing bacteria are capable of synthesizing aminopentol (Elling et al., 2022), indicating that these compounds may be produced by a range of different bacteria and not exclusively by methanotrophs. Adenosylhopane, which has been reported from α- and β-Proteobacteria as well as cyanobacteria (e.g., Talbot et al., 2007; Van Winden et al., 2012), was a minor BHP in these mats (<2%).
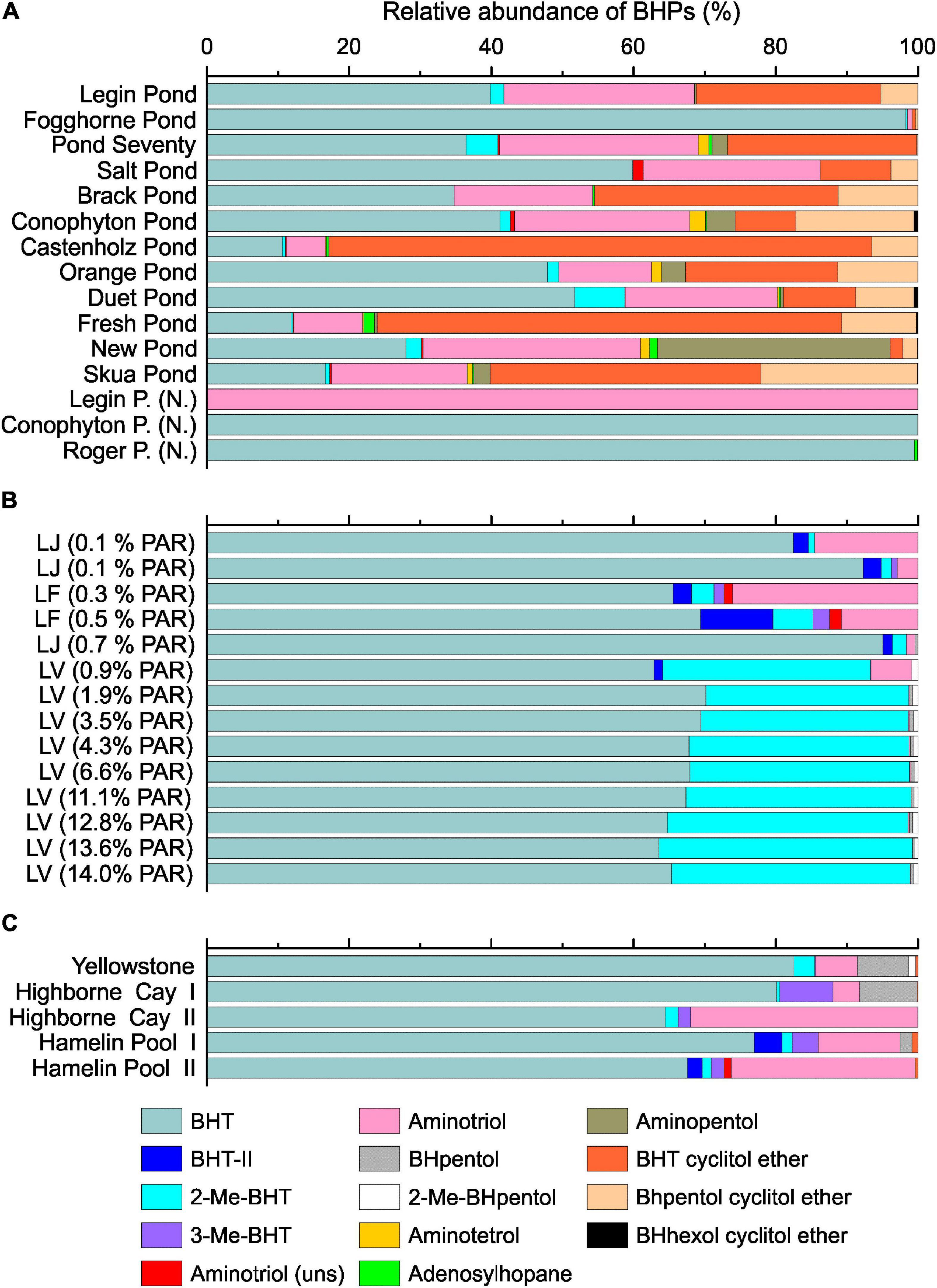
Figure 7. Bacteriohopanepolyols composition in the microbial mats from the meltwater ponds (A). Samples with (N.) indicate ‘Nostoc spheres’ that were collected in three different meltwater ponds. Panel (B) shows the relative BHP distribution from the three different Antarctic lakes: Lake Joyce (LJ), Lake Fryxell (LF) and Lake Vanda (LV). The brackets show the percentage of photosynthetically active radiation (PAR) that reached the microbial mats. The PAR was adopted from previous studies (Jungblut et al., 2016; Mackey et al., 2017b,2018; Matys et al., 2019). Panel (C) shows the relative abundance of BHPs in the microbial mats from the Yellowstone National Park (United States), Highborne Cay (Bahamas), and Hamelin Pool (Australia).
2-Methylated BHPs (2-Me-BHPs) were only detected as minor compounds (<10%, Figure 7A) and, notably, were lacking in the three ‘Nostoc sphere’ samples. The low abundance of 2-Me-BHT is surprising given that these compounds were previously suggested to be broadly prevalent in cyanobacteria including some species of Nostoc (Summons et al., 1999; Doughty et al., 2009). It was subsequently proposed that expression of the hpnP protein responsible for the production of 2-Me-BHT in cyanobacteria is an environmental stress response (Kulkarni et al., 2013; Ricci et al., 2017). For instance, an increasing 2-Me-BHT content with decreasing light intensity was observed (Matys et al., 2019). The high light intensity during austral summer may thus be a factor in the observed low abundance of 2-Me-BHT in the meltwater ponds. In addition, since not all cyanobacteria produce methylated BHPs (Talbot et al., 2008), the taxonomic makeup of the cyanobacteria could lean toward those devoid of the hpnP gene. Moreover, the primacy of a predominantly cyanobacterial origin has diminished as other bacterial sources of 2-Me-BHP are discovered (Bisseret et al., 1985; Rashby et al., 2007; Ricci et al., 2015; Elling et al., 2020).
Comparison of the Lipid Composition From Bacterial Mats Growing Under Different Environmental Conditions
Implications of Nutrient Supply on the Intact Polar Lipid Composition
The subtropical, hot spring, and Antarctic lake microbial mats showed a predominance of G-DAGs over other IPL-groups (20 – 60%), indicating that cyanobacteria likely play an important role in these settings. This agrees with previous DNA based studies showing that cyanobacteria are among the most abundant bacteria in Highborne Cay, Shark Bay, and the investigated Antarctic lakes (Dolhi et al., 2015; Mackey et al., 2015; Ruvindy et al., 2015; Matys et al., 2019). However, the microbial mats from (sub)tropical environments and Lake Vanda revealed a higher relative abundance of amino lipids (25–65%), such as BLs and OLs, than the mats from the meltwater ponds (5–15%, Figure 5). One explanation for the different abundance of amino and phospholipids between these sites is feasibly related to the availability of nitrogen and phosphorous. Low N/P ratios, commonly between 1 and 3, have been observed in the meltwater ponds (Howard-Williams et al., 1990; Sorrell et al., 2013), and Howard-Williams and Hawes (2007) argued that these ponds are primarily nitrogen limited. In Lake Vanda, Highborne Cay, and Hamelin Pool, typically, N/P-ratios above 20 have been reported (Atkinson, 1987; Priscu et al., 1989; Jabro, 2008). This suggests that P may be the limiting nutrient compared to the meltwater ponds, as suggested for Lake Vanda (Dillon et al., 2020). A replacement with N-bearing lipids in phospholipid-containing bacteria has been observed in various studies under P-limited conditions [see review by Schubotz (2019)]. This suggests that the low availability of phosphate in Lake Vanda and the subtropical mats leads to a substitution of phosphatidyl IPLs by aminolipids (Figure 8). Genes for the substitution of nitrogen for phosphorous in membrane lipids have been, for instance, recently detected in microbial mats from Lake Vanda (Dillon et al., 2020).
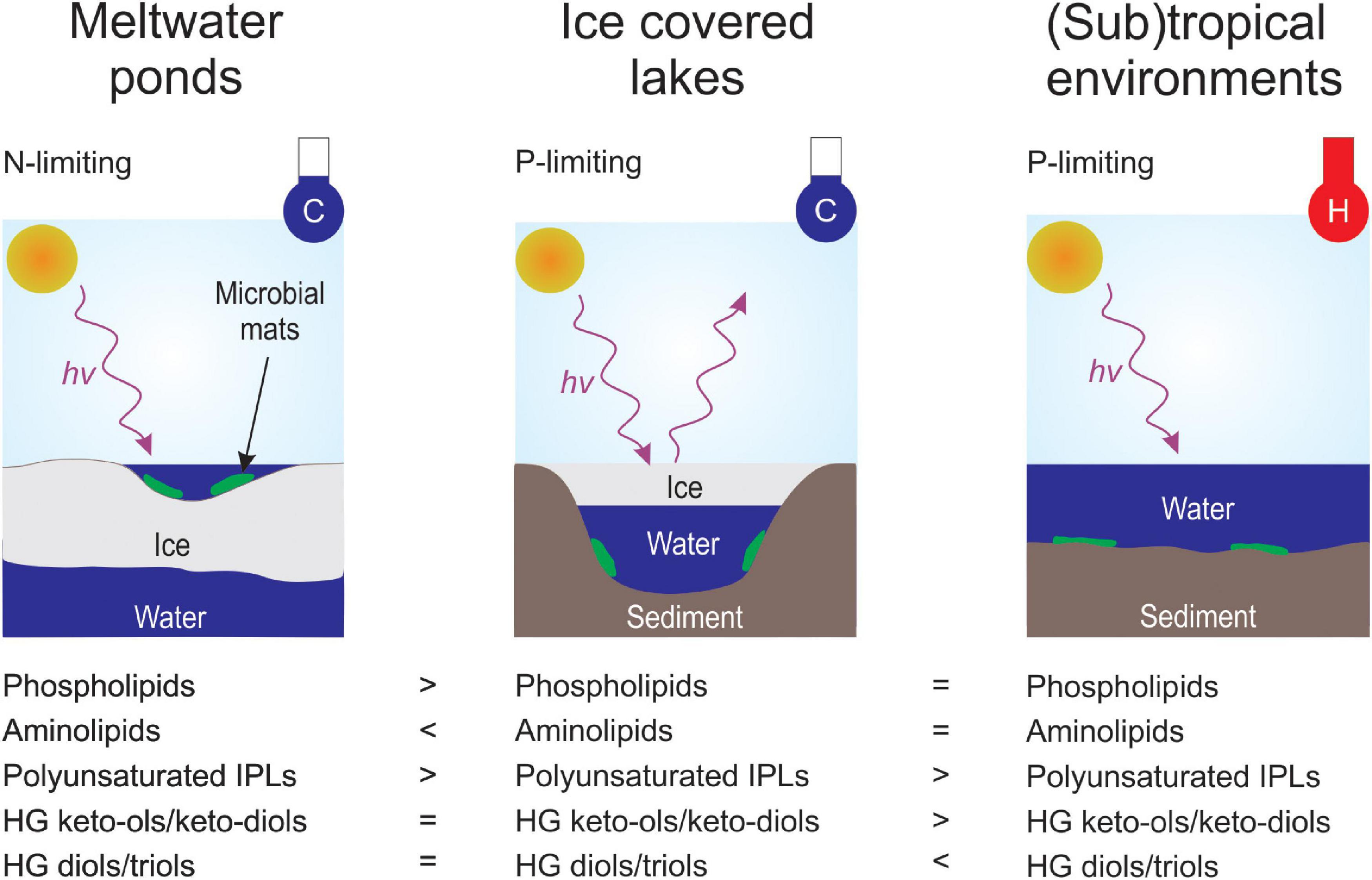
Figure 8. Conceptual sketch of the presenting controlling environmental parameters and the corresponding lipid membrane signatures. Environmental parameters include light regime (hv+), temperature in cold (C; blue thermometer) and warm (H; red thermometer), ice coverage and nutrient (N-limiting or P-limiting) availability.
Implications of Temperature on Intact Polar Lipid Composition
Besides the IPL head group composition, the properties of the core fatty acids were investigated. This analysis revealed that the acyl chains in the (sub)tropical microbial mats were more saturated than in samples from Antarctica (Supplementary Figure 3 and Supplementary Table 10). These observations agree with previous culture-based experiments, where the abundance of unsaturated fatty acids increased with decreasing growth temperature (e.g., Marr and Ingraham, 1962; Suutari et al., 1990; Gombos et al., 1994). In addition, a correlation between bacterial membrane permeability and the degree of saturation of fatty acids has been shown in various earlier studies (e.g., Van de Vossenberg et al., 1995; Murata and Los, 1997). This implies that fatty acid desaturation is an adaptive microbial response to cold environments (e.g., Marr and Ingraham, 1962; Suutari et al., 1990; Gombos et al., 1994) and helps cyanobacteria and other microbes to thrive under the extreme conditions of the Antarctic meltwater ponds and lakes (Figure 8).
Interestingly, Antarctic lake samples contained a higher degree of saturation for most IPL types compared to the microbial mats from the meltwater ponds (Supplementary Figure 3 and Supplementary Table 10). Since samples from both environments were collected at comparable in situ temperatures (Tables 1, 2), this indicates that additional factors, such as ice coverage, could affect the degree of unsaturation in the IPL acyl chain. The meltwater ponds mats are only ice-free during the height of austral summer but frozen during winter (Hawes et al., 2011). In contrast, Lakes Vanda, Joyce, and Fryxell are perennially covered by a thick layer of ice but remain liquid all year. Previous experiments observed an improved resistance to freezing among different bacterial genera when their cell membrane contained high quantities of unsaturated fatty acids (Goldberg and Eschar, 1977; Beal et al., 2001). Accordingly, microbes in the meltwater ponds may increase the extent of unsaturation to survive prolonged periods of freezing during austral winter.
Another potential factor could be the different light regimes of the Antarctic lakes and meltwater ponds. The microbial mats from the Antarctic lakes are habituated to low light intensities (PAR ∼0.1–14% incident irradiance, see Section “Materials and Methods”), whereas a higher median PAR of ∼45% was determined for 39 different meltwater ponds on the McMurdo Ice Shelf during austral summer (Pridmore et al., 1995). Therefore, we hypothesize that the high light intensity during austral summer in the meltwater ponds results in the preferred synthesis of polyunsaturated membrane lipids. This is supported by previous experiments with Synechococcus sp. PCC7942 which have shown that an increase in unsaturation of fatty acids enhanced the tolerance to grow under strong light intensity (Gombos et al., 1997). Furthermore, a recent study investigating lipid distributions on a millimeter-scale in microbial mats from Octopus spring suggested that unsaturated IPLs form the first barrier against strong light exposure (Wörmer et al., 2020). However, additional research is needed to fully understand the correlation between light exposure and lipid membrane composition (Figure 8).
Temperature Controls on Heterocyte Glycolipid and Bacteriohopanepolyol Composition
As observed with the IPLs, the HGs revealed substantial differences between the microbial mats from the Antarctic environments (meltwater ponds and lakes) compared to the (sub)tropical and hot spring samples (Figure 6). In general, the latter had a higher diversity of HGs and extended carbon chains compared to the Antarctic settings. However, a unique feature of the microbial mats from Antarctica is the high abundance of HG keto-ols and keto-diols, whereas (sub)tropical and hot spring microbial mats almost exclusively contained HG diols and triols (Figure 6). Similar observations have been made in cultured cyanobacteria, in which the proportion of HG diols has been found to increase with growth temperature (Bauersachs et al., 2014). The HDI26, a HG based proxy to reconstruct surface water temperatures (SWT) in lacustrine environments (Bauersachs et al., 2015, 2021), revealed substantial variations between the Antarctic meltwater ponds (HDI26: 0.24 – 0.68, SWT: –6 – 11°C) and lakes (HDI26: 0.32 – 0.54, SWT: – 5 – 5°C) compared to the (sub)tropical mats (HDI26: 0.90 – 0.97, SWT: 21 – 23°C). HG distribution patterns and the HDI26 thus express a significant climate-driven component, which may compensate for varying rates of oxygen diffusion into the heterocyte with temperature variation and provide cyanobacteria with an anaerobic microenvironment to allow for biological N2 fixation (Bauersachs et al., 2009a).
The microbial mats from the McMurdo Ice Shelf showed substantial variations of the BHP distribution pattern in the different meltwater ponds, which complicated the comparison with samples from other environments (Figure 7). In general, the mats from the meltwater ponds revealed a high relative abundance of cyclitol ethers, while these compounds were below the detection limit in most of the samples from the Antarctic lakes and (sub)tropical environments. High quantities of cyclitol ethers have previously been detected in microbial mats collected from the Kongsfjord near Svalbard (Rethemeyer et al., 2010). It was suggested that these compounds are essential to protect bacteria to grow under microbial stress, such as a low pH or growth with antibiotics (Schmerk et al., 2015). However, cyclitol ethers are detected in a wide range of bacteria, which precludes a clear biological assignment of these lipids (Talbot et al., 2008; Van Winden et al., 2012; Schmerk et al., 2015).
Moreover, some noteworthy distribution patterns were observed in the microbial mats from Antarctic lakes and (sub)tropical environments. The microbial mats from Lake Vanda showed a high abundance of 2-Me-BHPs (30 – 35%). Such a high abundance of methylated BHPs was not detected in any other investigated environment. Based on genetic analysis of the radical S-adenosylmethionine protein that catalyzes the methylation of hopanoids at the C-position, a recent study suggested that 2-Me-BHPs in Lake Vanda are produced by cyanobacteria (Matys et al., 2019). Given that cyanobacteria dominated the microbial community in the McMurdo meltwater ponds and the low abundance of 2-Me-BHPs in these mats, this suggests that the synthesis of 2-Me-hopanoids is either dependent on the cyanobacterial taxon or particular environmental conditions. Moreover, we detected some BHPs in the Antarctic lakes and (sub)tropical environments that were absent from the meltwater ponds. BHpentol and 2-Me-BHpentol, which are considered biomarkers for cyanobacteria (Talbot et al., 2008), were detected in the mats from Lake Vanda, Yellowstone, and Highborne Cay, for instance. Moreover, 3-Me-BHT which has been suggested as a biomarker for methanotrophic bacteria (Zundel and Rohmer, 1985; Simonin et al., 1996) was detected in the mats from Lake Joyce, Lake Fryxell, Highborne Cay, and Hamelin Pool. This indicates that methanotrophic bacteria may be an important part of the microbial community in these samples.
Lastly, most of the samples investigated here came from shallow and highly productive environments which are expected to be fully saturated with O2. Samples from the Bratina meltwater ponds originated from waters that have previously been shown to be oversaturated (>625 μM) with respect to O2 (Wait et al., 2006). Moreover, Lake Vanda and Lake Joyce samples were collected in the O2 oversaturated upper part of the water column (Table 2). Thus, we did not observe trends in IPL composition that would have been influenced by O2 availability. On the other hand, mat samples from Lake Fryxell originated from an environment with a lower pO2 (Table 2), particularly the sample that was collected at 9.7 m depth (PAR 0.3%). This sample revealed a high relative abundance of BHT-II compared to the other investigated samples (Figure 7). BHT-II has been detected in marine anaerobic ammonium-oxidizing (anammox) bacteria (Rush et al., 2014), and freshwater purple non-sulfur bacteria (Neunlist et al., 1988) and it has been widely used to investigate oxygen gradients in the marine water column (e.g., Sáenz et al., 2011; Kharbush et al., 2013). Accordingly, the high abundance of BHT-II suggests the presence of an anaerobic bacterial community in the microbial mat from 9.7 m depth in Lake Vanda. This agrees with a recent study that showed a high abundance of genes indicative of anaerobic respiration capacity in microbial mats collected at a depth of 9.8 m in Lake Vanda (Dillon et al., 2020). However, we note that Rush et al. (2014) identified BHT-II only in marine and not freshwater anammox bacteria. Thus, salinity-stratified non-marine environments such as Lake Fryxell appear to also host bacterial sources of this unique BHP.
Conclusion
The microbial mat communities in twelve meltwater ponds from the McMurdo Ice Shelf were investigated for their IPL, HG, and BHP contents and compared with 16S and 18S rRNA gene community compositions. IPL distribution patterns dominated by glycolipids are in accordance with the predominance of cyanobacteria in these environments. Our results revealed a high abundance of phospholipids in microbial mats from the meltwater ponds, whereas aminolipids substitute these in P-limited systems. Comparison with microbial mats in perennially ice-covered Antarctic lakes, (sub)tropical, and hot spring environments revealed that the microbes in the meltwater ponds produce highly unsaturated lipids likely to increase the fluidity of the lipid membrane in cold environments and protect the inner cell against high levels of solar irradiance during austral summer. Similarly, HGs produced by N2-fixing heterocytous cyanobacteria show unusually high relative proportions of HG keto-ols and keto-diols compared to cyanobacterial mats from (sub)tropical and hot spring environments; likely an adaption at low temperatures to maintain the integrity of the gas diffusion barrier and limit the inflow of O2 into the heterocyte.
Data Availability Statement
The datasets presented in this study can be found in online repositories. The names of the repository/repositories and accession number(s) can be found in the article/Supplementary Material.
Author Contributions
RES, IH, and TWE designed the research. TJM, IH, and RES collected samples. TWE, MJK, ADJ, TB, and TJM performed the research. TWE, ADJ, JLM, TB, and HG analyzed the data. TWE and RES wrote the manuscript with contributions from all co-authors. All authors contributed to the article and approved the submitted version.
Funding
This study was funded by the Nasa Exobiology program (grant number: 80Nssc19K0465 awarded to RES). TWE was also funded by the Alexander von Humboldt-Gesellschaft through the Feodor-Lynen-Fellowship. The sampling campaign for microbial mats from Lake Fryxell was funded by the National Science Foundation Division of Polar Programs, the Mc Murdo Long Term Ecological Research project (grant number: 1115245). Lake Joyce sampling campaigns were funded by the Nasa Exobiology program (grant numbers: NNX08AO19G and NNX13AI60G). JLM was funded by a United Kingdom Natural Environmental Research Council Great Western 4+ Doctoral Training Partnership. The collection of the microbial mats from Lake Vanda was funded by the New Zealand Ministry of Business, Innovation, and Employment (grant number: UOWX1401).
Conflict of Interest
The authors declare that the research was conducted in the absence of any commercial or financial relationships that could be construed as a potential conflict of interest.
Publisher’s Note
All claims expressed in this article are solely those of the authors and do not necessarily represent those of their affiliated organizations, or those of the publisher, the editors and the reviewers. Any product that may be evaluated in this article, or claim that may be made by its manufacturer, is not guaranteed or endorsed by the publisher.
Acknowledgments
We gratefully thank Marc Schallenburg of the University of Otago for the conductivity measurements and his assistance during sample collection. We further acknowledge logistic support by Antarctica New Zealand and the United States Antarctic Program. Juliana Drozd provided invaluable laboratory assistance.
Supplementary Material
The Supplementary Material for this article can be found online at: https://www.frontiersin.org/articles/10.3389/fmicb.2022.903621/full#supplementary-material
References
Amaral-Zettler, L. A., McCliment, E. A., Ducklow, H. W., and Huse, S. M. (2009). A method for studying protistan diversity using massively parallel sequencing of V9 hypervariable regions of small-subunit ribosomal RNA genes. PLoS One 4:e6372. doi: 10.1371/journal.pone.0006372
Archer, S. D. J., McDonald, I. R., Herbold, C. W., Lee, C. K., and Cary, C. S. (2015). Benthic microbial communities of coastal terrestrial and ice shelf Antarctic meltwater ponds. Front. Microbiol. 6:485. doi: 10.3389/fmicb.2015.00485
Atkinson, M. J. (1987). Low phosphorus sediments in a hypersaline marine bay. Estuar. Coast. Shelf Sci. 24, 335–347.
Bale, N. J., Hopmans, E. C., Dorhout, D., Stal, L. J., Grego, M., van Bleijswijk, J., et al. (2018). A novel heterocyst glycolipid detected in a pelagic N2-fixing cyanobacterium of the genus Calothrix. Org. Geochem. 123, 44–47.
Bauersachs, T., Compaoré, J., Hopmans, E. C., Stal, L. J., Schouten, S., and Sinninghe Damsté, J. S. (2009a). Distribution of heterocyst glycolipids in cyanobacteria. Phytochemistry 70, 2034–2039.
Bauersachs, T., Hopmans, E. C., Compaoré, J., Stal, L. J., Schouten, S., and Sinninghe Damsté, J. S. (2009b). Rapid analysis of long-chain glycolipids in heterocystous cyanobacteria using high-performance liquid chromatography coupled to electrospray ionization tandem mass spectrometry. Rapid Commun. Mass Spectrom. 23, 1387–1394. doi: 10.1002/rcm.4009
Bauersachs, T., Compaoré, J., Severin, I., Hopmans, E. C., Schouten, S., Stal, L. J., et al. (2011). Diazotrophic microbial community of coastal microbial mats of the southern North Sea. Geobiology 9, 349–359. doi: 10.1111/j.1472-4669.2011.00280.x
Bauersachs, T., Rochelmeier, J., and Schwark, L. (2015). Seasonal lake surface water temperature trends reflected by heterocyst glycolipid-based molecular thermometers. Biogeosciences 12, 3741–3751.
Bauersachs, T., Russell, J. M., Evans, T. W., Schwalb, A., and Schwark, L. (2021). A heterocyte glycolipid-based calibration to reconstruct past continental climate change. Nat. Commun. 12, 1–11. doi: 10.1038/s41467-021-22739-3
Bauersachs, T., Stal, L. J., Grego, M., and Schwark, L. (2014). Temperature induced changes in the heterocyst glycolipid composition of N2 fixing heterocystous cyanobacteria. Org. Geochem. 69, 98–105.
Bauersachs, T., Talbot, H. M., Sidgwick, F., Sivonen, K., and Schwark, L. (2017). Lipid biomarker signatures as tracers for harmful cyanobacterial blooms in the Baltic Sea. PLoS One 12:e0186360. doi: 10.1371/journal.pone.0186360
Beal, C., Fonseca, F., and Corrieu, G. (2001). Resistance to freezing and frozen storage of Streptococcus thermophilus is related to membrane fatty acid composition. J. Dairy Sci. 84, 2347–2356. doi: 10.3168/jds.S0022-0302(01)74683-8
Bisseret, P., Zundel, M., and Rohmer, M. (1985). 2β-Methylhopanoids from Methylobacterium organophilum and Nostoc muscorum, a new series of prokaryotic triterpenoids. Eur. J. Biochem. 150, 29–34. doi: 10.1111/j.1432-1033.1985.tb08982.x
Bolyen, E., Rideout, J. R., Dillion, M. R., Bokulich, N. A., Abnet, C. C., Al-Ghalith, G. A., et al. (2019). Reproducible, interactive, scalable and extensible microbiome data science using QIIME 2. Nat. Biotechnol. 37, 852–857.
Bratina, B. J., Stevenson, B. S., Green, W. J., and Schmidt, T. M. (1998). Manganese reduction by microbes from oxic regions of the Lake Vanda (Antarctica) water column. Appl. Environ. Microbiol. 64, 3791–3797. doi: 10.1128/AEM.64.10.3791-3797.1998
Brocks, J. J., Jarrett, A. J. M., Sirantoine, E., Hallmann, C., Hoshino, Y., and Liyanage, T. (2017). The rise of algae in Cryogenian oceans and the emergence of animals. Nature 548, 578–581. doi: 10.1038/nature23457
Callahan, B. J., McMurdie, P. J., Rosen, M. J., Han, A. W., Johnson, A. J. A., and Holmes, S. P. (2016). DADA2: high-resolution sample inference from Illumina amplicon data. Nat. Methods 13, 581–583. doi: 10.1038/nmeth.3869
Caporaso, J. G., Lauber, C. L., Walters, W. A., Berg-Lyons, D., Huntley, J., Fierer, N., et al. (2012). Ultra-high-throughput microbial community analysis on the Illumina HiSeq and MiSeq platforms. ISME J. 6, 1621–1624. doi: 10.1038/ismej.2012.8
Caporaso, J. G., Lauber, C. L., Walters, W. A., Berg-Lyons, D., Lozupone, C. A., Turnbaugh, P. J., et al. (2011). Global patterns of 16S rRNA diversity at a depth of millions of sequences per sample. PNAS 108, 4516–4522. doi: 10.1073/pnas.1000080107
Corsetti, F. A., Olcott, A. N., and Bakermans, C. (2006). The biotic response to Neoproterozoic snowball Earth. Palaeogeogr. Palaeoclimatol. Palaeoecol. 232, 114–130.
Debenham, F. (1919). A new mode if transportation by ice: the raised marine muds of South Victoria Land (Antarctica). Q. J. Geol. Soc. 75, 51–76.
Dillon, M. L., Hawes, I., Jungblut, A. D., MacKey, T. J., Eisen, J. A., Doran, P. T., et al. (2020). Environmental control on the distribution of metabolic strategies of benthic microbial mats in Lake Fryxell, Antarctica. PLoS One 15:e0231053. doi: 10.1371/journal.pone.0231053
Dolhi, J. M., Teufel, A. G., Kong, W., and Morgan-Kiss, R. M. (2015). Diversity and spatial distribution of autotrophic communities within and between ice-covered Antarctic lakes (McMurdo Dry Valleys). Limnol. Ocean. 60, 977–991.
Doughty, D. M., Hunter, R. C., Summons, R. E., and Newman, D. K. (2009). 2-Methylhopanoids are maximally produced in akinetes of Nostoc punctiforme: Geobiological implications. Geobiology 7, 524–532. doi: 10.1111/j.1472-4669.2009.00217.x
Elling, F. J., Evans, T. W., Hemingway, J. D., Kharbush, J. J., Nathan, V., Bayer, B., et al. (2022). Marine and terrestrial nitrifying bacteria are sources of diverse bacteriohopanepolyols. Geobiology 22, 399–420. doi: 10.1111/gbi.12484
Elling, F. J., Hemingway, J. D., Evans, T. W., Kharbush, J. J., Spieck, E., Summons, R. E., et al. (2020). Vitamin B12-dependent biosynthesis ties amplified 2-methylhopanoid production during oceanic anoxic events to nitrification. PNAS 117, 32996–33004. doi: 10.1073/pnas.2012357117
Evans, T. W., Wörmer, L., Lever, M. A., Lipp, J. S., Lagostina, L., Lin, Y.-S., et al. (2017). Size and composition of subseafloor microbial community in the Benguela upwelling area examined from intact membrane lipid and DNA analysis. Org. Geochem. 111, 86–100.
Fairchild, I. J., Fleming, E. J., Bao, H., Benn, D. I., Boomer, I., Dublyansky, Y. V., et al. (2016). Continental carbonate facies of a Neoproterozoic panglaciation, north-east Svalbard. Sedimentology 63, 443–497.
Gambacorta, A., Trincone, A., Soriente, A., and Sodano, G. (1999). Chemistry of glycolipids from the heterocysts of nitrogen-fixing cyanobacteria. Curr. Top. Phytochem. 2, 145–150.
Geiger, O., González-Silva, N., López-Lara, I. M., and Sohlenkamp, C. (2010). Amino acid-containing membrane lipids in bacteria. Prog. Lipid Res. 49, 46–60. doi: 10.1016/j.plipres.2009.08.002
Geiger, O., López-Lara, I. M., and Sohlenkamp, C. (2013). Phosphatidylcholine biosynthesis and function in bacteria. Biochim. Biophys. Acta 1831, 503–513. doi: 10.1016/j.bbalip.2012.08.009
Glasser, N., Goodsell, B., Copland, L., and Lawson, W. (2006). Debris characteristics and ice-shelf dynamics in the ablation region of the McMurdo Ice Shelf, Antarctica. J. Glaciol. 52, 223–234.
Goldberg, I., and Eschar, L. (1977). Stability of lactic acid bacteria to freezing their fatty acid composition. Appl. Environ. Microbiol. 33, 489–496.
Goldfine, H., and Hagen, P.-O. (1968). N-methyl groups in bacterial lipids III. phospholipids of hypomicrobia. J. Bacteriol. 95, 367–375. doi: 10.1128/jb.95.2.367-375.1968
Gombos, Z., Kanervo, E., Tsetkova, N., Sakamoto, T., Aro, E.-M., and Murata, N. (1997). Genetic enhancement of the ability to tolerate photoinhibition by ontroduction of unsaturated bonds into membrane glycerolipids. Plant Physiol. 115, 551–559. doi: 10.1104/pp.115.2.551
Gombos, Z., Wada, H., and Murata, N. (1994). The recovery of photosynthesis from low-temperature photoinhibition is accelerated by the unsaturation of membrane lipids: a mechanism of chilling tolerance. PNAS 91, 8787–8791. doi: 10.1073/pnas.91.19.8787
Greco, C., Andersen, D. T., Hawes, I., Bowles, A. M. C., Yallop, M. L., Barker, G., et al. (2020). Microbial diversity of pinnacle and conical microbial mats in the perennially ice-covered lake untersee, East Antarctica. Front. Microbiol. 11:607251. doi: 10.3389/fmicb.2020.607251
Hawes, I., Howard-Williams, C., and Pridmore, R. D. (1993). Environmental control of microbial biomass in the ponds of the McMurdo Ice Shelf, Antarctica. Arch. Hydrobiol. 127, 271–287.
Hawes, I., Howard-Williams, C., and Sorrell, B. (2014). Decadal timescale variability in ecosystem properties in the ponds of the McMurdo Ice Shelf, southern Victoria Land, Antarctica. Antarct. Sci. 26, 219–230.
Hawes, I., Jungblut, A. D., Matys, E. D., and Summons, R. E. (2018). The “Dirty Ice” of the McMurdo Ice Shelf: analogues for biological oases during the Cryogenian. Geobiology 16, 369–377. doi: 10.1111/gbi.12280
Hawes, I., Safi, K., Sorrell, B., Webster-Brown, J., and Arscott, D. (2011). Summer – winter transitions in Antarctic ponds I: the physical environment. Antarct. Sci. 23, 235–242.
Hoffman, P. F. (2016). Cryoconite pans on Snowball Earth: supraglacial oases for Cryogenian eukaryotes? Geobiology 14, 531–542. doi: 10.1111/gbi.12191
Hoffman, P. F., Abbot, D. S., Ashkenazy, Y., Benn, D. I., Brocks, J. J., Cohen, P. A., et al. (2017). Snowball Earth climate dynamics and Cryogenian geology-geobiology. Sci. Adv. 3, e1600983. doi: 10.1126/sciadv.1600983
Hoffman, P. F., Kaufman, A. J., Halverson, G. P., and Schrag, D. P. (1998). A Neoproterozoic snowball earth. Science 281, 1342–1346.
Howard-Williams, C., and Hawes, I. (2007). Ecological processes in Antarctic inland waters: interactions between physical processes and the nitrogen cycle. Antarct. Sci. 19, 205–217.
Howard-Williams, C., Pridmore, R. D., Broady, P. A., and Vincent, W. F. (1990). “Environmental and biological variability in the McMurdo Ice Shelf ecosystem,” in Antarctic Ecosystems, eds K. R. Kerry and G. Hempel (Berlin: Springer), 23–31.
Jabro, N. B. (2008). Microcosm Studies of Nutrient Cycling in Bahamian Stromatolites. College Park, MD: University of Maryland.
Jackson, E. E., Hawes, I., and Jungblut, A. D. (2021). 16S rRNA gene and 18S rRNA gene diversity in microbial mat communities in meltwater ponds on the McMurdo Ice Shelf, Antarctica. Polar Biol. 44, 823–836.
Johnson, B. W., Poulton, S. W., and Goldblatt, C. (2017). Marine oxygen production and open water supported an active nitrogen cycle during the Marinoan Snowball Earth. Nat. Commun. 8, 1–10. doi: 10.1038/s41467-017-01453-z
Jungblut, A. D., Allen, M. A., Burns, B. P., and Neilan, B. A. (2009). Lipid biomarker analysis of cyanobacteria-dominated microbial mats in meltwater ponds on the McMurdo Ice Shelf, Antarctica. Org. Geochem. 40, 258–269.
Jungblut, A. D., Hawes, I., Mackey, T. J., Krusor, M., Doran, P. T., Sumner, D. Y., et al. (2016). Microbial mat communities along an oxygen gradient in a perennially ice-covered Antarctic lake. Appl. Environ. Microbiol. 82, 620–630. doi: 10.1128/AEM.02699-15
Jungblut, A. D., Hawes, I., Mountfort, D., Hitzfeld, B., Dietrich, D. R., Burns, B. P., et al. (2005). Diversity within cyanobacterial mat communities in variable salinity meltwater ponds of McMurdo Ice Shelf, Antarctica. Environ. Microbiol. 7, 519–529. doi: 10.1111/j.1462-2920.2005.00717.x
Jungblut, A. D., and Neilan, B. A. (2010). “Cyanobacterial mats of the meltwater ponds on the McMurdo Ice Shelf,” in Microbial Mats, eds J. Seckbach and A. Oren (Dordrecht: Springer), 499–514.
Jungblut, A. D., Vincent, W. F., and Lovejoy, C. (2012). Eukaryotes in Arctic and Antarctic cyanobacterial mats. FEMS Microbiol. Ecol. 82, 416–428. doi: 10.1111/j.1574-6941.2012.01418.x
Kato, M., Sakai, M., Adachi, K., Ikemoto, H., and Sano, H. (1996). Distribution of betaine lipids in marine algae. Phytochemistry 42, 1341–1345.
Khan, N., Tuffin, M., Stafford, W., Cary, C., Lacap, D. C., Pointing, S. B., et al. (2011). Hypolithic microbial communities of quartz rocks from Miers Valley, McMurdo Dry Valleys, Antarctica. Polar Biol. 34, 1657–1668.
Kharbush, J. J., Ugalde, J. A., Hogle, S. L., Allen, E. E., and Aluwihare, L. I. (2013). Composite bacterial hopanoids and their microbial producers across oxygen gradients in the water column of the California current. Appl. Environ. Microbiol. 79, 7491–7501. doi: 10.1128/AEM.02367-13
Kulkarni, G., Wu, C.-H., and Newman, D. K. (2013). The general stress response factor EcfG regulates expression of the C-2 hopanoid methylase HpnP in Rhodopseudomonas palustris TIE-1. J. Bacteriol. 195, 2490–2498. doi: 10.1128/JB.00186-13
Lechte, M. A., Wallace, M. W., van Smeerdijk Hood, A., Li, W., Jiang, G., Halverson, G. P., et al. (2019). Subglacial meltwater supported aerobic marine habitats during Snowball Earth. PNAS 116, 25478–25483. doi: 10.1073/pnas.1909165116
Lezcano, Á, Sánchez-García, L., Quesada, A., Carrizo, D., Fernández-Martínez, M. Á, Cavalcante-Silva, E., et al. (2022). Comprehensive metabolic and taxonomic reconstruction of an ancient microbial mat from the McMurdo Ice Shelf (Antarctica) by integrating genetic, metaproteomic and lipid biomarker analyses. Front. Microbiol 13:799360. doi: 10.3389/fmicb.2022.799360
Macdonald, F. A., Schmitz, M. D., Strauss, J. V., Halverson, G. P., Gibson, T. M., Eyster, A., et al. (2018). Cryogenian of Yukon. Precambrian Res. 319, 114–143.
Mackey, T., Sumner, D. Y., Hawes, I., Leidman, S. Z., Andersen, D. T., and Jungblut, A. D. (2018). Stromatolite records of environmental change in perennially ice-covered Lake Joyce, McMurdo Dry Valleys, Antarctica. Biogeochemistry 137, 73–92.
Mackey, T. J., Sumner, D. Y., Hawes, I., Jungblut, A. D., Lawrence, J., Leidman, S., et al. (2017b). Increased mud deposition reduces stromatolite complexity. Geology 45, 663–666.
Mackey, T. J., Sumner, D. Y., Hawes, I., and Jungblut, A. D. (2017a). Morphological signatures of microbial activity across sediment and light microenvironments of Lake Vanda, Antarctica. Sediment. Geol. 361, 82–92.
Mackey, T. J., Sumner, D. Y., Hawes, I., Jungblut, A. D., and Andersen, D. T. (2015). Growth of modern branched columnar stromatolites in Lake Joyce, Antarctica. Geobiology 13, 373–390. doi: 10.1111/gbi.12138
Marr, A. G., and Ingraham, J. L. (1962). Effect of temperature on the composition of fatty acids in Escherichia coli. J. Bacteriol. 84, 1260–1267.
Matys, E. D., Mackey, T., Grettenberger, C., Mueller, E., Sumner, D. Y., Hawes, I., et al. (2019). Bacteriohopanepolyols across environmental gradients in Lake Vanda, Antarctica. Geobiology 17, 308–319. doi: 10.1111/gbi.12335
Matys, E. D., Sepúlveda, J., Pantoja, S., Lange, C. B., Caniupán, M., Lamy, F., et al. (2017). Bacteriohopanepolyols along redox gradients in the Humboldt Current System off northern Chile. Geobiology 15, 844–857. doi: 10.1111/gbi.12250
Moczydłowska, M. (2008). The Ediacaran microbiota and the survival of snowball Earth conditions. Precambrian Res. 167, 1–15.
Möke, F., Wasmund, N., Bauwe, H., and Hagemann, M. (2013). Salt acclimation of Nodularia spumigena CCY9414 — a cyanobacterium adapted to brackish water. Aquat. Microb. Ecol. 70, 207–214.
Murata, N., and Los, D. A. (1997). Membrane fluidity and temperature perception. Plant Physiol. 115, 875–879. doi: 10.1104/pp.115.3.875
Neunlist, S., Bisseret, P., and Rohmer, M. (1988). The hopanoids of the purple non-sulfur bacteria Rhodopseudomonas palustris and Rhodopseudomonas acidophila and the absolute configuration of bacteriohopanetetrol. Eur. J. Biochem. 171, 245–252. doi: 10.1111/j.1432-1033.1988.tb13783.x
Parada, A. E., Needham, D. M., and Fuhrman, J. A. (2016). Every base matters: assessing small subunit rRNA primers for marine microbiomes with mock communities, time series and global field samples. Environ. Microbiol. 18, 1403–1414. doi: 10.1111/1462-2920.13023
Pridmore, R. D., Vant, W. N., and Cummings, V. J. (1995). Factors affecting the water clarity of ponds on the McMurdo Ice Shelf, Antarctica. Antarct. Sci. 7, 145–148.
Priscu, J. C., Vincent, W. F., and Howard-Williams, C. (1989). Inorganic nitrogen uptake and regeneration in perennially ice-covered Lakes Fryxell and Vanda, Antarctica. J. Plankton Res. 11, 335–351.
Quast, C., Pruesse, E., Yilmaz, P., Gerken, J., Schweer, T., Yarza, P., et al. (2013). The SILVA ribosomal RNA gene database project: improved data processing and web-based tools. Nucleic Acids Res. 41, D590–D596. doi: 10.1093/nar/gks1219
R Core Team (2021). A Language and Environment for Statistical Computing. Vienna: R Foundation for Statistical Computing.
Raetz, C. R. H. (1986). Molecular genetics of membrane phospholipid synthesis. Annu. Rev. Genet. 20, 253–291. doi: 10.1146/annurev.ge.20.120186.001345
Rashby, S. E., Sessions, A. L., Summons, R. E., and Newman, D. K. (2007). Biosynthesis of 2-methylbacteriohopanepolyols by an anoxygenic phototroph. PNAS 104, 15099–15104. doi: 10.1073/pnas.0704912104
Rethemeyer, J., Schubotz, F., Talbot, H. M., Cooke, M. P., Hinrichs, K.-U., and Mollenhauer, G. (2010). Distribution of polar membrane lipids in permafrost soils and sediments of a small high Arctic catchment. Org. Geochem. 41, 1130–1145.
Rezanka, T., Viden, I., Go, J. V., Dor, I., and Dembitsky, V. M. (2003). Polar lipids and fatty acids of three wild cyanobacterial strains of the genus Chroococcidiopsis. Folia Microbiol. 48, 781–786. doi: 10.1007/BF02931514
Ricci, J. N., Michel, A. J., and Newman, D. K. (2015). Phylogenetic analysis of HpnP reveals the origin of 2-methylhopanoid production in Alphaproteobacteria. Geobiology 13, 267–277. doi: 10.1111/gbi.12129
Ricci, J. N., Morton, R., Kulkarni, G., Summers, M. L., and Newman, D. K. (2017). Hopanoids play a role in stress tolerance and nutrient storage in the cyanobacterium Nostoc punctiforme. Geobiology 15, 173–183. doi: 10.1111/gbi.12204
Rippka, R., Deruelles, J., Waterburry, J. B., Herdman, M., and Stanier, R. Y. (1979). Generic assignments, strain histories and properties of pure cultures of cyanobacteria. Microbiology 111, 1–61.
Rooney, A. D., Strauss, J. V., Brandon, A. D., and Macdonald, F. A. (2015). A Cryogenian chronology: two long-lasting synchronous Neoproterozoic glaciations. Geology 43, 459–462.
Rush, D., Sinninghe Damsté, J. S., Poulton, S. W., Thamdrup, B., Garside, A. L., Gonzalez, J. A., et al. (2014). Anaerobic ammonium-oxidising bacteria: a biological source of the bacteriohopanetetrol stereoisomer in marine sediments. Geochim. Cosmochim. Acta 140, 50–64.
Rütters, H., Sass, H., Cypionka, H., and Rullkötter, J. (2002). Phospholipid analysis as a tool to study complex microbial communities in marine sediments. J. Microbiol. Methods 48, 149–160. doi: 10.1016/s0167-7012(01)00319-0
Ruvindy, R., White, I. I. I. R. A., Neilan, B. A., and Burns, B. P. (2015). Unravelling core microbial metabolisms in the hypersaline microbial mats of Shark Bay using high-throughput metagenomics. ISME J. 10, 183–196. doi: 10.1038/ismej.2015.87
Sáenz, J. P., Wakeham, S. G., Eglinton, T. I., and Summons, R. E. (2011). New constraints on the provenance of hopanoids in the marine geologic record: bacteriohopanepolyols in marine suboxic and anoxic environments. Org. Geochem. 42, 1351–1362.
Schmerk, C. L., Welander, P. V., Hamad, M. A., Bain, K. L., Bernards, M. A., Summons, R. E., et al. (2015). Elucidation of the Burkholderia cenocepacia hopanoid biosynthesis pathway uncovers functions for conserved proteins in hopanoid-producing bacteria. Environ. Microbiol. 17, 735–750. doi: 10.1111/1462-2920.12509
Schouten, S., Villareal, T. A., Hopmans, E. C., Mets, A., Swanson, K. M., and Sinninghe Damsté, J. S. (2013). Endosymbiotic heterocystous cyanobacteria synthesize different heterocyst glycolipids than free-living heterocystous cyanobacteria. Phytochemistry 85, 115–121. doi: 10.1016/j.phytochem.2012.09.002
Schubotz, F. (2019). “Membrane homeostasis upon nutrient (C, N, P) limitation,” in Biogenesis of Fatty Acids, Lipids and Membranes. Handbook of Hydrocarbon and Lipid Microbiology, ed. O. Geiger (Cham: Springer), 823–847.
Shacat, J. A., Green, W. J., Decarlo, E. H., and Newell, S. (2004). The geochemistry of Lake Joyce, McMurdo Dry Valleys, Antarctica. Aquat. Geochem. 10, 325–352.
Simonin, P., Jürgens, U. J., and Rohmer, M. (1996). Bacterial triterpenoids of the hopane series from the prochlorophyte Prochlorothrix hollandica and their intracellular localization. Eur. J. Biochem. 241, 865–871. doi: 10.1111/j.1432-1033.1996.00865.x
Sjöling, S., and Cowan, D. A. (2003). High 16S rDNA bacterial diversity in glacial meltwater lake sediment, Bratina Island, Antarctica. Extremophiles 7, 275–282. doi: 10.1007/s00792-003-0321-z
Sohlenkamp, C., López-Lara, I. M., and Geiger, O. (2003). Biosynthesis of phosphatidylcholine in bacteria. Prog. Lipid Res. 42, 115–162.
Sorrell, B. K., Hawes, I., and Safi, K. (2013). Nitrogen and carbon limitation of planktonic primary production and phytoplankton-bacterioplankton coupling in ponds on the McMurdo Ice Shelf, Antarctica. Environ. Res. Lett. 8:035043.
Stal, L. J., van Gemerden, H., and Krumbein, W. E. (1985). Structure and development of a benthic marine microbial mat. FEMS Microbiol. Lett. 1, 111–125.
Sturt, H. F., Summons, R. E., Smith, K., Elvert, M., and Hinrichs, K.-U. (2004). Intact polar membrane lipids in prokaryotes and sediments deciphered by high-performance liquid chromatography/electrospray ionization multistage mass spectrometry—new biomarkers for biogeochemistry and microbial ecology. Rapid Commun. Mass Spectrom. 18, 617–628. doi: 10.1002/rcm.1378
Summons, R. E., Jahnke, L. L., Hope, J. M., and Logan, G. A. (1999). 2-Methylhopanoids as biomarkers for cyanobacterial oxygenic photosynthesis. Nature 400, 554–557. doi: 10.1038/23005
Suutari, M., Liukkonen, K., and Laakso, S. (1990). Ion permeability of the cytoplasmic membrane limits the maximum growth temperature of bacteria and archaea. Microbiology 136, 1469–1474. doi: 10.1111/j.1365-2958.1995.18050925.x
Talbot, H. M., Rohmer, M., and Farrimond, P. (2007). Rapid structural elucidation of composite bacterial hopanoids by atmospheric pressure chemical ionisation liquid chromatography/ion trap mass spectrometry. Rapid Commun. Mass Spectrom. 21, 880–892. doi: 10.1002/rcm.2911
Talbot, H. M., Summons, R., Jahnke, L., and Farrimond, P. (2003). Characteristic fragmentation of bacteriohopanepolyols during atmospheric pressure chemical ionisation liquid chromatography/ion trap mass spectrometry. Rapid Commun. Mass Spectrom. 17, 2788–2796. doi: 10.1002/rcm.1265
Talbot, H. M., Summons, R. E., Jahnke, L. L., Cockell, C. S., Rohmer, M., and Farrimond, P. (2008). Cyanobacterial bacteriohopanepolyol signatures from cultures and natural environmental settings. Org. Geochem. 39, 232–263.
Van de Vossenberg, J. L. C. M., Ubbink-Kok, T., Elferink, M. G. L., Driessen, A. J. M., and Konings, W. N. (1995). Ion permeability of the cytoplasmic membrane limits the maximum growth temperature of bacteria and archaea. Mol. Microbiol. 18, 925–932. doi: 10.1111/j.1365-2958.1995.18050925.x
Van Winden, J. F., Talbot, H. M., Kip, N., Reichart, G.-J., Pol, A., McNamara, N. P., et al. (2012). Bacteriohopanepolyol signatures as markers for methanotrophic bacteria in peat moss. Geochim. Cosmochim. Acta 77, 52–61.
Varin, T., Lovejoy, C., Jungblut, A. D., Vincent, W. F., and Corbeil, J. (2012). Metagenomic analysis of stress genes in microbial mat communities from Antarctica and the high Arctic. Appl. Environ. Microbiol. 78, 549–559. doi: 10.1128/AEM.06354-11
Vincent, W. F., and Quesada, A. (1994). “Ultraviolet radiation effects on cyanobacteria: implications for Antarctic microbial ecosystems,” in Ultraviolet Radiation in Antarctica: Measurments and Biological Effects, eds C. S. Weiler and P. A. Penhale (Hoboken, NJ: John Wiley & Sons), 111–124.
Wada, H., and Murata, N. (1998). “Membrane lipids in cyanobacteria,” in Lipids in Photosynthesis: Structure, Function and Genetics, eds S. Paul-André and M. Norio (Dordrecht: Springer), 65–81.
Wait, B. R., Webster-Brown, J. G., Brown, K. L., Healy, M., and Hawes, I. (2006). PChemistry and stratification of Antarctic meltwater ponds I: coastal ponds near Bratina Island, McMurdo Ice Shelf. Antarct. Sci. 18, 515–524.
Wörmer, L., Cires, S., Velazquez, D., Quesada, A., and Hinrichs, K.-U. (2012). Cyanobacterial heterocyst glycolipids in cultures and environmental samples: diversity and biomarker potential. Limnol. Ocean. 57, 1775–1788.
Wörmer, L., Gajendra, N., Schubotz, F., Matys, E. D., Evans, T. W., and Summons, R. E. (2020). A micrometer-scale snapshot on phototroph spatial distributions: mass spectrometry imaging of microbial mats in Octopus Spring, Yellowstone National Park. Geobiology 18, 742–759. doi: 10.1111/gbi.12411
Wörmer, L., Lipp, J. S., Schröder, J. M., and Hinrichs, K.-U. (2013). Application of two new LC–ESI–MS methods for improved detection of intact polar lipids (IPLs) in environmental samples. Org. Geochem. 59, 10–21.
Yilmaz, P., Parfrey, L. W., Yarza, P., Gerken, J., Pruesse, E., Quast, C., et al. (2014). The SILVA and “all-species Living Tree Project (LTP)” taxonomic frameworks. Nucleic Acids Res. 42, D643–D648. doi: 10.1093/nar/gkt1209
Keywords: cyanobacteria, Antarctica, intact polar lipid, heterocyte glycolipids, bacteriohopanepolyol, microbial mats, homeoviscous adaptation
Citation: Evans TW, Kalambokidis MJ, Jungblut AD, Millar JL, Bauersachs T, Grotheer H, Mackey TJ, Hawes I and Summons RE (2022) Lipid Biomarkers From Microbial Mats on the McMurdo Ice Shelf, Antarctica: Signatures for Life in the Cryosphere. Front. Microbiol. 13:903621. doi: 10.3389/fmicb.2022.903621
Received: 24 March 2022; Accepted: 11 May 2022;
Published: 10 June 2022.
Edited by:
Philippe M. Oger, UMR 5240 Microbiologie, Adaptation et Pathogénie (MAP), FranceReviewed by:
François Joseph Guyot, Muséum National d’Histoire Naturelle, FranceGuillemette Ménot, Université de Lyon, France
Copyright © 2022 Evans, Kalambokidis, Jungblut, Millar, Bauersachs, Grotheer, Mackey, Hawes and Summons. This is an open-access article distributed under the terms of the Creative Commons Attribution License (CC BY). The use, distribution or reproduction in other forums is permitted, provided the original author(s) and the copyright owner(s) are credited and that the original publication in this journal is cited, in accordance with accepted academic practice. No use, distribution or reproduction is permitted which does not comply with these terms.
*Correspondence: Roger E. Summons, cnN1bW1vbnNAbWl0LmVkdQ==; Thomas W. Evans, dGV2YW5zQG1hcnVtLmRl
†Present addresses: Thomas W. Evans, MARUM Center for Marine Environmental Sciences, Department of Geosciences, University of Bremen, Bremen, Germany; Maria J. Kalambokidis Department of Ecology, Evolution and Behavior, University of Minnesota, St. Paul, MN, United States; Tyler J. Mackey, Department of Earth and Planetary Sciences, The University of New Mexico, Albuquerque, NM, United States