- 1Center for Electromicrobiology, Department of Biology, Aarhus University, Aarhus, Denmark
- 2X-LAB, UHasselt, Diepenbeek, Belgium
The global production of unrecycled electronic waste is extensively growing each year, urging the search for alternatives in biodegradable electronic materials. Electroactive bacteria and their nanowires have emerged as a new route toward electronic biological materials (e-biologics). Recent studies on electron transport in cable bacteria—filamentous, multicellular electroactive bacteria—showed centimeter long electron transport in an organized conductive fiber structure with high conductivities and remarkable intrinsic electrical properties. In this work we give a brief overview of the recent advances in biodegradable electronics with a focus on the use of biomaterials and electroactive bacteria, and with special attention for cable bacteria. We investigate the potential of cable bacteria in this field, as we compare the intrinsic electrical properties of cable bacteria to organic and inorganic electronic materials. Based on their intrinsic electrical properties, we show cable bacteria filaments to have great potential as for instance interconnects and transistor channels in a new generation of bioelectronics. Together with other biomaterials and electroactive bacteria they open electrifying routes toward a new generation of biodegradable electronics.
Introduction: E-Waste as a Global Problem
The use of electronic devices is creating the world’s fastest-growing waste-stream, with currently 50 million tons of e-waste produced each year (Ryder and Zhao Houlin, 2019). This not only causes $62.5 billion in material value of resources in our spent devices to be dumped into landfill (Ryder and Zhao Houlin, 2019), but exposure to this e-waste has a plethora of negative effects on humans, even in utero (Grant et al., 2013). Thus, electronic waste forms a substantial problem for environmental and human wellbeing, for which alternatives should be found on a short term (Kang et al., 2020). Biodegradable electronics is a new field that aims to replace the harmful non-durable materials used in electronics with biodegradable alternatives. In this review we will discuss the recent advances of using biomaterials for biodegradable electronics and the possible role of electroactive bacteria and in particular cable bacteria, of which an overview is given in Figure 1.
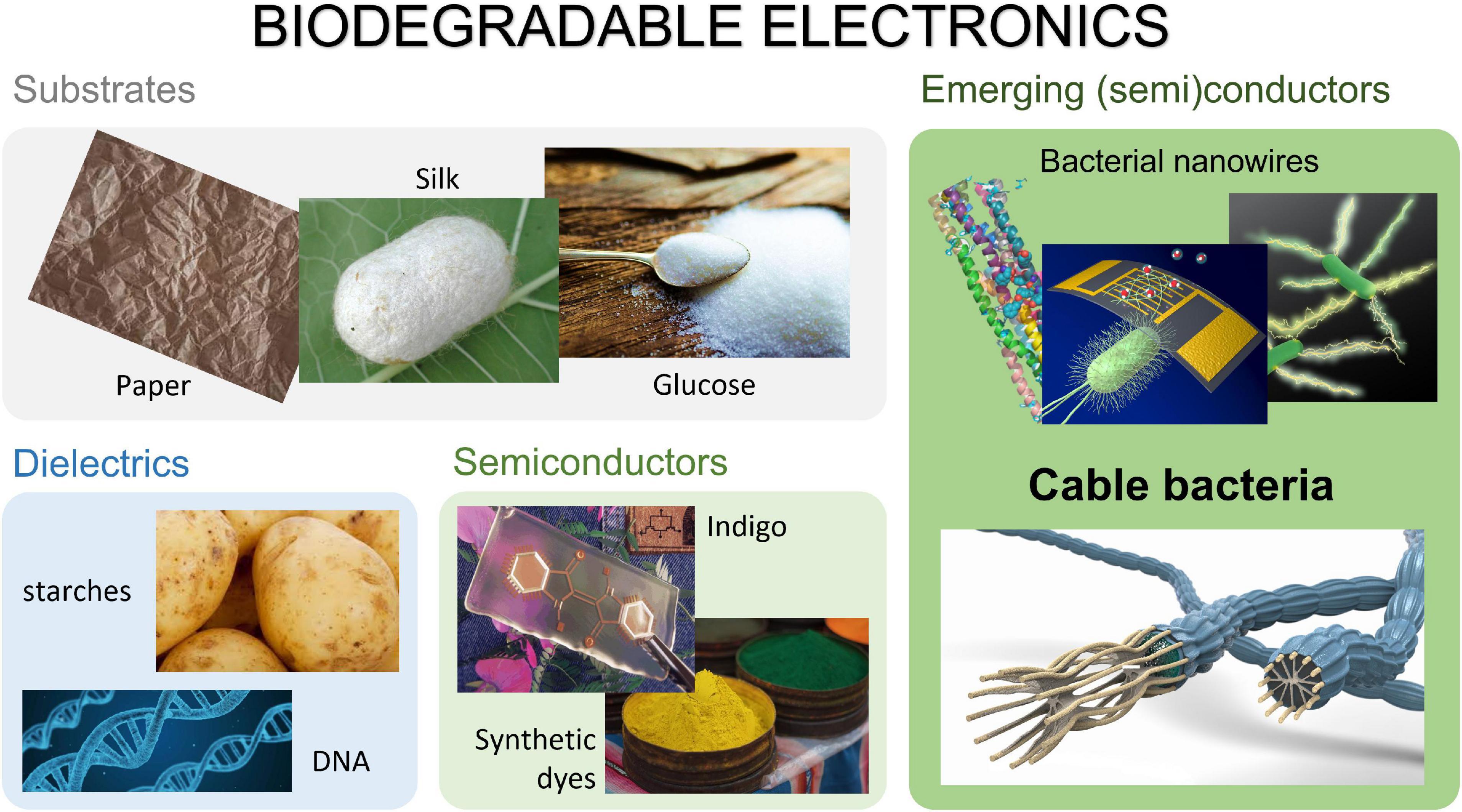
Figure 1. Several biomaterials that have potential in biodegradable electronics, electroactive bacteria and in particular cable bacteria are interesting upcoming candidates.
Advances in Biodegradable Electronics
Biodegradability might seem like a broad term since all materials will be degraded when left in nature after enough time. We will focus on so-called transient electronics: materials that have limited lifetimes before they disappear with the ultimate goal being bioresorption: the complete degradation of a device when immersed in biofluids. The kinetics of biodegradable materials depend on their chemical and morphological properties and the environmental conditions, such as temperature and pH and ionic content when in a solution (Kang et al., 2014). The lifetime of these devices can be accurately controlled by encapsulating the device with biodegradable material or by actively triggering the degradation reaction (Hwang et al., 2012; Kang et al., 2014, 2020). The number of defects through which water/vapor—which degrade the device—could leak can be tuned multiple ways, for example, by using multiple layers (Kang et al., 2014). Another option is doping materials to hinder their electrolysis (Seidel et al., 1990).
Biodegradable electronics can be found among inorganic, organic, and biological materials. Transience by bioresorption has for example been simulated for (semi-) conductors such as Silicon (Si), Germanium (Ge), SiGe and amorphous indium gallium zinc oxide (a-IGZO) in aqueous media. Some proposed non-biological transient dielectric materials include metal or semiconductor oxides and nitrides such as MgO, SiO2, and SiNx (Hwang et al., 2012). For example, the silicon and SiO2 undergo hydrolysis to form either harmless Si(OH)4 and H2, or Si(OH)4 and water (Seidel et al., 1990). This is a surface level effect, which allows for silicon’s use as encapsulation layer. Nevertheless, layers of SiO2 have defects through which water can permeate rapidly (<10 min), making it ineffective for such use (Lee et al., 2017). In this paper the focus will be on biodegradable materials originating from nature. They have the advantage that high-scale low-cost production is possible (Kang et al., 2020), because they are often solution based and allow low-temperature processing steps (Stadler et al., 2007). Furthermore, they have a high chemical compatibility with many other materials, and their properties can be tuned for their lifetime and morphology (Kang et al., 2020). Besides, organic monolayers can be combined with metals and plastics to create flexible electronics (Sekitani et al., 2009).
For biological biodegradable semiconductors, we will focus on materials that have proven conduction at least in the micrometer range, thereby excluding possible candidates like single peptides/proteins (Ing et al., 2018) and DNA (Beratan, 2019). A first interesting group of biological semiconductors are naturally occurring molecules such as indigo, which originates from several species of plants (Zollinger, 2003), but nowadays has become the most mass-produced industrial dye (Glowacki et al., 2011). These are planar molecules, which are connected through hydrogen bonding, resulting in tight π-stacking between neighboring molecules (Irimia-Vladu et al., 2012). This means that the orbitals which are not used in the intermolecular binding of the elements overlap and form conductive pathways. Despite having a high melting point, and low solubility, indigo can be degraded by enzymes (Campos et al., 2001), bacteria (Valdez-Vazquez et al., 2020), oxidizing agents (Prado et al., 2008) and light (in water) in timeframes down to a single day (Vautier et al., 2001), with a reported rate of 0.90 μmol L–1 min–1 (Vautier et al., 2001). In donor-acceptor solar cells, indigo acts like a good acceptor material (Glowacki et al., 2011). Another naturally occurring semiconductor is the pigment β-carotene, which can act as an electron donor in e.g., dye sensitized solar cells (Glowacki et al., 2011). However, this material is highly soluble in organic solvents, which makes certain measurements such as cyclic voltammetry challenging (Glowacki et al., 2011). Once dissolved it degrades with a reaction rate of 0.621 h–1 (Chen et al., 2014). The photo-to-electric conversion efficiency is very low when used in a dye sensitized solar cell (Suryana et al., 2013a). Eumelanins are a subclass of melanin which is found in skin pigment, which are natural semiconductors (Feig et al., 2018). Upon the absorption of water, a reaction occurs where free electrons and protons are released, self-doping the eumelanins. In 8 days, in vivo melanin implants can be nearly completely eroded and resorbed (Feig et al., 2018).
Instead of just considering naturally occurring biomolecules, it is interesting to look at their derivates to obtain organic semiconductors which are based on naturally occurring molecules as is reviewed by Glowacki et al. (2011). For example, indanthrene yellow G and indanthrene brilliant orange RK, are man-made pigments used in dyes that act as wide-bandgap semiconductors with high electron affinity. Other pigments can be derived from different sources to function as p-type electron donors like Quinacridone and Cibalakrot, with a larger bandgap (Glowacki et al., 2011). In 115 days, the conductivity of the former degraded to 80% of its original value (Daniel Głowacki et al., 2013). Gelatin is a conductive polymer obtained from collagen, of which the conductivity can be increased even further by combining it with conducting polymers such as Polyaniline (PANI) (Harrington and von Hippel, 1962; Li et al., 2006). Other biodegradable semiconductors which are neither natural nor nature-inspired include Poly(p-phenylene vinylene) (PPV), ambipolar polyselenophene, Buckminster fullerene, pentacene, and 5,50-bis-(7-dodecyl-9H-fluoren-2-yl)-2,20-bithiophene (DDFTTF).
Next to the biodegradable (semi) conducting materials, there is a much larger variety in isolating biomaterials that can be used as underlying substrates for the electronic circuitry, as described in Irimia-vladu et al. (2010b). The cheapest yet very familiar biodegradable substrate is paper. Despite its roughness, low-voltage active circuits can be realized on top of it using gravure and flexographic printing techniques. Hard gelatin capsules and caramelized glucose can be ingested and therefore used in bio-metabolizable electronics (Irimia-vladu et al., 2010b). Caramelized glucose is sensitive to moisture but has a smoothness close to that of glass. Poly(L-lactide-co-glycolide) (PLGA) are other sugar-based biopolymers obtained from pure L lactide, which can be used as substrate (Bettinger and Bao, 2010; Chanfreau et al., 2010). Though water can permeate through PLGA, which causes rapid failure (< 10 min) when they are used as encapsulation layer (Lee et al., 2017). Another familiar substrate is silk, a polypeptide polymer made up of fibroin and sericin, which have interchain hydrogen bonds, leading to the mechanical robustness of the silk fibers (Irimia-Vladu et al., 2012). Silk elicits no immune response and it safely dissolves and resorbs completely, making it safe to be implanted into the body. Furthermore, silk can be engineered to degrade under the desired conditions (Wang et al., 2008; Hu et al., 2011; Irimia-Vladu et al., 2012). A less common natural polymeric material produced by insects is shellac, a natural polyester copolymer, which can also be synthetically produced in multiple different grades and shades that can easily be cast to produce substrate foils (Irimia-Vladu et al., 2012). Of the mentioned substrates, glucose silk and shellac have very good surface smoothness. However, in general, biodegradable substrates need a smoothing layer to allow the fabrication of electronic components on top of them (Irimia-vladu et al., 2010b). An example of a material used for such a layer is polydimethylsiloxane (PDMS), which binds specific regions of the circuit to the substrate and provides strain isolation (Kim et al., 2009). The substrates described here are summarized with their dielectric constants and breakdown fields in Table 1.
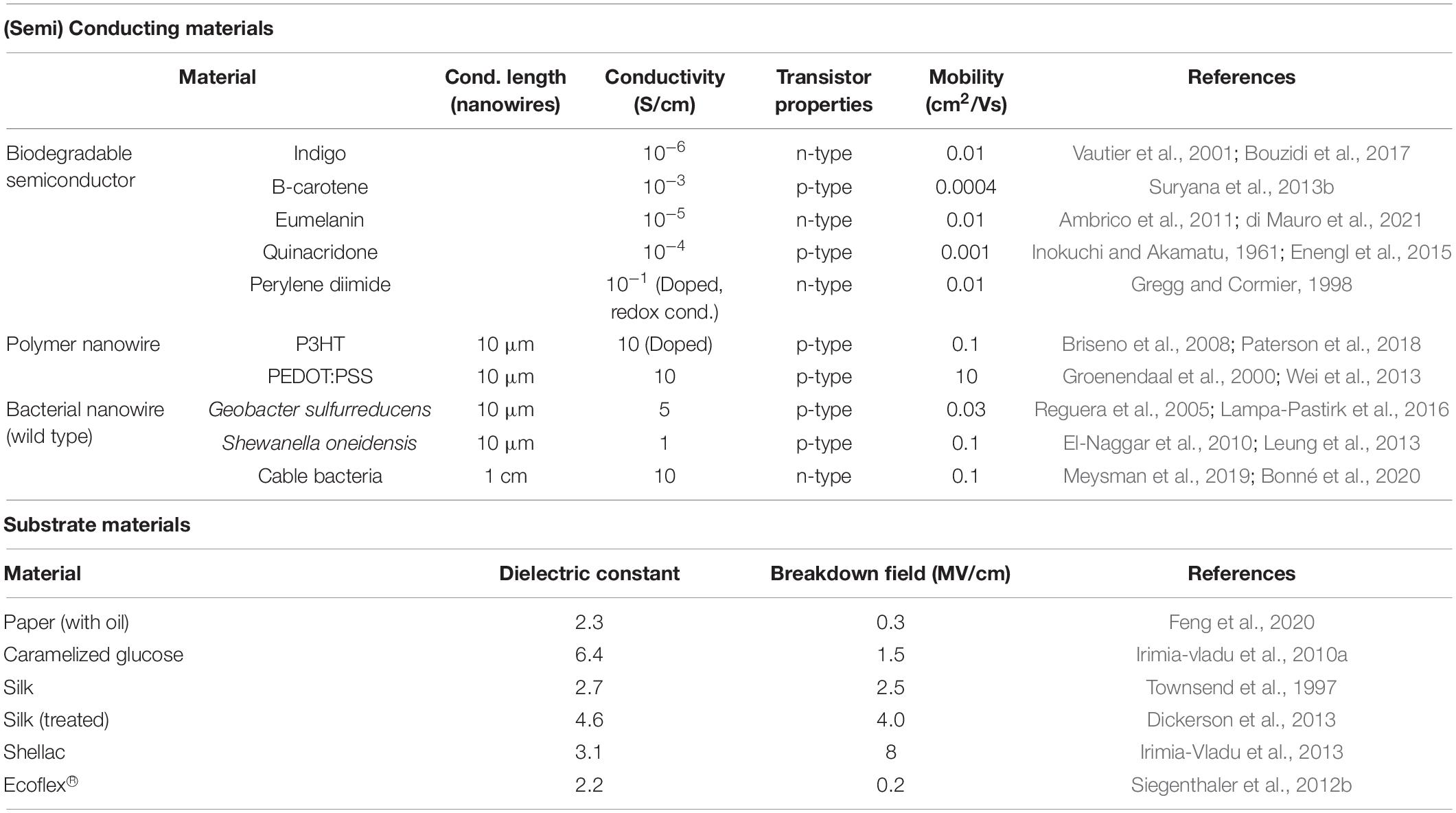
Table 1. An overview for different (semi) conducting materials and substrate materials for biodegradable electronics with their relevant characteristics measured in dry conditions. Bacterial nanowires stand out against the known biodegradable semiconductors for their high conductivity and mobility values. Cable bacteria top other nanowires for their excessive conduction length and n-type transistor behavior.
After looking at plant and animal based biodegradable organic substrates, one can look for substrates originating from microorganisms, an example of which is poly(4hydroxybutyrate) (P4HB), as described by Martin and Williams (2003) and Bettinger and Bao (2010). This material is produced by genetically engineered microorganisms, usually Escherichia coli K12, since it is very difficult to produce polymers fit for most applications through chemical synthesis (Martin and Williams, 2003). P4HB is strong, with a tensile strength comparable to high molecular weight polyethylene, yet flexible, with an elongation to break of 1,000% and is often extremely well tolerated in vivo (Martin and Williams, 2003). As for the semiconductors, there are biodegradable substrates which are neither naturally occurring nor nature inspired. For example, Ecoflex® is a completely biodegradable aliphatic-aromatic polyester which combines excellent mechanical properties, such as its tear-resistance, flexibility, and its resistance to fluctuations in water and humidity, with good processability of synthetic thermoplastics (Siegenthaler et al., 2012a). It is certified worldwide as compostable and approved for contact with food, however, this is only compostable in an industrial composter. A distinction is made between Ecoflex® F, based on fossil monomers, and Ecoflex® FS, a compound with poly(lactic acid) that has a higher rate of biodegradation. The material can also be blended with poly(lactic acid) (PLA), to obtain a blend called Ecovio.
Other materials crucial in the production of capacitors and advanced electrical components like Field-Effect Transistors (FETs) are dielectrics. These are electrical insulators which can be polarized by electric fields such as the previously mentioned biodegradable substrates, but also less sturdy materials. An abundant example is DNA, which can be obtained for example as waste material from the fishing industry and processed from water-based solutions (Irimia-Vladu et al., 2012). When DNA is used in an Organic Field-Effect Transistor (OFET), it displays significant hysteresis in the transfer characteristics, because of its high permittivity (Stadler et al., 2007). The separate nucleobases which compose DNA, adenine, cytosine, guanine, thymine, and uracil (which replaces thymine in RNA) could be used instead. These molecules are abundant and have low toxicity and cost (Irimia-vladu et al., 2010b). Another kind of biodegradable dielectric material are starches (Siegenthaler et al., 2012a). They consist of amylose and amylopectin polymers, forming a multilayer structure through hydrogen bonding. Starches obtained from different types of plants, such as corn or potato, differ, having an influence on the particle size and moisture absorption. Pure starch has multiple properties which makes it difficult to process and does not give it many applications. However, it is possible to chemically modify the starch to overcome these problems, though not enough to use it as substrate (Siegenthaler et al., 2012a). However, it can be used as such if the starch is blended with a hydrophobic polymer such as Ecoflex®.
Electroactive Bacteria for Bioelectronics
In the last decades, other extraordinary electronic materials were found in the world of microbiology: electroactive bacteria that make their own conductive wires. These electroactive microbes developed the ability to respire through minerals or other organisms (Summers et al., 2010; Shi et al., 2016; Sure et al., 2016), either through electron shuttles or as direct contact through self-made nanowires that form an electrical bridge between the bacterium and the mineral. When studying these bacteria in a bioelectrochemical system (BES), they were found to also respire through electrodes. The chosen conditions of the BES, like available substrates and electric potential of the electrode, can be tuned to enhance the nanowire production (Liu and Li, 2020; Yalcin et al., 2020). These bacterial nanowires are found in many forms and debate is still going on about its exact function and chemical structure (Creasey et al., 2018). Below we give an overview of nanowires that were found to be electrically conductive after isolation, both in wet and dry environments (Ing et al., 2018).
One organism intensively studied for its protein nanowires is Geobacter sulfurreducens (Reguera et al., 2005). Nanowires are often found in the form of c-type cytochromes: a heme containing protein with a Fe atom at its core. The prominent example is a nanowire made of a coiling stack of the cytochrome OmcS that consists of 6 heme groups (Wang et al., 2019) with measured conductivities in the order of 5 mS cm–1 (Malvankar et al., 2011; Yalcin et al., 2020). Another cytochrome nanowire made from 8-heme OmcZ proteins even reaches conductivities that are 1,000-fold higher (Yalcin et al., 2020). Next to cytochrome nanowires, a nanowire based on PilA proteins (nicknamed e-pili) have been described in many works (Reguera et al., 2005). Although controversy persists, there is ample evidence of these nanowires conducting electricity with conductivities in the range of 50–200 mS cm–1, respectively, for pH 7 and pH 2 (Adhikari et al., 2016). Another study found conductivities in the range of 1–5 S cm–1 with estimated mobilities in the range of 0.02 cm2 V–1 s–1 (Lampa-Pastirk et al., 2016). In another well-studied organism called Shewanella oneidensis, nanowires are found in the form of extensions of the outer membrane (Gorby et al., 2006; Pirbadian et al., 2014) that are packed with 20-heme protein complexes made of MtrA, MtrB, MtrC and OmcA cytochromes. These nanowires reach values of up to 1 S cm–1 (El-Naggar et al., 2010) and appear to have p-type transistor behavior with mobility values in the range of 0.1 cm2 V–1 s–1 (Leung et al., 2013). Besides these organisms a whole zoo of over 100 different electroactive bacteria (Logan et al., 2019) and their nanowires are either confirmed to have similar properties (Walker et al., 2018, 2019) or are waiting to be tested for their electronic potential.
Even though we can find some very good electrical characteristics for these nanowires, modifications to the wild-type nanowires can boost the conductivity values even further. The introduction of the PilA gene from Geobacter metallireducens in G. sulfurreducens, e.g., produces e-pili with conductivities in the order of 300 S cm–1 (Tan et al., 2017), while the inclusion of more aromatic rings like tryptophan in e-pili increased the conductivities to 100 S cm–1 at pH 7 and 1,000 S cm–1 at pH 2 (Tan et al., 2016). When thinking about applying these bacterial nanowires in biodegradable electronics, one might assume an intensive and costly process, but the recent discovery of nanowire production through genetic manipulation of Escherichia coli (Ueki et al., 2020) and the development of bottom-up fabrication of e-pili (Cosert et al., 2019) suggest a means of easier and cheaper production in the long term. Functionalization and adhesion of different substrates is also made easier with the possibility of decorating nanowires with peptides (Ueki et al., 2019). First proof-of-principle applications are developed as sensors (Smith et al., 2020), (limited) electricity production (Liu et al., 2020) and they are expected to play a role as conductors in bioelectronics (Lovley and Yao, 2021).
A Special Case: Cable Bacteria for Biodegradable Electronics
The main drawback for bacterial nanowires is the relatively short range of conduction, as they were only constructed over micrometer distances for now (Ing et al., 2018). Ten years ago, a new bacterium with the ability of transporting electrons over centimeter distances was discovered in the sea sediment: Cable bacteria (Pfeffer et al., 2012). They developed this mechanism to reach for both H2S in the deeper layer of the sediment and the O2 that is only available at the surface. Coupling the redox reactions of sulfide oxidation and oxygen reduction (Nielsen et al., 2010), it was hypothesized that cable bacteria could transport electrical currents over their centimeter long bodies (Pfeffer et al., 2012). This hypothesis appeared true when Meysman et al. (2019) measured conduction through dry filaments of cable bacteria and found it to be highly conductive with conductivities ranging in the order of 0.01–10 S cm–1, with one measurement even reaching 79 S cm–1. The current was shown to be flowing in an organized structure of around 10–60 parallel wires of each 50 nm in diameter that are present just under the outer membrane of the bacterium (Cornelissen et al., 2018) and that are interconnected at cell junctions (Thiruvallur Eachambadi et al., 2020).
The results presented in previous works show cable bacteria can overcome the micrometer range conduction previously present in electromicrobiology, as conduction has been shown over more than 1 cm, thereby proving by far the longest electron transport in a single biological structure (Meysman et al., 2019). One use of the conductive fibers in cable bacteria could be as an interconnector in a circuit, as they show low contact resistance (Bonné et al., 2020) and conductivities exceeding 10 S cm–1 (Meysman et al., 2019). The typical currents found in vivo are in the order of 0.1–1 nA for a single filament (Pfeffer et al., 2012; Schauer et al., 2014), but measurements on the conductive fiber sheaths show that single filaments can bear currents up to 0.3 μA at a DC bias of 1V (Bonné et al., 2020), and even higher values could be expected. This corresponds with a current density of 3 A mm–2, comparable to the typical allowed density in household copper wires (5–20 A mm–2). Next to its interconnection properties, it was shown that cable bacteria could work as transistors with FET mobilities in the range of 0.1 cm2 V–1 s–1 (Bonné et al., 2020), giving them a potential role in future computational circuits. Finally, next to direct current (DC), alternating current (AC) signals have been shown to pass through cable bacteria (Bonné et al., 2020). Showing visible degradation after 9–30 days in its near-natural environment during which time it has been exposed to mainly (micro-) oxic conditions (Supplementary Figure 1), this suggests that cable bacterium could be an interesting biodegradable material for bioelectronics.
To put the values found for cable bacteria in perspective, in Table 1 their initial electronic properties are compared to the previously discussed biodegradable semiconductors. Conductivity, mobility, and transistor properties for these materials were obtained by dry solid-state measurements like current-voltage-analysis, atomic force microscopy and field-effect transistor measurements (doped perylene diimide, treated in an electrochemical setup, being the only exception). It is clear that cable bacteria stand out with their high mobility values and conductivities that are a few orders of magnitude larger. Next to that, Table 1 also shows the state-of-the-art characteristics of the model (wild type) microbial nanowires of G. sulfurreducens and S. oneidensis and well-studied organic nanowires P3HT and PEDOT:PSS, also resulting from dry solid state measurements. It is apparent that already after a few years of measurements, cable bacteria can easily compete with materials that are studied for one or a few decades. Conductivity and mobility values of cable bacteria fibers can be found in the same range as organic electronics and wild type nanowires, with PEDOT:PSS outrunning alternatives in terms of mobility. However, cable bacteria really stand out for their n-type transistor behavior that is uncommon for biological materials and even more for the conduction length that is a few orders of magnitude higher than typical lengths for other biological and organic nanowires.
Conclusion, Challenges, and Outlook
The increasing amount of e-waste that is created worldwide has put an urge on the search for biodegradable electronics. In the mini-review presented in this work, we found that important progress was made in finding more sustainable materials in electronics, but their limited applicability in modern day devices shows they have a long way to go. An important element missing in most studies so far is a common and quantitative study on the rate and conditions of biodegradability of the different materials, which is crucial for their further investigation as bioelectronic materials. In the search for biological materials that could function as conductors or transistors in biodegradable electronics, interesting candidates are found in the world of electromicrobiology (Lovley, 2012), where electroactive bacteria like Geobacter and Shewanella species produce nanowires as electron transport conduits. Recent progress in the study of different proteins (Yalcin et al., 2020) and genetic manipulation has ramped up the conductivity values (Tan et al., 2016, 2017); modification of Escherichia coli has made PilA based nanowires easy to produce (Ueki et al., 2020). Yet, conduction lengths exceeding micrometers are yet to be shown, as well as the implementation in biodegradable electronic circuitry. An answer to this shortcoming might be cable bacteria that create nanowire networks that show electron transport over centimeters with high conductivities and electron mobility values.
Although the characteristics in Table 1 seem promising, research on cable bacteria is only in a young stage, and some limitations must be overcome to meet industrial requirements. Firstly, values for the conductivity can vary a few orders of magnitude among experiments, with Table 1 only displaying the order or magnitude of the highest measured values. This might be because all measurements were done on different species of cable bacteria taken from natural sediments that come from different field sites (Kjeldsen et al., 2019) and the variability found among cells within one organism (Bonné et al., 2020). Moreover, the measurement conditions were different for all filaments. As current through a bacterium decays exponentially in ambient air, a sample preparation time that takes 1 min longer (before placing the sample in a nitrogen environment or vacuum) might change the conductivity by a factor of 2. Although the true conductivity of the conductive fibers in cable bacteria is expected to be close to and possibly higher than the maximally reported value of 79 S cm–1, a more consistent set of values of the electronic properties is needed for the different species of cable bacteria. As a second limitation, all species of cable bacteria lack a pure culture so far, making upscaling difficult. Although some attempts have been made toward it, only a single-strain sediment culture was achieved until this point (Thorup et al., 2021). Finally, the conductive periplasmic fibers are yet to be extracted properly from cable bacteria, as it is expected that the pure fibers rather than the full filaments will be used in future electronics (Cosert et al., 2019). An easy and cheap method must be developed to either fully extract the fibers or produce them in a laboratory environment. It has to be emphasized that the chemical nature of the conductive fibers in cable bacteria is not yet discovered (Kjeldsen et al., 2019; Boschker et al., 2021; Thiruvallur Eachambadi et al., 2021), making it difficult to describe the electron transport mechanism, quantify the biodegradability of the material, and create a detailed forecast of the applicability in bioelectronics.
As a general conclusion, it can be stated that significant progress is being made toward biodegradable electronics, in terms of the usage and development of biomaterials and electroactive bacteria. Within the class of electroactive bacteria, cable bacteria show some very interesting features that could make them a potential long-range biodegradable interconnector or transistor in future durable electronics. Although the research field needs to overcome a few challenges before being able to get scaled up, the bacteria were only discovered 10 years ago and only in 2019 the first direct electrical measurements were made. If this blooming trend continues as it did for bacterial nanowires (Lovley and Walker, 2019), the road is open for cable bacteria fibers or cable bacteria inspired materials to be integrated together with a variety of complementary biomaterials and electroactive bacteria in our electronics in another 10 years from now. This new generation of electronics—being biodegradable—will dissolve in time together with the global e-waste problem.
Author Contributions
JM conceived the study. RB and KW wrote the manuscript with equal contribution. JL made Supplementary Figure 1. All authors did editing and proofreading.
Funding
This research was financially supported by the Research Foundation-Flanders (FWO project grant G013922N to JM) and Danish National Research Foundation (grant DNRF136 to JL).
Conflict of Interest
The authors declare that the research was conducted in the absence of any commercial or financial relationships that could be construed as a potential conflict of interest.
Publisher’s Note
All claims expressed in this article are solely those of the authors and do not necessarily represent those of their affiliated organizations, or those of the publisher, the editors and the reviewers. Any product that may be evaluated in this article, or claim that may be made by its manufacturer, is not guaranteed or endorsed by the publisher.
Acknowledgments
We thank the colleagues from X-LAB and PHYSXLAB from Hasselt University and for discussions.
Supplementary Material
The Supplementary Material for this article can be found online at: https://www.frontiersin.org/articles/10.3389/fmicb.2022.906363/full#supplementary-material
References
Adhikari, R. Y., Malvankar, N. S., Tuominen, M. T., and Lovley, D. R. (2016). Conductivity of individual Geobacter pili. RSC Adv. 6, 8354–8357. doi: 10.1039/c5ra28092c
Ambrico, M., Ambrico, P. F., Cardone, A., Ligonzo, T., Cicco, S. R., di Mundo, R., et al. (2011). Melanin layer on silicon: An attractive structure for a possible exploitation in bio-polymer based metal-insulator-silicon devices. Adv. Mat. 23, 3332–3336. doi: 10.1002/ADMA.201101358
Beratan, D. N. (2019). Why Are DNA and Protein Electron Transfer So Different? Ann. Rev. Phys. Chem. 70, 71–97. doi: 10.1146/annurev-physchem-042018-052353
Bettinger, C. J., and Bao, Z. (2010). Organic thin-film transistors fabricated on resorbable biomaterial substrates. Adv. Mat. 22, 651–655. doi: 10.1002/adma.200902322
Bonné, R., Hou, J. L., Hustings, J., Wouters, K., Meert, M., Hidalgo-Martinez, S., et al. (2020). Intrinsic electrical properties of cable bacteria reveal an Arrhenius temperature dependence. Sci. Rep. 1:19798. doi: 10.1038/s41598-020-76671-5
Boschker, H. T. S., Cook, P. L. M., Polerecky, L., Eachambadi, R. T., Lozano, H., Hidalgo-Martinez, S., et al. (2021). Efficient long-range conduction in cable bacteria through nickel protein wires. Nat. Commun. 12:3996. doi: 10.1038/s41467-021-24312-4
Bouzidi, A., Yahia, I. S., and El-Sadek, M. S. A. (2017). Novel and highly stable indigo (C.I. Vat Blue I) organic semiconductor dye: Crystal structure, optically diffused reflectance and the electrical conductivity/dielectric behaviors. Dyes Pigments 146, 66–72. doi: 10.1016/J.DYEPIG.2017.06.046
Briseno, A. L., Mannsfeld, S. C. B., Jenekhe, S. A., Bao, Z., and Xia, Y. (2008). Introducing organic nanowire transistors. Materials Today 11, 38–47. doi: 10.1016/S1369-7021(08)70055-5
Campos, R., Kandelbauer, A., Robra, K. H., Cavaco-Paulo, A., and Gübitz, G. M. (2001). Indigo degradation with purified laccases from Trametes hirsuta and Sclerotium rolfsii. J. Biotechnol. 89, 131-9. doi: 10.1016/S0168-1656(01)00303-0
Chanfreau, S., Mena, M., Porras-Domínguez, J. R., Ramírez-Gilly, M., Gimeno, M., Roquero, P., et al. (2010). Enzymatic synthesis of poly-L-lactide and poly-L-lactide-co-glycolide in an ionic liquid. Bioproc. Biosyst. Eng. 33, 629–638. doi: 10.1007/s00449-009-0388-8
Chen, L., Bai, G., Yang, R., Zang, J., Zhou, T., and Zhao, G. (2014). Encapsulation of β-carotene within ferritin nanocages greatly increases its water-solubility and thermal stability. Food Chem. 149, 307–312. doi: 10.1016/J.FOODCHEM.2013.10.115
Cornelissen, R., Bøggild, A., Thiruvallur Eachambadi, R., Koning, R. I., Kremer, A., Hidalgo-Martinez, S., et al. (2018). The Cell Envelope Structure of Cable Bacteria. Front. Microbiol. 9:3044. doi: 10.3389/fmicb.2018.03044
Cosert, K. M., Castro-Forero, A., Steidl, R. J., Worden, R. M., and Reguera, G. (2019). Bottom-up fabrication of protein nanowires via controlled self-assembly of recombinant Geobacter pilins. mBio 10:e02721–19 doi: 10.1128/mBio.02721-19
Creasey, R. C. G., Mostert, A. B., Nguyen, T. A. H., Virdis, B., Freguia, S., and Laycock, B. (2018). Microbial nanowires – Electron transport and the role of synthetic analogues. Acta Biomat. 69, 1–30. doi: 10.1016/j.actbio.2018.01.007
Daniel Głowacki, E., Irimia-Vladu, M., Kaltenbrunner, M., Ga, J., White, M. S., Monkowius, U., et al. (2013). Hydrogen-Bonded Semiconducting Pigments for Air-Stable Field-Effect Transistors. Adv. Materials 25, 1563–1569. doi: 10.1002/ADMA.201204039
di Mauro, E., Rho, D., and Santato, C. (2021). Biodegradation of bio-sourced and synthetic organic electronic materials towards green organic electronics. Nat. Commun. 1:3167. doi: 10.1038/s41467-021-23227-4
Dickerson, M. B., Fillery, S. P., Koerner, H., Singh, K. M., Martinick, K., Drummy, L. F., et al. (2013). Dielectric breakdown strength of regenerated silk fibroin films as a function of protein conformation. Biomacromolecules 14, 3509–3514. doi: 10.1021/BM4008452
El-Naggar, M. Y., Wanger, G., Leung, K. M., Yuzvinsky, T. D., Southam, G., Yang, J., et al. (2010). Electrical transport along bacterial nanowires from Shewanella oneidensis MR-1. Proc. Natl. Acad. Sci. 107, 18127–18131. doi: 10.1073/pnas.1004880107
Enengl, C., Enengl, S., Havlicek, M., Stadler, P., Glowacki, E. D., Scharber, M. C., et al. (2015). The Role of Heteroatoms Leading to Hydrogen Bonds in View of Extended Chemical Stability of Organic Semiconductors. Adv. Funct. Mat. 25, 6679–6688. doi: 10.1002/ADFM.201503241
Feig, V. R., Tran, H., and Bao, Z. (2018). Biodegradable Polymeric Materials in Degradable Electronic Devices. ACS Central Sci. 4, 337–348. doi: 10.1021/acscentsci.7b00595
Feng, D., Hao, J., Yang, L., Liao, R., Chen, X., and Li, J. (2020). Comparison of AC breakdown characteristics on insulation paper (pressboard) immersed by three-element mixed insulation oil and mineral oil. High Volt. 5, 298–305. doi: 10.1049/HVE.2019.0103
Glowacki, E. D., Leonat, L., Voss, G., Bodea, M., Bozkurt, Z., Irimia-Vladu, M., et al. (2011). Natural and nature-inspired semiconductors for organic electronics. Organic Semiconductors in Sensors and Bioelectronics IV (Bellingham:SPIE) M1–M81180. doi: 10.1117/12.892467
Gorby, Y. A., Yanina, S., McLean, J. S., Rosso, K. M., Moyles, D., Dohnalkova, A., et al. (2006). Electrically conductive bacterial nanowires produced by Shewanella oneidensis strain MR-1 and other microorganisms. Proc. Natl. Acad. Sci. U.S.A. 103, 11358–11363. doi: 10.1073/pnas.0604517103
Grant, K., Goldizen, F. C., Sly, P. D., Brune, M. N., Neira, M., van den Berg, M., et al. (2013). Health consequences of exposure to e-waste: A systematic review. Lancet Glob. Health 1, e350–e361. doi: 10.1016/S2214-109X(13)70101-3
Gregg, B. A., and Cormier, R. A. (1998). Liquid Crystal Perylene Diimide Films Characterized by Electrochemical, Spectroelectrochemical, and Conductivity versus Potential Measurements. J. Phys. Chem. B 102, 9952–9957. doi: 10.1021/JP982842E
Groenendaal, L., Jonas, F., Freitag, D., Pielartzik, H., and Reynolds, J. R. (2000). Poly(3,4-ethylenedioxythiophene) and Its Derivatives: Past, Present, and Future. Adv. Mat. 12, 481–494. doi: 10.1002/(SICI)1521-4095(200004)12:7<481::AID-ADMA481<3.0.CO;2-C
Harrington, W. F., and von Hippel, P. H. (1962). The Structure Of Collagen And Gelatin. Adv. Protein Chem. 16, 1–138. doi: 10.1016/S0065-3233(08)60028-5
Hu, X., Shmelev, K., Sun, L., Gil, E. S., Park, S. H., Cebe, P., et al. (2011). Regulation of silk material structure by temperature-controlled water vapor annealing. Biomacromolecules 12, 1686–1696. doi: 10.1021/bm200062a
Hwang, S.-W., Tao, H., Kim, D.-H., Cheng, H., Song, J.-K., Rill, E., et al. (2012). A Physically Transient Form of Silicon Electronics. Science 337, 1640–1644. doi: 10.1126/science.1226325
Ing, N. L., El-Naggar, M. Y., and Hochbaum, A. I. (2018). Going the Distance: Long-Range Conductivity in Protein and Peptide Bioelectronic Materials. J. Phys. Chem. B 122, 10403–10423. doi: 10.1021/acs.jpcb.8b07431
Inokuchi, H., and Akamatu, H. (1961). Electrical Conductivity of Organic Semiconductors. Solid State Phys.Adv. Res. Appl. 12, 93–148. doi: 10.1016/S0081-1947(08)60653-0
Irimia-vladu, B. M., Troshin, P. A., Reisinger, M., Shmygleva, L., Kanbur, Y., Schwabegger, G., et al. (2010a). Biocompatible and Biodegradable Materials for Organic Field-Effect Transistors. 4069–4076. doi: 10.1002/adfm.201001031
Irimia-vladu, M., Troshin, P. A., Reisinger, M., Schwabegger, G., Ullah, M., Schwoediauer, R., et al. (2010b). Environmentally sustainable organic field effect transistors. Organic Elect. 11, 1974–1990. doi: 10.1016/j.orgel.2010.09.007
Irimia-Vladu, M., Głowacki, E. D., Schwabegger, G., Leonat, L., Akpinar, H. Z., Sitter, H., et al. (2013). Natural resin shellac as a substrate and a dielectric layer for organic field-effect transistors. Green Chem. 15, 1473–1476. doi: 10.1039/C3GC40388B
Irimia-Vladu, M., Głowacki, E. D., Voss, G., Bauer, S., and Sariciftci, N. S. (2012). Green and biodegradable electronics. Materials Today 15, 340–346. doi: 10.1016/S1369-7021(12)70139-6
Kang, S. K., Hwang, S. W., Cheng, H., Yu, S., Kim, B. H., Kim, J. H., et al. (2014). Dissolution behaviors and applications of silicon oxides and nitrides in transient electronics. Adv. Funct. Materials 24, 4427–4434. doi: 10.1002/adfm.201304293
Kang, S. K., Yin, L., and Bettinger, C. (2020). The emergence of transient electronic devices. MRS Bull. 45, 87–95. doi: 10.1557/mrs.2020.19
Kim, D. H., Kim, Y. S., Wu, J., Liu, Z., Song, J., Kim, H. S., et al. (2009). Ultrathin silicon circuits with strain-isolation layers and mesh layouts for high-performance electronics on fabric, vinyl, leather, and paper. Adv. Materials 21, 3703–3707. doi: 10.1002/adma.200900405
Kjeldsen, K. U., Schreiber, L., Thorup, C. A., Boesen, T., Bjerg, J. T., Yang, T., et al. (2019). On the evolution and physiology of cable bacteria. Proc. Natl. Acad. Sci. 116:201903514. doi: 10.1073/pnas.1903514116
Lampa-Pastirk, S., Veazey, J. P., Walsh, K. A., Feliciano, G. T., Steidl, R. J., Tessmer, S. H., et al. (2016). Thermally activated charge transport in microbial protein nanowires. Sci. Rep. 6:23517. doi: 10.1038/srep23517
Lee, Y. K., Yu, K. J., Song, E., Barati Farimani, A., Vitale, F., Xie, Z., et al. (2017). Dissolution of Monocrystalline Silicon Nanomembranes and Their Use as Encapsulation Layers and Electrical Interfaces in Water-Soluble Electronics. ACS Nano 11, 12562–12572. doi: 10.1021/acsnano.7b06697
Leung, K. M., Wanger, G., El-Naggar, M. Y., Gorby, Y., Southam, G., Lau, W. M., et al. (2013). Shewanella oneidensis MR-1 Bacterial Nanowires Exhibit p-Type, Tunable Electronic Behavior. Nano Lett. 13, 2407–2411. doi: 10.1021/nl400237p
Li, M., Guo, Y., Wei, Y., MacDiarmid, A. G., and Lelkes, P. I. (2006). Electrospinning polyaniline-contained gelatin nanofibers for tissue engineering applications. Biomaterials 27, 2705–2715. doi: 10.1016/J.BIOMATERIALS.2005.11.037
Liu, D. F., and Li, W. W. (2020). Potential-dependent extracellular electron transfer pathways of exoelectrogens. Curr. Opin. Chem. Biol. 59, 140–146. doi: 10.1016/J.CBPA.2020.06.005
Liu, X., Gao, H., Ward, J. E., Liu, X., Yin, B., Fu, T., et al. (2020). Power generation from ambient humidity using protein nanowires. Nature 578, 550–554. doi: 10.1038/s41586-020-2010-9
Logan, B. E., Rossi, R., Ragab, A., and Saikaly, P. E. (2019). Electroactive microorganisms in bioelectrochemical systems. Nat. Rev. Microbiol. 17, 307–319. doi: 10.1038/s41579-019-0173-x
Lovley, D. R. (2012). Electromicrobiology. Annu. Rev. Microbiol. 66, 391–409 doi: 10.1146/annurev-micro-092611-150104
Lovley, D. R., and Walker, D. J. F. (2019). Geobacter Protein Nanowires. Front. Microbiol. 10:2078. doi: 10.3389/fmicb.2019.02078
Lovley, D. R., and Yao, J. (2021). Intrinsically Conductive Microbial Nanowires for ‘Green’ Electronics with Novel Functions. Trends Biotechnol. 39, 940–952. doi: 10.1016/J.TIBTECH.2020.12.005
Malvankar, N. S., Vargas, M., Nevin, K. P., Franks, A. E., Leang, C., Kim, B. C., et al. (2011). Tunable metallic-like conductivity in microbial nanowire networks. Nat. Nanotechnol. 6, 573–579. doi: 10.1038/nnano.2011.119
Martin, D. P., and Williams, S. F. (2003). Medical applications of poly-4-hydroxybutyrate: A strong flexible absorbable biomaterial. Biochem. Eng. J. 16, 97–105. doi: 10.1016/S1369-703X(03)00040-8
Meysman, F. J. R., Cornelissen, R., Trashin, S., Bonné, R., Hidalgo-Martinez, S., van der Veen, J., et al. (2019). A highly conductive fibre network enables centimetre-scale electron transport in multicellular cable bacteria. Nat. Commun. 10:4120. doi: 10.1038/s41467-019-12115-7
Nielsen, L. P., Risgaard-Petersen, N., Fossing, H., Christensen, P. B., and Sayama, M. (2010). Electric currents couple spatially separated biogeochemical processes in marine sediment. Nature 463, 1071–1074. doi: 10.1038/nature08790
Paterson, A. F., Singh, S., Fallon, K. J., Hodsden, T., Han, Y., Schroeder, B. C., et al. (2018). Recent Progress in High-Mobility Organic Transistors: A Reality Check. Adv. Materials 30:e1801079. doi: 10.1002/adma.201801079
Pfeffer, C., Larsen, S., Song, J., Dong, M., Besenbacher, F., Meyer, R. L., et al. (2012). Filamentous bacteria transport electrons over centimetre distances. Nature 491, 218–221. doi: 10.1038/nature11586
Pirbadian, S., Barchinger, S. E., Leung, K. M., Byun, H. S., Jangir, Y., Bouhenni, R. A., et al. (2014). Shewanella oneidensis MR-1 nanowires are outer membrane and periplasmic extensions of the extracellular electron transport components. Proc. Natl. Acad. Sci. U.S.A. 111, 12883–12888. doi: 10.1073/pnas.1410551111
Prado, A. G. S., Bolzon, L. B., Pedroso, C. P., Moura, A. O., and Costa, L. L. (2008). Nb2O5 as efficient and recyclable photocatalyst for indigo carmine degradation. Appl. Catalysis B 82, 219–224. doi: 10.1016/j.apcatb.2008.01.024
Reguera, G., McCarthy, K. D., Mehta, T., Nicoll, J. S., Tuominen, M. T., and Lovley, D. R. (2005). Extracellular electron transfer via microbial nanowires. Nature 435, 1098–1101. doi: 10.1038/nature03661
Ryder, G., and Zhao Houlin, H. (2019). The world’s e-waste is a huge problem. It’s also a golden opportunity. World Economic Forum Annual Meeting. Available online at: https://www.weforum.org/agenda/2019/01/how-a-circular-approach-can-turn-e-waste-into-a-golden-opportunity/. (Accessed on Feb 8 2021)
Schauer, R., Risgaard-Petersen, N., Kjeldsen, K. U., Tataru Bjerg, J. J., Jørgensen, B., Schramm, A., et al. (2014). Succession of cable bacteria and electric currents in marine sediment. ISME J. 8, 1314–1322. doi: 10.1038/ismej.2013.239
Seidel, H., Csepregi, L., Heuberger, A., and Baumgärtel, H. (1990). Anisotropic Etching of Crystalline Silicon in Alkaline Solutions: II. Influence of Dopants. J.Electrochem. Soc. 137, 3626–3632. doi: 10.1149/1.2086278
Sekitani, T., Yokota, T., Zschieschang, U., Klauk, H., Bauer, S., Takeuchi, K., et al. (2009). Organic nonvolatile memory transistors for flexible sensor arrays. Science 326, 1516–1519. doi: 10.1126/science.1179963
Shi, L., Dong, H., Reguera, G., Beyenal, H., Lu, A., Liu, J., et al. (2016). Extracellular electron transfer mechanisms between microorganisms and minerals. Nat. Rev. Microbiol. 10, 651–662. doi: 10.1038/nrmicro.2016.93
Siegenthaler, K. O., Künkel, A., Skupin, G., and Yamamoto, M. (2012a). Ecoflex® and ecovio®: Biodegradable, performance-enabling plastics. Adv. Polymer Sci. 245, 91–136. doi: 10.1007/12-2010-106
Siegenthaler, K. O., Künkel, A., Skupin, G., and Yamamoto, M. (2012b). Ecoflex® and ecovio®: Biodegradable, performance-enabling plastics. Adv. Polymer Sci. 245, 91–136.
Smith, A. F., Liu, X., Woodard, T. L., Fu, T., Emrick, T., Jiménez, J. M., et al. (2020). Bioelectronic protein nanowire sensors for ammonia detection. Nano Res. 5, 1479–1484. doi: 10.1007/S12274-020-2825-6
Stadler, P., Oppelt, K., Singh, T. B., Grote, J. G., Schwödiauer, R., Bauer, S., et al. (2007). Organic field-effect transistors and memory elements using deoxyribonucleic acid (DNA) gate dielectric. Organic Elect. 8, 648–654. doi: 10.1016/j.orgel.2007.05.003
Summers, Z. M., Fogarty, H. E., Leang, C., Franks, A. E., Malvankar, N. S., and Lovley, D. R. (2010). Direct exchange of electrons within aggregates of an evolved syntrophic coculture of anaerobic bacteria. Science 330, 1413–1415. doi: 10.1126/SCIENCE.1196526
Sure, S., Ackland, M. L., Torriero, A. A. J., Adholeya, A., and Kochar, M. (2016). Microbial nanowires: An electrifying tale. Microbiology 162, 2017–2028. doi: 10.1099/mic.0.000382
Suryana, R., and Khoiruddin Supriyanto, A. (2013a). Beta-carotene dye of daucus carota as sensitizer on dye-sensitized solar cell. Materials Sci. Forum 737, 15–19. doi: 10.4028/www.scientific.net/MSF.737.15
Suryana, R., and Khoiruddin Supriyanto, A. (2013b). Beta-carotene dye of daucus carota as sensitizer on dye-sensitized solar cell. Materials Sci. Forum 737, 15–19. doi: 10.4028/WWW.SCIENTIFIC.NET/MSF.737.15
Tan, Y., Adhikari, R. Y., Malvankar, N. S., Pi, S., Ward, J. E., Woodard, T. L., et al. (2016). Synthetic Biological Protein Nanowires with High Conductivity. Small 12, 4481–4485. doi: 10.1002/smll.201601112
Tan, Y., Adhikari, R. Y., Malvankar, N. S., War, J. E., Woodard, T. L., Nevin, K. P., et al. (2017). Expressing the geobacter metallireducens pila in geobacter sulfurreducens yields pili with exceptional conductivity. mBio 8, e02203–16. doi: 10.1128/mBio.02203-16
Thiruvallur Eachambadi, R., Bonné, R., Cornelissen, R., Hidalgo-Martinez, S., Vangronsveld, J., Meysman, F. J. R., et al. (2020). An Ordered and Fail-Safe Electrical Network in Cable Bacteria. Adv. Biosyst. 4:2000006. doi: 10.1002/adbi.202000006
Thiruvallur Eachambadi, R., Boschker, H. T. S., Franquet, A., Spampinato, V., Hidalgo-Martinez, S., Valcke, R., et al. (2021). Enhanced Laterally Resolved ToF-SIMS and AFM Imaging of the Electrically Conductive Structures in Cable Bacteria. Analytical Chem. 93, 7226–7234. doi: 10.1021/ACS.ANALCHEM.1C00298
Thorup, C., Petro, C., Bøggild, A., Ebsen, T. S., Brokjær, S., Nielsen, L. P., et al. (2021). How to grow your cable bacteria: Establishment of a stable single-strain culture in sediment and proposal of Candidatus Electronema aureum GS. Syst. Appl. Microbiol. 44:126236. doi: 10.1016/J.SYAPM.2021.126236
Townsend, P. H., Martin, S. J., Godschalx, J., Romer, D. R., Smith, D. W., Castillo, D., et al. (1997). Silk Polymer Coating with Low Dielectric Constant and High Thermal Stability for Ulsi Interlayer Dielectric. MRS Online Proc. Library 1, 9–17. doi: 10.1557/PROC-476-9
Ueki, T., Walker, D. J. F., Tremblay, P. L., Nevin, K. P., Ward, J. E., Woodard, T. L., et al. (2019). Decorating the Outer Surface of Microbially Produced Protein Nanowires with Peptides. ACS Synthetic Biol. 8, 1809–1817. doi: 10.1021/ACSSYNBIO.9B00131/SUPPL_FILE/SB9B00131_SI_001.PDF
Ueki, T., Walker, D. J. F., Woodard, T. L., Nevin, K. P., Nonnenmann, S. S., and Lovley, D. R. (2020). An Escherichia coli Chassis for Production of Electrically Conductive Protein Nanowires. ACS Synthetic Biol. 9, 647–654. doi: 10.1021/ACSSYNBIO.9B00506/SUPPL_FILE/SB9B00506_SI_001.PDF
Valdez-Vazquez, I., Robledo-Rizo, J. G., Muñoz-Páez, K. M., Pérez-Rangel, M., and de la Luz Ruiz-Aguilar, G. M. (2020). Simultaneous hydrogen production and decolorization of denim textile wastewater: kinetics of decolorizing of indigo dye by bacterial and fungal strains. Braz. J. Microbiol. 51, 701–709. doi: 10.1007/s42770-019-00157-4
Vautier, M., Guillard, C., and Herrmann, J. M. (2001). Photocatalytic Degradation of Dyes in Water: Case Study of Indigo and of Indigo Carmine. J. Catalysis 201, 46–59. doi: 10.1006/JCAT.2001.3232
Walker, D. J., Adhikari, R. Y., Holmes, D. E., Ward, J. E., Woodard, T. L., Nevin, K. P., et al. (2018). Electrically conductive pili from pilin genes of phylogenetically diverse microorganisms. ISME J. 12, 48–58. doi: 10.1038/ismej.2017.141
Walker, D. J. F., Martz, E., Holmes, D. E., Zhou, Z., Nonnenmann, S. S., and Lovley, D. R. (2019). The archaellum of methanospirillum hungatei is electrically conductive. mBio 10, e00579–19. doi: 10.1128/mBio.00579-19
Wang, F., Gu, Y., O’Brien, J. P., Yi, S. M., Yalcin, S. E., Srikanth, V., et al. (2019). Structure of Microbial Nanowires Reveals Stacked Hemes that Transport Electrons over Micrometers. Cell 177, 361.e–369.e. doi: 10.1016/j.cell.2019.03.029
Wang, Y., Rudym, D. D., Walsh, A., Abrahamsen, L., Kim, H. J., Kim, H. S., et al. (2008). In vivo degradation of three-dimensional silk fibroin scaffolds. Biomaterials 29, 3415–3428. doi: 10.1016/j.biomaterials.2008.05.002
Wei, Q., Mukaida, M., Naitoh, Y., and Ishida, T. (2013). Morphological Change and Mobility Enhancement in PEDOT:PSS by Adding Co-solvents. Adv. Materials 25, 2831–2836. doi: 10.1002/adma.201205158
Yalcin, S. E., O’Brien, J. P., Gu, Y., Reiss, K., Yi, S. M., Jain, R., et al. (2020). Electric field stimulates production of highly conductive microbial OmcZ nanowires. Nat. Chem. Biol. 10, 1136–1142. doi: 10.1038/s41589-020-0623-9
Zollinger, H. (2003). Color Chemistry Syntheses, Properties, and Applications of Organic Dyes and Pigments: Von H. Zollinger; Weinheim, Basel, Cambridge, New York, VCH Verlagsgesellschaft, 1987; XII, 367 Seiten mit 40 Bildern und 16 Tabellen; Format 17 cm × 24 cm, Pappband DM. 3rd revise. Zeitschrift fü Chemie 29, 259–268. doi: 10.1002/zfch.19890291016
Keywords: cable bacteria, long-range electron transport, bioelectronics, organic electronics, e-waste, e-biologics, microbial nanowires, biological semiconductor
Citation: Bonné R, Wouters K, Lustermans JJM and Manca JV (2022) Biomaterials and Electroactive Bacteria for Biodegradable Electronics. Front. Microbiol. 13:906363. doi: 10.3389/fmicb.2022.906363
Received: 28 March 2022; Accepted: 20 May 2022;
Published: 10 June 2022.
Edited by:
Amelia-Elena Rotaru, University of Southern Denmark, DenmarkReviewed by:
Kengo Inoue, University of Miyazaki, JapanAnnette Ruth Rowe, University of Cincinnati, United States
Joshua T. Atkinson, University of Southern California, United States
Copyright © 2022 Bonné, Wouters, Lustermans and Manca. This is an open-access article distributed under the terms of the Creative Commons Attribution License (CC BY). The use, distribution or reproduction in other forums is permitted, provided the original author(s) and the copyright owner(s) are credited and that the original publication in this journal is cited, in accordance with accepted academic practice. No use, distribution or reproduction is permitted which does not comply with these terms.
*Correspondence: Robin Bonné, cm9iaW4uYm9ubmVAYmlvLmF1LmRr
†These authors have contributed equally to this work