- 1Microbiology Research Group, Matís ohf., Reykjavík, Iceland
- 2ESBS, University of Strasbourg, Strasbourg, France
- 3EM3B Laboratory, BRM, IFREMER, Nantes, France
- 4Faculty of Biosciences, Department of Animal and Aquaculture Sciences, Norwegian University of Life Sciences, Ås, Norway
- 5Faculty of Food Science and Nutrition, University of Iceland, Reykjavik, Iceland
Beneficial bacteria promise to promote the health and productivity of farmed fish species. However, the impact on host physiology is largely strain-dependent, and studies on Arctic char (Salvelinus alpinus), a commercially farmed salmonid species, are lacking. In this study, 10 candidate probiotic strains were subjected to in vitro assays, small-scale growth trials, and behavioral analysis with juvenile Arctic char to examine the impact of probiotic supplementation on fish growth, behavior and the gut microbiome. Most strains showed high tolerance to gastric juice and fish bile acid, as well as high auto-aggregation activity, which are important probiotic characteristics. However, they neither markedly altered the core gut microbiome, which was dominated by three bacterial species, nor detectably colonized the gut environment after the 4-week probiotic treatment. Despite a lack of long-term colonization, the presence of the bacterial strains showed either beneficial or detrimental effects on the host through growth rate enhancement or reduction, as well as changes in fish motility under confinement. This study offers insights into the effect of bacterial strains on a salmonid host and highlights three strains, Carnobacterium divergens V41, Pediococcus acidilactici ASG16, and Lactiplantibacillus plantarum ISCAR-07436, for future research into growth promotion of salmonid fish through probiotic supplementation.
Introduction
Probiotics in aquaculture are defined as live or dead microorganisms administered through the feed or rearing environment that confer a health benefit to their host (Merrifield et al., 2010). Over the past decades, probiotics have attracted a growing interest as a promising new technology to enhance the health and productivity of farmed animals. Aquaculture is currently the fastest growing animal protein sector in the world, expected to continue providing a significant share of animal proteins as the global population increases (FAO, 2018). Research on the use of probiotics in aquaculture has demonstrated several benefits to farmed fish through increased disease resistance, higher survival, enhanced growth performance and improved water quality (Gatesoupe, 1999; Balcázar et al., 2006; Ringø et al., 2020). Probiotics can contribute to the sustainable development of the aquaculture sector, including a reduction of antibiotic use, decreased dependence on wild fish stocks for feed production and a reduced environmental footprint (Wang et al., 2008; Hai, 2015; Olmos et al., 2019). Previous studies have shown that increased disease resistance through probiotic supplementation can be achieved through competitive exclusion of pathogens (Vine et al., 2004a,b; Balcázar et al., 2006; Knipe et al., 2021), the production of antimicrobial compounds (Ravi et al., 2007; Muñoz-Atienza et al., 2013; Tan et al., 2016), or by enhancing the host immune response (Irianto and Austin, 2002; Nayak, 2010; Abumourad et al., 2013). This can lead both to reduced mortality and to increased growth due to reduced pathogen-induced stress (Gatesoupe, 1994; Queiroz and Boyd, 1998; Chang and Liu, 2002; Jha et al., 2015). Other mechanisms involved in growth rate enhancement through probiotic treatment include higher nutrient retention and digestibility through microbial enzymes aiding in nutrient breakdown and the microbial production of vitamins, fatty acids and other nutrients (Bagheri et al., 2008; Mohapatra et al., 2012; Dawood et al., 2019; Mohammadian et al., 2019; Ringø et al., 2020; Tarkhani et al., 2020). In addition, higher nutrient retention and enzymatic breakdown of otherwise inaccessible nutrients can lead to a lower feed conversion ratio and the usability of a wider range of protein sources, potentially replacing fish meal with more sustainable plant proteins in fish feeds (Van Doan et al., 2018; Jang et al., 2019; Serra et al., 2019). Apart from benefits toward health and growth, it has recently been shown that probiotics can influence the behavior of fish (Borrelli et al., 2016). Behavioral characteristics and stress coping styles, which are important indicators of animal welfare, have been linked to the brain-gut axis in fish (Ashley, 2007; Rosengren et al., 2018). Modulating behavior through diets and probiotic supplementation could therefore increase the wellbeing of farmed fish under high stocking densities (Banerjee and Ray, 2017; Soares et al., 2019). Despite large efforts in probiotics research to date, only one bacterial probiotic, a strain of the lactic acid bacterium Pediococcus acidilactici licensed under the brand name BACTOCELL® (Lallemand, Canada), has been approved for use in aquaculture in the European Union (Fečkaninová et al., 2017; Serra et al., 2019). In addition, it remains unclear how certain probiotic strains modulate the core fish gut microbiota or how they colonize the gut environment.
The aim of the present study was to evaluate the probiotic characteristics of 10 candidate probiotic bacterial strains through the analysis of in vitro assays and their in vivo activity on the growth, gut microbiome and behavior of Arctic char (Salvelinus alpinus), a commercially farmed salmonid species.
Materials and Methods
In vitro Strain Characterization
Culture Conditions
In total, 10 strains were selected for the in vitro assays and probiotic feeding trial (Table 1). These included strains previously showing probiotic properties in fish (Glutamicibacter bergerei 04–279, Enterococcus thailandicus 04–394), isolated from the fish gut environment (P. acidilactici ASG16), or assigned to genera with known probiotic candidates and showing probiotic characteristics, such as antimicrobial activity (Carnobacterium divergens V41, Carnobacterium maltaromaticum SF1944, Vagococcus fluvialis CD264, Lactococcus lactis SF1945, Latilactobacillus sakei SF1583) or fermentative capacity of feed constituents (Lactiplantibacillus plantarum ISCAR-07436, Levilactobacillus brevis ISCAR-07433). Two successive cultures of each strain were performed in 1 ml of appropriate medium in 2 ml-deep well plates at 26°C for 24 h. The Lactiplantibacillus, Levilactobacillus, Latilactobacillus and Pediococcus strains were grown in MRS broth (De Man, Rogosa, Sharpe, Biokar Diagnostics, Beauvais, France). The Carnobacterium, Vagococcus, Lactococcus and Enterococcus strains were grown in BHI broth (Brain Heart Infusion, Biokar Diagnostic, Beauvais, France) and Glutamicibacter in Zobell broth (4 g/L Tryptone, 1 g/L yeast extract, and 33.3 g/L aquarium salts). All strains were stored at −80°C in 15% (v/v) glycerol.
Gastric Juice Resistance
Deep wells with 24 h-cultures of each strain were centrifuged at 2576 g for 15 min. The supernatant was removed and the cells were washed twice with 1 ml of physiological water using a pipetting robot (VIAFLO 96/384, Integra Biosciences, France). Pellets were then resuspended in 1 ml of phosphate buffer saline solution (PBS, 0.2 M at pH 6). In total, 180 μl of the simulated gastric juice (3 mg/ml of pepsin in 5 g/L of NaCl adjusted at pH 2.5) were distributed into each well of a microplate and inoculated with 20 μl of the PBS suspension of strains. The resistance of the strains was evaluated after 0, 3, and 5 h at 26°C using miniaturized enumeration, as previously described by Wiernasz et al. (2017), on YEG medium (10 g/L Yeast Extract supplemented with 10 g/L of glucose). The sensitivity toward gastric juice was assessed according to the decrease in viable cell concentration after 5 h (Δlog = log CFU/ml at t0h−log CFU/ml at t5h). Experiments were performed in triplicate.
Fish Bile Tolerance
Fish bile was collected from European hakes (Merluccius mercluccius) and devil anglerfishes (Lophius budegassa) during the French oceanographic cruise EVHOE in November 2019.1 Bile acid was retrieved from both species by puncturing the gallbladder with sterile needles and syringes. Pooled fish bile was then stored at −20°C until use.
The probiotic strains were cultured in triplicate and incubated at 26°C for 24 h to reach approximately 108 CFU/ml. A 20 μl of each culture was then inoculated into 180 μl of fish bile solutions diluted in culture media (BHI, MRS or Zobell) to reach a final concentration of 20% fish bile. After 24 h of incubation, cell counts were performed with a miniaturized enumeration as described above. Resistance to fish bile was assessed by the difference of viable cell concentrations after growth, with or without fish bile.
Auto-Aggregation Assay
Auto-aggregation was measured by sedimentation characteristics of the candidate strains according to the protocol described by Tareb et al. (2013) with some modifications. Probiotic strains were grown in duplicates in 15 ml of appropriate medium for 48 h at 26°C. A 1.5 ml of each culture was then centrifuged for 5 min at 16200 g. The cells were then washed twice using PBS solution (0.2 M, pH 6) and finally resuspended in PBS to reach an initial OD600nm of 0.3 (ODi). Tubes were incubated at 20°C for 24 h. The absorbance of the upper phase was then measured (OD24h). The percentage of auto-aggregation was calculated according to the following formula:
Antimicrobial Activities
Antimicrobial activities of probiotic strains were assessed using a miniaturized spot-on-lawn method as previously described (Begrem et al., 2020). Briefly, 10 μl of 24 h-probiotic strain cultures were spotted onto soft agar plates inoculated with one of 24 target strains involved in fish diseases, human infections or seafood spoilage (Supplementary Table S1). Culture medium, incubation temperature and inoculation type were adapted according to the target strains (Supplementary Table S1). After 24 to 48 h, clear halos indicated growth inhibition. Toxic activity of BHI and MRS media was excluded by spotting media alone on agar plates with target strains.
Hemolytic Activity
Probiotic strains were isolated on blood Columbia agar (VWR, France) and incubated at 26°C or 37°C for 72 h. The β-hemolytic Staphylococcus aureus (ATCC 25923) was used as positive control. β-hemolysis capacity led to the formation of a clear halo around a colony and partial α-hemolysis led to a greenish-brown halo.
Antibiotic Resistance
Antibiotic resistance of probiotic strains was assessed according to the standard method procedure ISO 10932 (2010) for antimicrobial profiles. The minimum inhibitory concentration (MIC) was determined for nine antibiotics as recommended by EFSA (2012a) with the following concentration ranges: ampicillin (0.125–64 μg/ml), chloramphenicol (0.125–64 μg/ml), clindamycin (0.125–64 μg/ml), erythromycin (0.125–64 μg/ml), gentamicin (1–512 μg/ml), kanamycin (4–2048 μg/ml), streptomycin (4–2048 μg/ml), tetracycline (0.25–128 μg/ml) and vancomycin (0.125–64 μg/ml). The tests were performed in duplicates with an initial concentration of approximately 105 CFU/ml. Microplates were incubated at 26°C for 24 h under aerobic conditions. MICs were determined by measuring the absorbance at 600 nm with a spectrophotometer (Varioskan Lux, Thermo Fisher, France). Strains was considered sensitive when absorbance was inferior to 0.3.
Feed Preparation and Application of Strains
Basal fish feed was prepared with 9.8% (w/w) fish oil (Laxá, Iceland), 70.3% (w/w) fish meal (Laxá, Iceland), 18.9% (w/w) gelatinized wheat (R2 Agro, Denmark), and 1% (w/w) Farmix mineral and vitamin premix (Trouw Nutrition, The Netherlands). Pellets were prepared as described in Leeper et al. (in press)2. In brief, all ingredients were mixed in a standard food mixer (KitchenAid, United States) with a small volume of water and pelleted using a FL82 meat grinder (ADE, Germany). Pelleted feed was then dried in a commercial food dryer (Kreuzmayr, Austria) to a moisture content of less than 10%. The pellet size was approximately 0.5 mm. Protein, fat, water and ash contents of the feed were measured at the analytical service laboratory at Matís, Iceland and were 51.1, 17.6, 6.5, and 10.3%, respectively.
For probiotic supplementation of the diet, each probiotic strain was grown in 50 ml of appropriate liquid culture media on a shaking plate until the late exponential phase. Feed pellets were coated with the strains using sterile spray bottles to achieve a final cell count of 106 CFU g−1 feed. The coated pellets were subsequently air dried in a laminar flow hood for 2 h under regular mixing and stored for no more than 2 weeks at 4°C.
Experimental Set-up
Arctic char fry with approximately 1 g body weight were purchased from a commercial hatchery and transferred to a 1 m3 holding tank with a continuous freshwater supply. After an acclimation period of 3 weeks, fish were randomly selected from the holding tank and distributed into 23 circular tanks with 46 fish per tank in total. Water was supplied to the tanks at a rate of 1.75 L min−1, exchanging the tank volume of 15 L approximately every 9 min and creating a circular water movement in the tank. Water temperature was continuously logged with iButton DS1922L temperature loggers (Maxim Integrated, United States) and remained between 7.8 and 9.4°C throughout the experiment. Dissolved oxygen remained above 99% and was measured regularly using an oxygen probe (Oxyguard, Denmark). After an additional acclimatizing period of 5 days, the feeding trial was started. Each tank was supplied with either one of the 10 probiotic diets (no replicates were conducted due to a limited number of tanks available) or a control diet (in triplicate) for 4 weeks, after which all tanks received the control diet for the remaining 4 weeks (for a timeline of the experiment see Supplementary Figure S1). The feed was supplied to the tanks six times per day using a conveyor belt set to feeding 6.5% of the fish body weight per day. This value was higher than commercial feeding recommendations in order to ensure maximum uptake of feed over the trial period. Tanks were cleaned regularly to prevent excessive biofilm growth on the tank walls. The experiment was performed according to European and Icelandic guidelines under the license FE-1134 from the Icelandic Food and Veterinarian Authority and UST201707 from the Icelandic Environment Agency.
Growth Assessment and Sample Collection
All fish were batch weighed at the start of the feeding trial, at week 4 and at the end of the trial at week 8. The fish were fasted for 12 h prior to weighing and sample collection. Before weighing, the fish were anesthetized with 350 ppm of phenoxyethanol, gently dried with paper tissue and weighed on a precision scale (Shinko Denshi, Japan). The specific growth rate (SGR) was calculated with the following formula:
For gut histology and metataxonomic analysis, five fish per tank were randomly selected at week 4 and week 8, and euthanized with 500 ppm of phenoxyethanol. Fish were then transported on ice to the laboratory, rinsed first with ethanol and then with sterile laboratory grade water to remove transient bacteria from the skin. The fish were dissected and the mid and hindgut was removed, cut into approximately 1 mm wide segments and frozen at −80°C until DNA extraction. One 1 mm segment from each hindgut was fixed in freshly prepared 4% paraformaldehyde in 1 × PBS for 24 h at 4°C and then transferred to 70% ethanol at 4°C for long-term storage prior to histology.
Histology and FISH
Gut histology and 16S rRNA fluorescence in situ hybridization (FISH) were performed for fish in treatment groups P1 and P8 after week 4 and week 8 to determine the location of viable probiotic cells in the digesta and mucosa of the hindgut. These two groups were selected due to their increased growth rate compared to the control group. Fixed gut segments were dehydrated in successive baths of 80, 90, 95, and 100% ethanol, followed by a bath in xylene and embedding in paraffin. Paraffin embedded gut segments were cut into 5 μm sections on a CM1800 microtome (Leica, Germany) with MX35 Ultra microtome blades (Thermo scientific, United States) and deparaffinized with xylene followed by a wash in 100% ethanol. Histological sections were hybridized with a mix of Cy3 labeled probes CDV175 and CDV462 (treatment P1) or LBP457 (treatment P8), as well as Alexa488 labeled universal bacterial probe EUB338 (Supplementary Table S2). Hybridization and epifluorescence microscopy was performed as previously described in Knobloch et al. (2019) with the exception of using 40% formamide in the hybridization buffer. Epifluorescence images were processed in daime v. 2.2 (Daims et al., 2006).
Gut Metataxonomic Analysis
DNA Extraction, PCR, and Sequencing
DNA was extracted from defrosted fish guts as previously described in Leeper et al. (2022) using the QIAamp PowerFecal Pro DNA Kit (Qiagen) with modifications. Two negative extraction control samples containing only zirconia/silica beads and CD1 buffer were included and processed alongside the other samples. All samples were subjected to PCR amplification and Illumina sequencing of the partial 16S rRNA gene as previously described in Knobloch et al. (2021) using the universal prokaryotic primer pair S-D-Bact-0341-b-S-17 (5’-CCTACGGGNGGCWGCAG-3′) /S-D-Bact-0785-a-A-21 (5’-GACTACHVGGGTATCTAATCC-3′; Klindworth et al., 2013) and high-fidelity Q5 polymerase (New England Biolabs, United States).
Microbial Community Analysis
Bioinformatic analysis and subsequent statistical analysis were performed in R version 4.0.2 (R Core Team, 2020) implemented in RStudio (RStudio Team, 2016). Amplicon sequence variants (ASVs) were inferred using the R package DADA2 version 1.16.0 (Callahan et al., 2016). In brief, raw reads were truncated after 260 bp (forward read) and 240 bp (reverse read) and primer sequences were removed. After denoising and merging the forward and reverse reads, ASVs within the size range of 410 to 460 bp were retained and assigned a taxonomic rank using the Silva v138 reference database (Quast et al., 2013). ASVs present in either one of the negative controls above 1% of the relative abundance were removed from the dataset. Microbial community analysis was performed in R package phyloseq (McMurdie and Holmes, 2013). Differential abundance analysis was performed with an R-implementation3 of ANCOM (Mandal et al., 2015) using the Benjamini–Hochberg procedure and an alpha of 0.05. ASVs were considered significantly more or less abundant at a detection cut-off of 0.7. Plots were created with package ggplot2 (Wickham, 2009).
Behavioral Analysis
Stress response was analyzed based on time spent moving in a confined space according to Øverli et al. (2006). At time T1, six fish were randomly selected from each tank and placed individually into a 1 L glass beaker filled with 300 ml of water. Each beaker was separated by an opaque screen in order to prevent the fish from observing each other. Overhead lighting was uniform across all beakers. An overhead camera recorded the movement between 0 to 10 min and 20 to 30 min after transfer to the confined space. Time spent moving was calculated using the software Solomon Coder version 19.08.02 (Péter, 2011). Movement was defined as active locomotion transporting the fish further than an estimated 10% of its body length or active swimming against the walls of the tank. Differences between treatments were analyzed by analysis of variance (ANOVA). Dunnett’s comparison was conducted as post-hoc test, as well as independently to detect significant differences between individual treatments and the control group according to Hothorn (2016).
Results
In vitro Characterization of Candidate Probiotic Strains
The probiotic potential of the 10 bacterial strains was evaluated in vitro, measuring resistance to fish digestive conditions and auto-aggregation required for intestinal colonization, as well as antimicrobial activities against aquaculture pathogens and other undesirable bacterial species. In addition, safety of the strains was assessed by hemolytic capacity and antibiotic resistance evaluation.
All strains displayed high resistance to gastric juice and bile acid, particularly C. divergens V41 (P1) and L. lactis SF1945 (P4) which showed less than log 0.4 CFU/ml inhibition against gastric juice and no inhibition against bile acid (Figure 1). Interestingly, V. fluvialis CD264 (P3) showed increased growth with fish bile compared to the control. The percentage of auto-aggregation ranged from 43 to 85%, with the highest values for Lactobacillus sakei SF1583 (P10) and P. acidilactici ASG16 (P3) with 85 ± 7% and 81 ± 0.2%, respectively. A comparison between strains showed no significant difference for gastric juice resistance (ANOVA: F(9, 20) = [1.58], p > 0.1), but significant differences for fish bile resistance (ANOVA: F(9, 20) = [7.51], p < 0.001) and auto-aggregation (ANOVA: F(9, 20) = [4.37], p < 0.005; Figure 1).
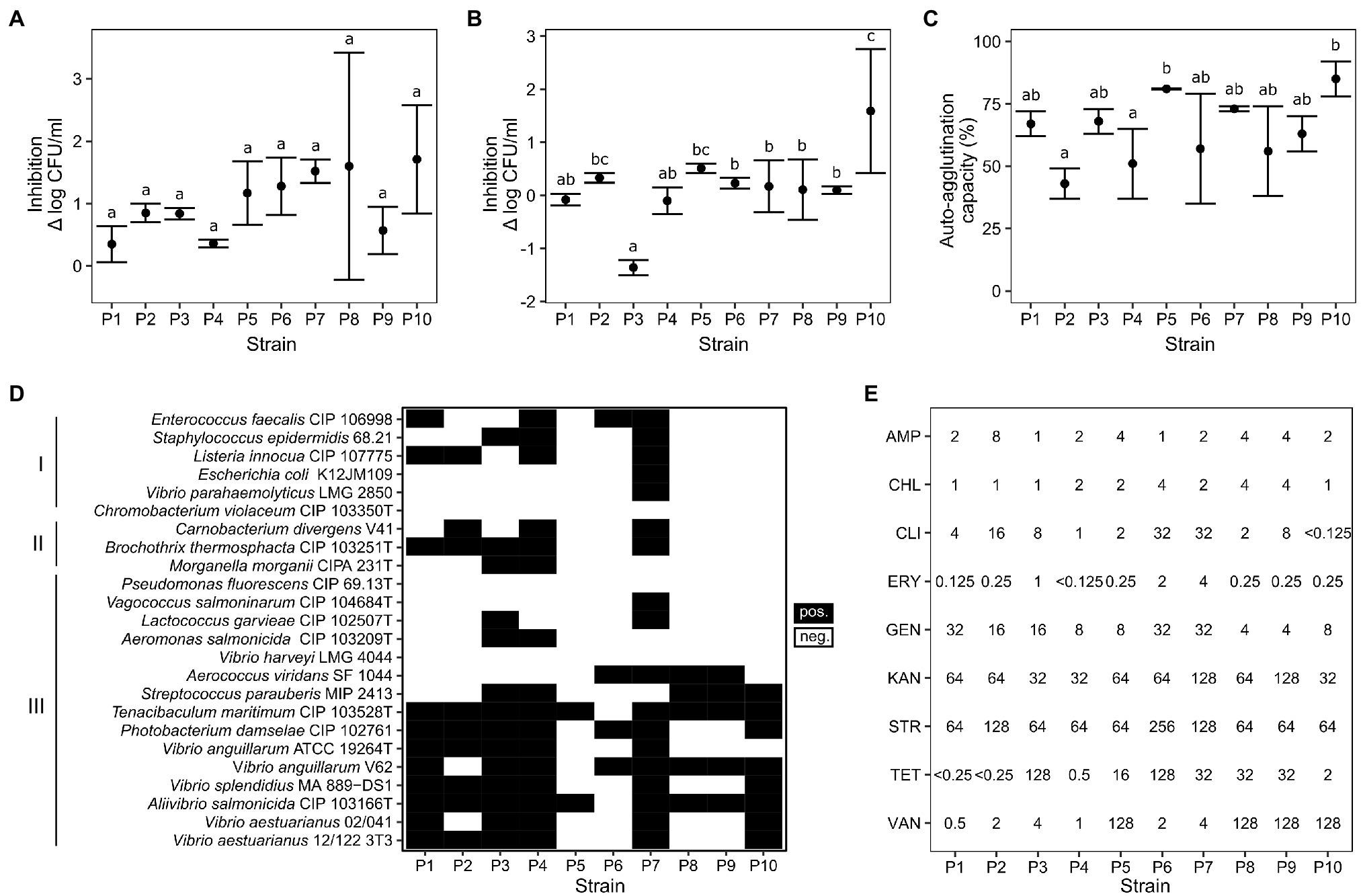
Figure 1. Results of in vitro assays. (A) Survival in simulated gastric juice after 5 h (ANOVA: F(9, 20) = [1.58], p > 0.1); (B) Survival in bile acid after 24 h (ANOVA: F(9, 20) = [7.51], p < 0.001); (C) Auto-aggregation capacity (ANOVA: F(9, 20) = [4.37], p < 0.005); (D) Antimicrobial activity profiles against bacteria associated with human infection (I), seafood spoilage (II), and fish diseases (III). (E) Minimal inhibitory concentrations against nine antibiotics in μg/ml (AMP: ampicillin, CHL: chloramphenicol, CLI: clindamycin, ERY: erythromycin, GEN: gentamicin, KAN: kanamycin, STR: streptomycin, TET: tetracycline, VAN: vancomycin). See selected EFSA cut-off values in Supplementary Table S3. P1: Carnobacterium divergens; P2: Carnobacterium maltaromaticum; P3: Vagococcus fluvialis; P4: Lactococcus lactis; P5: Pediococcus acidilactici; P6: Glutamicibacter bergerei; P7: Enterococcus thailandicus; P8: Lactiplantibacillus plantarum; P9: Levilactobacillus brevis; P10: Latilactobacillus sakei. Letters above error bars indicate significant differences (p < 0.05) between samples based on Tukey’s HSD post-hoc test.
Antimicrobial activity profiles were strain-dependent. Except P. acidilactici ASG16 and Arthrobacter bergerei 04–279, all LAB strains showed antimicrobial properties against the tested aquaculture pathogens (Figure 1). The Carnobacterium strains (P1, P2), L. lactis SF1945 (P4) and E. thailandicus 04–394 (P7) were also able to inhibit seafood spoilage organisms and Listeria sp.
No definite hemolytic activities were detected except for light green halos for V. fluvialis CD264 (P3) and L. sakei SF1583 (P10) which could possibly be related to H2O2 production (data not shown). Due to a lack of reported threshold values it was not possible to establish a resistance or sensitivity phenotype with high confidence for the tested strains. However, the resistance of the Lactiplantibacillus sp., L. lactis and the Pediococcus sp. can be assessed by comparison to EFSA guidelines available only for these feed additives species (Supplementary Table S3; EFSA, 2012a). The strain L. sakei SF1583 (P10) was found susceptible to all nine antibiotics. The obligate heterofermentative L. brevis (P9) seemed to display kanamycin and clindamycin resistances with MICs of 128 μg/ml and 8 μg/ml respectively, much higher than the EFSA cut-off values of 32 μg/ml and 1 μg/ml. The other strains showed intermediate resistance to streptomycin for L. lactis SF1945 (P4), tetracycline for P. acidilactici ASG16 (P5) and ampicillin for L. plantarum ISCAR-07436 (P8), with MIC values close to the reported cut-off values.
Fish Growth and Survival
The average SGR of Arctic char fry fed with the control diet was 2.36 ± 0.01% during the first 4 weeks of the trial (Figure 2). Only the group P1, supplemented with C. divergens V41, and P8, supplemented with L. plantarum ISCAR-07436, had a higher SGR of 2.47 and 2.40%, respectively. One-sample t-tests showed that the control group was either significantly higher or lower (p < 0.05) than each of the treatment groups apart from group P5, supplemented with P. acidilactici ASG16. Between week 4 and 8 of the growth trial, the average SGR of the control group was 2.08 ± 0.06%. All groups, apart from P9, previously fed with L. brevis ISCAR-07433, had a higher growth rate than the control group during this period. However, the control only significantly differed from treatment groups P2, P4 and P5. Across the entire 8-week growth period only groups P1, P5 and P8, with an SGR of with 2.36, 2.34 and 2.34%, respectively, had a higher SGR than the control group, which was significantly lower with an SGR of 2.24 ± 0.03%. The control group was only significantly higher than treatment group P9, which showed the slowest growth performance over the whole growth trial. No mortality occurred due to the treatments throughout the 8-week experiment.
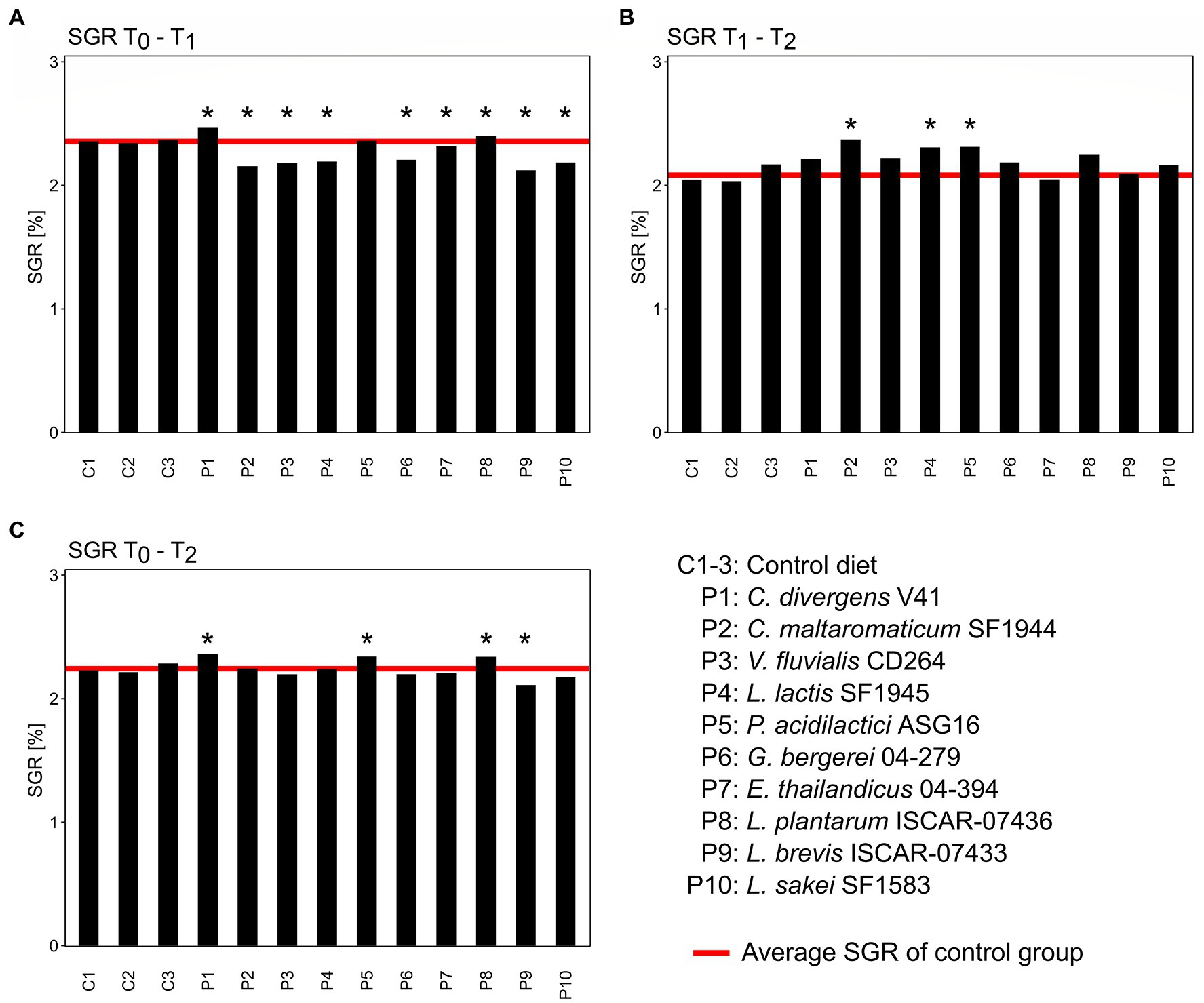
Figure 2. Specific growth rates (SGR) of the different treatment groups (P1–P10) compared to the control (C1–C3) between the first 4-week growth period (A), the second 4-week growth period (B) and the entire 8-week growth period (C). Asterisks above bars indicate significant difference of the control groups to the treatment group based on one-sample t-tests (p < 0.05).
Gut Microbiota
The gut microbiota of fish in the control group was dominated by an ASV assigned to the genus Mycoplasma, accounting for over 67% of the average relative abundance at time T1 and T2 (Figure 3A). The second and third most abundant ASVs were assigned to the genus Brevinema and to the family Ruminococcaceae, respectively. Although the relative abundance of both taxa varied strongly between samples, together they accounted for an average of 23% of the relative abundance in the control group across times T1 and T2. Each of the other taxa detected contributed less than 1% of the average relative abundance of the fish gut microbiota and were not present in all examined individuals.
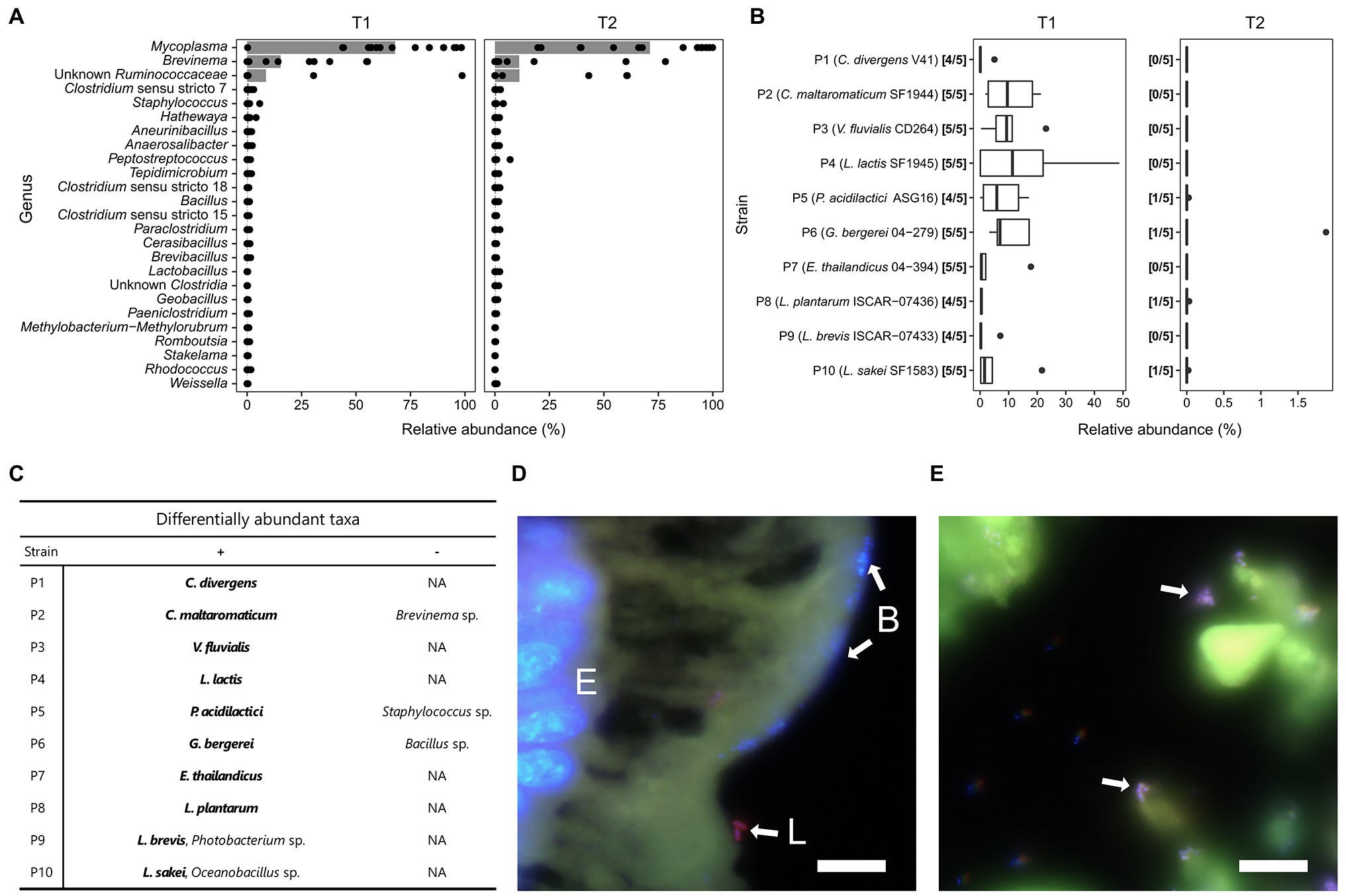
Figure 3. Description of Arctic char gut microbiota. (A) Relative abundance of genera (or higher taxon if genus is unknown) in fish guts of the control group (n = 15) at time T1 and T2. Gray bars indicate average relative abundance; (B) Relative abundance of probiotic strains detected in fish guts of the treatment groups (n = 5) at time T1 and T2. Positive detection was assumed if the 16S rRNA gene sequence matched the reference 16S rRNA gene of the respective probiotic strains with 100% sequence similarity; (C) Differentially abundant taxa for each treatment group with significantly higher (+) or lower (−) relative abundance compared to the control group. Probiotic strains are marked in bold. (D) FISH image of gut epithelium of treatment group P8 with nuclei of enterocytes (E) and other bacteria (B) stained blue (DAPI), and L. plantarum cells (L.) stained purple (DAPI + Cy3 + Alexa488). Bar: 10 μm; (E) FISH image of intestinal lumen of treatment group P1 with C. divergens cells marked purple (DAPI + Cy3 + Alexa488). Bar: 10 μm.
In the groups receiving feed supplemented with the probiotic strains, the percentage of each respective strain detected in the gut varied strongly by the individual examined and by the strain applied (Figure 3B). At time T1, the highest average relative abundances were found for P4, P2, P6, P3 and P5 with 16.4, 10.7, 10.1, 9.9 and 7.5%, respectively. In addition, the strains were not detected in all fish fed with the probiotics. One out of five individuals fed with either P1, P5, P8 and P9 did not harbor detectable levels of the corresponding strain at the time of sampling. At time T2, after being fed the control feed without probiotics for 4 weeks, only four strains were detected in one out of five individuals per treatment with 0.02 to 1.9% of the relative abundance (Figure 3B).
Although present in the fish gut until T1, the strains did not have a large impact on the abundance of the three dominant taxa found in the gut microbiome (Figure 3C). Only the group fed with P2 showed a significant reduction of Brevinema compared to the control group. Fish fed with P5 and P6 showed a significant reduction of the minor taxa Staphylococcus and Bacillus, respectively. Those fed with P9 and P10 harbored a higher proportion of Photobacterium and Oceanobacillus, respectively, compared to the control group.
FISH imaging of hindgut sections from fish supplemented with C. divergens V41 and L. plantarum ISCAR-07436 showed that intact cells were found exclusively outside of the mucus, compared to dense clusters of bacterial cells, likely belonging to the dominant Mycoplasma sp., found within the mucus layer of the fish gut epithelium (Figures 3D,E). No cells marked with C. divergens or L. plantarum specific probes were detected in fish gut section from time T2.
Behavioral Analysis
Behavior analysis was based on locomotive activity in a confined space by individuals in the control group (n = 18) compared to individuals in each of the other treatment groups (n = 6 per group). There was a significant effect of treatment in the first observation period based on ANOVA (F(10, 67) = 2.05, p = 0.04). Dunnett’s test indicated that the mean time spent moving was significantly different (Figure 4, p = 0.014) between the control group (255.3 ± 198.4 s) and the treatment group P7 receiving E. thailandicus 04–394 (543.6 ± 45.8 s). Average time spent moving was more similar between the control and treatment groups during the second observation period without significant differences between treatments based on ANOVA. However, Dunnett’s test showed a significant difference (p = 0.036) of the time spent moving between the control group (260.9 ± 140.9 s) and treatment group P9, previously fed with L. brevis ISCAR-07433 (479.9 ± 92.2 s).
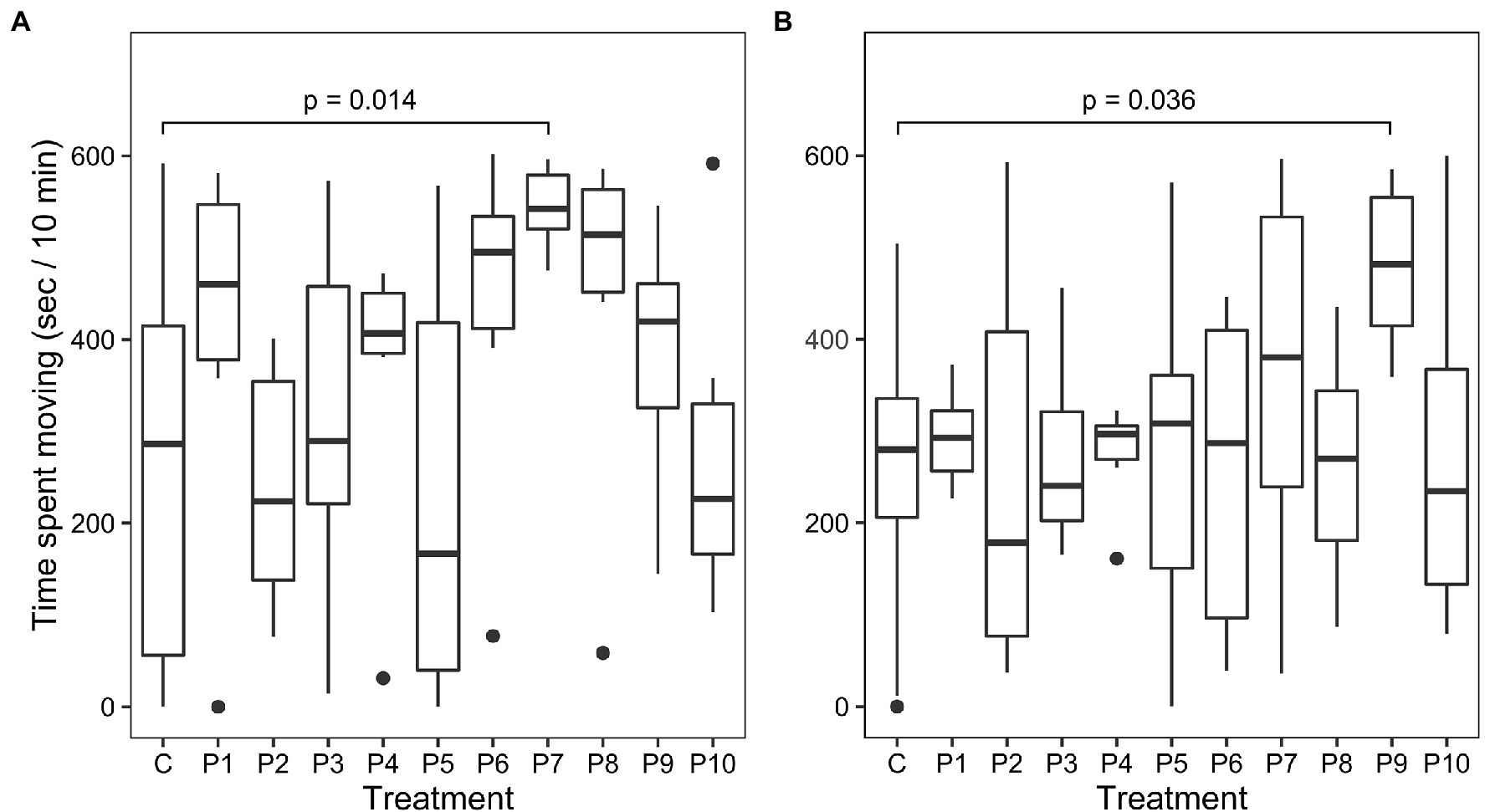
Figure 4. Time spent moving of control (C) and treatment groups (P1–P10) during confinement. (A) 0–10 min after transfer to confinement. (B) 20–30 min after transfer to confinement.
Discussion
In vitro assays can be useful for pre-selection of strains with putative probiotic properties in vivo (Vine et al., 2004a; Guo et al., 2010; Vinderola et al., 2017). In this study, no strain showed complete inhibition by gastric juice or bile acid indicating their survival in the fish gastrointestinal system. Strains C. divergens V41 and L. lactis SF1945 displayed the highest resistance in both tests making them highly suitable probiotic candidates in this regard. Average auto-aggregation capacity varied but remained above 40% for all strains. Previous studies on auto-aggregation of human and animal probiotic candidates showed similar values (Tareb et al., 2013; Krausova et al., 2019), indicating that all candidate strains could have the potential to adhere to epithelial cells, particularly Latilactobacillus sakei SF1583 and P. acidilactici ASG16 which displayed the highest values. However, as reported in Ouwehand and Salminen (2009), in vivo assays are needed to validate this hypothesis. Antimicrobial activity against specific pathogens and spoilage organisms was present in all strains, showing potential beneficial characteristics for application in aquaculture (Pan et al., 2008; Muñoz-Atienza et al., 2013; Yi et al., 2018). Antibiotic assays and hemolytic activities provided safety assessment of strains. These results can be useful for the Qualified Presumption of Safety (QPS) status of LAB deliberately introduced into the feed chain. No clear hemolytic activities were observed. However, the results seemed to show some antibiotic resistances. Among the LAB species with known cut-off values (EFSA, 2012a), L. brevis ISCAR-07433 is the only strain that probably harbors antibiotic resistance to clindamycin and kanamycin. Other putative resistances were observed for L. lactis SF1945, P. acidilactici ASG16 and L. plantarum ISCAR-07436. For strains without existing threshold values, the MICs of a large number of isolates should help to determine the cut-off values. Genome sequencing and analysis would be required to identify resistance genes and their transferability by mobile genetic elements and to further assess the risks of conferring resistance to fish and humans.
The SGR reported in this study is in good accordance with previous studies on growth rates of juvenile Arctic char for similar age groups (Jobling et al., 1993; Yang and Dick, 1994; Gunnarsson et al., 2011). Supplementation of probiotic strains impacted growth both throughout the treatment period (week 1 to 4) as well as after supplementation had ended (week 5 to 8). Fish fed with C. divergens V41 and with L. plantarum ISCAR-07436 showed an increased growth rate during the 4-week treatment period which led to a slightly increased growth rate over the total 8-week growth trial compared to the control group. Probiotics have previously been shown to increase the growth rate of farmed fish species as, for instance, in juvenile Labeo rohita fed with a combination of three probiotics (Bacillus subtilis, L. lactis and Saccharomyces cerevisiae; Mohapatra et al., 2012), in Oreochromis niloticus fed with the yeast S. cerevisiae (Lara-Flores et al., 2003), or in Paralichythys olivaceus fed with a strain of L. lactis (Nguyen et al., 2018). According to these studies, the impact on growth could be the result of microbial metabolites or the enzymatic digestion of feed constituents, chiefly proteins, aided by live probiotics. This, in turn, could lead to higher nutrient digestion and utilization by the host. The study by Nguyen et al. (2018) compared the gut metabolome of fish fed with and without probiotics showing that the gut environment of the probiotic-fed group was significantly enriched in metabolites such as citrulline, tricarboxylic acid cycle intermediates, short chain fatty acids, vitamins and taurine which could aid in host nutrition. Both Carnobacterium spp. and Lactiplantibacillus spp. have previously been isolated from the gut of different fish species and have shown proteolytic and lipolytic activity in vitro (Sahnouni et al., 2016). Hence the observed growth rate increase detected in this study could be the result of increased nutrient availability. The indication that the weight increase was sustained even after probiotic supplementation was terminated shows that even short-term application of these strains could promote a growth advantage during the juvenile development stage. The group fed with P. acidilactici ASG16 did not show an increased growth rate until the second half of the trial when the probiotic treatment was not applied anymore, indicating that the strain did not actively increase nutrient availability to the host. P. acidilactici is one of the most widely studied probiotic bacteria for fish and attributes of P. acidilactici supplementation include the reduction of bone deformation in developing fish (EFSA, 2012b), increased growth performance (Ashouri et al., 2018; Arani et al., 2020), increased stress resistance (Taridashti et al., 2017) and enhanced disease resistance or mucosal immune response (Rahimnejad et al., 2018; Hoseinifar et al., 2019). Whereas it is likely that no active nutritional benefit was gained through P. acidilactici in this study, it is possible that other beneficial properties of the strain conferred a health benefit in the host resulting in increased growth in the long term.
The other seven strains tested in this study did not promote growth benefits and even appeared to reduced growth during the treatment period. Most studies published on the effect of putative probiotic in farmed fish report positive correlations between probiotic supplementation and growth rates (Ringø et al., 2020). However, Hoseinifar et al. (2019) and Batista et al. (2016) showed significantly decreased growth rates in fish fed with a P. acidilactici strain and a multispecies probiotic, respectively, and several studies have shown a correlation between differences in the gut microbial composition and reduced growth (Chapagain et al., 2019; Jin et al., 2021). The reasons for decreased growth in this study are not clear. Four of the seven strains that induced slower growth rates in the fish were also detected at higher relative abundances in the gut, suggesting rapid growth of the strains in vivo. This could have led to, for instance, competition for nutrients otherwise available to the host, changes of the pH in the gut environment impairing nutrient absorption, crowding out of resident bacteria or causing local inflammation and other host immune reactions. Despite low growth while being fed the bacterial strains, six of the groups could regain their weight relative to the control group during the second period of the trial through compensatory growth. This phenomenon has previously been detected in Arctic char transferred to warm water after a period in suboptimal rearing in cold water (Mortensen and Damsgård, 1993). Only the group fed with L. brevis ISCAR-07433 showed a continuously decreased growth rate throughout the experiment, albeit without any cases of mortality. Future studies might focus on the cause of this interaction to elucidate bacterial characteristic to avoid when screening for growth enhancing probiotic strains for salmonid fish.
The gut microbiota of the juvenile Arctic char was dominated by three bacterial taxa, a Mycoplasma sp., Brevinema sp. and an unknown Ruminococcaceae. Mycoplasma has previously been described as a gut commensal across different wild and farmed salmonid species with potential mutualistic properties (Llewellyn et al., 2015; Mora-Sánchez et al., 2020; Rasmussen et al., 2021), suggesting an important association with its host in the present study. The sole cultivated member of the genus Brevinema is B. andersonii, an infectious spirochete (Defosse et al., 1995). However, less than 91% sequence similarity to this type species indicates that the bacterium detected in the Arctic char guts could belong to a separate genus. A sequence comparison to the NCBI nucleotide collection showed highest sequence similarity of the partial 16S rRNA gene to an uncultured bacterium detected in the Long-Jawed Mudsucker, Gillichthys mirabilis (96.3% sequence similarity), followed by a strain detected in the herbivorous marine fish Naso tonganus (96.1%), further highlighting its association with the gut of fish species. Likewise, the unknown Ruminococcaceae showed highest sequence similarity to an isolate from a fish species (Siganus canaliculatus) with 90.3% sequence similarity. The low abundance of the other taxa detected in the gut suggests that many are transient microorganisms and not members of the autochthonous gut microbiota. This is the first study characterizing the gut microbiota of farmed Arctic char. Compared to previous studies on wild Arctic char, the farmed fish appear to harbor a lower number of permanent members of the gut microbiota, but partially share the presence and high relative abundance of Mycoplasma and Brevinema (Hamilton et al., 2019; Element et al., 2020, 2021).
Probiotic treatment did not affect the relative abundance of the three dominant taxa in this study, with the exception of C. maltaromaticum SF1944 which was associated with a significantly reduced relative abundance of the Brevinema sp., possibly due to competition for the same niche. Brevinema, as well as Staphylococcus and Bacillus, the other two taxa with a significant reduction in abundance after probiotic treatment, have all been previously detected in farmed fish gut microbiomes, sometimes representing over a quarter of the relative community abundance (Parata et al., 2020; Skrodenytė-Arbačiauskienė et al., 2021; Leeper et al., 2022) and therefore likely benign to the host organism.
Overall, these results highlight the stability of the autochthonous gut microbiota toward perturbation by exogenous microorganisms. This is in contrast to previous studies which have shown that probiotic bacteria modulate the fish gut microbiome (Geraylou et al., 2013; Ramos et al., 2013; Lobo et al., 2014). This difference might be due the limited number of core gut microorganisms found in the juvenile Arctic char and therefore a lack of metabolic or functional redundancy which, in turn, necessitates a stable microbial community. This might also explain a lack of long-term survival of the strains in the gut as there may not have been a suitable niche for colonization. However, the presence of intact cells of C. divergens and L. plantarum in the gut, detected through FISH, indicates survival of these strains in the gut environment and the possibility of active metabolism. C. divergens has previously been detected in the gut of Arctic char (Ringø et al., 2006) which further points toward a functional, possibly mutualistic, role in the gut.
As in other animals, there is mounting evidence that the gut microbiome can modulate the behavior of fish via the gut-brain-axis and that probiotics can influence this interaction (Borrelli et al., 2016; Davis et al., 2016a,b). The current study shows first evidence of bacterial strains altering the stress response in a farmed salmonid. Whereas most strains did not show a significant difference in motility under confinement stress, fish fed with E. thailandicus 04–394 and L. brevis ISCAR-07433 showed significantly increased motility compared to the control group and thereby lower stress coping ability. It is not clear what mechanism triggers this response, but both treatments were also associated with slower growth during the treatment period and for L. brevis ISCAR-07433 even sustained slow growth after the treatment period had ended. Gut inflammation was not analyzed in this study, however, previous studies on mouse models have shown a correlation between inflammation and behavioral changes (Bercik et al., 2010; Guida et al., 2018). Hence, the E. thailandicus and L. brevis strains could have caused local immune responses in the gut which, in turn, could have led to inflammation, reduced nutrient absorption and behavioral changes.
Conclusion
Screening 10 bacterial strains for probiotic properties demonstrated both beneficial and adverse effects on host growth and behavior in juvenile Arctic char. In vitro assays can aid in the pre-selection of probiotic strains based on strain characteristics and safety status, however, there was no clear correlation between in vitro results and in vivo performances in this study. Based on small-scale growth trials, three putative probiotics, C. divergens V41, L. plantarum ISCAR-07436 and P. acidilactici ASG16, were highlighted as promising strains with the ability to enhance growth performance in juvenile Arctic char without altering behavioral characteristics or the gut microbiota. Moreover, C. divergens and L. plantarum are on the QPS list, with some strains currently being commercialized in food applications. Further studies are needed to analyze the benefits of these probiotic treatments during different growth stages and in different salmonid species, as well as their impact on performance throughout the entire rearing period. In-depth analysis of the metabolic potential of these strains in vivo will elucidate the beneficial interactions within the host organism and the underlying mechanisms of growth enhancement.
Data Availability Statement
Raw 16S rRNA gene sequences are deposited in the NCBI Sequence Read Archive under BioProject PRJNA791800.
Ethics Statement
The animal study was reviewed and approved by The Icelandic Food and Veterinarian Authority under licence FE-1134 and UST201707 from the Icelandic Environment Agency.
Author Contributions
SK, SS, LK, FL, DP, and VM contributed to the conception and design of the study. SK, SS, MD, and AL conducted field and laboratory work. AL provided expert advice on feed production and feeding trials. SK wrote the first draft of the manuscript. LK and DP wrote sections of the manuscript. All authors contributed to the article and approved the submitted version.
Funding
This study was supported by the EU H2020 Research and Innovation program (grant no. 818368) and the Icelandic AVS fund (grant R 17 018-17).
Conflict of Interest
The authors declare that the research was conducted in the absence of any commercial or financial relationships that could be construed as a potential conflict of interest.
Publisher’s Note
All claims expressed in this article are solely those of the authors and do not necessarily represent those of their affiliated organizations, or those of the publisher, the editors and the reviewers. Any product that may be evaluated in this article, or claim that may be made by its manufacturer, is not guaranteed or endorsed by the publisher.
Acknowledgments
We would like to thank Céline Garcia (LGPMM laboratory of IFREMER, La Tremblade, France) for providing indicator strains used in this study.
Supplementary Material
The Supplementary Material for this article can be found online at: https://www.frontiersin.org/articles/10.3389/fmicb.2022.912473/full#supplementary-material
Footnotes
1. ^http://dx.doi.org/10.17600/18000878
2. ^Leeper, A., Benhaïm, D., Smárason, B., Knobloch, S., Ómarsson, K., Bonnafoux, T., et al. (in press). Feeding black soldier Fly larvae (Hermetia illucens) reared on food waste alters gut characteristics of Atlantic salmon (Salmo salar). J. Insects as Food Feed.
References
Abumourad, I. M. K., Abbas, W. T., Awaad, E. S., Authman, M. M. N., El-Shafei, K., Sharaf, O. M., et al. (2013). Evaluation of Lactobacillus plantarum as a probiotic in aquaculture: emphasis on growth performance and innate immunity. J. Appl. Sci. Res. 9, 572–582.
Arani, M. M., Salati, A. P., Keyvanshokooh, S., and Safari, O. (2020). The effect of Pediococcus acidilactici on mucosal immune responses, growth, and reproductive performance in zebrafish (Danio rerio). Fish Physiol. Biochem. 471, 153–162. doi: 10.1007/S10695-020-00903-8
Ashley, P. J. (2007). Fish welfare: current issues in aquaculture. Appl. Anim. Behav. Sci. 104, 199–235. doi: 10.1016/J.APPLANIM.2006.09.001
Ashouri, G., Mahboobi Soofiani, N., Hoseinifar, S. H., Jalali, S. A. H., Morshedi, V., Van Doan, H., et al. (2018). Combined effects of dietary low molecular weight sodium alginate and Pediococcus acidilactici MA18/5M on growth performance, haematological and innate immune responses of Asian sea bass (Lates calcalifer) juveniles. Fish Shellfish Immunol. 79, 34–41. doi: 10.1016/J.FSI.2018.05.009
Bagheri, T., Hedayati, S. A., Yavari, V., Alizade, M., and Farzanfar, A. (2008). Growth, survival and gut microbial load of rainbow trout (Onchorhynchus mykiss) fry given diet supplemented with probiotic during the two months of first feeding. Turk. J. Fish. Aquat. Sci. 8, 43–48.
Balcázar, J. L., De Blas, I., Ruiz-Zarzuela, I., Cunningham, D., Vendrell, D., and Múzquiz, J. L. (2006). The role of probiotics in aquaculture. Vet. Microbiol. 114, 173–186. doi: 10.1016/J.VETMIC.2006.01.009
Banerjee, G., and Ray, A. K. (2017). The advancement of probiotics research and its application in fish farming industries. Res. Vet. Sci. 115, 66–77. doi: 10.1016/J.RVSC.2017.01.016
Batista, S., Medina, A., Pires, M. A., Moriñigo, M. A., Sansuwan, K., Fernandes, J. M. O., et al. (2016). Innate immune response, intestinal morphology and microbiota changes in Senegalese sole fed plant protein diets with probiotics or autolysed yeast. Appl. Microbiol. Biotechnol. 10016, 7223–7238. doi: 10.1007/S00253-016-7592-7
Begrem, S., Ivaniuk, F., Gigout-Chevalier, F., Kolypczuk, L., Bonnetot, S., Leroi, F., et al. (2020). New insight into antimicrobial compounds from food and marine-sourced Carnobacterium species through phenotype and genome analyses. Microorganisms 8:1093. doi: 10.3390/microorganisms8071093
Bercik, P., Verdu, E. F., Foster, J. A., MacRi, J., Potter, M., Huang, X., et al. (2010). Chronic gastrointestinal inflammation induces anxiety-Like behavior and alters central nervous system biochemistry in mice. Gastroenterology 139, 2102–2112.e1. doi: 10.1053/J.GASTRO.2010.06.063
Borrelli, L., Aceto, S., Agnisola, C., De Paolo, S., Dipineto, L., Stilling, R. M., et al. (2016). Probiotic modulation of the microbiota-gut-brain axis and behaviour in zebrafish. Sci. Report. 61, 1–9. doi: 10.1038/srep30046
Callahan, B. J., McMurdie, P. J., Rosen, M. J., Han, A. W., Johnson, A. J. A., and Holmes, S. P. (2016). DADA2: high-resolution sample inference from Illumina amplicon data. Nat. Methods 13, 581–583. doi: 10.1038/nmeth.3869
Chang, C.-I., and Liu, W.-Y. (2002). An evaluation of two probiotic bacterial strains, Enterococcus faecium SF68 and Bacillus toyoi, for reducing edwardsiellosis in cultured European eel, Anguilla anguilla L. J. Fish Dis. 25, 311–315. doi: 10.1046/J.1365-2761.2002.00365.X
Chapagain, P., Arivett, B., Cleveland, B. M., Walker, D. M., and Salem, M. (2019). Analysis of the fecal microbiota of fast- and slow-growing rainbow trout (Oncorhynchus mykiss). BMC Genomics 201, 1–11. doi: 10.1186/S12864-019-6175-2
Daims, H., Lücker, S., and Wagner, M. (2006). Daime, a novel image analysis program for microbial ecology and biofilm research. Environ. Microbiol. 8, 200–213. doi: 10.1111/j.1462-2920.2005.00880.x
Davis, D. J., Bryda, E. C., Gillespie, C. H., and Ericsson, A. C. (2016a). Microbial modulation of behavior and stress responses in zebrafish larvae. Behav. Brain Res. 311, 219–227. doi: 10.1016/J.BBR.2016.05.040
Davis, D. J., Doerr, H. M., Grzelak, A. K., Busi, S. B., Jasarevic, E., Ericsson, A. C., et al. (2016b). Lactobacillus plantarum Attenuates anxiety-related behavior and protects against stress-induced dysbiosis in adult zebrafish. Sci. Report. 6, 1–11. doi: 10.1038/srep33726
Dawood, M. A. O., Magouz, F. I., Salem, M. F. I., and Abdel-Daim, H. A. (2019). Modulation of digestive enzyme activity, blood health, oxidative responses and growth-related gene expression in GIFT by heat-killed Lactobacillus plantarum (L-137). Aquaculture 505, 127–136. doi: 10.1016/J.AQUACULTURE.2019.02.053
Defosse, D. L., Johnson, R. C., Paster, B. J., Dewhirst, F. E., and Fraser, G. J. (1995). Brevinema andersonii gen. Nov., sp. nov., an infectious spirochete isolated from the short-tailed shrew (Blarina brevicauda) and the White-footed mouse (Peromyscus leucopus). Int. J. Syst. Evol. Microbiol. 45, 78–84. doi: 10.1099/00207713-45-1-78
EFSA (2012a). Guidance on the assessment of bacterial susceptibility to antimicrobials of human and veterinary importance. EFSA J. 10:2740. doi: 10.2903/j.efsa.2012.2740
EFSA (2012b). Scientific opinion on the efficacy of Bactocell (Pediococcus acidilactici) when used as a feed additive for fish. EFSA J. 10:2886. doi: 10.2903/j.efsa.2012.2886
Element, G., Engel, K., Neufeld, J. D., Casselman, J. M., van Coeverden de Groot, P., Greer, C. W., et al. (2020). Seasonal habitat drives intestinal microbiome composition in anadromous Arctic char (Salvelinus alpinus). Environ. Microbiol. 22, 3112–3125. doi: 10.1111/1462-2920.15049
Element, G., Engel, K., Neufeld, J. D., Casselman, J. M., Van Coeverden De Groot, P. J., and Walker, V. K. (2021). Distinct intestinal microbial communities of two sympatric anadromous arctic salmonids and the effects of migration and feeding. Arct. Sci. 7, 634–654. doi: 10.1139/AS-2020-0011/SUPPL_FILE/AS-2020-0011SUPPLA.DOCX
FAO (2018). The State of World Fisheries and Aquaculture 2018-Meeting the Sustainable Development Goals. FAO Rome, Italy.
Fečkaninová, A., Koščová, J., Mudroňová, D., Popelka, P., and Toropilová, J. (2017). The use of probiotic bacteria against Aeromonas infections in salmonid aquaculture. Aquaculture 469, 1–8. doi: 10.1016/J.AQUACULTURE.2016.11.042
Gatesoupe, F.-J. (1994). Lactic acid bacteria increase the resistance of turbot larvae, Scophthalmus maximus, against pathogenic vibrio. Aquat. Living Resour. 7, 277–282. doi: 10.1051/ALR:1994030
Gatesoupe, F. J. (1999). The use of probiotics in aquaculture. Aquaculture 180, 147–165. doi: 10.1016/S0044-8486(99)00187-8
Geraylou, Z., Souffreau, C., Rurangwa, E., De Meester, L., Courtin, C. M., Delcour, J. A., et al. (2013). Effects of dietary arabinoxylan-oligosaccharides (AXOS) and endogenous probiotics on the growth performance, non-specific immunity and gut microbiota of juvenile Siberian sturgeon (Acipenser baerii). Fish Shellfish Immunol. 35, 766–775. doi: 10.1016/J.FSI.2013.06.014
Guida, F., Turco, F., Iannotta, M., De Gregorio, D., Palumbo, I., Sarnelli, G., et al. (2018). Antibiotic-induced microbiota perturbation causes gut endocannabinoidome changes, hippocampal neuroglial reorganization and depression in mice. Brain Behav. Immun. 67, 230–245. doi: 10.1016/J.BBI.2017.09.001
Gunnarsson, S., Imsland, A. K., Árnason, J., Gústavsson, A., Arnarson, I., Jónsson, J. K., et al. (2011). Effect of rearing temperatures on the growth and maturation of Arctic charr (Salvelinus alpinus) during juvenile and on-growing periods. Aquac. Res. 42, 221–229. doi: 10.1111/j.1365-2109.2010.02615.x
Guo, X. H., Kim, J. M., Nam, H. M., Park, S. Y., and Kim, J. M. (2010). Screening lactic acid bacteria from swine origins for multistrain probiotics based on in vitro functional properties. Anaerobe 16, 321–326. doi: 10.1016/J.ANAEROBE.2010.03.006
Hai, N. V. (2015). The use of probiotics in aquaculture. J. Appl. Microbiol. 119, 917–935. doi: 10.1111/JAM.12886
Hamilton, E. F., Element, G., van Coeverden de Groot, P., Engel, K., Neufeld, J. D., Shah, V., et al. (2019). Anadromous arctic char microbiomes: bioprospecting in the high arctic. Front. Bioeng. Biotechnol. 7:32. doi: 10.3389/FBIOE.2019.00032/BIBTEX
Hoseinifar, S. H., Hosseini, M., Paknejad, H., Safari, R., Jafar, A., Yousefi, M., et al. (2019). Enhanced mucosal immune responses, immune related genes and growth performance in common carp (Cyprinus carpio) juveniles fed dietary Pediococcus acidilactici MA18/5M and raffinose. Dev. Comp. Immunol. 94, 59–65. doi: 10.1016/J.DCI.2019.01.009
Hothorn, L. A. (2016). The two-step approach—a significant ANOVA F-test before Dunnett’s comparisons against a control—is not recommended. Commun. Stat. - Theory Methods 45, 3332–3343. doi: 10.1080/03610926.2014.902225
Irianto, A., and Austin, B. (2002). Use of probiotics to control furunculosis in rainbow trout, Oncorhynchus mykiss (Walbaum). J. Fish Dis. 25, 333–342. doi: 10.1046/J.1365-2761.2002.00375.X
ISO 10932 (2010). Milk and milk products — Determination of the minimal inhibitory concentration (MIC) of antibiotics applicable to bifidobacteria and non-enterococcal lactic acid bacteria (LAB). Geneva. Available at: https://www.iso.org/standard/46434.html [Accessed March 18, 2021].
Jang, W. J., Lee, J. M., Hasan, M. T., Lee, B. J., Lim, S. G., and Kong, I. S. (2019). Effects of probiotic supplementation of a plant-based protein diet on intestinal microbial diversity, digestive enzyme activity, intestinal structure, and immunity in olive flounder (Paralichthys olivaceus). Fish Shellfish Immunol. 92, 719–727. doi: 10.1016/J.FSI.2019.06.056
Jha, D. K., Bhujel, R. C., and Anal, A. K. (2015). Dietary supplementation of probiotics improves survival and growth of Rohu (Labeo rohita ham.) hatchlings and fry in outdoor tanks. Aquaculture 435, 475–479. doi: 10.1016/J.AQUACULTURE.2014.10.026
Jin, X., Chen, Z., Shi, Y., Gui, J.-F., and Zhao, Z. (2021). Response of gut microbiota to feed-borne bacteria depends on fish growth rate: a snapshot survey of farmed juvenile Takifugu obscurus. Microb. Biotechnol. 15, 683–702. doi: 10.1111/1751-7915.13741
Jobling, M., Jørgensen, E. H., Arnesen, A. M., and Ringø, E. (1993). Feeding, growth and environmental requirements of Arctic charr: a review of aquaculture potential. Aquac. Int. 1, 20–46. doi: 10.1007/BF00692662
Klindworth, A., Pruesse, E., Schweer, T., Peplies, J., Quast, C., Horn, M., et al. (2013). Evaluation of general 16S ribosomal RNA gene PCR primers for classical and next-generation sequencing-based diversity studies. Nucleic Acids Res. 41:e808. doi: 10.1093/nar/gks808
Knipe, H., Temperton, B., Lange, A., Bass, D., and Tyler, C. R. (2021). Probiotics and competitive exclusion of pathogens in shrimp aquaculture. Rev. Aquac. 13, 324–352. doi: 10.1111/RAQ.12477
Knobloch, S., Jóhannsson, R., and Marteinsson, V. (2019). Bacterial diversity in the marine sponge Halichondria panicea from Icelandic waters and host-specificity of its dominant symbiont “Candidatus Halichondribacter symbioticus”. FEMS Microbiol. Ecol. 95:220. doi: 10.1093/femsec/fiy220
Knobloch, S., Philip, J., Ferrari, S., Benhaïm, D., Bertrand, M., and Poirier, I. (2021). The effect of ultrasonic antifouling control on the growth and microbiota of farmed European sea bass (Dicentrarchus labrax). Mar. Pollut. Bull. 164:112072. doi: 10.1016/j.marpolbul.2021.112072
Krausova, G., Hyrslova, I., and Hynstova, I. (2019). In vitro evaluation of adhesion capacity, hydrophobicity, and auto-aggregation of newly isolated potential probiotic strains. Ferment 5:100. doi: 10.3390/FERMENTATION5040100
Lara-Flores, M., Olvera-Novoa, M. A., Guzmán-Méndez, B. E., and López-Madrid, W. (2003). Use of the bacteria streptococcus faecium and Lactobacillus acidophilus, and the yeast Saccharomyces cerevisiae as growth promoters in Nile tilapia (Oreochromis niloticus). Aquaculture 216, 193–201. doi: 10.1016/S0044-8486(02)00277-6
Lauzon, H. L., Gudmundsdottir, S., Steinarsson, A., Oddgeirsson, M., Martinsdottir, E., and Gudmundsdottir, B. K. (2010). Impact of probiotic intervention on microbial load and performance of Atlantic cod (Gadus morhua L.) juveniles. Aquaculture 310, 139–144. doi: 10.1016/J.AQUACULTURE.2010.10.017
Leeper, A., Ekmay, R., Knobloch, S., Skírnisdóttir, S., Varunjikar, M., Dubois, M., et al. (2022). Torula yeast in the diet of Atlantic Salmon (Salmo salar) and the impact on growth performance and gut microbiome. Sci. Rep. 12:567. doi: 10.1038/s41598-021-04413-2
Llewellyn, M. S., McGinnity, P., Dionne, M., Letourneau, J., Thonier, F., Carvalho, G. R., et al. (2015). The biogeography of the Atlantic salmon (Salmo salar) gut microbiome. ISME J. 105, 1280–1284. doi: 10.1038/ismej.2015.189
Lobo, C., Moreno-Ventas, X., Tapia-Paniagua, S., Rodríguez, C., Moriñigo, M. A., and de La Banda, I. G. (2014). Dietary probiotic supplementation (Shewanella putrefaciens Pdp11) modulates gut microbiota and promotes growth and condition in Senegalese sole larviculture. Fish Physiol. Biochem. 40, 295–309. doi: 10.1007/s10695-013-9844-0
Mandal, S., Van Treuren, W., White, R. A., Eggesbø, M., Knight, R., and Peddada, S. D. (2015). Analysis of composition of microbiomes: a novel method for studying microbial composition. Microb. Ecol. Health Dis. 26:7663. doi: 10.3402/mehd.v26.27663
McMurdie, P. J., and Holmes, S. (2013). Phyloseq: An R package for reproducible interactive analysis and graphics of microbiome census data. PLoS One 8:e61217. doi: 10.1371/journal.pone.0061217
Merrifield, D. L., Dimitroglou, A., Foey, A., Davies, S. J., Baker, R. T. M., Bøgwald, J., et al. (2010). The current status and future focus of probiotic and prebiotic applications for salmonids. Aquaculture 302, 1–18. doi: 10.1016/J.AQUACULTURE.2010.02.007
Mohammadian, T., Nasirpour, M., Tabandeh, M. R., and Mesbah, M. (2019). Synbiotic effects of β-glucan, mannan oligosaccharide and Lactobacillus casei on growth performance, intestine enzymes activities, immune-hematological parameters and immune-related gene expression in common carp, Cyprinus carpio: An experimental infection with Aeromonas hydrophila. Aquaculture 511:634197. doi: 10.1016/J.AQUACULTURE.2019.06.011
Mohapatra, S., Chakraborty, T., Prusty, A. K., Das, P., Paniprasad, K., and Mohanta, K. N. (2012). Use of different microbial probiotics in the diet of rohu, Labeo rohita fingerlings: effects on growth, nutrient digestibility and retention, digestive enzyme activities and intestinal microflora. Aquac. Nutr. 18, 1–11. doi: 10.1111/J.1365-2095.2011.00866.X
Mora-Sánchez, B., Pérez-Sánchez, T., and Balcázar, J. L. (2020). Phylogenetic analysis of intestinal microbiota reveals novel Mycoplasma phylotypes in salmonid species. Microb. Pathog. 145:104210. doi: 10.1016/J.MICPATH.2020.104210
Mortensen, A., and Damsgård, B. (1993). Compensatory growth and weight segregation following light and temperature manipulation of juvenile Atlantic salmon (Salmo salar L.) and Arctic charr (Salvelinus alpinus L.). Aquaculture 114, 261–272. doi: 10.1016/0044-8486(93)90301-E
Muñoz-Atienza, E., Gómez-Sala, B., Araújo, C., Campanero, C., Campo, R.del, Hernández, P. E., et al. (2013). Antimicrobial activity, antibiotic susceptibility and virulence factors of lactic acid Bacteria of aquatic origin intended for use as probiotics in aquaculture. BMC Microbiol. 1–22. doi:doi: 10.1186/1471-2180-13-15, 131
Nayak, S. K. (2010). Probiotics and immunity: A fish perspective. Fish Shellfish Immunol. 29, 2–14. doi: 10.1016/J.FSI.2010.02.017
Nguyen, T. L., Chun, W.-K., Kim, A., Kim, N., Roh, H. J., Lee, Y., et al. (2018). Dietary probiotic effect of Lactococcus lactis WFLU12 on low-molecular-weight metabolites and growth of olive flounder (Paralichythys olivaceus). Front. Microbiol. 9:2059. doi: 10.3389/FMICB.2018.02059
Olmos, J., Acosta, M., Mendoza, G., and Pitones, V. (2019). Bacillus subtilis, an ideal probiotic bacterium to shrimp and fish aquaculture that increase feed digestibility, prevent microbial diseases, and avoid water pollution. Arch. Microbiol. 2023, 427–435. doi: 10.1007/S00203-019-01757-2
Ouwehand, A. C., and Salminen, S. (2009). In vitro adhesion assays for probiotics and their in vivo relevance: a review. Microb. Ecol. Health Dis. 15, 175–184. doi: 10.1080/08910600310019886
Øverli, Ø., Sørensen, C., and Nilsson, G. E. (2006). Behavioral indicators of stress-coping style in rainbow trout: do males and females react differently to novelty? Physiol. Behav. 87, 506–512. doi: 10.1016/j.physbeh.2005.11.012
Pan, X., Wu, T., Zhang, L., Song, Z., Tang, H., and Zhao, Z. (2008). In vitro evaluation on adherence and antimicrobial properties of a candidate probiotic Clostridium butyricum CB2 for farmed fish. J. Appl. Microbiol. 105, 1623–1629. doi: 10.1111/J.1365-2672.2008.03885.X
Parata, L., Nielsen, S., Xing, X., Thomas, T., Egan, S., and Vergés, A. (2020). Age, gut location and diet impact the gut microbiome of a tropical herbivorous surgeonfish. FEMS Microbiol. Ecol. 96:179. doi: 10.1093/FEMSEC/FIZ179
Péter, A. (2011). Solomon coder (version beta 19.08.02): a simple solution for behavior coding. Computer Program. Available at: http//solomoncoder.com (Accessed May, 2020).
Pilet, M. F., Xavier, D., Rachel, B., Georges, N., Michel, D., and Piard, J. C. (1995). Evidence for two Bacteriocins produced by Carnobacterium piscicola and Carnobacterium divergens isolated from fish and active Against Listeria monocytogenes. J. Food Prot. 58, 256–262. doi: 10.4315/0362-028X-58.3.256
Quast, C., Pruesse, E., Yilmaz, P., Gerken, J., Schweer, T., Yarza, P., et al. (2013). The SILVA ribosomal RNA gene database project: improved data processing and web-based tools. Nucleic Acids Res. 41, D590–D596. doi: 10.1093/nar/gks1219
Queiroz, J. F., and Boyd, C. E. (1998). Effects of a bacterial inoculum in channel catfish ponds. J. World Aquacult. Soc. 29, 67–73. doi: 10.1111/J.1749-7345.1998.TB00300.X
R Core Team (2020). “R: A Language and Environment for Statistical Computing. R Foundation for Statistical Computing,” Vienna, Austria. Available at: https://www.R-project.org/in (Accessed August, 2020).
Rahimnejad, S., Guardiola, F. A., Leclercq, E., Ángeles Esteban, M., Castex, M., Sotoudeh, E., et al. (2018). Effects of dietary supplementation with Pediococcus acidilactici MA18/5M, galactooligosaccharide and their synbiotic on growth, innate immunity and disease resistance of rockfish (Sebastes schlegeli). Aquaculture 482, 36–44. doi: 10.1016/J.AQUACULTURE.2017.09.020
Ramos, M. A., Weber, B., Gonçalves, J. F., Santos, G. A., Rema, P., and Ozório, R. O. A. (2013). Dietary probiotic supplementation modulated gut microbiota and improved growth of juvenile rainbow trout (Oncorhynchus mykiss). Comp. Biochem. Physiol. Part A Mol. Integr. Physiol. 166, 302–307. doi: 10.1016/J.CBPA.2013.06.025
Rasmussen, J. A., Villumsen, K. R., Duchêne, D. A., Puetz, L. C., Delmont, T. O., Sveier, H., et al. (2021). Genome-resolved metagenomics suggests a mutualistic relationship between Mycoplasma and salmonid hosts. Commun. Biol. 41, 1–10. doi: 10.1038/s42003-021-02105-1
Ravi, A. V., Musthafa, K. S., Jegathammbal, G., Kathiresan, K., and Pandian, S. K. (2007). Screening and evaluation of probiotics as a biocontrol agent against pathogenic Vibrios in marine aquaculture. Lett. Appl. Microbiol. 45, 219–223. doi: 10.1111/J.1472-765X.2007.02180.X
Remenant, B., Borges, F., Cailliez-Grimal, C., Revol-Junelles, A. M., Marché, L., Lajus, A., et al. (2016). Draft genome sequence of Carnobacterium divergens V41, a Bacteriocin-producing strain. Genome Announc. 4, 1109–1125. doi: 10.1128/GENOMEA.01109-16
Ringø, E., Sperstad, S., Myklebust, R., Mayhew, T. M., and Olsen, R. E. (2006). The effect of dietary inulin on aerobic bacteria associated with hindgut of Arctic charr (Salvelinus alpinus L.). Aquac. Res. 37, 891–897. doi: 10.1111/J.1365-2109.2006.01509.X
Ringø, E., Doan, H.Van, Lee, S. H., Soltani, M., Hoseinifar, S. H., Harikrishnan, R., et al. (2020). Probiotics, lactic acid bacteria and bacilli: interesting supplementation for aquaculture. J. Appl. Microbiol. 129, 116–136. doi:doi: 10.1111/JAM.14628
Rosengren, M., Thörnqvist, P. O., Winberg, S., and Sundell, K. (2018). The brain-gut axis of fish: rainbow trout with low and high cortisol response show innate differences in intestinal integrity and brain gene expression. Gen. Comp. Endocrinol. 257, 235–245. doi: 10.1016/J.YGCEN.2017.09.020
RStudio Team (2016). Rstudio: Integrated Development Environment for r [Computer Software Manual]. Boston, MA: Author.
Sahnouni, F., Ringø, E., Maizi, A., Matallah-Boutiba, S. B. A., Chemlal, D., and Boutiba, Z. (2016). Biochemical and antibacterial potential of autochthonous Carnobacterium and Lactobacillus species isolated from gastrointestinal tract of coastal fish. J. Anim. Plant Sci 26, 1146–1155.
Serra, C. R., Almeida, E. M., Guerreiro, I., Santos, R., Merrifield, D. L., Tavares, F., et al. (2019). Selection of carbohydrate-active probiotics from the gut of carnivorous fish fed plant-based diets. Sci. Report. 91, 1–15. doi: 10.1038/s41598-019-42716-7
Skrodenytė-Arbačiauskienė, V., Virbickas, T., Lukša, J., Servienė, E., Blažytė-Čereškienė, L., and Kesminas, V. (2021). Gut microbiome of wild Baltic Salmon (Salmo salar L.) Parr. Microb. Ecol. 1, 1–5. doi: 10.1007/S00248-021-01910-9/FIGURES/2
Soares, M. C., Cable, J., Lima-Maximino, M. G., Maximino, C., and Xavier, R. (2019). Using fish models to investigate the links between microbiome and social behaviour: The next step for translational microbiome research? Fish. faf.12366:12366. doi: 10.1111/faf.12366
Tan, L. T.-H., Chan, K.-G., Lee, L.-H., and Goh, B.-H. (2016). Streptomyces Bacteria as potential probiotics in aquaculture. Front. Microbiol. 7:79. doi: 10.3389/FMICB.2016.00079
Tareb, R., Bernardeau, M., Gueguen, M., and Vernoux, J. P. (2013). In vitro characterization of aggregation and adhesion properties of viable and heat-killed forms of two probiotic Lactobacillus strains and interaction with foodborne zoonotic bacteria, especially Campylobacter jejuni. J. Med. Microbiol. 62, 637–649. doi: 10.1099/jmm.0.049965-0
Taridashti, F., Delafkar, K., Zare, A., and Azari-Takami, G. (2017). Effects of probiotic Pediococcus acidilactici on growth performance, survival rate, and stress resistance of Persian sturgeon (Acipenser persicus). J. Appl. Aquac. 29, 220–232. doi: 10.1080/10454438.2017.1363112
Tarkhani, R., Imani, A., Hoseinifar, S. H., Sarvi Moghanlou, K., and Manaffar, R. (2020). The effects of host-associated Enterococcus faecium CGMCC1.2136 on serum immune parameters, digestive enzymes activity and growth performance of the Caspian roach (Rutilus caspicus) fingerlings. Aquaculture 519:734741. doi: 10.1016/J.AQUACULTURE.2019.734741
Van Doan, H., Hoseinifar, S. H., Khanongnuch, C., Kanpiengjai, A., Unban, K., Van Kim, V., et al. (2018). Host-associated probiotics boosted mucosal and serum immunity, disease resistance and growth performance of Nile tilapia (Oreochromis niloticus). Aquaculture 491, 94–100. doi: 10.1016/J.AQUACULTURE.2018.03.019
Vinderola, G., Gueimonde, M., Gomez-Gallego, C., Delfederico, L., and Salminen, S. (2017). Correlation between in vitro and in vivo assays in selection of probiotics from traditional species of bacteria. Trends Food Sci. Technol. 68, 83–90. doi: 10.1016/J.TIFS.2017.08.005
Vine, N. G., Leukes, W. D., and Kaiser, H. (2004a). In vitro growth characteristics of five candidate aquaculture probiotics and two fish pathogens grown in fish intestinal mucus. FEMS Microbiol. Lett. 231, 145–152. doi: 10.1016/S0378-1097(03)00954-6
Vine, N. G., Leukes, W. D., Kaiser, H., Daya, S., Baxter, J., and Hecht, T. (2004b). Competition for attachment of aquaculture candidate probiotic and pathogenic bacteria on fish intestinal mucus. J. Fish Dis. 27, 319–326. doi: 10.1111/J.1365-2761.2004.00542.X
Wang, Y. B., Li, J. R., and Lin, J. (2008). Probiotics in aquaculture: challenges and outlook. Aquaculture 281, 1–4. doi: 10.1016/J.AQUACULTURE.2008.06.002
Wiernasz, N., Cornet, J., Cardinal, M., Pilet, M.-F., Passerini, D., and Leroi, F. (2017). Lactic acid Bacteria selection for biopreservation as a part of hurdle technology approach applied on seafood. Front. Mar. Sci. 4:119. doi: 10.3389/fmars.2017.00119
Yang, X., and Dick, T. A. (1994). Arctic char (Salvelinus alpinus) and rainbow trout (Oncorhynchus mykiss) differ in their growth and lipid metabolism in response to dietary polyunsaturated fatty acids. Can. J. Fish. Aquat. Sci. 51, 1391–1400. doi: 10.1139/f94-139
Keywords: probiotics, aquaculture, gut microbiome, salmonid, Mycoplasma, growth, behavior
Citation: Knobloch S, Skírnisdóttir S, Dubois M, Kolypczuk L, Leroi F, Leeper A, Passerini D and Marteinsson VÞ (2022) Impact of Putative Probiotics on Growth, Behavior, and the Gut Microbiome of Farmed Arctic Char (Salvelinus alpinus). Front. Microbiol. 13:912473. doi: 10.3389/fmicb.2022.912473
Edited by:
Harold J. Schreier, University of Maryland, Baltimore County, United StatesReviewed by:
Jesu Arockiaraj, SRM Institute of Science and Technology, IndiaDavid Pérez-Pascual, Institut Pasteur, France
Copyright © 2022 Knobloch, Skírnisdóttir, Dubois, Kolypczuk, Leroi, Leeper, Passerini and Marteinsson. This is an open-access article distributed under the terms of the Creative Commons Attribution License (CC BY). The use, distribution or reproduction in other forums is permitted, provided the original author(s) and the copyright owner(s) are credited and that the original publication in this journal is cited, in accordance with accepted academic practice. No use, distribution or reproduction is permitted which does not comply with these terms.
*Correspondence: Viggó Þ. Marteinsson, viggo@matis.is
†Present address: Stephen Knobloch, Senckenberg Biodiversity and Climate Research Centre, Frankfurt, Germany