- 1Metabolomics Research Center for Functional Materials, Kyungsung University, Busan, South Korea
- 2Department of SmartBio, Kyungsung University, Busan, South Korea
Riptortus pedestris insect indiscriminately acquires not only the symbiotic bacterium Burkholderia insecticola, but also entomopathogens that are abundant in the soil via feeding. However, it is unclear how the host insect survives oral infections of entomopathogens. A previous study suggested that serralysin, a potent virulence factor produced by Serratia marcescens, suppresses cellular immunity by degrading adhesion molecules, thereby contributing to bacterial pathogenesis. Here, we observed that S. marcescens orally administered to R. pedestris stably colonized the insect midgut, while not exhibiting insecticidal activity. Additionally, oral infection with S. marcescens did not affect the host growth or fitness. When co-incubated with the midgut lysates of R. pedestris, serralysin was remarkably degraded. The detoxification activity against serralysin was enhanced in the midgut extract of gut symbiont-colonizing insects. The mRNA expression levels of serralysin genes were negligible in M3-colonizing S. marcescens. M3-colonizing S. marcescens did not produce serralysin toxin. Immunoblot analyses revealed that serralysin was not detected in the M3 midgut region. The findings of our study suggest that orally infected S. marcescens lose entomopathogenicity through host-derived degrading factors and suppression of serralysin.
Introduction
Serratia species is frequently found in the midgut bacterial flora of almost all hematopoietic insects, such as mosquitoes and Rhodnius species (Demaio et al., 1996; Straif et al., 1998; Gonzalez-Ceron et al., 2003; Azambuja et al., 2004). The gram-negative bacterium, Serratia marcescens, acts as an opportunistic pathogen in a wide range of animals, including humans and insects (Raymann et al., 2018). Serratia marcescens is an entomopathogen that causes bacteremia in the insect hemolymph, resulting in rapid death (Grimont and Grimont, 1978; Chadwick et al., 1990). The gut commensal S. marcescens promotes mosquito permissiveness to arboviruses and facilitates arboviral infection via a secreted protein SmEnhancin (Wu et al., 2019). In the gut of a cockroach, Gromphadorhina portentosa (Madagascar), S. marcescens not only produces active molecule(s) with potent antibacterial properties but also exhibits anti-amoebic effects (Akbar et al., 2018). This entomopathogen produces a virulence factor, called serralysin, which shows insecticidal activity via hemolymph bleeding and inhibition of immune cell adhesion (Tambong et al., 2014; Ishii et al., 2014a,b). Notably, serralysin degrades the humoral immune factors in insects (Lee et al., 2017a). Serralysin is a conserved metalloprotease among various bacterial species and requires both zinc and a divalent cation for maximal catalysis (Bode et al., 1993; Belas et al., 2004). The entomopathogen Xenorhabdus has a serralysin-type metalloenzyme called PrtA (Massaoud et al., 2011). Proteus and Photorhabdus species also have the serralysin homologs ZapA and AprA, respectively, which inhibit antibacterial activity by destroying antimicrobial peptides, such as defensin and cecropin (Belas et al., 2004; Cabral et al., 2004). The serralysin family is grouped in the metzincin metalloprotease superfamily which is characterized by the zinc-binding motif (HEXXHXXGXXHZ; Bode et al., 1993; Salamone and Wodzinski, 1997).
Riptortus pedestris-Burkholderia insecticola symbiosis is a powerful model system for analyzing molecular cross-talks. The bean bug, R. pedestris (Hemiptera: Alydidae), possesses a specialized symbiotic organ in the posterior region of the midgut (referred to as the M4 crypt), where numerous crypts harbor specific gut symbionts of the β-proteobacterial genus, Burkholderia (Kikuchi et al., 2005). In this symbiosis model, Burkholderia-colonized insects (Sym-insects) and insects without gut symbionts (Apo-insects) can be generated in the laboratory depending on oral infection of Burkholderia cells, respectively (Lee et al., 2017b, 2019, 2022). However, the gut immune responses of R. pedestris to exogenous microbes have not yet been clearly elucidated. Using this model, we recently observed that orally infected entomopathogenic S. marcescens cells are resistant to the antimicrobial peptide, trialysin, produced in the salivary glands of R. pedestris (Lee et al., 2017a). However, it is unclear how the pathogenicity of S. marcescens is eventually suppressed in the midgut.
In this study, orally infected S. marcescens were found to colonize the midgut of R. pedestris, but did not exhibit any insecticidal activity against the host. Serralysin was markedly degraded by host-derived factors present in the M1 midgut region. The M3-colonizing S. marcescens cells did not produce serralysin. These results suggest that orally infected S. marcescens lose insecticidal activity through expression of host-derived degrading factors and suppression of serralysin molecules.
Materials and Methods
Insect Rearing and Serratia marcescens Inoculation
Riptortus pedestris was maintained in an insectary at 28°C under a long-day cycle of 16 h light and 8 h dark, as described previously (Lee et al., 2020). The nymphs were reared in clean plastic containers with soybean seeds and distilled water containing 0.05% ascorbic acid. The non-pigmented S. marcescens strain Db11 was cultured to mid-log phase at 37°C in the Luria-Bertani medium (BD Difco, United States; Lee et al., 2017a). Newly molted second-instar nymphs were inoculated with S. marcescens Db11 cells at a concentration of 107 cells/ml.
Fitness Measurement
To compare the effect of S. marcescens oral infection on the host fitness, nymphs were reared until adulthood and assessed for various fitness parameters, such as emergence rate, survival rate, dry weight, and body length (Lee et al., 2017b). Emergence was monitored daily by inspecting the late-fifth-instar nymphs and counting the number of newly molted adult insects. Survival rates were examined from the second instar to the adult stage when S. marcescens was orally infected. For dry weight measurements, adult males were immersed in acetone for 5 min and then completely dried in an oven at 70°C for 10 min.
Purification of Serralysin
Serralysin was purified from bacterial cultures according to a previous report (Lee et al., 2017a). Briefly, bacteria were grown at 30°C to an optical density at 600 nm (OD600) of 3.0 in the brain heart infusion medium (BD Difco, United States). The supernatant was filtered through a 0.2 μm pore filter (Whatman, United States), ultrafiltered, and replaced with buffer A (50 mM Tris-HCl, pH 8.0) using a Vivaspin 3,000 Da filter (10,000 × g, 30 min, 4°C; Sartorius, United States). The solutions were loaded onto a Mono-Q column equilibrated with buffer A (Metabolomics Research Center for Functional Materials, Kyungsung University; Agilent Technologies 1260 Infinity LC System, United States). The column was eluted with 30 ml of a linear gradient of NaCl (0–1 M) in buffer A at a flow rate of 0.5 ml/min. Active fractions were collected, N-terminally sequenced, and used for further experiments (Lee et al., 2017a).
Administration of Serralysin and Measurement of Survival Rate
Purified serralysin was sequentially diluted to a concentration of 1 μg/μl. Each serralysin solution at a different dose (2 μl aliquot) was orally infected or systemically injected into the joint of the hind leg that connects to the 3-day-old adult male, R. pedestris. The survival rate was monitored from 1 d after the systemic injection.
Preparation of Midgut Lysates From Apo- and Sym-Insects
Midguts from 10 male R. pedestris adults were collected in 500 μl of 10 mM phosphate buffer (PB; pH 7.0) containing a protease inhibitor cocktail (Sigma Aldrich, United States). The collected midguts were homogenized using disposable plastic pestles (SciLab Korea, South Korea). After centrifugation at 10,000 × g for 15 min at 4°C, the supernatant was ultrafiltered and replaced with buffer A (50 mM Tris–HCl, pH 8.0) using a Vivaspin 3,000 Da filter (10,000 × g, 30 min, 4°C; Sartorius, Germany). After buffer replacement, the solution was collected and used immediately for further experiments. The supernatant was heat-treated at 90°C for 5 min to inactivate the serralysin-degrading proteins and centrifuged at 20,000 × g for 10 min at 4°C. Bovine serum albumin was used for protein quantification of midgut lysates, and the native protein concentration was determined using the Bradford assay (Kruger, 2009).
Immunoblot Analyses
For immunoblot analyses, proteins from M3 lysate or hemolymph were separated on a 10% sodium dodecyl sulfate-polyacrylamide gel and transferred to a polyvinylidene difluoride membrane (Millipore, United States) using an electro-transfer blotter (Lee et al., 2017a). The membrane was blocked with 10% skim milk in Tris-buffered saline with Tween 20 (TBST) buffer (50 mM Tris-HCl, 150 mM NaCl, and 0.02% Tween 20) for 1 h and washed six times with the TBST buffer. After blocking, the membranes were incubated at room temperature for 1 h with the anti-rabbit serralysin antibody (dilution factor 1:5,000) in TBST containing 5% skimmed milk (Lee et al., 2017a). After washing with TBST six times, the membrane was incubated with horseradish peroxidase (HRP)-conjugated goat anti-rabbit IgG secondary antibody (dilution factor 1:10,000; Santa Cruz, United States) for 30 min. The membranes were washed seven times with TBST and visualized using HRP color development solution in accordance with the manufacturer’s instructions.
Isolation of Midgut-Colonizing Serratia marcescens Cells
M3 midguts were dissected from 10 fifth-instar nymphs and collected in 100 μl of 10 mM PB. M3 midguts were cut several times with fine scissors to break the midgut crypts. One milliliter of 10 mM PB was added to the midgut fragments by gentle pipetting. The solution was then filtered through a 5.0 μm pore to remove the midgut tissues. Isolated S. marcescens cells were gently washed with 10 mM PB and collected by centrifugation at 2,000 × g. The bacterial cell number was estimated using a hemocytometer.
Quantitative Real-Time Polymerase Chain Reaction
Total RNA was isolated from midgut-colonizing or cultured S. marcescens cells (OD600 nm = 1.0) using the TRIzol reagent (Invitrogen, United States) and RNeasy mini kit (Qiagen, United States), according to the manufacturer’s recommendations. Thereafter, 500 ng of total RNA was converted into cDNA using TOPscript RT DryMix containing oligo-dT primers (Enzynomics, South Korea). The synthesized cDNAs were diluted 20-fold, and qRT-PCR was performed on a QuantStudio™3 Real-Time PCR System (Thermo Fisher Scientific Inc., United States). The PCR conditions were as follows: 95°C for 10 min, followed by 40 cycles of 95°C for 10 s, 60°C for 15 s, and 72°C for 20 s. The primer sets used for qRT-PCR of serralysin genes are listed in Table 1. The comparative CT (∆∆CT) method was used to calculate the relative gene expression levels based on the 16S rRNA of S. marcescens cells as an internal control gene (Wilf et al., 2011). All analyses were performed using the QuantStudio™ Design & Analysis Software ver 1.5.2 (Thermo Fisher Scientific Inc.,).
Statistical Analysis
Statistical significance of the data was determined using an unpaired Student’s t-test, as provided in GraphPad Prism (ver. 8.0; GraphPad Software, United States).
Results
Midgut-Colonizing Serratia marcescens Cells Did Not Affect Host Fitness
Previous studies have shown that S. marcescens Db11 strain is highly pathogenic to Drosophila melanogaster and Apis mellifera when they are systemically injected with this bacterium (Flyg et al., 1980; Kurz et al., 2003; Raymann et al., 2018). However, orally infected S. marcescens Db11 cells can colonize the Drosophila midgut without any virulence (Nehme et al., 2007). Based on these reports, we hypothesized that orally infected S. marcescens cells could colonize the midgut of R. pedestris. The bean bug adults have five different midgut regions (Ohbayashi et al., 2015), M1, M2, M3, M4B, and M4. We examined the colony-forming units (CFUs) of S. marcescens in each midgut region at the indicated times (Figure 1A). Serratia marcescens cells were only detected in M1–M3 regions 2 days post-inoculation (Figures 1C,D). These results suggest that orally infected S. marcescens cells can pass through the digestive tract, such as the foregut region and colonize the midgut. The beneficial symbiont, B. insecticola, colonized M4B and M4 midgut regions (Figures 1C,D). This result is consistent with the previous report that no microorganism or color pigment can pass through M4B region except Burkholderia (Ohbayashi et al., 2015). When we examined the population of orally infected S. marcescens cells in M3 midgut region, the titers of S. marcescens were increased steadily during nymphal development and remained at constant levels of around 107 CFUs per individual in adulthood (Figure 1B).
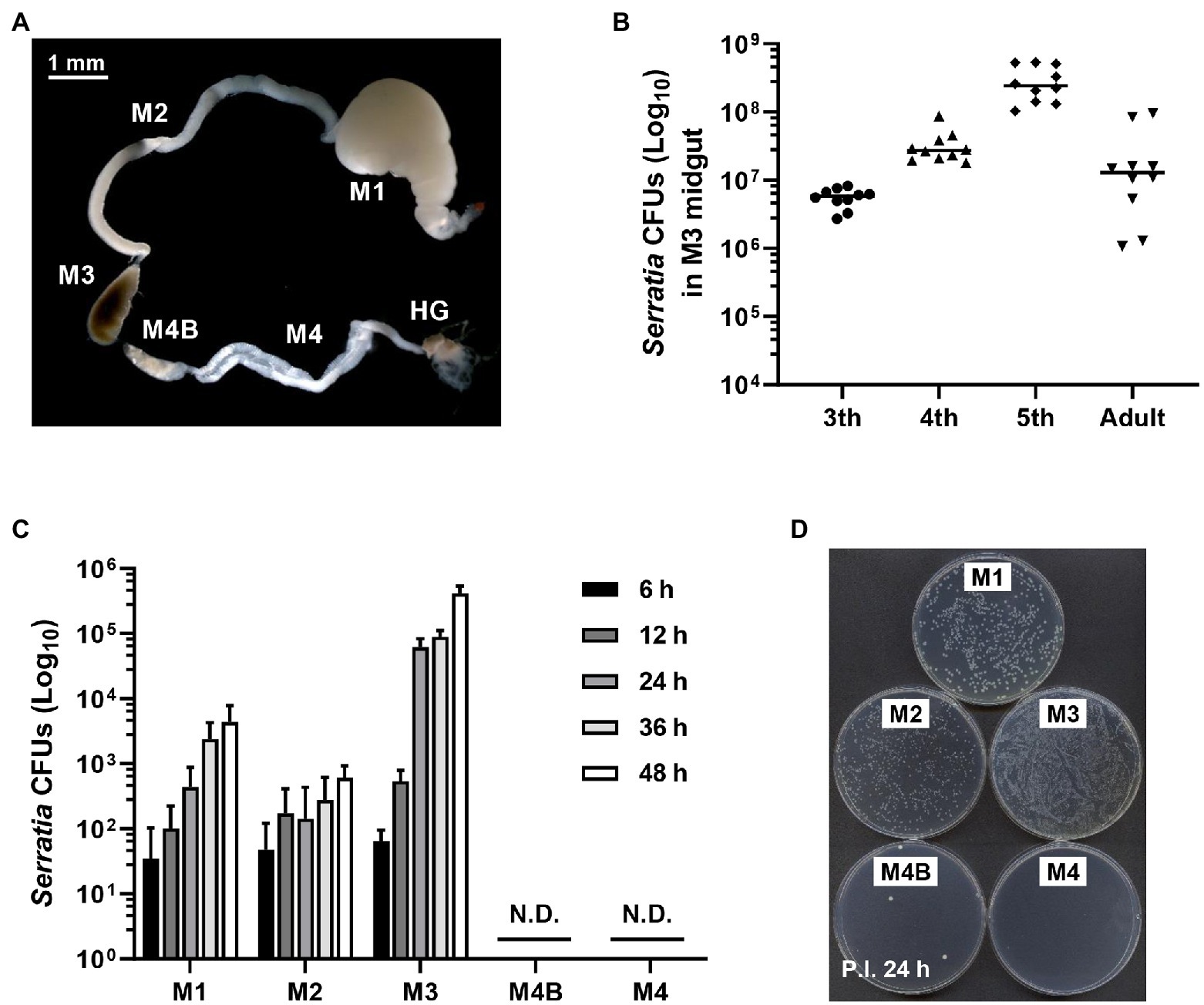
Figure 1. Colonization of orally infected Serratia marcescens. (A) Midgut (M1, M2, M3, M4B, and M4) and hindgut (HG) regions of the Riptortus pedestris insect. (B) Infection density of S. marcescens at third, fourth, fifth-instar stages and male adults. Means and standard deviations (SDs; n = 10) are shown as columns and error bars, respectively. (C) Colony-forming unit (CFU) quantification of infection densities of S. marcescens Db11 cells at 6, 12, 24, 36, and 48 h after inoculation. Horizontal lines in the graph indicate the mean values of S. marcescens CFUs. Error bars indicate the SD of the mean (n = 10). (N.D., not detected). (D) Colonies on agar plate of S. marcescens cells at an early stage of infection.
Based on the fact that Burkholderia gut symbionts positively affect the host fitness and immune responses (Kim et al., 2015a; Lee et al., 2017b), we investigated if orally infected S. marcescens is a potent entomopathogen that affects the survival rate and growth of the host. Furthermore, we speculated that Sym-insects with Burkholderia gut symbionts would be more resistant to orally infected S. marcescens than Apo-insects without symbionts. To investigate the effects of S. marcescens cells on either Sym- or Apo-insects, the adult emergence rates of R. pedestris were estimated after oral infection with cultured S. marcescens cells. Orally infected S. marcescens did not affect the growth of R. pedestris (Figure 2A). When the survival rates between Sym- and Apo-insects were compared, all second nymphs infected with S. marcescens were survived until adulthood (Figure 2B). Evaluation of body length and dry weight showed that orally infected S. marcescens did not have any effect on the host, regardless of the presence of symbiotic Burkholderia cells (Figure 2C). These results suggest that orally infected S. marcescens can colonize the midgut region without affecting host fitness.
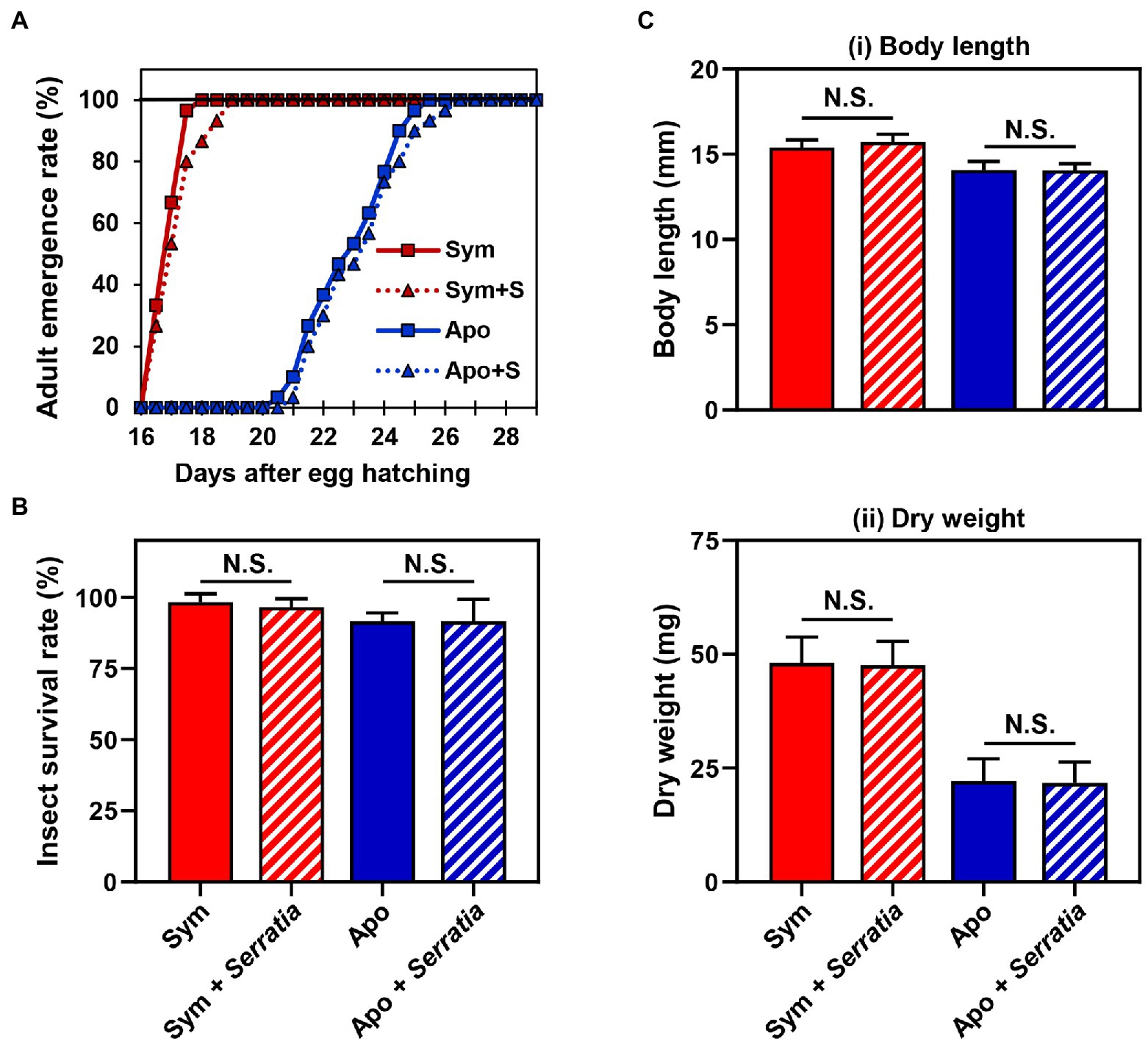
Figure 2. Effects of orally infected Serratia marcescens on Riptortus pedestris. Comparison of the (A) developmental rate, (B) survival rate, and (C) fitness parameters of male R. pedestris insects after oral infection with S. marcescens. The fitness effects of each group were analyzed by measuring the (i) body length and (ii) dry weight of male R. pedestris 5 days after adult emergence. Error bars indicate the SD of the mean (n = 30). (N.S., not significant).
Serralysin Is Specifically Degraded by the Midgut Lysate
Serralysin metalloprotease is the major toxic substance of S. marcescens in host insects (Ishii et al., 2014a). However, our results showed that oral infection with S. marcescens did not have any adverse effects on development of R. pedestris (Figure 2). These results indicate that orally infected S. marcescens does not show any insecticidal activity during the colonization of S. marcescens cells in insect gut. Therefore, to compare the insecticidal activity of serralysin based on the infection routes, the survival rate was investigated by oral infection or systemic injection of serralysin purified from the bacterial culture medium. Only systemic injection of serralysin showed insecticidal activity in a dose-dependent manner whereas all tested insects were survived after oral infection (Figure 3). The amino acid sequence of purified serralysin was identified as A-A-T-T-G-Y-D-A-V-D, corresponding to the 17th to 26th amino acids of serralysin 1 (Supplementary Figure 1).
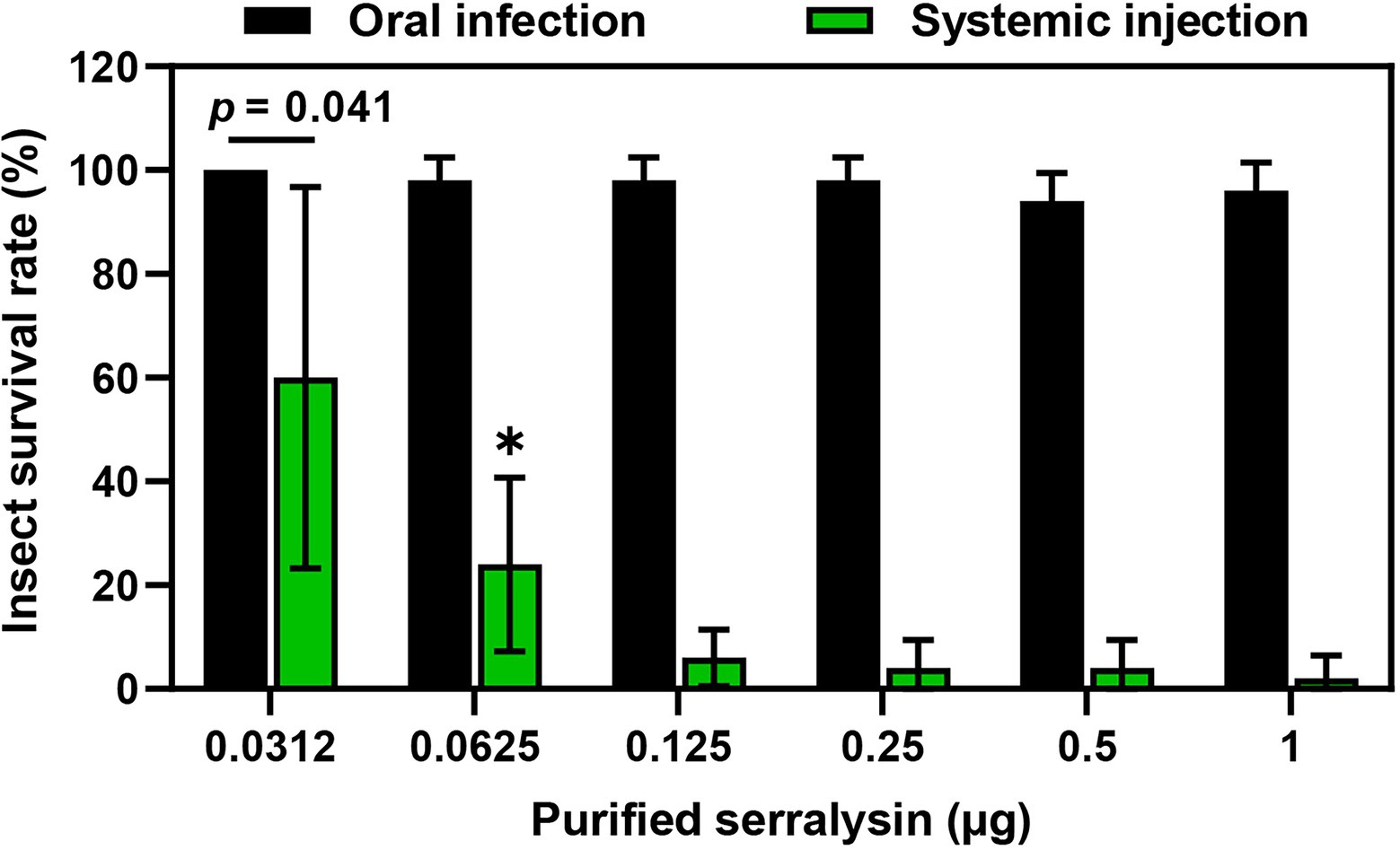
Figure 3. Insecticidal activity of serralysin. Purified serralysin was orally administered and systemically injected into the joint of the hind leg, which connects to the thorax of the insect. Survival was monitored 1 day after systemic injection. Data are expressed as the mean ± SD (n = 10) and are representative of five independent experiments. Asterisks indicate the significant differences between groups (*p < 0.01; unpaired t-test).
It is questionable how host insects are alive when they are orally infected with insecticidal serralysin. Our previous study provided a biochemical evidence that the antimicrobial peptide trialysin in R. pedestris salivary fluid was specifically hydrolyzed by serralysin, leading to the loss of its antimicrobial activity (Lee et al., 2017a). Based on these results, we hypothesized that the insecticidal activity of serralysin would be diminished during digestive tract passage and colonization by unknown factors, thereby facilitating host survival. To prove this hypothesis, purified serralysin was co-incubated with three midgut extracts of Apo-insects, and the activation of serralysin was investigated with immunoblot analyses. Purified serralysin was gradually degraded by the midgut extract in a dose-dependent manner (Figure 4). This result strongly suggests that a host-derived factor capable of degrading serralysin exists in the M1 midgut region (Figure 4A). To determine if the serralysin-degrading activity is derived from the hosts, Apo- or Sym-insects, extracts from the M1 midgut of both hosts were co-incubated with serralysin. Purified serralysin was completely removed by the M1 lysate of Sym-insects, but remained in the M1 lysate of Apo-insects (Figure 4B-i). Moreover, when the supernatant of heat-treated Sym- and Apo-M1 lysates was added to purified serralysin, the host-derived serralysin-degrading factor lost its biological activity (Figure 4B-ii). This result revealed that the serralysin-degrading factor in the M1 lysate is related to proteinous molecules.
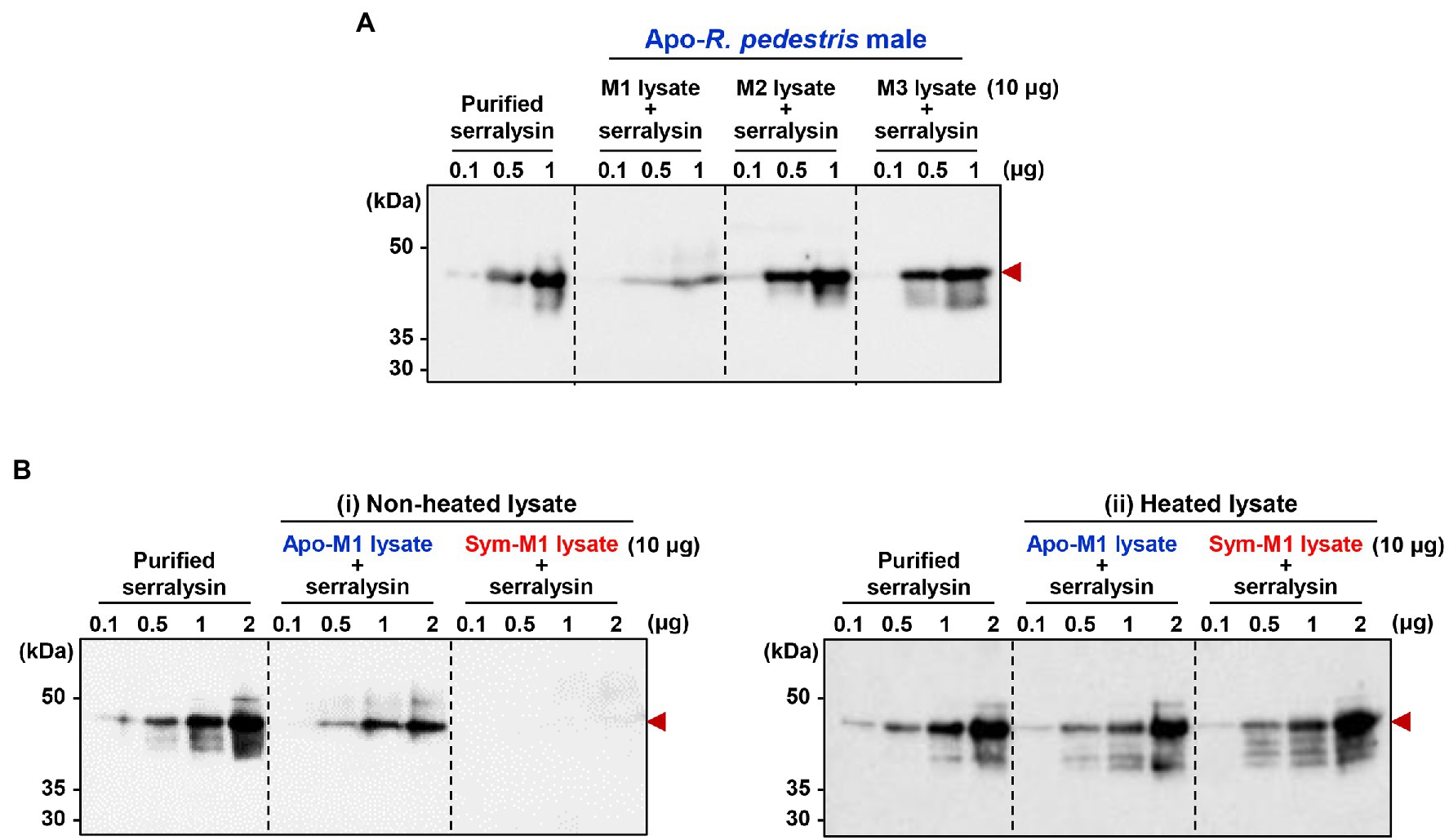
Figure 4. Inactivation of serralysin by midgut lysates. (A) Immunoblot patterns after co-incubation of purified serralysin with M1, M2, and M3 midgut lysates of insects without gut symbionts (Apo-insects). (B) Immunoblot patterns after co-incubation of purified serralysin with M1 midgut lysates of Apo- and Burkholderia-colonized insects (Sym-insects). M1 extracts were prepared (i) without and (ii) with heat treatment, respectively. The reaction conditions were as follows: In total, 10 μg of each midgut lysate was incubated with different concentrations of purified serralysin for 1 h at 28°C. Arrowheads indicate the bands of purified serralysin.
M3-Colonizing Serratia marcescens Cells Do Not Produce Serralysin
Although a serralysin-degrading factor from R. pedestris existed in M1 region (Figure 4), orally infected S. marcescens cells still colonized the M3 midgut region (Figure 1). To understand the host-gut microbe interactions in M3 region, we investigated if serralysin was detected in M3 tissue. Serralysin was not detected in the M3 tissue (Figure 5). This result provides the possibility that S. marcescens cells in M3 region do not produce serralysin during colonization of the host gut. Based on this result, we investigated that serralysin expression in M3 region can be maintained at a low level or completely suppressed. Genome analysis based on the National Center for Biotechnology Information (NCBI) public database showed that two serralysin genes were present in S. marcescens Db11. The nucleotide and putative amino acid sequences of serralysin 1 and serralysin 2 showed a similarity of 66.5 and 59.0%, respectively (Supplementary Figure 1). In addition, serralysin 1 and 2 had a catalytic domain related to metzincin at the N-terminus region (data not shown). The phylogenetic analysis revealed that serralysin 1 and serralysin 2 formed independent clades, respectively (Supplementary Figures 2, 3).
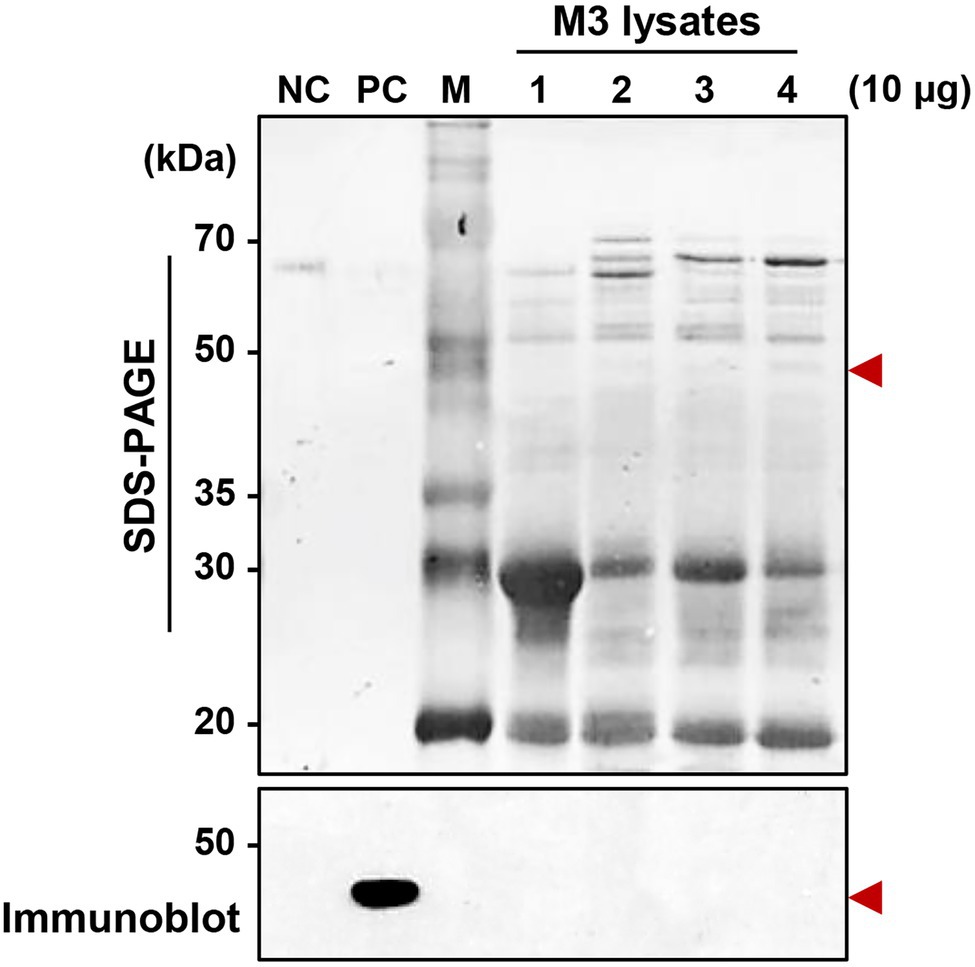
Figure 5. Detection of serralysin in the M3 midgut region of Riptortus pedestris. Sodium dodecyl sulfate-polyacrylamide gel electrophoresis (SDS-PAGE; upper layer) and immunoblot (bottom layer) of M3 lysate after oral infection of male Sym insects with S. marcescens. Serratia marcescens cells (1.0 × 107 CFUs/ml) were orally infected into 3-day-old adult male R. pedestris insects. After infection for 48, 72, and 96 h, M3 tissues were collected from the insects, and the induction of serralysin was analyzed using specific antibodies. Arrowheads indicate the bands of purified serralysin. Negative control (NC): bovine serum albumin (BSA; 0.5 μg); positive control (PC): purified serralysin (0.5 μg); M: ladder marker. Columns 1, 2, 3, and 4 indicate the naïve M3, S. marcescens colonized-M3 (48 h post-inoculation (P.I.)), P.I. 72 h, and P.I. 96 h, respectively.
When comparing mRNA expression levels between serralysin genes in cultured S. marcescens, no difference in the expression level was found (Supplementary Figure 4). The transcriptional levels of serralysin genes were compared between in vitro-cultured S. marcescens and M3-colonizing S. marcescens cells. The expression levels of serralysin genes in M3-colonizing S. marcescens cells were significantly lower than those of in vitro-cultured S. marcescens (Figure 6A). Under systemic injection, M3-colonizing S. marcescens cells did not produce serralysin whereas in vitro-cultured S. marcescens cells secreted serralysin (Figure 6B).
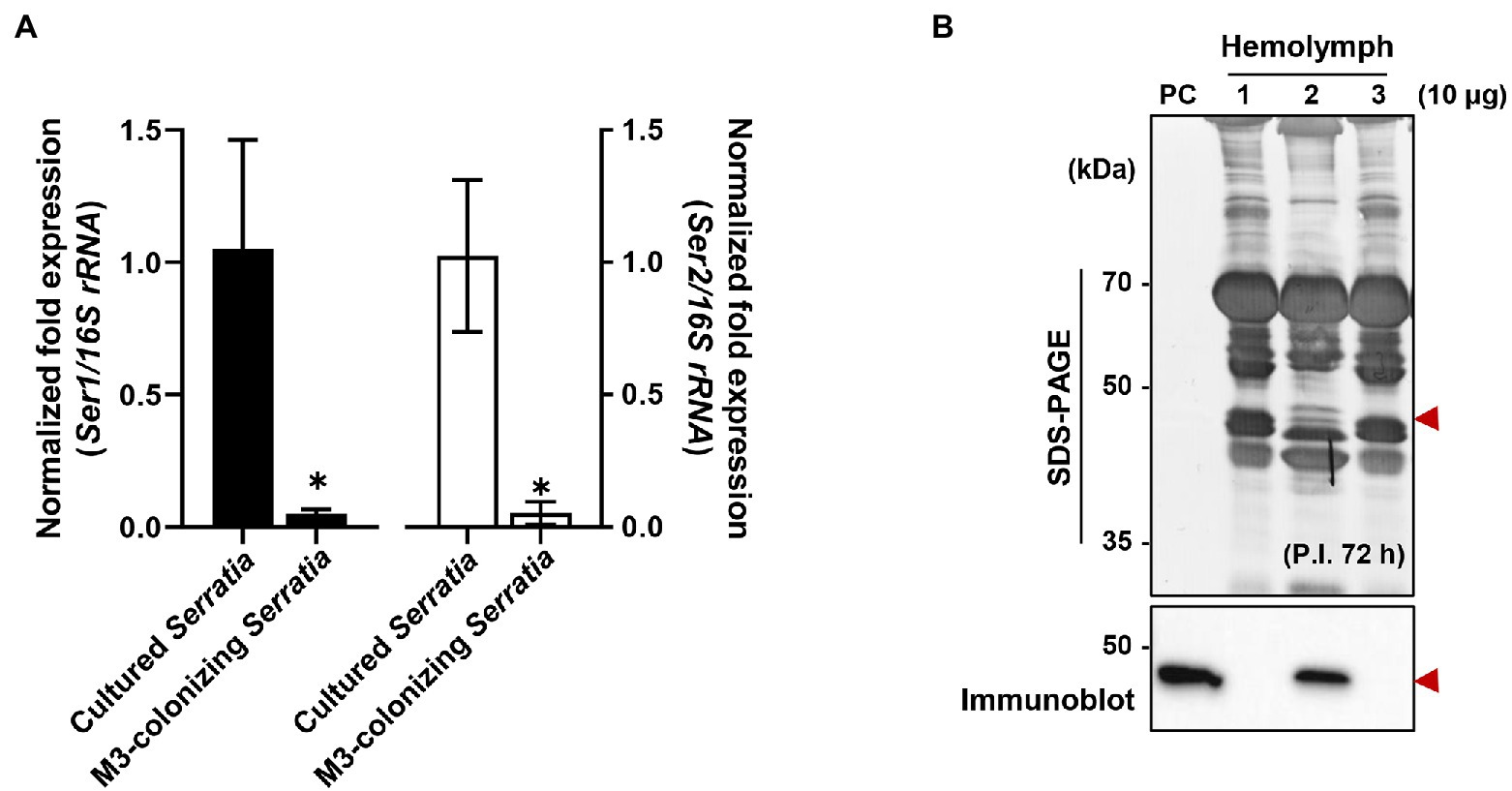
Figure 6. Suppression of serralysin production in M3-colonizing Serratia marcescens cells. (A) The mRNA expression levels of serralysin genes in cultured S. marcescens and M3-colonizing S. marcescens. The expression levels of serralysin genes were normalized to the expression levels of each cultured S. marcescens, which was set as 1. Data are expressed as the mean ± SD (n = 4). (*p < 0.01; unpaired t-test). The Genbank accession numbers of protein sequences are as follows: serralysin 1 (CDG14870.1) and serralysin 2 (CDG11942.1). (B) SDS-PAGE (upper layer) and immunoblot (bottom layer) of whole-body hemolymph after systemic injection of S. marcescens into male Sym insects. Serratia marcescens cells (1.0 × 105 CFUs) systemically injected into 3-day-old adult male Riptortus pedestris insects for immune induction. After injection for 3 h at 28°C, immune-challenged hemolymph was collected from the insects, and induction of serralysin was analyzed using specific antibodies. Arrowheads indicate the bands of purified serralysin. PC: purified serralysin (0.5 μg). Columns 1, 2, and 3 indicate phosphate-buffered saline (PBS) injection, cultured S. marcescens injection, and M3-colonizing S. marcescens injection, respectively.
Discussion
In a previous study, we demonstrated that serralysin, a toxin produced by S. marcescens cells, neutralized the antimicrobial activity of trialysin in the salivary of R. pedestris, resulting in suppressing the host immune response (Lee et al., 2017a). When systemically inoculated into the host, serralysin exhibited insecticidal activity (Figure 3). Based on this result, we hypothesized that the serralysin toxin of orally infected S. marcescens could kill the host insect. However, orally infected S. marcescens cells were found only to colonize the midgut without insecticidal activity (Figure 1). These results led to the idea that orally infected S. marcescens cells may have been converted into non-pathogenic microorganisms and then lost their insecticidal activity.
Insect detoxification systems inactivate toxic substances, greatly reducing biological effects by entomopathogenic microorganisms (Hillyer, 2016). In the insect midgut, the mechanism of resistance against toxic substances is composed of complex responses of the defense system, including alteration of toxin-binding proteins (Bravo et al., 2011), sequestration of the toxin by lipophorin (Ma et al., 2012), increased production of primary enzymes (Tartar et al., 2009; Grizanova et al., 2019; Wu et al., 2020), and antioxidant activity (Dubovskiy et al., 2008). These biochemical reactions require various enzymes, such as esterases, uridine diphosphate-glycosyltransferases, cytochrome P450s, glutathione-S-transferases, and monooxygenases (Ramsey et al., 2010; Feng et al., 2021). These enzymes play important roles in the detoxification of toxic substances, such as insecticides and plant secondary metabolites (Li et al., 2007). In this study, we revealed that a host-derived factor that can degrade serralysin is present in the M1 midgut region of R. pedestris (Figure 4A). The serralysin-degrading activity was completely lost after heat treatment (Figure 4B). Taken together, the host-derived factors for degrading serralysin are proteinous molecules, such as detoxifying enzymes or their complexes. As serine proteases, such as trypsin and chymotrypsin, have a serine hydroxyl residue at the active site and are involved in regulation of enzyme activity, they were candidates for host-derived serralysin-degrading factors (Aiyappa and Harris, 1976). The proteolytic activity of metalloproteases including serralysin was completely inhibited by EDTA, a chelating agent for divalent metal cations (Kida et al., 2007; Ishii et al., 2014a,b). The inhibitor screening for serralysin metalloprotease can be a good strategy to identify host-derived factors.
Orally infected S. marcescens cells did not produce serralysin during colonization of the midgut. Transcriptome analysis by RNA sequencing of both gut-colonizing bacteria and cultured bacteria showed that the expression patterns of several genes were completely different (Ohbayashi et al., 2019). When differentially expressed genes were classified into clusters of orthologous groups of the NCBI public database, the expression levels of most genes involved in cell motility, secretion, vesicular transport, and extracellular structures were downregulated in the gut-colonized bacteria (Baumann, 2005; McCutcheon and Moran, 2012; Ohbayashi et al., 2019). Among them, Type II and type IV secretion systems are involved in the extracellular transport of cell wall-degrading enzymes and endotoxins (Sandkvist, 2001; Murdoch et al., 2011; Gallique et al., 2017; Ohbayashi et al., 2019). There are changes in gut-colonizing bacteria, such as small size, increased stress sensitivity, loss of motility, and alteration of cell surfaces (Kim et al., 2013, 2015b; Ohbayashi et al., 2019). Based on these results, we investigated that the gene expression pattern of M3-colonizing S. marcescens would be different from that of free-living S. marcescens. As expected, the transcriptional and translational expression levels of serralysin genes dramatically decreased in M3-colonizing S. marcescens (Figure 6). This explained the reason the insecticidal serralysin was not found in M3 tissue.
The previous report that lipopolysaccharide (LPS) components are associated with the insecticidal activity of S. marcescens may provide a molecular evidence that M3-colonizing S. marcescens cannot produce serralysins. In general, LPS is a requisite component for maintaining the physiological properties, such as permeability and biofilm formation (Herlax et al., 2005; Ishii et al., 2014b; Hathroubi et al., 2016). The LPS layer comprises three regions: lipid A moieties, core oligosaccharides, and O-antigen polysaccharides. Among them, the O-antigen residue is highly associated with bacterial pathogenicity. The waaE mutant of Aeromonas hydrophila which has an O-antigen-deficient LPS is avirulent and loses the ability to stimulate the pro-phenoloxidase system in Tenebrio molitor larvae (Noonin et al., 2010). Enterohemorrhagic Escherichia coli O157 requires an LPS O-antigen for effective insecticidal activity against Bombyx mori (Miyashita et al., 2012). Similarly, the wecA gene of S. marcescens which is involved in the biosynthesis of LPS O-antigen is an important factor for the apoptotic death of host immune cells in B. mori (Ishii et al., 2012). The culture supernatant of the S. marcescens wecA mutant exhibits reduced hemolymph bleeding activity compared to that of the wild-type strain (Ishii et al., 2014b). In S. marcescens, rough-type LPS lacking O-antigen components induced significantly low hemolytic activity (Poole and Braun, 1988; Kurz et al., 2003). Symbiotic Burkholderia colonizing the M4 crypt lacks an O-antigen residue in R. pedestris (Kim et al., 2015b). These molecular evidences suggest the possibility that M3-colonizing S. marcescens cells may lack the LPS O-antigen.
In summary, we revealed that serralysin produced by orally infected S. marcescens cells was degraded by the host-derived factor present in the M1 tissue of R. pedestris. In addition, S. marcescens cells colonized in the M3 region did not produce the entomopathogenic serralysin. This study will provide insight into how orally infected entomopathogenic bacteria can colonize the host midgut without insecticidal activity and the molecular interactions between them in the gut.
Data Availability Statement
The original contributions presented in the study are included in the article/Supplementary Material, further inquiries can be directed to the corresponding authors.
Author Contributions
JL and D-WL conceived and designed the study and wrote the manuscript. JL performed the experiments and analyzed the data. All authors contributed to the article and approved the submitted version.
Funding
This research was supported by the Basic Science Research Program through the National Research Foundation of Korea (NRF) and funded by the Ministry of Education (NRF-2020R1I1A1A0106704312). This research was supported by a grant from the Korea Basic Science Institute (National Research Facilities and Equipment Center) and funded by the Ministry of Education (2019R1A6C1010044).
Conflict of Interest
The authors declare that the research was conducted in the absence of any commercial or financial relationships that could be construed as a potential conflict of interest.
Publisher’s Note
All claims expressed in this article are solely those of the authors and do not necessarily represent those of their affiliated organizations, or those of the publisher, the editors and the reviewers. Any product that may be evaluated in this article, or claim that may be made by its manufacturer, is not guaranteed or endorsed by the publisher.
Acknowledgments
We would like to thank Bok Luel Lee and Jong Uk Kim (Pusan National University) for providing serralysin antibodies for this study.
Supplementary Material
The Supplementary Material for this article can be found online at: https://www.frontiersin.org/articles/10.3389/fmicb.2022.913113/full#supplementary-material
References
Aiyappa, P., and Harris, J. (1976). The extracellular metalloprotease of Serratia marcescens: I. Purification and characterization. Mol. Cell. Biochem. 13, 95–100. doi: 10.1007/BF01837059
Akbar, N., Siddiqui, R., Iqbal, M., Sagathevan, K., and Khan, N. (2018). Gut bacteria of cockroaches are a potential source of antibacterial compound (s). Lett. Appl. Microbiol. 66, 416–426. doi: 10.1111/lam.12867
Azambuja, P., Feder, D., and Garcia, E. (2004). Isolation of Serratia marcescens in the midgut of Rhodnius prolixus: impact on the establishment of the parasite Trypanosoma cruzi in the vector. Exp. Parasitol. 107, 89–96. doi: 10.1016/j.exppara.2004.04.007
Baumann, P. (2005). Biology of bacteriocyte-associated endosymbionts of plant sap-sucking insects. Annu. Rev. Microbiol. 59, 155–189. doi: 10.1146/annurev.micro.59.030804.121041
Belas, R., Manos, J., and Suvanasuthi, R. (2004). Proteus mirabilis ZapA metalloprotease degrades a broad spectrum of substrates, including antimicrobial peptides. Infect. Immun. 72, 5159–5167. doi: 10.1128/IAI.72.9.5159-5167.2004
Bode, W., Gomis-Rüth, F.-X., and Stöckler, W. (1993). Astacins, serralysins, snake venom and matrix metalloproteinases exhibit identical zinc-binding environments (HEXXHXXGXXH and met-turn) and topologies and should be grouped into a common family, the ‘metzincins’. FEBS Lett. 331, 134–140. doi: 10.1016/0014-5793(93)80312-I
Bravo, A., Likitvivatanavong, S., Gill, S. S., and Soberón, M. (2011). Bacillus thuringiensis: a story of a successful bioinsecticide. Insect Biochem. Mol. Biol. 41, 423–431. doi: 10.1016/j.ibmb.2011.02.006
Cabral, C., Cherqui, A., Pereira, A., and Simões, N. (2004). Purification and characterization of two distinct metalloproteases secreted by the entomopathogenic bacterium Photorhabdus sp. strain Az29. Appl. Environ. Microbiol. 70, 3831–3838. doi: 10.1128/AEM.70.7.3831-3838.2004
Chadwick, J., Caldwell, S., and Chadwick, P. (1990). Adherence patterns and virulence for galleria mellonella larvae of isolates of Serratia marcescens. J. Invertebr. Pathol. 55, 133–134. doi: 10.1016/0022-2011(90)90044-7
Demaio, J., Pumpuni, C. B., Kent, M., and Beier, J. C. (1996). The midgut bacterial flora of wild Aedes triseriatus, Culex pipiens, and Psorophora columbiae mosquitoes. Am. J. Trop. Med. Hyg. 54, 219–223. doi: 10.4269/ajtmh.1996.54.219
Dubovskiy, I., Martemyanov, V., Vorontsova, Y., Rantala, M., Gryzanova, E., and Glupov, V. (2008). Effect of bacterial infection on antioxidant activity and lipid peroxidation in the midgut of Galleria mellonella L. larvae (Lepidoptera, Pyralidae). Comp. Biochem. Physiol. C Toxicol. Pharmacol. 148, 1–5. doi: 10.1016/j.cbpc.2008.02.003
Feng, K., Luo, J., Ding, X., and Tang, F. (2021). Transcriptome analysis and response of three important detoxifying enzymes to Serratia marcescens Bizio (SM1) in Hyphantria cunea (Drury)(Lepidoptera: Noctuidae). Pestic. Biochem. Physiol. 178:104922. doi: 10.1016/j.pestbp.2021.104922
Flyg, C., Kenne, K., and Boman, H. G. (1980). Insect pathogenic properties of Serratia marcescens: phage-resistant mutants with a decreased resistance to Cecropia immunity and a decreased virulence to Drosophila. Microbiology 120, 173–181. doi: 10.1099/00221287-120-1-173
Gallique, M., Bouteiller, M., and Merieau, A. (2017). The type VI secretion system: a dynamic system for bacterial communication? Front. Microbiol. 8:1454. doi: 10.3389/fmicb.2017.01454
Gonzalez-Ceron, L., Santillan, F., Rodriguez, M. H., Mendez, D., and Hernandez-Avila, J. E. (2003). Bacteria in midguts of field-collected Anopheles albimanus block Plasmodium vivax sporogonic development. J. Med. Entomol. 40, 371–374. doi: 10.1603/0022-2585-40.3.371
Grimont, P. A., and Grimont, F. (1978). The genus Serratia. Annu. Rev. Microbiol. 32, 221–248. doi: 10.1146/annurev.mi.32.100178.001253
Grizanova, E., Krytsyna, T., Surcova, V., and Dubovskiy, I. (2019). The role of midgut nonspecific esterase in the susceptibility of Galleria mellonella larvae to Bacillus thuringiensis. J. Invertebr. Pathol. 166:107208. doi: 10.1016/j.jip.2019.107208
Hathroubi, S., Hancock, M., Bosse, J., Langford, P., Tremblay, Y., Labrie, J., et al. (2016). Surface polysaccharide mutants reveal that absence of O antigen reduces biofilm formation of Actinobacillus pleuropneumoniae. Infect. Immun. 84, 127–137. doi: 10.1128/IAI.00912-15
Herlax, V., De Alaniz, M., and Bakás, L. (2005). Role of lipopolysaccharide on the structure and function of α-hemolysin from Escherichia coli. Chem. Phys. Lipids 135, 107–115. doi: 10.1016/j.chemphyslip.2005.02.009
Hillyer, J. F. (2016). Insect immunology and hematopoiesis. Dev. Comp. Immunol. 58, 102–118. doi: 10.1016/j.dci.2015.12.006
Ishii, K., Adachi, T., Hamamoto, H., and Sekimizu, K. (2014a). Serratia marcescens suppresses host cellular immunity via the production of an adhesion-inhibitory factor against immunosurveillance cells. J. Biol. Chem. 289, 5876–5888. doi: 10.1074/jbc.M113.544536
Ishii, K., Adachi, T., Hara, T., Hamamoto, H., and Sekimizu, K. (2014b). Identification of a Serratia marcescens virulence factor that promotes hemolymph bleeding in the silkworm, Bombyx mori. J. Invertebr. Pathol. 117, 61–67. doi: 10.1016/j.jip.2014.02.001
Ishii, K., Adachi, T., Imamura, K., Takano, S., Usui, K., Suzuki, K., et al. (2012). Serratia marcescens induces apoptotic cell death in host immune cells via a lipopolysaccharide-and flagella-dependent mechanism. J. Biol. Chem. 287, 36582–36592. doi: 10.1074/jbc.M112.399667
Kida, Y., Inoue, H., Shimizu, T., and Kuwano, K. (2007). Serratia marcescens serralysin induces inflammatory responses through protease-activated receptor 2. Infect. Immun. 75, 164–174. doi: 10.1128/IAI.01239-06
Kikuchi, Y., Meng, X.-Y., and Fukatsu, T. (2005). Gut symbiotic bacteria of the genus Burkholderia in the broad-headed bugs Riptortus clavatus and Leptocorisa chinensis (Heteroptera: Alydidae). Appl. Environ. Microbiol. 71, 4035–4043. doi: 10.1128/AEM.71.7.4035-4043.2005
Kim, J. K., Lee, J., Huh, Y. R., Jang, H. A., Kim, C.-H., Yoo, J. W., et al. (2015a). Burkholderia gut symbionts enhance the innate immunity of host Riptortus pedestris. Dev. Comp. Immunol. 53, 265–269. doi: 10.1016/j.dci.2015.07.006
Kim, J. K., Son, D. W., Kim, C.-H., Cho, J. H., Marchetti, R., Silipo, A., et al. (2015b). Insect gut symbiont susceptibility to host antimicrobial peptides caused by alteration of the bacterial cell envelope. J. Biol. Chem. 290, 21042–21053. doi: 10.1074/jbc.M115.651158
Kim, J. K., Won, Y. J., Nikoh, N., Nakayama, H., Han, S. H., Kikuchi, Y., et al. (2013). Polyester synthesis genes associated with stress resistance are involved in an insect-bacterium symbiosis. Proc. Natl. Acad. Sci. U. S. A. 110, E2381–E2389. doi: 10.1073/pnas.1303228110
Kruger, N. J. (2009). “The Bradford method for protein quantitation,” in The Protein Protocols Handbook. ed. J. M. Walker (Totowa, NJ: Springer), 17–24.
Kurz, C. L., Chauvet, S., Andrès, E., Aurouze, M., Vallet, I., Michel, G. P., et al. (2003). Virulence factors of the human opportunistic pathogen Serratia marcescens identified by in vivo screening. EMBO J. 22, 1451–1460. doi: 10.1093/emboj/cdg159
Lee, J., Cha, W. H., and Lee, D.-W. (2022). Multiple precursor proteins of Thanatin isoforms, an antimicrobial peptide associated With the gut Symbiont of Riptortus pedestris. Front. Microbiol. 12:796548. doi: 10.3389/fmicb.2021.796548
Lee, J., Kim, C.-H., Jang, H. A., Kim, J. K., Kotaki, T., Shinoda, T., et al. (2019). Burkholderia gut symbiont modulates titer of specific juvenile hormone in the bean bug Riptortus pedestris. Dev. Comp. Immunol. 99:103399. doi: 10.1016/j.dci.2019.103399
Lee, D. J., Lee, J., Jang, H. A., Ferrandon, D., and Lee, B. L. (2017a). An antimicrobial protein of the Riptortus pedestris salivary gland was cleaved by a virulence factor of Serratia marcescens. Dev. Comp. Immunol. 67, 427–433. doi: 10.1016/j.dci.2016.08.009
Lee, J., Mao, X., Lee, Y. S., Lee, D. J., Kim, J., Kim, J. K., et al. (2020). Putative host-derived growth factors inducing colonization of Burkholderia gut symbiont in Riptortus pedestris insect. Dev. Comp. Immunol. 104:103570. doi: 10.1016/j.dci.2019.103570
Lee, J., Park, K.-E., Lee, S. A., Jang, S. H., Eo, H. J., Jang, H. A., et al. (2017b). Gut symbiotic bacteria stimulate insect growth and egg production by modulating hexamerin and vitellogenin gene expression. Dev. Comp. Immunol. 69, 12–22. doi: 10.1016/j.dci.2016.11.019
Li, X., Schuler, M. A., and Berenbaum, M. R. (2007). Molecular mechanisms of metabolic resistance to synthetic and natural xenobiotics. Annu. Rev. Entomol. 52, 231–253. doi: 10.1146/annurev.ento.51.110104.151104
Ma, G., Rahman, M. M., Grant, W., Schmidt, O., and Asgari, S. (2012). Insect tolerance to the crystal toxins Cry1Ac and Cry2Ab is mediated by the binding of monomeric toxin to lipophorin glycolipids causing oligomerization and sequestration reactions. Dev. Comp. Immunol. 37, 184–192. doi: 10.1016/j.dci.2011.08.017
Massaoud, M. K., Marokházi, J., and Venekei, I. (2011). Enzymatic characterization of a serralysin-like metalloprotease from the entomopathogen bacterium, Xenorhabdus. Biochim. Biophys. Acta Proteins Proteom. 1814, 1333–1339. doi: 10.1016/j.bbapap.2011.05.008
McCutcheon, J. P., and Moran, N. A. (2012). Extreme genome reduction in symbiotic bacteria. Nat. Rev. Microbiol. 10, 13–26. doi: 10.1038/nrmicro2670
Miyashita, A., Iyoda, S., Ishii, K., Hamamoto, H., Sekimizu, K., and Kaito, C. (2012). Lipopolysaccharide O-antigen of enterohemorrhagic Escherichia coli O157: H7 is required for killing both insects and mammals. FEMS Microbiol. Lett. 333, 59–68. doi: 10.1111/j.1574-6968.2012.02599.x
Murdoch, S. L., Trunk, K., English, G., Fritsch, M. J., Pourkarimi, E., and Coulthurst, S. J. (2011). The opportunistic pathogen Serratia marcescens utilizes type VI secretion to target bacterial competitors. J. Bacteriol. 193, 6057–6069. doi: 10.1128/JB.05671-11
Nehme, N. T., Liégeois, S., Kele, B., Giammarinaro, P., Pradel, E., Hoffmann, J. A., et al. (2007). A model of bacterial intestinal infections in Drosophila melanogaster. PLoS Pathog. 3:e173. doi: 10.1371/journal.ppat.0030173
Noonin, C., Jiravanichpaisal, P., Söderhäll, I., Merino, S., Tomás, J. M., and Söderhäll, K. (2010). Melanization and pathogenicity in the insect, Tenebrio molitor, and the crustacean, Pacifastacus leniusculus, by Aeromonas hydrophila AH-3. PLoS One 5:e15728. doi: 10.1371/journal.pone.0015728
Ohbayashi, T., Futahashi, R., Terashima, M., Barrière, Q., Lamouche, F., Takeshita, K., et al. (2019). Comparative cytology, physiology and transcriptomics of Burkholderia insecticola in symbiosis with the bean bug Riptortus pedestris and in culture. ISME J. 13, 1469–1483. doi: 10.1038/s41396-019-0361-8
Ohbayashi, T., Takeshita, K., Kitagawa, W., Nikoh, N., Koga, R., Meng, X.-Y., et al. (2015). Insect’s intestinal organ for symbiont sorting. Proc. Natl. Acad. Sci. U. S. A. 112, E5179–E5188. doi: 10.1073/pnas.1511454112
Poole, K., and Braun, V. (1988). Influence of growth temperature and lipopolysaccharide on hemolytic activity of Serratia marcescens. J. Bacteriol. 170, 5146–5152. doi: 10.1128/jb.170.11.5146-5152.1988
Ramsey, J. S., Rider, D. S., Walsh, T. K., De Vos, M., Gordon, K., Ponnala, L., et al. (2010). Comparative analysis of detoxification enzymes in Acyrthosiphon pisum and Myzus persicae. Insect Mol. Biol. 19, 155–164. doi: 10.1111/j.1365-2583.2009.00973.x
Raymann, K., Coon, K. L., Shaffer, Z., Salisbury, S., and Moran, N. A. (2018). Pathogenicity of Serratia marcescens strains in honey bees. MBio 9:e01649-18. doi: 10.1128/mBio.01649-18
Salamone, P., and Wodzinski, R. (1997). Production, purification and characterization of a 50-kDa extracellular metalloprotease from Serratia marcescens. Appl. Microbiol. Biotechnol. 48, 317–324. doi: 10.1007/s002530051056
Sandkvist, M. (2001). Type II secretion and pathogenesis. Infect. Immun. 69, 3523–3535. doi: 10.1128/IAI.69.6.3523-3535.2001
Straif, S. C., Mbogo, C. N. M., Toure, A. M., Walker, E. D., Kaufman, M., Toure, Y. T., et al. (1998). Midgut bacteria in Anopheles gambiae and An. Funestus (Diptera: Culicidae) from Kenya and Mali. J. Med. Entomol. 35, 222–226. doi: 10.1093/jmedent/35.3.222
Tambong, J., Xu, R., Sadiku, A., Chen, Q., Badiss, A., and Yu, Q. (2014). Molecular detection and analysis of a novel metalloprotease gene of entomopathogenic Serratia marcescens strains in infected Galleria mellonella. Can. J. Microbiol. 60, 203–209. doi: 10.1139/cjm-2013-0864
Tartar, A., Wheeler, M. M., Zhou, X., Coy, M. R., Boucias, D. G., and Scharf, M. E. (2009). Parallel metatranscriptome analyses of host and symbiont gene expression in the gut of the termite Reticulitermes flavipes. Biotechnol. Biofuels 2:25. doi: 10.1186/1754-6834-2-25
Wilf, N. M., Williamson, N. R., Ramsay, J. P., Poulter, S., Bandyra, K. J., and Salmond, G. P. (2011). The RNA chaperone, Hfq, controls two luxR-type regulators and plays a key role in pathogenesis and production of antibiotics in Serratia sp. ATCC 39006. Environ. Microbiol. 13, 2649–2666. doi: 10.1111/j.1462-2920.2011.02532.x
Wu, P., Sun, P., Nie, K., Zhu, Y., Shi, M., Xiao, C., et al. (2019). A gut commensal bacterium promotes mosquito permissiveness to arboviruses. Cell Host Microbe 25, 101.e5–112.e5. doi: 10.1016/j.chom.2018.11.004
Keywords: insect symbiosis, serralysin, detoxification, Riptortus pedestris, Serratia marcescens Db11
Citation: Lee J and Lee D-W (2022) Insecticidal Serralysin of Serratia marcescens Is Detoxified in M3 Midgut Region of Riptortus pedestris. Front. Microbiol. 13:913113. doi: 10.3389/fmicb.2022.913113
Edited by:
Robert Czajkowski, University of Gdansk, PolandReviewed by:
Xiaoli Bing, Nanjing Agricultural University, ChinaLuis Felipe Muriel-Millán, Universidad Nacional Autónoma de México, Mexico
Copyright © 2022 Lee and Lee. This is an open-access article distributed under the terms of the Creative Commons Attribution License (CC BY). The use, distribution or reproduction in other forums is permitted, provided the original author(s) and the copyright owner(s) are credited and that the original publication in this journal is cited, in accordance with accepted academic practice. No use, distribution or reproduction is permitted which does not comply with these terms.
*Correspondence: Junbeom Lee, d2luZDIwMDBuZXRAa3MuYWMua3I=; Dae-Weon Lee, ZGFld2VvbmxlZUBrcy5hYy5rcg==