- 1Skryabin Institute of Bioengineering, Research Center of Biotechnology of the Russian Academy of Sciences, Moscow, Russia
- 2Faculty of Biology, Lomonosov Moscow State University, Moscow, Russia
- 3Department of Microbiology, University of Bayreuth, Bayreuth, Germany
- 4Instituto de Microbiologia Paulo de Góes, Universidade Federal do Rio de Janeiro, Rio de Janeiro, Brazil
- 5Department of Marine Science, The University of Texas at Austin, Austin, TX, United States
- 6SciBear OU, Tallinn, Estonia
Magnetosome synthesis in magnetotactic bacteria (MTB) is regarded as a very ancient evolutionary process that dates back to deep-branching phyla. Magnetotactic bacteria belonging to one of such phyla, Nitrospirota, contain the classical genes for the magnetosome synthesis (e.g., mam, mms) and man genes, which were considered to be specific for this group. However, the recent discovery of man genes in MTB from the Thermodesulfobacteriota phylum has raised several questions about the inheritance of these genes in MTB. In this work, three new man genes containing MTB genomes affiliated with Nitrospirota and Thermodesulfobacteriota, were obtained. By applying reconciliation with these and the previously published MTB genomes, we demonstrate that the last common ancestor of all Nitrospirota was most likely not magnetotactic as assumed previously. Instead, our findings suggest that the genes for magnetosome synthesis were transmitted to the phylum Nitrospirota by horizontal gene transfer (HGT), which is the first case of the interphylum transfer of magnetosome genes detected to date. Furthermore, we provide evidence for the HGT of magnetosome genes from the Magnetobacteriaceae to the Dissulfurispiraceae family within Nitrospirota. Thus, our results imply a more significant role of HGT in the MTB evolution than deemed before and challenge the hypothesis of the ancient origin of magnetosome synthesis.
Introduction
Bacteria that have an ability to form magnetosomes, magnetotactic bacteria (MTB), were found in phylogenetically distant taxa such as Pseudomonadota (former Proteobacteria, replaced by Oren and Garrity, 2021), Nitrospirota, Omnitrophota, Latescibacterota, Planctomycetota, Nitrospinota, Hydrogenedentota, Elusimicrobiota, Fibrobacterota, Riflebacteria, Bdellovibrionota, UBA10199, and Thermodesulfobacteriota [the taxon Deltaproteobacteria has been reclassified into four phyla: Thermodesulfobacteriota, Myxococcota, SAR324, and Bdellovibrionota (Waite et al., 2020)] (Lin et al., 2018, 2020b; Uzun et al., 2020; Gareev et al., 2021). Magnetotactic bacteria are primarily found in stratified aquatic environments, where magnetosomes in couple with aerotaxis help cells react to environmental fluctuations (Lefèvre and Bazylinski, 2013). That behavior is called magneto-aerotaxis or, simply, magnetotaxis (Frankel et al., 1997). The question of when did magnetotaxis emerge and how did it spread among such distant taxa is still a matter of debate. The first hypotheses concerning the origin of magnetotaxis appeared after discovering that the type of magnetosome mineral, magnetite or greigite, reflects phylogenetic affiliation. It has been suggested that magnetite-based and greigite-based magnetotaxis emerged independently (DeLong et al., 1993). Later, as data on the diversity of MTB increased, the idea of a polyphyletic origin was abandoned (Abreu et al., 2011), especially after discovering Desulfamplus magnetovallimortis BW-1, capable of synthesizing crystals of both types (Lefèvre et al., 2013a,b). One of the latest assumptions was that magnetotaxis is an ancient physiological trait with a single origin. The magnetosome gene cluster’s (MGC) evolutionary history mainly displays vertical inheritance, accompanied by multiple independent losses during bacterial diversification (Lin et al., 2018, 2020a). According to the latest data, it has been suggested that a common ancestor with MGC most likely appeared before the divergence of the phyla Nitrospirota and Pseudomonadota, which are considered to be deep-branching phyla (Lin et al., 2017b). Alternatively, it was proposed that MGC could be transferred undetectably early between the base of these phyla soon after divergence (Lin et al., 2017b). It could have happened during the mid-Archean Eon or even earlier (Lin et al., 2020b; Uzun et al., 2020). It has also been proposed that some ancient organisms could form primitive magnetosomes and, therefore, bacterial magnetotaxis would be a primal physiological process on Earth (Lin et al., 2020a,b). Magnetosomes could help ancient bacteria to be protected against environmental stresses on early Earth (e.g., ultraviolet radiation, toxic reactive oxygen species; Lin et al., 2020a). This was supported by the presence of MTB in groups close to the last bacterial common ancestor (LBCA), which led to the assumption that the closest descendants of LBCA or even the last universal common ancestor (LUCA) potentially could synthesize primitive magnetosomes (Lin et al., 2020b). The lack of evidence for horizontal gene transfer (HGT) above the class level, including transfer between phyla, indeed supports the theory of vertical inheritance accompanied by multiple losses of MGC. At the same time, the evolutionary history of MGC at lower ranks is intricate (Lin et al., 2018). For example, there is evidence for HGT before and after the delineation of Magnetospirillum, Magnetovibrio, and Magnetospira genera (Monteil et al., 2018, 2020) and within the order Magnetococcales (Koziaeva et al., 2019), suggesting a more complex evolutionary history of MGC at lower taxonomic ranks (families, genera, and species ranks).
Some magnetosome genes are essential for magnetosome formation. These genes called mam genes, nine of them (mamA, -В, -M, -K, -P, -Q, -E, -O, and -I), can be found in all MGCs (Uebe and Schüler, 2016; Lin et al., 2017a). Except for mam genes, there are some group-specific genes. For example, mms genes are found only in Pseudomonadota, Nitrospinota, and SAR324, mad genes – in Thermodesulfobacteriota and Nitrospirota, and man genes were initially found only in the genomes of Nitrospirota phylum (Uebe and Schüler, 2016). However, several genomes of Thermodesulfobacteriota containing man genes were recently obtained (Uzun et al., 2020). Group-specific genes are assumed to be involved in the magnetosomes’ different shapes and sizes. However, their functions are not known for certain (Uebe and Schüler, 2016). Also, the feoAm and feoBm genes are known to be involved in iron transport for magnetosome synthesis (Uebe and Schüler, 2016).
In the current study, we sequenced and analyzed the genomes of the three magnetotactic bacteria previously identified in a freshwater lake Beloe Bordukovskoe (Koziaeva et al., 2020). As a result, we propose two novel Candidatus genera and three novel species of man-genes containing MTB. The comparative genome analysis and tree reconciliations revealed the instances of interfamily and interphylum HGT of magnetosome genes in the deep-branching phyla Thermodesulfobacteriota and Nitrospirota. Our findings contribute to understanding of the origin and evolution of MGC.
Materials and methods
Sampling, microscopic observation, and DNA extraction
Water and sediment samples were collected from freshwater Lake Beloe Bordukovskoe to form a three-liter microcosm. Magnetotactic bacteria from this microcosm were concentrated using the MTB-CoSe approach as described in Koziaeva et al. (2020). Part of the magnetically concentrated cells was used for DNA isolation, and the rest were fixed in paraformaldehyde for morphology analyses. The DNA was used in the present study for metagenome sequencing. Fluorescence in situ hybridization combined with transmission electron microscopy (FISH-TEM) was conducted as described before (Li et al., 2017) with specific probe LBB01 (Koziaeva et al., 2020). For high-resolution TEM (HRTEM), the same grids prepared for conventional TEM were imaged using a Tecnai G2 F20 FEG (FEI, United States) operated at 200 kV and equipped with a 4 k × 4 k Gatan UltraScan 1,000 CCD camera. Measurements and fast Fourier transform (FFT) from the HRTEM images were obtained using Digital Micrograph software (Gatan, United States).
Genome sequencing, assembly, annotation, and metabolic pathways reconstructions
To obtain sufficient DNA for metagenomic sequencing, whole-genome amplification was carried out using the multiple displacement amplification technique with the Genomiphi V2 DNA Amplification Kit (GE Healthcare, United States). This approach has been widely used previously in various works (Kolinko et al., 2015; Monteil et al., 2019; Zhang et al., 2020b). The amplified DNA was purified by sodium acetate precipitation. All stages of work with DNA were carried out according to the manufacturer’s recommendations.
For the DNA obtained after precipitation, metagenomic sequencing was performed. Short and long reads were obtained using the DNBSEQ (MGI) and Oxford Nanopore Technologies (ONT) platforms, respectively. A DNA library was constructed using the MGIEasy universal DNA library prep to obtain short reads. DNA library sequencing was performed using the DNBSEQ-G400 platform (MGI Tech, China) with pair-end 150-bp reads. Raw reads were quality checked with FastQC v.0.11.9.1 Low-quality reads were removed using Trimmomatic v0.39 (Bolger et al., 2014). A DNA library was constructed using the NEBNext Companion Module for ONT Ligation Sequencing kit to obtain long reads. The library sequencing was performed on a MinION sequencing device (Oxford Nanopore Technologies, UK) using an R9.4.1 flow cell (FLO-MIN106D). Guppy v3.4.4, available from the Oxford Nanopore Technology community website, was used for basecalling, demultiplexing, and quality trimming ONT-passed long reads.
Long and short trimmed reads were hybrid de novo assembled using SPAdes v3.13.0 with the “-meta” flag (Bankevich et al., 2012). Metagenome assembled genomes (MAGs) reconstruction was conducted using the MaxBin2 v2.2.7 (Wu et al., 2015), METABAT2 v2.15 (Kang et al., 2019), and Busy Bee Web (Laczny et al., 2017) with standard parameters. The DAS Tool v1.1.3 was used for choosing consensus assemblies for the obtained MAGs (Sieber et al., 2018). The MAG LBB01 was manually curated and reassembled. Briefly, short and long reads were mapped to the resulting assembly using Bowtie 2 v2.3.5.110 (Langmead and Salzberg, 2012) and Minimap2 v2.17 (Li, 2018), respectively. The mapped reads were assembled into a circular genome with Unicycler v0.4.6 (Wick et al., 2017). The quality metrics were assessed using the QUAST v5.0.2 (Gurevich et al., 2013). The genome coverages were evaluated by QualiMap 2 v2.2.29 (Okonechnikov et al., 2016) and Bowtie 2 v2.3.2 (Langmead and Salzberg, 2012). Genome completeness and contamination were estimated using CheckM v1.1.3 (Parks et al., 2015). RefineM v0.1.2 (Parks et al., 2017) was used to remove contamination based on taxonomic assignments. The identification of protein-coding sequences and primary annotation was performed using the NCBI Prokaryotic Genome Annotation Pipeline (PGAP v5.3; Tatusova et al., 2016) and Rapid Annotations Subsystems Technology (RAST) online service (Aziz et al., 2008). The putative MGCs were determined using local BLAST and comparison with reference sequences of magnetotactic bacteria. The protein-coding sequences were annotated using the Kyoto Encyclopedia of Genes and Genomes (KEGG) framework (Kanehisa and Sato, 2020). Functional pathway prediction was performed using KEGG Mapper.
Phylogenetic analyses and genome index calculation
The GTDB-Tk v1.6.0 (Chaumeil et al., 2019) “classify_wf” command was used to find 120 single-copy bacterial marker protein sequences, construct their concatenated multiple alignments, and get the MAG’s taxonomic assignment using the GTDB r202 database (Parks et al., 2018). For genome-based phylogenetic analyses, all available MTB genomes from different phyla and non-MTB Nitrospirota and Thermodesulfobacteriota genomes from the GTDB r95 database were selected (Supplementary Table S1). The protein sequences of the same MGC gene (Mad26, Mad25, Mad24, Mad23, MamO-Cter, Man6, Man5, Man4, MamQ, MamE, MamI, MamA, Mad2, MamB, MamQ-2, Mad31, MamM, MamP, Man3, Mad10, Man2, MamK, Man1) from different MTB taxonomic groups were independently aligned using MAFFT (Katoh and Standley, 2013). PhyloSuite v1.2.2 (Zhang et al., 2020a) was used to concatenate MGC protein sequences. For MamA, -B, E, -I, -K, -M, -P, -Q protein sequences, and for the concatenated MGC protein sequences, the total number of protein sequences was reduced. This reduction was by taking off sequences from genomes that do not belong to Nitrospirota or Thermodesulfobacteriota phyla. This procedure was necessary to reconcile in the Notung because the program does not work with trees with over 150 representatives. Maximum-likelihood phylogenetic trees were built with IQ-TREE v1.6.12 (Nguyen et al., 2015) using evolutionary models selected by ModelFinder (Wong et al., 2017). Branch supports were obtained with 1,000 ultrafast bootstraps (Hoang et al., 2017). Trees were visualized with iTOL v6.5 (Letunic and Bork, 2019). One hundred and twenty single-copy bacterial marker protein sequences tree (hereinafter called «species tree») was rooted to Fusobacteriota (Coleman et al., 2021). Trees of the protein sequences of the same MGC gene (hereafter called «protein trees») were rooted to midpoint.
The average nucleotide identity (ANI) was calculated using the FastANI v1.33 tool (Jain et al., 2018). Average amino acid identity (AAI) was calculated using CompareM v0.1.2 (No Title).2 Digital DNA–DNA hybridization (dDDH) values were determined using Genome-to-Genome Distance Calculator (GGDC) v3.0 online software (Meier-Kolthoff et al., 2013). The pairwise percentage of conserved proteins (POCP) was calculated using the script runPOCP.sh (Pantiukh and Grouzdev, 2017; Grouzdev et al., 2018), based on the previously described approach (Qin et al., 2014).
Reconciliation
The evolution of proteins involved in magnetosome biogenesis was studied by reconciling protein trees and their concatenation with the species tree. Reconciliation is a method of annotating gene trees (protein trees in this work) with evolutionary events along with mapping them onto a species tree (Duchemin et al., 2018). Two programs, Notung v.2.9 (Stolzer et al., 2012) and Ranger-DTL v2.0 (Bansal et al., 2018), were used for reconciliation. Notung algorithm captures gene duplication (D), transfer (T), and loss (L) driving tree incongruence and infers all optimal solutions to finally report the complete and temporally feasible event histories giving the data. Notung was used with standard parameters: D = 1.5, T = 3, L = 1. Ranger-DTL, in turn, not only assigns one of the possible evolutionary events to nodes on the protein tree but also gives the probability of an ongoing evolutionary event, thereby refining the Notung results. Ranger-DTL analysis was run with “Ranger-DTL” command and 100 simulations with default parameters (D = 2, T = 3, L = 1). The “AggregateRanger” command was used to compute support values for the most frequent mappings that are the donor species. The reconciliation results of Notung were protein trees showing the most likely evolutionary paths taken. Ranger-DTL produced text files that indicated the most likely evolution events that may have occurred within the 100 simulations for each leaf node of the studied protein tree. Further, the results of all 100 simulations were aggregated into one resulting file. This file contained information on how many times out of 100 certain evolutionary events were found for each leaf node. Notung and Ranger-DTL reconciliations for each protein tree were recorded in a table (Supplementary Table S2). Based on data from the table, the probability of a particular evolutionary event was calculated.
Results
Morphology of MTB cells and magnetosomes
In the previous study (Koziaeva et al., 2020), sediment samples collected from freshwater Lake Beloe Bordukovskoe contained an abundant population of MTB with various morphology. In that article six MTB were identified using 16S rRNA and MamK phylogenetic analyses combined with the FISH-TEM approach. Among them, there were two Nitrospirota MTB (LBB01 and LBB02) and one Thermodesulfobacteriota MTB (LBB04; Koziaeva et al., 2020). The population was dominated by a magnetotactic vibrio LBB01 allowing further analyses of its cell and magnetosome morphology using FISH-TEM.
The LBB01 probe hybridized only with vibrioid-shaped bacteria, as observed in Figures 1A–C. The phase-contrast image of a magnetically enriched sample (Figure 1A) showed vibrioid-shaped and ovoid bacteria. Figure 1B shows that all cells hybridized with the domain-specific probe EUB. Whereas with the specific probe on LBB01, only vibrioid-shaped bacteria showed fluorescence (Figure 1C). TEM images of the same area used for FISH analysis (Figure 1D) revealed that this MTB group presented a thick chain of magnetosomes organized along the long axis of the bacterial cell body (Figure 1E). The observed magnetosomes were anisotropic (Figure 1F) and presented [111] as the elongation axis. In addition, the fast Fourier transform (FFT) pattern of crystalline structure corresponded to magnetite (Figure 1G). LBB01 cells were 2.0 ± 0.4 μm long and 0.5 ± 0.1 μm wide (n = 32). They contained 33 ± 9 magnetosomes per cell (n = 32), which formed one bundle of bullet-shaped magnetosomes located close to each other. The bundle consisted of two to three twisted filaments of magnetosomes. The tips of the crystals were not always sequentially oriented and were sometimes oriented in opposite directions. The structure of the chains resembled that in strains MWB-1 and Ca. Magnetobacterium bavaricum TM-1 (Li et al., 2015). Immature crystals were found in different parts of the chains. A detailed analysis of the magnetosome size showed that they varied within 108 ± 21.1 nm × 45 ± 8.1 nm (length × width) with a shape factor of 0.45 (n = 1,061; Supplementary Figure S1).
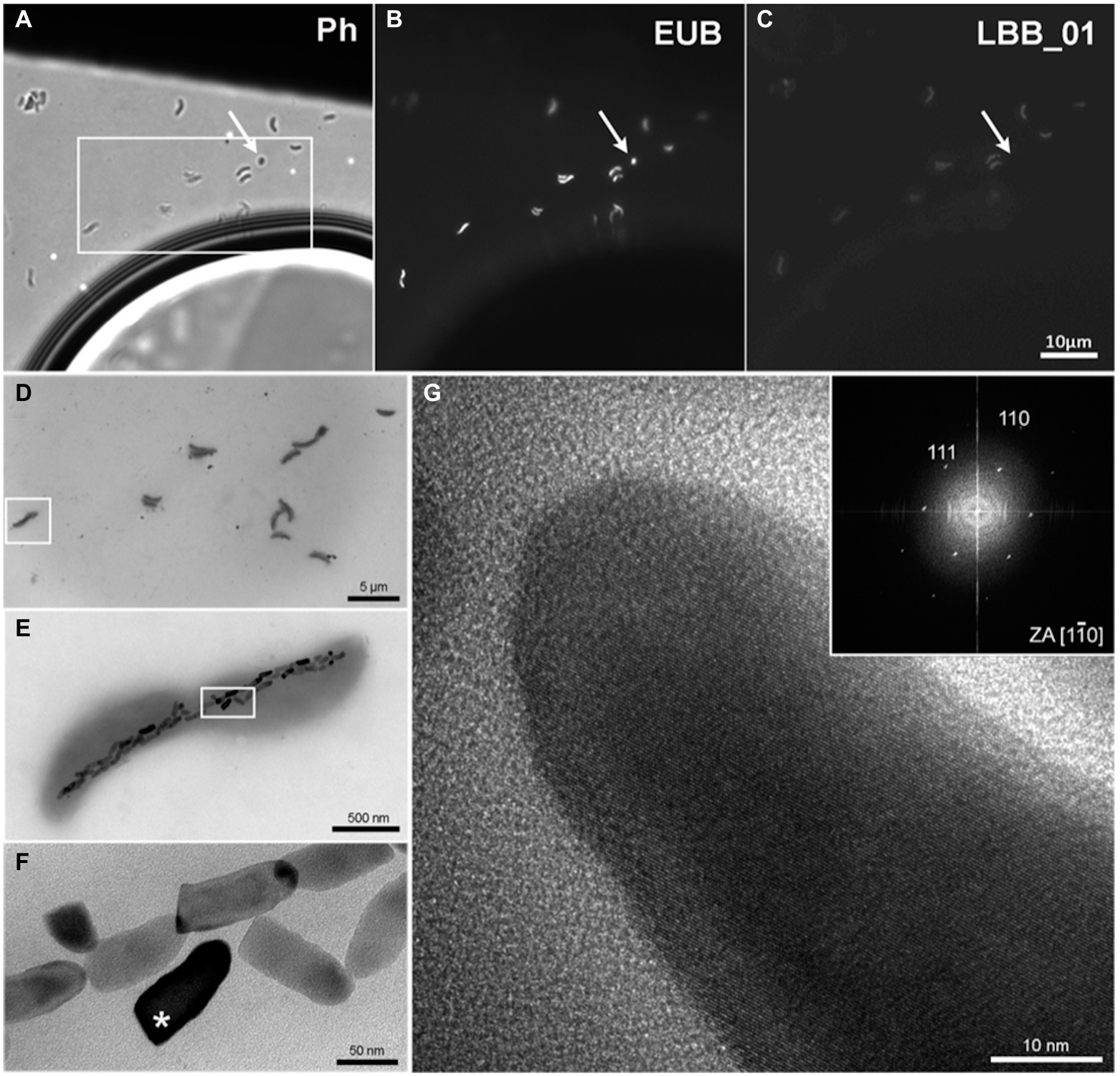
Figure 1. FISH-TEM of the MTB Ca. Magnetomonas plexicatena LBB01 and HRTEM of its magnetosomes. (A) phase contrast image of magnetic enrich environmental sample on Formvar coated TEM grids. (B) bacteria observed on image A hybridized with EUB probe. (C) image after hybridization with the specific design probe for Ca. Magnetomonas plexicatena species. The arrow highlights a small coccus present in the sample that was not hybridized with the specific probe. (D) TEM image of the same region marked as a box (A). (E) higher magnification of the square area (D) showing a chain of anisotropic magnetosomes organized along the long axis of the cell. (F) higher magnification image of the area inside the square (E). Magnetosomes in this cell demonstrate rough edges and a bullet shape. (G) HRTEM of magnetosome mark with an asterisk (F). The FFT of the crystalline structure of the magnetosome supports that they consist of magnetite that presents an [111] elongation axis.
Besides, as already described in our recently published article (Koziaeva et al., 2020), a small number of ovoid- and rod-shaped magnetotactic cells were present, which represented LBB02 and LBB04, respectively. LBB02 were ~1.5 μm long and 1.2 μm wide and contained two chains of elongated bullet-shaped magnetosomes. LBB02 is closely related to Ca. Magnetominusculus xianensis HCH-1, which morphology has not been identified previously (Lin et al., 2017b). LBB02 and HCH-1 had a high 16S rRNA gene similarity (98.2%). Probably, HCH-1 and other members of the genus Ca. Magnetominusculus may be ovoid-shaped. Considering the previous results (Koziaeva et al., 2020), LBB04 is a rod-shaped cell ~2.5 μm long and 1.1 μm wide with disorganized elongated bullet-shaped magnetosomes.
Genome reconstruction and phylogenomic analyses
The genomes of the enriched MTB were assembled from metagenome sequencing. To this end, we generated 115,589,666 (2 × 150-bp) short paired-end (14.9 Gb) and 304,996 long reads (2.1 Gb of data, mean read length of 3,459 bp, and N50 of 5,251 bp). A hybrid assembly and metagenome-assembled genomes (MAG) reconstruction resulted in three MAGs that met the criteria for Genome taxonomy database (GTDB) representative genomes (Parks et al., 2018): completeness ≥50% and contamination <5%. Full or partial 16S rRNA sequences were detected, helping to link genomic data to the morphology from the previous work. First, a complete circular chromosome of the LBB01 genome, 3.27 Mbp long with a GC composition of 42.0%, was assembled (Supplementary Table S3). Also, a high-quality draft genome for LBB02 was obtained with a GC content of 47.0% and 3.27 Mbp in size. Besides, a draft genome for LBB04, with a length of 4.49 Mbp and a GC content of 50.5%, was obtained.
According to GTDB, the reconstructed genomes of LBB01 and LBB02 were assigned to candidate family Ca. Magnetobacteriaceae of the phylum Nitrospirota, while LBB04 was assigned to the order Syntrophales of the Thermodesulfobacteriota phylum (Supplementary Table S3). The phylogenomic tree based on 120 single-copy protein sequences (Figure 2) confirmed that LBB01 and LBB02 belong to Ca. Magnetobacteriaceae family and LBB04 – to Thermodesulfobacteriota phylum. The genomes formed five clades within Ca. Magnetobacteriaceae, likely corresponding to five genera. One of the clades included the previously described strain Ca. Magnetobacterium casensis MYR-1 (Li et al., 2010). LBB01 genome formed a separate cluster together with the nDJH6bin1, nDJH13bin19, nDJH8bin8, and nDJH14bin5 genomes. Another clade included LBB02, Ca. Magnetominusculus xianensis HCH-1, nMYbin6, MYbin6, nDJH5bin4, nHCHbin2, HCHbin1, nDJH8bin6, nDJH14bin7, nDJH8bin13, and nDJH13bin15 (Lin et al., 2017b, 2018, 2020b). The other two clades include single genomes of Ca. Magnetomicrobium cryptolimnococcus XYC and nDJH13bin3, respectively (Zhang et al., 2021). Two Nitrospirota genomes, nDJH8bin7 and nDJH14bin9, belong to a separate family. To confirm the results of the phylogenetic analyses, genomic indices were calculated. The average amino acid identity (AAI) values within the designated clades of Ca. Magnetobacteriaceae ranged from 75% to 100%, while values between them ranged from 55% to 62% (Supplementary Table S4). It has been recently proposed that members of the same genus can have 65%–95% AAI values (Konstantinidis et al., 2017). Considering the branching of the phylogenomic tree and the AAI values, LBB01 can be affiliated to a separate genus (AAI < 65%), whereas LBB02 belongs to the genus Ca. Magnetominusculus (AAI > 65%). The percentage of orthologous conserved proteins (POCP) between genomes of the same clade was around 60% or higher, supporting the conclusions drawn from the phylogenetic and AAI analyses (Supplementary Table S4). To determine taxonomy at the species level, average nucleotide identity (ANI) and digital DNA–DNA hybridization (dDDH) were calculated (Supplementary Tables S5, S6). As a result, ANI and dDDH values for LBB01 and LBB02 with closely related genomes were below the species separation threshold (<95%–96% and <70% respectively; Goris et al., 2007; Auch et al., 2010), indicating that LBB01 and LBB02 represent two novel species. Therefore, we propose names Ca. Magnetomonas plexicatena and Ca. Magnetominusculus linsii for LBB01 and LBB02, respectively.
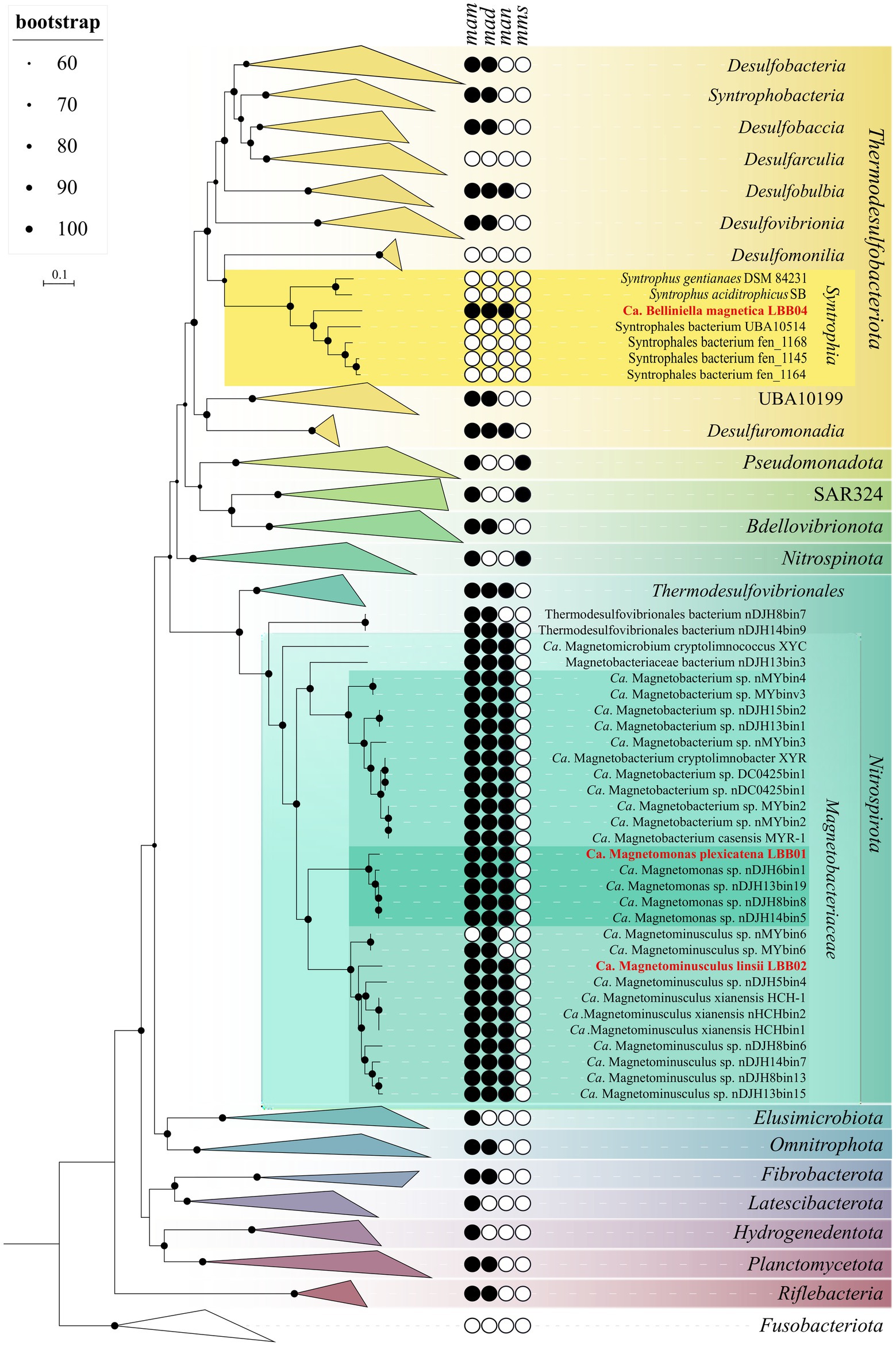
Figure 2. Maximum-likelihood phylogenomic tree of all previously known and the MTB genomes obtained in this work and their close non-MTB relatives. The tree was inferred from concatenated 120 bacterial single-copy marker proteins constructed with evolutionary model LG + F + I + G4. Branch supports were obtained with 1,000 ultrafast bootstraps. The scale bar represents amino acid substitutions per site. The complete tree is shown in figshare data (Uzun et al., 2022). Genomes obtained in this work are highlighted in red.
As already mentioned, the genome of LBB04 clustered with representatives of the Syntrophales order within the Thermodesulfobacteriota phylum. According to the relative evolutionary divergence values and genome-based taxonomy proposed by the GTDB database, the order Syntrophales consists of 17 families. However, only two of them, Syntrophaceae and Smithellaceae, include validly described species. LBB04 appears phуlogenetically distant from any of the known Syntrophales strains. The most closely related cultivated strains were Syntrophus aciditrophicus SB and Syntrophus gentianae DSM 84231 with 93.5%16S rRNA sequence similarity according to EzBioCloud (Yoon et al., 2017). According to the criteria for the description of new taxa, the level of similarity of 16S rRNA sequences for members of the same genus is more than 94.5%–95.0% (Yarza et al., 2014; Konstantinidis et al., 2017). In addition, the AAI values between LBB04 and species of Syntrophus and Smithella were 57%–58%, which is lower than the AAI values between these genera (59%–60%). AAI values between organisms below 65% indicate the need to assign them to different genera (Konstantinidis et al., 2017). Thus, based on the results of phylogenetic analysis, 16S rRNA sequence similarity, and AAI values, we assign LBB04 to a new genus and species and propose the name Ca. Belliniella magnetica.
Genomic reconstruction of key metabolic functions
Genome analysis suggests that LBB01 contains the key enzymes to exploit the Wood–Ljungdahl pathway for CO2 fixation, the trait that appears common among Nitrospirota MTB. It has been previously hypothesized that Ca. Magnetobacterium casensis, a closely related species to LBB01, might utilize the reductive tricarboxylic acid cycle (rTCA) as an additional pathway for CO2 assimilation (Lin et al., 2014). However, we found that LBB01, LBB02, Ca. Magnetobacterium casensis and other related Nitrospirota lack the key enzymes for rTCA, i.e., fumarate reductase, ATP-citrate (pro-S)-lyase (aclAB), and isocitrate dehydrogenase (idh), suggesting that Wood–Ljungdahl is likely the only CO2 fixation pathway present in these organisms. Besides, in LBB01 and LBB02, the Tricarboxylic acid cycle (TCA) appears to be incomplete as genes encoding several essential enzymes, such as succinate dehydrogenase (EC 1.3.5.1) and succinyl-CoA synthetase (EC 6.2.1.5), are not found in their genomes.
Heterotrophic utilization of glucose is possible by glycolysis (Embden–Meyerhof pathway). LBB02 contains the full set of glycolytic enzymes, whereas LBB01 lacks 6-phosphofructokinase (EC 2.7.1.11) but can alternatively utilize diphosphate-fructose-6-phosphate-1-phosphotransferase (EC 2.7.1.90) for conversion of fructose-6-phosphate to fructose-1,6-diphosphate (Alves et al., 1997).
In contrast to Ca. Magnetobacterium casensis, LBB01 and LBB02 appear to be unable for complete denitrification. In both organisms, the nitrate reduction to nitrite by NarGHI and nitrous oxide to nitrogen by NosZ are possible. Nitrogen fixation in LBB01 and LBB02 should not occur.
The complete set of genes associated with dissimilatory sulfur oxidation, dsrABCHEF, aprAB, and sat, was found in the genome of LBB01. Although their operation in the reverse (reductive) direction cannot be excluded entirely without the experimental evidence, the presence of dsrEFH is typically associated with dissimilatory sulfur oxidation. Alternatively, the pathway may be exploited to obtain energy through the disproportionation of elemental sulfur or thiosulfate, as has been recently shown for a related Nitrospirota bacterium (Umezawa et al., 2020).
Since the genome of LBB04 is highly fragmented, the reliable reconstruction of its metabolic abilities is difficult. Nonetheless, it seems to include most genes of the essential pathways (biosynthesis of amino acids, purines, pyrimidines, and several important cofactors), as well as many enzymes for TCA and glycolysis. However, LBB04 is extremely poor in genes associated with sulfur and nitrogen cycling. It may utilize acetate or produce due to acetyl-CoA synthetase [EC:6.2.1.1] and pyruvate ferredoxin oxidoreductase [EC:1.2.7.1]. LBB04 might be able to degrade benzoate and other aromatic compounds, as it has enzymes containing benzoyl-CoA reductase domain (BcrC/BadD/HgdB). This trait is common to the cultivated Syntrophia, which degrade benzoate in association with hydrogen-consuming microorganisms (McInerney et al., 2007).
Magnetosome gene cluster reconstruction
Assuming the current view that MGC had vertical inheritance accompanied by many losses is true, there would be a probability of finding individual MGC genes in non-MTB genomes. Therefore, the search for MGC genes was initially carried out in all genomes of the Nitrospirota phylum, presented in the GTDB r95 database (Supplementary Table S7), using local BLASTp and MGC protein sequences of Ca. Magnetomonas plexicatena LBB01 as a reference. As a result, no MGC genes have been found in non-MTB genomes.
Next, the magnetosomes synthesis genes were detected in the reconstructed MTB genomes (Figure 3). Considering that a circular chromosome has been assembled for Ca. Magnetomonas plexicatena LBB01, its MGC was taken as a reference for comparative analysis of magnetosome gene clusters containing man genes. The MGC of Ca. Magnetomonas plexicatena LBB01 consisted of three regions located on the three separate chromosome loci. The main MGC region (22 Kb) include mad26, mad25, mad24, mad23, mamO-Cter, man6, man5, man4, mamQ, mamE, mamI, mamA, mad2, mamB, mamQ-2, mad31, mamM, mamP, man3, mad10, man2, mamK, man1 genes. A second region (2.7 Kb), located 276.8 Kb away from the main region, contains the feoA and feoB genes. These genes are homologs of the mad30 and mad17 genes, respectively, and are involved in iron transport for magnetosome synthesis. The third region (2.6 Kb) is located at a distance of 1.7 Mb from the second region and is composed of genes mad28, mad29, and a gene encoding a hypothetical protein.
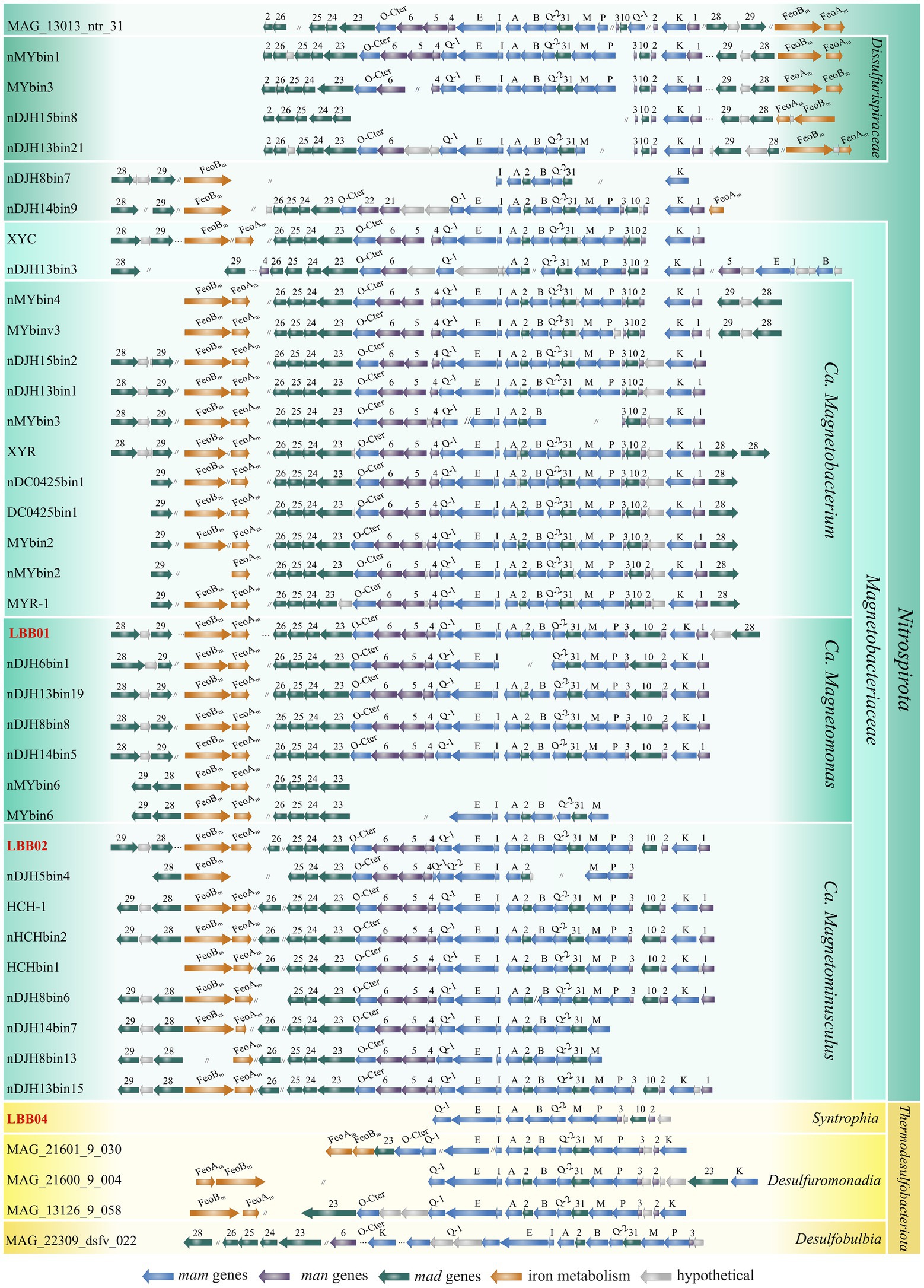
Figure 3. Comparison of the MGC regions in MTB genomes affiliated with the Nitrospirota and Thermodesulfobacteriota phyla. Genomes obtained in this work are highlighted in red. Full names for strains can be found in Supplementary Table S1.
The MGC of Ca. Magnetominusculus linsii LBB02 also includes three regions with a similar gene content to MGC of Ca. Magnetomonas plexicatena LBB01, except for the mad26 gene that was in another contig. Moreover, the gene content and synteny of the mad26-man1 region in the assembled genomes are similar in all known magnetosome gene clusters from Nitrospirota phylum, confirming that the gene synteny is highly conserved in MGCs across Nitrospirota MTB (Zhang et al., 2021). It is also notable that two mad28 genes were observed in the MGC of Ca. Magnetomonas plexicatena LBB01. Previously the duplication of this gene was detected only in Ca. Magnetobacterium cryptolimnobacter XYR (Zhang et al., 2021). Besides, there is a difference in MGC composition between representatives of Ca. Magnetobacteriaceae and Dissulfurispiraceae families. As indicated before, the region containing the feoAB genes is far from the main MGC region of LBB01 that belonged to Ca. Magnetobacteriaceae. The MGCs of the Dissulfurispiraceae look highly compact because the feoAB region is located in up to four genes from the main region. The MGC of Ca. Belliniella magnetica LBB04 contained only some of the genes present in Ca. Magnetomonas plexicatena LBB01. In the MGC of LBB04, two features attract attention. First, the man2 and man3 genes, previously thought to be specific for Nitrospirota, were detected. Second, all mad genes, except for mad10, were absent from loci where they were present in LBB01. The absence of these genes probably can be explained by the low completeness of the studied genome. However, if these genes are presented in the genome, they should occupy other loci than those in LBB01. Surprisingly, man-containing MGCs of other Thermodesulfobacteriota contained mad2 and mad31 genes at the same positions as in LBB01, although the same genes in the same order, like in LBB04, were presented. The part of MGCs (from mamQ-1 to man2) of other Thermodesulfobacteriota was similar to the part of MGCs of LBB01 and other Nitrospirota.
Reconstruction of the evolutionary paths for MGC of man-containing MTB
As the first step in further studying the evolution of MTB containing man genes, a phylogenomic tree of MTB and non-MTB genomes from the Nitrospirota and Thermodesulfobacteriota phyla was built (Figure 4). According to this tree, all man-containing MTB were divided into three groups: Dissulfurispiraceae group, Magnetobacteriaceae group, and man-containing Thermodesulfobacteriota group. The group of man-containing Thermodesulfobacteriota contained MTB representatives from different classes of the Thermodesulfobacteriota phylum. In the Nitrospirota, man-containing MTB were divided into two groups, Dissulfurispiraceae and Magnetobacteriaceae, because they were separated from each other by non-MTB groups.
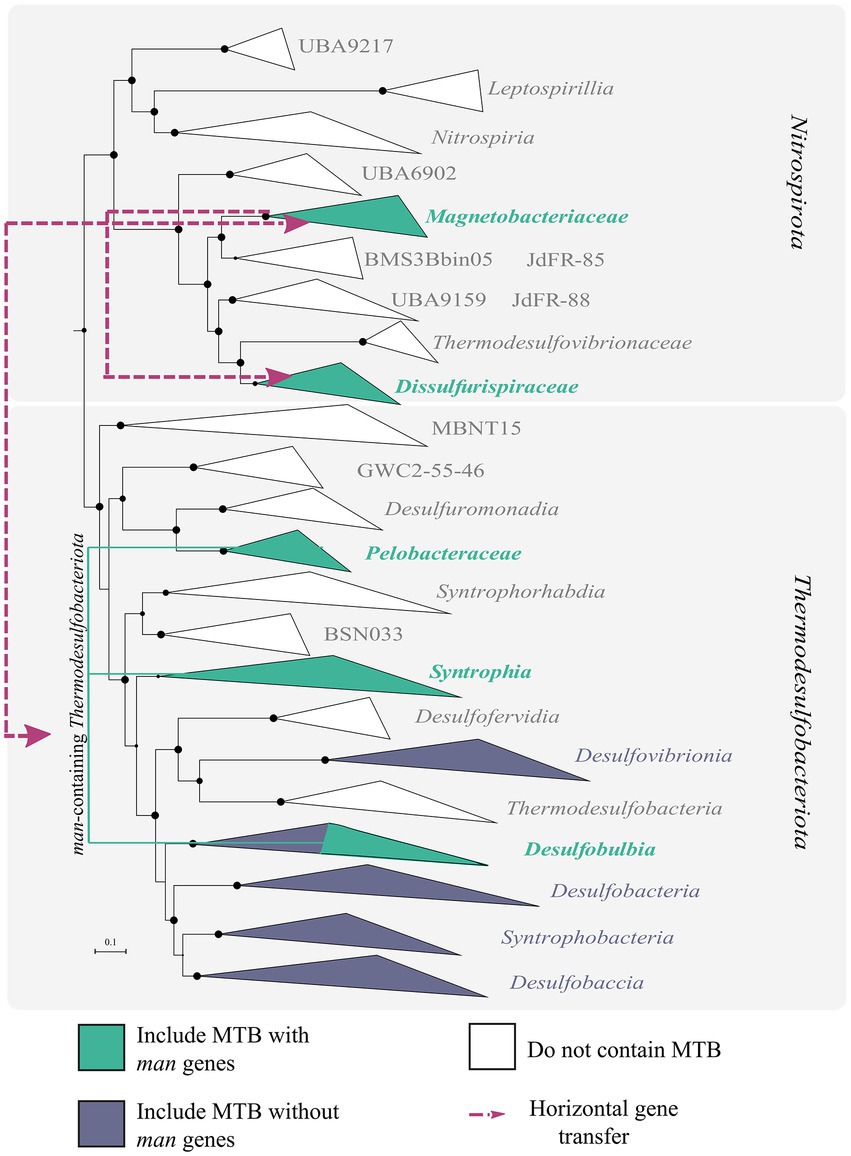
Figure 4. Results of reconciliation for man-containing genomes from the Nitrospirota and Thermodesulfobacteriota phyla. A maximum-likelihood phylogenomic tree was built from concatenated 120 bacterial single-copy marker proteins using evolutionary model LG + F + I + G4. Branch supports were obtained with 1,000 ultrafast bootstraps. The scale bar represents amino acid substitutions per site. Branches colored in green indicate groups that include MTB representatives. Violet-colored branches include MTB representatives without man genes in MGCs. White branches do not have MTB members. Purple lines indicate the direction of horizontal gene transfer (HGT) of MGC.
The first group, the Dissulfurispiraceae group, contained two families from the Nitrospirota phylum: the Dissulfurispiraceae (Umezawa et al., 2021; previously named UBA9935) and the family called DUZI01 in the GTDB r202 database. According to GTDB, the Dissulfurispiraceae family contained 10 representative genomes, four of which (nMYbin1, MYbin3, nDJH15bin8, and nDJH13bin21) were affiliated to MTB (Supplementary Table S1). DUZI01 family had only one genome, Nitrospirae bacterium MAG_10313_ntr_31, which belonged to MTB. The Dissulfurispiraceae group on the species tree clustered separately from the second man-containing MTB group within the Nitrospirota, the Magnetobacteriaceae group. This group contained 29 MTB genomes from the Ca. Magnetobacteriaceae family and two genomes (nDJH14bin9 and nDJH8bin7) from a new, yet unnamed in GTDB r202 database, family. Non-MTB representatives were not found in this group. Considering how distant Dissulfurispiraceae is from Magnetobacteriaceae on the species tree, it is crucial to understand how MGCs were inherited in the MTB of these groups. To answer this question, we conducted a reconciliation analysis. Reconciliation is a method of annotating protein trees with evolutionary events along with mapping them onto a species tree (Duchemin et al., 2018). Two programs, Notung v.2.9 (Stolzer et al., 2012) and Ranger-DTL v2.0 (Bansal et al., 2018), were used for reconciliation (see materials and methods for details). As a result, in 90.9% of cases (20 of 22 MGC protein tree reconciliations) according to Notung and in 95.5% (21 of 22 trees) according to Ranger-DTL, MGC genes of the Dissulfurispiraceae group were acquired horizontally from the Magnetobacteriaceae group [Supplementary Figure S2; Supplementary Table S2, figshare data “Reconciliation_results” (Uzun et al., 2022)]. The reconciliation results of the concatenated protein tree also confirmed the horizontal inheritance of MGC in Dissulfurispiraceae group from the Magnetobacteriaceae group. MGC from the Magnetobacteriaceae group was most likely transferred to the last common ancestor of the Dissulfurispiraceae group. This MGC was then inherited vertically by future lineages and eventually lost in the non-MTB lineages of the Dissulfurispiraceae group. Alternatively, in a less likely scenario, the MGC from the Magnetobacteriaceae group was transferred specifically to the family Dissulfurispiraceae, and from there, by HGT, to the family DUZI01. Thus, based on the results obtained, the MGC genes in the Dissulfurispiraceae group were acquired horizontally from the Magnetobacteriaceae group.
The reconciliation analysis also revealed that MGCs in the Magnetobacteriaceae group were obtained by HGT in 54.5% of cases (12 of 22 trees) according to Ranger-DTL and 59% (13 of 22 trees) according to Notung. Of all HGT cases, the most common were transfers from the Thermodesulfobacteriota phylum, which accounted for 46.2% (6 of 13 trees) according to Ranger-DTL and 69.2% (9 of 13 trees) according to Notung. Transfers from other phyla (Bdellovibrionota, Riflebacteria, Planctomycetota, Omnitrophota) were much less likely to occur. The vertical inheritance of genes from the last common man-containing magnetotactic ancestor was observed in 45.5% of cases (in 10 out of 22 trees) according to Ranger-DTL and 41% (9 of 22 trees) according to Notung. However, a vertical inheritance pattern was observed in protein trees containing representatives solely from Nitrospirota (Man1, -2, -4, -5 trees) or Nitrospirota and Thermodesulfobacteriota (Mad2, -10, -24, -25, -31 trees) phyla. Since these proteins can only be found in a limited number of taxa, their vertical inheritance results may be explained by the fact that the programs cannot analyze these evolutionary paths in sufficient detail. In the meantime, a reconciliation of the concatenated protein tree and individual Mam protein trees suggests that MTB from the Magnetobacteriaceae group likely acquired their MGCs by HGT.
The third group, the Thermodesulfobacteriota group, includes five MTB genomes affiliated to classes Syntrophia (LBB04), Desulfobulbia (MAG_13126_9_058, MAG_21600_9_004, MAG_21601_9_030), and Desulfuromonadia (MAG_22309_dsfv_022) from the Thermodesulfobacteriota phylum. It is noteworthy that MTB are rare in these classes. Interestingly, on the concatenated magnetosome protein trees, MTB from this group clustered with MTB affiliated with the Nitrospirota phylum [figshare data “Mam_protein_trees” (Uzun et al., 2022)], but not with Thermodesulfobacteriota. Analysis of the reconciliation data revealed that MGCs of the man-containing Thermodesulfobacteriota group were acquired horizontally with 100.0% probability (19 of 19 trees) according to Ranger-DTL and 94.7% (18 of 19 trees) according to Notung. Moreover, MGCs were transferred to this group from the Magnetobacteriaceae group with a 73.6% probability (14 of 19 trees) according to Ranger-DTL and 52.6% (10 of 19 trees) according to Notung. The likelihood of vertical inheritance was extremely low: 0% according to Ranger-DTL and 5.3% (1 tree of 19) - to Notung. The reconciliation data of the concatenated protein tree also confirmed that the MGC in the man-containing Thermodesulfobacteriota group was obtained by HGT. According to Notung, this transfer originated from Bdellovibrionota, while Ranger-DTL showed inheritance from the Magnetobacteriaceae group. At the same time, within the man-containing Thermodesulfobacteriota group, it is not entirely clear how MGCs were inherited. It could be a horizontal transfer to the ancestor of all Thermodesulfobacteriota followed by further vertical inheritance and multiple losses of MGC in most genomes of the entire phyla. Alternatively, it could be a horizontal transfer to one specific bacterium after delineation of the major classes, followed by further transfers to other species. Since MGC horizontal gene transfer has been independently detected from the Magnetobacteriaceae group to the Thermodesulfobacteriota group and vice versa, this reinforces the HGT of MGC between the two groups (Figure 4). This is, to our knowledge, the first detected case of inter-phylum HGT of MGC. However, more data is required for clarification, which of the groups served as a donor and which as a recipient.
Discussion
Our knowledge about magnetotactic Nitrospirota increased significantly in the last years since Ca. Magnetobacterium bavaricum, the first Nitrospirota MTB, was discovered (Spring et al., 1993). Nitrospirota representatives have been found worldwide in freshwater and marine ecosystems (Lin et al., 2012a,b; Xu et al., 2018; Qian et al., 2019). Unfortunately, there is no available pure culture of Nitrospirota MTB, making it difficult to accurately characterize their physiology, ecology, and magnetosome formation process. All known magnetotactic Nitrospirota were studied using culture-independent techniques (Spring et al., 1993; Lefèvre et al., 2010; Lefevre et al., 2011; Lin et al., 2011, 2012a, 2014; Xu et al., 2018; Qian et al., 2019), and the morphology of many Nitrospirota MTB is known through the FISH-TEM approaches (Lefevre et al., 2011; Lin et al., 2012a; Qian et al., 2019; Zhang et al., 2021). In this study, two novel Nitrospirota genomes were reconstructed, which expanded MTB’s genomic diversity. For one of them, the genome of LBB01, the whole circular chromosome was successfully obtained, the first complete genome of magnetotactic Nitrospirota. Using the LBB01 genome as a reference allowed further comparative analyses of magnetosome genes and metabolic features. Comparative analysis showed common traits for Nitrospirota MTB. MGC of all genomes, with some exceptions, consisted of three separate clusters that were highly conservative, which was also noted by Zhang et al. (2021). No principal differences were found in metabolic features between the magnetotactic Nitrospirota genomes. Consistent with previous studies, all of the magnetotactic representatives appear to have the ability for autotrophy using Wood–Ljungdahl and heterotrophy by glycolysis via Embden–Meyerhof pathways (Lin et al., 2014; Zhang et al., 2021). In addition, all Nitrospirota MTB are potentially capable of dissimilatory sulfur oxidation. At the same time, MTB that belong to Ca. Magnetobacteriaceae showed high diversity in cell morphology and magnetosome chain organization. So, MTB of the genus Ca. Magnetobacterium represent rod-shaped bacteria of various sizes that contain up to thousand magnetosomes per cell (Spring et al., 1993; Lin et al., 2014), Ca. Magnetominusculus are small ovoid-shaped bacteria with several bundles of magnetosome chains, Ca. Magnetomonas are vibrioid-shaped bacteria with a single bundle of chains, Ca. Magnetoovum and Ca. Cryptolimnococcus are large ovoid-shaped MTB forming multiple bundles of chains (Lefevre et al., 2011; Kolinko et al., 2015). Accordingly, the results of phylogenetic inference supported by differences in genomic features and morphology, LBB01 and LBB02 have been defined and described as a novel genus and two novel species within Ca. Magnetobacteriaceae family, respectively.
Compared to Nitrospirota, MTB from Thermodesulfobacteriota phylum represented a minor fraction of the Lake Beloe Bordukovskoe MTB community. Despite this, in our previous work (Koziaeva et al., 2020), we managed to take pictures of LBB04 from the Thermodesulfobacteriota phylum using the FISH-TEM approach. It turned out that LBB04 had the same bullet-shaped magnetosomes as LBB01 and LBB02 from the Nitrospirota phylum. In this work, we obtained a genome of strain LBB04 affiliated with the order Syntrophales and detected man genes in its MGC. Previously, man genes have already been detected in the MTB of Thermodesulfobacteriota phylum (Uzun et al., 2020). However, these genes were initially considered to be found exclusively in the Nitrospirota phylum (Lin et al., 2014). The obtained new data allowed us to investigate several issues about the origin and evolution of man-containing MGCs.
As mentioned earlier, two theories have been proposed regarding the appearance of MGC in the MTB from the Nitrospirota phylum (Lin et al., 2017b). In the first and most frequently cited hypothesis, MGC in Nitrospirota was acquired vertically from the last common ancestor of Nitrospirota and Pseudomonadota. According to the second, less speculated view, MGC was horizontally transferred undetectably early between these two phyla soon after their delineation. These suggestions were proposed a couple of years ago when mentioned phyla clustered together on the magnetotactic phylogenetic tree. Since then, many new data on MTB diversity have emerged, providing an opportunity to explore these two suggestions in more detail.
Assuming the first suggestion about the vertical MGC inheritance is true, there should have been MGC losses within the Nitrospirota phylum since this phylum has many non-MTB representatives. Wang and Chen (2017) have already made this assumption during the analysis of the 16S rRNA sequences tree of the Nitrospirota and Pseudomonadota representatives known at that time. However, they concluded that the number of losses must have been enormously high, which had never been documented before. Using the new genomic data, we tested this hypothesis by building the species tree [Figure 2, figshare data (Uzun et al., 2022)]. On the resulting tree, MTB represented the minor part of Nitrospirota members, and a huge number of losses indeed should have occurred in case of the common inheritance of magnetotactic trait within this phylum. Thus, our result confirms the conclusions of Wang and Chen, also suggesting that such a scenario is unlikely to occur. Apart from that, currently, Nitrospirota and Pseudomonadota phyla are located distantly on the species tree, with many phyla that include non-MTB representatives positioned between them. In the case of a common inheritance of their MGCs, the number of losses that should have occurred increases many times, which is even more unlikely. Also, we suggest that if MGC losses within the whole Nitrospirota phylum did occur, it would be possible to detect the parts of the MGCs that remained after the losses. However, the search for MGC residues in non-MTB genomes of the Nitrospirota phylum did not reveal even a single gene for magnetosome synthesis. This may indicate that either the MGCs are always entirely removed in case of losses, or the genes were never present in these genomes. Considering all these results, we conclude that with a high probability, the last common ancestor of all Nitrospirota was not magnetotactic.
Next, we considered the second hypothesis, which assumes the horizontal inheritance of the man-containing MGC. For this, reconciliation tools were used, and statistical calculations of obtained results were carried out. Eventually, several new HGT events were detected. First, the reconciliation analysis shows that the Magnetobacteriaceae group donated MGC to the Dissulfurispiraceae group by HGT with high probability. Second, it has been found that in the Magnetobacteriaceae group, MGCs with a high probability were also obtained by HGT from other phyla, which, however, cannot be yet identified. The fact that man-containing MGCs in this group are highly conserved also provides further evidence for the recent transfer of magnetosome synthesis genes. Current results suggest that, with different probabilities, the donors for MGC could be MTB from Thermodesulfobacteriota, Bdellovibrionota, Riflebacteria, Planctomycetota, Omnitrophota phyla. However, none of the reconciliation results showed the probability of MGC inheritance in the Magnetobacteriaceae group from the MTB of the Pseudomonadota phylum, as suggested earlier. Within the Magnetobacteriaceae group, reconciliation results revealed vertical inheritance of MGCs. This is the first reported case of HGT of magnetosome synthesis genes among MTB families within the phylum Nitrospirota. Also, to the best of our knowledge, the first case of interphylum horizontal genes transfer of MGC was reported in this work. These results significantly refine the second, previously much less studied, theory of MGC inheritance in the Nitrospirota phylum.
Given the presence of man genes in the Thermodesulfobacteriota phylum, the next question was how the members of this group inherited man-containing MGCs. According to the reconciliation results, MGCs in the Thermodesulfobacteriota phylum were most likely obtained by HGT from the Magnetobacteriaceae group of the phylum Nitrospirota. Thus, our study reveals that magnetosome synthesis genes were horizontally transferred between MTB belonging to different phyla. The fact that these MTB from different phyla were detected in the same habitat, Lake Beloe Bordukovskoe, increases the probability that HGT could occur. The detection of the interphylum HGT calls into question the anciency of the origin of magnetosome synthesis genes, as the MGCs could have been transferred horizontally into these deep-branching phyla in later evolutionary periods. Although the timepoint when magnetosome synthesis emerged cannot be determined based on the currently available data, our findings suggest that it could occur after the delineation of Nitrospirota and Pseudomonadota, as at least one of these phyla acquired the MGC horizontally. This implies that the origin might be dated to a later time point than previously suggested mid-Archaeon (Lin et al., 2017b). Based on the obtained results, it can be assumed that the horizontal transfer of MGCs plays a more significant role in MTB evolution than thought previously. The possibility of interphylum transfers should be considered in further analyses of MTB evolution. Future works should focus on gathering further MTB genome sequences and more thorough reconstructions of the evolutionary history of MGC in different phylogenetic groups.
Taxonomic consideration
Candidatus Magnetomonas
Magnetomonas (Ma.gne.to.mo’nas. Gr. n. magnes, − etos a magnet; N.L. pref. magneto- pertaining to a magnet; N.L. fem. n.monas unit, monad; N.L. fem. n. Magnetomonas a magnetic monad).
Candidatus Magnetomonas plexicatena
Magnetomonas plexicatena (ple.xi.ca.te’na.L. past part.plexus interwoven; L. fem. n.catena chain; N.L. fem. n. plexicatena an interwoven chain).
Vibrioid-shaped, cell size 2.0 ± 0.4 μm long and 0.5 ± 0.1 μm wide, form magnetite magnetosomes organized in chains along the long axis of the bacterial cell body. Magnetosomes present a mean length of 108 ± 21.1 nm and a mean width of 45 ± 8.1 nm. Potentially capable of chemolithoautotrophy with the oxidation of sulfur compounds and carbon assimilation by Wood–Ljungdahl pathway. Potentially capable of heterotrophy by glycolysis. Not capable of nitrogen fixation. The reference strain is LBB01. The genome reference sequence of LBB01 is CP049016. G + C content 42.0%.
Candidatus Magnetominusculus linsii
Magnetominusculus linsii (lin’si.i. N.L. gen. masc. n. linsii, of Lins, named after Ulysses Lins, a Brazilian microbiologist, who made a significant contribution to the study of magnetotactic bacteria).
Small ovoid cells 1.5 μm long and 1.2 μm wide, form two bundles of bullet-shaped magnetite magnetosomes. Potentially capable of chemolithoautotrophy with the oxidation of sulfur compounds and carbon assimilation by Wood–Ljungdahl pathway. Potentially capable of heterotrophy by glycolysis. Not capable of nitrogen fixation. The reference strain is LBB02. The genome reference sequence of LBB02 is JAKOEO000000000. G + C content 47.0%.
Candidatus Belliniella
Belliniella (Bel.li.ni.el’la. N.L. fem. n. Belliniella, named in honor of Salvatore Bellini, an Italian microbiologist, who was one of the discoverers of magnetotactic bacteria).
Candidatus Belliniella magnetica
Belliniella magnetica (mag.ne’ti.ca. L. fem. adj. magnetica, of magnetic, referring to intracellular magnetite particles).
Rod-shaped cells ~2.5 μm long and 1.1 μm wide, form elongated magnetosomes not organized in chains. May utilize acetate. Potentially capable for glycolysis and degrading of benzoate and other aromatic compounds. The genome reference sequence of LBB04 is JAKOEP000000000. G + C content 50.4%.
Data availability statement
The datasets presented in this study can be found in online repositories. The names of the repository/repositories and accession number(s) can be found in the article/Supplementary material.
Author contributions
MU, VK, and DG conceived and designed experiments. MK performed bioinformatics data processing. MU, MD, and DG carried out the phylogenetic and comparative genomic analyses. PL performed FISH-TEM analysis. VK, MU, MD, and DG analyzed the data and drafted the original manuscript. All authors contributed to the article and approved the submitted version.
Funding
The reported study was partially funded by RFBR (project number 20-34-90116) and the Ministry of Science and Higher Education of the Russian Federation.
Acknowledgments
We thank Aharon Oren for his expert guidance in nomenclature. Bioinformatic analyses were performed using computing resources at the Core Research Facility “Bioengineering” (Research Center of Biotechnology RAS) and SciBear OU (https://sci-bear.com/).
Conflict of interest
The authors declare that the research was conducted in the absence of any commercial or financial relationships that could be construed as a potential conflict of interest.
Publisher’s note
All claims expressed in this article are solely those of the authors and do not necessarily represent those of their affiliated organizations, or those of the publisher, the editors and the reviewers. Any product that may be evaluated in this article, or claim that may be made by its manufacturer, is not guaranteed or endorsed by the publisher.
Supplementary material
The Supplementary Materials for this article can be found online at: https://www.frontiersin.org/articles/10.3389/fmicb.2022.945734/full#supplementary-material
Footnotes
References
Abreu, F., Cantão, M. E., Nicolás, M. F., Barcellos, F. G., Morillo, V., Almeida, L. G., et al. (2011). Common ancestry of iron oxide- and iron-sulfide-based biomineralization in magnetotactic bacteria. ISME J. 5, 1634–1640. doi: 10.1038/ismej.2011.35
Alves, A. M. C. R., Euverink, G. J. W., Bibb, M. J., and Dijkhuizen, L. (1997). Identification of ATP-dependent phosphofructokinase as a regulatory step in the glycolytic pathway of the actinomycete Streptomyces coelicolor A3(2). Appl. Environ. Microbiol. 63, 956–961. doi: 10.1128/aem.63.3.956-961.1997
Auch, A. F., von Jan, M., Klenk, H. P., and Göker, M. (2010). Digital DNA-DNA hybridization for microbial species delineation by means of genome-to-genome sequence comparison. Stand. Genomic Sci. 2, 117–134. doi: 10.4056/sigs.531120
Aziz, R. K., Bartels, D., Best, A. A., Dejongh, M., Disz, T., Edwards, R. A., et al. (2008). The RAST server: rapid annotations using subsystems technology. BMC Genomics 9, 1–15. doi: 10.1186/1471-2164-9-75
Bankevich, A., Nurk, S., Antipov, D., Gurevich, A. A., Dvorkin, M., Kulikov, A. S., et al. (2012). SPAdes: a new genome assembly algorithm and its applications to single-cell sequencing. J. Comput. Biol. 19, 455–477. doi: 10.1089/cmb.2012.0021
Bansal, M. S., Kellis, M., Kordi, M., and Kundu, S. (2018). RANGER-DTL 2.0: rigorous reconstruction of gene-family evolution by duplication, transfer and loss. Bioinformatics 34, 3214–3216. doi: 10.1093/bioinformatics/bty314
Bolger, A. M., Lohse, M., and Usadel, B. (2014). Trimmomatic: a flexible trimmer for Illumina sequence data. Bioinformatics 30, 2114–2120. doi: 10.1093/bioinformatics/btu170
Chaumeil, P., Mussig, A. J., Parks, D. H., and Hugenholtz, P. (2019). GTDB-Tk: a toolkit to classify genomes with the genome taxonomy database. Bioinformatics 36, 1925–1927. doi: 10.1093/bioinformatics/btz848
Coleman, G. A., Davín, A. A., Mahendrarajah, T. A., Szánthó, L. L., Spang, A., Hugenholtz, P., et al. (2021). A rooted phylogeny resolves early bacterial evolution. Science 372:eabe0511. doi: 10.1126/science.abe0511
DeLong, E. F., Frankel, R. B., and Bazylinski, D. A. (1993). Multiple evolutionary origins of Magnetotaxis in Bacteria. Science 259, 803–806. doi: 10.1126/science.259.5096.803
Duchemin, W., Gence, G., Chifolleau, A. M. A., Arvestad, L., Bansal, M. S., Berry, V., et al. (2018). RecPhyloXML: a format for reconciled gene trees. Bioinformatics 34, 3646–3652. doi: 10.1093/bioinformatics/bty389
Frankel, R. B., Bazylinski, D. A., Johnson, M. S., and Taylor, B. L. (1997). Magneto-aerotaxis in marine coccoid bacteria. Biophys. J. 73, 994–1000. doi: 10.1016/S0006-3495(97)78132-3
Gareev, K. G., Grouzdev, D. S., Kharitonskii, P. V., Kosterov, A., Koziaeva, V. V., Sergienko, E. S., et al. (2021). Magnetotactic bacteria and magnetosomes: basic properties and applications. Magnetochemistry 7:86. doi: 10.3390/magnetochemistry7060086
Goris, J., Konstantinidis, K. T., Klappenbach, J. A., Coenye, T., Vandamme, P., and Tiedje, J. M. (2007). DNA-DNA hybridization values and their relationship to whole-genome sequence similarities. Int. J. Syst. Evol. Microbiol. 57, 81–91. doi: 10.1099/ijs.0.64483-0
Grouzdev, D. S., Rysina, M. S., Bryantseva, I. A., Gorlenko, V. M., and Gaisin, V. A. (2018). Draft genome sequences of “Candidatus Chloroploca asiatica” and “Candidatus Viridilinea mediisalina”, candidate representatives of the Chloroflexales order: phylogenetic and taxonomic implications. Stand. Genomic Sci. 13:24. doi: 10.1186/s40793-018-0329-8
Gurevich, A., Saveliev, V., Vyahhi, N., and Tesler, G. (2013). QUAST: quality assessment tool for genome assemblies. Bioinformatics 29, 1072–1075. doi: 10.1093/bioinformatics/btt086
Hoang, D. T., Chernomor, O., Von Haeseler, A., Minh, B. Q., and Vinh, L. S. (2017). UFBoot2: improving the ultrafast bootstrap approximation. Mol. Biol. Evol. 35, 518–522. doi: 10.1093/molbev/msx281
Jain, C., Rodriguez-R, L. M., Phillippy, A. M., Konstantinidis, K. T., and Aluru, S. (2018). High throughput ANI analysis of 90K prokaryotic genomes reveals clear species boundaries. Nat. Commun. 9:5114. doi: 10.1038/s41467-018-07641-9
Kanehisa, M., and Sato, Y. (2020). KEGG mapper for inferring cellular functions from protein sequences. Protein Sci. 29, 28–35. doi: 10.1002/pro.3711
Kang, D. D., Li, F., Kirton, E., Thomas, A., Egan, R., An, H., et al. (2019). MetaBAT 2: an adaptive binning algorithm for robust and efficient genome reconstruction from metagenome assemblies. PeerJ 7, 1–13. doi: 10.7717/peerj.7359
Katoh, K., and Standley, D. M. (2013). MAFFT multiple sequence alignment software version 7: improvements in performance and usability. Mol. Biol. Evol. 30, 772–780. doi: 10.1093/molbev/mst010
Kolinko, S., Richter, M., Glöckner, F.-O., Brachmann, A., and Schüler, D. (2015). Single-cell genomics of uncultivated deep-branching magnetotactic bacteria reveals a conserved set of magnetosome genes. Environ. Microbiol. 18, 21–37. doi: 10.1111/1462-2920.12907
Konstantinidis, K. T., Rosselló-Móra, R., and Amann, R. (2017). Uncultivated microbes in need of their own taxonomy. ISME J. 11, 2399–2406. doi: 10.1038/ismej.2017.113
Koziaeva, V. V., Alekseeva, L. M., Uzun, M. M., Leão, P., Sukhacheva, M. V., Patutina, E. O., et al. (2020). Biodiversity of magnetotactic bacteria in the freshwater Lake Beloe Bordukovskoe, Russia. Microbiology 89, 348–358. doi: 10.1134/s002626172003008x
Koziaeva, V., Dziuba, M., Leão, P., Uzun, M., Krutkina, M., and Grouzdev, D. (2019). Genome-based metabolic reconstruction of a novel uncultivated freshwater magnetotactic coccus “Ca. magnetaquicoccus inordinatus” UR-1, and proposal of a candidate family “Ca. Magnetaquicoccaceae.” Front. Microbiol. 10, 1–20. doi: 10.3389/fmicb.2019.02290
Laczny, C. C., Kiefer, C., Galata, V., Fehlmann, T., Backes, C., and Keller, A. (2017). BusyBee web: metagenomic data analysis by bootstrapped supervised binning and annotation. Nucleic Acids Res. 45, W171–W179. doi: 10.1093/nar/gkx348
Langmead, B., and Salzberg, S. L. (2012). Fast gapped-read alignment with bowtie 2. Nat. Methods 9, 357–359. doi: 10.1038/nmeth.1923
Lefèvre, C. T., Abreu, F., Schmidt, M. L., Lins, U., Frankel, R. B., Hedlund, B. P., et al. (2010). Moderately thermophilic magnetotactic bacteria from hot springs in Nevada. Appl. Environ. Microbiol. 76, 3740–3743. doi: 10.1128/AEM.03018-09
Lefèvre, C. T., and Bazylinski, D. A. (2013). Ecology, diversity, and evolution of magnetotactic bacteria. Microbiol. Mol. Biol. Rev. 77, 497–526. doi: 10.1128/MMBR.00021-13
Lefevre, C. T., Frankel, R. B., Abreu, F., Lins, U., and Bazylinski, D. A. (2011). Culture-independent characterization of a novel, uncultivated magnetotactic member of the Nitrospirae phylum. Environ. Microbiol. 13, 538–549. doi: 10.1111/j.1462-2920.2010.02361.x
Lefèvre, C. T., Trubitsyn, D., Abreu, F., Kolinko, S., de Almeida, L. G. P., de Vasconcelos, A. T. R., et al. (2013a). Monophyletic origin of magnetotaxis and the first magnetosomes. Environ. Microbiol. 15, 2267–2274. doi: 10.1111/1462-2920.12097
Lefèvre, C. T., Trubitsyn, D., Abreu, F., Kolinko, S., Jogler, C., de Almeida, L. G. P., et al. (2013b). Comparative genomic analysis of magnetotactic bacteria from the Deltaproteobacteria provides new insights into magnetite and greigite magnetosome genes required for magnetotaxis. Environ. Microbiol. 15, 2712–2735. doi: 10.1111/1462-2920.12128
Letunic, I., and Bork, P. (2019). Interactive tree of life (iTOL) v4: recent updates and new developments. Nucleic Acids Res. 47, W256–W259. doi: 10.1093/nar/gkz239
Li, H. (2018). Minimap2: pairwise alignment for nucleotide sequences. Bioinformatics 34, 3094–3100. doi: 10.1093/bioinformatics/bty191
Li, J., Menguy, N., Gatel, C., Boureau, V., Snoeck, E., Patriarche, G., et al. (2015). Crystal growth of bullet-shaped magnetite in magnetotactic bacteria of the Nitrospirae phylum. J. R. Soc. Interface 12:20141288. doi: 10.1098/rsif.2014.1288
Li, J., Pan, Y., Liu, Q., Yu-Zhang, K., Menguy, N., Che, R., et al. (2010). Biomineralization, crystallography and magnetic properties of bullet-shaped magnetite magnetosomes in giant rod magnetotactic bacteria. Earth Planet. Sci. Lett. 293, 368–376. doi: 10.1016/j.epsl.2010.03.007
Li, J., Zhang, H., Menguy, N., Benzerara, K., Wang, F., Lin, X., et al. (2017). Single-cell resolution study of uncultured magnetotactic bacteria via fluorescence-coupled electron microscopy. Appl. Environ. Microbiol. 83, e00409–e00417. doi: 10.1128/AEM.00409-17
Lin, W., Deng, A., Wang, Z., Li, Y., Wen, T., Wu, L.-F., et al. (2014). Genomic insights into the uncultured genus “Candidatus Magnetobacterium,” in the phylum Nitrospirae. ISME J. 8, 2463–2477. doi: 10.1038/ismej.2014.94
Lin, W., Jogler, C., Schüler, D., and Pan, Y. (2011). Metagenomic analysis reveals unexpected subgenomic diversity of magnetotactic bacteria within the phylum Nitrospirae. Appl. Environ. Microbiol. 77, 323–326. doi: 10.1128/AEM.01476-10
Lin, W., Kirschvink, J. L., Paterson, G. A., Bazylinski, D. A., and Pan, Y. (2020a). On the origin of microbial magnetoreception. Natl. Sci. Rev. 7, 472–479. doi: 10.1093/nsr/nwz065
Lin, W., Li, J., and Pan, Y. (2012a). Newly isolated but uncultivated Magnetotactic bacterium of the phylum Nitrospirae from Beijing, China. Appl. Environ. Microbiol. 78, 668–675. doi: 10.1128/AEM.06764-11
Lin, W., Pan, Y., and Bazylinski, D. A. (2017a). Diversity and ecology of and biomineralization by magnetotactic bacteria. Environ. Microbiol. Rep. 9, 345–356. doi: 10.1111/1758-2229.12550
Lin, W., Paterson, G. A., Zhu, Q., Wang, Y., Kopylova, E., Li, Y., et al. (2017b). Origin of microbial biomineralization and magnetotaxis during the Archean. Proc. Natl. Acad. Sci. 114, 2171–2176. doi: 10.1073/pnas.1614654114
Lin, W., Wang, Y., Li, B., and Pan, Y. (2012b). A biogeographic distribution of magnetotactic bacteria influenced by salinity. ISME J. 6, 475–479. doi: 10.1038/ismej.2011.112
Lin, W., Zhang, W., Paterson, G. A., Zhu, Q., Zhao, X., Knight, R., et al. (2020b). Expanding magnetic organelle biogenesis in the domain Bacteria. Microbiome 8, 152–113. doi: 10.1186/s40168-020-00931-9
Lin, W., Zhang, W., Zhao, X., Roberts, A. P., Paterson, G. A., Bazylinski, D. A., et al. (2018). Genomic expansion of magnetotactic bacteria reveals an early common origin of magnetotaxis with lineage-specific evolution. ISME J. 12, 1508–1519. doi: 10.1038/s41396-018-0098-9
McInerney, M. J., Rohlin, L., Mouttaki, H., Kim, U., Krupp, R. S., Rios-Hernandez, L., et al. (2007). The genome of Syntrophus aciditrophicus: life at the thermodynamic limit of microbial growth. Proc. Natl. Acad. Sci. 104, 7600–7605. doi: 10.1073/pnas.0610456104
Meier-Kolthoff, J. P., Auch, A. F., Klenk, H. P., and Göker, M. (2013). Genome sequence-based species delimitation with confidence intervals and improved distance functions. BMC Bioinformatics 14:60. doi: 10.1186/1471-2105-14-60
Monteil, C. L., Grouzdev, D. S., Perrière, G., Alonso, B., Rouy, Z., Cruveiller, S., et al. (2020). Repeated horizontal gene transfers triggered parallel evolution of magnetotaxis in two evolutionary divergent lineages of magnetotactic bacteria. ISME J. 14, 1783–1794. doi: 10.1038/s41396-020-0647-x
Monteil, C. L., Perrière, G., Menguy, N., Ginet, N., Alonso, B., Waisbord, N., et al. (2018). Genomic study of a novel magnetotactic Alphaproteobacteria uncovers the multiple ancestry of magnetotaxis. Environ. Microbiol. 20, 4415–4430. doi: 10.1111/1462-2920.14364
Monteil, C. L., Vallenet, D., Menguy, N., Benzerara, K., Barbe, V., Fouteau, S., et al. (2019). Ectosymbiotic bacteria at the origin of magnetoreception in a marine protist. Nat. Microbiol. 4, 1088–1095. doi: 10.1038/s41564-019-0432-7
Nguyen, L.-T. T., Schmidt, H. A., Von Haeseler, A., and Minh, B. Q. (2015). IQ-TREE: a fast and effective stochastic algorithm for estimating maximum-likelihood phylogenies. Mol. Biol. Evol. 32, 268–274. doi: 10.1093/molbev/msu300
Okonechnikov, K., Conesa, A., and García-Alcalde, F. (2016). Qualimap 2: advanced multi-sample quality control for high-throughput sequencing data. Bioinformatics 32, 292–294. doi: 10.1093/bioinformatics/btv566
Oren, A., and Garrity, G. M. (2021). Valid publication of the names of forty-two phyla of prokaryotes. Int. J. Syst. Evol. Microbiol. 71:004645. doi: 10.1099/ijsem.0.005056
Pantiukh, K., and Grouzdev, D. (2017). POCP-matrix Calculation for a Number of Genomes. FigShare. Code. doi: 10.6084/m9.figshare.5602957.v1
Parks, D. H., Chuvochina, M., Waite, D. W., Rinke, C., Skarshewski, A., Chaumeil, P.-A., et al. (2018). A standardized bacterial taxonomy based on genome phylogeny substantially revises the tree of life. Nat. Biotechnol. 36, 996–1004. doi: 10.1038/nbt.4229
Parks, D. H., Imelfort, M., Skennerton, C. T., Hugenholtz, P., Tyson, G. W., Centre, A., et al. (2015). CheckM: assessing the quality of microbial genomes recovered from isolates, single cells, and metagenomes. Genome Res. 25, 1043–1055. doi: 10.1101/gr.186072.114.Freely
Parks, D. H., Rinke, C., Chuvochina, M., Chaumeil, P.-A., Woodcroft, B. J., Evans, P. N., et al. (2017). Recovery of nearly 8,000 metagenome-assembled genomes substantially expands the tree of life. Nat. Microbiol. 2, 1533–1542. doi: 10.1038/s41564-017-0012-7
Qian, X. X., Liu, J., Menguy, N., Li, J., Alberto, F., Teng, Z., et al. (2019). Identification of novel species of marine magnetotactic bacteria affiliated with Nitrospirae phylum. Environ. Microbiol. Rep. 11, 330–337. doi: 10.1111/1758-2229.12755
Qin, Q. L., Xie, B. B., Zhang, X. Y., Chen, X. L., Zhou, B. C., Zhou, J., et al. (2014). A proposed genus boundary for the prokaryotes based on genomic insights. J. Bacteriol. 196, 2210–2215. doi: 10.1128/JB.01688-14
Sieber, C. M. K., Probst, A. J., Sharrar, A., Thomas, B. C., Hess, M., Tringe, S. G., et al. (2018). Recovery of genomes from metagenomes via a dereplication, aggregation and scoring strategy. Nat. Microbiol. 3, 836–843. doi: 10.1038/s41564-018-0171-1
Spring, S., Amann, R., Ludwig, W., Schleifer, K. H., Van Gemerden, H., and Petersen, N. (1993). Dominating role of an unusual magnetotactic bacterium in the microaerobic zone of a freshwater sediment. Appl. Environ. Microbiol. 59, 2397–2403. doi: 10.1128/aem.59.8.2397-2403.1993
Stolzer, M., Lai, H., Xu, M., Sathaye, D., Vernot, B., and Durand, D. (2012). Inferring duplications, losses, transfers and incomplete lineage sorting with nonbinary species trees. Bioinformatics 28, i409–i415. doi: 10.1093/bioinformatics/bts386
Tatusova, T., Dicuccio, M., Badretdin, A., Chetvernin, V., Nawrocki, P., Zaslavsky, L., et al. (2016). NCBI prokaryotic genome annotation pipeline. Nucleic Acids Res. 44, 6614–6624. doi: 10.1093/nar/gkw569
Uebe, R., and Schüler, D. (2016). Magnetosome biogenesis in magnetotactic bacteria. Nat. Rev. Microbiol. 14, 621–637. doi: 10.1038/nrmicro.2016.99
Umezawa, K., Kojima, H., Kato, Y., and Fukui, M. (2020). Disproportionation of inorganic sulfur compounds by a novel autotrophic bacterium belonging to Nitrospirota. Syst. Appl. Microbiol. 43:126110. doi: 10.1016/j.syapm.2020.126110
Umezawa, K., Kojima, H., Kato, Y., and Fukui, M. (2021). Dissulfurispira thermophila gen. Nov., sp. nov., a thermophilic chemolithoautotroph growing by sulfur disproportionation, and proposal of novel taxa in the phylum Nitrospirota to reclassify the genus Thermodesulfovibrio. Syst. Appl. Microbiol. 44:126184. doi: 10.1016/j.syapm.2021.126184
Uzun, M., Alekseeva, L., Krutkina, M., Koziaeva, V., and Grouzdev, D. (2020). Unravelling the diversity of magnetotactic bacteria through analysis of open genomic databases. Scientific Data 7, 252–213. doi: 10.1038/s41597-020-00593-0
Uzun, M., Koziaeva, V., Dziuba, M., Leão, P., Krutkina, M., and Grouzdev, D. (2022). Reconciliation results. figshare. Collection. doi: 10.6084/m9.figshare.c.5873954.v1
Waite, D. W., Chuvochina, M., Pelikan, C., Parks, D. H., Yilmaz, P., Wagner, M., et al. (2020). Proposal to reclassify the proteobacterial classes Deltaproteobacteria and Oligoflexia, and the phylum Thermodesulfobacteria into four phyla reflecting major functional capabilities. Int. J. Syst. Evol. Microbiol. 70, 5972–6016. doi: 10.1099/ijsem.0.004213
Wang, S., and Chen, Y. (2017). Origin of magnetotaxis: vertical inheritance or horizontal transfer? Proc. Natl. Acad. Sci. 114, E5016–E5018. doi: 10.1073/pnas.1706937114
Wick, R. R., Judd, L. M., Gorrie, C. L., and Holt, K. E. (2017). Unicycler: resolving bacterial genome assemblies from short and long sequencing reads. PLoS Comput. Biol. 13, e1005595–e1005522. doi: 10.1371/journal.pcbi.1005595
Wong, T. K. F., Jermiin, L. S., Minh, B. Q., Kalyaanamoorthy, S., and von Haeseler, A. (2017). ModelFinder: fast model selection for accurate phylogenetic estimates. Nat. Methods 14, 587–589. doi: 10.1038/nmeth.4285
Wu, Y., Simmons, B. A., and Singer, S. W. (2015). MaxBin 2.0: an automated binning algorithm to recover genomes from multiple metagenomic datasets. Bioinformatics 32, 605–607. doi: 10.1093/bioinformatics/btv638
Xu, C., Zhang, W., Pan, H., Du, H., and Xiao, T. (2018). Distribution and diversity of magnetotactic bacteria in sediments of the Yellow Sea continental shelf. J. Soils Sediments 18, 2634–2646. doi: 10.1007/s11368-018-1912-8
Yarza, P., Yilmaz, P., Pruesse, E., Glöckner, F. O., Ludwig, W., Schleifer, K.-H., et al. (2014). Uniting the classification of cultured and uncultured bacteria and archaea using 16S rRNA gene sequences. Nat. Rev. Microbiol. 12, 635–645. doi: 10.1038/nrmicro3330
Yoon, S.-H., Ha, S.-M., Kwon, S., Lim, J., Kim, Y., Seo, H., et al. (2017). Introducing EzBioCloud: a taxonomically united database of 16S rRNA gene sequences and whole-genome assemblies. Int. J. Syst. Evol. Microbiol. 67, 1613–1617. doi: 10.1099/ijsem.0.001755
Zhang, D., Gao, F., Jakovlić, I., Zou, H., Zhang, J., Li, W. X., et al. (2020a). PhyloSuite: an integrated and scalable desktop platform for streamlined molecular sequence data management and evolutionary phylogenetics studies. Mol. Ecol. Resour. 20, 348–355. doi: 10.1111/1755-0998.13096
Zhang, W., Ji, R., Liu, J., Pan, Y., Wu, L.-F., and Lin, W. (2020b). Two Metagenome-assembled genome sequences of Magnetotactic Bacteria in the order Magnetococcales. Microbiol. Resour. Announce. 9, 21–23. doi: 10.1128/mra.00363-20
Keywords: magnetotactic bacteria, magnetosome, Nitrospirota, horizontal gene transfer, HGT
Citation: Uzun M, Koziaeva V, Dziuba M, Leão P, Krutkina M and Grouzdev D (2022) Detection of interphylum transfers of the magnetosome gene cluster in magnetotactic bacteria. Front. Microbiol. 13:945734. doi: 10.3389/fmicb.2022.945734
Edited by:
Andrew Paul Jackson, University of Liverpool, United KingdomReviewed by:
Arturo Becerra, National Autonomous University of Mexico, MexicoLei Yan, Heilongjiang Bayi Agricultural University, China
L. F. Wu, Centre National de la Recherche Scientifique, France
Copyright © 2022 Uzun, Koziaeva, Dziuba, Leão, Krutkina and Grouzdev. This is an open-access article distributed under the terms of the Creative Commons Attribution License (CC BY). The use, distribution or reproduction in other forums is permitted, provided the original author(s) and the copyright owner(s) are credited and that the original publication in this journal is cited, in accordance with accepted academic practice. No use, distribution or reproduction is permitted which does not comply with these terms.
*Correspondence: Denis Grouzdev, denisgrouzdev@gmail.com
†These authors have contributed equally to this work and share first authorship