- 1State Key Laboratory of Grassland Agro-Ecosystems, Lanzhou University, Lanzhou, China
- 2Key Laboratory of Grassland Livestock Industry Innovation, Ministry of Agriculture and Rural Affairs, Lanzhou University, Lanzhou, China
- 3College of Pastoral Agriculture Science and Technology, Lanzhou University, Lanzhou, China
- 4Institute of Qinghai-Tibetan Plateau, Southwest Minzu University, Chengdu, China
A total of 14 Festuca sinensis seed lots were collected from different geographical locations on the Qinghai-Tibet Plateau to study the seed microbiota and determine the abiotic (temperature, precipitation, and elevation) and biotic (Epichloë sinensis infection rate) factors likely to shape the seed microbiome. The 14 seed lots had different bacterial and fungal structures and significantly different diversities (p < 0.05). The α-diversity indices of the bacteria were significantly correlated with precipitation (p < 0.05), whereas those of the fungi were significantly correlated with temperature (p < 0.05). Microbiota analysis showed that Proteobacteria, Cyanobacteria, and Bacteroidetes were the most abundant bacteria at the phylum level in the seeds, and Ascomycota and Basidiomycota were the most abundant fungi. β-diversity analysis suggested large differences in the microbial communities of each sample. Redundancy analysis showed that temperature and precipitation were the main environmental factors that drive variations in the microbial community, at the medium-high elevation (3,000–4,500 m), the impact of temperature and precipitation on microbial community is different, and the other elevations that effect on microbial community were basically identical. Spearman's correlation analysis showed that the relative abundances of the most abundant bacterial phyla were significantly correlated with temperature (p < 0.05), whereas those of the most abundant fungal phyla were significantly correlated with precipitation (p < 0.05). E. sinensis infection rates were significantly correlated with elevation and temperature (p < 0.05). These results suggest that temperature and precipitation are the key factors driving the microbial community, that temperature and elevation also had a great influence on the E. sinensis infection rate, and that environmental factors (temperature and elevation) may further affect the microbial community by regulating the E. sinensis infection rate.
Introduction
Festuca sinensis, a native cool-season perennial grass species, is distributed across cold and semi-arid regions of China. It is important for grassland production and establishment, restoration of degraded grasslands, and ecological management of the Qinghai-Tibet Plateau of China (Lin et al., 2018). Seeds represent one of the most crucial stages in a plant's life history (Finch-Savage and Bassel, 2015). Microorganisms inside and on the surface of seeds play the important roles in the germination and development of seedlings (Nelson, 2018). During seed germination, a new compartment of microbiome is created. Recent omics-based analyses have shown that plant seeds contain beneficial plant-genotype-specific microbes, which can be vertically transmitted from one plant generation to the next (Johnston-Monje et al., 2016; Adam et al., 2018). Therefore, seed microbes play the important roles in the seed itself and in plant growth (Hashsham et al., 2000). Although studies have reported the importance of seed microbiota, there are no reports on the microbiota of F. sinensis seed. Therefore, research on F. sinensis seeds and their microbiota is of great significance for the production and applications of F. sinensis.
Some studies have shown that microbial communities are significantly affected by plant species, the host environment, host genotype, host age, and many other factors (Tannenbaum et al., 2020). Environmental factors, such as temperature, precipitation, and elevation, could be the main factors that affect microbial diversity (Jiang et al., 2016). However, most reports have only focused on the relationships between environmental factors and the soil microbial community. Some studies have found that the soil microbial community may be affected by total carbon (C) and total nitrogen (N) in the soil (Schimel et al., 2007). Some studies have shown that different tillage practices for wheat (Triticum aestivum) can change the microbial diversity in the soil (Lupwayi et al., 1998). However, few studies have highlighted the effects of environmental factors on the microbial diversity of seeds. Klaedtke et al. (2016) found that environmental factors had an important influence on the structure of seed microbiota. However, the relationships between environmental factors (such as temperature, precipitation, and elevation) and microbial diversity of F. sinensis seeds have not been reported.
F. sinensis is frequently infected by an asexual, symptomless Epichloë species that has been identified as Epichloë sinensis (Song et al., 2015; Tian et al., 2020). The recent studies have produced conflicting results regarding Epichloë endophytes in pasture microbiomes. For example, Nissinen et al. (2019) demonstrated that resident E. coenophiala, as a keystone species, had divergent effects on bacterial and fungal communities in the leaf endosphere of F. arundinacea and shaped fungal but not bacterial communities. However, Tannenbaum et al. identified the effects of E. festucae var. lolii on bacterial microbiomes of pooled young perennial ryegrass seedlings (Tannenbaum et al., 2020). These conflicting results suggest that more research on the influence of Epichloë endophytes on microbial communities is needed to understand their mechanisms. Therefore, it is of substantial importance to study the microbiota diversity of different F. sinensis seeds and their relationships with E. sinensis infection rates to clarify the effects of Epichloë endophyte infection on the host seed microbiota.
As environmental factors are very important for the formation of seed microbiota, we wanted to clarify the effects of varied environmental conditions on F. sinensis seed microbiota, using seeds collected from different locations in the Qinghai-Tibet Plateau. Therefore, the aims of this study were to 1) identify the seed microbiome diversity of F. sinensis seeds, 2) identify the abiotic and biotic factors that are likely involved in shaping the microbiome of F. sinensis seeds, and 3) reveal the relationships among E. sinensis infection rate, the microbiota, and environmental factors.
Materials and methods
Seed materials
A total of 14 seed lots of F. sinensis were collected from different locations on the Qinghai-Tibet Plateau, as shown in Table 1 and Supplementary Figure S1. The E. sinensis infection rate in these seed lots was detected by the aniline blue staining method (Nan, 1996). A total of 100 seeds per seed lot were tested, and the E. sinensis infection rate was calculated (Christensen et al., 2008).
DNA extraction
Each seed lot had three replicates, with 50 mg of seeds per replication. The total genomic DNA of each replication was extracted using the CTAB/SDS method after homogenization using a pestle and mortar (Ren et al., 2006). Seed powder (50 mg) was placed in a 2-ml microcentrifuge tube containing 1,000 μl of CTAB buffer and 20 μl of lysozyme. After the sample incubated at 65°C for 2–3 h with occasional mixing, the sample was vortexed briefly and then centrifuged for 10 min at 12,000 rpm. Then, 950 μl of the supernatant was mixed with 950 μl chloroform/isoamyl alcohol (24:25 v/v). Again, after totally mixing by vortexing, the sample was centrifuged for 10 min at 12,000 rpm. After repeating this step, the supernatant was mixed with ice-cold isopropanol (the volume of ice-cold isopropanol is three-quarters of the volume of the supernatant), and the tube was inverted five times to precipitate nucleic acids. The sample was centrifuged at 12,000 rpm for 10 min, and the precipitate was washed two times with 1 ml of 75% ethanol. The remaining small amount of liquid was collected by further centrifugation and then sucked out with a pipette tip. The pellets were air-dried for 2 h. Then, 50 μl ddH2O was added to dissolve DNA samples. Then, 1 μl RNase A was added to digest RNA, and the mixture was incubated at 37°C for 15 min. DNA was diluted to 1 μg/L with sterile water after its purity was quantified using a NanoDrop 2000 spectrophotometer (Thermo Fisher Scientific, Waltham, MA, USA) (Zheng et al., 2018).
Polymerase chain reaction amplification and high-throughput sequencing
Specific primers with barcodes were synthesized to amplify the bacterial 16s rRNA V4–V5 region (515F:5'-ACTCCTACGGGAGCAGCA-3'; 806R:5'-GGACTACHVGGGTWTCTAAT-3') and the fungal ITS1 or ITS2 region (ITS5-1737F: 5'-CTTGGTCATTTAGAGGAAGTAA-3'; ITS2-2043R: 5'-GCTGCGTTCTTCATCGATGC-3') to assess the composition of both bacterial and fungal communities (Klindworth et al., 2013). Phusion® Hi-Fi PCR Master Mix (New England Biolabs, Ipswich, MA, USA) was used for all PCR. Each PCR mixture contained 5 μl genomic DNA (40–60 ng), 1.5 μl forward primer (10 μm), 1.5 μl reverse primer (10 μM), 1 μl Toyobo, 1 μl KOD FX Neo Buffer (2X), 10 μl dNTP (2 mM), and ddH2O was added for a total volume of 50 μl. PCR was performed under the following conditions: one cycle at 95°C for 10 min, 15 cycles at 95°C for 1 min, 50°C for 1 min, 72°C for 1 min, and finally 72°C for 7 min. PCR products were subjected to 2% agarose gel electrophoresis for purification and magnetic beads were purified using a TruSeq®DNA PCR-Free Sample Preparation Kit (Illumina, San Diego, CA, USA). A DNA library was constructed after homogenization and quantified using Qubit and q-PCR (AB 9902). After the library was qualified, NovaSeq6000 was used for sequencing at Genepioneer Biotechnologies (Nanjing, China) with separate runs for the 16S rRNA and ITS amplicon pools (Reuter et al., 2015). All quality sequence files supporting the findings of this study are available in the NCBI Sequence Read Archive (SRA) under BioProject ID PRJNA844205.
Microbial diversity analysis
To maximize the quantity and quality of reliable sequences, the following protocols were performed. TrimGalore (version 0.4.2) software filtered out bases with a terminal mass of less than 20, which might comprise adapter and short sequences less than 100 bp in length. After merging via FLASH2 (V1.2.7) software and removing low-quality sequences, the primer sequences were trimmed using Mothur (version 1.41.1) (Schloss et al., 2009). Sequences less than 100 bp in length or with an error rate of more than two were discarded by USEARCH (version 10.0). High-quantity and high-reliability sequences were clustered into operational taxonomic units (OTUs) with 97% similarity using the UPARSE algorithm and singleton OTUs (with only one read) were removed.
Bacterial and fungal OTU representative sequences were classified taxonomically through BLAST alignment against the SSUrRNA database of SILVA138 (http://www.arb-silva.de/) and the Unit (v8.2) fungal ITS database (http://unite.ut.ee/), respectively (Bryant and Frigaard, 2006; Liu et al., 2012). The OTU tables were rarefied to the sample containing the lowest number of sequences, with a threshold of >10,000 sequences (all samples with < 10,000 sequences were removed from analyses prior to the rarefaction step). A subsequent analysis of diversity was performed based on the output-normalized data. QIIME software (version 1.9.1) was used to compute the diversity indices of Chao1, Simpson, Shannon, and the abundance-based coverage estimator (ACE) based on OTU numbers to study the diversity and structure of the microbial community of F. sinensis seeds (Shade et al., 2017).
Unweighted pair-group method with arithmetic means (UPGMA) cluster analysis was performed to interpret the distance matrix using average linkage which conducted using the QIIME software (version 1.9.1). Principal component analysis (PCA) based on the unweighted UniFrac distances (Bray–Curtis) (QIIME software, version 1.9.1) was used to examine the differences in microbial community structures among 14 seed lot samples based on the relative abundances of OTUs. PCA was performed using the Ade4 package and ggplot2 package of R software (Version 2.15.3).
Statistical analysis
Meteorological data were obtained from the China Meteorological Center (http://data.cma.cn). Based on the longitude, latitude, and elevation of each sampling point, the thin plate smoothing spline algorithm in package Anusplin 4.4 (Version 4.4, Canberra, Australia http://fennerschool.anu.edu.au/files/anusplin44.pdf) was used to calculate the kriging difference to obtain the monthly mean temperature (MMT) and monthly mean precipitation (MMP) at each sampling point from 2006 to 2015. The growing season of F. sinensis is from April to August, and the average temperature and precipitation from April to August were calculated as the mean temperature and precipitation during the growing season. Microsoft Excel 2020 was used to calculate the MMT, MMP, growing monthly mean temperature (GMMT), and growing monthly mean precipitation (GMMP). Spearman's correlation analysis in SPSS 23.0 (version 23.0; SPSS Inc, Chicago, IL, USA) was used to analyze the relationship among environmental factors, E. sinensis infection rate, and microbial diversity, and statistical significance was set at p < 0.05.
One-way analysis of variance (ANOVA) was used to determine statistically significant differences in microbial community diversity among the 14 groups using SPSS 23.0 (version 23.0; SPSS Inc, Chicago, IL, USA). When ANOVA indicated a significant difference, Fisher's least significant difference (LSD) test was applied to conduct multiple pairwise comparisons, and statistical significance was set at p < 0.05.
Redundancy analysis (RDA) was performed using Canoco 5 software (Microcomputer Power, Ithaca, New York, USA), as discussed by Braak (1994). In RDA, microbial parameters were used as “species,” and the ordination axes were constrained to be linear combinations of the environmental factors (i.e., MMT, MMP, GMMT, and GMMP). Thus, this analysis allowed the relationships between environmental factors and microbial parameters to be directly compared. Using the Monte Carlo permutation test (number of permutations), the significance of the environmental factors in accounting for the observed variance in the microbial parameters can be assessed with p-values. In the RDA diagram, positively correlated variables are indicated by arrows pointing in the same direction, negatively correlated variables point in opposite directions, and perpendicular variables are uncorrelated. In addition, the arrow length is a measure of the relative importance of environmental factors in explaining the variances of the microbial parameters. To more clearly understand the impacts of elevation, the samples were divided into 3 groups, including high elevation (2,500–3,000 m), medium-high elevation (3,000–4,500 m), and extremely high elevation (4,500–5,500 m). Additionally, a total of 14 samples were also analyzed together and compared.
Results
Sequencing annotation
After 16S rRNA sequencing, an average of 104,769 tags was detected for each sample, and 65,201 tags were obtained on average for each sample after quality control, with the efficiency of quality control reaching 62.28%. Sequences were clustered into OTUs with 97% identity, and a total of 3,003 OTUs were obtained. Taxonomic annotations of these OTUs were conducted using the SILVA138 database. There were 2,862 (95.30%) OTUs that could be annotated in the database.
After ITS sequencing, an average of 103,696 tags was detected for each sample. The quantity of effective data under quality control reached 65,156 for each sample, and the quality control efficiency reached 62.88%. The OTUs were clustered with 97% identity and 636 OTUs were identified. Taxonomic annotation was performed using the OTUs sequence and the UNITE databases, and 592 (93.08%) OTUs were annotated.
Microbiota diversity in seeds
OTUs level
Both the rarefaction of the sequencing depth (Supplementary Figure S2) and coverage estimators (Supplementary Table S2) indicated that the sequencing depth was sufficient to represent the microbial composition of the samples and to reveal the microbial community structure.
A total of 2,862 bacterial OTUs were obtained by clustering, whereas a total of 1,062 bacterial OTUs were obtained by clustering in the Venn petals (Figure 1A). In addition, 117 OTUs were shared by bacterial communities of these 14 seed lots, accounting for 11.02% of the total number of OTUs. Ecotype S6 had the largest number of unique OTUs (252), followed by ecotypes S4 (248), and S11 (225). Ecotypes S5 and S8 had the lowest numbers of unique OTUs (170), followed by ecotype S7 (180). A total of 592 fungal OTUs were obtained by clustering, whereas a total of 253 fungal OTUs were obtained by clustering in the Venn petals (Figure 1B), and 85 OTUs were shared by fungal communities of these 14 seed lots, accounting for 33.60% of the total number of OTUs. Ecotype S11 had the highest number of unique OTUs (139), followed by ecotype S3 (126). Ecotypes S6 (87), S8 (87), S9 (90), and S4 (90) had the lowest numbers of OTUs.
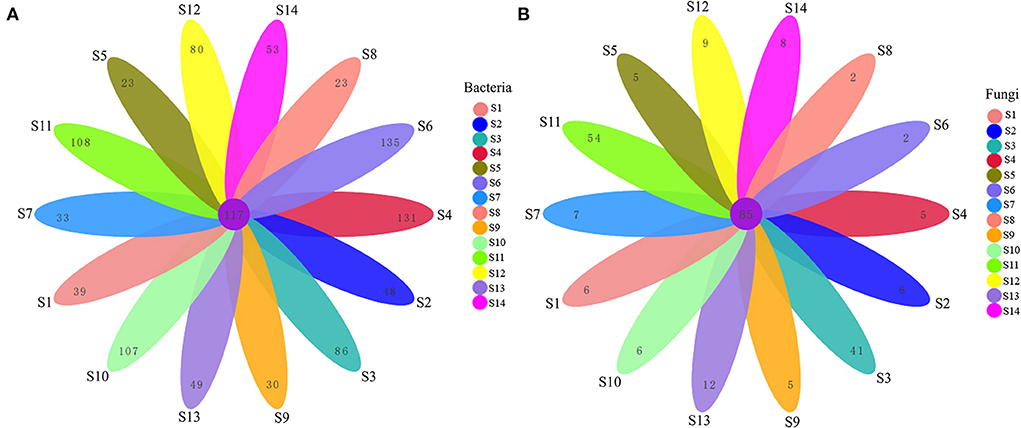
Figure 1. Venn petals of bacteria (A) and fungi (B) at the OTUS level showing the number of commonly and uniquely expressed genes collected the Festuca sinensis seeds from different geographical locations.
Relative abundance of the bacterial and fungal phyla
The relative abundances of the bacterial phyla are shown in Figure 2A. These phyla included Proteobacteria, Cyanobacteria, Bacteroidetes, Chloroflexi, Actinobacteria, Acidobacteria, Firmicutes, Elusimicrobiota, and Verrucomicrobiota. Proteobacteria, Cyanobacteria, and Bacteroidetes were the most abundant phyla. The microbiomes of each seed lot were different. The relative abundances of Proteobacteria in seeds of ecotypes S2, S6, S7, S11, S12, S14, S8, S9, S10, and S13 were over 50%, and Proteobacteria was the most abundant bacterial phylum in the seeds. The relative abundances of the three most abundant phyla in each seed lot are shown in Supplementary Table S2. The relative abundances of Proteobacteria in the seeds of ecotype S13 were significantly higher than those in the seeds of ecotypes S1, S4, S3, S5, S7, S11, S12, S14, and S10 (p < 0.05). The relative abundance of Cyanobacteria in the seeds of ecotype S3 was significantly higher than those in the seeds of ecotypes S2, S9, and S13 (p < 0.05). The relative abundance of Bacteroidota in seeds of ecotype S9 was highest and was significantly higher than those in seeds of the other ecotypes (p < 0.05). The relative abundance of Chloroflexi in the seeds of ecotype S5 was significantly higher than those in the seeds of the other ecotypes (p < 0.05).
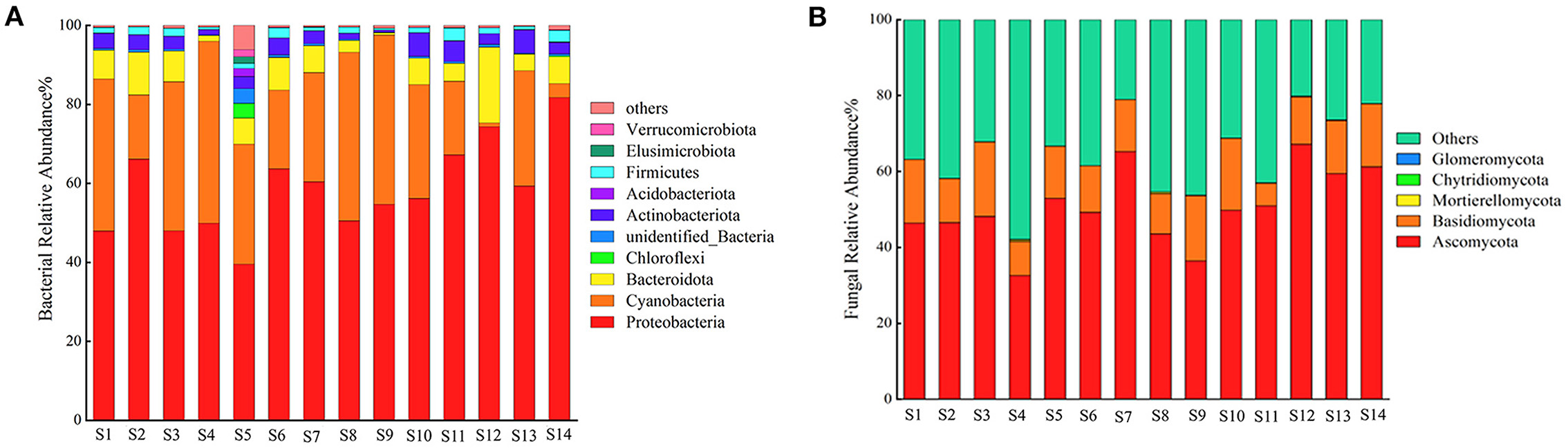
Figure 2. The relative abundance of the top 10 phyla of bacteria in seed lot from 14 ecotypes 16s ribosomal RNA sequencing (A) and fungi in seed lot from 14 ecotypes ITS sequencing (B).
The relative abundances of the fungal phyla are shown in Figure 2B. These phyla include Ascomycota, Basidiomycota, Mortierellomycota, Chytridiomycota, and Glomeromycota. Ascomycota and Basidiomycota were the most abundant fungi, with relative abundances of > 50%. Ecotype S9 had the highest relative abundance of Ascomycota (Supplementary Table S2), which was significantly higher than those in seeds of ecotypes S3 and S12 (p < 0.05). The relative abundance of Ascomycota in seeds of ecotype S3 was significantly lower than those in seeds of ecotypes S7 and S9 (p < 0.05). The relative abundances of Basidiomycota in seeds of ecotypes S4 and S14 were significantly higher than those in seeds of ecotypes S3 and S8 (p < 0.05), and the relative abundance of Basidiomycota in seeds of ecotype S8 was significantly lower than that in seeds of ecotypes S1, S4, S12, and S14 (p < 0.05).
α-diversity of bacterial and fungal communities in seeds
There were significant differences among α-diversity indices of the bacteria of different ecotypes (Table 2). The Chao richness index of ecotype S116 was significantly higher than those of ecotypes S2, S12, S8, S9, and S13 (p < 0.05). The Shannon index of ecotype S9 was significantly higher than that of ecotype S14 (p < 0.05). The Simpson indices of ecotypes S2, S5, S6, S14, S8, and S9 were significantly higher than those of ecotypes S3 and S12 (p < 0.05). The ACE indices of ecotypes S4, S3, and S11 were significantly higher than those of ecotypes S2, S5, S12, S8, S9, S10, and S13 (p < 0.05).
The Chao richness index of fungi in ecotype S11 was significantly higher than that in ecotype S8 (p < 0.05; Table 2) and was not significantly different from that of other ecotypes. The Shannon indices of ecotypes S5 and S14 were significantly higher than those of ecotypes S8 and S13 (p < 0.05) and were not significantly different from those of the other ecotypes. Simpson indices of ecotypes S1, S2, S5, and S14 were significantly higher than those of ecotype S13 (p < 0.05) and were not significantly different from those of the other ecotypes. The ACE index of ecotype S11 was significantly higher than that of ecotype S8 (p < 0.05) and was not significantly different from those of the other ecotypes.
β-diversity of bacterial and fungal communities in seeds
A heat map of the beta diversity measurements is shown in Figure 3. For the bacterial community (Figure 3A), the distances of the samples based on weighted UniFrac were between 0.068 and 0.693, and the distances of the samples based on unweighted UniFrac were between 0.544 and 0.847. For the fungal community (Figure 3B), the distances of the samples based on weighted UniFrac were between 0.104 and 0.790, and the distances of the samples based on unweighted UniFrac were between 0.309 and 0.730. Overall, the UniFrac distances were relatively large.
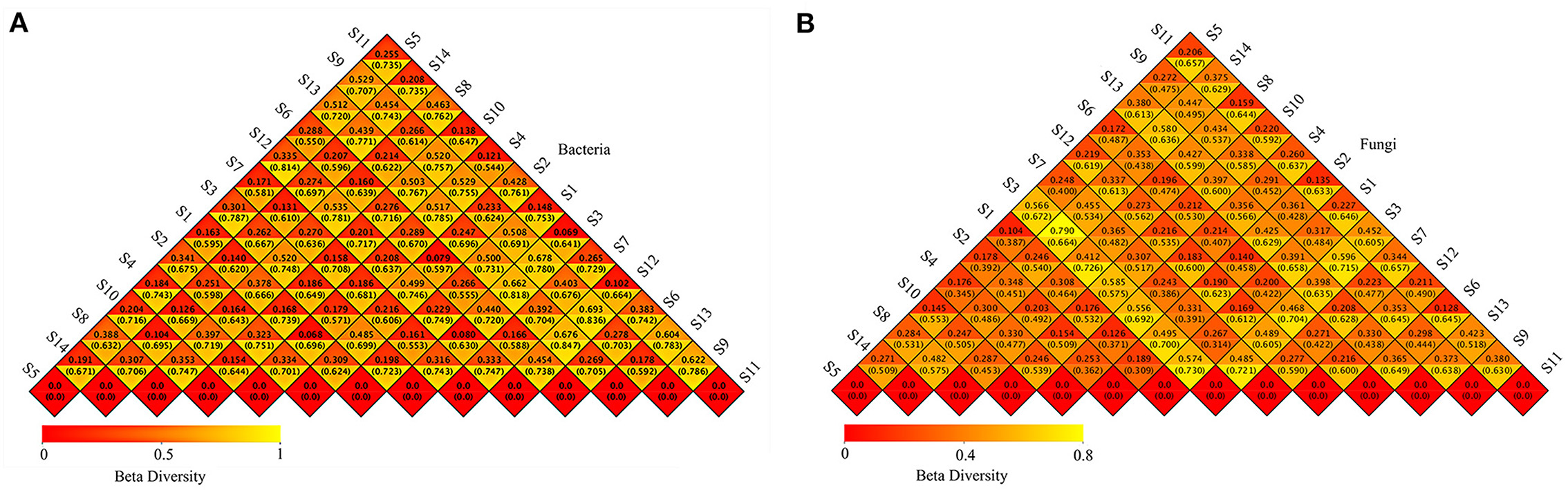
Figure 3. Beta diversity of bacterial (A) and fungal (B) heat map based on the UniFrac distance. The upper triangle is the weighted distance. The distance from the lower triangles is the unweighted distance.
UPGMA cluster and PCA
This study used both UPGMA cluster tree and PCA to cluster the F. sinensis seeds based on unweighted UniFrac (Figure 4). The UPGMA cluster analysis was performed using the unweighted UniFrac distance matrix, and the clustering results were integrated with the relative abundance column chart at the phylum taxon level for each sample. The phylogenetic tree showed that the bacterial communities were divided into four three groups (Figure 4A), and the fungal communities were divided into two major groups (Figure 4B), with each group containing samples from different areas of the Qinghai-Tibet Plateau. The KMO values for PCA of the bacteria (0.847, Supplementary Table S3) and fungi (0.938, Supplementary Table S3) are big enough to run multivariate analysis. For bacterial communities (Figure 4C), the first and second components explained 10.83 and 8.47% of the variances, respectively. For fungal communities (Figure 4D), the first and second components explained 9.66 and 7.4% of the variances, respectively. The results showed that the bacterial communities clustered more distinctively than the fungal communities. The bacterial communities of seed ecotypes S5, S1, S14, and S7 were less similar to those in seed ecotypes S8, S9, S2, S13, and S4, S11, S3, and S12, respectively. The fungal communities of seed ecotypes S11, S13, S12, and S3 were clustered together; however, they were less similar to those in seed ecotypes S10, S8, S6, S14, S9, S7, S1, S2, S4, and S5.
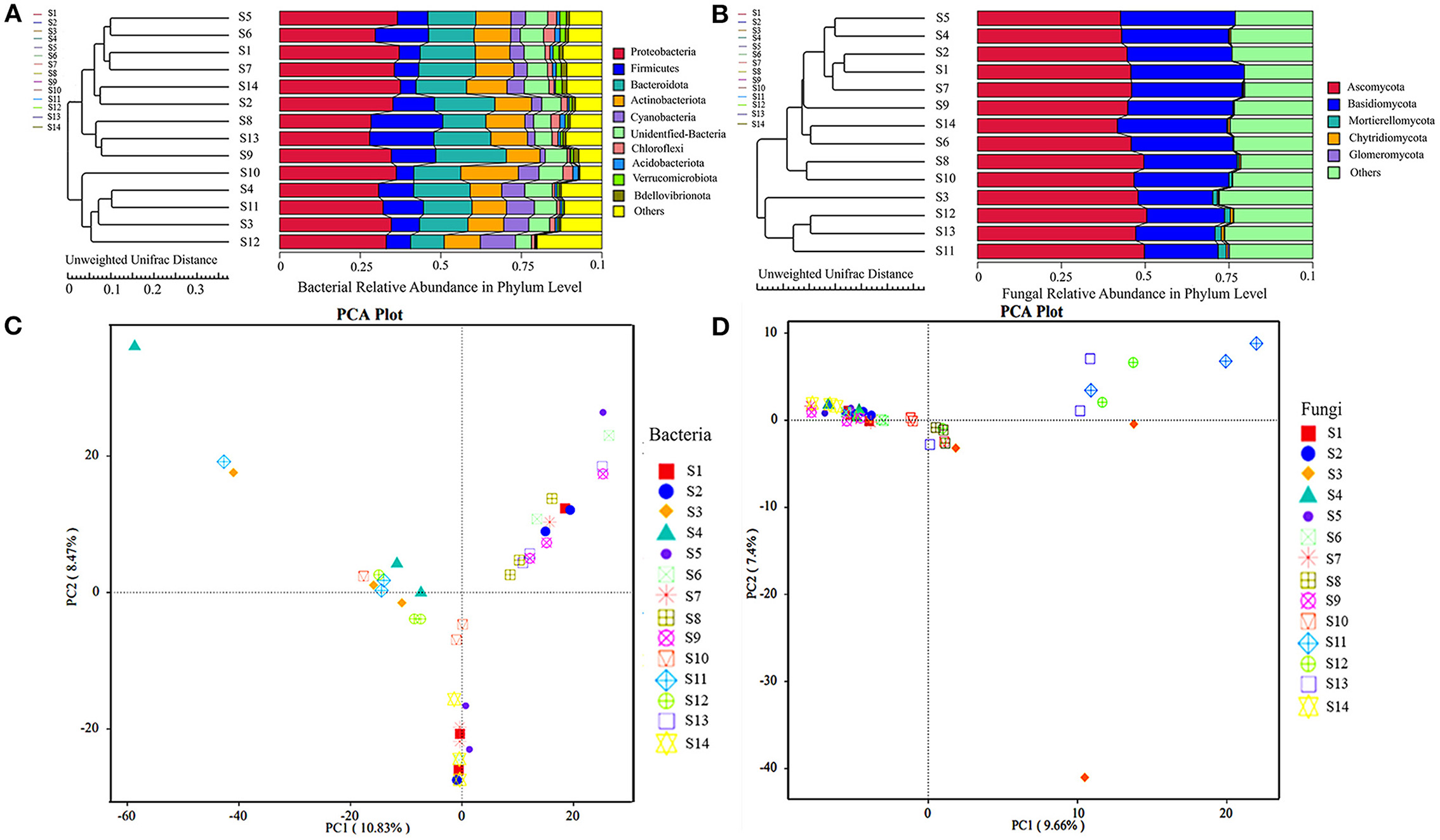
Figure 4. Analysis of F. sinensis seeds microbial communities with unweighted UniFrac. Principal component analysis (PCA) of bacterial (A) and fungal community (B) with unweighted UniFrac. Symbols represent different F. sinensis seeds. UPGMA tree based on unweighted UniFrac distance at the phylum level. Explanation: On the left is the UPGMA cluster tree structure, and on the right is the species relative abundance distribution at the phylum level for each sample. The phylogenetic relationship of the bacteria and fungi was determined by PCR sequencing portions of the 16S rRNA gene (C) and ITS gene (D).
Correlation analysis
Correlation analysis between α-diversity and environmental factors
Analysis of correlations among α-diversity and environmental factors (Table 3) showed that the Chao richness index and ACE index of bacteria were significantly negatively correlated with MMP and GMMP (p < 0.05). However, the Simpson index of bacteria was significantly positively correlated with MMP and GMMP (p < 0.05). The Chao richness index and the ACE index of fungi were significantly negatively correlated with MMT and GMMT (p < 0.05). The E. sinensis infection rate was significantly positively correlated with MMT and GMMT (p < 0.05) and significantly negatively correlated with elevation (p < 0.05).
Correlation analysis of the relative abundances of the most abundant bacteria and fungi with environmental factors
The correlation of the relative abundances of the three most abundant bacterial phyla (Proteobacteria, Cyanobacteria, and Bacteroidetes) with environmental factors was analyzed (Table 4). The relative abundance of Proteobacteria was significantly positively correlated with MMP and GMMP (p < 0.05). The relative abundance of Cyanobacteria was very significantly negatively correlated with MMP and GMMP (p < 0.01). The relative abundance of Bacteroidetes was significantly positively correlated with MMT and GMMT (p < 0.05). The correlation of relative abundances of the two most abundant fungal phyla (Ascomycota and Basidiomycota) with environmental factors was also analyzed (Table 4). The relative abundance of Ascomycota was significantly positively correlated with MMP and GMMP (p < 0.05).
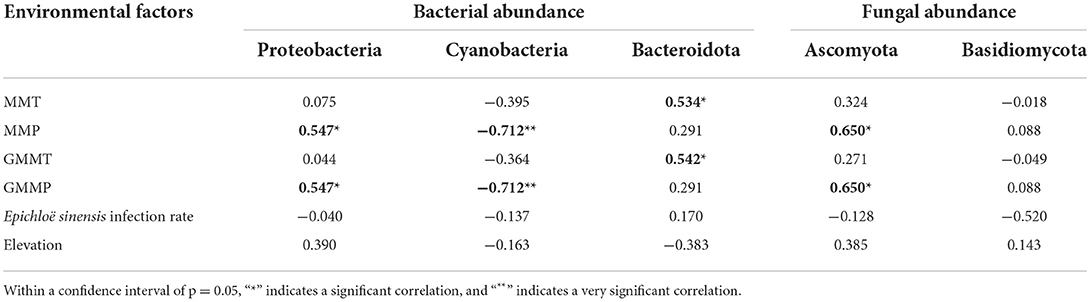
Table 4. Correlation coefficient between the relative abundance of the most abundance bacteria and fungi and environmental factors.
The relationship between the composition of microbial communities and environmental factors
Through Monte Carlo permutation, the ranking results of the four axes could better reflect the relationship between environmental factors and microbial communities (Supplementary Tables S4–S7). The relationships between environmental factors and abundances of bacterial and fungal at the phylum and genus levels were analyzed on the overall elevation, high elevation, medium-high elevation, and extremely high elevation (Supplementary Table S8). On the overall elevation, MMP and GMMT had significant effects on the variation in the bacterial phyla and genera (p < 0.05), with explained degrees of variation being 42.4, 21.0, 48.4, and 17.9%, respectively (Supplementary Table S8). MMP and MMT had a significant influence on the variation in the fungal phyla (p < 0.05, Supplementary Table S8), and their explained degrees of variation were 29.3 and 23.2%, respectively. MMP and GMMP had significant effects on the variation in the fungal genera, with explained degrees of variation of 21.7 and 17.8%, respectively (Supplementary Table S8). These results indicated that temperature and precipitation were the main environmental factors that affected the spatial differentiation of microbial communities. E. sinensis infection rates had a certain effect on the relative abundance of the phyla and genera of fungi (the explained degrees of variation were 7.4 and 9.2%, respectively). Elevation had the smallest effect on the phyla and genera of bacteria, with explained degrees of variation of only 1.0 and 0.4%, respectively. GMMT had the smallest effect on the phyla and genera of fungi, with explained degrees of variation of only 2.5 and 2.1%, respectively. On the high elevation, medium-high elevation and extremely high elevation the result of all axes were F < 0.1, p = 1 (Supplementary Table S8).
Redundancy analysis, as tested by Monte Carlo permutation on the bacterial phyla (Figure 5), fungal phyla (Figure 6), bacterial genera (Figure 7), and fungal genera (Figure 8) of samples at the overall elevation (2,589–5,197 m), high elevation (2,589–3,000 m), medium-high elevation (3,000–4,500 m), and extremely high elevation (4,500–5,197 m), identified the environmental factors (MMT, GMMT, MMP, and GMMP) that were significantly correlated with the microbial community. Environmental factors (GMMP, MMP, GMMT, and MMT) were positively correlated with the abundances of Chloroflexi, Elusimicrobiota, Acidobacteriota, Verrucomicrobiota, and Bateroidota at overall elevation (Figure 5A), high elevation (Figure 5B), and extremely high elevation (Figure 5D), however, negatively correlated with the abundance of Cyanobacteria at overall elevation, high elevation, and extremely high elevation (Figures 5A,B,D). The elevation was positively correlated with the abundances of Cyanobacteria at overall elevation and medium-high elevation (Figures 5A,C), whereas negatively correlated with the abundances of Cyanobacteria at high elevation and extremely high elevation (Figures 5B,D). At medium-high elevation group, GMMT and MMT were positively correlated with the abundance of Elusimicrobiota, Acidobacteriota, Chloroflexi, and Actinobacteriota; however, GMMP and MMP were negatively correlated with them (Figure 5C).
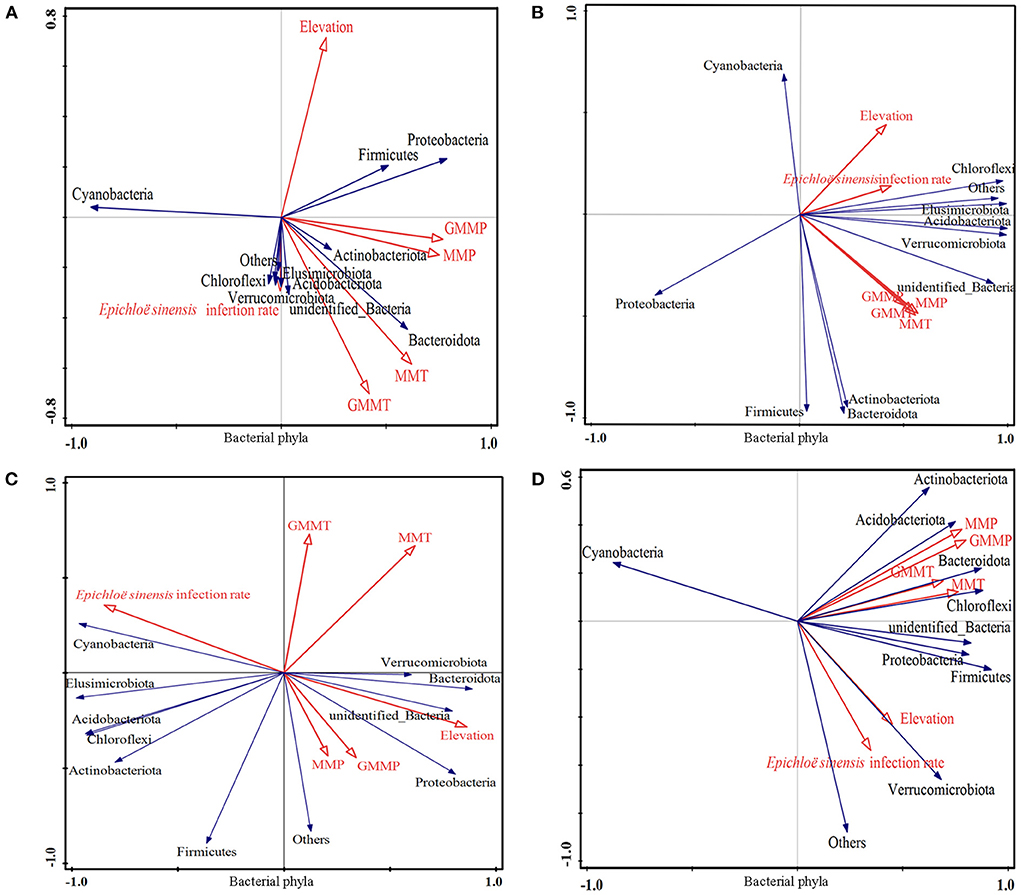
Figure 5. Effect of environmental factors on bacterial phyla. (A) on overall elevation (2,589–5,197 m). (B) on high elevation (2,589–3,000 m); (C) on medium-high elevation (3,000–4,500 m); (D) and on extremely high elevation (4,500–5,197 m). The microbial parameters (expressed as response variables in the RDA) were presented as black line vectors, and the environmental factors (explanatory variables) were presented as red line vectors. MMT, monthly mean temperature; MMP, monthly mean precipitation; GMMT, growing monthly mean temperature; GMMP, growing monthly mean precipitation.
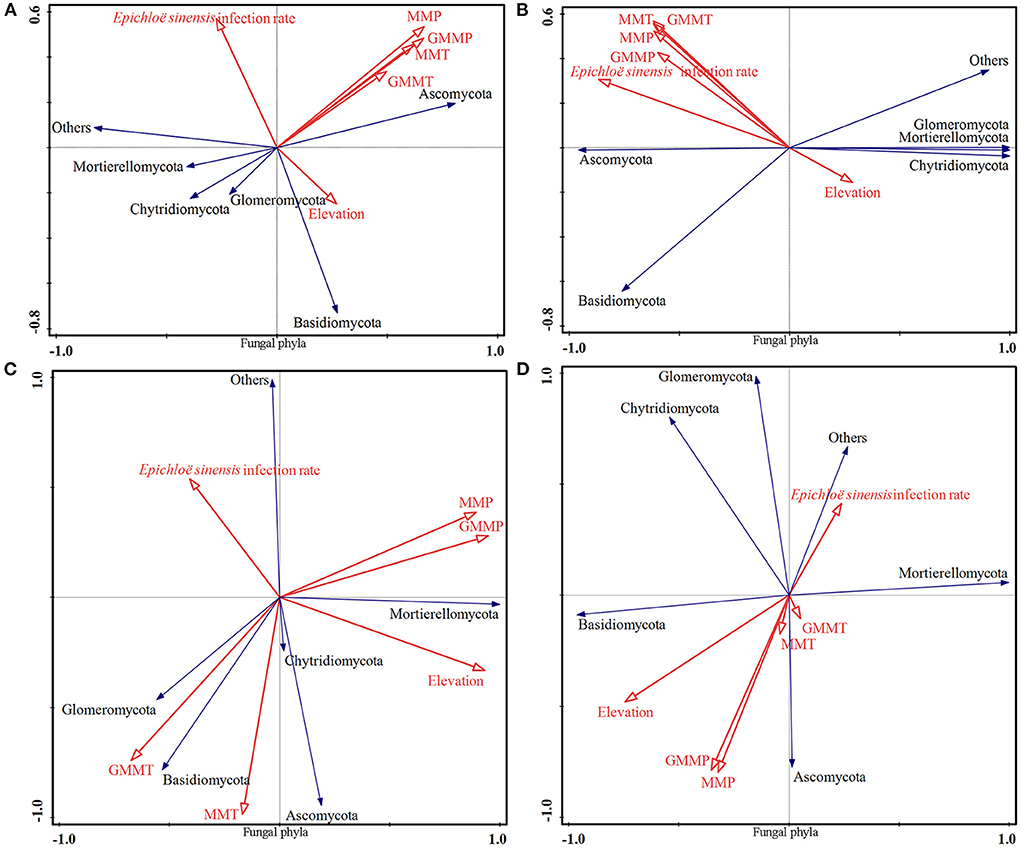
Figure 6. Effect of environmental factors on fungal phyla. (A) on overall elevation (2,589–5,197 m). (B) on high elevation (2,589–3,000 m); (C) on medium-high elevation (3,000–4,500 m); (D) and on extremely high elevation (4,500–5,197 m). The microbial parameters (expressed as response variables in the RDA) were presented as black line vectors, and the environmental factors (explanatory variables) were presented as red line vectors. MMT, monthly mean temperature; MMP, monthly mean precipitation; GMMT, growing monthly mean temperature; GMMP, growing monthly mean precipitation.
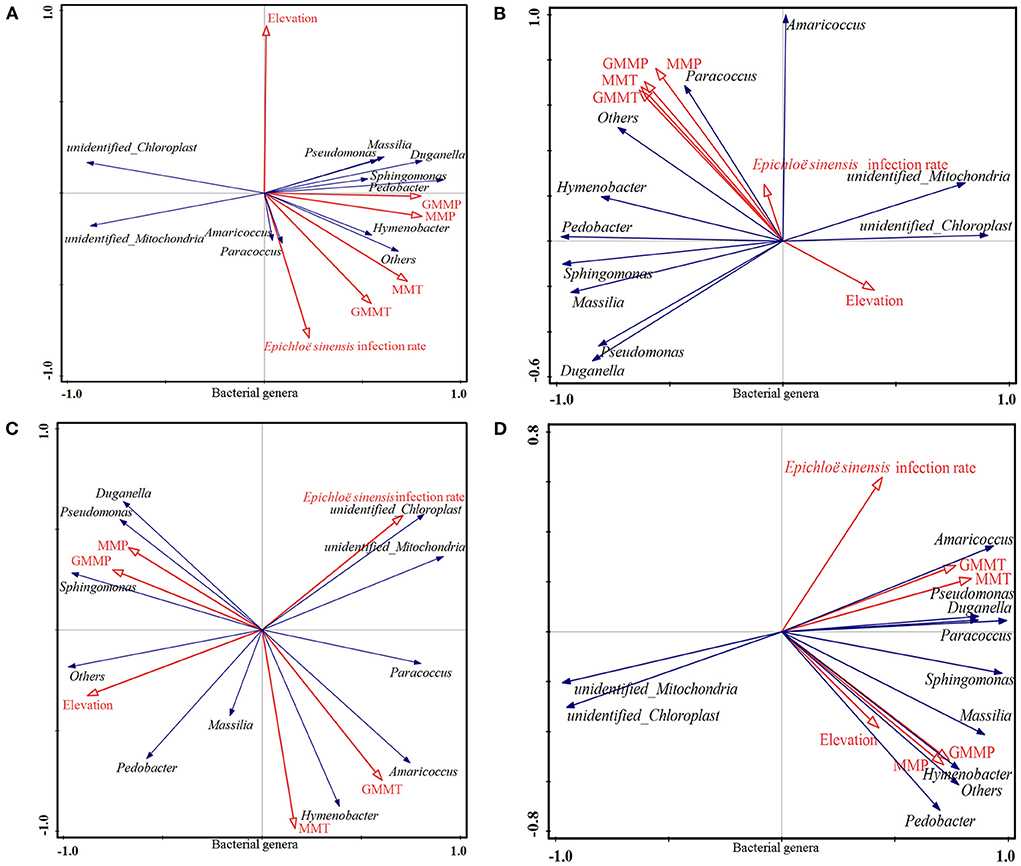
Figure 7. Effect of environmental factors on bacterial genera. (A) on overall elevation (2,589–5,197 m). (B) on high elevation (2,589–3,000 m); (C) on medium-high elevation (3,000–4,500 m); (D) and on extremely high elevation (4,500–5,197 m). The microbial parameters (expressed as response variables in the RDA) were presented as black line vectors, and the environmental factors (explanatory variables) were presented as red line vectors. MMT, monthly mean temperature; MMP, monthly mean precipitation; GMMT, growing monthly mean temperature; GMMP, growing monthly mean precipitation.
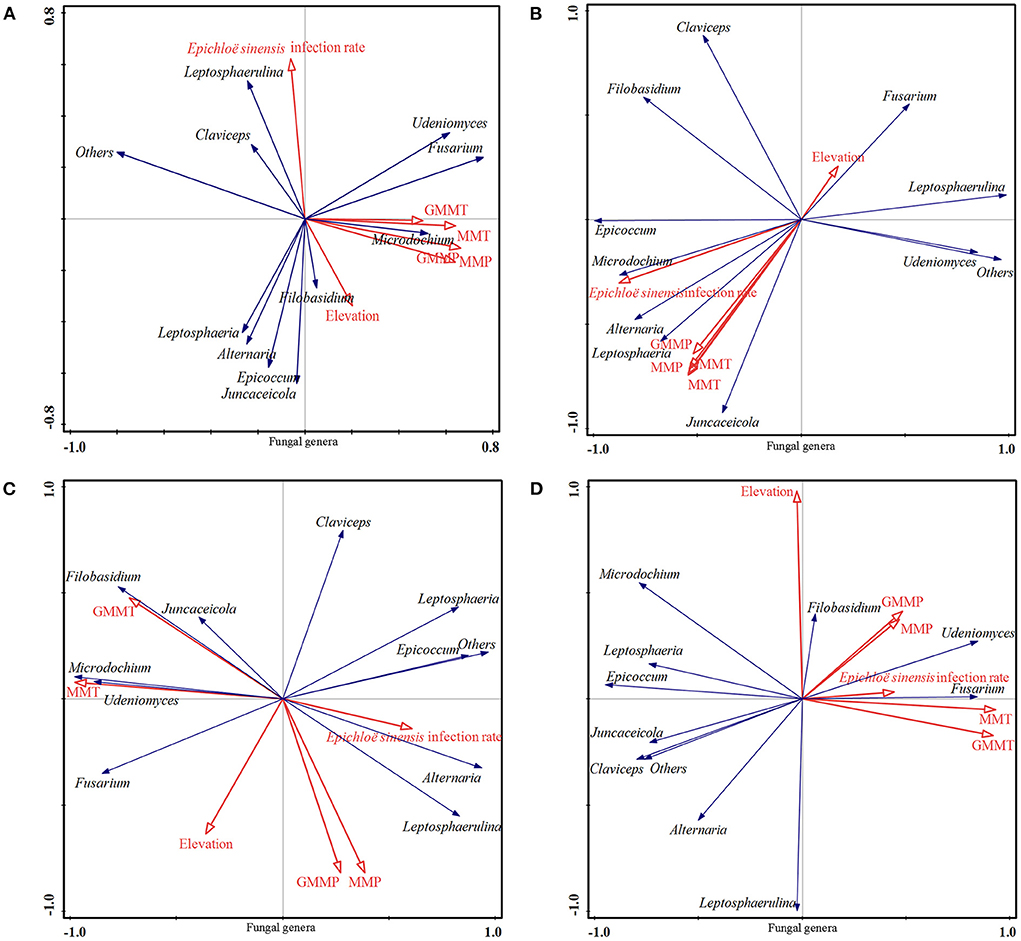
Figure 8. Effect of environmental factors on fungal genera. (A) on overall elevation (2,589–5,197 m). (B) on high elevation (2,589–3,000 m); (C) on medium-high elevation (3,000–4,500 m); (D) and on extremely high elevation (4,500–5,197 m). The microbial parameters (expressed as response variables in the RDA) were presented as black line vectors, and the environmental factors (explanatory variables) were presented as red line vectors. MMT, monthly mean temperature; MMP, monthly mean precipitation; GMMT, growing monthly mean temperature; GMMP, growing monthly mean precipitation.
Environmental factors (MMT, GMMT, MMP, and GMMP) were significantly correlated with the fungal community at the phyla levels (Figure 6). Environmental factors (GMMP, MMP, GMMT, and MMT) were positively correlated with the abundances of Ascomycota (Figures 6A,B,D). The elevation was positively correlated with the abundance of Ascomycota, Basidiomycota, whereas E. sinensis infection rate was negatively correlated with the abundance of Ascomycota and Basidiomycota (Figures 6A,D). At the medium-high elevation, the GMMP and MMP were positively correlated with the abundance of Ascomycota, Chytridiomycota, and Mortierellomycota, whereas negatively correlated with the abundances of Basidiomycota and Glomeromycota (Figure 6C), GMMT and MMT were positively correlated with the abundances of Basidiomycota and Glomeromycota, whereas negatively correlated with the abundances of Ascomycota, Chytridiomycota, and Mortierellomycota (Figure 6C).
As shown in Figure 7, environmental factors (GMMP, MMP, GMMT, and MMT) were positively correlated with the abundances of Amaricoccus, Sphingomonas, Pseudomonas, Paracoccus, Pedobacter, Massilia, Duganella, and Hymenobacter, whereas negatively correlated with the abundances of unidentified_Chloroplast and unidentified_Mitochondria at overall elevation, high elevation, and extremely high elevation (Figures 7A,B,D). For all four groups, the elevation was positively correlated with the abundances of Pseudomonas and Duganella; however, E. sinensis infection rate was negatively correlated with them (Figure 7). At medium-high elevation group, GMMT and MMT were positively correlated with the abundance of Pedobacter, Massilia, Amaricoccus, and Paracoccus, whereas GMMP and MMP were negatively correlated with them (Figure 7C). The GMMP and MMP were positively correlated with the abundance of Duganella, Pseudomonas, and Sphingomonas; however, GMMP and MMP were negatively correlated with them (Figure 7C).
As shown in Figure 8, environmental factors (GMMP, MMP, GMMT, and MMT) were positively correlated with the abundances of Juncaceicola, Filobasidium, Microdochium, Fusarium, and Udeniomyces at overall elevations, high elevations, and extremely high elevation (Figures 8A,B,D). For all four groups, elevation was positively correlated with the abundances of Fusarium and Udeniomyces, and E. sinensis infection rate was negatively correlated with the abundances of Fusarium, Udeniomyces (Figure 8). At the medium-high elevation, GMMP and MMP were positively correlated with the abundance of Leptosphaerulina, Alternaria, Epicoccum, and Leptosphaeria, whereas negatively correlated with the abundances of Juncaceicola, Filobasidium, Microdochium, and Udeniomyces. GMMP and MMP were positively correlated with the abundance of Juncaceicola, Filobasidium, Microdochium, and Udeniomyces, whereas negatively correlated with the abundances of Leptosphaerulina, Alternaria, Epicoccum, and Leptosphaeria (Figure 8C).
Discussion
There is a rich microbial community comprising a diverse range of bacteria (Truyens et al., 2015) and fungi (Shearin et al., 2018) on the surfaces and interiors of plant seeds. In this study, we characterized the seed microbiota from 14 different locations on the Qinghai-Tibet Plateau. A total of 64 bacterial phyla and six fungal phyla were found in the F. sinensis seeds by high-throughput sequencing. Moreover, α-diversity identified the important environment factors (temperature and precipitation) and β-diversity further showed that the microbial community structure varied greatly among different collection sites of the Qinghai-Tibet Plateau, which demonstrated that the microbiota diversity of F. sinensis seeds had a strong relationship with the geographical distribution. In general, the results suggested that the microbiome in different F. sinensis seed lots had different components. Previous research found a total of 54 genera and 129 species of bacteria were isolated from the surface and interior of more than 30 types of crop seeds. Among these bacteria, Proteobacteria was the main phylum, and Firmicutes, Actinobacteria, and Bacteroidetes were the second most common (Liu et al., 2012). Our study was consistent with the results of previous studies. Proteobacteria play a key role in phylogenetic, ecological, and pathogenic processes and participate in energy metabolism, including oxidation and photosynthesis of organic and inorganic compounds (Zhang et al., 2018). Cyanobacteria produce phycoerythrin and phycocyanin, two photosynthetic pigments characteristic of Rhodophyta or red algae, and one or two other smaller groups (Edelman et al., 1967). Cyanobacteria are also well recognized as producers of a wide array of bioactive metabolites including toxins and potential drug candidates (Walton and Berry, 2016). Thus, Cyanobacteria may improve plant growth and seed germination (Chua et al., 2019). Bacteroidetes are increasingly regarded as specialists in the degradation of high molecular weight organic matter, namely, proteins and carbohydrates (Thomas et al., 2011). A possible explanation for our findings is that the climate of the Qinghai-Tibet Plateau leads to more Cyanobacteria in the F. sinensis seeds. The higher amount of Cyanobacteria is more conducive to photosynthesis in F. sinensis, which makes it better adapted for growth in the Qinghai-Tibet Plateau. Ascomycota and Basidiomycota were the most abundant fungi, consistent with the previous studies conducted on alpine meadows in the Yushu Tibetan Autonomous Prefecture, which showed that most fungi belonged to Ascomycota (Chen et al., 2017), Ascomycota mainly decompose cellulose and lignin, and its growth may depend on more readily available energy sources, such as soluble carbohydrates (Osono and Takeda, 2002). Basidiomycota mainly produce lignin-modifying enzymes that degrade lignin (Osono et al., 2003). Ascomycota and Basidiomycota are widely distributed in plants, aquatic ecosystems, and soil, in different proportions (Vandenkoornhuyse et al., 2002). These microorganisms play the important roles in seed health and production.
The coexistence of microbial symbionts and hosts contributes to host adaptation to the natural environment. In turn, the composition of the plant microbial community responds to the environment and the host, making it possible for the plant to benefit. Seeds are involved in the transmission of microorganisms from one plant generation to another and consequently may act as the initial inoculum source for the plant microbiota (Rezki et al., 2018). Seeds have evolved in association with diverse microbial assemblages that may influence plant growth and health (Gibbons et al., 2013). Microbial communities and structures vary and can be influenced by many factors. Previous studies have found that both seed and soil types affect microbial communities (Buyer et al., 1999). Different environmental conditions affect the propagation, infection, and transmission of various microbes, and different species carry different types and numbers of microbes. The same seed can carry different fungi under different growth conditions (Thomas et al., 2016). Environmental factors have different effects on the abundance and diversity of seed microorganisms. High-altitude ecosystems are generally characterized by low temperatures, variable precipitation, decreased atmospheric pressure, and soil nutrient stress, which have major impacts on biodiversity (Moran-Tejeda et al., 2013). We found that precipitation and temperature were the dominant drivers of the bacterial and fungal diversity gradients (Nottingham et al., 2018; Shen et al., 2020). Seeds of different ecotypes carried abundant amounts of different microorganisms that adapt to different environments, including elevation, temperature, and humidity. Some studies found that microbial species richness and diversity were closely correlated with temperature and that microbial diversity decreased under extreme and polar temperatures (Sharp et al., 2014). In our study, temperature and precipitation were the main environmental factors that drive variations in the microbial community. Further correlation analysis showed that the most abundant bacterial phyla were significantly correlated with temperature and the most abundant fungal phyla were significantly correlated with precipitation.
A possible explanation is that temperature and precipitation became the dominant environmental factors that affect bacterial and fungal community diversity in the Qinghai-Tibet Plateau, which is characterized by low temperature and low precipitation due to high elevation. Precipitation has a strong impact on humidity, temperature, and other conditions in the air, which influence the growth of microorganisms. Some studies have shown that certain bacterial populations respond immediately to an increase in soil moisture once the monsoon rains arrive, although the relative abundances of most bacterial phyla showed less variation during the course of the study (McHugh et al., 2014). In this study, it is possible that precipitation affected local atmospheric conditions such as temperature and humidity, which further affected the abundance and diversity of the microbial communities. The E. sinensis infection rate in F. sinensis seeds on the Qinghai-Tibet Plateau was systematically investigated. The asexual Epichloë is extant in the embryos of seeds and is transmitted through the seeds (Wang et al., 2018). There have been extensive studies and reports on the influence of Epichloë endophytes on host performance, including disease and insect resistance, and cold and drought tolerance (Chen et al., 2016; Xia et al., 2018; Bu et al., 2019). However, there are relatively few reports on the effects of Epichloë endophytes on microbial communities, and research results are inconsistent. One study suggested that Epichloë endophytes can affect the microbial diversity in the roots and rhizosphere (Liu et al., 2021). Another study has reported that E. festucae var. lolii strains AR1 and AR37 significantly changed the rhizosphere bacterial community composition of the host perennial ryegrass but had no significant effect on the Pseudomonas community (Li et al., 2017). These studies have shown that Epichloë endophytes had different effects on different microbial communities. However, in this study, E. sinensis infection rate did not affect the relative abundance of the most abundant microbiota. In this study, F. sinensis seeds were obtained from a high-altitude areas of the Qinghai-Tibet Plateau, where the temperature and precipitation differ from those of the plain area. Correlation analysis showed that the Epichloë sinensis infection rate was significantly correlated with temperature and elevation, which further confirmed our hypothesis. Thus, this study provides some clues for studying the effects of Epichloë endophytes on seed microbiota.
The E. sinensis infection rate was also affected by environmental factors, which were significantly negatively correlated with elevation and positively correlated with temperature. Bacon and Siegel (1988) noted a decrease in the seed endophyte infection rate and vegetative tissue of tall fescue after the plants had experienced hot and dry summers and cold winters. Ju et al. found through growth chamber experiments that temperature appears to be a major variable that affects the fluctuation of endophyte frequency in plant tissues (Ju et al., 2006). Previous studies found that high concentrations of carbon dioxide significantly affect the infection frequency of endophytic fungi in tall fescue, and precipitation may promote this grass–fungal symbiosis, leading to higher endophyte infection frequency (Brosi et al., 2011). These studies indicate that endophytic fungi are susceptible to various environmental factors. In this study, F. sinensis seed lots were obtained from different sites on the Qinghai-Tibet Plateau; possibly, the combination of both host genotype and environment interactions led to the variations among the microbiomes of these 14 seed lots. The relationship between endophyte infection rate and host growth environment has not been confirmed as the research has been limited. Some reports have shown that endophyte infection rates of Elymus tangutorum, Lolium rigidum, and F. rubra decreased with increasing elevation (Bazely et al., 2007; Kirkby et al., 2011; Shi et al., 2020). However, other studies on F. ovina, F. eskia, Deschampsia flexuosa, and Poa trivialis did not find a relationship between endophyte infection rate and elevation (Bazely et al., 2007; Granath et al., 2007; Gonzalo-Turpin et al., 2010). Some studies have shown that the frequency of E. alsodes in Poa alsodes was positively correlated with July maximum temperatures, July precipitation, and soil nitrogen and phosphorous (Shymanovich and Faeth, 2019).
Conclusion
The microbiota of F. sinensis seeds of 14 different ecotypes collected from the Qinghai-Tibet Plateau were rich in diversity and were significantly affected by two environmental factors: temperature and precipitation; however, at the medium-high elevation (3,000–4,500 m), the impact of temperature and precipitation on microbial community is different. E. sinensis infection rates in the host F. sinensis seeds varied among different geographic locations, which varied in environmental factors affecting host growth, including elevation and temperature. However, the specific mechanisms of the variations require further investigation.
Data availability statement
The data presented in the study are deposited in the NCBI Sequence Read Archive (SRA) repository, accession number BioProject ID PRJNA844205.
Author contributions
PT designed the experiment, revised, and polished the manuscript and provide guidance for data analysis. YG conducted data analysis and draft the manuscript. YC and QZ provided the experimental materials. ZN provided guidance for samples collection and experimental design. YL and JL helped in data analysis. All authors contributed to the article and approved the submitted version.
Funding
This study was supported by the National Nature Science Foundation of China (31971768), Gansu transportation department science and technology project 2021-07 and Gansu transportation department science and technology project 2021-28.
Conflict of interest
The authors declare that the research was conducted in the absence of any commercial or financial relationships that could be construed as a potential conflict of interest.
Publisher's note
All claims expressed in this article are solely those of the authors and do not necessarily represent those of their affiliated organizations, or those of the publisher, the editors and the reviewers. Any product that may be evaluated in this article, or claim that may be made by its manufacturer, is not guaranteed or endorsed by the publisher.
Supplementary material
The Supplementary Material for this article can be found online at: https://www.frontiersin.org/articles/10.3389/fmicb.2022.956489/full#supplementary-material
References
Adam, E., Bernhart, M., Müller, H., Winkler, J., and Berg, G. (2018). The Cucurbita pepo seed microbiome: genotype-specific composition and implications for breeding. Plant Soil 422, 35–49. doi: 10.1007/s11104-016-3113-9
Bacon, C. W., and Siegel, M. R. (1988). Endophyte parasitism of tall fescue. J. Product. Agric. 1, 45–55. doi: 10.2134/jpa1988.0045
Bazely, D. R., Ball, J. P., Vicari, M., Tanentzap, A. J., Bérenger, M., Rakocevic, T., et al. (2007). Broad-scale geographic patterns in the distribution of vertically-transmitted, asexual endophytes in four naturally-occurring grasses in Sweden. Ecography 30, 367–374. doi: 10.1111/j.0906-7590.2007.04985.x
Braak, C. J. F. (1994). Canonical community ordination. Part I: Basic theory and linear methods. Ecoscience 1, 127–140. doi: 10.1080/11956860.1994.11682237
Brosi, G. B., Mcculley, R. L., Bush, L. P., Nelson, J. A., Classen, A. T., and Norby, R. J. (2011). Effects of multiple climate change factors on the tall fescue–fungal endophyte symbiosis: infection frequency and tissue chemistry. New Phytol. 189, 797–805. doi: 10.1111/j.1469-8137.2010.03532.x
Bryant, D. A., and Frigaard, N. U. (2006). Prokaryotic photosynthesis and phototrophy illuminated. Trends Microbiol. 14, 488–496. doi: 10.1016/j.tim.2006.09.001
Bu, Y. Y., Guo, P., Ji, Y. L., Zhang, S. T., Yu, H. S., and Wang, Z. W. (2019). Effects of Epichloë sinica on Roegneria kamoji seedling physiology under PEG-6000 simulated drought stress. Symbiosis 77, 123–132. doi: 10.1007/s13199-018-0570-3
Buyer, J. S., Roberts, D. P., and Russek-Cohen, E. (1999). Microbial community structure and function in the spermosphere as affected by soil and seed type. Can. J. Microbiol. 45, 138–144. doi: 10.1139/w98-227
Chen, N., He, R. L., Chai, Q., Li, C. J., and Nan, Z. B. (2016). Transcriptomic analyses giving insights into molecular regulation mechanisms involved in cold tolerance by Epichloë endophyte in seed germination of Achnatherum inebrians. Plant Growth Regul. 80, 367–375. doi: 10.1007/s10725-016-0177-8
Chen, Y. L., Deng, Y., Ding, J. Z., Hu, H. W., Xu, T. L., Li, F., et al. (2017). Distinct microbial communities in the active and permafrost layers on the Tibetan Plateau. Mol. Ecol. 26, 6608–6620. doi: 10.1111/mec.14396
Christensen, M. J., Bennett, R. J., Ansari, H. A., Koga, H., Johnson, R. D., Bryan, G. T., et al. (2008). Epichloë endophytes grow by intercalary hyphal extension in elongating grass leaves. Fungal Genet. Biol. 45, 84–93. doi: 10.1016/j.fgb.2007.07.013
Chua, M., Erickson, T. E., Merritt, D. J., Chilton, A., and Muñoz-Rojas, M. (2019). Bio-priming seeds with cyanobacteria: effects on native plant growth and soil properties. Restor. Ecol. 28, S168–S176. doi: 10.1111/rec.13040
Edelman, M., Swinton, D., Schiff, J. A., Epstein, H., and Zeldin, B. (1967). Deoxyribonucleic acid of the blue-green algae (Cyanophyta). Bacteriol. Rev. 31, 315–331. doi: 10.1128/br.31.4.315-331.1967
Finch-Savage, W. E., and Bassel, G. W. (2015). Seed vigour and crop establishment: extending performance beyond adaptation. J. Exp. Bot. 67, 567–591. doi: 10.1093/jxb/erv490
Gibbons, S. M., Caporaso, J. G., Pirrung, M., Field, D., Knight, R., and Gilbert, J. A. (2013). Evidence for a persistent microbial seed bank throughout the global ocean. Proc. Natl. Acad. Sci. U.S.A. 110, 4651–4655. doi: 10.1073/pnas.1217767110
Gonzalo-Turpin, H., Barre, P., Gibert, A., Grisard, A., West, C. C., and Hazard, L. (2010). Co-occurring patterns of endophyte infection and genetic structure in the alpine grass, Festuca eskia: implications for seed sourcing in ecological restoration. Conserv. Genet. 11, 877–887. doi: 10.1007/s10592-009-9927-8
Granath, G., Vicari, M., Bazely, D. R., Ball, J. P., Puentes, A., and Rakocevic, T. (2007). Variation in the abundance of fungal endophytes in fescue grasses along altitudinal and grazing gradients. Ecography 30, 422–430. doi: 10.1111/j.0906-7590.2007.05027.x
Hashsham, S. A., Fernandez, A. S., Dollhopf, S. L., Dazzo, F. B., Hickey, R. F., Tiedje, J. M., et al. (2000). Parallel processing of substrate correlates with greater functional stability in methanogenic bioreactor communities perturbed by glucose. Appl. Environ. Microbiol. 66, 4050–4057. doi: 10.1128/AEM.66.9.4050-4057.2000
Jiang, L. F., Song, M. K., Yang, L., Zhang, D. Y., Sun, Y. T., Shen, Z. G., et al. (2016). Exploring the influence of environmental factors on bacterial communities within the rhizosphere of the cu-tolerant plant, Elsholtzia splendens. Sci. Rep. 6, 36302. doi: 10.1038/srep36302
Johnston-Monje, D., Lundberg, D. S., Lazarovits, G., Reis, V. M., and Raizada, M. N. (2016). Bacterial populations in juvenile maize rhizospheres originate from both seed and soil. Plant Soil 405, 337–355. doi: 10.1007/s11104-016-2826-0
Ju, H. J., Hill, N. S., Abbott, I., and Ingram, K. T. (2006). Temperature influences on endophyte growth in tall fescue. Crop Sci. 46, 404–412. doi: 10.2135/cropsci2005.0282
Kirkby, K. A., Pratley, J. E., Hume, D. E., Faville, M. J., An, M., and Wu, H. (2011). Incidence of endophyte neotyphodium occultans in Lolium rigidum from Australia. Weed Res. 51, 261–272. doi: 10.1111/j.1365-3180.2011.00846.x
Klaedtke, S., Jacques, M. A., Raggi, L., Préveaux, A., Bonneau, S., Negri, V., et al. (2016). Terroir is a key driver of seed-associated microbial assemblages. Environ. Microbiol. 18, 1792–1804. doi: 10.1111/1462-2920.12977
Klindworth, A., Pruesse, E., Schweer, T., Peplies, J., Quast, C., Horn, M., et al. (2013). Evaluation of general 16S ribosomal RNA gene PCR primers for classical and next-generation sequencing-based diversity studies. Nucleic Acids Res. 41, e1–e1. doi: 10.1093/nar/gks808
Li, F., Guo, Y. E., Christensen, M. J., Gao, P., Li, Y. Z., and Duan, T. Y. (2017). An arbuscular mycorrhizal fungus and Epichloë festucae var. lolii reduce Bipolaris sorokiniana disease incidence and improve perennial ryegrass growth. Mycorrhiza 28, 159–169. doi: 10.1007/s00572-017-0813-9
Lin, W. H., Wang, X. X., Wang, J. J., Nzabanita, C., Xu, W. B., Yang, L., et al. (2018). Intra- and interspecific competition of Elymus Nutans griseb and Festuca Sinensis keng. EX EB alexeev infected by Epichloë endophyte. Bangladesh J. Bot. 47, 699–709.
Liu, H., Wu, M., Liu, J. M., Gao, Y. B., and Ren, A. Z. (2021). Endophyte infection influences arbuscular mycorrhizal fungi communities in rhizosphere soils of host as opposed to non-host grass. Eur. J. Soil Sci. 72, 995–1009. doi: 10.1111/ejss.12996
Liu, Y., Zuo, S., Xu, L. W., Zou, Y. Y., and Song, W. (2012). Study on diversity of endophytic bacterial communities in seeds of hybrid maize and their parental lines. Arch. Microbiol. 194, 1001–1012. doi: 10.1007/s00203-012-0836-8
Lupwayi, N. Z., Rice, W. A., and Clayton, G. W. (1998). Soil microbial diversity and community structure under wheat as influenced by tillage and crop rotation. Soil Biol. Biochem. 30, 1733–1741. doi: 10.1016/S0038-0717(98)00025-X
McHugh, T. A., Koch, G. W., and Schwartz, E. (2014). Minor changes in soil bacterial and fungal community composition occur in response to monsoon precipitation in a semiarid grassland. Microb. Ecol. 68, 370–378. doi: 10.1007/s00248-014-0416-3
Moran-Tejeda, E., Ignacio Lopez-Moreno, J. I., and Beniston, M. (2013). The changing roles of temperature and precipitation on snowpack variability in Switzerland as a function of altitude. Geophys. Res. Lett. 40, 2131–2136. doi: 10.1002/grl.50463
Nan, Z. B. (1996). Incidence and distribution of endophytic fungi in seeds of some native and introduced grasses in China. Acta Pratacult Sin. 5, 13–17.
Nelson, E. B. (2018). The seed microbiome: Origins, interactions, and impacts. Plant Soil 422, 7–34. doi: 10.1007/s11104-017-3289-7
Nissinen, R., Helander, M., Kumar, M., and Saikkonen, K. (2019). Heritable Epichloë symbiosis shapes fungal but not bacterial communities of plant leaves. Sci. Rep. 9, 5253. doi: 10.1038/s41598-019-41603-5
Nottingham, A. T., Fierer, N., Turner, B. L., Whitaker, J., Ostle, N. J., McNamara, N. P., et al. (2018). Microbes follow Humboldt: temperature drives plant and soil microbial diversity patterns from the Amazon to the Andes. Ecology 99, 2455–2466. doi: 10.1002/ecy.2482
Osono, T., Fukasawa, Y., and Takeda, H. (2003). Roles of diverse fungi in larch needle-litter decomposition. Mycologia 95, 820–826. doi: 10.1080/15572536.2004.11833041
Osono, T., and Takeda, H. (2002). Comparison of litter decomposing ability among diverse fungi in a cool temperate deciduous forest in Japan. Mycologia 94, 421–427. doi: 10.1080/15572536.2003.11833207
Ren, X. L., Zhu, X. Y., Warndorff, M., Bucheli, P., and Shu, Q. Y. (2006). DNA extraction and fingerprinting of commercial rice cereal products. Food Res. Int. 39, 433–439. doi: 10.1016/j.foodres.2005.09.006
Reuter, J. A., Spacek, D. V., and Snyder, M. P. (2015). High-throughput sequencing technologies. Mol. Cell 58, 586–597. doi: 10.1016/j.molcel.2015.05.004
Rezki, S., Campion., C., Simoneau., P., Jacques, M. A., Shade, A., and Barret, M. (2018). Assembly of seed-associated microbial communities within and across successive plant generations. Plant Soil 422, 67–79. doi: 10.1007/s11104-017-3451-2
Schimel, J., Balser, T. C., and Wallenstein, M. (2007). Microbial stress-response physiology and its implications for ecosystem function. Ecology 88, 1386–1394. doi: 10.1890/06-0219
Schloss, P. D., Westcott, S. L., Ryabin, T., Hall, J. R., Hartmann, M., Hollister, E. B., et al. (2009). Introducing mothur: open-source, platform-independent, community-supported software for describing and comparing microbial communities. Appl. Environ. Microbiol. 75, 7537–7541. doi: 10.1128/AEM.01541-09
Shade, A., Jacques, M. A., and Barrett, M. (2017). Ecological patterns of seed microbiome diversity, transmission, and assembly. Curr. Opin. Microbiol. 37, 15–22. doi: 10.1016/j.mib.2017.03.010
Sharp, C. E., Brady, A. L., Sharp, G. H., Grasby, S. E., Stott, M. B., and Dunfield, P. F. (2014). Humboldt's spa: microbial diversity is controlled by temperature in geothermal environments. ISME J. 8, 1166–1174. doi: 10.1038/ismej.2013.237
Shearin, Z. R. C., Filipek, M., Desai, R., Bickford, W. A., Kowalski, K. P., and Clay, K. (2018). Fungal endophytes from seeds of invasive, non-native Phragmites australis and their potential role in germination and seedling growth. Plant Soil 422, 183–194. doi: 10.1007/s11104-017-3241-x
Shen, C. C., Gunina, A., Luo, Y., Wang, J. J., He, J. Z., Kuzyakov, Y., et al. (2020). Contrasting patterns and drivers of soil bacterial and fungal diversity across a mountain gradient. Environ. Microbiol. 22, 3287–3301. doi: 10.1111/1462-2920.15090
Shi, Q., Matthew, C., Liu, W. H., and Nan, Z. B. (2020). Alkaloid contents in Epichloë endophyte-infected Elymus tangutorum sampled along an elevation gradient on the Qinghai-Tibetan Plateau. Agronomy-Basel 10, 1812. doi: 10.3390/agronomy10111812
Shymanovich, T., and Faeth, S. H. (2019). Environmental factors affect the distribution of two Epichloë fungal endophyte species inhabiting a common host grove bluegrass (Poa alsodes). Ecol. Evol. 9, 6624–6642. doi: 10.1002/ece3.5241
Song, M. L., Chai, Q., Li, X. Z., Yao, X., Li, C. J., Christensen, M. J., et al. (2015). An asexual Epichloë endophyte modifies the nutrient stoichiometry of wild barley (Hordeum brevisubulatum) under salt stress. Plant Soil 387, 153–165. doi: 10.1007/s11104-014-2289-0
Tannenbaum, I., Kaur, J., Mann, R., Sawbridge, T., and Spangenberg, G. (2020). Profiling the Lolium perenne microbiome: from seed to seed. Phytobiomes J. 4, 281–289. doi: 10.1094/PBIOMES-03-20-0026-R
Thomas, D. C., Vandegrift, R., Ludden, A., Carroll, G. C., and Roy, B. A. (2016). Spatial ecology of the fungal genus xylaria in a tropical cloud forest. Biotropica 48, 381–393. doi: 10.1111/btp.12273
Thomas, F., Hehemann, J. H., Rebuffet, E., Czjzek, M., and Michel, G. (2011). Environmental and gut bacteroidetes: the food connection. Front. Microbiol. 2, 93. doi: 10.3389/fmicb.2011.00093
Tian, P., Xu, W. B., Li, C. J., Song, H., Wang, M. N., Schardl, C. L., et al. (2020). Phylogenetic relationship and taxonomy of a hybrid Epichloë species symbiotic with Festuca sinensis. Mycol. Prog. 19, 1069–1081. doi: 10.1007/s11557-020-01618-z
Truyens, S., Weyens, N., Cuypers, A., and Vangronsveld, J. (2015). Bacterial seed endophytes: genera, vertical transmission and interaction with plants. Environ. Microbiol. Rep. 7, 40–50. doi: 10.1111/1758-2229.12181
Vandenkoornhuyse, P., Baldauf, S. L., Leyval, C., Straczek, J., and Young, J. P. W. (2002). Extensive fungal diversity in plant roots. Science 295, 2051–2052. doi: 10.1126/science.295.5562.2051
Walton, K., and Berry, J. P. (2016). Indole alkaloids of the Stigonematales (Cyanophyta): chemical diversity, biosynthesis and biological activity. Mar. Drugs 14, 73. doi: 10.3390/md14040073
Wang, M. Y., McGill, C. R., Southward, R. C., He, X. Z., Card, S. D., and Rolston, M. P. (2018). Epichloë fungal endophyte colonisation and seed quality in developing grass florets-effect of different fertiliser applications. N. Z. J. Agric. Res. 61, 27–41. doi: 10.1080/00288233.2017.1383275
Xia, C., Li, N. N., Zhang, Y. W., Li, C. J., Zhang, X. X., and Nan, Z. B. (2018). Role of Epichloë endophytes in defense responses of cool-season grasses to pathogens: a review. Plant Dis. 102, 2061–2073. doi: 10.1094/PDIS-05-18-0762-FE
Zhang, B. G., Zhang, J., Liu, Y., Guo, Y. Q., Shi, P., and Wei, G. H. (2018). Biogeography and ecological processes affecting root-associated bacterial communities in soybean fields across China. Sci. Total Environ. 627, 20–27. doi: 10.1016/j.scitotenv.2018.01.230
Keywords: Festuca sinensis, seed microbiota, environmental factors, temperature, precipitation, Epichloë sinensis infection rate
Citation: Gao Y, Chen Y, Luo Y, Liu J, Tian P, Nan Z and Zhou Q (2022) The microbiota diversity of Festuca sinensis seeds in Qinghai-Tibet Plateau and their relationship with environments. Front. Microbiol. 13:956489. doi: 10.3389/fmicb.2022.956489
Received: 30 May 2022; Accepted: 01 July 2022;
Published: 03 August 2022.
Edited by:
Juan Sun, Qingdao Agricultural University, ChinaReviewed by:
Chao Yang, Qingdao Agricultural University, ChinaWu Zhang, Lingnan Normal University, China
Copyright © 2022 Gao, Chen, Luo, Liu, Tian, Nan and Zhou. This is an open-access article distributed under the terms of the Creative Commons Attribution License (CC BY). The use, distribution or reproduction in other forums is permitted, provided the original author(s) and the copyright owner(s) are credited and that the original publication in this journal is cited, in accordance with accepted academic practice. No use, distribution or reproduction is permitted which does not comply with these terms.
*Correspondence: Pei Tian, tianp@lzu.edu.cn