- 1Université de Pau et des Pays de l’Adour, E2S UPPA, CNRS, IPREM UMR 525—Bât. IBEAS, BP1155, Pau, France
- 2La Rochelle Université, CNRS, UMR 7266 LIENSs (Littoral Environnement et Sociétés)—2, rue Olympe de Gouges, Bât. ILE, La Rochelle, France
Marine environments are expected to be one of the most affected ecosystems by climate change, notably with increasing ocean temperature and ocean acidification. In marine environments, microbial communities provide important ecosystem services ensuring biogeochemical cycles. They are threatened by the modification of environmental parameters induced by climate change that, in turn, affect their activities. Microbial mats, ensuring important ecosystem services in coastal areas, are well-organized communities of diverse microorganisms representing accurate microbial models. It is hypothesized that their microbial diversity and metabolic versatility will reveal various adaptation strategies in response to climate change. Thus, understanding how climate change affects microbial mats will provide valuable information on microbial behaviour and functioning in changed environment. Experimental ecology, based on mesocosm approaches, provides the opportunity to control physical-chemical parameters, as close as possible to those observed in the environment. The exposure of microbial mats to physical-chemical conditions mimicking the climate change predictions will help to decipher the modification of the microbial community structure and function in response to it. Here, we present how to expose microbial mats, following a mesocosm approach, to study the impact of climate change on microbial community.
1. Introduction
In the marine environment, climate change is predicted to have great and long-term impacts (Pachauri, 2014; IPCC, 2021). The increase in air temperature due to the enhanced greenhouse effect will impact first the surface water temperature and then the deep ocean temperature (Orr et al., 2005; Pachauri, 2014; Hutchins and Fu, 2017; IPCC, 2021). The global surface temperature of the ocean is projected to exceed 2°C (relative to 1850–1900 temperatures) for the 22nd century according to RCP8.5 (Pachauri, 2014). Moreover, global warming is predicted to release faster CO2 into the atmosphere, increasing its concentration from 390 ppm to 700 ppm by 2100 (Pachauri, 2014). This rise will lead to a decrease in pH between 0.3 and 0.4 units. Ocean acidification is associated with increasing concentrations of HCO3–, CO2, and H+ and decreasing concentrations of CO32–. Thus, the global carbon cycle is directly affected by ocean acidification. Ocean models also predicted a decline in the dissolved oxygen inventory of 1–7% by 2100 (Schmidtko et al., 2017). These physical-chemical changes will have a strong impact on marine life. Microorganisms are abundant and diverse in the oceans, with 4 × 1029 cells in the deep oceanic subsurface, 5 × 1028 cells in the upper oceanic sediment and 1 × 1029 cells in the oceans (Flemming and Wuertz, 2019). They dominate the metabolic activity, for example, ensuring approximately half of the global primary production of the oceans (O’Brien et al., 2016). They play a central role in the biogeochemical cycles, as well as in the exchange of trace gases that have direct impacts on local climate (O’Brien et al., 2016). They are also crucial for the good functioning of the aquatic ecosystems being at the basis of food webs. Thus, marine microbial communities are expected to play a central role in the response of the ecosystem faced with environmental change because of their key functions in the oceans (Azam and Malfatti, 2007).
Microbial mats are laminated microbial structures (van Gemerden, 1993) observed at the water-sediment interface in several environments (Fourçans et al., 2004; Bolhuis et al., 2014; Mazière et al., 2021). They show a vertical stratification according to different physical-chemical gradients such as oxygen, sulphide, and pH. In such organization different interacting metabolisms coexist allowing microbial mats to be self-sustaining structure ensuring important ecosystem services in coastal areas (Pajares et al., 2015). In response to environmental changes, microbial mats can show various strategies due to their versatility and large diversity, as well as to their functional resilience (Bordenave et al., 2007). They represent ideal microbial community model to study the effect of global changes.
Current climate change effects on microbial communities can be investigated but it is difficult to simulate the conditions induced by climate change in situ, especially the acidification that remains difficult to control in open natural environments. Different approaches have been developed to study climate change effects on organisms inhabiting marine environments. Some studies focused on specific natural environments defined as models where one or several environmental parameters are similar to those predicted by IPCC, such as hot springs to simulate a high water temperature (Kitidis et al., 2011; Danovaro et al., 2017). However, the microorganisms inhabiting these environments are already adapted to the physical-chemical changes occurring on them and no temporal changes comparable to those due to climate change are observed. Moreover, the environmental complexity does not allow a definitive conclusion on the cause(s) of the observed changes. Thus, the precise impact on the environmental functioning and the dynamic of the microbial community in response to climate change cannot be studied.
Global changes are rapid simultaneous changes of environmental factors due to anthropogenic activities which threaten marine microorganisms. So far, little knowledge has been acquired to predict the effect of combined alteration of several environmental factors on marine microbial communities. Understanding the microbial communities shifts in response to the modification of environmental factors would allow to predict the effect of global changes on ecosystem functioning (Pajares et al., 2015). In order to gain such knowledge, it is thus necessary to develop accurate and controlled strategies to expose model microbial community to controlled climate change conditions. The mesocosms are a good approach because they allow the implementation of artificial scenarios by controlling physical-chemical parameters mimicking as close as possible the natural environment or the environmental conditions to be simulated (Cravo-Laureau and Duran, 2014). It is also possible to distinguish each factor independently allowing thus the characterization of their precise impact. Other advantages are that replicates and analysis can be multiplied, as well as manipulations can be performed without the constraints encountered in situ (Petersen et al., 2009). Data collection and analysis are thus facilitated. Mesocosms are enclosed ecosystem experiments that have gained in popularity as research tools in ecological science, particularly in the study of coastal aquatic environments (Petersen et al., 2009). Mesocosms have been developed for many applications, including the study of environmental stress (Pajares et al., 2012), hydrocarbon degradation (Cravo-Laureau and Duran, 2014), simulated oil spill (Chronopoulou et al., 2013), climate change simulation (Stewart et al., 2013; Mazière et al., 2022). Mesocosms have been used to analyse the behaviour of specific microbial communities (Chronopoulou et al., 2013; Stauffert et al., 2013), and of complex microbial structure such as biofilm (Agogué et al., 2014) and microbial mats (Mazière et al., 2022). They allow the control of different physical-chemical parameters and the association of them that can act synergistically (Baragi et al., 2015; Baragi and Anil, 2016; Li et al., 2016; Mazière et al., 2022), which thus allows to disentangle the impact of the studied factors from other environmental factors (Cravo-Laureau and Duran, 2014). So far, most studies have focused on the impact on one specific population or microorganisms (Widdicombe et al., 2009; Beman et al., 2011; Gingold et al., 2013; Guanyong et al., 2017; Lee et al., 2017; Tan et al., 2019) in a specific environmental compartment, such as water column and deep-sea benthos (Danovaro et al., 2017). However, the investigation of complex microbial assemblages is necessary to obtain data representative of the reality (Dimitriou et al., 2017). For that, a good microbial community model, representing a complex network of microorganisms, diversified at both the taxonomic and functional levels, is required. Microbial mats have been shown to represent good microbial community models in experimental ecology, particularly revealing the effect of oil spills on microbial community structure (Bordenave et al., 2004b,2007) and functioning (Bordenave et al., 2004a,2008). Moreover, microorganisms inhabiting microbial mats are well-adapted to physical-chemical fluctuations (Fourçans et al., 2006) making them adequate models to study climate change in microbial ecosystems. To the best of our knowledge, only a few studies have reported the impact of climate change on microbial mats (Verleyen et al., 2010; Mazière et al., 2022). In this paper, an experimental methodology is proposed where microbial mats were used as a microbial community model in a mesocosm experiment, representative of coastal areas, which showed to be a good way for deciphering climate change effect on complex microbial community.
2. Materials and methods
Climate change was simulated by ocean acidification and warming according to the most pessimistic scenario (RCP8.5) predicted by IPCC (Pachauri, 2014).
2.1. Mesocosms design
Microbial mats for mesocosm experiments came from a non-exploited salt marsh located in Ars-en-Ré (46°13′29.9″N 1°31′07.5″W, Ré Island, France). These microbial mats were selected as microbial model to study the climate change impact because they presented layers more developed than those from exploited ponds (Mazière et al., 2021). A constant water height of 3 cm was maintained above them by the salt marsh owner to prevent microbial mats from being exposed to the air. Microbial mats were sampled with a core collector (2 cm mat and sediment depth), and transferred while maintaining their integrity, into mesocosms boxes (48 × 33 cm). Their transport to the laboratory was done without water, in the dark and at room temperature to avoid the destruction of the vertical structure.
Four sets of six mesocosms were built, each representing a treatment (Figure 1). The water was distributed independently from the seawater reservoir on each microbial mats via stainless steel taps. This seawater was filtered at 80 μm and passed under UV light to prevent the presence of other marine organisms in the mesocosms system. The salinity was adjusted to that measured in situ [60 practical salinity unit (psu)] by adding salt coming from the salt marshes of the Ré Island.
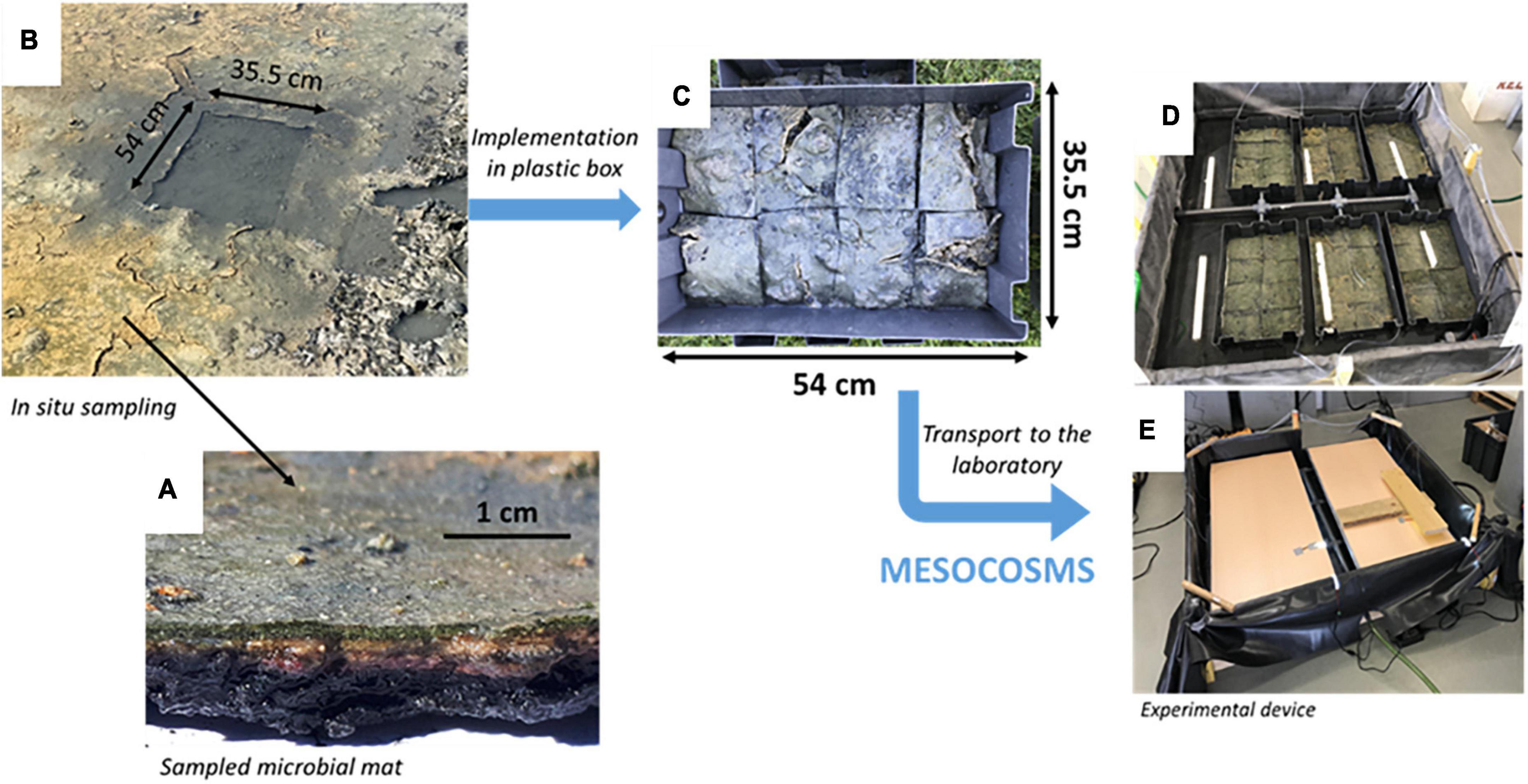
Figure 1. In situ sampling of the microbial mats for mesocosms experiment. Microbial mats were placed in containers with the sediment samples and transported to the laboratory for the mesocosms experiment. (A) Sampled microbial mat and its laminated structure. (B) In situ sampling of the microbial mat with a mold. (C) Implementation of the sampled microbial mat cut in eight parts to transfer it by avoiding damaging it as much as possible in a representing one mesocosm. (D) Six mesocosms circled by a pool representing one treatment. (E) The same than panel (D) with the light system above.
A stable water level was maintained at 3 cm above the mat with a water outlet in front of its inlet. The microbial mats in salt marshes were under a continuous flow-through water depending on the evaporation rate and thus, of the daily temperature making the determination of the water flux difficult. The microbial mats in the mesocosms were under a continuous slow (0.5 L/h; dilution rate estimated around 10–5/h; water turnover in 1h20) flow-through water diffusion simulating a slow water inlet occurring in salt marshes, which ensures water renewal supplying natural nutrients for the development of microbial mats (Figure 1). The microbial mats were illuminated by LED (TOP-24H company by SYLED, France) 12 h a day following the day/night cycle observed during the spring in France (Figure 1). They provided white cold light colour, limiting the evaporation rate, and intensity of 12 ± 1 μmol.photons.m–2.s–1 (corresponding to 874 ± 70 lux) (HOBO Pendant® Temperature/Light Data Logger, Onset Computer Corporation, USA) on the surface of the microbial mats. The LED approximates a solar spectrum according to the manufacturer’s instructions. It is certified like a natural light, used for the cultivation of aquatic plants.
For each treatment, the desired temperature water above the microbial mats was maintained by placing the six containers with the sediment samples in a thermostated water bath with a pump (EHEIM universal 600, Germany) connected to a thermoregulating device (Teco®) (Figure 2).
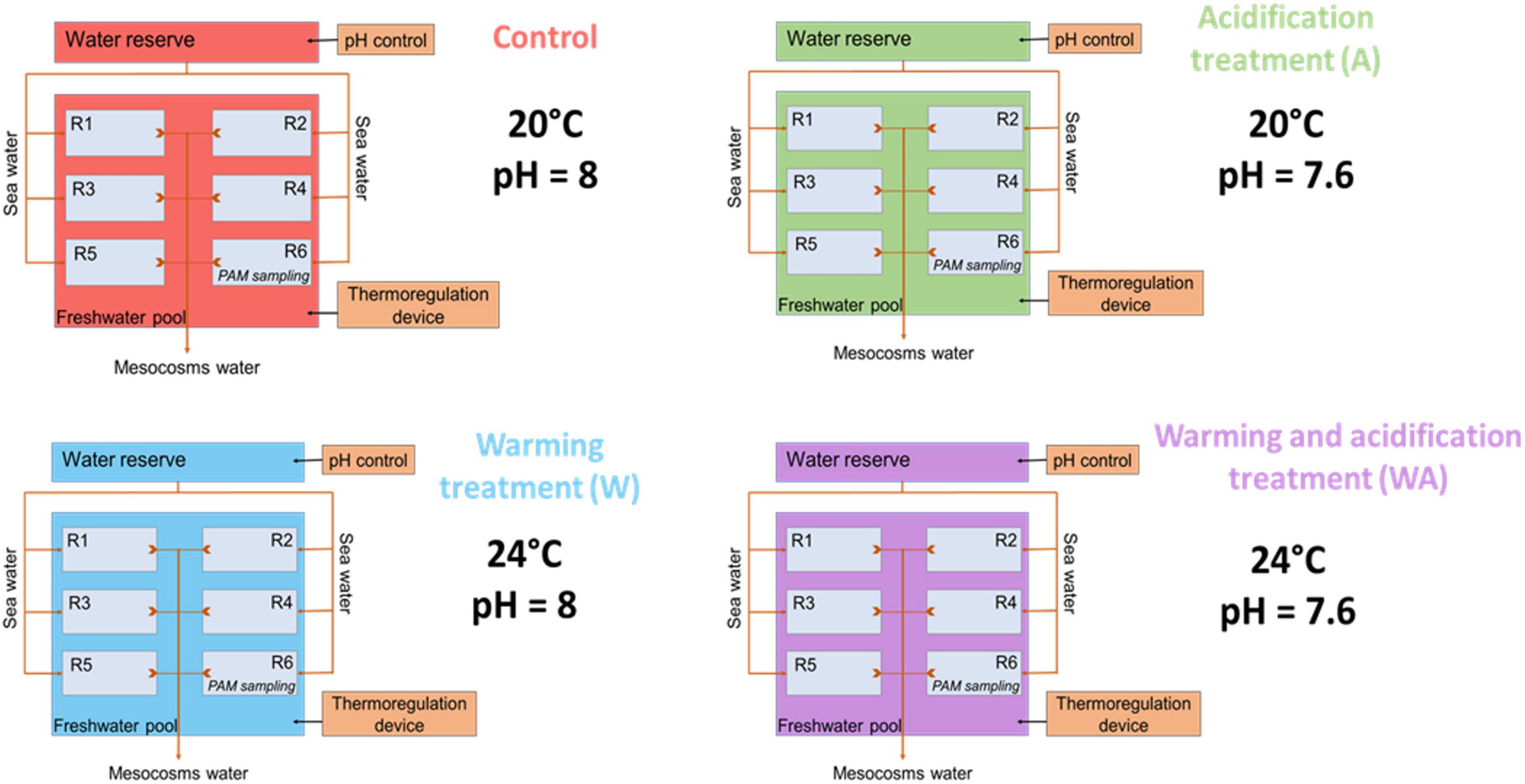
Figure 2. Schematic representation of the experimental design with the control (C), acidification (A), warming (W), and mixed (WA) treatments. The letter R represents the replicates. The first five replicates were used for sampling to perform the analysis listed in Table 1 and the sixth was dedicated to evaluate the photosynthetic activity potential measuring the chlorophyll a fluorescence by pulse amplitude modulation (PAM) and physical-chemical gradients. This figure was modified from Mazière (2021) and Mazière et al. (2022).
2.2. Simulated scenarios
In order to acclimate the microbial mats to their new environment, a stabilisation period was applied (Stauffert et al., 2013; Gette-Bouvarot et al., 2015) maintaining the temperature (20°C) and salinity (60 psu) observed in situ. In this experiment, this period lasted 5 weeks ending when the physical-chemical parameters (salinity, temperature, pH, and dissolved oxygen concentration) in the water above microbial mats were stabilised for 1 week [see figure 2 in Mazière et al. (2022) and figure A in its Supplementary Materials].
A control (C) in which the parameters were not changed, and three treatments were then applied to the microbial mats for seven additional weeks [see figure A in the Supplementary Materials of Mazière et al. (2022)]. The first treatment was the warming treatment (W) in which the temperature was increased by 0.5°C every 2 days for 2 weeks until reaching 24°C. The acidification treatment (A) represented the second condition. Water acidification was performed in the water reservoir with the addition of CO2 (IKS aquastar, iks ComputerSysteme GmbH, Germany), allowing a drop of initial water pH of 0.1 unit every 4 days for 2 weeks until reaching a decrease of the initial pH of the water reservoir equal to 0.4 unit, i.e., 7.6. The water pH in the water reservoir was precisely monitored with a pH probe (826 pH mobile, Ω Metrohm, Swiss) to adjust the pH on IKS device by taking into account the alkalinity using the seacarb package on RStudio software (version 4.2.1© RStudio, R Core Team, 2020). This probe was calibrated in standard solutions (pH 4, 7, and 10, HANNA (instruments, France). The third treatment combined warming and acidification treatments (WA). Acidification and water warming were performed over 2 weeks to mimic a continuous temporal variation and not a sudden change in these parameters like the predicted IPCC scenarios (Pachauri, 2014). The mesocosms were then maintained with the stable conditions for five further weeks.
Many parameters were monitored to allow the most accurate experimental ecology approach possible, combining physical-chemical, diversity, functional, and biochemical analyses (Table 1). The sampling strategy was described by Mazière et al. (2022). Briefly, measurement of all these parameters was done before the change of conditions (t9) and regularly during their variation and stabilization (t16, t23, t30, t37, t44, t51, and t58). Among the six replicates, one was specifically dedicated for the evaluation of the community’s maximum potential for photosynthetic activity by measuring the chlorophyll a fluorescence with pulse amplitude modulation (PAM) as described in Mazière et al. (2022). The specific replicate was dedicated to PAM analysis because sampling for the analyses listed in Table 1 were performed immediately beforehand, leading to a suspension of biological material, which can disturb PAM analysis.
2.3. DNA extraction and sequencing
Microbial mats subsamples of 0.25 g were done and used for DNA extraction using the DNeasy PowerSoil kit (Qiagen) according to the manufacturer’s instructions. The bacterial V3-V4 region of the 16S rRNA gene was amplified using the primers 344F (5′-ACGGRAGGCAGCAG-3′) and 801R_m (5′-ACCAGGGTATCTAATCCT-3′) (Liu et al., 2007). PCR mix consisted in 12.5 μL of AmpliTaq Gold 360 master mix (Applied Biosystems), 1 μL of each primer (10 μM) and 1 μL of genomic DNA, in a final volume of 25 μL (adjusted with distilled water). All amplifications were performed on a Veriti 96 Well Thermal Cycler (Applied Biosystem) using the following PCR program: 10 min at 95°C, 30 cycles of 30 s at 95°C, 30 s at 63°C, and 40 s at 72°C, and finally, 10 min at 72°C. The sequencing was performed by the Genomic platform of Roscoff (France), using Illumina MiSeq technology.
2.4. Statistical analyses
The bioinformatic analyses on the DNA raw data obtained after sequencing were performed using the SAMBA (Standardized and Automated MetaBarcoding Analyses workflow) (v3.0.0) workflow written by SeBiMER, the IFREMER’s Bioinformatics Core Facility (Noël et al., 2021). Two replicates of the control condition at t44 and t58 were removed because they didn’t pass the quality control test during the data integrity step of the workflow. The taxonomic affiliation was performed against the Silva database v138 (Quast et al., 2012; Yilmaz et al., 2013) with 99% of similarities. All calculations and statistical analyses were performed on RStudio software (version 4.2.1 (RStudio, R Core Team, 2020). The statistical analyses used to interpret the physical-chemical data, the pigmentary composition, and the extracellular polymeric substances (EPS) concentration were described in Mazière et al. (2022). Non-metric multi-dimensional scaling (NMDS) analysis was based on the Bray–Curtis distance matrices calculated from the bacterial Amplicon Sequence Variants (ASVs) table (Supplementary Table 1). In order to define whether the treatment at a sampling time explained the variance among the Bray–Curtis distance matrices, a permutational multivariate analysis of variance (PERMANOVA) was performed with the adonis function of the vegan package. Linear discriminant analysis effect size (LEfSe) (Segata et al., 2011) on the 1,000 more abundant ASVs was performed on Galaxy web application to determine bacterial genera biomarkers for each treatment. The non-parametric Kruskal–Wallis sum-rank test (α = 0.05) was performed to detect taxa with significant differential abundance. The biological consistency was investigated by performing a pairwise Wilcoxon test (α = 0.05). A linear discriminant analysis (LDA) threshold score of 2.0 was applied.
3. Results and discussion
Although the decrease of 0.4 unit of pH (upH) in the seawater reservoir of the acidification treatments was effective, the water pH was not significantly different between the treatments [see figure 2 in Mazière et al. (2022) and figures B, D in its Supplementary Materials]. Such phenomenon has been previously described; it can be explained by abiotic factors controlling the CO2 chemistry in seawater (Zeebe and Wolf-Gladrow, 2001) and by biotic factors linked to oxygenic photosynthesis (Giordano et al., 2005; Crawfurd et al., 2011; Ma et al., 2019). Thus, it can be hypothesized that the acidification might result in CaCO3 dissolution, which in turn consume the protons in excess counteracting the acidification (Middelburg et al., 2020), explaining that pH was not decreased in our experiment. Regarding the biotic factors, it is known that many microbial species possess mechanisms allowing the storage of high CO2 concentrations named carbon concentration mechanisms (CCMs). The CCMs have been described in oxygenic phototrophs like algae (Giordano et al., 2005) and cyanobacteria (Ma et al., 2019). The storage of the CO2 added for acidification in CCMs might also explain why the decrease of pH was not observed in our experiment. However, when the maximum CO2 concentration capacity of the CCMs will be reached in the cells, it could be expected to observe a pH decrease in the water column due to the added CO2 (Black et al., 2019). Moreover, the dissolved oxygen concentration was observed to be more increased than in the other treatments, likely due to an increase in photosynthesis because of the carbon input (Black et al., 2019; Mazière et al., 2022). As expected, the temperature increased by 4°C from the initial temperature in the warming treatments [see figure 2 in Mazière et al. (2022)]. An increase in salinity was also observed in the warming treatments probably because of water evaporation [see figure 2 in Mazière et al. (2022)].
Thus, the mesocosms experiment allowed to change and maintain the conditions as expected (acidification of the water input and water warming on the mesocosms) (Petersen et al., 2009; Cravo-Laureau and Duran, 2014; Mazière et al., 2022). Such changes in environmental conditions are difficult to produce in situ because of the environmental complexity (day/night alternation, weather hazards, human impact…). By changing the conditions one by one, the changes in the other measured parameters could be attributed to the treatment. In our study, the warming treatment impacted the salinity whereas the acidification treatment affected the dissolved oxygen concentration. Thus, the mesocosms were effective to control the physical-chemical parameters for simulating climate change.
After changing the conditions, the bacterial community composition differed in the acidification treatment 3 weeks after (t44) in comparison to the other treatments (Figure 3) (PERMANOVA, p < 0.05). At the end of the experiment (t58), no more difference was observed between the acidification treatment and the control and warming treatments (Figure 3), suggesting that the bacterial community was resilient. However, the bacterial community composition differed, certainly due to the WA treatment (PERMANOVA, p < 0.05).
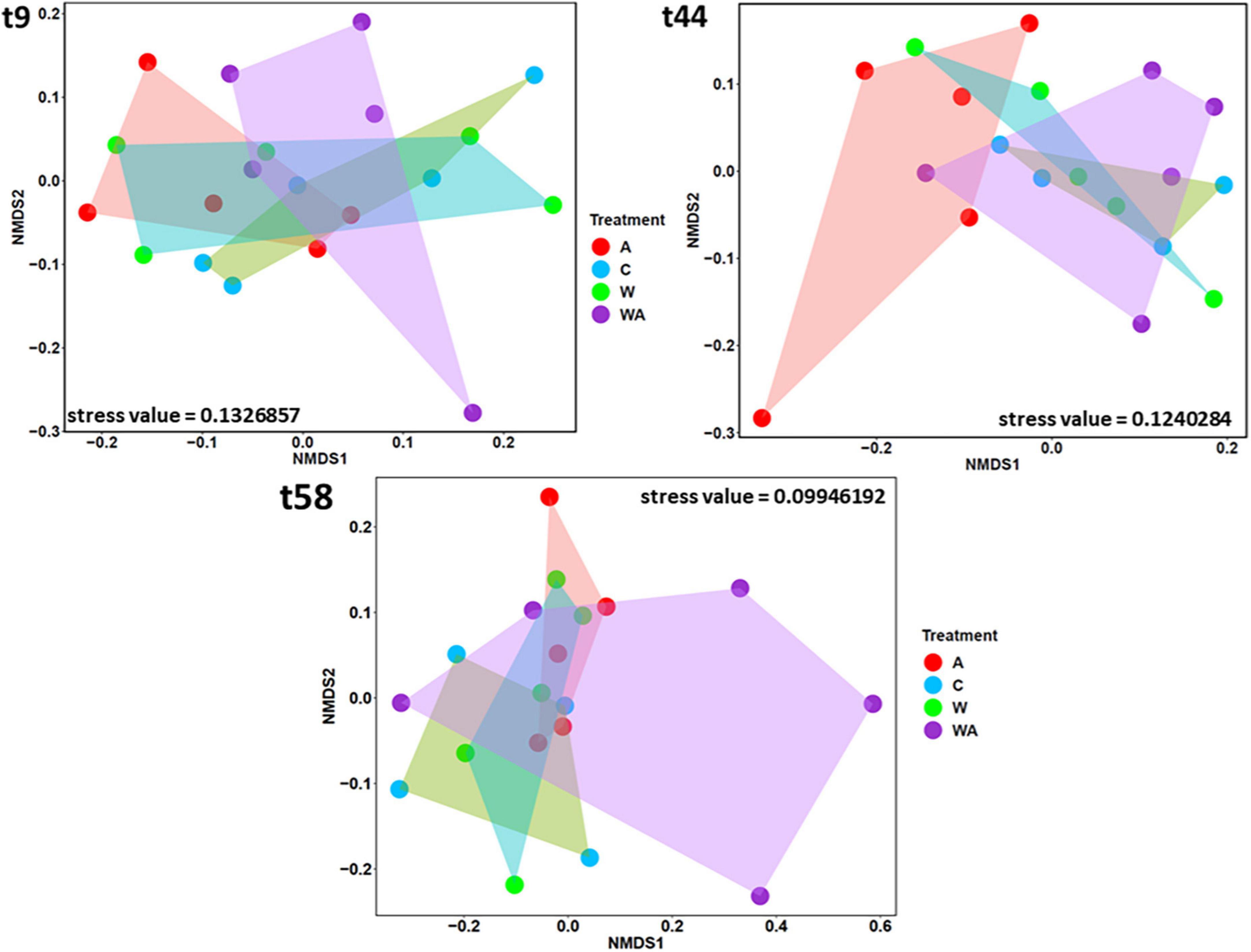
Figure 3. Comparison of bacterial communities according to the treatments. The Non-metric Multi-dimensional Scaling (NMDS), based on the bacterial 16S rRNA gene sequences, was performed to compare the bacterial communities according to the treatments [control (C), acidification (A), warming (W), and warming and acidification together (WA)] at the beginning (t9), the middle (t44), and the end (t58) of the experiment. The shapes correspond to the replicates (n = 5, except for control n = 4 at t44 and t58).
Indeed, LEfSe analyses on the 1,000 more abundant ASVs revealed bacterial taxa differentially abundant between the four treatments at t58. LEfSe revealed that the acidification was characterized by bacterial biomarkers, genera significantly more abundant in the acidification treatment, belonging to genera affiliated to Rhodobacteraceae and Sulfurimonas (Figure 4). The Sulfurimonas species are widespread in the environment and present large flexibility to colonize various habitats due to their versatile energy metabolisms and their adaptive abilities (Han and Perner, 2015). They play key roles in sulphur, hydrogen, nitrogen, oxygen, and carbon cycles (Han and Perner, 2015). The Sulfurimonas species are known to grow over a large range of pH (Inagaki, 2003; Takai et al., 2006) with the capacity to tolerate oxygen (Sievert et al., 2008), features allowing Sulfurimonas to outcompete under the conditions observed after acidification in our experiment. The Rhodobacteraceae play a key role in biogeochemical cycles and are often eukaryote mutualists (Simon et al., 2017). The abundance of Rhodobacteraceae has ever been shown to be affected under a high pCO2 (Hu et al., 2021). Desulfuromonas genus, sulphate reducer bacteria (SRB) obligate anaerobe and obligate sulphur reducer (Margulis and Chapman, 2009), was revealed as a bacterial biomarker in the warming treatment (Figure 4). SRB are known to exhibit versatile metabolism, an asset to adapt to the modification of environmental conditions in various ecosystems (Giloteaux et al., 2013), particularly in microbial mats (Fourçans et al., 2008). The WA treatment presented Guyparkeria, Ilumatobacter, and MAT-CR-H6-H10 as bacterial biomarkers (Figure 4). Guyparkeria genus includes autotrophic sulphur oxidizers (Nosalova et al., 2022). Ilumatobacter species are generally found in contaminated places by hydrocarbons (Peng et al., 2015; Papale et al., 2020). MAT-CR-H6-H10 was described in a hypersaline microbial mat (Kirk Harris et al., 2013). The results suggest that the different treatments simulating climate change impact bacteria playing a role in the sulfur cycle. The acidification treatments (A and WA) affected sulfur oxidizers while the warming treatment impacted sulphate reducers.
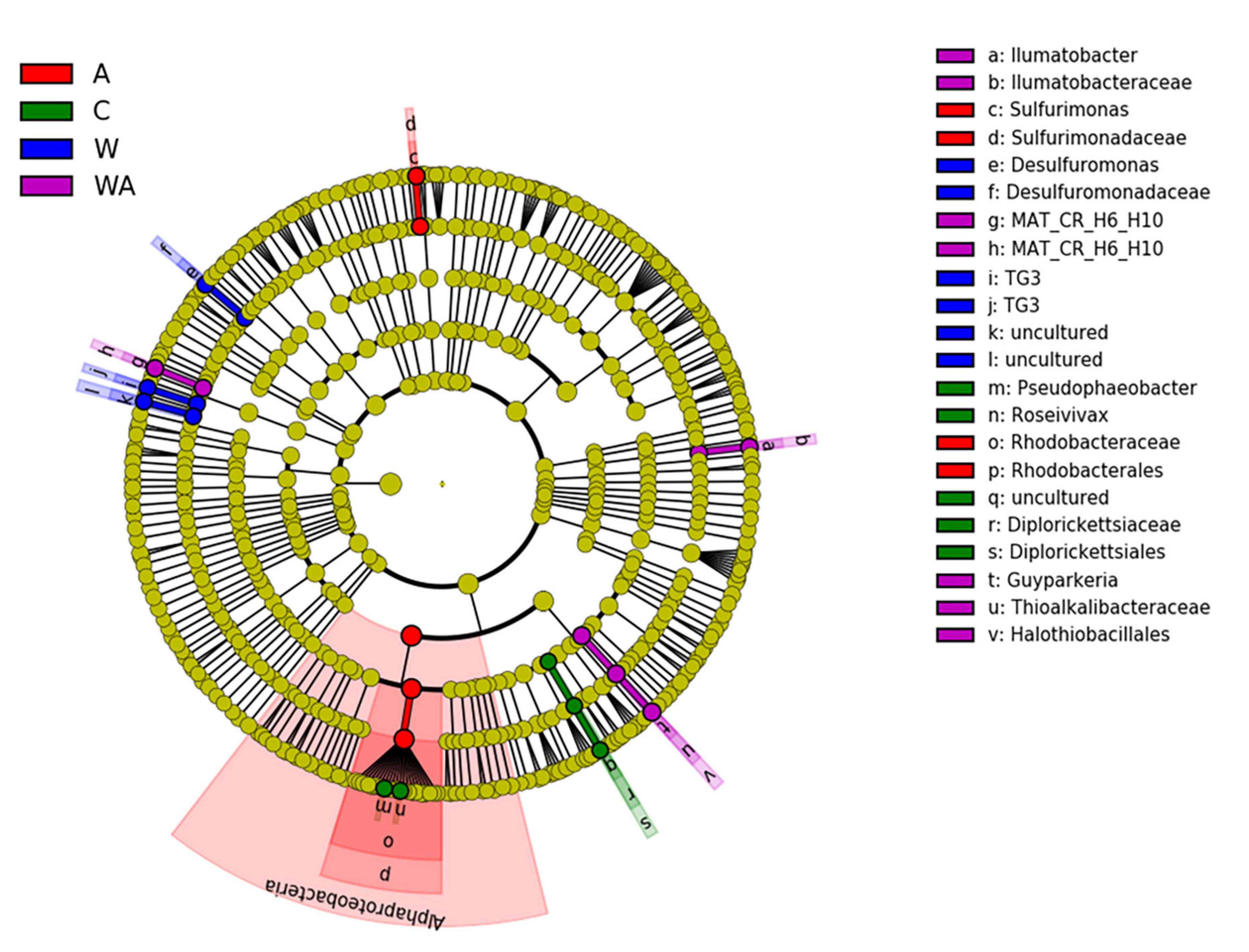
Figure 4. Linear discriminant analysis effect (LefSe). Comparison of bacterial communities at t58 in the different treatments [control (C), acidification (A), warming (W), and warming and acidification together (WA)] by linear discriminant analysis effect size (LEfSe). The analysis was performed with the 1,000 more abundant bacterial ASVs of each treatment.
Concomitantly, the increasing of the concentration of bound carbohydrate EPS in the acidification treatment was observed (Mazière et al., 2022) indicating that EPS are involved in the adaptation of communities to their environment as previously proposed (Dupraz and Visscher, 2005; Hubas, 2018; Prieto-Barajas et al., 2018). The most probable explanation is that the studied microbial mats are already naturally exposed to fluctuating environmental conditions (pH, light, temperature, salinity, etc.) with a great amplitude in salt marshes (Fourçans et al., 2004, 2006, 2008; Abed et al., 2007; Boujelben et al., 2012; Baumann et al., 2015; Mazière et al., 2021). Therefore, they are already confronted to temperature such those predicted by the IPCC for 2100. Changes in the bacterial metabolism probably enabled bacteria to maintain a high activity potential when exposed to the conditions of the simulated scenarios. Noteworthy, a change in the pigment composition of the microbial mats submitted to acidification was observed (Mazière et al., 2022), that suggested a shift in the photosynthetic capacities corresponding to a modification in the photosynthetic communities or their metabolism (Mazière et al., 2022). The microbial mats maintained in mesocosms allowed us to observe the effect of the acidification alone on photosynthetic microorganisms, which was not observable in the treatment combining both acidification and warming.
To summarize, the acidification treatment affected the photosynthetic communities by changing the pigment proportion of the microbial mat, which probably resulted from a change in either their composition or their metabolisms (Figure 5). Concomitantly, an increase of dissolved oxygen concentration was observed in the water, likely due to an increase of the photosynthesis (Figure 5). The modifications of the conditions generated by the acidification impacted also the sulfur-oxidizers belonging to the Sulfurimonas genus and the Rhodobacteraceae family, which were significantly more abundant in this condition (Figure 4). In contrast, the warming of the water, characterized by an upsurge of salinity due to evaporation (Figure 5), increased the relative abundance of the sulphate-reducers Desulfurimonas (Figure 4). Finally, the warming and acidified treatment resulted in an increase of the water salinity but the dissolved oxygen concentration was not impacted (Figure 5), affecting the sulfur-oxidizers Guyparkeria (Figure 4).
Maintaining microbial mats in mesocosms following an experimental ecology approach allowed to apply conditions to simulate climate change, which is difficult to perform in situ. It also made possible to increase the number of replicates and analysis strengthening the statistical analyses. We were thus able to unveil the relationships between the microorganisms inhabiting the microbial mat and their environment, helpful information to refine the hypotheses of the impact of climate change at the ecosystem level. More generally, the gained information provides new insights to better understand the importance of microbial mats in their environment.
4. Conclusion and perspectives
Climate change is already happening, but it is difficult to determine its effect on complex microbial assemblages, particularly because in situ simulation experiments are difficult to perform. An alternative to overcome this difficulty is to follow an experimental ecology approach maintaining complex microbial community in mesocosm applying conditions simulating predicted climate change conditions. Microbial mats used allowed to unveil microbial structural modification in response to climate change. The mesocosm approach mimics in situ conditions excluding environmental complexity, which was useful to characterize the impact of each changed parameter independently. The total cost of the mesocosms set up was less than 3.000$. The described method in this paper allowed to simulate the change of two parameters, the water pH and temperature, alone and associated. The regular monitoring of several abiotic and biotic parameters and the presence of five replicates led to the complete description of the ecosystem. After applying climate change scenario, several physical-chemical and biological modifications were observed, which provide new insights on microbial mats behaviour. The gained information is useful to understand the impact of climate change at the ecosystem level. Microbial mats, easy to handle and to maintain in mesocosm, are thus precious complex microbial community model to investigate ecological issues of concern for coastal marine ecosystems. Mesocosms experiment can mimic as close as possible the environment but it can’t reproduce all in situ variations and stresses. The method presented could therefore be improved by adding rainfall cycles or seasonal variations. Additional analyses could be performed to specify the functional role of microbial mats in its environment, such as vertical microprofiling of oxygen and sulphur, or a day/night monitoring. This method could be adapted with additional climate change parameters as for example, deoxygenation or the alternance of drought and extreme rainfall. Other issues could also be investigated with this system, such as the impact of oil pollution, metals contamination, organic enrichment, etc. The presented method could also be extended on sediments coming from aquatic environments, as for example estuaries, beach, deep ocean, but also rivers, lakes, by adjusting the sampling method and the parameters applied.
Data availability statement
The raw data supporting the conclusions of this article will be made available by the authors, without undue reservation.
Author contributions
CM: formal analysis, methodology, and validation—original draft. RD, CD, and CC-L: funding acquisition, project administration, resources, and supervision. All authors: conceptualization, writing—review and editing, contributed to the article, and approved the submitted version.
Funding
CM was supported by a Ph.D. grant from E2S-UPPA program and the Région Nouvelle-Aquitaine. We thank the funding support from the European programme ERANETMED AQUASALT (NMED-0003-01) and from the ACI politique d’établissement Université de La Rochelle.
Acknowledgments
The authors are grateful to the salterns owner Michel Jauffrais and all the people who participated in this work. The content of this manuscript is a part of the CM’s thesis.
Conflict of interest
The authors declare that the research was conducted in the absence of any commercial or financial relationships that could be construed as a potential conflict of interest.
Publisher’s note
All claims expressed in this article are solely those of the authors and do not necessarily represent those of their affiliated organizations, or those of the publisher, the editors and the reviewers. Any product that may be evaluated in this article, or claim that may be made by its manufacturer, is not guaranteed or endorsed by the publisher.
Supplementary material
The Supplementary Material for this article can be found online at: https://www.frontiersin.org/articles/10.3389/fmicb.2023.1039658/full#supplementary-material
References
Abed, R. M. M., Kohls, K., and de Beer, D. (2007). Effect of salinity changes on the bacterial diversity, photosynthesis and oxygen consumption of cyanobacterial mats from an intertidal flat of the Arabian Gulf. Environ. Microbiol. 9, 1384–1392. doi: 10.1111/j.1462-2920.2007.01254.x
Agogué, H., Mallet, C., Orvain, F., De Crignis, M., Mornet, F., and Dupuy, C. (2014). Bacterial dynamics in a microphytobenthic biofilm: a tidal mesocosm approach. J. Sea Res. 92, 36–45.
Aminot, A., and Kérouel, R. (2007). Dosage automatique des nutriments dans les eaux marines: Méthodes d’analyse en milieu marin. Méthodes en flux continu. Paris: QUAE.
Azam, F., and Malfatti, F. (2007). Microbial structuring of marine ecosystems. Nat. Rev. Microbiol. 5, 782–791.
Baragi, L. V., and Anil, A. C. (2016). Synergistic effect of elevated temperature, pCO2 and nutrients on marine biofilm. Mar. Pollut. Bull. 105, 102–109. doi: 10.1016/j.marpolbul.2016.02.049
Baragi, L. V., Khandeparker, L., and Anil, A. C. (2015). Influence of elevated temperature and pCO2 on the marine periphytic diatom Navicula distans and its associated organisms in culture. Hydrobiologia 762, 127–142.
Baumann, H., Wallace, R. B., Tagliaferri, T., and Gobler, C. J. (2015). Large natural pH, CO2 and O2 fluctuations in a temperate tidal salt marsh on diel, seasonal, and interannual time scales. Estuaries Coasts 38, 220–231.
Beman, J. M., Chow, C.-E., King, A. L., Feng, Y., Fuhrman, J. A., Andersson, A., et al. (2011). Global declines in oceanic nitrification rates as a consequence of ocean acidification. Proc. Natl. Acad. Sci. 108, 208–213. doi: 10.1073/pnas.1011053108
Black, J. G., Stark, J. S., Johnstone, G. J., McMinn, A., Boyd, P., McKinlay, J., et al. (2019). In-situ behavioural and physiological responses of Antarctic microphytobenthos to ocean acidification. Sci. Rep. 9:1890. doi: 10.1038/s41598-018-36233-2
Bolhuis, H., Silvia, M., and Stal, L. J. (2014). Molecular ecology of microbial mats. FEMS Microbiol. Ecol. 90, 335–350.
Bordenave, S., Fourçans, A., Blanchard, S., Goñi-Urriza, M. S., Caumette, P., and Duran, R. (2004a). Structure and functional analyses of bacterial communities changes in microbial mats following petroleum exposure. Ophelia 58, 195–203.
Bordenave, S., Goñi-Urriza, M. S., Caumette, P., and Duran, R. (2007). Effects of heavy fuel oil on the bacterial community structure of a pristine microbial mat. Appl. Environ. Microbiol. 73, 6089–6097. doi: 10.1128/AEM.01352-07
Bordenave, S., Goñi-Urriza, M. S., Vilette, C., Blanchard, S., Caumette, P., and Duran, R. (2008). Diversity of ring-hydroxylating dioxygenases in pristine and oil contaminated microbial mats at genomic and transcriptomic levels. Environ. Microbiol. 10, 3201–3211. doi: 10.1111/j.1462-2920.2008.01707.x
Bordenave, S., Jézéquel, R., Fourçans, A., Budzinski, H., Merlin, F. X., Fourel, T., et al. (2004b). Degradation of the “Erika” oil. Aquat. Living Resour. 17, 261–267.
Boujelben, I., Gomariz, M., Martínez-García, M., Santos, F., Peña, A., López, C., et al. (2012). Spatial and seasonal prokaryotic community dynamics in ponds of increasing salinity of Sfax solar saltern in Tunisia. Antonie Van Leeuwenhoek 101, 845–857. doi: 10.1007/s10482-012-9701-7
Chronopoulou, P.-M., Fahy, A., Coulon, F., Païssé, S., Goñi-Urriza, M. S., Peperzak, L., et al. (2013). Impact of a simulated oil spill on benthic phototrophs and nitrogen-fixing bacteria in mudflat mesocosms. Environ. Microbiol. 15, 242–252. doi: 10.1111/j.1462-2920.2012.02864.x
Cravo-Laureau, C., and Duran, R. (2014). Marine coastal sediments microbial hydrocarbon degradation processes: contribution of experimental ecology in the omics’ era. Front. Microbiol. 5:39. doi: 10.3389/fmicb.2014.00039
Crawfurd, K. J., Raven, J. A., Wheeler, G. L., Baxter, E. J., and Joint, I. (2011). The response of thalassiosira pseudonana to long-term exposure to increased CO2 and decreased pH. PLoS One 6:e26695. doi: 10.1371/journal.pone.0026695
Danovaro, R., Corinaldesi, C., Dell’Anno, A., and Rastelli, E. (2017). Potential impact of global climate change on benthic deep-sea microbes. FEMS Microbiol. Lett. 364:fnx214.
Dimitriou, P. D., Papageorgiou, N., Geropoulos, A., Kalogeropoulou, V., Moraitis, M., Santi, I., et al. (2017). A novel mesocosm setup for benthic-pelagic coupling experiments. Limnol. Oceanogr. Methods 15, 349–362.
Dupraz, C., and Visscher, P. T. (2005). Microbial lithification in marine stromatolites and hypersaline mats. Trends Microbiol. 13, 429–438. doi: 10.1016/j.tim.2005.07.008
Flemming, H.-C., and Wuertz, S. (2019). Bacteria and archaea on earth and their abundance in biofilms. Nat. Rev. Microbiol. 17, 247–260.
Fourçans, A., Oteyza, T. G., Wieland, A., Solé, A., Diestra, E., Bleijswijk, J., et al. (2004). Characterization of functional bacterial groups in a hypersaline microbial mat community (Salins-de-Giraud, Camargue, France). FEMS Microbiol. Ecol. 51, 55–70. doi: 10.1016/j.femsec.2004.07.012
Fourçans, A., Ranchou-Peyruse, A., Caumette, P., and Duran, R. (2008). Molecular analysis of the spatio-temporal distribution of sulfate-reducing bacteria (SRB) in camargue (France) hypersaline microbial mat. Microb. Ecol. 56, 90–100. doi: 10.1007/s00248-007-9327-x
Fourçans, A., Solé, A., Diestra, E., Ranchou-Peyruse, A., Esteve, I., Caumette, P., et al. (2006). Vertical migration of phototrophic bacterial populations in a hypersaline microbial mat from Salins-de-Giraud (Camargue, France). FEMS Microbiol. Ecol. 57, 367–377. doi: 10.1111/j.1574-6941.2006.00124.x
Garet, M. J., and Moriarty, D. J. W. (1996). Acid extraction of tritium label from bacterial DNA in clay sediment. J. Microbiol. Methods 25, 1–4. doi: 10.1007/BF02097401
Gette-Bouvarot, M., Mermillod-Blondin, F., Lemoine, D., Delolme, C., Danjean, M., Etienne, L., et al. (2015). The potential control of benthic biofilm growth by macrophytes—a mesocosm approach. Ecol. Eng. 75, 178–186.
Giloteaux, L., Duran, R., Casiot, C., Bruneel, O., Elbaz-Poulichet, F., Goñi-Urriza, M. S., et al. (2013). Three-year survey of sulfate-reducing bacteria community structure in Carnoulès acid mine drainage (France), highly contaminated by arsenic. FEMS Microbiol. Ecol. 83, 724–737. doi: 10.1111/1574-6941.12028
Gingold, R., Moens, T., and Rocha-Olivares, A. (2013). Assessing the response of nematode communities to climate change-driven warming: a microcosm experiment. PLoS One 8:e66653. doi: 10.1371/journal.pone.0066653
Giordano, M., Beardall, J., and Raven, J. A. (2005). CO2 concentrating mechanisms in algae: mechanisms, environmental modulation, and evolution. Annu. Rev. Plant Biol. 56, 99–131. doi: 10.1002/jez.2367
Guanyong, O., Wang, H., Ranran, S., and Guan, W. (2017). The dinoflagellate Akashiwo sanguinea will benefit from future climate change: the interactive effects of ocean acidification, warming and high irradiance on photophysiology and hemolytic activity. Harmful Algae 68, 118–127. doi: 10.1016/j.hal.2017.08.003
Han, Y., and Perner, M. (2015). The globally widespread genus Sulfurimonas: versatile energy metabolisms and adaptations to redox clines. Front. Microbiol. 6:989. doi: 10.3389/fmicb.2015.00989
Hu, C., Li, X., He, M., Jiang, P., Long, A., and Xu, J. (2021). Effect of ocean acidification on bacterial metabolic activity and community composition in oligotrophic oceans, inferred from short-term bioassays. Front. Microbiol. 12:583982. doi: 10.3389/fmicb.2021.583982
Hubas, C. (2018). Biofilms, Tapis et Agrégats Microbiens: vers Une Vision Unificatrice (HDR (Habilitation à Diriger les Recherches)). Muséum National D’Histoire Naturelle. Available online at: https://doi.org/10.5281/zenodo.3784703 (accessed September 8, 2022).
Hutchins, D. A., and Fu, F. (2017). Microorganisms and ocean global change. Nat. Microbiol. 2:17058.
Inagaki, F. (2003). Sulfurimonas autotrophica gen. nov., sp. nov., a novel sulfur-oxidizing -proteobacterium isolated from hydrothermal sediments in the mid-okinawa trough. Int. J. Syst. Evol. Microbiol. 53, 1801–1805. doi: 10.1099/ijs.0.02682-0
IPCC (2021). “Climate change 2021: the physical science basis. contribution of working group I to the sixth assessment report of the intergovernmental panel on climate change. summary for policymakers,” in Sixth Assessment Report of the Intergovernmental Panel on Climate Change, eds V. Masson-Delmotte, P. Zhai, and A. Pirani (Geneva: IPCC).
Kirk Harris, J., Gregory Caporaso, J., Walker, J. J., Spear, J. R., Gold, N. J., Robertson, C. E., et al. (2013). Phylogenetic stratigraphy in the Guerrero Negro hypersaline microbial mat. ISME J. 7, 50–60. doi: 10.1038/ismej.2012.79
Kitidis, V., Laverock, B., McNeill, L. C., Beesley, A., Cummings, D., Tait, K., et al. (2011). Impact of ocean acidification on benthic and water column ammonia oxidation. Geophys. Res. Lett. 38:L21603.
Lavergne, C., Beaugeard, L., Dupuy, C., Courties, C., and Agogué, H. (2014). An efficient and rapid method for the enumeration of heterotrophic prokaryotes in coastal sediments by flow cytometry. J. Microbiol. Methods 105, 31–38. doi: 10.1016/j.mimet.2014.07.002
Lee, M. R., Torres, R., and Manríquez, P. H. (2017). The combined effects of ocean warming and acidification on shallow-water meiofaunal assemblages. Mar. Environ. Res. 131, 1–9. doi: 10.1016/j.marenvres.2017.09.002
Li, W., Xu, X., Fujibayashi, M., Niu, Q., Tanaka, N., and Nishimura, O. (2016). Response of microalgae to elevated CO2 and temperature: impact of climate change on freshwater ecosystems. Environ. Sci. Pollut. Res. 23, 19847–19860. doi: 10.1007/s11356-016-7180-5
Liu, Z., Lozupone, C., Hamady, M., Bushman, F. D., and Knight, R. (2007). Short pyrosequencing reads suffice for accurate microbial community analysis. Nucleic Acids Res. 35:e120. doi: 10.1093/nar/gkm541
Ma, J., Wang, P., Wang, X., Xu, Y., and Paerl, H. W. (2019). Cyanobacteria in eutrophic waters benefit from rising atmospheric CO2 concentrations. Sci. Total Environ. 691, 1144–1154.
Margulis, L., and Chapman, M. J. (2009). “Chapter one - kingdom prokaryotae (Bacteria, Monera, Prokarya),” in Kingdoms and Domains, 4th Edn, eds L. Margulis and M. J. Chapman (London: Academic Press).
Mazière, C. (2021). Exploration and Study of the Impact of Climate Change on Microbial Mats in the Nouvelle-Aquitaine Region. France: Université de Pau et des Pays de l’Adour.
Mazière, C., Agogué, H., Cravo-Laureau, C., Cagnon, C., Lanneluc, I., Sablé, S., et al. (2021). New insights in bacterial and eukaryotic diversity of microbial mats inhabiting exploited and abandoned salterns at the Ré Island (France). Microbiol. Res. 252:126854. doi: 10.1016/j.micres.2021.126854
Mazière, C., Bodo, M., Perdrau, M. A., Cravo-Laureau, C., Duran, R., Dupuy, C., et al. (2022). Climate change influences chlorophylls and bacteriochlorophylls metabolism in hypersaline microbial mat. Sci. Total Environ. 802:149787. doi: 10.1016/j.scitotenv.2021.149787
Middelburg, J. J., Soetaert, K., and Hagens, M. (2020). Ocean alkalinity, buffering and biogeochemical processes. Rev. Geophys. 58:e2019RG000681.
Noël, C., Quintric, L., Cormier, A., Leroi, L., and Durand, P. (2021). SAMBA: Standardized and Automated MetaBarcoding Analyses workflow. Available online at: https://doi.org/10.48546/WORKFLOWHUB.WORKFLOW.156.1 (accessed September 8, 2022).
Nosalova, L., Piknova, M., Bonova, K., and Pristas, P. (2022). Deep subsurface hypersaline environment as a source of novel species of halophilic sulfur-oxidizing bacteria. Microorganisms 10:995. doi: 10.3390/microorganisms10050995
O’Brien, P. A., Morrow, K. M., Willis, B. L., and Bourne, D. G. (2016). Implications of ocean acidification for marine microorganisms from the free-living to the host-associated. Front. Mar. Sci. 3:14. doi: 10.3389/fmars.2016.00047
Orr, J. C., Fabry, V. J., Aumont, O., Bopp, L., Doney, S. C., and Feely, R. A. (2005). Anthropogenic ocean acidification over the twenty-first century and its impact on calcifying organisms. Nature 437, 681–686. doi: 10.1038/nature04095
Pachauri, R. K. (2014). “Climate change 2014: synthesis report. contribution of working groups I, II and III to the fifth assessment report of the intergovernmental panel on climate change,” in Climate Change 2014: Synthesis Report, eds The Core Writing Team, R. K. Pachauri, and L. Meyer (Geneva: IPCC).
Pajares, S., Bonilla-Rosso, G., Travisano, M., Eguiarte, L. E., and Souza, V. (2012). Mesocosms of aquatic bacterial communities from the cuatro cienegas basin (Mexico): a tool to test bacterial community response to environmental stress. Microb. Ecol. 64, 346–358. doi: 10.1007/s00248-012-0045-7
Pajares, S., Souza, V., and Eguiarte, L. E. (2015). Multivariate and phylogenetic analyses assessing the response of bacterial mat communities from an ancient oligotrophic aquatic ecosystem to different scenarios of long-term environmental disturbance. PLoS One 10:e0119741. doi: 10.1371/journal.pone.0119741
Papale, M., Rappazzo, A., Mikkonen, A., Rizzo, C., Moscheo, F., Conte, A., et al. (2020). Bacterial diversity in a dynamic and extreme sub-arctic watercourse (pasvik river, norwegian arctic). Water 12:3098.
Pascal, P.-Y., Dupuy, C., Richard, P., Mallet, C., telet, E. A., du, C., et al. (2009). Seasonal variation in consumption of benthic bacteria by meio- and macrofauna in an intertidal mudflat. Limnol. Oceanogr. 54, 1048–1059.
Peng, M., Zi, X., and Wang, Q. (2015). Bacterial community diversity of oil-contaminated soils assessed by high throughput sequencing of 16S rRNA genes. Int. J. Environ. Res. Public. Health 12, 12002–12015. doi: 10.3390/ijerph121012002
Petersen, J., Kennedy, V. S., Dennison, W., and Kemp, W. M. (2009). Enclosed Experimental Ecosystems and Scale: Tools For Understanding and Managing Coastal Ecosystems. Berlin: Springer.
Prieto-Barajas, C. M., Valencia-Cantero, E., and Santoyo, G. (2018). Microbial mat ecosystems: structure types, functional diversity, and biotechnological application. Electron. J. Biotechnol. 31, 48–56.
Quast, C., Pruesse, E., Yilmaz, P., Gerken, J., Schweer, T., Yarza, P., et al. (2012). The SILVA ribosomal RNA gene database project: improved data processing and web-based tools. Nucleic Acids Res. 41, D590–D596. doi: 10.1093/nar/gks1219
R Core Team (2020). R: A language and environment for statistical computing. Vienna: R Foundation for Statistical Computing. Available online at: https://www.R-project.org/
Schmidtko, S., Stramma, L., and Visbeck, M. (2017). Decline in global oceanic oxygen content during the past five decades. Nature 542, 335–339. doi: 10.1038/nature21399
Segata, N., Izard, J., Waldron, L., Gevers, D., Miropolsky, L., Garrett, W. S., et al. (2011). Metagenomic biomarker discovery and explanation. Genome Biol. 12:R60.
Sievert, S. M., Scott, K. M., Klotz, M. G., Chain, P. S. G., Hauser, L. J., and Hemp, J. (2008). Genome of the epsilonproteobacterial chemolithoautotroph sulfurimonas denitrificans. Appl. Environ. Microbiol. 74, 1145–1156. doi: 10.1128/AEM.01844-07
Simon, M., Scheuner, C., Meier-Kolthoff, J. P., Brinkhoff, T., Wagner-Döbler, I., Ulbrich, M., et al. (2017). Phylogenomics of Rhodobacteraceae reveals evolutionary adaptation to marine and non-marine habitats. ISME J. 11, 1483–1499. doi: 10.1038/ismej.2016.198
Stauffert, M., Cravo-Laureau, C., Jezequel, R., Barantal, S., Cuny, P., Gilbert, F., et al. (2013). Impact of oil on bacterial community structure in bioturbated sediments. PLoS One 8:e65347.
Stewart, R. I. A., Dossena, M., Bohan, D. A., Jeppesen, E., Kordas, R. L., and Ledger, M. E. (2013). “Chapter two - mesocosm experiments as a tool for ecological climate-change research,” in Advances in Ecological Research, Global Change in Multispecies Systems: Part 3, eds G. Woodward and E. J. O’Gorman (Cambridge, MA: Academic Press).
Takai, K., Suzuki, M., Nakagawa, S., Miyazaki, M., Suzuki, Y., Inagaki, F., et al. (2006). Sulfurimonas paralvinellae sp. nov., a novel mesophilic, hydrogen- and sulfur-oxidizing chemolithoautotroph within the Epsilonproteobacteria isolated from a deep-sea hydrothermal vent polychaete nest, reclassification of Thiomicrospira denitrificans as Sulfurimonas denitrificans comb. nov. and emended description of the genus Sulfurimonas. Int. J. Syst. Evol. Microbiol. 56, 1725–1733. doi: 10.1099/ijs.0.64255-0
Tan, Y.-H., Lim, P.-E., Beardall, J., Poong, S.-W., and Phang, S.-M. (2019). A metabolomic approach to investigate effects of ocean acidification on a polar microalga Chlorella sp. Aquat. Toxicol. 217:105349. doi: 10.1016/j.aquatox.2019.105349
Verleyen, E., Sabbe, K., Hodgson, D. A., Grubisic, S., Taton, A., Cousin, S., et al. (2010). Structuring effects of climate-related environmental factors on Antarctic microbial mat communities. Aquat. Microb. Ecol. 59, 11–24.
Widdicombe, S., Dashfield, S. L., McNeill, C. L., Needham, H. R., Beesley, A., McEvoy, A., et al. (2009). Effects of CO2 induced seawater acidification on infaunal diversity and sediment nutrient fluxes. Mar. Ecol. Prog. Ser. 379, 59–75.
Yilmaz, P., Wegener Parfrey, L., Yarza, P., Gerken, J., Pruesse, E., Quast, C., et al. (2013). The SILVA and “all-species living tree project (LTP)” taxonomic frameworks. Nucleic Acids Res. 42, D643–D648. doi: 10.1093/nar/gkt1209
Keywords: global change, ocean warming, ocean acidification, microbial mat, experimental ecology, mesocosms
Citation: Mazière C, Duran R, Dupuy C and Cravo-Laureau C (2023) Microbial mats as model to decipher climate change effect on microbial communities through a mesocosm study. Front. Microbiol. 14:1039658. doi: 10.3389/fmicb.2023.1039658
Received: 08 September 2022; Accepted: 30 May 2023;
Published: 15 June 2023.
Edited by:
Tony Gutierrez, Heriot-Watt University, United KingdomReviewed by:
Andrew Decker Steen, The University of Tennessee, Knoxville, United StatesPanagiotis D. Dimitriou, University of Crete, Greece
Copyright © 2023 Mazière, Duran, Dupuy and Cravo-Laureau. This is an open-access article distributed under the terms of the Creative Commons Attribution License (CC BY). The use, distribution or reproduction in other forums is permitted, provided the original author(s) and the copyright owner(s) are credited and that the original publication in this journal is cited, in accordance with accepted academic practice. No use, distribution or reproduction is permitted which does not comply with these terms.
*Correspondence: C. Mazière, Y2FtaWxsZS5tYXppZXJlQHVuaXYtcGF1LmZy; C. Cravo-Laureau, Y3Jpc3RpYW5hLmNyYXZvLWxhdXJlYXVAdW5pdi1wYXUuZnI=