- 1Centre of Microbial and Plant Genetics, KU Leuven, Leuven, Belgium
- 2Department of Biosystems, Laboratory of Gene Technology, KU Leuven, Leuven, Belgium
- 3Institute of Biology, Leiden University, Leiden, Netherlands
- 4Laboratoire de Biodiversité et Biotechnologie Microbiennes, Sorbonne Université, CNRS, Observatoire Océanologique, Banyuls-sur-Mer, France
Recent changes in the taxonomy of the Pseudomonadaceae family have led to the delineation of three new genera (Atopomonas, Halopseudomonas and Stutzerimonas). However, the genus Pseudomonas remains the most densely populated and displays a broad genetic diversity. Pseudomonas are able to produce a wide variety of secondary metabolites which drives important ecological functions and have a great impact in sustaining their lifestyles. While soilborne Pseudomonas are constantly examined, we currently lack studies aiming to explore the genetic diversity and metabolic potential of marine Pseudomonas spp. In this study, 23 Pseudomonas strains were co-isolated with Vibrio strains from three marine microalgal cultures and rpoD-based phylogeny allowed their assignment to the Pseudomonas oleovorans group (Pseudomonas chengduensis, Pseudomonas toyotomiensis and one new species). We combined whole genome sequencing on three selected strains with an inventory of marine Pseudomonas genomes to assess their phylogenetic assignations and explore their metabolic potential. Our results revealed that most strains are incorrectly assigned at the species level and half of them do not belong to the genus Pseudomonas but instead to the genera Halopseudomonas or Stutzerimonas. We highlight the presence of 26 new species (Halopseudomonas (n = 5), Stutzerimonas (n = 7) and Pseudomonas (n = 14)) and describe one new species, Pseudomonas chaetocerotis sp. nov. (type strain 536T = LMG 31766T = DSM 111343T). We used genome mining to identify numerous BGCs coding for the production of diverse known metabolites (i.e., osmoprotectants, photoprotectants, quorum sensing molecules, siderophores, cyclic lipopeptides) but also unknown metabolites (e.g., ARE, hybrid ARE-DAR, siderophores, orphan NRPS gene clusters) awaiting chemical characterization. Finally, this study underlines that marine environments host a huge diversity of Pseudomonadaceae that can drive the discovery of new secondary metabolites.
Introduction
Pseudomonas spp. are ubiquitous bacteria able to colonize a wide range of environments such as soil, stream and ground waters or associated to plants (Ramos, 2004). Their occurrence in soil or associated to diverse plant crops is constantly examined and their implication in fundamental ecological processes is well established (Wasi et al., 2013; Dignam et al., 2019; Yadav et al., 2021). So far, Pseudomonas spp. have been randomly isolated from seawater, sediments or associated to higher organisms, demonstrating that they naturally occur in marine environments and occasionally represent a large majority of the studied bacterial communities (Amer et al., 2015; Jiang et al., 2015; Viggor et al., 2015; Berner et al., 2018). Several Pseudomonas species are also fish pathogens and have detrimental impacts on aquaculture around the world (e.g., P. plecoglossicida, P. baetica, P. stutzeri, P. anguilliseptica or P. alcaligenes) (Lopez et al., 2012; Xu et al., 2015; Wiklund, 2016; Beaton et al., 2018). However, most of the studies are using 16S rRNA gene sequences to perform taxonomic affiliations and this gene is not sufficiently discriminant to differentiate Pseudomonas strains at the species level, which results in misidentifications and underestimations of diversity (Amer et al., 2015; Jiang et al., 2015; Viggor et al., 2015; Berner et al., 2018).
The genus Pseudomonas, originally made of several groups and subgroups (Lalucat et al., 2020; Girard et al., 2021), was recently divided into four and the Pseudomonadaceae family now contains three supplementary genera (Atopomonas, Halopseudomonas and Stutzerimonas) (Rudra and Gupta, 2021; Lalucat et al., 2022). The genera Halopseudomonas and Stutzerimonas are corresponding to the former P. pertucinogena and P. stutzeri groups, while the genus Aptomonas only includes one species, A. hussainii (previously P. hussaini). Consequently, the genus Pseudomonas is now made of 14 groups (P. aeruginosa, P. anguilliseptica, P. fluorescens, P. linyingensis, P. lutea, P. massiliensis, P. oleovorans, P. oryzihabitans, P. pohangensis, P. putida, P. resinovorans, P. rhizosphaerae, P. straminea and P. syringae) and several orphan groups (Girard et al., 2021).
In order to thrive in an extremely wide range of environments Pseudomonas spp. display a great metabolic diversity and their metabolite production appears to be an important strategy in sustaining their lifestyles (Kraemer, 2004; Götze and Stallforth, 2019; Girard et al., 2020a). Indeed, these molecules are often involved in important ecological functions (e.g., iron-scavenging, swarming motility, biofilm formation, pathogenicity, cooperation or antagonism) and a large majority have anti-microbial properties (anti-bacterial, anti-fungal or anti-viral) (Gross and Loper, 2009). Pseudomonas spp. have the ability to assemble intriguing compounds and their metabolic potential his supported by the prevalence, in their genomes, of very diverse biosynthetic systems [e.g., non-ribosomal peptide synthetases (NRPSs), polyketides synthases (PKSs, hybrid systems) but also by the presence of many orphan Biosynthetic Gene Clusters (BGCs)] encoding for the production of unknown metabolites (Gross and Loper, 2009; Girard et al., 2020a). Over the last decade, the expansion of affordable sequencing technologies and advances in bioinformatics has led to the genome mining (or genome-guided) discovery of a wide diversity of compounds (de Bruijn et al., 2007) and marine bacteria were shown to produce novel secondary metabolites with unique chemical structure leading the development of new drugs (Petersen et al., 2020). Marine Pseudomonas were recently pinpointed as a prolific source for molecules of biotechnological interest (Carroll et al., 2019; Bollinger et al., 2020), however, studies linking accurate taxonomic affiliation and metabolic potential of strains are still missing.
In this study, we used the rpoD gene to taxonomically assign twenty-three Pseudomonas strains co-isolated with Vibrio strains from three marine microalgal cultures: Chaetoceros calcitrans, Chaetoceros gracilis and Isochrysis galbana affinis Tahiti. We performed whole genome sequencing on three selected strains and inventoried all marine Pseudomonas isolates with available genomic sequences. We then used whole genome analyses (Average Nucleotide Identity (ANIb), digital DNA–DNA hybridization (dDDH)) to taxonomically re-assign 77 marine strains previously identified as Pseudomonas sp., P. fluorescens or P. putida. Based on a polyphasic approach, we show that two isolates, 536 and 293, represent a novel species within the P. oleovorans group, Pseudomonas chaetocerotis sp. nov. (type strain 536T = LMG 31766T = DSM 111343T). Ultimately, we used a combination of online tools and phylogenetic analyses to explore the metabolic potential of marine Pseudomonas and give an overview of the nature and diversity of their BGCs.
Materials and methods
Isolation and culture conditions
The diversity of Vibrio species associated to three marine microalgal species, Chaetoceros calcitrans, Chaetoceros gracilis and Isochrysis galbana affinis Tahiti, was examined between February and June 2018. Microalgal cultures were directly plated on Thiosulfate-Citrate-Bile salts-Sucrose (TCBS) and incubated at 25°C for 48 h. Vibrio typically grow on TCBS as large green or yellow colonies. Pseudomonas strains appear as small green colonies on TCBS and as small cream colonies on marine agar (MA). A total of 125 colonies were picked randomly and isolated on MA plates. After isolation, strains were identified based on the gyrB gene, suitable for taxonomic identification of Vibrio isolates [data not shown; (Girard et al., 2018)], and revealed the presence of 23 Pseudomonas isolates. Therefore, the taxonomic affiliations of Pseudomonas isolates were assessed using the rpoD gene (Girard et al., 2020b).
rpoD sequencing
PCR reactions were performed as previously described in Girard et al. (2020a). The cell lysates of the 23 Pseudomonas strains were used as PCR templates. PCR amplifications were performed using the primers PsEG30F and PsEG790R (Mulet et al., 2009), and KAPA2G Fast HotStart ReadyMix (Sigma–Aldrich, Saint-Louis, Missouri, United States). Cycling conditions were as follows: initial denaturation at 95°C for 5 min followed by 30 cycles of annealing at 60°C for 30 s, extension at 72°C for 30 s and denaturation at 95°C for 15 s, and reactions were completed at 72°C for 2 min. PCR products were purified using the GenElute PCR Clean-Up kit (Sigma–Aldrich Saint-Louis, Missouri, United States). Purified PCR products were then sequenced using the same set of primers (PsEG30F and PsEG790R) by Sanger sequencing (Macrogen Europe, Amsterdam, The Netherlands) to obtain a final fragment of approximately 650 bp.
Phenotypic, biochemical and chemotaxonomic characterization
Pseudomonas strain 536T was grown on LB agar for 24 h at 30°C and cell morphology and flagellation were observed by using a HITACHI type H7500 transmission electron microscope and a negative-staining technique. For negative staining, Pseudomonas strain 536T was fixed with 2.5% glutaraldehyde and stained with 0.1% uranyl acetate. Growth in LB broth for 2 days was assessed at 4, 16, 25, 28, 37 and 41°C, at pH 5, 6, 8 and 10 and with 0, 1, 2, 4, 5, 8 and 10% (w/v) NaCl. Phenotypic characterization was assed using the GEN III MicroPlate (Biolog) following the manufacturer’s instruction and the API 20 NE kit (bioMérieux) in LB at 30°C. Whole-cell fatty acids composition was determined by FAME (Fatty Acid Methyl Ester) and respiratory quinones were extracted, and confirmed using HPLC (Collins and Jones, 1981; Sasser, 2001). P. toyotomiensis JCM 15604T and P. chengduensis DSM 26382T were used as reference strains and assessed in the same growth conditions.
Whole genome sequencing
In order to validate the rpoD-based taxonomic affiliation, the genome of one representative strain for each rpoD cluster, namely 402, 536 and 718, was sequenced. Genomic DNA was extracted using the Gentra Puregene Yeast/Bact. Kit (Qiagen, Hilden, Germany). Nextera XT library preparation kit and the Illumina MiSeq sequencer were used for genome sequencing (BASECLEAR, Leiden, The Netherlands). Libraries were sequenced using a paired-end approach (2 × 150 bp) and the genome coverage was routinely above 40 X. The quality of the Illumina reads was assessed using FastQC v. 0.11.9 and Trimmomatic v. 0.38 for adapter clipping, quality trimming (LEADING:3 TRAILING:3 SLIDINGWINDOW:4.15), and minimum length exclusion (>50 bp) (Bolger et al., 2014). De novo genome assembly was performed with the SPAdes assembler v. 3.13.0 (Bankevich et al., 2012). Strain 536T was re-sequenced using Nanopore technology (Oxford Nanopore Technology, London, United Kingdom). The quality of the dataset of nanopore reads was assessed with the NanoPack software suite (De Coster et al., 2018), and combined with Illumina using the hybrid assembler Unicycler v0.4.8 (Wick et al., 2017).
Phylogenetic and whole genome analyses
We inventoried all genomic sequences of Pseudomonas strains isolated from marine sources available on NCBI. We searched among three categories, unaffiliated Pseudomonas sp. strains (November 2021),1 and strains affiliated to P. fluorescens (November 2021)2 and P. putida (November 2021).3 Indeed, these two species also represent the largest groups within the Pseudomonas genus and NCBI affiliations at the species level are the most likely to be incorrect (Girard et al., 2021). The first phylogenetic analysis included the rpoD sequences of our 23 Pseudomonas isolates from marine microalgal cultures (Supplementary Table S1), the type strains of the P. oleovorans group and P. anguilliseptica (outgroup). The second rpoD-based phylogenetic analysis included 322 type strains (277 Pseudomonas, 23 Halopseudomonas, 14 Stutzerimonas, 7 other Pseudomonadaceae and Cellvibrio japonicus as the outgroup; Supplementary Table S2) and 61 marine Pseudomonas strains (Supplementary Table S3). The trees showing the phylogenetic relationships of environmental Pseudomonas isolates with the type strains were constructed using maximum-likelihood methods in MEGA-X (best evolutionary model; Tamura et al., 2011) and annotated with iTOL (Letunic and Bork, 2019). The genomes of type strains (275 Pseudomonas, 22 Halopseudomonas, 14 Stutzerimonas; Supplementary Table S2) and environemental strains (Supplementary Table S3) were used for whole genome analyses. Average nucleotide identity (ANI) values were calculated using the PYANI v0.2.10 with default parameters (Pritchard et al., 2016). When ANIb values were considered as ambiguous (i.e., between 95 and 96.5%), digital DNA–DNA Hybridization (dDDH) were calculated using the online tool, the Genome-to-Genome Distance Calculator GGDC (March 2022).4
BGCs analyses
We first used antiSMASH 6.0 to identify and annotate secondary metabolites BGCs (Blin et al., 2021). The MIBiG cluster comparison and ClusterBlast packages, now allow to detect potential unexplored forms of BGCs but also to easily identify identical BGCs in other strains. As not every known BGCs (published) is yet registered in the database, it thus considerably facilitates identifications and comparisons. The synteny of each BGC was manually inspected. NRPS clusters were checked to verify the expected domain organization (i.e., siderophores, lipopeptides) and the online PKS/NRPS analysis tool5 was used to delineate and extract A-domains for amino-acid sequence predictions (Rokni-Zadeh et al., 2012; Ye et al., 2013; Girard et al., 2020a).
Results and discussion
Pseudomonas isolates from marine microalgal cultures
A total of twenty-three Pseudomonas strains were co-isolated with Vibrio species on TCBS agar plates from three marine microalgal cultures, Chaetoceros calcitrans (n = 9), Chaetoceros gracilis (n = 12) and Isochrysis galbana affinis Tahiti (n = 2), and identified using rpoD amplicon analysis (Supplementary Table S1). All isolates belong to the Pseudomonas genus, clustering within the P. oleovorans group and affiliated to two known species, P. chengduensis (n = 19) and P. toyotomiensis (n = 2), and one new Pseudomonas species (n = 2; Supplementary Figure S1). Pseudomonas strains were previously isolated from cyanobacterial or phytoplanktonic blooms (Ansari et al., 2015; Choi et al., 2016; Park et al., 2016; Berner et al., 2018; Sinha et al., 2019). However, this is the first study reporting Pseudomonas isolates from the P. oleovorans group associated with marine diatom species. Diatoms from the genus Chaetoceros are not only the most widespread and abundant in marine habitats worldwide but also the richest in term of number of species (Li et al., 2017) which suggests that Pseudomonas strains could evolve in Chaetoceros blooms in diverse marine environments. Furthermore, Chaetoceros calcitrans, Chaetoceros gracilis and Isochrysis galbana affinis Tahiti are the most frequently used species in aquaculture, especially as food in bivalve and shrimp marinicultures, but can also be used for the production of biodiesel (Brown et al., 1997; Kwangdinata et al., 2014; Marquez et al., 2019). Considering the economic and ecological importance of these microalgae and the tight relationships between Pseudomonas spp. and plants in terrestrial environments (commensal, pathogenic and biocontrol strains; Höfte and De Vos, 2007; Girard et al., 2020a), it would be of great interest to study in depth relationships between Pseudomonas spp. and marine diatoms. Finally, Vibrio spp. are the most studied bacteria, in terms of diversity, abundance and distribution, in marine environments around the world (Zhang et al., 2018). TCBS is a selective medium commonly use for the enumeration of cultivable Vibrio spp. from all kinds of samples. Previous studies have highlighted that other bacteria can grow on this medium, such as Burkholderia cepacia, Aeromonas salmonicida, A. caviae, A. hydrophila, A. sobria, Chromobacterium violaceum, Listonella damsela, Shewanella putrefaciens, Flavobacterium meningosepticum and Pasteurella sp. (Hervio-Heath et al., 2002). However, this is the first study reporting the isolation of different Pseudomonas species on TCBS.
Taxonomic affiliations of marine Pseudomonas
Three isolates were selected to confirm rpoD-based taxonomic affiliation and whole genome sequencing was performed on strains 536, 402 and 718. ANIb values confirmed that Pseudomonas strains 402 (96.60%) and 718 (97.37%) are belonging to, respectively, P. chengduensis and P. toyotomiensis, while strain 536 represents a new species and was included in the following dataset for further analyses. Most of NCBI Pseudomonas genomes were belonging to strains isolated from soils or plants and only 77 genomes of strains isolated from marine sources were available, 3.7% of Pseudomonas sp. (65/1775), 3.7% of P. putida (7/190) and 1.8% of P. fluorescens (5/272). A large majority of these genomes were obtained in the course of broad metagenomics projects (e.g., Tara Ocean) and thus are highly fragmented (Supplementary Table S3). Consequently, we were only able to retrieve the rpoD gene in 61 genomes and these 61 strains were thus included in the rpoD-based phylogeny (Figure 1). However, whole genome analysis allowed us to conclude on the taxonomic status of the 77 strains (e.g., ANIb, Table 1; Supplementary Table S4). Following the recent changes in the Pseudomonadaceae family, our analyses show that almost half of the strains were belonging to the genus Pseudomonas while the remaining are members of the newly described genera, Halopseudomonas (n = 12) and Stutzerimonas (n = 27) (Table 1; Figure 1). All strains previously classified as P. putida and P. fluorescens were incorrectly affiliated at the species level (Table 1). Solely 22 strains were affiliated to known species while the remaining represented 26 new species, Halopseudomonas sp. #1 to 5, Stutzerimonas sp. #1 to 7 and Pseudomonas sp. #1 to 14 (Pseudomonas #3 corresponding to P. chaetocerotis sp. nov.; Table 1). These results highlight the great genetic diversity, yet unexplored, of strains pertaining to the Pseudomonadaceae family within marine samples.
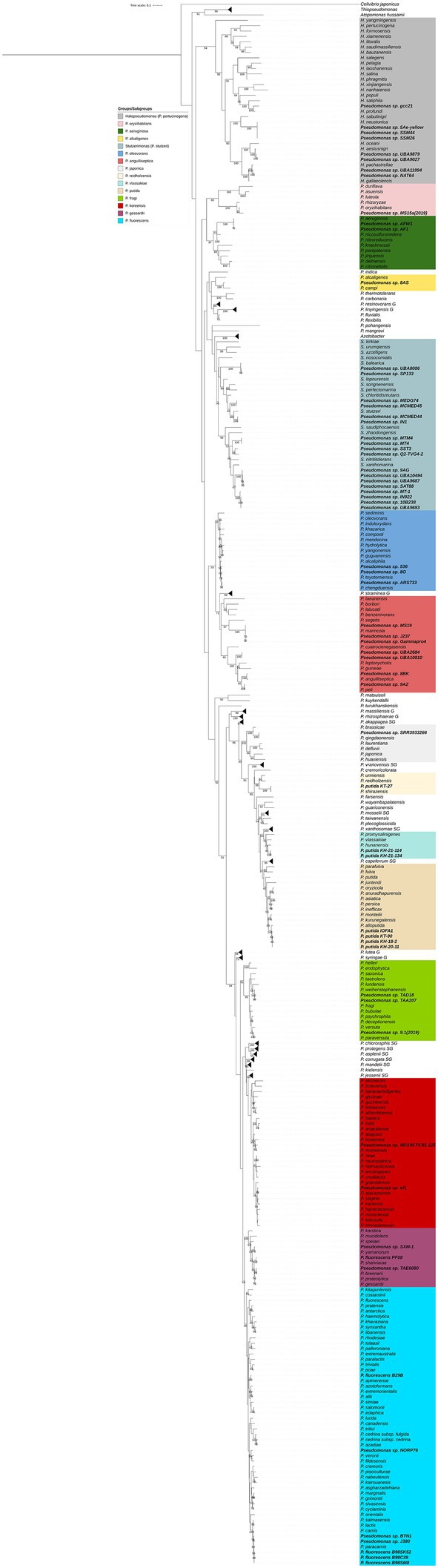
Figure 1. Phylogenetic tree of the Pseudomonadaceae based on the rpoD gene of type strains (Supplementary Table S2) and environment strains (Supplementary Table S3). The maximum likelihood phylogenetic tree was constructed using the GTR + G + I model (MEGA-X). Bootstrap values were calculated based on 1,000 replications and only bootstrap values higher than 50% are shown. Environmental strains are highlighted in bold. C. japonicus is used as the outgroup.
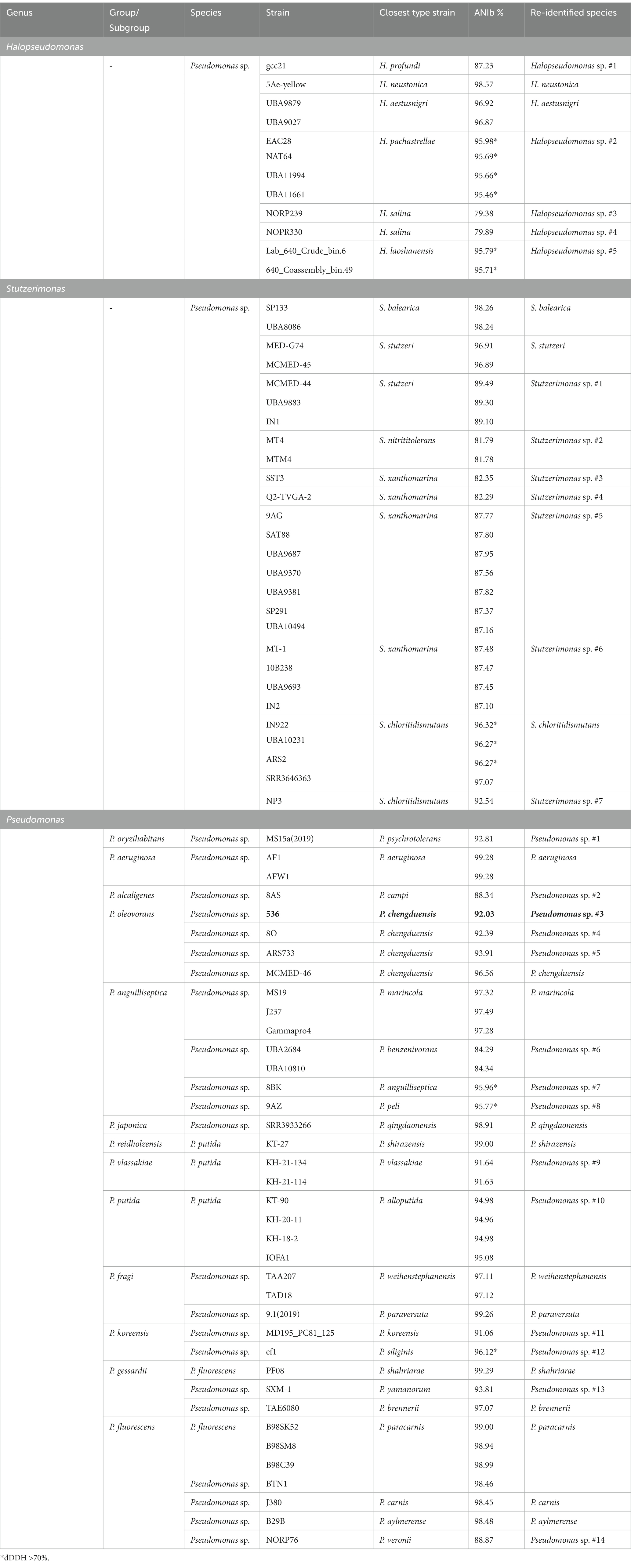
Table 1. Phylogenetic affiliation based on ANIb values for the 77 strains, previously not assigned or incorrectly assigned at the species level (Supplementary Table S4). Accession numbers are shown in Supplementary Table S3.
Pseudomonas chaetocerotis sp. nov.
The highest ANIb values between strain 536 and the type strains from the P. oleovorans group were observed when compared to P. chengduensis DSM 26382T (92.04%) and P. toyotomiensis JCM 15604T (91.92%; Supplementary Table S4). dDDH calculations confirmed the new species assignment with values below 70% for hybridization with P. chengduensis DSM 26382T (47.40%) and P. toyotomiensis JCM 15604T (47.20%). P. chengduensis DSM 26382T and P. toyotomiensis JCM 15604T were thus selected as reference strains for biochemical and chemotaxonomic characterization. The genomic features of strain 536 are: genome size 5.3 Mbp, scaffold count 44 and gene count 5,113 (coding 4,980; NCBI Prokaryotic Genome Annotation Pipeline, PGAP). Cells of strain 536 were 2.02–3.07 μm long and 0.82–1.19 μm wide, facultative anaerobic, Gram negative, and motile with a single polar flagellum (Supplementary Figure S2). Pseudomonas strain 536 was able to grow at 16–41°C, at pH 5–10 and in the presence of 0–8% (w/v) NaCl. Colonies appeared are small green colonies on TCBS and small cream colonies on MA after 48 h at 25°C; and as smalgram.
l, irregular, pale irregular colonies on LB agar after 24 h at 30°C. The biochemical characteristics of strain 536T and the two references strains P. toyotomiensis JCM 15604T and P. chengduensis DSM 26382T are detailed in Supplementary Table S5. Strain 536T can be easily differentiated from the two nearest relatives by a negative oxidase test or by growth on medium with antibiotic Aztreonam. The fatty acid profile of strain 536T is shown in Supplementary Table S6. The major fatty acid are C18:1 ω7c (35.81%), C16:1 ω7c/iso-C15:0 2-OH (22.90%), C16:0 (15.69%) and C12:0 (8.68%), a pattern similar to the two closest type strains, P. toyotomiensis JCM 15604T and P. chengduensis DSM 26382T. The respiratory quinones of strain 536T are Q-9 (97%), Q-8 (2%) and Q-10 (1%). The rpoD and whole genome analyses confirmed these affiliations and phenotypic characteristics further discriminated strain 536T from their closest phylogenetic neighbors P. toyotomiensis JCM 15604T and P. chengduensis DSM 26382T. Finally, chemotaxonomic phenotypic and genomic characteristics allowed the distinction from previously described species in the P. oleovorans group and thus, the description a new species, Pseudomonas chaetocerotis sp., with Pseudomonas strain 536T (=LMG 31766T = DSM 111343T) as the type strain (protologue; Table 2; Appendix A).
Proposal of transfer of species to the novel genera
We propose the transfer of 3 species, not transferred by Rudra and Gupta, 2021, to the genus Halopseudomonas that belong to the P. pertucinogena group in our study and in the corresponding references [P. laoshanensis (Wang et al., 2021), P. nanhaiensis (Pang et al., 2021) and P. yangmingensis (Wong and Lee, 2014)]. These transfers are also supported by results of Girard et al. (2020a,b), Girard et al. (2021), and Appendix A.
Genomics of secondary metabolites production by marine Pseudomonadaceae
A sub-selection of 37 genomes, excluding identical strains (i.e., ANIb values >99%), were surveyed for BGCs involved in secondary metabolite production. BGCs found in these 37 genomes included osmoprotectants, photo-protectants, quorum sensing molecules, siderophores, cyclic lipopeptides (CLPs) and numerous orphan NRPS gene clusters. Results are presented in Figure 2.
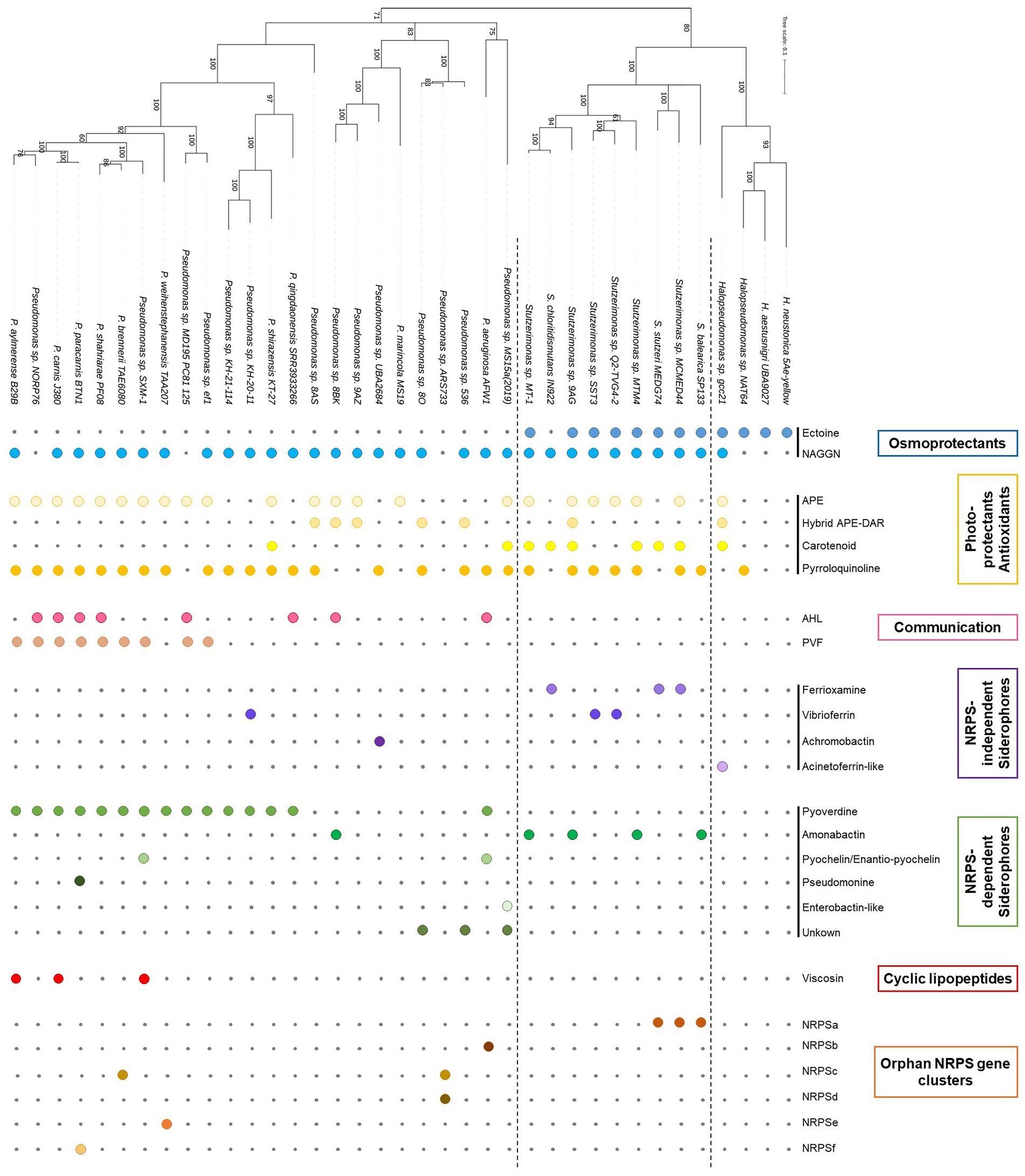
Figure 2. Genes and clusters involved in secondary metabolites production. The presence of a gene/cluster is symbolized by a colored circle, while the absence is indicated by a grey dot. NRPS, Non-ribosomal peptide synthetase.
Osmoprotectants
Osmoprotection seems to be a fundamental mechanism to cope with high salinity level exhibited in marine ecosystems. Early on, N-acetylglutaminylglutamine amide (NAGGN) was shown to be a dominant osmolyte used by Pseudomonas spp. when subjected to osmotic stress (D’Souza-Ault et al., 1993; Pocard et al., 1994). Lately, the NAGGN cluster was show to be widespread in Pseudomonas (Alam et al., 2021) and, more generally, in bacterial genomes (Sagot et al., 2010). Interestingly, while the NAGGN cluster is present in the genome of almost all Pseudomonadaceae strains, it seems that the ectoine BGC is confined to Halopseudomonas and Stutzerimonas strains (Figure 2). The original ectoine BGC, ectABC, encodes for a diaminobutyric acid (DABA) acetyltransferase (EctA), DABA aminotransferase (EctB) and ectoine synthase (EctC). However, numerous operon variants have been identified in halophilic γ-Proteobacteria, including an ectR (MarR-type transcriptional regulator gene), an ectD (ectoine hydroxylase gene) and/or an ask (aspartate kinase) (Schwibbert et al., 2011). Stutzerimonas stutzeri (P. stutzeri) was shown to produce hydroxyectoine via a ectABCD-ask cluster as observed in all Stutzerimonas genomes (Supplementary Table S7; Seip et al., 2011). On the other hand, the ectoine BGC present in Halopseudomonas strains were either an ectABCD-ask, like Stutzerimonas strains, or an ectABC-ask similarly to Vibrio parahaemolyticus (Supplementary Table S7), indicating the possible production of the osmolyte hydroxyectoine or ectoine. A phylogeny of the ectBC genes showed high similarity with the rpoD phylogeny revealing that this BGC has evolved in accordance to the evolutionary history of both genera (Supplementary Figure S3). However, two Stutzerimonas strains, S. nosocomialis and S. kirkiae, were accommodating a shorter version of this BGC, respectively, ectBCD-ask and ectBC-ask and were standing out of the ectBC phylogeny, indicating a recent acquisition/modification of this cluster (V3/V4; Supplementary Figure S3).
Photoprotectants/antioxidant
Antioxidants have a great application potential particularly in health and food industry and bacteria were recently pinpointed as a cost-effective way to produce this type of compounds (Ram et al., 2020). Antioxydants can also turn to be potential antimicrobials or quorum sensing inhibitors (Gökalsın et al., 2017; Ram et al., 2020). Among them, carotenoids have potential application in cancer prevention, reversal of multidrug resistance, reduction of virulence (via quorum quenching) but also as additives in food industry (Ram et al., 2020). Carotenoid production from Pseudomonas strains has been reported (Beuttler et al., 2011) and novel chemical structure were discovered using marine Pseudomonas (i.e., sponge associated isolate; Okadaxanthin; Miki et al., 1994). We observed in our genomes two type of Zeaxanthin-like BGCs, the crtE-idi-XYIBZ organization, found both in Pseudomonas, Halopseudomonas and Stutzerimonas strains, as previously reported for Enterobacteriaceae strains (Sedkova et al., 2005), and the unique organization crtE-idi-XYIB, found in P. shirazensis KT-27 (Supplementary Table S8). In other strains either the crtE upstream, the idi or the crtIBZ downstream the crtY are incomplete or missing, probably due to the fact that some genomes are highly fragmented.
Aryl polyenes (APE) is a highly abundant class of natural products that are functionally related to anti-oxidative carotenoids and APE BGCs are widespread among bacteria (Cimermancic et al., 2014; Schöner et al., 2016). So far, four classes of APEs have been described, the xanthomonadin and derivatives, the hybrid APE – dialkylresorcinol (DAR) arcuflavin, the hybrid APE-DAR flexirubin and derivatives and a flexirubin-like APE found in E. coli and V. fischeri (Cimermancic et al., 2014; Schöner et al., 2016). We found different variants of the APE BGCs in most of the analyzed genomes, all pertaining to the fourth class with organizations similar to E. coli and V. fischeri (APEV1-V3; Figure 3). Interestingly, we also found three types of hybrid APE-DAR BGCs with unique organizations and compositions that cannot be affiliated to the known classes (hybrid APE-DARV1-V3; Figure 3; Cimermancic et al., 2014; Schöner et al., 2014). Particularly, the type strain of P. chaetocerotis sp. nov., as well as Pseudomonas sp. 8O, carry a unique hybrid APE-DAR BGC, with an APE BGC similar to E. coli and V. fischeri connected to a DAR BGC (APE-DARV1; Figure 3). Likewise, the DAR part of these hybrid APE-DAR BGCs diverge from the 2,5 dialkylresorcinol (HPR) BGC, well-known for its antifungal activity, a biocontrol asset for crop associated Pseudomonas (HPR, Figure 3; Calderón et al., 2014; Biessy et al., 2019). Thus, a conscientious work of chemical characterization needs to be done to investigate the structural diversity of this type of compounds. Furthermore, even if it was recently demonstrated that APE genes are essential for E. coli to form biofilms (Johnston et al., 2021), little is known about the biological and ecological functions of this metabolite family.
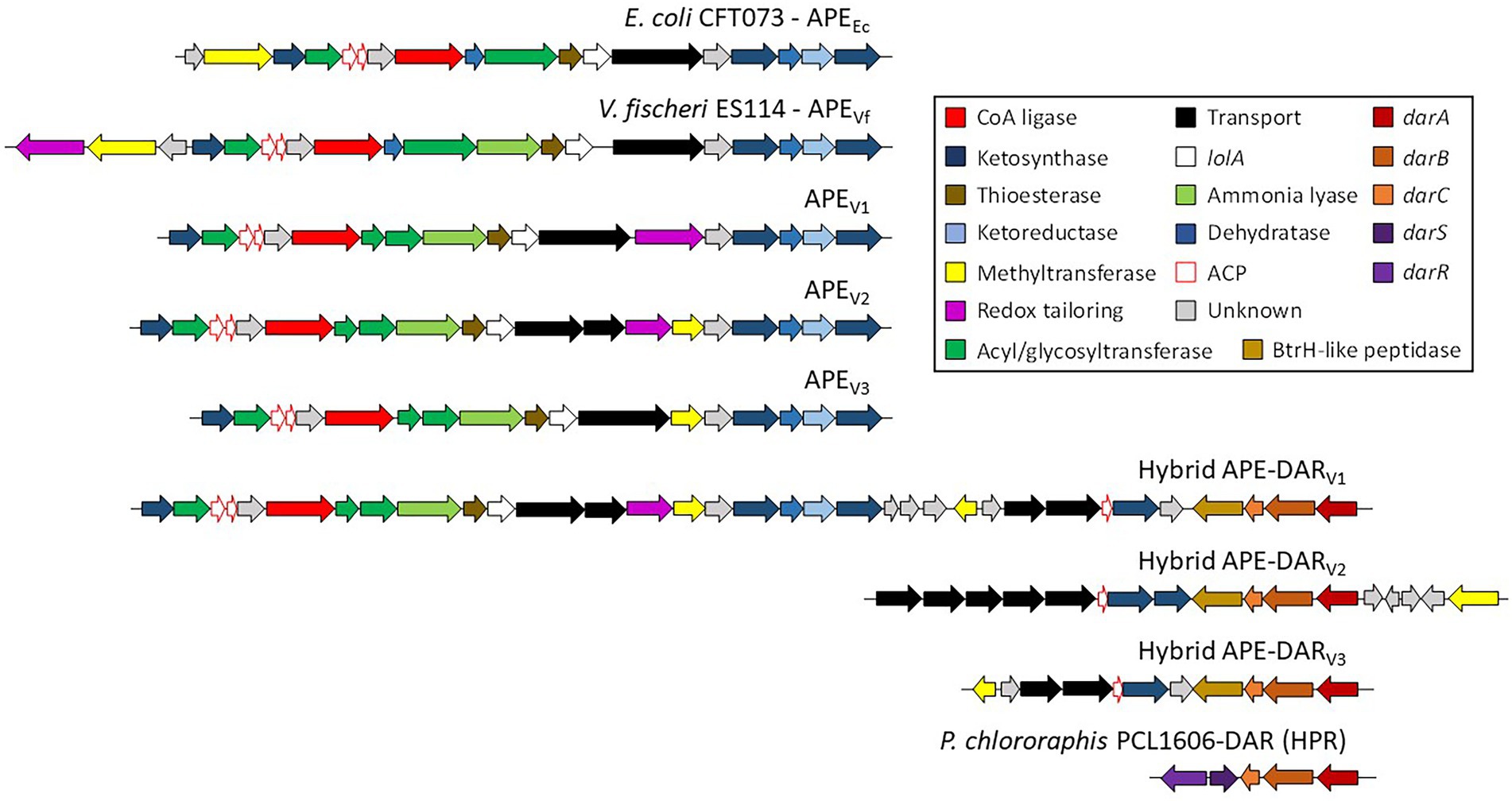
Figure 3. Known and proposed gene clusters involved in the biosynthesis of APE, DAR and Hybrid APE-DAR. All genes are scaled to the depicted size.
Pyrroloquinoline quinone (PQQ) was shown to be a plant growth promoting factor produced by P. fluorescens. The pqqFABCDEMKJIH cluster was identified as the source of this metabolite and it was demonstrated that all genes were essential for the production of PQQ (Choi et al., 2008). A truncated version of this cluster, pqqFABCDEMIH, is also present in P. putida KT2440 and is involved in the production of gluconic acid allowing the solubilization of mineral phosphates (An and Moe, 2016). PQQ BGCs are widespread in Pseudomonadaceae genomes and the classical organization pqqABCDE was conserved among the analyzed genomes, however many variants (i.e., presence or absence of the “accessory” genes pqqFHIJKM) were identified and potentially represent new forms of PQQ with similar or unknown functions (Supplementary Table S8).
Cell-to-cell communication
Quorum sensing (QS) genes were only found in Pseudomonas and were absent from Halopseudomonas and Stutzerimonas genomes. All LuxI homologs identified in this study are clustering with known Pseudomonas AHL synthases (Supplementary Table S8; Supplementary Figure S4). One divergent LuxI homolog was identified in Pseudomonas sp. 8BK and, interestingly, this is the first study reporting QS in strains pertaining to the P. anguilliseptica group (Supplementary Figure S4). Only two strains harbored two QS gene pairs, P. aeruginosa AFW1 carried the classical lasI/R and rhlI/R gene pairs, likewise most P. aeruginosa strains (Juhas et al., 2005), and Pseudomonas sp. NORP76, pertaining to the P. fluorescens subgroup, with one LuxI clustering with PmrI from P. wayambapalatensis RW10S2 and the second with PfsI from P. fuscovaginae UBP0736. Multiple communication systems, homologous to the luxI/luxR gene pair, have been identified among Pseudomonas genomes (Supplementary Table S9; Juhas et al., 2005). N-acyl-homoserine lactones (AHLs) based communication regulates numerous biological functions (e.g., virulence, biofilm formation) in Pseudomonas spp., but also the production of secondary metabolites (e.g., mupirocin, phenazine, cyclic lipopeptides) (Chin-A-Woeng et al., 1998; El-Sayed et al., 2001; Dubern et al., 2006; Arp et al., 2018). In marine environments, AHL were also shown to mediate interactions with cyanobacteria (Van Mooy et al., 2012), thus further studies are needed to chemically characterize AHL diversity among marine Pseudomonas.
Pyrazine-derived compounds are well known to coordinate communal behavior among bacteria (Silva-Junior, 2018). Pseudomonas strains typically produce pyrazine N-oxides (PNOs) through the Pseudomonas virulence factor BGC (pvfABCD) and PVF autoinducers regulate the expression of many genes involved in virulence, colonization and competition (Kretsch et al., 2018, 2021). Pvf genes are widely distributed among Proteobacteria and have been identified, in this study, among strains belonging to the P. fluorescens group (Figure 2; Supplementary Table S10). In marine environments, the autoinducer 3,5-dimethylpyrazin-2-ol (DPO) regulate virulence factor production and biofilm formation (Eickhoff and Bassler, 2018). Recently, DPO was also described to mediate interactions with bacteriophages (Silpe and Bassler, 2019; Silpe et al., 2020). Together both AHLs and PVF autoinducers may be the key to understand the interactions between Pseudomonas and other organisms in marine environments.
Siderophores
Oceans are an iron limiting environment and siderophore production is a major mechanism for marine heterotrophic bacteria but it also plays an important role in the oceanic biogeochemical cycling of iron (Mawji et al., 2008). Numerous NRPS-dependent and independent BGCs for siderophore production were identified in our marine Pseudomonadaceae genomes. We observed a greater diversity of siderophores BGCs among Pseudomonas strains (n = 8). Several BGCs are very common in Pseudomonas genomes, such as pyoverdines, pyochelin, enantio-pyochelin or pseudomonine (Gross and Loper, 2009; Garrido-Sanz et al., 2016), while others have never been detected or sporadically in very few Pseudomonas strains. Indeed, vibrioferrin production was previously reported in P. fragi (Stanborough et al., 2018) and achromobactin was characterized in P. syringae strains (Berti and Thomas, 2009; Owen and Ackerley, 2011) while amonabactin has never reported in Pseudomonas strains. Interestingly, Pseudomonas sp. MS15a(2019), carries an enterobactin-like cluster, excluding, in comparison to the original cluster present in E.coli DSM 30083, the presence of three genes (fepE, entD and entH, Figure 4A). Enterobactin production has never been reported for Pseudomonas strains, however the ClusterBlast function of antismash allowed us to identify identical (P. oryzihabitans S00005, P. oleovorans AG1002, Pseudomonas sp. Snoq117.2 and 1766) or similar clusters in many Pseudomonas genomes.
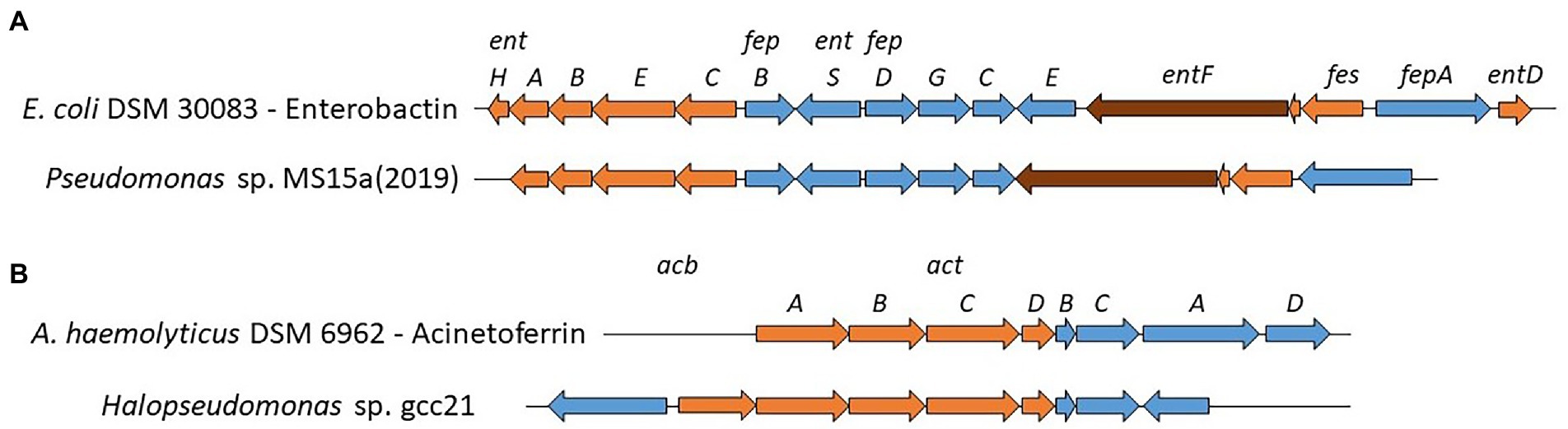
Figure 4. Known and proposed gene clusters involved in the biosynthesis of Enterobactin (A) and Acinetoferrin (B). All genes are scaled to the depicted size. Biosynthesis genes are highlighted in orange (NRPS in brown) and transport genes in blue.
The most common NRPS-based siderophores among fluorescent Pseudomonas are the pyoverdines (Meyer et al., 2008; Biessy et al., 2019). A first attempt of classification was made based on pyoverdines secreted by P. aeruginosa strains and three classes were defined. Later on, the structural characterization of a large amount of pyoverdines have led to the expansion of these classes (Cezard et al., 2014). The structural diversity of pyoverdines is well characterized, and they all consist of three distinct structural parts, a quinoline-1-carboxylic acid containing a chromophore, a dicarboxylic acid or its monoamide, and a peptide chain comprising 6–14 amino acids (Visca et al., 2007). Both the chromophore and the peptide chain of pyoverdines are synthesized by NRPSs. While the chromophore part, encoded by pvdL, is identical and common to all Pseudomonas strains, the primary difference among pyoverdines lies in their peptide chain and thus the NRPSs organizations. Initial studies on P. aeruginosa identified 3 NRPS genes, namely pvdIJD, encoding for 8 modules (4, 2 and 2) (Ravel and Cornelis, 2003). Subsequently, diverse pyoverdine BGCs were described in Pseudomonas strains (e.g., P. syringae, P. putida and P. fluorescens) differing in the number of gene, gene organization, modules composition and thus peptide chain length and composition (Ravel and Cornelis, 2003; Ye et al., 2013). Each module contains domains with different functions, with the essential ones being the condensation domain (C-domain, formation of the peptide bond), thiolation (T-domain) and peptide carrier protein (PCP), and adenylation domain (A-domain, amino acid selection) (Ye et al., 2013). A-domains, allowing with a certain specificity the selection and sequential incorporation of amino acids into the peptidic chain, enable to predict the sequence of the peptide (Bachmann and Ravel, 2009). All strains pertaining to the P. fluorescens and P. putida groups and the P. aeruginosa AFW1 have a pyoverdine BGC (Supplementary Table S10). A-domains phylogeny, including Pseudomonas strains with characterized pyoverdines (Supplementary Table S11) and A-domains extracted from the genomes of our marine strains (Supplementary Table S10), allowed the prediction of amino-acid peptide sequence for 9/15 strains (Table 3; Supplementary Figure S5). The remaining strains have a fragmented pyoverdine BGC making the predictions impossible. Most of the predicted amino-acid peptide sequence were identical to previously described type I and II pyoverdines. Nonetheless, two unusual peptide sequence were identified in Pseudomonas sp. KH-21-114 (Lys-Asp-Ser-Orn) and P. shirazensis KT-27 (Ser-Lys-His-Asp-Orn), and such short peptide sequence have never been described. However, further studies are needed to determine if these pyoverdines are actually produced and remain functional. Another unusual BGC coding for a NRPS-based siderophore was identified in the genome of three strains, Pseudomonas sp. MS15a(2019) (P. oryzihabitans group), Pseudomonas sp. 8O and P. chaetocerotis 536T (P. oleovorans group). A-domains phylogeny allowed the prediction of a peptide composed of 6 amino acids Asp-Dab-Ser-Orn-Ser-Orn (not done for P. chaetocerotis 536T, second part of the BGC too fragmented). An identical BGC was shown to be responsible for the production of an hydroxamate-based siderophore by P. mendocina ymp, however this siderophore still awaits chemical characterization (Awaya and DuBois, 2008).
On the other hand, less diversity was observed in Stutzerimonas strains (n = 3) while only one strain of Halopseudomonas carried a siderophore BGC. Stutzerimonas strains carried, as previously reported, either an amonabactin, a ferrioxamine or a vibrioferrin BGC (Zawadzka et al., 2006; Essén et al., 2007). Halopseudomonas sp. gcc21, possess an acinetoferrin-like BGC, with the actA gene upstream the biosynthesis genes (orange Figure 4B) and an extra biosynthesis gene upstream acbA coding for a pyridoxal-dependent decarboxylase (Pfam: PF0082). Interestingly, there are no hits (ClusterBlast, antismash) with other Halopseudomonas strains but with Alkanindiges illinoisensis DSM 15370 and Acinetobacter strains. Considering the fact that no other Halopseudomonas strain carries such siderophore BGC, we believe that this acinetoferrin-like BGC has been acquired recently in the evolution of this strain.
Cyclic lipopeptides
CLPs are biosurfactants made of a fatty acid tail attached to a cyclic oligopeptide with a wide range of antibacterial and antifungal activities (Geudens and Martins, 2018; Götze and Stallforth, 2019). Pseudomonas CLPs are involved numerous ecological functions such as biocontrol activity, bacterial motility or biofilm formation (Geudens and Martins, 2018). Similarly to pyoverdines, CLPs are assembled by NRPSs and from the modularity of these enzymes comes a wide diversity of variants, classified in several families based on the size and nature of their oligopeptide (Geudens and Martins, 2018). CLP BGCs are absent from Halopseudomonas and Stutzerimonas genomes. Most Pseudomonas strains analyzed here do not carry CLP BGCs but their presence was revealed in the genomes of three strains from the P. fluorescens group, namely Pseudomonas sp. SXM-1, P. carnis J380 and P. aylmerense B29B. All BGCs were composed of three NRPS genes, coding, respectively, for 2, 4 and 3 modules, in a split organization where the first biosynthetic gene is separated from the two others (Supplementary Table S10). This allowed us to conclude their affiliation to the Viscosin family and a phylogenetic analysis based on the concatenated NRPS proteins, including known members of the Viscosin family (Supplementary Table S12) and the three strains cited above, is shown in Supplementary Figure S6. Members of this CLP family are well-known to be involved, for soilborne and plant associated Pseudomonas, in swarming motility and antagonism, but their function within marine ecosystems remains unknown.
Unknown clusters
We detected numerous putative NRPS clusters, one among Stutzerimonas strains (NRPSa) and 5 among Pseudomonas strains (NRPSb to f, Figure 2). Accession numbers for these NRPSs genes can be found in Supplementary Table S10. To date, most Pseudomonas secondary metabolites are NRPS based (Gross and Loper, 2009) and NRPSs are a promising source for the discovery of novel bioactive natural products (Challis, 2008). Beside the putative NRPS clusters, we observed the presence of many RIPP-like genes within the analyzed genomes (1 to 4 RIPP-genes by genome). Ribosomally synthesized and post-translationally modified peptides (RiPPs) are a diverse group of bioactive compounds (Arnison et al., 2013) and RIPP genes are widespread among prokaryotic genomes (Skinnider et al., 2016). These orphan NRPS and RIPP clusters represent a real challenge for microbiologists and chemists in the discovery of new chemical structure and potent biological activities.
Conclusion
We studied here the genetic diversity together with the metabolic potential of marine Pseudomonadaceae. We showed that marine environments host a wide diversity of Pseudomonadaceae and highlight the need to further explore their diversity, distribution, and seasonality in marine environments. The identification of BGCs responsible for secondary metabolites production in Pseudomonas, Stutzerimonas and Halopseudomonas genomes allowed us to identify new producers of known metabolites and new variants of BGCs possibly coding for the production of new metabolites. Numerous strains, including the type strain of the newly described species P. chaetocerotis, require further work to chemically characterize these new compounds, particularly the new variants of siderophores (pyoverdines, acinetoferrin and enterobactin) and APEs, but also new metabolites such as the hybrid APE-DARs and the new NRPS-dependent siderophore. Finally, the majority of these different classes of metabolites have well-defined ecological functions for Pseudomonads in terrestrial environments but a tremendous amount of work is still needed to understand their role and importance within marine ecosystems.
Data availability statement
The datasets presented in this study can be found in online repositories. The names of the repository/repositories and accession number(s) can be found in the article/Supplementary material.
Author contributions
LG: conceptualization, data analysis, investigation, and writing – original draft and editing. CL: methodology, data analysis, and writing – review and editing. VN and RM: writing – review and editing and funding acquisition. JB: resources, formal analysis, writing – review and editing, and funding acquisition. All authors contributed to the article and approved the submitted version.
Funding
This work was funded by Sorbonne Universités (JB), supported by the EOS grant 30650620 (LG and RM). CL was supported by the Research Foundation – Flanders grant 1S64720N and the Research Council of KU Leuven grant PDMt2/21/038.
Acknowledgments
We thank the technical support of EMBRC-France, whose French state funds are managed by the ANR within the Investments of the Future program under reference ANR-10-INBS-02. We acknowledge the BioPIC an Bio2Mar platforms (CNRS-Sorbonne Université, Oceanological Observatory of Banyuls-sur-Mer) and particularly Marie-Line Escande for technical support and TEM preparation and image generation.
Conflict of interest
The authors declare that the research was conducted in the absence of any commercial or financial relationships that could be construed as a potential conflict of interest.
Publisher’s note
All claims expressed in this article are solely those of the authors and do not necessarily represent those of their affiliated organizations, or those of the publisher, the editors and the reviewers. Any product that may be evaluated in this article, or claim that may be made by its manufacturer, is not guaranteed or endorsed by the publisher.
Supplementary material
The Supplementary material for this article can be found online at: https://www.frontiersin.org/articles/10.3389/fmicb.2023.1071039/full#supplementary-material
Footnotes
1. ^https://www.ncbi.nlm.nih.gov/genome/browse/#!/prokaryotes/13508/
2. ^https://www.ncbi.nlm.nih.gov/genome/?term=pseudomonas+fluorescens
3. ^https://www.ncbi.nlm.nih.gov/genome/browse/#!/prokaryotes/174/
References
Alam, K., Islam, M. M., Li, C., Sultana, S., Zhong, L., Shen, Q., et al. (2021). Genome mining of pseudomonas species: diversity and evolution of metabolic and biosynthetic potential. Molecules 26:7524. doi: 10.3390/molecules26247524
Amann, C., Taraz, K., Budzikiewicz, H., and Meyer, J.-M. (2000). The siderophores of Pseudomonas fluorescens 18.1 and the importance of cyclopeptidic substructures for the recognition at the cell surface. Zeitschrift für Naturforschung C 55, 671–680. doi: 10.1515/znc-2000-9-1001
Amer, R. A., Mapelli, F., El Gendi, H. M., Barbato, M., Goda, D. A., Corsini, A., et al. (2015). Bacterial diversity and bioremediation potential of the highly contaminated marine sediments at el-max district (Egypt, Mediterranean Sea). Biomed. Res. Int. 2015, 1–17. doi: 10.1155/2015/981829
An, R., and Moe, L. A. (2016). Regulation of pyrroloquinoline quinone-dependent glucose dehydrogenase activity in the model rhizosphere-dwelling bacterium pseudomonas putida KT2440. Appl. Environ. Microbiol. 82, 4955–4964. doi: 10.1128/AEM.00813-16
Ansari, M. I., Harb, M., Jones, B., and Hong, P.-Y. (2015). Molecular-based approaches to characterize coastal microbial community and their potential relation to the trophic state of Red Sea. Sci. Rep. 5:9001. doi: 10.1038/srep09001
Arnison, P. G., Bibb, M. J., Bierbaum, G., Bowers, A. A., Bugni, T. S., Bulaj, G., et al. (2013). Ribosomally synthesized and post-translationally modified peptide natural products: overview and recommendations for a universal nomenclature. Nat. Prod. Rep. 30, 108–160. doi: 10.1039/C2NP20085F
Arp, J., Götze, S., Mukherji, R., Mattern, D. J., García-Altares, M., Klapper, M., et al. (2018). Synergistic activity of cosecreted natural products from amoebae-associated bacteria. Proc. Natl. Acad. Sci. U. S. A. 115, 3758–3763. doi: 10.1073/pnas.1721790115
Awaya, J. D., and DuBois, J. L. (2008). Identification, isolation, and analysis of a gene cluster involved in iron acquisition by Pseudomonas mendocina ymp. Biometals 21, 353–366. doi: 10.1007/s10534-007-9124-5
Bachmann, B. O., and Ravel, J. (2009). Chapter 8. Methods for in silico prediction of microbial polyketide and nonribosomal peptide biosynthetic pathways from DNA sequence data. Meth. Enzymol. 458, 181–217. doi: 10.1016/S0076-6879(09)04808-3
Bankevich, A., Nurk, S., Antipov, D., Gurevich, A. A., Dvorkin, M., Kulikov, A. S., et al. (2012). SPAdes: A new genome assembly algorithm and its applications to single-cell sequencing. J. Comput. Biol. 19, 455–477. doi: 10.1089/cmb.2012.0021
Beaton, A., Lood, C., Cunningham-Oakes, E., MacFadyen, A., Mullins, A. J., Bestawy, W. E., et al. (2018). Community-led comparative genomic and phenotypic analysis of the aquaculture pathogen Pseudomonas baetica a390T sequenced by ion semiconductor and Nanopore technologies. FEMS Microbiol. Lett. 365. doi: 10.1093/femsle/fny069
Beiderbeck, H., Risse, D., Budzikiewicz, H., and Taraz, K. (1999). A new pyoverdin from Pseudomonas aureofaciens. Zeitschrift für Naturforschung C 54, 1–5. doi: 10.1515/znc-1999-1-202
Berner, C., Bertos-Fortis, M., Pinhassi, J., and Legrand, C. (2018). Response of microbial communities to changing climate conditions during summer cyanobacterial blooms in the Baltic Sea. Front. Microbiol. 9:1562. doi: 10.3389/fmicb.2018.01562
Berti, A. D., and Thomas, M. G. (2009). Analysis of achromobactin biosynthesis by pseudomonas syringae pv. Syringae B728a. J. Bacteriol. 191, 4594–4604. doi: 10.1128/JB.00457-09
Beuttler, H., Hoffmann, J., Jeske, M., Hauer, B., Schmid, R. D., Altenbuchner, J., et al. (2011). Biosynthesis of zeaxanthin in recombinant pseudomonas putida. Appl. Microbiol. Biotechnol. 89, 1137–1147. doi: 10.1007/s00253-010-2961-0
Biessy, A., Novinscak, A., Blom, J., Léger, G., Thomashow, L. S., Cazorla, F. M., et al. (2019). Diversity of phytobeneficial traits revealed by whole-genome analysis of worldwide-isolated phenazine-producing pseudomonas spp. Environ. Microbiol. 21, 437–455. doi: 10.1111/1462-2920.14476
Blin, K., Shaw, S., Kloosterman, A. M., Charlop-Powers, Z., van Wezel, G. P., Medema, M. H., et al. (2021). antiSMASH 6.0: improving cluster detection and comparison capabilities. Nucleic Acids Res. 49, W29–W35. doi: 10.1093/nar/gkab335
Bolger, A. M., Lohse, M., and Usadel, B. (2014). Trimmomatic: a flexible trimmer for Illumina sequence data. Bioinformatics 30, 2114–2120. doi: 10.1093/bioinformatics/btu170
Bollinger, A., Thies, S., Katzke, N., and Jaeger, K. (2020). The biotechnological potential of marine bacteria in the novel lineage of pseudomonas pertucinogena. Microb. Biotechnol. 13, 19–31. doi: 10.1111/1751-7915.13288
Brown, M. R., Jeffrey, S. W., Volkman, J. K., and Dunstan, G. A. (1997). Nutritional properties of microalgae for mariculture. Aquaculture 151, 315–331. doi: 10.1016/S0044-8486(96)01501-3
Budzikiewicz, H., Kilz, S., Taraz, K., and Meyer, J.-M. (1997). Identical pyoverdines from Pseudomonas fluorescens 9AW and from Pseudomonas putida 9BW. Zeitschrift für Naturforschung C 52, 721–728. doi: 10.1515/znc-1997-11-1202
Calderón, C. E., de Vicente, A., and Cazorla, F. M. (2014). Role of 2-hexyl, 5-propyl resorcinol production by pseudomonas chlororaphis PCL1606 in the multitrophic interactions in the avocado rhizosphere during the biocontrol process. FEMS Microbiol. Ecol. 89, 20–31. doi: 10.1111/1574-6941.12319
Carroll, A. R., Copp, B. R., Davis, R. A., Keyzers, R. A., and Prinsep, M. R. (2019). Marine natural products. Nat. Prod. Rep. 36, 122–173. doi: 10.1039/C8NP00092A
Cezard, C., Farvacques, N., and Sonnet, P. (2014). Chemistry and biology of pyoverdines, pseudomonas primary siderophores. CMC 22, 165–186. doi: 10.2174/0929867321666141011194624
Challis, G. L. (2008). Genome mining for novel natural product discovery. J. Med. Chem. 51, 2618–2628. doi: 10.1021/jm700948z
Chin-A-Woeng, T. F. C., Bloemberg, G. V., van der Bij, A. J., van der Drift, K. M. G. M., Schripsema, J., Kroon, B., et al. (1998). Biocontrol by phenazine-1-carboxamide-producing pseudomonas chlororaphis PCL1391 of tomato root rot caused by Fusarium oxysporum f. sp. radicis-lycopersici. Mol. Plant-Microbe Interact. 11, 1069–1077. doi: 10.1094/MPMI.1998.11.11.1069
Choi, S.-B., Kim, J.-G., Jung, M.-Y., Kim, S.-J., Min, U.-G., Si, O.-J., et al. (2016). Cultivation and biochemical characterization of heterotrophic bacteria associated with phytoplankton bloom in the Amundsen Sea polynya, Antarctica. Deep-Sea Res. II Top. Stud. Oceanogr. 123, 126–134. doi: 10.1016/j.dsr2.2015.04.027
Choi, O., Kim, J., Kim, J.-G., Jeong, Y., Moon, J. S., Park, C. S., et al. (2008). Pyrroloquinoline quinone is a plant growth promotion factor produced by Pseudomonas fluorescens B16. Plant Physiol. 146, 657–668. doi: 10.1104/pp.107.112748
Cimermancic, P., Medema, M. H., Claesen, J., Kurita, K., Wieland Brown, L. C., Mavrommatis, K., et al. (2014). Insights into secondary metabolism from a global analysis of prokaryotic biosynthetic gene clusters. Cells 158, 412–421. doi: 10.1016/j.cell.2014.06.034
Collins, M. D., and Jones, D. (1981). Distribution of isoprenoid quinone structural types in bacteria and their taxonomic implication. Microbiol. Rev. 45, 316–354.
Demange, P., Bateman, A., Mertz, C., Dell, A., Piemont, Y., and Abdallah, M. A. (1990). Bacterial siderophores: structures of pyoverdins Pt, siderophores of Pseudomonas tolaasii NCPPB 2192, and pyoverdins Pf, siderophores of Pseudomonas fluorescens CCM 2798. Identification of an unusual natural amino acid. Biochemistry 29, 11041–11051. doi: 10.1021/bi00502a005
D’Souza-Ault, M. R., Smith, L. T., and Smith, G. M. (1993). Roles of N-acetylglutaminylglutamine amide and glycine betaine in adaptation of Pseudomonas aeruginosa to osmotic stress. Appl. Environ. Microbiol. 59, 473–478. doi: 10.1128/aem.59.2.473-478.1993
de Bruijn, I., de Kock, M. J. D., Yang, M., de Waard, P., van Beek, T. A., and Raaijmakers, J. M. (2007). Genome-based discovery, structure prediction and functional analysis of cyclic lipopeptide antibiotics in pseudomonas species. Mol. Microbiol. 63, 417–428. doi: 10.1111/j.1365-2958.2006.05525.x
De Coster, W., D’Hert, S., Schultz, D. T., Cruts, M., and Van Broeckhoven, C. (2018). NanoPack: visualizing and processing long-read sequencing data. Bioinformatics 34, 2666–2669. doi: 10.1093/bioinformatics/bty149
Dignam, B. E. A., O’Callaghan, M., Condron, L. M., Raaijmakers, J. M., Kowalchuk, G. A., and Wakelin, S. A. (2019). Impacts of long-term plant residue management on soil organic matter quality, pseudomonas community structure and disease suppressiveness. Soil Biol. Biochem. 135, 396–406. doi: 10.1016/j.soilbio.2019.05.020
Dubern, J.-F., Lugtenberg, B. J. J., and Bloemberg, G. V. (2006). The ppuI-rsaL-ppuR quorum-sensing system regulates biofilm formation of pseudomonas putida PCL1445 by controlling biosynthesis of the cyclic lipopeptides putisolvins I and II. J. Bacteriol. 188, 2898–2906. doi: 10.1128/JB.188.8.2898-2906.2006
Eickhoff, M. J., and Bassler, B. L. (2018). SnapShot: bacterial quorum sensing. Cells 174, 1328–1328.e1. doi: 10.1016/j.cell.2018.08.003
El-Sayed, A. K., Hothersall, J., and Thomas, C. M. (2001). Quorum-sensing-dependent regulation of biosynthesis of the polyketide antibiotic mupirocin in Pseudomonas fluorescens NCIMB 10586. Microbiology 147, 2127–2139. doi: 10.1099/00221287-147-8-2127
Essén, S. A., Johnsson, A., Bylund, D., Pedersen, K., and Lundström, U. S. (2007). Siderophore production by pseudomonas stutzeri under aerobic and anaerobic conditions. Appl. Environ. Microbiol. 73, 5857–5864. doi: 10.1128/AEM.00072-07
Garrido-Sanz, D., Meier-Kolthoff, J. P., Göker, M., Martín, M., Rivilla, R., and Redondo-Nieto, M. (2016). Genomic and genetic diversity within the Pseudomonas fluorescens complex. PLoS One 11:e0150183. doi: 10.1371/journal.pone.0150183
Georgias, H., Taraz, K., Budzikiewicz, H., Geoffroy, V., and Meyer, J.-M. (1999). The structure of the pyoverdin from Pseudomonas fluorescens 1.3. structural and biological relationships of pyoverdins from different strains. Zeitschrift für Naturforschung C 54, 301–308. doi: 10.1515/znc-1999-5-602
Geudens, N., and Martins, J. C. (2018). Cyclic lipodepsipeptides from pseudomonas spp. – biological swiss-army knives. Front. Microbiol. 9:1867. doi: 10.3389/fmicb.2018.01867
Girard, L., Höfte, M., and Mot, R. D. (2020a). Lipopeptide families at the interface between pathogenic and beneficial pseudomonas-plant interactions. Crit. Rev. Microbiol. 46, 397–419. doi: 10.1080/1040841X.2020.1794790
Girard, L., Lantoine, F., Lami, R., Vouvé, F., Suzuki, M. T., and Baudart, J. (2018). Genetic diversity and phenotypic plasticity of AHL-mediated quorum sensing in environmental strains of vibrio mediterranei. ISME J. 13, 159–169. doi: 10.1038/s41396-018-0260-4
Girard, L., Lood, C., Höfte, M., Vandamme, P., Rokni-Zadeh, H., van Noort, V., et al. (2021). The ever-expanding pseudomonas genus: description of 43 new species and partition of the pseudomonas putida group. Microorganisms 9:1766. doi: 10.3390/microorganisms9081766
Girard, L., Lood, C., Rokni-Zadeh, H., van Noort, V., Lavigne, R., and De Mot, R. (2020b). Reliable identification of environmental pseudomonas isolates using the rpoD gene. Microorganisms 8:1166. doi: 10.3390/microorganisms8081166
Gökalsın, B., Aksoydan, B., Erman, B., and Sesal, N. C. (2017). Reducing virulence and biofilm of Pseudomonas aeruginosa by potential quorum sensing inhibitor carotenoid: zeaxanthin. Microb. Ecol. 74, 466–473. doi: 10.1007/s00248-017-0949-3
Götze, S., and Stallforth, P. (2019). Structure, properties, and biological functions of nonribosomal lipopeptides from pseudomonads. Nat. Prod. Rep. 37, 29–54. doi: 10.1039/C9NP00022D
Gross, H., and Loper, J. E. (2009). Genomics of secondary metabolite production by pseudomonas spp. Nat. Prod. Rep. 26, 1408–1446. doi: 10.1039/b817075b
Hervio-Heath, D., Colwell, R. R., Derrien, A., Robert-Pillot, A., Fournier, J. M., and Pommepuy, M. (2002). Occurrence of pathogenic vibrios in coastal areas of France. J. Appl. Microbiol. 92, 1123–1135. doi: 10.1046/j.1365-2672.2002.01663.x
Höfte, M., and De Vos, P. (2007). “Plant pathogenic Pseudomonas species” in Plant-Associated Bacteria. ed. S. S. Gnanamanickam (Dordrecht: Springer Netherlands), 507–533.
Jiang, Y.-F., Ling, J., Wang, Y.-S., Chen, B., Zhang, Y.-Y., and Dong, J.-D. (2015). Cultivation-dependent analysis of the microbial diversity associated with the seagrass meadows in Xincun Bay, South China Sea. Ecotoxicology 24, 1540–1547. doi: 10.1007/s10646-015-1519-4
Johnston, I., Osborn, L. J., Markley, R. L., McManus, E. A., Kadam, A., Schultz, K. B., et al. (2021). Identification of essential genes for Escherichia coli aryl polyene biosynthesis and function in biofilm formation. NPJ Biofilms Microbiomes 7:56. doi: 10.1038/s41522-021-00226-3
Juhas, M., Eberl, L., and Tummler, B. (2005). Quorum sensing: the power of cooperation in the world of pseudomonas. Environ. Microbiol. 7, 459–471. doi: 10.1111/j.1462-2920.2005.00769.x
Kraemer, S. M. (2004). Iron oxide dissolution and solubility in the presence of siderophores. Aquat. Sci. Res. Across Bound. 66, 3–18. doi: 10.1007/s00027-003-0690-5
Kretsch, A. M., Morgan, G. L., Acken, K. A., Barr, S. A., and Li, B. (2021). Pseudomonas virulence factor pathway synthesizes autoinducers that regulate the secretome of a pathogen. ACS Chem. Biol. 16, 501–509. doi: 10.1021/acschembio.0c00901
Kretsch, A. M., Morgan, G. L., Tyrrell, J., Mevers, E., Vallet-Gély, I., and Li, B. (2018). Discovery of (Dihydro)pyrazine N-oxides via genome mining in pseudomonas. Org. Lett. 20, 4791–4795. doi: 10.1021/acs.orglett.8b01944
Kwangdinata, R., Raya, I., and Zakir, M. (2014). Production of biodiesel from lipid of phytoplankton Chaetoceros calcitrans through ultrasonic method. Sci. World J. 2014, 1–5. doi: 10.1155/2014/231361
Lalucat, J., Gomila, M., Mulet, M., Zaruma, A., and García-Valdés, E. (2022). Past, present and future of the boundaries of the pseudomonas genus: proposal of Stutzerimonas gen. Nov. Syst. Appl. Microbiol. 45:126289. doi: 10.1016/j.syapm.2021.126289
Lalucat, J., Mulet, M., Gomila, M., and García-Valdés, E. (2020). Genomics in bacterial taxonomy: impact on the genus pseudomonas. Genes 11:139. doi: 10.3390/genes11020139
Letunic, I., and Bork, P. (2019). Interactive tree of life (iTOL) v4: recent updates and new developments. Nucleic Acids Res. 47, W256–W259. doi: 10.1093/nar/gkz239
Li, Y., Boonprakob, A., Gaonkar, C. C., Kooistra, W. H. C. F., Lange, C. B., Hernández-Becerril, D., et al. (2017). Diversity in the globally distributed diatom genus Chaetoceros (Bacillariophyceae): three new species from warm-temperate waters. PLoS One 12:e0168887. doi: 10.1371/journal.pone.0168887
Linget, C., Azadi, P., MacLeod, J. K., Dell, A., and Abdallah, M. A. (1992). Bacterial siderophores: the structures of the pyoverdins of Pseudomonas fluorescens ATCC 13525. Tetrahedron Lett. 33, 1737–1740. doi: 10.1016/S0040-4039(00)91719-2
Lopez, J. R., Dieguez, A. L., Doce, A., De la Roca, E., De la Herran, R., Navas, J. I., et al. (2012). Pseudomonas baetica sp. nov., a fish pathogen isolated from wedge sole, Dicologlossa cuneata (Moreau). IJSEM 62, 874–882. doi: 10.1099/ijs.0.030601-0
Marquez, A., Lodeiros, C., Loor, A., Revilla, J., Da Costa, F., and Sonnenholzner, S. (2019). Microalgae diet for juveniles of Spondylus limbatus. Aquacult Int 27, 323–335. doi: 10.1007/s10499-018-0327-2
Mawji, E., Gledhill, M., Milton, J. A., Tarran, G. A., Ussher, S., Thompson, A., et al. (2008). Hydroxamate siderophores: occurrence and importance in the Atlantic Ocean. Environ. Sci. Technol. 42, 8675–8680. doi: 10.1021/es801884r
Meyer, J.-M., Gruffaz, C., Raharinosy, V., Bezverbnaya, I., Schäfer, M., and Budzikiewicz, H. (2008). Siderotyping of fluorescent pseudomonas: molecular mass determination by mass spectrometry as a powerful pyoverdine siderotyping method. Biometals 21, 259–271. doi: 10.1007/s10534-007-9115-6
Miki, W., Otaki, N., Yokoyama, A., Izumida, H., and Shimidzu, N. (1994). Okadaxanthin, a novel C50-carotenoid from a bacterium, pseudomonas sp. KK10206C associated with marine sponge, Halichondria okadai. Experiential 50, 684–686. doi: 10.1007/BF01952874
Mulet, M., Bennasar, A., Lalucat, J., and García-Valdés, E. (2009). An rpoD-based PCR procedure for the identification of pseudomonas species and for their detection in environmental samples. Mol. Cell. Probes 23, 140–147. doi: 10.1016/j.mcp.2009.02.001
Owen, J. G., and Ackerley, D. F. (2011). Characterization of pyoverdine and achromobactin in pseudomonas syringae pv. Phaseolicola 1448a. BMC Microbiol. 11:218. doi: 10.1186/1471-2180-11-218
Pang, Y., Zhang, Y., Chen, M., Lu, W., Chen, M., Yan, Y., et al. (2021). Pseudomonas nanhaiensis sp. nov., a lipase-producing bacterium isolated from deep-sea sediment of the South China Sea. Antonie Van Leeuwenhoek 114, 1791–1804. doi: 10.1007/s10482-021-01639-y
Park, B. S., Joo, J.-H., Baek, K.-D., and Han, M.-S. (2016). A mutualistic interaction between the bacterium pseudomonas asplenii and the harmful algal species Chattonella marina (Raphidophyceae). Harmful Algae 56, 29–36. doi: 10.1016/j.hal.2016.04.006
Petersen, L.-E., Kellermann, M. Y., and Schupp, P. J. (2020). “Secondary metabolites of marine microbes: from natural products chemistry to chemical ecology” in YOUMARES 9 - the oceans: Our research, our future. eds. S. Jungblut, V. Liebich, and M. Bode-Dalby (Cham: Springer International Publishing), 159–180.
Pocard, J. A., Smith, L. T., Smith, G. M., and Le Rudulier, D. (1994). A prominent role for glucosylglycerol in the adaptation of Pseudomonas mendocina SKB70 to osmotic stress. J. Bacteriol. 176, 6877–6884. doi: 10.1128/jb.176.22.6877-6884.1994
Pritchard, L., Glover, R. H., Humphris, S., Elphinstone, J. G., and Toth, I. K. (2016). Genomics and taxonomy in diagnostics for food security: soft-rotting enterobacterial plant pathogens. Anal. Methods 8, 12–24. doi: 10.1039/C5AY02550H
Ram, S., Mitra, M., Shah, F., Tirkey, S. R., and Mishra, S. (2020). Bacteria as an alternate biofactory for carotenoid production: A review of its applications, opportunities and challenges. J. Funct. Foods 67:103867. doi: 10.1016/j.jff.2020.103867
Ramos, J.-L. (2004). Pseudomonas: Volume 1 Genomics, Life Style and Molecular Architecture. Boston, MA: Springer US: Imprint: Springer.
Ravel, J., and Cornelis, P. (2003). Genomics of pyoverdine-mediated iron uptake in pseudomonads. Trends Microbiol. 11, 195–200. doi: 10.1016/S0966-842X(03)00076-3
Rokni-Zadeh, H., Li, W., Sanchez-Rodriguez, A., Sinnaeve, D., Rozenski, J., Martins, J. C., et al. (2012). Genetic and functional characterization of cyclic lipopeptide white-line-inducing principle (WLIP) production by rice rhizosphere isolate pseudomonas putida RW10S2. Appl. Environ. Microbiol. 78, 4826–4834. doi: 10.1128/AEM.00335-12
Ruangviriyachai, C., Uría Fernández, D., Schäfer, M., and Budzikiewicz, H. (2004). Structure proposal for a new pyoverdin from a Thai Pseudomonas putida strain 1. Spectroscopy 18, 453–458. doi: 10.1155/2004/394872
Rudra, B., and Gupta, R. S. (2021). Phylogenomic and comparative genomic analyses of species of the family Pseudomonadaceae: proposals for the genera Halopseudomonas gen. Nov. and Atopomonas gen. Nov., merger of the genus Oblitimonas with the genus Thiopseudomonas, and transfer of some misclassified species of the genus pseudomonas into other genera. IJSEM 71, 71. doi: 10.1099/ijsem.0.005011
Sagot, B., Gaysinski, M., Mehiri, M., Guigonis, J.-M., Le Rudulier, D., and Alloing, G. (2010). Osmotically induced synthesis of the dipeptide N-acetylglutaminylglutamine amide is mediated by a new pathway conserved among bacteria. Proc. Natl. Acad. Sci. U. S. A. 107, 12652–12657. doi: 10.1073/pnas.1003063107
Sasser, M. (2001). “Identification of bacteria by gas chromatography of cellular fatty acids,” in Technical Note 101 (Newark, Del.: Microbial ID, Inc).
Schöner, T. A., Fuchs, S. W., Reinhold-Hurek, B., and Bode, H. B. (2014). Identification and biosynthesis of a novel xanthomonadin-dialkylresorcinol-hybrid from Azoarcus sp. BH72. PLoS One 9:e90922. doi: 10.1371/journal.pone.0090922
Schöner, T. A., Gassel, S., Osawa, A., Tobias, N. J., Okuno, Y., Sakakibara, Y., et al. (2016). Aryl polyenes, a highly abundant class of bacterial natural products, are functionally related to antioxidative carotenoids. Chembiochem 17, 247–253. doi: 10.1002/cbic.201500474
Schwibbert, K., Marin-Sanguino, A., Bagyan, I., Heidrich, G., Lentzen, G., Seitz, H., et al. (2011). A blueprint of ectoine metabolism from the genome of the industrial producer Halomonas elongata DSM 2581T: Ectoine metabolism from the Halomonas genome. Environ. Microbiol. 13, 1973–1994. doi: 10.1111/j.1462-2920.2010.02336.x
Sedkova, N., Tao, L., Rouvière, P. E., and Cheng, Q. (2005). Diversity of carotenoid synthesis gene clusters from environmental Enterobacteriaceae strains. Appl. Environ. Microbiol. 71, 8141–8146. doi: 10.1128/AEM.71.12.8141-8146.2005
Seip, B., Galinski, E. A., and Kurz, M. (2011). Natural and engineered hydroxyectoine production based on the pseudomonas stutzeri ectABCD-ask gene cluster. Appl. Environ. Microbiol. 77, 1368–1374. doi: 10.1128/AEM.02124-10
Silpe, J. E., and Bassler, B. L. (2019). A host-produced quorum-sensing autoinducer controls a phage lysis-lysogeny decision. Cells 176, 268–280.e13. doi: 10.1016/j.cell.2018.10.059
Silpe, J. E., Bridges, A. A., Huang, X., Coronado, D. R., Duddy, O. P., and Bassler, B. L. (2020). Separating functions of the phage-encoded quorum-sensing-activated antirepressor qtip. Cell Host Microbe 27, 629–641.e4. doi: 10.1016/j.chom.2020.01.024
Silva-Junior, E. A. (2018). Pyrazines from bacteria and ants: convergent chemistry within an ecological niche. Sci. Rep. 8:2595. doi: 10.1038/s41598-018-20953-6
Sinha, R. K., Krishnan, K. P., Thomas, F. A., Binish, M. B., Mohan, M., and Kurian, P. J. (2019). Polyphasic approach revealed complex bacterial community structure and function in deep sea sediment of ultra-slow spreading southwest Indian ridge. Ecol. Indic. 96, 40–51. doi: 10.1016/j.ecolind.2018.08.063
Skinnider, M. A., Johnston, C. W., Edgar, R. E., Dejong, C. A., Merwin, N. J., Rees, P. N., et al. (2016). Genomic charting of ribosomally synthesized natural product chemical space facilitates targeted mining. Proc. Natl. Acad. Sci. U. S. A. 113, E6343–E6351. doi: 10.1073/pnas.1609014113
Stanborough, T., Fegan, N., Powell, S. M., Tamplin, M., and Chandry, P. S. (2018). Vibrioferrin production by the food spoilage bacterium Pseudomonas fragi. FEMS Microbiol. Lett. 365. doi: 10.1093/femsle/fnx279
Tamura, K., Peterson, D., Peterson, N., Stecher, G., Nei, M., and Kumar, S. (2011). MEGA5: molecular evolutionary genetics analysis using maximum likelihood, evolutionary distance, and maximum parsimony methods. Mol. Biol. Evol. 28, 2731–2739. doi: 10.1093/molbev/msr121
Tappe, R., Taraz, K., Budzikiewicz, H., Meyer, J.-M., and Lefèvre, J. F. (1993). Structure elucidation of a pyoverdin produced by Pseudomonas aeruginosa ATCC 27 853. J. Prakt. Chem. 335, 83–87. doi: 10.1002/prac.19933350113
Teintze, M., Hossain, M. B., Barnes, C. L., Leong, J., and Van der Helm, D. (1981). Structure of ferric pseudobactin: a siderophore from a plant growth promoting Pseudomonas. Biochemistry 20, 6446–6457. doi: 10.1021/bi00525a025
Van Mooy, B. A. S., Hmelo, L. R., Sofen, L. E., Campagna, S. R., May, A. L., Dyhrman, S. T., et al. (2012). Quorum sensing control of phosphorus acquisition in Trichodesmium consortia. ISME J. 6, 422–429. doi: 10.1038/ismej.2011.115
Viggor, S., Jõesaar, M., Vedler, E., Kiiker, R., Pärnpuu, L., and Heinaru, A. (2015). Occurrence of diverse alkane hydroxylase alkB genes in indigenous oil-degrading bacteria of Baltic Sea surface water. Mar. Pollut. Bull. 101, 507–516. doi: 10.1016/j.marpolbul.2015.10.064
Visca, P., Imperi, F., and Lamont, I. L. (2007). Pyoverdine siderophores: from biogenesis to biosignificance. Trends Microbiol. 15, 22–30. doi: 10.1016/j.tim.2006.11.004
Wang, M.-Q., Zhang, C.-S., Yu, L.-N., Yang, W.-Q., Jiao, K., Gong, K.-J., et al. (2021). Pseudomonas laoshanensis sp. nov., isolated from peanut field soil. Arch. Microbiol. 203, 829–834. doi: 10.1007/s00203-020-02067-8
Wasi, S., Tabrez, S., and Ahmad, M. (2013). Use of pseudomonas spp. for the bioremediation of environmental pollutants: a review. Environ. Monit. Assess. 185, 8147–8155. doi: 10.1007/s10661-013-3163-x
Wick, R. R., Judd, L. M., Gorrie, C. L., and Holt, K. E. (2017). Unicycler: resolving bacterial genome assemblies from short and long sequencing reads. PLoS Comput. Biol. 13:e1005595. doi: 10.1371/journal.pcbi.1005595
Wiklund, T. (2016). Pseudomonas anguilliseptica infection as a threat to wild and farmed fish in the Baltic Sea. Microbiol. Aust. 37:135. doi: 10.1071/MA16046
Wong, B.-T., and Lee, D.-J. (2014). Pseudomonas yangmingensis sp. nov., an alkaliphilic denitrifying species isolated from a hot spring. J. Biosci. Bioeng. 117, 71–74. doi: 10.1016/j.jbiosc.2013.06.006
Xu, J., Zeng, X., Jiang, N., Zhou, Y., and Zeng, L. (2015). Pseudomonas alcaligenes infection and mortality in cultured Chinese sturgeon, Acipenser sinensis. Aquaculture 446, 37–41. doi: 10.1016/j.aquaculture.2015.04.014
Yadav, A. N., Kour, D., Kaur, T., Devi, R., Yadav, A., Dikilitas, M., et al. (2021). Biodiversity, and biotechnological contribution of beneficial soil microbiomes for nutrient cycling, plant growth improvement and nutrient uptake. Biocatal. Agric. Biotechnol. 33:102009. doi: 10.1016/j.bcab.2021.102009
Ye, L., Ballet, S., Hildebrand, F., Laus, G., Guillemyn, K., Raes, J., et al. (2013). A combinatorial approach to the structure elucidation of a pyoverdine siderophore produced by pseudomonas putida isolate and the use of pyoverdine as a taxonomic marker for typing P. putida subspecies. Biometals 26, 561–575. doi: 10.1007/s10534-013-9653-z
Zawadzka, A. M., Vandecasteele, F. P. J., Crawford, R. L., and Paszczynski, A. J. (2006). Identification of siderophores of pseudomonas stutzeri. Can. J. Microbiol. 52, 1164–1176. doi: 10.1139/w06-077
Zhang, X., Lin, H., Wang, X., and Austin, B. (2018). Significance of vibrio species in the marine organic carbon cycle—A review. Sci. China Earth Sci. 61, 1357–1368. doi: 10.1007/s11430-017-9229-x
Appendix A: New species descriptions
Description of Pseudomonas chaetocerotis sp. nov. (protologue Table 2)
(chae.to.ce.ro’tis. N.L. gen. n. chaetocerotis of the diatom genus Chaetoceros)
The type strain is 536T (LMG 31766T = DSM 111343T) and was isolated from a culture of Chaetoceros calcitrans, Leucate, France in 2018. Its G + C content is 62.40 mol % (calculated based on its genome sequence). The 16 rRNA gene and whole genome sequence of 536T are publicly available through the accession numbers MW333026 and JACFYX000000000, respectively.
Description of Halopseudomonas laoshanensis comb. nov.
(lao.shan.en’sis. N.L. masc./fem. Adj. laoshanensis, of Laoshan mountain, referring to the geographical origin of the type strain)
Basonym: Pseudomonas laoshanensis.
The description of this species is as given by Wang et al. (2021) for Pseudomonas laoshanensis.
Type strain: Y22T = CGMCC 1.16552T = JCM 32580T = KCTC 62385T.
Description of Halopseudomonas nanhaiensis comb. nov.
(nan.hai.en’sis. N.L. masc./fem. Adj. nanhaiensis, pertaining to Nanhai, a sea in South China where the sample was isolated)
Basonym: Pseudomonas nanhaiensis.
The description of this species is as given by Pang et al. (2021) for Pseudomonas nanhaiensis.
Type strain: SCS 2-3T = GDMCC 1.2219T = JCM 34440T.
Description of Halopseudomonas yangmingensis comb. nov.
(yang.ming.en’sis. N.L. masc./fem. Adj. yangmingensis, pertaining to the Yang-Ming National Park, Taiwan, Republic of China, from where the organism was isolated)
Basonym: Pseudomonas yangmingensis.
The description of this species is as given by Wong and Lee (2014) for Pseudomonas yangmingensis.
Type strain: CRS1T = DSM 24213T.
Keywords: Pseudomonas chaetocerotis sp. nov., Pseudomonas, Halopseudomonas, Stutzerimonas, genome mining of natural products, phylogenetics
Citation: Girard L, Lood C, De Mot R, van Noort V and Baudart J (2023) Genomic diversity and metabolic potential of marine Pseudomonadaceae. Front. Microbiol. 14:1071039. doi: 10.3389/fmicb.2023.1071039
Edited by:
John R. Battista, Louisiana State University, United StatesReviewed by:
Graciela Dias, Federal University of Rio de Janeiro, BrazilMunusamy Madhaiyan, Temasek Life Sciences Laboratory, Singapore
Copyright © 2023 Girard, Lood, De Mot, van Noort and Baudart. This is an open-access article distributed under the terms of the Creative Commons Attribution License (CC BY). The use, distribution or reproduction in other forums is permitted, provided the original author(s) and the copyright owner(s) are credited and that the original publication in this journal is cited, in accordance with accepted academic practice. No use, distribution or reproduction is permitted which does not comply with these terms.
*Correspondence: Julia Baudart, YmF1ZGFydEBvYnMtYmFueXVscy5mcg==; Léa Girard, bGd2Lm1pY3JvYmlvbG9neS5jb25zdWx0aW5nQGdtYWlsLmNvbQ==