- 1Departamento de Microbiología, Laboratorio Tecnológico del Uruguay, Montevideo, Uruguay
- 2Latitud, Fundación LATU, Montevideo, Uruguay
- 3Instituto Nacional de Investigación Agropecuaria, INIA, Tacuarembó, Uruguay
- 4Departamento de Salud Pública, Facultad de Medicina, Universidad Nacional Autónoma de México, Mexico City, Mexico
- 5Instituto de Ciencia y Tecnología de Sistemas Alimentarios Sustentables, UEDD INTA-CONICET, Buenos Aires, Argentina
- 6Departamento de Bacteriología y Virología, Facultad de Medicina, Universidad de la República, Montevideo, Uruguay
Introduction: Shiga toxin-producing Escherichia coli (STEC) is a zoonotic pathogen that cause food-borne diseases in humans. Cattle and derived foodstuffs play a known role as reservoir and vehicles, respectively. In Uruguay, information about the characteristics of circulating STEC in meat productive chain is scarce. The aim was to characterize STEC strains recovered from 800 bovine carcasses of different slaughterhouses.
Methods: To characterize STEC strains we use classical microbiological procedures, Whole Genome Sequencing (WGS) and FAO/WHO risk criteria.
Results: We analyzed 39 STEC isolated from 20 establishments. They belonged to 21 different O-groups and 13 different H-types. Only one O157:H7 strain was characterized and the serotypes O130:H11(6), O174:H28(5), and O22:H8(5) prevailed. One strain showed resistance in vitro to tetracycline and genes for doxycycline, sulfonamide, streptomycin and fosfomycin resistance were detected. Thirty-three strains (84.6%) carried the subtypes Stx2a, Stx2c, or Stx2d. The gene eae was detected only in two strains (O157:H7, O182:H25). The most prevalent virulence genes found were lpfA (n = 38), ompA (n = 39), ompT (n = 39), iss (n = 38), and terC (n = 39). Within the set of STEC analyzed, the majority (81.5%) belonged to FAO/WHO’s risk classification levels 4 and 5 (lower risk). Besides, we detected STEC serotypes O22:H8, O113:H21, O130:H11, and O174:H21 belonged to level risk 2 associate with diarrhea, hemorrhagic colitis or Hemolytic-Uremic Syndrome (HUS). The only O157:H7 strain analyzed belonged to ST11. Thirty-eight isolates belonged to the Clermont type B1, while the O157:H7 was classified as E.
Discussion: The analyzed STEC showed high genomic diversity and harbor several genetic determinants associated with virulence, underlining the important role of WGS for a complete typing. In this set we did not detect non-O157 STEC previously isolated from local HUS cases. However, when interpreting this findings, the low number of isolates analyzed and some methodological limitations must be taken into account. Obtained data suggest that cattle constitute a local reservoir of non-O157 serotypes associated with severe diseases. Other studies are needed to assess the role of the local meat chain in the spread of STEC, especially those associated with severe diseases in humans.
1. Introduction
Shiga toxin-producing Escherichia coli (STEC) includes more than 1,000 different serotypes defined according to somatic “O” and flagellar “H” antigens combinations. The set of these serotypes associated with severe diseases in humans is classically called enterohemorrhagic E. coli (EHEC) (Croxen et al., 2013; FAO and WHO, 2019; Malahlela et al., 2022).
The human illnesses that STEC produce range from mild pathologies like watery diarrhea (WD), severe bloody diarrhea (BD) to life-threatening entities such as hemorrhagic colitis (HC) and hemolytic-uremic syndrome (HUS). The latter is defined by the presence of thrombocytopenia, mechanical hemolytic anemia and multi-organ ischemic damage (Rivas et al., 2016).
STEC strains can also be grouped into two serological categories, STEC O157 and STEC non-O157. Escherichia coli O157:H7 is the leading serotype of STEC isolated clinically, and generally produce more severe illnesses than non-O157:H7 STEC ones. In the last decade, non-O157 STEC strains with atypical virulent characteristics (e.g., eae negative) linked to cases of severe human diseases have been recovered worldwide. The main non-O157 serogroups in Europe include O26, O80, and O145, while in United States O26, O45, O103, and O111 are the most common [Hadler et al., 2011; EFSA and ECDC (European Food Safety Authority and European Centre for Disease Prevention and Control), 2022].
The global impact of STEC infection has been estimated in 43.1 acute cases per 100,000 persons, with 3,890 annual cases of HUS and more than 100 deaths (Majowicz et al., 2014). Centers for Disease Control and Prevention (CDC) reported that the incidence of STEC infection was 5.9 per 100,000 persons during 2018, a 26% increase over the incidence from 2015 to 2017 (Tack et al., 2019). New Zealand communicated a mean annual incidence of 0.8 per 100,000 persons (2.6/100,000 children under 5 years), France 0.49 per 100,000 persons (3.1/100.000 children under 5 years) and China 0.57 per 100,000 persons [0.38/100.000 children under 5 years; Vally et al., 2012; EFSA and ECDC (European Food Safety Authority and European Centre for Disease Prevention and Control), 2019; Feng et al., 2022]. On the other hand, Latin America has an endemic STEC infection, with most cases located in the south of the continent. In Uruguay it is estimated that occur between 10 and 15 HUS cases per year, and the incidence rate would be 0.5/100.000 inhabitants and 4 to 5/100,000 children under 5 years old (Varela et al., 2008). In neighboring Argentina, which has a robust surveillance system, HUS incidence during 2021 was 0.6/100.000 people and 5.95/100,000 children under 5 years of age, with a lethality of 1.7% (BEN 611-SE29 – Boletín epidemiológico nacional N611 SE29, 2022). It has been estimated that STEC infections in South America cause approximately 2% of acute diarrhea cases and 20–30% of BD (Torres et al., 2018).
As a result of their genetic plasticity, STEC of the same serotype may have different virulence profiles and represent different human health risks. This characteristic can also lead to the emergence of hybrid strains. In that sense, in 2011, a hybrid EAEC-STEC O104:H4 caused an important outbreak in Europe. Between 1992 and 2012, four EAEC-STEC hybrid strains were associated with small outbreak and sporadic cases of HUS (Koutsoumanis et al., 2020; Torti et al., 2021). This situation highlights the need to advance in the analysis and estimation of the virulent potential of local STEC strains (Rasko et al., 2011; Robins-Browne et al., 2016).
The ability of STEC to cause damage has been classically related to their capacity to produce different variants of the Shiga toxin (Stx). These variants are classified into two types: Stx1 (which consists of three variants Stx1a, Stx1c, and Stx1d) and Stx2 (that included variants Stx2a, Stx2b, Stx2c, Stx2d, Stx2e, Stx2f, and Stx2g). Stx1a, Stx2a, Stx2c, and Stx2d are linked to severe human diseases but there is no definitive or conclusive association, since it has been seen that the type of phage that contains the stx gene, the site where it was inserted and the combination of other genes can affect the virulence of STEC (Scheutz et al., 2012).
It has been postulated that without adherence of STEC to the intestinal epithelium, Stx production alone is considered insufficient to cause serious diseases. Therefore, Stx production and adhesion capacity would be important attributes to determining the course of STEC infections. The major adherence factor is intimin encoded by the eae gene, which is located in a pathogenicity island called LEE (Locus of Enterocyte Effacement). However, there are severe disease case reports caused by LEE-negative STEC (Newton, 2009). Thus, it is also interesting to evaluate STEC’s ability to produce human damage the presence of genes linked to other adhesion mechanisms: aggR, aaiC, saa, sab, paa, efa1, ompA, ompT, lpfA, toxB, the LAA island (Locus of Adhesion and Autoaggregation) using the hes gene as marker, as well as other virulence factors associated with adaptation and toxicity (Paton and Paton, 2002; Kaper et al., 2004; Herold et al., 2009; Montero et al., 2017).
Cattle is the principal reservoir of STEC strains (both, O157 and non-O157 ones), along with other ruminants such as sheep and goats, and from them STEC could be transmitted directly to humans, or through of foods, included meat and milk, or water contaminated with their feces (Farrokh et al., 2013; Menge, 2020). The presence of STEC in meat for human consumption is considered a hazard and has two clearly defined negative impacts. One, the most important, on human health and the other economically related to the losses determined by this production chain, especially in countries like Uruguay, where the majority of meat production is exported to increasingly demanding markets.
As far as we know, up to now there are no many local reports about the presence of STEC in bovine carcasses nor of its microbiological characteristics analyzed using WGS.
The aim of the study was to characterize phenotypically and genotypically a set of STEC strains recovered from bovine carcasses to have an initial approximation to knowledge of the circulating strains.
2. Materials and methods
2.1. Detection and isolation of STEC
For 2 years (August 2018–July 2020), covering the four seasons, samples of randomly selected half-carcasses of 37 abattoirs from all over the country (13 for internal supply and 24 for export; representing 88% of those enabled for bovine slaughter in Uruguay in 2018) were taken 24 h after sacrifice, with or without intervention (steam vacuum, water washing by arc with sprinklers, application of lactic acid 2–4% at room temperature), being cooled to 4°C. The number of samples per establishment was proportional to their participation in the 2017-year national slaughter, from a minimum of 1 sample to maximum 73. Between 1 and 7 abattoir visits were made.
Carcass swabs were obtained, following the methodology previously described by Brusa in 2017 (Brusa et al., 2017). Briefly, the total surface of the half-carcasses comprising both external and internal face, was covered with a pre moistened sponge (Whirl-Pak, Merck, Germany).
Stomacher bags containing the sponges were placed into coolers with ice and sent to be processed within 18 h. Upon arrival at the laboratory, 100 ml of pre-warmed modified Tryptone Soy Broth (mTSB; Neogen, United States) were added to the sponges and incubated at 42 ± 1°C for 18–22 h. All samples were analyzed by real time PCR using the BAX® System kit “STEC Screening assay for stx and eae” genes (Hygiena, United States).
If a positive signal for stx was detected samples (both, eae positive or eae negative) were streaked onto two MacConkey (MAC; Oxoid, United Kingdom) plates and, subsequently, in two consecutive eosin-methylene blue–Levine (EMB-Lev, Oxoid, United Kingdom) plates and incubated 37 ± 1°C for 18 h. The confluent growth zones were screened for the stx1, stx2, and eae genes by endpoint multiplex PCR (Paton and Paton, 1998). If the stx1 or stx2 genes were detected, up to 50 colonies per sample were selected for PCR confirmation.
Those samples that were simultaneously positive for both stx and eae genes, were also screened for the presence of E. coli O157:H7 using the BAX® System kit “Real time E. coli O157:H7” (Hygiena, United States). Positive samples were processed by immunomagnetic separation with Dynabeads® anti-E. coli O157 (Thermo Fisher Scientific, United States) following manufacturer’s instructions, and plated onto Sorbitol MAC agar supplemented with cefixime–potassium tellurite (CT-SMAC, Oxoid, United Kingdom) and onto CHROMagar™ O157 (Chromagar, France). Typical sorbitol negative colonies and mauve colored colonies, respectively, were agglutinated using the “E.coli O157 Latex Test Kit “(Thermo Scientific, United States).
Presumptive STEC strains were isolated on Trypticase soy agar (TSA, Oxoid, United Kingdom), and kept in 1 ml Trypticase soy broth (TSB, Oxoid, United Kingdom) with 0.5 ml of glycerol at-80°C for further phenotypic and genotypic characterization.
2.2. Phenotypic identification, antimicrobial susceptibility tests, and serotyping of STEC strains
Confirmation of isolates as E. coli was performed using the “NCCombo 66 panel” on the MicroScan autoSCAN-4 system (Beckman Coulter Inc., United States), according to the manufacturer’s instructions. “Neg MIC 44” panel was also used to determine the Minimum Inhibitory Concentration (MIC) to 33 antimicrobials (Beckman Coulter Inc, 2021). Disk diffusion susceptibility tests were made following the recommendations for Enterobacteriaceae of the Clinical and Laboratory Standards Institute (CLSI, 2019). The following 14 antibiotics were tested: ampicillin 10 μg (AMP10); cefuroxime 30 μg (CXM30); ceftriaxone 30 μg (CRO30); fosfomycin/trometamol 200 μg (FOT200); ceftazidime 30 μg (CAZ30); cefepime 30 μg (FEP30); imipenem 10 μg (IPM10); amoxicillin-clavulanic acid 30 μg (AMC30); cefoxitin 30 μg (FOX 30); trimethoprim-sulfamethoxazole 25 μg (SXT25); ciprofloxacin 5 μg (CIP 5); amikacin 30 μg (AK30); gentamicin 10 μg (CN10); and meropenem 10 μg (MEM10) (Oxoid, United Kingdom). Result interpretation was done according to CLSI breakpoints (Clinical and Laboratory Standards Institute, 2019). Escherichia coli ATCC 25922 was used as quality control.
Serotypes were determined at the Universidad Nacional Autónoma de México, using classical Ørskov and Ørskov′s agglutination assay with rabbit serum (SERUNAM, Mexico) obtained against 187 somatic antigens and 53 flagellar antigens of E. coli (Peirano et al., 2018).
2.3. Whole genome sequencing and genotypic characterization
The strains to be studied were thawed and inoculated into TSA agar plates. After an overnight incubation at 37 ± 1°C, five morphologically identical colonies of the pure culture were selected and genomic DNA was extracted and then purified using the DNeasy Blood & Tissue Kit (QIAGEN, Germany), following the manufacturer’s instructions. Genomic libraries were created with the TruSeq Nano DNA Kit – 350pb library (Illumina, United States) and sequencing was performed using the Illumina NovaSeq 150PE platform at Macrogen Inc. (Seoul, South Korea). The accession numbers to the sequences are available in Supplementary Table S1. Read quality was analyzed with FASTQC (Andrews, 2010) and low-quality positions were removed using Sickle (Joshi and Fass, 2011). Subsequently, the reads are assembled into contigs using Velvet in two steps; first velveth converts the reads into k-mers using a hash table, and secondly velvetg assembles the overlapping k-mers into contigs through a Bruijn graph discarding contigs with a length below the 250 bp (Zerbino and Birney, 2008). The web tools of the Center for Genomic Epidemiology (CGE) were used to evaluate the in silico molecular characterization of the sequenced strains. The multilocus sequence type was determined using MLST Finder 2.0 against the E. coli #1 set, including adenylate kinase (adk), fumarate hydratase (fumC), DNA gyrase (gyrB), isocitrate/isopropylmalate dehydrogenase (icd), malate dehydrogenase (mdh), adenylosuccinate dehydrogenase (purA), and ATP/GTP binding motif (recA) genes (Larsen et al., 2012). Virulence Finder 2.0 was used to search for virulence genes and ResFinder 4.1 was used to identify the AMR genes, setting an identity threshold of 90% with a minimum length protein of 60% (Camacho et al., 2009; Joensen et al., 2014; Zankari et al., 2017; Bortolaia et al., 2020). The stx subtyping and the following additional virulence factors were searched by in silico PCR, using IPCress (Slater and Birney, 2005) and the primers described in Table 1 for: Efa1 adherence factor (efa1), LAA Hemagglutinin (hes), outer membrane protein A (ompA), STEC autoagglutination adhesin (saa), an autotransporter that contributes to biofilm formation (sab), and an urease-associated protein (ureC).
Molecular serotyping was made with SerotypeFinder 2.0 using an identity threshold of 85% with a minimum length of 60% (Joensen et al., 2015).
Genomic sequences were also analyzed to determine phylogroups with ClermonTyping (Beghain et al., 2018; Clermont et al., 2019). Finally, the strains were classified with the FAO/WHO criteria to evaluate their potential risk (World Health Organization and Food and Agriculture Organization of the United Nations, 2018).
3. Results
3.1. Presence of stx genes in carcasses and selected STEC strains for a detailed analysis
From the 800 carcasses analyzed, 179 (22.3%, CI 95 19.5–25.3%) were positive to stx genes, representing 29 of the 37 (78.4%) slaughterhouses sampled 20 for export and nine for local supply (Figure 1). In 25 of them, at least one isolate was recovered. To continue with the characterization analysis, STEC strains from all the positive slaughterhouses were selected taking into account the number of stx positive samples from the screening of each establishment. However, in 5 of the them with positive isolates in the initial stage, it was not possible to continue with the characterization since when the strains were thawed they did not present a positive stx signal again. Of the 800 samples analyzed, only in 2 we recovered E. coli O157:H7 (prevalence 0.25%, CI 95 0–0.6%). Since both isolates came from to the same establishment, with the same sampling date and the same virulence profile (stx2c/eae), we opted for the WGS characterization of only one of the strains. All the 39 selected strains were identified as Escherichia coli using the MicroScan panels. Three isolates were unable to use sorbitol: U20 (O157:H7), U9 (O174:H21), and U22 (O-:H21).
3.2. Serotyping using classical procedure and serotype finder database
Serotyping of the isolates showed that the 39 WGS confirmed STEC strains belonged to 20 different O-groups and 13 different H types were identified, as well as one strain that was nontypeable (O-) (Table 2). Only one O157:H7 strain was included and among the 38 non-O157 isolates, the serotypes O130:H11 (6), O174:H28(5), and O22:H8 (5) prevailed. As can be seen in Table 2, there are some strains that could not be serotyped or gave inconsistent results. The isolates O182:H25 (U5), O88:H25 (U6), and O20:H7 (U32) were serotyped by Serotype Finder. Two isolates defined by agglutination test as O156 (U8, U27) could not be identified as such by the Serotype Finder. Some discrepancies could also be observed in the results obtained when assigning the serotype by agglutination or when doing so using the Serotype Finder. Strain U31 belonged to O159:H28 by agglutination and was molecularly identified as O130:H11, also the strain U39 was found to be O99:H19 by serology and was O10:H42 by aligning sequences with CGE databases.
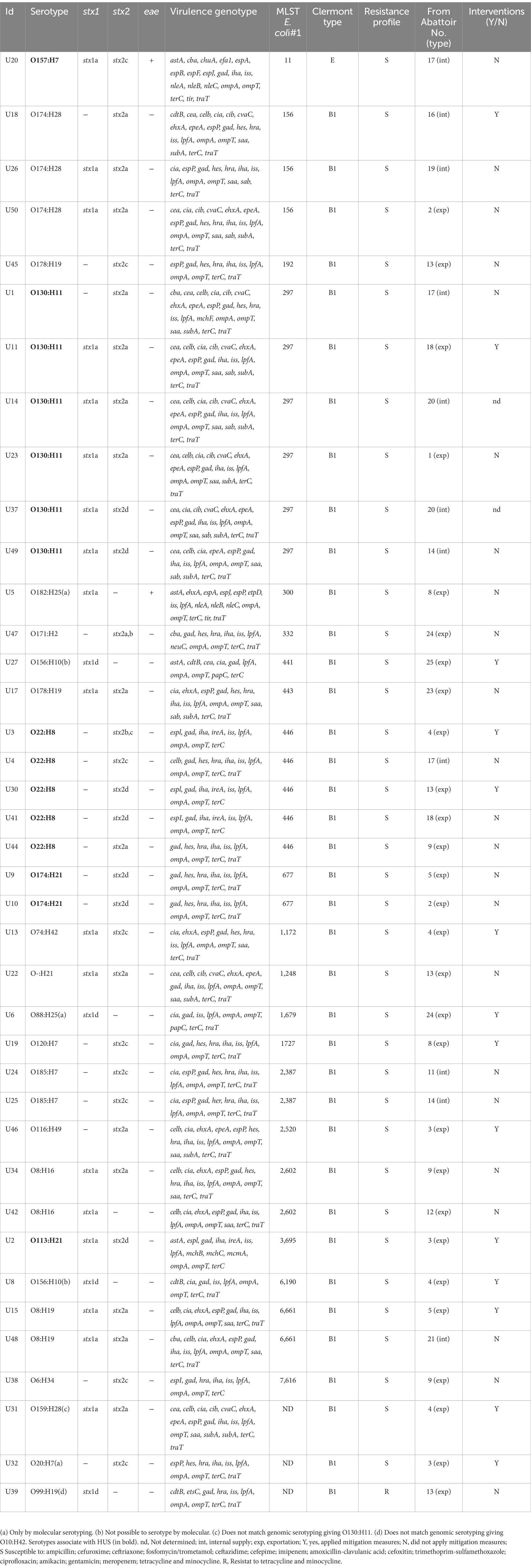
Table 2. Characteristics of STEC strains isolated in Uruguay between 2018 and 2020 from bovine carcasses.
3.3. Antibiotic susceptibility profiles and resistance genes
None of the 39 STEC strains analyzed showed resistance to the antimicrobials tested by disk diffusion (see the list of antibiotics tested by this procedure above). In one isolate O120:H7, the fosfomycin resistance gene fosA7 was detected with 94.1% of identity but no phenotypic resistance to this antibiotic was expressed. One O99:H19 strain had the resistance genes for: doxycycline, tetracycline, minocycline – tetB (100% identity), sulfonamides – sul2 (100% id) and streptomycin – aph(3″)-Ib and aph(6)-Id (100% id). Resistance to tetracycline (TE) and minocycline (MIN) was confirmed by Microscan showing MICs values >8 μg/ml to both. Resistance to doxycycline, streptomycin or sulfonamides was not tested by disk diffusion or MicroScan.
3.4. Types of stx genes and subtypes. Other virulence genes, phylogenetic grouping, and sequence typing
The stx subtypes carried by isolated strains were distinguished by in silico-PCR, showing that six strains (15%) carried stx1 gene only: stx1a (n = 2) and stx1d (n = 4). Seventeen strains (43%) carried stx2 only: stx2a (n = 4), stx2b (n = 2), stx2c (n = 7), and stx2d (n = 4). Sixteen strains (41%) harbored combinations of stx1 and stx2 genes: stx1a/stx2a (n = 11), stx1a/stx2c (n = 2), and stx1a/stx2d (n = 3). Thirty-three strains (84.6% of all isolates) carried the subtypes associated with high pathogenic potency, stx2a, stx2c, or stx2d.
Among the STEC strains investigated, a high variability of virulence gene profiles was identified (Table 2). One O130:H11 strain possessed the highest number of E. coli virulence-associated gene targets, with 21 genes detected. In addition to the stx2a gene, this strain presents genes associated with pathogenicity islands such as iha, and with plasmids epeA (pO113) and espP and ehxA (pO157). In relation to the amount of virulence factors, this strain is followed by three O174:H28 (20 virulence genes) and the O157:H7 with 19 virulence of the searched genes (Table 2). The virulence profile was examined in detail to detect hybrid strains searching for virulence markers founded in LEE-negative strains of animal or human origin, which were recognized in others pathotypes; ipaH as an indicator of enteroinvasive E. coli (EIEC), and aggR, aat, and aaiC as markers of enteroaggregative E. coli (EAEC). None of these genes was found in the 39 analyzed strains. The intimin coding gene eae was detected only in two strains, the O157:H7 and one O182:H25. The most prevalent genes found included those corresponding to adherence factors (Figure 2), such as lpfA coding long polar fimbriae (n = 38) and iha coding IrgA homolog adhesin (n = 30), outer membrane proteins ompA (n = 39) and ompT (n = 39); the serum resistance factor genes iss (n = 38) and traT (n = 34); the tellurite resistance gene terC (n = 39); the gene that codes for the glutamate decarboxylase gad (n = 36) and confers acid tolerance, and the bacteriocin colicin-Ia cia (n = 23). The iron utilization gene chuA was only present in the O157:H7 isolate (Table 2).
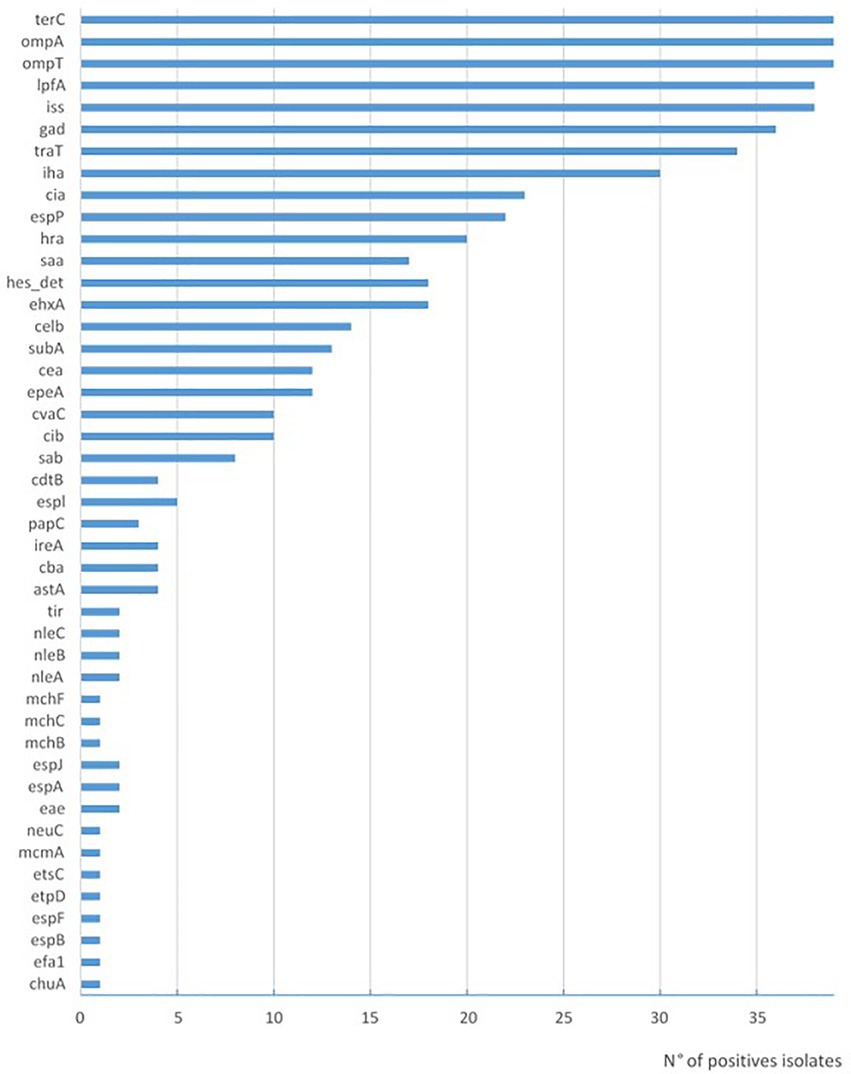
Figure 2. Prevalence of virulence genes in STEC strains isolated in Uruguay between 2018 and 2020 from chilled bovine carcasses.
The majority (n = 38, 97.4%) of the isolates included in this study belonged to the phylogenetic group (Clermont type) B1, while the O157:H7 was classified as E.
The list of identified STs by MLST Finder 2.0 is shown in Table 2. Strains of the same serogroup and the same ST did not necessarily shared the same virulotype, evidencing again the genomic plasticity of these bacteria. For example, the three O174:H28 isolates of ST156 present three different virulence profiles, the six strains O130:H11 ST297 are distributed in four profiles, like the five strains of O22:H8 ST446 (Table 2).
3.5. Risk groups
The distribution of the strains non-O157 in the risk groups indicated by the FAO/WHO, with the linked serogroups, were as follows: level 1 “stx2a + eae/aggR”: 0 isolated; Level 2 “stx2a”: 7 isolates (O22:H8{2}, O113:H21{1}, O130:H11{2}, O174:H21{2}); level 3”stx2c + eae”: none; level 4 “stx1a + eae”: 1 isolated (O182:H25); level 5 “other stx subtypes”: 30 isolates (Figure 3).
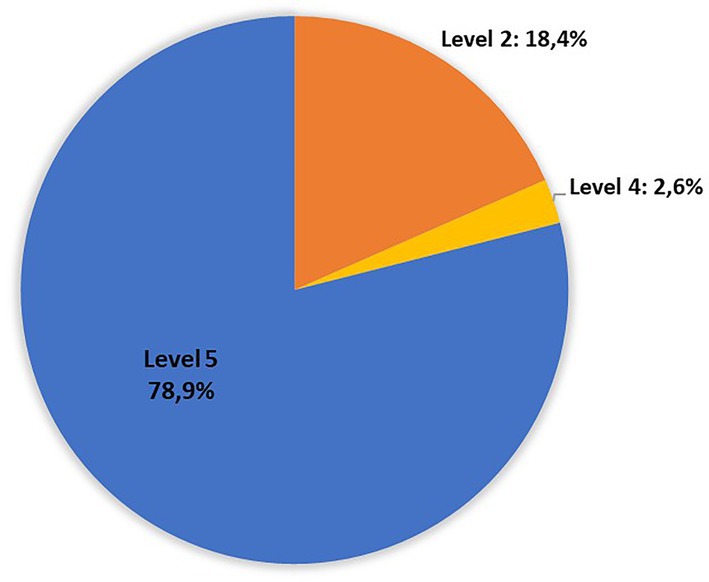
Figure 3. Distribution of non-O157 isolates recovered in Uruguay between 2018 and 2020 from chilled bovine carcasses in the risk levels defined by FAO/WHO: Level 1 “stx2a + eae/aggR”: 0 isolated; Level 2 “stx2a”: 7 isolates (O22:H8{2}, O113:H21{1}, O130:H11{2}, O174:H21{2}); Level 3”stx2c + eae”: none; Level 4 “stx1a + eae”: 1 isolated (O182:H25); Level 5 “other stx subtypes”: 30 isolates (World Health Organization and Food and Agriculture Organization of the United Nations, 2018).
4. Discussion
To our knowledge, this is the first local study on the presence of STEC in bovine carcasses ready to enter the production line, that includes the complete microbiological characteristics of the strains involved, such serotype, stx subtyping, sequence type, virulence and resistance genes.
In this work it was possible to estimate a 0.25% (CI 95 0–0.6%) prevalence of O157:H7 in chilled bovine carcasses (2 positives in 800 samples, both from the same internal supply slaughterhouse). Other published works reported prevalences of 2.7% in 258 refrigerated carcasses in Mexico (Varela-Hernández et al., 2007), 2.8% in 576 pre intervention carcasses on New Zealand (Stromberg et al., 2015), 3% in 132 carcasses in Ireland (Carney et al., 2006), 2.4% over 300 carcasses in Istanbul (Gun et al., 2003), 0.5% in a study carried out in Ethiopia with a similar sampling methodology as the used in our investigation (Abdissa et al., 2017). Also, a study carried out in export abattoirs in Argentina without post-slaughter interventions, reports a prevalence of 2.6% processed by immunomagnetic separation (Masana et al., 2011) and none O157:H7 was found in another study that analyzed pools of samples from 641 carcasses in an abattoir with a comprehensive HACCP design (Brusa et al., 2017). The lower prevalence found in our study may be due in part, to the fact that most STEC come from carcasses (500, 62%) of slaughterhouses that declared to apply some mitigation measures. Other types of study would be necessary to confirm or reject this hypothesis.
This study is the first experience in Uruguay working with this number of DNA sequences obtained by WGS of non-O157 STEC isolated from chilled bovine carcasses. Reports of these serotypes linked to human outbreaks have been increasing (Bettelheim and Goldwater, 2014). In Uruguay, in HUS cases with recovery of STEC, the serogroups O26, O111 and O145 predominate (Varela et al., 2008). In Argentina, with a robust surveillance system, non-O157 STEC are linked to 26% of HUS cases, with the most frequent serogroups being O145, O26, O121, and O103 (BIV 560 – Boletín integrado de Vigilancia N560 SE30, 2021). None of these serogroups were recovered in this work. This may be due to the low number of tested strains and some bias in their selection or to the fact that these strains are not as frequent in relation to other STEC in bovines. Another possible reason may be that we did not use immuno-concentration procedures nor chromogenic media that allow us to selectively identify them; or that its reservoir is not beef cattle. Also to that the STEC strains that produce HUS may come from other sources as indicated by EFSA and FAO/WHO (World Health Organization and Food and Agriculture Organization of the United Nations, 2018; Koutsoumanis et al., 2020).
Some of the serotypes analyzed corresponded to FAO/WHO level 2: O22:H8, O113:H21, O130:H11, and O174:H21. These serotypes have been previously isolated from patients with diarrhea, HC or HUS (Alonso et al., 2016; Commereuc et al., 2016; Oderiz et al., 2018). The knowledge of the origin of the STEC that cause severe disease in humans and their way of transmission continues to be a great question and therefore a challenge in studies related to these bacteria. Therefore, it would be of great importance to carry out continuous monitoring between strains isolated from humans and bovines as well as from other potential reservoirs to determine their relationship and eventual origin.
At the time of serotyping, we found some discordant results between both techniques used and some discrepancies could also be observed in the results obtained when assigning the serogroup by serology or when doing so using the Serotype Finder. The same occurred when Castro et al. compared PCR and WGS results to define E. coli serogroups (Castro et al., 2021). Some hypotheses to explain these discrepancies in our study are that we are dealing with new variants or new serogroups not yet described, and therefore not updated in the database used, or strains that cross-react with sera from other serogroups. Also, discrepant results may be due to sequence modifications in the O-antigen biosynthesis gene clusters (O-AGCs; Iguchi et al., 2015). More research could be done using new databases as they emerge.
Most of the strains (84%) carried stx2 alone or associated with stx1, which has been shown to be related with more virulent lineages. At the same time, Stx2a is epidemiologically associated with an increase of the excretion levels of STEC O157 from cattle and of the transmission between animals. It could be because it is more rapidly produced than other Stx subtypes and limits cellular proliferation of bovine epithelial cells (Galarce et al., 2021). Only two isolates had the eae gene and none carried aggR, two of the most relevant virulence markers. Ninety-four point nine % were LEE-negative, but had different adhesin-encoding genes, including lpfA, ehaA, and saa (Table 2). LpfA is the major subunit of the fimbrial protein, able to bind fibronectin, laminin, and collagen IV (Farfan et al., 2011). EhaA is an autotransporter protein related with biofilm and cellular aggregation; while Saa is involved in adhesion to HEp-2 cells (Montero et al., 2019). Moreover, some recently acquired pathogenicity islands (PAI) could collaborate to its adhesion, such as LAA. Some genes are used as markers for PAI modules, for example hes is a marker for module I and iha and lesP are markers for module II (Vélez et al., 2020). Parts of this PAI was identified in 76.9% of the strains. Its acquisition is probably a recent evolutionary event in STEC (Montero et al., 2017), which could have contributed to the emergence of highly virulent LEE-negative strains. Other toxin-encoding genes detected were ehxA (46% of the isolates), subA (33%), and cdtB (10%), the last two have only been detected in LEE-negative strains. The plasmid-encoded enterohemolysin (EhxA) has the ability to form pores in several eukaryotic cell membranes and is widely distributed in STEC strains (Hua et al., 2021). SubAB integrates the AB5 toxin family. It is highly toxic for a range of cell types, induces vacuolization, and has a synergic effect with Stx2 in human glomerular endothelial cells damage, contributing to the development of HUS (Tsutsuki et al., 2022). Last, CDT causes irreversible G2/M arrest, death and inhibition of proliferation of human endothelial cells (Vélez et al., 2020). We must emphasize that no hybrid strain was found in this study.
An O99:H19 strain carried the sul2 genes; tet(B); aph(3″)-Ib; and aph(6)-Id; responsible for resistance to sulfonamides, tetracycline and streptomycin, respectively. Tetracycline resistance was confirmed with the MicroScan system, which yielded a MIC value >8 μg/ml. This occurs most frequently by the acquisition of genes that code for ribosomal protection proteins, efflux pumps, or also by enzymatic inactivation (Thaker et al., 2010; Gasparrini et al., 2020). The presence of these resistance and the tetB gene in STEC has been previously described in isolates from Chile and Irish cattle (Galarce et al., 2021). Many of the genes involved in antibiotic resistance are located in mobile elements, which emphasizes the fact that STEC can serve also as reservoirs for resistance genes that could be passed to other pathogenic or commensal microorganisms. In another STEC, the O120:H7, the gene fosA7 (which confers resistance to fosfomycin) was found. The presence of this gene has been previously reported in E. coli and Salmonella spp. from animal and human origins (Gomi et al., 2017; Li et al., 2021), being an important concern in human health. Also, the presence of this gene in STEC from bovine feces and in a STEC O145:H25 isolated from an HUS case have been reported in our country (Mota et al., 2020; Umpiérrez et al., 2022).
The majority of STEC analyzed in this study (38/39) belonged to the B1 phylogroup, a finding similar to that previously reported in calves from Brazil (Coura et al., 2017). Escherichia coli belonging to the phylogroups A, B1, and D are the commensal and intestinal pathogenic strains, while the strains associated with extra-intestinal infection in humans belong to groups B2 and D. In animals, the predominance of B1 strains is informed, followed by A, B2 strains and, to a lesser extent, D (Ndegwa et al., 2022). The only O157:H7 strain included belonged to the phylogroup E. Although the E. coli strains of the found phylogroups (B1 and E) are associated with diseases in humans, it is very difficult to establish a link between phylogenetic groups and severity of infection in humans.
The ST297 was the most frequently detected. Strains of this ST were detected worldwide from non-human and human hosts and are associated with illness in humans and animals. This ST was predicted from genomes of STEC strains isolated from humans and beef in Chile and has been identified as the predominant lineage in cattle from Sweden (Carter et al., 2016), and in cattle, milk, pigs and water from farms in China (Peng et al., 2019). We also identified 5 isolates of ST446 (O22:H8), this serogroup was described to have a protector effect versus O157:H7 competing at the bovine recto-anal junction, making non-O157 carrying-calves less susceptible to O157:H7 colonization (Martorelli et al., 2017). Also the ST443, found in this study, was identified in cattle and beef samples from Chile and Uruguay before. The O157:H7 ST11 has been described as the principal clone identified from human infections in Argentina, Brazil, Paraguay and Uruguay and its expansion can be responsible for causing severe disease, as HUS (Vélez et al., 2020).
A quantitative risk assessment carried out in Argentine abattoirs that applied HACCP published in 2020, concludes that only 10% of HUS cases would be linked to beef consumption. In that work it is also inferred that the food with the highest risk is ground beef and that no case of HUS would be expected from the consumption of beef cuts (Brusa et al., 2020). It would be convenient for a similar study to be carried out in Uruguay.
It is interesting to see that strains of the same serogroup, ST and Clermont type were recovered from different establishments located in different regions. This may be due to the fact that several slaughterhouses are often supplied by the same animal breeders favoring the distribution of identical STEC strains to different establishments. Another particular case is that of the U14 and U37 strains, both are O130:H11 that come from the same slaughterhouse from different carcasses and on the same date but showed differences in their virulence profile. This may be due to the instability (by loss or gain) of genes encoding different virulence attributes and highlights the plasticity of the E. coli genome.
5. Conclusion
This is the first local work carried out with the aim of studying the STEC present in bovine carcasses, without performing any serogroup pre-selection, as is generally done in meat industry and control agencies. The idea was to have a notion of the diversity of the circulating STEC strains, their associated virulence factors, their resistance profile and estimate the potencial risk to produce severe human diseases.
Thus, 39 isolates were studied; one O157:H7 and 38 non-O157 corresponding to 19 different O-groups, resulting the most frequent serotypes: O130:H11 (six strains), O174:H28(5), and O22:H8 (5), all of them previously isolated from humans and animals/derived foods in other countries.
Using WGS it was possible to study the presence of multiple virulence factors, resistance genes and determine the sequence type and phylogroup of this set of STEC. This tool is still very expensive for us and is not routinely used for epidemiological surveillance nor to characterize this group of pathogens in our country. However, this work gave us the possibility to show its potential and obtain a lot of information that would have been very laborious to achieve with traditional PCR techniques.
Beyond the bias in the selection of the STEC analyzed, the obtained data suggest that cattle constitute a local reservoir of non-O157 serotypes associated with severe diseases in humans. In that sense, although most of the isolates studied belonged to the least risky FAO/WHO levels, some of the serotypes analyzed corresponding to level 2 (O22:H8, O113:H21, O130:H11, and O174:H21) were previously isolated in other countries from patients with diarrhea, HC or HUS. Other studies are needed to define the local participation of these serotypes in these human pathologies.
Anyway, the information gathered in the present study help to a better estimation of the risk from non-O157 STEC in the beef industry of Uruguay and American region. Since other vehicles can be involved in the local spread of STEC, studies similar to this, incorporating analysis by WGS, should be performed including a larger number of strains recovered form beef, other foods and environment to have a better epidemiological link to cases of human STEC infections.
Data availability statement
The data presented in the study are deposited in the NCBI repository, accession number of each strain is detailed on the supplementary files: https://dataview.ncbi.nlm.nih.gov/object/PRJNA914614?reviewer=4qmj8hptqcfl104prsud5h1ujs.
Author contributions
PM, IM, SL, GL, and GV contributed to conception and design of the study. PM performed the most of the analytical part and AN was the responsible for the serological serotyping of the strains. All authors contributed to manuscript revision, read, and approved the submitted version.
Funding
This work was supported by Agencia Nacional de Investigación e Innovación (ANII), Fondo Sectorial Innovagro – Inocuidad, Grant: FSA_I_2017_1_140224.
Acknowledgments
Felipe Schelotto for their contributions to the manuscript and technical assistance; Ana Caetano for their help in the preparation of reagents and culture media; Ana María Maquieira, Lucía Trujillo and Santiago Ultra from the Microbiology Department of LATU; Juan Manuel Burghi, María De La Paz Xavier and Carlos Méndez for their participation in sampling process. Stefano Morabito for his support and guidance.
Conflict of interest
The authors declare that the research was conducted in the absence of any commercial or financial relationships that could be construed as a potential conflict of interest.
Publisher’s note
All claims expressed in this article are solely those of the authors and do not necessarily represent those of their affiliated organizations, or those of the publisher, the editors and the reviewers. Any product that may be evaluated in this article, or claim that may be made by its manufacturer, is not guaranteed or endorsed by the publisher.
Supplementary material
The Supplementary material for this article can be found online at: https://www.frontiersin.org/articles/10.3389/fmicb.2023.1130170/full#supplementary-material
References
Abdissa, R., Haile, W., Fite, A. T., Beyi, A. F., Agga, G. E., Edao, B. M., et al. (2017). Prevalence of Escherichia coli O157:H7 in beef cattle at slaughter and beef carcasses at retail shops in Ethiopia. BMC Infect. Dis. 17:277. doi: 10.1186/s12879-017-2372-2
Alonso, M. Z., Krüger, A., Sanz, M. E., Padola, N. L., and Lucchesi, P. M. A. (2016). Serotypes, virulence profiles and stx subtypes of Shigatoxigenic Escherichia coli isolated from chicken derived products. Rev. Argent. Microbiol. 48, 325–328. doi: 10.1016/j.ram.2016.04.009
Andrews, S. (2010). FastQC: A Quality Control Tool for High Throughput Sequence Data. Available at: http://www.bioinformatics.babraham.ac.uk/projects/fastqc/ (Accessed December 12, 2022).
Bai, X., Zhao, A., Lan, R., Xin, Y., Xie, H., Meng, Q., et al. (2013). Shiga toxin-producing Escherichia coli in yaks (Bos grunniens) from the Qinghai-Tibetan plateau, China. PLoS ONE 8:e65537. doi: 10.1371/journal.pone.0065537
Beckman Coulter Inc (2021). https://media.beckmancoulter.com/-/media/diagnostics/products/microbiology/conventional-panels/docs/microscan-gram-neg-and-pos-panel-ous.pdf (Accessed December 12, 2022).
Beghain, J., Bridier-Nahmias, A., Le Nagard, H., Denamur, E., and Clermont, O. (2018). ClermonTyping: an easy-to-use and accurate in silico method for Escherichia genus strain phylotyping. Microb. Genom. 4:e000192. doi: 10.1099/mgen.0.000192
BEN 611-SE29 – Boletín epidemiológico nacional N611 SE29 (2022). https://bancos.salud.gob.ar/sites/default/files/2022-07/BEN-611-SE-29.pdf (Accessed December 12, 2022).
Bettelheim, K. A., and Goldwater, P. N. (2014). Serotypes of non-O157 Shigatoxigenic Escherichia coli (STEC). Adv. Microbiol. 4, 377–389. doi: 10.4236/aim.2014.47045
BIV 560 – Boletín integrado de Vigilancia N560 SE30 (2021). Available at: https://bancos.salud.gob.ar/sites/default/files/2021-09/biv_560_se_30_con_informe_SUH.pdf (Accessed December 12, 2022).
Bortolaia, V., Kaas, R. S., Ruppe, E., Roberts, M. C., Schwarz, S., Cattoir, V., et al. (2020). Res finder 4.0 for predictions of phenotypes from genotypes. J. Antimicrob. Chemother. 75, 3491–3500. doi: 10.1093/jac/dkaa345
Brusa, V., Costa, M., Padola, N. L., Etcheverría, A., Sampedro, F., Fernandez, P. S., et al. (2020). Quantitative risk assessment of haemolytic uremic syndrome associated with beef consumption in Argentina. PLoS One 15:e0242317. doi: 10.1371/journal.pone.0242317
Brusa, V., Restovich, V., Galli, L., Teitelbaum, D., Signorini, M., Brasesco, H., et al. (2017). Isolation and characterization of non-O157 Shiga toxin-producing Escherichia coli from beef carcasses, cuts and trimmings of abattoirs in Argentina. PLoS One 12:e0183248. doi: 10.1371/journal.pone.0183248
Camacho, C., Coulouris, G., Avagyan, V., Ma, N., Papadopoulos, J., Bealer, K., et al. (2009). BLAST+: architecture and applications. BMC Bioinformatics 10:421. doi: 10.1186/1471-2105-10-421
Carney, E., O’Brien, S. B., Sheridan, J. J., McDowell, D. A., Blair, I. S., and Duffy, G. (2006). Prevalence and level of Escherichia coli O157 on beef trimmings, carcasses and boned head meat at a beef slaughter plant. Food Microbiol. 23, 52–59. doi: 10.1016/j.fm.2004.12.001
Carter, M. Q., Quinones, B., He, X., Zhong, W., Louie, J. W., Lee, B. G., et al. (2016). An environmental Shiga toxin-producing Escherichia coli O145 clonal population exhibits high-level phenotypic variation that includes virulence traits. Appl. Environ. Microbiol. 82, 1090–1101. doi: 10.1128/AEM.03172-15
Castro, V. S., Ortega Polo, R., Figueiredo, E. E. D. S., Bumunange, E. W., McAllister, T., King, R., et al. (2021). Inconsistent PCR detection of Shiga toxin-producing Escherichia coli: insights from whole genome sequence analyses. PLoS One 16:e0257168. doi: 10.1371/journal.pone.0257168
Clermont, O., Dixit, O. V. A., Vangchhia, B., Condamine, B., Dion, S., Bridier-Nahmias, A., et al. (2019). Characterization and rapid identification of phylogroup G in Escherichia coli, a lineage with high virulence and antibiotic resistance potential. Environ. Microbiol. 21, 3107–3117. doi: 10.1111/1462-2920.14713
Clinical and Laboratory Standards Institute (2019). Performance Standards for Antimicrobial Susceptibility Testing. CLSI supplement M100. Table 2A, Enterobacteriaceae M02 and M07.
Commereuc, M., Weill, F.-X., Loukiadis, E., Gouali, M., Gleizal, A., Kormann, R., et al. (2016). Recurrent hemolytic and uremic syndrome induced by Escherichia coli. Medicine 95:e2050. doi: 10.1097/MD.0000000000002050
Coura, F. M., de Araújo Diniz, S., Mussi, J. M. S., Silva, M. X., Lage, A. P., and Heinemann, M. B. (2017). Characterization of virulence factors and phylogenetic group determination of Escherichia coli isolated from diarrheic and non-diarrheic calves from Brazil. Folia Microbiol. 62, 139–144. doi: 10.1007/s12223-016-0480-9
Croxen, M. A., Law, R. J., Scholz, R., Keeney, K. M., Wlodarska, M., and Finlay, B. B. (2013). Recent advances in understanding enteric pathogenic Escherichia coli. Clin. Microbiol. Rev. 26, 822–880. doi: 10.1128/CMR.00022-13
EFSA and ECDC (European Food Safety Authority and European Centre for Disease Prevention and Control) (2019). The European Union one health 2018 Zoonoses report. EFSA J. 17:e05926. doi: 10.2903/j.efsa.2019.5926
EFSA and ECDC (European Food Safety Authority and European Centre for Disease Prevention and Control) (2022). The European Union one health 2021 Zoonoses report. EFSA J. 20:e07666. doi: 10.2903/j.efsa.2022.7666
FAO and WHO. (2019). Attributing Illness Caused by Shiga Toxin-Producing Escherichia coli (STEC) to Specific Foods. Microbiological Risk Assessment Series no. 32. Rome: FAO
Farfan, M. J., Cantero, L., Vidal, R., Botkin, D. J., and Torres, A. G. (2011). Long polar fimbriae of enterohemorrhagic Escherichia coli O157:H7 bind to extracellular matrix proteins. Infect. Immun. 79, 3744–3750. doi: 10.1128/IAI.05317-11
Farrokh, C., Jordan, K., Auvray, F., Glass, K., Oppegaard, H., Raynaud, S., et al. (2013). Review of Shiga-toxin-producing Escherichia coli (STEC) and their significance in dairy production. Int. J. Food Microbiol. 162, 190–212. doi: 10.1016/j.ijfoodmicro.2012.08.008
Feng, J., Xu, K., Shi, X., Xu, L., Liu, L., Wang, F., et al. (2022). Incidence and cost of haemolytic uraemic syndrome in urban China: a national population-based analysis. BMC Nephrol. 23:122. doi: 10.1186/s12882-022-02746-2
Franz, E., van Hoek, A. H. A. M., Wuite, M., van der Wal, F. J., de Boer, A. G., Bouw, E., et al. (2015). Molecular Hazard identification of non-O157 Shiga toxin-producing Escherichia coli (STEC). PLoS One 10:e0120353. doi: 10.1371/journal.pone.0120353
Galarce, N., Sánchez, F., Escobar, B., Lapierre, L., Cornejo, J., Alegría-Morán, R., et al. (2021). Genomic epidemiology of Shiga toxin-producing Escherichia coli isolated from the livestock-food-human Interface in South America. Animals 11:1845. doi: 10.3390/ani11071845
Gasparrini, A. J., Markley, J. L., Kumar, H., Wang, B., Fang, L., Irum, S., et al. (2020). Tetracycline-inactivating enzymes from environmental, human commensal, and pathogenic bacteria cause broad-spectrum tetracycline resistance. Commun. Biol. 3:241. doi: 10.1038/s42003-020-0966-5
Gomi, R., Matsuda, T., Matsumura, Y., Yamamoto, M., Tanaka, M., Ichiyama, S., et al. (2017). Whole-genome analysis of antimicrobial-resistant and Extraintestinal pathogenic Escherichia coli in river. Water. Appl. Environ. Microbiol. 83:e02703-16. doi: 10.1128/AEM.02703-16
Gun, H., Yilmaz, A., Turker, S., Tanlasi, A., and Yilmaz, H. (2003). Contamination of bovine carcasses and abattoir environment by Escherichia coli O157:H7 in Istanbul. Int. J. Food Microbiol. 84, 339–344. doi: 10.1016/S0168-1605(02)00476-2
Hadler, J. L., Clogher, P., Hurd, S., Phan, Q., Mandour, M., Bemis, K., et al. (2011). Ten-year trends and risk factors for non-O157 Shiga toxin–producing Escherichia coli found through Shiga toxin testing, Connecticut, 2000–2009. Clin. Infect. Dis. 53, 269–276. doi: 10.1093/cid/cir377
Herold, S., Paton, J. C., and Paton, A. W. (2009). Sab, a novel autotransporter of locus of enterocyte effacement-negative Shiga-toxigenic Escherichia coli O113:H21, contributes to adherence and biofilm formation. Infect. Immun. 77, 3234–3243. doi: 10.1128/IAI.00031-09
Hua, Y., Zhang, J., Jernberg, C., Chromek, M., Hansson, S., Frykman, A., et al. (2021). Molecular characterization of the Enterohemolysin gene (ehxA) in clinical Shiga toxin-producing Escherichia coli isolates. Toxins 13:71. doi: 10.3390/toxins13010071
Iguchi, A., Iyoda, S., Seto, K., Morita-Ishihara, T., Scheutz, F., and Ohnishi, M. (2015). Escherichia coli O-genotyping PCR: a comprehensive and practical platform for molecular O serogrouping. J. Clin. Microbiol. 53, 2427–2432. doi: 10.1128/JCM.00321-15
Joensen, K. G., Scheutz, F., Lund, O., Hasman, H., Kaas, R. S., Nielsen, E. M., et al. (2014). Real-time whole-genome sequencing for routine typing, surveillance, and outbreak detection of verotoxigenic Escherichia coli. J. Clin. Microbiol. 52, 1501–1510. doi: 10.1128/JCM.03617-13
Joensen, K. G., Tetzschner, A. M. M., Iguchi, A., Aarestrup, F. M., and Scheutz, F. (2015). Rapid and easy in Silico serotyping of Escherichia coli isolates by use of whole-genome sequencing data. J. Clin. Microbiol. 53, 2410–2426. doi: 10.1128/JCM.00008-15
Joshi, N. A., and Fass, J. N. (2011). Sickle: A sliding-window, adaptive, quality-based trimming tool for FastQ files (Version 1.21) [Software]. Available at: https://github.com/najoshi/sickle (Accessed December 12, 2022).
Kaper, J. B., Nataro, J. P., and Mobley, H. L. (2004). Pathogenic Escherichia coli. Nat. Rev. Microbiol. 2, 123–140. doi: 10.1038/nrmicro818
Koutsoumanis, K., Allende, A., Alvarez-Ordóñez, A., Bover-Cid, S., Chemaly, M., Davies, R., et al. (2020). Scientific opinion on the pathogenicity assessment of Shiga toxin-producing Escherichia coli (STEC) and the public health risk posed by contamination of food with STEC. EFSA J. 18:5967. doi: 10.2903/j.efsa.2020.5967
Larsen, M. V., Cosentino, S., Rasmussen, S., Friis, C., Hasman, H., Marvig, R. L., et al. (2012). Multilocus sequence typing of total-genome-sequenced bacteria. J. Clin. Microbiol. 50, 1355–1361. doi: 10.1128/JCM.06094-11
Li, M., Wang, K., Tang, A., Tang, A., Chen, A., and Huang, Z. (2021). Investigation of the genes involved in the outbreaks of Escherichia coli and salmonella spp. in the United States. Antibiotics 10:1274. doi: 10.3390/antibiotics10101274
Majowicz, S. E., Scallan, E., Jones-Bitton, A., Sargeant, J. M., Stapleton, J., Angulo, F. J., et al. (2014). Global incidence of human Shiga toxin-producing Escherichia coli infections and deaths: a systematic review and knowledge synthesis. Foodborne Pathog. Dis. 11, 447–455. doi: 10.1089/fpd.2013.1704
Malahlela, M. N., Cenci-Goga, B. T., Marufu, M. C., Fonkui, T. Y., Grispoldi, L., Etter, E., et al. (2022). Occurrence, serotypes and virulence characteristics of Shiga-toxin-producing Escherichia coli isolates from goats on communal rangeland in South Africa. Toxins 14:353. doi: 10.3390/toxins14050353
Martorelli, L., Albanese, A., Vilte, D., Cantet, R., Bentancor, A., Zolezzi, G., et al. (2017). Shiga toxin-producing Escherichia coli (STEC) O22:H8 isolated from cattle reduces E. coli O157:H7 adherence in vitro and in vivo. Vet. Microbiol. 208, 8–17. doi: 10.1016/j.vetmic.2017.06.021
Masana, M. O., D’Astek, B. A., Palladino, P. M., Galli, L., Del Castillo, L. L., Carbonari, C., et al. (2011). Genotypic characterization of non-O157 Shiga toxin-producing Escherichia coli in beef abattoirs of Argentina. J. Food Prot. 74, 2008–2017. doi: 10.4315/0362-028X.JFP-11-189
Menge, C. (2020). The role of Shiga toxins in STEC colonization of cattle. Toxins 12:607. doi: 10.3390/toxins12090607
Montero, D. A., Canto, F. D., Velasco, J., Colello, R., Padola, N. L., Salazar, J. C., et al. (2019). Cumulative acquisition of pathogenicity islands has shaped virulence potential and contributed to the emergence of LEE-negative Shiga toxin-producing Escherichia coli strains. Emerg. Microbes. Infect. 8, 486–502. doi: 10.1080/22221751.2019.1595985
Montero, D. A., Velasco, J., Del Canto, F., Puente, J. L., Padola, N. L., Rasko, D. A., et al. (2017). Locus of adhesion and autoaggregation (LAA), a pathogenicity island present in emerging Shiga toxin-producing Escherichia coli strains. Sci. Rep. 7:7011. doi: 10.1038/s41598-017-06999-y
Mota, M. I., Vázquez, S., Cornejo, C., Alessandro, B., Braga, V., Caetano, A., et al. (2020). Does Shiga toxin-producing Escherichia coli and Listeria monocytogenes contribute significantly to the burden of antimicrobial resistance in Uruguay? Front. Vet Sci. 7:583930. doi: 10.3389/fvets.2020.583930
Ndegwa, E., O’Brien, D., Matthew, K., Wang, Z., and Kim, J. (2022). Shiga toxin subtypes, serogroups, phylogroups, RAPD genotypic diversity, and select virulence markers of Shiga-toxigenic Escherichia coli strains from goats in mid-Atlantic US. Microorganisms 10:1842. doi: 10.3390/microorganisms10091842
Newton, H. J. (2009). Shiga toxin–producing Escherichia coli strains negative for locus of enterocyte effacement. Emerg. Infect. Dis. 15, 372–380. doi: 10.3201/eid1503.080631
Oderiz, S., Leotta, G. A., and Galli, L. (2018). Detección y caracterización de Escherichia coli productor de toxina Shiga en niños atendidos en un hospital pediátrico interzonal de la ciudad de La Plata. Rev. Argent. Microbiol. 50, 341–350. doi: 10.1016/j.ram.2017.08.008
Paton, A. W., and Paton, J. C. (1998). Detection and characterization of Shiga toxigenic Escherichia coli by using multiplex PCR assays for stx 1, stx 2, eae a, enterohemorrhagic E. coli hly A, rfb O111, and rfb O157. J. Clin. Microbiol. 36, 598–602. doi: 10.1128/JCM.36.2.598-602.1998
Paton, A. W., and Paton, J. C. (2002). Direct detection and characterization of Shiga toxigenic Escherichia coli by multiplex PCR for stx1, stx2, eae, ehxA, and saa. J. Clin. Microbiol. 40, 271–274. doi: 10.1128/JCM.40.1.271-274.2002
Peirano, V., Bianco, M. N., Navarro, A., Schelotto, F., and Varela, G. (2018). Diarrheagenic Escherichia coli associated with acute gastroenteritis in children from Soriano, Uruguay. Can. J. Infect. Dis. Med. Microbiol. 8:8387218. doi: 10.1155/2018/8387218
Peng, Z., Liang, W., Hu, Z., Li, X., Guo, R., Hua, L., et al. (2019). O-serogroups, virulence genes, antimicrobial susceptibility, and MLST genotypes of Shiga toxin-producing Escherichia coli from swine and cattle in Central China. BMC Vet. Res. 15:427. doi: 10.1186/s12917-019-2177-1
Rasko, D. A., Webster, D. R., Sahl, J. W., Bashir, A., Boisen, N., Scheutz, F., et al. (2011). Origins of the E. coli strain causing an outbreak of hemolytic-uremic syndrome in Germany. N. Engl. J. Med. 365, 709–717. doi: 10.1056/NEJMoa1106920
Rivas, M., Chinen, I., and Guth, B. E. C. (2016). “Enterohemorrhagic (Shiga toxin-producing) Escherichia coli” in Escherichia coli in the Americas. ed. A. Torres (Cham: Springer), 97–123.
Robins-Browne, R. M., Holt, K. E., Ingle, D. J., Hocking, D. M., Yang, J., and Tauschek, M. (2016). Are Pathotypes still relevant in the era of whole-genome sequencing? Front. Cell. Infect. Microbiol. 6:141. doi: 10.3389/fcimb.2016.00141
Scheutz, F., Teel, L. D., Beutin, L., Piérard, D., Buvens, G., Karch, H., et al. (2012). Multicenter evaluation of a sequence-based protocol for subtyping Shiga toxins and standardizing Stx nomenclature. J. Clin. Microbiol. 50, 2951–2963. doi: 10.1128/JCM.00860-12
Slater, G. S. C., and Birney, E. (2005). Automated generation of heuristics for biological sequence comparison. BMC Bioinformatics 6:31. doi: 10.1186/1471-2105-6-31
Stromberg, Z. R., Baumann, N. W., Lewis, G. L., Sevart, N. J., Cernicchiaro, N., Renter, D. G., et al. (2015). Prevalence of enterohemorrhagic Escherichia coli O26, O45, O103, O111, O121, O145, and O157 on hides and preintervention carcass surfaces of feedlot cattle at harvest. Foodborne Pathog. Dis. 12, 631–638. doi: 10.1089/fpd.2015.1945
Tack, D. M., Marder, E. P., Griffin, P. M., Cieslak, P. R., Dunn, J., Hurd, S., et al. (2019). Preliminary incidence and trends of infections with pathogens transmitted commonly through food-foodborne diseases active surveillance network, 10 U.S. sites, 2015–2018. MMWR Morb. Mortal. Wkly Rep. 68, 369–373. doi: 10.15585/mmwr.mm6816a2
Thaker, M., Spanogiannopoulos, P., and Wright, G. D. (2010). The tetracycline resistome. Cell. Mol. Life Sci. 67, 419–431. doi: 10.1007/s00018-009-0172-6
Torres, A. G., Amaral, M. M., Bentancor, L., Galli, L., Goldstein, J., Krüger, A., et al. (2018). Recent advances in Shiga toxin-producing research in Latin America. Microorganisms 6:100. doi: 10.3390/microorganisms6040100
Torti, J. F., Cuervo, P., Nardello, A., and Pizarro, M. (2021). Epidemiology and characterization of Shiga toxin-producing Escherichia coli of hemolytic uremic syndrome in Argentina. Cureus 13:e17213. doi: 10.7759/cureus.17213
Tsutsuki, H., Zhang, T., Yahiro, K., Ono, K., Fujiwara, Y., Iyoda, S., et al. (2022). Subtilase cytotoxin from Shiga-toxigenic Escherichia coli impairs the inflammasome and exacerbates enteropathogenic bacterial infection. IScience 25:104050. doi: 10.1016/j.isci.2022.104050
Umpiérrez, A., Ernst, D., Cardozo, A., Torres, A., Fernández, M., Fraga, M., et al. (2022). Non-O157 Shiga toxin-producing Escherichia coli with potential harmful profiles to humans are isolated from the faeces of calves in Uruguay. Austral J. Vet. Sci. 54, 45–53. doi: 10.4067/S0719-81322022000200045
Vally, H., Hall, G., Dyda, A., Raupach, J., Knope, K., Combs, B., et al. (2012). Epidemiology of Shiga toxin producing Escherichia coli in Australia, 2000-2010. BMC Public Health 12:63. doi: 10.1186/1471-2458-12-63
Varela, G., Chinen, I., Gadea, P., Miliwebsky, E., Mota, M., González, S., et al. (2008). Detección y caracterización de Escherichia coli productor de toxina Shiga a partir de casos clínicos y de alimentos en Uruguay. Rev. Argent. Microbiol. 40, 93–100.
Varela-Hernández, J. J., Cabrera-Diaz, E., Cardona-López, M. A., Ibarra-Velázquez, L. M., Rangel-Villalobos, H., Castillo, A., et al. (2007). Isolation and characterization of Shiga toxin-producing Escherichia coli O157:H7 and non-O157 from beef carcasses at a slaughter plant in Mexico. Int. J. Food Microbiol. 113, 237–241. doi: 10.1016/j.ijfoodmicro.2006.06.028
Vélez, M. V., Colello, R., Etcheverría, A. I., Vidal, R. M., Montero, D. A., Acuña, P., et al. (2020). Distribution of locus of adhesion and autoaggregation and hes Gene in STEC strains from countries of Latin America. Curr. Microbiol. 77, 2111–2117. doi: 10.1007/s00284-020-02062-8
Wang, Y., Tang, C., Yu, W., Xia, M., and Yue, H. (2010). Distribution of serotypes and virulence-associated genes in pathogenic Escherichia coli isolated from ducks. Avian Pathol. 39, 297–302. doi: 10.1080/03079457.2010.495742
World Health Organization and Food and Agriculture Organization of the United Nations (2018). Shiga toxin-producing Escherichia coli (STEC) and food: attribution, characterization, and monitoring: report. World Health Organization. Available at: https://apps.who.int/iris/handle/10665/272871 (Accessed February 17, 2023).
Zankari, E., Allesøe, R., Joensen, K. G., Cavaco, L. M., Lund, O., and Aarestrup, F. M. (2017). Point finder: a novel web tool for WGS-based detection of antimicrobial resistance associated with chromosomal point mutations in bacterial pathogens. J. Antimicrob. Chemother. 72, 2764–2768. doi: 10.1093/jac/dkx217
Keywords: STEC, bovine carcasses, virulence factors, WGS, Uruguay
Citation: Mussio P, Martínez I, Luzardo S, Navarro A, Leotta G and Varela G (2023) Phenotypic and genotypic characterization of Shiga toxin-producing Escherichia coli strains recovered from bovine carcasses in Uruguay. Front. Microbiol. 14:1130170. doi: 10.3389/fmicb.2023.1130170
Edited by:
Vinicius Castro, University of Lethbridge, CanadaReviewed by:
Maxsueli Machado, Federal University of Rio de Janeiro, BrazilFrancesco Pomilio, Istituto Zooprofilattico Sperimentale dell'Abruzzo e del Molise, Italy
Copyright © 2023 Mussio, Martínez, Luzardo, Navarro, Leotta and Varela. This is an open-access article distributed under the terms of the Creative Commons Attribution License (CC BY). The use, distribution or reproduction in other forums is permitted, provided the original author(s) and the copyright owner(s) are credited and that the original publication in this journal is cited, in accordance with accepted academic practice. No use, distribution or reproduction is permitted which does not comply with these terms.
*Correspondence: Paula Mussio, cGF1bXVzc2lvQGdtYWlsLmNvbQ==; Gustavo Varela, Z3ZhcmVsYUBoaWdpZW5lLmVkdS51eQ==