- 1Department of Biotechnology and Food Science, Norwegian University of Science and Technology, Trondheim, Norway
- 2Section for Contaminants and Biohazards, Institute of Marine Research, Bergen, Norway
Aeromonas are widespread in aquatic environments and are considered emerging pathogens in humans and animals. Multidrug resistant (MDR) Aeromonas circulating in the aquatic environment and food production chain can potentially disseminate antimicrobial resistance (AMR) to humans via the foodborne route. In this study, we aimed to investigate AMR and virulence factors of 22 Aeromonas strains isolated from ready-to-eat (RTE) seafood. A multilocus phylogenetic analysis (MLPA) using the concatenated sequences of six housekeeping genes (gyrB, rpoD, gyrA, recA, dnaJ, and dnaX) in the 22 Aeromonas genomes and average nucleotide identity (ANI) analysis revealed eight different species; A. caviae, A. dhakensis, A. hydrophila, A. media, A. rivipollensis, A. salmonicida, A. bestiarum, and A. piscicola. The presence of virulence genes, AMR genes and mobile genetic elements (MGEs) in the Aeromonas genomes was predicted using different databases. Our data showed that the genes responsible for adherence and motility (Msh type IV pili, tap type IV pili, polar flagella), type II secretion system (T2SS) and hemolysins were present in all strains, while the genes encoding enterotoxins and type VI secretion system (T6SS) including major effectors were highly prevalent. Multiple AMR genes encoding β-lactamases such as cphA and blaOXA were detected, and the distribution of those genes was species-specific. In addition, the quinolone resistance gene, qnrS2 was found in a IncQ type plasmid of the A. rivopollensis strain A539. Furthermore, we observed the co-localization of a class I integron (intl1) with two AMR genes (sul1 and aadA1), and a Tn521 transposon carrying a mercury operon in A. caviae strain SU4-2. Various MGEs including other transposons and insertion sequence (IS) elements were identified without strongly associating with detected AMR genes or virulence genes. In conclusion, Aeromonas strains in RTE seafood were potentially pathogenic, carrying several virulence-related genes. Aeromonas carrying multiple AMR genes and MGEs could potentially be involved in the dissemination and spread of AMR genes to other bacterial species residing in the same environment and possibly to humans. Considering a One-Health approach, we highlight the significance of monitoring AMR caused by Aeromonas circulating in the food chain.
1. Introduction
Aeromonas are Gram-negative bacteria, ubiquitous in aquatic environments, including estuarine and brackish water (Martin-Carnahan and Joseph, 2005). Psychrophilic A. salmonicida and some mesophilic Aeromonas are responsible for fish diseases such as furunculosis and motile Aeromonas septicemia (MAS), while many species are opportunistic human pathogens (Janda and Abbott, 2010; Beaz-Hidalgo and Figueras, 2013). The genus Aeromonas comprises at least 31 species, and among them, A. hydrophila, A. caviae, and A. veronii are the major species that frequently involved in human gastroenteritis and extraintestinal infections (Fernández-Bravo and Figueras, 2020). Although the role of Aeromonas as a true enteropathogen has been controversial, several studies have suggested that more attention should be given to the genus Aeromonas as emerging foodborne pathogens (Teunis and Figueras, 2016; Wu et al., 2018; Hoel et al., 2019). Aeromonas have occasionally been recognized as the source of foodborne outbreaks (Zhang et al., 2012; Ventura et al., 2015). The occurrence of mesophilic Aeromonas in water and food including ready-to-eat seafood (RTE) has frequently been reported, and their pathogenic potential has been determined based on the analysis of virulence-associated toxin genes (Pablos et al., 2011; Hoel et al., 2017; Lee et al., 2021). The pathogenesis of Aeromonas is complex and multifactorial as several virulence factors related to adherence, motility, secretion, and toxins are involved (Tomás, 2012; Fernández-Bravo and Figueras, 2020).
Antimicrobial resistance (AMR) is an emerging threat to public health around the globe. The extensive use and abuse of antimicrobial drugs for humans and animals, and the spread of resistant bacteria within and between these sectors and the environment, has contributed to the increased emergence and spread of AMR (Mcewen and Collignon, 2018). Resistant bacteria residing in the food chain can spread to humans via the foodborne route (Founou et al., 2016; EFSA Panel on Biological Hazards (BIOHAZ), 2021). In the food chain, food animals including fish, vegetables, as well as food-producing environments are considered important reservoirs of resistant bacteria (EFSA Panel on Biological Hazards (BIOHAZ), 2021). Bacteria can be intrinsically resistant or acquire new resistance mechanisms by obtaining genetic materials located in mobile genetic elements (MGEs) such as plasmids or transposons, where the latter phenomenon is known as horizontal gene transfer (HGT; Piotrowska and Popowska, 2015; Founou et al., 2016).
The usage of antimicrobial drugs for humans and animals in Norway is very restrictive compared to most other countries (NORM/NORM-VET, 2020). Only two antimicrobial agents are legally used in aquaculture in Norway, and a substantial decrease in antimicrobial usage has been implemented in aquaculture since the top in 1987 (Love et al., 2020). However, antibiotic residues can reach aquatic environments, not only through use in aquaculture, but also through routes like agricultural run-off or improper wastewater treatment (Sanseverino et al., 2018). The occurrence of multidrug resistant (MDR) Aeromonas strains has been reported from RTE seafood on the Norwegian market (Lee et al., 2021) as well as other types of food including marine bivalves (Stratev and Odeyemi, 2016; Albini et al., 2022). In addition, Aeromonas have been shown to carry multiple AMR genes as well as MGEs, implying their potential to transfer AMR genes to other bacterial species (Piotrowska and Popowska, 2015; Dubey et al., 2022a,b).
Nevertheless, available information on AMR of Aeromonas is limited since it is based on phenotypic resistance patterns, or the screening of target AMR genes. Thus, the characterization of AMR genes and MGEs of MDR Aeromonas would be necessary to understand their resistance mechanisms and assess their potential to spread AMR genes. With the development of whole genome sequencing (WGS) technology and bioinformatics tools, it is possible to perform a more comprehensive analysis on obtaining AMR and virulence gene profiles in bacterial genomes. In recent years, comparative genomic analysis on the AMR or virulence genes has enabled us to evaluate the potential role of Aeromonas in spreading AMR to other bacteria, as well as to predict the pathogenic potential of the Aeromonas (Dubey et al., 2022a,b; Erickson et al., 2023; Song et al., 2023). However, to our knowledge, such analysis has not been conducted on the Aeromonas residing in the food chain, particularly in relation to RTE seafood. Therefore, in this study, whole genome sequences of 22 Aeromonas isolated from RTE seafood were obtained to explore the presence of all AMR and virulence genes in their genomes. In addition, the presence of MGEs was examined to investigate their potential to disseminate AMR or virulence genes to other bacterial species.
2. Materials and methods
2.1. Bacterial collection
In total, 79 Aeromonas isolates were investigated in this study. Among the 79 isolates, 26 were previously isolated from retail sushi products (Hoel et al., 2015), and 43 were isolated from different types of RTE seafood including retail sushi, salmon loins, oysters, and scallops (Lee et al., 2021). All of these 69 isolates were identified as Aeromonas spp. based on partial gyrB gene sequencing in previous studies (Hoel et al., 2017; Lee et al., 2021). In addition, ten presumptive Aeromonas isolates originating from a salmon processing environment (SPE) were donated by Thomassen et al. (2022, 2023), and subjected to gyrB gene sequencing for species identification in the present study.
2.2. Species identification by partial gyrB gene sequencing
Genomic DNA was extracted from 1 mL of overnight cultures grown in Tryptone Soy Broth (TSB; Oxoid, Oslo, Norway) at 37°C using the protocol for Gram-negative bacteria in the GeneJET Genomic DNA Purification Kit (Thermo Fisher Scientific, Oslo, Norway). For PCR amplification, the primers gyrB3F (5′-TCCGGCGGTCTGCACGGCGT-3′) and gyrB14R (5′-TTGTCCGGGTTGTACTCGTC-3′) were used to amplify an approximately 1,100 bp gyrB gene (Yáñez et al., 2003). All PCR reactions were performed with 25 μL containing 1 x PCR buffer (1.5 mM MgCI2), 200 μM of each nucleotide, 0.4 μM each primer, 2.5 U Taq polymerase (Qiagen, Oslo, Norway) and 50–100 ng DNA template. PCR amplification was as follows: initial denaturation at 95°C for 15 min, 35 cycles of denaturation at 95°C for 30 s, annealing at 52°C for 30 s, and extension at 72°C for 60 s, followed by a final extension at 72°C for 7 min. PCR products were visualized by electrophoresis in a 1.5% agarose gel (SeaKem, Lonza Group Ltd., Basel, Switzerland) in 1 × TAE buffer. PCR products were purified with the GeneJET PCR Purification Kit (Thermo Fisher Scientific). DNA sequencing was performed by Eurofins Genomics (Ebersberg, Germany), and the sequences were compared with available sequences (> 97% nucleotide BLAST similarity) in the GenBank database in the National Center for Biotechnology Information (NCBI). A phylogenetic analysis was conducted based on the gyrB gene sequence of the 79 isolates and 11 relevant reference strains by the neighbor-joining (NJ) method with bootstrapping (1,000 replicates) using MEGA 11 version 11.0.10 (Tamura et al., 2021), according to the method described by Lee et al. (2021). The list of the reference strains is shown in Supplementary Table S1A.
2.3. Antimicrobial susceptibility testing (AST)
Among the 79 isolates, AST was performed for 36 isolates from Hoel et al. (2015) and Thomassen et al. (2022, 2023), while the antimicrobial susceptibility profile of 43 Aeromonas isolates was obtained from the previous study by Lee et al. (2021). AST was conducted by a disk diffusion method according to the recommendation of the Clinical and Laboratory Standards Institute (CLSI, 2016). In brief, the fresh inoculum of each isolate was evenly spread by a sterile cotton swab on Mueller-Hinton agar (MHA; Oxoid, Oslo, Norway). A maximum of five antibiotic disks were placed on each plate on the surface before incubation at 35°C for 16–18 h. After incubation, the diameter of the inhibition zone around the disks was measured, and the degree of susceptibility was categorized as sensitive, intermediate, or resistant according to CLSI criteria (CLSI, 2014, 2016). The resistance pattern of each isolate was examined against 15 antimicrobials belonging to 9 antimicrobial classes including aminoglycosides: gentamicin (10 μg) and tobramycin (10 μg), amphenicols: florfenicol (30 μg) and trimethoprim/sulfamethoxazole (1.25/23.75 μg), carbapenems: imipenem (10 μg) and meropenem (10 μg), cephalosporins: cefotaxime (30 μg) and ceftriaxone (30 μg), macrolides: erythromycin (15 μg) and oxolinic acid (2 μg), penicillin: ampicillin (10 μg) and mecillinam (10 μg), quinolones: ciprofloxacin (5 μg), tetracyclines: doxycycline (30 μg) and tetracycline (30 μg; Oxoid). Based on the antimicrobial susceptibility pattern, MDR strains were defined as being resistant to at least one antimicrobial agent in three or more antimicrobial classes (Magiorakos et al., 2012).
2.4. WGS and genome assembly
To select the isolates of interest for WGS analysis, the 79 Aeromonas isolates were first divided into eight different groups representing eight different Aeromonas species based on the gyrB gene sequences. Depending on their source of isolation and antimicrobial susceptibility pattern, 22 Aeromonas isolates were chosen for WGS. Total genomic DNA was extracted from 1 mL of overnight cultures grown in TSB (Oxoid) at 37°C, using the Genomic Micro AX Bacteria Gravity kit (A&A biotechnology, Poland) according to the manufacturer’s protocol. The quality of the DNA was checked on agarose gel, and DNA concentrations were estimated by spectrophotometric measurement using BioTek PowerWave XS (Winooski, VT, United States), Take3 plate and Gen5 2.0 software (BioTek Instruments Inc., Winooski, VT, United States). DNA samples were shipped on ice overnight to the Norwegian Sequencing Center (Ullevål University Hospital, Oslo, Norway) for WGS. Sequencing libraries were prepared using the Nextera DNA Flex Library Prep kit (Illumina, United States). Sequencing was performed using Illumina MiSeq platform (Illumina, United States), with 2 × 300 bp chemistry. The raw paired-end reads were cleaned and quality trimmed using BBDuk1 and assembled using SPAdes v3.15.4 (Bankevich et al., 2012). Draft genome assemblies were annotated using the NCBI Prokaryotic Genome Annotation Pipeline (Tatusova et al., 2016). The genome assemblies of the 22 isolates were deposited in the NCBI Genbank with accession numbers (JAOPLB000000000-JAOPLW000000000) (Supplementary Table S1B).
2.5. Multilocus phylogenetic analysis (MLPA) and average nucleotide identity (ANI) analysis
The housekeeping gene sequences of 10 reference strains (either type strains or representative strains) were retrieved from NCBI Genbank (Supplementary Table S1A). For MLPA, six housekeeping gene sequences (gyrB, rpoD, gyrA, recA, dnaJ, and dnaX) were extracted from each of the 22 Aeromonas genomes by blasting the nucleotide sequences of 10 reference strains against the assembled genomes. Multiple sequence alignments of each housekeeping gene were performed using CLUSTAL W (Thompson et al., 1994) implemented in MEGA11. The aligned sequences were then concatenated and re-aligned to concatenated sequences (4,172 bp). Genetic distances between the sequences were calculated using Kimura’s two-parameter model (Kimura, 1980) and a phylogenetic tree was constructed by the NJ method with bootstrapping (1,000 replicates) using MEGA 11 (Tamura et al., 2021). To confirm the tree topology, a phylogenetic tree was also created using the maximum-likelihood (ML) method with bootstrapping (100 replicates). To verify the taxonomy, ANI values between the genomes including 22 isolates and eight reference strains were calculated using FastANI (Jain et al., 2018). ANI matrix was clustered by scripy’s UPGMA and an ANIclustermap was created using the ANIclustermap pipeline (Shimoyama, 2022). The list of the reference genomes used for ANI analysis is included in Supplementary Table S1C.
2.6. Prediction of virulence genes, AMR genes, and MGEs
Genes associated with pathogenic bacteria virulence factors were identified by using VFanalyzer based on the virulence factors database (VFDB; Liu et al., 2019), where the threshold for virulence factor detection was set at 80%. The profile of virulence factors was visualized using the package pheatmap in R studio v.4.2.2 (R Core Team, 2022). The presence of AMR genes in bacterial genomes was predicted using the NCBI AMRFinderPlus v3.10.45 (Feldgarden et al., 2019), and ResFinder v4.1 (Bortolaia et al., 2020) with default settings (the threshold for AMR gene identification was set at 90%). PlasmidFinder v2.1 (Carattoli et al., 2014) was used to identify plasmids in the genome assemblies where the threshold for plasmid identification was set at 80%. MGEs were identified using MobileElementFinder v1.0.3 (Johansson et al., 2021) with default settings (minimum sequence identity of 90%). Circular maps of chromosomes and plasmids were visualized using the Proksee software (Grant et al., 2023).
3. Results
3.1. MLPA and ANI value
The phylogenetic tree based on the gyrB gene sequences (a continuous stretch of 929 bp) of the 79 isolates and relevant reference strains was constructed by the NJ method for species identification (Supplementary Figure S1). The MLPA of the 22 Aeromonas isolates selected for WGS and relevant reference strains was performed based on the concatenated sequences (4,172 bp) of six housekeeping genes (gyrB, rpoD, gyrA, recA, dnaJ and dnaX) by the NJ method (Figure 1). An identical tree-topology was obtained by the phylogenetic analysis using the ML method, confirming the robustness of the NJ tree (data not shown). The constructed tree comprised two main clusters; one cluster included the species A. salmonicida, A. piscicola, A. bestiarum, and A. popoffii, and the other cluster included the species A. hydrophila, A. dhakensis, A. caviae, A. media, A. rivipollensis, and A. paramedia [one of the A. media species complex suggested by Talagrand-Reboul et al. (2017)]. All isolates of interest clustered to the respective reference strains. In addition, the same clustering of the isolates to the reference strains, with one exception, was observed in further phylogenetic analysis based on each of the housekeeping gene sequence gyrB (908 bp), rpoD (657 bp), gyrA (709 bp), recA (598 bp), dnaJ (800 bp) and dnaX (500 bp; data not shown). One exception was the clustering of SU58-3 with A. piscicola observed in the tree based on the dnaX gene sequence, while each of the other five gene sequences of SU58-3 clustered with A. bestairum.
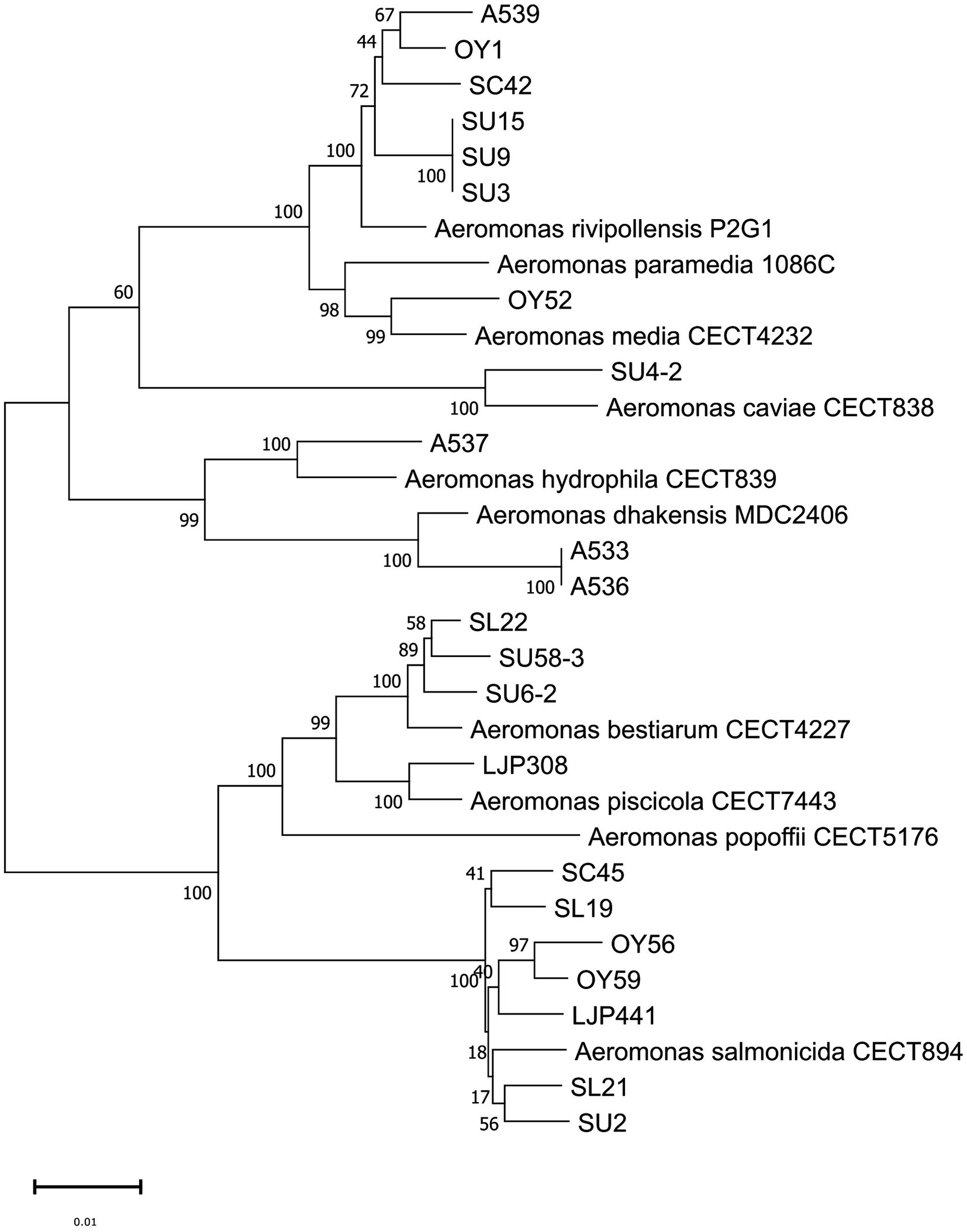
Figure 1. Multilocus phylogenetic analysis (MLPA) based on the concatenated sequence of six housekeeping genes (gyrB, rpoD, gyrA, recA, dnaJ, and dnaX, in total 4,172 bp) by the neighbor-joining method showing the relationship of 22 Aeromonas isolates and 10 reference strains. Numbers at nodes indicate bootstrap values (1,000 replicates) and the scale bar, 0.01 indicates the evolutionary distance of nucleotide substitution per site. The sequence data of the reference strains was retrieved from NCBI Genbank (Supplementary Table S1).
Considering the ANI cutoff value of ≥ 96% for strains belonging to the same species, the ANI values between the 22 isolates and reference genome (Figure 2) supported the phylogenetic clustering observed in the tree shown in Figure 1. Our ANI analysis between 22 isolates and reference genomes confirmed the eight different species among our isolates. In addition, an ANI value of 99.9–100% was observed between the two isolates A533 and A536 from retail sushi product in 2015, as well as among the three isolates SU3, SU9 and SU15 from retail sushi in 2019, indicating that those isolates share high enough genomic similarity to be considered identical strains. Thus, only one genome representing the identical strains (A533 and SU3, respectively) was included in the final dataset of 19 Aeromonas strains for the prediction of virulence and AMR genes.
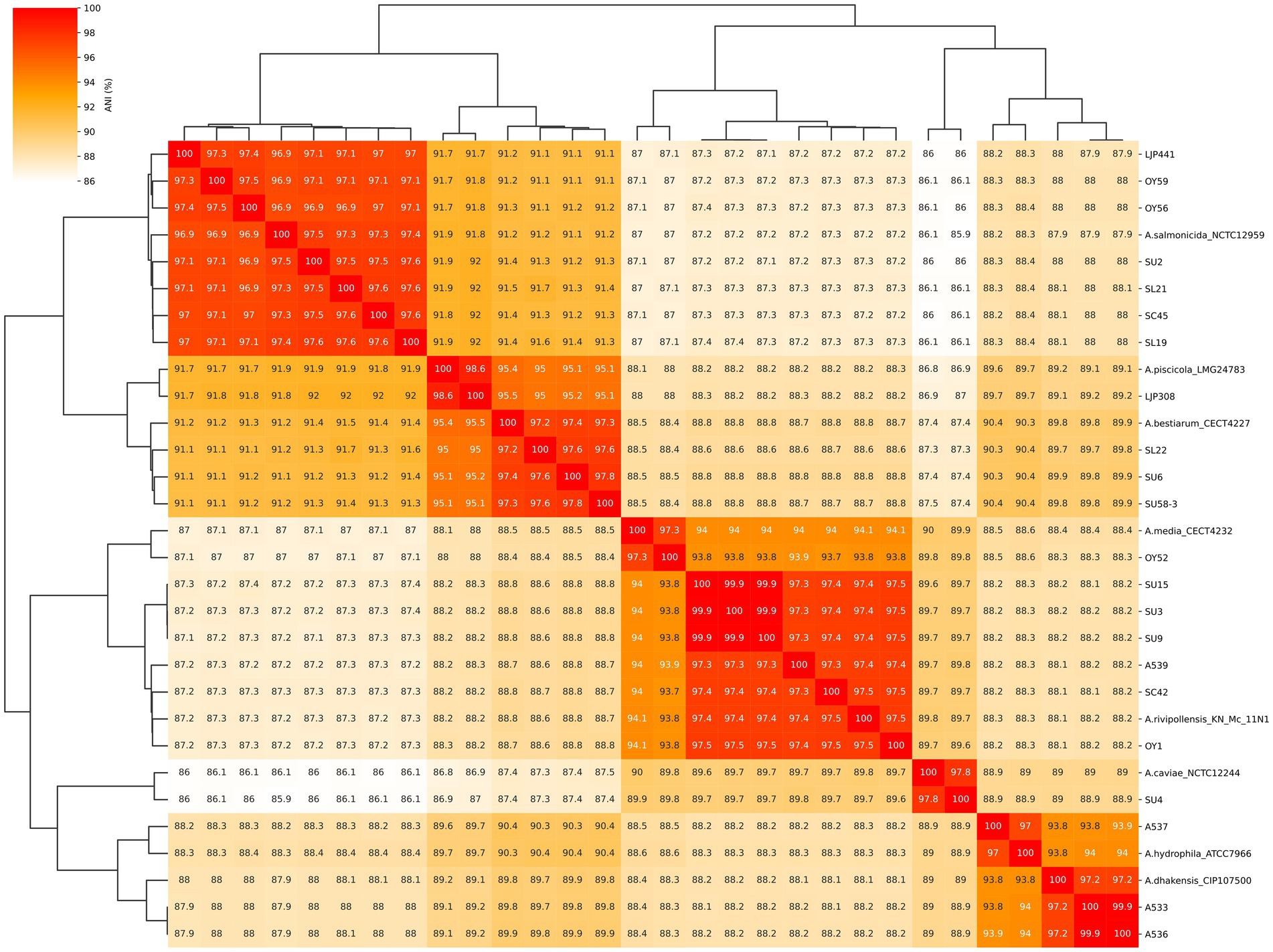
Figure 2. Heatmap of average nucleotide identity (ANI) values among the 22 Aeromonas isolates and 8 reference genome assemblies. The numbers represent the ANI values (%) between two genome sequences. The reference strains (NCBI Genbank accession number) used in this study were Aeromonas bestiarum CECT 4227 (NZ_CDDA00000000), A. caviae NCTC 12244 (NZ_LS483441), A. dhakensis CIP 107500 (NZ_CDBH00000000), A. hydrophila ATCC 7966 (NC_008570), A. media CECT 4232 (NZ_CDBZ00000000), A. piscicola LMG 24783 (NZ_CDBL00000000), A. rivipollensis KN-Mc-11N1(NZ_UAPT00000000) and A. salmonicida NCTC 12959 (NZ_UAPT00000000).
3.2. Virulence factors
Major virulence factors examined in this study include five categories; (i) adherence: the genes encoding type I and type IV pili, (ii) motility: the genes encoding polar and lateral flagella, (iii) immune evasion: the gene encoding capsules and lipopolysaccharide (LPS) O-antigens, (iv) secretion systems: the genes encoding type II secretion system (T2SS), type III secretion system (T3SS), and type VI secretion system (T6SS), and (v) toxins: the genes encoding cytotoxic and cytotonic enterotoxins, and exotoxins. Over 250 genes encoding multiple virulence factors were identified among the 19 Aeromonas strains, and virulence gene profiles of the 19 genomes were compared to the profiles of two reference genomes: A. hydrophila subsp. hydrophila ATCC 7966, which is a well-characterized type strain originally isolated from a tin of milk with a fishy odor (Seshadri et al., 2006), and A. salmonicida subsp. salmonicida A449, which is a type strain isolated from a brown trout with furunculosis (Reith et al., 2008) (Figure 3). Overall, the strains belonging to A. piscicola, A. bestiarum, A. salmonicida, A. hydrophila, and A. dhakensis contained more virulence factors than the strains belonging to A. caviae, A. media, and A. rivipollensis.
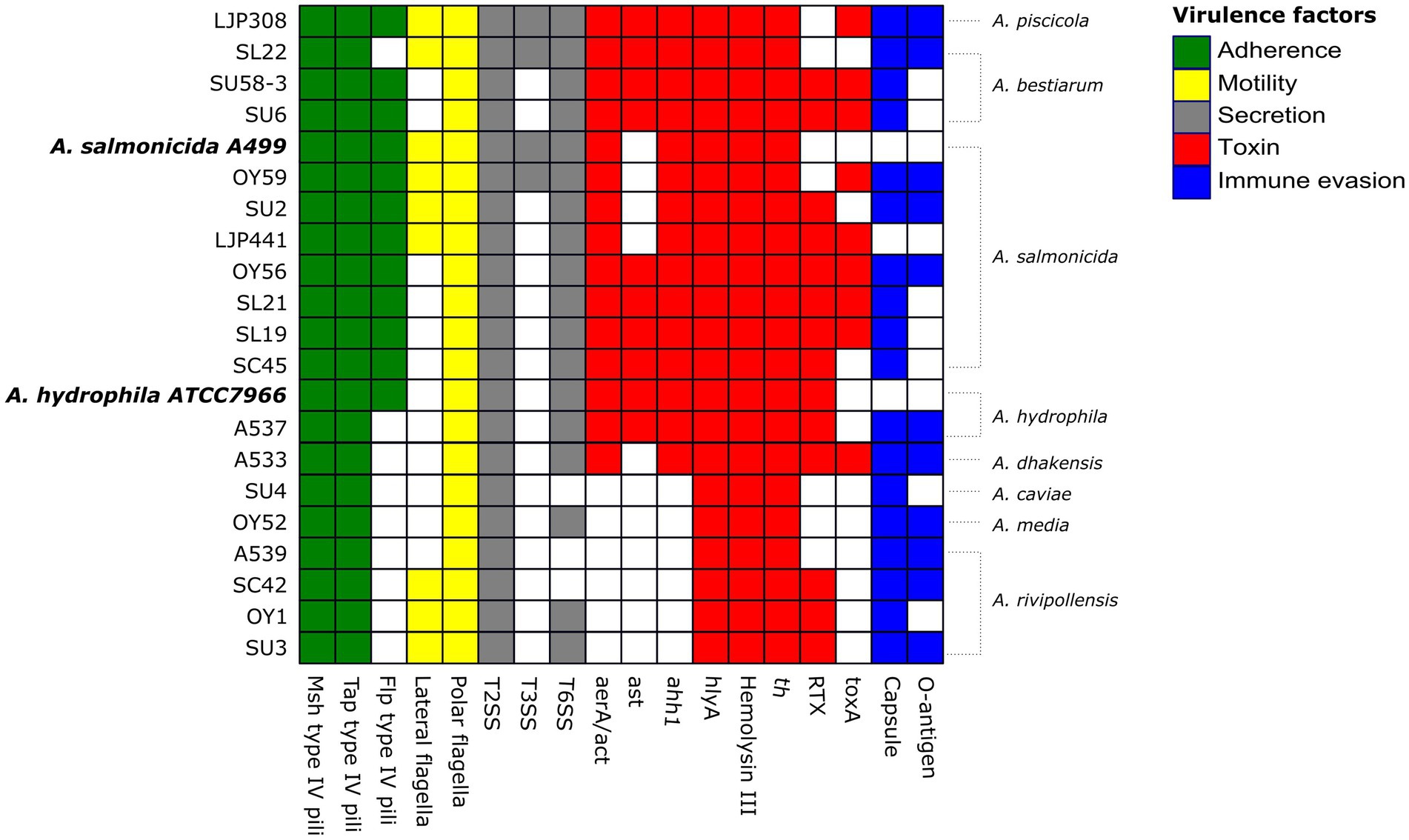
Figure 3. Heatmap showing the distribution of virulence factors across the 19 Aeromonas strains and 2 reference strains A. hydrophila subsp. hydrophila ATCC 7966 (NC_008570) and A. salmonicida subsp. salmonicida A449 (NC_009348, NC_009350). The presence of each virulence factor belonging to five categories is highlighted in different colors as follows; adherence (in green), motility (yellow), secretion (grey), toxin (red) and immune evasion (blue), and the absence of the virulence factor is marked in white color.
3.2.1. Adherence and motility
In this study, the presence of three distinct type IV pili was examined in 19 Aeromonas strains and those included were (i) mannose-sensitive haemagglutinin (Msh) pili, (ii) Tap pili, and (iii) Flp pili. Two reference strains contained the genes encoding all three type IV pili. Msh pili was also present in all 19 strains, while only ten contained a complete 17 gene cluster (msh-A,-B,-C,-D,-E,-F,-G,-H,-I,-I1,-J,-K,-L,-M,-N,-O,-P,-Q) encoding the Msh pili and those were the strains A. hydrophila A537, A. rivipollensis OY1, SU3, SC42, A539, A. salmonicida SU2 OY56, LJP441, and A. bestiarum SU6, SU58-3. The rest of the strains lacked some of the genes such as mshA or mshQ. In addition, over 14 genes including tapD encoding Tap pili, were found in all 19 strains, while Flp type IV pili were only detected in the A. salmonicida, A. piscicola and A. bestiarum strains (except for SL22). Like two references strains, type I pili were detected in 12 strains belonging to A. dhakenis, A. caviae, A. piscicola, A bestiarum, and A. salmonicida (except for SL21).
Moreover, over 50 genes encoding polar flagella were detected in all 19 strains as well as in two reference strains. Two major flagellin genes required for optimal polar flagella functions, flaA and flaB, were identified in most of the strains except for four strains A. rivipollensis A539, SU3, SC42, and A. caviae SU4-2. Other genes encoding flagellar motor components including fliG, fliM, fliN, motX, motY, pomA(A2), and pomB(B2) were found in all 19 strains. On the contrary, about 36 genes encoding lateral flagella including laf genes were detected in eight strains including A. rivipollensis, SU3, SC42, OY1, A. salmonicida SU2, OY59, LJP441, A. bestiarum SL22, and A. piscicola LJP308. The genes encoding the lateral flagella were observed in the reference strain A. salmonicida A449, but not in the A. hydrophila ATCC 7966.
3.2.2. Immune evasion
Most of the Aeromonas strains contained genes encoding capsules except for A. salmonicida LJP441. Genes encoding the LPS O-antigens were identified in 11 strains, including A. dhakensis A533, A. hydrophila A537, A. media OY52, A. rivipollensis A539, SU3, SC42, A. salmonicida SU2, OY56, OY59, A. bestiarum SL22 and A. piscicola LJP308. On the contrary, none of the reference strains had the genes encoding the capsules or LPS O-antigens.
3.2.3. Secretion systems
Genes encoding T2SS were detected in all examined strains including two reference strains. Like the A. hydrophila ATCC7966, most of the strains did not contain the genes encoding T3SS. Only three strains including A. bestiarum SL22, A. piscicola LJP308 and A. salmonicida OY59 had over 40 genes encoding T3SS, like the A. salmonicida A449; however, the bifunctional toxin gene, aexT, was only detected in the reference strain. Moreover, over 20 genes encoding T6SS, including major effectors such as hcp (or hcp1), vgrG1, vasH, or vasK, were detected in most of Aeromonas strains, except for three strains including A. rivipollensis A539, SC42, and A. caviae SU4-2. Both reference strains contained the genes encoding T6SS; however, some of the effector genes such as hcp and vgG were absent in the genome of A. salmonicida A499.
3.2.4. Toxin genes
All 19 Aeromonas strains contained the genes encoding hemolysin HlyA (hlyA), hemolysin III, and thermostable hemolysin, like both reference strains. Genes encoding the extracellular heat-liable hemolysin (ahh1) and the aerolysin AerA/cytotoxic enterotoxin Act (aerA/act) were also detected in both reference strains and most of our strains, except for the A. caviae, A. media and A. rivipollensis. The heat-stable cytotonic enterotoxin gene (ast) was found in the A. hydrophila ATCC 7966 as well as nine strains including A. hydrophila A537, A. bestiarum SU6, SU58-3, SL22, A. piscicola LJP308 and A. salmonicida SL19, SL21, SC45, OY56. In addition, six genes encoding the repeat toxins (RTX: rtx-A,-B,-C,-D,-E,-H) were detected in the A. hydrophila ATCC 7966 and ten strains including A. hydrophila A537, A. dhakensis A533, A. bestiarum SU6, SU58-3, and all of the A. salmonicida strains except for OY59. Three strains of A. rivipollensis SU3, SC42, OY1 possessed only the rtxA gene. Furthermore, the exotoxin A (ETA) gene, toxA was detected in nine strains including A. dhakensis A533, A. salmonicida SL19, SL22, OY56, OY59, LJP441, A. bestiarum SU6, SU58-3, and A. piscicola LJP308, unlike the reference strains.
3.2.5. Other virulence factors
The adeG gene encoding efflux pump autoinducer, related to biofilm formation was detected in A. bestiarum strain SU58-3. Iron uptake genes, basG or basB were detected in two strains A. dhakensis A533 and A. hydrophila A537. The catalase-peroxidase gene, katG, associated with stress adaptation was detected in all of the A. caviae, A. media and A. rivipollensis strains. The neuB2 gene encoding O-linked flagellar glycosylation was detected in two strains A. media OY52 and A. salmonicida SU2. More detailed information on virulence gene profile is available in Supplementary Table S3.
3.3. AMR
Phenotypic antimicrobial susceptibility profiles of 79 Aeromonas isolates (Supplementary Table S2) showed that all isolates were resistant to ampicillin as expected. Resistance to erythromycin, florfenicol and oxolinic acid was observed in 57, 48, and 22% of the strains, respectively. Reduced susceptibility to oxolinic acid was observed in 34% of the isolates. In addition, resistance (10%) or reduced susceptibility (11%) to imipenem was mostly observed in A. salmonicida, A. bestiarum and A. dhakensis strains. Resistance to cefotaxime was found in the A. rivipollensis strain SU2 and SU3, while reduced susceptibility to cefotaxime and ceftriaxone was observed in about 30% of the strains. One A. salmonicida strain (OY59) was resistant to both imipenem and meropenem, and another two A. salmonicida strains (OY60 and 61) showed reduced susceptibility to tobramycin. The A. caviae strain SU4-2 showed reduced susceptibility to trimethoprim/sulfamethoxazole. None of the Aeromonas strains were resistant to mecillinam, ciprofloxacin, doxycycline, tetracycline, or gentamycin. Moreover, about 58% of the Aeromonas strains were considered MDR. Most MDR strains were originally isolated from RTE seafood, and they were mainly resistant to ampicillin (penicillins), erythromycin (macrolides), florfenicol (amphenicols) and oxolinic acid (quinolones). On the other hand, none of the strains originated from the SPE was considered MDR.
Furthermore, WGS confirmed that all 19 Aeromonas strains contained multiple AMR genes in their genomes and revealed the presence of different classes of β-lactamases (Table 1). The class B metallo-β-lactamases (MBL) group was dominated by cphA1 and cphA5 found in the A. salmonicida, A. bestiarum, and A. piscicola strains, as well as cphA2 detected in the A. dhakensis and A. hydrophila strains. However, genes belonging to class B MBL group were not detected in any of the A. caviae, A. media and A. rivipollensis. Genes belonging to class C β-lactamases (blaAQU, blaMOX, and cepS) were detected only in four strains including A. caviae SU4-2, A dhakensis A533, A. hydrophila A537, and A. media OY52. All 19 Aeromonas strains contained different types of blaOXA genes belonging to the class D β-lactamases group. Identified blaOXA type genes were species-specific as following; blaOXA-12 (in A. dhakensis, A. hydrophila), blaOXA-780 (in A. caviae), blaOXA-427 (in A. media and A. rivipollensis), and blaOXA-956 (in A. salmonicida, A. bestiarum, and A. piscicola). The class A β-lactamases group including extended spectrum β-lactamases (ESBL) was not found in any of the Aeromonas strains. Other than β-lactamases, the sulfonamide resistance gene, sul1 and the aminoglycoside resistant gene, aadA1 were detected in the A. caviae SU4-2, and the quinolone resistance gene, qnrS2 was detected in the A. rivipollensis A539. In addition, two genes encoding a major facilitator superfamily (MFS) efflux pump protein, tet(E) and qacEΔ1, were found in the A. caviae SU4-2 while only tet(E) was detected in the A. hydrophila A537.
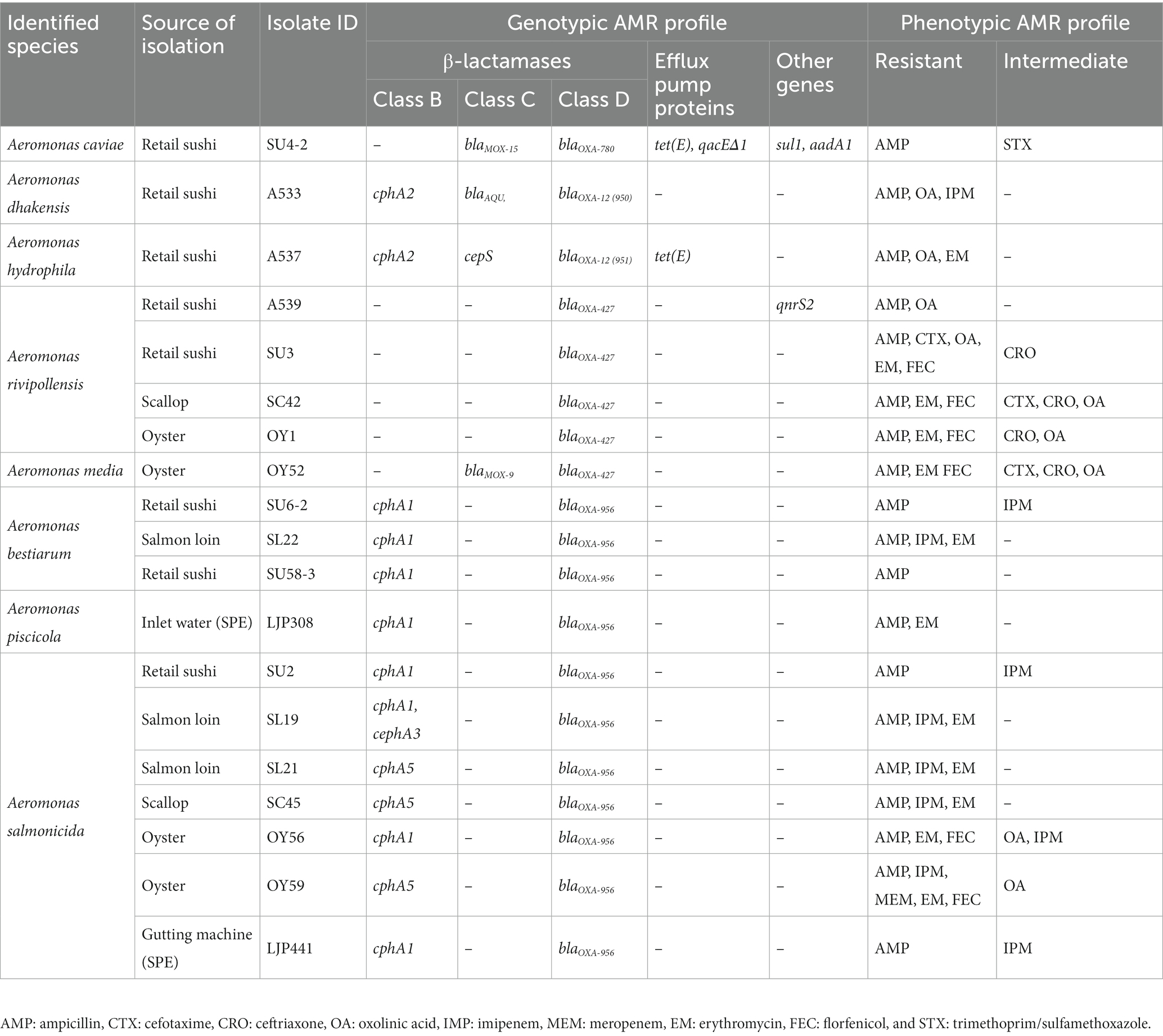
Table 1. Genotypic and phenotypic antimicrobial resistance (AMR) profile of 19 Aeromonas strains isolated from RTE seafood and a salmon processing environment (SPE).
3.4. MGEs
The presence of MGEs including plasmids, transposons, and insertion sequence (IS) elements was examined in the 19 Aeromonas strains. A. rivipollensis A539 was the only strain that contained a plasmid, which belonged to the IncQ1 group. A circular map of the plasmid (6,535 bp) shows the presence of two replication proteins repA and repC, the mobilization protein mobA, as well as the quinolone resistance gene, qnrS2 (Figure 4A).
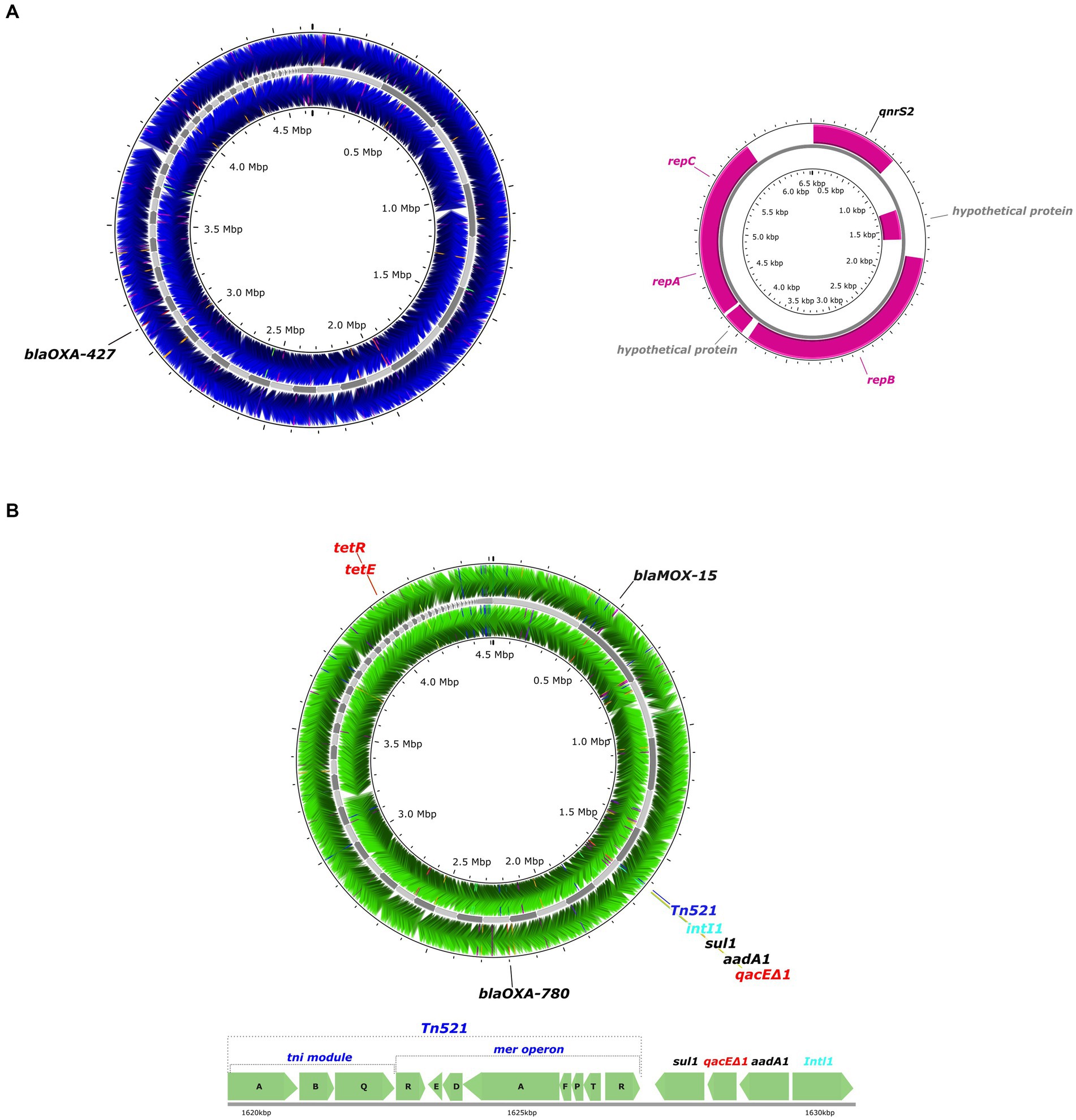
Figure 4. Circular maps of whole genome sequences of the Aeromonas A539 and SU4-2 strains isolated from retail sushi products. (A) Circular maps of the whole genome sequence of A. rivipollensis A539 strain (blue) showing the loci for the resistance gene (black) and its plasmid showing the loci for the resistance genes (black) and replication or mobilization genes (pink). (B) A circular map of the whole genome sequence of Aeromonas caviae SU4-2 strain (green) showing the loci for the resistance genes (black), a transposon (dark blue), an integron (light blue), and efflux pump proteins (red).
All Aeromonas strains except for A. piscicola LJP308 carried at least one transposase as a part of their IS elements, which belongs to different IS families such as IS1595, IS3, IS481, IS4, IS1634, IS5, IS21, IS30, IS66, IS110, IS200/IS605, IS256, IS630, and ISAs1 (Supplementary Table S4). Among the 19 strains, the A. caviae strain SU4-2 carried the highest number (21) of IS elements, and they were widely distributed in the chromosome. In addition, composite transposons (cn) together with their respective IS elements were identified in 12 strains including A. hydrophila A537, A. salmonicida SL19, OY56, and all strains of A. bestiarum and A. rivipollensis. In contrast, a non-composite transposon (Tn) was only detected in the A. caviae strain SU4-2. A circular map of the A. caviae SU4-2 genome shows the presence of the transposon Tn512, containing the mer operon encoding mercury-resistance, as well as a class I integron (IntI1; Figure 4B). Two AMR genes sul1 and aadA1, together with an efflux pump gene, qacEΔ1, were located between Tn512 and IntI. In addition, a transcription repressor, tet(R), was located next to a tetracycline efflux MFS efflux pump, tet(E).
4. Discussion
In this study, 79 Aeromonas isolates from RTE seafood and a SPE were subjected to species identification using gyrB gene sequencing, and these isolates were tested for susceptibility against 15 antimicrobial agents. Based on the gyrB gene sequence identity, 22 isolates representing eight different Aeromonas species were selected for WGS considering their source of isolation and phenotypic resistance patterns. A MLPA was performed based on the concatenated sequences of six housekeeping genes in the whole genome sequences of 22 isolates for taxonomy classification. The whole genomes of 19 Aeromonas strains were further examined to predict the presence of virulence genes, AMR genes as well as MGEs using different databases.
Identification of Aeromonas strains at the species level is still controversial and challenging due to the genetic heterogenicity in the genus Aeromonas (Janda and Abbott, 2010; Fernández-Bravo and Figueras, 2020). Molecular identification methods using 16S ribosomal RNA gene or housekeeping genes such as gyrB or rpoD, have been widely used for defining species and assessing the phylogenetic relationships within genus Aeromonas (Yáñez et al., 2003; Soler et al., 2004). Nevertheless, previous studies have shown the limitation of using a single gene sequence to infer the phylogeny of closely related Aeromonas species, due to the different phylogenetic resolution of protein-coding genes or possible horizontal gene transfer or recombination (Navarro and Martínez-Murcia, 2018). Currently, a MLPA of concatenated sequences of five or more housekeeping genes has been recommended for the correct identification of Aeromonas species (Martínez-Murcia et al., 2011; Navarro and Martínez-Murcia, 2018). In recent years, WGS technology has become more common, and the number of available Aeromonas genome sequences in the NCBI database is constantly increasing (Fernández-Bravo and Figueras, 2020). ANI is considered as one of the most robust methods to compare genomic relatedness among strains, with organisms belonging to the same species showing ≥ 95–96% ANI value (Richter and Rosselló-Móra, 2009). Currently, an ANI value of 96% is the recommended cutoff to define species boundaries of Aeromonas (Colston et al., 2014). ANI analysis has been used for the taxonomic verification of Aeromonas species (Figueras et al., 2014; Beaz-Hidalgo et al., 2015).
In our study, the phylogeny of the 22 isolates were analyzed by the MLPA based on the concatenated sequences of six housekeeping genes. The constructed tree by MLPA showed the robust topology with high supporting values by both NJ and ML methods. The phylogenetic clustering of the 22 isolates to eight different Aeromonas species could be verified by the ANI value ≥ 96%. We also constructed the six different phylogenetic trees based on each of the housekeeping gene sequences that extracted from the whole genome sequences. The species identity of 22 isolates based on each of the six gene markers and the concatenated sequences of the six gene markers was identical. One exceptional clustering of SU58-3 with A. piscicola was found based on the dnaX gene sequence. Nevertheless, we can conclude that the species identity of SU58-3 can be confirmed to be A. bestiarum by the ANI value of 97% between SU58-3 and A. bestiarum, while the ANI value was less than 95% between SU58-3 and A. piscicola. Additionally, the clustering of the dnaX gene sequence of the SU 58–3 with A. piscicola unlike other gene sequences suggested that using single gene sequence such as dnaX gene might not be specific enough to distinguish the closely related species such as A. piscicola and A. bestiarum.
Mesophilic Aeromonas strains are considered emerging pathogens in humans, causing gastrointestinal and extraintestinal infections with various clinical manifestations (Tomás, 2012; Fernández-Bravo and Figueras, 2020). Aeromonas pathogenicity is considered a multifactorial process, and the presence of several virulence factors can enable Aeromonas to adhere, invade, and destroy the host cells (Tomás, 2012; Fernández-Bravo and Figueras, 2020). Genes related to adherence, motility, immune evasion, secretion, and toxin production were the major virulence factors of Aeromonas strains examined in this study.
Aeromonas have two independent flagella systems for motility: polar flagella, required for the motility in a liquid environment (swimming) and lateral flagella, which is needed for the movement across a solid surface (swarming) (Kirov et al., 2002). While both flagella systems are involved in the early colonization of human intestinal cells as well as biofilm formation, not all mesophilic Aeromonas can produce a lateral flagella system (Kirov et al., 2004). In our study, 47% of 19 Aeromonas strains had the genes encoding the lateral flagella including laf genes, which are the major genes for lateral flagella biosynthesis (Canals et al., 2006a). However, the absence of the lateral flagella system in the reference strain A. hydrophila ATCC 7966 might imply that they might be not absolutely required for the virulence mechanism (Seshadri et al., 2006). Moreover, all 19 strains carried over 50 genes expressing polar flagella, while two major flagellin genes flaA and flaB were detected only in 15 strains. A previous study by Canals et al. (2006b) showed that the double mutant flaA and flaB caused the loss of polar flagella and reduced adherence and biofilm formation, implying that only those strains containing these two genes are likely to have the polar flagella with optimal functions.
Among type IV pili detected in mesophilic Aeromonas spp., Msh bundle-forming pili are known to be the major adherence system responsible for cell adherence, colonization, and biofilm formation (Kirov et al., 1999; Hadi et al., 2012). A complete gene cluster of Msh pili (mshA-Q) observed in ten strains in our study, have been characterized from A. veronii bv. sobria and these genes are required for the optimal function of cell adherence and biofilm formation (Hadi et al., 2012). In particular, mshQ is known to play an important role in the Msh pili biosynthesis of A. hydrophila (Qin et al., 2014). In the genome of A. hydrophila ATCC7966, some of the genes mshA, mshk, mshP were absent, implying that not all the 17 genes might be required for the adherence function of other species. In addition, genes encoding Tap pili were detected in all strains, including a TapD protein (tapABCD), which is known to be associated with the assembly and functionality of Msh pili as well as extracellular secretion of aerolysin and proteases, contributing to the T2SS (Pepe et al., 1996; Hadi et al., 2012). Moreover, the distribution of the genes encoding Flp pili was species-related, since those genes were only detected in the A. piscicola, A. bestiarum, and A. salmonicida strains. While very little is known about the specific role of Flp pili in mesophilic Aeromonas, the Flp pili of psychrophilic A. salmonicida strains have previously been identified and found not essential for their virulence (Boyd et al., 2008).
Bacterial cell surface polysaccharides including capsules, LPS O-antigens, and S-layers play important roles in immune evasion of many pathogens including Aeromonas (Rasmussen-Ivey et al., 2016). In this study, the genes encoding capsules and O-antigens were detected among our Aeromonas strains. The capsule covers the outer layers of the bacterial cell wall and is an important virulence factor of Aeromonas that helps prevent phagocytosis by host cells and acts as a barrier to toxin substances (Merino and Tomás, 2015). The O-antigen is the most surface-exposed LPS, acting as a colonization factor, and previous studies have shown that Aeromonas strains lacking O-antigen were unable to colonize hosts and have reduced expression of T3SS components (Merino et al., 1996; Vilches et al., 2004). Among 19 strains, most of had the gene encoding capsule as a virulence factor, whereas 11 strains representing each of the species except for A. caviae having both capsule and O-antigen, are likely involved in the virulence mechanisms of colonizing and invading host cells. However, none of the genes encoding capsule or O-antigen were detected in two reference genomes based on the VFDB. Considering that LPS O-antigen structure of the A. salmonicida strains A449 was previously characterized by the previous study (Wang et al., 2005), we cannot rule out the presence of those genes in both reference genomes. It might be that current database of VFDB is not fully updated with the genes associated with capsules or O-antigens of Aeromonas.
Of six secretion systems identified in Gram-negative bacteria, only T2SS, T3SS and T6SS were detected in the 19 strains. T2SS (detected in all strains) is an essential pathway for the pathogenesis of Aeromonas, since it is involved in the extracellular secretion of virulence factors such as DNase, protease, hemolysin and aerolysin (Sandkvist, 2001; Lowry et al., 2014). Both T3SS and T6SS are considered virulence markers of Aeromonas (Tomás, 2012). T3SS is a needle-like structure, injecting effectors directly into host cells, different T3SS effectors such as AexT, AexU, AopP, AopO and AopH have previously been identified in virulent A. hydrophila and A. salmoncidia strains (Burr et al., 2003; Sha et al., 2007). On the other hand, other studies have shown that both environmental and epidemic A. hydrophila strains lacked the genes encoding T3SS, implying that alternate secretion system is more critical for the virulence of A. hydrophila and probably other species as well (Seshadri et al., 2006; Pang et al., 2015). Like T3SS, T6SS could also inject their effectors into the host cells, and four effectors including Hcp (hemolysin coregulated protein), VgrG (valine-glycine repeat protein G), vasH (sigma factor 54 activator) and vasK are typical characteristics of the T6SS (Suarez et al., 2008, 2010). A previous study has showed that deletion of two genes hcp1 and vgG1 in virulent A. hydrophila strains significantly reduced their virulence (Tekedar et al., 2019). Besides, the T6SS has also been characterized from non-pathogenic Aeromonas strains (Pang et al., 2015; Rasmussen-Ivey et al., 2016). In our study, the T6SS including two genes (hcp1 and vgG1) was highly prevalent among the strains, implying their potential roles in pathogenicity and biofilm formation, while most of the strain lacked the genes encoding T3SS.
Two main types of enterotoxins are present in Aeromonas spp.: cytotoxic and cytotonic (Tomás, 2012). Cytotoxic enterotoxins include Act and aerolysin, known as the main virulence factors of A. hydrophila, and responsible for hemolytic, cytotoxic and enterotoxic activity (Chopra et al., 1993; Chopra and Houston, 1999). Two types of cytotonic enterotoxin include heat-liable Alt, and heat-stable Ast (Chopra et al., 1994). Hemolysins are cytotoxic and pore-forming toxins produced by pathogenic bacteria, and two hemolysin genes (hlyA and aerA) have been detected from all virulent A. hydrophila strains from the previous studies (Wong et al., 1998; Heuzenroeder et al., 1999). In addition, a previous study by Wang et al. (2003) showed that the combination of aerA and ahh1 genes seemed to be the most cytotoxic genotype of hemolysins, identified from all virulent A. hydrophila. In our study, three hemolysin genes encoding hemolysinA (hlyA), thermostable hemolysins (th) and hemolysinIII (hlyIII) were detected in all strains. Both aerA and ahh1 encoding aerolysin and extracellular hemolysin were detected in the A. dhakensis, A. hydrophila, A. bestiarum, A. piscicola and A. salmonicida strains, implying their potential for cytotoxic effects. Moreover, a RTX operon consisting of six genes (rtxACHBDE) were detected in some of the A. hydrophila, A. dhakensis, A. bestiarum and A. salmonicida strains, while only rtxA (exotoxin) was detected in the A. rivipollensis strains. A previous study by Suarez et al. (2012) showed that rtxA plays an important role in host cell rounding and apoptosis.
Our data showed the presence of multiple AMR genes in the Aeromonas strains regardless of the source of isolation. A large proportion of AMR genes detected in our Aeromonas strains belonged to the Ambler class B, C, and D β-lactamases, and species-specific distribution of β-lactamases genes was observed in accordance with previous observations (Fosse et al., 2003; Chen et al., 2012; Dubey et al., 2022a). Aeromonas spp. could produce β-lactamases which confer resistance to a broad spectrum of β-lactams antibiotic by hydrolyzing the four-membered β-lactam ring of antibiotics, and Ambler class B, C, and D β-lactamases are known as three major classes of chromosomally mediated β-lactamases detected in Aeromonas (Janda and Abbott, 2010).
Among the Ambler class B β-lactamases, the most prevalent gene among the 19 Aeromonas genomes was cphA. Previous research has reported that cphA gene is considered intrinsic among environmental Aeromonas spp., showing carbapenems-hydrolyzing activity (Balsalobre et al., 2009). In our study, cphA β-lactamases such as cphA1, cphA2, or cphA5 were detected in the chromosome of several strains, which showed phenotypic resistance or reduced susceptibility to imipenem or meropenem (carbapenems). The presence of those genes in the Aeromonas strains is likely to confer their phenotypic resistance to carbapenems. In addition, we observed the species-specific distribution of cphA genes, in accordance with previous studies (Rossolini et al., 1995; Chen et al., 2012). The class B β-lactamase genes, particularly cphA seem to be prevalent among Aeromonas spp., as these genes have frequently been observed in both environmental and clinical isolates of Aeromonas (Rossolini et al., 1995; Balsalobre et al., 2009; Wu et al., 2012).
Of the 19 strains, only four strains contained the genes belonging to the Ambler class C β-lactamases, associated with the resistance mechanisms of many β-lactam antibiotics, including narrow spectrum cephalosporins, third generation cephalosporins, but less active on fourth generation cephalosporins (Bush et al., 1995). Class C β-lactamases have been identified in both chromosomes and plasmids of Aeromonas spp. originating from various sources (Piotrowska et al., 2017; Ebmeyer et al., 2019; Piccirilli et al., 2022). The class C β-lactamases genes detected in our study were blaMOX-9 (in A. media), blaMOX-15 (in A. caviae), blaAQU (in A. dhakensis), and cepS (in A. hydrophila). Among these, blaMOX variants from blaMOX-3 to blaMOX-12 have previously been found in both environmental and clinical isolates of Aeromonas spp. (Ye et al., 2010; Piotrowska et al., 2017). In addition, blaMOX-9 was previously found in the transposon of A. media species and considered as a mobile antibiotic resistance gene (Ebmeyer et al., 2019; Piccirilli et al., 2022), whereas this gene detected in our study was encoded in the chromosome of the A. media strain. Moreover, the presence of blaAQU was observed in clinical isolates of A. dhakensis showing cefotaxime resistance (Wu et al., 2013), and cepS together with blaOXA-12 and cphA7 genes were detected in clinical strains of A. hydrophila strains showing carbapenem resistance (Hilt et al., 2020). Both blaAQU and cepS have also been detected in environmental isolates of Aeromonas spp. (Wang et al., 2021; Dubey et al., 2022a).
Among the Ambler class D β-lactamases, oxacillin-hydrolyzing type β-lactamases (OXAs) was observed in all 19 strains. OXAs can confer resistance not only to penicillin, but also to cephalosporins and carbapenems (Evans and Amyes, 2014). OXAs have been identified among several Gram-negative bacteria, including Aeromonas spp. (Poirel et al., 2010). The first OXA-like gene identified in the chromosome of Aeromonas spp. was designated as blaOXA-12, and blaOXA-12 associated genes including new variants such as blaOXA-427, blaOXA-780, blaOXA-830 and blaOXA-956 are considered innate in Aeromonas spp. (Rasmussen et al., 1994; Chen et al., 2019). Accordingly, four different blaOXA genes (blaOXA-12, blaOXA-427, blaOXA-780, blaOXA-956) detected in our study were chromosomally encoded in the Aeromonas genomes, and a species-related distribution was observed. Previous studies have also reported the presence of blaOXA genes in the Aeromonas spp. isolated from environmental (Moura et al., 2012; Piotrowska et al., 2017) and clinical samples (Hilt et al., 2020; Tang et al., 2020).
Other than β-lactamases, the quinolone resistance gene qnrS2 was present in the A. rivipollensis strain A539 showing phenotypic resistance to quinolones. Furthermore, the sulfonamide resistant gene sul1 was detected in the A. caviae strain SU4-2, that phenotypically showed reduced susceptibility to trimethoprim/sulfamethoxazole. On the other hand, some discrepancies between genotypic and phenotypic resistance were observed. For instance, phenotypic resistance to erythromycin or florfenicol could not be predicted based on the AMR gene profiles, and no correlation was found between the aminoglycoside resistance gene, aadA1 and the phenotypic resistance of the A. caviae strain SU4-2. Both AMRFinder and ResFinder databases have been constructed with high accuracy in predicting genotype–phenotype concordance for some foodborne pathogens; however, the outcomes may depend on the bacterial species, the type of antibiotics and the associated mechanism of resistance (Feldgarden et al., 2019; Bortolaia et al., 2020). In addition, incorrect phenotypic data might be the reason for discrepancies, since repeating phenotypic testing could have resolved most discrepancies between phenotypes and predicted genotypes using ResFinder (Zankari et al., 2013). Moreover, multidrug efflux pump proteins were detected from the two strains A. caviae SU4-2 and A. hydrophila A537. The MFS efflux pump gene, qacEΔ1 linked to sulfonamide (sul1) and aminoglycoside (aadA1) resistance genes was observed in the A. caviae strain SU4-2, while another efflux pump gene, tet(E) linked to the tet(R) was found in both A. caviae SU4-2 and A. hydrophila A537 strains. Similarly, the qacEΔ1 together with other resistance genes (sul1, aadA2) were also detected in A. caviae and A. hydrophila strains isolated from fish (Dubey et al., 2022a). In addition, the tet(E) has previously been identified in Aeromonas (Dubey et al., 2022b; Erickson et al., 2023).
A pool of genes located in MGEs such as plasmids, transposons, and integrons are considered flexible and transferrable, and may be associated with virulence factors, toxic compounds as well as antibiotic resistance (Piotrowska and Popowska, 2015). Several studies have reported the presence of MGEs in Aeromonas strains isolated from aquatic environments and fish, and their association with resistance or virulence determinants (McIntosh et al., 2008; del Castillo et al., 2013; Dubey et al., 2022a; Song et al., 2023). In our study, an IncQ plasmid carrying qnrS2 was detected in the A. rivipollensis strain A539 isolated from a retail sushi product. Other studies have suggested the strong relationship between the IncQ plasmid and qnrS2, where they have observed the IncQ plasmids harboring qnrS2 in Aeromonas strains isolated from fish and water (Cattoir et al., 2008; Majumdar et al., 2011; Han et al., 2012). Moreover, we found the co-localization of a transposon Tn521 containing a mercury operon and a class I integron (Int11) with two AMR genes (sul1, aadA1) and an efflux pump protein (qacEΔ1) in the A. caviae strain SU4-2, implying co-resistance of mercury and antibiotic resistance. Co-resistance occurs when resistance determinants to heavy metals and antibiotics are harbored together in the same MGEs, and this co-localization could result in co-selection mechanisms of other genes in the same elements (Chapman, 2003; Baker-Austin et al., 2006). Some studies have reported the IncA/C plasmids of Aeromonas spp. carrying the mercury operon and AMR genes in the class I integron (McIntosh et al., 2008; del Castillo et al., 2013;). Besides, several transposons and IS elements detected in Aeromonas spp. have strongly been associated with β-lactamases in previous studies (Girlich et al., 2011; Picão et al., 2013). However, we found no strong association between other MGEs identified in our Aeromonas strains and AMR genes.
5. Conclusion
In the present study, 22 Aeromonas strains isolated from RTE seafood and a SPE were classified into eight different Aeromonas species based on the MLPA and ANI analysis. Most strains contained several genes encoding major virulence factors related to adherence, motility, immune evasion, secretion systems, and toxins, and in particular, we observed the high incidence of enterotoxins, T6SS and its major effectors. Multiple AMR genes encoding class B, C and D β-lactamases were found in all Aeromonas strains and their distribution was species-related. In addition, the presence of other AMR genes located in MGEs such as an IncQ type plasmid in the A. rivipollensis strain A539 and a transposon and a class I integron in the A. caviae SU4-2 indicates their potential to disseminate AMR genes to other bacteria. Considering that most Aeromonas strains were isolated from RTE seafood, our study suggests that Aeromonas strains circulating in the food chain could potentially be pathogenic and act as a vector for dissemination of AMR genes to other bacteria residing in the same environments. Thus, we highlight the importance of collecting more knowledge of AMR and mobilome in the genus Aeromonas to understand their ability to transfer AMR within the food chain, and the potential risk of AMR caused by Aeromonas circulating in the food chain should be carefully monitored.
Data availability statement
The datasets presented in this study can be found in online repositories. The names of the repository/repositories and accession number(s) can be found in the article/Supplementary material and in the NCBI database under accession number: PRJNA877469.
Author contributions
H-JL: manuscript preparation, methodology, bioinformatics analysis, editing, and submission. JS: methodology, bioinformatics analysis, and editing. JL: editing and supervision. B-TL, SH, and AJ: conceptualization, editing, and supervision. All authors contributed to the article and approved the submitted version.
Funding
This work was funded by Norwegian University of Science and Technology (NTNU). H-JL was supported by a PhD grant from NTNU, as part of the OPTiMAT project.
Acknowledgments
The authors would like to thank Selina Chuan Jakobsen for her technical assistance.
Conflict of interest
The authors declare that the research was conducted in the absence of any commercial or financial relationships that could be construed as a potential conflict of interest.
Publisher’s note
All claims expressed in this article are solely those of the authors and do not necessarily represent those of their affiliated organizations, or those of the publisher, the editors and the reviewers. Any product that may be evaluated in this article, or claim that may be made by its manufacturer, is not guaranteed or endorsed by the publisher.
Supplementary material
The Supplementary material for this article can be found online at: https://www.frontiersin.org/articles/10.3389/fmicb.2023.1175304/full#supplementary-material
Footnotes
References
Albini, E., Orso, M., Cozzolino, F., Sacchini, L., Leoni, F., and Magistrali, C. F. (2022). A systematic review and meta-analysis on antimicrobial resistance in marine bivalves. Front. Microbiol. 13:1040568. doi: 10.3389/fmicb.2022.1040568
Baker-Austin, C., Wright, M. S., Stepanauskas, R., and McArthur, J. V. (2006). Co-selection of antibiotic and metal resistance. Trends Microbiol. 14, 176–182. doi: 10.1016/j.tim.2006.02.006
Balsalobre, L. C., Dropa, M., Lincopan, N., Mamizuka, E. M., Matté, G. R., and Matté, M. H. (2009). Detection of metallo-beta-lactamases-encoding genes in environmental isolates of Aeromonas hydrophila and Aeromonas jandaei. Lett. Appl. Microbiol. 49, 142–145. doi: 10.1111/j.1472-765X.2009.02625.x
Bankevich, A., Nurk, S., Antipov, D., Gurevich, A. A., Dvorkin, M., Kulikov, A. S., et al. (2012). SPAdes: a new genome assembly algorithm and its applications to single-cell sequencing. J. Comput. Biol. 19, 455–477. doi: 10.1089/cmb.2012.0021
Beaz-Hidalgo, R., and Figueras, M. J. (2013). Aeromonas spp. whole genomes and virulence factors implicated in fish disease. J. Fish Dis. 36, 371–388. doi: 10.1111/jfd.12025
Beaz-Hidalgo, R., Hossain, M. J., Liles, M. R., and Figueras, M. J. (2015). Strategies to avoid wrongly labelled genomes using as example the detected wrong taxonomic aliation for Aeromonas genomes in the Genbank database. PLoS One 10:e0115813. doi: 10.1371/journal.pone.0115813
Bortolaia, V., Kaas, R. S., Ruppe, E., Roberts, M. C., Schwarz, S., Cattoir, V., et al. (2020). ResFinder 4.0 for predictions of phenotypes from genotypes. J. Antimicrob. Chemother. 75, 3491–3500. doi: 10.1093/jac/dkaa345
Boyd, J. M., Dacanay, A., Knickle, L. C., Touhami, A., Brown, L. L., Jericho, M. H., et al. (2008). Contribution of type IV pili to the virulence of Aeromonas salmonicida subsp. salmonicida in Atlantic salmon (Salmo salar L.). Infect. Immun. 76, 1445–1455. doi: 10.1128/IAI.01019-07
Burr, S. E., Stuber, K., and Frey, J. (2003). The ADP-ribosylating toxin, AexT, from Aeromonas salmonicida subsp. salmonicida is translocated via a type III secretion pathway. J. Bacteriol. 185, 6583–6591. doi: 10.1128/JB.185.22.6583-6591
Bush, K., Jacoby, G. A., and Medeiros, A. A. (1995). A functional classification scheme for beta-lactamases and its correlation with molecular structure. Antimicrob. Agents Chemother. 39, 1211–1233. doi: 10.1128/AAC.39.6.1211
Canals, R., Altarriba, M., Vilches, S., Horsburgh, G., Shaw, J. G., Tomás, J. M., et al. (2006a). Analysis of the lateral flagellar gene system of Aeromonas hydrophila AH-3. J. Bacteriol. 188, 852–862. doi: 10.1128/JB.188.3.852-862.2006
Canals, R., Ramirez, S., Vilches, S., Horsburgh, G., Shaw, J. G., Tomás, J. M., et al. (2006b). Polar flagellum biogenesis in Aeromonas hydrophila. J. Bacteriol. 188, 542–555. doi: 10.1128/JB.188.2.542-555.2006
Carattoli, A., ZankarI, E., García-Fernández, A., Larsen, M. V., Lund, O., Villa, L., et al. (2014). In silico detection and typing of plasmids using PlasmidFinder and plasmid multilocus sequence typing. Antimicrob. Agents Chemother. 58, 3895–3903. doi: 10.1128/AAC.02412-14
Cattoir, V., Poirel, L., Aubert, C., Soussy, C. J., and Nordmann, P. (2008). Unexpected occurrence of plasmid-mediated quinolone resistance determinants in environmental Aeromonas spp. Emerg. Infect. Dis. 14, 231–237. doi: 10.3201/eid1402.070677
Chapman, J. S. (2003). Disinfectant resistance mechanisms, cross-resistance, and co-resistance. Int. Biodeterior. Biodegradation 51, 271–276. doi: 10.1016/S0964-8305(03)00044-1
Chen, P. L., Ko, W. C., and Wu, C. J. (2012). Complexity of β-lactamases among clinical Aeromonas isolates and its clinical implications. J. Microbiol. Immunol. Infect. 45, 398–403. doi: 10.1016/j.jmii.2012.08.008
Chen, Q., Zhou, W., Qian, C., Shen, K., Zhu, X., Zhou, D., et al. (2019). OXA-830, a novel chromosomally encoded extended-spectrum class D β-lactamase in Aeromonas simiae. Front. Microbiol. 10:2732. doi: 10.3389/fmicb.2019.02732
Chopra, A. K., and Houston, C. W. (1999). Enterotoxins in Aeromonas-associated gastroenteritis. Microbes Infect. 1, 1129–1137. doi: 10.1016/s1286-4579(99)00202-6
Chopra, A. K., Houston, C. W., Peterson, J. W., and Jin, G. F. (1993). Cloning, expression, and sequence analysis of a cytolytic enterotoxin gene from Aeromonas hydrophila. Can. J. Microbiol. 39, 513–523. doi: 10.1139/m93-073
Chopra, A. K., Pham, R., and Houston, C. W. (1994). Cloning and expression of putative cytotonic enterotoxin-encoding genes from Aeromonas hydrophila. Gene 139, 87–91. doi: 10.1016/0378-1119(94)90528-2
CLSI. (2014). Performance Standards for Antimicrobial Susceptibility Testing of Bacteria Isolated From Aquatic Animals: Second Informational Supplement CLSI Document VET03/VET04-S2. Wayne, PA: Clinical and Laboratory Standards Institute.
CLSI. (2016). Methods for antimicrobial dilution and disk susceptibility testing of infrequently isolated or fastidious Bacteria (3rd). Wayne, PA: CLSI guidline M45. Clinical and Laboratory Standards Institute.
Colston, S. M., Fullmer, M. S., Beka, L., Lamy, B., Gogarten, J. P., and Graf, J. (2014). Bioinformatic genome comparisons for taxonomic and phylogenetic assignments using Aeromonas as a test case. MBio 5:e02136. doi: 10.1128/mBio.02136-14
del Castillo, C. S., Hikima, J., Jang, H. B., Nho, S. W., Jung, T. S., and Wongtavatchai, J. (2013). Comparative sequence analysis of a multidrug-resistant plasmid from Aeromonas hydrophila. Antimicrob. Agents Chemother. 57, 120–129. doi: 10.1128/AAC.01239-12
Dubey, S., Ager-Wick, E., Kumar, J., Karunasagar, I., Karunasagar, I., Peng, B., et al. (2022a). Aeromonas species isolated from aquatic organisms, insects, chicken, and humans in India show similar antimicrobial resistance profiles. Front. Microbiol. 13:1008870. doi: 10.3389/fmicb.2022.1008870
Dubey, S., Ager-Wick, E., Peng, B., Evensen, Ø., Sørum, H., and Munang'andu, H. M. (2022b). Characterization of virulence and antimicrobial resistance genes of Aeromonas media strain SD/21-15 from marine sediments in comparison with other Aeromonas spp. Front. Microbiol. 13:1022639. doi: 10.3389/fmicb.2022.1022639
Ebmeyer, S., Kristiansson, E., and Larsson, D. G. J. (2019). CMY-1/MOX-family AmpC β-lactamases MOX-1, MOX-2 and MOX-9 were mobilized independently from three Aeromonas species. J. Antimicrob. Chemother. 74, 1202–1206. doi: 10.1093/jac/dkz025
EFSA Panel on Biological Hazards (BIOHAZ)Koutsoumanis, K., Allende, A., Álvarez-Ordóñez, A., Bolton, D., Bover-Cid, S., et al. (2021). Role played by the environment in the emergence and spread of antimicrobial resistance (AMR) through the food chain. Eur. Food Safety Authority J. 19:e06651. doi: 10.2903/j.efsa.2021.6651
Erickson, V. I., Alfifi, A., Hounmanou, Y. G. M., Sana, M. J., Christensen, J. P., and Dalsgaard, A. (2023). Genomic traits of Aeromonas veronii isolated from slaughtered Danish broilers. Vet. Microbiol. 283:109772. doi: 10.1016/j.vetmic.2023.109772
Evans, B. A., and Amyes, S. G. (2014). OXA β-lactamases. Clin. Microbiol. Rev. 27, 241–263. doi: 10.1128/CMR.00117-13
Feldgarden, M., Brover, V., Haft, D. H., Prasad, A. B., Slotta, D. J., Tolstoy, I., et al. (2019). Validating the AMRFinder tool and resistance gene database by using antimicrobial resistance genotype-phenotype correlations in a collection of isolates. Antimicrob. Agents Chemother. 63, e00483–e00419. doi: 10.1128/AAC.00483-19
Fernández-Bravo, A., and Figueras, M. J. (2020). An update on the genus Aeromonas: taxonomy, epidemiology, and pathogenicity. Microorganisms. 8:129. doi: 10.3390/microorganisms8010129
Figueras, M. J., Beaz-Hidalgo, R., Hossain, M. J., and Liles, M. R. (2014). Taxonomic affiliation of new genomes should be verified using average nucleotide identity and multilocus phylogenetic analysis. Genome Announc. 2, e00927–e00914. doi: 10.1128/genomeA.00927-14
Fosse, T., Giraud-Morin, C., and Madinier, I. (2003). Phénotypes de résistance aux beta-lactamines dans le genre Aeromonas [phenotypes of beta-lactam resistance in the genus Aeromonas]. Pathol. Biol. (Paris) 51, 290–296. doi: 10.1016/s0369-8114(03)00027-0
Founou, L. L., Founou, R. C., and Essack, S. Y. (2016). Antibiotic resistance in the food chain: a developing country-perspective. Front. Microbiol. 7:1881. doi: 10.3389/fmicb.2016.01881
Girlich, D., Poirel, L., and Nordmann, P. (2011). Diversity of clavulanic acid-inhibited extended-spectrum β-lactamases in Aeromonas spp. from the Seine River, Paris, France. Antimicrob. Agents Chemother. 55, 1256–1261. doi: 10.1128/AAC.00921-10
Grant, J. R., Enns, E., Marinier, E., Mandal, A., Herman, E. K., Chen, C. Y., et al. (2023). Proksee: in-depth characterization and visualization of bacterial genomes. Nucleic Acids Res. gkad326. doi: 10.1093/nar/gkad326
Hadi, N., Yang, Q., Barnett, T. C., Tabei, S. M., Kirov, S. M., and Shaw, J. G. (2012). Bundle-forming pilus locus of Aeromonas veronii bv. Sobria. Infect Immun. 80, 1351–1360. doi: 10.1128/IAI.06304-11
Han, J. E., Kim, J. H., Choresca, C. H. Jr., Shin, S. P., Jun, J. W., Chai, J. Y., et al. (2012). A small IncQ-type plasmid carrying the quinolone resistance (qnrS2) gene from Aeromonas hydrophila. Lett. Appl. Microbiol. 54, 374–376. doi: 10.1111/j.1472-765X.2012.03208.x
Heuzenroeder, M. W., Wong, C. Y., and Flower, R. L. (1999). Distribution of two hemolytic toxin genes in clinical and environmental isolates of Aeromonas spp.: correlation with virulence in a suckling mouse model. FEMS Microbiol. Lett. 174, 131–136. doi: 10.1111/j.1574-6968.1999.tb13559.x
Hilt, E. E., Fitzwater, S. P., Ward, K., de St Maurice, A., Chandrasekaran, S., Garner, O. B., et al. (2020). Carbapenem resistant Aeromonas hydrophila carrying blacphA7 isolated from two solid organ transplant patients. Front. Cell. Infect. Microbiol. 10:563482. doi: 10.3389/fcimb.2020.563482
Hoel, S., Mehli, L., Bruheim, T., Vadstein, O., and Jakobsen, A. N. (2015). Assessment of microbiological quality of retail fresh sushi from selected sources in Norway. J. Food Prot. 78, 977–982. doi: 10.4315/0362-028X.JFP-14-480
Hoel, S., Vadstein, O., and Jakobsen, A. N. (2017). Species distribution and prevalence of putative virulence factors in mesophilic Aeromonas spp. isolated from fresh retail sushi. Front. Microbiol. 8:931. doi: 10.3389/fmicb.2017.00931
Hoel, S., Vadstein, O., and Jakobsen, A. N. (2019). The significance of mesophilic Aeromonas spp. in minimally processed ready-to-eat seafood. Microorganisms. 7:91. doi: 10.3390/microorganisms7030091
Jain, C., Rodriguez-R, L. M., Phillippy, A. M., Konstantinidis, K. T., and Aluru, S. (2018). High throughput ANI analysis of 90K prokaryotic genomes reveals clear species boundaries. Nat. Commun. 9:5114. doi: 10.1038/s41467-018-07641-9
Janda, J. M., and Abbott, S. L. (2010). The genus Aeromonas: taxonomy, pathogenicity, and infection. Clin. Microbiol. Rev. 23, 35–73. doi: 10.1128/CMR.00039-09
Johansson, M. H. K., Bortolaia, V., Tansirichaiya, S., Aarestrup, F. M., Roberts, A. P., and Petersen, T. N. (2021). Detection of mobile genetic elements associated with antibiotic resistance in Salmonella enterica using a newly developed web tool: MobileElementFinder. J. Antimicrob. Chemother. 76, 101–109. doi: 10.1093/jac/dkaa390
Kimura, M. (1980). A simple method for estimating evolutionary rates of base substitutions through comparative studies of nucleotide sequences. J. Mol. Evol. 16, 111–120. doi: 10.1007/BF01731581
Kirov, S. M., Castrisios, M., and Shaw, J. G. (2004). Aeromonas flagella (polar and lateral) are enterocyte adhesins that contribute to biofilm formation on surfaces. Infect. Immun. 72, 1939–1945. doi: 10.1128/IAI.72.4.1939-1945.2004
Kirov, S. M., O'Donovan, L. A., and Sanderson, K. (1999). Functional characterization of type IV pili expressed on diarrhea-associated isolates of Aeromonas species. Infect. Immun. 67, 5447–5454. doi: 10.1128/IAI.67.10.5447-5454.1999
Kirov, S. M., Tassell, B. C., Semmler, A. B., O'Donovan, L. A., Rabaan, A. A., and Shaw, J. G. (2002). Lateral flagella and swarming motility in Aeromonas species. J. Bacteriol. 184, 547–555. doi: 10.1128/JB.184.2.547-555.2002
Lee, H. J., Hoel, S., Lunestad, B. T., Lerfall, J., and Jakobsen, A. N. (2021). Aeromonas spp. isolated from ready-to-eat seafood on the Norwegian market: prevalence, putative virulence factors and antimicrobial resistance. J. Appl. Microbiol. 130, 1380–1393. doi: 10.1111/jam.14865
Liu, B., Zheng, D., Jin, Q., Chen, L., and Yang, J. (2019). VFDB 2019: a comparative pathogenomic platform with an interactive web interface. Nucleic Acids Res. 47, D687–D692. doi: 10.1093/nar/gky1080
Love, D. C., Fry, J. P., Cabello, F., Good, C. M., and Lunestad, B. T. (2020). Veterinary drug use in United States net pen Salmon aquaculture: implications for drug use policy. Aquaculture 518:734820. doi: 10.1016/j.aquaculture.2019.734820
Lowry, R., Balboa, S., Parker, J. L., and Shaw, J. G. (2014). Aeromonas flagella and colonisation mechanisms. Adv. Microb. Physiol. 65, 203–256. doi: 10.1016/bs.ampbs.2014.08.007
Magiorakos, A. P., Srinivasan, A., Carey, R. B., Carmeli, Y., Falagas, M. E., Giske, C. G., et al. (2012). Multidrug-resistant, extensively drug-resistant and pandrug-resistant bacteria: an international expert proposal for interim standard definitions for acquired resistance. Clin. Microbiol. Infect. 18, 268–281. doi: 10.1111/j.1469-0691.2011.03570.x
Majumdar, T., Das, B., Bhadra, R. K., Dam, B., and Mazumder, S. (2011). Complete nucleotide sequence of a quinolone resistance gene (qnrS2) carrying plasmid of Aeromonas hydrophila isolated from fish. Plasmid 66, 79–84. doi: 10.1016/j.plasmid.2011.05.001
Martin-Carnahan, A., and Joseph, S. W. (2005). “Aeromonadales” in Bergey’s Manual of Systematic Bacteriology: Volume Two. The Proteobacteria Part B the Gammaproteobacteria. eds. D. J. Brenner, N. R. Krieg, J. T. Staley, G. M. Garrity, D. R. Boone, and P. Vos (Boston, MA: Springer).
Martínez-Murcia, A. J., Monera, A., Saavedra, M. J., Oncina, R., Lopez-Alvarez, M., Lara, E., et al. (2011). Multilocus phylogenetic analysis of the genus Aeromonas. Syst. Appl. Microbiol. 34, 189–199. doi: 10.1016/j.syapm.2010.11.014
McEwen, S. A., and Collignon, P. J. (2018). Antimicrobial resistance: a one health perspective. Microbiol Spectr. 6:2. doi: 10.1128/microbiolspec.ARBA-0009-2017
McIntosh, D., Cunningham, M., Ji, B., Fekete, F. A., Parry, E. M., Clark, S. E., et al. (2008). Transferable, multiple antibiotic and mercury resistance in Atlantic Canadian isolates of Aeromonas salmonicida subsp. Salmonicida is associated with carriage of an IncA/C plasmid similar to the Salmonella enterica plasmid pSN254. J. Antimicrob. Chemother. 61, 1221–1228. doi: 10.1093/jac/dkn123
Merino, S., Rubires, X., Aguillar, A., Guillot, J. F., and Tomas, J. M. (1996). The role of the O-antigen lipopolysaccharide on the colonization in vivo of the germfree chicken gut by Aeromonas hydrophila serogroup O:34. Microb. Pathog. 20, 325–333. doi: 10.1006/mpat.1996.0031
Merino, S., and Tomás, J. M. (2015). Bacterial capsules and evasion of immune responses. eLS 1–10. doi: 10.1002/9780470015902.a0000957.pub
Moura, A., Pereira, C., Henriques, I., and Correia, A. (2012). Novel gene cassettes and integrons in antibiotic-resistant bacteria isolated from urban wastewaters. Res. Microbiol. 163, 92–100. doi: 10.1016/j.resmic.2011.10.010
Navarro, A., and Martínez-Murcia, A. (2018). Phylogenetic analyses of the genus Aeromonas based on housekeeping gene sequencing and its influence on systematics. J. Appl. Microbiol. 125, 622–631. doi: 10.1111/jam.13887
NORM/NORM-VET. (2020). Usage of antimicrobial agents and occurrence of antimicrobial resistance in Norway. Tromsø/Oslo. Available at: https://www.fhi.no/en/publ/2021/norm-and-norm-vet/. Accessed February 1, 2023.
Pablos, M., Huys, G., Cnockaert, M., Rodríguez-Calleja, J. M., Otero, A., Santos, J. A., et al. (2011). Identification and epidemiological relationships of Aeromonas isolates from patients with diarrhea, drinking water and foods. Int. J. Food Microbiol. 147, 203–210. doi: 10.1016/j.ijfoodmicro.2011.04.006
Pang, M. B., Jiang, J. W., Xie, X., Wu, Y. F., Dong, Y. H., and Kwok, A. H. Y. (2015). Novel insights into the pathogenicity of epidemic Aeromonas hydrophila ST251 clones from comparative genomics. Sci. Rep. 5:9833. doi: 10.1038/srep09833
Pepe, C. M., Eklund, M. W., and Strom, M. S. (1996). Cloning of an Aeromonas hydrophila type IV pilus biogenesis gene cluster: complementation of pilus assembly functions and characterization of a type IV leader peptidase/N-methyltransferase required for extracellular protein secretion. Mol. Microbiol. 19, 857–869. doi: 10.1046/j.1365-2958.1996.431958.x
Picão, R. C., Cardoso, J. P., Campana, E. H., Nicoletti, A. G., Petrolini, F. V., Assis, D. M., et al. (2013). The route of antimicrobial resistance from the hospital effluent to the environment: focus on the occurrence of KPC-producing Aeromonas spp. and Enterobacteriaceae in sewage. Diagn. Microbiol. Infect. Dis. 76, 80–85. doi: 10.1016/j.diagmicrobio.2013.02.001
Piccirilli, A., Antonelli, A., D'Andrea, M. M., Cherubini, S., Perilli, M., and Rossolini, G. M. (2022). Molecular and kinetic characterization of MOX-9, a plasmid-mediated enzyme representative of a novel sublineage of MOX-type class C β-lactamases. Antimicrob. Agents Chemother. 66:e0059522. doi: 10.1128/aac.00595-22
Piotrowska, M., and Popowska, M. (2015). Insight into the mobilome of Aeromonas strains. Front. Microbiol. 6:494. doi: 10.3389/fmicb.2015.00494
Piotrowska, M., Przygodzińska, D., Matyjewicz, K., and Popowska, M. (2017). Occurrence and variety of β-lactamase genes among Aeromonas spp. isolated from urban wastewater treatment plant. Front. Microbiol. 8:863. doi: 10.3389/fmicb.2017.00863
Poirel, L., Naas, T., and Nordmann, P. (2010). Diversity, epidemiology, and genetics of class D beta-lactamases. Antimicrob. Agents Chemother. 54, 24–38. doi: 10.1128/AAC.01512-08
Qin, Y. X., Yan, Q. P., Mao, X. X., Chen, Z., and Su, Y. Q. (2014). Role of MshQ in MSHA pili biosynthesis and biofilm formation of Aeromonas hydrophila. Genet. Mol. Res. 13, 8982–8996. doi: 10.4238/2014
R Core Team. (2022). R: A Language and Environment for Statistical Computing. R Foundation for Statistical Computing, Vienna, Austria. Available at: https://www.R-project.org/.
Rasmussen, B. A., Keeney, D., Yang, Y., and Bush, K. (1994). Cloning and expression of a cloxacillin-hydrolyzing enzyme and a cephalosporinase from Aeromonas sobria AER 14M in Escherichia coli: requirement for an E. coli chromosomal mutation for efficient expression of the class D enzyme. Antimicrob. Agents Chemother. 38, 2078–2085. doi: 10.1128/aac.38.9.2078
Rasmussen-Ivey, C. R., Figueras, M. J., McGarey, D., and Liles, M. R. (2016). Virulence factors of Aeromonas hydrophila: in the wake of reclassification. Front. Microbiol. 7:1337. doi: 10.3389/fmicb.2016.01337
Reith, M. E., Singh, R. K., Curtis, B., Boyd, J. M., Bouevitch, A., Kimball, J., et al. (2008). The genome of Aeromonas salmonicida subsp. salmonicida A449: insights into the evolution of a fish pathogen. BMC Genomics 9:427. doi: 10.1186/1471-2164-9-427
Richter, M., and Rosselló-Móra, R. (2009). Shifting the genomic gold standard for the prokaryotic species definition. Proc. Natl. Acad. Sci. U. S. A. 106, 19126–19131. doi: 10.1073/pnas.0906412106
Rossolini, G. M., Zanchi, A., Chiesurin, A., Amicosante, G., Satta, G., and Guglielmetti, P. (1995). Distribution of cphA or related carbapenemase-encoding genes and production of carbapenemase activity in members of the genus Aeromonas. Antimicrob. Agents Chemother. 39, 346–349. doi: 10.1128/AAC.39.2.346
Sandkvist, M. (2001). Type II secretion and pathogenesis. Infect. Immun. 69, 3523–3535. doi: 10.1128/IAI.69.6.3523-3535.2001
Sanseverino, I., Navarro Cuenca, A., Loos, R., Marinov, D., and Lettieri, T. (2018). State of the Art on the contribution of water to antimicrobial resistance. EUR 29592 EN. Publications Office of the European Union, Luxembourg.
Seshadri, R., Joseph, S. W., Chopra, A. K., Sha, J., Shaw, J., Graf, J., et al. (2006). Genome sequence of Aeromonas hydrophila ATCC 7966T: jack of all trades. J. Bacteriol. 188, 8272–8282. doi: 10.1128/JB.00621-06
Sha, J., Wang, S. F., Suarez, G., Sierra, J. C., Fadl, A. A., Erova, T. E., et al. (2007). Further characterization of a type III secretion system (T3SS) and of a new effector protein from a clinical isolate of Aeromonas hydrophila–part I. Microb. Pathog. 43, 127–146. doi: 10.1016/j.micpath.2007.05.002
Shimoyama, Y. (2022). ANIclustermap: A tool for drawing ANI clustermap between all-vs-all microbial genomes [computer software]. Available from: https://github.com/moshi4/ANIclustermap
Soler, L., Yáñez, M. A., Chacon, M. R., Aguilera-Arreola, M. G., Catalan, V., Figueras, M. J., et al. (2004). Phylogenetic analysis of the genus Aeromonas based on two housekeeping genes. Int. J. Syst. Evol. Microbiol. 54, 1511–1519. doi: 10.1099/ijs.0.03048-0
Song, Y., Wang, L. F., Zhou, K., Liu, S., Guo, L., Ye, L. Y., et al. (2023). Epidemiological characteristics, virulence potential, antimicrobial resistance profiles, and phylogenetic analysis of Aeromonas caviae isolated from extra-intestinal infections. Front. Cell. Infect. Microbiol. 13:1084352. doi: 10.3389/fcimb.2023.1084352
Stratev, D., and Odeyemi, O. A. (2016). Antimicrobial resistance of Aeromonas hydrophila isolated from different food sources: a mini-review. J. Infect. Public Health 9, 535–544. doi: 10.1016/j.jiph.2015.10.006
Suarez, G., Khajanchi, B. K., Sierra, J. C., Erova, T. E., Sha, J., and Chopra, A. K. (2012). Actin cross-linking domain of Aeromonas hydrophila repeat in toxin a (RtxA) induces host cell rounding and apoptosis. Gene 506, 369–376. doi: 10.1016/j.gene.2012.07.012
Suarez, G., Sierra, J. C., Erova, T. E., Sha, J., Horneman, A. J., and Chopra, A. K. (2010). A type VI secretion system effector protein, VgrG1, from Aeromonas hydrophila that induces host cell toxicity by ADP ribosylation of actin. J. Bacteriol. 192, 155–168. doi: 10.1128/JB.01260-09
Suarez, G., Sierra, J. C., Sha, J., Wang, S., Erova, T. E., Fadl, A. A., et al. (2008). Molecular characterization of a functional type VI secretion system from a clinical isolate of Aeromonas hydrophila. Microb. Pathog. 44, 344–361. doi: 10.1016/j.micpath.2007.10.005
Talagrand-Reboul, E., Roger, F., Kimper, J. L., Colston, S. M., Graf, J., Latif-Eugenín, F., et al. (2017). Delineation of taxonomic species within complex of species: Aeromonas media and related species as a test case. Front. Microbiol. 8:621. doi: 10.3389/fmicb.2017.00621
Tamura, K., Stecher, G., and Kumar, S. (2021). MEGA11: molecular evolutionary genetics analysis version 11. Mol. Biol. Evol. 38, 3022–3027. doi: 10.1093/molbev/msab120
Tang, L., Huang, J., She, J., Zhao, K., and Zhou, Y. (2020). Co-occurrence of the blaKPC-2 and Mcr-3.3 gene in Aeromonas caviae SCAc2001 isolated from patients with diarrheal disease. Infect Drug Resist. 13, 1527–1536. doi: 10.2147/IDR.S245553
Tatusova, T., DiCuccio, M., Badretdin, A., Chetvernin, V., Nawrocki, E. P., Zaslavsky, L., et al. (2016). NCBI prokaryotic genome annotation pipeline. Nucleic Acids Res. 44, 6614–6624. doi: 10.1093/nar/gkw569
Tekedar, H. C., Abdelhamed, H., Kumru, S., Blom, J., Karsi, A., and Lawrence, M. L. (2019). Comparative genomics of Aeromonas hydrophila secretion systems and mutational analysis of hcp1 and vgrG1 genes from T6SS. Front. Microbiol. 9:3216. doi: 10.3389/fmicb.2018.03216
Teunis, P., and Figueras, M. J. (2016). Reassessment of the enteropathogenicity of mesophilic Aeromonas species. Front. Microbiol. 7:1395. doi: 10.3389/fmicb.2016.01395
Thomassen, G. M. B., Krych, L., Knøchel, S., and Mehli, L. (2023). Bacterial community development and diversity during the first year of production in a new salmon processing plant. Food Microbiol. 109:104138. doi: 10.1016/j.fm.2022.104138
Thomassen, G. M. B., Reiche, T., Tennfjord, C. E., and Mehli, L. (2022). Antibiotic resistance properties among Pseudomonas spp. associated with salmon processing environments. Microorganisms. 10:1420. doi: 10.3390/microorganisms10071420
Thompson, J., Higgins, D. G., and Gibson, T. J. (1994). CLUSTAL W: improving the sensitivity of progressive multiple sequence alignment through sequence weighting, position-specific gap penalties and weight matrix choice. Nucleic Acids Res. 22, 4673–4680. doi: 10.1093/nar/22.22.4673
Tomás, J. M. (2012). The main Aeromonas pathogenic factors. ISRN Microbiol. 2012:256261. doi: 10.5402/2012/256261
Ventura, R. J., Muhi, E., de los Reyes, V. C., Sucaldito, M. N., and Tayag, E. (2015). A community-based gastroenteritis outbreak after typhoon Haiyan, Leyte, Philippines, 2013. Western Pac. Surveill. Response J. 6, 1–6. doi: 10.5365/wpsar.2014.5.1.010
Vilches, S., Urgell, C., Merino, S., Chacon, M. R., Soler, L., Castro-Escarpulli, G., et al. (2004). Complete type III secretion system of a mesophilic Aeromonas hydrophila strain. Appl. Environ. Microbiol. 70, 6914–6919. doi: 10.1128/AEM.70.11.6914-6919.2004
Wang, G., Clark, C. G., Liu, C., Pucknell, C., Munro, C. K., Kruk, T. M., et al. (2003). Detection and characterization of the hemolysin genes in Aeromonas hydrophila and Aeromonas sobria by multiplex PCR. J. Clin. Microbiol. 41, 1048–1054. doi: 10.1128/JCM.41.3.1048-1054
Wang, Y., Hou, N., Rasooly, R., Gu, Y., and He, X. (2021). Prevalence and genetic analysis of chromosomal mcr-3/7 in Aeromonas from U.S. animal-derived samples. Front. Microbiol. 12:667406. doi: 10.3389/fmicb.2021.667406
Wang, Z., Vinogradov, E., Larocque, S., Harrison, B. A., Li, J., and Altman, E. (2005). Structural and serological characterization of the O-chain polysaccharide of Aeromonas salmonicida strains A449, 80204 and 80204-1. Carbohydr. Res. 340, 693–700. doi: 10.1016/j.carres.2005.01.009
Wong, C. Y. F., Heuzenroeder, M. W., and Flower, R. L. P. (1998). Inactivation of two haemolytic toxin genes in Aeromonas hydrophila attenuates virulence in a suckling mouse model. Microbiology 144, 291–298. doi: 10.1099/00221287-144-2-291
Wu, C. J., Chen, P. L., Wu, J. J., Yan, J. J., Lee, C. C., Lee, H. C., et al. (2012). Distribution and phenotypic and genotypic detection of a metallo-β-lactamase, CphA, among bacteraemic Aeromonas isolates. J. Med. Microbiol. 61, 712–719. doi: 10.1099/jmm.0.038323-0
Wu, C. J., Figueras, M. J., Chen, P. L., and Ko, W. C. (2018) in Aeromonas in Handbook of Foodborne Diseases. ed. D. Liu, vol. 22. 1st ed (Boca Raton, FL: Imprint CRC Press), 12.
Wu, C. J., Wang, H. C., Chen, P. L., Chang, M. C., Sunny, S. H., Chou, P. H., et al. (2013). AQU-1, a chromosomal class C β-lactamase, among clinical Aeromonas dhakensis isolates: distribution and clinical significance. Int. J. Antimicrob. Agents 42, 456–461. doi: 10.1016/j.ijantimicag.2013.08.002
Yáñez, M. A., Catalan, V., Apráiz, D., Figueras, M. J., and Martínez-Murcia, A. J. (2003). Phylogenetic analysis of members of the genus Aeromonas based on gyrB gene sequences. Int. J. Syst. Evol. Microbiol. 53, 875–883. doi: 10.1099/ijs.0.02443-0
Ye, Y., Xu, X. H., and Li, J. B. (2010). Emergence of CTX-M-3, TEM-1 and a new plasmid-mediated MOX-4 AmpC in a multiresistant Aeromonas caviae isolate from a patient with pneumonia. J. Med. Microbiol. 59, 843–847. doi: 10.1099/jmm.0.016337-0
Zankari, E., Hasman, H., Kaas, R. S., Seyfarth, A. M., Agersø, Y., Lund, O., et al. (2013). Genotyping using whole-genome sequencing is a realistic alternative to surveillance based on phenotypic antimicrobial susceptibility testing. J. Antimicrob. Chemother. 68, 771–777. doi: 10.1093/jac/dks496
Keywords: Aeromonas , antimicrobial resistance, virulence factors, mobile genetic elements, whole genome sequences, multilocus phylogenetic analysis
Citation: Lee H-J, Storesund JE, Lunestad B-T, Hoel S, Lerfall J and Jakobsen AN (2023) Whole genome sequence analysis of Aeromonas spp. isolated from ready-to-eat seafood: antimicrobial resistance and virulence factors. Front. Microbiol. 14:1175304. doi: 10.3389/fmicb.2023.1175304
Edited by:
Ming-Chi Li, National Cheng Kung University, TaiwanReviewed by:
Sanath H. Kumar, Central Institute of Fisheries Education (ICAR), IndiaChristopher John Grim, United States Food and Drug Administration, United States
Maria Jose Figueras, University of Rovira i Virgili, Spain
Copyright © 2023 Lee, Storesund, Lunestad, Hoel, Lerfall and Jakobsen. This is an open-access article distributed under the terms of the Creative Commons Attribution License (CC BY). The use, distribution or reproduction in other forums is permitted, provided the original author(s) and the copyright owner(s) are credited and that the original publication in this journal is cited, in accordance with accepted academic practice. No use, distribution or reproduction is permitted which does not comply with these terms.
*Correspondence: Hye-Jeong Lee, hyejeong.lee@ntnu.no