- Department of Agricultural Biotechnology and Department of Food and Animal Biotechnology, Research Institute of Agriculture and Life Sciences, Seoul National University, Seoul, Republic of Korea
The emergence of antimicrobial resistance (AMR) Escherichia coli has noticeably increased in recent years worldwide and causes serious public health concerns. As alternatives to antibiotics, bacteriophages are regarded as promising antimicrobial agents. In this study, we isolated and characterized a novel jumbo phage EJP2 that specifically targets AMR E. coli strains. EJP2 belonged to the Myoviridae family with an icosahedral head (120.9 ± 2.9 nm) and a non-contractile tail (111.1 ± 0.6 nm), and contained 349,185 bp double-stranded DNA genome with 540 putative ORFs, suggesting that EJP2 could be classified as jumbo phage. The functions of genes identified in EJP2 genome were mainly related to nucleotide metabolism, DNA replication, and recombination. Comparative genomic analysis revealed that EJP2 was categorized in the group of Rak2-related virus and presented low sequence similarity at the nucleotide and amino acid level compared to other E. coli jumbo phages. EJP2 had a broad host spectrum against AMR E. coli as well as pathogenic E. coli and recognized LPS as a receptor for infection. Moreover, EJP2 treatment could remove over 80% of AMR E. coli biofilms on 96-well polystyrene, and exhibit synergistic antimicrobial activity with cefotaxime against AMR E. coli. These results suggest that jumbo phage EJP2 could be used as a potential biocontrol agent to combat the AMR issue in food processing and clinical environments.
Introduction
Escherichia coli is a main opportunistic pathogen that commonly colonizes in the gastrointestinal tract of both animals and humans (Kim et al., 2021), causing a range of intestinal and extra-intestinal disease. Various antibiotics have been applied orally or via injection to control this bacteria, but E. coli species have represented a capability to easily acquire the resistance genes by horizontal gene transfer (Poirel et al., 2018). Antimicrobial resistance (AMR) E. coli, such as Extended-Spectrum Beta-Lactamase (ESBL)-producing E. coli, exhibit a wide spectrum of resistance against all β-lactam antibiotics and other class of antibiotics such as fluoroquinolones, aminoglycosides (Coque et al., 2008; Dd Pitout, 2013). The emergence and prevalence of AMR in E. coli has become serious public health threats (Roca et al., 2015) and a number of studies have reported the isolation of AMR E. coli from patients, animals, and food chain (Rupp and Fey, 2003; Poirel et al., 2018; Park et al., 2019; Martak et al., 2022). Hence, World Health Organization (WHO) declared that AMR E. coli is one of the most urgent strains for which novel antimicrobials are needed (Shrivastava et al., 2018). Currently, the use of antibiotics is limited due to rapid acquisition of AMR in E. coli (Zurfuh et al., 2016; Parvez and Khan, 2018; Wu et al., 2018), so the development of alternative antimicrobial agents is necessary to address these concerns.
Bacteriophages (phages) are viruses that infect specific bacterial species and are the most abundant biological entities on earth (Bragg et al., 2014). In comparison to conventional antibiotics, phages have several advantages for use as alternative antimicrobial agents, such as harmlessness to human and commensal bacteria, host specificity, and low cost for production compared to development of novel antibiotics (Loc-Carrillo and Abedon, 2011). Phages are classified into two categories based on their life cycles; virulent and temperate phage (Kutter and Sulakvelidze, 2004) and the use of virulent phage is considered as a suitable strategy to control pathogens (Hassan et al., 2021). After the phage products, Listshield (Intralytix, Inc., Baltimore, MD, United States), was firstly approved by U.S. Food and Drug Administration (FDA) in 2006, a variety of phage products have commodified to prevent E. coli infection (Huang et al., 2022). E. coli phages (coliphages) are commonly isolated from environment. Although many studies of coliphages in terms of genetics and molecular biology have provided insights into the phage biology (Kutter and Sulakvelidze, 2004), but what we know about phage is just the tip of the iceberg.
Phages with a genome size from 200 to 500 kb are classified as jumbo phages (Yuan and Gao, 2017). There are over 22,000 registered phages in the NCBI database, and out of these, 581 phages can be classified as jumbo phages. Jumbo phages have distinct characteristics compared to phage with genome size under 200 kb. Nazir et al. (2021) reported that most jumbo phages morphologically represent head and tail sizes of over 100 nm (Nazir et al., 2021). Jumbo phages possess genomes that contain numerous genes associated with genome replication, modification and nucleotide metabolism, enabling them to replicate independently from the host. Genes with similar function in jumbo phage genomes are typically dispersed or organized into sub-clusters throughout the genome (Ceyssens et al., 2014; Guan and Bondy-Denomy, 2020). Transcription of early phage genes is commonly regulated by phage-encoded RNA polymerases (RNAPs) (Mesyanzhinov et al., 2002; Leskinen et al., 2016; Imam et al., 2019), and non-virion RANP is responsible for transcription of middle or late phage genes (Orekhova et al., 2019). In addition, jumbo phages possess a range of tRNAs and aminoacyl-tRNA synthetases, which can replace cleaved host tRNA to maintain translation of viral proteins. Iyer et al. (2021) analyzed that the number of tRNA genes in jumbo phages ranges from 4.5 to 22 per genome. The abundance of transcription and translation-related genes enables the post-infection development of jumbo phages, which implies a high level of independence from the host molecular machinery (Ceyssens et al., 2014; Lavysh et al., 2016). This independence appears to confer broad host ranges to jumbo phages, as in the case of Xanthomonas citri jumbo phage XacN1 (Yoshikawa et al., 2018).
In this study, we isolated and characterized E. coli jumbo phage EJP2, which has a genome size of 349,185 bp (accession no. OQ411014) with low sequence homology to the other jumbo phages currently known. We investigated its biological and genomic features of EJP2, and revealed that EJP2 recognized LPS as a phage receptor. EJP2 presented a broad host range, biofilm removal activity and exhibited synergistic antimicrobial efficacy against AMR E. coli when used in combination with cefotaxime (CTX) treatment. These results would help to expand our knowledge of jumbo phages and suggest the potential of EJP2 as alternative antimicrobial agents for biocontrol of AMR E. coli.
Materials and methods
Bacterial strains, plasmids and growth conditions
The bacterial strains used in this study are listed in Table 1. All bacteria were grown in Luria-Bertani (LB) broth and agar plates at 30°C and/or 37°C supplemented with appropriate antibiotics: ampicillin (Amp), 50 μg/ml; carbenicillin (Car), 100 μg/ml; kanamycin (Kan), 50 μg/ml; acridine orange (AO), 100 μg/ml, while isopropyl β-D-1-thiogalactopyranoside (IPTG) was added at a concentration of 50 or 100 μM.
Bacteriophage isolation and propagation
Animal fecal samples were collected in Seoul, South Korea. Phage isolation was conducted as previously described with some modifications (Saad et al., 2019). Sample was homogenized with 50 ml of sodium chloride-magnesium sulfate (SM) buffer (50 mM Tris–HCl, pH 7.5, 100 mM NaCl and 8 mM MgSO4·7H2O). Large particles were excluded by centrifuge (5,000 × g, 10 min, 4°C) and chloroform was added to supernatant for removal of residual bacteria. After centrifugation, supernatants were discarded to remove small phages and the pellet was suspended with 5 ml of SM buffer. This process was repeated three times. The resultant lysate was spotted on the bacterial lawn containing host cell. Briefly, 100 μl of cultured host cell (E. coli FORC82) was inoculated into 5 ml of LB soft agar [0.3% (supplemented with appropriate antibiotic and IPTG, if necessary)]. Mixture was poured on LB agar plates and solidified for 30 min. Ten microliters of serially diluted (10-fold) phage lysates were spotted on the bacterial lawn and dried for 20 min at room temperature. The plates were incubated at 30°C for at least 12 h to obtain single plaques. Small single plaques were picked with the sterile tip and eluted in 250 μl of SM buffer for further purification. This purification step was repeated at least three times. For phage propagation, host strain was incubated at 30°C for 2 h 30 min. The phage lysate was added into bacterial culture at a multiplicity of infection (MOI) of 1 and incubated for 4 h. The propagated phages were precipitated with polyethylene glycol (PEG) 6,000 and concentrated by CsCl density gradient ultracentrifugation (78,500 × g, 2 h, 4°C) (Kim H. et al., 2019).
Morphological analysis by TEM
Each purified phage stock dilutions (4 μl, approximately 109 PFU/mL) were placed on carbon-coated copper grids for 60 s and the excess phage was removed with filter paper. Equal volume of 2% aqueous uranyl acetate (pH 4.0) were added for 90 s to negatively stain the phage particles. Phages were examined by transmission electron microscopy (TEM; LEO 912AB transmission electron microscope; Carl Zeiss, Wezlar, Germany) at a 120-kV accelerating voltage, and images were scanned at the National Instrumentation Center for Environmental Management (Seoul, South Korea). Phage were morphologically classified according to the guidelines of the International Committee on Taxonomy of Viruses (ICTV) (Walker et al., 2022).
Bacteriophage spot assay
The bacterial lawn was prepared as described by (Kim and Ryu, 2011). Briefly, 200 μl of cultured host cells was inoculated into 5 ml of LB soft agar [0.3% agar (supplemented with the appropriate antibiotic and IPTG, if necessary)]. This mixture was poured onto LB agar plates and solidified for 30 min. Ten microliters of serially diluted (10-fold) phage lysates were spotted on the bacterial lawn and dried at room temperature for 20 min. The plates were incubated for 12 h at 30°C, and the phage plaques were monitored.
Bacterial challenge assays
One milliliter of LB broth was inoculated with overnight culture of E. coli FORC82. Bacterial cultures were incubated at 30 and 37°C for 2 h and infected with phage EJP2 at a MOI of 1. Optical density at 600 nm was measured every 1 h after phage infection until 12 h. SM buffer was added as a negative control. The experiments were conducted in triplicate.
Sequencing of phage DNA and bioinformatics analysis
Phage genomic DNA was extracted by phenol-chloroform method as previously described (Sambrook and Russell, 2001). The purified phage DNA was sequenced using the Illumina Miseq platform (Illumina, San Diego, CA, United States) and assembled with the SPAdeS v.3.13.0 (Bankevich et al., 2012) at Sanigen Inc., South Korea. The ORFs were predicted by using Glimmer3 (Delcher et al., 2007), GeneMarkS (Besemer et al., 2001), and RAST annotation server1 (Aziz et al., 2008; Overbeek et al., 2014). The annotated data were assorted and arranged by using Artemis (Carver et al., 2008). The tRNA sequence in the phage genome were analyzed by tRNAscan-SE program (Lowe and Eddy, 1997). The functions of phage proteins were predicted by using NCBI BLASTp and InterProscan program (Altschul et al., 1990; Jones et al., 2014). Sequence alignment among EJP2 and other phages was performed using ClustalW (Thompson et al., 1994) using amino acid sequences of phage terminase large subunit (TerL), major capsid protein (MCP) and portal vertex protein. Relationships among the phage genome sequences were inferred using neighbor-joining method (Saitou and Nei, 1987) and the phylogenetic tree was constructed using MEGA-X v10.0.5 (Kumar et al., 2018). The bootstrap value of 5,000 replicates represented the evolutionary history of the analyzed taxa (Felsenstein, 1985). The evolutionary distances were represented using p-distance method (Nei and Kumar, 2000). Dot plot analysis was conducted using Gepard v1.40 (Krumsiek et al., 2007).
Acridine orange and PNA treatment for curing the plasmids
Acridine orange (AO) was treated to E. coli FORC82 in order to cure the plasmids of E. coli FORC82 (Hirota, 1960). An overnight culture of E. coli FORC82 was sub-cultured to 3 ml of fresh LB broth with AO (100 μg/ml) and incubated at 37°C for 24 h. This process was repeated five times and serially diluted (10-fold) bacterial cells were streaked on LB agar plate. Plasmid curing was confirmed by colony PCR.
To cure pFORC82_1 which harbors a gene encoding ESBL from E. coli FORC82 cells, we used two peptide nucleic acids (PNAs, RepE-PNA1 and RepE-PNA2), which are composed of complementary sequences including predicted ribosome binding site and start codon, respectively, against replication initiation protein E of pFORC82_1 (Panagene, Daejeon, South Korea) (Table 2). All PNAs were covalently conjugated with peptide KFFKFFKFFK to improve cell-penetrating efficiency (Good et al., 2001). E. coli FORC82 cells were harvested at early log phase and diluted to 105 CFU/ml. The 20 μM RepE-PNA2 or the combination of PNA2 and Amp (50 μg/ml) were added to bacterial cell culture. The optical density at 600 nm of bacterial cell culture was measured every 15 min up to 7 h. The PNAs-treated cells were plated on the LB/Amp plates. Several colonies resuspended with 20 μl of PBS were spotted on LB and LB/Amp agar plate to distinguish the sensitivity against Amp. We selected clone which was sensitive to Amp and named the strain as E. coli PS01.
Construction of Tn5 transposon mutant library and screening phage resistance mutants
A Tn5 transposon mutant library was generated using the EZ-Tn5 kit as described by the manufacturer (LucigenⓇ, Middleton, WI, United States) with some modifications. Briefly, kanamycin resistance gene in plasmid pKD13 was amplified by polymerase chain reaction (PCR), wherein DNA fragment had inverted repeat sequence on both end (CTGTCTCTTATACACATCT). Purified DNA fragment (approximately 250 ng/μL) was mixed with 100% glycerol and EZ-Tn5 transposase and the mixture was incubated for 30 min at room temperature to construct transposome. One microliter of transposome was transformed by electroporation to E. coli PS01. To screen the phage-resistant clone, pools of transformants were mixed with EJP2 (approximately 109 PFU/mL) and incubated at 30°C for 30 min and the mixture was spread on LB/Kan agar plate. Surviving colonies were isolated by streaking three times on LB/Kan agar to remove the effect of remaining phages. Phage resistance against EJP2 was confirmed by spot assay. The locus of transposon insertion was identified by whole genome sequencing (WGS) using Illumina NextSeq platform (Illumina, San Diego, CA, United States). The sequenced DNA fragments were assembled using CLC Genomics Workbench 9.0.
Plasmid construction
The plasmids and oligonucleotides used in this study are listed in Table 1 and Table 3. Plasmid pFORC82_waaR (pUHE21-2 lacIq::waaR), which expresses FORC82_0109 gene (putative LPS α-1,2 glycosyltransferase), was constructed using isothermal assembly with the two DNA fragments; a PCR amplified linearized pUHE21-2 lacIq plasmid, and a PCR amplified FORC82_0109 gene. The two DNA fragments had overlapped sequences (20 bp) with each other (Gibson et al., 2009) and were inserted into the pFORC82_0109 by incubating at 50°C for 1 h in reaction buffer (25% PEG-8000, 500 nM Tris–HCl [pH 7.5], 50 mM MgCl2, 50 mM DTT, 1 mM of dNTPs, and 5 mM Nicotinamide adenine dinucleotide). The assembled plasmid was transformed to E. coli PS01 strain for complementation test.
Biofilm inhibition assay
Overnight cultures of E. coli FORC82 and other AMR E. coli strains were diluted 1:100 in 2 ml of fresh LB broth. EJP2 was added at MOI 0.1 or 1 in each well of a 96-well polystyrene plate containing E. coli FORC82 and incubated at 25°C without shaking for 48 h. Two hundred microliter of LB broth was used as a negative control. After incubation, biofilm staining was conducted as described by Cha et al. (2019) with some modifications. All wells were washed with PBS three times to remove vegetable cells. Biofilm was fixed with 95% methanol for 15 min and stained by 0.1% crystal violet (CV) for 30 min. Each well was washed with PBS to remove the residual CV. All wells were filled with 200 μl of 33% glacial acetic acid and incubated for 45 min at RT for dissolution of biofilm. The optical density at 570 nm of each well was measured.
Phage-antibiotic synergy
EJP2 phage was treated with cefotaxime (CTX) to investigate synergistic effect with antibiotics. Overnight-cultured E. coli FORC82 cells was sub-cultured 1:100 into fresh LB broth. EJP2 was treated to bacterial culture (5 × 106 CFU/ml) at a MOI of 1 with or without sublethal concentration of CTX (64 μg/ml). Mixtures were incubated at 30°C for 12 h. E. coli FORC82 cells were obtained every 1 h up to 3 h and diluted (10-fold) bacterial cells were plated on LB plates. The number of E. coli FORC82 cells was determined by counting the number of colonies on LB plates. The bacterial culture treated with LB broth was used as negative control.
Statistical analysis
GraphPad Prism 5 software was used for statistical analysis. Statistical analysis was conducted by one-way analysis of variance (ANOVA) with Tukey’s multiple comparison tests among the experimental groups (95% confidence interval). The significant differences among the experimental groups are marked with asterisks. p < 0.05 (*), or p < 0.001 (***).
Results and discussion
Isolation and morphological analysis of jumbo phage EJP2
The novel E. coli phage EJP2 was isolated from animal feces without filtration (Supplementary Figure S1). EJP2 could infect AMR E. coli FORC82 strain that contains mcr-1-harboring plasmid (Kim J. et al., 2019) and formed small clear plaques on LB soft agar (0.7%) over the bacterial lawn of E. coli FORC82. EJP2 plaques were rarely visible on a high concentration of LB soft agar (0.7%) (Figure 1A), indicating that EJP2 exhibits characteristic in common with Escherichia jumbo phages, in which plaque size decreased as the agar concentration increased (Saad et al., 2019).
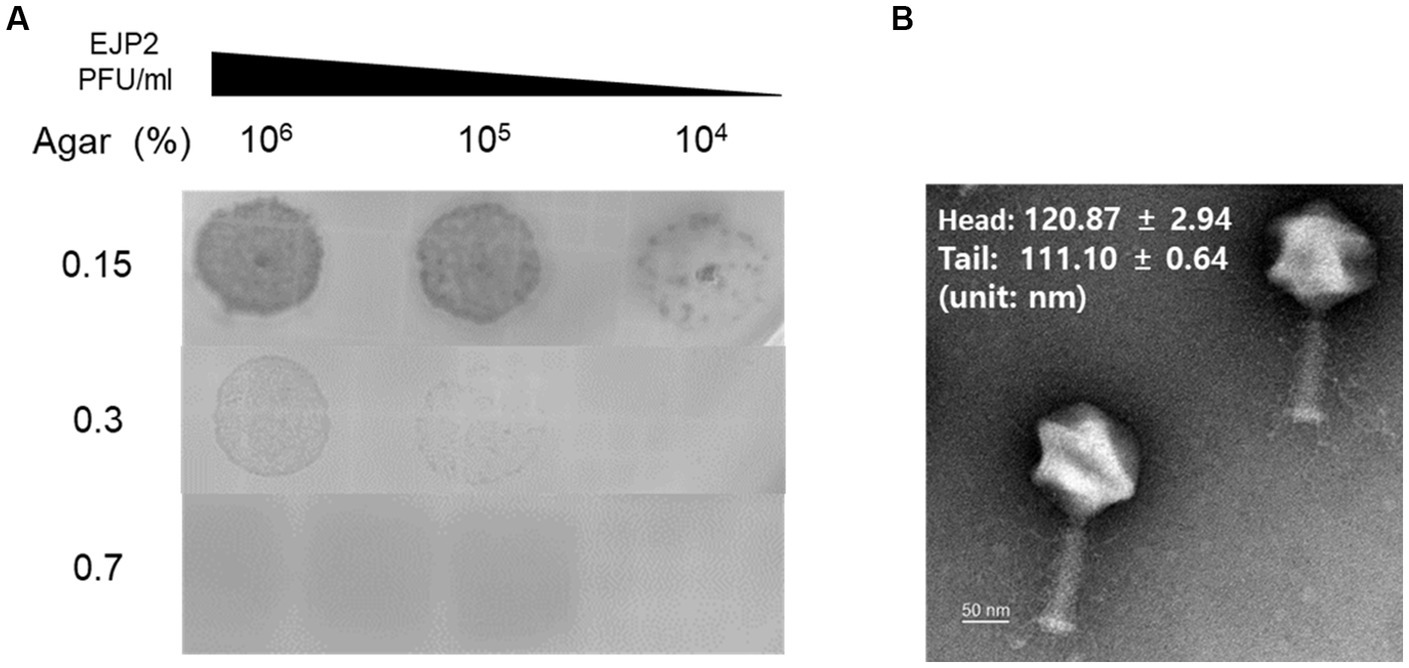
Figure 1. (A) Comparison of plaque size of EJP2 depending on the concentration of agar in LB soft agar. The concentrations of agar are shown on the left side of (A). The larger plaques of EJP2 were observed at low agar concentration. (B) Transmission electron micrographs of phage EJP2. Phage EJP2 particles were negatively stained with 2% aqueous uranyl acetate (pH 4.0). EJP2 belongs to the Myoviridae family. Bar, 50 nm.
Of over 220 jumbo phages reported to date, more than over hundred phages were classified as Myoviridae family (Yuan and Gao, 2017). The morphological analysis using transmission electron microscopy (TEM) demonstrated that phage EJP2 belongs to the Myoviridae family. Both the diameter of icosahedral head (120.9 ± 2.9 nm) and the length of contractile tail (111.1 ± 0.6 nm) were over 100 nm (Figure 1B), suggesting that EJP2 has a similar morphological feature compared to large virions classified as jumbo phages.
Bacterial growth inhibition efficacy of EJP2
Sixty-seven AMR E. coli isolates, pathogenic E. coli strains, and other Gram-negative strains were used to determine the host range of EJP2. Among 67 AMR E. coli strains, which were classified by the Clermont phylotyping (Clermont et al., 2013), EJP2 inhibited the growth of 33 AMR E. coli strains in phylogroup A (19/38), B1 (9/13), B2 (2/4), D (2/4), and E (1/6) (Figure 2A). This result indicates that EJP2 showed different host spectrum compared to those of five JEP coliphages reported by Kim et al. (2021). EJP2 showed a broader host range than those of JEP1, JEP6, JEP7 and JEP8 phages. JEP4 phage could infect AMR E. coli strain in phylogroup A (28/38) and D (3/4) (Kim et al., 2021), but EJP2 showed a broader inhibition spectrum against major phylogroups of AMR E. coli (A, B1, B2, D, and E) than JEP4 phage. EJP2 was also capable of forming plaques against Enterohemorrhagic E. coli (EHEC), Enterotoxigenic E. coli (ETEC), Enteroaggregative E. coli (EAEC), and Shigella flexneri (Figure 2B). In bacterial challenge assay, EJP2 could retard the growth of E. coli FORC82 4 h after infection at 30°C and 2 h after infection at 37°C (Supplementary Figure S2). The broad host spectrum of EJP2 implies its potential utility as biocontrol agents in clinical or food applications.
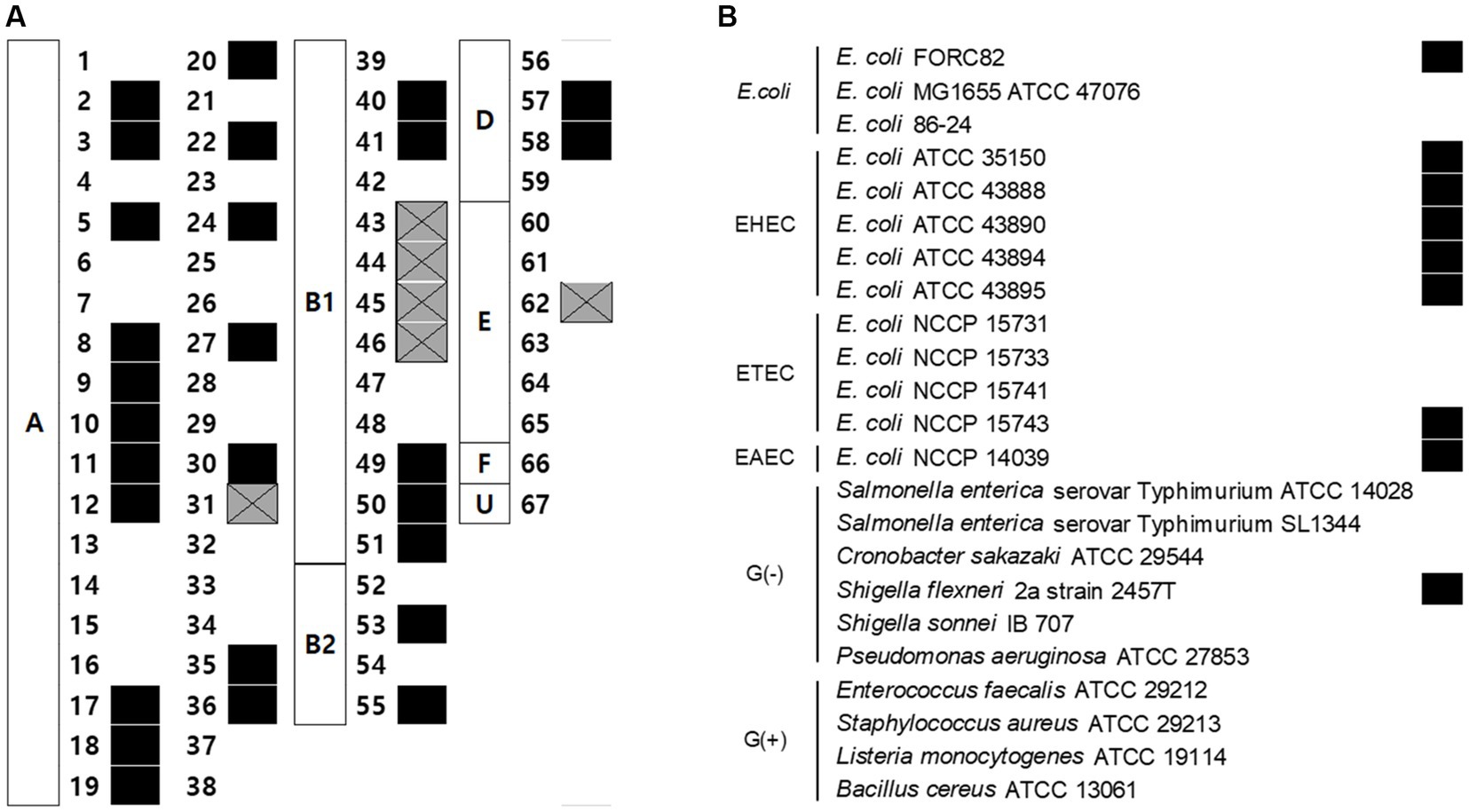
Figure 2. Antimicrobial spectrum of phage EJP2. (A) The susceptibility of 67 AMR E. coli against EJP2 was examined by using the spot assay. Alphabet in the white box indicates the phylogenetic groups of AMR E. coli. (B) Host range of EJP2 against pathogenic E. coli, other Gram-negative bacteria, and Gram-positive bacteria was determined by spot assay. Black squares indicate that EJP2 can form a plaque on the lawn of indicated bacterial strains. Gray squares with X mark present the growth inhibition zone. FORC, Food-borne pathogen Omics Research Center; ATCC, American Type Culture Collection; NCCP, National Culture Collection for Pathogens; EHEC, Enterohemorrhagic E. coli; ETEC, Enterotoxigenic E. coli; EAEC, Enteroaggregative E. coli; G(−), Gram-negative bacteria; G(+), Gram-positive bacteria.
Genomic and phylogenetic analysis of EJP2
Genomic characteristics of phage EJP2 were identified through whole-genome analysis. EJP2 possesses a 349,185 bp circular double-stranded DNA genome with average G + C content 37% and was thus classified as jumbo phage. EJP2 contains 540 putative ORFs and 6 genes encoding tRNAs (Figure 3A). Most of predicted genes (471 ORFs) encode hypothetical proteins with unknown functions. Of the 540 putative ORFs, only a small subset (12.8%, 69/540) were assigned putative functions (Supplementary Table S1). The analysis of phage life cycle is essential for developing phage therapeutic agent because temperate phages have the inherent capacity to transfer genes associated with bacterial virulence or antibiotic resistance by transduction (Howard-Varona et al., 2017). No genes associated with lysogenization, such as integrase, excisionase, transposase, superinfection immunity, repressor, and genome attachment site (attP) were predicted in the EJP2 genome, suggesting that EJP2 may be a virulent phage (Fogg et al., 2011; Davies et al., 2016). In addition, antibiotics resistance and virulence-associated genes were not identified in EJP2. BlastN analysis revealed that the whole genome of phage EJP2 shares less than 14% nucleotide identity with registered E. coli jumbo phages. Dot plot analysis presented low sequence homology with five Escherichia jumbo phages and one Salmonella jumbo phage at both the nucleotide and amino acid level (Supplementary Figure S3).
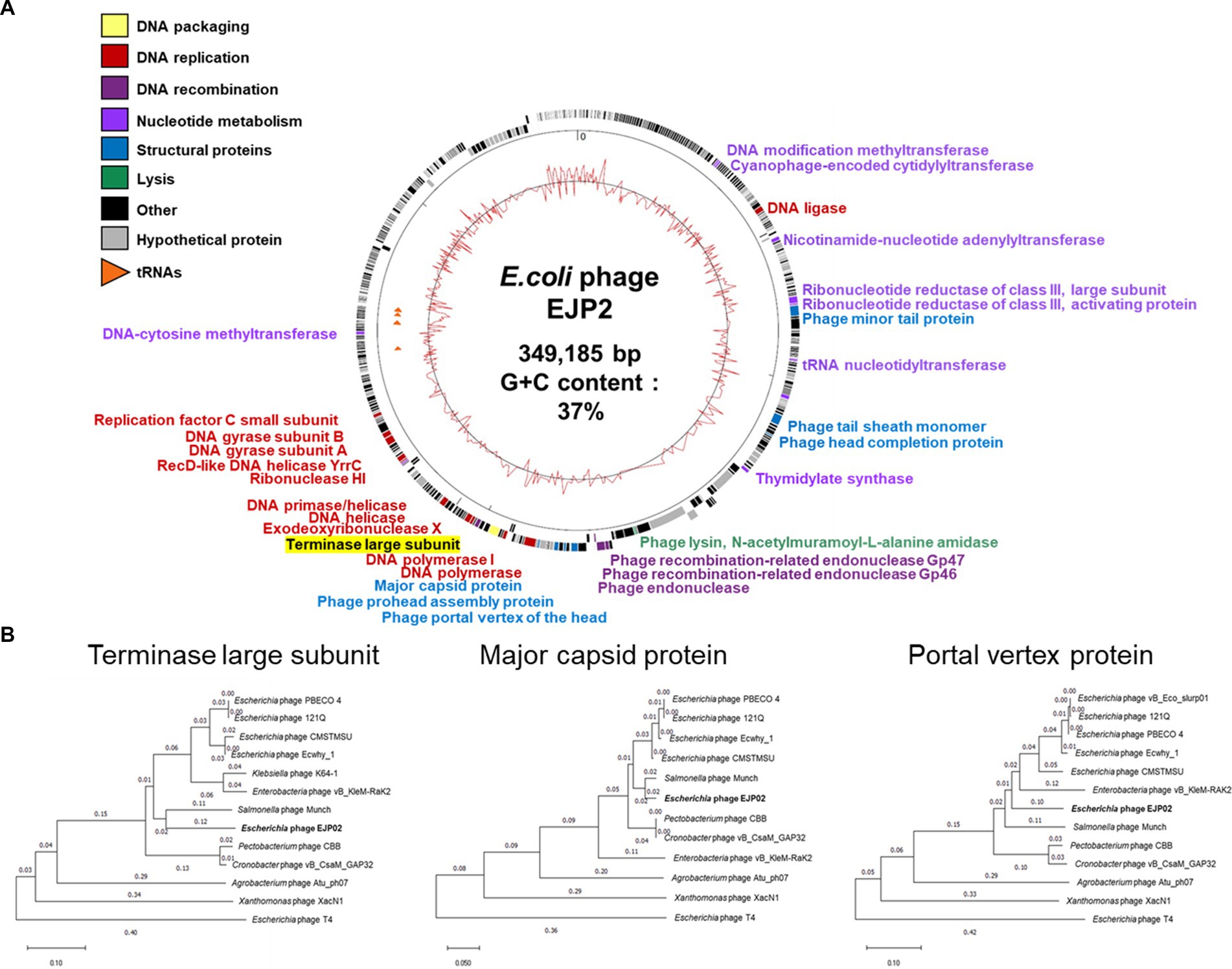
Figure 3. (A) Whole-genome map of phage EJP2. Predicted ORFs with the corresponding gene products are arranged on the EJP2 genome. Functional groups are categorized into colors. The GC content is presented as the inner track. (B) Phylogenetic tree comparing the terminase large subunit, major capsid protein, and portal vertex protein of phage EJP2 among other jumbo phages. Jumbo phages were selected as they share homology with the amino acid sequences of indicated protein using BlastP. Phage T4 was selected as an outgroup phage that is not closely related to the corresponding sequence of EJP2. Sequence relationships were inferred using the neighbor-joining method and evolutionary distances were computed using p-distance method by MegaX software.
As different from the small phage genomes, the putative functional genes of EJP2 were scattered throughout its genome. The function of ORFs were categorized into 6 groups; nucleotide metabolism, DNA replication, DNA recombination, DNA packaging, structure proteins, and lysis (Figure 3A). One noticeable features of jumbo phages is that they possess their own enzymes for DNA replication, recombination, and transcription, capable of self-replication independent of host machinery (Iyer et al., 2021). In EJP2 genome, four ORFs (EJP02_150, EJP02_151, EJP02_301, and EJP02_303) were annotated as ribonucleotide reductase subunit whose composes enzyme for synthesis of deoxyribonucleotides from ribonucleotides with the help of glutaredoxin 1 (EJP02_192) and thioredoxin (EJP02_485) (Dwivedi et al., 2013; Sengupta and Holmgren, 2014). Two ORFs were predicted as DNA polymerase (EJP02_261) and DNA polymerase I (EJP02_270) (Supplementary Table S1). EJP2 also encoded other 9 genes associated with DNA replication and 3 genes associated with DNA recombination, respectively. In addition to various genes involved in DNA metabolism, EJP2 possesses two proteins (RNA polymerase sigma factor D and RNA ligase) for its own transcription (Supplementary Table S1). Overall, the results of genome annotation of EJP2 suggest the evolution of EJP2 toward reduced dependency on the host bacterium. This finding would be helpful to explain the broad host spectrum of phage EJP2.
The MCP, TerL protein and portal vertex protein are major conserved proteins in phage genomes and are thus used as phylogenetic markers to organize the phage families through single gene analysis (Smith et al., 2013; Prevelige and Cortines, 2018). Analysis of phylogenetic trees based on these three genes revealed that EJP2 is grouped with Rak2-like phage family (Šimoliūnas et al., 2013), which includes E. coli phage PBECO4 (Kim et al., 2013), E. coli phage 121Q (accession number: NC_025447), Cronobacter phage vB_CsaM_GAP32 (Abbasifar et al., 2014), Enterobacteria phage vB_PcaM_CBB (Buttimer et al., 2017), and Salmonella phage Munch (accession number: MK268344.1). EJP2 appeared to be evolutionally closest to Salmonella phage Munch for three proteins (Figure 3B).
Phage EJP2 receptor analysis using Tn5 insertion mutant library
The host of EJP2, E. coli FORC82, harbors three F+ plasmids encoding various antibiotics resistance genes (Kim J. et al., 2019). We conducted plasmid curing by using acridine orange and PNA treatments to remove pFORC82_1, which carries most AMR genes. Unexpectedly, acridine orange treatment cured the pFORC82_2 (data not shown). Treatments of PNAs targeting RBS or start codon of plasmid replication protein (RepE) resulted in E. coli PS01 that was sensitive to β-lactam antibiotics (Supplementary Figure S4A). E. coli PS01 showed no difference in phage sensitivity compared to the E. coli FORC82 (Supplementary Figure S4B). We confirmed the deletion of about 6.3 kb region containing class A extended-spectrum β-lactamase (blaCTX-M-65) gene between IS26 family transposase genes (Tnp26), which is known to form transposons carrying antibiotic resistance genes (Harmer and Hall, 2016; Supplementary Figure S4C). A Tn5 insertional mutant library of E. coli PS01 was constructed to screen for phage EJP2 resistance. Two EJP2-resistant colonies were obtained and Tn5 insertion sites were identified by WGS as putative waaO (FORC82_RS00495, LPS 3-α galactosyltransferase) and putative waaR (FORC82_RS00500, LPS α-1,2 glucosyltransferase), both of which are associated with biosynthesis of LPS (Figure 4A). LPS 3-α galactosyltransferase is an enzyme that adds the galactose (GalI) to the first glucose (GlcI) to form branches of LPS (Pradel et al., 1992). Other reports describe that waaO gene encodes LPS α-1,3-glucosyltransferase or LPS α-1,3-galactosyltransferase, which adds hexose II residue to glucose I of outer core of LPS (Shibayama et al., 1998; Qian et al., 2014). LPS α-1,2 glucosyltransferase is an enzyme that adds the third glucose (GlcIII) to the second glucose (GlcII) in the outer core of E. coli LPS (Shibayama et al., 1999). Phage resistant mutant with Tn5 insertion in putative waaR gene was designated as E. coli PRS07. The susceptibility of E. coli PS01 and E. coli PRS07 to EJP2 was examined by spot assay. As expected, EJP2 could not form plaques on the lawn of E. coli PRS07 (Figure 4B). When the putative waaR gene was complemented using inducible plasmid pUHE21-2 lacIq, the sensitivity of EJP2 against PRS07 was completely restored, even without IPTG induction (Figure 4B). These results indicated that EJP2 recognizes LPS as a phage receptor and a third glucose of LPS outer core, or O-antigen would be required for EJP2 infection. LPS produced by E. coli varies depending on the pathogen type and this is due to the diversity in O-antigen structure (Liu et al., 2020). Moreover, the core region of LPS is known to have five different types and distribution of LPS core types is diverse among different phylogroups (Leclercq et al., 2021). Because of these differences in LPS structure, the sensitivity against EJP2 was different among E. coli strains (Figure 2).
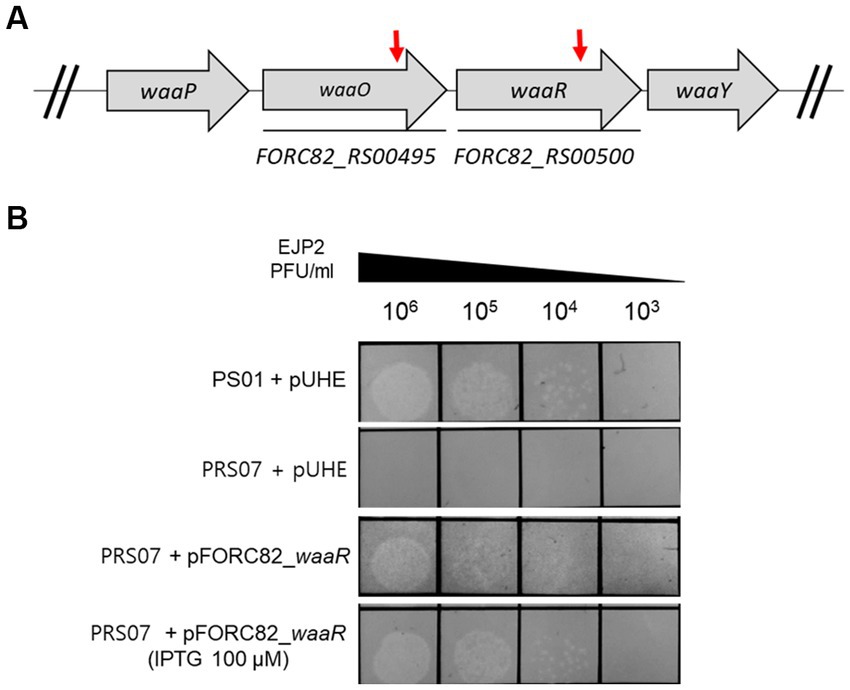
Figure 4. EJP2 receptor analysis. Schematic representation of the genes associated with LPS biosynthesis in E. coli FORC82 (A). Red arrows indicate genes disrupted by transposon insertion. Locus tags of genes were presented below each arrow. Complementation of the LPS biosynthesis gene (putative waaR, FORC82_RS00500) to identify the restored susceptibility against EJP2 in E. coli PS01 (B). The concentration of IPTG is presented in parentheses. LPS is a phage EJP2 receptor. One representative result of triplicate experiments is shown.
Biofilm inhibition assay
Biofilm formation by AMR E. coli cells can pose a serious threat in human health and food industry because this structure is generally resistant to the human immune system and shows increased-tolerance against antibiotic treatments (Sharma et al., 2016; Zhou et al., 2022). Bacteriophages are known to efficiently remove biofilms (Pires et al., 2017), and the ability of EJP2 to eradicate biofilms formed by AMR E. coli was tested. Before phage treatment, we tried to screen AMR E. coli strain whose biofilm-forming ability was higher than E. coli FORC82. AMR E. coli isolate 62 showed significantly higher biofilm formation on polystyrene surfaces than E. coli FORC82 (Figure 5A), thus we used E. coli isolate 62 for biofilm inhibition assay. EJP2 was added to each well at MOI 1 and 0.1 and its treatment reduced biofilm formation by more than 80% compared to the positive control (Figure 5B). These results imply that anti-biofilm capacity of EJP2 would be helpful in reducing disease caused by AMR E. coli infection such as catheter-associated urinary tract infection.
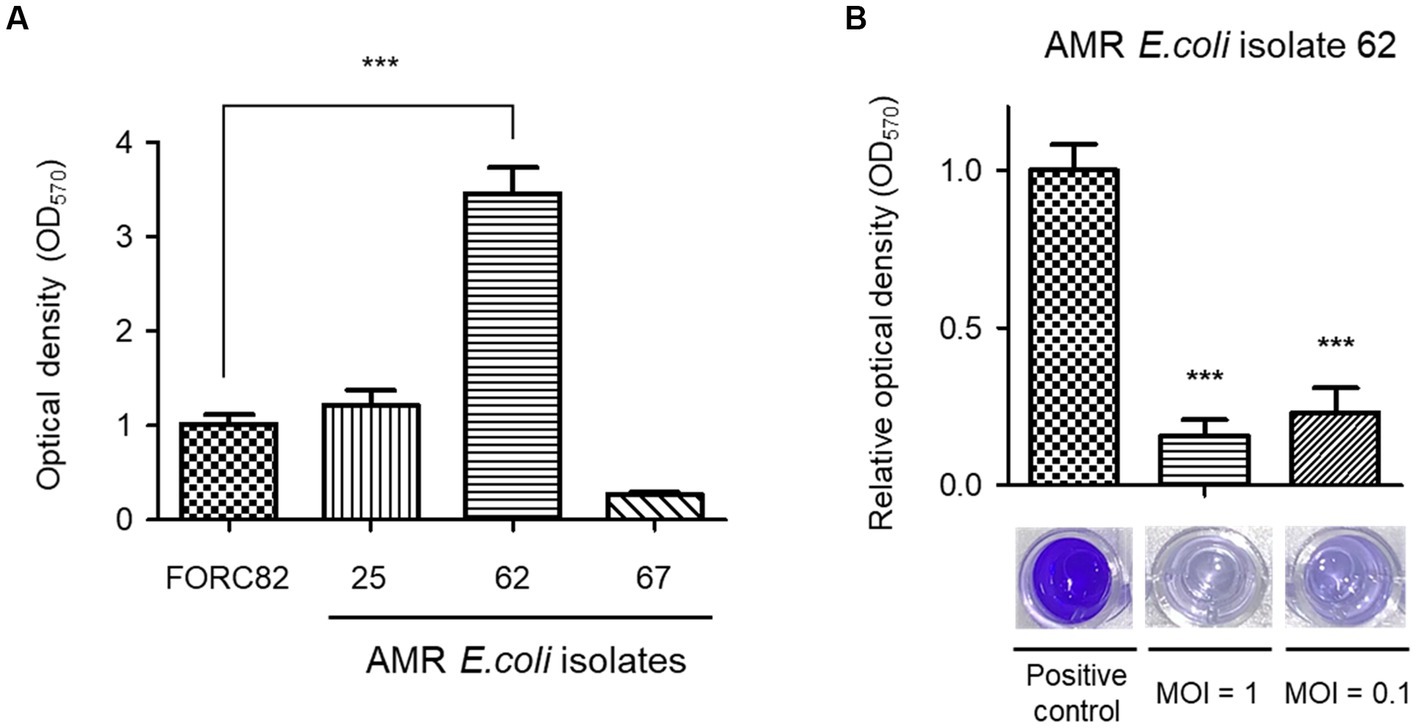
Figure 5. Comparison of biofilm formation of 4 AMR E. coli strains in 96-well polystyrene microplates (A). Biofilm removal efficacy of phage EJP2 (B). Biofilms of AMR E. coli isolate 62 were treated with EJP2 at a MOI of 1 and 0.1 and incubated for 48 h. Each column represents the mean of triplicate experiments, and error bars indicate the standard deviation. One representative result of triplicate experiments is shown. ***p < 0.001.
EJP2 and CTX synergy against AMR Escherichia coli FORC82
CTX, one of third generation cephalosporins, was selected to investigate its synergistic antimicrobial effects. CTX is considered by WHO as the “highest priority critically important antimicrobials” for human medicine (World Health Organization, 2017) and the E. coli FORC82 was highly resistant to CTX (MIC ≥128 μg/ml, data not shown). The combination of EJP2 and sublethal concentration of CTX (64 μg/ml) significantly reduced E. coli FORC82 population after 3 h of treatment compared to the separate treatment of EJP2 or CTX (Figure 6). Two studies reported synergistic bacterial lysis by combining CTX treatment with T4 and two other T4-like phages, CTX treatment shortened the latent period of those phages (Comeau et al., 2007; Ryan et al., 2012). The present study revealed that EJP2 recognizes LPS as a phage receptor (Figure 4). LPS is considered to be a permeability barrier against hydrophilic and hydrophobic compounds (Lehman and Grabowicz, 2019) and it is well-known that LPS truncation or modification increases susceptibility of E. coli against antibiotics, and detergents. LPS truncation caused by T4 phage infection led to hypersensitivity against food grade surfactant (Zhong et al., 2020) and rough type LPS by rfa gene knockouts increased sensitivity of E. coli to colistin (Burmeister et al., 2020). Similar to these findings, the increased susceptibility of E. coli FORC82 to CTX in combination treatment with EJP2 may be due to LPS modification caused by EJP2 infection, as bacteria generally modify their receptors to avoid the phage infection (Labrie et al., 2010). The synthesis of at least the third glucose in outer core of LPS is necessary for EJP2 infection (Figure 4), it is possible that EJP2-resistant E. coli FORC82 may have rough type LPS, potentially making CTX more accessible to outer membrane proteins, such as OmpC, and OmpF (Goltermann et al., 2022; Masi et al., 2022). The observed synergy between EJP2 and CTX suggests that EJP2 could be used as alternative and/or adjuvants to antibiotics, potentially reducing the use of antibiotics. For better understanding the mechanism of action about synergistic antimicrobial effects, further investigation is needed.
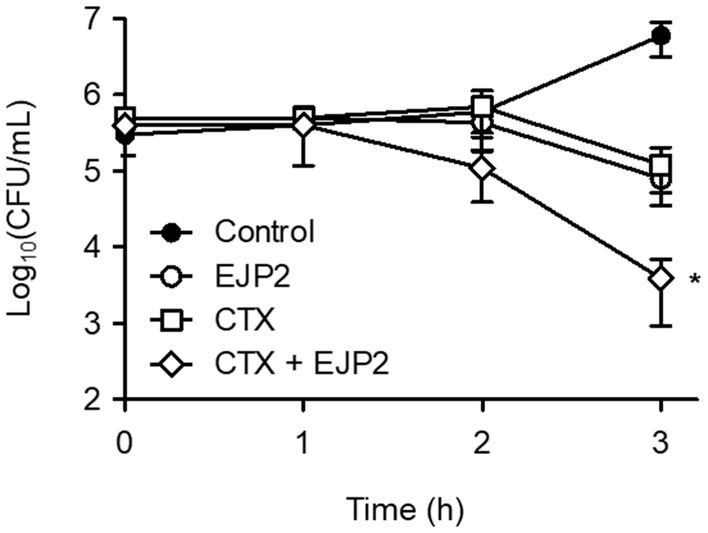
Figure 6. EJP2 and CTX synergy against E. coli FORC82. The antimicrobial activity of single treatment (EJP2, or CTX), and their combination were evaluated. Each Colony Forming Unit (CFU) was numerated after 3 h inoculation. The means with standard deviation of triplicate experiments are shown. *p < 0.05.
Conclusion
In this work, we have described AMR E. coli-specific jumbo phage EJP2 which was isolated from animal feces. EJP2 belongs to the Myoviridae family with a head and tail size of over 100 nm. Its genome contains 349,185 bp with 540 ORFs encoding genes for DNA replication, DNA recombination and nucleotide metabolism, DNA packaging, structural proteins, lysis, 6 tRNAs, and many genes whose functions remain unknown. Phylogenetic analyses of TerL, MCP, and portal vertex protein places EJP2 in a clade similar to E. coli and Salmonella jumbo phages, but with low sequence homology, suggesting a novel lineage for EJP2. Phage EJP2 exhibits a wide host range, biofilm removal activity, and synergistic effect with CTX against AMR E. coli, making it potentially a good candidate for the development of a biocontrol agent against diseases caused by AMR E. coli strains.
Data availability statement
The datasets presented in this study can be found in online repositories. The name of the repository and accession number can be found below: NCBI: OQ411014.
Author contributions
DJ, HK, and SR conceived and designed the experiments. DJ and HK carried out the main body of research, performed the experiments and bioinformatics analysis, and wrote the manuscript. DJ and YL contributed in phage isolation. JK contributed in provide a source and an information about AMR E. coli FORC82. SR supervised the work progress and edited the manuscript. All authors have read and approved the final manuscript.
Funding
This research was supported by a grant of the Korea Health Technology R&D Project through the Korea Health Industry Development Institute (KHIDI), funded by the Ministry of Health & Welfare, Republic of Korea (grant number: HI21C0901).
Conflict of interest
The authors declare that the research was conducted in the absence of any commercial or financial relationships that could be construed as a potential conflict of interest.
Publisher’s note
All claims expressed in this article are solely those of the authors and do not necessarily represent those of their affiliated organizations, or those of the publisher, the editors and the reviewers. Any product that may be evaluated in this article, or claim that may be made by its manufacturer, is not guaranteed or endorsed by the publisher.
Supplementary material
The Supplementary material for this article can be found online at: https://www.frontiersin.org/articles/10.3389/fmicb.2023.1194435/full#supplementary-material
Footnotes
References
Abbasifar, R., Griffiths, M. W., Sabour, P. M., Ackermann, H.-W., Vandersteegen, K., Lavigne, R., et al. (2014). Supersize me: Cronobacter sakazakii phage GAP32. Virology 460-461, 138–146. doi: 10.1016/j.virol.2014.05.003
Altschul, S. F., Gish, W., Miller, W., Myers, E. W., and Lipman, D. J. (1990). Basic local alignment search tool. J. Mol. Biol. 215, 403–410. doi: 10.1016/S0022-2836(05)80360-2
Aziz, R. K., Bartels, D., Best, A. A., Dejongh, M., Disz, T., Edwards, R. A., et al. (2008). The RAST server: rapid annotations using subsystems technology. BMC Genomics 9. doi: 10.1186/1471-2164-9-75
Bankevich, A., Nurk, S., Antipov, D., Gurevich, A. A., Dvorkin, M., Kulikov, A. S., et al. (2012). SPAdes: a new genome assembly algorithm and its applications to single-cell sequencing. J. Comput. Biol. 19, 455–477. doi: 10.1089/cmb.2012.0021
Besemer, J., Lomsadze, A., and Borodovsky, M. (2001). GeneMarkS: a self-training method for prediction of gene starts in microbial genomes. Implications for finding sequence motifs in regulatory regions. Nucleic Acids Res. 29, 2607–2618. doi: 10.1093/nar/29.12.2607
Bragg, R., Van Der Westhuizen, W., Lee, J.-Y., Coetsee, E., and Boucher, C. (2014). “Bacteriophages as potential treatment option for antibiotic resistant bacteria” in Infectious diseases and nanomedicine I (Midtown Manhattan, New York City: Springer), 97–110.
Burmeister, A. R., Fortier, A., Roush, C., Lessing, A. J., Bender, R. G., Barahman, R., et al. (2020). Pleiotropy complicates a trade-off between phage resistance and antibiotic resistance. Proc. Natl. Acad. Sci. 117, 11207–11216. doi: 10.1073/pnas.1919888117
Buttimer, C., Hendrix, H., Oliveira, H., Casey, A., Neve, H., Mcauliffe, O., et al. (2017). Things are getting hairy: Enterobacteria bacteriophage vB_PcaM_CBB. Front. Microbiol. 8:44. doi: 10.3389/fmicb.2017.00044
Carver, T., Berriman, M., Tivey, A., Patel, C., Böhme, U., Barrell, B. G., et al. (2008). Artemis and ACT: viewing, annotating and comparing sequences stored in a relational database. Bioinformatics 24, 2672–2676. doi: 10.1093/bioinformatics/btn529
Ceyssens, P.-J., Minakhin, L., Van Den Bossche, A., Yakunina, M., Klimuk, E., Blasdel, B., et al. (2014). Development of giant bacteriophage ϕKZ is independent of the host transcription apparatus. J. Virol. 88, 10501–10510. doi: 10.1128/JVI.01347-14
Cha, Y., Chun, J., Son, B., and Ryu, S. (2019). Characterization and genome analysis of staphylococcus aureus podovirus CSA13 and its anti-biofilm capacity. Viruses, 11:54.
Clermont, O., Christenson, J. K., Denamur, E., and Gordon, D. M. (2013). The Clermont Escherichia coli phylo-typing method revisited: improvement of specificity and detection of new phylo-groups. Environ. Microbiol. Rep. 5, 58–65. doi: 10.1111/1758-2229.12019
Comeau, A. M., Tétart, F., Trojet, S. N., Prere, M.-F., and Krisch, H. (2007). Phage-antibiotic synergy (PAS): β-lactam and quinolone antibiotics stimulate virulent phage growth. PLoS One 2:e799. doi: 10.1371/journal.pone.0000799
Coque, T., Baquero, F., and Canton, R. (2008). Increasing prevalence of ESBL-producing Enterobacteriaceae in Europe. Eur. Secur. 13:19044. doi: 10.2807/ese.13.47.19044-en
Datsenko, K. A., and Wanner, B. L. (2000). One-step inactivation of chromosomal genes in Escherichia coli K-12 using PCR products. Proc. Natl. Acad. Sci. 97, 6640–6645. doi: 10.1073/pnas.120163297
Davies, E. V., Winstanley, C., Fothergill, J. L., and James, C. E. (2016). The role of temperate bacteriophages in bacterial infection. FEMS Microbiol. Lett. 363. doi: 10.1093/femsle/fnw015
Dd Pitout, J. (2013). Enterobacteriaceae that produce extended-spectrum β-lactamases and AmpC β-lactamases in the community: the tip of the iceberg? Curr. Pharm. Des. 19, 257–263. doi: 10.2174/138161213804070348
Delcher, A. L., Bratke, K. A., Powers, E. C., and Salzberg, S. L. (2007). Identifying bacterial genes and endosymbiont DNA with glimmer. Bioinformatics 23, 673–679. doi: 10.1093/bioinformatics/btm009
Dwivedi, B., Xue, B., Lundin, D., Edwards, R. A., and Breitbart, M. (2013). A bioinformatic analysis of ribonucleotide reductase genes in phage genomes and metagenomes. BMC Evol. Biol. 13, 1–17. doi: 10.1186/1471-2148-13-33
Felsenstein, J. (1985). Phylogenies and the comparative method. Am. Nat. 125, 1–15. doi: 10.1086/284325
Ferrieres, L., Hemery, G., Nham, T., Guerout, A. M., Mazel, D., Beloin, C., et al. (2010). Silent mischief: bacteriophage mu insertions contaminate products of Escherichia coli random mutagenesis performed using suicidal transposon delivery plasmids mobilized by broad- host- range RP4 conjugative machinery. J. Bacteriol. 192, 6418–6427. doi: 10.1128/JB.00621-10
Fogg, P., Rigden, D., Saunders, J., Mccarthy, A., and Allison, H. (2011). Characterization of the relationship between integrase, excisionase and antirepressor activities associated with a superinfecting Shiga toxin encoding bacteriophage. Nucleic Acids Res. 39, 2116–2129. doi: 10.1093/nar/gkq923
Gibson, D. G., Young, L., Chuang, R.-Y., Venter, J. C., Hutchison, C. A., and Smith, H. O. (2009). Enzymatic assembly of DNA molecules up to several hundred kilobases. Nat. Methods 6, 343–345. doi: 10.1038/nmeth.1318
Goltermann, L., Zhang, M., Ebbensgaard, A. E., Fiodorovaite, M., Yavari, N., Løbner-Olesen, A., et al. (2022). Effects of LPS composition in Escherichia coli on antibacterial activity and bacterial uptake of antisense peptide-PNA conjugates. Front. Microbiol. 13. doi: 10.3389/fmicb.2022.877377
Good, L., Awasthi, S. K., Dryselius, R., Larsson, O., and Nielsen, P. E. (2001). Bactericidal antisense effects of peptide–PNA conjugates. Nat. Biotechnol. 19, 360–364. doi: 10.1038/86753
Guan, J., and Bondy-Denomy, J. (2020). Intracellular organization by jumbo bacteriophages. J. Bacteriol. 203, e00362–e00320. doi: 10.1128/JB.00362-20
Harmer, C. J., and Hall, R. M. (2016). IS26-mediated formation of transposons carrying antibiotic resistance genes. MSphere 1. doi: 10.1128/mSphere.00038-16
Hassan, A. Y., Lin, J. T., Ricker, N., and Anany, H. (2021). The age of phage: friend or foe in the new dawn of therapeutic and biocontrol applications? Pharmaceuticals 14:199. doi: 10.3390/ph14030199
Hirota, Y. (1960). The effect of acridine dyes on mating type factors in Escherichia coli. Proc. Natl. Acad. Sci. U. S. A. 46, 57–64. doi: 10.1073/pnas.46.1.57
Howard-Varona, C., Hargreaves, K. R., Abedon, S. T., and Sullivan, M. B. (2017). Lysogeny in nature: mechanisms, impact and ecology of temperate phages. ISME J. 11, 1511–1520. doi: 10.1038/ismej.2017.16
Huang, Y., Wang, W., Zhang, Z., Gu, Y., Huang, A., Wang, J., et al. (2022). Phage products for fighting antimicrobial resistance. Microorganisms 10:1324. doi: 10.3390/microorganisms10071324
Imam, M., Alrashid, B., Patel, F., Dowah, A. S., Brown, N., Millard, A., et al. (2019). vB_PaeM_MIJ3, a novel jumbo phage infecting Pseudomonas aeruginosa, possesses unusual genomic features. Front. Microbiol. 10:2772. doi: 10.3389/fmicb.2019.02772
Iyer, L. M., Anantharaman, V., Krishnan, A., Burroughs, A. M., and Aravind, L. (2021). Jumbo phages: a comparative genomic overview of core functions and adaptions for biological conflicts. Viruses 13:63. doi: 10.3390/v13010063
Jones, P., Binns, D., Chang, H.-Y., Fraser, M., Li, W., Mcanulla, C., et al. (2014). InterProScan 5: genome-scale protein function classification. Bioinformatics 30, 1236–1240. doi: 10.1093/bioinformatics/btu031
Kim, M. S., Hong, S. S., Park, K., and Myung, H. (2013). Genomic analysis of bacteriophage PBECO4 infecting Escherichia coli O157: H7. Arch. Virol. 158, 2399–2403. doi: 10.1007/s00705-013-1718-3
Kim, J., Hwang, B. K., Choi, H., Wang, Y., Choi, S. H., Ryu, S., et al. (2019). Characterization of mcr-1-harboring plasmids from pan drug-resistant Escherichia coli strains isolated from retail raw chicken in South Korea. Microorganisms 7:344. doi: 10.3390/microorganisms7090344
Kim, H., Kim, M., Bai, J., Lim, J.-A., Heu, S., and Ryu, S. (2019). Colanic acid is a novel phage receptor of Pectobacterium carotovorum subsp. carotovorum phage POP72. Front. Microbiol. 10. doi: 10.3389/fmicb.2019.00143
Kim, J., Park, H., Ryu, S., and Jeon, B. (2021). Inhibition of antimicrobial-resistant Escherichia coli using a broad host range phage cocktail targeting various bacterial phylogenetic groups. Front. Microbiol. 12:699630. doi: 10.3389/fmicb.2021.699630
Kim, M., and Ryu, S. (2011). Characterization of a T5-like coliphage, SPC35, and differential development of resistance to SPC35 in Salmonella enterica serovar typhimurium and Escherichia coli. Appl. Environ. Microbiol. 77, 2042–2050. doi: 10.1128/AEM.02504-10
Krumsiek, J., Arnold, R., and Rattei, T. (2007). Gepard: a rapid and sensitive tool for creating dotplots on genome scale. Bioinformatics 23, 1026–1028. doi: 10.1093/bioinformatics/btm039
Kumar, S., Stecher, G., Li, M., Knyaz, C., and Tamura, K. (2018). MEGA X: molecular evolutionary genetics analysis across computing platforms. Mol. Biol. Evol. 35, 1547–1549. doi: 10.1093/molbev/msy096
Kutter, E., and Sulakvelidze, A. (2004). Bacteriophages: Biology and applications Boca Raton, Florida, United States: CRC press.
Labrie, S. J., Samson, J. E., and Moineau, S. (2010). Bacteriophage resistance mechanisms. Nat. Rev. Microbiol. 8, 317–327. doi: 10.1038/nrmicro2315
Lavysh, D., Sokolova, M., Minakhin, L., Yakunina, M., Artamonova, T., Kozyavkin, S., et al. (2016). The genome of AR9, a giant transducing Bacillus phage encoding two multisubunit RNA polymerases. Virology 495, 185–196. doi: 10.1016/j.virol.2016.04.030
Leclercq, S. O., Branger, M., Smith, D. G., and Germon, P. (2021). Lipopolysaccharide core type diversity in the Escherichia coli species in association with phylogeny, virulence gene repertoire and distribution of type VI secretion systems. Microbial. Genomics 7. doi: 10.1099/mgen.0.000652
Lehman, K. M., and Grabowicz, M. (2019). Countering gram-negative antibiotic resistance: recent progress in disrupting the outer membrane with novel therapeutics. Antibiotics 8:163. doi: 10.3390/antibiotics8040163
Leskinen, K., Blasdel, B. G., Lavigne, R., and Skurnik, M. (2016). RNA-sequencing reveals the progression of phage-host interactions between φR1-37 and Yersinia enterocolitica. Viruses 8:111. doi: 10.3390/v8040111
Liu, B., Furevi, A., Perepelov, A. V., Guo, X., Cao, H., Wang, Q., et al. (2020). Structure and genetics of Escherichia coli O antigens. FEMS Microbiol. Rev. 44, 655–683. doi: 10.1093/femsre/fuz028
Loc-Carrillo, C., and Abedon, S. T. (2011). Pros and cons of phage therapy. Bacteriophage 1, 111–114. doi: 10.4161/bact.1.2.14590
Lowe, T. M., and Eddy, S. R. (1997). tRNAscan-SE: A program for improved detection of transfer RNA genes in genomic sequence. Nucleic Acids Res. 25, 955–964. doi: 10.1093/nar/25.5.955
Martak, D., Guther, J., Verschuuren, T. D., Valot, B., Conzelmann, N., Bunk, S., et al. (2022). Populations of extended-spectrum β-lactamase-producing Escherichia coli and Klebsiella pneumoniae are different in human-polluted environment and food items: A multicentre European study. Clin. Microbiol. Infect. 28, 447.e7. e414–447.e14. doi: 10.1016/j.cmi.2021.07.022
Masi, M., Vergalli, J., Ghai, I., Barba-Bon, A., Schembri, T., Nau, W. M., et al. (2022). Cephalosporin translocation across enterobacterial OmpF and OmpC channels, a filter across the outer membrane. Commun. Biol. 5:1059. doi: 10.1038/s42003-022-04035-y
Mesyanzhinov, V. V., Robben, J., Grymonprez, B., Kostyuchenko, V. A., Bourkaltseva, M. V., Sykilinda, N. N., et al. (2002). The genome of bacteriophage φKZ of Pseudomonas aeruginosa. J. Mol. Biol. 317, 1–19. doi: 10.1006/jmbi.2001.5396
Nazir, A., Ali, A., Qing, H., and Tong, Y. (2021). Emerging aspects of jumbo bacteriophages. Infect. Drug Resist. 14, 5041–5055. doi: 10.2147/IDR.S330560
Nei, M., and Kumar, S. (2000). Molecular evolution and phylogenetics. Oxford: Oxford University Press.
Orekhova, M., Koreshova, A., Artamonova, T., Khodorkovskii, M., and Yakunina, M. (2019). The study of the phiKZ phage non-canonical non-virion RNA polymerase. Biochem. Biophys. Res. Commun. 511, 759–764. doi: 10.1016/j.bbrc.2019.02.132
Overbeek, R., Olson, R., Pusch, G. D., Olsen, G. J., Davis, J. J., Disz, T., et al. (2014). The SEED and the rapid annotation of microbial genomes using subsystems technology (RAST). Nucleic Acids Res. 42, D206–D214. doi: 10.1093/nar/gkt1226
Park, H., Kim, J., Ryu, S., and Jeon, B. (2019). Predominance of blaCTX-M-65 and blaCTX-M-55 in extended-spectrum β-lactamase-producing Escherichia coli from raw retail chicken in South Korea. J. Global Antimicrob. Resist. 17, 216–220. doi: 10.1016/j.jgar.2019.01.005
Parvez, S., and Khan, A. U. (2018). Hospital sewage water: a reservoir for variants of New Delhi metallo-β-lactamase (NDM)-and extended-spectrum β-lactamase (ESBL)-producing Enterobacteriaceae. Int. J. Antimicrob. Agents 51, 82–88. doi: 10.1016/j.ijantimicag.2017.08.032
Pires, D. P., Melo, L. D., Boas, D. V., Sillankorva, S., and Azeredo, J. (2017). Phage therapy as an alternative or complementary strategy to prevent and control biofilm-related infections. Curr. Opin. Microbiol. 39, 48–56. doi: 10.1016/j.mib.2017.09.004
Platt, R., Drescher, C., Park, S.-K., and Phillips, G. J. (2000). Genetic system for reversible integration of DNA constructs and lacZ gene fusions into the Escherichia coli chromosome. Plasmid 43, 12–23. doi: 10.1006/plas.1999.1433
Poirel, L., Madec, J.-Y., Lupo, A., Schink, A.-K., Kieffer, N., Nordmann, P., et al. (2018). Antimicrobial resistance in Escherichia coli. Microbiology. Spectrum 6:6.4. 14. doi: 10.1128/microbiolspec.ARBA-0026-2017
Pradel, E., Parker, C. T., and Schnaitman, C. A. (1992). Structures of the rfaB, rfaI, rfaJ, and rfaS genes of Escherichia coli K-12 and their roles in assembly of the lipopolysaccharide core. J. Bacteriol. 174, 4736–4745. doi: 10.1128/jb.174.14.4736-4745.1992
Prevelige, P. E. Jr., and Cortines, J. R. (2018). Phage assembly and the special role of the portal protein. Curr. Opin. Virol. 31, 66–73. doi: 10.1016/j.coviro.2018.09.004
Qian, J., Garrett, T. A., and Raetz, C. R. (2014). In vitro assembly of the outer core of the lipopolysaccharide from Escherichia coli K-12 and Salmonella typhimurium. Biochemistry 53, 1250–1262. doi: 10.1021/bi4015665
Roca, I., Akova, M., Baquero, F., Carlet, J., Cavaleri, M., Coenen, S., et al. (2015). The global threat of antimicrobial resistance: science for intervention. New Microbes New Infect. 6, 22–29. doi: 10.1016/j.nmni.2015.02.007
Rupp, M. E., and Fey, P. D. (2003). Extended spectrum β-lactamase (ESBL)-producing Enterobacteriaceae. Drugs 63, 353–365. doi: 10.2165/00003495-200363040-00002
Ryan, E. M., Alkawareek, M. Y., Donnelly, R. F., and Gilmore, B. F. (2012). Synergistic phage-antibiotic combinations for the control of Escherichia coli biofilms in vitro.FEMS Immunol. Med. Microbiol. 65, 395–398. doi: 10.1111/j.1574-695X.2012.00977.x
Saad, A. M., Soliman, A. M., Kawasaki, T., Fujie, M., Nariya, H., Shimamoto, T., et al. (2019). Systemic method to isolate large bacteriophages for use in biocontrol of a wide-range of pathogenic bacteria. J. Biosci. Bioeng. 127, 73–78. doi: 10.1016/j.jbiosc.2018.07.001
Saitou, N., and Nei, M. (1987). The neighbor-joining method: a new method for reconstructing phylogenetic trees. Mol. Biol. Evol. 4, 406–425.
Sambrook, J., and Russell, D. (2001). Molecular cloning: a laboratory manual 1. 3rd Cold Spring Harbor. New York, NY: 6.4–6.12
Sengupta, R., and Holmgren, A. (2014). Thioredoxin and glutaredoxin-mediated redox regulation of ribonucleotide reductase. World J. Biol. Chem. 5, 68–74. doi: 10.4331/wjbc.v5.i1.68
Sharma, G., Sharma, S., Sharma, P., Chandola, D., Dang, S., Gupta, S., et al. (2016). Escherichia coli biofilm: development and therapeutic strategies. J. Appl. Microbiol. 121, 309–319. doi: 10.1111/jam.13078
Shibayama, K., Ohsuka, S., Sato, K., Yokoyama, K., Horii, T., and Ohta, M. (1999). Four critical aspartic acid residues potentially involved in the catalytic mechanism of Escherichia coli K-12 WaaR. FEMS Microbiol. Lett. 174, 105–109. doi: 10.1111/j.1574-6968.1999.tb13555.x
Shibayama, K., Ohsuka, S., Tanaka, T., Arakawa, Y., and Ohta, M. (1998). Conserved structural regions involved in the catalytic mechanism of Escherichia coli K-12 WaaO (RfaI). J. Bacteriol. 180, 5313–5318. doi: 10.1128/JB.180.20.5313-5318.1998
Shrivastava, S. R., Shrivastava, P. S., and Ramasamy, J. (2018). World health organization releases global priority list of antibiotic-resistant bacteria to guide research, discovery, and development of new antibiotics. J. Med. Society 32:76. doi: 10.4103/jms.jms_25_17
Šimoliūnas, E., Kaliniene, L., Truncaitė, L., Zajančkauskaitė, A., Staniulis, J., Kaupinis, A., et al. (2013). Klebsiella phage vB_KleM-RaK2—A giant singleton virus of the family Myoviridae. PLoS One 8. doi: 10.1371/journal.pone.0060717
Smith, K. C., Castro-Nallar, E., Fisher, J. N., Breakwell, D. P., Grose, J. H., and Burnett, S. H. (2013). Phage cluster relationships identified through single gene analysis. BMC Genom. 14, 1–16. doi: 10.1186/1471-2164-14-410
Soncini, F. C., Véscovi, E. G., and Groisman, E. A. (1995). Transcriptional autoregulation of the Salmonella typhimurium phoPQ operon. J. Bacteriol. 177, 4364–4371. doi: 10.1128/jb.177.15.4364-4371.1995
Thompson, J. D., Higgins, D. G., and Gibson, T. J. (1994). CLUSTAL W: improving the sensitivity of progressive multiple sequence alignment through sequence weighting, position-specific gap penalties and weight matrix choice. Nucleic Acids Res. 22, 4673–4680. doi: 10.1093/nar/22.22.4673
Walker, P. J., Siddell, S. G., Lefkowitz, E. J., Mushegian, A. R., Adriaenssens, E. M., Alfenas-Zerbini, P., et al. (2022). Recent changes to virus taxonomy ratified by the international committee on taxonomy of viruses (2022). Arch. Virol. 167, 2429–2440. doi: 10.1007/s00705-022-05516-5
World Health Organization. (2017). Critically important antimicrobials for human medicine: ranking of antimicrobial agents for risk management of antimicrobial resistance due to non-human use.
Wu, C., Wang, Y., Shi, X., Wang, S., Ren, H., Shen, Z., et al. (2018). Rapid rise of the ESBL and mcr-1 genes in Escherichia coli of chicken origin in China, 2008–2014. Emerg. Microbes Infect. 7, 1–10. doi: 10.1038/s41426-018-0033-1
Yoshikawa, G., Askora, A., Blanc-Mathieu, R., Kawasaki, T., Li, Y., Nakano, M., et al. (2018). Xanthomonas citri jumbo phage XacN1 exhibits a wide host range and high complement of tRNA genes. Sci. Rep. 8:4486. doi: 10.1038/s41598-018-22239-3
Yuan, Y., and Gao, M. (2017). Jumbo bacteriophages: an overview. Front. Microbiol. 8:403. doi: 10.3389/fmicb.2017.00403
Zhong, Z., Emond-Rheault, J.-G., Bhandare, S., Lévesque, R., and Goodridge, L. (2020). Bacteriophage-induced lipopolysaccharide mutations in Escherichia coli lead to hypersensitivity to food grade surfactant sodium dodecyl sulfate. Antibiotics 9:552. doi: 10.3390/antibiotics9090552
Zhou, F., Wang, D., Hu, J., Zhang, Y., Tan, B. K., and Lin, S. (2022). Control measurements of Escherichia coli biofilm: A review. Foods 11:2469. doi: 10.3390/foods11162469
Zurfuh, K., Poirel, L., Nordmann, P., Nüesch-Inderbinen, M., Hächler, H., and Stephan, R. (2016). Occurrence of the plasmid-borne mcr-1 colistin resistance gene in ESBL-producing Enterobacteriacae in river water and imported vegetable samples in Switzerland. Antimicrob Agents Chemother 60, 2594–2595. doi: 10.1128/AAC.00066-16
Keywords: bacteriophage, jumbo phage, antimicrobial resistance, Escherichia coli , antimicrobial agent, genome, phylogenetic analysis
Citation: Jo D, Kim H, Lee Y, Kim J and Ryu S (2023) Characterization and genomic study of EJP2, a novel jumbo phage targeting antimicrobial resistant Escherichia coli. Front. Microbiol. 14:1194435. doi: 10.3389/fmicb.2023.1194435
Edited by:
Mark Willcox, University of New South Wales, AustraliaReviewed by:
Joseph Atia Ayariga, Alabama State University, United StatesHector Quezada, Hospital Infantil de México Federico Gómez, Mexico
Copyright © 2023 Jo, Kim, Lee, Kim and Ryu. This is an open-access article distributed under the terms of the Creative Commons Attribution License (CC BY). The use, distribution or reproduction in other forums is permitted, provided the original author(s) and the copyright owner(s) are credited and that the original publication in this journal is cited, in accordance with accepted academic practice. No use, distribution or reproduction is permitted which does not comply with these terms.
*Correspondence: Sangryeol Ryu, sangryu@snu.ac.kr
†These authors have contributed equally to this work