- 1Instituto de Tecnologia Química e Biológica António Xavier (ITQB NOVA), Universidade Nova de Lisboa, Oeiras, Portugal
- 2Department of Life Sciences Core Facilities, Weizmann Institute of Science, Rehovot, Israel
- 3Instituto Universitário Militar, Centro de Investigação da Academia Militar (CINAMIL), Unidade Militar Laboratorial de Defesa Biológica e Química (UMLDBQ), Lisbon, Portugal
- 4Department of Chemical and Biological Physics, Weizmann Institute of Science, Rehovot, Israel
- 5Department of Chemical Research Support, Weizmann Institute of Science, Rehovot, Israel
- 6Instituto de Biologia Experimental e Tecnológica (iBET), Oeiras, Portugal
Arsenic (As) is a toxic heavy metal widely found in the environment that severely undermines the integrity of water resources. Bioremediation of toxic compounds is an appellative sustainable technology with a balanced cost-effective setup. To pave the way for the potential use of Deinococcus indicus, an arsenic resistant bacterium, as a platform for arsenic bioremediation, an extensive characterization of its resistance to cellular insults is paramount. A comparative analysis of D. indicus cells grown in two rich nutrient media conditions (M53 and TGY) revealed distinct resistance patterns when cells are subjected to stress via UV-C and methyl viologen (MV). Cells grown in M53 demonstrated higher resistance to both UV-C and MV. Moreover, cells grow to higher density upon exposure to 25 mM As(V) in M53 in comparison with TGY. This analysis is pivotal for the culture of microbial species in batch culture bioreactors for bioremediation purposes. We also demonstrate for the first time the presence of polyphosphate granules in D. indicus which are also found in a few Deinococcus species. To extend our analysis, we also characterized DiArsC2 (arsenate reductase) involved in arsenic detoxification and structurally determined different states, revealing the structural evidence for a catalytic cysteine triple redox system. These results contribute for our understanding into the D. indicus resistance mechanism against stress conditions.
Introduction
Arsenic presents high toxicity and has been classified as carcinogenic, mutagenic and teratogenic element (Machado et al., 1999; National Research Council, 2001; Wang et al., 2017). Due to the electronic configuration of its valence orbitals, it presents a unique chemical nature that allows several changes in oxidation states and bonding configurations, forming a wide diversity of inorganic and organic species (O’Day, 2006). In nature, four oxidation states are found, namely −3 (arsine), 0 (arsenic), +3 (arsenite) and +5 (arsenate); the latter two are of the utmost interest due to their high toxicity and predominance in aquatic environments (Bissen et al., 2003; James et al., 2017). Arsenite, As(III), is mainly present in groundwater under reducing conditions while arsenate, As(V) is present in oxidizing conditions (Bissen et al., 2003; James et al., 2017). As a metalloid, arsenic is a pnictogen found in group 15 of the periodic table and presents high physicochemical similarities with phosphorus (Dani, 2011; Fekry et al., 2011; Tawfik and Viola, 2011; Varadwaj et al., 2022). The uptake of arsenate by cells follows through the phosphorus pathways mainly by phosphate transporters (Rosenberg et al., 1977; Jiang et al., 2014; Garbinski et al., 2019). Arsenate (AsO4−3) and phosphate (PO4−3) share similar chemical speciation, thus as a chemical analog of phosphorus, arsenate is able to act as substrate for several enzymes, leading to harmful cellular effects (Strawn, 2018). To cope with arsenic, most living organisms have evolved mechanisms to circumvent its toxicity. Most bacterial species bear an arsenic resistance (ars) operon that encompasses at least three main core genes: (1) an arsenic responsive repressor (arsR), (2) As(III) efflux permease (arsB) and (3) arsenate reductase (arsC) (Páez-Espino et al., 2009; Wang et al., 2016; Yang and Rosen, 2016). Deinococcus indicus Wt/1aT was isolated from an arsenic contaminated aquifer in West Bengal, India and it is the first known species of the Deinococcus genus able to withstand high concentrations of both arsenate and arsenite (Suresh et al., 2004). D. indicus contains the typical arsRBC operon that encodes two regulatory proteins (ArsR1 and ArsR2), two arsenate reductases (ArsC2 and ArsC3) and an arsenite efflux pump protein (ArsB). Besides this ars operon, it also encodes an additional arsenate reductase, ArsC1 (Suresh et al., 2004; Tawfik and Viola, 2011; Ranganathan et al., 2023).
The Deinococcus genus is widespread in the environment, colonizing extreme habitats such as deserts, hot springs and polar regions (Hirsch et al., 2004; De Groot et al., 2005; Makk et al., 2016; Jin et al., 2019). Members of this genus present high resistance to radiation and desiccation (Jin et al., 2019). Nevertheless, with the exception of Deinococcus radiodurans, the model organism for radiation resistance, and Deinococcus geothermalis, a biofilm forming organism that heavily affects the paper industry, most Deinococcus sp. have been poorly characterized to date (Kolari et al., 2001, 2003; Peltola et al., 2008; Slade and Radman, 2011; Gerber et al., 2015; Lim et al., 2019). D. radiodurans and D. geothermalis have shown high efficiency in the detoxification of toxic materials (Brim et al., 2000, 2003; Misra et al., 2012; Choi et al., 2017; Manobala et al., 2019). Nonetheless, before exploring the bioremediation capabilities of an organism, the characterization of its resistance to cellular insults is imperative. Moreover, the analysis of culture conditions is vital for the application of microbial species in batch bioreactors for toxic waste removal (Tekere, 2019). So far, D. indicus ability to survive under UV-B radiation was evaluated and no information is available regarding oxidative stress resistance (Suresh et al., 2004).
Here, D. indicus resistance to UV-C, oxidative stress and arsenate was investigated in two rich nutritional media (M53 and TGY). Interestingly, a media-dependent response was observed for UV-C and methyl viologen (MV) damage, with D. indicus presenting higher resistance in M53. Upon exposure to As(V), D. indicus was able to grow in the presence of 25 mM of As(V) and to tolerate 50 mM of As(V) while D. radiodurans had its growth compromised in the presence of 50 fold lower concentration. Polyphosphate granules have been shown to be involved in heavy metal detoxification in different microorganisms, and indeed elemental analysis by cryogenic scanning transmission electron microscopy with energy dispersive X-ray spectroscopy (STEM-EDX) revealed the presence of prominent polyphosphate granules in D. indicus. To extend our understanding of arsenic resistance in D. indicus, the crystal structure of DiArsC2 arsenate reductase was determined in two states: native and bound to arsenic.
Materials and methods
Deinococcus cell growth
Deinococcus radiodurans R1 and D. indicus Wt/1aT (MTCC 4913) were grown either in: M53 medium, 1.0% (w/v) casein yeast peptone (Sigma-Aldrich), 0.5% (w/v) yeast extract (VWR Chemicals), 0.5% (w/v) glucose (Carl Roth) and 0.5% (w/v) NaCl (Merck); or Tryptone Glucose Yeast extract (TGY) medium, 1.0% (w/v) tryptone (VWR Chemicals), 0.5% (w/v) yeast extract (VWR Chemicals) and 0.1% (w/v) glucose (Carl Roth). For all assays, cells were grown at 30°C and 150 rpm agitation; for solid cultures, 1.5% (w/v) agar-agar (Carl Roth) was added. For each assay, two sequential subcultures were grown for 14–16 h prior to the final culture. Final cultures were diluted to an early-exponential phase either (Optical density at 600 nm (OD600) of 0.2) for arsenate exposure growth curves and STEM-EDX or (OD600 = 0.5) for oxidative stress, in-plate arsenate stress and UV assays.
Oxidative and arsenate stress response
Oxidative and arsenate stress assays were performed by adapting the disk diffusion method (Bauer et al., 1966; Hudzicki, 2012). Briefly, 400 μl of cells grown in either M53 or TGY (at an OD600 = 2) were evenly spread on the surface of an agar plate (120.5 × 120.5 mm) using a cotton swab and allowed to dry for 15 min before the application of the disks (6 mm, PRAT DUMAS). Afterwards, oxidative stress inducer compounds or arsenic (20 μl) were applied on top of the disk in increasing concentrations. Plates were incubated for 3 days and halos were measured using Fiji/ImageJ (Schindelin et al., 2012). Oxidative stress compounds: (1) Methyl viologen dichloride, MV (C12H14Cl2N2· xH2O, Sigma-Aldrich); (2) Hydrogen peroxide 30% (w/w), H2O2 (Sigma-Aldrich). Arsenic stress compound: sodium arsenate dibasic heptahydrate 98%, As(V), (HAsNa2O4 · 7H2O, Sigma Aldrich).
UV-C radiation survival curves
Ultraviolet (UV) irradiations were performed at UV-C (254 nm) with a fluence of 3 mW/cm2 throughout the assay. Fluence was assessed using a MS-100 optical radiometer with a MS 125 UVC sensor (Ultra-Violet Products, Upland, CA). D. indicus cells grown in either M53 and TGY (at an OD600 = 2) were serially diluted and plated in duplicate for each dose. Plates were air dried for 15 min and placed under the UV lamp to be exposed from 300 to 1800 J/m2 (in increments of 300 J/m2 which corresponds to an exposure of 10 s). Afterwards, plates were incubated for 3 days and the colony forming units (CFU) were assessed. The average CFU mL−1 of non-irradiated sample aliquots represented 100% survival. The surviving fraction at a given dose is the average CFU mL−1 from each irradiated sample (N) divided by the average CFU mL−1 of the non-irradiated sample (N0). Survival curves were obtained by plotting the logarithm of N/N0 versus the dose. To determine the survival curve parameters, the multi-hit model was applied (equation 1), S(D) = Survival fraction (where 1 is 100%); D = Dose (J/m2); K = inactivation constant and n = extrapolation number (obtained by the intercept of the extrapolated semi-log straight-line), (Severin et al., 1983; Kowalski et al., 2000; Kowalski, 2009). For statistical intercomparison of the different D10 values extrapolated from the survival curves of M53 and TGY a Student’s t-test was used and Ρ ≤ 0.05 were considered statistically significant (De La Vega et al., 2005).
Arsenic resistance growth curves
Arsenic resistance was assessed by adding As(V) to broth cultures (OD600 = 0.3) for both M53 and TGY. D. indicus cells were incubated with 10, 25 and 50 mM while D. radiodurans was incubated with 0.05, 0.5, 1, 5, 10, and 20 mM of As(V). Growth curves were obtained by plotting the measured OD600 versus time.
SEM of Deinococcus indicus
Deinococcus indicus cells were grown in M53 or TGY in 24 well plates for 3 days at 30°C. Grown cells were washed with Phosphate Buffer Saline (PBS) and applied over a lamella. Samples were fixed using a mixture of 2.5% glutaraldehyde and 1% formaldehyde in 0.1 M sodium cacodylate buffer for 1 h at room temperature. Afterwards, samples were washed 3 times with 0.1 M of sodium cacodylate buffer and dehydrated by sequential washing with increasing concentration of ethanol solutions (50, 70, 90, and 100%). Ethanol was removed and tert-butyl alcohol was added. Samples were incubated at 4°C for 30 min followed by 1 h in a vacuum desiccator and kept at room temperature until the scanning electron microscopy (SEM) analyses were performed. Samples were incubated for 10 min with 2% solution of osmium tetroxide and gold sputtered with 8 nm gold in an electron sputter (Cressington 108). Imaging was performed using a Hitachi TM3030Plus scanning electron microscope, at 1.5 kV.
STEM imaging and EDX of Deinococcus indicus
Deinococcus indicus cells were grown in M53 broth media (OD600 of 0.3) and washed twice with M53 at 4000 rpm, 4°C. Afterwards, cells were diluted to an appropriated concentration and 4 μL deposited onto a glow-discharged Quantifoil copper grid. The grids were blotted, and plunged into liquid ethane with an automated plunger (EM-GP, Leica Microsystems). STEM imaging was performed under cryogenic conditions with a field-emission Talos F200X (S)TEM microscope (Thermo Fisher Scientific) at 200 kV accelerating voltage (extraction voltage 3,850 V, gun lens 4, spot size 9), with a condenser aperture of 70 μm in microprobe mode with a semi convergence angle of 2.1 mrad. Energy-dispersive X-ray spectroscopy (EDX) was performed using an energy dispersive spectrometer encompassing a Super-X G2 detector that features 4 silicon drift detector (SDDs) for substantially enhanced sensitivity, which is critical for trace element detection, with 8 μs dwell time per pixel, and dispersion of 5 eV per channel
Co-localized Deinococcus indicus-granule size metrics
To identify D. indicus cells and the granules residing within them, grids were imaged using a Talos Arctica 200 kV FEG STEM (Thermo Fisher Scientific) applying the same settings described above with a few variations: spot size 4, camera length 160 mm and 6.66 nm pixel size with 1 μs dwell time per pixel. To quantify the size and distribution of granules within the bacteria, we used tiling and stitching images taken using TFS Tomography software to cover large field of view with multiple bacteria. Stitching was done using Fiji Grid/Collection stitching plugin (Preibisch et al., 2009). Segmentation of the individual bacteria and individual granules residing within them was performed and the number of granules and their average and total size within each bacterium was measured. To segment the bacteria and granules, we used a workflow combining Ilastik (Berg et al., 2019), pixel classifier followed by further processing using dedicated Fiji macro (Schindelin et al., 2012). We used multiple fields of view from different conditions for training two-stage machine-learning classifier in Ilastik “autocontext” approach to classify pixels into four categories: bacteria, granules, background and unclassified. The trained classifier was applied to the stitched images in Fiji (Schindelin et al., 2012). Bacteria were segmented based on connected component analysis of a filled mask of all pixels classified as bacteria, followed by size (1.6 < size [μm2] < 6) and shape filtering (circularity >0.2). Granules were then segmented based on connected component analysis of a mask of all pixels classified as granules within segmented bacteria and further filtered by size (0.012 < size [μm2] < 0.12) and shape (circularity >0.3). Manual correction was applied to correct the segmentation of some missed or falsely detected bacteria. The size and number of all valid bacteria and granules were extracted and analyzed.
Sequence alignment and phylogenetic tree
Multiple sequence alignments were performed using ClustalW (Thompson et al., 1994). Aligned sequences were used as input and the phylogenetic tree was generated using maximum likelihood (ML) in IQ-TREE2 (version 2.2.2.6) (Minh et al., 2020), software with the mod LG + G4 substitution model. The model was selected by running the first tree using the model finder option (Kalyaanamoorthy et al., 2017). The final tree was generated by performing bootstrap analysis of 1,000 data sets and was treated and displayed using the Interactive Tree Of Life (iTOL) (version 6) web service (Letunic and Bork, 2021).
Protein expression and purification
DiArsC2 gene was cloned into a pET28a(+) plasmid that contains an His-tag followed by a TEV cleavage site (GenScript Inc.). The overexpression of DiArsC2 was obtained in Escherichia coli BL21 (Gold) cells transformed with the plasmid pET28a(+)-DiArsC2 and grown in Luria-Bertani (LB) medium at 37°C, 180 rpm. Cells at an OD600 of 0.7 were induced with 500 μM of isopropyl β-D-thiogalactopyranoside (NZYTech) and grown overnight at 20°C. Afterward, the cells were harvested and resuspended in lysis buffer [20 mM HEPES pH 7, 10% glycerol, 300 mM NaCl, 10 mM MgCl2, 1 μg/ml DNase I and 0.1 mg/ml Lysozyme (Sigma-Aldrich)]. Cells were disrupted by 5 cycles of freeze and thaw, and the soluble fraction containing overexpressed DiArsC2 was obtained by centrifugation at 25,931 x g, 20 min at 4°C. The supernatant was loaded onto a His Trap excel column (Cytiva) using as binding buffer 20 mM HEPES pH 7, 250 mM NaCl, 10% (v/v) glycerol, and 10 mM imidazole, and the elution buffer was 20 mM HEPES pH 7, 250 mM NaCl, 10% (v/v) glycerol and 1 M imidazole. DiArsC2 eluted at 300 mM of imidazole and was further loaded onto a desalting column (Hipreptm 26/10 Desalting, Cytiva) using 20 mM HEPES pH 7, 250 mM NaCl, 10% glycerol (v/v). Afterward, the DiArsC2 His-tag was cleaved by adding Tobacco Etch Virus (TEV) protease (Sigma) at 12°C with gentle shaking for 14 h. DiArsC2 was loaded onto His Trap excel (Cytiva) using the same buffers as described above. The final purification step was done by size exclusion chromatography (Superdex 75 10/300 GL, Cytiva), with the buffer 20 mM HEPES pH 7 and 250 mM NaCl. The protein was pure as judged by sodium dodecyl sulphate polyacrylamide gel electrophoresis analysis and concentrated to 21 mg/mL prior to being used for crystallization trials.
Crystallization and X-ray diffraction data collection
Crystallization screens for DiArsC2 were setup at the nL scale in a Crystallization Robot Mosquito LCP (SPT Labtech) with triple sitting drop 96-well plate (TTP Labtech) and using commercial screens Structure 1 and 2 (Molecular Dimensions). Needle- and plate-like crystals appeared in several conditions, three of which were selected for further optimization: #37 (0.2 M Sodium acetate trihydrate, 0.1 M Tris pH 8.5 and 30% (w/v) PEG 4000); #46 (0.05 M Potassium phosphate monobasic and 20% (w/v) PEG 8000); and #47 (15% (w/v) PEG1500). These were optimized by vapor diffusion using 2 μl hanging drops equilibrated against 500 μl reservoir solution in XRL 24-well crystallization plates (Molecular Dimensions), at 20 and 4°C with varying ratios of protein and crystallization solution in each drop. For the final optimized crystallization condition, a ratio of 1:1 (protein to crystallization solution) with 0.2 M Sodium acetate trihydrate, 0.1 M Tris pH 8.5 and 20% (w/v) PEG 4000 for DiArsC2 native (henceforth referred to as DiArsC2) at 4°C. In the case of DiArsC2 bound to arsenic (DiArsC2-As), a ratio of 1:0.8 (protein to crystallization solution) was used using 0.2 M Sodium acetate trihydrate, 0.1 M Tris pH 8.5 and 30% (w/v) PEG 4000, plus 0.2 μl of 0.1 M As(V) at 20°C. DiArsC2 crystals appeared after 7 days while DiArsC2-As crystals appeared after 3 days. Crystals were dipped in a cryoprotecting solution that consisted of the crystallization buffer supplemented with 10% (v/v) PEG 400 and flash-cooled in liquid nitrogen.
Structure determination, refinement, and quality assessment
DiArsC2 crystals were initially screened in-house using Cu Kα radiation with 1.5418 Å wavelength in a Bruker AXS Proteum Pt135 CCD detector system coupled to an Incoatec Microfocus X-ray Source with Montel mirrors. Bruker Proteum software package were used to process and scale the images. The structure was solved by Molecular Replacement using MORDA (Vagin and Lebedev, 2015) and using as phasing model the structure of arsenate reductase from Bacillus subtilis (PDB code: 1JL3) (Bennett et al., 2001). In addition DiArsC2-As crystal was also tested in-house and the structure was solved by PHASER (McCoy et al., 2007) in the PHENIX suite (Adams et al., 2010; Liebschner et al., 2019) using the DiArsC2 as phasing model.
Diffraction data to higher resolution were collected at the XALOC beamline BL13 of the ALBA Synchrotron (Barcelona, Spain) (Juanhuix et al., 2014). The images were processed with autoPROC (Vonrhein et al., 2011), which makes use of XDS (Kabsch, 2010) and the CCP4 suite (Potterton et al., 2003) for integration and conversion of integrated intensities to structure factors. Each dataset was integrated with XDS, followed by POINTLESS (Evans, 2011) for space-group determination, scaling and merging with AIMLESS and application of an anisotropic resolution cut-off at CC1/2 < 0.3 with STARANISO (Tickle et al., 2018). The crystals belonged to the monoclinic space group P21 and contained two molecules per asymmetric unit, as estimated by the Mathews coefficient probability (Matthews, 1968; Kantardjieff and Rupp, 2003). The data processing statistics are given in Supplementary Table S1. Higher resolution crystallographic structures for both DiArsC2 and DiArsC2-As were determined by molecular replacement using PHASER-MR (McCoy et al., 2007) via the CCP4 Graphics User Interface (Potterton et al., 2003) or PHENIX Suite (Adams et al., 2010; Liebschner et al., 2019), and using the DiArsC2-As structure obtained from the in-house data as the phasing model. For DiArsC2, an initial refinement was done with REFMAC (Murshudov et al., 1997) and continued with PHENIX.REFINE (Terwilliger et al., 2007; Adams et al., 2010; Afonine et al., 2012) while for DiArsC2-As refinements were done using PHENIX.REFINE. Throughout the refinement, the model was periodically checked and corrected with COOT against σA-weighted 2|Fo|-|Fc| and |Fo|-|Fc| electron-density maps. Solvent molecules were added manually by inspection of electron-density maps in COOT (Emsley and Cowtan, 2004). TLS (translation-libration-screw) reciprocal space refinement was carried out for both structures. Hydrogen atoms were included in calculated positions with the PHENIX.READYSET tool and isotropic displacement parameters (ADPs) were refined for all non-hydrogen atoms. The final structures were validated using MOLPROBITY (Chen et al., 2010). Refinement statistics are given in Supplementary Table S2. Structure factors and associated structure coordinates of DiArsC2 and DiArsC2-As were deposited in the Protein Data bank (Berman et al., 2003) with accession code 8P6M and 8P5N, respectively (Supplementary Table S2).
A simple ensemble refinement protocol in PHENIX was run for both final structures DiArsC2 and DiArsC2-As considering one domain per independent molecule in the asymmetric unit, of the crystal structure, and using 80% of the non-hydrogen atoms to include in the TLS fitting of the atomic displacement parameters (ADPs) from a previous refinement with isotropic ADPs (Burnley et al., 2012). The secondary structure was determined running PROCHECK within CCP4 Graphics User Interface (Laskowski et al., 1993; Potterton et al., 2003). Figures of the structures were prepared using PyMOL (Delano, 2022).
Results
Deinococcus indicus response to oxidative stress and UV-C
Deinococcus species are known for their extreme tolerance to radiation and oxidative stress (Lim et al., 2019). To evaluate D. indicus tolerance, we subjected the cells grown in M53 and TGY to hydrogen peroxide (H2O2) and methyl viologen (MV). MV is known to catalyze the formation of superoxide anion (O2•-), (Halliwell and Gutteridge, 1999; Halliwell, 2006). D. indicus cells presented higher resistance to H2O2 than to MV in both media (Figure 1). Upon exposure to MV, cells grown in M53 showed higher resistance than the ones grown in TGY. In fact, at 20 mM of MV, cells in M53 were neither inhibited nor had their cell growth compromised. However, the same was not observed when directly applying H2O2, in which case the cells responded in the same way independently of the culture medium (Figure 1B). D. indicus cells were also exposed to UV-C irradiation. It is known that when UV radiation is applied to microbial populations a slight delay is often observed before the onset of an exponential decay (Cerf, 1977; Munakata et al., 1991; Pruitt and Kamau, 1993; Kowalski et al., 2000; Kowalski, 2009). This effect is usually named as a shoulder curve due to its shape and for this reason the multi-hit model was applied to determine curve parameters and extrapolate the D10 (i.e., the dose required to yield 10% survival) for the cells grown in both culture media (Severin et al., 1983; Kowalski et al., 2000; Kowalski, 2009). Interestingly, in M53 cells exhibited significant higher resistance to UV-C in contrast to the ones grown in TGY (Figure 2). This was verified by calculating the D10 values (Ρ ≤ 0.05): in M53 a dose of 809 ± 66 J/m2 (30s exposure) is required to eliminate 90% of cells, while in TGY 619 ± 82 J/m2 (20s exposure) was sufficient to achieve the same result.
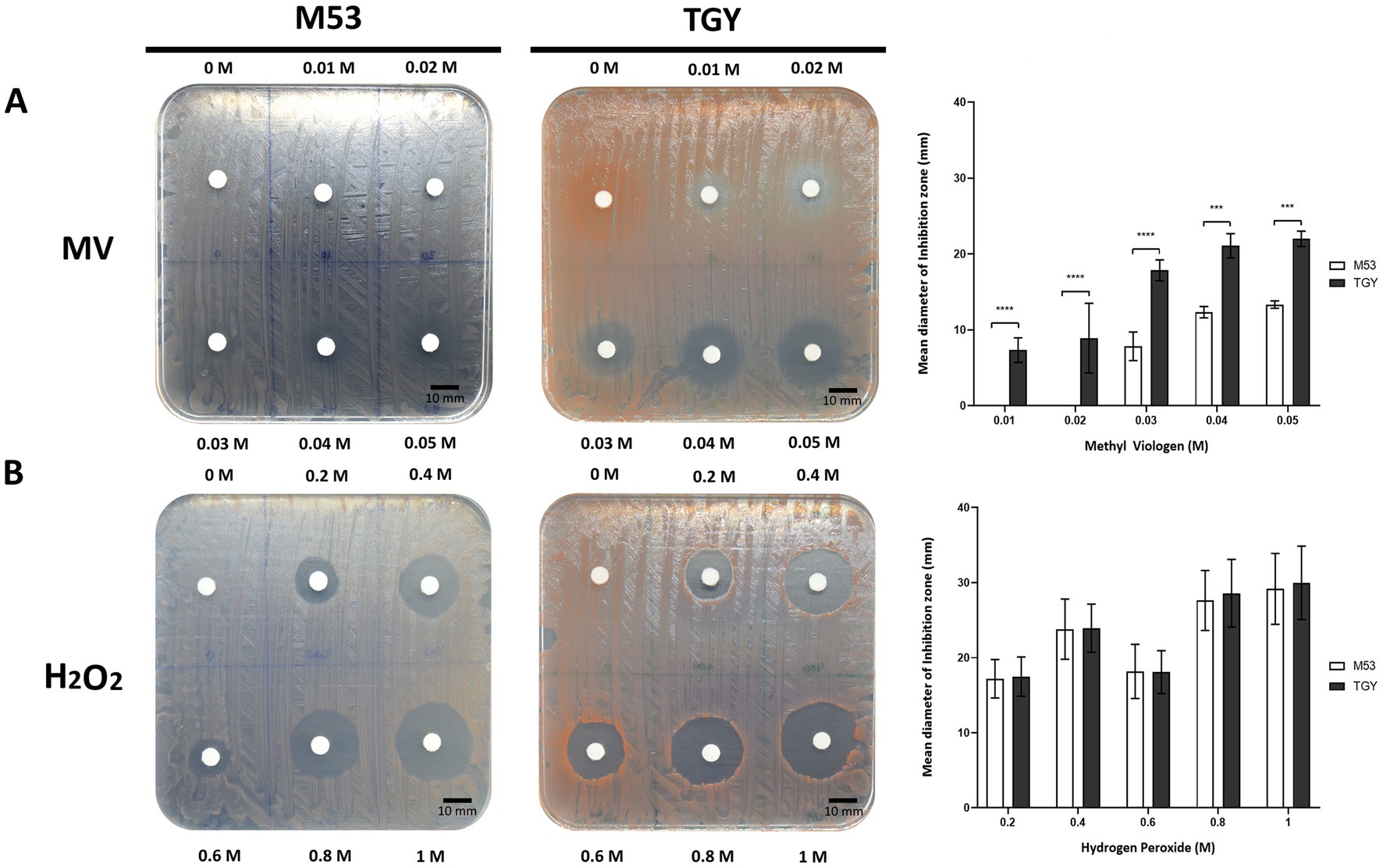
Figure 1. Survival of D. indicus at mid-exponential phase (OD600 = 2.0) to different oxidative stress conditions. (A) Cells exposed to methyl viologen (MV). (B) Cells exposed to hydrogen peroxide (H2O2). (A,B) Representative images of adapted Kirby-Bauer assays to cells grown in M53 and TGY exposed to increasing concentrations (left and middle panels). Inhibition halos correspond to the mean diameter of the inhibition zone (mm), (right panel). The error bars represent the standard deviations from three replicates of four independent experiments (n = 4). p-values were obtained by Two-Way ANOVA. p ≤ 0.001 and p ≤ 0.0001 are represented as: *** and ****, respectively.
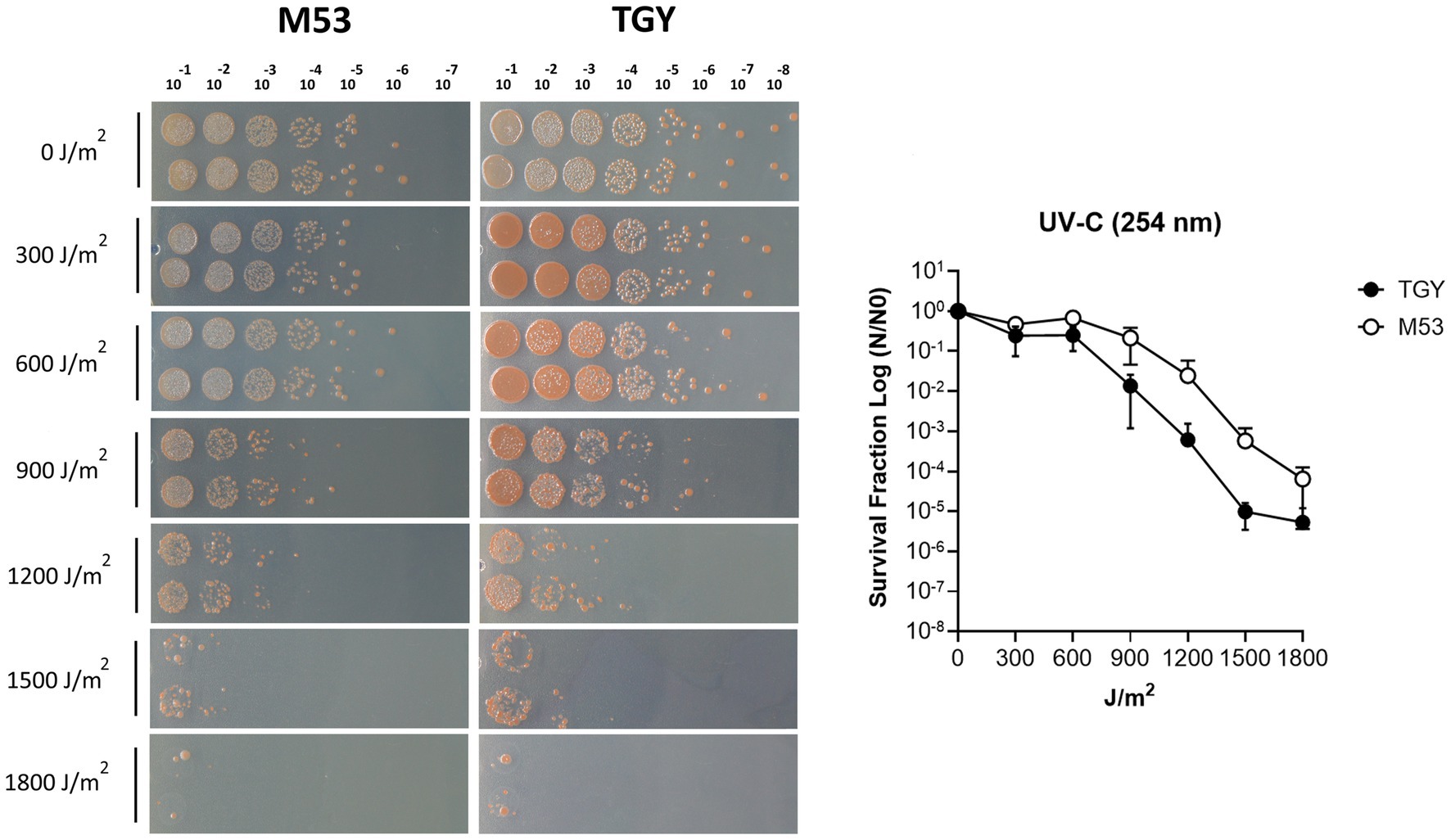
Figure 2. UV-C irradiation survival of D. indicus in M53 and TGY. (A) Representative image of D. indicus CFUs exposed to increasing doses of UV-C (254 nm) radiation in M53 and TGY. (B) Survival curves of D. indicus. The survival fraction was calculated by dividing the CFUs of UV-C irradiated cells (N) by the CFUs of non-irradiated cells (N0). Filled circles correspond to cells grown in TGY while open circles correspond to cells grown in M53. The error bars represent the standard deviation of four independent experiments (n = 4).
Arsenate tolerance assays
Deinococcus indicus Wt/1aT was originally isolated from an aquifer in West Bengal, India, and it was reported to survive 10 mM of As(V) in nutrient broth medium (Suresh et al., 2004). To evaluate its ability to cope with higher concentrations of As(V), D. indicus and D. radiodurans (non-arsenic resistant specie) were exposed to As(V) in their early exponential growth phase (OD600 = 0.3). D. indicus was able to grow in the presence of 25 mM As(V) in both media cultures. However, an extended lag phase was observed and at 50 mM As(V), cells were unable to grow (Figure 3A). Nevertheless, cells harvested and applied to fresh media were able to recover their growth in normal conditions (Supplementary Figure S1). Regarding D. radiodurans, different As(V) concentrations were tested, namely 0.05, 0.5, 1, 5, 10, and 20 mM, our results show that in the presence of 5, 10, or 20 mM, the cells are not able to recover the growth after 24H (Figure 3B). Cells were only able to display normal growth in 50 μM As(V), a 500-fold lower concentration than applied to D. indicus. When exposed to 1 mM, an evident decrease in growth was observed (Figure 3B). This observation was more evident in cells grown in TGY than for those grown in M53.
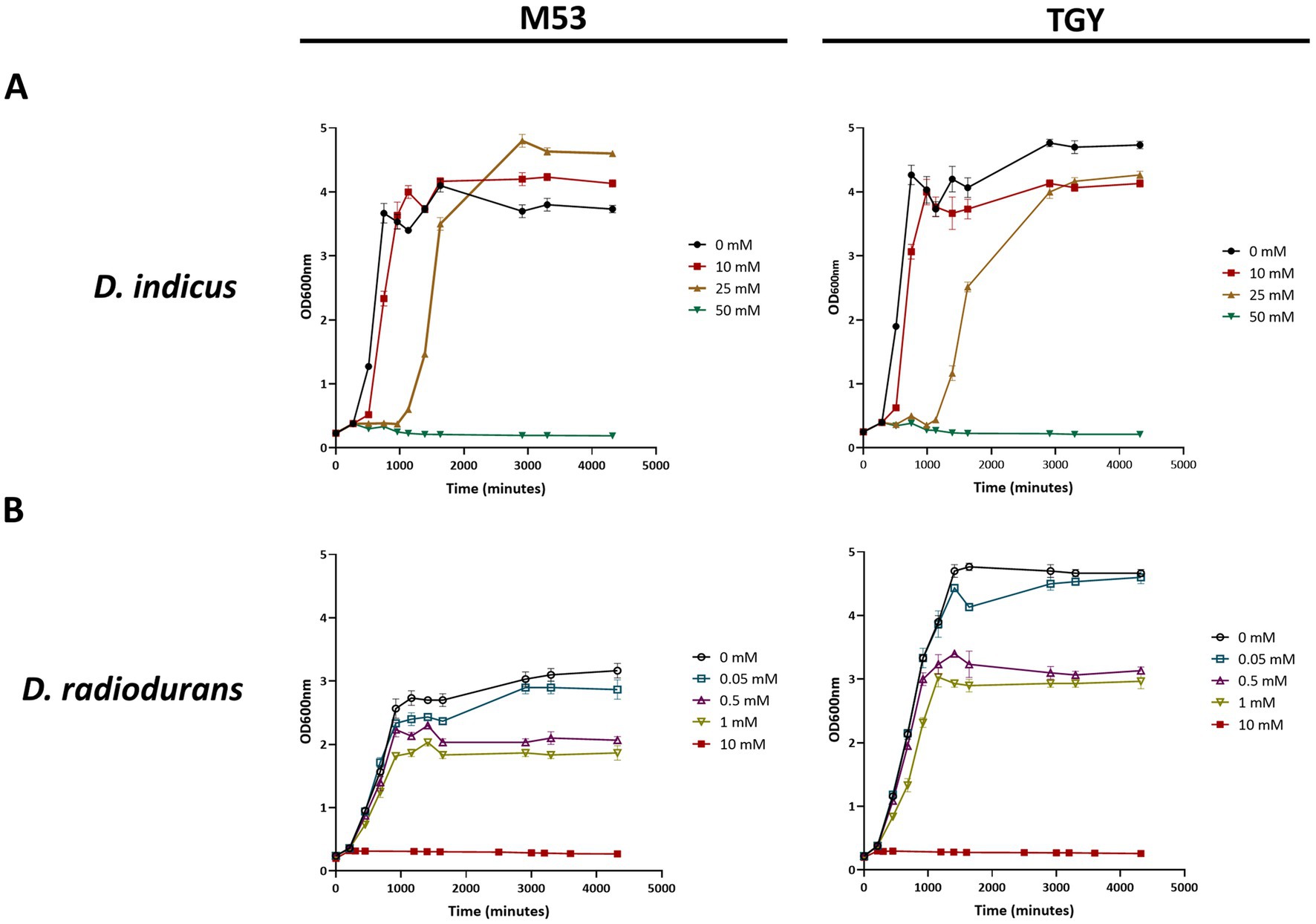
Figure 3. Growth curves in TGY and M53 media cultures of Deinococcus cells exposed to increasing concentrations of As(V) at an early exponential phase (OD600 = 0.3). (A) D. indicus, filled circles correspond to 0 mM of As(V), filled squares correspond to 10 mM of As(V), filled right side up triangles correspond to 25 mM of As(V) and filled upside down triangles correspond to 50 mM of As(V). (B) D. radiodurans, open circles correspond to 0 mM of As(V), open squares correspond to 0.05 mM of As(V), open right side up triangles correspond to 0.5 mM of As(V), open upside down triangles correspond to 1 mM of As(V) and filled squares correspond to 10 mM of As(V).
To further assess their resistance to As(V) in a solid matrix, D. indicus and D. radiodurans were exposed to increasing concentrations of arsenate ranging from 200 mM to 1 M (Figure 4). Interestingly, in the solid matrix D. indicus was not fully inhibited by 1 M of As(V) in either culture medium. Nevertheless, partial inhibition halos (reduction of cellular density) were observed in cells grown in M53 upon submission to 600 mM to 1 M of As(V). In contrast, D. radiodurans is inhibited by 200 mM of arsenate (Figure 4).
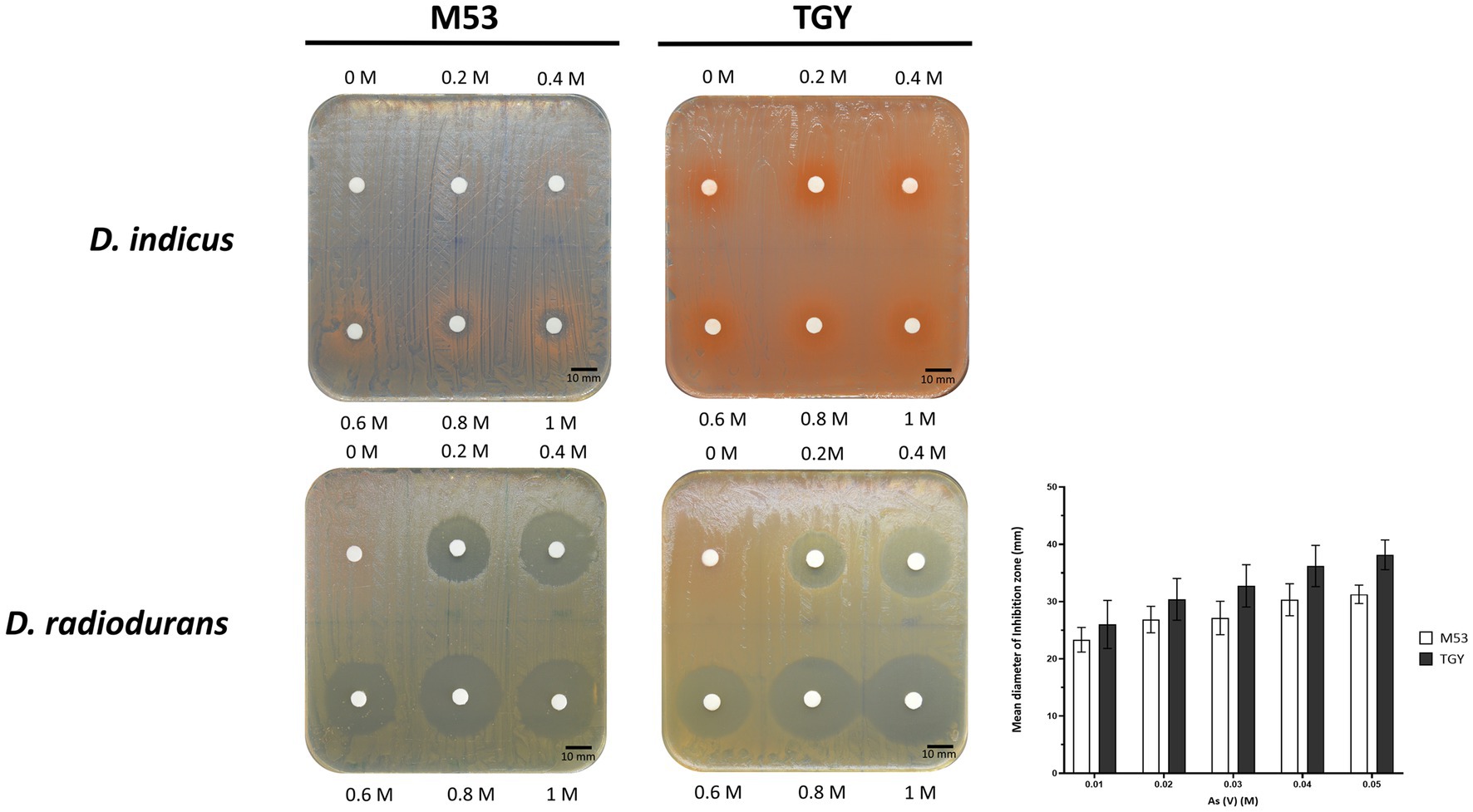
Figure 4. Survival of D. indicus and D. radiodurans in solid matrix (M53 and TGY culture media) in response to As(V). Cells at mid-exponential phase (OD600 = 2.0) were exposed to increasing concentrations of As(V) using an adapted Kirby-Bauer assay (Bauer et al., 1966; Hudzicki, 2012). Inhibition halos correspond to the mean diameter of inhibition zone (mm). The error bars represent the standard deviations from three replicates of four independent experiments (n = 4).
SEM of Deinococcus indicus
Previously, it was demonstrated that D. indicus presents growth media-induced morphotypes displaying a reversible behavior from single cells to multi-cell chain morphology (Chauhan et al., 2019b). To explore this capacity and understand if differences in morphotypes account for the behavior disparities observed against cell insults, D. indicus grown in M53 and TGY media were observed through Scanning Electron Microscopy (SEM). SEM data revealed small differences on the size of the cells, where D. indicus grown in TGY presented slightly lower cell lengths. However, no differences in cell chain clustering were observed (Figure 5). Nevertheless, the addition of 500 μM MnCl2 seems to affect cells grown in TGY, where cell septation is compromised and cell length is vastly increased (Supplementary Figure S2).
Cryo-STEM EDX of Deinococcus indicus
Polyphosphate-like granules (pPLGs) are known to be involved in the sequestration of cation ions as well as in heavy-metal detoxification (Peverly et al., 1978; Urech et al., 1978; Scott and Palmer, 1990; Keasling, 1997; Kulakovskaya, 2018). D. radiodurans, Deinococcus murrayi and Deinococcus proteolyticus present pPLGs in their native compositions, but no information is available for D. indicus (Thornley et al., 1965; Sleytr et al., 1976; Ferreira et al., 1997; Eltsov and Dubochet, 2005; Copeland et al., 2012). Wolf et al. (2015) was carried out to image D. indicus in native conditions at the early-exponential phase in M53 medium. Through the ImageJ macro previously trained using ilastik “autocontext” approach, we were able to rapidly quantify and segment D. indicus cells and their granules using Cryo-STEM stitched images (Figure 6A). We observed a wide heterogeneity of electron dense granules distribution ranging from 0 to 8 granules per cell (Figure 6B). Overall, from the 435 cells analyzed 31.8% of the cells had no granules, followed by 17.2% of the cells containing 1 or 2 granules. Only 1.4 and 0.5% of the cells contained 7 and 8 granules, respectively (Figure 6B). The average granule area (n = 803) was 0.040 ± 0.019 μm2 with the smallest granules measuring 0.012 μm2 while the biggest granule measuring 0.107 μm2 (Figure 6C). EDX spectral analysis detected the enrichment in phosphorus of the intracellular granules, confirming that these structures are indeed polyphosphate-like granules (pPLGs) (Figure 7). The main counter ion found was magnesium (Supplementary Table S3).
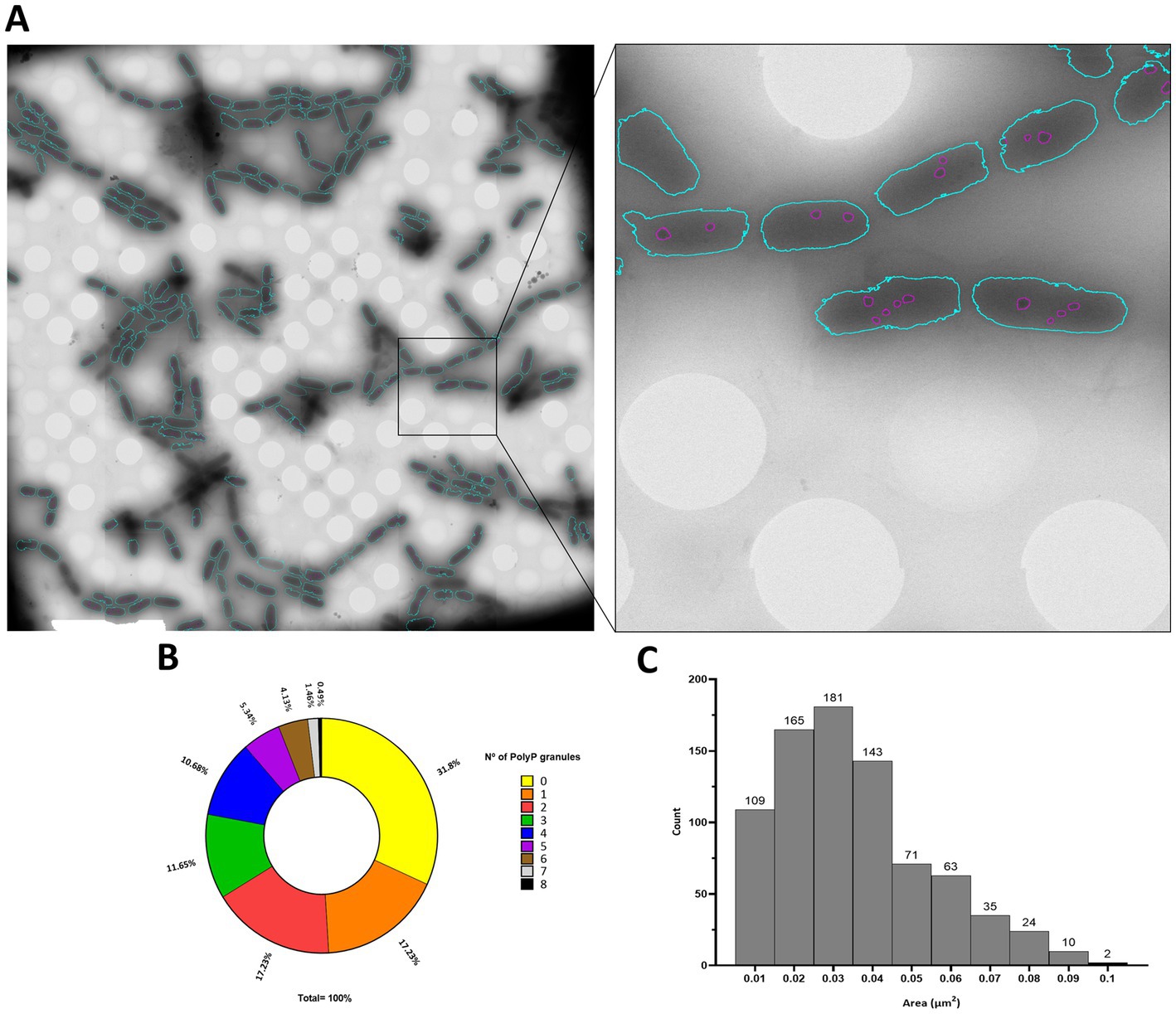
Figure 6. PolyP granules analysis by Cryo-STEM. (A) D. indicus STEM stitched image segmented trough the trained classifier, detected bacteria are colored in blue and granules within the bacteria are colored in purple. (B) Percentage of cells containing PolyP granules. (C) Area (μm2) of D. indicus PolyP granules.
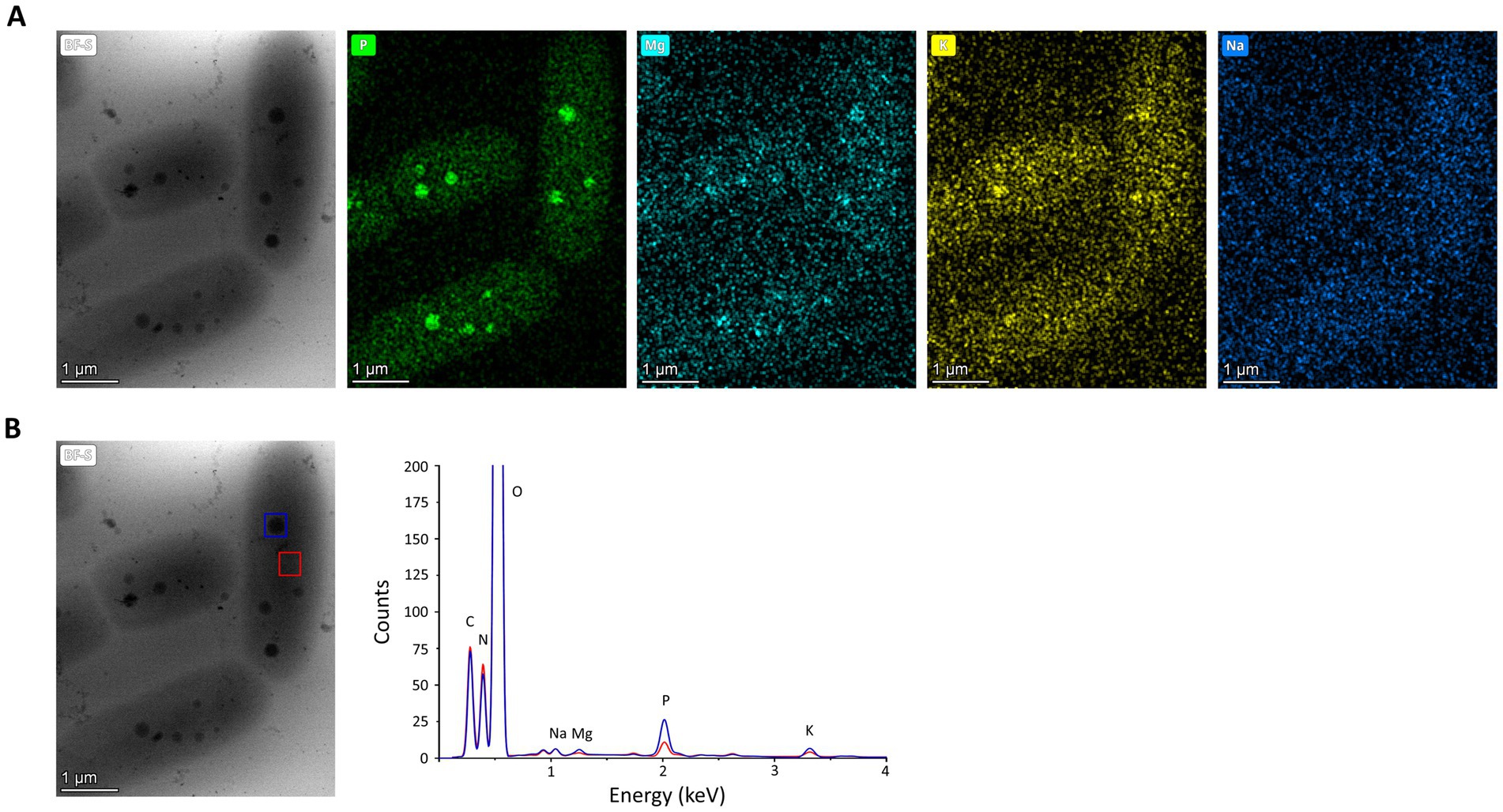
Figure 7. EDX analysis of D. indicus cells grown in M53. (A) Cryo-STEM images of control condition for which EDX spectra were measured, and elemental mapping for phosphorus (green), magnesium (cyan), potassium (yellow), and sodium (dark blue). (B) Blue square highlights a polyphosphate granule and red square a cytosol region, used to obtain the superimposed EDX spectra the polyphosphate granule (blue line) and the cytosol (red line).
DiArsC2 (arsenate reductase) phylogenetic analysis
In order to explore the difference between the two species, D. radiodurans and D. indicus, to the arsenate resistance we analyzed the arsenate reductases that belong to the ars operon, which are able to convert As(V) into As(III) (Mukhopadhyay and Rosen, 2002). It is predicted that D. indicus possesses three genes that encode cytosolic arsenate reductases, DiArsC1, DiArsC2, and DiArsC3 while D. radiodurans possesses two arsenate reductases, DrArsC1 and DrArsC2 (Figure 8). There are three families of cytosolic arsenate reductases: one belongs to the E. coli R773 plasmid and comprises glutaredoxin (Grx)-coupled enzymes, while another class belongs to the Staphylococcus aureus Plasmid pI258 comprising the thioredoxin (Trx)-coupled enzymes (Ji et al., 1994; Oden et al., 1994; Shi et al., 1999; Messens et al., 2002a; Messens and Silver, 2006) and the final class belongs to eukaryotic organisms, encompassing the ACR2p from Saccharomyces cerevisiae (Mukhopadhyay and Rosen, 1998; Mukhopadhyay et al., 2000). A sequence analysis of the different protein sequence, shows that the three arsenate reductases from D. indicus, DiArsC1, DiArsC2 and DiArsC3 belong to three branches (Figure 8). Interestingly, DiArsC1 and DrArsC1 branch closely to the E. coli ArsC from the Grx-linked prokaryotic family (Figure 8; Gladysheva et al., 1994; Rosen, 1999). DiArsC3 and DrArsC2 branch closely to Thermus thermophilus ArsC that belongs to the Trx-linked prokaryotic ArsC reductases family. DiArsC2 branch closely to Desulfovibrio alaskensis G20 ArsC3 (Nunes et al., 2014), which are in the main branch with S. aureus pI258 ArsC (Ji et al., 1994; Rosen, 1999; Figure 8). Since DiArsC2 did not cluster together with any of D. radiodurans ArsCs, we decided to further unveil the structural mechanism for arsenate detoxification.
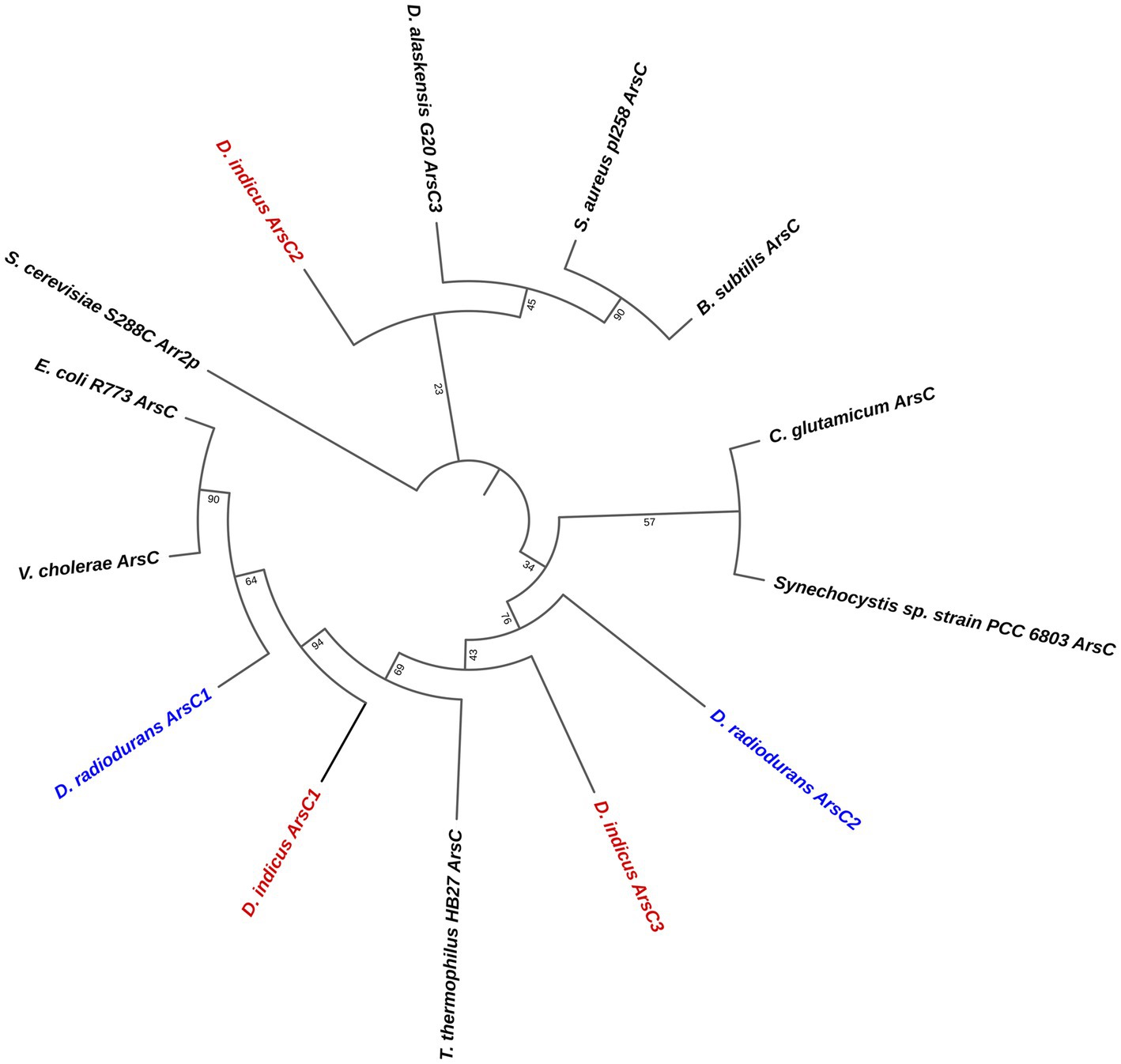
Figure 8. Phylogenetic tree of the three families of cytosolic arsenate reductases. Accession numbers for the amino acid sequences are: B. subtilis (P45947), Corynebacterium glutamicum (Q8NQC6), D. indicus ArsC1 (GHG23001.1), D. indicus ArsC2 (WP_088246862.1), D. indicus ArsC3 (WP_191300003.1), D. radiodurans ArsC1 (UDK99340.1), D. radiodurans ArsC2 (ANC72982.1), Desulfovibrio alaskensis G20 (WP_011368603.1), E. coli R773 (P08692.1), Saccharomyces cerevisiae S288C Arr2P (DAA11614.1) S. aureus pl258 (P0A006), Synechocystis sp. PCC 6803 (P74313),Thermus thermophilus HB27 (AAS81844.1) and Vibrio cholerae (Q9KQ39). The three D. indicus ArsC and two D. radiodurans ArsC are shown in red and blue, respectively.
DiArsC2 and DiArsC2-As structural insights
The crystal structures of DiArsC2 and DiArsC2-As were determined and refined at resolutions of 1.65 and 1.50 Å, respectively. The space group of both crystal structures is P21, presenting two molecules in the asymmetric unit. DiArsC2 and DiArsC2-As structures were refined to final Rfactor/Rfree values of 0.224/0.254 and 0.152/0.172, respectively (Supplementary Table S2). The superposition of both chains based on the secondary structure matching algorithm yields a root-mean-square deviation (r.m.s.d.) of 0.6 Å between the superimposed Cα carbon atoms and 127 aligned amino acid residues. The largest deviations are in a loop region between residues 88–100 that presents high flexibility and for DiArsC2 it lacks electron density for this region (Figures 9A,B). In fact, in DiArsC2 it was not possible to build the protein chain between the residues 89–96 for Chain A and 89–93 for Chain B (Figure 9B). Thus, the structural analysis for both structures were performed with Chain B. Ensemble refinements (Burnley et al., 2012) were performed for DiArsC2 and DiArsC2-As (leading to Rfactor/Rfree values of 0.221/0.255 and 0.160/0.177 respectively) where the most flexible regions were residues 31–36 and the loop 86–97 that contains the two conserved cysteines (Cys87 and Cys94) involved in As(V) reduction (Figures 9C, 10). The loop that contains the conserved catalytic Cys15 does not show a high degree of structural flexibility between the two structures here presented.
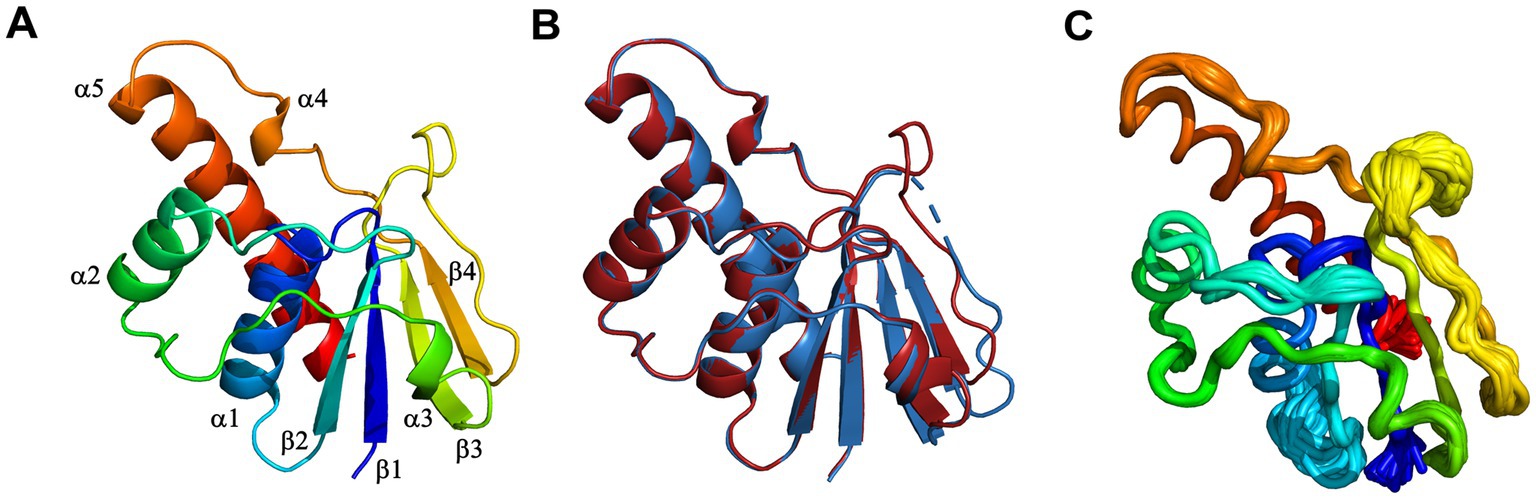
Figure 9. X-ray crystal structure of D. indicus arsenate reductase ArsC2. (A) Cartoon representation of the DiArsC2-As, rainbow colored from blue (N-terminal) to red (C-terminal). (B) Superposition of the DiArsC2-As (red) with the DiArsC2 (blue) monomers. (C) Cα tube representation of the DiArsC2-As monomer from the Ensemble refinement calculations.
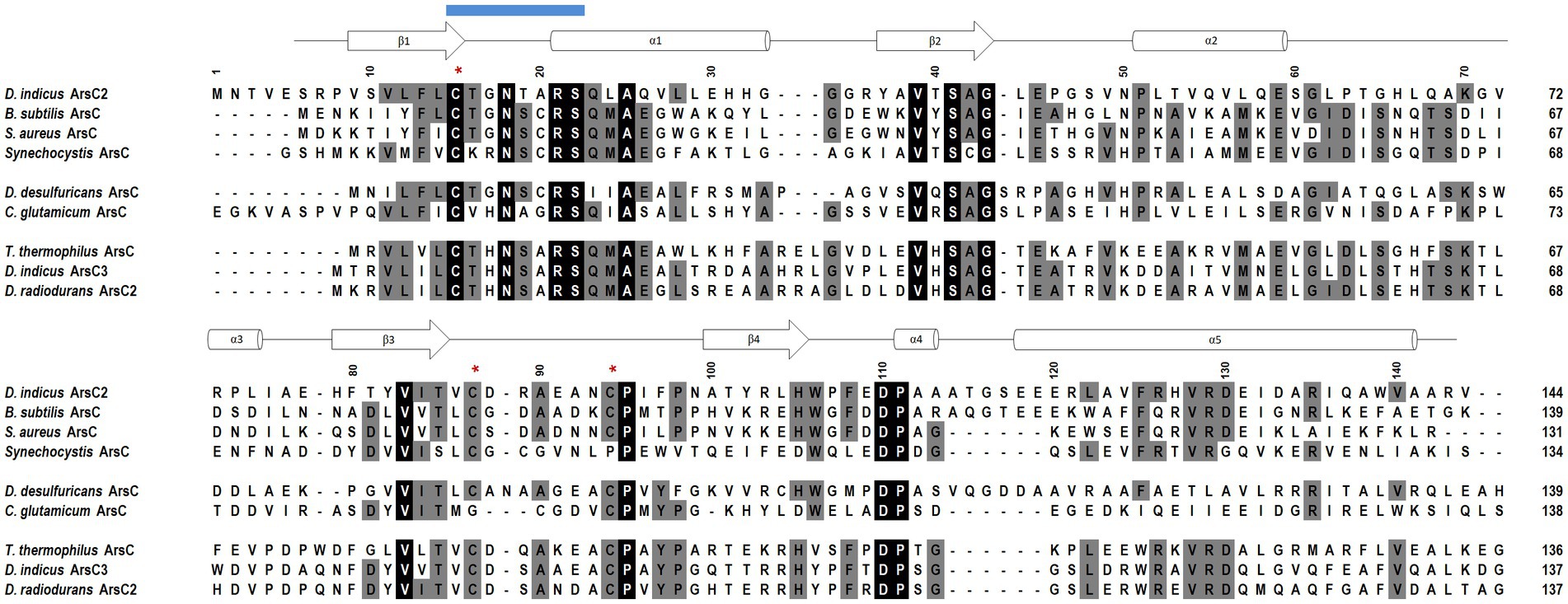
Figure 10. Amino acid sequence alignment of the D. indicus arsenate reductase ArsC2 with the Trx-linked family ArsC. The percentage identity of the ArsC of Trx-linked family with D. indicus ArsC2 (WP_088246862.1) is showed below. B. subtilis (34%, P45947); S. aureus pl258 (29%, P0A006); Synechocystis sp. PCC 6803 (27%, P74313); D. desulfuricans IC1 (26%, QCC86315.1); C. glutamicum (19%, Q8NQC6); T. thermophilus HB27 (28%, AAS81844.1); D. indicus ArsC3 (30%, WP_191300003.1); D. radiodurans ArsC2 (31%, ANC72982.1). DiArsC2 secondary structure is shown above the alignment, and is indicated as α-helices and β-chains based on the output from PROCHECK (Laskowski et al., 1993). The three cysteine residues are marked with a red *. Amino acid residues that are part of the phosphate-binding loop are marked with a blue line. Strictly conserved amino acids represented as black boxes, whereas gray boxes represent the mostly conserved residues among the selected sequences.
DiArsC2 structures are composed of an α/β domain and four parallel β-strands which are flanked by three helices and two small helices (Figures 10, 11). These crystal structures are similar to the arsenate reductases from B. subtilis (Bennett et al., 2001), (PDB 1JL3) and S. aureus, (PDB 1LJL) (Messens et al., 2002b). The crystal structures of DiArsC2 and DiArsC2-As presented an r.m.s.d. value of 1.3 Å with 127 aligned amino acid residues with the arsenate reductases from B. subtilis (Bennett et al., 2001) (PDB 1JL3). The arsenate reductase from S. aureus, (PDB 1LJL) presents an r.m.s.d. value of 1.0 Å and 119 aligned amino acid residues with DiArsC2 and an r.m.s.d. value of 1.2 Å and 121 aligned amino acid residues with DiArsC2-As.
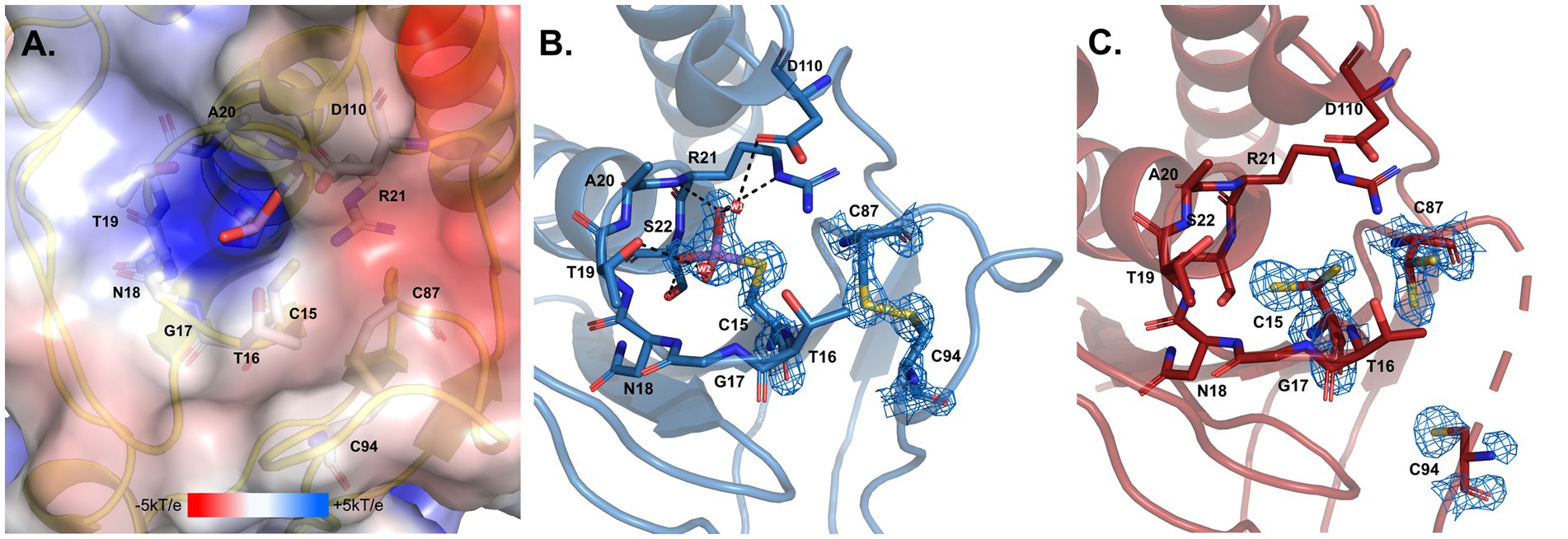
Figure 11. Arsenate catalytic site of D. indicus ArsC2. (A) Electrostatic potential mapped on the molecular surface of the pocket where arsenate enters, calculated by APBS (Baker et al., 2001; Dolinsky et al., 2004) integrated in the PYMOL (Delano, 2022). (B) DiArsC2–As and (C) DiArsC2 catalytic centers. In all the panels structures are shown in cartoon representation, with selected amino acid residues and arsenate drawn as sticks. In (B,C), the final σA-weighted 2|Fo|–|Fc| electron density map is represented at the 2 σ and 1 σ contour levels, respectively around the cysteine residues and the arsenate ligand. In (A–C) carbons are colored in gray, blue and red, respectively; nitrogen in dark blue, oxygen in red, sulfur in yellow, and arsenic in purple. Two water molecules W1 and W2 are represented as small red spheres. Black dashes represent the hydrogen bond interaction with neighboring atoms to the arsenate and the water molecules.
The reduction mechanism of arsenate involves three cysteines, Cys15, Cys87, and Cys94. In the case of the DiArsC2-As structure, a disulfide bridge between Cys87 and Cys94 is formed. Although the protein was co-crystallized in the presence of arsenate (AsO43−), the density observed close to the catalytic Cys15 suggests the presence of AsHO32−. This molecule is located in a positively charged pocket and establishes a network of hydrogen bonds with the main-chain nitrogen atoms of residues G17NTARS22, Thr16 and Ser22 hydroxyl and Arg21Nε groups, as well as water molecules (Figures 11A,B). Cysteine 15 is part of a well-known phosphate-binding loop (motif, CX5R) of LMW PTPases which is conserved in the Trx-linked ArsC family (Figure 10; Bennett et al., 2001). In the native structure, there is no species present in this pocket, and all the residues in the phosphate binding loop G17NTARS22, are in a similar position as in the Arsenate-bound structure. Although no electron density was observed for the region 89–93 (Chain B), weak density for Cys94 was obtained (Figure 11C). Cys15 and Cys87 are involved in the reduction of As(V) to As(III), and in DiArsC2 structure both residues were refined with a double side-chain conformation, suggesting the presence of a mixed state (Figure 11C).
Discussion
Deinococcus genus is ubiquitously present in nature and is able to tolerate high levels of radiation insults (Jin et al., 2019). D. indicus is a Gram-negative and arsenic resistant bacterium that presents a rod-shaped morphology in contrast to most Deinococcus sp. including D. radiodurans that present a coccus morphology (Suresh et al., 2004). It is known that arsenic induces oxidative stress in both eukaryotic and prokaryotic organisms (Huang et al., 2010; Ciprandi et al., 2012; Belfiore et al., 2013; Andres and Bertin, 2016; Shah and Damare, 2018; Castro-Severyn et al., 2019; Hu et al., 2020; De Francisco et al., 2021). Here, we report an extensive characterization of D. indicus resistance to oxidative stress, UV-C and arsenate. Oxidative stress analysis revealed that different culture conditions promote dissimilar responses to oxidative stress agents, and D. indicus presents a higher resistance to MV when grown in M53 than in TGY, while the same resistance pattern is observed in both media when exposing the cells to H2O2. Interestingly, D. indicus cells are more resistant to H2O2 than to MV. In bacteria, an increase in the expression of superoxide dismutase (SOD) and catalases has been observed upon exposure to arsenic (Weiss et al., 2009; Ciprandi et al., 2012; Belfiore et al., 2013; Andres and Bertin, 2016; Shah and Damare, 2018). It is predicted that D. indicus has two superoxide dismutases (SOD), a MnSOD (accession number: GHG29236.1) a Cu/Zn SOD (accession number GHG15276.1) and one catalase (accession number: GHG14730.1). Moreover, both the superoxide anion and H2O2 can damage proteins containing iron sulfur clusters with the release of Fe2+ that can react with hydrogen peroxide, leading to the formation of hydroxyl radicals by the Fenton reaction (Koppenol, 1993; Valentine et al., 1998; Kehrer, 2000; Kohen and Nyska, 2002). D. indicus also possesses one DNA-binding protein under starved conditions, Dps (accession number: GHG25447.1). D. radiodurans Dps proteins are known to sequester iron and manganese (Ishikawa et al., 2003; Santos et al., 2015). D. indicus Dps presents high identity (75.5%) with DrDps1 which is known to utilize hydrogen peroxide for the oxidation of Fe2+ to Fe3+(Santos et al., 2015). In addition, it has been previously reported that D. radiodurans protein extracts present a higher ROS scavenging ability than in E. coli, namely 30 fold-higher for H2O2 and 6 fold higher for O2.- (Tian et al., 2004).
So far, there is no information regarding UV-C resistance of D. indicus. In fact, D. indicus was screened for UV-B when first isolated by Suresh and co-workers, presenting 2–4% survival at 50000 J/m2 in nutrient broth media (Suresh et al., 2004). Here, we showed that UV-C resistance in D. indicus is media-dependent: cells grown in M53 require a dose of 809 ± 66 J/m2 to eliminate 90% of cells, while cells grown in TGY required a lower dose of 619 ± 82 J/m2.
Moreover, D. indicus cells grown in M53 were also more resistant than the ones in TGY when subjected to MV. Interestingly, it has been previously reported that D. radiodurans response to UV-C exposure is also media-dependent (Chen et al., 2023). We can postulate that this media dependent response to MV and UV-C could indicate similar resistance mechanisms are in place and they act in a media dependent way, however the molecular mechanisms involved need to be further investigated.
Previously, it was demonstrated that D. indicus Wt/1aT can survive to 10 mM of arsenate As(V) (Suresh et al., 2004). To further extend arsenic resistance characterization, herein we showed that D. indicus is able to reach control conditions in the presence of 25 mM of As(V). Interestingly, in M53 medium and in the presence of 10 mM and 25 mM of arsenate, cells presented higher OD600 compared to control conditions, while the same behavior was not observed in TGY. This could be due to higher resistance to oxidative stress conditions, or perhaps other transcripts are modulated when cells are grown in M53. Moreover, an extended lag phase in the presence of 25 mM of arsenate before resuming normal growth was observed in both media although it was more pronounce when the cells were grown in TGY. This is a fairly common effect, for instance, methyl-mercury acetate causes an extended lag-phase on Rhodopseudomonas capsulata before resumption of normal growth (Nielsen and Sojka, 1979; Brochiero et al., 1984; Jaiganesh et al., 2012; Aljerf and AlMasri, 2018). In E. coli, cadmium quantum dots caused a longer lag-phase until normal proliferation was restored (Jaiganesh et al., 2012). Moreover, D. indicus growth was suppressed by the presence of 50 mM of As(V). However, upon As(V) removal, D. indicus was able to restore normal growth conditions. In this case, an extended lag-phase similar to post-antibiotic effect (PAE) noted on antibiotic applications was also observed (Zhanel et al., 1991; Li et al., 2016; Srimani et al., 2017). PAE is characterized as the time period after total removal of antibiotic during which no growth is observed. Our data for arsenic resistance is consistent with that for D. indicus strain DR1 isolated from the wetlands of Dadri, Uttar Pradesh, India (Chauhan et al., 2017, 2019a). Regarding D. radiodurans, no lag phase was observed upon exposure to 0.5 and 1 mM of As(V), even though cell growth was compromised. No differences were observed between media cultures in D. radiodurans and the same pattern was verified by exposing the cells to As(V) in a solid matrix. In contrast, D. indicus is able to survive up to 1 M of As(V) in solid matrix.
Since D. indicus presents higher resistance to MV, UV-C and a faster response and growth in M53 when submitted to As(V), a STEM-EDX analysis was performed. Here we reported for the first time the presence of polyphosphate-like granules (pPLGs) in D. indicus. Moreover, more than 30% of the cells contained no granules while a few cells contained up to 8 granules. This high heterogeneity reveals high plasticity in PolyP granules of D. indicus cells, and could indicate that cells adopt specific roles in the community, specializing in PolyP storage to gain an advantage upon environmental changes. pPLGs are also known to be involved in heavy-metal detoxification (Pan-Hou et al., 2001, 2002; Alvarez and Jerez, 2004; Ruiz et al., 2011; Orell et al., 2012; Jasso-Chávez et al., 2019; Sanz-Luque et al., 2020). In Anabaena cylindrica a higher accumulation of aluminum was observed on cells exhibiting pPLGs that were grown in a phosphate-rich medium (Annette et al., 1985). It is known that polyphosphate kinases (PPKs) are involved in the formation of inorganic polyphosphate (polyP) and pPLGs (Kornberg, 1999). In E. coli, higher resistance to Hg2+ was observed after the introduction of ppk into the merA-deleted mer operon (Pan-Hou et al., 2001, 2002). Moreover, it was proposed that polyP was involved in conversion of Hg2+ to a less toxic molecule via chelation mechanisms (Pan-Hou et al., 2001, 2002). Nevertheless, further analysis of D. indicus ability to utilize polyP for arsenic detoxification is required, especially since D. radiodurans also presents pPLGs and is sensitive to arsenic. It would be vital to understand if D. indicus pPLGs aid on the detoxification of arsenic either being directly involved or indirectly.
In order to complement our studies and to further understand the resistance to arsenate by D. indicus the arsenate reductase DiArsC2 was chosen since it did not cluster with any of ArsC from D. radiodurans. Thus, we undertook the structural studies of DiArsC2 and we were able to crystallize two different forms of the enzyme: native (DiArsC2) and bound to arsenite (DiArsC2-As). The crystal structures present high similarities with the B. subtillis ArsC that belongs to the Trx-linked family (Bennett et al., 2001). Based on the conservation of the 3 cysteines (Cys15, Cys87, and Cys94) (Figure 10) and structural analysis (Figures 9, 11), we infer the As(V) mechanism of reduction is identical to the one proposed for B. subtillis, i.e., a triple redox system (Bennett et al., 2001). The DiArsc2-As structure represents the final stage, where Cys15 is bound to As(III), however the ligand cannot be released since Cys87 forms a disulfide bridge with Cys94 (Figure 11). Moreover, the arsenate is located in a positive-charged pocket which is accessible from the external environment. Since in our DiArsC2 structure the disulfide bridge between these two residues is not formed (at least in chain B, where density is observed) this could indicate that an As(V) reduction event already took place in the DiArsC2-As structure, leading to the formation of the Cys87 – Cys94 disulfide bridge (Figure 11). The lack of mobility of the PTPase loop (where Cys15 is located) together with the high flexibility of the loop that contains Cys87 and Cys94 suggests that Cys87 is the residue responsible for the release of As(III) bound to the Cys15 (Figure 9). In fact this loop is readily accessible by the thioredoxin reductase system to reduce the Cys87 – Cys94 disulfide bridge and allow a new As(V) reduction cycle to occur (Messens et al., 1999; Bennett et al., 2001). It would be interesting to assess whether the presence of Cys87 in the DiArsC2 structure is essential for the ligation of As(V). Moreover, the mutation of Cys87 could allow DiArsC2 to be used has a tool for of As(V) removal by inhibiting the release of As(III).
Conclusion
Arsenic contamination severely damages ecosystems and compromises the integrity of water resources essential for human life. D. indicus is an arsenic resistant organism belonging to Deinococcaceae family that present high resistant to UV radiation. Here we performed a characterization of stress resistance of D. indicus and provide insights toward exploiting D. indicus as a tool for bioremediation. D. indicus exhibited a media-dependent response upon exposure to UV-C and MV, and demonstrated higher resistance in M53 compared to TGY. Moreover, cells grown in M53 upon exposure to 25 mM As(V) exhibited higher growth (OD600). This may indicate that optimization of culture conditions could lead to a higher resistance to cellular insults including arsenic stress. Using STEM-EDX we were able to detect the presence of polyphosphate granules in D. indicus. Nevertheless, more studies are required to infer the potential utilization as a path for the detoxification process via arsenic bioaccumulation. Additionally, the D. indicus ars operon contains two arsenate reductases (DiArsC2 and DiArsC3). The DiArsC2 structure revealed detailed insights into the molecular mechanism of the arsenate reduction, in which As(V) is converted to As(III) and remains bound to Cys15. These findings, contribute to the knowledge to apply D. indicus in bioremediation of arsenic either by following a cell-based approach or at the protein level using DiArsC has a tool to remove As(V).
Data availability statement
The original contributions presented in the study are included in the article/Supplementary material, further inquiries can be directed to the corresponding author.
Author contributions
AG and CR: conceptualization and experimental design. AG, BS, WA, SW, ME, PM, and CR: methodology. CR, AG, SW, ME, and PK: STEM experiments. CR, AG, SW, OG, and DR: software, granules analysis, and data curation. WA and AG: SEM experiments. AG, BS, CR, and PM: protein purification and structure determination. AG and CR: UV-C, oxidative, and As(V) experiments. AG and CR analyzed the data and wrote the manuscript. CR: investigation, supervision, and project administration. AG, BS, DR, WA, PK, OG, SW, ME, PM, and CR: review and editing the manuscript. CR, SW, and ME: funding acquisition. All authors contributed to the article and approved the submitted version.
Funding
This study was financially supported by the Portuguese Fundação para a Ciência e Tecnologia (FCT), grants PTDC/BIA-BQM/31317/2017, Project MOSTMICRO-ITQB with references UIDB/04612/2020 and UIDP/04612/2020, and LS4FUTURE Associated Laboratory (LA/P/0087/2020). This project has received funding from the European Union’s Horizon 2020 research and innovation program under grant agreement No. 857203. AG and BS are recipients of FCT grants SFRH/BD/06723/2020 and SFRH/BD/08066/2020, respectively. CR is recipient of FCT Institutional CEEC. Cryo-electron microscopy studies received partial support from the Weizmann Institute of Science (The Irving and Cherna Moskowitz Center for Nano and BioNano Imaging), and from the European Union (ERC-AdV grant, CryoSTEM, 101055413 to ME). This work benefited from access to the Weizmann Institute Electron Microscopy Unit, an Instruct-ERIC centre through the Access proposal PID: 19879.
Acknowledgments
We would like to acknowledge the ALBA Synchrotron Light Facility with the collaboration of ALBA staff for the data collection of DiArsC2 structures performed at BL13-XALOC beamline. The image analysis was made available thanks to the de Picciotto Cancer Cell Observatory In Memory of Wolfgang and Ruth Lesser of the MICC Life Sciences Core Facilities Weizmann Institute of Science Israel. Teresa Silva and Cristina Timóteo from the Microbial Cell Production and Protein Purification and Characterization Research facilities at ITQB-NOVA are acknowledged for providing the competent cells of Escherichia coli strains and to purify TEV protease.
Conflict of interest
The authors declare that the research was conducted in the absence of any commercial or financial relationships that could be construed as a potential conflict of interest.
Publisher’s note
All claims expressed in this article are solely those of the authors and do not necessarily represent those of their affiliated organizations, or those of the publisher, the editors and the reviewers. Any product that may be evaluated in this article, or claim that may be made by its manufacturer, is not guaranteed or endorsed by the publisher.
Supplementary material
The Supplementary material for this article can be found online at: https://www.frontiersin.org/articles/10.3389/fmicb.2023.1240798/full#supplementary-material
References
Adams, P. D., Afonine, P. V., Bunkóczi, G., Chen, V. B., Davis, I. W., Echols, N., et al. (2010). PHENIX: A comprehensive Python-based system for macromolecular structure solution. Acta Crystallogr. Sect. D Biol. Crystallogr. 66, 213–221. doi: 10.1107/S0907444909052925
Afonine, P. V., Grosse-Kunstleve, R. W., Echols, N., Headd, J. J., Moriarty, N. W., Mustyakimov, M., et al. (2012). Towards automated crystallographic structure refinement with phenix.Refine. Acta Crystallogr. Sect. D Biol. Crystallogr. 68, 352–367. doi: 10.1107/S0907444912001308
Aljerf, L., and AlMasri, N. (2018). A gateway to metal resistance: bacterial response to heavy metal toxicity in the biological environment. Ann. Adv. Chem. 2, 032–044. doi: 10.29328/journal.aac.1001012
Alvarez, S., and Jerez, C. A. (2004). Copper ions stimulate polyphosphate degradation and phosphate efflux in Acidithiobacillus ferrooxidans. Appl. Environ. Microbiol. 70, 5177–5182. doi: 10.1128/AEM.70.9.5177
Andres, J., and Bertin, P. N. (2016). The microbial genomics of arsenic. FEMS Microbiol. Rev. 40, 299–322. doi: 10.1093/femsre/fuv050
Annette, P., Ljerka, K., Bergman, B., and Godfrie, M. R. (1985). Accumulation of aluminium by Anabaena cylindrica into polyphosphate granules and cell walls: an X-ray energy-dispersive microanalysis study. J. Gen. Microbiol. 131, 2545–2548.
Baker, N. A., Sept, D., Joseph, S., Holst, M. J., and McCammon, J. A. (2001). Electrostatics of nanosystems: application to microtubules and the ribosome. Proc. Natl. Acad. Sci. U. S. A. 98, 10037–10041. doi: 10.1073/pnas.181342398
Bauer, A. W., Kirby, W. M., Sherris, J. C., and Turck, M. (1966). Antibiotic susceptibility testing by a standardized single disk method. Am. J. Clin. Pathol. 45, 493–496. doi: 10.1308/rcsann.2013.95.7.532
Belfiore, C., Ordoñez, O. F., and Farías, M. E. (2013). Proteomic approach of adaptive response to arsenic stress in Exiguobacterium sp. S17, an extremophile strain isolated from a high-altitude Andean Lake stromatolite. Extremophiles 17, 421–431. doi: 10.1007/s00792-013-0523-y
Bennett, M. S., Guan, Z., Laurberg, M., and Su, X. D. (2001). Bacillus subtilis arsenate reductase is structurally and functionally similar to low molecular weight protein tyrosine phosphatases. Proc. Natl. Acad. Sci. U. S. A. 98, 13577–13582. doi: 10.1073/pnas.241397198
Berg, S., Kutra, D., Kroeger, T., Straehle, C. N., Kausler, B. X., Haubold, C., et al. (2019). Ilastik: interactive machine learning for (bio)image analysis. Nat. Methods 16, 1226–1232. doi: 10.1038/s41592-019-0582-9
Berman, H., Henrick, K., and Nakamura, H. (2003). Announcing the worldwide protein data Bank. Nat. Struct. Biol. 10:980. doi: 10.1038/nsb1203-980
Bissen, M., Frimmel, F. H., and Ag, C. (2003). Arsenic – a review. part i: occurrence, toxicity, speciation, mobility. Acta Hydrochim. Hydrobiol. 31, 9–18. doi: 10.1002/aheh.200390025
Brim, H., McFarlan, S. C., Fredrickson, J. K., Minton, K. W., Zhai, M., Wackett, L. P., et al. (2000). Engineering Deinococcus radiodurans for metal remediation in radioactive mixed waste environments. Nat. Biotechnol. 18, 85–90. doi: 10.1038/71986
Brim, H., Venkateswaran, A., Kostandarithes, H. M., Fredrickson, J. K., and Daly, M. J. (2003). Engineering Deinococcus geothermalis for bioremediation of high-temperature radioactive waste environments. Appl. Environ. Microbiol. 69, 4575–4582. doi: 10.1128/AEM.69.8.4575-4582.2003
Brochiero, E., Bonaly, J., and Mestre, J. C. (1984). Toxic action of hexavalent chromium on Euglena gracilis cells strain Z grown under heterotrophic conditions. Arch. Environ. Contam. Toxicol. 13, 603–608. doi: 10.1007/BF01056339
Burnley, B. T., Afonine, P. V., Adams, P. D., and Gros, P. (2012). Modelling dynamics in protein crystal structures by ensemble refinement. elife 1, e00311–e00329. doi: 10.7554/eLife.00311
Castro-Severyn, J., Pardo-Esté, C., Sulbaran, Y., Cabezas, C., Gariazzo, V., Briones, A., et al. (2019). Arsenic response of three Altiplanic Exiguobacterium strains with different tolerance levels against the metalloid species: a proteomics study. Front. Microbiol. 10:2161. doi: 10.3389/fmicb.2019.02161
Cerf, O. (1977). A review: tailing of survival curves of bacterial spores. J. Appl. Bacteriol. 42, 1–19. doi: 10.1111/j.1365-2672.1977.tb00665.x
Chauhan, D., Srivastava, P. A., Agnihotri, V., Yennamalli, R. M., and Priyadarshini, R. (2019a). Structure and function prediction of arsenate reductase from Deinococcus indicus DR1. J. Mol. Model. 25:15. doi: 10.1007/s00894-018-3885-3
Chauhan, D., Srivastava, P. A., Ritzl, B., Yennamalli, R. M., Cava, F., and Priyadarshini, R. (2019b). Amino acid-dependent alterations in cell wall and cell morphology of deinococcus indicus DR1. Front. Microbiol. 10:1449. doi: 10.3389/fmicb.2019.01449
Chauhan, D., Srivastava, A., Yennamalli, R. M., and Priyadarshini, R. (2017). Draft genome sequence of Deinococcus indicus DR1, a novel strain isolated from a freshwater wetland. Genome Announc. 5, e00754–e00717. doi: 10.1128/genomeA.00754-17
Chen, V. B., Arendall, W. B., Headd, J. J., Keedy, D. A., Immormino, R. M., Kapral, G. J., et al. (2010). MolProbity: all-atom structure validation for macromolecular crystallography. Acta Crystallogr. Sect. D Biol. Crystallogr. 66, 12–21. doi: 10.1107/S0907444909042073
Chen, Y., Zhang, Q., Wang, D., Shu, Y.-G., and Shi, H. (2023). Memory effect on the survival of Deinococcus radiodurans after exposure in near space. Microbiol. Spectr. 11:e0347422. doi: 10.1128/spectrum.03474-22
Choi, M. H., Jeong, S. W., Shim, H. E., Yun, S. J., Mushtaq, S., Choi, D. S., et al. (2017). Efficient bioremediation of radioactive iodine using biogenic gold nanomaterial-containing radiation-resistant bacterium, Deinococcus radiodurans R1. Chem. Commun. 53, 3937–3940. doi: 10.1039/c7cc00720e
Ciprandi, A., Baraúna, R. A., Santos, A. V., Gonçalves, E. C., Carepo, M. S. P., Schneider, M. P. C., et al. (2012). Proteomic response to arsenic stress in chromobacterium violaceum. J. Integr. OMICS 2, 69–73. doi: 10.5584/jiomics.v2i1.84
Copeland, A., Zeytun, A., Yassawong, M., Nolan, M., Lucas, S., Hammon, N., et al. (2012). Complete genome sequence of the orange-red pigmented, radioresistant Deinococcus proteolyticus type strain (MRPT). Stand. Genomic Sci. 6, 240–250. doi: 10.4056/sigs.2756060
Dani, S. U. (2011). The arsenic for phosphorus swap is accidental, rather than a facultative one, and the question whether arsenic is nonessential or toxic is quantitative, not a qualitative one. Sci. Total Environ. 409, 4889–4890. doi: 10.1016/j.scitotenv.2011.05.044
De Francisco, P., Martín-González, A., Rodriguez-Martín, D., and Díaz, S. (2021). Interactions with arsenic: mechanisms of toxicity and cellular resistance in eukaryotic microorganisms. Int. J. Environ. Res. Public Health 18:12226. doi: 10.3390/ijerph182212226
De Groot, A., Chapon, V., Servant, P., Christen, R., Fischer-Le Saux, M., Sommer, S., et al. (2005). Deinococcus deserti sp. nov., a gamma-radiation-tolerant bacterium isolated from the Sahara Desert. Int. J. Syst. Evol. Microbiol. 55, 2441–2446. doi: 10.1099/ijs.0.63717-0
De La Vega, U. P., Rettberg, P., Douki, T., Cadet, J., and Horneck, G. (2005). Sensitivity to polychromatic UV-radiation of strains of Deinococcus radiodurans differing in their DNA repair capacity. Int. J. Radiat. Biol. 81, 601–611. doi: 10.1080/09553000500309374
Delano, W. L. (2022). He PyMOL molecular graphics system, Version 2.3. Delano Scientific: San Carlos
Dolinsky, T. J., Nielsen, J. E., McCammon, J. A., and Baker, N. A. (2004). PDB2PQR: an automated pipeline for the setup of Poisson-Boltzmann electrostatics calculations. Nucleic Acids Res. 32, W665–W667. doi: 10.1093/nar/gkh381
Eltsov, M., and Dubochet, J. (2005). Fine structure of the Deinococcus radiodurans nucleoid revealed by cryoelectron microscopy of vitreous sections. J. Bacteriol. 187, 8047–8054. doi: 10.1128/JB.187.23.8047-8054.2005
Emsley, P., and Cowtan, K. (2004). Coot: model-building tools for molecular graphics. Acta Crystallogr. Sect. D Biol. Crystallogr. 60, 2126–2132. doi: 10.1107/S0907444904019158
Evans, P. R. (2011). An introduction to data reduction: space-group determination, scaling and intensity statistics. Acta Crystallogr. Sect. D Biol. Crystallogr. 67, 282–292. doi: 10.1107/S090744491003982X
Fekry, M. I., Tipton, P. A., and Gates, K. S. (2011). Kinetic consequences of replacing the internucleotide phosphorus atoms in DNA with arsenic. ACS Chem. Biol. 6, 127–130. doi: 10.1021/cb2000023
Ferreira, A. C., Nobre, M. F., Rainey, F. A., Silva, M. T., Wait, R., Burghardt, J., et al. (1997). Deinococcus geothermalis sp. nov. and Deinococcus murrayi sp. nov., two extremely radiation-resistant and slightly thermophilic species from hot springs. Int. J. Syst. Bacteriol. 47, 939–947. doi: 10.1099/00207713-47-4-939
Garbinski, L. D., Rosen, B. P., and Chen, J. (2019). Pathways of arsenic uptake and efflux. Environ. Int. 126, 585–597. doi: 10.1016/j.envint.2019.02.058.Pathways
Gerber, E., Bernard, R., Castang, S., Chabot, N., Coze, F., Dreux-Zigha, A., et al. (2015). Deinococcus as new chassis for industrial biotechnology: biology, physiology and tools. J. Appl. Microbiol. 119, 1–10. doi: 10.1111/jam.12808
Gladysheva, T. B., Oden, K. L., and Rosen, B. P. (1994). Properties of the arsenate reductase of plasmid R773. Biochemistry 33, 7288–7293. doi: 10.1021/bi00189a033
Halliwell, B. (2006). Redox biology is a fundamental theme of aerobic life. Plant Physiol. 141, 312–322. doi: 10.1104/pp.106.077073.312
Halliwell, B., and Gutteridge, J. M. (1999) in Free radicals in biology and medicine. eds. B. Halliwell and J. M. Gutteridge. 3rd ed (Oxford: Oxford University Press)
Hirsch, P., Gallikowski, C. A., Siebert, J., Peissl, K., Kroppenstedt, R., Schumann, P., et al. (2004). Deinococcus frigens sp. nov., Deinococcus saxicola sp. nov., and Deinococcus marmoris sp. nov., low temperature and draught-tolerating, UV-resistant bacteria from continental Antarctica. Syst. Appl. Microbiol. 27, 636–645. doi: 10.1078/0723202042370008
Hu, Y., Li, J., Lou, B., Wu, R., Wang, G., Lu, C., et al. (2020). The role of reactive oxygen species in arsenic toxicity. Biomol. Ther. 10:240. doi: 10.3390/biom10020240
Huang, A., Teplitski, M., Rathinasabapathi, B., and Ma, L. (2010). Characterization of arsenic-resistant bacteria from the rhizosphere of arsenic hyperaccumulator Pteris vittata. Can. J. Microbiol. 56, 236–246. doi: 10.1139/W10-005
Hudzicki, J. (2012). Kirby-Bauer disk diffusion susceptibility test protocol author information. Am. Soc. Microbiol., 1–13.
Ishikawa, T., Mizunoe, Y., Kawabata, S., Takade, A., Harada, M., Wai, S. N., et al. (2003). The iron-binding protein Dps confers hydrogen peroxide stress resistance to Campylobacter jejuni. J. Bacteriol. 185, 1010–1017. doi: 10.1128/JB.185.3.1010-1017.2003
Jaiganesh, T., Rani, D. V., and Girigoswami, A. (2012). Spectroscopically characterized cadmium sulfide quantum dots lengthening the lag phase of Escherichia coli growth. Spectrochim. Acta - part A Mol. Biomol. Spectrosc. 92, 29–32. doi: 10.1016/j.saa.2012.02.044
James, K. A., Meliker, J. R., and Nriagu, J. O. (2017). “Arsenic” in International encyclopedia of public health. ed. S. R. Quah (Academic Press), 170–175.
Jasso-Chávez, R., Lira-Silva, E., González-Sánchez, K., Larios-Serrato, V., Mendoza-Monzoy, D. L., Pérez-Villatoro, F., et al. (2019). Marine archaeon methanosarcina acetivorans enhances polyphosphate metabolism under persistent cadmium stress. Front. Microbiol. 10, 1–10. doi: 10.3389/fmicb.2019.02432
Ji, G., Garber, E. A. E., Armes, L. G., Chen, C. M., Fuchs, J. A., and Silver, S. (1994). Arsenate reductase of Staphylococcus aureus plasmid pI258. Biochemistry 33, 7294–7299. doi: 10.1021/bi00189a034
Jiang, W., Hou, Q., Yang, Z., Zhong, C., Zheng, G., Yang, Z., et al. (2014). Evaluation of potential effects of soil available phosphorus on soil arsenic availability and paddy rice inorganic arsenic content. Environ. Pollut. 188, 159–165. doi: 10.1016/j.envpol.2014.02.014
Jin, M., Xiao, A., Zhu, L., Zhang, Z., Huang, H., and Jiang, L. (2019). The diversity and commonalities of the radiation-resistance mechanisms of Deinococcus and its up-to-date applications. AMB Express 9:138. doi: 10.1186/s13568-019-0862-x
Juanhuix, J., Gil-Ortiz, F., Cuní, G., Colldelram, C., Nicolás, J., Lidón, J., et al. (2014). Developments in optics and performance at BL13-XALOC, the macromolecular crystallography beamline at the Alba synchrotron. J. Synchrotron Radiat. 21, 679–689. doi: 10.1107/S160057751400825X
Kabsch, W. (2010). XDS. Acta Crystallogr. Sect. D Biol. Crystallogr 66, 125–132. doi: 10.1107/S0907444909047337
Kalyaanamoorthy, S., Minh, B. Q., Wong, T. K. F., Von Haeseler, A., and Jermiin, L. S. (2017). ModelFinder: fast model selection for accurate phylogenetic estimates. Nat. Methods 14, 587–589. doi: 10.1038/nmeth.4285
Kantardjieff, K. A., and Rupp, B. (2003). Matthews coefficient probabilities: Improved estimates for unit cell contents of proteins, DNA, and protein–nucleic acid complex crystals. Protein Sci. 12, 1865–1871. doi: 10.1110/ps.0350503
Keasling, J. D. (1997). Regulation of intracellular toxic metals and other cations by hydrolysis of polyphosphate. Ann. N. Y. Acad. Sci. 829, 242–249. doi: 10.1111/j.1749-6632.1997.tb48579.x
Kehrer, J. P. (2000). The Haber-Weiss reaction and mechanisms of toxicity. Toxicology 149, 43–50. doi: 10.1016/S0300-483X(00)00231-6
Kohen, R., and Nyska, A. (2002). Oxidation of biological systems: oxidative stress phenomena, antioxidants, redox reactions, and methods for their Quanti?Cation. Toxicolofic Pathol. 30, 620–650. doi: 10.1080/0192623029016672
Kolari, M., Nuutinen, J., Rainey, F. A., and Salkinoja-Salonen, M. S. (2003). Colored moderately thermophilic bacteria in paper-machine biofilms. J. Ind. Microbiol. Biotechnol. 30, 225–238. doi: 10.1007/s10295-003-0047-z
Kolari, M., Nuutinen, J., and Salkinoja-Salonen, M. S. (2001). Mechanisms of biofilm formation in paper machine by Bacillus species: the role of Deinococcus geothermalis. J. Ind. Microbiol. Biotechnol. 27, 343–351. doi: 10.1038/sj.jim.7000201
Koppenol, W. H. (1993). The centennial of the Fenton reaction. Free Radic. Biol. Med. 15, 645–651. doi: 10.1016/0891-5849(93)90168-T
Kornberg, A. (1999). “Inorganic polyphosphate: A molecule of many functions” in Progress in molecular and subcellular biology. eds. H. C. Schroder and W. E. G. Muller (Berlin Heidelberg: Springer-Verlag), 1–5.
Kowalski, W. (2009). “Mathematical modeling of UV disinfection” in Ultraviolet germicidal irradiation handbook: UVGI for air and surface disinfection (Berlin: Springer Berlin, Heidelberg), 1–501.
Kowalski, W. J., Bahnfleth, W. P., Witham, D. L., Severin, B. F., and Whittam, T. S. (2000). Mathematical modeling of ultraviolet germicidal irradiation for air disinfection. Quant. Microbiol. 2, 249–270. doi: 10.1023/A:1013951313398
Kulakovskaya, T. (2018). Inorganic polyphosphates and heavy metal resistance in microorganisms. World J. Microbiol. Biotechnol. 34:139. doi: 10.1007/s11274-018-2523-7
Laskowski, R. A., MacArthur, M. W., Moss, D. S., and Thornton, J. M. (1993). PROCHECK: a program to check the stereochemical quality of protein structures. J. Appl. Crystallogr. 26, 283–291. doi: 10.1107/s0021889892009944
Letunic, I., and Bork, P. (2021). Interactive tree of life (iTOL) v5: an online tool for phylogenetic tree display and annotation. Nucleic Acids Res. 49, W293–W296. doi: 10.1093/nar/gkab301
Li, B., Qiu, Y., Shi, H., and Yin, H. (2016). The importance of lag time extension in determining bacterial resistance to antibiotics. Analyst 141, 3059–3067. doi: 10.1039/c5an02649k
Liebschner, D., Afonine, P. V., Baker, M. L., Bunkoczi, G., Chen, V. B., Croll, T. I., et al. (2019). Macromolecular structure determination using X-rays, neutrons and electrons: recent developments in Phenix. Acta Crystallogr. Sect. D Struct. Biol. 75, 861–877. doi: 10.1107/S2059798319011471
Lim, S., Jung, J. H., Blanchard, L., and De Groot, A. (2019). Conservation and diversity of radiation and oxidative stress resistance mechanisms in Deinococcus species. FEMS Microbiol. Rev. 43, 19–52. doi: 10.1093/femsre/fuy037
Machado, A. F., Hovland, D. N., Pilafas, S., and Collins, M. D. (1999). Teratogenic response to arsenite during neurulation: relative sensitivities of C57BL/6J and SWV/Fnn mice and impact of the splotch allele. Toxicol. Sci. 51, 98–107. doi: 10.1093/toxsci/51.1.98
Makk, J., Tóth, E. M., Anda, D., Pál, S., Schumann, P., Kovács, A. L., et al. (2016). Deinococcus budaensis sp. Nov., a mesophilic species isolated from a biofilm sample of a hydrothermal spring cave. Int. J. Syst. Evol. Microbiol. 66, 5345–5351. doi: 10.1099/ijsem.0.001519
Manobala, T., Shukla, S. K., Subba Rao, T., and Dharmendira Kumar, M. (2019). A new uranium bioremediation approach using radio-tolerant Deinococcus radiodurans biofilm. J. Biosci. 44, 1–9. doi: 10.1007/s12038-019-9942-y
Matthews, B. W. (1968). Solvent content of protein crystals. J. Mol. Biol. 33, 491–497. doi: 10.1016/0022-2836(68)90205-2
McCoy, A. J., Grosse-Kunstleve, R. W., Adams, P. D., Winn, M. D., Storoni, L. C., and Read, R. J. (2007). Phaser crystallographic software. J. Appl. Crystallogr. 40, 658–674. doi: 10.1107/S0021889807021206
Messens, J., Hayburn, G., Desmyter, A., Laus, G., and Wyns, L. (1999). The essential catalytic redox couple in arsenate reductase from Staphylococcus aureus. Biochemistry 38, 16857–16865. doi: 10.1021/bi9911841
Messens, J., Martins, J. C., Brosens, E., Van Belle, K., Jacobs, D. M., Willem, R., et al. (2002a). Kinetics and active site dynamics of Staphylococcus aureus arsenate reductase. J. Biol. Inorg. Chem. 7, 146–156. doi: 10.1007/s007750100282
Messens, J., Martins, J. C., Van Belle, K., Brosens, E., Desmyter, A., De Gieter, M., et al. (2002b). All intermediates of the arsenate reductase mechanism, including an intramolecular dynamic disulfide cascade. Proc. Natl. Acad. Sci. U. S. A. 99, 8506–8511. doi: 10.1073/pnas.132142799
Messens, J., and Silver, S. (2006). Arsenate reduction: thiol cascade chemistry with convergent evolution. J. Mol. Biol. 362, 1–17. doi: 10.1016/j.jmb.2006.07.002
Minh, B. Q., Schmidt, H. A., Chernomor, O., Schrempf, D., and Woodhams, M. D., von Haeseler, A. von von Haeseler, A., and Lanfear, R. (2020). IQ-TREE 2: new models and efficient methods for phylogenetic inference in the genomic era. Mol. Biol. Evol., 37, 1530–1534, doi: 10.1093/molbev/msaa015
Misra, C. S., Appukuttan, D., Kantamreddi, V. S. S., Rao, A. S., and Apte, S. K. (2012). Recombinant D. radiodurans cells for bioremediation of heavy metals from acidic/neutral aqueous wastes. Bioeng. Bugs 3, 44–48. doi: 10.4161/bbug.3.1.18878
Mukhopadhyay, R., and Rosen, B. P. (1998). Saccharomyces cerevisiae ACR2 gene encodes an arsenate reductase. FEMS Microbiol. Lett. 168, 127–136. doi: 10.1016/S0378-1097(98)00430-3
Mukhopadhyay, R., and Rosen, B. P. (2002). Arsenate reductases in prokaryotes and eukaryotes. Environ. Health Perspect. 110, 745–748. doi: 10.1289/ehp.02110s5745
Mukhopadhyay, R., Shi, J., and Rosen, B. P. (2000). Purification and characterization of Acr2p, the Saccharomyces cerevisiae arsenate reductase. J. Biol. Chem. 275, 21149–21157. doi: 10.1074/jbc.M910401199
Munakata, N., Saito, M., and Hieda, K. (1991). Inactivation action spectra of bacillus subtilis spores in extended ultraviolet wavelengths (50–300nm) obtained with synchrotron radiation. Photochem. Photobiol. 54, 761–768. doi: 10.1111/j.1751-1097.1991.tb02087.x
Murshudov, G. N., Vagin, A. A., and Dodson, E. J. (1997). Refinement of macromolecular structures by the maximum-likelihood method. Acta Crystallogr. Sect. D Biol. Crystallogr. 53, 240–255. doi: 10.1107/S0907444996012255
National Research Council (2001). “National Research Council (US) Subcommittee to Update the 1999 Arsenic,” in Drinking Water Report. Arsenic in Drinking Water: 2001 Update. Washington, DC: National Academies Press (US).
Nielsen, A. M., and Sojka, G. A. (1979). Photoheterotrophic utilization of acetate by the wild type and an acetate-adapted mutant of Rhodopseudomonas capsulata. Arch. Microbiol. 120, 39–42. doi: 10.1007/BF00413270
Nunes, C. I. P., Brás, J. L. A., Najmudin, S., Moura, J. J. G., Moura, I., and Carepo, M. S. P. (2014). ArsC3 from Desulfovibrio alaskensis G20, a cation and sulfate-independent highly efficient arsenate reductase. J. Biol. Inorg. Chem. 19, 1277–1285. doi: 10.1007/s00775-014-1184-8
O’Day, P. A. (2006). Chemistry and mineralogy of arsenic. Elements 2, 77–83. doi: 10.2113/gselements.2.2.77
Oden, K. L., Gladysheva, T. B., and Rosen, B. P. (1994). Arsenate reduction mediated by the plasmid-encoded ArsC protein is coupled to glutathione. Mol. Microbiol. 12, 301–306. doi: 10.1111/j.1365-2958.1994.tb01018.x
Orell, A., Navarro, C. A., Rivero, M., Aguilar, J. S., and Jerez, C. A. (2012). Inorganic polyphosphates in extremophiles and their possible functions. Extremophiles 16, 573–583. doi: 10.1007/s00792-012-0457-9
Páez-Espino, D., Tamames, J., De Lorenzo, V., and Cánovas, D. (2009). Microbial responses to environmental arsenic. Biometals 22, 117–130. doi: 10.1007/s10534-008-9195-y
Pan-Hou, H., Kiyono, M., Kawase, T., Omura, T., and Endo, G. (2001). Evaluation of ppk -specified polyphosphate as a mercury remedial tool. Biol. Pharm. Bull. 24, 1423–1426. doi: 10.1248/bpb.24.1423
Pan-Hou, H., Kiyono, M., Omura, H., Omura, T., and Endo, G. (2002). Polyphosphate produced in recombinant Escherichia coli confers mercury resistance. FEMS Microbiol. Lett. 207, 159–164. doi: 10.1111/j.1574-6968.2002.tb11045.x
Peltola, M., Kanto Öqvist, C., Ekman, J., Kosonen, M., Jokela, S., Kolari, M., et al. (2008). Quantitative contributions of bacteria and of Deinococcus geothermalis to deposits and slimes in paper industry. J. Ind. Microbiol. Biotechnol. 35, 1651–1657. doi: 10.1007/s10295-008-0409-7
Peverly, J. H., Adamec, J., and Parthasarathy, M. V. (1978). Association of Potassium and some Other Monovalent Cations with occurrence of polyphosphate bodies in Chlorella pyrenoidosa. Plant Physiol. 62, 120–126. doi: 10.1104/pp.62.1.120
Potterton, E., Briggs, P., Turkenburg, M., and Dodson, E. (2003). Biological crystallography A graphical user interface to the CCP4 program suite. Acta Crystallogr. Sect. D Biol. Crystallogr. 59, 1131–1137. doi: 10.1107/S0907444903008126
Preibisch, S., Saalfeld, S., and Tomancak, P. (2009). Globally optimal stitching of tiled 3D microscopic image acquisitions. Bioinformatics 25, 1463–1465. doi: 10.1093/bioinformatics/btp184
Pruitt, K. M., and Kamau, D. N. (1993). Mathematical models of bacterial growth, inhibition and death under combined stress conditions. J. Ind. Microbiol. 12, 221–231. doi: 10.1007/BF01584194
Ranganathan, S., Sethi, D., Kasivisweswaran, S., Ramya, L., Priyadarshini, R., and Yennamalli, R. M. (2023). Structural and functional mapping of ars gene cluster in Deinococcus indicus DR1. Comput. Struct. Biotechnol. J. 21, 519–534. doi: 10.1016/j.csbj.2022.12.015
Rosen, B. P. (1999). Families of arsenic transporters. Trends Microbiol. 7, 207–212. doi: 10.1016/S0966-842X(99)01494-8
Rosenberg, H., Gerdes, R. G., and Chegwidden, K. (1977). Two systems for the uptake of phosphate in Escherichia coli. J. Bacteriol. 131, 505–511. doi: 10.1128/jb.131.2.505-511.1977
Ruiz, O. N., Alvarez, D., Gonzalez-Ruiz, G., and Torres, C. (2011). Characterization of mercury bioremediation by transgenic bacteria expressing metallothionein and polyphosphate kinase. BMC Biotechnol. 11, 1–18. doi: 10.1186/1472-6750-11-82
Santos, S. P., Mitchell, E. P., Franquelim, H. G., Castanho, M. A. R. B., Abreu, I. A., and Romão, C. V. (2015). Dps from Deinococcus radiodurans: oligomeric forms of Dps1 with distinct cellular functions and Dps2 involved in metal storage. FEBS J. 282, 4307–4327. doi: 10.1111/febs.13420
Sanz-Luque, E., Bhaya, D., and Grossman, A. R. (2020). Polyphosphate: A multifunctional metabolite in Cyanobacteria and algae. Front. Plant Sci. 11, 1–21. doi: 10.3389/fpls.2020.00938
Schindelin, J., Arganda-Carreras, I., Frise, E., Kaynig, V., Longair, M., Pietzsch, T., et al. (2012). Fiji: an open-source platform for biological-image analysis. Nat. Methods 9, 676–682. doi: 10.1038/nmeth.2019
Scott, J. A., and Palmer, S. J. (1990). Sites of cadmiun uptake in bacteria used for biosorption. Appl. Microbiol. Biotechnol. 33, 221–225. doi: 10.1007/BF00176529
Severin, B. F., Suidan, M. T., and Engelbrecht, R. S. (1983). Kinetic modeling of U.V. disinfection of water. Water Res. 17, 1669–1678. doi: 10.1016/0043-1354(83)90027-1
Shah, S., and Damare, S. R. (2018). Differential protein expression in a marine-derived Staphylococcus sp. NIOSBK35 in response to arsenic(III). Biotech 8:287. doi: 10.1007/s13205-018-1307-y
Shi, J., Vlamis-Gardikas, A., Åslund, F., Holmgren, A., and Rosen, B. P. (1999). Reactivity of glutaredoxins 1, 2, and 3 from Escherichia coli shows that glutaredoxin 2 is the primary hydrogen donor to ArsC-catalyzed arsenate reduction. J. Biol. Chem. 274, 36039–36042. doi: 10.1074/jbc.274.51.36039
Slade, D., and Radman, M. (2011). Oxidative stress resistance in Deinococcus radiodurans. Microbiol. Mol. Biol. Rev. 75, 133–191. doi: 10.1128/MMBR.00015-10
Sleytr, U. B., Silva, M. T., Kocur, M., and Lewis, N. F. (1976). The fine structure of Micrococcus radiophilus and Micrococcus radioproteolyticus. Arch. Microbiol. 107, 313–320. doi: 10.1007/BF00425346
Srimani, J. K., Huang, S., Lopatkin, A. J., and You, L. (2017). Drug detoxification dynamics explain the postantibiotic effect. Mol. Syst. Biol. 13:948. doi: 10.15252/msb.20177723
Strawn, D. G. (2018). Review of interactions between phosphorus and arsenic in soils from four case studies. Geochem. Trans. 19, 10–13. doi: 10.1186/s12932-018-0055-6
Suresh, K., Reddy, G. S. N., Sengupta, S., and Shivaji, S. (2004). Deinococcus indicus sp. nov., an arsenic-resistant bacterium from an aquifer in West Bengal. India. Int. J. Syst. Evol. Microbiol. 54, 457–461. doi: 10.1099/ijs.0.02758-0
Tawfik, D. S., and Viola, R. E. (2011). Arsenate replacing phosphate: alternative life chemistries and ion promiscuity. Biochemistry 50, 1128–1134. doi: 10.1021/bi200002a
Tekere, M. (2019). Microbial bioremediation and different bioreactors designs applied. Biotechnol. Bioeng., 1–19. doi: 10.5772/intechopen.83661
Terwilliger, T. C., Grosse-Kunstleve, R. W., Afonine, P. V., Moriarty, N. W., Zwart, P. H., Hung, L. W., et al. (2007). Iterative model building, structure refinement and density modification with the PHENIX AutoBuild wizard. Acta Crystallogr. Sect. D Biol. Crystallogr. 64, 61–69. doi: 10.1107/S090744490705024X
Thompson, D., Higgins, D. G., and Gibson, T. J. (1994). CLUSTAL W: improving the sensitivity of progressive multiple sequence alignment through sequence weighting, position-specific gap penalties and weight matrix choice. Nucleic Acids Res. 22, 4673–4680. doi: 10.1093/nar/22.22.4673
Thornley, M. J., Horne, R. W., and Glauert, A. M. (1965). The fine structure of micrococcus radiodurans. Arch. Mikrobiol. 51, 267–289. doi: 10.1007/BF00408143
Tian, B., Wu, Y., Sheng, D., Zheng, Z., Gao, G., and Hua, Y. (2004). Chemiluminescence assay for reactive oxygen species scavenging activities and inhibition on oxidative damage of DNA in Deinococcus radiodurans. Luminescence 19, 78–84. doi: 10.1002/bio.761
Tickle, I. J., Flensburg, C., Keller, P., Paciorek, W., Sharff, A., Vonrhein, C., et al. (2018). Staraniso. Glob. Phasing Ltd. Cambridge.
Urech, K., Dürr, M., Boller, T., Wiemken, A., and Schwencke, J. (1978). Localization of polyphosphate in vacuoles of Saccharomyces cerevisiae. Arch. Microbiol. 116, 275–278. doi: 10.1007/BF00417851
Vagin, A., and Lebedev, A. (2015). MoRDa, an automatic molecular replacement pipeline. Found. Adv. 71:S19. doi: 10.1107/S2053273315099672
Valentine, J. S., Wertz, D. L., Lyons, T. J., Liou, L. L., Goto, J. J., and Gralla, E. B. (1998). The dark side of dioxygen biochemistry. Curr. Opin. Chem. Biol. 2, 253–262. doi: 10.1016/S1367-5931(98)80067-7
Varadwaj, A., Varadwaj, P. R., Marques, H. M., and Yamashita, K. (2022). The Pnictogen bond: the covalently bound arsenic atom in molecular entities in crystals as a Pnictogen bond donor. Molecules 27, 23–29. doi: 10.3390/molecules27113421
Vonrhein, C., Flensburg, C., Keller, P., Sharff, A., Smart, O., Paciorek, W., et al. (2011). Data processing and analysis with the autoPROC toolbox. Acta Crystallogr. Sect. D Biol. Crystallogr. 67, 293–302. doi: 10.1107/S0907444911007773
Wang, L. K., Wang, M. H. S., Hung, Y. T., Shammas, N. K., and Chen, J. P. (2017). Handbook of advanced industrial and hazardous wastes management. Boca Raton: CRC Press.
Wang, L., Zhuang, X., Zhuang, G., and Jing, C. (2016). Arsenic resistance strategy in Pantoea sp. IMH: organization, function and evolution of ars genes. Sci. Rep. 6:39195. doi: 10.1038/srep39195
Weiss, S., Carapito, C., Cleiss, J., Koechler, S., Turlin, E., Coppee, J. Y., et al. (2009). Enhanced structural and functional genome elucidation of the arsenite-oxidizing strain Herminiimonas arsenicoxydans by proteomics data. Biochimie 91, 192–203. doi: 10.1016/j.biochi.2008.07.013
Wolf, S. G., Rez, P., and Elbaum, M. (2015). Phosphorus detection in vitrified bacteria by cryo-STEM annular dark-field analysis. J. Mic. 260, 227–233. doi: 10.1111/jmi.12289
Yang, H. C., and Rosen, B. P. (2016). New mechanisms of bacterial arsenic resistance. Biom. J. 39, 5–13. doi: 10.1016/j.bj.2015.08.003
Keywords: metals, UV-C, oxidative stress, PolyP granules, arsenate reductase, arsenate
Citation: Gouveia AG, Salgueiro BA, Ranmar DO, Antunes WDT, Kirchweger P, Golani O, Wolf SG, Elbaum M, Matias PM and Romão CV (2023) Unraveling the multifaceted resilience of arsenic resistant bacterium Deinococcus indicus. Front. Microbiol. 14:1240798. doi: 10.3389/fmicb.2023.1240798
Edited by:
Melina Kerou, University of Vienna, AustriaReviewed by:
John R. Battista, Louisiana State University, United StatesPatricia De Francisco, Spanish National Research Council (CSIC), Spain
Min-Kyu Kim, Korea Atomic Energy Research Institute (KAERI), Republic of Korea
Copyright © 2023 Gouveia, Salgueiro, Ranmar, Antunes, Kirchweger, Golani, Wolf, Elbaum, Matias and Romão. This is an open-access article distributed under the terms of the Creative Commons Attribution License (CC BY). The use, distribution or reproduction in other forums is permitted, provided the original author(s) and the copyright owner(s) are credited and that the original publication in this journal is cited, in accordance with accepted academic practice. No use, distribution or reproduction is permitted which does not comply with these terms.
*Correspondence: Célia V. Romão, cmromao@itqb.unl.pt