- Department of Biotechnology, Indian Institute of Technology Hyderabad, Sangareddy, Telangana, India
Myxobacteria are an intriguing group of social-behavior-depicting microbes with unique physiological characteristics such as fruiting body formation, gliding motility, and predation, encompassing the largest genomes (>9 Mb) within the Eubacteria kingdom. These soil-dwelling organisms are crucial for lignocellulosic biomass degradation, which has both ecological and industrial significance. While previous studies have demonstrated polysaccharide deconstruction abilities in a few myxobacterial species, we aim to elucidate the distribution of their Carbohydrate Active Enzymes (CAZymes) domains per organism, with a focus on proteins involved in the catabolism of critical polysaccharides such as cellulose, lignin, xylan, starch, pectin, fructan, chitin, and dextran, across 61 high-quality sequenced myxobacterial genomes. Our findings reveal that 3.5% of the total genes at the median level have domains related to CAZyme functions across different myxobacterial families. Notably, family Archangiaceae (4.4%) and Myxococcaceae (3.7%) members exhibit the most significant genomic diversity and potential for degrading multiple substrates within lignocellulosic biomass. These plentiful CAZymes probably enable these majorly soil-harboring myxobacteria to breakdown various carbohydrate substrates into simpler biological molecules, which not only allow these organisms to sustain in poor-nutrient environments but also enable them to be critical players in carbon cycling and organic matter decomposition. Overall, it can be postulated that myxobacteria have an unexplored genomic potential that may play an integral role in the degradation of recalcitrant plant biomass, potentially influencing soil health and composition. This study further suggests the critical ecological importance of these CAZymes in sustaining the balance of terrestrial ecosystems and diverse industrial applications.
Highlights
• Polysaccharides are the most abundant polymers, making up the Earth’s biomass.
• Polysaccharide degradation is well-known to be carried out by diverse microorganisms; however, there is more to be explored concerning the novel organisms that can degrade these biomolecules efficiently along with understanding the reactions carried out in this process.
• Soil-dwelling myxobacteria, model organisms for our study, are unique and under-studied social-behavior-depicting microbes.
• This research investigated the genomic potential of the 61 myxobacterial organisms to encode carbohydrate-active enzymes that break down various substrates, including lignocellulosic biomass, predominantly in their habitat.
Introduction
Carbohydrates, primarily polysaccharides such as cellulose, starch, xylan, chitin, lignin, pectin, fructan, dextran, etc., (Berlemont and Martiny, 2015) are the most abundant naturally occurring polymeric biomolecules with numerous imperative biological, pharmacological, and industrial applications (Mohammed et al., 2021) such as textile processing, detergent production, bleaching of paper and prebiotic production (Chettri et al., 2020). As per recent studies, the most abundant biologically renewable resource on earth is the lignocellulosic biomass (LCB; Yousuf et al., 2020), which is primarily composed of cellulose, hemicellulose, and lignin (Cortes-Tolalpa et al., 2017). LCB captures attention as it can serve as an alternative to fossil fuels and pave the way to become the source of biofuels, to meet the ever-increasing demand for energy (Zoghlami and Paës, 2019; Inyang et al., 2022). Other important polysaccharides are xylan, a component of hemicellulose (Bajpai, 2009); chitin, which is abundant in the shells of arthropods like crabs, shrimps, and insects, and produced by bacteria and fungi (Mohammed et al., 2021); pectin, mostly found in plant cell walls and peels of citrus fruits (Mudgil, 2017); fructans, which are reserve carbohydrates (Stick and Williams, 2009) and dextrans, which are microbial water-soluble exopolysaccharides (Silva et al., 2017). The utilization of LCB or other polysaccharides requires physiochemical processing and degradation (Ezeilo et al., 2017; Devi et al., 2022) by diverse Carbohydrate-Active enZymes (CAZymes; Ezeilo et al., 2017; Zerva et al., 2021). Based on their mechanisms of action, these CAZymes can be classified into three categories, i.e., (1) enzymes involved in their assembly or glycosyltransferases (GT), and (2) enzymes involved in their breakdown—glycoside hydrolases (GH), polysaccharide lyases (PL), carbohydrate esterases (CE), and (3) accessory functions that contain auxiliary activities (AAs) and carbohydrate-binding modules (CBMs). GHs carry out the hydrolysis of the glycosidic linkage, GTs help in the making of glycosidic bonds, PLs cause the breakdown of glycosidic bonds in a non-hydrolytic manner, CEs hydrolyses the carbohydrate esters, AAs are redox enzymes that play a role in association with other CAZymes, and CBMs help in adhesion to the carbohydrate molecule upon which the enzymes act (Lombard et al., 2014). Therefore, the understanding of enzymatic reactions behind these processes can help in improving strategies to cope with the recalcitrant nature of some polysaccharides toward degradation (Ezeilo et al., 2017), further helping in utilizing their potential more efficiently.
Using a diversity of enzymes specific to each carbohydrate substrate, microorganisms degrade miscellaneous carbohydrates in their ecological niches such as the soil (Yeager et al., 2017), oceans (Sichert and Cordero, 2021), or the human gut (Flint et al., 2012) and maintain equilibrium in those ecosystems. Based on the abundance of these enzymes, LCB decomposition potential has been reported for bacteria, fungi, as well as protozoa (Harindintwali et al., 2022). Recent metagenomic studies of rhizosphere microorganisms have shown a plenitude of carbohydrate-active enzymes in Actinobacteria, Proteobacteria, Bacteroidetes, Verrucomicrobia, Gemmatimonadetes and Firmicutes (Tashkandi and Baz, 2023). Bacteroidetes members have also been reported to encode a lot of carbohydrate-degrading genes, playing a critical role in carbohydrate digestion in our gut (Flint et al., 2012). The cellulose-degrading potential of Pseudomonas, Streptococcus, and Bacillus species has been widely utilized with several applications in the paper, food, and biofuel industries (Zhang and Dong, 2022). However, myxobacteria, which are a notable group of large-genome size non-pathogenic soil-dwelling social microbes, have not been investigated extensively.
Myxobacteria belong to the order Myxococcales within the phylum Proteobacteria, however, recently, the International Committee on Systematics of Prokaryotes (ICSP) classified them under a new phylum, Myxococcota (Oren and Garrity, 2021; Panda et al., 2022). They are a fascinating group of prokaryotes depicting complex social behavior in the form of fruiting body formation, social motility or swarming, biofilm formation, and predation (Muñoz-Dorado et al., 2016; Zusman et al., 2007; Kaiser et al., 2010). Most of these organisms are aerobic with gigantic genomes ranging from 9 to 16 Mbps, with a few exceptions (Mohr, 2018). Myxobacteria are typically soil bacteria that can thrive in almost any kind of environment, be it temperate regions, tropical rain forests, arctic tundra, deserts, or marine/saline environments. They are known as ‘wolf-pack’ predators and can prey upon bacteria (both, Gram-positive and Gram-negative bacteria) and fungi through toxic secretions into the extracellular environment (Marshall and Whitworth, 2019). An industrially and clinically pertinent characteristic of myxobacteria is that they have a remarkable potential to produce a plethora of secondary metabolites showing a broad spectrum of biological activities such as anti-bacterial, anti-fungal, immunosuppressive effects, and insecticidal, anti-malarial, and herbicidal activities (Reichenbach, 2001; de Frias et al., 2018). Overall, these diverse aspects make myxobacteria quite extraordinary and distinct from other bacteria and, therefore, intriguing to study.
Order Myxococcales have been classified into three broad suborders, nine families, 20 genera, and > 200 species (Figure 1; Supplementary Table S1; Mohr, 2018). Most of the members are soil microbes, with a few being marine; however, only some organisms are well studied for their carbohydrate degradation activities, mainly in the context of predation, where these enzymes help in the breakdown of the cell wall of prey bacteria (Zhou et al., 2021; Li Y. et al., 2022; Zhang et al., 2023). Sorangium cellulosum of the suborder Sorangiineae decomposes cellulose and crystalline cellulose. Most of its strains can also degrade starch, xylan, and chitin (Li Y. et al., 2022; Reichenbach, 2005). Sandaracinus amylolyticus, another Sorangiineae member, degrades starch and has characterized proteins with domains involved in degrading chitin, agar, and cellulose reported in its genome (Sharma et al., 2016). The Archangiaceae family has several genera among which, Cystobacter fuscus and Stigmatella aurantiaca are well known to degrade chitin whereas Archangium gephyra does not degrade chitin (Sharma and Subramanian, 2017). Chitin degrading activity has also been reported in Corallococcus sp. EGB (Zhao et al., 2021) and Myxococcus fulvus (Shahbaz and Yu, 2020), both of which belong to the suborder Cystobacterineae.
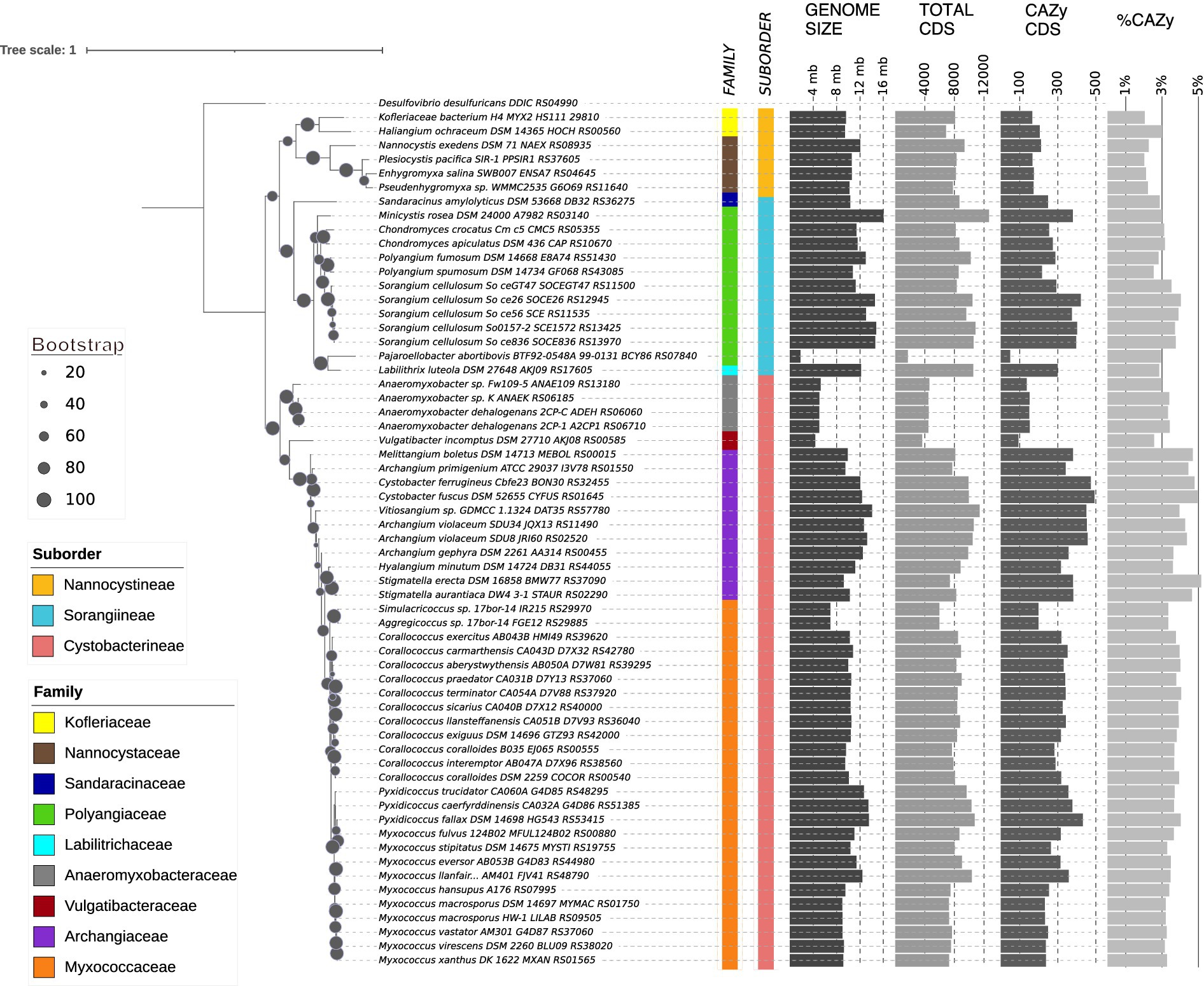
Figure 1. 16 s rRNA phylogenetic tree depicting the myxobacterial evolutionary relationship at suborder, family, and genus levels along with CAZyme distribution. Each label contains the organism’s name with strain and 16S rRNA locus tag. Bootstrap values are represented by the diameter of the circles on the branches of the phylogenetic tree according to the scale shown on the left. Taxonomic classification of the three suborders and associated nine families are represented by the color strips according to the left-bottom legends. Bar graphs on the right represent the genome size, CDS counts, CAZyme domain containing CDS counts, and percentage of CAZyme domain containing CDSs.
Although the carbohydrate degrading potential of a few myxobacterial species has been previously studied, this study aims to perform a high-throughput pan-phylum genomic study to explore the distribution, architecture, and functions of these carbohydrate degrading enzymes (CAZymes). This study investigated 61 myxobacterial genomes (majorly complete and a few draft assemblies) and analyzed their CAZymes to untap their polysaccharide-degradation genomic potential. The study highlights varied CAZymes with diverse domain architecture among myxobacterial families, challenging assumptions about their genome size and CAZyme density correlation.
Methods
Selection of organisms
Sixty-one reference and representative myxobacterial organisms were selected from NCBI RefSeq based on representation of each suborder and family level taxonomy and best possible chromosome-level assembly (draft assemblies were selected only when chromosomal level assembly was not available). Based on assembly and annotation files, a genome statistics table is created to showcase their genomic features such as taxonomy, assembly, NCBI genome ID, genome size, GC%, contigs, CDS number, %CAZyme CDS as identified by custom shell scripts (Figure 1; Supplementary Table S1). %CAZyme CDS were calculated per genome from the number of CDSs having at least one CAZyme domain divided by the total CDSs in that genome.
Phylogenetic analysis using 16 s rRNA sequences
16 s rRNA sequences of all myxobacterial organisms along with outgroup, Desulfovibrio desulfuricans, were extracted and multiple sequence alignment was performed using MUSCLE v3.8.1551. The GTR + GAMMA+I model of RAxML v8.2.12 was used to build the phylogeny tree with 100 bootstraps. Visualization of the resultant tree was done using iTOL v6 (Letunic and Bork, 2021),1 along with suborder and family information mapping.
Domain-based homology detection and domain architecture construction
CAZyme domains were identified from the myxobacterial protein sequences by performing hmmscan (Finn et al., 2011; HMMER 3.3.2 installed in Nov 2020) against the dbCAN-HMMdb-V9 profile database downloaded from http://www.cazy.org/, using default parameters in domtblout output format. The output was parsed using a shell script to sort the output as per overlapped/redundant domains by retaining high-quality matches and calculating the covered fraction of hmm profiles. The parsed and sorted data was represented in the form of ‘domain architecture’ wherein the domains were arranged in order of their position in the protein sequence using a custom R script.
CAZyme distribution
The counts of proteins per organism containing domains specific to each CAZyme category were calculated to obtain the distribution values from the architecture. The distribution was obtained at two levels, i.e., CAZyme broad category level and CAZyme family/subfamily level. Custom shell and Python scripts were written to perform these tasks.
Curation of specific CAZyme domains, their architecture and distribution
The different types of CAZymes and their EC numbers were identified through literature search (Joshi et al., 2021; Hamid et al., 2013; Kumar and Chandra, 2020; Li et al., 2021; Krivorotova and Sereikaite, 2014; Khalikova et al., 2005; Ng and Randy, 2011). The list of CAZyme domains associated with each enzyme was identified by searching respective “EC Number” in CAZydb. The protein modular architecture specific to those domains was extracted using custom shell scripts. The enzyme-specific domains were also explored to showcase their occurrence in an alone/ duplicate/ combination manner with similar or different domains.
Correlation and regression analysis
Correlation analyses were carried out using the lm function in R and the plots for these analyses were made using ggplot2. We performed correlation analysis between genome size and the total number of CAZyme domains per organism and similarly between genome size and distribution of each of the six CAZyme categories individually.
Principal component analysis
The principal component analysis was carried out using the prcomp method and ggplot2 package in R, further clustering using family and suborder information. The principal components used for this analysis were the counts of the six CAZyme categories-AA, GH, GT, CBM, PL, and CE per organism.
Results
Our studied dataset represents the whole myxobacterial taxonomy
Our dataset included 61 order Myxococcales organisms, which has ~70% (42/61), ~10% (6/61), and ~ 20% (13/61) representation in three suborders, i.e., Cystobacterineae, Nannocystineae and Sorangiineae, respectively (Figure 1; Supplementary Table S1). We selected these organisms to capture the vast diversity of myxobacteria from all taxonomic lineages with a priority of having a complete genome. As Myxococcus and Corallococcus spp. from family Myxococcaceae are the most studied and characterized myxobacteria (Garcia and Müller, 2014), family Myxococcaceae within the suborder Cystobacterineae have the highest representation (26/61; ~40%) in our dataset. Other predominant families, i.e., Archangiaceae and Polyangiaceae have 11 members each representing ~20% of the total dataset whereas the rest of the six families have 1–4 members each. Out of 26 unique genera, Myxococcus and Corallococcus have 10 and 11 members, respectively. Most of these organisms are soil-dwelling, isolated from diverse lignocellulose biomass present in different countries. However, all suborder Nannocystineae members are present in marine high-salt environments. It is also noteworthy to mention that Myxococcus fulvus HW-1 is the only member from the suborder Cystobacterineae that has been isolated from the marine environment (Albataineh and Stevens, 2018).
Apart from their habitat and taxonomy, most organisms in the studied dataset have a 9–16 Mb genome size (which is higher than the average bacterial genome size) suggesting their complex metabolic and regulatory implications (Whitworth et al., 2021). Anaeromyxobacter spp., Vulgatibacter incomptus, and Pajaroellobacter abortibovis are exceptions as they have smaller genome size (<5 Mb) and a few other physiological characteristics such as respiration, predation, etc., different form the rest of the myxobacteria (Sanford et al., 2002; Yamamoto et al., 2014). This dataset includes several largest genomes known so far including Minicystis rosea (16.04 Mb genome; Kellner et al., 2018; Pal et al., 2021), Sorangium spp. (11–14 Mb genomes; Land et al., 2015), followed by family Archangiaceae and Myxococcaceae members. We found that the myxobacterial family-wise mean genome size is almost 3–4 times greater than the previously reported average bacterial genome size, ∼3.87 Mb (diCenzo and Finan, 2017). The highest count of total coding sequences (CDS) is present in M. rosea and the lowest in P. abortibovis. As anticipated, encoded genes per genome show a good correlation with genome size (R2 = 0.96). The GC content ranges from ~66–75% in our dataset except for P. abortibovis with 47.45%. Bacterial GC content can range from 16 to 75% (Lightfield et al., 2011) with Anaeromyxobacter dehalogenans 2CP-C at the apex having 74.9% and the other myxobacteria are located in the upper quartile of this range.
Myxobacteria harbor a diverse CAZyme repertoire across their monophyletic taxonomic lineages
First, we employed 16S rRNA sequences of all 61 myxobacterial organisms to build a maximum-likelihood phylogenetic tree to comprehend their evolutionary relationship (Figure 1). This phylogeny was able to perfectly cluster the organisms into distinct clades based on their taxonomic classification at suborder, family, and genus levels. This phylogeny depicts two major clades differentiating into suborder Cystobacterineae members and Nannocystineae and Sorangiineae members together, further separated into their subclades. All families but Archangiaceae show a monophyletic distribution, thereby making a single clade for all members at the genus and species level. However, one of the Archangium species within the family Archangiaceae is present at two different branches suggesting the requisite of better phylogenetic markers for their classification.
To understand the relative distribution of CAZyme domains and associated polysaccharide degradation capabilities of order Myxococcales, we functionally characterized all myxobacterial protein sequences based on CAZyme domains using the HMMER suite’s hmmscan module against the dbCAN CAZyme database. We also mapped the organism-wise CAZyme CDS distribution to the phylogeny alongside the total CDSs (Figure 1) and we saw that some species have a higher CAZyme density. Our analysis reported that myxobacteria utilize almost 2–5% of the total CDSs in carbohydrate-degrading enzyme functions. This study further revealed that members of the suborder Cystobacterineae in the dataset we curated have a slightly higher proportion of CAZyme domains in their genomes as compared to suborder Sorangiineae with median values of 3.66 and 3.11%, respectively (Figure 2A), with the lowest, 2.16%, in suborder Nannocystineae. Pairwise Welch’s t-test showed that the CAZyme CDSs are significantly higher in Cystobacterineae (p value = 1.3e−05) and Sorangiineae as compared to Nannocystineae (p-value = 0.0003). However, as we have more representation from Cystobacterineae members in the present dataset, future studies should include more members from other suborders to confirm this correlation. The lower abundance in marine organisms can be explained by their presence in the marine salt-tolerant ecosystem as the meso and bathypelagic layers of the ocean do not have as much availability of organic material for such bacterial species to break down (Baltar et al., 2009). Family-wise proportions (Figure 2A) depict that the highest %CAZyme CDSs are present in the family Archangiaceae with a median value of 4.44% and lowest in the family Nannocystaceae with 2.16%. However, more representation is needed from the marine organisms in future analyses.
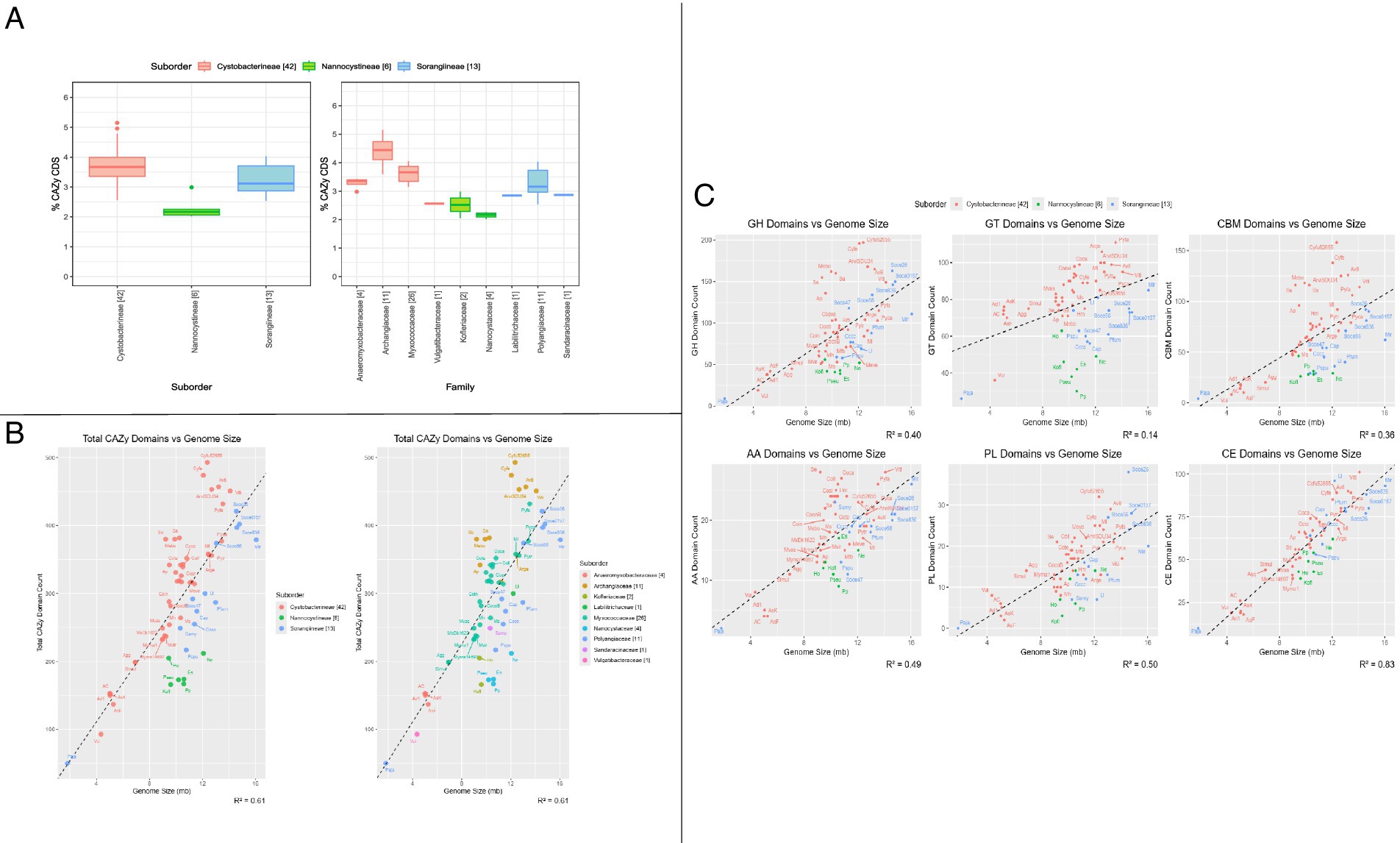
Figure 2. CAZymes distribution and correlation with genome size. (A) Box whisker plots depict %CDS containing CAZyme domains per organism, segregated at suborder level and family level. At the family level, the first four families belong to the suborder Cystobacterineae, the next two families belong to the suborder Nannocystineae, and the last three belong to the suborder Sorangiineae. (B) Organism-wise correlation analysis between genome size and CAZyme distribution counts (the sum of counts of all 6 CAZyme categories—GH, GT, AA, PL, CE, CBM)—showing (a) suborder & (b) family-wise classification. (C) Organism-wise correlation analysis between genome size and domain count of each CAZyme category (AA, CBM,CE, GH, GT, PL) showing suborder-wise classification. In all panels, the numbers mentioned in brackets in the legend and the x-axis depict the number of organisms in that suborder/family. The abbreviations correspond to the short tags we assigned to each organism. The full organism name corresponding to each tag is given in Supplementary Table S1.
The overall genomic potential for carbohydrate metabolism can be estimated by counting the number of translated CDSs having at least one CAZyme domain from any of the six categories (AA, CBM, CE GH, GT, PL) and we found that the highest number of CAZyme domain containing CDSs is 493/9943 (Supplementary Table S1) in the Archangiaceae member Cystobacter fuscus DSM 52655 which thrives in soil and herbivorous animals’ dung (Reichenbach, 2005). We further observed that the five topmost organisms with the highest CAZyme potential have been identified within the family Archangiaceae itself, whereas the lowest number of CAZymes is seen in the Polyangiaceae member, P. abortibovis, which is a pathogenic parasite with 1.8 Mb genome size probably having a limited requirement for carbohydrate degradation, and still, it has 2.94% CAZyme representation at the gene level. The highest and lowest proportions of CAZymes are present in Stigmatella erecta DSM 16858 at 5.14% and Plesiocystis pacifica SIR-1 with 2.01% CAZyme CDSs in its genome (Figure 2A). Based on this, we hypothesize that CAZyme density is not dependent on the number of CDSs the organism has. To further confirm this, we checked the correlation between genome size and CAZyme proteins (Figure 2B), which revealed that most of the family Archangiaceae members encode more CAZymes as compared to other families. Although we see this potential in Archangiaceae through our comparative genomics approach, experimental validation is required to confirm the expression of these CDSs. Overall, owing to several positive and negative outliers, we found a suboptimal/slightly positive correlation between genome size and CAZyme counts (R2 ~ 0.61). Several organisms deviate from the trendline, and this highlights that despite their relatively smaller genome size, their immense CAZyme potential is an aspect that must be explored and utilized further.
This analysis provided an organism-wise distribution for all 61 organisms for all CAZyme categories, their families, and subfamilies (Supplementary Table S2). We found that the most abundant CAZyme category is GH followed by GT, CE, CBMs, AA, and PL with median values of 84, 77, 63, 62, 19, 14 and sample standard deviation 44.73, 18.52, 20.58, 34.76, 6.45, 7.64, respectively. Archangiaceae members have approximately two times more GHs (160) encoded in their genomes as compared to Polyangiaceae (111) and Myxococcaceae (83), at the median scale. Irrespective of having the largest genome size, Minicystis rosea, and other Sorangium spp. organisms have almost 30–45% lesser number of GHs, suggesting that Archangiaceae members have the largest genomic potential in terms of carbohydrate degradation as supported by their glycosyl hydrolases encoded proteins. To our surprise, we do not see any major difference in other categories such as GT, PL, CE, and AAs at the median level suggesting their less variation across the dataset. However, CBM numbers showed a large variation of up to two times in Archangiaceae (116) as compared to Polyangiaceae (54) and Myxococcaceae (63), at the median scale, which is almost in correlation with GH numbers. Correlation studies between each CAZyme category with the genome size suggested no correlation for GTs, low correlation for GHs and CBMs (R2 value ~0.38), moderate correlation (R2 value ~0.50) for AA and PL, and good correlation for CEs (R2 value 0.83; Figure 2C).
In the process of seeing an overall picture of the distribution of the major CAZyme families and subfamilies across all taxonomic families, we created a ball and dot map of all categories which have an average total of >10 proteins at all taxonomic families (Figure 3). As expected from our above findings, the Archangiaceae family has a greater relative abundance in nearly all major GH families, which are the key domains in polysaccharide degradation. The most abundant subfamilies are CE1 with acetyl xylan esterase activity (Dilokpimol et al., 2022), and other major families include GT4, GT2, GH13, CBM50, and GH23 which are most abundant in either Archangiaceae, Myxococcaceae, or Labilitrichaceae. Among these, relevant to our study are GH13 which has dual roles with α-amylase starch degrading activity as well as glucan-1,6-α-d-glucosidase dextran degrading activity, GH23 which has endochitinase activity, and CE4 involved in acetyl xylan esterase activity.
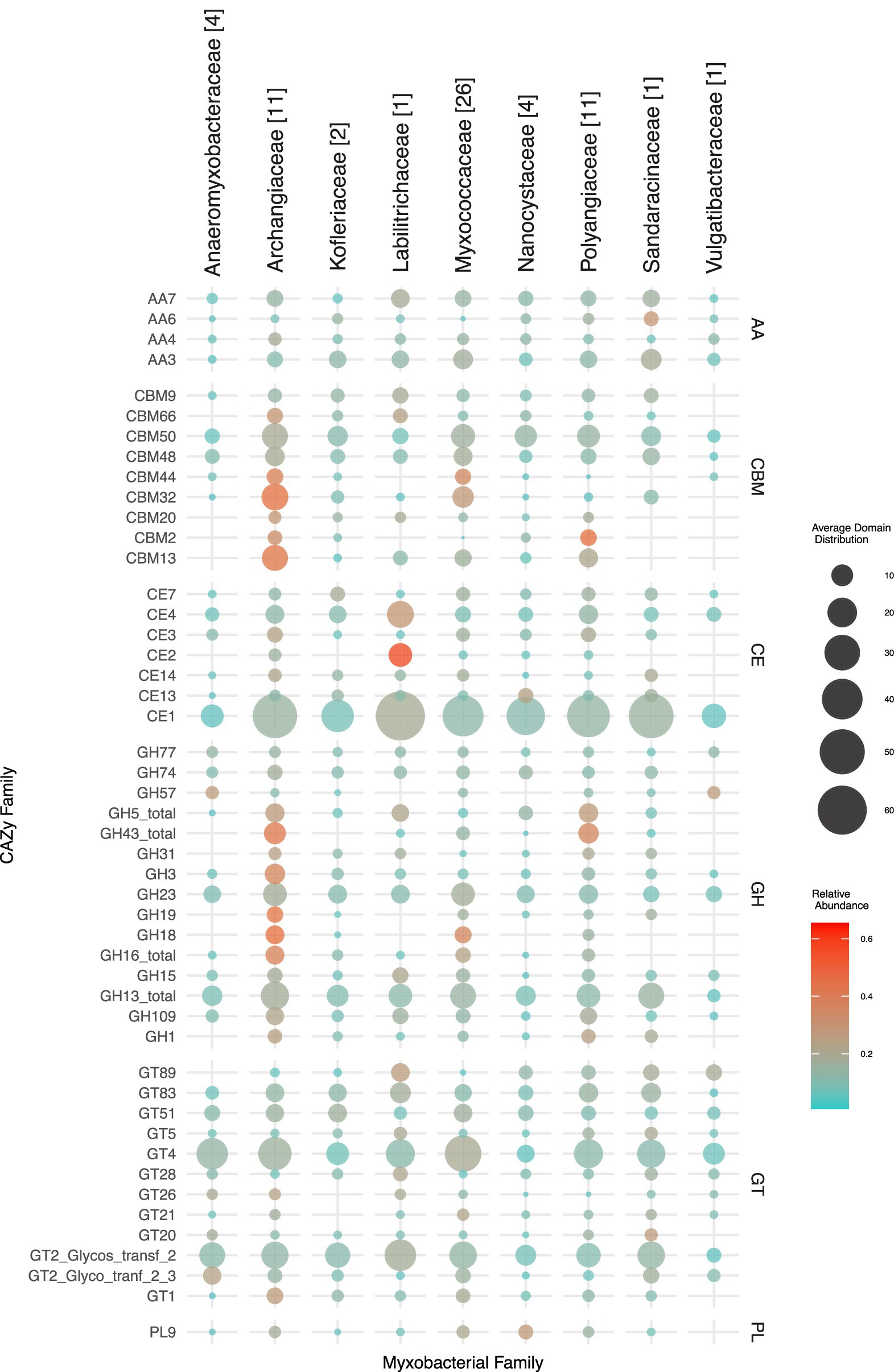
Figure 3. Taxonomy family-wise representation of predominant CAZyme families in all six CAZyme categories. The mean family-wise values are depicted which are proportional to the diameter of the circles according to the scale shown on the right-bottom. The color of the circles represents the family-wise relative abundance which gives an idea about how much abundance a myxobacterial family has of a particular CAZyme family compared to the rest of the myxobacterial families. Thus, the color range should be analyzed row-wise; blue represents low relative abundance and red represents high relative abundance. The numbers in brackets next to family names (on the top) represent the number of organisms in that family.
We further wanted to analyze if CAZyme density could be used as a factor to differentiate the taxonomic clusters at the suborder and family level and for that we further utilized the distribution counts of the six CAZyme categories to perform principal component analysis (Supplementary Figure 1). The two axes, PC1 and PC2, showed 72.72 and 10.86% variability, respectively. We observed that at both levels, some clusters were overlapping, justified to some extent by exceptional cases like P. abortibovis which have extremely low CAZyme counts, and some members of the family Archangiaceae which have relatively high CAZyme counts in most categories.
CAZymes could play an important role in the myxobacterial lifestyle
After having looked at the CAZyme domain distribution broadly, we emphasized on their functionality based on their actions against major carbohydrate substrates—cellulose, xylan, lignin, pectin, chitin, starch, dextran, and fructan (Supplementary Tables S3, S4). Being majorly soil bacteria, we anticipate that myxobacteria do have a role to play in the degradation of lignocellulosic biomass. Lignocellulosic biomass presents a complex and intricate matrix that is not readily accessible for enzymatic degradation. However, glycoside hydrolases (GHs) and related enzymes exhibit the capability to break down these structures (Ezeilo et al., 2017), a process that holds significant implications for biofuel production. Consequently, such investigations contribute to the expanding knowledge of these potentially economically valuable enzymes and their natural sources.
Using the enzyme-specific EC numbers (curated from literature) as a query in the CAZyme database, we first curated a list of CAZyme families that are specific to the enzymatic degradation of each of our chosen substrates (Supplementary Table S3), followed by their distribution across all myxobacteria (Figure 4). Many CAZyme families act on a single carbohydrate substrate but some are bifunctional and involved in the degradation of more than one substrate (the last few domains on the x-axis). For example, GH13 is involved in the degradation of two substrates- starch and dextran. This bifunctionality may be possible because amylopectin (a starch component) and dextran are both α-1,6 linked (Hondoh et al., 2008; Yang et al., 2022) chains and thus they both can get catabolized by the same enzymatic action, i.e., α-glycosidases (Vera-Ponce de León et al., 2020). Overall, for all enzymes again, the higher abundance is seen in Archangiaceae, Myxococcaceae, and Polyangiaceae members, which needs to be validated experimentally.
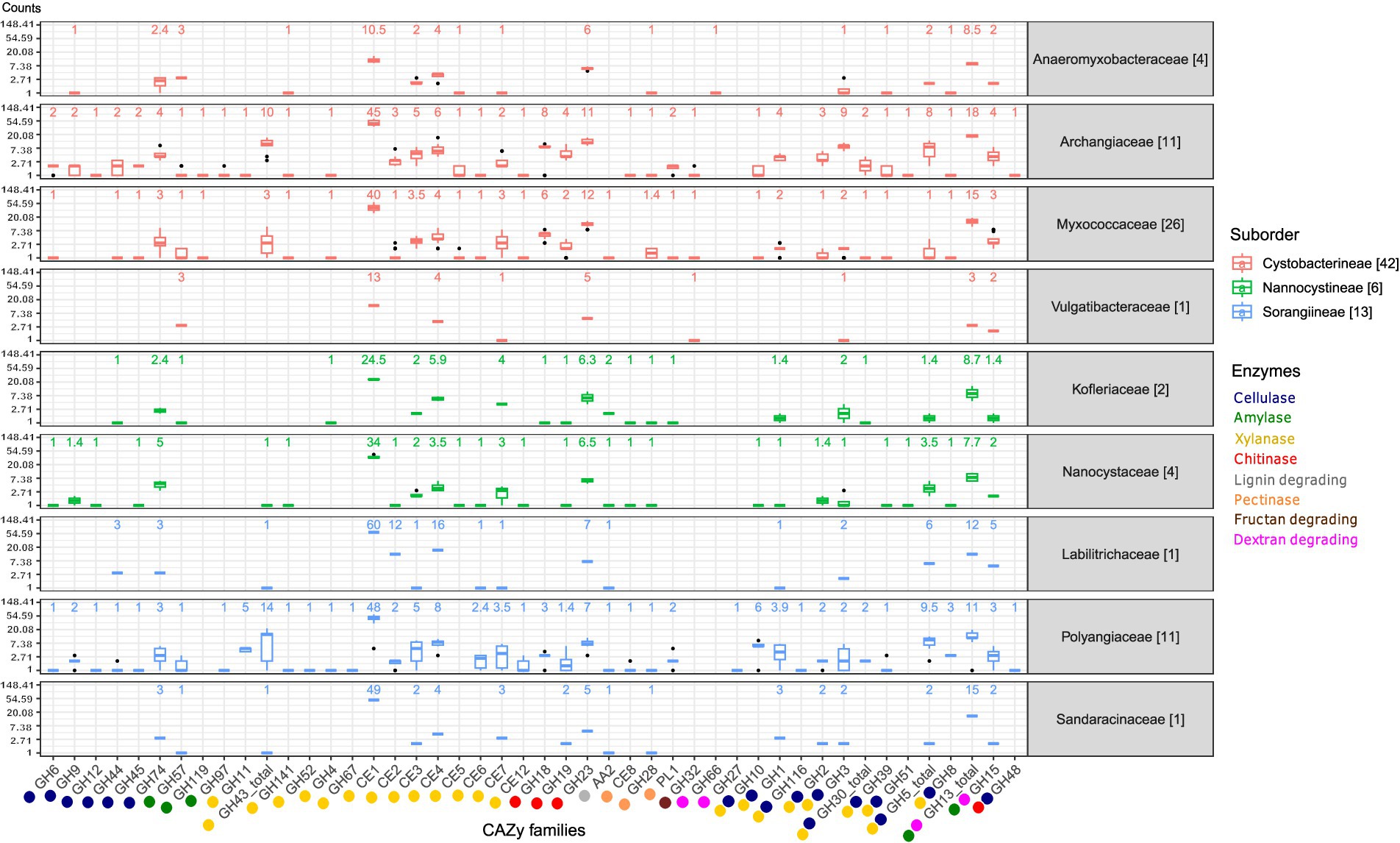
Figure 4. Box whisker plots showing the median count of lignocellulose enzyme-specific CAZyme families in the myxobacterial families on a logarithmic scale.The CAZyme families are labeled on the x-axis and their corresponding enzymatic function is depicted by the colors below, explained on the lower right side. CAZyme families which are involved in two enzyme activities are represented with both their respective enzyme colors. In the top region of each myxobacterial family’s panel, the mentioned numbers are the median values of that box plot. The box plots are colored according to the suborder. The numbers mentioned in brackets in the legend and beside the family names depict the number of organisms in that family/suborder.
Myxobacteria are potent lignocellulosic biomass degraders
Cellulose degradation
The prime component of lignocellulosic biomass, cellulose is cleaved by different types of cellulases (See Supplementary Table S3 for enzyme sub-class wise domains and distribution). Seventeen GH families are involved in this catabolic activity, of which six (GH6, GH9, GH12, GH44, GH45, GH74) are unique to cellulase activity in our study, while the others are bifunctional (Figure 4). For 90% of these 11 bifunctional GH domains, the other substrate recognized is xylan while the one remaining, GH48 acts on chitin. The distribution of cellulase domains is highest in the suborder Cystobacterineae owing to the presence of large numbers in a few organisms; however, the median is higher for the suborder Sorangiineae (Figure 5A). The family Archangiaceae harbors 36 cellulase proteins at the median level and Polyangiaceae with 24 cellulase proteins at the median level (Figure 5B) suggesting these organisms have the most cellulose degrading genomic potential (Figures 4, 5B).
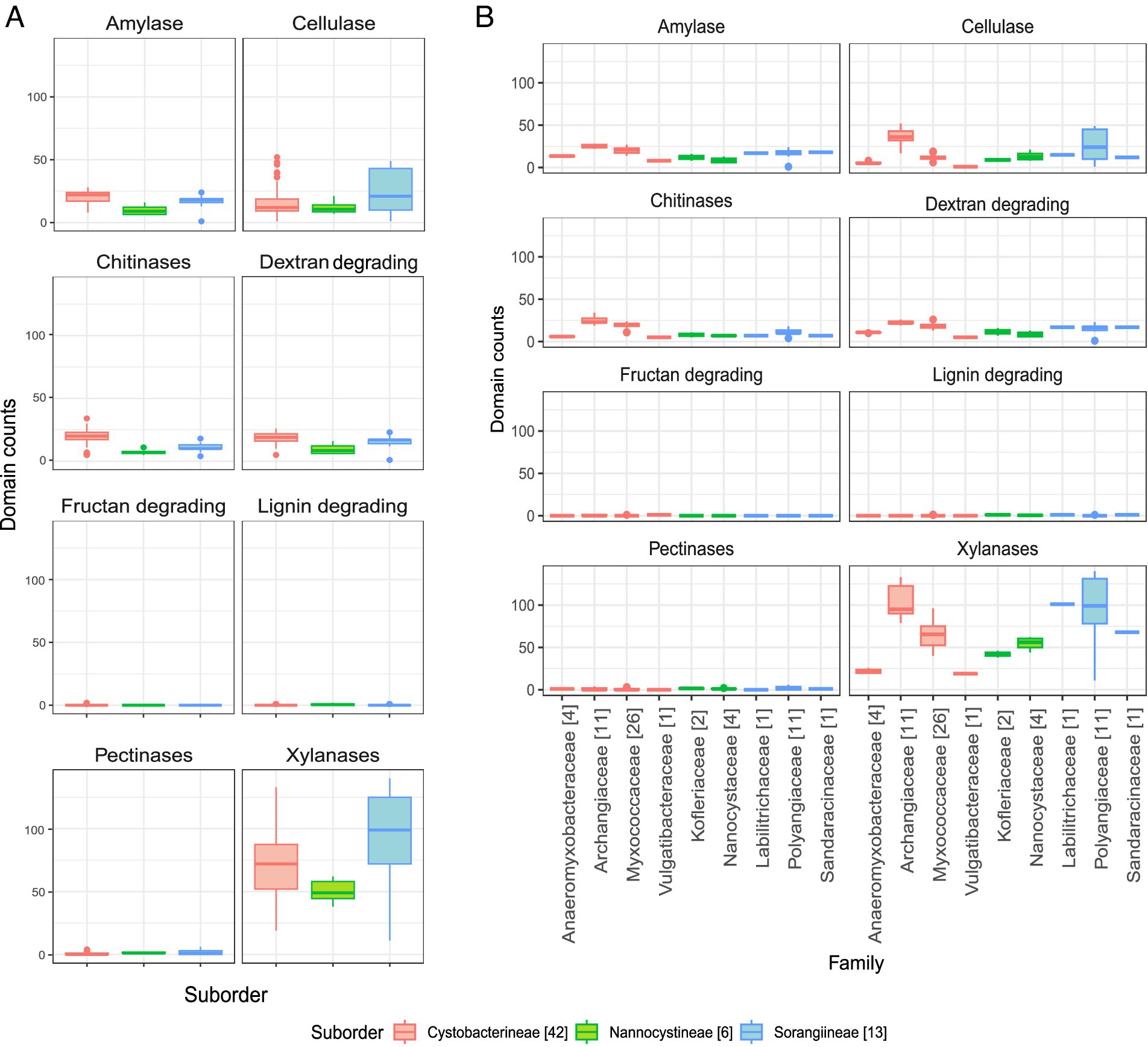
Figure 5. Distribution of specific lignocellulose enzymes. (A) Enzyme-wise box whisker plots representing the suborder level distribution of the respective CAZyme domains. (B) Enzyme-wise box whisker plots showing the family-level distribution of the respective CAZyme domains. The numbers mentioned in brackets in the legend depict the number of organisms in that family. The y-axis scales in all panels are kept the same for comparative visualization.
In Polyangiaceae members, cellulose degradation has already been well-reported (Schneiker et al., 2007), both computationally and experimentally. However, within the family Myxococcaceae, cellulases have been reported in a few studies such as encoded by the cel9-cel48 gene cluster in Myxobacter sp. AL-1 (Ramírez-Ramírez et al., 2008). The Polyangiaceae central tendencies in our study, however, are on the lower side due to the exceptional case of the pathogen P. abortibovis; the median shoots up to 30 proteins when calculated excluding this pathogen. The members of both these families mostly inhabit the soil ecosystem, in decaying plant material, dung of herbivores, and wood/bark (Reichenbach, 2005) all of which are rich sources of cellulose. Thus, their CAZyme potential reflects their habitat and role in the ecosystem.
The Archangiaceae member C. ferrugineus Cbfe23 exhibits the highest number of cellulase-associated domain-containing proteins with a total of 52 (Supplementary Table S4). Following closely are S. cellulosum So ce836 and S. cellulosum So ce26, each containing 49 cellulase domain-containing proteins (Supplementary Table S4). Vulgatibacter species from the family Vulgatibacteraceae and Haliangium ochraceum SMP-2T (different strain - Haliangium ochraceum DSM 14365 used in our study) from Kofleriaceae are known to be non-cellulose degrading (Yamamoto et al., 2014). This non-degradative characteristic is evident from their lower domain distribution in our dataset as well (Figures 4, 5B).
The cellulase GH domains can occur alone, in duplicates, or paired with other CAZyme domains (Figure 6), which possibly means that they can perform their action independently and in association. GH6, GH9, GH48, and GH51 have both endocellulase and exocellulase (or cellobiohydrolase activity; Supplementary Table S3). Notably, GH6 is predominantly associated with CBM4, and occasionally with CBM3 and CBM37. Among these associated domains, CBM4 is implicated in the degradation of amorphous cellulose and xylan but not crystalline cellulose; CBM3 targets cellulose and, less frequently, chitin; while CBM37 has a broad polysaccharide binding potential, as documented in the CAZyme database. GH9 domains are majorly associated with CBM30, CBM4, and CMB3 which are well known to have cellulose, xylan, or chitin-binding function. It is interesting to note that the endocellulase domain GH74 domain is typically present alone in most identified proteins; however, when it co-occurs with other domains, it is linked with GH33, which has trans-sialidase activity. Additionally, GH10, GH1, GH116, GH2, GH3, GH30 (and its sub-families), GH39, GH51, GH5 (and its sub-families), and GH8 are commonly involved in the degradation of both cellulose and xylan.
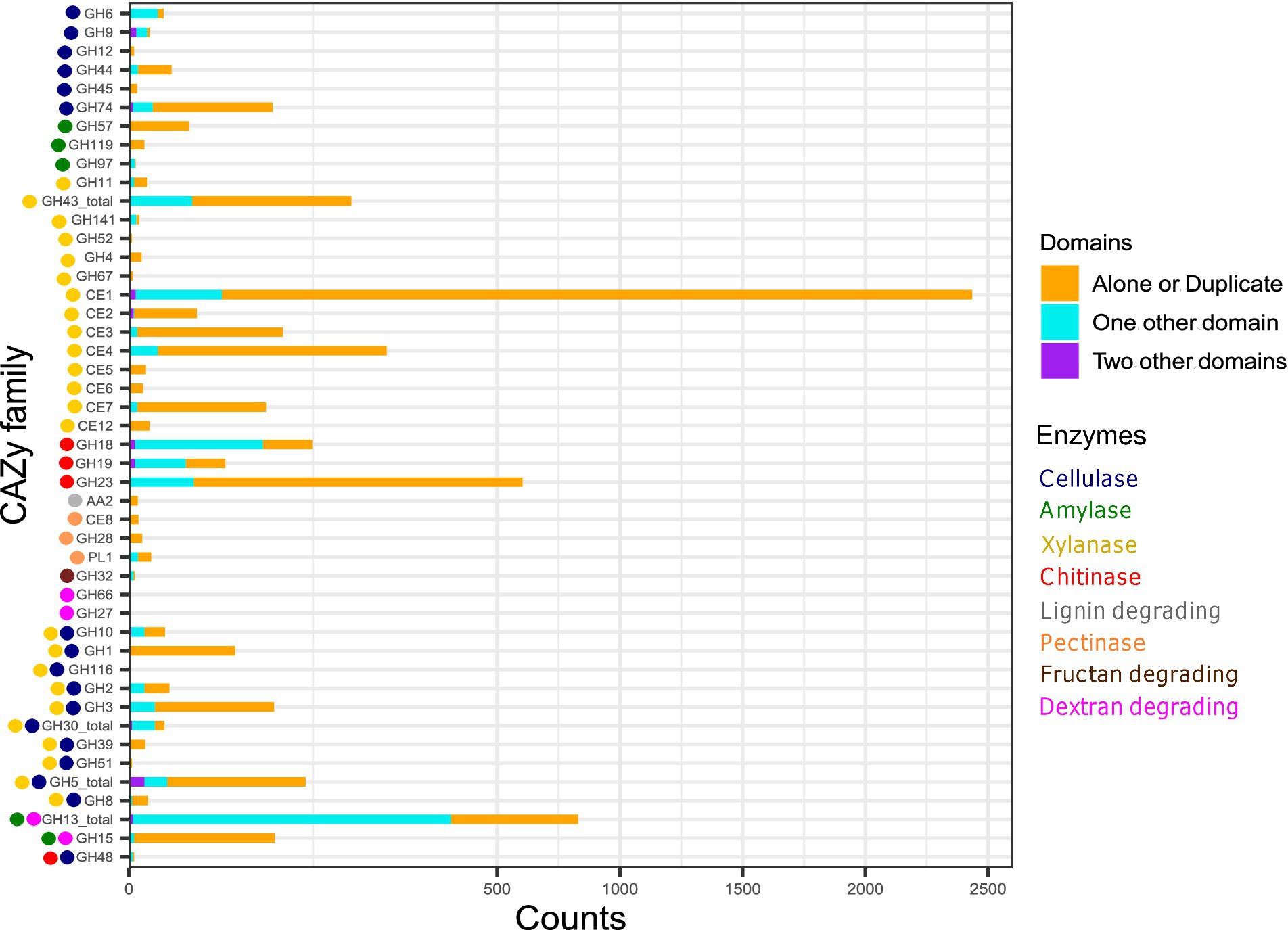
Figure 6. The stacked bar plot representing the relative frequency of CAZyme domains in diverse combinatorial patterns. The CAZyme domains either occurring alone (or in multiple copies) or with one or multiple accessory domain indicate their independent/ dependent mode of action with one or two functions. The X-axis scale is expanded within 0–500 counts.
We further observed that some cellulase CAZyme families were specifically present in a few organisms or families or suborders. GH10 (former cellulase family F), GH12 (former cellulase family H), GH8 (former cellulase family D), and GH9 (former cellulase family E) were predominantly present in genus all under study Sorangium spp. and a few members of family Archangiaceae (Supplementary Table S3). More importantly, these members were almost absent in the most abundant family Myxococcaceae except for sporadic distribution in a few organisms. Among the cellobiases (beta-glucosidases) function, we found a unique family, GH116, which is only present in Sorangium cellulosum So ce56 (locus_tag = SCE_RS22635); even the taxonomic distribution in CAZyme database shows its exceptionally low occurrence in Proteobacteria phylum. Blastp analysis reveals its closest homologs in Acetivibrio cellulolyticus, a phylum Bacillota member known for its efficient crystalline cellulose degradation capabilities. Overall, this further suggests the novel origin and functional importance of GH116 protein in Sorangium cellulosum So ce56. GH48 (former cellulase family L) and GH51 are also interesting candidates to explore further as they are only present in several family Archangiaceae members and almost absent in other myxobacteria. Overall, cellulase protein distribution indicates the huge cellulose degradation genomic potential within Sorangium spp. and less-explored family Archangiaceae members.
Lignin degradation
To enhance the fermentation rate of cellulose and hemicellulose, lignin must first be removed which can be performed by bacteria, fungi, bacteria-fungal co-culturing, or enzymatic pre-treatment (Li X. et al., 2022). Our analysis indicates that auxiliary activities (AA) families AA1 and AA2 are implicated in various ligninolytic processes. Specifically, AA1, which exhibits laccase activity, is absent in all organisms examined in this study. In contrast, AA2, which is associated with lignin peroxidase, manganese peroxidase, and versatile peroxidase activities, appears infrequently and cannot be correlated with their taxonomy. Overall, lignin-degradative domains are not prominently represented in our dataset (Figures 4, 5) and in every case we saw, AA2 domain does not co-occur with any other CAZyme domain (Figure 6) which shows its independent functions here- but this would be better understood in other organisms which have more representation of AA2 domain and its architecture combinations.
Xylan degradation
Plant hemicellulose predominantly comprises xylan, a polysaccharide structurally similar to cellulose but composed of D-xylose monomers (Bajpai, 2009). Cellulose hydrolysis is facilitated by hemicellulose-degrading enzymes, a critical factor for enhancing lignocellulosic biomass degradation in industrial applications (Busk et al., 2014). xynA, a xylanase-encoding gene has been thoroughly studied in Sorangium cellulosum So9733-1 (Wang et al., 2011). Xylan degradation is mediated by xylanases, the most diverse class of enzymes in our dataset, spanning 24 different CAZyme families. Consequently, there are more xylanase domain-containing proteins per organism compared to those targeting other polysaccharide substrates. Nearly 60% of these CAZyme families are specific to xylan deconstruction, with carbohydrate esterases (CEs) being highly represented; no other polysaccharide-degrading enzyme category in our dataset encompasses as many as eight CE families (Supplementary Table S3).
The function of feruloyl esterases and acetyl xylan esterases is primarily mediated by CE domains. Notably, family CE1, involved in both enzymatic actions, is the most prevalent domain (Figure 4), ranging from five proteins in the Sorangiineae pathogenic member P. abortibovis to 64 proteins in the Archangiaceae member Vitiosangium sp. GDMCC 1.1324. At the suborder and family levels, significant xylan-degrading potential is observed in Sorangiineae (Figure 5A), particularly within the Polyangiaceae family and Archangiaceae members (Figures 4, 5B). S. cellulosum So0157-2 exhibits the highest xylanase distribution with 140 proteins, followed by S. cellulosum So ce836 and S. cellulosum So ce26 with 138 and 137 proteins, respectively.
Most specific xylan-degrading domains occur independently (Figure 6), though GH43 and its subfamilies, as well as CE1, can possess accessory domains. GH43 subfamilies co-occur with several carbohydrate-binding modules (CBMs): CBM6 which interacts with amorphous cellulose, β-1,4-xylan, and glucan; CBM66, which binds with terminal fructoside residue of fructans; and CBM13, which binds mannose in plants and xylan in bacteria. CE1 domain is occasionally accompanied by a few other CAZyme modules such as CBM20, PL22, CE7, and CBM32.
One of the β-xylosidases, GH52 CAZyme family, is uniquely distributed only within four out of five Sorangium spp. members. These Sorangium proteins show maximum homology with Agarivorans spp. members from Gammaproteobacteria with 98% query coverage and ~ 50% identity. Similarly, the GH67 domain functioning as α-glucuronidase is also present only in Sorangium spp. with closest relatives in Gemmatimonadaceae bacterium having >80% query coverage and > 60% identity.
Myxobacteria encode a diverse set of enzymes to degrade other important polysaccharide substrates
Starch degradation
At the suborder level, Cystobacterineae members have the highest median values for amylase domain-containing proteins (Figure 5A). Previously, α-amylases, AmyM (Li et al., 2015), and CoMA (Zhou et al., 2018) have been characterized in the Cystobacterineae member Corallococcus sp. EGB. Another multifunctional amylase, AmyC, has been characterized within the same species (Wu et al., 2015). Looking at the family level, Archangiaceae members have the highest median value of 25 proteins (Figures 4, 5B). The Archangiaceae members – C. fuscus DSM 52655, A. violaceum SDU34, and A. violaceum SDU8 have equal numbers and the highest counts with a value of 28 proteins (Supplementary Table S4). Starch-degrading domains are also found in Myxococcaceae, with a median count value of 21 proteins. The order Sorangiineae and family Sandaracinaceae member, S. amylolyticus DSM 53668, is a species known to degrade starch (Sharma et al., 2016) and it has 18 proteins with starch-degrading domains. Starch degradative domains include α-amylases associated GH13, GH57, GH119, β-amylases functioning GH14, γ-amylases associated GH15, GH97. GH13 and its subfamilies as well as GH15 are bifunctional domains with both dextran and starch degrading functions (Figures 4, 6). GH13 subfamiles GH13_11 and GH13_10, were found to be associated with CBM48 in 143 and 102 proteins, respectively which makes it the most frequent accessory domain for GH13 in our dataset. Other accessory domains include CBM20, CBM32, starch binding CBM25 the α-glucans amylose, amylopectin, pullulan binding CBM41, starch binding CBM69 which is also distantly related to CBM20 and CBM48.
GH119 domain is involved in α-amylase function, and it is specifically present in all family Archangiaceae members and only one genus in the family Myxococcaceae, i.e., Corallococcus members. GH119 is closely related to the previously well-known α-amylase family, GH57. These myxobacterial GH119 homologs have close homologs in Cohnella and Paenibacillus spp. of phylum Bacillota (Firmicutes) as indicated by >95% query coverage and > 60% identity suggesting their putative evolutionary relationship with the latter.
Chitin degradation
Endochitinase activities are predominantly shown by GH18, GH19, GH23, and GH48 CAZyme families. A recent study has reported a GH19 enzyme, C25GH19A, which is chitin-induced in Corallococcus silvisoli c25j2 (Zhou et al., 2024). An anti-fungal GH18 chitinase, CcCti1, was also identified and further studied in Corallococcus sp. EGB (Li et al., 2019). Based on the distribution of these families, the suborder Cystobacterineae members demonstrate encoding potential for chitin degradation (Figure 5A). Within this suborder, members of the family Archangiaceae exhibit the highest median number of chitinase domain-containing proteins, with a median value of 23 (Figures 4, 5B). Vitiosangium sp. GDMCC 1.1324 possesses the highest number of chitin-degrading domains, totalling 34 (Supplementary Table S4). Among Archangiaceae, previous studies have shown that members of the genus Cystobacter can degrade chitin but Archangium gephyra cannot (Sharma and Subramanian, 2017; Lang et al., 2015). Following the previous study, our study shows that A. gephyra DSM 2261 has a lower count of chitinase-associated domain-containing proteins as compared to a higher count (19 proteins) in the Cystobacter members C. fuscus DSM 52655 and C. ferrugineus Cbfe23 (29 and 30 proteins respectively). Among Myxococcaceae, chitin-degrading enzyme domains have been previously reported in Corallococcus species and Myxococcus species (Shahbaz and Yu, 2020; Li et al., 2019). With a median value of 20 proteins, Myxococcaceae members stand second highest after Archangiaceae. Chitinase domains GH18, GH19, and GH23 showcase endochitinase activity among which the first two are always majorly associated with other domains whereas the last one majorly works independently (Figure 6). GH18 plays a role in chitobiosidase activity as well and in our data, it is majorly associated with several CBMs, such as CBM12, CBM13, CBM2, CBM44, CBM5, CBM8, CBM13, CBM61, and CBM 37. Similarly, GH19 is present with CBM12, CBM13, CBM2, and CBM50. However, GH23 is mostly independent (515 proteins) but when associated with an accessory domain, it is one or two or three replicates of CBM50 itself. This suggests that CBM50 has some additional evolutionary relatedness with GH23. GH48 acts both on cellulose as well as chitin (endochitinase activity). Our study revealed that this domain is only present in a few members of the family Archangiaceae along with two members of the family Polyangiaceae, i.e., Minicystis rosea DSM 24000, Polyangium fumosum DSM 14668.
Dextran degradation
Suborder Cystobacterineae has the highest genomic potential for dextran degradation as well (Figure 5A). Here again the most potent are the members from the underlying Archangiaceae family (Figures 4, 5B) with a median of 22 proteins having dextran degrading domains. A violaceum SDU8 has 26 proteins with dextranase domains which is the maximum value in this dataset. GH66 and GH49 play a role in dextranase activity, GH13 with its subfamilies and GH15 are involved in glucan-1,6-α-d-glucosidases functioning and GH27 in glucan-1,6-α-isomaltosidases. GH49 also has a role to play in dextran 1,6-α-isomaltotriosidase activity but GH49 is not identified in any of the genomes in this study.
The GH66 domain is only present in Anaeromyxobacter sp. Fw109-5, which depicts the closest homologs in Thermoanaerobacter spp. from phylum Bacillota (Firmicutes) with >95% query coverage and ~ 45% identity. Both of these are facultative Fe (III)-reducing bacteria, and such homology possibly suggests the shared evolutionary relationship between these two homologs. Similarly, the GH27 domain which is involved in glucan-1,6-α-isomaltosidases function occurs only in Sorangium cellulosum So ce836 and not in any other genome. Its closest homologs are present in the Verrucomicrobiia bacterium from phylum Verrucomicrobiota, with >70% query coverage, >40% identity, and e-values of <1e−135, showcasing good sequence homologs.
Fructan degradation
Fructan degradation is catalyzed by fructan exohydrolases which function with the help of the GH32 domain. GH32-containing proteins are not too abundant in this myxobacterial dataset (Figures 4, 5). This domain is only present in six proteins (Figure 6), out of which it occurs independently in two proteins and the other four, with two copies of domain CBM66 which is well-known for fructan binding.
Pectin degradation
CE8, GH28, and PL1 are the domain families substantial for pectinase activity CE8 having pectin methylesterase activity, GH28 for endo-polygalacturonases, and PL1 having pectin lyase activity are the domain families substantial for pectinase activity. We could identify only a few pectinases in our dataset (Figures 4, 5), where the highest number is seen in S. cellulosum So ce26 with 6 domains. Pectin-degrading pathways have also been earlier reported in S. cellulosum (Schneiker et al., 2007). Overall, CE8 was found in only 3 proteins which are encoded by Archangium violaceum genomes (Figure 6) and our analysis reveals that all 3 of those proteins have varying architecture, one having CE8 in triplicates, one with the domain PL9_4, and the other with the pectinase specific PL1 itself. GH28 is found in 18 proteins overall, while mostly present alone, this domain could also be found associated with one accessory domain which is PL9_2, or two accessory domains PL9_2 and PL9_4 (Figure 6). The accessory PL9_4 plays a role in the peptidoglycan lyase activity, while no specific activity has been characterized for PL9_2 as per the CAZyme database. PL1 mostly occurs alone, or it can be with other accessory domains such as PL9, mannose/xylan binding CBM13, the rhamnose binding CBM67, and CBM37.
Overall, our data indicates that the Archangiaceae, Myxococcaceae, and Polyangiaceae families have significant carbohydrate-degrading genomic potential, warranting further exploration, experimental validation, and utilization. Conversely, the pathogenic Pajaroellobacter demonstrates the lowest distribution of CAZymes across all classes, which is consistent with expectations for a pathogenic organism that has reduced genome size. For all enzyme categories, the lower values of Nannocystineae could be attributed to their marine habitat (Ivanova et al., 2010; Figure 7) and thereby reduced carbohydrate degradation but more representation and experimental validation are required in future studies. This is supported by earlier reports during the initial characterization of Nannocystis exedens, which noted the absence of starch and cellulose degradation (Reichenbach, 1970). Collectively, these findings from the current dataset suggest that CAZyme potential is higher in soil-dwelling myxobacterial species compared to their marine counterparts, though further studies are needed to confirm this trend (Figure 7).
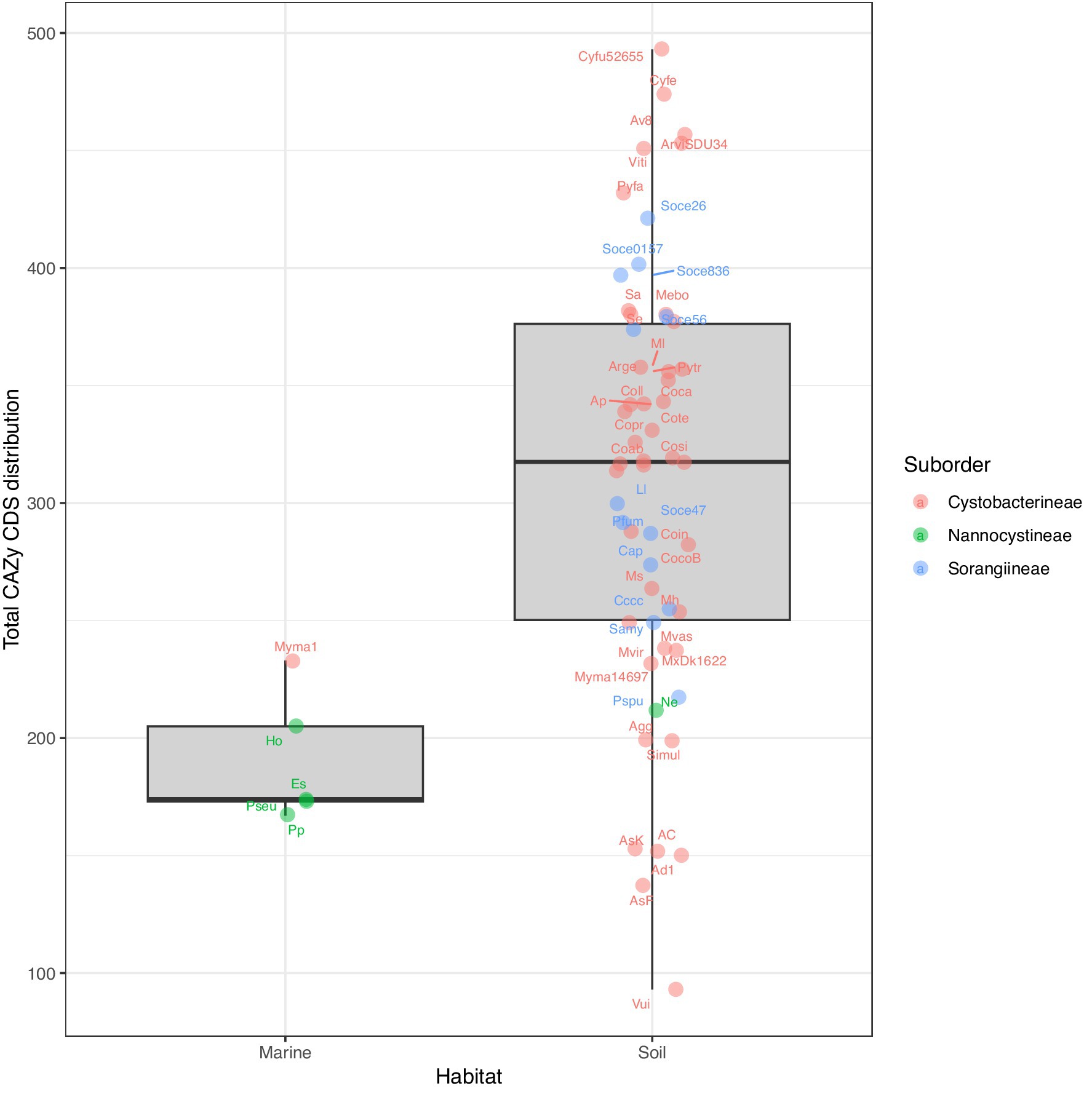
Figure 7. Boxplots showing the organism-wise preferred habitat and respective CAZyme domain distribution of the 61 myxobacteria in our dataset, categorized by suborder. The abbreviations correspond to the short tags, assigned to each organism. The full organism name corresponding to each tag has been provided in the Supplementary Table S1.
Discussion
Myxobacteria, ubiquitous across various habitats, exhibit their highest diversity in steppe and warm climatic zones. Notably, certain species within the genera Myxococcus, Corallococcus, Archangium, and Nannocystis are also found in harsher environments such as deserts and high-altitude regions. These topsoil bacteria are frequently isolated from the dung of herbivorous animals and decaying plant material, which serve as well-known predominant sources of complex polysaccharides (Reichenbach, 1999). The polysaccharide catabolism potential of organisms using the carbohydrate-active enzymes (CAZyme) database mapped on their encoded genes can underscore their value for industrial applications. It has been previously shown that Gemmatimonadetes, Actinobacteria, and Proteobacteria members are crucial for nutrient cycling because of their high CAZyme potential (Zhou et al., 2023). Streptomyces, Micromonospora, a few Mycobacterium and Propionibacterium strains have enriched carbohydrate-active enzyme (CAZyme) domains, which have been found to help in lignocellulose biomass degradation (Lewin et al., 2016). The role of Streptomyces species in nutrient cycling has been elucidated in several studies (Book et al., 2016; Sidar et al., 2024; Takasuka et al., 2013; Book et al., 2014). Insect associated Streptomyces species with genome sizes in the range of 6.9–7.4 Mb encode 130–185 CAZyme proteins with percentage CAZyme proteins in the range of 2.3–2.9% (Book et al., 2014), which helps them in sustaining their relationship with insects. A metagenomic analysis of an anaerobic digestion microbiome exposed to acidosis revealed that Bacteroidetes MAGs constitute 8.8% ± 1.8% of CAZyme genes whereas Firmicutes MAGs with 3.6% ± 1.1%, Spirochaetes MAGs with 2.9% ± 1.2% (Bertucci et al., 2019) and a smaller percentage of myxobacterial MAGS was also present in such ecosystem.
In this study, we revealed the genomic potential of polysaccharide catabolism in a diverse representation of myxobacterial species by diving deep into analyzing the CAZyme domains involved in degrading the major substrates cellulose, starch, xylan, fructan, lignin, pectin, dextran, and chitin. Members of Sorangiineae, especially Sorangium cellulosum have been well-known as cellulose degraders as the name suggests, however, the genomic background of these activities was not much studied. Our research fills this gap and reveals that many other myxobacterial species possess considerable, yet underexplored, capacities for polysaccharide deconstruction. The degradation of polysaccharides, especially lignocellulosic biomass, holds immense potential for biofuel production. Previous research has shown that overexpression of certain plant-associated domains, like glycosyltransferases (GTs), can enhance biomass formation (Yang et al., 2017). This concept could be explored and applied to harness the converse catabolic activities of myxobacteria for increasing efficiency.
Our findings indicate substantial variability in genomic CAZyme potential among these large-genome harboring myxobacterial families and organisms, with some species exhibiting high potential for substrate-specific degradative capabilities despite having relatively small genomes. Notably, members of the soil-dwelling Archangiaceae family demonstrated a remarkable richness in CAZymes. It is important to recognize and mention that deriving biological conclusions from high-throughput studies such as ours, which involve multi-organism and multi-level comparisons, heavily relies on the quality of publicly available genome assemblies. Most of the assemblies used in our study have complete genomes with high-quality genome annotations, however, a few of them are incomplete, multi-contig assemblies that may not capture the total encoding potential. The inclusion of these draft assemblies was to avail the maximum taxonomic and genomic diversity available at the time of data curation. Despite these limitations, our deep analysis has unveiled new avenues of knowledge.
While we performed this analysis at three levels- suborder, family, and organism, there may be cases where a bacterium/its family may have immense genomic potential but is not expressing the same when it comes to its expression in its life cycle. Genomic studies alone cannot address these limitations, necessitating extensive experimental validation. We urge experimental researchers to verify whether high counts of substrate-degrading CAZyme domains correlate with increased degradation efficiency. Nonetheless, the carbohydrate degradation potential highlighted in our study is substantial and provides a basis for designing experiments to harness this capability for practical applications. The dataset used in the study is taxonomically broad but still limited to certain well-studied myxobacterial groups. This could introduce phylogenetic bias, where the observed patterns in CAZyme distribution might not be generalizable to other, less-studied phylogenetically close groups. We acknowledge this limitation and suggest the inclusion of more taxa in future studies to provide a more complete picture.
In conclusion, we present a comprehensive high-throughput genomic analysis of the Carbohydrate Active enZymes-encoding potential of 61 myxobacteria members (order Myxococcales or currently known phylum Myxococcota), examining their genomic diversity at the levels of suborder, family, and genus, as well as their functionality in terms of singular or multiple enzymatic activities. Comparative analysis among members of order Myxococcales revealed variations in carbohydrate utilization potential and recommended that lignocellulose biomass degradation is not universally conserved and similar within these groups of organisms. Such carbohydrate degradation analyses lay a preliminary foundation for utilizing myxobacterial organisms in diverse paper-pulp, food-beverage, textile, and biofuel industries.
Data availability statement
Publicly available datasets were analyzed in this study. The accession numbers to these datasets are available in the “U” column of Supplementary Table 1.
Author contributions
NS: Investigation, Methodology, Resources, Validation, Writing – original draft, Writing – review & editing, Data curation, Formal analysis, Visualization. GS: Investigation, Methodology, Resources, Validation, Writing – original draft, Writing – review & editing, Conceptualization, Funding acquisition, Project administration, Supervision.
Funding
The author(s) declare that financial support was received for the research and/or publication of this article. NS acknowledges the fellowship by IIT Hyderabad. GS is supported by the IIT Hyderabad seed grant and the DST-INSPIRE Faculty Grant by the Government of India.
Conflict of interest
The authors declare that the research was conducted in the absence of any commercial or financial relationships that could be construed as a potential conflict of interest.
Generative AI statement
The author(s) declare that no Gen AI was used in the creation of this manuscript.
Publisher’s note
All claims expressed in this article are solely those of the authors and do not necessarily represent those of their affiliated organizations, or those of the publisher, the editors and the reviewers. Any product that may be evaluated in this article, or claim that may be made by its manufacturer, is not guaranteed or endorsed by the publisher.
Supplementary material
The Supplementary material for this article can be found online at: https://www.frontiersin.org/articles/10.3389/fmicb.2025.1550287/full#supplementary-material
SUPPLEMENTARY FIGURE 1 | Principal component analysis based on the organism-wise distribution of the six CAZyme Categories—GH, GT, AA, PL, CE, CBM—showing (a) suborder & (b) family-wise clustering. The numbers mentioned in brackets in the legend and X-axis depict the number of organisms in that suborder/family.
SUPPLEMENTARY TABLE S1 | Detailed genome statistics information for all 61 myxobacterial genomes showcases their taxonomy information, abbreviated tags used throughout the study, genome size, GC content, CDS information, CAZy-specific CDS density, RNA information, source file RefSeq links, and individual category and total CAZyme domain distribution counts. Columns represented as heatmaps range from red as the lowest, yellow in the middle, and green as the highest value in that column. Columns (B, C, and D) are color-coded as per their family taxonomy.
SUPPLEMENTARY TABLE S2 | Distribution of identified encoded proteins per organism per CAZyme family. Distribution implies the total number of CDSs having that unique domain per protein.
SUPPLEMENTARY TABLE S3 | Distribution of proteins per organism per CAZyme family per lignocellulose enzyme. Distribution implies the total number of CDSs having that unique domain per protein.
SUPPLEMENTARY TABLE S4 | Organism-wise distribution of each broad lignocellulose enzyme along with organism taxonomy.
SUPPLEMENTARY TABLE S5 | Details of myxobacterial strains under study and their ecological niche from where the respective strain has been isolated.
Abbreviations
CAZy, Carbohydrate Active Enzymes; LCB, lignocellulosic biomass; GH, glycoside hydrolases; GT, glycosyl transferases; PL, polysaccharide lyases; CE, carbohydrate esterases; AA, auxiliary activities; CBM, carbohydrate-binding modules; NCBI, National Center for Biotechnology Information; CDS, Coding Sequence; GC%, Guanine-Cytosine percentage.
Footnotes
References
Albataineh, H., and Stevens, D. C. (2018). Marine Myxobacteria: a few good halophiles. Mar. Drugs 16:209. doi: 10.3390/md16060209
Bajpai, P. (2009). “Xylanases” in Encyclopedia of microbiology (third edition). ed. M. Schaechter (Oxford: Academic Press), 600–612.
Baltar, F., Arístegui, J., Sintes, E., van Aken, H. M., Gasol, J. M., and Herndl, G. J. (2009). Prokaryotic extracellular enzymatic activity in relation to biomass production and respiration in the meso- and bathypelagic waters of the (sub)tropical Atlantic. Environ. Microbiol. 11, 1998–2014. doi: 10.1111/j.1462-2920.2009.01922.x
Berlemont, R., and Martiny, A. C. (2015). Genomic potential for polysaccharide deconstruction in Bacteria. Appl. Environ. Microbiol. 81, 1513–1519. doi: 10.1128/AEM.03718-14
Bertucci, M., Calusinska, M., Goux, X., Rouland-Lefèvre, C., Untereiner, B., Ferrer, P., et al. (2019). Carbohydrate hydrolytic potential and redundancy of an anaerobic digestion microbiome exposed to acidosis, as uncovered by metagenomics. Appl. Environ. Microbiol. 85, e00895–e00819. doi: 10.1128/AEM.00895-19
Book, A. J., Lewin, G. R., McDonald, B. R., Takasuka, T. E., Doering, D. T., Adams, A. S., et al. (2014). Cellulolytic Streptomyces strains associated with herbivorous insects share a phylogenetically linked capacity to degrade lignocellulose. Appl. Environ. Microbiol. 80, 4692–4701. doi: 10.1128/AEM.01133-14
Book, A. J., Lewin, G. R., McDonald, B. R., Takasuka, T. E., Wendt-Pienkowski, E., Doering, D. T., et al. (2016). Evolution of high cellulolytic activity in symbiotic Streptomyces through selection of expanded gene content and coordinated gene expression. PLoS Biol. 14:e1002475. doi: 10.1371/journal.pbio.1002475
Busk, P. K., Lange, M., Pilgaard, B., and Lange, L. (2014). Several genes encoding enzymes with the same activity are necessary for aerobic fungal degradation of cellulose in nature. PLoS One 9:e114138. doi: 10.1371/journal.pone.0114138
Chettri, D., Verma, A. K., and Verma, A. K. (2020). Innovations in CAZyme gene diversity and its modification for biorefinery applications. Biotechnology Reports. 28:e00525. doi: 10.1016/j.btre.2020.e00525
Cortes-Tolalpa, L., Salles, J. F., and van Elsas, J. D. (2017). Bacterial synergism in lignocellulose biomass degradation – complementary roles of degraders as influenced by complexity of the carbon source. Front. Microbiol. 8:1628. doi: 10.3389/fmicb.2017.01628
de Frias, U. A., Pereira, G. K. B., Guazzaroni, M.-E., and Silva-Rocha, R. (2018). Boosting secondary metabolite production and discovery through the engineering of novel microbial biosensors. Biomed. Res. Int. 2018:7021826. doi: 10.1155/2018/7021826
Devi, A., Bajar, S., Kour, H., Kothari, R., Pant, D., and Singh, A. (2022). Lignocellulosic biomass valorization for bioethanol production: a circular bioeconomy approach. Bioenergy Res. 15, 1820–1841. doi: 10.1007/s12155-022-10401-9
diCenzo, G. C., and Finan, T. M. (2017). The divided bacterial genome: structure, function, and evolution. Microbiol. Mol. Biol. Rev. 81, e00019–e00017. doi: 10.1128/MMBR.00019-17
Dilokpimol, A., Verkerk, B., Li, X., Bellemare, A., Lavallee, M., Frommhagen, M., et al. (2022). Screening of novel fungal carbohydrate esterase family 1 enzymes identifies three novel dual feruloyl/acetyl xylan esterases. FEBS Lett. 596, 1932–1943. doi: 10.1002/1873-3468.14322
Ezeilo, U. R., Zakaria, I. I., Huyop, F., and Wahab, R. A. (2017). Enzymatic breakdown of lignocellulosic biomass: the role of glycosyl hydrolases and lytic polysaccharide monooxygenases. Biotechnol. Biotechnol. Equip. 31, 1–16. doi: 10.1080/13102818.2017.1330124
Finn, R. D., Clements, J., and Eddy, S. R. (2011). HMMER web server: interactive sequence similarity searching. Nucleic Acids Res. 39, W29–W37. doi: 10.1093/nar/gkr367
Flint, H. J., Scott, K. P., Duncan, S. H., Louis, P., and Forano, E. (2012). Microbial degradation of complex carbohydrates in the gut. Gut Microbes 3, 289–306. doi: 10.4161/gmic.19897
Garcia, R., and Müller, R. (2014). “The family Myxococcaceae” in The prokaryotes: Deltaproteobacteria and Epsilonproteobacteria. eds. E. Rosenberg, E. F. DeLong, S. Lory, E. Stackebrandt, and F. Thompson (Berlin, Heidelberg: Springer), 191–212.
Hamid, R., Khan, M. A., Ahmad, M., Ahmad, M. M., Abdin, M. Z., Musarrat, J., et al. (2013). Chitinases: an update. J. Pharm. Bioallied Sci. 5, 21–29. doi: 10.4103/0975-7406.106559
Harindintwali, J. D., Wang, F., Yang, W., Zhou, J., Muhoza, B., Mugabowindekwe, M., et al. (2022). Harnessing the power of cellulolytic nitrogen-fixing bacteria for biovalorization of lignocellulosic biomass. Ind. Crop. Prod. 186:115235. doi: 10.1016/j.indcrop.2022.115235
Hondoh, H., Saburi, W., Mori, H., Okuyama, M., Nakada, T., Matsuura, Y., et al. (2008). Substrate recognition mechanism of alpha-1,6-glucosidic linkage hydrolyzing enzyme, dextran glucosidase from Streptococcus mutans. J. Mol. Biol. 378, 913–922. doi: 10.1016/j.jmb.2008.03.016
Inyang, V., Laseinde, O. T., and Kanakana, G. M. (2022). Techniques and applications of lignocellulose biomass sources as transport fuels and other bioproducts. Int. J. Low-Carbon Technolog. 17, 900–909. doi: 10.1093/ijlct/ctac068
Ivanova, N., Daum, C., Lang, E., Abt, B., Kopitz, M., Saunders, E., et al. (2010). Complete genome sequence of Haliangium ochraceum type strain (SMP-2T). Stand. Genomic Sci. 2, 96–106. doi: 10.4056/sigs.69.1277
Kaiser, D., Robinson, M., and Kroos, L. (2010). Myxobacteria, polarity, and multicellular morphogenesis. Cold Spring Harb. Perspect. Biol. 2:a000380. doi: 10.1101/cshperspect.a000380
Kellner, S., Spang, A., Offre, P., Szöllősi, G. J., Petitjean, C., and Williams, T. A. (2018). Genome size evolution in the Archaea. Emerg. Topics in Life Sci. 2, 595–605. doi: 10.1042/ETLS20180021
Khalikova, E., Susi, P., and Korpela, T. (2005). Microbial dextran-hydrolyzing enzymes: fundamentals and applications. Microbiol. Mol. Biol. Rev. 69, 306–325. doi: 10.1128/MMBR.69.2.306-325.2005
Krivorotova, T., and Sereikaite, J. (2014). Determination of fructan exohydrolase activity in the crude extracts of plants. Electron. J. Biotechnol. 17, 329–333. doi: 10.1016/j.ejbt.2014.09.005
Kumar, A., and Chandra, R. (2020). Ligninolytic enzymes and its mechanisms for degradation of lignocellulosic waste in environment. Heliyon. 6:e03170. doi: 10.1016/j.heliyon.2020.e03170
Land, M., Hauser, L., Jun, S.-R., Nookaew, I., Leuze, M. R., Ahn, T.-H., et al. (2015). Insights from 20 years of bacterial genome sequencing. Funct. Integr. Genomics 15, 141–161. doi: 10.1007/s10142-015-0433-4
Lang, E., Schumann, P., Tindall, B. J., Mohr, K. I., and Spröer, C. (2015). Reclassification of Angiococcus disciformis, Cystobacter minus and Cystobacter violaceus as Archangium disciforme comb. nov., Archangium minus comb. nov. and Archangium violaceum comb. nov., unification of the families Archangiaceae and Cystobacteraceae, and emended descriptions of the families Myxococcaceae and Archangiaceae. Int. J. Syst. Evol. Microbiol. 65, 4032–4042. doi: 10.1099/ijsem.0.000533
Letunic, I., and Bork, P. (2021). Interactive tree of life (iTOL) v5: an online tool for phylogenetic tree display and annotation. Nucleic Acids Res. 49, W293–W296. doi: 10.1093/nar/gkab301
Lewin, G. R., Carlos, C., Chevrette, M. G., Horn, H. A., McDonald, B. R., Stankey, R. J., et al. (2016). Evolution and ecology of Actinobacteria and their bioenergy applications. Ann. Rev. Microbiol. 70, 235–254. doi: 10.1146/annurev-micro-102215-095748
Li, F., Foucat, L., and Bonnin, E. (2021). Effect of solid loading on the behaviour of pectin-degrading enzymes. Biotechnol. Biofuels 14:107. doi: 10.1186/s13068-021-01957-3
Li, X., Shi, Y., Kong, W., Wei, J., Song, W., and Wang, S. (2022). Improving enzymatic hydrolysis of lignocellulosic biomass by bio-coordinated physicochemical pretreatment—a review. Energy Rep. 8, 696–709. doi: 10.1016/j.egyr.2021.12.015
Li, Z., Wu, J., Zhang, B., Wang, F., Ye, X., Huang, Y., et al. (2015). AmyM, a novel Maltohexaose-forming α-amylase from Corallococcus sp. strain EGB. Appl Environ Microbiol. 81, 1977–1987. doi: 10.1128/AEM.03934-14
Li, Z., Xia, C., Wang, Y., Li, X., Qiao, Y., Li, C., et al. (2019). Identification of an endo-chitinase from Corallococcus sp. EGB and evaluation of its antifungal properties. Int. J. Biol. Macromol. 132, 1235–1243. doi: 10.1016/j.ijbiomac.2019.04.056
Li, Y., Zhou, X., Zhang, X., Xu, Z., Dong, H., Yu, G., et al. (2022). A myxobacterial GH19 lysozyme with bacteriolytic activity on both gram-positive and negative phytopathogens. AMB Express 12:54. doi: 10.1186/s13568-022-01393-y
Lightfield, J., Fram, N. R., and Ely, B. (2011). Across bacterial Phyla, distantly-related genomes with similar genomic GC content have similar patterns of amino acid usage. PLoS One 6:e17677. doi: 10.1371/journal.pone.0017677
Lombard, V., Golaconda Ramulu, H., Drula, E., Coutinho, P. M., and Henrissat, B. (2014). The carbohydrate-active enzymes database (CAZy) in 2013. Nucleic Acids Res. 42, D490–D495. doi: 10.1093/nar/gkt1178
Marshall, R. C., and Whitworth, D. E. (2019). Is “wolf-pack” predation by antimicrobial Bacteria cooperative? Cell behaviour and predatory mechanisms indicate profound selfishness, even when working alongside kin. Bioessays. 41:e1800247. doi: 10.1002/bies.201800247
Mohammed, A. S. A., Naveed, M., and Jost, N. (2021). Polysaccharides; classification, chemical properties, and future perspective applications in fields of pharmacology and biological medicine (a review of current applications and upcoming potentialities). J. Polym. Environ. 29, 2359–2371. doi: 10.1007/s10924-021-02052-2
Mohr, K. I. (2018). Diversity of Myxobacteria-we only see the tip of the iceberg. Microorganisms. 6:E84. doi: 10.3390/microorganisms6030084
Mudgil, D. (2017). “The interaction between insoluble and soluble Fiber” in Dietary Fiber for the prevention of cardiovascular disease. ed. R. A. Samaan (Oxford: Academic Press), 35–59.
Muñoz-Dorado, J., Marcos-Torres, F. J., García-Bravo, E., Moraleda-Muñoz, A., and Pérez, J. (2016). Myxobacteria: moving, killing, feeding, and surviving together. Front. Microbiol. 7:7. doi: 10.3389/fmicb.2016.00781
Ng, T. B., and Randy, C. (2011). Cellulase: types, actions, mechanisms and uses. Cellulase: Types and Action, Mechanism and Uses., 251–263.
Oren, A., and Garrity, G. M. (2021). Valid publication of the names of forty-two phyla of prokaryotes. Int. J. Syst. Evol. Microbiol. 71. doi: 10.1099/ijsem.0.005056
Pal, S., Sharma, G., and Subramanian, S. (2021). Complete genome sequence and identification of polyunsaturated fatty acid biosynthesis genes of the myxobacterium Minicystis rosea DSM 24000T. BMC Genomics 22:655. doi: 10.1186/s12864-021-07955-x
Panda, A., Islam, S. T., and Sharma, G. (2022). Harmonizing prokaryotic nomenclature: fixing the fuss over phylum name flipping. MBio 13, e00970–e00922. doi: 10.1128/mbio.00970-22
Ramírez-Ramírez, N., Romero-García, E. R., Calderón, V. C., Avitia, C. I., Téllez-Valencia, A., and Pedraza-Reyes, M. (2008). Expression, characterization and synergistic interactions of Myxobacter Sp. AL-1 Cel9 and Cel48 Glycosyl hydrolases. Int. J. Mol. Sci. 9, 247–257. doi: 10.3390/ijms9030247
Reichenbach, H. (1970). Nannocystis exedens gen. Nov., spec. Nov., a new myxobacterium of the family Sorangiaceae. Arch. Mikrobiol. 70, 119–138. doi: 10.1007/BF00412203
Reichenbach, H. (1999). The ecology of the myxobacteria. Environ. Microbiol. 1, 15–21. doi: 10.1038/sj.jim.7000025
Reichenbach, H. (2001). Myxobacteria, producers of novel bioactive substances. J. Ind. Microbiol. Biotechnol. 27, 149–156.
Reichenbach, H. (2005). “Order VIII. Myxococcales” In: D. J. Brenner, N. R. Krieg, J. T. Staley, and G. M. Garrity (eds.), Bergey’s manual of systematic bacteriology, second edition, (The Proteobacteria), part C (The Alpha-, Beta-, Delta-, and Epsilonproteobacteria). (New York: Springer). 2, 1059–1072.
Sanford, R. A., Cole, J. R., and Tiedje, J. M. (2002). Characterization and description of Anaeromyxobacter dehalogenans gen. Nov., sp. nov., an aryl-Halorespiring facultative anaerobic Myxobacterium. Appl. Environ. Microbiol. 68, 893–900. doi: 10.1128/AEM.68.2.893-900.2002
Schneiker, S., Perlova, O., Kaiser, O., Gerth, K., Alici, A., Altmeyer, M. O., et al. (2007). Complete genome sequence of the myxobacterium Sorangium cellulosum. Nat. Biotechnol. 25, 1281–1289. doi: 10.1038/nbt1354
Shahbaz, U., and Yu, X. (2020). Cloning, isolation, and characterization of novel chitinase-producing bacterial strain UM01 (Myxococcus fulvus). J. Genet. Eng. Biotechnol. 18:45. doi: 10.1186/s43141-020-00059-1
Sharma, G., Khatri, I., and Subramanian, S. (2016). Complete genome of the starch-degrading Myxobacteria Sandaracinus amylolyticus DSM 53668T. Genome Biol. Evol. 8, 2520–2529. doi: 10.1093/gbe/evw151
Sharma, G., and Subramanian, S. (2017). Unravelling the complete genome of Archangium gephyra DSM 2261T and evolutionary insights into Myxobacterial Chitinases. Genome Biol. Evol. 9, 1304–1311. doi: 10.1093/gbe/evx066
Sichert, A., and Cordero, O. X. (2021). Polysaccharide-Bacteria interactions from the Lens of evolutionary ecology. Front. Microbiol. 12:705082. doi: 10.3389/fmicb.2021.705082
Sidar, A., Voshol, G. P., El-Masoudi, A., Vijgenboom, E., and Punt, P. J. (2024). Highly variable domain architecture in carbohydrate-active enzymes highlights Streptomyces as promising resource for rice straw bioconversion. Bioresource Technol. Reports. 25:101775. doi: 10.1016/j.biteb.2024.101775
Silva, S. S., Fernandes, E. M., Pina, S., Silva-Correia, J., Vieira, S., Oliveira, J. M., et al. (2017). “2.11 polymers of biological origin☆” in Comprehensive biomaterials II. ed. P. Ducheyne (Oxford: Elsevier), 228–252.
Stick, R. V., and Williams, S. J. (2009). “Disaccharides, oligosaccharides and polysaccharides” in Carbohydrates: The essential molecules of life. eds. R. V. Stick and S. J. Williams. Second ed (Oxford: Elsevier), 321–341.
Takasuka, T. E., Book, A. J., Lewin, G. R., Currie, C. R., and Fox, B. G. (2013). Aerobic deconstruction of cellulosic biomass by an insect-associated Streptomyces. Sci. Rep. 3:1030. doi: 10.1038/srep01030
Tashkandi, M., and Baz, L. (2023). Function of CAZymes encoded by highly abundant genes in rhizosphere microbiome of Moringa oleifera. Saudi J. Biolog. Sci. 30:103578. doi: 10.1016/j.sjbs.2023.103578
Joshi, N., Upadhyay, D., and Andhare, P. (2021). A study on amylase: review. Int. J. Biol., Pharmacy Allied Sci. 10, 333–340. doi: 10.31032/IJBPAS/2021/10.4.1037
Vera-Ponce de León, A., Jahnes, B. C., Duan, J., Camuy-Vélez, L. A., and Sabree, Z. L. (2020). Cultivable, host-specific Bacteroidetes symbionts exhibit diverse Polysaccharolytic strategies. Appl. Environ. Microbiol. 86, e00091–e00020. doi: 10.1128/AEM.00091-20
Wang, S.-Y., Hu, W., Lin, X.-Y., Wu, Z.-H., and Li, Y.-Z. (2011). A novel cold-active xylanase from the cellulolytic myxobacterium Sorangium cellulosum So9733-1: gene cloning, expression, and enzymatic characterization. Appl. Microbiol. Biotechnol. 93, 1503–1512. doi: 10.1007/s00253-011-3480-3
Whitworth, D. E., Sydney, N., and Radford, E. J. (2021). Myxobacterial genomics and post-genomics: a review of genome biology, genome sequences and related ‘omics studies. Microorganisms. 9:2143. doi: 10.3390/microorganisms9102143
Wu, J., Xia, B., Li, Z., Ye, X., Chen, Q., Dong, W., et al. (2015). Molecular cloning and characterization of a novel GH13 saccharifying α-amylase AmyC from Corallococcus sp. EGB. Starch - Stärke. 67, 810–819. doi: 10.1002/star.201400258
Yamamoto, E., Muramatsu, H., and Nagai, K. (2014). Vulgatibacter incomptus gen. Nov., sp. nov. and Labilithrix luteola gen. Nov., sp. nov., two myxobacteria isolated from soil in Yakushima Island, and the description of Vulgatibacteraceae fam. Nov., Labilitrichaceae fam. Nov. and Anaeromyxobacteraceae fam. Nov. Int. J. Syst. Evol. Microbiol. 64, 3360–3368. doi: 10.1099/ijs.0.063198-0
Yang, W., Su, L., Wang, L., Wu, J., and Chen, S. (2022). Alpha-glucanotransferase from the glycoside hydrolase family synthesizes α(1–6)-linked products from starch: features and synthesis pathways of the products. Trends Food Sci. Technol. 128, 160–172. doi: 10.1016/j.tifs.2022.08.001
Yang, Y., Yoo, C. G., Guo, H.-B., Rottmann, W., Winkeler, K. A., Collins, C. M., et al. (2017). Overexpression of a domain of unknown function 266-containing protein results in high cellulose content, reduced recalcitrance, and enhanced plant growth in the bioenergy crop Populus. Biotechnol. Biofuels 10:74. doi: 10.1186/s13068-017-0760-x
Yeager, C. M., Gallegos-Graves, L. V., Dunbar, J., Hesse, C. N., Daligault, H., and Kuske, C. R. (2017). Polysaccharide degradation capability of Actinomycetales soil isolates from a semiarid grassland of the Colorado plateau. Appl. Environ. Microbiol. 83, e03020–e03016. doi: 10.1128/AEM.03020-16
Yousuf, A., Pirozzi, D., and Sannino, F. (2020). (eds.). Chapter 1 - Fundamentals of lignocellulosic biomass. Lignocellulosic biomass to liquid biofuels, Academic Press, 1–15. Available at: https://www.sciencedirect.com/science/article/pii/B9780128159361000010
Zerva, A., Pentari, C., Ferousi, C., Nikolaivits, E., Karnaouri, A., and Topakas, E. (2021). Recent advances on key enzymatic activities for the utilisation of lignocellulosic biomass. Bioresour. Technol. 342:126058. doi: 10.1016/j.biortech.2021.126058
Zhang, G., and Dong, Y. (2022). Design and application of an efficient cellulose-degrading microbial consortium and carboxymethyl cellulase production optimization. Front. Microbiol. 13:957444. doi: 10.3389/fmicb.2022.957444
Zhang, L., Dong, C., Wang, J., Liu, M., Wang, J., Hu, J., et al. (2023). Predation of oomycetes by myxobacteria via a specialized CAZyme system arising from adaptive evolution. ISME J. 17, 1089–1103. doi: 10.1038/s41396-023-01423-y
Zhao, Y., Wang, Y., Xia, C., Li, X., Ye, X., Fan, Q., et al. (2021). Whole-genome sequencing of Corallococcus sp. strain EGB reveals the genetic determinants linking taxonomy and predatory behavior. Genes (Basel) 12:1421. doi: 10.3390/genes12091421
Zhou, X., Chen, X., Qi, X., Zeng, Y., Guo, X., Zhuang, G., et al. (2023). Soil bacterial communities associated with multi-nutrient cycling under long-term warming in the alpine meadow. Front. Microbiol. 14:14. doi: 10.3389/fmicb.2023.1136187
Zhou, J., Li, Z., Zhang, H., Wu, J., Ye, X., Dong, W., et al. (2018). Novel Maltogenic amylase CoMA from Corallococcus sp. strain EGB catalyzes the conversion of Maltooligosaccharides and soluble starch to maltose. Appl. Environ. Microbiol. 84, e00152–e00118. doi: 10.1128/AEM.00152-18
Zhou, Y., Yi, S., Zang, Y., Yao, Q., and Zhu, H. (2021). The predatory Myxobacterium Citreicoccus inhibens gen. Nov. sp. nov. showed antifungal activity and Bacteriolytic property against Phytopathogens. Microorganisms 9:2137. doi: 10.3390/microorganisms9102137
Zhou, X., Zhou, X., Zhang, X., Dong, H., Dong, Y., and Zhu, H. (2024). Secretory CAZymes profile and GH19 enzymes analysis of Corallococcus silvisoli c25j21. Front. Microbiol. 15:1324153. doi: 10.3389/fmicb.2024.1324153
Zoghlami, A., and Paës, G. (2019). Lignocellulosic biomass: understanding recalcitrance and predicting hydrolysis. Front. Chem. 7:7. doi: 10.3389/fchem.2019.00874
Keywords: myxobacteria, comparative genomics, carbohydrate active enzymes (CAZyme), polysaccharide degradation, soil-dwelling bacteria, lignocellulosic biomass
Citation: Saraf N and Sharma G (2025) Comparative genomic insight into the myxobacterial carbohydrate-degrading potential. Front. Microbiol. 16:1550287. doi: 10.3389/fmicb.2025.1550287
Edited by:
Reeta Goel, G. B. Pant University of Agriculture and Technology, IndiaReviewed by:
Kuan-ting Hsin, National Taiwan University, TaiwanYanfang Wang, University of Groningen, Netherlands
Lina Baz, King Abdulaziz University, Saudi Arabia
Copyright © 2025 Saraf and Sharma. This is an open-access article distributed under the terms of the Creative Commons Attribution License (CC BY). The use, distribution or reproduction in other forums is permitted, provided the original author(s) and the copyright owner(s) are credited and that the original publication in this journal is cited, in accordance with accepted academic practice. No use, distribution or reproduction is permitted which does not comply with these terms.
*Correspondence: Gaurav Sharma, c2hhcm1hR0BidC5paXRoLmFjLmlu