- 1College of Zhongbei, Nanjing Normal University, Danyang, Jiangsu, China
- 2Jiangsu Provincial Key Laboratory of Materials Cycling and Pollution Control, School of Geographical Sciences, Nanjing Normal University, Nanjing, China
- 3School of Environment, Nanjing Normal University, Nanjing, China
- 4Jiangsu Center for Collaborative Innovation in Geographical Information Resource Development and Application, Nanjing, China
Introduction: Ammonium (NH4+) and nitrate (NO3−) are the two main forms of inorganic nitrogen (N) that exist in soil and both can be absorbed and utilized by plants. As a vast and crucial biome, soil microorganisms are responsible for mediating the inorganic N assimilation process and enhancing nitrogen use efficiency. Understanding how these microorganisms assimilate different forms of inorganic nitrogen is crucial. There are a handful of microorganisms that play a dominant role in the process of soil inorganic nitrogen assimilation and have a significant advantage in abundance. However, microbial preferences for ammonium or nitrate, as well as differences in their metabolic pathways under co-existing ammonium and nitrate conditions, remain unclear.
Methods: In this study, two microbial strains with nitrogen assimilation advantages, Burkholderia sp. M6-3 and Arthrobacter sp. M7-15 were isolated from an acidic Chinese soil and then incubated by different sources of inorganic N to investigate their N preferences. Furthermore, RNA sequencing-based transcriptome analysis was used to map the metabolic pathways of the two strains and explore their explanatory potential for N preferences.
Results: The results showed that strain M6-3 preferred to utilize NH4+ while strain M7-15 preferred to utilize NO3−. Although both strains shared similar nitrogen metabolic pathways, the differential expression of the glutamine synthetase-coding gene glnA played a crucial role in regulating their inorganic N preferences. This inconsistency in glnA expression may be attributed to GlnR, a global regulator of nitrogen utilization.
Discussion: This research strengthens the theoretical basis for exploring the underlying causes of differential preferences for inorganic N forms and provided key clues for screening functional microorganisms to ultimately enhance inorganic nitrogen use efficiency.
1 Introduction
Nitrogen (N) is essential for all life on Earth (Stevens, 2019). Multiple forms of N are present in soil, including inorganic N [ammonium (NH4+) and nitrate (NO3−)] and low molecular weight organic N [such as amino acids and microbial biomass nitrogen (MBN)], which can be absorbed and utilized by plants (Beeckman et al., 2018). In agroecological systems, NH4+ and NO3− are the most critical N sources for crops (Cui et al., 2017; Zhu Y. et al., 2021). Soil NO3− and NH4+ concentrations are often insufficient for crop growth; applying additional N fertilizer to agricultural soils is a common practice to improve crop yields and ensure food security (Fageria and Baligar, 2005). Studies indicate that most crops exhibit differential preferences for inorganic N forms. For example, wheat and maize preferentially absorb NO3− (Zhang et al., 2016), while rice shows a strong preference for NH4+ (Zhao et al., 2013). Similarly, soil microorganisms also display distinct inorganic N preferences. Microorganisms generally prefer to utilize NH4+ in soil (Christie and Wasson, 2001), possibly due to the lower energy requirement for NH4+ assimilation (Murphy et al., 2003). In contrast, other researchers found that even in alkaline soils with a high NH4+/NO3− ratio, microbial assimilation of NO3− remained dominant (Rochester et al., 1992). Certain studies have been conducted on the reasons for plants’ preference for NH4+ or NO3−, and it has been found that functional genes for ammonium and nitrate/nitrite transport may play a key role in their N preference (Hildebrand, 2005). However, the intrinsic causes of microbial preference for NH4+ or NO3− are unclear.
Soil microorganisms are essential for ecosystem multifunctionality (Wagg et al., 2014). One of the underlying mechanisms is the assimilation process in which microorganisms absorb inorganic N, reducing their losses and enhancing N use efficiency (Zhu J. et al., 2021; Wang et al., 2024). The assimilation of inorganic N by soil microorganisms is strongly related to their N metabolic pathways (Pan et al., 2023). Numerous studies have investigated the assimilation processes of NH4+ and NO3−and found that NO3− is the primary source of inorganic N used by most bacteria, yeasts, and algae (González et al., 2006; Wang and Song, 2024). NO3− is reduced to NO2− by assimilatory nitrate reductases (Nas) after being transported into the cell by nitrate transporters, followed by reduction to NH4+ by nitrite reductase (Jin et al., 2019). NH4+ is a crucial compound for nitrogen assimilation in many biological systems (Zhang L. J. et al., 2020) and can also be incorporated into the carbon skeleton through the glutamate dehydrogenase (GDH) or glutamine synthetase-glutamate synthase (GS-GOGAT) pathways (Mara et al., 2018; Gao et al., 2025) after entering the cell via ammonium transport family protein (Amt) transport (Andrade and Oliver., 2007; Williamson et al., 2022). In this series of processes, multiple functional genes for nitrogen metabolism are activated and expressed (Tu et al., 2019). After cells transport nitrate or nitrite into the cytoplasm through active transport by NrT/NrTA-regulated transporter proteins (Richardson et al., 2001), nitrate is reduced by nasAB-encoded Nas to nitrite, nirBD-encoded nitrite reductases reduce nitrite to ammonium (Zhang et al., 2015), and external nitrite can also be taken up and directly reduced to ammonium (Lledó et al., 2005). The ammonium produced by the nitrite reductase reaction is incorporated into the carbon skeleton via the GDH or GS–GOGAT pathways. The GDH and GS–GOGAT pathways are two pathways of ammonium assimilation, some microorganisms use only one of them strictly while others use both pathways (Gao et al., 2025). Thus, microbial utilization of inorganic N is closely related to its nitrogen metabolism pathways and functional gene expression. However, it is unclear whether microbial preference for inorganic N in the assimilation process is controlled by the difference in metabolic pathways or the expression level of functional genes mentioned above.
When adding multiple forms of N, either inorganic or organic, the majority of N was immobilized in microbial biomass (Kaštovská and Šantrůčková, 2011; Tahovská et al., 2013). Initially, all soil microorganisms were recognized to jointly participate in N assimilation and immobilization (Schimel, 1995). Recent studies proposed that N assimilation is performed by a relatively select group of microorganisms rather than a large number of seemingly redundant groups of microbial taxa (McGuire and Treseder, 2010). This finding was further supported by the fact that only a few groups of microorganisms contributed to 82–88% of the assimilated N in meadow soil, using a quantitative 15N-labeled stable isotope probing approach (Dong et al., 2022; Morrissey et al., 2018), and also evidenced in shelf environments (Wawrik et al., 2012). Therefore, it is feasible to isolate dominant N-assimilating strains that prefer different inorganic N sources, which can facilitate the study of their assimilation differences.
This study aims to address the following issues in microbial nitrogen metabolism research: (1) the molecular mechanisms underlying the differential preferences for inorganic nitrogen in two soil bacteria; and (2) the differences in nitrogen utilization regulation of the two bacteria when different forms of inorganic nitrogen serve as nitrogen sources. Using Burkholderia sp. M6-3 and Arthrobacter sp. M7-15 isolated from acidic upland soils, we sought to: (1) Characterize strain specific nitrogen source preferences through phenotypic analysis; (2) Elucidate the differences in dynamic gene expression under mixed nitrogen conditions through transcriptomic analysis; and (3) Identify conserved regulatory networks and strain specific molecular switches that control nitrogen metabolism. These integrated approaches are aimed at understanding microbial nitrogen utilization strategies and providing a theoretical basis for improving nitrogen use efficiency in agricultural systems.
2 Materials and methods
2.1 Strains and growth conditions
The test strains Burkholderia sp. M6-3 and Arthrobacter sp. M7-15 (Figure 1) were isolated from an acidic upland soil (Liu et al., 2023), which came from a long-term field fertilization experiment site (28°15′30″N, 116°20′24″E) started in 1986 at the Jiangxi Institute of Red Soil and Germplasm Resources, China. The area has a subtropical monsoon climate with an average annual rainfall of 1,537 mm and an average annual temperature of 17.5°C. The soil is derived from Quaternary red clay, and the cropping system is the spring corn-autumn corn-winter idle system. Soil sampling was conducted in three plots. Surface soil (0–15 cm) was collected using a five-point sampling method from each plot, mixed thoroughly, and sieved through a 2 mm mesh. Three replicates of soil samples were collected. Soil pH was 4.90, soil electrical conductivity was 27.20 μS cm−1, organic carbon content was 7.14 g kg−1, and total nitrogen was 0.92 g kg−1 (Zhong et al., 2010; Wang et al., 2015).
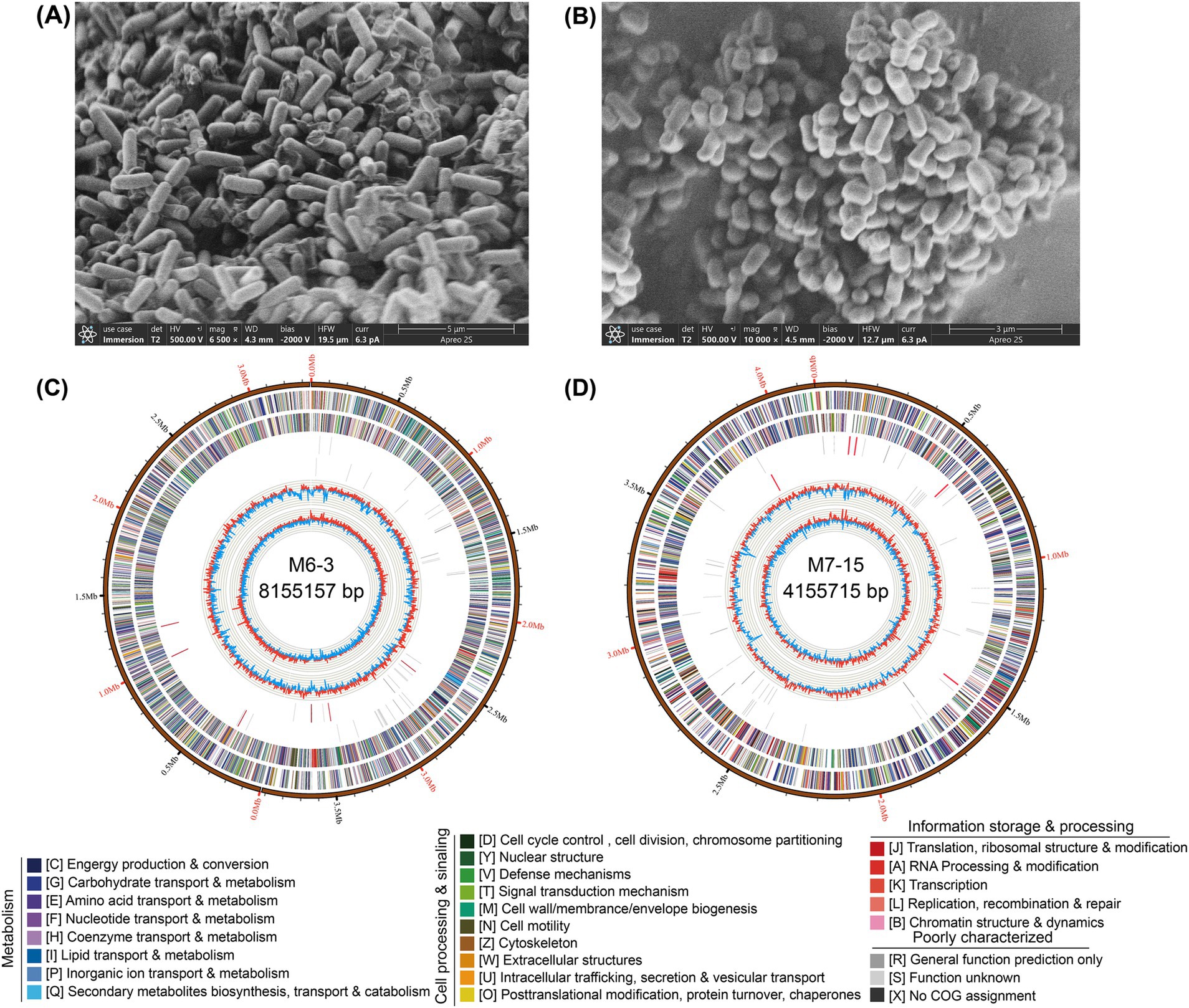
Figure 1. Morphology and genome information of Burkholderia sp. M6-3 (A,C) and Arthrobacter sp. M7-15 (B,D). The circular genome map is displayed from the outer circle to innermost, as follows: scale marks of the genome; protein-coding genes on the forward strand; protein-coding genes on the reverse strand; tRNA (black) and rRNA (red) genes on the forward strand; tRNA (black) and rRNA (red) genes on the reverse strand; GC content; GC skew. Protein-coding genes are color coded according to their COG categories.
The media required for the strain’s growth were prepared as follows: liquid nutrient agar medium (NA medium) (Mishra, 2019): 10 g peptone, 3 g beef extract and 5 g NaCl, dissolved in 1000 mL of water (pH 7.0), and autoclaved at 121°C for 20 min. Nitrogen-free medium (NF medium) (Patil et al., 2020): 10 g glucose, 0.2 g K2HPO4, 0.2 g MgSO4, 0.2 g NaCl, 0.1 g K2SO4, 1.0 g CaCO3, dissolved in 1000 mL of water (pH 7.0), autoclaved at 121°C for 20 min (glucose alone autoclaved at 115°C for 30 min).
Inorganic nitrogen at 4 μmol N g−1 soil and glucose at 500 μg C g−1 soil were added to activate the soil nitrogen assimilation and enrich the N-assimilating microorganisms (Morrissey et al., 2018). The dominant microbial groups with the highest relative abundance were obtained by high-throughput sequencing of total soil DNA, and individual strains were isolated from the NF medium coated with soil suspension. A total of 154 isolated bacteria were incubated. Bacterial genomic DNA was extracted using the Rapid Bacterial Genomic DNA Isolation Kit (Sangon Biotech). A 25 μL PCR reaction mixture was employed to amplify the selected suspected strains, consisting of 12.5 μL of PCR Master Mix, 0.5 μL of each primer (27F: 5′-AGAGTTTGATCCTGGCTCAG-3′, 5 μM; 1492R: 5′-TACGGYTACCTTGTTAYGACTT-3′, 5 μM) 10.5 μL of ddH₂O, and 1 μL of DNA template (Haas et al., 2011). The amplification conditions were as follows: 94°C for 5 min; 35 cycles of denaturation at 94°C for 30 s, annealing at 58°C for 30 s, and extension at 72°C for 90 s; followed by a final extension at 72°C for 7 min. The PCR products were analyzed by agarose gel electrophoresis to assess quantity and quality. Sequencing was performed by Sangon Biotech (Shanghai) Co., Ltd. (Shanghai, China) using an Applied Biosystems™ 3730XL Genetic Analyzer (Thermo Fisher Scientific) with bidirectional sequencing. Raw sequencing data were edited and assembled using Sequencher 5.0 software (Gene Codes Corporation). The resulting sequences were subjected to BLAST alignment against the NCBI GenBank database. The sequences of the strains were compared to the sequences of the dominant microorganisms in the soil, then the strains Burkholderia sp. M6-3 and Arthrobacter sp. M7-15, which had the closest sequence, was isolated.
2.2 Whole genome sequencing of strains
Both strains were sequenced at Guangdong Magigene Biotechnology Co., Ltd. (Guangzhou, China) on the Illumina Hiseq1500 platform. For the libraries that passed the quality inspection, paired-end 150 bp (PE150) sequencing was performed on an on the Illumina Hiseq 1,500 platform. Sequencing data were filtered and assembled using a de novo assembly strategy (Metzker, 2010). Pure third-generation sequencing (TGS) data were assembled with SMRT Link v5.1.0 (Jayakumar and Sakakibara, 2019), while hybrid assembly integrating second- and third-generation sequencing data was performed with Unicycler (Wick et al., 2017). Genomic data of the strains were annotated by the KEGG (Kyoto Encyclopedia of Genes and Genomes) (Kanehisa and Goto, 2000), and a genome-wide Blast1 similarity search (E-value < 1−10, minimal alignment length percentage > 40%) was performed against on databases, including COG, KEGG, GO, NR, and Swiss-Prot (Pan et al., 2017; Sun et al., 2019). Sequencing data were used to map the nitrogen metabolism pathway and identify functional genes based on KEGG annotation. The raw genomic data were deposited in the NCBI Sequence Read Archive database under the accession number PRJNA891540.
2.3 Strain growth under different inorganic nitrogen sources
Strains of Burkholderia sp. M6-3 and Arthrobacter sp. M7-15 were inoculated into the NA medium and incubated at 200 r/min 30°C until the proliferation phase, and the bacteria were collected, washed three times with saline, and resuspended.
Four treatments, nitrogen-free medium (Control) and with additions of KNO3 (NO3−), NH4Cl (NH4+) and KNO3 + NH4Cl (NO3− + NH4+), were considered. The concentration of each nitrogen source in the medium was made at 100 mg N L−1 (pH = 7.0 ± 0.2). The parameters of the culture medium were determined based on the pre-experiment (Supplementary Figures 1, 2). Each treatment was autoclaved at 121°C for 20 min, injected with 100 μL of resuspended bacteria and incubated at 30°C 200 r/min. All treatments were repeated at least thrice. The biomass accumulation was determined for every 6 h by measuring the optical density at 600 nm (OD600) using a BioTek Synergy™ HT Multidetection microplate reader. Ammonium, nitrite and nitrate contents were measured with a San++ continuous flow analyzer (Skalar, Breda, Netherlands). Microbial biomass nitrogen (MBN) was determined by collecting all the bacteria in the medium, freeze-drying them, using a Microwave Digestion System (TANK 40, SINEO, Shanghai, China), and then using a total organic carbon analyzer (TOC-L CPH, Shimadzu, Japan).
2.4 Transcriptome analysis by RNA sequencing
For each treatment, bacteria were collected by centrifugation at 6000 r/min for 5 min during the proliferation phase and stored at −80°C refrigerator after quick-freezing with liquid nitrogen. Total RNA was extracted from the collected organisms using the TRIzol method. Quality checks of extracted RNA samples were conducted using a NanoDrop One Spectrophotometer (Thermo Scientific, Waltham, MA, United States) and Agilent 4,200 Tape Station (Santa Clara, CA, United States). Ribosomal RNA (rRNA) was removed from the total RNA using an Epicenter Ribo-Zero rRNA Removal Kit (Madison, WI, United States). Each treatment included 3 replicates, and the extracted RNAs were pooled in equal proportions for sequencing. RNA sequencing was performed by Guangdong Magigene Biotechnology Co., Ltd. (Guangzhou, China).
Library preparation was carried out with a NEBNext® Ultra II™ Directional RNA Library Prep Kit (Illumina, San Diego, CA, United States) according to the standard protocol. The library was subjected to quality control, and PE150 sequencing was performed using the Illumina high-throughput sequencing platform. The raw data files obtained from sequencing were transformed into raw sequencing sequences (Raw Reads) by Base Calling (Ledergerber and Dessimoz, 2011), and the results were stored in FASTQ (fq) file format. Sequence quality control and data filtering was performed with fastp (Chen et al., 2018), and rRNA reads were filtered out before analysis. The ribosome contamination was removed by Bowtie 2 (Langmead and Salzberg, 2012), and the sequencing quality was analyzed by RSeQC (Wang et al., 2012). The read count was calculated using RSEM (Li and Dewey, 2011). The read counts of transcripts were normalized using the “tximport” package (Soneson et al., 2015) to calculate Fragments Per Kilobase of transcript per Million mapped reads (FPKM), which can eliminate the effects of gene length and differences in sequencing depth on the calculation of gene expression. GFOLD (v1.1.4) was employed to identify differentially expressed genes (DEGs) across different inorganic N treatments (Feng et al., 2012). GFOLD ranks differentially expressed genes from RNA-seq data, relying on the posterior distribution of log fold change, to overcome the limitations of p-value, providing stable and biologically relevant results (Takahashi et al., 2022). The significance cutoff was set at 0.01 (−sc 0.01), and a |GFOLD value| > 1 was also required for 2-fold change or greater (Yang et al., 2017; Adachi et al., 2024). The genes were annotated using the KEGG database. After annotating each gene into a KEGG functional category, and comparisons were made in terms of that functional category (Guo et al., 2005). RNA-seq data have been submitted to the NCBI Sequence Read Archive (SRA) under the BioProject accession PRJNA891352.
2.5 Statistical analysis
Statistical analyses were conducted using SPSS (V20.0, IBM, United States). The one-way analysis of variance (ANOVA) was employed to assess the significant differences in OD600, inorganic nitrogen content and biomass among treatments (p < 0.05, n = 3, Duncan’s test).
3 Results
3.1 Strain morphology and genomic characteristics
Visualized on solid medium, both strains, Burkholderia sp. M6-3 and Arthrobacter sp. M7-15 formed moist, white, and round colonies with neatly defined edges and raised centers. The diameter of each colony is approximately 1.0 mm. When observed using scanning electron microscopy (SEM), Burkholderia sp. M6-3 exhibits a rod shape, measuring 0.6–0.7 μm × 1.0–2.2 μm (Figure 1A), while Arthrobacter sp. M7-15 also displays a rod shape, measuring 0.4–0.5 μm × 0.8–1.2 μm (Figure 1B).
The whole genome maps of two strains were visualized using Circos. Each genome comprises both coding and non-coding regions that are essential for transcriptional regulation, translational regulation, epigenetic functions, and other biological processes. The genome size of strain M6-3 was 8,155,157 bp, with a GC content of 61.6%. This strain contained two chromosomes and two plasmids (Figure 1C). The total number of coding genes was 7,989, and the length of coding regions accounted for 83.53% of the genome. The non-coding RNA repertoire included 63 tRNAs, 18 rRNAs, and 5 sRNAs (Supplementary Table 1). In contrast, the genome size of strain M7-15 was 4,155,715 bp, with a GC content of 63.31%. This strain contained one chromosome (Figure 1D). The number of coding genes was 4,092, with coding regions constituting 87.95% of the genome. The non-coding RNA in this strain included 57 tRNAs and 15 rRNAs (Supplementary Table 1).
3.2 Preference for inorganic nitrogen of two strains
The growth characteristics of both strains were assessed using OD600 at various sources of inorganic nitrogen. Both M6-3 and M7-15 exhibited relatively longer lag and exponential growth phases in response to NO3− treatment compared to the treatments with NH4+ and NO3− + NH4+ (Figure 2). For the NO3− + NH4+ treatment, the actual lag phase may be less than 6 h, indicating that the response of both strains to NH4+ is more rapid than to NO3−. While the growth curves of both strains did not show statistically significant differences between the NH4+ and NO3− + NH4+ treatments, significant differences were observed between the NO3− and NH4+ or NO3− + NH4+ treatments (p < 0.05) (Figure 2).
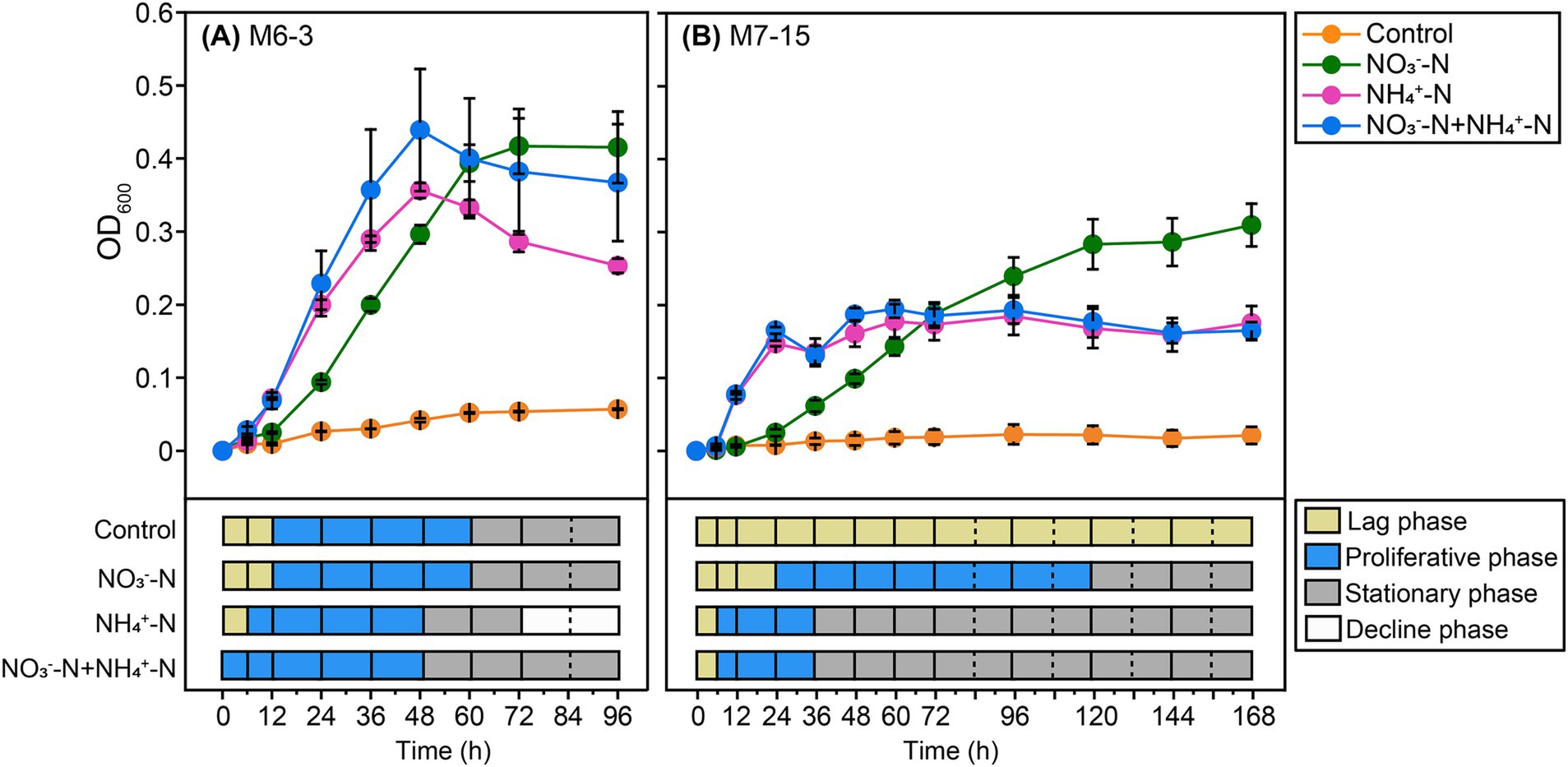
Figure 2. Growth of Burkholderia sp. M6-3 (A) and Arthrobacter sp. M7-15 (B) under different inorganic nitrogen application. Linear graphs indicate the growth curve of the bacterial strains in terms of the change in OD600 value. The time axis indicates the different time periods of bacterial strain growth. The different periods of strains growth were classified according to whether there was a significant difference between their OD600 values (p < 0.05, n = 3, Student’s T-test). Control refers nitrogen-free treatment. Bars indicate the standard deviation (n = 3).
For strain M6-3, the measured nitrate content exhibited a decrease of 4.40 ± 2.90 mg L−1 over the 24 h period, and a decrease of 13.87 ± 1.47 mg L−1 over the 72 h period in the NO3− treatment. The measured ammonium content decreased by 11.80 ± 2.79 mg L−1 during the 24 h interval and by 19.33 ± 2.07 mg L−1 during the 72 h interval in the NH4+ treatment. The decrease in nitrate content observed in the NO3− treatment was significantly smaller than those noted for ammonium content in the NH4+ treatment. In the mixed nitrate and ammonium N treatment, total inorganic N decreased by 13.13 ± 4.97 mg L−1, with nitrate and ammonium contributing 2.06 ± 2.28 mg L−1 and 11.07 ± 2.93 mg L−1, respectively (Figures 3A–C). These findings indicate that strain M6-3 preferentially utilizes ammonium N.
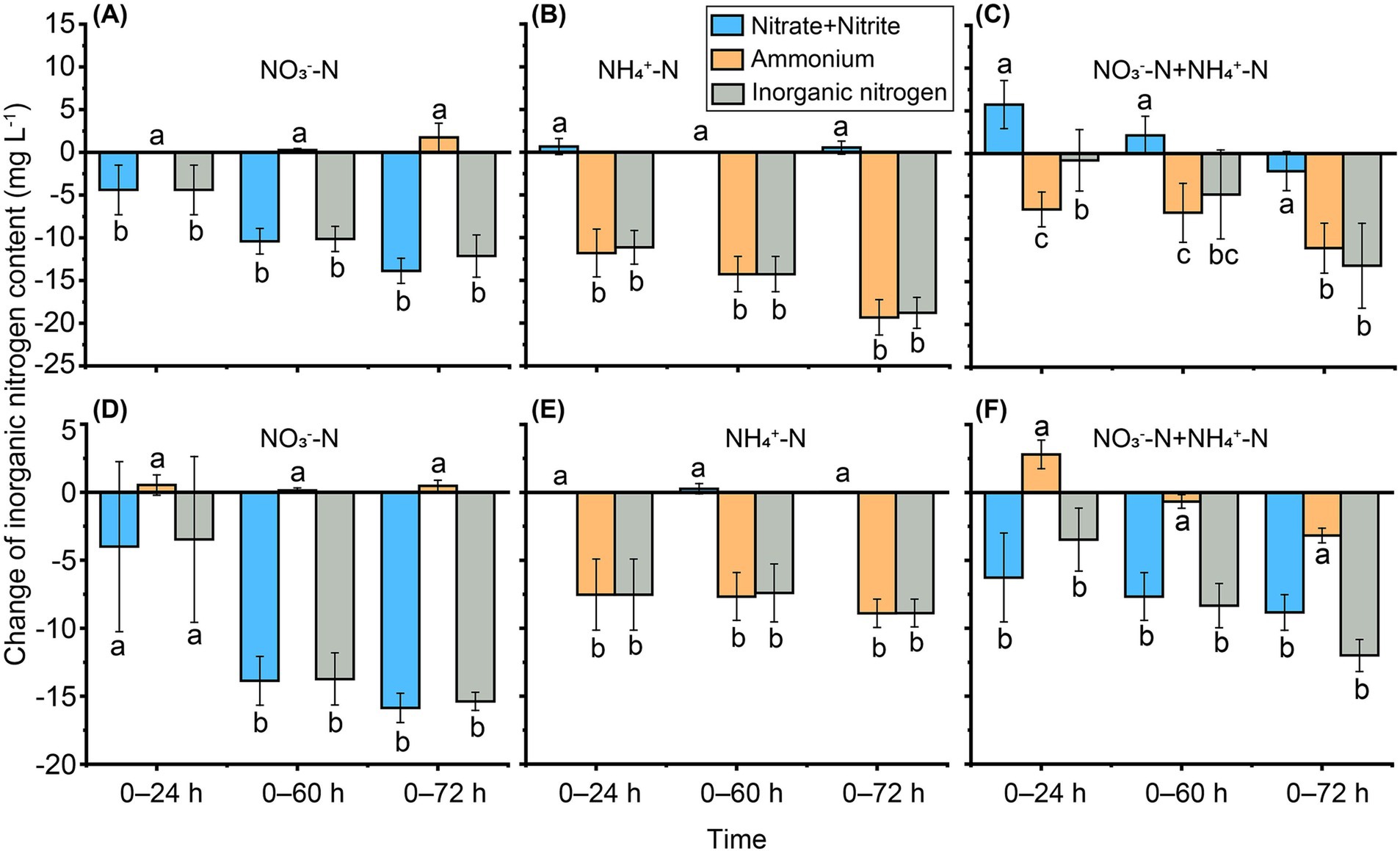
Figure 3. Changes in inorganic nitrogen contents during 72 h of incubation with Burkholderia sp. M6-3 (A–C) and Arthrobacter sp. M7-15 (D–F). “0–24 h,” “0–60 h” and “0–72 h” indicate the changes of inorganic nitrogen content from 0 h to 24 h, from 0 h to 60 h and from 0 h to 72 h, respectively. Bars indicate the standard deviation, different letters indicate significant differences (p < 0.05, n = 3, Duncan’s test).
For strain M7-15, the measured nitrate content exhibited a decrease of 4.00 ± 10.83 mg L−1 over a 24 h period, and a decrease of 15.85 ± 1.07 mg L−1 over the 72 h period in the NO3− treatment. The measured ammonium content decreased by 7.53 ± 2.61 mg L−1 during the 24 h interval and by 8.90 ± 1.04 mg L−1 during the 72 h interval in the NH4+ treatment. The decrease in nitrate content observed in the NO3− treatment was significantly greater than the decrease in ammonium content noted in the NH4+ treatment. In the mixed treatment of nitrate and ammonium nitrogen, the total inorganic nitrogen decreased by 12.00 ± 1.18 mg L−1, with contributions from nitrate and ammonium amounting to 8.83 ± 1.31 mg L−1 and 3.17 ± 0.54 mg L−1, respectively (Figures 3D–F). These findings indicate that the strain M7-15 preferentially utilizes nitrate nitrogen.
3.3 Expression profiles of nitrogen metabolism-related genes
For strain M6-3, a total of 1,302 DEGs was identified from the sample groups NO3−-N vs. NH4+-N, of which 593 were significantly up-regulated and 709 were significantly down-regulated (Figures 4A,B). In the sample groups NO3−-N vs. NH4+-N for strain M7-15, a total of 430 DEGs were identified, with 238 significantly up-regulated and 192 significantly down-regulated (Figures 4C,D). Following KEGG annotation, 13 and 10 types of genes were selected to map nitrogen metabolic pathways for strains M6-3 and M7-15, respectively. The results indicated that both M6-3 and M7-15 shared a similar nitrogen metabolic pathway, except for the NtrC family genes in the two-component system (Figure 5). After nitrate is transported into the cell via NrT/NrTA-regulated transporter proteins, it is reduced to nitrite by Nas, then reduced to NH4+ by nitrite reductase, and then incorporated into the carbon skeleton by GDH or GS-GOGAT pathway. Based on the mapped nitrogen metabolism pathway, it is feasible to investigate the underlying causes of the differential preferences for inorganic N.
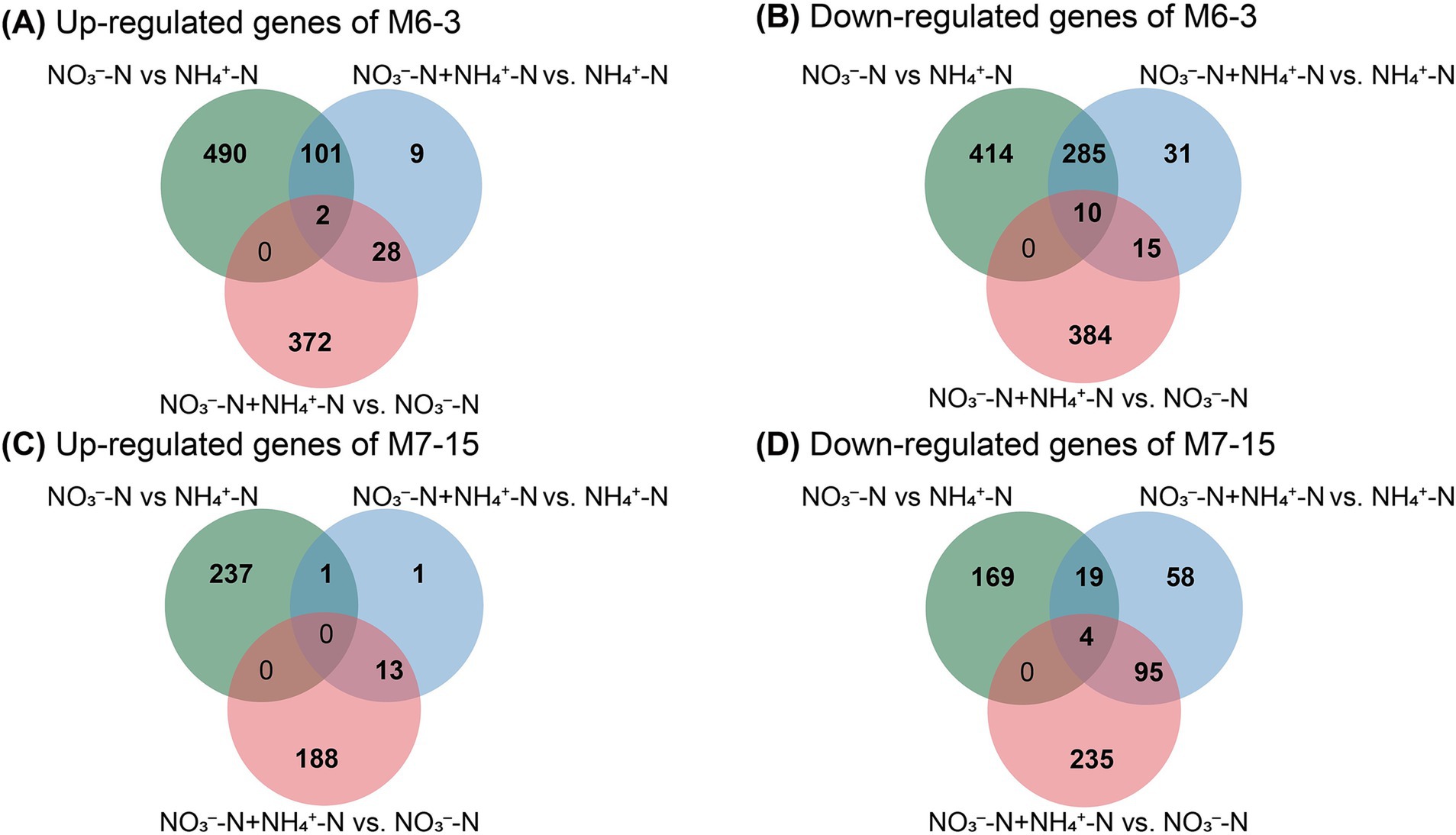
Figure 4. The intersections of differentially expressed genes (DEGs) of Burkholderia sp. M6-3 (A,B) and Arthrobacter sp. M7-15 (C,D) under different inorganic nitrogen treatments.
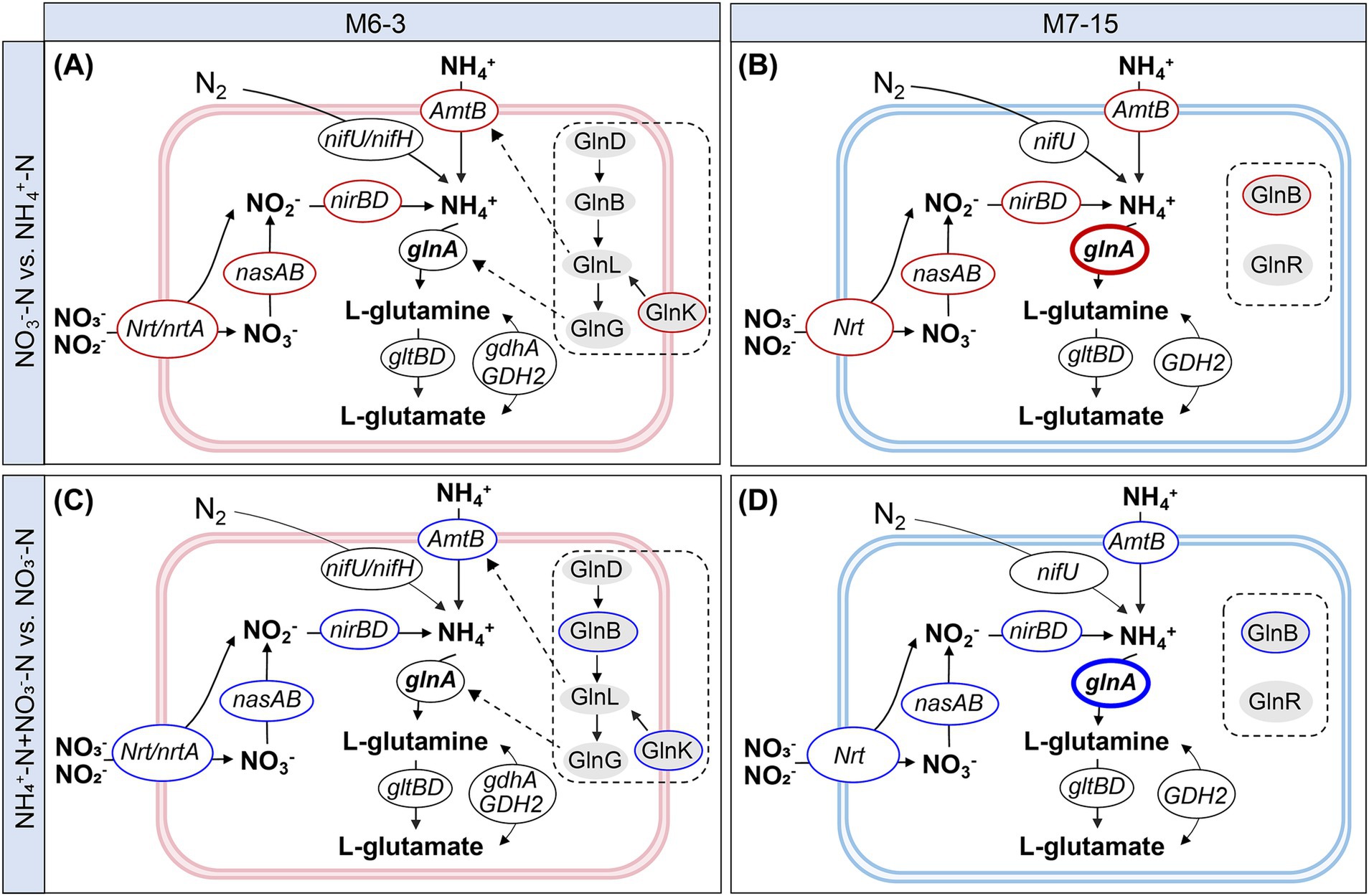
Figure 5. Differential transcript expression of functional genes for nitrogen metabolism in ammonium nitrogen, nitrate nitrogen and mixed nitrogen sources by Burkholderia sp. M6-3 (A,C) and Arthrobacter sp. M7-15 (B,D). The significance cutoff was set at 0.01 (−sc 0.01), and a |GFOLD value| > 1 was also required for 2-fold change or greater. Red borders indicate genes significantly up-regulated (Gfold >1); blue borders indicate genes significantly down-regulated (Gfold < −1), and black borders indicate no significant difference. Gray ovals indicate the nitrogen regulatory factors associate with the two-component regulatory system.
From the sample groups NO3−-N vs. NH4+-N, the nitrate/nitrite transport and reduction genes Nrt/NrtA, nasAB, nirBD, the ammonium transporter AmtB and the nitrogen status sensor GlnB/GlnK were significantly up-regulated in both strains M6-3 and M7-15. However, the glutamine synthetase-coding gene glnA was significantly up-regulated only in strain M7-15, while no significant change was observed in M6-3 (Figures 5A,B). In the sample groups NH4+-N + NO3−-N vs. NO3−-N, the genes Nrt/NrtA, nasAB, nirBD, AmtB, and GlnB/GlnK were significantly down-regulated for both strain M6-3 and M7-15. Additionally, the glnA gene showed significant down-regulation only in M7-15 but not in M6-3 (Figures 5C,D). In the sample groups NH4+-N + NO3−-N vs. NH4+-N, the gene GlnB in M6-3 and the gene nirBD in M7-15 were significantly down-regulated, while the other genes had no significant differences (Supplementary Figure 3).
4 Discussion
4.1 Response of two bacterial strains to different inorganic nitrogen
This study found that M6-3 and M7-15 exhibited longer lag phases and exponential growth phases under NO3−-N treatment (Figure 2), which might be related to the metabolic mechanism of nitrate N. The utilization of nitrate N usually requires its reduction to ammonium N through the nitrate reductase system, a process that may involve energy consumption and the induction of gene expression, thereby leading to an extended lag phase (Wu et al., 2016). Notably, the lag phases of both strains were shortened under NO3−-N + NH4+-N mixed treatment (Figure 2), suggesting that the presence of NH4+-N might accelerate growth initiation through a synergistic effect or metabolic shortcut. This phenomenon is consistent with the reported characteristics of certain strains that preferentially utilize ammonium nitrogen (Geisseler et al., 2010). Although there was no statistical difference in the growth curves between NH4+-N and NO3−-N + NH4+-N treatments for both strains, the biomass was slightly higher under NO3−-N + NH4+-N treatment compared to pure NH4+-N conditions, which might reflect that diverse nitrogen forms are beneficial for increasing gene richness and thus promoting microbial growth (Ameer et al., 2025). However, the specific molecular mechanism underlying this difference requires further experimental verification.
The strains showed different preferences for different inorganic nitrogen. Inoculated with M6-3, the reduction of ammonium N was 1.4 times of the reduction of nitrate N under the NH4+-N or NO3−-N treatment (19.33 ± 2.07 mg L−1 vs. 13.87 ± 1.47 mg L−1), and ammonium N reduction was 5.4 times that of nitrate N reduction under the NO3−-N + NH4+-N treatment (11.07 ± 2.93 mg L−1 vs. 2.06 ± 2.28 mg L−1) during 0–72 h. Inoculated with M7-15, the reduction of nitrate N was 1.8 times greater than the reduction of ammonium N under the NH4+-N or NO3−-N treatment (15.85 ± 1.07 mg L−1 vs. 8.90 ± 1.04 mg L−1), and 2.8 times greater than the reduction in ammonium N under the NO3−-N + NH4+-N treatment (8.83 ± 1.31 mg L−1 vs. 3.17 ± 0.54 mg L−1) during 0–72 h. The results indicated that the M6-3 preferred to absorb ammonium N, and M7-15 preferred to absorb nitrate N (Figure 3). Previous studies on inorganic N utilization have often found that ammonium N is more preferentially immobilized by microorganisms than nitrate N (Christie and Wasson, 2001; Geisseler et al., 2010), possibly because the lower energy requirement for ammonium N assimilation into microbial cells (Murphy et al., 2003), and possibly due to ammonium N can inhibit nitrate N assimilation. Thus, ammonium appears to be a more readily available direct source of nitrogen for microorganisms, while the corresponding nitrate is more difficult to utilize directly. But some studies have revealed a higher microbial demand for nitrate in grassland and forest soil, which may be attributed to nitrogen limitation resulting from low NH4+ concentrations in the soil (Hatch et al., 2000; Song et al., 2025). In the absence of or low levels of ammonium concentration within the growth environment, bacterial nitrate removal rates can reach up to 82% (Burger and Jackson, 2003; Huang et al., 2021).
Previous research has demonstrated that the preference of microorganisms for ammonium or nitrate is contingent upon their species and growth conditions (Bengtsson et al., 2003; Huang et al., 2021; Li et al., 2017). In addition to growth conditions, changes in the strains themselves will have a more direct impact on their preference for ammonium or nitrate, so the study investigates the underlying reasons for this preference by examining the characteristics and gene transcription differences among microbial strains.
4.2 Transcriptional difference and metabolic mechanism of two strains under different inorganic nitrogen
The nitrogen metabolism pathways were similar between Burkholderia sp. M6-3 and Arthrobacter sp. M7-15, except for the NtrC family genes in a two-component system, which serves as the regulators of nitrogen assimilation (North et al., 2023). Both strains contained nitrate/nitrite transport and reduction genes and organic substance degradation and synthesis genes, including NrT/NrTA, nasA/B, nirB/D, gdhA/GDH2, glnA, and gltB/D (Tu et al., 2017; Tu et al., 2019). In this study, nitrate/nitrite is converted to NH4+ through transport and reduction regulated by the genes NrT/NrTA, nasA/B, and nirB/D. Additionally, NH4+ can also be directly transported into the cell via AmtB. In the NH4+ assimilation pathway, it was found that the expression of genes encoding GDH was generally at a low level, and both M6-3 and M7-15 mainly used the GS–GOGAT pathway for ammonium utilization rather than the GDH pathway (Figure 6), even though more energy expenditure required (Wu et al., 2016). In addition, under ammonium-limited conditions, the ammonium transporter (AmtB) can capture extracellular NH4+ into the cell through active transport, but this process is subject to strict regulation by the nitrogen regulatory system (Liu et al., 2020).

Figure 6. The expression levels of major nitrogen metabolism genes in Burkholderia sp. M6-3 (A) and Arthrobacter sp. M7-15 (B) under different inorganic nitrogen treatment.
The presence of NH4+ significantly repressed the expression of nitrate and nitrite transport and reduction genes, and the ability to utilize nitrate of microbial cells under high concentrations of NH4+ will be greatly repressed (Flores et al., 2005; Martínez-Espinosa et al., 2007). In both strains M6-3 and M7-15, compared to NH4+-N treatments, genes Nrt/NrtA, nasAB, and nirBD were significantly up-regulated in treatments where only NO3−-N was present (Figures 5A,B), and they were significantly down-regulated in NO3−-N + NH4+-N treatment compared to NO3−-N treatment (Figures 5C,D). In M6-3, NH4+ severely inhibited the expression of the genes for nitrate utilization in the treatment of ammonium-nitrate coexistence, while no other response occurred for other nitrogen assimilation genes, which resulted in a preferential utilization of ammonium over nitrate by M6-3.
However, different from M6-3, in the case of the same inhibition by NH4+, the glutamine synthetase gene glnA in M7-15 gave feedback to this inhibition, causing this strain to assimilate more nitrate. Gene glnA in M7-15 was significantly up-regulated in NO3−-N treatment compared with NH4+-N (Figure 5B), which was significantly down-regulated in NO3−-N + NH4+-N treatment compared with NO3−-N (Figure 5D). This may be one of the reasons why the assimilation of M7-15 to nitrate N is higher than that to ammonium N. The glnA gene encodes glutamine synthetase, which is the central enzyme for nitrogen assimilation. Glutamine is a significant nitrogen source that is required for the organism (Zhang L. J. et al., 2020; Zhang W. et al., 2020), which not only provides glutamine for biosynthesis, but also cooperates with GOGAT to assimilate ammonium (Martínez-Espinosa et al., 2006). Studies have shown that glutamate synthetase and glutamine synthetase enabled the growth of strain in a medium with low ammonium concentration or only nitrate as a single nitrogen source (Pire et al., 2014), and glnA and gltBD genes were important genes for the growth of the strain in pure nitrate nitrogen medium. Therefore, the transcriptional expression of the strains themselves was an important factor in their response to the variable environment (Wang et al., 2022), and nitrogen-assimilating strains show different preferences in the face of different inorganic nitrogen sources by regulating the transcriptional expression of their functional genes. The glnA gene was significantly up-regulated in NO3−-N treatment, resulting in more nitrogen sources being utilized. Other researchers have also found a significant advantage in the expression of the glnA gene within a nitrate-preferred strain of Bacillus megaterium (You et al., 2022). The gene glnA seems to be the key factor for the difference in ammonium or nitrate preferences by strain M7-15. The difference in gene glnA expression in the two strains might be related to the nitrogen two-component system, and further investigation revealed a large difference in the nitrogen regulatory factors of the two strains.
Within the NtrB-NtrC two-component system, abundant nitrogen regulatory factors annotated to M6-3, including GlnD, GlnB, GlnK, GlnL (ntrB), and GlnG (ntrC), among which GlnB and GlnK, as members of the PII protein family, act as sensors of cell nitrogen status (Coutts et al., 2002). At low concentrations of NH4+, GlnK is fully uridylylated, allowing AmtB to actively transport NH4+. Meanwhile, GlnK/GlnB signals to the GlnL and GlnG layers for regulation of gene glnA expression. At higher NH4+ concentrations, however, GlnK binds to AmtB and inactivates it, rendering it unable to transport NH4+ (Thomas et al., 2000; Arcondéguy et al., 2001). In M7-15, no complete two-component regulatory system genes were identified, but a nitrogen state sensor GlnB, and GlnR, functioning as a global regulator involved in nitrogen metabolism (Wang et al., 2014), were isolated, potentially regulating the transcription of glnA and may even simultaneously regulate nitrite reduction (Tiffert et al., 2008). Researchers have found that during cell growth, glnA is transcribed by different promoters depending upon the different nitrogen utilization pathways of cell growth (Kramer et al., 1996). And Wang et al. (2022) found that the response of bacterial communities to changes in survival conditions was mainly due to significant changes in the transcriptional patterns of different genes. Therefore, the difference in glnA transcription under ammonium or nitrate treatments contributes to the preference of strain M7-15 for nitrate.
4.3 Limitations and implications
This study advances our understanding of microbial nitrogen source utilization by combining the phenotypic growth characteristics of Burkholderia sp. M6-3 and Arthrobacter sp. M7-15 with whole-genome and transcriptomic analyses. The dynamic transcriptional response was elucidated by RNA sequencing, and the molecular mechanism of the nitrogen source preference strategy was revealed. While our study provides novel insights into microbial nitrogen preference mechanisms, we acknowledge several limitations in the experimental design. Firstly, the current work relies on pure culture experiments under controlled laboratory conditions, without considering the influence of environmental factors (such as pH) on the metabolism of strains (Fuess et al., 2019), nor can it fully summarize the complex interactions and environmental fluctuations existing in natural soil ecosystems (Zengler and Zaramela, 2018). Combining microcosm experiments or in-situ verification will help explore the metabolic differences of microorganisms towards ammonium N and nitrate N in complex environments, which may be the direction of our further research. Second, although three biological replicates were included for transcriptomic analysis, the use of pooled RNA samples (equal proportions of three replicates) may have averaged out subtle expression variations among individual samples, potentially masking strain-specific responses. However, GFOLD was selected for this study due to its unique strengths in handling single-sample data. Unlike traditional methods that rely on replicate-based variance estimation, GFOLD calculates a generalized fold change (GFC) using posterior distributions of log fold changes, which effectively accounts for uncertainty in single-replicate datasets (Feng et al., 2012). This approach has been extensively validated in studies with limited biological replicates (Huang et al., 2024; Sheikh et al., 2024), where GFOLD outperformed other methods in ranking DEGs under similar conditions. Notably, GFOLD’s conservative ranking of DEGs reduces false positives while maintaining biological relevance, which is critical for studies with sparse data. The researchers demonstrated the robustness of GFOLD in data analysis despite the constraint of single biological replicates (Sheikh et al., 2024). The coincidence between the transcriptome results and the inorganic nitrogen preference characteristics of the strains also proved the reliability of the results.
Despite these limitations, our study establishes a foundational framework for understanding strain-specific nitrogen preference and highlights key regulatory genes (e.g., glnA) that warrant further exploration. These findings provide critical guidance for designing targeted experiments to unravel the intricate interplay between microbial genetics and nitrogen cycling in agricultural soils.
5 Conclusion
In this study, we isolated two nitrogen-assimilating bacterial strains, Burkholderia sp. M6-3 and Arthrobacter sp. M7-15, from acidic dryland red soil. The conclusion highlights key genetic and pathway insights: The differential nitrogen source preferences (NH4+-N for M6-3, NO3−-N for M7-15) are underpinned by distinct gene regulatory patterns. Notably, the glutamine synthetase-coding gene glnA emerges as a critical factor. Its significant upregulation in M7-15, absent in M6-3, correlates with M7-15’s nitrate preference. Additionally, the global regulator GlnR is proposed as a potential modulator of nitrogen metabolism gene expression, potentially explaining the glnA-related discrepancies. While both strains share similar nitrogen metabolism pathways (involving genes like Nrt/NrtA, nasAB, nirBD, AmtB, and GlnB/GlnK), regulatory variations in key genes drive their divergent preferences. These findings identify glnA and regulatory elements like GlnR as pivotal in shaping nitrogen source utilization strategies. This work strengthens the theoretical foundation for understanding microbial nitrogen preference mechanisms and offers critical clues for screening functional microorganisms to enhance inorganic nitrogen use efficiency.
Data availability statement
The datasets presented in this study can be found in online repositories. The names of the repository/repositories and accession number(s) can be found here: https://www.ncbi.nlm.nih.gov/genbank/, PRJNA891540, PRJNA891352.
Author contributions
RL: Data curation, Methodology, Visualization, Writing – original draft, Writing – review & editing, Formal analysis, Investigation, Supervision. HQ: Resources, Validation, Writing – original draft, Writing – review & editing. QW: Data curation, Formal analysis, Software, Writing – review & editing. CC: Investigation, Project administration, Resources, Writing – review & editing. YJ: Supervision, Validation, Visualization, Writing – review & editing. HD: Supervision, Validation, Visualization, Writing – review & editing. CH: Conceptualization, Supervision, Funding acquisition, Investigation, Writing – original draft, Writing – review & editing. WZ: Funding acquisition, Investigation, Resources, Supervision, Validation, Writing – review & editing.
Funding
The author(s) declare that financial support was received for the research and/or publication of this article. This work was funded by the National Natural Science Foundation of China (grant numbers 42277287, 42077033, and 42207344) and the Natural Science Research Project in Universities of Jiangsu Province (24KJB210015).
Conflict of interest
The authors declare that the research was conducted in the absence of any commercial or financial relationships that could be construed as a potential conflict of interest.
Generative AI statement
The authors declare that no Gen AI was used in the creation of this manuscript.
Publisher’s note
All claims expressed in this article are solely those of the authors and do not necessarily represent those of their affiliated organizations, or those of the publisher, the editors and the reviewers. Any product that may be evaluated in this article, or claim that may be made by its manufacturer, is not guaranteed or endorsed by the publisher.
Supplementary material
The Supplementary material for this article can be found online at: https://www.frontiersin.org/articles/10.3389/fmicb.2025.1559884/full#supplementary-material
Footnotes
References
Adachi, Y., Terakura, S., Osaki, M., Okuno, Y., Sato, Y., Sagou, K., et al. (2024). Cullin-5 deficiency promotes chimeric antigen receptor T cell effector functions potentially via the modulation of JAK/STAT signaling pathway. Nat. Commun. 15:10376. doi: 10.1038/s41467-024-54794-x
Ameer, M. J., Liu, Y., Zhao, X., Yan, S., and Qu, T. (2025). Effect of different synthetic nitrogen forms and levels on nitrification and denitrification key genes abundances: implications for oligotrophic forest soil management. Nitrogen 6:4. doi: 10.3390/nitrogen6010004
Andrade, S. L. A., and Einsle, O. (2007). The Amt/Mep/Rh family of ammonium transport proteins (review). Mol. Membr. Biol. 24, 357–365. doi: 10.1080/09687680701388423
Arcondéguy, T., Jack, R., and Merrick, M. (2001). P(II) signal transduction proteins, pivotal players in microbial nitrogen control. Microbiol. Mol. Biol. Rev. 65, 80–105. doi: 10.1128/mmbr.65.1.80-105.2001
Beeckman, F., Motte, H., and Beeckman, T. (2018). Nitrification in agricultural soils: impact, actors and mitigation. Curr. Opin. Biotechnol. 50, 166–173. doi: 10.1016/j.copbio.2018.01.014
Bengtsson, G., Bengtson, P., and Mansson, K. F. (2003). Gross nitrogen mineralization-, immobilization-, and nitrification rates as a function of soil C/N ratio and microbial activity. Soil Biol. Biochem. 35, 143–154. doi: 10.1016/S0038-0717(02)00248-1
Burger, M., and Jackson, L. E. (2003). Microbial immobilization of ammonium and nitrate in relation to ammonification and nitrification rates in organic and conventional cropping systems. Soil Biol. Biochem. 35, 29–36. doi: 10.1016/S0038-0717(02)00233-X
Chen, S., Zhou, Y., Chen, Y., and Gu, J. (2018). Fastp: an ultra-fast all-in-one FASTQ preprocessor. Bioinformatics 34, i884–i890. doi: 10.1093/bioinformatics/bty560
Christie, P., and Wasson, E. A. (2001). Short-term immobilization of ammonium and nitrate added to a grassland soil. Soil Biol. Biochem. 33, 1277–1278. doi: 10.1016/S0038-0717(00)00237-6
Coutts, G., Thomas, G., Blakey, D., and Merrick, M. (2002). Membrane sequestration of the signal transduction protein GlnK by the ammonium transporter AmtB. EMBO J. 21, 536–545. doi: 10.1093/emboj/21.4.536
Cui, J., Yu, C., Qiao, N., Xu, X., Tian, Y., and Ouyang, H. (2017). Plant preference for NH4+ versus NO3− at different growth stages in an alpine agroecosystem. Field Crop Res. 201, 192–199. doi: 10.1016/j.fcr.2016.11.009
Dong, W. L., Yang, Q., George, T. S., Yin, H. Q., Wang, S., Bi, J. J., et al. (2022). Investigating bacterial coupled assimilation of fertilizer-nitrogen and crop residue-carbon in upland soils by DNA-qSIP. Sci. Total Environ. 845:157279. doi: 10.1016/j.scitotenv.2022.157279
Fageria, N. K., and Baligar, V. C. (2005). Enhancing nitrogen use efficiency in crop plants. Adv. Agron. 88, 97–185. doi: 10.1016/S0065-2113(05)88004-6
Feng, J., Meyer, C. A., Wang, Q., Liu, J. S., Shirley Liu, X., and Zhang, Y. (2012). GFOLD: a generalized fold change for ranking differentially expressed genes from RNA-seq data. Bioinformatics 28, 2782–2788. doi: 10.1093/bioinformatics/bts515
Flores, E., Frías, J. E., Rubio, L. M., and Herrero, A. (2005). Photosynthetic nitrate assimilation in cyanobacteria. Photosynth. Res. 83, 117–133. doi: 10.1007/s11120-004-5830-9
Fuess, L. T., Zaiat, M., and do Nascimento, C. A. O. (2019). Novel insights on the versatility of biohydrogen production from sugarcane vinasse via thermophilic dark fermentation: impacts of pH-driven operating strategies on acidogenesis metabolite profiles. Bioresour. Technol. 286:121379. doi: 10.1016/j.biortech.2019.121379
Gao, Y., Zhang, Q., Chen, Y., Yang, Y., Zhou, C., Yu, J., et al. (2025). Ammonia-assimilating bacteria promote wheat (Triticum aestivum) growth and nitrogen utilization. Microorganisms 13:43. doi: 10.3390/microorganisms13010043
Geisseler, D., Horwath, W. R., Joergensen, R. G., and Ludwig, B. (2010). Pathways of nitrogen utilization by soil microorganisms-A review. Soil Biol. Biochem. 42, 2058–2067. doi: 10.1016/j.soilbio.2010.08.021
González, P. J., Correia, C., Moura, I., Brondino, C. D., and Moura, J. J. G. (2006). Bacterial nitrate reductases: molecular and biological aspects of nitrate reduction. J. Inorg. Biochem. 100, 1015–1023. doi: 10.1016/j.jinorgbio.2005.11.024
Guo, Z., Zhang, T., Li, X., Wang, Q., Xu, J., Yu, H., et al. (2005). Towards precise classification of cancers based on robust gene functional expression profiles. BMC Bioinformatics 6:58. doi: 10.1186/1471-2105-6-58
Haas, B. J., Gevers, D., Earl, A. M., Feldgarden, M., Ward, D. V., Giannoukos, G., et al. (2011). Chimeric 16S rRNA sequence formation and detection in sanger and 454-pyrosequenced PCR amplicons. Genome Res. 21, 494–504. doi: 10.1101/gr.112730.110
Hatch, D. J., Jarvis, S. C., Parkinson, R. J., and Lovell, R. D. (2000). Combining field incubation with nitrogen-15 labelling to examine nitrogen transformations in low to high intensity grassland management systems. Biol. Fertil. Soils 30, 492–499. doi: 10.1007/s003740050028
Hildebrand, M. (2005). Cloning and functional characterization of ammonium transporters from the marine diatom Cylindrotheca fusiformis (Bacillariophyceae). J. Phycol. 41, 105–113. doi: 10.1111/j.1529-8817.2005.04108.x
Huang, X., Jiang, D., Ni, J., Xie, D., and Li, Z. (2021). Removal of ammonium and nitrate by the hypothermia bacterium Pseudomonas putida Y-9 mainly through assimilation. Environ. Technol. Innov. 22:101458. doi: 10.1016/j.eti.2021.101458
Huang, Y. H., Qiu, Y. R., Zhang, Q. L., Cai, M. C., Yu, H., Zhang, J. M., et al. (2024). Genomic and transcriptomic profiling of peripheral T cell lymphoma reveals distinct molecular and microenvironment subtypes. Cell Rep. Med. 5:101416. doi: 10.1016/j.xcrm.2024.101416
Jayakumar, V., and Sakakibara, Y. (2019). Comprehensive evaluation of non-hybrid genome assembly tools for third-generation PacBio long-read sequence data. Brief. Bioinform. 20, 866–876. doi: 10.1093/bib/bbx147
Jin, P., Chen, Y., Yao, R., Zheng, Z., and Du, Q. (2019). New insight into the nitrogen metabolism of simultaneous heterotrophic nitrification-aerobic denitrification bacterium in mRNA expression. J. Hazard. Mater. 371, 295–303. doi: 10.1016/j.jhazmat.2019.03.023
Kanehisa, M., and Goto, S. (2000). KEGG: Kyoto encyclopedia of genes and genomes. Nucleic Acids Res. 28, 27–30. doi: 10.1093/nar/28.1.27
Kaštovská, E., and Šantrůčková, H. (2011). Comparison of uptake of different N forms by soil microorganisms and two wet-grassland plants: A pot study. Soil Biol. Biochem. 43, 1285–1291. doi: 10.1016/j.soilbio.2011.02.021
Kramer, J. G., Wyman, M., Zehr, J. P., and Capone, D. G. (1996). Diel variability in transcription of the structural gene for glutamine synthetase (glnA) in natural populations of the marine diazotrophic cyanobacterium Trichodesmium thiebautii. FEMS Microbiol. Ecol. 21, 187–196. doi: 10.1016/S0168-6496(96)00055-4
Langmead, B., and Salzberg, S. L. (2012). Fast gapped-read alignment with bowtie 2. Nat. Methods 9, 357–359. doi: 10.1038/nmeth.1923
Ledergerber, C., and Dessimoz, C. (2011). Base-calling for next-generation sequencing platforms. Brief. Bioinform. 12, 489–497. doi: 10.1093/bib/bbq077
Li, B., and Dewey, C. N. (2011). RSEM: accurate transcript quantification from RNA-Seq data with or without a reference genome. BMC Bioinformatics 12:323. doi: 10.1186/1471-2105-12-323
Li, Y., Wang, Y., Fu, L., Gao, Y., Zhao, H., and Zhou, W. (2017). Aerobic-heterotrophic nitrogen removal through nitrate reduction and ammonium assimilation by marine bacterium Vibrio sp. Y1-5. Bioresour. Technol. 230, 103–111. doi: 10.1016/j.biortech.2017.01.049
Liu, R., Jiang, Y. B., Deng, H., Zhang, Y. P., Han, C., and Zhong, W. H. (2023). Isolation and whole genome analysis of bacterial strains assimilating inorganic nitrogen in acidic dryland red soils. Acta Microbiol Sin. 63, 3157–3172. doi: 10.13343/j.cnki.wsxb.20220889
Liu, G., Vijayaraman, S. B., Dong, Y., Li, X., Andongmaa, B. T., Zhao, L., et al. (2020). Bacillus velezensis LG37: transcriptome profiling and functional verification of GlnK and MnrA in ammonia assimilation. BMC Genomics 21:215. doi: 10.1186/s12864-020-6621-1
Lledó, B., Marhuenda-Egea, F. C., Martínez-Espinosa, R. M., and Bonete, M. J. (2005). Identification and transcriptional analysis of nitrate assimilation genes in the halophilic archaeon Haloferax mediterranei. Gene 361, 80–88. doi: 10.1016/j.gene.2005.07.011
Mara, P., Fragiadakis, G. S., Gkountromichos, F., and Alexandraki, D. (2018). The pleiotropic effects of the glutamate dehydrogenase (GDH) pathway in Saccharomyces cerevisiae. Microb. Cell Factories 17:170. doi: 10.1186/s12934-018-1018-4
Martínez-Espinosa, R. M., Esclapez, J., Bautista, V., and Bonete, M. J. (2006). An octameric prokaryotic glutamine synthetase from the haloarchaeon Haloferax mediterranei. FEMS Microbiol. Lett. 264, 110–116. doi: 10.1111/j.1574-6968.2006.00434.x
Martínez-Espinosa, R. M., Lledó, B., Marhuenda-Egea, F. C., and Bonete, M. J. (2007). The effect of ammonium on assimilatory nitrate reduction in the haloarchaeon Haloferax mediterranei. Extremophiles 11, 759–767. doi: 10.1007/s00792-007-0095-9
McGuire, K. L., and Treseder, K. K. (2010). Microbial communities and their relevance for ecosystem models: decomposition as a case study. Soil Biol. Biochem. 42, 529–535. doi: 10.1016/j.soilbio.2009.11.016
Metzker, M. L. (2010). Sequencing technologies — the next generation. Nat. Rev. Genet. 11, 31–46. doi: 10.1038/nrg2626
Morrissey, E. M., Mau, R. L., Schwartz, E., Koch, B. J., Hayer, M., and Hungate, B. A. (2018). Taxonomic patterns in the nitrogen assimilation of soil prokaryotes. Environ. Microbiol. 20, 1112–1119. doi: 10.1111/1462-2920.14051
Murphy, D. V., Recous, S., Stockdale, E. A., Fillery, I. R. P., Jensen, L. S., Hatch, D. J., et al. (2003). Gross nitrogen fluxes in soil: theory, measurement and application of 15N pool dilution techniques. Adv. Agron. 79, 69–118. doi: 10.1016/S0065-2113(02)79002-0
North, H., McLaughlin, M., Fiebig, A., and Crosson, S. (2023). The Caulobacter NtrB-NtrC two-component system bridges nitrogen assimilation and cell development. J Bacteriol. 205:e0018123. doi: 10.1128/jb.00181-23
Pan, J., Sun, Y., Yao, W., Mao, H., Zhang, Y., and Zhu, M. (2017). Complete genome sequence of the Vibrio vulnificus strain VV2014DJH, a human-pathogenic bacterium isolated from a death case in China. Gut Pathog. 9:67. doi: 10.1186/s13099-017-0216-7
Pan, W., Zhou, J., Tang, S., Wu, L., Ma, Q., Marsden, K. A., et al. (2023). Utilisation and transformation of organic and inorganic nitrogen by soil microorganisms and its regulation by excessive carbon and nitrogen availability. Biol. Fertil. Soils 59, 379–389. doi: 10.1007/s00374-023-01712-w
Patil, S. V., Mohite, B. V., Patil, C. D., Koli, S. H., Borase, H. P., and Patil, V. S. (2020). “Azotobacter” in Beneficial microbes in agro-ecology. eds. N. Amaresan, K. Annapurna, K. Kumar, M. Senthil Kumar, and A. Sankaranarayanan (Amsterdam: Academic Press), 397–426.
Pire, C., Martínez-Espinosa, R. M., Pérez-Pomares, F., Esclapez, J., and Bonete, M. J. (2014). Ferredoxin-dependent glutamate synthase: involvement in ammonium assimilation in Haloferax mediterranei. Extremophiles 18, 147–159. doi: 10.1007/s00792-013-0606-9
Richardson, D. J., Berks, B. C., Russell, D. A., Spiro, S., and Taylor, C. J. (2001). Functional, biochemical and genetic diversity of prokaryotic nitrate reductases. Cell. Mol. Life Sci. 58, 165–178. doi: 10.1007/PL00000845
Rochester, I., Constable, G., and Macleod, D. A. (1992). Preferential nitrate immobilization in alkaline soils. Soil Res. 30, 737–749. doi: 10.1071/SR9920737
Schimel, J. (1995). “Ecosystem consequences of microbial diversity and community structure” in Arctic and alpine biodiversity: Patterns, causes and ecosystem consequences. eds. F. S. Chapin and C. Körner (Berlin: Springer-Verlag Press), 239–254.
Sheikh, S., Fu, C. J., Brown, M. W., and Baldauf, S. L. (2024). The Acrasis kona genome and developmental transcriptomes reveal deep origins of eukaryotic multicellular pathways. Nat. Commun. 15:10197. doi: 10.1038/s41467-024-54029-z
Soneson, C., Love, M. I., and Robinson, M. D. (2015). Differential analyses for RNA-seq: transcript-level estimates improve gene-level inferences. F1000Res 4:1521. doi: 10.12688/f1000research.7563.2
Song, L., Liao, J., Ma, F., Wang, S., Yan, Y., Chen, C., et al. (2025). Nitrogen additions increase soil microbial nitrate- rather than ammonium- immobilization. Biol. Fertil. Soils. doi: 10.1007/s00374-025-01896-3
Stevens, C. J. (2019). Nitrogen in the environment. Science 363, 578–580. doi: 10.1126/science.aav8215
Sun, L., Yu, D., Wu, Z., Wang, C., Yu, L., Wei, A., et al. (2019). Comparative transcriptome analysis and expression of genes reveal the biosynthesis and accumulation patterns of key flavonoids in different varieties of zanthoxylum bungeanum leaves. J. Agric. Food Chem. 67, 13258–13268. doi: 10.1021/acs.jafc.9b05732
Tahovská, K., Kaňa, J., Bárta, J., Oulehle, F., Richter, A., and Šantrůčková, H. (2013). Microbial N immobilization is of great importance in acidified mountain spruce forest soils. Soil Biol. Biochem. 59, 58–71. doi: 10.1016/j.soilbio.2012.12.015
Takahashi, H., Yang, G., Yoneshiro, T., Abe, Y., Ito, R., Yang, C., et al. (2022). MYPT1-PP1β phosphatase negatively regulates both chromatin landscape and co-activator recruitment for beige adipogenesis. Nat. Commun. 13:5715. doi: 10.1038/s41467-022-33363-0
Thomas, G., Coutts, G., and Merrick, M. (2000). The glnK amtB operon. A conserved gene pair in prokaryotes. Trends Genet. 16, 11–14. doi: 10.1016/s0168-9525(99)01887-9
Tiffert, Y., Supra, P., Wurm, R., Wohlleben, W., Wagner, R., and Reuther, J. (2008). The streptomyces coelicolor GlnR regulon: identification of new GlnR targets and evidence for a central role of GlnR in nitrogen metabolism in actinomycetes. Mol. Microbiol. 67, 861–880. doi: 10.1111/j.1365-2958.2007.06092.x
Tu, Q., He, Z., Wu, L., Xue, K., Xie, G., Chain, P., et al. (2017). Metagenomic reconstruction of nitrogen cycling pathways in a CO2-enriched grassland ecosystem. Soil Biol. Biochem. 106, 99–108. doi: 10.1016/j.soilbio.2016.12.017
Tu, Q., Lin, L., Cheng, L., Deng, Y., and He, Z. (2019). NCycDB: a curated integrative database for fast and accurate metagenomic profiling of nitrogen cycling genes. Bioinformatics 35, 1040–1048. doi: 10.1093/bioinformatics/bty741
Wagg, C., Bender, S. F., Widmer, F., and van der Heijden, M. G. A. (2014). Soil biodiversity and soil community composition determine ecosystem multifunctionality. Proc. Natl. Acad. Sci. USA 111, 5266–5270. doi: 10.1073/pnas.1320054111
Wang, X., Han, C., Zhang, J., Huang, Q., Deng, H., Deng, Y., et al. (2015). Long-term fertilization effects on active ammonia oxidizers in an acidic upland soil in China. Soil Biol. Biochem. 84, 28–37. doi: 10.1016/j.soilbio.2015.02.013
Wang, Y., Li, C., Duan, N., Li, B., Ding, X. M., Yao, Y. F., et al. (2014). GInR negatively regulates the transcription of the alanine dehydrogenase encoding gene ald in Amycolatopsis mediterranei U32 under nitrogen limited conditions via specific binding to its major transcription initiation site. PLoS One 9:e104811. doi: 10.1371/journal.pone.0104811
Wang, X., and Song, L. (2024). Advances in the study of NO3− immobilization by microbes in agricultural soils. Nitrogen 5, 927–940. doi: 10.3390/nitrogen5040060
Wang, L., Wang, S., and Li, W. (2012). RSeQC: quality control of RNA-seq experiments. Bioinformatics 28, 2184–2185. doi: 10.1093/bioinformatics/bts356
Wang, D., Wang, Y., Liu, L., Chen, Y., Wang, C., Li, Y.-Y., et al. (2022). Response and resilience of anammox consortia to nutrient starvation. Microbiome 10:23. doi: 10.1186/s40168-021-01212-9
Wang, X., Zhou, M., Zhu, B., Brüggemann, N., Zhang, W., and Butterbach-Bahl, K. (2024). Land use types affect soil microbial NO3− immobilization through changed fungal and bacterial contribution in alkaline soils of a subtropical montane agricultural landscape. Biol. Fertil. Soils 60, 237–252. doi: 10.1007/s00374-023-01787-5
Wawrik, B., Boling, W. B., Van Nostrand, J. D., Xie, J., Zhou, J., and Bronk, D. A. (2012). Assimilatory nitrate utilization by bacteria on the West Florida shelf as determined by stable isotope probing and functional microarray analysis. FEMS Microbiol. Ecol. 79, 400–411. doi: 10.1111/j.1574-6941.2011.01226.x
Wick, R. R., Judd, L. M., Gorrie, C. L., and Holt, K. E. (2017). Unicycler: resolving bacterial genome assemblies from short and long sequencing reads. PLoS Comput. Biol. 13:e1005595. doi: 10.1371/journal.pcbi.1005595
Williamson, G., Brito, A. S., Bizior, A., Tamburrino, G., Dias Mirandela, G., Harris, T., et al. (2022). Coexistence of ammonium transporter and channel mechanisms in Amt-Mep-Rh twin-his variants impairs the filamentation signaling capacity of fungal Mep2 transceptors. MBio 13:e0291321. doi: 10.1128/mbio.02913-21
Wu, C., Xiong, W., Dai, J., and Wu, Q. (2016). Kinetic flux profiling dissects nitrogen utilization pathways in the oleaginous green alga Chlorella protothecoides. J. Phycol. 52, 116–124. doi: 10.1111/jpy.12374
Yang, Y., Chen, L., Gu, J., Zhang, H., Yuan, J., Lian, Q., et al. (2017). Recurrently deregulated lncRNAs in hepatocellular carcinoma. Nat. Commun. 8:14421. doi: 10.1038/ncomms14421
You, Y., Chi, Y., Chen, X., Wang, J., Wang, R., Li, R., et al. (2022). A sustainable approach for bioremediation of secondary salinized soils: studying remediation efficiency and soil nitrate transformation by bioaugmentation. Chemosphere 300:134580. doi: 10.1016/j.chemosphere.2022.134580
Zengler, K., and Zaramela, L. S. (2018). The social network of microorganisms – how auxotrophies shape complex communities. Nat. Rev. Microbiol. 16, 383–390. doi: 10.1038/s41579-018-0004-5
Zhang, W., Gao, H., Huang, Y., Wu, S., Tian, J., Niu, Y., et al. (2020). Glutamine synthetase gene glnA plays a vital role in curdlan biosynthesis of Agrobacterium sp. CGMCC 11546. Int. J. Biol. Macromol. 165, 222–230. doi: 10.1016/j.ijbiomac.2020.09.152
Zhang, J., Lan, T., Müller, C., and Cai, Z. (2015). Dissimilatory nitrate reduction to ammonium (DNRA) plays an important role in soil nitrogen conservation in neutral and alkaline but not acidic rice soil. Soil Biol. Biochem. 15, 523–531. doi: 10.1007/s11368-014-1037-7
Zhang, J., Wang, J., Müller, C., and Cai, Z. (2016). Ecological and practical significances of crop species preferential N uptake matching with soil N dynamics. Soil Biol. Biochem. 103, 63–70. doi: 10.1016/j.soilbio.2016.08.009
Zhang, L. J., Xie, Y., Ding, L. Y., Qiao, X. J., and Tao, H. C. (2020). Highly efficient ammonium removal through nitrogen assimilation by a hydrogen-oxidizing bacterium, Ideonella sp. TH17. Environ. Res. 191:110059. doi: 10.1016/j.envres.2020.110059
Zhao, X. Q., Guo, S. W., Shinmachi, F., Sunairi, M., Noguchi, A., Hasegawa, I., et al. (2013). Aluminium tolerance in rice is antagonistic with nitrate preference and synergistic with ammonium preference. Ann. Bot. 111, 69–77. doi: 10.1093/aob/mcs234
Zhong, W., Gu, T., Wang, W., Zhang, B., Lin, X., Huang, Q., et al. (2010). The effects of mineral fertilizer and organic manure on soil microbial community and diversity. Plant Soil 326, 511–522. doi: 10.1007/s11104-009-9988-y
Zhu, J., Jansen-Willems, A., Müller, C., and Dörsch, P. (2021). Topographic differences in nitrogen cycling mediate nitrogen retention in a subtropical, N-saturated forest catchment. Soil Biol. Biochem. 159:108303. doi: 10.1016/j.soilbio.2021.108303
Keywords: inorganic nitrogen assimilation, nitrogen preference, bacterial strains, RNA-seq analysis, nitrogen metabolic pathway
Citation: Liu R, Qin H, Wang Q, Chu C, Jiang Y, Deng H, Han C and Zhong W (2025) Transcriptome analysis of nitrogen assimilation preferences in Burkholderia sp. M6-3 and Arthrobacter sp. M7-15. Front. Microbiol. 16:1559884. doi: 10.3389/fmicb.2025.1559884
Edited by:
Lidong Shen, Nanjing University of Information Science and Technology, ChinaReviewed by:
Khan Mohd. Sarim, Rudjer Boskovic Institute, CroatiaShuai Liu, Qingdao University of Science and Technology, China
Copyright © 2025 Liu, Qin, Wang, Chu, Jiang, Deng, Han and Zhong. This is an open-access article distributed under the terms of the Creative Commons Attribution License (CC BY). The use, distribution or reproduction in other forums is permitted, provided the original author(s) and the copyright owner(s) are credited and that the original publication in this journal is cited, in accordance with accepted academic practice. No use, distribution or reproduction is permitted which does not comply with these terms.
*Correspondence: Cheng Han, Y2hlbmdoYW5AbmpudS5lZHUuY24=
†These authors have contributed equally to this work and share first authorship