- 1Department of General Medicine, Kyorin University School of Medicine, Tokyo, Japan
- 2Department of Traumatology and Critical Care Medicine, Kyorin University School of Medicine, Tokyo, Japan
- 3Antimicrobial Resistance Research Center, National Institute of Infectious Disease, Tokyo, Japan
- 4Research Center for Drug and Vaccine Development, National Institute of Infectious Diseases, Tokyo, Japan
- 5Department of Medical Technology, Faculty of Health Sciences, Kyorin University, Tokyo, Japan
- 6Center for Data Science Education and Research, Kyorin University, Tokyo, Japan
The prevalence of extended-spectrum β-lactamase (ESBL)-producing Escherichia coli (E. coli) is a global health concern due to the multidrug antimicrobial resistance in extraintestinal pathogenic E. coli (ExPEC). ExPEC causes severe infections such as bloodstream infections, meningitis, and sepsis. Uropathogenic E. coli (UPEC), a subset of ExPEC, is responsible for urinary tract infections (UTIs), ranging from asymptomatic bacteriuria and cystitis to more severe conditions, such as pyelonephritis, bacteremia, and sepsis (urosepsis). Although ESBL-producing E. coli may have a significant impact on patient outcomes, comparisons of genotype and virulence factors between ESBL-producing and non-ESBL-producing E. coli have not fully elucidated the factors influencing its pathogenicity. Therefore, in the present study, we analyzed the genotypes and virulence-associated genes of ESBL-producing strains isolated from the blood of patients with UTIs to determine the characteristics of ESBL-producing UPEC strains associated with severe infections. Most of the clinical isolates belonged to phylogroup B2, with the exception of three strains from phylogroup D. The MLST was ST131, followed by ST73, ST95, and ST38, which are commonly found in UPEC strains. Intriguingly, ST131 strains were associated with fewer sepsis cases compared to non-ST131 strains (8 of 38 cases by ST131 and 5 of 8 cases by non-ST131 [OR, 0.16; 95% CI, 0.038–0.873; p = 0.031]). In silico analysis of 23 clinical isolates revealed that the genes detected in all strains may play a significant role in the pathogenesis of invasive UTIs. Clustering and gene locus analysis highlighted the genotype-MLST dependence of UPEC-specific virulence-associated genes. ST38-specific strains were atypical, characterized by the absence of several UPEC-specific genes, including pap loci, pathogenicity island marker (malX), and ompT, as well as the presence of genes encoding Ycb fimbriae and a Type 3 secretion system, which are typically found in enteropathogenic E. coli (EPEC). These results suggest that the virulence of clinical isolates causing invasive infections can vary, and that the pathogenicity of UPEC should be considered when analyzing the correlation between MLST and the repertoire of virulence-associated genes.
1 Introduction
Multidrug-resistant extended-spectrum β-lactamase (ESBL)-producing E. coli is often isolated from a variety of extraintestinal infections in humans, including bloodstream infection, lower respiratory tract infection, surgical site infection, meningitis, biliary tract infection (BTI), and urinary tract infection (UTI) (Dunn et al., 2019). In 2017, an estimated 197,400 individuals in the Unites States contracted infections caused by ESBL-producing Enterobacteriaceae, resulting in 9,100 deaths. The annual medical costs associated with these infections were projected to exceed 1 billion dollars (CDC, 2019). ESBL-producing E. coli is widespread in both community and healthcare settings, posing a significant public health threat due to the associated high mortality rate (Sato et al., 2024; Jernigan et al., 2020; Naghavi et al., 2024). While WHO surveillance (WHO, 2021) is ongoing, it is evident that ESBL-producing E. coli serves as both a relevant and representative indicator of the scope and trends in the AMR crisis. Moreover, it significantly contributes to human morbidity and mortality, while imposing a substantial economic burden on healthcare systems (Temkin et al., 2018). Furthermore, the use of carbapenem antibiotics and colistin, which are the last-line treatments for infections caused by ESBL-producing E. coli, has increased significantly in recent years, raising concerns about the possibility of a future lack of effective treatment options.
Uropathogenic E. coli (UPEC) is responsible for approximately 80% of UTIs (Foxman and Brown, 2003; Svanborg and Godaly, 1997), encompassing a diverse range of pathologies, from noninvasive infections such as asymptomatic bacteriuria and cystitis to more invasive UTIs such as pyelonephritis, which may progress to bacteremia and sepsis (urosepsis), potentially leading to life-threatening infections. UPEC produces a range of virulence factors that facilitate colonization of the urinary tract, including fimbriae, iron utilization, serum resistance, evasion of the innate immune response, and various toxins. These genetic factors significantly influence the clinical progression of UTIs. Analysis of virulence-related genes in the genomes of numerous clinical isolates (Abe et al., 2008; Wang et al., 2014; Kuhnert et al., 2000) reveals that the most representative UPEC-specific genes are primarily located within pathogenicity islands (Svanborg and Godaly, 1997; Shah et al., 2019; Terlizzi et al., 2017; Rezatofighi et al., 2021). However, the genes in the pathogenic islands vary through recombination and mobilization. Therefore, the complex pathogenesis of UPEC remains unclear (Rezatofighi et al., 2021).
The pathogenicity of ESBL-producing E. coli is higher than that of non-ESBL-producing E. coli (Lee et al., 2018; Naghavi et al., 2024). While the proportion of ESBL-producing E. coli isolates is increasing in both urinary tract and bloodstream infections (Ilmavirta et al., 2023), E. coli responsible for invasive UTIs remains poorly characterized (Pitout et al., 2005; O’Boyle et al., 2023). Although antibiograms guide antibiotic selection for UTIs, empiric therapy commonly employs penicillin or broad-spectrum cephalosporins with β-lactamase inhibitors, and fluoroquinolones (Edited by the Japanese Association for Infectious Diseases and the Japanese Society for Chemotherapy, 2023; Nelson et al., 2024). However, susceptibilities to these antibiotics are declining (WHO, 2022). In severe cases, cephamycins (e.g., cefmetazole), fosfomycin, or faropenem are considered, with carbapenems as the preferred first-line treatment (Edited by the Japanese Association for Infectious Diseases and the Japanese Society for Chemotherapy, 2023; Nelson et al., 2024).
For developing effective diagnostic and therapeutic strategies for infections caused by E. coli strains producing ESBLs, which are increasingly becoming difficult to treat owing to development of drug resistance, it is crucial to identify the factors associated with disease severity. Therefore, in this study, we aimed to evaluate previously reported representative UPEC specific virulence-associated genes (Johnson and Stell, 2000; Yamamoto et al., 1995) in ESBL-producing E. coli strains from bloodstream infections caused by UTIs, using PCR. In addition, we aimed to identify virulence-associated genes through genome sequencing and in silico analysis of non-ST131 and randomly selected ST131 strains.
2 Materials and methods
2.1 Cases of ESBL-producing Escherichia coli infections and clinical isolates
Between May 2017 and November 2022, ESBL-producing E. coli were prospectively collected from the blood samples as part of routine medical practices of patients visiting the Department of Emergency Medicine and Emergency General Medicine, Kyorin University Hospital. A total of 60 ESBL-producing E. coli strains were obtained and analyzed using bacterial colonies obtained from the first blood agar inoculum (stored at −80°C). Identification of ESBL-producing E. coli and drug susceptibility testing were performed using a BD Phoenix™ system (BD Diagnostics, Franklin Lakes, NJ, United States) with an NMIC/ID-441 panel and the system automatically performs assays, determines the results of MICs (Murata et al., 2023). To assess the ESBL producing-phenotype, the double-disk synergy test (DDST) was performed (CLSI [M100], 31st ed.). The first isolate from each patient was considered the primary strain, and duplicates were excluded. The primary source of infection and genotypes of the bacterial strains are shown in Table 1. After colony formation on blood agar plates from the initial blood inoculation, clinical isolates were stored at −80°C until further analysis.
Bacteremia was defined as the detection of bacteria in blood cultures using the BACT/ALERT ®3D (bioMérieux, Marcy-l’Étoile, France). Urine culture results were used solely as the basis for diagnosing UTIs, in conjunction with clinical symptoms. Bacterial detection in urine samples was conducted through routine examination by streaking of 2 μL of urine samples on sheep blood agar plates, followed by incubation at 37°C for 18 h. If the result was positive, colonies that formed on the sheep blood agar were further analyzed for E. coli identification and the ESBL-producing phenotype. Sepsis was determined based on clinical criteria: a suspected or proven focus of infection along with an acute elevation of Sequential Organ Failure Assessment score ≥2 points, which serves as a proxy for organ dysfunction, in accordance with the Japanese Clinical Practice Guidelines for Management of Sepsis and Septic Shock (2016 and 2020).
2.2 Genotyping by PCR and analysis of virulence-associated gene retention
E. coli strains were cultured in Lysogeny Broth (LB) or on LB agar at 37°C. DNA was extracted following the instructions provided with the Wizard® Genomic DNA Purification Kit (Promega, Madison, United States), according to the manufacturer’s instructions. Phylogeny determination was performed according to Clermont’s scheme (Clermont et al., 2013; Clermont et al., 2019). Representative virulence-associated genes (papAH, papGII, papGIII, cnf1, hlyA, kpsMTII, fyuA, iutA, usp. malX, traT, ompT, fimH, and csgA), commonly associated with UPEC, were detected by PCR. PCR was conducted using the primers listed in Table 2. The ST131 genotype was determined using a CicaGeneus® E. coli POT kit (Kanto Chemical Co., Ltd.), according to the manufacturer’s instructions, and negative results were considered non-ST131.
2.3 Whole-genome sequencing and genotyping: de novo assembly and annotation
Clinical isolates were cultured in LB broth at 37°C for 15 h, and genomic DNA was extracted using the Wizard® HMW DNA Extraction Kit (Promega, Madison, United States), according to the manufacturer’s instructions. Libraries were prepared using the QIAseq FX DNA Library Kit (Qiagen, Hilden, Germany) according to the manufacturer’s instructions. Paired-end sequencing was performed on the DNBSEQ platform. Sequence reads were assembled de novo into contigs using Shovill version 1.1.0.1 Genomic annotation was performed using Prokka version 1.14.6.2 Genomic data for the 23 strains were deposited in GenBank (BioProject ID: PRJDB18240).
2.4 In silico analysis
Phylogroup, MLST, serotype, as well as FimH, and FumC type were analyzed using ClermonTyping,3 MLST version 2.23.0,4 SerotypeFinder 2.0, and CHTyper 1.0 (Center for Genomic Epidemiology5), respectively.
ABRicate version 1.0.16 was used to detect virulence-associated genes, antibiotic-resistance genes, and plasmids using the default parameters. The databased used included E. coli_VF,7 Resfinder (Zankari et al., 2012; Feldgarden et al., 2019), and the National Center for Biotechnology Information (NCBI) AMRFinderPlus (Feldgarden et al., 2019). Protein or DNA sequence searches were performed using the Basic Local Alignment Search Tool (BLAST) at NCBI8 to predict gene function.
2.5 Clustering analysis
Cluster analysis, heatmap generation, and dendrograms construction were performed using Seaborn version 0.12.02 (statistical data visualization9). The reference strain for ESBL-producing ST131 UPEC included EC598 (GenBank accession No. HG941718), CFT073 (GenBank accession No. AE014075.1), UTI89 (GenBank accession No. CP000243), and 536 (GenBank accession No. CP000247). Additionally, genomes of UPEC strains from phylogroups D-38 and D-69, with assembly status from EngteroBase, were also included in the analysis (Supplementary Table S1).
2.6 Statistical analyses
Data were presented as counts and percentages, means with standard deviations (SD), or medians with interquartile ranges (25th–75th percentiles). Numerical and categorical variables were analyzed using the Student’s t-test or Fisher’s exact test. Statistical significance was set at p < 0.05. Odds ratio (OR) with 95% confidence intervals (CI) were also calculated. All statistical analyses were performed using the GraphPad Prism 8 software (GraphPad Software Inc., La Jolla, CA, United States).
3 Results
3.1 Genotypes, antibiotic resistance, and the set of virulence-associated genes of clinical isolates
Among the 60 bloodstream infections caused by ESBL-producing E. coli, 76.7% were UTIs (46 cases), with the remainder attributed to BTIs and gastrointestinal perforations (Table 1). Of the 46 UTI cases, three urine cultures were not examined due to patient conditions, and two were negative, likely due to prior antibiotic use. This study analyzed only clinical isolates from blood samples. Phylogenetic groups are summarized in Table 1. Of the UPEC strains, 43 (93.5%) belonged to phylogroup B2, while the remaining three strains were classified under phylogroup D (Table 1). Urosepsis was observed in 8 of 38 cases infected with ST131 strains and in 5 of 8 cases infected with non-ST131 strains (Table 3). The incidence was significantly lower in patients with ST131 infections compared to those with non-ST131 infections [OR, 0.16; 95% CI, 0.038–0.873; p = 0.031]. All strains were susceptible to imipenem/cilastatin, meropenem, cefmetazole, and latamoxef. On the other hand, approximately 80% of the strains exhibited non-susceptibility (R or I) to fluoroquinolones (Table 4). Resistance rates for ciprofloxacin and levofloxacin were 89.6 and 87.5%, respectively, for ST131 strains, compared to 75 and 50.0%, respectively, for non-ST131 strains.
3.2 Virulence-associated genes in clinical isolates analyzed by PCR
Several factors related to colonization, iron acquisition, and serum resistance contribute to UPEC pathogenicity (Terlizzi et al., 2017). We selected key UPEC virulence-associated genes for assessment via PCR (Table 5; Supplementary Table S2). The csgA, fimH, and fyuA genes were detected in all strains. In contrast, malX, usp. and ompT genes were detected in all phylogroup B2 strains but not in phylogroup D strains. Additionally, traT and iutA genes were detected in more than half of the B2 strains, while none of the phylogroup D strains tested positive for these genes. Overall, phylogroup D strains exhibited significantly fewer virulence-associated genes than phylogroup B2 strains (Table 5; Supplementary Table S2). Notably, papAH and papGII or papGIII genes, which are associated with P fimbriae (P pili) and the development of pyelonephritis (Ambite et al., 2019), were absent in all phylogroup D strains.
3.3 Whole-genome analysis and characterization of clinical isolates
Fifteen ST131 strains, randomly selected from 38 identified using the POT kit, were subjected to whole-genome analysis alongside eight non-ST131 strains. This in silico analysis focused on genotype, serotype, FimH and FumC types, resistance genes, and the presence of pathogenic genes (Table 6).
Genome analysis confirmed that the MLST results were consistent with the PCR findings, with the exception of KYE057. Although identified as ST131 using a POT kit, KYE057 was determined to belong to the ST131 lineage based on a single nucleotide substitution (16G → A) in the adk gene, and was included in the ST131 group for statistical analysis. Non-ST131 strains were classified as ST95, ST73, or ST1193 in phylogroup B2 and ST38 within phylogroup D. Consistent with previous studies (Pitout et al., 2022), the ST1193 strain exhibited a disruption of lacY due to a frameshift mutation (Supplementary Table S3). Regarding O antigen type, O25 was the most prevalent (14 strains), followed by O16 and O75, all commonly associated with UPEC. The ESBL genotypes were CTX-M27 (11 strains), CTX-M15 (7 strains), CTX-M14 (4 strains), and CTX-M8 (1 strain). Notably, the ST131-O25:H4 H30 pandemic clones were associated with CTX-M15 and CTC-M27, while the three ST38 strains were associated with CTX-M14 (Table 6).
3.4 Virulence-associated genes in clinical isolates analyzed in silico
In silico analysis of virulence genes using ABRicate (Supplementary Table S4) revealed that ST131 clinical isolates, including the UPEC ST131 type strain EC958, carried a significantly fewer number of virulence-associated genes compared to non-ST131 strains (<0.0001; Table 7). Well-known UPEC-specific genes, including ompT, usp. and malX, were detected in all phylogroup B2 strains in both PCR and in silico analyses. However, these genes were absent in the ST38 strains.
Cluster analysis of clinical isolates, including UPEC reference strains, was performed based on the presence of virulence genes, with each clade showing genotype-dependent clustering (Figure 1A). In terms of MLST-specific genes, the ST38 clinical isolates uniquely harbored genes associated with the epa (members of secondary T3SS-epa), esp (EPEC-T3SS secreted protein, T3SS-esp), ycb, and the hlyE (Figure 1B; Supplementary Table S4) genes. Notable, most of the ST131-, ST95-, ST73, and ST1193 specific genes were hypothetical (Figure 1B; Supplementary Table S4).
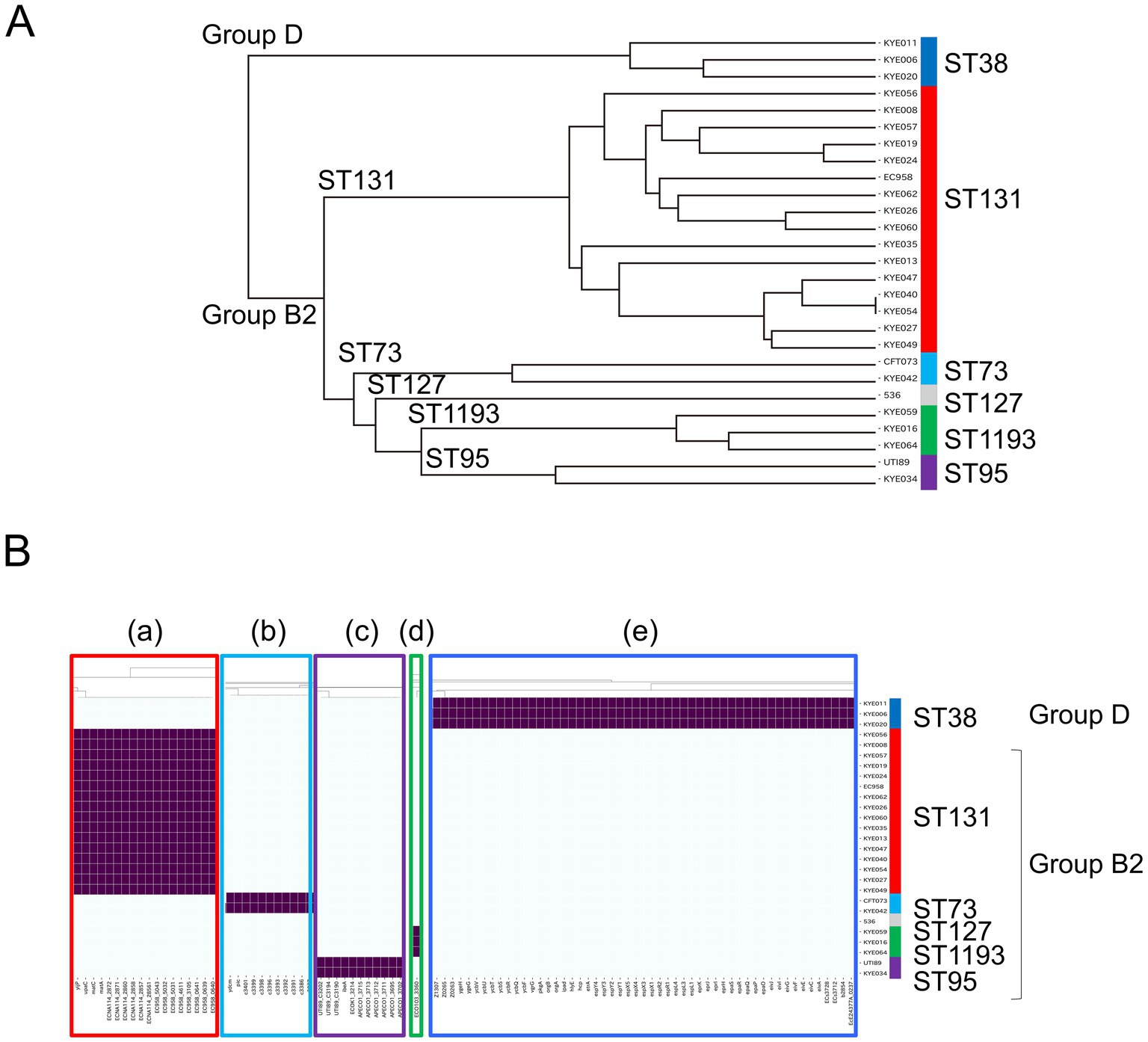
Figure 1. The ESBL-producing UPEC clinical isolates and representative UPEC strains clustered according to virulence-associated gene carriage detected by genome analysis. The presence of the gene is indicated with purple. Dendrograms of bacterial strains were deposited to distinguish the results (A). The genes specifically detected in the ST131 (a), ST73 (b), ST95 (c), ST1193 (d), and ST38 (e) strains were shown (B). Phylogroups B2 and D are indicated with Group B2 and D.
3.5 Homologs of virulence-associated genes and gene loci and their functional implications
The hypothetical genes identified within MLST-specific genes were analyzed using a BLAST search with amino acid sequences and gene order from the Prokka data. Based on the results from both ABRicate and Prokka, gene homologs or genes with multiple designations were further analyzed and annotated (Supplementary Table S5). Genes within the same operon or functional gene loci were subsequently grouped together (Supplementary Table S6).
Accordingly, T6SS gene members were found upstream and downstream of c3400, present in ST131 and other phylogroup B2 strains (Supplementary Table S7). T6SS is classified into three distinct groups: T6SS-1 to -3, based on their genetic structures. Nucleotide sequence analysis revealed that c3400 encoded tssF and tssG of T6SS-1. Other T6SS-1 gene homologs were consistently found around c3400 genes in other phylogroup B2 strains. Furthermore, the utilization of these homologs appeared to be MLST-dependent (Supplementary Table S7). The presence of T6SS-1 homologs was evalulated using the genomes of seven ST38 strains and 161 ST69 strains isolated from UTIs in EnteroBase (Supplementary Table S1). In contrast to the phylogroup B2 strains, c3400 and associated T6SS-1 homologs were absent in the genomes of ST38 and ST69 strains in EnteroBase (data not shown).
Based on the gene loci (Supplementary Tables S6, S8, S9), clustering analysis was performed, including the four well-known virulence genes (cnf1, ompT, traT, and usp; Supplementary Table S9). The T6SS apparatus requires the construction of at least 13 genes for its assembly (Journet and Cascales, 2016; Navarro-Garcia et al., 2019). As previously reported, tssM was predictably absent in ST131-O25 strains (Cummins et al., 2023) and KYE034 (ST95) (Figure 2; Supplementary Tables S7–S9).
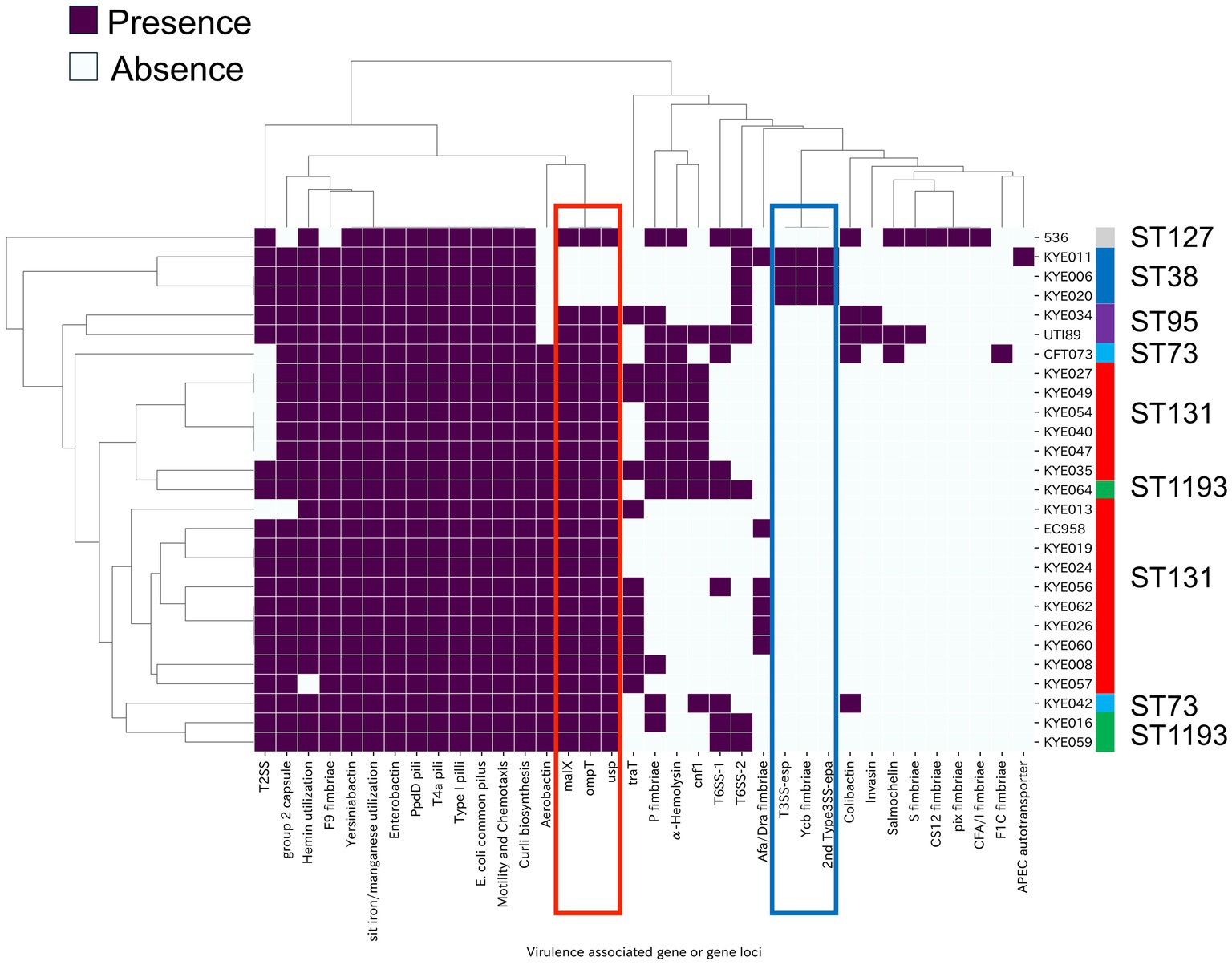
Figure 2. Hierarchical clustering of the bacteria based on the virulence-associated gene loci and genes profiles. Purple cells indicated the gene loci containing the predicted functional gene sets (Supplementary Tables S6, S8, S9). The genes that were specifically not detected in ST38 strains were indicated with a red box. The gene loci that were specifically detected in ST38 strains were indicated with a blue box.
The aec genes were predicted to be members of the T6SS-2 family (Supplementary Table S7). With the exception of ST131, more than two of the T6SS-2 gene homologs were found in all strains, including ST38 (Supplementary Table S7). However, most T6SS-2 genes were lacking in KYE042 and also in CFT073 as shown in the previous data (Journet and Cascales, 2016). Therefore, functional T6SS-2 loci were absent in both ST131 and ST73 strains (Figure 2; Supplementary Tables S7–S9). Similar to T6SS-1 genes, the utilization of T6SS-2 gene homologs appeared to be MSLT dependent (Supplementary Table S7).
P fimbria, associated with pyelonephritis and UTIs (Ambite et al., 2019; Kuehn et al., 1992; Lane and Mobley, 2007), are encoded by 11 pap (pyelonephritis-associated pili) genes (Supplementary Table S6). Whole-genome analysis revealed that none of the pap genes were present in ST38 strains. In several phylogroup B2 strains, the pap operon was disrupted by an IS insertion downstream of papI, and these strains were negative for papAH and papG genes by PCR (Supplementary Tables S2, S8). Of the 38 ST131 isolates, 10 were positive for papAH and papG, suggesting they could likely express functional P fimbriae. This proportion was significantly lower compared to the non-ST131-phylogroup B2 isolates, where 4 out of 5 were positive for these genes (10 of 38; OR, 0.089; 95% CI, 0.0071–0.7031; p = 0.032).
Functional T3SS-esp., the second T3SS-epa, Ycb fimbriae genes, and hlyE were found only in the ST38 strains (Figure 2; Table 8; Supplementary Tables S8, S9). ST69, belonging to phylogroup D, is frequently detected in UPEC strains. The presence of these genes was evaluated using the genomes of ST38 strains and ST69 strains (Supplementary Table S1). Accordingly, ycb genes were detected in all ST38 strains but were absent in the ST69 strains examined. In contrast, the second T3SS-epa, T3SS-esp genes, and hlyE were detected in all ST38 strains and in more than 90% of ST69 strains (data not shown).
Gene loci associated with attachment and colonization (type I pili, curli biosynthesis, F9 fimbriae, PpdD pili, T4a pili, E. coli common pilus), iron acquisition systems (enterobactin, yersiniabactin, and Sit iron/manganese utilization system), as well as motility, chemotaxis, and Group 2 capsules, were present in all strains except KYE013 (Figure 2; Table 8; Supplementary Table S9). Consequently, fewer secretion systems were detected in ST131 strains compared to non-ST131 strains (Table 9).
4 Discussion
The pathogenicity of ESBL-producing E. coli clinical isolates requires further elucidation; however, it remains insufficiently understood. In this study, we analyzed the genotypes and virulence-associated genes of ESBL-producing E. coli, focusing on strains that cause bloodstream infections, particularly those associated with UTIs.
Most clinical isolates were identified as ST131, with some belonging to ST73, ST95, and ST1193 in phylogroup B2. These findings are consistent with previous findings indicating that the most prevalent genotype among ESBL-producing UPEC strains consists of typical extraintestinal pathogenic E. coli (ExPEC) strains responsible for bloodstream infections (Clermont et al., 2013; Manges et al., 2019). ST73 and ST95 (Masui et al., 2022), known as “classic STs,” are highly prevalent in ExPEC, particularly when sample selection does not focus on AMR phenotypes (Gibreel et al., 2012; Yamaji et al., 2018; Fibke et al., 2019; Li et al., 2023). These STs are frequently associated with UTIs and bloodstream infections (Riley, 2014). ST1193, an emerging clone (Johnson et al., 2019), is linked to outbreaks and is imitating ST131 in terms of prevalence (Pitout et al., 2022; Tchesnokova et al., 2019). Although previous antimicrobial treatments may introduce bias, the high resistance rates observed in ST131 strains highlight the need for alternative therapeutic strategies (Table 4). ST38 is among the top 10 human pandemic lineages (Roy Chowdhury et al., 2023); however, it has been poorly investigated, with the exception of comprehensive phylogenomic analyses (Manges et al., 2019).
For the analysis of representative UPEC-specific genes by PCR, primers were designed for the kpsMT, papAH, and papG genes using homologous regions from available genomes (data not shown). The primers toned to be meticulously designed, considering the polymorphisms observed in bacterial surface protein genes. Accordingly, only three genes were detected in all the strains, while nine were not detected in the ST38 strain by PCR (Table 5; Supplementary Table S2). These results were somewhat contradictory, given the involvement of ST38 strains in invasive infections, prompting us to conduct whole-genome and in-silico analyses. The number of virulence-associated genes in ST38 strains was similar to that in the non-ST131 phylogroup B2 strains, and higher than that in the ST131 strains (Table 7; Supplementary Table S4). Further annotation and identification of gene loci to clarify the repertoire of virulence-associated genes highlighted the specificity of ST38 (Figure 2).
papGII has been identified as the only gene associated with invasive infections and severe UTIs (Lane and Mobley, 2007; Biggel et al., 2020). Despite the disruption of the locus in several B2 strains by IS, the P fimbriae gene was detected in all B2 strains. It is possible that some pap genes may have been lost during evolution, or that pap-deficient genes may have been horizontally transmitted in these strains. The ST38 strains were distinguished from the B2 clinical isolates based on the absence of P fimbriae genes and the presence of several EPEC genes (Figure 1B; Supplementary Tables S4, S8). These findings suggest that the ST38 strain, along with B2 strains that have incomplete pap genes, may rely on alternative factors for invasive infection.
The ycb operon has been found in some E. coli strains, but not all, and is present as a hidden system in the nonpathogenic E. coli strain K12 (Korea et al., 2010; Hsiao et al., 2016). The ycb operon plays a critical role in E. coli entry into HCT-8 cells, a human ileocecal epithelial cell line (Hsiao et al., 2016). Further gene function analyses will allow for further elucidation of Ycb expression and function in ST38 strains.
T6SS is a secretion system found in Gram-negative bacteria that is often associated with pathogenic strains and absent in nonpathogenic strains (Navarro-Garcia et al., 2019). Although the structure of the apparatus is conserved, the T6SS effector proteins vary. The T6SS in E. coli plays a role in the toxicity to bacterial and eukaryotic cells (Journet and Cascales, 2016).
Meanwhile, in silico analysis revealed the presence of the T6SS-1 gene locus near c3400 in all phylogroup B2 strains, but not in the ST38 strains (see Supplementary Tables S4, S7, S8). T6SS-1 is linked to enteroaggregative E. coli (EAEC) and avian pathogenic E. coli (Journet and Cascales, 2016), even though it is absent in phylogroup D strains, as observed in avian pathogenic E. coli (Tantoso et al., 2022). Overall, it is likely that several T6SS-1 genes are absent in the trains evaluated in the present study. Previous data have shown that the deletion of T6SS-1 genes in CFT073 do not affect colonization in the bladders and kidneys of CBA/J mice (Lloyd et al., 2009). Further analysis of T6SS expression is needed to understand the biological roles of these gene loci.
The functional T6SS-2 loci were found in all strains except for ST131 and ST73. E. coli T6SS-2 was found in EAEC and linked to phylogroups D to F, but not to B2 (Chen et al., 2021). Consistent with previous studies, T6SS-2 was also detected in ST95 strains (Tantoso et al., 2022). According to Prokka analysis, genes encoding Rhs-family proteins, predicted to be effector proteins, were found upstream of the vgrG gene in ST38 strains (data not shown) (Günther et al., 2022), suggesting the utilization of different effectors in these and other B2 strains. T6SS-2 affects colonization, survival, and invasion (Zhou et al., 2012). Consequently, the absence of the T6SS-2 locus in ST131 may have reduced its invasiveness.
Since the E. coli_VF database contains more virulence genes than the VFDB database, we used the E. coli_VF database for our analysis. The ST131 strains had fewer virulence-associated genes, particularly secretion system genes, compared to the non-ST131 strains (Table 9). ST131 strains are recognized as virulent UPEC clones, and are often isolated from invasive infections (Biggel et al., 2020). In contrast, ST131 isolates showed lower virulence in mouse models than other isolates (Johnson et al., 2012). In the present study, a lower frequency of sepsis cases was observed for ST131 strains, with the secretion system genes—associated with the pathogenicity of EPEC—being less abundant in the most prevalent genotype, ST131. This finding correlates with the number of sepsis cases, even with limited clinical isolates. Although the relationship between the number of virulence genes and disease severity remains unclear (Merino et al., 2020), the number of virulence-associated genes were affected by secretion system genes (Table 9).
The increase in ESBL-producing E. coli can be attributed to the growing difficulty in treating UTIs, which complicates patient management (Foxman and Brown, 2003; Flores-Mireles et al., 2015). In the present study, the repertoires of virulence genes were found to be associated with MLST, with notable differences between ST38 strains and other B2 group strains. ST38 NDM-5-producing E. coli isolates have caused an outbreak in the Czech Republic (Chudejova et al., 2024). Genomic characterization and pathogenicity of clinical ST38 isolates are essential to monitor future trends. Moreover, ST131 strains revealed a lower frequency of virulence-associated genes than the other strains, with fewer secretion system genes. These results corroborate previous findings and suggest that the pathogenicity may vary among ESBL-producing UPEC strains causing invasive infections. To our knowledge, this study is the first to suggest that ST131 strains may exhibit lower pathogenicity than non-ST131 strains, based on the analysis of the number of virulence-associated genes and clinical data. However, the limited number of strains—all collected from the suburbs of Tokyo—necessitates a cautious interpretation of these findings. Future studies employing larger and geographically diverse strains, together with comprehensive clinical data, can definitively assess this relationship. Overall, the diversity in virulence of ESBL-producing UPEC strains causing severe infections highlights the need for further investigations to develop more effective treatment strategies.
Data availability statement
The datasets presented in this study can be found in online repositories. The names of the repository/repositories and accession number(s) can be found in the article/Supplementary material.
Ethics statement
The studies involving humans were approved by Faculty of Medicine Research Ethics Committee, Kyorin University. The studies were conducted in accordance with the local legislation and institutional requirements. The participants provided their written informed consent to participate in this study.
Author contributions
MT: Conceptualization, Writing – original draft, Writing – review & editing, Formal analysis, Investigation, Methodology. TH: Conceptualization, Funding acquisition, Methodology, Project administration, Supervision, Writing – original draft, Writing – review & editing. TS: Formal analysis, Investigation, Resources, Writing – original draft, Writing – review & editing. YT: Formal analysis, Writing – original draft, Writing – review & editing. LM: Formal analysis, Methodology, Writing – original draft, Writing – review & editing. KKo: Data curation, Formal analysis, Methodology, Visualization, Writing – original draft, Writing – review & editing. AA: Data curation, Formal analysis, Methodology, Writing – original draft, Writing – review & editing. KKi: Methodology, Writing – original draft, Writing – review & editing. SY: Investigation, Methodology, Resources, Writing – original draft, Writing – review & editing. RY: Formal analysis, Investigation, Writing – original draft, Writing – review & editing. TO: Data curation, Formal analysis, Methodology, Writing – original draft, Writing – review & editing. TM: Funding acquisition, Methodology, Project administration, Supervision, Writing – original draft, Writing – review & editing, Conceptualization.
Funding
The author(s) declare that financial support was received for the research and/or publication of this article. This work was supported by JSPS KAKENHI grant nos. 22K09535 and 24K11640.
Acknowledgments
We thank Prof. T. Sato for the assistance with the statistical analysis in this research.
Conflict of interest
The authors declare that the research was conducted in the absence of any commercial or financial relationships that could be construed as a potential conflict of interest.
Generative AI statement
The author(s) declare that no Gen AI was used in the creation of this manuscript.
Publisher’s note
All claims expressed in this article are solely those of the authors and do not necessarily represent those of their affiliated organizations, or those of the publisher, the editors and the reviewers. Any product that may be evaluated in this article, or claim that may be made by its manufacturer, is not guaranteed or endorsed by the publisher.
Supplementary material
The Supplementary material for this article can be found online at: https://www.frontiersin.org/articles/10.3389/fmicb.2025.1571121/full#supplementary-material
Footnotes
1. ^https://github.com/tseemann/shovill
2. ^https://github.com/tseemann/prokka
3. ^http://clermontyping.iame-research.center
4. ^https://github.com/tseemann/mlst
5. ^https://cge.food.dtu.dk/services/SerotypeFinder/, https://cge.food.dtu.dk/services/CHTyper/
6. ^https://github.com/tseemann/abricate
7. ^https://github.com/phac-nml/Ecoli_vf
8. ^https://blast.ncbi.nlm.nih.gov/Blast.cgi?PROGRAM=blastp&PAGE_TYPE=BlastSearch&BLAST_SPEC=&LINK_LOC=blasttab&LAST_PAGE=blastn
References
Abe, C. M., Salvador, F. A., Falsetti, I. N., Vieira, M. A. M., Blanco, J., Blanco, J. E., et al. (2008). Uropathogenic Escherichia coli (UPEC) strains may carry virulence properties of diarrhoeagenic E. coli. FEMS Immunol. Med. Microbiol. 52, 397–406. doi: 10.1111/j.1574-695X.2008.00388.x
Adams-Sapper, S., Diep, B. A., Perdreau-Remington, F., and Riley, L. W. (2013). Clonal composition and community clustering of drug-susceptible and -resistant Escherichia coli isolates from bloodstream infections. Antimicrob. Agents Chemother. 57, 490–497. doi: 10.1128/AAC.01025-12
Ambite, I., Butler, D. S. C., Stork, C., Grönberg-Hernández, J., Köves, B., Zdziarski, J., et al. (2019). Fimbriae reprogram host gene expression – divergent effects of P and type 1 fimbriae. PLoS Pathog. 15:e1007671. doi: 10.1371/journal.ppat.1007671
Biggel, M., Xavier, B. B., Johnson, J. R., Nielsen, K. L., Frimodt-Møller, N., Matheeussen, V., et al. (2020). Horizontally acquired papGII-containing pathogenicity islands underlie the emergence of invasive uropathogenic Escherichia coli lineages. Nat. Commun. 11:5968. doi: 10.1038/s41467-020-19714-9
CDC (2019). Antibiotic resistance threats in the United States, 2019. Atlanta, Georgia: U.S. Department of Health and Human Services.
Chen, X., Liu, W., Li, H., Yan, S., Jiang, F., Cai, W., et al. (2021). Whole genome sequencing analysis of avian pathogenic Escherichia coli from China. Vet. Microbiol. 259:109158. doi: 10.1016/j.vetmic.2021.109158
Chudejova, K., Sourenian, T., Palkovicova, J., Stredanska, K., Nechutna, L., Vlkova, K., et al. (2024). Genomic characterization of ST38 NDM-5-producing Escherichia coli isolates from an outbreak in the Czech Republic. Antimicrob. Agents Chemother. 68:e0013324. doi: 10.1128/aac.00133-24
Clermont, O., Christenson, J. K., Denamur, E., and Gordon, D. M. (2013). The Clermont Escherichia coli phylo-typing method revisited: improvement of specificity and detection of new phylo-groups. Environ. Microbiol. Rep. 5, 58–65. doi: 10.1111/1758-2229.12019
Clermont, O., Dixit, O. V. A., Vangchhia, B., Condamine, B., Dion, S., Bridier-Nahmias, A., et al. (2019). Characterization and rapid identification of phylogroup G in Escherichia coli, a lineage with high virulence and antibiotic resistance potential. Environ. Microbiol. 21, 3107–3117. doi: 10.1111/1462-2920.14713
Cummins, E. A., Moran, R. A., Snaith, A. E., Hall, R. J., Connor, C. H., Dunn, S. J., et al. (2023). Parallel loss of type VI secretion systems in two multi-drug-resistant Escherichia coli lineages. Microb. Genom. 9:001133. doi: 10.1099/mgen.0.001133
Darvishi, M. (2016). Virulence factors profile and antimicrobial resistance of Acinetobacter baumannii strains isolated from various infections recovered from immunosuppressive patients. Biomed. Pharmacol. J. 9, 1057–1062. doi: 10.13005/bpj/1048
Desloges, I., Taylor, J. A., Leclerc, J., Brannon, J. R., Portt, A., Spencer, J. D., et al. (2019). Identification and characterization of OmpT-like proteases in uropathogenic Escherichia coli clinical isolates. Microbiology 8:e915. doi: 10.1002/mbo3.915
Dunn, S. J., Connor, C., and McNally, A. (2019). The evolution and transmission of multi-drug resistant Escherichia coli and Klebsiella pneumoniae: the complexity of clones and plasmids. Curr. Opin. Microbiol. 51, 51–56. doi: 10.1016/j.mib.2019.06.004
JAID/JSC (2023). “XI. Urinary tract Infections,” in The JAID/JSC Guide to Clinical Management of Infectious Diseases. (Japanese Association for Infectious Diseases and the Japanese Society for Chemotherapy), p280–304.
Feldgarden, M., Brover, V., Haft, D. H., Prasad, A. B., Slotta, D. J., Tolstoy, I., et al. (2019). Validating the AMRFinder tool and resistance gene database by using antimicrobial resistance genotype-phenotype correlations in a collection of isolates. Antimicrob. Agents Chemother. 63:e00483-19. doi: 10.1128/AAC.00483-19
Fibke, C. D., Croxen, M. A., Geum, H. M., Glass, M., Wong, E., Avery, B. P., et al. (2019). Genomic epidemiology of major Extraintestinal pathogenic Escherichia coli lineages causing urinary tract infections in young women across Canada. Open Forum Infect. Dis. 6:ofz431. doi: 10.1093/ofid/ofz431
Flores-Mireles, A. L., Walker, J. N., Caparon, M., and Hultgren, S. J. (2015). Urinary tract infections: epidemiology, mechanisms of infection and treatment options. Nat. Rev. Microbiol. 13, 269–284. doi: 10.1038/nrmicro3432
Foxman, B., and Brown, P. (2003). Epidemiology of urinary tract infections: transmission and risk factors, incidence, and costs. Infect. Dis. Clin. N. Am. 17, 227–241. doi: 10.1016/S0891-5520(03)00005-9
Gibreel, T. M., Dodgson, A. R., Cheesbrough, J., Fox, A. J., Bolton, F. J., and Upton, M. (2012). Population structure, virulence potential and antibiotic susceptibility of uropathogenic Escherichia coli from Northwest England. J. Antimicrob. Chemother. 67, 346–356. doi: 10.1093/jac/dkr451
Günther, P., Quentin, D., Ahmad, S., Sachar, K., Gatsogiannis, C., Whitney, J. C., et al. (2022). Structure of a bacterial Rhs effector exported by the type VI secretion system. PLoS Pathog. 18:e1010182. doi: 10.1371/journal.ppat.1010182
Hsiao, F. S.-H., Sutandy, F. R., Syu, G.-D., Chen, Y.-W., Lin, J.-M., and Chen, C.-S. (2016). Systematic protein interactome analysis of glycosaminoglycans revealed YcbS as a novel bacterial virulence factor. Sci. Rep. 6:28425. doi: 10.1038/srep28425
Ilmavirta, H., Ollgren, J., Räisänen, K., Kinnunen, T., Hakanen, A. J., Jalava, J., et al. (2023). Increasing proportions of extended-spectrum β-lactamase-producing isolates among Escherichia coli from urine and bloodstream infections: results from a nationwide surveillance network, Finland, 2008 to 2019. Euro Surveill. 28:2200934. doi: 10.2807/1560-7917.ES.2023.28.43.2200934
Jernigan, J. A., Hatfield, K. M., Wolford, H., Nelson, R. E., Olubajo, B., Reddy, S. C., et al. (2020). Multidrug-resistant bacterial infections in U.S. hospitalized patients, 2012–2017. N. Engl. J. Med. 382, 1309–1319. doi: 10.1056/NEJMoa1914433
Johnson, T. J., Elnekave, E., Miller, E. A., Munoz-Aguayo, J., Flores Figueroa, C., Johnston, B., et al. (2019). Phylogenomic analysis of Extraintestinal pathogenic Escherichia coli sequence type 1193, an emerging multidrug-resistant clonal group. Antimicrob. Agents Chemother. 63:e01913-18. doi: 10.1128/AAC.01913-18
Johnson, J. R., Porter, S. B., Zhanel, G., Kuskowski, M. A., and Denamur, E. (2012). Virulence of Escherichia coli clinical isolates in a murine Sepsis model in relation to sequence type ST131 status, fluoroquinolone resistance, and virulence genotype. Infect. Immun. 80, 1554–1562. doi: 10.1128/IAI.06388-11
Johnson, J. R., and Stell, A. L. (2000). Extended virulence genotypes of Escherichia coli strains from patients with Urosepsis in relation to phylogeny and host compromise. J. Infect. Dis. 181, 261–272. doi: 10.1086/315217
Journet, L., and Cascales, E. (2016). The type VI secretion system in Escherichia coli and related species. EcoSal Plus 7. doi: 10.1128/ecosalplus.esp-0009-2015
Korea, C., Badouraly, R., Prevost, M., Ghigo, J., and Beloin, C. (2010). Escherichia coli K-12 possesses multiple cryptic but functional chaperone–usher fimbriae with distinct surface specificities. Environ. Microbiol. 12, 1957–1977. doi: 10.1111/j.1462-2920.2010.02202.x
Kuehn, M. J., Heuser, J., Normark, S., and Hultgren, S. J. (1992). P pili in uropathogenic E. coli are composite fibres with distinct fibrillar adhesive tips. Nature 356, 252–255. doi: 10.1038/356252a0
Kuhnert, P., Boerlin, P., and Frey, J. (2000). Target genes for virulence assessment of Escherichia coli isolates from water, food and the environment. FEMS Microbiol. Rev. 24, 107–117. doi: 10.1111/j.1574-6976.2000.tb00535.x
Lane, M. C., and Mobley, H. L. T. (2007). Role of P-fimbrial-mediated adherence in pyelonephritis and persistence of uropathogenic Escherichia coli (UPEC) in the mammalian kidney. Kidney Int. 72, 19–25. doi: 10.1038/SJ.KI.5002230
Lee, C.-C., Lee, C.-H., Hong, M.-Y., Hsieh, C.-C., Tang, H.-J., and Ko, W.-C. (2018). Propensity-matched analysis of the impact of extended-spectrum β-lactamase production on adults with community-onset Escherichia coli, Klebsiella species, and Proteus mirabilis bacteremia. J. Microbiol. Immunol. Infect. 51, 519–526. doi: 10.1016/j.jmii.2017.05.006
Li, D., Elankumaran, P., Kudinha, T., Kidsley, A. K., Trott, D. J., Jarocki, V. M., et al. (2023). Dominance of Escherichia coli sequence types ST73, ST95, ST127 and ST131 in Australian urine isolates: a genomic analysis of antimicrobial resistance and virulence linked to F plasmids. Microb. Genom. 9:mgen001068. doi: 10.1099/mgen.0.001068
Lloyd, A. L., Henderson, T. A., Vigil, P. D., and Mobley, H. L. T. (2009). Genomic Islands of Uropathogenic Escherichia coli contribute to virulence. J. Bacteriol. 191, 3469–3481. doi: 10.1128/JB.01717-08
Manges, A. R., Geum, H. M., Guo, A., Edens, T. J., Fibke, C. D., and Pitout, J. D. D. (2019). Global Extraintestinal pathogenic Escherichia coli (ExPEC) lineages. Clin. Microbiol. Rev. 32:e00135-18. doi: 10.1128/CMR.00135-18
Masui, T., Nakano, R., Nakano, A., Saito, K., Suzuki, Y., Kakuta, N., et al. (2022). Predominance of CTX-M-9 group among ESBL-producing Escherichia coli isolated from healthy individuals in Japan. Microb. Drug Resist. 28, 355–360. doi: 10.1089/mdr.2021.0062
Merino, I., Porter, S. B., Johnston, B., Clabots, C., Thuras, P., Ruiz-Garbajosa, P., et al. (2020). Molecularly defined extraintestinal pathogenic Escherichia coli status predicts virulence in a murine sepsis model better than does virotype, individual virulence genes, or clonal subset among E. coli ST131 isolates. Virulence 11, 327–336. doi: 10.1080/21505594.2020.1747799
Murata, M., Kosai, K., Akamatsu, N., Matsuyama, Y., Oda, M., Wakamatsu, A., et al. (2023). Diagnostic performance of BD Phoenix CPO detect panels for detection and classification of Carbapenemase-producing gram-negative bacteria. Microbiol. Spectr. 11:e0089723. doi: 10.1128/spectrum.00897-23
Naghavi, M., Vollset, S. E., Ikuta, K. S., Swetschinski, L. R., Gray, A. P., Wool, E. E., et al. (2024). Global burden of bacterial antimicrobial resistance 1990–2021: a systematic analysis with forecasts to 2050. Lancet 404, 1199–1226. doi: 10.1016/S0140-6736(24)01867-1
Navarro-Garcia, F., Ruiz-Perez, F., Cataldi, Á., and Larzábal, M. (2019). Type VI secretion system in pathogenic Escherichia coli: structure, role in virulence, and acquisition. Front. Microbiol. 10:1965. doi: 10.3389/fmicb.2019.01965
Nelson, Z., Aslan, A. T., Beahm, N. P., Blyth, M., Cappiello, M., Casaus, D., et al. (2024). Guidelines for the prevention, diagnosis, and Management of Urinary Tract Infections in pediatrics and adults. JAMA Netw. Open 7:e2444495. doi: 10.1001/jamanetworkopen.2024.44495
O’Boyle, N., Douce, G. R., Farrell, G., Rattray, N. J. W., Schembri, M. A., Roe, A. J., et al. (2023). Distinct ecological fitness factors coordinated by a conserved Escherichia coli regulator during systemic bloodstream infection. Proc. Natl. Acad. Sci. 120:e2212175120. doi: 10.1073/pnas.2212175120
Pitout, J. D. D., Laupland, K. B., Church, D. L., Menard, M. L., and Johnson, J. R. (2005). Virulence factors of Escherichia coli isolates that produce CTX-M-type extended-Spectrum β-lactamases. Antimicrob. Agents Chemother. 49, 4667–4670. doi: 10.1128/AAC.49.11.4667-4670.2005
Pitout, J. D. D., Peirano, G., Chen, L., DeVinney, R., and Matsumura, Y. (2022). Escherichia coli ST1193: following in the footsteps of E. coli ST131. Antimicrob. Agents Chemother. 66:e0051122. doi: 10.1128/aac.00511-22
Rezatofighi, S. E., Mirzarazi, M., and Salehi, M. (2021). Virulence genes and phylogenetic groups of uropathogenic Escherichia coli isolates from patients with urinary tract infection and uninfected control subjects: a case-control study. BMC Infect. Dis. 21:361. doi: 10.1186/s12879-021-06036-4
Riley, L. W. (2014). Pandemic lineages of extraintestinal pathogenic Escherichia coli. Clin. Microbiol. Infect. 20, 380–390. doi: 10.1111/1469-0691.12646
Roy Chowdhury, P., Hastak, P., DeMaere, M., Wyrsch, E., Li, D., Elankumaran, P., et al. (2023). Phylogenomic analysis of a global collection of Escherichia coli ST38: evidence of interspecies and environmental transmission? mSystems 8:e0123622. doi: 10.1128/msystems.01236-22
Sato, T., Uemura, K., Yasuda, M., Maeda, A., Minamoto, T., Harada, K., et al. (2024). Traces of pandemic fluoroquinolone-resistant Escherichia coli clone ST131 transmitted from human society to aquatic environments and wildlife in Japan. One Health 18:100715. doi: 10.1016/J.ONEHLT.2024.100715
Shah, C., Baral, R., Bartaula, B., and Shrestha, L. B. (2019). Virulence factors of uropathogenic Escherichia coli (UPEC) and correlation with antimicrobial resistance. BMC Microbiol. 19:204. doi: 10.1186/s12866-019-1587-3
Svanborg, C., and Godaly, G. (1997). Bacterial virulence in urinary tract infection. Infect. Dis. Clin. N. Am. 11, 513–529. doi: 10.1016/S0891-5520(05)70371-8
Tantoso, E., Eisenhaber, B., Kirsch, M., Shitov, V., Zhao, Z., and Eisenhaber, F. (2022). To kill or to be killed: pangenome analysis of Escherichia coli strains reveals a tailocin specific for pandemic ST131. BMC Biol. 20:146. doi: 10.1186/s12915-022-01347-7
Tchesnokova, V. L., Rechkina, E., Larson, L., Ferrier, K., Weaver, J. L., Schroeder, D. W., et al. (2019). Rapid and extensive expansion in the United States of a new multidrug-resistant Escherichia coli clonal group, sequence type 1193. Clin. Infect. Dis. 68, 334–337. doi: 10.1093/cid/ciy525
Temkin, E., Fallach, N., Almagor, J., Gladstone, B. P., Tacconelli, E., and Carmeli, Y. (2018). Estimating the number of infections caused by antibiotic-resistant Escherichia coli and Klebsiella pneumoniae in 2014: a modelling study. Lancet Glob. Health 6, e969–e979. doi: 10.1016/S2214-109X(18)30278-X
Terlizzi, M. E., Gribaudo, G., and Maffei, M. E. (2017). UroPathogenic Escherichia coli (UPEC) infections: virulence factors, bladder responses, antibiotic, and non-antibiotic antimicrobial strategies. Front. Microbiol. 8:1566. doi: 10.3389/fmicb.2017.01566
Wang, Y., Zhao, S., Han, L., Guo, X., Chen, M., Ni, Y., et al. (2014). Drug resistance and virulence of uropathogenic Escherichia coli from Shanghai, China. J. Antibiot. (Tokyo) 67, 799–805. doi: 10.1038/ja.2014.72
WHO (2021). WHO Integrated Global Surveillance on ESBL-Producing E. coli Using a One Health Approach Implementation and Opportunities : World Health Organization.
WHO (2022). Global Antimicrobial Resistance and Use Surveillance System (GLASS) Report 2022 : World Health Organization.
Yamaji, R., Rubin, J., Thys, E., Friedman, C. R., and Riley, L. W. (2018). Persistent pandemic lineages of Uropathogenic Escherichia coli in a college community from 1999 to 2017. J. Clin. Microbiol. 56:e01834-17. doi: 10.1128/JCM.01834-17
Yamamoto, S., Terai, A., Yuri, K., Kurazono, H., Takeda, Y., and Yoshida, O. (1995). Detection of urovirulence factors in Escherichia coli by multiplex polymerase chain reaction. FEMS Immunol. Med. Microbiol. 12, 85–90. doi: 10.1111/j.1574-695X.1995.tb00179.x
Zankari, E., Hasman, H., Cosentino, S., Vestergaard, M., Rasmussen, S., Lund, O., et al. (2012). Identification of acquired antimicrobial resistance genes. J. Antimicrob. Chemother. 67, 2640–2644. doi: 10.1093/jac/dks261
Keywords: ESBL-producing Escherichia coli, uropathogenic Escherichia coli, extraintestinal pathogenic Escherichia coli, virulence-associated genes, urosepsis, bloodstream infections, ST131
Citation: Tanaka M, Hanawa T, Suda T, Tanji Y, Minh LN, Kondo K, Azam AH, Kiga K, Yonetani S, Yashiro R, Ohmori T and Matsuda T (2025) Comparative analysis of virulence-associated genes in ESBL-producing Escherichia coli isolates from bloodstream and urinary tract infections. Front. Microbiol. 16:1571121. doi: 10.3389/fmicb.2025.1571121
Edited by:
Miklos Fuzi, Independent Researcher, Seattle, WA, United StatesReviewed by:
Okon Okwong Kenneth, Federal University, Wukari, NigeriaMikaeel Young, Baylor University, United States
Saskia Camille Flament Simon, University of Santiago de Compostela, Spain
Copyright © 2025 Tanaka, Hanawa, Suda, Tanji, Minh, Kondo, Azam, Kiga, Yonetani, Yashiro, Ohmori and Matsuda. This is an open-access article distributed under the terms of the Creative Commons Attribution License (CC BY). The use, distribution or reproduction in other forums is permitted, provided the original author(s) and the copyright owner(s) are credited and that the original publication in this journal is cited, in accordance with accepted academic practice. No use, distribution or reproduction is permitted which does not comply with these terms.
*Correspondence: Tomoko Hanawa, dGhhbmF3YUBrcy5reW9yaW4tdS5hYy5qcA==; Takeaki Matsuda, dG1hdHN1ZGFAa3Mua3lvcmluLXUuYWMuanA=
†These authors have contributed equally to this work
‡ORCID: Yasunori Tanji, https://orcid.org/0009-0009-7006-3756