- 1Medical Molecular Biology Laboratory, School of Medicine, Jinhua University of Vocational Technology, Jinhua, China
- 2Key Laboratory of Medical Genetics of Zhejiang Province, Key Laboratory of Laboratory Medicine, Ministry of Education, School of Laboratory Medicine and Life Sciences, Institute of Biomedical Informatics, Wenzhou Medical University, Wenzhou, China
Background: Owing to the rapid emerging of multidrug-, even pandrug-resistant pathogens, and lack of new antibiotics, the older antibiotic, fosfomycin, has been reused in recent years in the clinical practice, especially for treatment of uropathogen infections. With the increased use of fosfomycin, bacterial resistance to it has also increased drastically. Elucidating the resistance mechanism to the antimicrobial has become an urgent task.
Methods: The putative fosfomycin resistance gene fosC3 was cloned, and minimal inhibitory concentrations were determined by the agar dilution method. Enzyme kinetic parameters were measured by high-performance liquid chromatography. Bioinformatics analysis was applied to understand the evolutionary characteristics of FosC3.
Results: The A. caviae strain DW0021 exhibited high level resistance to several antimicrobials including kanamycin, streptomycin, chloramphenicol, florfenicol, tetracycline, and especially higher to fosfomycin (> 1,024 μg/mL), while genome annotation indicated that no function-characterized resistance gene was associated with fosfomycin resistance. A novel functional gene designated fosC3 responsible for fosfomycin resistance was identified in the chromosome of A. caviae DW0021. Among the function-characterized proteins, FosC3 shared the highest amino acid similarity of 58.65% with FosC2. No mobile genetic element (MGE) was found surrounding the fosC3 gene. The recombinant pMD19-fosC3/DH5α displayed a MIC value of 32 μg/mL to fosfomycin, which revealed a 128-fold increase of MIC value to fosfomycin compared to the control pMD19/E. coli DH5α (0.25 μg/mL). FosC3 was phylogenetically close to FosC2 and exhibited a kcat and Km of 82,442 ± 1,475 s−1, 70.99 ± 4.31 μM, respectively, and a catalytic efficiency of (1.2 ± 0.3) × 103 μM−1·s−1.
Conclusion: In this work, a novel functional fosfomycin thiol transferase, FosC3, which shared the highest protein sequence similarity with FosC2, was identified in A. caviae. The fosfomycin inactivation enzyme FosC3 could effectively inactivate fosfomycin by chemical modification. It is implied that such mechanism facilitates A. caviae to respond to fosfomycin exposure, thereby enhancing survival. However, fosC3 was not related with any MGE, which differs from many other fosfomycin thiol transferase genes. As a result, fosC3 is not expected to be transmitted to other species through horizontal gene transfer mechanism. Our findings will contribute to the resistance mechanism of the common pathogenic A. caviae.
Introduction
Classified under the Gammaproteobacteria, order Aeromonadales, and family Aeromonadaceae, Aeromonas spp. are Gram-negative facultative anaerobes and isolated from numerous sources such as animals, water, soil, and food. As a result, both immunocompromised and immunocompetent humans are more likely to be infected by Aeromonas (Pessoa et al., 2022). Common diseases are gastroenteritis, bacteremia, septicemia, etc. (Fernández-Bravo and Figueras, 2020).
The wastewater discharged from hospitals and aquaculture farms is a significant source of multidrug-resistant pathogens and Aeromonas spp. in these aquatic environments exhibit a broad spectrum of antibiotic resistance profile (Pessoa et al., 2022). In addition, antibiotic resistance genes could be transmitted between Aeromonas via mobile genetic elements such as integrons and plasmids (Bello-López et al., 2019).
The resistance to fosfomycin tends to rise in the settings with use of more fosfomycin and among multi-drug resistant pathogens (Falagas et al., 2019). Three major fosfomycin resistance mechanisms are as follows: (i) reduced permeability to fosfomycin in consequence of mutated fosfomycin intake genes (uhpT etc.), (ii) mutations in the fosfomycin target gene murA, which takes part in the biosynthesis of peptidoglycan, and (iii) fosfomycin-inactivating enzymes (FosA etc.) (Zurfluh et al., 2020). Fosfomycin-inactivating enzymes are generally associated with mobile genetic elements and therefore have a critical impact in the horizontal transfer of fosfomycin resistance (Güneri et al., 2022). Antibiotic modification by fosfomycin-modifying enzymes is one of the acquired resistance mechanisms (Falagas et al., 2019). Inactivation of fosfomycin could be achieved through (i) addition of the sulphydryl group to C1 of the epoxide ring in fosfomycin (FosA and FosC2); (ii) nucleophilic addition of l-Cys or bacillithiol to fosfomycin (FosB); (iii) addition of H2O to the C1 position of epoxide ring in fosfomycin (FosX); (iv) phosphorylation of the phosphate group to monophosphate (FomA and FosC) and conversion of monophosphate to diphosphate (FomB) in fosfomycin (Falagas et al., 2019).
Up to date, at least 10 types of fos genes have been discovered, e.g., fosA, fosB, fosC, and so on (Yang et al., 2019). fosC2 is mainly responsible for fosfomycin resistance in Enterobacteriaceae (Yang et al., 2019). In this work, we identified and characterized a novel chromosome-encoded fosfomycin resistance gene designated fosC3 from a A. caviae strain DW0021.
Materials and methods
Bacterial strains and plasmids
The bacteria and plasmids used in this work were listed in Table 1. DW0021 was isolated from a soil sample at an animal farm in Wenzhou, China. The taxonomic classification analysis was a combination of 16S rRNA gene homology and whole-genome average nucleotide identity (ANI). The recommended 95% threshold of ANI was used for species delimitation (Richter and Rosselló-Móra, 2009).
Minimum inhibitory concentration determination
The antimicrobial agents used in antimicrobial susceptibility testing were in Table 2, which included streptomycin, kanamycin, chloramphenicol, florfenicol, tetracycline, fosfomycin and so on. The antimicrobial susceptibility testing was performed following the antimicrobial susceptibility testing standard M100 (34th Edition, 2024) from the Clinical and Laboratory Standards Institute.
Molecular cloning of the fosC3 gene
Primers were designed using Primer Premier1 (PREMIER Biosoft International, Palo Alto, CA) and SnapGene2 (Table 3). The open reading frame (ORF) of fosC3 with the promoter region was amplified and inserted into the T-Vector pMD19 with the T4 DNA ligase (Takara Bio, Inc., Dalian, China). The constructed recombinant plasmid was transformed into E. coli DH5α using the calcium chloride method and cultured on Luria-Bertani agar plates containing 100 μg/mL ampicillin. The inserted sequences were verified by sequencing (Shanghai Sunny Biotechnology Co., Ltd., Shanghai, China).
Expression and purification of the FosC3 enzyme
The ORF of fosC3 was PCR-amplified and inserted into the pCold I vector with the cleavage sites of thrombin, restriction endonucleases EcoRI and XbaI (Table 3). The recombinant plasmid was transformed into E. coli BL21. FosC3 was overexpressed and purified as described previously (Qing et al., 2004). After the OD600 reached 0.6 at 37°C, FosC3 enzyme induction occurred in the presence of 0.1 mM isopropyl-β-d-thiogalactoside, followed by 20 h cultivation at 16°C. Bacteria were centrifugated (5,000 × g, 10 min) at 4°C, resuspended in lysis buffer (20 mM Tris–HCl, 150 mM NaCl, 3 mM β-mercaptoethanol, 0.5% Nonidet-P-40, pH 8.0), and disintegrated by sonication. The cell debris was eliminated through centrifugation (12,000 × g, 30 min) at 4°C. Subsequently, the lysates were incubated with pre-equilibrated nickel-nitrilotriacetic acid (Ni-NTA) agarose resin (Beyotime Biotechnology, Shanghai, China) for 8 h at 4°C under slow agitation. The recombinant protein purification was achieved using standard Ni-NTA affinity chromatography. The His6 tag was removed by incubation with thrombin for 4 h at 37°C. The concentration of the purified FosC3 protein was measured by SDS-PAGE and the BCA protein assay kit (Thermo Fisher Scientific, Rockford, IL, United States).
Enzyme kinetic parameter determination of the FosC3 enzyme
The kinetic assay was based on high-performance liquid chromatography (HPLC) and the methods described previously (Rigsby et al., 2005). The components of reaction mixture were listed in Table 4. After 5 min of incubation at 37°C, the reaction was terminated by 900 μL solution contain 90 and 10% volume of mobile phase A (100 mM KH2PO3-H3PO3) and B (methanol), respectively. Then the mixture was centrifugated at 12,000 rpm for 10 min. 700 μL supernatant was injected into a 250 mm × 4.6 mm Elite C-18 column (GL Sciences, Shanghai, China) with a flow rate of 800 μL/min. Analysis was done by the Accela UHPLC system (Thermo Fisher Scientific, Rockford, IL, United States) under the 200 nm wavelength.
Whole genome sequencing, genome assembly, and annotation
The whole-genome sequencing was processed on the Illumina NovaSeq (paired-end, 2 × 150 bp) and PacBio RS II (20 kbp library) platforms (Shanghai Personal Biotechnology Co., Ltd., Shanghai, China), respectively. The genome sequence was obtained through Unicycler assembly pipeline (Wick et al., 2017). The long reads were assembled by miniasm (Li, 2016) and the genome was polished with Illumina short reads by Racon (Vaser et al., 2017). Protein-, tRNA-and rRNA-coding sequences (CDSs) were found by Prodigal (Hyatt et al., 2010), ARAGORN (Laslett and Canback, 2004) and Barrnap3, respectively. The promoter region was predicated by BPROM (Solovyev, 2011). The annotation of protein sequences was based on alignment of the predicted CDSs to the NCBI nr database (Sayers et al., 2021), the Swiss-Prot database (Bateman et al., 2023), and the Comprehensive Antibiotic Resistance Database (CARD) (Alcock et al., 2022) using DIAMOND blastp (Buchfink et al., 2021). The genome of DW0021 was served as the reference genome and the other 9 A. caviae genomes sharing the highest nucleotide identity with DW0021 were used for comparison (Table 5). Comparison of different A. caviae genomes was visualized by CGView Comparison Tool (Grant et al., 2012). The molecular weight and isoelectric point (pI) of protein sequences were calculated by EMBOSS pepstats (Rice et al., 2000).
Phylogenetic tree reconstruction and model building
Entrez Direct4 and GNU Parallel (Tange, 2021) were used to retrieve sequences from the NCBI databases. Samtools (Danecek et al., 2021) and SeqKit (Shen et al., 2016) were used to manipulate fasta sequences. FosC3 and the other sequences were aligned with L-INS-i strategy by MAFFT (Katoh and Standley, 2013) and printed by r-msa (Bodenhofer et al., 2015). The maximum-likelihood tree of FosC3 and its homologous sequences was reconstructed with LG + G4 substitution model, tested by 1,000 bootstrap replicates and visualized by IQ-TREE 2 (Minh et al., 2020), UFBoot2 (Hoang et al., 2018) and ggtree (Yu, 2020), respectively. The structure of FosC3 and FosC3 bound fosfomycin was modeled by AlphaFold 3 and SWISS-MODEL using the fosfomycin resistance protein with bound fosfomycin (SMTL ID: 5v3d.1) as template (Waterhouse et al., 2018; Abramson et al., 2024).
Gene synteny analysis
The fosC3 gene, along with its flanking regions, were used as queries and searched against the NCBI non-redundant nucleotide database to infer synteny. Comparison of gene clusters was generated by clinker (Gilchrist and Chooi, 2021).
Nucleotide sequence accession numbers
The GenBank accession numbers of the sequences of the A. caviae DW0021 chromosome, plasmid pAECA21-3829 and fosC3 gene were CP128475, CP128476, and OR187734, respectively.
Results and discussion
Antibiotic resistance pattern and genomic analysis of DW0021
Among 13 antimicrobials tested, A. caviae DW0021 showed high MIC values (≥ 32 μg/mL) to 10 of them, including kanamycin (512 μg/mL), tobramycin (128 μg/mL), streptomycin (128 μg/mL), chloramphenicol (128 μg/mL), florfenicol (64 μg/mL), tetracycline (128 μg/mL), and especially higher to fosfomycin (>1,024 μg/mL) (Table 2).
To analyze the molecular resistance mechanism, the complete genome of A. caviae DW0021 was sequenced, and it consisted of a chromosome and a plasmid designated pAECA21-3829. The chromosome was 4,589,869 bp in length with 61.43% GC content, encoding 4,086 proteins, 126 tRNAs and 31 rRNAs. pAECA21-3829 was 3,829 bp in length, harboring 6 CDSs (Table 6). The 16S rRNA gene sequence analysis indicated that DW0021 was phylogenetically closer to Aeromonas spp., and genome wide sequence comparison of DW0021 with those genomes available in the NCBI genome database revealed that it shared the highest whole-genome ANI (97.99%) with the A. caviae type strain NCTC12244 (genome assembly accession number: GCA_900476005.1). Therefore, the strain DW0021 was classified into A. caviae and named A. caviae DW0021. The annotation result of antimicrobial resistance genes was listed in Table 7. Thirteen antimicrobial resistance genes (≥ 80% similarity with the function-characterized resistance genes in the CARD database) that related to six classes of antimicrobial agents were annotated, including aminoglycoside [aph(3″)-Ib, aph(6)-Id, and two copies of aac(6′)-Ib9], β-lactam (blaOXA-10, blaOXA-504, and blaMOX-3), fluoroquinolone (qnrVC4), phenicol (cmlA5 and floR), sulfonamide (sul1), and tetracycline [tet(E) and tet(A)]. All the antimicrobial resistance genes were encoded on the chromosome, while the plasmid pAECA21-3829 was free of an antimicrobial resistance gene.
Comparative genomic analysis of DW0021 and the other A. caviae strains was shown in Figure 1. It revealed that the genome of A. caviae DW0021 was similar to the other 9 A. caviae genomes in large part.
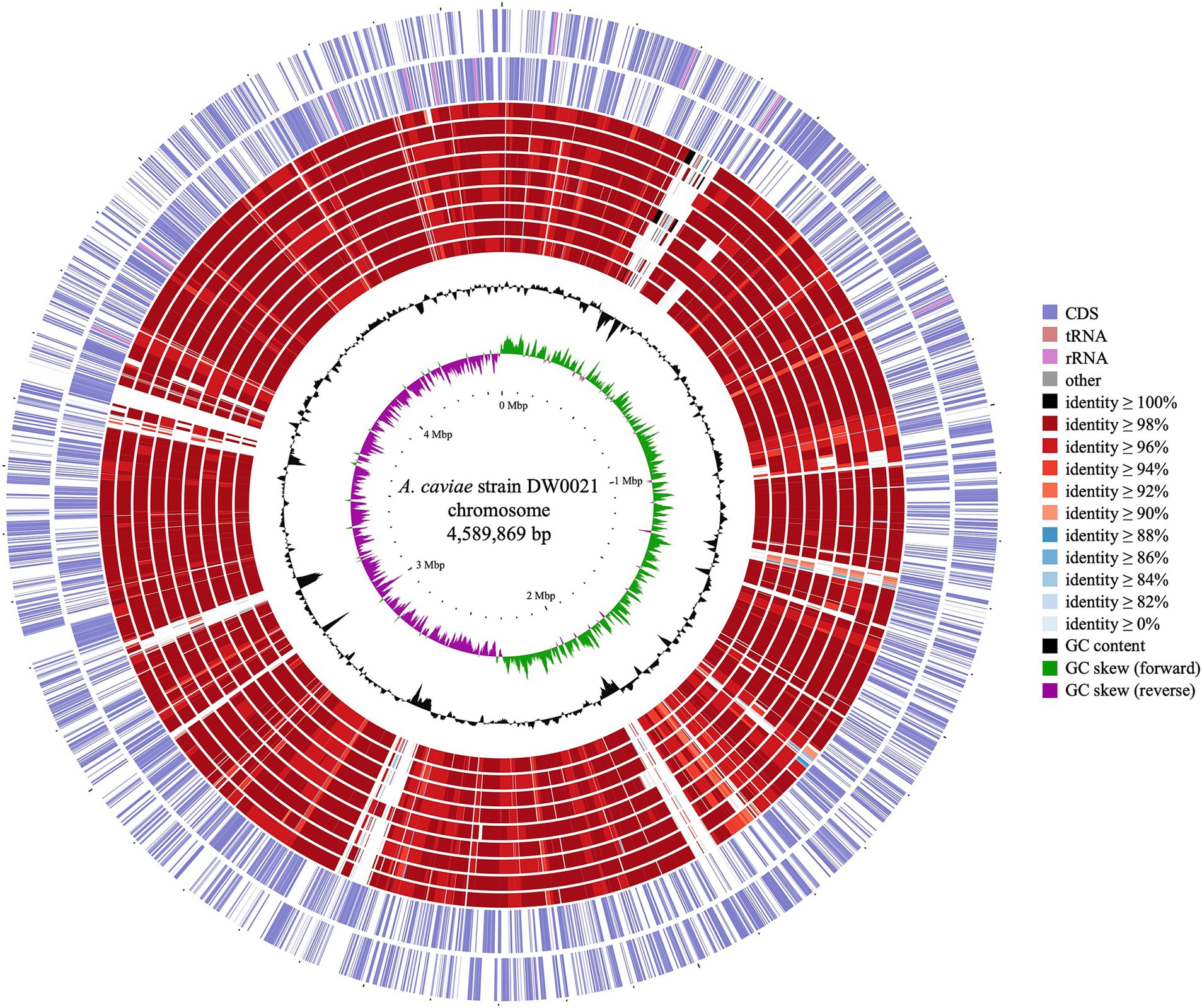
Figure 1. Genome map of Aeromonas caviae DW0021 and other A. caviae strains. Circles (from outside to inside) 1–2, indicated forward and reverse strand of the DW0021 chromosome (CP128475.1); 3–11 were the chromosomes of A. caviae KAM376 (AP024402.1), A. caviae FAHZZU2447 (CP100392.1), A. caviae FDAARGOS_75 (CP062801.1), A. caviae FDAARGOS_72 (CP062787.1), A. caviae NUITM-VA2 (AP025280.1), A. caviae 211703 (CP092181.1), A. caviae NCTC12244 (LS483441.1), A. caviae 71442 (CP084350.1), and A. caviae R25-6 (CP025705.1), respectively; 12–13 were GC content and GC skew of the DW0021 chromosome, respectively.
fosC3 showing resistance to fosfomycin
Although A. caviae DW0021 exhibited high level MIC to fosfomycin, the 13 predicted resistance genes from the whole genome were not associated with the resistance to the antimicrobial. A novel fosfomycin resistance mechanism would be present in A. caviae DW0021, which may be affiliated with an unidentified antimicrobial resistance gene. To confirm the speculation, the annotation result of the genome sequence was examined and the deduced protein sequence encoded by one fosC2-like gene sharing 99.25% coverage and 59.09% identity with FosC2 (BAJ10053.1) was found. The fosC2-like gene (designated fosC3 in this work) was cloned and confirmed to be functional. The recombinant pMD19-fosC3/DH5α displayed a 128-fold increase of MIC value to fosfomycin (32 μg/mL) compared to pMD19/DH5α (0.25 μg/mL), however, no significantly reduced susceptibility to the other antimicrobial agents identified (Table 2).
Generally, the bacteria with the fosfomycin-modifying genes (fos and fom genes) showed high MIC levels to fosfomycin. For example, P. syringae PB-5123 carrying a fosC gene demonstrated a MIC of 1,024 mg/mL to fosfomycin (García et al., 1995). Compared to the recipients, the transformant DH10B (pS-fosC2) with fosC2 showed reduced susceptibility (MIC > 256 μg/mL, increased > 512-fold) (Wachino et al., 2010), and the FosC2AS-producing E. coli recombinant also showed high level of resistance to fosfomycin (MIC > 256 μg/mL, increased > 64-fold) (Ortiz de la Rosa et al., 2022). Other reports also revealed high or increased MIC levels when a strain harbored a fosfomycin resistance gene or a mutated one. E. coli KAM32/pSP72/Vf-murA had an MIC of 3,000 μg/mL to fosfomycin (Kumar et al., 2009). Amino acid substituted MurA found in resistant isolates further raised the MIC for fosfomycin by more than 8-fold (≥ 1,024 mg/L) compared with the strains expressing wild-type MurA (Takahata et al., 2010). The fosfomycin-resistant subpopulations overexpressed murA also resulted in a 10-fold increase (from 0.064 to 0.64 mg/L) (Campos da Campos et al., 2020). Another gene called abrp conferred a 4-fold decreased susceptibility to fosfomycin (from 64 mg/L to 256 mg/L) in A. baumannii (Li et al., 2016). Mutations in the structure of glpT and uhpT showed increased MICs to fosfomycin (≥ 256 mg/L) (Takahata et al., 2010).
The 402 bp ORF of fosC3 gene encoded a 133 amino acid enzyme with a molecular weight of 14.87 kDa and pI of 5.96. To further study the properties of the novel enzyme, FosC3 was over-expressed (Supplementary Figure S1A) and purified (Supplementary Figure S1B). Enzyme kinetic assays of FosC3 based on HPLC (Supplementary Figure S2) manifested a kcat and Km of 82,442 ± 1,475 s−1, 70.99 ± 4.31 μM, respectively, which indicated that FosC3 could inactivate fosfomycin with a catalytic efficiency (kcat/Km) of (1.2 ± 0.3) × 103 μM−1·s−1. Most kinetic analyses of fosfomycin thiol transferases were conducted on FosAs (Table 8). FosA typically demonstrated a high catalytic efficiency (≥ 103), which may be a result of selection pressures exerted by the clinical use of fosfomycin. Although the kcat of FosC3 (82,442 ± 1,475 s−1) was significantly more than that of other fosfomycin thiol transferases, no notable difference of catalytic efficiency was observed. The results indicate that the FosC3 was probably less or equal active than the FosA enzyme variants.
Comparative analysis of fosC3 with other fos genes
fosC3 was a novel fosfomycin-modifying gene. The phylogenetic tree of FosC3 and the other function-characterized fosfomycin-modifying enzymes was depicted in Figure 2. In the phylogenetic tree, FosC3 was on a branch that was close to FosC2 and FosG. Among the function-characterized Fos proteins, FosC3 shared the highest amino acid similarity of 58.65% (99.25% coverage and 59.09% identity) with FosC2. FosC3 also shared > 50% similarities with FosG, FosK, FosA5, FosA6, FosA, FosL1, FosA8, FosA2, FosA3, FosA7.5, FosA4, and FosA7, but < 40% with the rest fosfomycin thiol transferases.
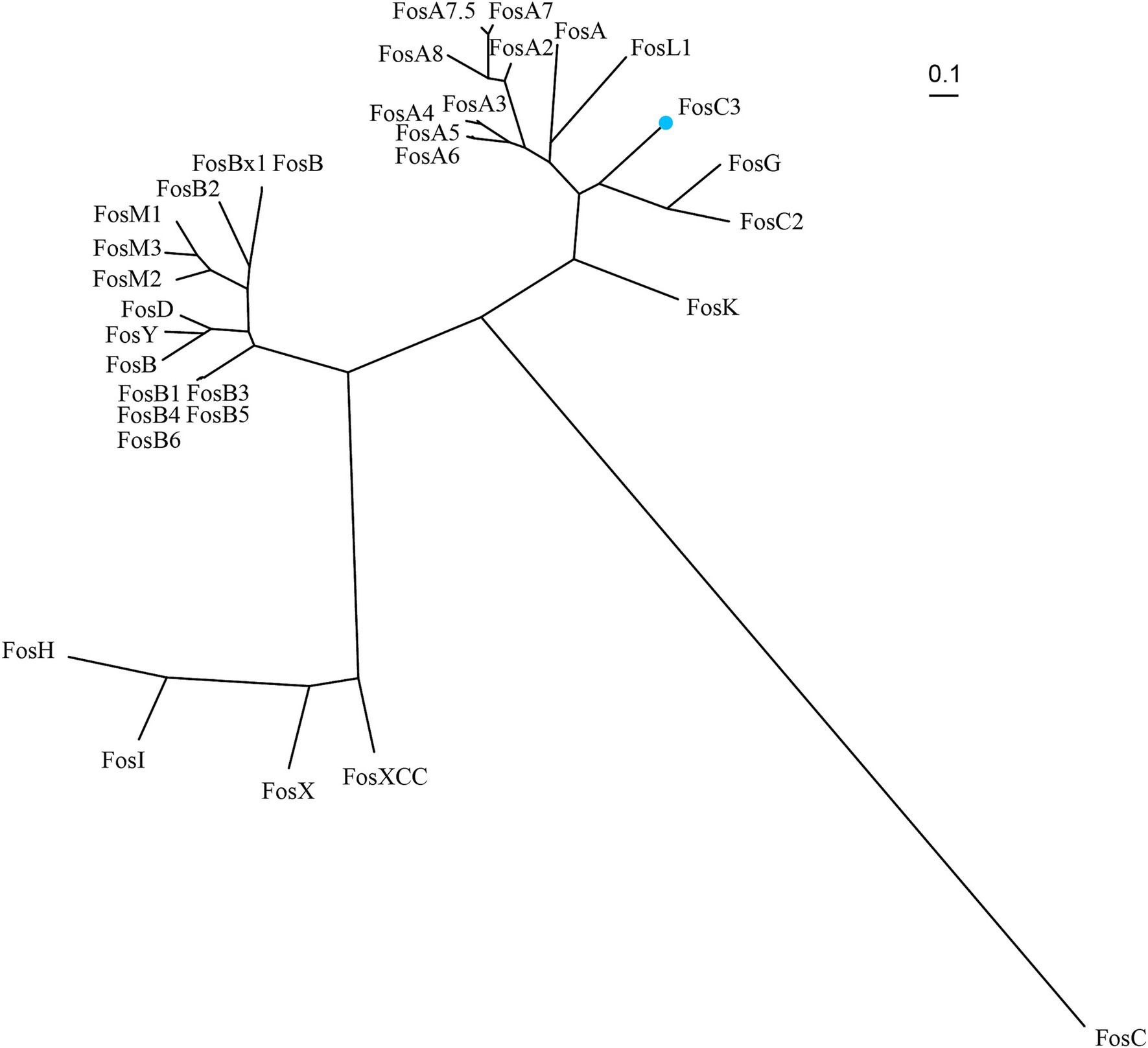
Figure 2. Unrooted phylogenetic tree of FosC3 and other function-characterized fosfomycin-modifying enzymes. FosC3 (blue dot) was in the clade that close to FosG and FosC2. Accession numbers: FosC3 (XBY83663.1), FosA (AAG04518.1), FosA2 (ACC85616.1), FosA3 (AEG78825.1), FosA4 (BAP18892.1), fosA5 (AJE60855.1), FosA6 (AMQ12811.1), FosA7 (KKE03230.1), FosA7.5 (ANQ03635.1), FosA8 (QEI22965.1), FosB (AAP08996.1), FosB1 (BAE05988.1), FosB2 (AAP27834.1), FosB3 (ADX95999.1), FosB4 (ALM24139.1), FosB5 (ALN12426.1), FosB6 (ALM24145.1), FosBx1 (QLF01382.1), FosC (CAA83855.1), FosC2 (BAJ10053.1), FosD (BAG12271.1), FosG (RTB44598.1), FosH (ADF48907.1), FosI (AFJ38137.1), FosK (BAO79518.1), FosL1 (QHR93773.1), FosM1 (DAC85639.1), FosM2 (DAC85640.1), FosM3 (DAC85641.1), FosX (CWV56762.1), FosXCC (AIF29598.1), FosY (QTE33800.1), and FosB (EHS19134.1).
FosC3 possessed the similar functional residues that were similar to FosA. Although FosA and FosC2 were two distinct enzymes, both could inactivate fosfomycin through glutathione S-transferase activity (Wachino et al., 2010). FosA proteins possessed residues that were responsible for the dimer interface loop (Figure 3, amino acid residues 53 to 58 in red frames), Mn2+ (residues 7H, 62H, 108E, blue frames) and K+ binding (93E, green frames), and fosfomycin binding (9 T, 60Y, 88 K, 92S, 96S, 98Y, purple frames) (Klontz et al., 2017). Multiple sequence alignment of FosC3 and its homologous fosfomycin-modifying enzymes revealed that FosC3 contained the similar residues (Figure 3) and may be able to inactivate fosfomycin through glutathione S-transferase activity (Wachino et al., 2010). The notable divergence was related to the dimer interface loop among fosfomycin thiol transferases, while those residues involved in Mn2+ coordination, K+ binding, and fosfomycin binding were identical. FosC3, FosC2, FosG and FosK were five residues shorter, and that of FosA and FosL1 were three and two, which implied that the loop could cross the dimer interface relatively directly (Klontz et al., 2017). The enzyme was predicted to a homo-dimer (Supplementary Figure S3) with residues 9T, 46W, 60Y, 88K, 92S, 98Y, 117R to bind with fosfomycin. FosC3 was also predicted to form metal complexes with K+ (90N, 92S, 94G, 96S) and Mn2+ (7H, 62H, 108E).
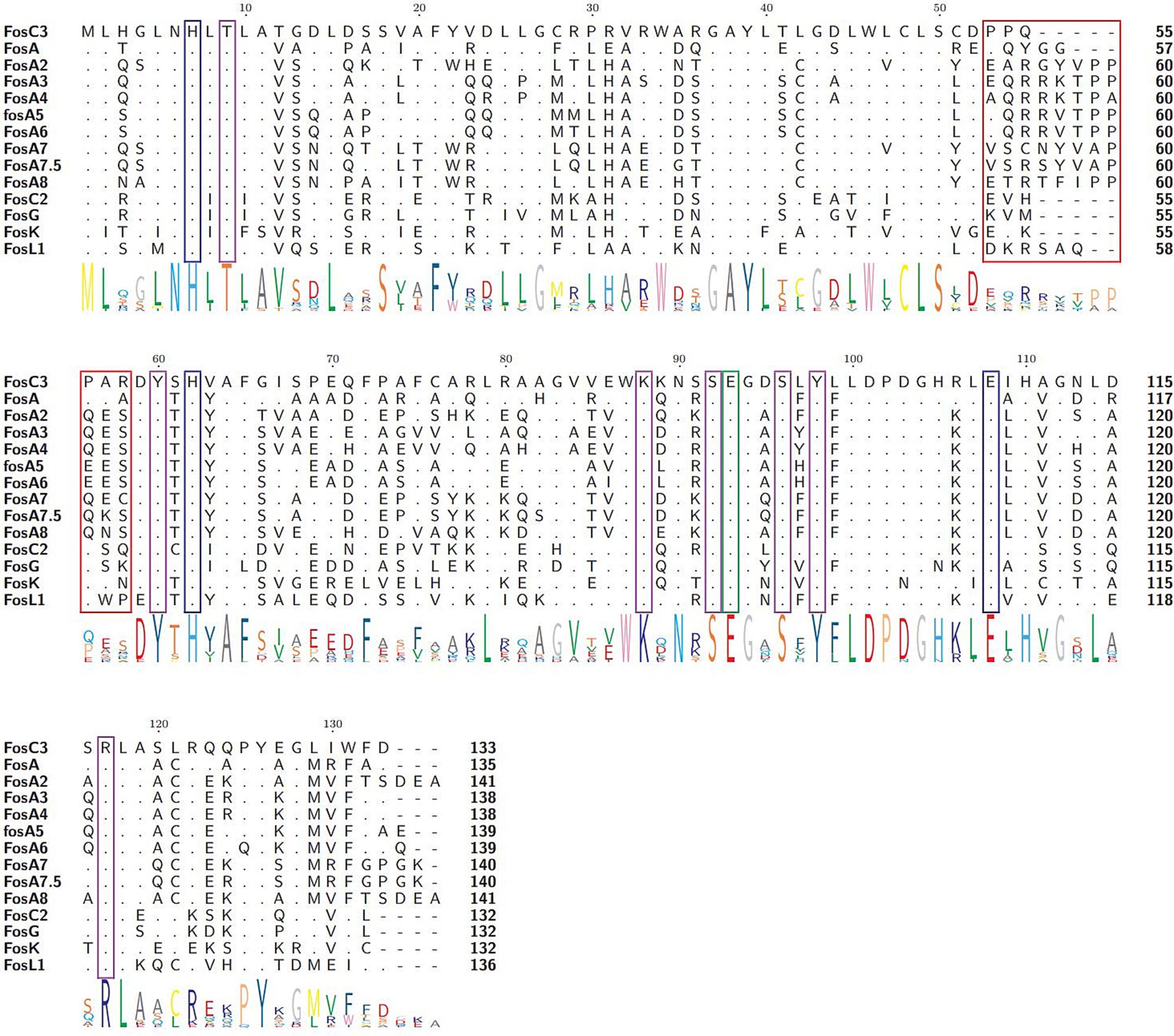
Figure 3. Multiple sequence alignment of FosC3 and its homologous fosfomycin-modifying enzymes. The length of each sequence was labeled on the right. The logo size at the bottom indicated the degree of conservation at that residue. Dots and hyphens represented identical residues and gaps, respectively. Amino acid residues in the red, blue, green, and purple frames manifested the dimer interface loop of enzymes, with residues involved in Mn2+ coordination, K+ binding loop, and fosfomycin binding, respectively. Accession numbers: FosC3 (XBY83663.1), FosA (AAG04518.1), FosA2 (ACC85616.1), FosA3 (AEG78825.1), FosA4 (BAP18892.1), fosA5 (AJE60855.1), FosA6 (AMQ12811.1), FosA7 (KKE03230.1), FosA7.5 (ANQ03635.1), FosA8 (QEI22965.1), FosC2 (BAJ10053.1), FosG (RTB44598.1), FosK (BAO79518.1), and FosL1 (QHR93773.1).
Aeromonas spp. could be a reservoir of fosC3 genes. It was found that 54 FosC3-like sequences (annotated as putative fosfomycin-modifying enzymes) sharing > 90.0% coverage and > 80.0% identity with FosC3 in the NCBI non-redundant database were all from Aeromonas spp. Most of them were found in A. caviae (31/54, 57.4%), and the others were from Aeromonas spp. (16/54, 29.6%), A. dhakensis (4/54, 7.4%), A. bivalvium (2/54, 3.7%) and A. enteropelogenes (1/54, 1.9%), respectively (Supplementary Table S1).
The fosC3 and fosC3-like genes were located within a conserved genomic region (Figure 4). No mobile genetic element was identified around the flanking regions of fosC3 and fosC3-like genes (> 80% identity). fosC3 was surrounded by upstream genes encoding FxsA family protein, aspartate ammonia-lyase, and anaerobic C4-dicarboxylate transporter, and downstream genes of fosC3 were ribosomal protein and so on. The structure of the fosC3-related fragment was similar to several other chromosomal fragments of A. caviae strains. The result indicated that fosC3 may be a gene in a conserved genomic region of A. caviae strains. fosC2AS was also encoded in a region without MGE (Ortiz de la Rosa et al., 2022). However, other fos genes such as fosC2 and fosA genes were found related with the MGEs. The fosC2 gene was found in a class 1 integron accompanied by dfrA17 and aadA5 encoded in a plasmid (Wachino et al., 2010). fosA was first discovered on Tn2921 in a plasmid (Navas et al., 1990). Other plasmid-borne fosA genes such as fosA3 (Wachino et al., 2010), fosA5 (Ma et al., 2015), fosA6 (Guo et al., 2016) and fosA8 (Poirel et al., 2019) were also identified.
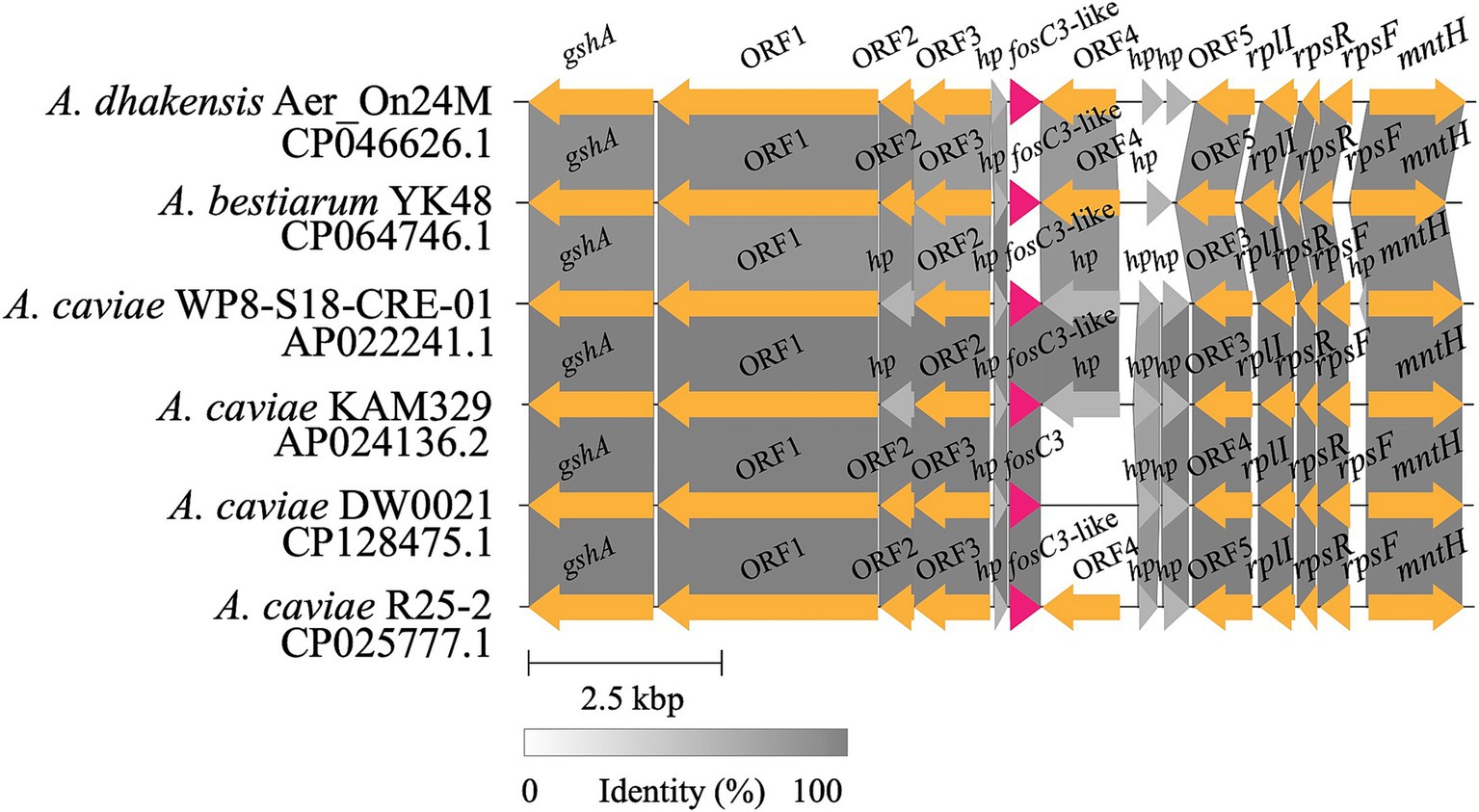
Figure 4. Alignments of the fosC3 and fosC3-like genes clusters. A grey linked region indicated a ≥ 80% identity. fosC3 and fosC3-like genes were highlighted in red. Genes annotated with ORF were those without official gene symbols. hp, hypothetical genes.
Conclusion
A novel gene fosC3 that encoding fosfomycin thiol transferases was identified on the chromosome of A. caviae DW0021, and the kinetic and functional properties of the FosC3 enzyme were measured. FosC3 shared the highest amino acid similarity of 58.65% (99.25% coverage and 59.09% identity) with FosC2 and phylogenetically related to FosC2 and FosG. FosC3 was able to inactive fosfomycin with a catalytic efficiency (kcat/Km) of (1.2 ± 0.3) × 103 μM−1·s−1. This work contributed to the study on mechanism of fosfomycin resistance in pathogenic Aeromonas species such as A. caviae.
Data availability statement
The datasets presented in this study can be found in online repositories. The names of the repository/repositories and accession number(s) can be found in the article/Supplementary material.
Author contributions
JL: Methodology, Writing – original draft, Writing – review & editing, Data curation, Formal analysis. RZ: Data curation, Methodology, Writing – original draft, Formal analysis. YY: Data curation, Formal analysis, Methodology, Writing – original draft. HL: Conceptualization, Writing – review & editing. DL: Conceptualization, Methodology, Writing – review & editing. QB: Conceptualization, Methodology, Writing – review & editing. CF: Conceptualization, Formal analysis, Methodology, Visualization, Writing – original draft, Writing – review & editing.
Funding
The author(s) declare that financial support was received for the research and/or publication of this article. This study was supported by the Science & Technology Project of Jinhua City, China (2022-2-013), Zhejiang Provincial Natural Science Foundation of China (LGD22C040006), the Science & Technology Project of Wenzhou City, China (N20210001), and the Scientific Research Fund Project of Zhejiang Provincial Department of Education (Y202455702).
Acknowledgments
The authors would like to acknowledge all study participants and individuals who contributed to this study.
Conflict of interest
The authors declare that the research was conducted in the absence of any commercial or financial relationships that could be construed as a potential conflict of interest.
Generative AI statement
The author(s) declare that no Gen AI was used in the creation of this manuscript.
Publisher’s note
All claims expressed in this article are solely those of the authors and do not necessarily represent those of their affiliated organizations, or those of the publisher, the editors and the reviewers. Any product that may be evaluated in this article, or claim that may be made by its manufacturer, is not guaranteed or endorsed by the publisher.
Supplementary material
The Supplementary material for this article can be found online at: https://www.frontiersin.org/articles/10.3389/fmicb.2025.1577167/full#supplementary-material
SUPPLEMENTARY FIGURE S1 | (A) Over-expression of enzyme. lane 1, expression without IPTG induction; 2, sediment; 3, supernatant; 4, supernatant (pCold I-fosC3/BL21); 5, sediment (pCold I-fosC3/BL21); M, 180 kDa marker. (B) Purification of enzyme. Lane M, 10-180 kDa marker; lane 1-4, EK digestion for 12 h, 8 h, 4 h, and 0 h under 4°C, respectively; lane 5, FosC3.
SUPPLEMENTARY FIGURE S2 | HPLC chromatogram. Retention time (0–6 min): 3.32 min: unknown peak, 3.97 min: product peak, 4.68 min: GSH peak, 5.05 min: unknown peak.
SUPPLEMENTARY FIGURE S3 | (A) Cartoon representation of the structure of FosC3 and (B) FosC3 bound with fosfomycin (residues 9T, 46W, 60Y, 88K, 92S, 98Y, 117R). (C) Metal coordination with FosC3. K+: 90N, 92S, 94G, 96S and Mn2+: 7H, 62H, 108E.
Footnotes
References
Abramson, J., Adler, J., Dunger, J., Evans, R., Green, T., Pritzel, A., et al. (2024). Accurate structure prediction of biomolecular interactions with AlphaFold 3. Nature 630, 493–500. doi: 10.1038/s41586-024-07487-w
Alcock, B. P., Huynh, W., Chalil, R., Smith, K. W., Raphenya, A. R., Wlodarski, M. A., et al. (2022). CARD 2023: expanded curation, support for machine learning, and resistome prediction at the comprehensive antibiotic resistance database. Nucleic Acids Res. 51, D690–D699. doi: 10.1093/nar/gkac920
Bateman, A., Martin, M.-J., Orchard, S., Magrane, M., Ahmad, S., Alpi, E., et al. (2023). UniProt: the universal protein knowledgebase in 2023. Nucleic Acids Res. 51, D523–D531. doi: 10.1093/nar/gkac1052
Bello-López, J. M., Cabrero-Martínez, O. A., Ibáñez-Cervantes, G., Hernández-Cortez, C., Pelcastre-Rodríguez, L. I., Gonzalez-Avila, L. U., et al. (2019). Horizontal gene transfer and its association with antibiotic resistance in the genus Aeromonas spp. Microorganisms 7:363. doi: 10.3390/microorganisms7090363
Bodenhofer, U., Bonatesta, E., Horejš-Kainrath, C., and Hochreiter, S. (2015). Msa: an R package for multiple sequence alignment. Bioinformatics 31, 3997–3999. doi: 10.1093/bioinformatics/btv494
Brown, D. W., Schaab, M. R., Birmingham, W. R., and Armstrong, R. N. (2009). Evolution of the antibiotic resistance protein, FosA, is linked to a catalytically promiscuous progenitor. Biochemistry 48, 1847–1849. doi: 10.1021/bi900078q
Buchfink, B., Reuter, K., and Drost, H.-G. (2021). Sensitive protein alignments at tree-of-life scale using DIAMOND. Nat. Methods 18, 366–368. doi: 10.1038/s41592-021-01101-x
Cao, M., Bernat, B. A., Wang, Z., Armstrong, R. N., and Helmann, J. D. (2001). FosB, a cysteine-dependent Fosfomycin resistance protein under the control of ςW, an Extracytoplasmic-function ς factor in Bacillus subtilis. J. Bacteriol. 183, 2380–2383. doi: 10.1128/JB.183.7.2380-2383.2001
da Campos, A. C., Andrade, N. L., Couto, N., Mutters, N. T., de Vos, M., Rosa, A. C. d. P., et al. (2020). Characterization of fosfomycin heteroresistance among multidrug-resistant Escherichia coli isolates from hospitalized patients in Rio de Janeiro, Brazil. J. Glob. Antimicrob. Resist. 22, 584–593. doi: 10.1016/j.jgar.2020.04.026
Danecek, P., Bonfield, J. K., Liddle, J., Marshall, J., Ohan, V., Pollard, M. O., et al. (2021). Twelve years of SAMtools and BCFtools. Gigascience 10:giab008. doi: 10.1093/gigascience/giab008
Falagas, M. E., Athanasaki, F., Voulgaris, G. L., Triarides, N. A., and Vardakas, K. Z. (2019). Resistance to fosfomycin: mechanisms, frequency and clinical consequences. Int. J. Antimicrob. Agents 53, 22–28. doi: 10.1016/j.ijantimicag.2018.09.013
Fernández-Bravo, A., and Figueras, M. J. (2020). An update on the genus Aeromonas: taxonomy, epidemiology, and pathogenicity. Microorganisms 8:129. doi: 10.3390/microorganisms8010129
Fillgrove, K. L., Pakhomova, S., Newcomer, M. E., and Armstrong, R. N. (2003). Mechanistic diversity of fosfomycin resistance in pathogenic microorganisms. J. Am. Chem. Soc. 125, 15730–15731. doi: 10.1021/ja039307z
Fillgrove, K. L., Pakhomova, S., Schaab, M. R., Newcomer, M. E., and Armstrong, R. N. (2007). Structure and mechanism of the genomically encoded fosfomycin resistance protein, FosX, from Listeria monocytogenes. Biochemistry 46, 8110–8120. doi: 10.1021/bi700625p
García, P., Arca, P., and Evaristo Suárez, J. (1995). Product of fosC, a gene from Pseudomonas syringae, mediates fosfomycin resistance by using ATP as cosubstrate. Antimicrob. Agents Chemother. 39, 1569–1573
Gilchrist, C. L. M., and Chooi, Y.-H. (2021). Clinker & clustermap.Js: automatic generation of gene cluster comparison figures. Bioinformatics 37, 2473–2475. doi: 10.1093/bioinformatics/btab007
Grant, J. R., Arantes, A. S., and Stothard, P. (2012). Comparing thousands of circular genomes using the CGView comparison tool. BMC Genomics 13:202. doi: 10.1186/1471-2164-13-202
Güneri, C. Ö., Stingl, K., Grobbel, M., Hammerl, J. A., and Kürekci, C. (2022). Different fosA genes were found on mobile genetic elements in Escherichia coli from wastewaters of hospitals and municipals in Turkey. Sci. Total Environ. 824:153928. doi: 10.1016/j.scitotenv.2022.153928
Guo, Q., Tomich, A. D., McElheny, C. L., Cooper, V. S., Stoesser, N., Wang, M., et al. (2016). Glutathione-S-transferase FosA6 of Klebsiella pneumoniae origin conferring fosfomycin resistance in ESBL-producing Escherichia coli. J. Antimicrob. Chemother. 71, 2460–2465. doi: 10.1093/jac/dkw177
Hoang, D. T., Chernomor, O., von Haeseler, A., Minh, B. Q., and Vinh, L. S. (2018). UFBoot2: improving the ultrafast bootstrap approximation. Mol. Biol. Evol. 35, 518–522. doi: 10.1093/molbev/msx281
Hyatt, D., Chen, G.-L., LoCascio, P. F., Land, M. L., Larimer, F. W., and Hauser, L. J. (2010). Prodigal: prokaryotic gene recognition and translation initiation site identification. BMC Bioinformatics 11:119. doi: 10.1186/1471-2105-11-119
Katoh, K., and Standley, D. M. (2013). MAFFT multiple sequence alignment software version 7: improvements in performance and usability. Mol. Biol. Evol. 30, 772–780. doi: 10.1093/molbev/mst010
Klontz, E. H., Tomich, A. D., Günther, S., Lemkul, J. A., Deredge, D., Silverstein, Z., et al. (2017). Structure and dynamics of FosA-mediated Fosfomycin resistance in Klebsiella pneumoniae and Escherichia coli. Antimicrob. Agents Chemother. 61, e01572–e01517. doi: 10.1128/AAC.01572-17
Kumar, S., Parvathi, A., Hernandez, R., Cadle, K., and Varela, M. F. (2009). Identification of a novel UDP-N-acetylglucosamine enolpyruvyl transferase (MurA) from Vibrio fischeri that confers high fosfomycin resistance in Escherichia coli. Arch. Microbiol. 191, 425–429. doi: 10.1007/s00203-009-0468-9
Laslett, D., and Canback, B. (2004). ARAGORN, a program to detect tRNA genes and tmRNA genes in nucleotide sequences. Nucleic Acids Res. 32, 11–16. doi: 10.1093/nar/gkh152
Li, H. (2016). Minimap and miniasm: fast mapping and de novo assembly for noisy long sequences. Bioinformatics 32, 2103–2110. doi: 10.1093/bioinformatics/btw152
Li, X., Quan, J., Yang, Y., Ji, J., Liu, L., Fu, Y., et al. (2016). Abrp, a new gene, confers reduced susceptibility to tetracycline, glycylcine, chloramphenicol and fosfomycin classes in Acinetobacter baumannii. Eur. J. Clin. Microbiol. Infect. Dis. 35, 1371–1375. doi: 10.1007/s10096-016-2674-0
Lu, W., Zhou, S., Ma, X., Xu, N., Liu, D., Zhang, K., et al. (2024). fosA11, a novel chromosomal-encoded fosfomycin resistance gene identified in Providencia rettgeri. Microbiol. Spectr. 12, e02542–e02523. doi: 10.1128/spectrum.02542-23
Ma, Y., Xu, X., Guo, Q., Wang, P., Wang, W., and Wang, M. (2015). Characterization of fosA5, a new plasmid-mediated fosfomycin resistance gene in Escherichia coli. Lett. Appl. Microbiol. 60, 259–264. doi: 10.1111/lam.12366
Minh, B. Q., Schmidt, H. A., Chernomor, O., Schrempf, D., Woodhams, M. D., von Haeseler, A., et al. (2020). IQ-TREE 2: new models and efficient methods for phylogenetic inference in the genomic era. Mol. Biol. Evol. 37, 1530–1534. doi: 10.1093/molbev/msaa015
Navas, J., León, J., Arroyo, M., and García Lobo, J. M. (1990). Nucleotide sequence and intracellular location of the product of the fosfomycin resistance gene from transposon Tn2921. Antimicrob. Agents Chemother. 34, 2016–2018
Ortiz de la Rosa, J.-M., Nordmann, P., Zong, Z., and Poirel, L. (2022). Aliidiomarina shirensis as possible source of the Integron-and plasmid-mediated Fosfomycin resistance gene fosC2. Antimicrob. Agents Chemother. 66:e0222721. doi: 10.1128/aac.02227-21
Pessoa, R. B. G., de Oliveira, W. F., Correia, M. T. D. S., Fontes, A., and Coelho, L. C. B. B. (2022). Aeromonas and human health disorders: clinical approaches. Front. Microbiol. 13:868890. doi: 10.3389/fmicb.2022.868890
Poirel, L., Vuillemin, X., Kieffer, N., Mueller, L., Descombes, M.-C., and Nordmann, P. (2019). Identification of FosA8, a plasmid-encoded Fosfomycin resistance determinant from Escherichia coli, and its origin in Leclercia adecarboxylata. Antimicrob. Agents Chemother. 63, e01403–e01419. doi: 10.1128/AAC.01403-19
Qing, G., Ma, L.-C., Khorchid, A., Swapna, G. V. T., Mal, T. K., Takayama, M. M., et al. (2004). Cold-shock induced high-yield protein production in Escherichia coli. Nat. Biotechnol. 22, 877–882. doi: 10.1038/nbt984
Rice, P., Longden, I., and Bleasby, A. (2000). EMBOSS: the European molecular biology open software suite. Trends Genet. 16, 276–277. doi: 10.1016/s0168-9525(00)02024-2
Richter, M., and Rosselló-Móra, R. (2009). Shifting the genomic gold standard for the prokaryotic species definition. Proc. Natl. Acad. Sci. USA 106, 19126–19131. doi: 10.1073/pnas.0906412106
Rife, C. L., Pharris, R. E., Newcomer, M. E., and Armstrong, R. N. (2002). Crystal structure of a genomically encoded fosfomycin resistance protein (FosA) at 1.19 a resolution by MAD phasing off the L-III edge of Tl(+). J. Am. Chem. Soc. 124, 11001–11003. doi: 10.1021/ja026879v
Rigsby, R. E., Brown, D. W., Dawson, E., Lybrand, T. P., and Armstrong, R. N. (2007). A model for glutathione binding and activation in the fosfomycin resistance protein, FosA. Arch. Biochem. Biophys. 464, 277–283. doi: 10.1016/j.abb.2007.04.035
Rigsby, R. E., Fillgrove, K. L., Beihoffer, L. A., and Armstrong, R. N. (2005). Fosfomycin resistance proteins: a nexus of glutathione transferases and epoxide hydrolases in a metalloenzyme superfamily. Methods Enzymol. 401, 367–379. doi: 10.1016/S0076-6879(05)01023-2
Sayers, E. W., Bolton, E. E., Brister, J. R., Canese, K., Chan, J., Comeau, D. C., et al. (2021). Database resources of the National Center for biotechnology information. Nucleic Acids Res. 50, D20–D26. doi: 10.1093/nar/gkab1112
Shen, W., Le, S., Li, Y., and Hu, F. (2016). SeqKit: a cross-platform and ultrafast toolkit for FASTA/Q file manipulation. PLoS One 11:e0163962. doi: 10.1371/journal.pone.0163962
Solovyev, V. (2011). “V. Solovyev, a Salamov (2011) automatic annotation of microbial genomes and metagenomic sequences” in Metagenomics and its applications in agriculture, biomedicine and environmental studies. ed. R. W. Li (Hauppauge, NY: Nova Science Publishers), 61–78.
Takahata, S., Ida, T., Hiraishi, T., Sakakibara, S., Maebashi, K., Terada, S., et al. (2010). Molecular mechanisms of fosfomycin resistance in clinical isolates of Escherichia coli. Int. J. Antimicrob. Agents 35, 333–337. doi: 10.1016/j.ijantimicag.2009.11.011
Vaser, R., Sović, I., Nagarajan, N., and Šikić, M. (2017). Fast and accurate de novo genome assembly from long uncorrected reads. Genome Res. 27, 737–746. doi: 10.1101/gr.214270.116
Wachino, J., Yamane, K., Suzuki, S., Kimura, K., and Arakawa, Y. (2010). Prevalence of Fosfomycin resistance among CTX-M-producing Escherichia coli clinical isolates in Japan and identification of novel plasmid-mediated Fosfomycin-modifying enzymes. Antimicrob. Agents Chemother. 54, 3061–3064. doi: 10.1128/AAC.01834-09
Waterhouse, A., Bertoni, M., Bienert, S., Studer, G., Tauriello, G., Gumienny, R., et al. (2018). SWISS-MODEL: homology modelling of protein structures and complexes. Nucleic Acids Res. 46, W296–W303. doi: 10.1093/nar/gky427
Wick, R. R., Judd, L. M., Gorrie, C. L., and Holt, K. E. (2017). Unicycler: resolving bacterial genome assemblies from short and long sequencing reads. PLoS Comput. Biol. 13:e1005595. doi: 10.1371/journal.pcbi.1005595
Yang, T.-Y., Lu, P.-L., and Tseng, S.-P. (2019). Update on fosfomycin-modified genes in Enterobacteriaceae. J. Microbiol. Immunol. Infect. 52, 9–21. doi: 10.1016/j.jmii.2017.10.006
Yu, G. (2020). Using ggtree to visualize data on tree-like structures. Curr. Protoc. Bioinformatics 69:e96. doi: 10.1002/cpbi.96
Keywords: fosfomycin, Aeromonas caviae , FosC3, enzyme kinetics, antibiotic resistance
Citation: Lu J, Zhang R, Yu Y, Lou H, Li D, Bao Q and Feng C (2025) Identification of a novel chromosome-encoded fosfomycin resistance gene fosC3 in Aeromonas caviae. Front. Microbiol. 16:1577167. doi: 10.3389/fmicb.2025.1577167
Edited by:
John Osei Sekyere, University of Pretoria, South AfricaReviewed by:
Joseph Sahayarayan Jesudass, Alagappa University, IndiaSura Alasadi, Nahrain University, Iraq
Dipta Chandra Pal, University of Dhaka, Bangladesh
Brenda Solange Ayzanoa Canales, Universidad Peruana Cayetano Heredia, Peru
Sulmaz Reshi, Hamdard Institute of Medical Sciences and Research (HIMSR), India
Copyright © 2025 Lu, Zhang, Yu, Lou, Li, Bao and Feng. This is an open-access article distributed under the terms of the Creative Commons Attribution License (CC BY). The use, distribution or reproduction in other forums is permitted, provided the original author(s) and the copyright owner(s) are credited and that the original publication in this journal is cited, in accordance with accepted academic practice. No use, distribution or reproduction is permitted which does not comply with these terms.
*Correspondence: Dong Li, bGlkb25nQHdtdS5lZHUuY24=; Qiyu Bao, YmFvcXlAZ2Vub21pY3MuY24=; Chunlin Feng, MTg1ODc3NDQ0NTJAMTg5LmNu