- 1Southern Marine Science and Engineering Guangdong Laboratory (Zhuhai), Ministry of Agriculture Key Laboratory of Animal Nutrition and Feed Science in South China, Institute of Animal Science, Guangdong Academy of Agricultural Sciences, Guangzhou, China
- 2Guangdong Provincial Key Laboratory of Animal Nutrition Regulation, College of Animal Science, South China Agricultural University, Guangzhou, China
- 3Southern Marine Science and Engineering Guangdong Laboratory (Zhuhai), Department of Ecology, Jinan University, Guangzhou, China
- 4Key Laboratory of Xinjiang Feed Biotechnology, Feed Research Institute, Xinjiang Academy of Animal Science, Ürümqi, China
- 5Agri-Food and Biosciences Institute, Hillsborough, United Kingdom
Introduction: Asparagopsis taxiformis (A. taxiformis) has shown great potential to mitigate methane (CH4) emissions in recent years. This study aims to evaluate the impact of A. taxiformis on methane emissions and to fill the knowledge gap regarding its mechanisms of action in affecting CH4 metabolism and rumen fermentation.
Methods: The experimental design consisted of a control group (CON) and test groups supplemented with 2% (Low), 5% (Mid), and 10% (High) of dried and freeze-dried treatment A. taxiformis, respectively, for 48 h of in vitro rumen fermentation. The optimal combination strategy for mitigating CH4 emissions was confirmed by analyzing nutrient degradation, CH4 production and rumen fermentation parameters, and the mechanism of action was analyzed by metagenomic and metabolomic approaches.
Results and discussion: The results showed that freeze-dried treatment had better potential to mitigate CH4 emissions than dried treatment, and supplementation of freeze-dried treatments at Low, Mid, and High groups significantly reduced CH4 production by 32.44%, 98.53%, and 99.33%, respectively. However, the High group exhibited a huge negative impact on rumen fermentation. Therefore, subsequent analyses focused on the Low and Mid groups to explore the underlying mechanisms. Metagenomics analyses showed that supplementation of freeze-dried treatment with the Mid-level supplementation significantly increased the relative abundance of propionate-producing bacteria such as Prevotella, Ruminobacter, and Succinivibrio, while inhibited acetate-producing bacteria such as Ruminococcus, altered the pattern of volatile fatty acid (VFA) synthesis in the rumen, and reduced H2 availability for methanogenesis and promoted propionate production, indirectly alleviating CH4 production. Moreover, by suppressing the relative abundance of Methanobrevibacter, CH4 production in the rumen was directly suppressed. Furthermore, KEGG pathway analysis showed that A. taxiformis significantly inhibited the abundance of K00399, methyl-coenzyme M reductase alpha subunit, which directly inhibited CH4 synthesis. Metabolomics analysis of A. taxiformis supplementation significantly enriched ketoglutarate, malate, isocitrate, and melatonin, which may have reduced the release of rumen fermented H2, thereby mitigating CH4 emissions. In summary, freeze-dried treatment A. taxiformis at the 5% supplementation level achieved the optimal balance between CH4 mitigation and rumen fermentation efficiency.
1 Introduction
The agricultural sector is a major source of CH4 emissions, with livestock production contributing 37% of global CH4 emissions (Sun et al., 2023). Ruminal fermentation in ruminants is livestock’s primary source of CH4 emissions, resulting in a 2%–12% loss of total energy intake (Johnson and Johnson, 1995). Among all anthropogenic CH4 emissions, ruminant fermentation accounts for approximately 33%, making it the second largest source after fossil fuel activities (Crippa et al., 2021), while CH4 is 28 times more thermogenic than carbon dioxide (CO2). Therefore, effective CH4 mitigation strategies are crucial to reducing environmental hazards and enhancing the growth performance of ruminants. The rumen of ruminants is a complex ecosystem in which bacteria facilitate carbohydrate decomposition, and methanogenic archaea collaborate with these bacteria to produce CH4 by utilizing H2 generated through bacterial fermentation (Lyu and Liu, 2018). Dietary modulation of rumen microbiota represents the most viable strategy for CH4 mitigation, with feed additives demonstrating particular promise (Patra et al., 2017). Among these, chemical inhibitors have shown marked efficacy: 3-Nitrooxypropanol (3-NOP) reduces emissions by 20%–60% through competitive inhibition of methyl-coenzyme M reductase (MCR) (Jayanegara et al., 2018), while melatonin (10−3 M) achieves 50% reduction by decreasing Methanobacterium abundance and protozoa number (Fu et al., 2023). Natural additives, particularly seaweeds, exhibit comparable potential. The brown seaweed Sargassum mcclurei (2% DM) by decreasing Methanobacterium abundance and increasing propionate-producing bacteria, leading to an 18.85% CH4 reduction (Li et al., 2024).
Intriguingly, recent studies have demonstrated that the red seaweed A. taxiformis exhibits a potent inhibitory effect on CH4 emissions, capable of mitigating over 95% of CH4 when added to the diets (under both in vivo and in vitro experimental conditions) (Machado et al., 2016b; Kinley et al., 2020; Min et al., 2021; Glasson et al., 2022). The A. taxiformis is rich in halomethanes, haloalkanes, haloketones and haloacids, of which bromoform (CHBr3) is considered to be the most abundant (Woolard et al., 1979). These active ingredients confer on A. taxiformis the ability to efficiently inhibit CH4 by affecting the abundance of methanogenic archaea and by reacting with reduced vitamin B12 to produce an enzymatic inhibition that limits the rate of the methyltransferase step in CH4 synthesis (Wood et al., 1968). The efficacy of A. taxiformis in mitigating rumen CH4 emissions is significantly influenced by both supplementation levels (Machado et al., 2016b; Makkar et al., 2016), and treatment methods. Treatments at different temperatures (freeze-dried and dried) significantly affected the volatilization of methane-inhibiting haloalkanes and the cellular integrity of A. taxiformis (Vucko et al., 2017). Currently, freeze-dried treatment A. taxiformis is widely used as a CH4 inhibitor (Kinley et al., 2016b; Machado et al., 2016b; Kinley et al., 2020). However, considering the added cost of the application, it is also necessary to evaluate the CH4 emission mitigation effect of dried treatment (more convenience and low cost) of A. taxiformis.
We hypothesized that different treatments and supplementation levels may have different inhibitory effects on CH4 due to different levels of retention and content of bioactive compounds.
Therefore, the objectives of this study were to evaluate the effect of different treatments and supplementation levels of A. taxiformis on mitigating CH4 emissions and to assess the effect on rumen fermentation by analyzing in vitro rumen fermentation gas production, nutrient degradation, and rumen fermentation parameters. The mechanism of A. taxiformis in inhibiting rumen CH4 production was explored using metagenomics and metabolomics approaches.
2 Materials and methods
2.1 Animal experiment permit
This study was conducted in August 2023 at the Institute of Animal Science, Guangdong Academy of Agricultural Sciences, Guangzhou, China. Three Holstein cows were used in the experiment. The animal experiment protocol complied with the requirements of experimental animal welfare and ethics, as well as the regulations of the Ministry of Science and Technology of China. It was performed following the European Directive 2010/63/EU and S.I. No. 543 of 2012.
2.2 Collection and preparation of Asparagopsis taxiformis
We focused on A. taxiformis because it is a characteristic species of Guangdong coastal ecosystems, and more importantly, it exhibits exceptional performance in mitigating CH4 emissions. Accordingly, in this experiment, the red seaweed A. taxiformis was used as a supplement to mitigate ruminal CH4 emissions. The seaweed was harvested from Naozhou Island (Zhanjiang, Guangdong, China, 20°54′–21°10′N, 109°00–109°15′E). Asparagopsis taxiformis was submerged in fresh water for 1 min and then dried with a roller. The dried seaweed was divided into two parts, with one part subjected to conventional dried and the other to freeze-dried. Samples were dried at 65°C for 48 h as a dried treatment, and samples were frozen at −80°C overnight, then freeze-dried by Christ ALPHA2-4LSC (Chirst, Germany) at −50°C for 48 h under 0.1 mbar vacuum. Both treatments resulted in the seaweed being milled to a 1 mm particle size (Machado et al., 2018) and stored at −20°C until use in subsequent experiments.
2.3 Experimental design and treatment
2.3.1 Seaweed bioactive components analysis
The determination of bioactive compounds in A. taxiformis samples was performed by Boende Testing Center (Qingdao, China). For FT-IR characterization, samples were homogenized with potassium bromide (1:100 w/w) and pressed into pellets and analyzed using a NiColet iS5 (Thermo Scientific, United States). For HPLC analysis, 200 mg of each sample were subjected to methanol extraction (20 mL, 10 min ultrasonication), filtered through 0.45 μm membranes, and analyzed using an Agilent 1260 (Agilent, United States) equipped with an SB-C18 column (250 mm × 4.6 mm, 5 μm). Subsequent LC-MS analysis was conducted on a Thermo TRACE 1610 (Thermo Scientific, United States) with a TG-5SILMS column (30 m × 0.25 mm × 0.25 μm), employing EI ionization at 1.0 mL/min flow rate. The mobile phase consisted of (A) 0.1% formic acid with 5 mM ammonium acetate and (B) methanol: acetonitrile (1:1 v/v), with detection in SCAN mode.
2.3.2 In vitro rumen fermentation system
On the day of the experiment, to minimize individual variation, we selected three healthy Holstein cows with similar age (2.8 ± 0.5 years) and body weight (510 ± 25 kg) that were fed the same diet. The rumen fluid was collected 2 h after morning feeding. Rumen fluid samples were from donor animals using a stomach tube-based rumen fluid sampler (Anscitech, China). The sampler was orally inserted through the esophagus into the rumen following proper restraint. Approximately 200 mL of rumen fluid was manually aspirated from the rumen using a sterile syringe, with the first 50 mL of collected fluid being discarded to minimize salivary contamination. Between sampling procedures, the device was thoroughly rinsed with warm distilled water to prevent cross-contamination. The rumen fluids collected from the three cows were pooled in equal volumes (1:1:1), filtered through four layers of sterile gauze. The mixed fluid was then stored in a CO₂ flushed thermos preheated to 39°C for immediate transport to the laboratory.
The rumen buffer solution was prepared according to the method described by Menke and Steingass (1988). The solution is consisted of 400 mL H₂O, 0.1 mL solution A (containing CaCl₂·2H₂O 13.2 g/L, MnCl₂·4H₂O 10.0 g/L, CoCl₂·6H₂O 1.0 g/L, and FeCl₃·6H₂O 8.0 g/L), 200 mL solution B (NaHCO₃ 39 g/L), and 200 mL solution C (Na₂HPO₄ 5.7 g/L, KH₂PO₄ 6.2 g/L, MgSO₄·7H₂O 0.6 g/L), 1 mL resazurin (0.1% w/v) and 40 mL reducing solution (95 mL H₂O, 4 mL 1 N NaOH, and 625 mg Na₂S·9H₂O).
The rumen fluid and buffer solution are mixed evenly in a ratio of 1:2 (25 mL:50 mL). The mixing process is always carried out under constant temperature water at 39°C and carbon dioxide flushing. This study used fermentation bottles (100 mL), each containing 500 mg of fermentation substrate (forage: concentrates = 60:40) (Choi et al., 2022) and 75 mL of mixed rumen fluid. The fermentation substrate consisted of corn straw and concentrate supplementation. Both components were dried, milled to 1 mm, and stored in a desiccator until use in subsequent experiments. Different treatments of A. taxiformis (DM) were supplemented at 2, 5, and 10% (Low, Mid, and High) of the fermentation substrate DM. The control group (CON) without supplemented with A. taxiformis. Each supplementation level had 6 replicates, totaling 42 fermentation bottles, the experiment was repeated twice and 6 samples were randomly selected for subsequent experimental analysis. The bottle is filled with carbon dioxide and sealed with a butyl stopper and an aluminum cap to ensure the anaerobic environment required for fermentation. This study of in vitro rumen fermentation was carried out for 48 h in a constant temperature water shaker at 39°C and 85 rpm. In this experiment, the nutrient composition of the fermentation substrate is shown in Table 1.
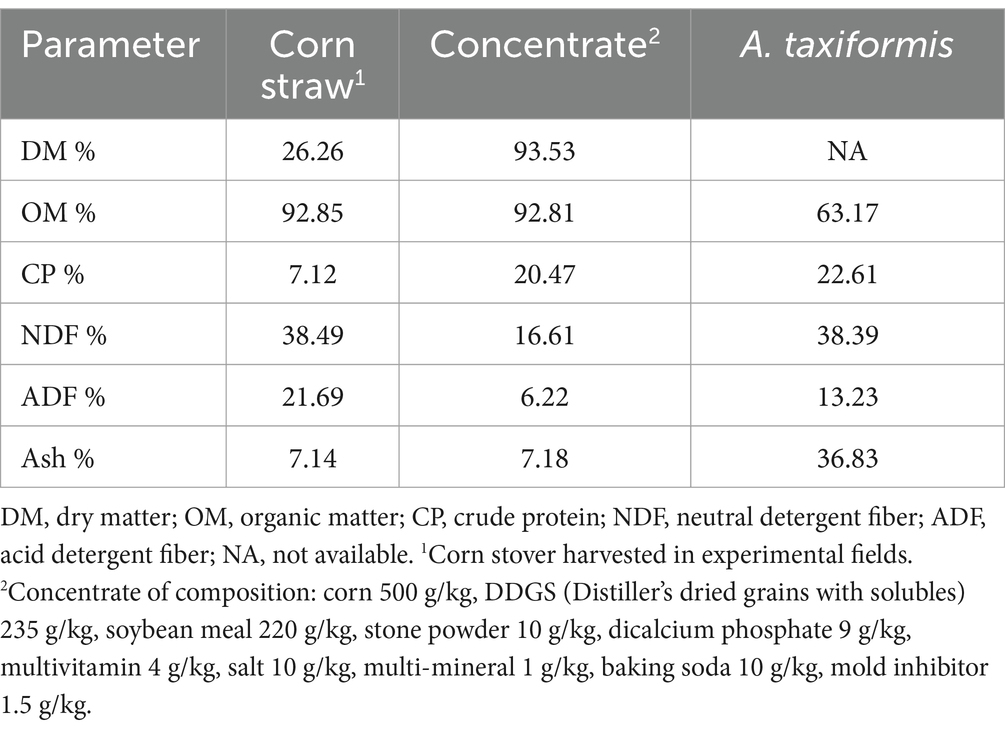
Table 1. Chemical composition of substrates and Asparagopsis taxiformis used in the in vitro rumen fermentation (DM basis).
2.3.3 Gas collection and composition analysis
At 2, 4, 8, 12, 24, and 48 h of the experiment, the total gas production (TGP) by fermentation in each bottle was collected using a 30 mL syringe. The gas sample was stored in aluminum foil airbags until analysis. The gas composition (CH₄, CO₂, and H₂) was analyzed using an SP-2060 T Gas Chromatograph (Tianpu, China) equipped with a thermal conductivity detector (TCD), and two serially connected stainless steel columns: a 5A molecular sieve column (3 mm × 3 m, 60–80 mesh Chromosorb) followed by a TDX-01 carbon molecular sieve column (3 mm × 1 m, 60–80 mesh Chromosorb). The analysis was performed under isothermal conditions at 100°C with the detector maintained at 100°C. High-purity argon (≥99.999%) was used as carrier gas at a flow rate of 30 mL/min and operating pressure of 0.5 MPa. Gas samples (1 mL) were injected using gas-tight syringes. Quantification was achieved by external calibration with certified standard gas mixtures containing 10% CH4, 20% CO2, and 2% H2 in argon balance. TGP, CH4, CO2, and H2 volume normalized on a dry matter basis (mL/g DM).
2.3.4 Fermentation substrate chemical analysis
The DM content of the seaweed and fermentation substrate was determined by achieving constant weight at 105°C (AOAC, 2000). Organic matter was measured as the loss in the muffle furnace (Yiheng, China) at 550°C for 8 h (AOAC, 2000). Neutral detergent fiber, and acid detergent fiber (NDF and ADF), were measured according to the method described by Van Soest et al. (1991) using the ANKOM 200i Fiber Analyzer (ANKOM Technology, United States). Crude protein (CP) was determined by KjelMaster K-375 (BUCHI, CH) and the nitrogen concentration was multiplied by the conversion factor of 6.25 (Mariotti et al., 2008). After the fermentation is completed, the nylon bags were rinsed with laboratory tap water until the water ran clear, then dried at 65°C for 48 h and the remaining substrate was weighed. The dry matter disappearance (DMD), neutral detergent fiber degradation (NDFD), acid detergent fiber degradation (ADFD) and crude protein degradation (CPD) during the fermentation process are calculated. The DM, NDF, ADF and CP contents in the fermentation residue were measured by the above methods.
DMD (%) was determined by weight difference before and after fermentation, with corrections made for blank bag losses.
2.3.5 Rumen fermentation parameters analysis
After fermentation, put the bottle in an ice water bath for 30 min to stop fermentation. The pH of rumen fluid was immediately measured using a calibrated PHSJ-4F pH meter (Leici, China). The remaining fermentation substrate was collected in nylon bags for further analysis. Individual fermentation fluid samples were stored at −80°C for VFA, ammonia (NH3-N), rumen microbial protein (MCP), metagenomic, and metabolomics analysis. For VFA concentration analysis, the sample was centrifuged at 5,400 rpm for 10 min. Then, 1 mL of the supernatant and 0.2 mL of the metaphosphoric acid solution containing 2-ethylbutyric acid (2 g/L) as internal standard were taken, mixed, and placed in an ice water bath for more than 30 min. The VFA concentration was centrifuged again at 10,000 rpm for 10 min, and the supernatant was used to measure the VFA concentration using Agilent 6890 N (Agilent, United States). Gas chromatography operating conditions have been described by Erwin et al. (1961). NH3-N concentration was determined using the UV-2600 Spectrophotometer (Unico, China) method as described by Broderick and Kang (1980). MCP concentration was determined using the Coomassie Brilliant Blue method with bovine serum albumin (BSA) as standard (Sedmak and Grossberg, 1977). A standard curve (0–1,000 μg/mL BSA) was established for quantification. 100 mg Coomassie Brilliant Blue in 50 mL 95% ethanol, then adding 100 mL 85% phosphoric acid, and diluting to 1 L with distilled water. Rumen fluid samples were centrifuged at 12,000 × g for 15 min. Then, 100 μL supernatant was mixed with 1 mL dye reagent, incubated for 10 min, and measured at 595 nm Spectronic 200 (Thermo Scientific, United States).
2.3.6 DNA extraction, library construction, and metagenomic sequencing
According to the manufacturer’s instructions, total genomic DNA was extracted from rumen fermentation fluid samples using the MagAtrract PowerSoil Pro DNA Kit (Omega Bio-tek, Norcross, GA, United States). DNA purity was verified with A260/A280 ratios of 1.8–2.0 and A260/A230 ratios >1.7 to exclude protein or carbohydrate contamination, coupled with TBS-380 fluorometer quantification (≥20 ng/μL).
DNA extract was fragmented to an average size of about 400 bp using Covaris M220 (Gene Company Limited, China) for paired-end library construction. Paired-end library was constructed using NEXTFLEX Rapid DNA-Seq (Bioo Scientific, Austin, TX, United States). Adapters containing the full complement of sequencing primer hybridization sites were ligated to the blunt end of fragments. Paired-end sequencing was performed on Illumina NovaSeq (Illumina Inc., San Diego, CA, United States) at Majorbio Bio-Pharm Technology Co., Ltd. (Shanghai, China) using NovaSeq 6000 S4 Reagent Kit v1.5 (300 cycles) according to the manufacturer’s instructions.1 Sequence data associated with this project have been deposited in the NCBI Short Read Archive database (Accession Number: PRJNA1148834).
2.3.7 Quality control sample and UHPLC-MS/MS analysis
The LC-MS/MS analysis of the sample was conducted on a Thermo UHPLC-Q Exactive HF-X system equipped with an ACQUITY HSS T3 column (100 mm × 2.1 mm i.d., 1.8 μm; Waters, United States) at Majorbio Bio-Pharm Technology Co. Ltd. (Shanghai, China). Each sample was spiked with internal standards (2-chloro-L-phenylalanine, lidocaine, and glyceryl trioleate at 1 μg/mL) for quantification and system monitoring.
The pretreatment of LC/MS raw data was performed by Progenesis QI (Waters Corporation, Milford, United States) software. To control batch effects, samples were randomized across acquisition sequences with pooled quality control (QC) samples analyzed every 10 injections. Data normalization combined internal standard correction and QC-based LOESS regression. A three-dimensional data matrix in CSV format was exported. This three-dimensional matrix included: sample information, metabolite name, and mass spectral response intensity. Internal standard peaks and any known false positive peaks (including noise, column bleed, and derivatized reagent peaks) were removed from the data matrix, deredundant, and peak pooled. At the same time, the metabolites were identified by searching databases, and the main databases were the HMDB,2 Metlin,3 and Majorbio Database.
2.4 Statistical analysis
The resulting model was analyzed with an ANOVA. For nutrient degradation (DMD, NDFD, and ADFD), total gas produced, gas composition (CH4, CO2, and H2), and fermenter parameters (pH, VFA, NH3-N, and MCP), data were tested for normality (Shapiro–Wilk) and statistically significant mean differences were tested for using parametric ANOVA (with Dunn) as appropriate. All ANOVA analyses were performed using IBM SPSS (27.0) software. Statistically significant means were considered when p < 0.05, while p < 0.1 was considered a tendency toward statistical significance. The metagenome and metabolome data were processed by Shanghai Meiji Company. The data were analyzed on the free online platform of Majorbio Cloud Platform (https://cloud.majorbio.com, accessed May 10, 2024).
3 Results
3.1 Effect of different treatments on the bioactive components of Asparagopsis taxiformis
The top 10 bioactive components of the compositional ratio of A. taxiformis after different treatments are listed in Table 2. The main bioactive components in freeze-dried A. taxiformis are Bromoacetic acid (10.28%), Catechi (6.25%), Rosmarinic acid (3.48%), Dibromoiodomethane (2.91%), Coumaric acid (2.71%), P-Bromophenol (2.36%), Hesperidine (2.32%), 2,4,6-Tribromothiophenol (2.28%), Daidzein (2.26%), 2,4-Dibromophenol (1.78%), respectively.
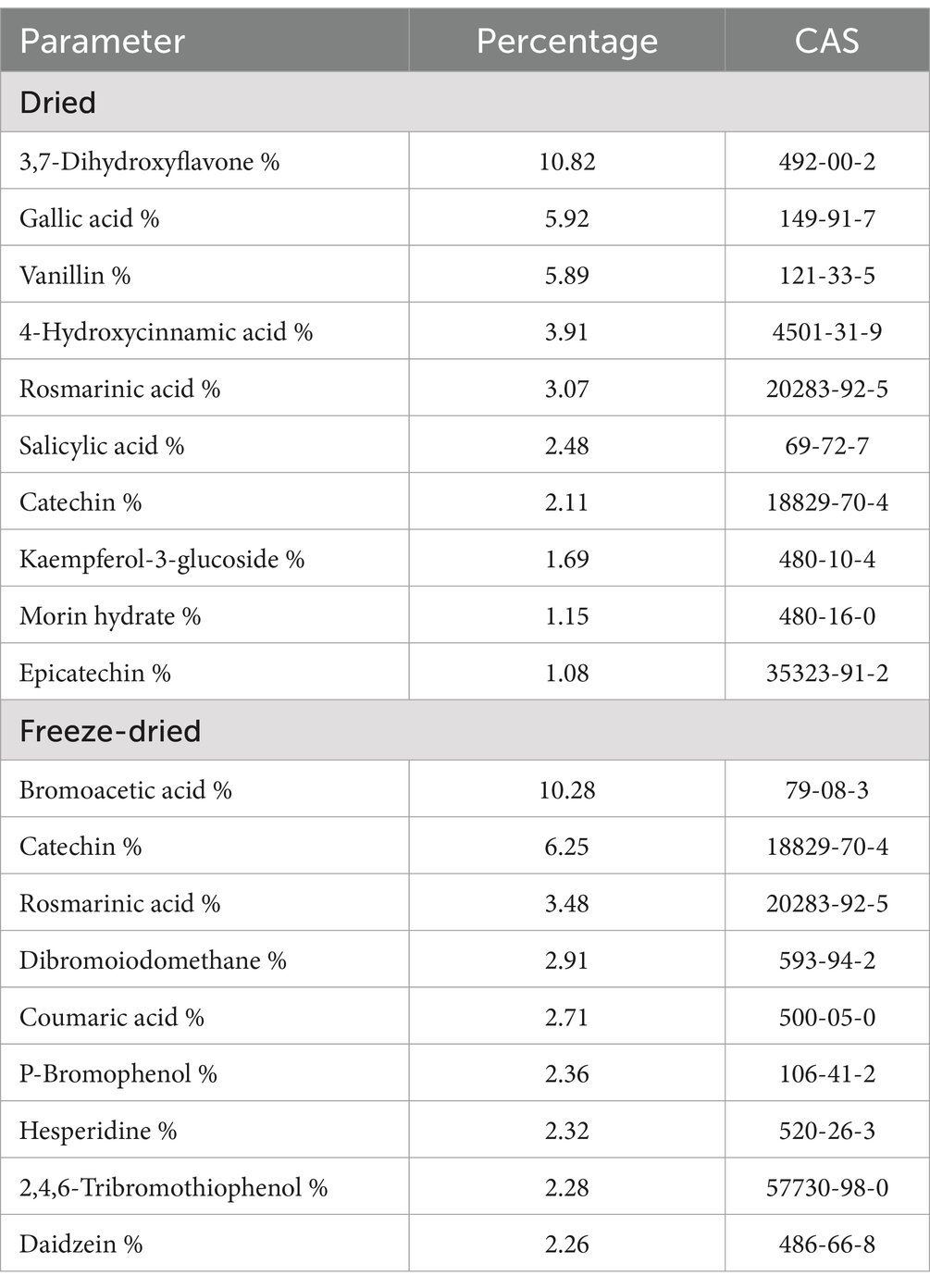
Table 2. Effect of different treatments on composition of the main bioactive components (top 10) in Asparagopsis taxiformis.
The main bioactive components in dried A. taxiformis are 3,7-Dihydroxyflavon (10.82%), Gallic acid (5.92%), Vanillin (5.89%), 4-Hydroxycinnamic acid (3.91%), Rosmarinic acid (3.07%), Salicylic acid (2.48%), Catechin (2.11%), Kaempferol-3-glucoside (1.69%), Morin hydrate (1.15%), Epicatechin (1.08%), respectively.
3.2 Effect of Asparagopsis taxiformis supplementation on gas production parameters and CH4 production
In the current study, the total gas production (TGP) in the CON was 293.02 ± 12.02 mL/g DM. TGP in the Mid and High groups after supplementation dried treatment of A. taxiformis was 243.63 ± 21.02 and 189.72 ± 40.10 mL/g DM respectively, significantly lower than that in the CON (p < 0.01) (Figure 1A). Supplementation with dried A. taxiformis significantly reduced CH4 production (Figure 1B). Compared with the CH4 production of 26.89 ± 1.56 mL/g DM in the control group, the Low group (27.65 ± 3.21 mL/g DM) did not alleviate the methane production. The Mid group produced 17.51 ± 2.77 mL/g DM of CH4, and the High group produced 4.61 ± 1.07 mL/g DM. Compared with the CON, the CH4 production decreased by 34.52% (p < 0.01) and 82.89% (p < 0.01) in the Mid and High groups, respectively.
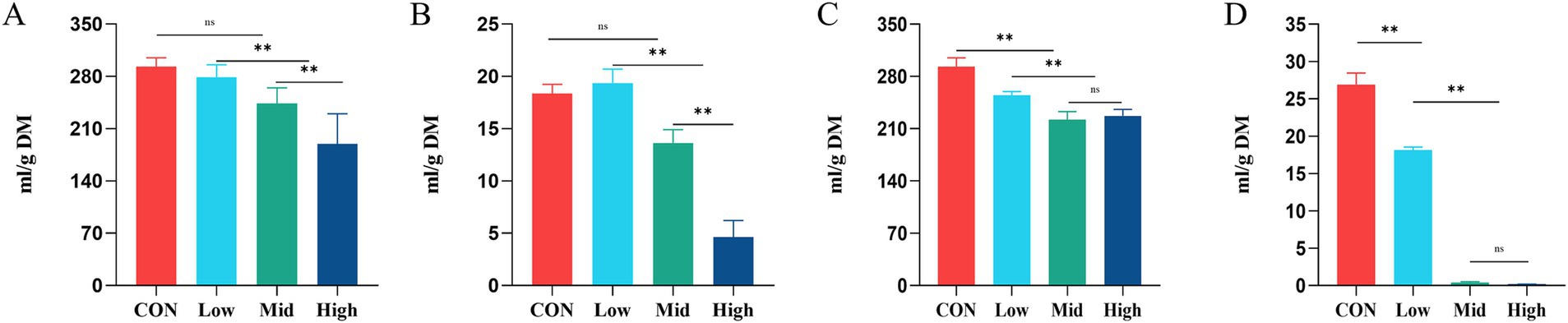
Figure 1. Effect of different treatments and supplementation levels of Asparagopsis taxiformis on total gas production (TGP) and methane (CH4) emission from in vitro rumen fermentation. (A) Total gas TGP at dried treatment. (B) CH4 emission at dried treatment. (C) TGP at freeze-dried treatment. (D) CH4 emission at freeze-dried treatment. CON, control group; Low, CON plus 2% A. taxiformis; Mid, CON plus 5% A. taxiformis; High, CON plus 10% A. taxiformis. *<0.05, **<0.01, ***<0.001.
Unlike the dried A. taxiformis, the freeze-dried A. taxiformis showed the greater potential to reduce the TGP and CH4. As shown in Figure 1C, all three freeze-dried A. taxiformis supplementation levels significantly reduced TGP from in vitro rumen fermentation. Compared with CON, the TGP of Low, Mid, and High groups were decreased by 13.02% (p < 0.01), 24.18% (p < 0.01), and 22.56% (p < 0.01), respectively. The Low group produced 18.11 ± 0.45 mL/g DM of CH4, and the reduction effect reached 32.44% (p < 0.01). The CH4 production was almost completely suppressed in the Mid and High groups, and only 0.38 ± 0.12 and 0.17 ± 0.02 mL/g DM CH4 were produced. The CH4 reduction reached 98.53% (p < 0.01) and 99.33% (p < 0.01), respectively (Figure 1D).
In the current study, the potential of freeze-dried A. taxiformis to mitigate methane emissions is significantly better than that of dried treatment at the same supplementation level. When supplementation dried A. taxiformis, the H2 production increased significantly only in the High group (p < 0.01). When supplementing the freeze-died treatment of A. taxiformis, the H2 production increased significantly in both the Mid group (p < 0.01) and High group (p < 0.01). Furthermore, the production of CO2 was significantly reduced only in the freeze-dried treatment High group (p < 0.01). Supplementary Table S1 presents the effect of A. taxiformis supplementation on the gas production parameters observed in this study.
3.3 Effect of Asparagopsis taxiformis supplementation on nutrient degradation
Supplementing with A. taxiformis significantly inhibits CH4 emissions, making it necessary to investigate further whether its supplementation negatively affects nutrient degradation and rumen fermentation and explore the mechanisms behind the reduction in CH4 emissions. The negative impact of A. taxiformis supplementation on nutrient degradation was affected by the treatment method and supplementation level. As shown in Table 3, when the supplementation level was greater than 5%, a decrease in DMD, NDFD, ADFD, and CPD was observed in both dried and freeze-dried treatments. A high level of supplementation significantly inhibits the degradation of these nutrients. In the dried treatment, the High group reduced DMD, NDFD, ADFD, and CPD by 3.69% (p < 0.01), 11.25% (p < 0.01), 7.70% (p < 0.01), and 36% (p < 0.01), respectively.
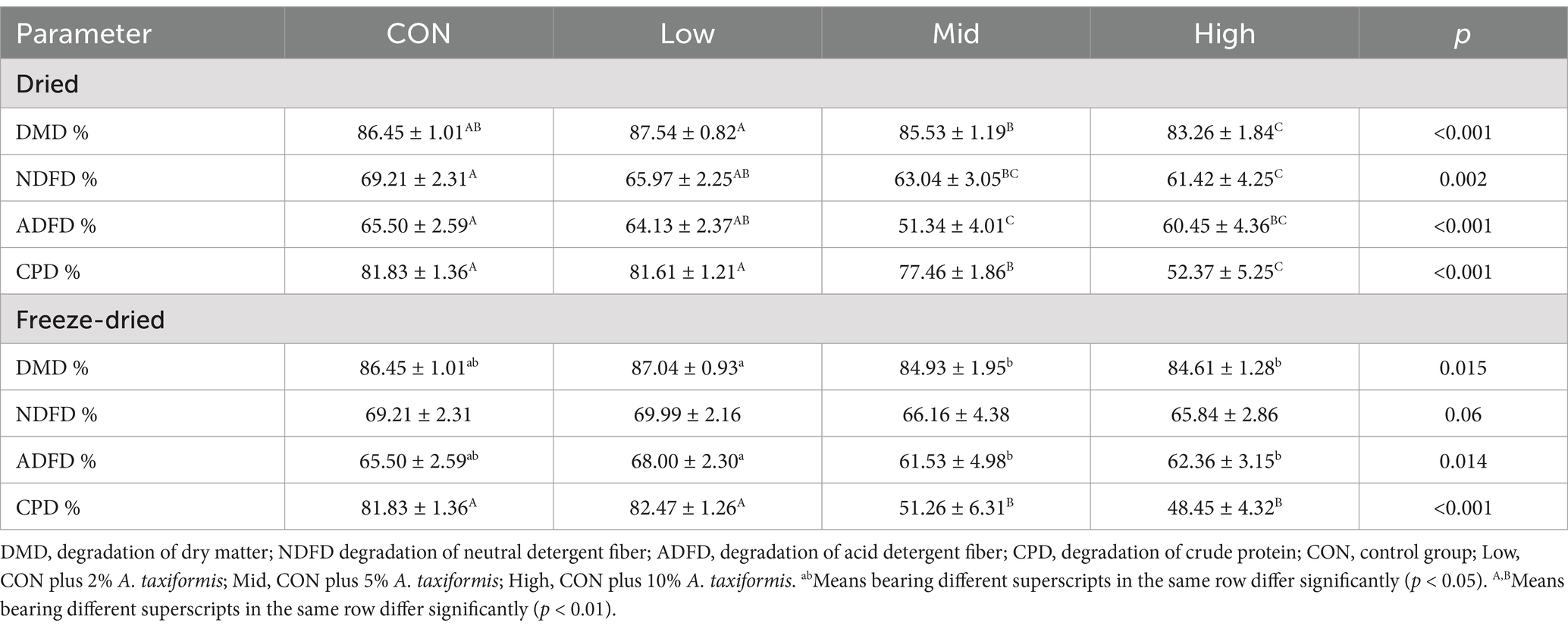
Table 3. Effect of the two treatments and different supplementation levels Asparagopsis taxiformis on nutrient degradation in vitro rumen fermentation.
In addition, with supplementation freeze-dried A. taxiformis, the inhibitory effect on degradation of nutrients except CP is alleviated, and the high group reduced DMD, NDFD, and ADFD by 2.12% (p < 0.01), 4.86% (p < 0.01), and 4.79% (p < 0.01), respectively. It is worth noting that CPD was significantly reduced in both freeze-dried Mid and High groups, which were reduced by 37.35% (p < 0.01) and 40.79% (p < 0.01) compared with the CON.
3.4 Effect of Asparagopsis taxiformis supplementation on rumen fermentation parameters
Rumen fermentation parameters are important indicators of rumen internal environment stability and can reflect changes in rumen fermentation function. Adding A. taxiformis affects rumen CH4 production and rumen fermentation parameters from in vitro rumen fermentation (Table 4). After the dried treatment, the supplementation of A. taxiformis in the Low and Mid groups significantly reduced the pH of rumen fluid (p < 0.01). NH3-N, MCP, and total VFA concentrations were not affected, but individual VFA concentrations changed along with supplementation levels. The acetate, isobutyrate, and isovalerate concentrations were all significantly reduced (p < 0.05). The Mid group produced 32.73 ± 3.87 mmol/L of acetate and 0.83 ± 0.11 mmol/L of isobutyrate, and the High group produced 1.35 ± 0.14 mmol/L of isovalerate both of which were the lowest levels in the dried. The decrease in acetate concentration was the main reason for the lowest total VFA in the Mid group. The ratio of acetate to propionate (A:P ratio) in the CON was 2.65, and with increasing supplementation levels, the A:P ratio decreased to 2.26, 2.19, and 1.99 (p = 0.04), respectively.
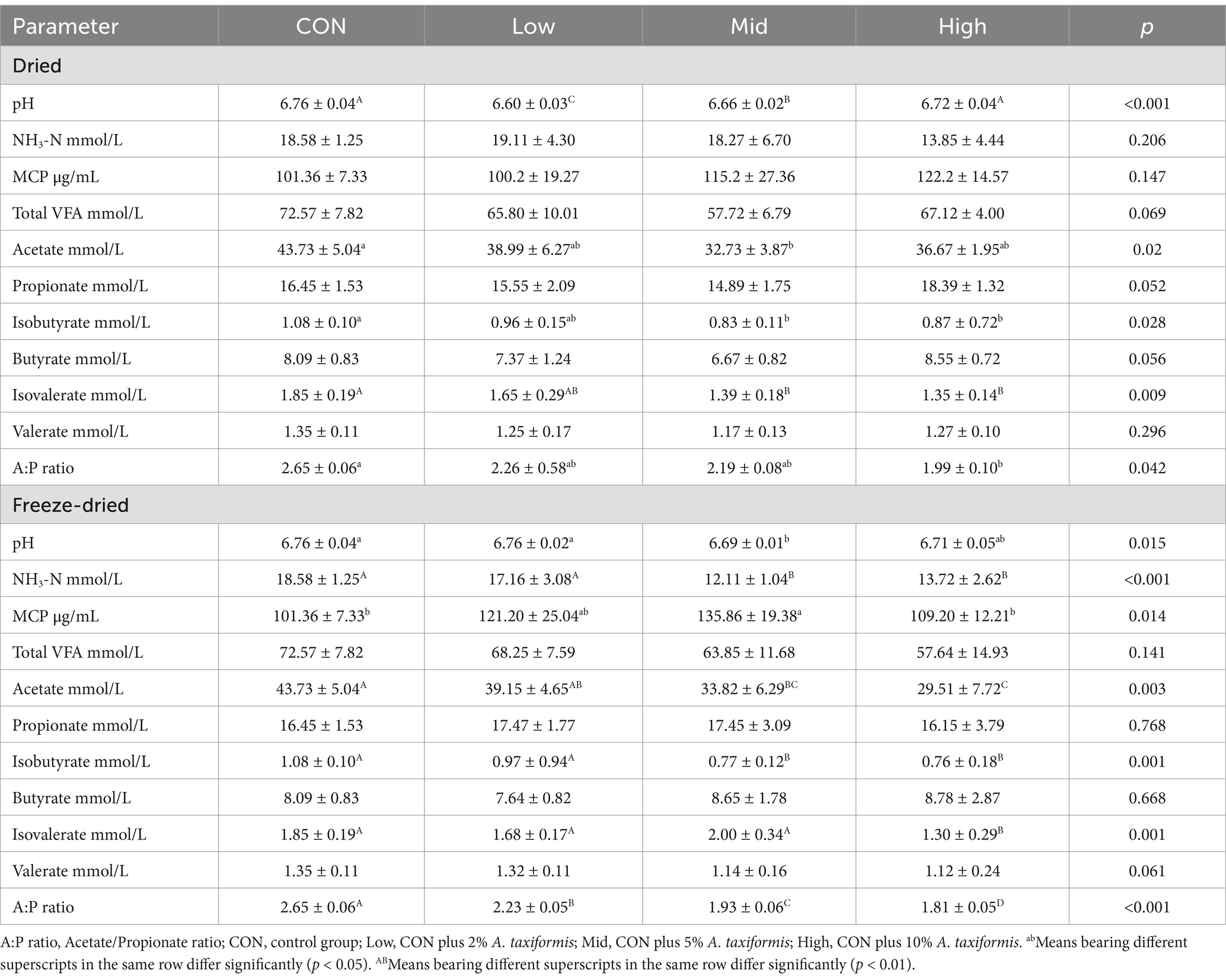
Table 4. Effect of the two treatments and different supplementation levels Asparagopsis taxiformis on pH, NH3-N, MCP, and VFA profiles in vitro.
The changes in pH and NH3-N in the freeze-dried maintained the same trend, both decreasing in the Mid and High groups and lowest in the Mid group (Table 4). The NH3-N concentration in the Mid and High groups was reduced by 34.82% (p < 0.01) and 26.15% (p < 0.01) compared with the CON. However, the MCP concentration in the Mid group was 135.86 ± 19.38 μg/mL, which was 34.03% (p < 0.05) higher than that in the CON (101.36 μg/mL). The concentration of total VFA has a downward trend with increasing supplementation levels, but it is not significant (p = 0.141). Increased supplementation levels caused significant decreases in acetate (p < 0.01), isobutyrate (p < 0.01), and isovalerate (p < 0.01) concentrations. In the High group, the concentration of acetate was 29.51 ± 7.72 mmol/L, which was 32.51% lower than the 43.73 ± 5.04 mmol/L in the CON, while isobutyrate and isovalerate also decreased by 29.62% and 29.72%. In the freeze-dried treatment, the acetate is significantly reduced, but the propionate does not change significantly, which causes a significant decrease in the A:P ratio (p < 0.01). After supplementing three levels of A. taxiformis, the A:P ratios were 2.23, 1.93, and 1.81, respectively.
Based on the above experimental results, we suggest that freeze-dried treatment of A. taxiformis is more effective in alleviating CH4 emissions. However, the High group showed a huge negative impact on rumen fermentation and was not fit for application, thus we selected the Low and Mid groups samples for metagenomic testing to further analyze the function and mechanism of A. taxiformis in decreasing CH4 production.
3.5 Effect of Asparagopsis taxiformis supplementation on the composition of microbial communities
3.5.1 Bacterial community effects under Asparagopsis taxiformis supplementation
The study mainly observed the effect of supplementing A. taxiformis on the community structure of rumen bacteria at the genus level. In terms of bacterial α-diversity, the Ace index (Figure 2A) and Chao index (Figure 2B) had no significant differences among the three groups (p > 0.05). The Mid group significantly increased the Shannon index (p < 0.01) (Figure 2C). It also significantly decreased the Simpson index (p < 0.01) (Figure 2D), but there was no significant difference between the CON and Low groups. In terms of bacterial β-diversity, the results showed that the microorganisms in the three groups were separated (R = 0.772, p < 0.01) (Figure 2E). The Venn diagram shows that at the genus level, the three groups have a total of 2,970 common species. There were 46, 35, and 63 unique species in the CON, Low, and Mid groups, respectively (Figure 2F). At the genus level, we analyzed the ruminal top 15 species in relative abundance. Among the annotated species, Prevotella, Ruminobacter, Ruminococcus, Succinivbrio, and Eubacterium are the dominant genera in the three groups (Figure 2G). Further observation of the effect of A. taxiformis supplementation on the microbial bacterial community structure revealed that the relative abundance of the dominant bacterial genera was significantly influenced by the level of freeze-dried A. taxiformis supplementation (Figure 2H). Compared with the CON group, the Mid group significantly increased the relative abundance of Prevotella (p < 0.05). Compared with the other two groups, the Mid group had a significantly higher relative abundance of Ruminobacter (p < 0.01), and Succinivbrio (p < 0.01), and there was no difference between the CON and Low groups (p > 0.05). The relative abundance of Ruminococcus significantly decreased along with increasing levels of A. taxiformis supplementation in the three groups (p < 0.01). The relative abundance of Eubacterium in the Mid group was not different from that in the CON, but was significantly lower than in the Low group (p < 0.01). When the LDA threshold is >4, we found that Prevotella, Ruminobacter, and Succinivbrio were significantly enriched, indicating that their relative abundance increased significantly in the Mid group (Figure 2I). The changes in bacterial communities at the phylum and species levels are shown in Supplementary Figures S1, S2.
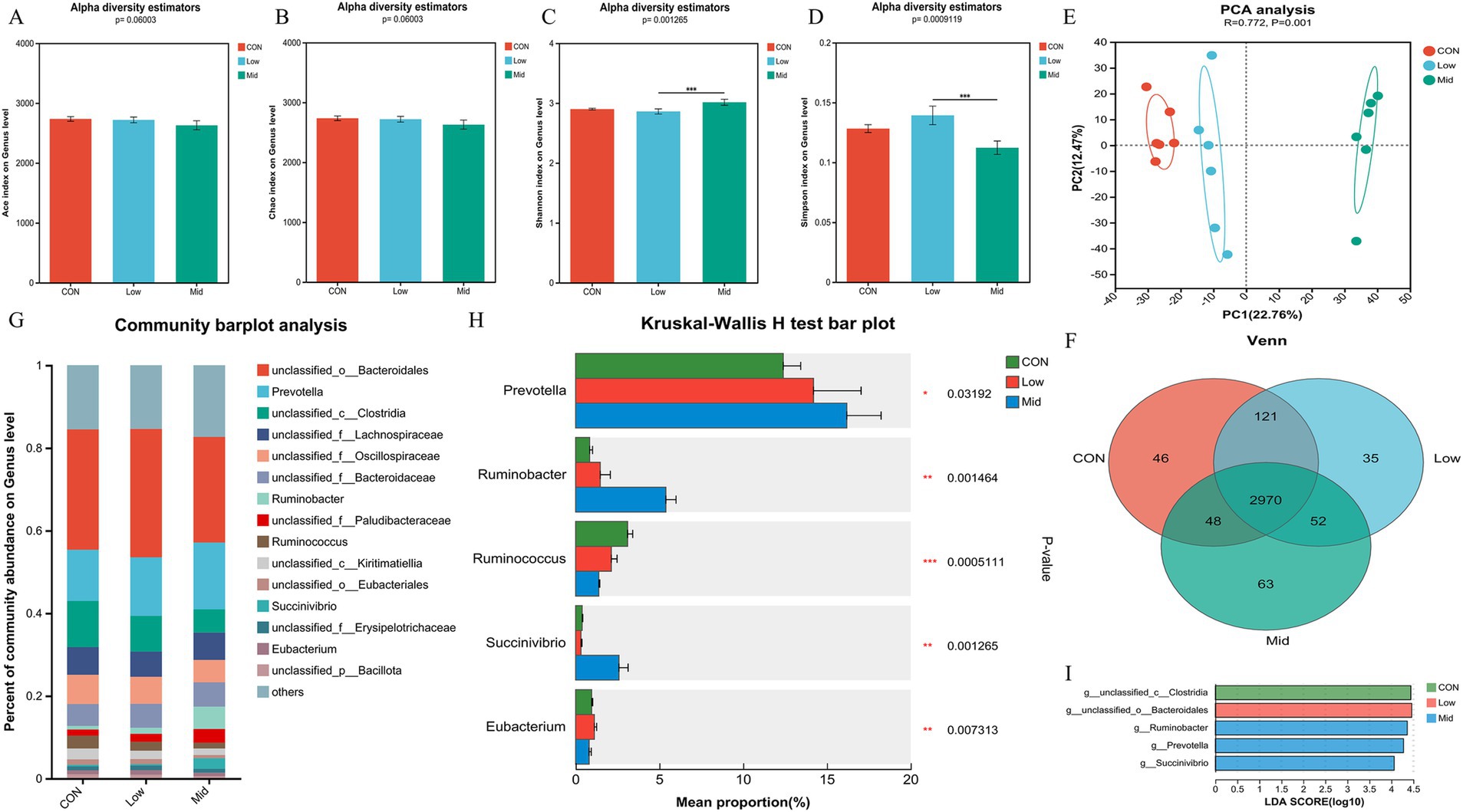
Figure 2. Effect of different treatments and supplementation levels of Asparagopsis taxiformis on rumen bacterial composition in the in vitro rumen fermentation. (A) Ace index on genus level. (B) Chao index on genus level. (C) Shannon index on genus level. (D) Simpson index on genus level. (E) Beta diversity. (F) Venn diagram on genus level. (G) Relative abundances of the 15 most abundant genus-level across all three groups. (H) Differences in bacterial genus levels by metagenomics sequencing. (I) The LDA values of different species among the three groups on genus level (LDA > 4). CON, control group; Low, CON plus 2% A. taxiformis; Mid, CON plus 5% A. taxiformis; High, CON plus 10% A. taxiformis. *<0.05, **<0.01, ***<0.001.
3.5.2 Archaeal community effects under Asparagopsis taxiformis supplementation
The effect of A. taxiformis supplementation on the archaeal community were also observed at the genus level. Different from bacteria, Ace (Figure 3A), Chao (Figure 3B), and Simpson (Figure 3D) indexes were all significantly decreased (p < 0.01), and the Shannon (Figure 3C) index was significantly increased (p < 0.01) in the Mid group. However, there was no difference between the Low and CON groups. The result of PCA principal component analysis showed that the archaeal communities of the three groups of samples were separated (R = 0.989, p < 0.01) (Figure 3E). The result of the Venn diagram showed that there were 118 archaeal species in the three groups (Figure 3F), with 3, 2, and 6 unique archaeal species, respectively. Methanobrevibacter and Methanosphaera are the dominant genera at the genus level (Figure 3G). The species difference histograms (Figure 3H) illustrate that there is no significant difference in the relative abundance of archaea in the CON and Low groups. However, in the Mid group, the relative abundance of Methanobrevibacter (p < 0.01) was significantly decreased, and the abundance of Methanosphaera (p < 0.01), Methanocorpusculum (p < 0.01), and Methanomicrobium (p < 0.01) were significantly increased. Setting the LDA score >4, the results also showed that Methanobrevibacter was significantly enriched in the CON group, and Methanosphaera was significantly enriched in the Mid group, which is consistent with the species difference results (Figure 3I). Supplementary Figures S3, S4 show the changing trends in archaea at the phylum and species level.
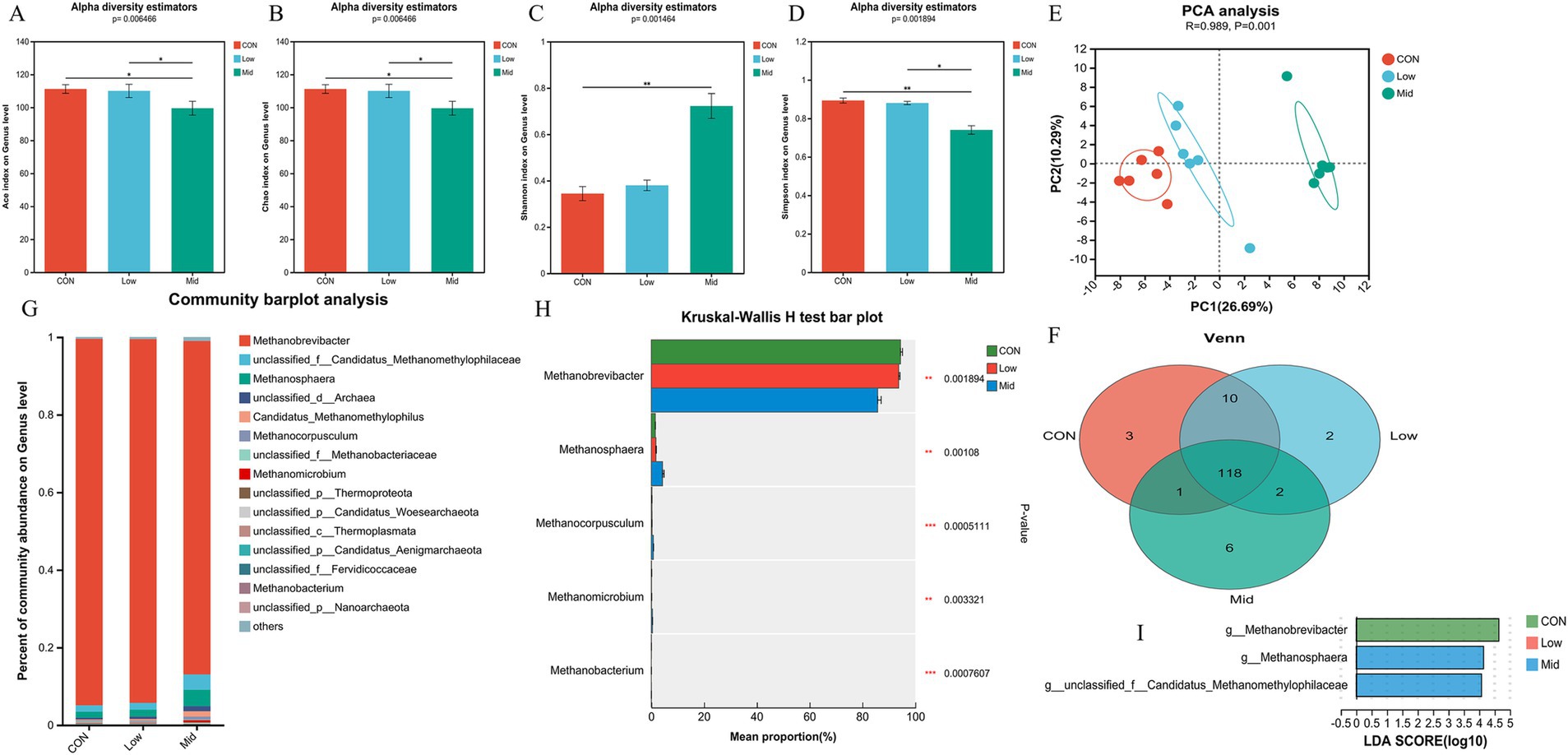
Figure 3. Effect of different treatments and supplementation levels of Asparagopsis taxiformis on rumen archaea composition in the in vitro rumen fermentation. (A) Ace index on genus level. (B) Chao index on genus level. (C) Shannon index on genus level. (D) Simpson index on genus level. (E) Beta diversity. (F) Venn diagram on genus level. (G) Relative abundances of the 15 most abundant genus-level across all three groups. (H) Differences in archaea genus levels by metagenomics sequencing. (I) The LDA values of different species among the three groups on genus level (LDA > 4). CON, control group; Low, CON plus 2% A. taxiformis; Mid, CON plus 5% A. taxiformis; High, CON plus 10% A. taxiformis. *<0.05, **<0.01, ***<0.001.
3.6 Effect of Asparagopsis taxiformis supplementation on KEGG pathways in rumen
3.6.1 KEGG functional pathway enrichment analysis
To further investigate the reasons for the reduction in methane emissions, the effect of A. taxiformis supplementation on carbon metabolism and methane metabolism in different groups were analyzed. As shown in Figure 4A, the relative abundance of some KEGG pathways such as the Biosynthesis of secondary metabolites, Biosynthesis of amino acids, and Biosynthesis of cofactors increased significantly (p < 0.01). In contrast, Microbial metabolism in diverse environments, Quorum sensing, and Purine metabolism decreased significantly (p < 0.05) in the Mid group. We primary focus on the changes in the CH4 metabolism pathway, the relative abundance of methane metabolism significantly decreased with increasing supplementation levels of A. taxiformis (p < 0.01) (Figure 4B). Gene sets related to methane metabolism were screened for further analysis. In the Mid group, the relative abundance of Metabolic pathways, Methane metabolism, Microbial metabolism in diverse environments, Carbon metabolism, Biosynthesis of secondary metabolites, and Glycolysis/Gluconeogenesis was significantly reduced (p < 0.01), and Carbon fixation pathways in prokaryotes, Pyruvate metabolism, Propanoate metabolism, and Taurine and hypotaurine metabolism was significantly increased (p < 0.01) (Figure 5A).
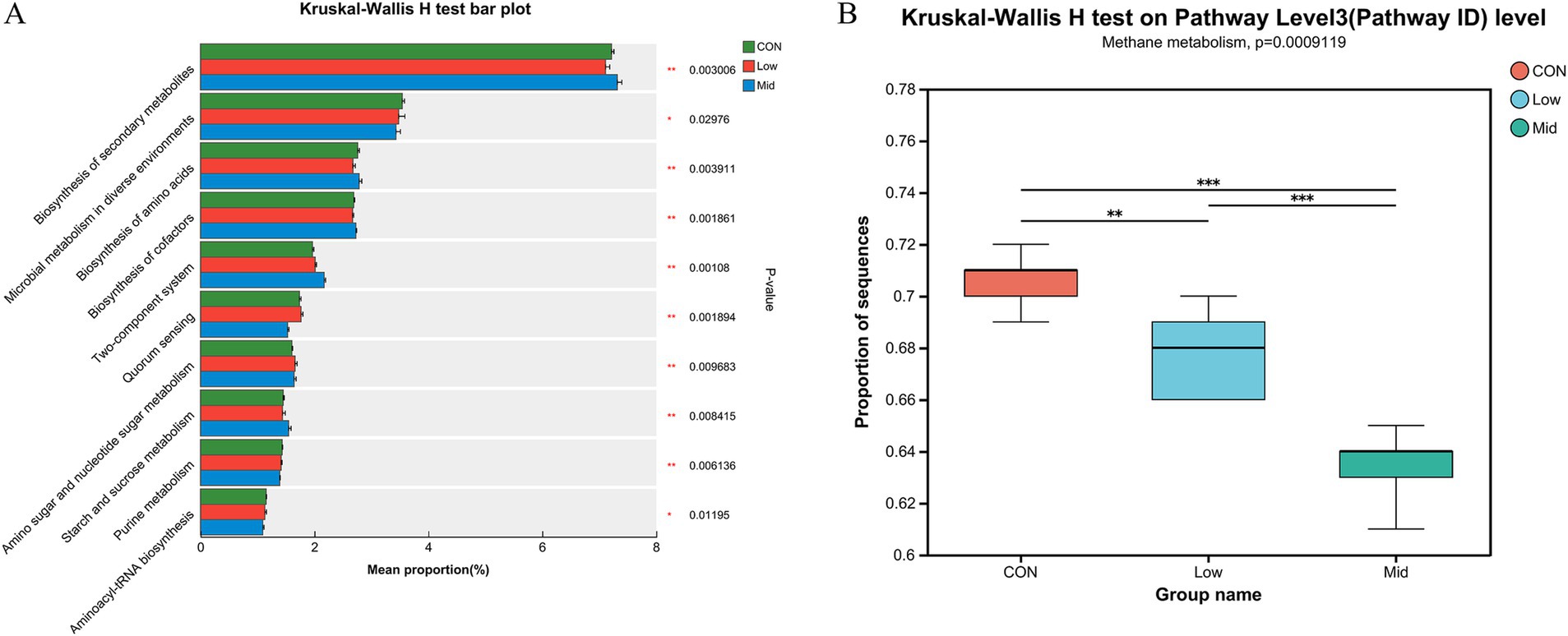
Figure 4. Effect of different levels of freeze-dried treatment Asparagopsis taxiformis in the in vitro rumen fermentation KEGG pathway. (A) Differences in pathway level 3 (Pathway ID). (B) Differences in methane metabolism among the three groups. CON, control group; Low, CON plus 2% A. taxiformis; Mid, CON plus 5% A. taxiformis; High, CON plus 10% A. taxiformis. *<0.05, **<0.01, ***<0.001.
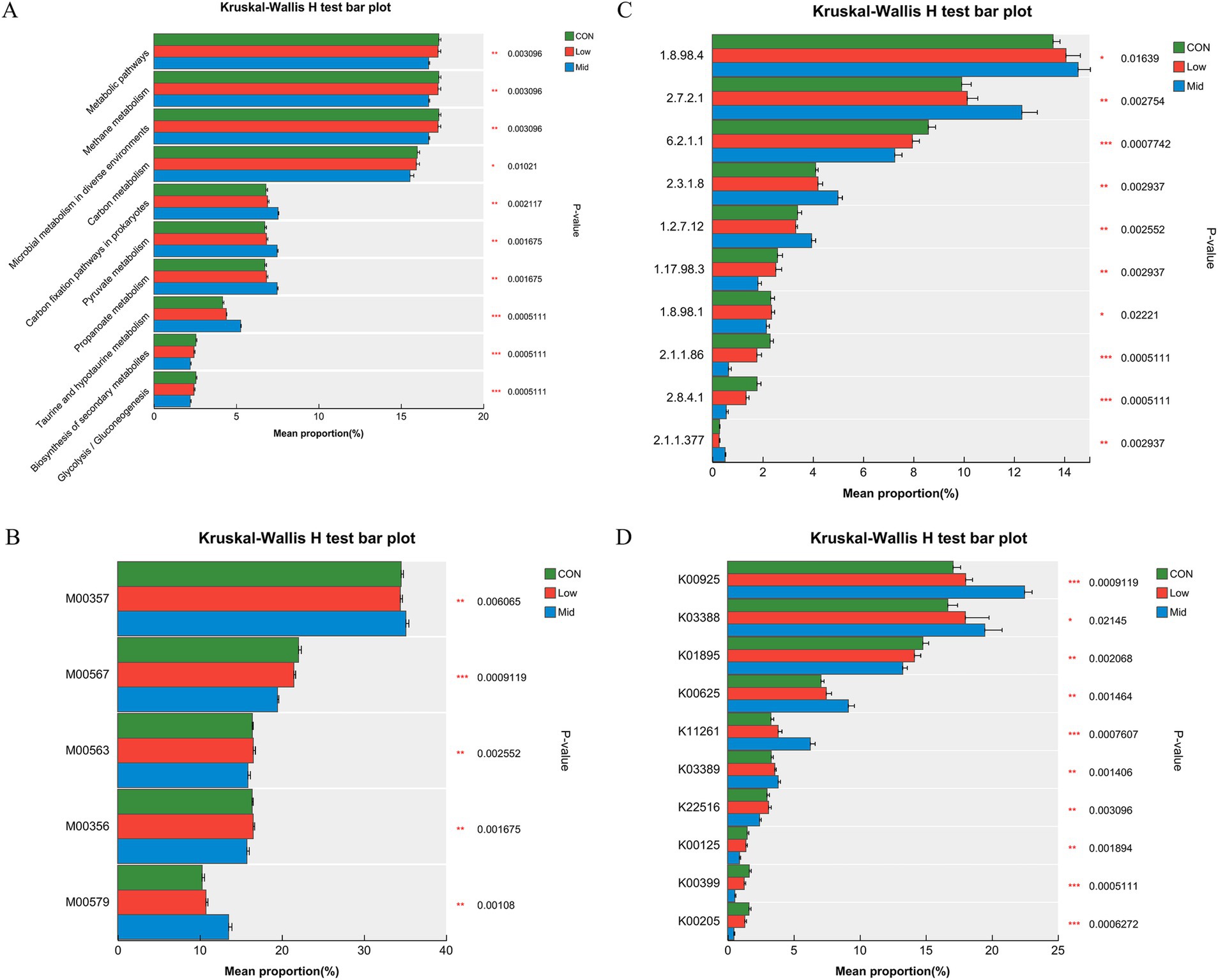
Figure 5. Effect of different levels of Asparagopsis taxiformis supplementation on methane metabolism function level. (A) Differences in methane metabolism at Pathway level 3. (B) Differences in methane metabolism at Module. (C) Differences in methane metabolism at Enzyme. (D) Differences in methane metabolism at KO (KEGG Orthology). CON, control group; Low, CON plus 2% A. taxiformis; Mid, CON plus 5% A. taxiformis; High, CON plus 10% A. taxiformis. *<0.05, **<0.01, ***<0.001.
3.6.2 Enrichment analysis of KEGG pathway related to CH4 metabolism
Furthermore, the reasons for reduced CH4 production were explored at different functional levels. The impact on CH4 metabolism resulted in changes at the module, enzyme, and KEGG Orthology (KO) levels. The top 10 relative abundances of each function were analyzed. M00357 and M00579 significantly increased by the Mid group of A. taxiformis (p < 0.01). However, M00567, M00563, and M00356 are significantly decreased (p < 0.01) (Figure 5B). Figure 5C shows the change in the relative abundance of enzymes in the pathway. The relative abundance of enzymes such as 1.8.98.4 (coenzyme F420), 2.7.2.1 (acetate kinase), and 2.3.1.8 (phosphate acetyltransferase) were significantly increased (p < 0.05), while those of 1.17.98.3 (formate dehydrogenase) and 1.8.98.1 (dihydromethanophenazine) were significantly decreased (p < 0.05). It is worth noting that 6.2.1.1 (acetyl coenzyme A synthetase), 2.1.1.86, and 2.8.4.1 (methyl-CoM reductase) were significantly reduced (p < 0.01) in the two groups of supplementing A. taxiformis, but it was more significant in the Mid group. The relative abundance of K00925 (acetate kinase), K03388 (heterodisulfide reductase subunit A2), K00625 (phosphate acetyltransferase), K11261 (formylmethanofuran dehydrogenase subunit E), and K03389 (heterodisulfide reductase subunit B2) gradually increases (p < 0.01), while the relative abundance of K01895 (acetyl-CoA synthetase), K22516 (coenzyme F420 alpha subunit), K00125 (coenzyme F420 beta subunit), K00399 (methyl-coenzyme M reductase alpha subunit), and K00205 (4Fe-4S ferredoxin) gradually decreases (p < 0.01) (Figure 5D).
3.7 Effect of Asparagopsis taxiformis in different supplementation levels respond to rumen metabolome
Further analysis was conducted to investigate the effect of supplementing different levels of A. taxiformis on the in vitro rumen fermentation metabolites. Using the anion and cation mix combined analysis method, unit variance conversion, and confidence level 0.95, PCA analysis was performed on the metabolite composition of the CON, Low, and Mid groups. The results indicate that the separation trend of the Mid group from the CON and Low groups is obvious, indicating that in the Mid group, metabolites changed significantly (Figure 6A). The PLS-DA results showed that the metabolites of the mixed mode of CON, Low, and Mid groups were completely separated, indicating that the supplementation of A. taxiformis significantly disrupted the in vitro rumen fermentation metabolite profile (Figure 6B). The PLS-DA simulation verification results showed that the R2 of the mixed mode was above Q2 (Figure 6B), the model fit was good, the predictability was strong, and it was suitable for subsequent data analysis. According to the PLS-DA results, the differential metabolites screened out by the projected variable importance (VIP) of the multivariate analysis PLS-DA model were combined with the fold change and p value (VIP > 1, p < 0.05) as the difference between the two groups. Figure 6C shows that the Low group had 311 metabolites significantly upregulated and 168 downregulated compared to the CON. In the Mid group, A. taxiformis supplementation led to the up-regulation of 516 metabolites and the down-regulation of 247 metabolites (Figure 6D). Detailed changes in metabolites are provided in Supplementary Tables S2, S3.
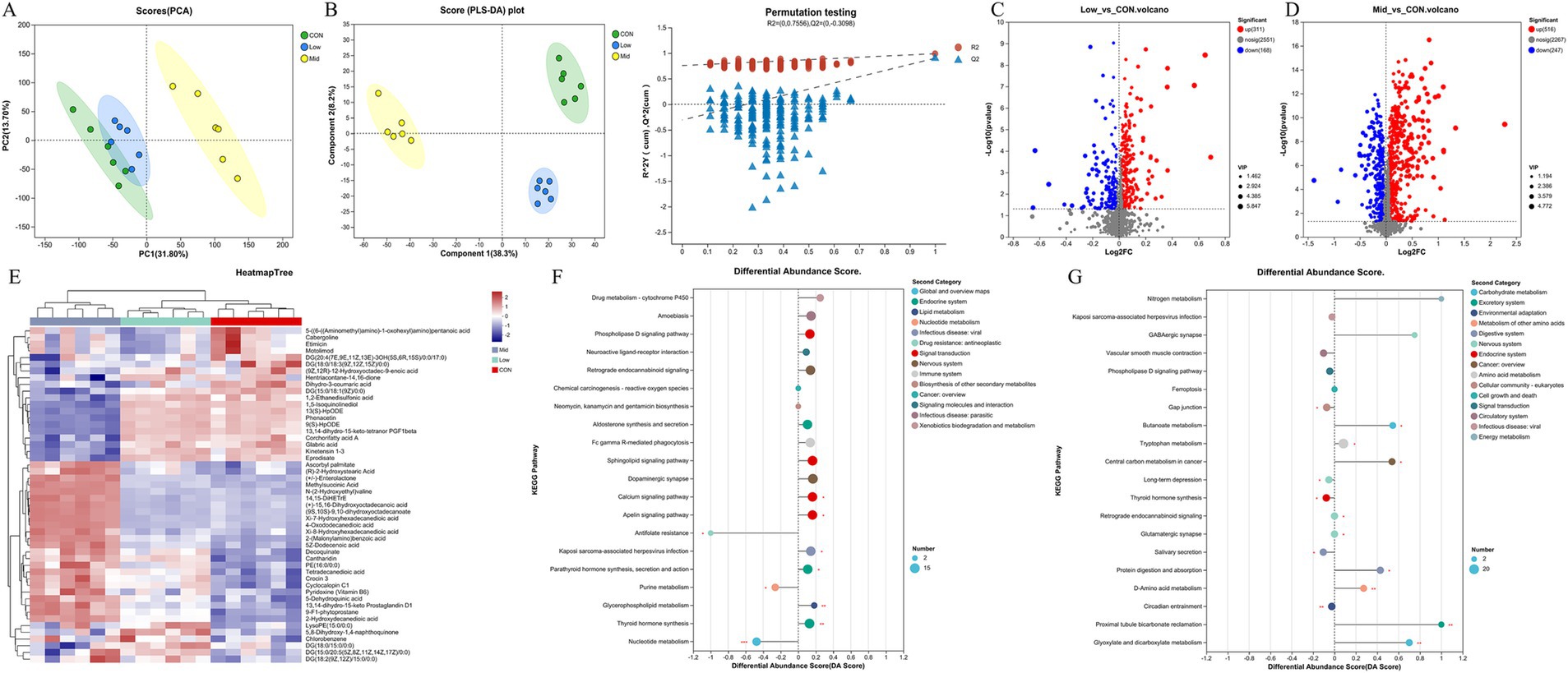
Figure 6. Effect of Asparagopsis taxiformis supplementation on the metabolomics of rumen fluid from in vitro rumen fermentation. (A) Principal component analysis (PCA) scores of metabolites in rumen fluid at different supplementation levels. (B) PLS-DA scores plots. R2 was 0.7556 and Q2 was 0.3098. (C) Volcano plot of differential metabolites between Low and CON groups. (D) Volcano plot of differential metabolites between Mid and CON groups. (E) Hierarchical clustering analysis of differential metabolites in rumen fluid at different levels of A. taxiformis supplementation. (F) Diagram of enrichment scores of differential metabolites in functional pathways in the Low group compared with the CON. (G) Diagram of enrichment scores of differential metabolites in functional pathways in the Mid group compared with the CON. CON, control group; Low, CON plus 2% A. taxiformis; Mid, CON plus 5% A. taxiformis; High, CON plus 10% A. taxiformis. *<0.05, **<0.01, ***<0.001.
To visualize relevant differences in the in vitro ruminal fermentation metabolites, hierarchical cluster analysis was performed using heatmaps (Figure 6E). Five distinct clusters were formed among these differential metabolites. The results showed that 24 metabolites, including Ascorbyl palmitate, (R)-2-Hydroxystearic Acid, and Methylsuccinic Acid, could be classified into cluster 3. The expression of metabolites in cluster 3 in the Mid group was significantly higher than in the CON and Low groups. At the same time, 16 metabolites such as Corchorifatty acid A can be classified into cluster 2. The expression of metabolites in cluster 2 in the Mid group was lower than in the other two groups. The two groups were compared with CON to analyze the effect of A. taxiformis supplementation on the enrichment of differential metabolites in KEGG pathways. The differences in differential metabolite expression in the KEGG pathways are illustrated for the Low group (Figure 6F) and the Mid group (Figure 6G), compared to the CON group.
4 Discussion
The main objectives of this study were to evaluate the effect of different supplementation levels of A. taxiformis in different treatments on reducing rumen fermentation CH4 production and to explore the mechanisms behind CH4 emissions. It is worth noting that the freeze-dried treatment demonstrated a stronger inhibitory effect on rumen fermentation CH4 production at supplementation levels ≥5% (Mid and High), reducing CH4 production by more than 98% (Figure 1). The result agrees with the report by Roque et al. (2019) who reported a 95% reduction in CH4 production with 5% supplementation of organic matter from A. taxiformis. Although the dried treatment of A. taxiformis ≥5% (Mid and High), showed a lower effect than freeze-dried treatment, it still reduced CH4 emissions by 34.52% to 82.89%.
The high efficiency of freeze-dried A. taxiformis in mitigating CH4 was expected. It is well known that freeze-dried can inhibit mutation and protect cell structure during the sublimation process, which can retain bioactive components to the maximum extent (Ratti, 2001; Vucko et al., 2017). This preservation would help maintain the content of haloalkanes, which were key ingredient in mitigating CH4 in A. taxiformis. Dibromoiodomethane as a haloalkanes, is a structural analog of MCR and competes to inhibit methyl transfer reactions during methanogenesis (Liu et al., 2011), and P-Bromophenol and 2,4,6-Tribromothiophenol and 2,4-Dibromophenol as polyphenol derivatives compete to inhibit the transfer of the H2 to the pathway of CH4 production, which are all reactions that further strengthen the inhibitory effect on CH4 (Ramdani et al., 2023).
In contrast, dried reduces the concentration of heat-sensitive phytochemicals, such as haloalkanes and polyphenol, which are critical for the antimethanogenic activity. This is the main reason why relevant bioactive components were not detected in the dried treatment. The loss of these compounds occurs due to thermal instability and oxidative reactions during dehydration (Wong and Cheung, 2001; Magnusson et al., 2014; Ling et al., 2015). In addition, dried alters the composition of nutrients such as proteins and the integrity of the cell wall, which can exacerbate the volatilization of bioactive components from A. taxiformis and reduce their potential as CH4 inhibitors (Ling et al., 2015).
In this experiment, the supplementation levels of dried treatment reduced CH4 by 34.52%, and 82.89%, respectively, while freeze-dried treatment reduced CH4 by 32.44%, 98.53%, and 99.33%, respectively. These results indicate that at the same level, freeze-dried treatment has greater potential to mitigate CH4, consistent with the above description that freeze-dried can better preserve bioactive components. However, a limitation of this study is that the CHBr3 concentrations in the different treatments and supplementation levels were not tested, preventing the provision of concrete evidence regarding changes in CHBr3 concentration.
The degradation of dietary nutrients is an important indicator to measure the degree of nutrient utilization by animals (Li et al., 2023). The results demonstrate that both supplementation level and treatment method influence nutrient degradation. It is noteworthy that significant differences were observed in ADFD (61.53 vs. 51.34) and CPD (51.26 vs. 77.46) between the freeze-dried and dried treatments under mid-level supplementation. The freeze-dried treatment may enhance the digestibility of ADF while preserving a higher amount of rumen-protected protein. When the supplementation level of A. taxiformis is more than 5%, both treatment methods significantly negative affect nutrient degradation, which is consistent with previous study results (Kinley et al., 2016a). The additional level of A. taxiformis more than 5% may affect the fermentation stability and inhibit the activity of carbohydrate digestive enzymes, thus affecting the degradation of nutrients (Abbott et al., 2020). CH4 is the final product of anaerobic fermentation of carbohydrates in the rumen of ruminants, and H2 plays an important role in this process (Antonius et al., 2023). In the rumen, propionate production consumes H2 and this pathway is considered an important H2 sink (Ungerfeld, 2020; Wasson et al., 2022). Therefore, changes in VFA fermentation patterns will also affect CH4 emissions (Sena et al., 2024). In this study, we observed no significant effect on total VFA concentration, although there was a downward trend with increasing supplementation levels in the freeze-dried treatment (Table 4). This study observed that acetate concentration was lower in the freeze-dried treatment compared to the dried treatment, with acetate concentration decreasing by 22.62%–32.51% as supplementation levels increased. Propionate concentration had no effect in all treatment groups compared to the CON. In the dried treatment, only the A:P ratio in the High group significantly decreased by 24.90%. In the freeze-dried treatment, the A:P ratio decreased by 15.84%, 27.17%, and 31.69% along with increasing supplement levels. The A:P ratio directly reflects the net balance of H2 during rumen fermentation, indicating the trend of methanogenic substrates and thus the potential of strategies to mitigate CH4 emissions (Machado et al., 2016a). This study indicated that the decrease in acetate concentration after supplementation with A. taxiformis might be the main reason for the decrease in the A:P ratio, and this effect was more effective in the freeze-dried treatment than in the dried treatment, and also more effective in the high supplementation level than in the low supplementation level.
In the current study, NH3-N concentration was significantly reduced only in the freeze-dried Mid and High groups. Several factors such as CPD, protozoa number, and MCP synthesis in the rumen of ruminants jointly affect the concentration of NH3-N (Kelln et al., 2023). In the freeze-dried treatment, the Low and Mid groups exhibited significantly higher MCP concentrations compared to the CON group. This result may be due to the freeze-dried A. taxiformis affecting the rumen environment, accelerating the rate at which microorganisms use NH3-N to synthesize MCP, resulting in a decrease in NH3-N concentration and an increase in MCP (Li et al., 2023). However, when the supplementation level is too high, it will inhibit the microbial synthesis of MCP.
Freeze-dried treatment A. taxiformis was more effective than other treatment methods in mitigating CH4 emissions and had a reduced negative impact on rumen nutrient degradation. Although the High group achieved the best CH4 mitigation in the freeze-dried treatment, it had a significant adverse effect on rumen fermentation parameters. We therefore conclude that the Mid group represents the optimal balance between CH4 emission reduction and rumen fermentation efficiency. To further explore the mechanism of freeze-dried A. taxiformis in mitigating CH4, we selected three groups of samples (CON, Low, and Mid group) for metagenomic and metabolomics analysis.
Rumen microbiome plays a crucial role in the rumen, and regulating the structure of the rumen microbiome is a common strategy to mitigate CH4 emissions (Fu et al., 2023). In this experiment, the supplementation of A. taxiformis significantly affected bacterial diversity, with the relative abundance of Prevotella, Ruminobacter, and Succinivibrio increased significantly in the Mid group (Figure 2H). The increase in the relative abundance of these bacteria can alleviate rumen CH4 emissions by competing for H2 utilization (Liu et al., 2022; Fu et al., 2023; Khiaosa-Ard et al., 2023). All three genera mentioned above can ferment carbohydrates in the rumen to produce succinate (Russell and Rychlik, 2001), which is subsequently converted into propionate through fermentation by Selenomonas (Van Gylswyk, 1995). The relative abundance of Selenomonas also significantly increased in the Mid group, although this genus accounted for a relatively low abundance in this experiment. This succinate pathway is the main pathway of propionate production in the rumen (Van Gylswyk, 1995; Jeyanathan et al., 2014). This result suggests that the Mid group facilitated the propionate production pathway by affecting the relative abundance of certain bacteria compared to the CON, explaining the trend of increased propionate production in the Mid group and contributing to its strong inhibition of CH4 production. Additionally, a significant decrease in the relative abundance of Ruminococcus was observed, which functions in the rumen as degraded cellulose (Baba et al., 2019). The effect was stronger in the Mid group, explaining the observed decrease in fiber degradation. The fermentation of fiber by cellulose-degrading bacteria such as Ruminococcus produces by-products including acetate and H2 (Palakawong Na Ayudthaya et al., 2018). Reducing acetate production similarly contributes to CH4 reduction (Jayanegara et al., 2014). At the bacterial species level, the relative abundance of Clostridia_bacterium and Oscillospiraceae_bacterium, both belonging to the Firmicutes, was significantly reduced in the Mid group (Supplementary Figure S2). Firmicutes play an important role in the degradation of carbohydrates such as starch, cellulose, and hemicellulose (Ahmad et al., 2020). The reduction in their relative abundance could also explain the decreased fiber degradation and acetate concentration in the Mid group. After supplementation of freeze-dried A. taxiformis, the effect on bacterial community structure with increasing levels altered the pattern of rumen VFA fermentation, promoting propionate production and inhibiting acetate production. This leads to a lower A:P ratio, which promotes the transfer of H2 to the propionate pathway and reduces the amount of H2 available for the methanogenic pathway, thereby mitigating CH4 emissions.
It is well known that Methanobrevibacter is the dominant archaeal species in the rumen, accounting for approximately 61%–74% of the total archaeal community, and is responsible for the production of CH4 (Choi et al., 2022; Pitta et al., 2022). This study observed a significant reduction in richness and increased diversity of archaeal community species in the Mid group compared to the CON and Low groups (Figure 3H). The relative abundance of Methanobrevibacter was significantly lower in the Low and Mid groups, with a more substantial reduction observed in the Mid group. Lowing the relative abundance of Methanobrevibacter is typical of CH4 inhibition. Previous studies have shown that a decreased in the relative abundance of Methanobrevibacter is directly responsible for the decreased in rumen CH4 production (Roque et al., 2019; Künzel et al., 2022; Jia et al., 2023). Additionally, the relative abundances of Methanosphaera, Methanomicrobium, and Methanocorpusculum were significantly higher in the Mid group. Both Methanobrevibacter and Methanosphaera are major methanogenic archaea in the rumen of ruminants. However, the relative abundance of Methanobrevibacter is positively correlated with CH4 production, whereas Methanosphaera is negatively correlated with methane production (Fricke et al., 2006; Cunha et al., 2019; Jia et al., 2023). This is because Methanobrevibacter utilizes the hydrogenotrophic pathway (M00567) consume 1 mol of CO2 to produce 1 mol of CH4, whereas Methanosphaera operates through methylotrophic pathway (M00356) consume 4 mol of methanol to produce 3 mol of CH4 (Cunha et al., 2019; Jia et al., 2023). Therefore, when the carbon source from rumen fermentation remains constant, the higher relative abundance of Methanosphaera results in less CH4 production. The results of this study indicated that the trends in the relative abundances of these two archaea might be the main reasons for the 98.53% CH4 reduction observed in the Mid group.
Overall, supplementation with freeze-dried A. taxiformis at 5% could indirectly mitigate CH4 emissions by affecting the bacterial community to alter the fermentation pattern of VFAs and reduce H2 transfer to the methanogenic pathway. Furthermore, it directly inhibited CH4 production in the rumen by altering the relative abundance of archaeal communities, particularly methanogenic archaea. Changes in the microbial community induced alterations in rumen fermentation patterns and functions, which strongly reduced rumen CH4 emissions.
Metagenomics analysis also revealed significant alterations in the KEGG pathways of the Mid group compared to the CON and Low groups. Specifically, the pathways for methane metabolism (ko00680) and carbon metabolism (ko01200) pathways were significantly suppressed, whereas the propionate metabolism pathway (ko 00640) was markedly enriched. These findings align with our experimental result, where a significant reduction in CH4 was observed. In the ko00680, we identified differences in the abundance of four modules: M00357 (acetate ≥ CH4), M00567 (CO2 ≥ CH4), M00563 (methylamine/dimethylamine/trimethylamine ≥ CH4), and M00356 (methanol ≥ CH4). Methanogenesis can be categorized into three pathways based on the substrate: hydrogenotrophic (M00567), aceticlastic (M00357), and methylotrophic (M00563 and M00356) (Lyu et al., 2018). In module M00357, the increased abundance of K00925 (acetate kinase [EC:2.7.2.1]) and K00625 (phosphate acetyltransferase [EC:2.3.1.8]) promoted the conversion of acetate to Acetyl-CoA (Latimer and Ferry, 1993). Changes in microbial abundance led to reduced acetate production and enhanced acetate depletion in the methanogenic pathway, contributing to a 22.66% decrease in acetate concentration in the freeze-dried Mid group compared to the CON. Additionally, the reduced abundance of M00567 in this study led to a reduction in K11261 (formylmethanofuran dehydrogenase subunit E [EC:1.2.7.12]) and K00205 (4Fe-4S ferredoxin) abundance, thus inhibiting the step in the conversion of substrate CO2 to Foemyl-MFR in the hydrogenotrophic pathway (Vorholt et al., 1996; Wagner et al., 2016). In the Mid group, M00563 and M00356, representing methylotrophic CH4-producing modules, showed significantly lower abundance, thus reducing CH4 production via this pathway (Figures 5C,D).
In the freeze-dried treatment of Mid group, supplementation with A. taxiformis reduced the abundance of K00399 (methyl-coenzyme M reductase alpha subunit [EC:2.8.4.1]). It inhibited the final reaction step of CH4 generation in the rumen. All three methanogenic pathways are catalyzed by MCR, a key enzyme in the final step of methane formation common to all methanogenic pathways (Lyu et al., 2018; Thauer, 2019). During this reaction, K00399 mediates the reduction of MCR by the thiol, coenzyme B (HS-CoB) to form a reductively form the heterodisulfide (CoM-SS-CoB) and CH4 (Lyu et al., 2018). Furthermore, CoM-SS-CoB reductive regeneration to HS-CoB and coenzyme M (HS-CoM) (Hedderich and Thauer, 1988). Previous studies have shown that the redox potential Eo′ of the CoM-S-S-CoB/HS-CoM + HS-CoB pairing is −140 mV under standard conditions (Tietze et al., 2003). Moreover, the MCR is active only when the redox potential of the CoM-S-S-CoB/HS-CoM + HS-CoB pair is well below the standard redox potential of −140 mV (Thauer, 2019). The significant increase in abundance at K03388 (heterodisulfide reductase subunit A2 [EC:1.8.98.4]) and K03389 (heterodisulfide reductase subunit B2 [EC:1.8.98.4]) facilitated the process of CoM-S-S-CoB reduction to generate HS-CoM and HS-CoB, resulting in an elevated redox potential Eo′ for the CoM-S-S-CoB/HS-CoM + HS-CoB pair, which decreased MCR activity, thereby inhibiting CH4 emission.
Metabolomics allows for analyzing rumen fermentation metabolites in vitro and identifying biomarkers that reflect the physiological status of ruminants (Fontanesi, 2016). In the current study, the Proximal Tubule Bicarbonate Reclamation metabolic pathway was significantly enriched in the Mid group compared to the CON. As shown in Supplementary Table S2, the major differential metabolites within this pathway were L-glutamate, ketoglutarate, and malate. L-glutamate produces ketoglutarate, which enters the tricarboxylic acid cycle (TCA) (Hinca et al., 2021). The Mid group also significantly enriched the TCA metabolic pathway, with ketoglutarate, isocitrate, and malate as the main differential metabolites. The accumulation of these three TCA cycle upstream metabolites suggests a potential inhibited of dehydrogenation reaction in the TCA process (Choi et al., 2021), this inhibition would limit H2 and CO2 during sugar metabolism, thereby reducing substrate for CH4 production. We hypothesize that the bioactive compounds of A. taxiformis may target key TCA enzymes, though direct mechanistic evidence requires future in vitro enzymology studies.
Furthermore, we observed that the highest enrichment in the Mid group was in the Tryptophan metabolic. Within this pathway, melatonin was identified as a differential metabolite. Besides its well-known roles in anti-inflammatory, sleep-promoting, mood-improving, reproductive, and immune-enhancing activities, melatonin’s antioxidant function is widely recognized (Tan et al., 2015). Several studies have shown that melatonin can regulate the activity of intestinal microbes or their metabolites and improve intestinal physiology (Rahman et al., 2005; Ren et al., 2018; Fu et al., 2023). Recent studies have shown that melatonin mitigates about 50% of CH4 emissions in the rumen of dairy cows by decreasing the relative abundance and production of CH4 substrates by methanogenic archaea (Fu et al., 2023). In the present study, melatonin was significantly enriched in both the Tryptophan Metabolic and Circadian Entrainment pathways. The elevated expression of melatonin may play a role in reducing methane emissions.
5 Conclusion
Supplementation with 5% freeze-dried A. taxiformis significantly mitigated in vitro rumen fermentation CH₄ emissions, reducing CH₄ by 98.53%. The potential mechanism of A. taxiformis on the rumen contributed was drawn in Figure 7 to this substantial reduction in CH₄ emissions. Thereof, the supplementation of A. taxiformis increased the abundance of Prevotella, Ruminobacter, and Succinivibrio, and reduced the abundance of Ruminococcus, resulting in a decrease in the net H₂ balance and indirectly mitigated CH₄ emissions. Supplementation also directly influenced methanogenesis pathway by altering the abundance of key archaea. Specifically, the upregulation of K03388 (heterodisulfide reductase subunit A2) and K03389 (heterodisulfide reductase subunit B2) facilitated the reduction of CoM-SS-CoB to HS-CoB and HS-CoM, inhibiting MCR enzyme activity. Concurrently, the downregulation of K00399 (methyl-coenzyme M reductase alpha subunit) further inhibited MCR reduction to generate CH₄, collectively suppressing the final step of CH₄ production in the rumen and reducing overall CH₄ emissions. Moreover, melatonin expression was significantly upregulated, reducing CH₄ emissions through its dual role as an antioxidant and microbial modulator. These findings suggest that A. taxiformis supplementation may offer a promising and biologically plausible approach to sustainable ruminant production. Further animal studies are warranted to comprehensively evaluate the long-term CH₄ reduction potential of A. taxiformis, its effects on animal health and performance, as well as its impacts on milk and meat quality, and economic feasibility.
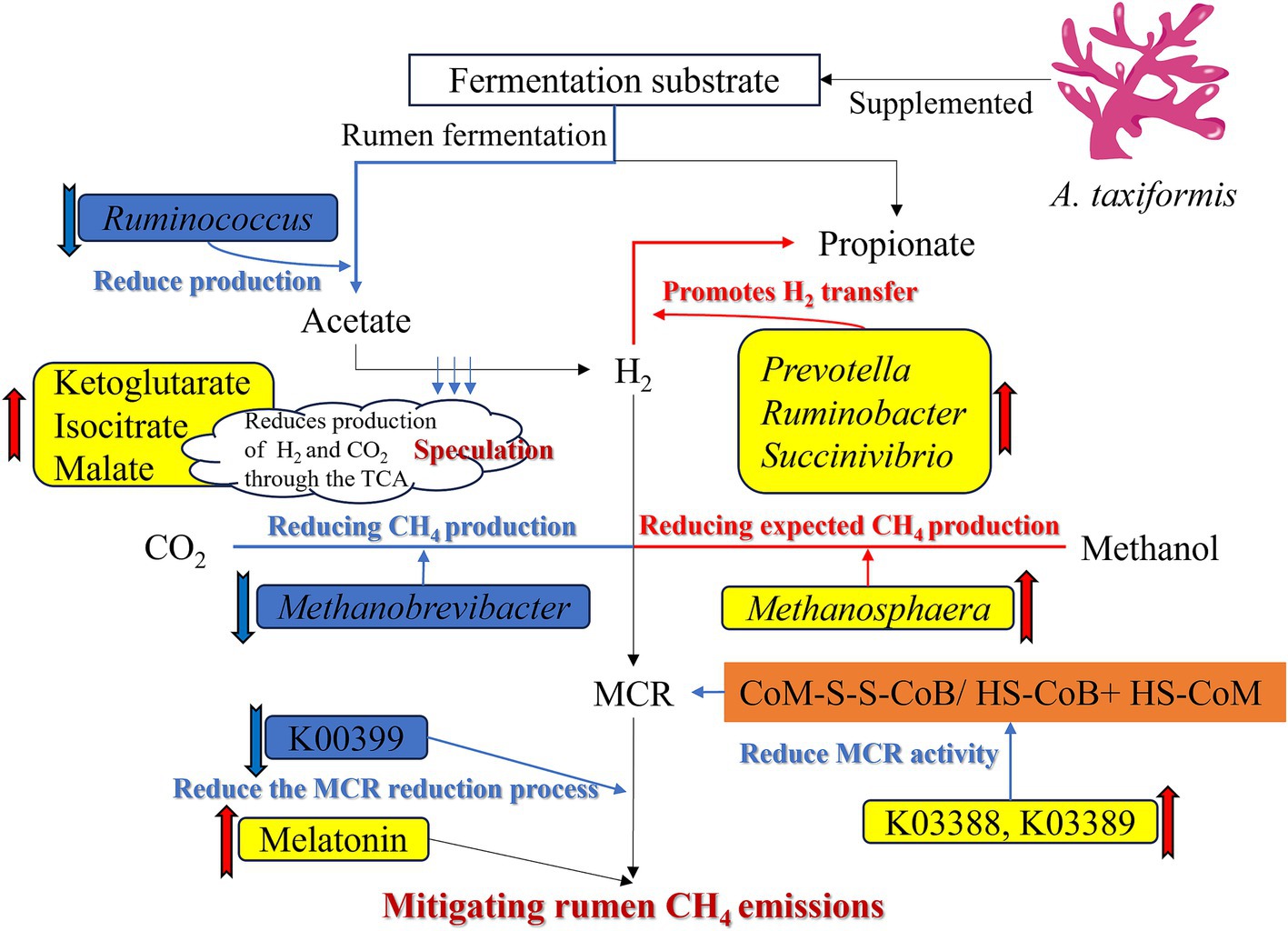
Figure 7. Mechanism of supplementation of Asparagopsis taxiformis to alleviate methane emission in the in vitro rumen fermentation.
Data availability statement
The datasets presented in this study can be found in online repositories. The names of the repository/repositories and accession number(s) can be found at: https://www.ncbi.nlm.nih.gov/genbank/, PRJNA1148834.
Ethics statement
The animal experiment protocol complied with the requirements of experimental animal welfare and ethics, as well as the regulations of the Ministry of Science and Technology of China. It was performed following the European Directive 2010/63/EU and S.I. No. 543 of 2012. The study was conducted in accordance with the local legislation and institutional requirements.
Author contributions
SL: Data curation, Formal analysis, Investigation, Writing – original draft. YS: Data curation, Investigation, Methodology, Writing – review & editing. SC: Data curation, Writing – review & editing. TG: Funding acquisition, Writing – review & editing. XT: Writing – review & editing. ZZ: Writing – review & editing. JS: Supervision, Writing – review & editing. YY: Supervision, Writing – review & editing. QW: Project administration, Writing – review & editing. DL: Conceptualization, Writing – review & editing. LM: Conceptualization, Funding acquisition, Supervision, Writing – original draft, Writing – review & editing.
Funding
The author(s) declare that financial support was received for the research and/or publication of this article. This study was supported by the Southern Marine Science and Engineering Guangdong Laboratory (Zhuhai) (SML2024SP028), Guangdong Modern Agro-industry Technology Research System (2024CXTD13), China Scholarship Council (202408440440), National Natural Science Foundation of China (32271684), Guangzhou Science and technology planning project (2023A04J0793), and Open project of Xinjiang Key Laboratory of Feed Biotechnology for Herbivorous Livestock (XJSLSW-2023002).
Conflict of interest
The authors declare that the research was conducted in the absence of any commercial or financial relationships that could be construed as a potential conflict of interest.
Generative AI statement
The authors declare that no Gen AI was used in the creation of this manuscript.
Publisher’s note
All claims expressed in this article are solely those of the authors and do not necessarily represent those of their affiliated organizations, or those of the publisher, the editors and the reviewers. Any product that may be evaluated in this article, or claim that may be made by its manufacturer, is not guaranteed or endorsed by the publisher.
Supplementary material
The Supplementary material for this article can be found online at: https://www.frontiersin.org/articles/10.3389/fmicb.2025.1586456/full#supplementary-material
Footnotes
References
Abbott, D. W., Aasen, I. M., Beauchemin, K. A., Grondahl, F., Gruninger, R., Hayes, M., et al. (2020). Seaweed and seaweed bioactives for mitigation of enteric methane: challenges and opportunities. Animals 10:2432. doi: 10.3390/ani10122432
Ahmad, A. A., Yang, C., Zhang, J., Kalwar, Q., Liang, Z., Li, C., et al. (2020). Effects of dietary energy levels on rumen fermentation, microbial diversity, and feed efficiency of yaks (Bos grunniens). Front. Microbiol. 11:625. doi: 10.3389/fmicb.2020.00625
Antonius, A., Pazla, R., Putri, E. M., Negara, W., Laia, N., Ridla, M., et al. (2023). Effectiveness of herbal plants on rumen fermentation, methane gas emissions, in vitro nutrient digestibility, and population of protozoa. Vet World 16, 1477–1488. doi: 10.14202/vetworld.2023.1477-1488
AOAC (2000). Official methods of analysis of the Association of Official Analytical Chemists. Rockville, United States: AOAC.
Baba, Y., Matsuki, Y. U., Takizawa, S., Suyama, Y., Tada, C., Fukuda, Y., et al. (2019). Pretreatment of lignocellulosic biomass with cattle rumen fluid for methane production: fate of added rumen microbes and indigenous microbes of methane seed sludge. Microbes Environ. 34, 421–428. doi: 10.1264/jsme2.ME19113
Broderick, G. A., and Kang, J. H. (1980). Automated simultaneous determination of ammonia and total amino acids in ruminal fluid and in vitro media. J. Dairy Sci. 63, 64–75. doi: 10.3168/jds.S0022-0302(80)82888-8
Choi, Y., Lee, S. J., Kim, H. S., Eom, J. S., Jo, S. U., Guan, L. L., et al. (2022). Red seaweed extracts reduce methane production by altering rumen fermentation and microbial composition in vitro. Front Vet Sci 9:985824. doi: 10.3389/fvets.2022.985824
Choi, I., Son, H., and Baek, J.-H. (2021). Tricarboxylic acid (TCA) cycle intermediates: regulators of immune responses. Life 11:69. doi: 10.3390/life11010069
Crippa, M., Solazzo, E., Guizzardi, D., Monforti-Ferrario, F., Tubiello, F. N., and Leip, A. (2021). Food systems are responsible for a third of global anthropogenic GHG emissions. Nat Food 2, 198–209. doi: 10.1038/s43016-021-00225-9
Cunha, C. S., Marcondes, M. I., Veloso, C. M., Mantovani, H. C., Pereira, L. G. R., Tomich, T. R., et al. (2019). Compositional and structural dynamics of the ruminal microbiota in dairy heifers and its relationship to methane production. J. Sci. Food Agric. 99, 210–218. doi: 10.1002/jsfa.9162
Erwin, E. S., Marco, G. J., and Emery, E. M. (1961). Volatile fatty acid analyses of blood and rumen fluid by gas chromatography. J Diary Sci. 44, 1768–1771. doi: 10.3168/jds.S0022-0302(61)89956-6
Fontanesi, L. (2016). Metabolomics and livestock genomics: insights into a phenotyping frontier and its applications in animal breeding. Anim. Front. 6, 73–79. doi: 10.2527/af.2016-0011
Fricke, W. F., Seedorf, H., Henne, A., Krüer, M., Liesegang, H., Hedderich, R., et al. (2006). The genome sequence of Methanosphaera stadtmanae reveals why this human intestinal archaeon is restricted to methanol and H2 for methane formation and ATP synthesis. J. Bacteriol. 188, 642–658. doi: 10.1128/JB.188.2.642-658.2006
Fu, Y., Yao, S., Wang, T., Lu, Y., Han, H., Liu, X., et al. (2023). Effects of melatonin on rumen microorganisms and methane production in dairy cow: results from in vitro and in vivo studies. Microbiome 11:196. doi: 10.1186/s40168-023-01620-z
Glasson, C. R. K., Kinley, R. D., de Nys, R., King, N., Adams, S. L., Packer, M. A., et al. (2022). Benefits and risks of including the bromoform containing seaweed Asparagopsis in feed for the reduction of methane production from ruminants. Algal Res. 64:102673. doi: 10.1016/j.algal.2022.102673
Hedderich, R., and Thauer, R. K. (1988). Methanobacterium thermoautotrophicum contains a soluble enzyme system that specifically catalyzes the reduction of the heterodisulfide of coenzyme M and 7-mercaptoheptanoylthreonine phosphate with H2. FEBS Lett. 234, 223–227. doi: 10.1016/0014-5793(88)81339-5
Hinca, S. B., Salcedo, C., Wagner, A., Goldeman, C., Sadat, E., Aibar, M. M. D., et al. (2021). Brain endothelial cells metabolize glutamate via glutamate dehydrogenase to replenish TCA-intermediates and produce ATP under hypoglycemic conditions. J. Neurochem. 157, 1861–1875. doi: 10.1111/jnc.15207
Jayanegara, A., Sarwono, K. A., Kondo, M., Matsui, H., Ridla, M., Laconi, E. B., et al. (2018). Use of 3-nitrooxypropanol as feed additive for mitigating enteric methane emissions from ruminants: a meta-analysis. Ital. J. Anim. Sci. 17, 650–656. doi: 10.1080/1828051X.2017.1404945
Jayanegara, A., Wina, E., and Takahashi, J. (2014). Meta-analysis on methane mitigating properties of saponin-rich sources in the rumen: influence of addition levels and plant sources. Asian Australas. J. Anim. Sci. 27, 1426–1435. doi: 10.5713/ajas.2014.14086
Jeyanathan, J., Martin, C., and Morgavi, D. P. (2014). The use of direct-fed microbials for mitigation of ruminant methane emissions: a review. Animal 8, 250–261. doi: 10.1017/S1751731113002085
Jia, P., Dong, L.-f., Tu, Y., and Diao, Q.-y. (2023). Bacillus subtilis and Macleaya cordata extract regulate the rumen microbiota associated with enteric methane emission in dairy cows. Microbiome 11:229. doi: 10.1186/s40168-023-01654-3
Johnson, K. A., and Johnson, D. E. (1995). Methane emissions from cattle. J. Anim. Sci. 73, 2483–2492. doi: 10.2527/1995.7382483x
Kelln, B. M., Penner, G. B., Acharya, S. N., McAllister, T. A., McKinnon, J. J., Saleem, A. M., et al. (2023). Effect of mixtures of legume species on ruminal fermentation, methane, and microbial nitrogen production in batch and continuous culture (RUSITEC) systems. Can. J. Anim. Sci. 103, 326–337. doi: 10.1139/cjas-2022-0095
Khiaosa-Ard, R., Mahmood, M., Mickdam, E., Pacífico, C., Meixner, J., and Traintinger, L.-S. (2023). Winery by-products as a feed source with functional properties: dose–response effect of grape pomace, grape seed meal, and grape seed extract on rumen microbial community and their fermentation activity in RUSITEC. J Anim Sci Biotechnol 14:92. doi: 10.1186/s40104-023-00892-7
Kinley, R. D., de Nys, R., Vucko, M. J., Machado, L., and Tomkins, N. W. (2016a). The red macroalgae Asparagopsis taxiformis is a potent natural antimethanogenic that reduces methane production during in vitro fermentation with rumen fluid. Anim. Prod. Sci. 56, 282–289. doi: 10.1071/AN15576
Kinley, R. D., Martinez-Fernandez, G., Matthews, M. K., de Nys, R., Magnusson, M., and Tomkins, N. W. (2020). Mitigating the carbon footprint and improving productivity of ruminant livestock agriculture using a red seaweed. J. Clean. Prod. 259:120836. doi: 10.1016/j.jclepro.2020.120836
Kinley, R. D., Vucko, M. J., Machado, L., and Tomkins, N. W. (2016b). In vitro evaluation of the antimethanogenic potency and effects on fermentation of individual and combinations of marine macroalgae. Am. J. Plant Sci. 7, 2038–2054. doi: 10.4236/ajps.2016.714184
Künzel, S., Yergaliyev, T., Wild, K. J., Philippi, H., Petursdottir, A. H., Gunnlaugsdottir, H., et al. (2022). Methane reduction potential of Brown seaweeds and their influence on nutrient degradation and microbiota composition in a rumen simulation technique. Front. Microbiol. 13:889618. doi: 10.3389/fmicb.2022.889618
Latimer, M. T., and Ferry, J. G. (1993). Cloning, sequence analysis, and hyperexpression of the genes encoding phosphotransacetylase and acetate kinase from Methanosarcina thermophila. J. Bacteriol. 175, 6822–6829. doi: 10.1128/jb.175.21.6822-6829.1993
Li, S., Sun, Y., Guo, T., Liu, W., Tong, X., Zhang, Z., et al. (2024). Sargassum mcclurei mitigating methane emissions and affecting rumen microbial community in in vitro rumen fermentation. Animals 14:2057. doi: 10.3390/ani14142057
Li, S., Zeng, H., Wang, C., and Han, Z. (2023). Effect of methionine Hydroxy analog on Hu sheep digestibility, rumen fermentation, and rumen microbial community in vitro. Meta 13:169. doi: 10.3390/metabo13020169
Ling, A. L. M., Yasir, S., Matanjun, P., and Abu Bakar, M. F. (2015). Effect of different drying techniques on the phytochemical content and antioxidant activity of Kappaphycus alvarezii. J. Appl. Phycol. 27, 1717–1723. doi: 10.1007/s10811-014-0467-3
Liu, Z., Wang, K., Nan, X., Cai, M., Yang, L., Xiong, B., et al. (2022). Synergistic effects of 3-nitrooxypropanol with fumarate in the regulation of propionate formation and methanogenesis in dairy cows in vitro. Appl. Environ. Microbiol. 88, e01908–e01921. doi: 10.1128/aem.01908-21
Liu, H., Wang, J., Wang, A., and Chen, J. (2011). Chemical inhibitors of methanogenesis and putative applications. Appl. Microbiol. Biotechnol. 89, 1333–1340. doi: 10.1007/s00253-010-3066-5
Lyu, Z., Shao, N., Akinyemi, T., and Whitman, W. B. (2018). Methanogenesis. Curr. Biol. 28, R727–R732. doi: 10.1016/j.cub.2018.05.021
Machado, L., Magnusson, M., Paul, N. A., Kinley, R., de Nys, R., and Tomkins, N. (2016a). Dose-response effects of Asparagopsis taxiformis and Oedogonium sp. on in vitro fermentation and methane production. J. Appl. Phycol. 28, 1443–1452. doi: 10.1007/s10811-015-0639-9
Machado, L., Magnusson, M., Paul, N. A., Kinley, R., de Nys, R., and Tomkins, N. (2016b). Identification of bioactives from the red seaweed Asparagopsis taxiformis that promote antimethanogenic activity in vitro. J. Appl. Phycol. 28, 3117–3126. doi: 10.1007/s10811-016-0830-7
Machado, L., Tomkins, N., Magnusson, M., Midgley, D. J., de Nys, R., and Rosewarne, C. P. (2018). In vitro response of rumen microbiota to the antimethanogenic red macroalga Asparagopsis taxiformis. Microb. Ecol. 75, 811–818. doi: 10.1007/s00248-017-1086-8
Magnusson, M., Mata, L., De Nys, R., and Paul, N. A. (2014). Biomass, lipid and fatty acid production in large-scale cultures of the marine macroalga Derbesia tenuissima (Chlorophyta). Mar. Biotechnol. 16, 456–464. doi: 10.1007/s10126-014-9564-1
Makkar, H. P. S., Tran, G., Heuzé, V., Giger-Reverdin, S., Lessire, M., Lebas, F., et al. (2016). Seaweeds for livestock diets: a review. Anim. Feed Sci. Technol. 212, 1–17. doi: 10.1016/j.anifeedsci.2015.09.018
Mariotti, F., Tomé, D., and Mirand, P. P. (2008). Converting nitrogen into protein—beyond 6.25 and Jones' factors. Crit. Rev. Food Sci. Nutr. 48, 177–184. doi: 10.1080/10408390701279749
Menke, K. H., and Steingass, H. J. A. R. D. (1988). Estimation of the energetic feed value obtained from chemical analysis and in vitro gas production using rumen fluid. Anim Res Dev. 28, 7–55.
Min, B. R., Parker, D., Brauer, D., Waldrip, H., Lockard, C., Hales, K., et al. (2021). The role of seaweed as a potential dietary supplementation for enteric methane mitigation in ruminants: challenges and opportunities. Anim Nutr 7, 1371–1387. doi: 10.1016/j.aninu.2021.10.003
Palakawong Na Ayudthaya, S., Van De Weijer, A. H. P., Van Gelder, A. H., Stams, A. J. M., De Vos, W. M., and Plugge, C. M. (2018). Organic acid production from potato starch waste fermentation by rumen microbial communities from Dutch and Thai dairy cows. Biotechnol. Biofuels 11, 1–15. doi: 10.1186/s13068-018-1012-4
Patra, A., Park, T., Kim, M., and Yu, Z. (2017). Rumen methanogens and mitigation of methane emission by anti-methanogenic compounds and substances. J Anim Sci Biotechnol 8:13. doi: 10.1186/s40104-017-0145-9
Pitta, D., Indugu, N., Narayan, K., and Hennessy, M. (2022). Symposium review: understanding the role of the rumen microbiome in enteric methane mitigation and productivity in dairy cows. J. Dairy Sci. 105, 8569–8585. doi: 10.3168/jds.2021-21466
Rahman, M. A., Azuma, Y., Fukunaga, H., Murakami, T., Sugi, K., Fukushi, H., et al. (2005). Serotonin and melatonin, neurohormones for homeostasis, as novel inhibitors of infections by the intracellular parasite chlamydia. J. Antimicrob. Chemother. 56, 861–868. doi: 10.1093/jac/dki331
Ramdani, D., Yuniarti, E., Jayanegara, A., and Chaudhry, A. S. (2023). Roles of essential oils, polyphenols, and saponins of medicinal plants as natural additives and anthelmintics in ruminant diets: a systematic review. Animals 13:767. doi: 10.3390/ani13040767
Ratti, C. (2001). Hot air and freeze-drying of high-value foods: a review. J. Food Eng. 49, 311–319. doi: 10.1016/S0260-8774(00)00228-4
Ren, W., Wang, P., Yan, J., Liu, G., Zeng, B., Hussain, T., et al. (2018). Melatonin alleviates weanling stress in mice: involvement of intestinal microbiota. J. Pineal Res. 64:e12448. doi: 10.1111/jpi.12448
Roque, B. M., Brooke, C. G., Ladau, J., Polley, T., Marsh, L. J., Najafi, N., et al. (2019). Effect of the macroalgae Asparagopsis taxiformis on methane production and rumen microbiome assemblage. Anim Microbiome. 1:3. doi: 10.1186/s42523-019-0004-4
Russell, J. B., and Rychlik, J. L. (2001). Factors that alter rumen microbial ecology. Science 292, 1119–1122. doi: 10.1126/science.1058830
Sedmak, J. J., and Grossberg, S. E. (1977). A rapid, sensitive, and versatile assay for protein using Coomassie brilliant blue G250. Anal. Biochem. 79, 544–552. doi: 10.1016/0003-2697(77)90428-6
Sena, F., Portugal, P. V., Dentinho, M. T., Paulos, K., Costa, C., Soares, D. M., et al. (2024). Effects of sunflower oil infusions of Asparagopsis taxiformis on in vitro ruminal methane production and biohydrogenation of polyunsaturated fatty acids. J. Dairy Sci. 107, 1472–1484. doi: 10.3168/jds.2023-23506
Sun, J., Zhao, G., and Li, M. M. (2023). Using nutritional strategies to mitigate ruminal methane emissions from ruminants. Front Agricult Sci Engineer 10, 390–402. doi: 10.15302/J-FASE-2023504
Tan, D.-X., Manchester, L. C., Esteban-Zubero, E., Zhou, Z., and Reiter, R. J. (2015). Melatonin as a potent and inducible endogenous antioxidant: synthesis and metabolism. Molecules 20, 18886–18906. doi: 10.3390/molecules201018886
Thauer, R. K. (2019). Methyl (alkyl)-coenzyme M reductases: nickel F-430-containing enzymes involved in anaerobic methane formation and in anaerobic oxidation of methane or of short chain alkanes. Biochemistry 58, 5198–5220. doi: 10.1021/acs.biochem.9b00164
Tietze, M., Beuchle, A., Lamla, I., Orth, N., Dehler, M., Greiner, G., et al. (2003). Redox potentials of methanophenazine and CoB-S-S-CoM, factors involved in electron transport in methanogenic archaea. Chembiochem 4, 333–335. doi: 10.1002/cbic.200390053
Ungerfeld, E. M. (2020). Metabolic hydrogen flows in rumen fermentation: principles and possibilities of interventions. Front. Microbiol. 11:589. doi: 10.3389/fmicb.2020.00589
Van Gylswyk, N. O. (1995). Succiniclasticum ruminis gen. Nov., sp. nov., a ruminal bacterium converting succinate to propionate as the sole energy-yielding mechanism. Int. J. Syst. Evol. Microbiol. 45, 297–300.
Van Soest, P., Robertson, J., and Lewis, B. (1991). Symposium: carbohydrate methodology, metabolism, and nutritional implications in dairy cattle. J. Dairy Sci. 74, 3583–3597.
Vorholt, J. A., Vaupel, M., and Thauer, R. K. (1996). A polyferredoxin with eight [4Fe–4S] clusters as a subunit of molybdenum formylmethanofuran dehydrogenase from Methanosarcina barkeri. Eur. J. Biochem. 236, 309–317. doi: 10.1111/j.1432-1033.1996.t01-1-00309.x
Vucko, M. J., Magnusson, M., Kinley, R. D., Villart, C., and de Nys, R. (2017). The effects of processing on the in vitro antimethanogenic capacity and concentration of secondary metabolites of Asparagopsis taxiformis. J. Appl. Phycol. 29, 1577–1586. doi: 10.1007/s10811-016-1004-3
Wagner, T., Ermler, U., and Shima, S. (2016). The methanogenic CO2 reducing-and-fixing enzyme is bifunctional and contains 46 [4Fe-4S] clusters. Science 354, 114–117. doi: 10.1126/science.aaf9284
Wasson, D. E., Yarish, C., and Hristov, A. N. (2022). Enteric methane mitigation through Asparagopsis taxiformis supplementation and potential algal alternatives. Front Anim Sci 3:999338. doi: 10.3389/fanim.2022.999338
Wong, K., and Cheung, P. C. (2001). Influence of drying treatment on three Sargassum species. J. Appl. Phycol. 13, 43–50. doi: 10.1023/A:1008149215156
Wood, J. M., Kennedy, F. S., and Wolfe, R. S. (1968). Reaction of multihalogenated hydrocarbons with free and bound reduced vitamin B12. Biochemistry 7, 1707–1713. doi: 10.1021/bi00845a013
Keywords: methane synthesis, microbial regulation, low carbon, ruminant, methane inhibitor, Asparagopsis taxiformis
Citation: Li S, Sun Y, Cao S, Guo T, Tong X, Zhang Z, Sun J, Yang Y, Wang Q, Li D and Min L (2025) Asparagopsis taxiformis mitigates ruminant methane emissions via microbial modulation and inhibition of methyl-coenzyme M reductase. Front. Microbiol. 16:1586456. doi: 10.3389/fmicb.2025.1586456
Edited by:
Anusorn Cherdthong, Khon Kaen University, ThailandReviewed by:
Maharach Matra, Mahasarakham University, ThailandRittikeard Prachumchai, Rajamangala University of Technology Thanyaburi, Thailand
Copyright © 2025 Li, Sun, Cao, Guo, Tong, Zhang, Sun, Yang, Wang, Li and Min. This is an open-access article distributed under the terms of the Creative Commons Attribution License (CC BY). The use, distribution or reproduction in other forums is permitted, provided the original author(s) and the copyright owner(s) are credited and that the original publication in this journal is cited, in accordance with accepted academic practice. No use, distribution or reproduction is permitted which does not comply with these terms.
*Correspondence: Dagang Li, bGlkYWdhbmdAZ2RhYXMuY24=; Li Min, bWlubGlAZ2RhYXMuY24=